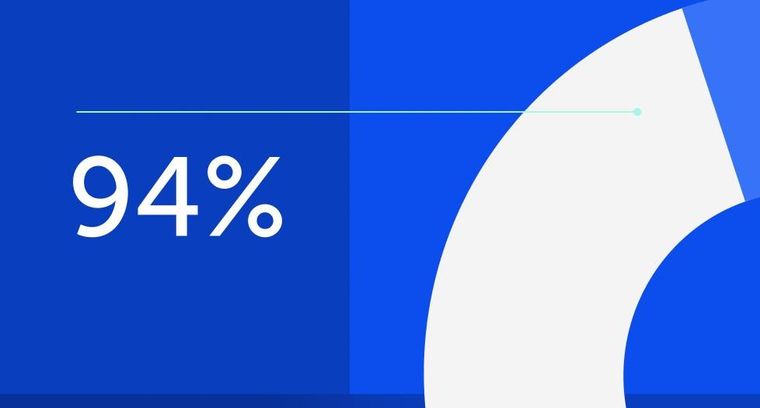
94% of researchers rate our articles as excellent or good
Learn more about the work of our research integrity team to safeguard the quality of each article we publish.
Find out more
REVIEW article
Front. Immunol., 01 December 2022
Sec. Viral Immunology
Volume 13 - 2022 | https://doi.org/10.3389/fimmu.2022.1050478
This article is part of the Research TopicCommunity Series in Immunometabolic Mechanisms Underlying the Severity of COVID-19, volume IIView all 8 articles
Our review summarizes the evidence that COVID-19 can be complicated by SARS-CoV-2 infection of immune cells. This evidence is widespread and accumulating at an increasing rate. Research teams from around the world, studying primary and established cell cultures, animal models, and analyzing autopsy material from COVID-19 deceased patients, are seeing the same thing, namely that some immune cells are infected or capable of being infected with the virus. Human cells most vulnerable to infection include both professional phagocytes, such as monocytes, macrophages, and dendritic cells, as well as nonprofessional phagocytes, such as B-cells. Convincing evidence has accumulated to suggest that the virus can infect monocytes and macrophages, while data on infection of dendritic cells and B-cells are still scarce. Viral infection of immune cells can occur directly through cell receptors, but it can also be mediated or enhanced by antibodies through the Fc gamma receptors of phagocytic cells. Antibody-dependent enhancement (ADE) most likely occurs during the primary encounter with the pathogen through the first COVID-19 infection rather than during the second encounter, which is characteristic of ADE caused by other viruses. Highly fucosylated antibodies of vaccinees seems to be incapable of causing ADE, whereas afucosylated antibodies of persons with acute primary infection or convalescents are capable. SARS-CoV-2 entry into immune cells can lead to an abortive infection followed by host cell pyroptosis, and a massive inflammatory cascade. This scenario has the most experimental evidence. Other scenarios are also possible, for which the evidence base is not yet as extensive, namely productive infection of immune cells or trans-infection of other non-immune permissive cells. The chance of a latent infection cannot be ruled out either.
The SARS-CoV-2 virus has brought many surprises to the scientific community. One of them is the virus’ ability to infect immune cells. Numerous and rapidly growing evidence of this ability inspired us to write this literature review. Studies accumulated to date show that among immune cells SARS-CoV-2 predominantly infects professional and nonprofessional phagocytes. The observation that infecting cells are mostly phagocytes may reflect the fact that they are simply at the forefront of the body’s defense against viral infection. These cells engage various mechanisms of pathogen internalization and, in particular, phagocytosis.
Evolution has equipped some immune cells with the ability to use various variants of pathogen internalization by endocytosis, as a defense mechanism to destroy pathogens, and to utilize their peptides for antigen presentation. Phagocytosis is defined as the internalization process of particles larger than 0.5 µm in diameter (1). This process is the most ancient form of endocytosis, and the origin of proteins involved in this type of endocytic pathway can be traced back to prokaryotes (2, 3). The act of phagocytosis is a fundamental process that is playing a key role in immunity (4). Phagocytosis can be accompanied by activation of the inflammatory pathway, which contributes to the elimination of the pathogen by inhibiting its spread (5).
The cell that carries out the process of phagocytosis in an animal or human body is called a phagocyte. Phagocytic cells consist of many subsets that perform highly diverse functions to maintain our health. At the same time, these cells also play a role in pathology of infectious or endogenous nature (1, 6). Several types of immune system cells are professional phagocytes, such as neutrophils, macrophages, and dendritic cells (1, 6, 7). There are also cells that can be called nonprofessional phagocytes. For example, B-cells mainly internalize antigens through endocytosis (8), but there is some evidence that these cells have an active phagocytic capability and undergo immune activation upon phagocytosis (9, 10).
Infection of phagocytes with a virus can lead to serious malfunction of these cells, greatly undermining the protective role of the immune system. Our review focused on phagocytes, but we know that viruses capable of infecting phagocytes can be internalized by host cells not necessarily by phagocytosis. Thus, some viruses have learned to take advantage of different variants of endocytic receptor-mediated internalization pathways, including but not limited by phagocytosis, to enter and infect the target cell (11–13).
Moreover, it has already been shown that SARS-CoV-2 can transfer its replication competent RNA into the host cell cytoplasm by rapid clathrin-mediated endocytosis (14). Therefore, we consider the process of virus internalization, which leads to viral infection of phagocytes, in terms of the endocytosis and, in particular, phagocytosis mechanisms.
Some viruses can reprogram biology of phagocytic cells by infecting and using them as vehicles for the spread, and/or persistence in tissues of host organisms (15). In this review, we focus on how SARS-CoV-2 hacks into endocytic pathways to penetrate and infect an immune cell.
SARS-CoV-2 belongs to the category of enveloped viruses. These viruses enter cells via fusion of their envelope with cellular membranes, which might be represented by outer plasma or endosomal membrane (12, 16, 17). For coronaviruses, including SARS-CoV-2, entry into endosomes and its pH dependent release from endolysosomes are key features of the host cells entry (18).
SARS-CoV-2 cell penetration is initiated by binding of viral S glycoprotein to host cell receptors. Angiotensin-converting enzyme-2 (ACE-2) is the most studied and well characterized SARS-CoV-2 cell entry receptor (19). The interaction of the ACE-2 receptor with the viral receptor binding domain (RBD) of the S-protein causes conformational changes in both protein subunits (S1 and S2), after which the covalent bond in S2 subunit is cleaved by the host protease, leading to fusion of the viral and cell membranes, and allowing the viral genome to enter the cell cytoplasm.
To achieve the membrane fusion two sequential cleavages of the S glycoprotein are required. The first cleavage is performed by a protease (Furin) at the S1/S2 site in the host cell during S protein biosynthesis in the infected cells. The second cleavage is performed by one of two transmembrane proteases at the S2′ cleavage site when the virus reaches the next target cell. One of these proteases is the plasma membrane serine protease TMPRSS2 and the other is the endosomal cysteine protease cathepsin L (19). Cleavage of the S2′ site by TMPRSS2 occurs on the cell surface, whereas cleavage of this site by cathepsin L happens in the endosomal compartment after ACE2-mediated endocytosis. SARS-CoV-2 virus like other coronaviruses (20) can enter and leave cells through the endolysosomal system (19). SARS-CoV-2 and some related viruses can leave this system and replicate in autophagosomes in the cytosol [reviewed in (18)].
While it was discovered that there is little to no expression of ACE-2 on most human peripheral blood immune cells it was also found that ACE-2 is highly expressed on tissue macrophages (21, 22) including alveolar macrophages, and macrophages of arteries (21, 23).
Moreover, the research group of Sefik et al. revealed that ACE2 expression was higher in infected human macrophages. Blockade of ACE2 with antibodies significantly reduced the ability of the virus to infect macrophages, suggesting that the ACE2 receptor may mediate virus entry into some human pulmonary macrophages (22).
It has been shown that monocytes can also express detectable levels of ACE2 mRNA and protein (24).
However, SARS-CoV-2 transcripts were found in some migratory dendritic cells, monocyte-derived alveolar macrophages, and tissue resident macrophages, which do not express ACE2 (25). Single-cell sequence analysis of myeloid cells positive for SARS-CoV-2 RNA showed that most of them do not express known viral entry factors (26). How does the virus get into these cells? It is likely that other than ACE2 cell entry receptors are also involved. They may play an additional role in SARS-Cov-2 penetration mechanisms into different target cells, including viral entry into immune cells (27).
It has been shown that several molecules can bind SARS-CoV-2 and function as additional receptors or coreceptors, helping the virus to attach to the cell surface. Among them C-lectin proteins such as DC-SIGN, L-SIGN (28, 29), ACRG-1 (30, 31), and transmembrane proteins: KREMEN1 (30, 31), Neuropilin-1 (32), AXL receptor tyrosine kinase (33), Basigin (34, 35), and Dipeptidyl peptidase-4 (36). A list of these potential receptors, their alternative names, and information on their expression in immune cells is presented in Table 1.
Table 1 Potential receptors and co-receptors for SARS-CoV-2 entry into immune cells other than ACE-2.
For effective viral entry into a host cell receptor expression in this cell must be complemented by the expression of proteases capable of cleaving the S-protein, as described above. Is anything known about TMPRSS2 and cathepsin-L expression in immune cells? Devaprasad et al. have shown that “a small fraction of circulating immune cells (including dendritic cells, monocytes, T cells) in the human peripheral blood mononuclear cells of COVID-19 patients express ACE2 and TMPRSS2” (50). Cathepsin-L, like other cathepsins B and H, is expressed constitutively in most immune cells (51) and therefore most likely participates in the promotion of SARS-CoV-2 entry into these cell.
The ability to infect immune cells is related to the disease pathogenesis of many enveloped viruses, such as representatives of Orthomyxoviridae family (influenza virus), Rhabdoviridae (rabies and vesicular stomatitis virus), Flaviviridae (Dengue, Hepatitis C and Zika viruses) (15, 52). The list can be expanded further with the representatives of Togaviridae (Chikungunya virus), Herpesviridae (cytomegalovirus), Paramyxoviridae (Respiratory syncytial virus), Retroviridae (HIV-1), Coronaviridae, and many others (15).
For the Coronaviridae family the phenomenon of such infection has been also documented for alpha- (53, 54) and beta coronaviruses (55–59). Some of the viruses listed use phagocytic immune cells as a repository for persistence in a form of latent infection, but some viruses employ these cells for productive infection (15).
It was hypothesized early in the pandemic that certain phagocytic cells, such as monocytes and macrophages, contribute to pathological local tissue inflammation and cytokine storm in patients with COVID-19, presumably being infected with SARS-CoV-2 (60). Subsequently, the hypothesis of a link between the development of severe immunopathology in patients with COVID-19 and infection of immune cells with the virus was reinforced. Indeed, evidence has been obtained that certain professional and nonprofessional phagocytes can be infected with SARS-CoV-2 in vivo and ex vivo, and such infection appears to be associated with severe inflammation. Our review focuses on summarizing and analyzing this evidence. We examined the possible mechanisms of infection, the consequences of this infection, and its potential role in the pathogenesis of COVID-19.
There are several lines of evidence demonstrating that SARS-CoV-2 can infect and replicate in professional and nonprofessional phagocytic immune cells. The detection of viral replication and translation products in an immune cell is interpreted as the presence of an infection.
The first line comes from autopsy reports, which arrived from research teams of different countries (61–66). The second line - derives from cell culture experiments (64–71). These lines of evidence are complemented by testing of blood (66), as well as bronchoalveolar lavage fluid samples from patients with COVID-19 (25) and analysis of transgenic mice model experiments (22, 67). In a few studies the titers of the infectious SARS-CoV-2 virus produced by different types of immune cells were measured (64, 70, 72, 73). In some other studies, the infectivity of the SARS-CoV-2 pseudovirus was assessed (74, 75).
Supplemental Table 1 summarizes the results of 19 studies in which evidence of viral infection of immune cells was obtained. The table lists the types of infected cells, as well as methods and techniques for detecting infection, and whether the virus titer was determined when there was evidence of productive infection. In addition, the table provides information on studies showing antibody-dependent enhancement of SARS-CoV-2 infection of immune cells (66, 72, 74, 75).
In summary, many researchers have concluded that immune cells are vulnerable targets for SARS-CoV-2 infection.
SARS-CoV-2 infected macrophages can be found in the lungs (25, 62, 63, 66) spleen, lymph nodes (61), as well as adipose (fat) tissues (65) of deceased patients with COVID-19. Information about adipose tissue infection is reported in the preprint, the study which has not been peer-reviewed (65). Infected cells were detected among macrophages from bronchoalveolar lavage fluid samples (25) and among blood monocytes taken from COVID-19 patients (66).
Immune cells that are infected by the virus are mainly represented by professional and nonprofessional phagocytes. The first category includes monocytes, macrophages, and dendritic cells. The second category includes B-cells.
Let’s take a closer look at the representatives of cells, for which SARS-CoV-2 infection was detected. The studies described below most often point to an abortive viral infection, which, however, triggers the production of many pro-inflammatory cytokines. Supplemental Table 1 includes a brief description of the studies we discuss in this review, listing the cells for which evidence of virus infection was obtained, as well as the methods used in the studies.
Monocytes are the largest circulating cells in the peripheral blood. They are round cells with a kidney-shaped nucleus. Monocytes can be recruited into tissues and differentiate into macrophages as well as dendritic cells, which are the front line of defense against invading pathogens. Monocytes are essential for functioning of both innate and adaptive immune systems. In addition to being precursors of other immune cells, they directly regulate immune pathways and are capable of phagocytosis. In the adult body monocytes differentiate out of precursors in bone marrow under the cascade of growth factors and stay in the circulation between 3 and 6 days patrolling the endothelial wall for the possible signal to be recruited into damaged tissue. Already in circulation, monocytes can perform their immune defense functions, and start a differentiation process (76). In blood monocytes are exposed to the number of circulating factors of endogenous and infectious origin and can be activated by these factors (77). The lifespan of circulating monocytes is rather short, and most of them, if they do not differentiate into other cells, undergo programmed cell death approximately a few days after leaving the bone marrow. After short term circulation in blood vessels, monocytes are genetically programmed to differentiate into tissue macrophages, monocyte derived dendritic cells; alternatively, they must undergo apoptosis or pyroptosis (76, 78, 79).
There is substantial evidence that human monocytes obtained from the blood of healthy donors can become infected with the virus ex vivo (56–58). In addition, infected monocytes have been found in individuals suffering from the disease (25, 56, 57). Thus, the research of Junqueira et al, show that about 6% of blood monocytes of patients with severe form of COVID-19 have evidence of replicating SARS-CoV-2 genomes and viral proteins translation (66). Supplemental Table 1 lists the studies that have provided evidence of monocytes viral infection and the methods used in these studies.
Macrophages are a heterogeneous population of innate immune scavenging cells. They use both endocytosis and phagocytosis to provide protection against infection and unwanted-self components. These large phagocytes, discovered by Élie Metchnikoff, winner of the 1908 Nobel Prize, build an innate immune network in all human and animal tissues. In healthy status, macrophage dynamically controls tissue turn-over (80). When trauma occurs or pathogens invade, resident macrophages are first to provide anti-pathogen response and to active acute inflammation. At this stage, circulating monocytes are recruited to differentiate into dendritic cells and macrophages. Once the danger is eliminated, local macrophages will signal immune and somatic cells to resolve inflammation, heal the damage, and restore tissue homeostasis (6). The lifespan of macrophages varies from a few days to months or even years. Especially long-lived are resident macrophages (63, 76).
Although macrophages offer protection against infection by playing a key role in the immune defense system, they can cause serious immunopathology. Among the immunopathological conditions directly related to the improper functioning of macrophages, which turn from friends into life-threatening enemies, are sepsis (5), cancer (81), diabetes (82, 83), and heart disease (84).
Macrophage-mediated chronic inflammation is another immunopathological condition associated with malfunctioning macrophages, and it is amplified by hyperglycemia (85, 86) and dyslipidemia (87). Therefore, such inflammation is particularly harmful and can even become life-threatening for people with obesity (88), metabolic syndrome, and diabetes (89, 90). The question of how these chronic diseases with metabolic dysfunction particularly aggravate the course of COVID-19, leading to life-threatening complications, must be addressed (91). Perhaps knowledge of the pathological function of macrophages in chronic inflammation can help us find answers to this question.
Ex vivo polarized macrophages can efficiently engulf SARS-CoV-2. However, instead of being inactivated in these cells, the virus sometimes starts replicating and translating its proteins (67, 71).
According to the study of Lv at al. classically activated M1, but not alternatively activated M2 macrophages can be productively infected by SARS-CoV-2 and help spread the virus (67). In contrast, Boumaza et al. found that M1 and M2 macrophages were equally infected and concluded that macrophage polarization in vitro did not affect permissiveness to SARS-CoV-2 (69).
Yuichi Mitsui et al. found that one factor in the differentiation of macrophages into the type that is susceptible to viral infection is the lymphokine IL-10. This lymphokine is often found in elevated concentrations in the bloodstream of patients with severe COVID-19. Thus, due to this lymphokine, alveolar macrophages become M2c macrophages, which are susceptible to SARS-CoV-2 infection, and the infection leads to increased inflammation in the alveoli. The results of the study are published in a preprint (92). Thus, considering research results of (67, 71, 92) we cannot yet clearly say which type of macrophages (M1 or M2) are more susceptible to SARS-CoV-2 infection. This is clearly an important question, and further research should soon shed light on the problem and help find an answer.
How does the virus get into the macrophages it infects? One possible route of infection of residential lung macrophages is phagocytosis of epithelial cells infected with SARS-CoV-2 (73). However, FcγR-mediated phagocytosis of antibody-virus complexes instead of infected cells may also be involved in such infection (see below).
In the Supplemental Table 1 a brief description of the studies, in which evidence of macrophage viral infection was obtained is given. This table lists subsets of macrophages that were found to be infected and includes their Cluster of Differentiation (CD) markers as well as the methods used in these studies.
Infected macrophages probably lose the ability to function properly, and their dysfunction may play an important role in the pathogenesis of COVID-19, which is described in more detail below. Because of the viral infection, macrophages infiltrating the lungs may be in a hyperactivated state. Such a state probably contributes to a positive feedback loop of release of proinflammatory cytokines by these macrophages and attraction of cytotoxic effector cells, increasing tissue damage at the site of infection (93). Some researchers have called the massive macrophage activation phenomenon during COVID-19, which leads to acute respiratory distress syndrome (ARDS), the “macrophage activation syndrome”. ARDS is the major cause of high fatality in SARS-CoV-2-associated pneumonia (94). It is highly likely that a viral infection of macrophages contributes to their reprogramming and leads to this pathological development.
Dendritic cells (DCs) have outer membranes that form protrusions looking like tree-like structures, which is why they got their name as dendritic. These cells are capable of amoeboid movements that allow them to travel in tissues, including tight junctions of epithelia. DCs enable bridging between innate and the adaptive arms of the immune system (95). The main function of these cells is the presentation of antigens to immunocompetent cells. The antigen presentation may or may not involve phagocytosis. DCs can digest pathogen proteins into peptides that are getting represented on the major histocompatibility complex molecules recognized by T cells (95). The life span of DCs depends upon their belonging to different subsets and is a subject of debate (96).
Ex vivo cell culturing demonstrated that monocyte derived dendritic cells (moDC) can be targets of SARS-CoV-1 (97, 98) and SARS-CoV-2 abortive infection (68). The observed infection of DCs induced the production of multiple proinflammatory cytokines capable of provoking interferon-mediated cell death (68).
SARS-CoV-2 transcripts were found in some migratory dendritic cells indicating likely viral infection (25). Larsen et al. observed that the virus can effectively infect and replicate in plasmacytoid dendritic cells (pDCs) causing their chronic activation (73). Experimental evidence suggests that the virus enters these cells via clathrin-mediated endocytosis (73). According to Larsen et al, chronic activation of pDCs, which is accompanied by their release of large amounts of IFN-I, can hyper-stimulate macrophages, causing a cytokine storm in patients with COVID-19 (73).
Supplemental Table 1 includes a brief description of the studies in which evidence of dendritic cells viral infection was obtained, the results of these studies, as well as the methods used.
There is also a mechanism for dendritic cells to participate in the spread of the virus via the trans-dissemination pathway. DCs capable of transferring their cargo in a form of intact pathogens or protein complexes to other cells for antigen presentation. During this process, DCs transport intact viruses, from the sites of peripheral infection, in which the pathogen was encountered to the lymphoid tissues, where the cargo can be transferred to other antigen-presenting cells. This process is mediated by endocytic uptake of antigens or intact pathogens into late endosomal structures that are named multivesicular bodies/endosomes. These multivesicular endosomes are becoming exosomes, formed by “turned inside out” endosomes. After cargo uptake, multivesicular endosomes are directed to antigen processing through the lysosomal system or return to the cell surface, where they excrete out their cargo, and recycle surface receptors by returning them back to the plasma membrane [reviewed in (99)].
This process, created by evolution for efficient antigen presentation, has been hijacked by some viruses such as HIV and used for their dissimilation (99). The evidence is getting accumulated that coronaviruses employ this mechanism as well. DCs and many other immune cells express a C-type lectin receptor, namely DC-SIGN, which can bind the viral S-glycoprotein glycans (29). This binding possibly allows replication-competent SARS-CoV-2 to travel as a passenger with C-lectin-expressing cells, probably in multivesicular endosomes, without undergoing inactivation. Thus, DCs or other immune cells can transport the virus to the receptor-positive target cell, whereby the virus gains access to the permissive cell. In this way, DC-SIGN molecules on the surface of immune cells allow the virus not only to be taken up by the cells, but also to travel and gain access to other cell types, causing the so-called trans-infection. In this way, it has been demonstrated that C-type lectin receptors mediate SARS-CoV-2 trans-infection and can contribute to the spread of the virus (28, 29).
Interestingly, this spread infection mechanism has been shown for SARS-CoV-1. Experimental evidence has been obtained that S-glycoprotein-mediated virus entry into some host cells can be enhanced by DCs virus transmission via the viral coreceptor DC-SIGN (100).
B-lymphocytes, also called B-cells, are round-shaped cells that play a critical role in the functioning of the adaptive humoral immune system. They are responsible for the production of antigen-specific antibodies. B-cells internalize antigens through endocytosis (8), or phagocytosis (9, 10). Life span of B-cells depend on the category they belong to: there are short-lived B-cells with a lifespan of a few days and there are those that live years (101).
To date, two research teams have provided evidence that B-lymphocytes can be susceptible to SARS-CoV-2 infection ex vivo (64, 75). Pontelli et al. (64) demonstrated that the infection can be productive. Interestingly, a close relative of SARS-CoV-2 namely SARS-CoV-1 virus is capable of infecting human B-cells in vitro. Infection occurs through antibody mediated FcR-dependent infection route (102).
Supplemental Table 1 includes a brief description of the studies in which evidence of B-cells viral infection was obtained, their results, as well as the methods used in these studies.
Phagocytic immune cells, including macrophages and monocytes, have an effective system for inactivating ingested pathogens. During internalization, the pathogen is captured by the cell into the endosome/phagosome, which then, together with the captured pathogen inside, undergoes several changes that include ‘maturation’ steps, with the internal pH gradually decreasing, making it more and more acidic. These steps represent a chain of fusion and fission events with endocytic organelles through a “kiss-and-run” mechanism to obtain the molecules needed for each endosome/phagosome stage. The stages of endosome maturation include the early, intermediate, and late phase (103, 104). At the end of the maturation process the endosome/phagosome with its contents fuses with the lysosome forming an endolysosome or, in particular phagolysosome, with acidic, hydrolytic contents, which can effectively kill and digest the pathogens consumed (104, 105).
Obviously, viruses that survive in immune cells after internalization use this important process of endosome maturation to their advantage. Some of them have developed escape mechanisms that allow not only to survive this endosome/phagosome transformation process, but also to start replication in the phagocytic cell.
Interestingly, it has been shown that the SARS-CoV-2 is internalized through the pH-dependent endocytic pathway (106, 107). Moreover, blocking transportation of the endosome and its acidification can inhibit SARS-CoV-2 infection (106, 107). To enter the permissive cell through endocytic pathway, the viral S-protein must undergo proteolytic cleavage by host membrane protease cathepsin L along with conformational changes. The fact that blocking acidification inhibits viral infection may indicate that the S protein cleavage by cathepsin L along with S protein conformational changes are pH dependent.
As mentioned in the introductory section of this review, enveloped viruses penetrate host cells by fusing their envelopes with cell membranes, which can be represented by endosomal membranes. Membrane fusion mechanisms can be of two types: pH-independent and pH-dependent (17). In the pH-dependent case, which we are more interested in, the interaction between the virus and the cell entry receptor leads to the obligatory endocytosis of the virus-receptor complex. Then the gradual step-by-step acidification of the endosomes, during their maturation process, promotes the viral glycoprotein cleavage by host proteases, its conformational change, followed by the fusion of the viral and endosomal membranes along with the release of the viral genetic material into the host cell (17).
It is appealing to think that a similar pH dependent mechanism is used by SARS-CoV-2 to escape the endosome/phagosome during its maturation process. Most likely, the pathogen internalization pathway with gradual stepwise acidification can provide the necessary low pH to promote proteolytic cleavage along with the conformational change in the S-protein required for the fusion of the viral and endosomal membranes followed by the entry of viral genomic RNA into the host phagocytic cell.
At the beginning stages of COVID-19 pandemic Matthew Park wrote a short commentary entitled “Macrophages: a Trojan horse in COVID-19?”. The commentary summarized experimental evidence by Feng et al. [published as a preprint (61)] that according to Park suggests that infected by SARS-CoV-2 macrophages could contribute to viral spread, and excessive inflammation during COVID-19 (108). It is possible that infected monocytes could play a similar role. Monocytes can become important and specialized viral reservoirs distributed throughout the body that store large amounts of viral genetic material, keeping it infective.
Indeed, according to experimental evidence [reviewed in (15)] infected monocytes can cross the blood-tissue barrier and deliver virions “as specific parcels” into the central nervous system, playing the role of the “Trojan horse”, which is a known way of dissemination for HIV, HCV, HCMV and Japanese encephalitis viruses.
However, to use the immune cell as a vehicle for spreading infection, the virus must undergo a productive infectious cycle or, in the case of the virus persistence, its infectivity in the host immune cell must be maintained. Indeed, several types of viruses can travel with immune cells, retaining viral replicative and infectious capacity until these immune cells meet permissive target cells that can be infected by these viruses (15). Therefore, immune cells may serve as a virus transport device.
Some immune cells express C lectin proteins such as SIGLEC1 (CD168), and/or DC-SIGN (CD209) on their surface (Table 1). These proteins have a high affinity for the outer glycoproteins of some viruses. As mentioned above, a subset of DCs belong to this kind of immune cells. They express DC-SIGN, which can bind the S-glycoprotein SARS-CoV-2.
The ability to bind and transport viruses through this and other mechanisms is an evolutionary acquisition of DCs for the antigen presentation process. However, this binding, sometimes allows the virus to hijack the adaptive evolutionary created mechanism, with the result that immune cells transport replication competent virus and promote the infection of other cell types (28, 29). Thus, lectin molecules on the surface of one cell type, such as immune cell, can be utilized by the virus to travel and access cells of another type, causing what is called trans-infection. Being involved in the process, immune cells may spread the virus but do not themselves become infected. These kinds of scenarios can lead not only to the spread of the virus to permissive cells, but also to severe functional abnormalities of immune cells.
Lv et al. observed the release of virions from infected alveoli macrophages ex vivo, and these virions were capable of infecting other cell types (67). In a humanized transgenic mouse model, M1 macrophages were found to promote lung infection with SARS-CoV-2. This research group concluded that SARS-CoV-2 can complete its life cycle in macrophages. This group's researchers strongly suspect that these immune cells are involved in spreading the infection within the tissue by acting as a vehicle (67). The suspicion that strengthens the Trojan horse hypothesis was further tested in a transgenic mouse model, in which alveolar macrophages were found to be able to promote SARS-CoV-2 infection of the murine lungs. Another research group showed in ex vivo cell culture experiments that the virus infects monocytes and macrophages without forming new infectious virions in these immune cells, but with retained infectivity for other target cells. This is how trans-infection occurs, when non-permissive immune cells associated with SARS-CoV-2 infect other permissive non-immune cells (109).
Many studies (64, 69, 71) demonstrate that immune cell virus infection proceeds abortively, without formation of infectious virions. Similar abortive infection of human immune cells, which included blood monocytes and lung macrophages was discovered by Junqueira et al. (66). The authors of the study found that up to 6% of blood monocytes in COVID-19 patients can be infected with SARS-CoV-2. However, the infectious virus was not detected in the cultures of infected monocytes, instead the signs of monocytes pyroptotic deaths were noticed that were consistent with observation of the study of Sefik et al. (22).
Abortive SARS-CoV-2 infection of human monocyte-derived immune cells such as macrophages and dendritic cells was also shown in research of Zheng et al. group (68). Therefore, spread of the virus by immune cells through productive infection in these cells seems unlikely, but transinfection of other target cells is probable.
Different research teams observe diverse scenarios under various experimental settings. Both the stages of myeloid-lineage cell differentiation, the vectors as well as states of their polarization and activation can create restrictive or permissive cellular conditions for the virus. For example, the type of infection, namely, whether the monocyte and its progeny can be infected productively or undergo programmed cell death in the form of pyroptosis, whether they will be an effective carrier of the intact virus to other target permissive cells or not, depends on the state of monocyte differentiation (15).
Monocytes, predominantly derived from the progenitors in the bone marrow, differentiate into macrophages or dendritic cells after circulating in the bloodstream for about 3-6 days. This differentiation is characterized by changes in the transcriptome, epigenome, metabolism, and secretome, which determine the key regulatory activities of macrophages and DCs in tissues (76, 78, 79).
Summarizing the experimental data one can imagine that in some immune cells the infection is abortive, in others it has a latent or productive form. There is the possibility of trans-infection of target cells by attaching the virus to the C lectins of immune cells, transporting it as a passenger on or in these cells and then infecting the target permissive cells of other type (28, 29, 109). Some possible scenarios of immune cell infection are shown in Figure 1.
Figure 1 Three scenarios of SARS-CoV-2 infection of immune cells: (I) entry of SARS-CoV-2 into immune cells triggers pyroptosis and inflammatory cascade, (II) entry of virus into cells leads to productive infection or, (III) entry of virus promotes its transfer to primary permissive target cells, causing their infection. The second and third scenarios are consistent with the "Trojan horse" hypothesis.
Studies performed on transgenic humanized mice and in cell culture showed that SARS-CoV-2 replication in macrophages triggers inflammatory death (pyroptosis) of these cells, which likely stimulates the hyperinflammatory cascade associated with COVID-19 (22). Pyroptosis is a form of lytic programmed cell death that happens most frequently during infection with pathogens having the capability to enter and survive in the cells of the host organism (110, 111). This type of suicide cell death occurs mainly in immune cells such as macrophages that are responsible for the inflammatory process, however other cell types such as epithelial cells can also undergo pyroptosis (110, 112). Thus, infection-induced pyroptosis probably represents an important line of defense, carried out not only by immune cells, but also by epithelial barrier tissues.
The pyroptosis promotes the release of immunogenic cellular content, including damage-associated molecular patterns, and inflammatory cytokines such as interleukin-1β (IL-1β) (110). The cytokine release provokes inflammation, which, being part of the body’s normal immune defense mechanism against infection, can be harmful if it is dysregulated. For example, increased uncontrolled inflammation can lead to sepsis (110).
The physiological function of inflammation and pyroptosis is to trigger the expression of immune genes and attract lymphocytes to the site of infection, thereby controlling pathogen invasion (113). In addition, pyroptosis of infected cells prevents the virus from completing its replication cycle, therefore it is an important part of the antiviral immune defense mechanism (113, 114).
Not surprisingly, because of these properties of pyroptosis, the release of infectious virions by the infected macrophage is largely prohibited and was observed only after treatment of the cell with inhibitors of the inflammasome pathway, which prevent pyroptosis (66). Thus, there is no evidence of a complete viral cycle naturally occurring in macrophages in the study by Junqueira et al. (66). However, despite the lack of detection of the productive cycle of the virus, the study showed that signs of pyroptosis in the blood plasma of COVID-19 patients correlate with the development of severe disease. Therefore, Junqueira et al. concluded that abortive viral infection of macrophages, due to pyroptosis, triggers the excessive production of inflammatory cytokines, which enhances the hyperinflammatory response to SARS-CoV-2.
These findings were further supported by experimental data that the virus induces inflammasome formation and activation, which leads to pyroptosis in experimentally infected primary monocytes from healthy donors and COVID-19 patients (70).
Inflammasomes are cytosolic multiprotein complexes that are a functional part of the mechanisms of the innate immune system (113, 115). Activation of the inflammasome stimulates secretion of pro-inflammatory cytokines such as IL-1β and interleukin 18 (113, 115). Therefore, it is not surprising that SARS-CoV-2-infected blood monocytes and lung macrophages had activated inflammasomes. It has been demonstrated that these activated inflammasomes and programmed cell death via pyroptosis increases the secretion of proinflammatory cytokines, including IL-1ß by SARS-CoV-2-infected monocytes (70). Super elevated cytokine secretion promotes tissue immune cell infiltration, which through over secretion of proteases and reactive oxygen species can cause damage to the lung and other human organs.
To what extent does a physiological immune defense mechanism, such as pyroptosis, whose purpose, among other things, is to prevent the spread of infection, become dysfunctional because of infection with SARS-CoV-2 macrophages, monocytes, or other immune cells? To what extent does this infection contribute to the aberrant inflammatory feedback loop? These questions need answers.
The authors of the study Junqueira et al. examined whether the macrophages in the lung autopsies of diseased COVID-19 patients were infected with SARS-CoV-2 and whether they had activated inflammasomes. They found that a smaller proportion of macrophages were infected, but a larger proportion had activated inflammasomes. This ratio indicates that pyroptotic death of infected cells leads to the release of potent inflammatory mediators, causing activation of inflammasomes in the larger macrophage population (66).
The observation of this phenomenon and other evidence led to the development of the idea that viral infection of immune cells, triggering death of these cells through pyroptosis, is the massive driving force behind the development of particularly severe forms of the disease and fatal COVID-19 cases. The idea is becoming more and more popular in the research community (22, 66, 70).
Lv et al. observed the release of infectious virions from infected macrophages ex vivo (67). Interestingly, it has been shown that the N-protein SARS-CoV-2 can antagonize cellular inflammatory responses by inhibiting pyroptosis in human monocytes (116). It remains to be shown if this inhibition can lead to a productive viral infection of immune cells.
It has been demonstrated earlier that SARS-CoV-1 infected macrophages induce little or no IFN-β, suggesting that suppression of the immune response leads to uncontrolled viral replication in the respiratory epithelium cells (117). Perhaps a similar scenario is realized with SARS-CoV-2.
Infection of immune cells can occur in two ways, which are probably synergistic. That is, a cell can become infected with a virus by viral interaction with a cell receptor without assistance of antibodies or with such assistance. In the case of the latter, antibodies help to infect the immune cell. Below we will discuss these two pathways, but it should be remembered that they are not mutually exclusive and, most likely, each of these pathways of virus penetration into the cell increases the probability of infection. In the “Introduction” section, we gave a list of receptors and co-receptors (Table 1) that, in addition to the ACE 2 receptor, can be used by SARS-CoV-2 to penetrate immune cells.
Direct infection of primary human SARS-CoV-2 monocytes using cell entry receptors and without any antibody assistance was demonstrated by Rodrigues et al. (71). This group, like Sefik et al. (22), found that the virus can replicate in healthy donor monocytes cultured ex vivo, and this process causes NLRP3 inflammasome activation as well as cell death. NLRP3 is a protein mainly expressed in macrophages and is encoded by the NLRP3 gene located on human chromosome 1. The protein acts as a sensory molecule and, together with other proteins, forms the NLRP3 inflammasome, a protein complex essential to the innate immune system functioning (118).
The process of monocyte infection detected by Sefik et al. (22) and some other research teams (68) did not require antibodies for the virus cell entry. Similar finding was done by Pontelli, et al. This research team revealed that monocytes, as well as both B and T lymphocytes, were susceptible to direct SARS-CoV-2 infection in vitro that didn’t require antibodies. The discovery of the phenomenon raises the question related to the identification of receptors that can be used by the virus to enter the cell.
Antibody-dependent enhancement (ADE) of infection is a phenomenon in which virus-antibody complexes interact with immune cells carrying complement or Fc-receptors and promote internalization of the virus, maintaining infectivity and virus potential for latent, abortive, or productive infection (119–122). In other words, antiviral antibodies facilitate the entry of the virus into immune target cells by hijacking the phagocytic FcγR or complement pathways, which naturally evolved to fight infection. Although antibodies are usually protective and beneficial to organisms, surprisingly, they can promote a viral infection instead of stopping it. It has been shown that virion binding to non-neutralizing or sub-neutralizing antibodies can lead to more efficient FcγR mediated viral uptake by the target cell causing ADE (121).
The ADE phenomenon has been described for many viruses including representative of families Flaviviridae (Dengue virus), Retroviridae (HIV-1), Orthomyxoviridae (Influenza virus), Paramyxoviridae (Respiratory syncytial virus), Filoviridae (Ebola virus), and Togaviridae (Chikungunya virus) (122–124).
The ADE has been also documented for Coronaviridae including alpha- (53, 54) and beta coronaviruses (55–59). ADE could increase the severity of several viral infections (75) although no definitive role for ADE in human coronavirus infections has been demonstrated so far. However, it is well established that feline infectious peritonitis virus can replicate in monocytes and macrophages, entering these cells via antibody dependent mechanism. This replication can trigger fatal peritonitis in cats (53, 54). Therefore, some researchers hypothesize that the pathogenesis of SARS (56–59) and COVID-19 (55, 125–127) diseases may be associated with ADE. Its role in establishing potential latent virus infection is also discussed (55).
Interestingly, the research group of Junqueira et al. discovered that monocyte SARS-CoV-2 infection can be dependent on antiviral antibodies. It is worth noting that only antibodies from COVID-19 infected patients, and not from the plasma of vaccinated individuals, were able to contribute to the type of cellular infection observed by this group (66).
It is well established that in many viral diseases, prior sensitization of the humoral immune response, in the form of prior infection or vaccination, is a prerequisite for ADE upon subsequent antigen exposure (121). However, according to Junqueira et al. (66), for SARS-CoV-2 the scenario is different; antibody mediated virus entry can occur during primary viral infection.
Sanchez-Zuno et al. (128) suggested that currently there is no sufficient evidence that ADE promotes the spread of SARS-CoV-2 in infected host organisms. Therefore, ADE in COVID-19 is best described as “virus antibody dependent entry into the cell”, or “antibody-dependent inflammation”, which does not necessarily lead to productive viral infection, meaning that ADE can lead to abortive or latent SARS-CoV-2 types of infection instead. Other research team suggested the name Fc-mediated viral entry (FVE) (129).
However, as mentioned above, even abortive infection of immune cells can lead to powerful amplification of inflammatory cascades with aberrant feedback loops, which can promote severe form of COVID-19. A similar scenario occurs with other viruses, particularly the Dengue virus, for which it has been noted that ADE has been associated with the development of a “cytokine storm”, which involves the massive release of inflammatory cytokines and other mediators (130).
How does a virus use antibodies to enter an immune cell? The process resembles the piggybacking approach, in which “piggyback” is an IgG antibody and the entry gates to a pig barn are Fcγ receptors (FcγRs) of immune phagocytic cells (131, 132). It is thought that myeloid cells expressing FcγRs, such as monocytes and macrophages, dendritic cells, and some granulocytes, can become ADE targets through phagocytic uptake of immune complexes (128).
Most likely cooperation between conventional cell entry receptors such as ACE2 and Fc receptors occur and both types of receptors are working synergistically. For example, Wang et al. found that ACE2 can function as an additional receptor in the FcγR-dependent ADE of SARS-CoV-2 infection (74). A possible mechanism of antibody-mediated infection via Fcγ receptors is shown in Figure 2. The virus probably escapes the endosome/phagosome during the maturation of this organelle, which is accompanied by its acidification, as shown in Figure 2A. Two scenarios can be imagined of this escape (Figure 2B). They differ in the affinity of the antibody and the resistance of this affinity to a pH decrease in an endosome/phagosome. The first scenario: in the case of high antibody affinity, which is also unaffected by a pH decrease, the virus is reliably retained by the antibodies within the immune complex in the endosome/phagosome. It cannot escape. However, second scenario is possible: in the case of low antibody affinity, which can be further decreased with the acidification of the endosome/phagosome during its maturation, the antibodies are losing their “grasp” and the virus acquires the ability to escape from the immune complex and consequently, also the endosome/phagosome.
Figure 2 Hypothetical ways in which the virus escapes phagosomes. (A) The virus leaves the endosome/phagosome during its maturation and acidification. (B) Antibodies that firmly retain the virus in the immune complex most likely prevent it from leaving the organelle as shown in the upper part of the figure. Antibodies not firmly holding the virus allow it to escape from the organelle into the cytoplasm, as shown in the lower part of the figure. Perhaps acidification of the endosome/phagosome during its maturation reduces the affinity of some antibodies to the virus, promoting the exit of the virus from the immune complex and facilitating its exit from this organelle.
FcγRs belong to the family of immunoglobulin proteins. They are located on the surface of immune cells and function as molecules that bind Fc-chains of the IgG molecule (133). This binding might trigger phagocytosis of the virus-antibody complex (131). One of the natural functions of FcγRs is to help phagocytic immune cells to capture, and “ingest” the pathogen. Internalization of the pathogen should lead to its inactivation and presentation of the pathogen derived antigens by the phagocytic cell. However, some viruses have developed the ability to avoid inactivation in the immune cell.
Three FcγR groups have been described for human cell types: FcγRI (CD 64), FcγRIIA/B (CD32), and FcγRIIIA/B (CD16). All receptors are expressed in various combinations on the surface of different immune cells (133). FcγRs vary in their affinity for IgG, such as FcγRI is a high-affinity activating receptor that binds to monomeric IgG molecules, FcγRII and FcγRIII are low-affinity receptors that require high avidity binding by IgG-pathogen immune complexes. Among FcγRII receptors FcγRIIA and FcγRIIC are activating receptors and FcγRIIB is an inhibitory receptor (122, 133).
Experimental evidence suggests that SARS-CoV-1 can start replication in immune cells after entering via FcγRII (CD32) (56, 102, 134). It was specifically shown that the expression of two types of receptors by immune cells: FcγRIIa and FcγRIIb induces ADE by SARS-CoV-1 (56). The author of another study, while observing SARS patients, found that the severity of the disease correlates with the FcγRIIa allelic polymorphism. In patients with FcγRIIa allelic isoform that can interact with both IgG1 and IgG2, the disease was more severe compared to patients with the FcγRIIa isoform capable of binding only IgG2 (134). Thus, it appears that obtained evidence points to CD32 as the major receptor for SARS-CoV-1 entry.
It has been discovered that FcγRIIB is involved in infection of B-cells by SARS-CoV-2 (75). However, it is likely that additional receptors function in antibody-mediated immune cell entry of SARS-CoV-2 compared to SARS-CoV-1. Thus, team of Junqueira et al. found that blocking CD16 or CD64 strongly inhibited infection by SARS-CoV-2 of monocytes, whereas blocking the other receptors had no major effect (66). This experimental evidence points to CD16 or CD64 as major virus entry receptors for SARS-CoV-2.
The Fcγ receptors used by MERS-CoV, SARS-CoV-1, and SARS-C0V-2 for immune cell infection are listed in Table 2.
Table 2 FcγRs receptors involved in the antibody-dependent infection of immune cells by beta-coronaviruses.
Immune responses in severe COVID-19 patients stand out with a predominance of low fucosylated IgG antibodies (141). Although Fc afucosylation is supposed to be a regulatory layer of adaptive immunity (142), many studies reported its role in COVID-associated pathology (141, 143–145).
Core fucose is a component of N-linked glycans within the Fc fragment of the antibody. Addition of fucose does not cause structural changes (146) but strongly decreases the affinity of receptor binding (147) and the efficiency of antibody-dependent reactions (148). A schematic representation of the interaction of an antibody with the phagocytic Fc gamma RIII receptor and the effect of a fucose residue on this interaction is shown in Figure 3. Afucosylation is not the stripping of existing fucose, but simply fucosylation that has not happened. Core afucosylation seems to be an adaptive mechanism for the response to membrane-bound antigens (142). Since afucosylated IgG are shown to have a higher receptor-binding affinity and thus more pronounced effector functions, Fc afucosylation might be a regulatory feature to adjust adaptive humoral immunity to fight enveloped pathogens. However, it can also promote autoimmune pathology (149–151) and aggravate infectious diseases (152–154).
Figure 3 Distinction between fucosylated and afucosylated antibodies. Fucosylated antibodies have a lower affinity for the cellular receptor FcγRIIIa (CD16a) compared to afucosylated antibodies. Consequently, these antibodies contribute less to the internalization of the pathogen by cells possessing this receptor than do afucosylated antibodies.
Afucosylated IgG antibodies have been shown to contribute to the pathogenesis of COVID-19 through their proinflammatory action (141). In particular, Chakraborty et al. showed the link between low fucosylation of viral S protein-specific antibodies and the release of proinflammatory cytokines, such as IL-6 and TNFα, during SARS-CoV-2 infection (143). Another study confirmed these results and revealed that high titers of afucosylated IgG antibodies targeting S protein induce inflammation promoted by alveolar macrophages and activate platelets as well as endothelial cells (144). Authors observed the normalization of antibody fucosylation in several weeks after infection.
In their next study, Chakraborty et al. found out that afucosylated IgG antibodies were non-neutralizing and could be detected in severe COVID-19 patients but not in those with mild disease or vaccinated (145). Noteworthy, van Coillie et al. reported in their preprint that the first dose of mRNA vaccine induced transient formation of low fucosylated IgG1 in people naive to SARS-CoV-2, but not in people who encountered this antigen, although the extent of this formation was lower than in those with severe COVID-19 (155).
Immune complexes with low fucosylation from patients with severe COVID-19 induced immune cell infiltration of lung tissue in model animals, whereas highly sialylated and fucosylated mRNA vaccine-elicited IgG did not cause inflammation in lungs or proinflammatory cytokine release (145).
Junqueira et al. investigated how SARS-CoV-2 infects monocytes and observed that highly afucosylated antibodies from COVID-19 patients but not from vaccinees significantly increased virus uptake through FcγRIII (66).
Thus, there is growing evidence that IgG antibodies with afucosylated Fc fragments play an important role in the pathogenesis of viral infections and particularly in COVID-19.
Preliminary data indicate that a pathway other than FcγR-mediated cell entry may be involved in SARS-CoV-2 ADE. Okuya et al. obtained evidence that an antibody-dependent pathway of virus entry mediated by the complement component 1q plays a role in cell infection with this virus. However, immune cells were not investigated as target cells in this study (156).
Complement component 1q (C1q) is a protein complex involved in the bridging of innate and adaptive immune systems. Okuya et al. provide a review of the literature showing that C1q-mediated ADE has been proposed for several viruses, including HIV, EBOV, Marburg virus, and human parvoviruses (156). This ADE mechanism is based on the binding of C1q receptors on the cell surface with virus-antibody C1q complexes, which results in enhanced attachment of the virus to target cells. The mechanism is like FcγR mediated virus cell entry, but instead of FcγR another cellular receptor is used namely - C1q receptor. Therefore, C1q serves as an additional bridge connecting virus-antibody complexes to the cell plasma membrane. These complexes are then taken up by the phagocytic cell and end up in the endosome/phagosome (Figure 4).
Figure 4 Hypothetical mechanism of SARS-CoV-2 entry into a cell via complement receptors. The low affinity of the antibody, which causes a loose connection to the virus, allows the virus to escape the complex and fuse its envelope to the phagosome membrane, thus introducing its genome into the cell cytoplasm.
Junqueira et al. observed that patient plasma promoted viral infection of healthy donor monocytes in vitro much more than purified IgG antibodies against S-protein (66). The authors of the study concluded that an antibody-independent plasma component may contribute to the infection (66). It is possible that C1q is the plasma component responsible for the effect observed by the study authors.
ADE of coronaviruses can be promoted by antibodies to the spike (S) glycoprotein. This observation was done for SARS-CoV-1 (59, 102, 157) and SARS-CoV-2 (127, 158).
A high neutralization titer of antibodies to the SARS-CoV-2 spike-protein in the plasma of patients with COVID-19 has been shown to predict survival (159). However, different antibodies have various potential to neutralize the virus and cause ADE; a neutralization potential does not exclude a potential for ADE. Anti S-protein immune serum, while inhibiting receptor mediated viral entry into a permissive host cell, may increase infection of human monocyte-derived macrophages (59) and cultured B-cells (102, 157) by SARS-CoV-1 via Fc-receptor binding.
Some antibodies can have both neutralizing and ADE effects. Antibodies targeting S-protein that neutralized most variants of SARS-CoV-1 viruses enhanced immune cell entry of the mutant virus. The mechanism of enhancement might involve the interaction of antibodies with conformational epitopes in the viral ACE-2-binding domain (160). Antibodies targeting different S-protein variants of the SARS-CoV-1 virus can neutralize the virus or facilitate its entry into the cell. If anti-S antibodies target a human-derived virus protein, they neutralize the virus. The same antibodies enhance virus entry into the cell if the virus has been adapted for growth in palm civet and acquired a mutated gene encoding the S-protein in the process of adaptation.
The ACE-2 receptor-binding domain of S-protein can mediate antibody-dependent virus entry. Five amino acid substitutions in the S-protein region from positions 248 to 501 in adapted human and civet viruses are probably responsible for this effect (60). Interesting, human immunodominant epitopes of the SARS-CoV-1 have been shown to cause both enhancing and neutralizing effects in non-human primates. In rhesus macaques, the S-protein peptides S471–503, S604–625, and S1164–1191 triggered antibodies that efficiently prevented infection. In contrast, peptide S597–603 elicited antibodies that enhanced infection both in vitro and in vivo (57).
Wang et al. noticed that two neutralizing monoclonal antibodies enhanced the ability of the SARS-CoV-2 pseudovirus to infect B-lymphocytes, but another neutralizing monoclonal antibody was not able to help the virus infect these cells (75). Interestingly, the antibody that was unable to cause ADE was capable to bind only “up” position of RBD of S-protein, while antibodies associated with ADE could bind to RBDs in S-trimer with both “up” and “down” states (75).
Certain epitopes of the S-protein are particularly prone to be targeted by antibodies that promote ADE. These observations were done by Zhou at al. using convalescent plasma from donors. The group revealed that enhancement versus neutralization by SARS-CoV-2 antibodies associates with distinct epitopes on the RBD of S-protein (127).
When studying the ADE phenomenon in vitro, researchers noticed the so-called antibody concentration effect, which perhaps is related to the fate of the virus-antibody complex inside the phagocytic cell (157) (Figure 5). This effect is that some antibodies can neutralize the virus in a wide range of concentrations and others in a narrow range; they can neutralize the virus at a high concentration but help the virus to infect cells at a lower concentration. Fc-mediated SARS-CoV-2 uptake by cell (causing ADE) might peak as neutralization potency of the relevant antibody decreases (129). In other words, ADE might happen at sub-neutralizing antibody concentrations.
Figure 5 Distinction between antibodies that are more or less likely to cause ADE. Antibodies capable and incapable of causing ADEs differ both at the level of epitope recognition and by the type of antibody itself. Thus, antibodies to certain antigenic epitopes are more prone to cause ADEs than antibodies directed at other viral epitopes. In addition, afucosylated antibodies are more likely to cause ADEs compared to fucosylated antibodies. However, even antibodies capable of inducing ADE at one concentration can neutralize the virus at another and be protective against the virus.
The existence of this effect indicates that the binding constants of viral antigenic epitopes to antibodies play a key role in determining whether an antibody will neutralize the virus or become an ADE trigger in complex with the virus.
There is evidence that the binding constant between the Fc region of an antibody and the Fc receptor of a phagocytic cell also plays an important role in promoting ADE. Antibody fucosylation lowers the FcγRIIIa/CD16a affinity (140). As was mentioned above patients with severe acute COVID-19 have increased concentration of antiviral IgGs that are afucosylated in their Fc region (141, 143, 144). These antibodies bind much better to CD16, and this enhanced binding probably facilitates antibody-mediated entry of SARS-CoV-2 cells into CD16+ immune cells, ultimately promoting infection of these cells (66).
How common are antibodies that are capable of ADE of SARS-CoV-2 in the human population? ADE-capable antibodies were found in almost half of the acute COVID-19 patients (156) and in a significant proportion of convalescent plasmas of recovered patients (129). Among 93 plasma samples tested, 90 were capable of inducing ADEs ex vivo (74).
Summarizing the above information, we can identify several scenarios of phagocyte interaction with the virus (Figure 6). The first scenario involves the normal, natural course of events, designed by evolution to protect hosts from pathogens. The virus in complex with antibodies is taken up by the phagocyte via the Fc-receptor and enters the endosome/phagosome, which fuses with the lysosome to form the endo- or phagolysosome. During this fusion process, the virus is inactivated and destroyed, its proteins are used for antigen presentation (161) or released from the cell by degranulation (Figure 6A). The second scenario involves the survival of the virus (Figure 6B), which might result in the phagocyte infection (Figure 6C). In such a case, nature has developed a backup plan that programs the phagocytic cell to commit suicide by pyroptosis (I). This cell program prevents infection spread and attracts other immune cells to a site of infection. However, mass cell pyroptosis can cause uncontrolled inflammation, tissue damage, and severe complications of the disease. Alternatively, a productive virus infection of an immune cell can occur (II). Finally, trans-infection is possible. Such infection occurs when immune cells deliver a replication-competent virus as a parcel to the permissive cells. The harm to the host organism from all of these scenarios is obvious.
Figure 6 Antibody-dependent phagocytosis of SARS-CoV-2 in norm and pathology. (A) The phagocyte destroys the internationalized pathogen through the phagolysosomal pathway and presents its antigens via the major histocompatibility complexes (MHC I and MHC II). (B) The phagocyte engulfs but cannot inactivate SARS-CoV-2 and becomes infected. It is likely that the infection occurs due to the synergistic action of cell entry receptors and Fc receptors. (C) The virus escapes from the endosome/phagosome and the escape results in (I) abortive or (II) productive viral infection.
Available information indicates that SARS-CoV-2 is capable of infecting of phagocytic immune cells. There is evidence that professional phagocytes, such as monocytes, macrophages, and dendritic cells, as well as nonprofessional ones, such as B cells, can be targets of viral infection, which can be abortive or productive. Viral entry can be direct via cell-entry receptors or mediated by antibodies and Fc-receptors of immune cells. Most likely both types of receptors act synergistically and cooperate in helping the virus to infect a phagocytic immune cell.
In addition, trans-infection of target cells with SARS-CoV-2 virus has been demonstrated. The virus can attach itself to the receptors of an immune cell, travel with this cell as a passenger, maintaining the status of replication ability, reach the permissive target cell and infect it.
Regardless of how a virus enters immune cells or travels with them, it can cause pathological reactions. They can manifest themselves in the spread of a viral infection and/or in mass death of phagocytic cells via pyroptosis, accompanied by uncontrolled inflammatory cascades.
The mechanisms of penetration of replication-competent SARS-CoV-2 into immune cells deserve careful study because there are many blind spots related to the identification of cell receptors, proteases, and other molecules that may promote this process.
The ability of the virus to cause ADE by infecting immune cells should be particularly studied. SARS-CoV-2 most likely uses multiple mechanisms to enter cells via Fc receptors and cause enhanced inflammation. We also have a poor understanding of why some antibodies may contribute to ADE and some may not. Perhaps the difference in conformational stability of S protein epitopes (162), which can be facilitated by lowering pH during pathological development of COVID-19 (163–165) plays a role in increasing the probability of ADE. The role of afucosylated antibodies in the ADE process also deserves special attention.
Finally, the most important question that must be addressed is how ADE affects the severity of COVID-19.
OM: Developing the idea of writing such a review, writing the main text, designing, and drawing illustrations. YN: Organizing regular manuscript discussions, conceptualization and reviewing the progress. DL: Writing the section “IgG afucosylation contributes to Fc-mediated virus phagocytic uptake, infection and disease severity, participation in regular discussion of the manuscript content. YY: Concept of introduction, outline of information presentation and design of Figure 4. JK: Concept and outline of information presentation, writing, the manuscript, design of tables. All authors contributed to the article and approved the submitted version.
This research was funded by the Tomsk State University Development Programme (Priority 20-30), and by the Program of Fundamental Research in the Russian Federation for 2021–2030 period (project No. 121052600299-1).
The authors are grateful to Dr. Dmitry Mazurov for his careful reading of the text and constructive criticism, which helped to significantly improve this review.
Authors OM and DL was employed by Sendai Viralytics, LLC.
The remaining authors declare that the research was conducted in the absence of any commercial or financial relationships that could be construed as a potential conflict of interest.
All claims expressed in this article are solely those of the authors and do not necessarily represent those of their affiliated organizations, or those of the publisher, the editors and the reviewers. Any product that may be evaluated in this article, or claim that may be made by its manufacturer, is not guaranteed or endorsed by the publisher.
The Supplementary Material for this article can be found online at: https://www.frontiersin.org/articles/10.3389/fimmu.2022.1050478/full#supplementary-material
1. Gordon S. Phagocytosis: An immunobiologic process. Immunity (2016) 44(3):463–75. doi: 10.1016/j.immuni.2016.02.026
2. Yutin N, Wolf MY, Wolf YI, Koonin EV. The origins of phagocytosis and eukaryogenesis. Biol Direct (2009) 4:9. doi: 10.1186/1745-6150-4-9
3. Mills DB. The origin of phagocytosis in earth history. Interface Focus (2020) 10(4):20200019. doi: 10.1098/rsfs.2020.0019
4. Rosales C, Uribe-Querol E. Phagocytosis: A fundamental process in immunity. BioMed Res Int (2017) 2017:9042851. doi: 10.1155/2017/9042851
5. Hortova-Kohoutkova M, Tidu F, De Zuani M, Sramek V, Helan M, Fric J. Phagocytosis-inflammation crosstalk in sepsis: New avenues for therapeutic intervention. Shock (2020) 54(5):606–14. doi: 10.1097/SHK.0000000000001541
6. Gordon S, Pluddemann A. Tissue macrophages: Heterogeneity and functions. BMC Biol (2017) 15(1):53. doi: 10.1186/s12915-017-0392-4
7. Uribe-Querol E, Rosales C. Phagocytosis: Our current understanding of a universal biological process. Front Immunol (2020) 11:1066. doi: 10.3389/fimmu.2020.01066
8. Roberts AD, Davenport TM, Dickey AM, Ahn R, Sochacki KA, Taraska JW. Structurally distinct endocytic pathways for b cell receptors in b lymphocytes. Mol Biol Cell (2020) 31(25):2826–40. doi: 10.1091/mbc.E20-08-0532
9. Zhu Q, Zhang M, Shi M, Liu Y, Zhao Q, Wang W, et al. Human b cells have an active phagocytic capability and undergo immune activation upon phagocytosis of mycobacterium tuberculosis. Immunobiology (2016) 221(4):558–67. doi: 10.1016/j.imbio.2015.12.003
10. Martinez-Riano A, Bovolenta ER, Mendoza P, Oeste CL, Martin-Bermejo MJ, Bovolenta P, et al. Antigen phagocytosis by b cells is required for a potent humoral response. EMBO Rep (2018) 19(9):e46016. doi: 10.15252/embr.201846016
11. Ghigo E. A dilemma for viruses and giant viruses: Which endocytic pathway to use to enter cells? Intervirology (2010) 53(5):274–83. doi: 10.1159/000312912
12. Cossart P, Helenius A. Endocytosis of viruses and bacteria. Cold Spring Harb Perspect Biol (2014) 6(8):a016972. doi: 10.1101/cshperspect.a016972
13. Grove J, Marsh M. The cell biology of receptor-mediated virus entry. J Cell Biol (2011) 195(7):1071–82. doi: 10.1083/jcb.201108131
14. Bayati A, Kumar R, Francis V, McPherson PS. Sars-Cov-2 infects cells after viral entry Via clathrin-mediated endocytosis. J Biol Chem (2021) 296:100306. doi: 10.1016/j.jbc.2021.100306
15. Nikitina E, Larionova I, Choinzonov E, Kzhyshkowska J. Monocytes and macrophages as viral targets and reservoirs. Int J Mol Sci (2018) 19(9):2821. doi: 10.3390/ijms19092821
16. Plemper RK. Cell entry of enveloped viruses. Curr Opin Virol (2011) 1(2):92–100. doi: 10.1016/j.coviro.2011.06.002
17. Cosset FL, Lavillette D. Cell entry of enveloped viruses. Adv Genet (2011) 73:121–83. doi: 10.1016/B978-0-12-380860-8.00004-5
18. Khan N, Chen X, Geiger JD. Role of endolysosomes in severe acute respiratory syndrome coronavirus-2 infection and coronavirus disease 2019 pathogenesis: Implications for potential treatments. Front Pharmacol (2020) 11:595888. doi: 10.3389/fphar.2020.595888
19. Jackson CB, Farzan M, Chen B, Choe H. Mechanisms of sars-Cov-2 entry into cells. Nat Rev Mol Cell Biol (2022) 23(1):3–20. doi: 10.1038/s41580-021-00418-x
20. Burkard C, Verheije MH, Wicht O, van Kasteren SI, van Kuppeveld FJ, Haagmans BL, et al. Coronavirus cell entry occurs through the endo-/Lysosomal pathway in a proteolysis-dependent manner. PLoS Pathog (2014) 10(11):e1004502. doi: 10.1371/journal.ppat.1004502
21. Song X, Hu W, Yu H, Zhao L, Zhao Y, Zhao X, et al. Little to no expression of angiotensin-converting enzyme-2 on most human peripheral blood immune cells but highly expressed on tissue macrophages. Cytometry A (2020) 1–10. doi: 10.1002/cyto.a.24285
22. Sefik E, Qu R, Junqueira C, Kaffe E, Mirza H, Zhao J, et al. Inflammasome activation in infected macrophages drives covid-19 pathology. Nature (2022) 606:585–93. doi: 10.1038/s41586-022-04802-1
23. Hu W, Song X, Yu H, Zhao L, Zhao Y, Zhao Y. Further comments on the role of ace-2 positive macrophages in human lung. Cytometry A (2021) 1–7. doi: 10.1002/cyto.a.24484
24. Rutkowska-Zapala M, Suski M, Szatanek R, Lenart M, Weglarczyk K, Olszanecki R, et al. Human monocyte subsets exhibit divergent angiotensin I-converting activity. Clin Exp Immunol (2015) 181(1):126–32. doi: 10.1111/cei.12612
25. Grant RA, Morales-Nebreda L, Markov NS, Swaminathan S, Querrey M, Guzman ER, et al. Circuits between infected macrophages and T cells in sars-Cov-2 pneumonia. Nature (2021) 590(7847):635–41. doi: 10.1038/s41586-020-03148-w
26. Delorey TM, Ziegler CGK, Heimberg G, Normand R, Yang Y, Segerstolpe A, et al. Covid-19 tissue atlases reveal sars-Cov-2 pathology and cellular targets. Nature (2021) 595(7865):107–13. doi: 10.1038/s41586-021-03570-8
27. Zhang Q, Xiang R, Huo S, Zhou Y, Jiang S, Wang Q, et al. Molecular mechanism of interaction between sars-Cov-2 and host cells and interventional therapy. Signal Transduct Target Ther (2021) 6(1):233. doi: 10.1038/s41392-021-00653-w
28. Thepaut M, Luczkowiak J, Vives C, Labiod N, Bally I, Lasala F, et al. Dc/L-sign recognition of spike glycoprotein promotes sars-Cov-2 trans-infection and can be inhibited by a glycomimetic antagonist. PLoS Pathog (2021) 17(5):e1009576. doi: 10.1371/journal.ppat.1009576
29. Lempp FA, Soriaga LB, Montiel-Ruiz M, Benigni F, Noack J, Park YJ, et al. Lectins enhance sars-Cov-2 infection and influence neutralizing antibodies. Nature (2021) 598(7880):342–7. doi: 10.1038/s41586-021-03925-1
30. Gu Y, Cao J, Zhang X, Gao H, Wang Y, Wang J, et al. Receptome profiling identifies Kremen1 and Asgr1 as alternative functional receptors of sars-Cov-2. Cell Res (2022) 32(1):24–37. doi: 10.1038/s41422-021-00595-6
31. Hoffmann M, Pöhlmann S. Novel sars-Cov-2 receptors: Asgr1 and Kremen1. Cell Res (2022) 32(1):1–2. doi: 10.1038/s41422-021-00603-9
32. Cantuti-Castelvetri L, Ojha R, Pedro LD, Djannatian M, Franz J, Kuivanen S, et al. Neuropilin-1 facilitates sars-Cov-2 cell entry and infectivity. Science (2020) 370(6518):856–60. doi: 10.1126/science.abd2985
33. Wang S, Qiu Z, Hou Y, Deng X, Xu W, Zheng T, et al. Axl is a candidate receptor for sars-Cov-2 that promotes infection of pulmonary and bronchial epithelial cells. Cell Res (2021) 31(2):126–40. doi: 10.1038/s41422-020-00460-y
34. Wang K, Chen W, Zhang Z, Deng Y, Lian JQ, Du P, et al. Cd147-spike protein is a novel route for sars-Cov-2 infection to host cells. Signal Transduct Target Ther (2020) 5(1):283. doi: 10.1038/s41392-020-00426-x
35. Behl T, Kaur I, Aleya L, Sehgal A, Singh S, Sharma N, et al. Cd147-spike protein interaction in covid-19: Get the ball rolling with a novel receptor and therapeutic target. Sci Total Environ (2022) 808:152072. doi: 10.1016/j.scitotenv.2021.152072
36. Li Y, Zhang Z, Yang L, Lian X, Xie Y, Li S, et al. The mers-cov receptor Dpp4 as a candidate binding target of the sars-Cov-2 spike. iScience (2020) 23(8):101400. doi: 10.1016/j.isci.2020.101400
37. O'Neill AS, van den Berg TK, Mullen GE. Sialoadhesin - a macrophage-restricted marker of immunoregulation and inflammation. Immunology (2013) 138(3):198–207. doi: 10.1111/imm.12042
38. Soilleux EJ, Morris LS, Leslie G, Chehimi J, Luo Q, Levroney E, et al. Constitutive and induced expression of dc-sign on dendritic cell and macrophage subpopulations in situ and in vitro. J Leukoc Biol (2002) 71(3):445–57. doi: 10.1189/jlb.71.3.445
39. Geijtenbeek TBH, Gringhuis SI. Signalling through c-type lectin receptors: Shaping immune responses. Nat Rev Immunol (2009) 9(7):465–79. doi: 10.1038/nri2569
40. Harris RL, van den Berg CW, Bowen DJ. Asgr1 and Asgr2, the genes that encode the asialoglycoprotein receptor (Ashwell receptor), are expressed in peripheral blood monocytes and show interindividual differences in transcript profile. Mol Biol Int (2012) 2012:283974. doi: 10.1155/2012/283974
41. Protein-Atlas-Database. Available at: https://www.Proteinatlas.Org/Ensg00000183762-Kremen1 (Accessed July 12, 2022).
42. Roy S, Bag AK, Singh RK, Talmadge JE, Batra SK, Datta K. Multifaceted role of neuropilins in the immune system: Potential targets for immunotherapy. Front Immunol (2017) 8:1228. doi: 10.3389/fimmu.2017.01228
43. Lemke G. Biology of the Tam receptors. Cold Spring Harb Perspect Biol (2013) 5(11):a009076. doi: 10.1101/cshperspect.a009076
44. DeBerge M, Glinton K, Subramanian M, Wilsbacher LD, Rothlin CV, Tabas I, et al. Macrophage axl receptor tyrosine kinase inflames the heart after reperfused myocardial infarction. J Clin Invest (2021) 131(6):e139576. doi: 10.1172/JCI139576
45. Protein-Atlas-Database. Available at: https://www.Proteinatlas.Org/Ensg00000167601-Axl/Immune+Cell (Accessed July 12, 2022).
46. Zhu X, Song Z, Zhang S, Nanda A, Li G. Cd147: A novel modulator of inflammatory and immune disorders. Curr Med Chem (2014) 21(19):2138–45. doi: 10.2174/0929867321666131227163352
47. Shao S, Xu Q, Yu X, Pan R, Chen Y. Dipeptidyl peptidase 4 inhibitors and their potential immune modulatory functions. Pharmacol Ther (2020) 209:107503. doi: 10.1016/j.pharmthera.2020.107503
48. Zheng M, Karki R, Williams EP, Yang D, Fitzpatrick E, Vogel P, et al. Tlr2 senses the sars-Cov-2 envelope protein to produce inflammatory cytokines. Nat Immunol (2021) 22(7):829–38. doi: 10.1038/s41590-021-00937-x
49. Protein-Atlas-Database. Available at: https://www.Proteinatlas.Org/Ensg00000137462-Tlr2 (Accessed July 27, 2022).
50. Devaprasad A, Pandit A. Enrichment of sars-Cov-2 entry factors and interacting intracellular genes in tissue and circulating immune cells. Viruses (2021) 13(9):1757. doi: 10.3390/v13091757
51. Perisic Nanut M, Sabotic J, Jewett A, Kos J. Cysteine cathepsins as regulators of the cytotoxicity of nk and T cells. Front Immunol (2014) 5:616. doi: 10.3389/fimmu.2014.00616
52. Klepper A, Branch AD. Macrophages and the viral dissemination super highway. EC Microbiol (2015) 2(3):328–36
53. Hohdatsu T, Yamada M, Tominaga R, Makino K, Kida K, Koyama H. Antibody-dependent enhancement of feline infectious peritonitis virus infection in feline alveolar macrophages and human monocyte cell line U937 by serum of cats experimentally or naturally infected with feline coronavirus. J Vet Med Sci (1998) 60(1):49–55. doi: 10.1292/jvms.60.49
54. Takano T, Tomiyama Y, Katoh Y, Nakamura M, Satoh R, Hohdatsu T. Mutation of Neutralizing/Antibody-dependent enhancing epitope on spike protein and 7b gene of feline infectious peritonitis virus: Influences of viral replication in Monocytes/Macrophages and virulence in cats. Virus Res (2011) 156(1-2):72–80. doi: 10.1016/j.virusres.2010.12.020
55. Wen J, Cheng Y, Ling R, Dai Y, Huang B, Huang W, et al. Antibody-dependent enhancement of coronavirus. Int J Infect Dis (2020) 100:483–9. doi: 10.1016/j.ijid.2020.09.015
56. Jaume M, Yip MS, Kam YW, Cheung CY, Kien F, Roberts A, et al. Sars cov subunit vaccine: Antibody-mediated neutralisation and enhancement. Hong Kong Med J (2012) 18(Suppl 2):31–6.
57. Wang Q, Zhang L, Kuwahara K, Li L, Liu Z, Li T, et al. Immunodominant sars coronavirus epitopes in humans elicited both enhancing and neutralizing effects on infection in non-human primates. ACS Infect Dis (2016) 2(5):361–76. doi: 10.1021/acsinfecdis.6b00006
58. Liu L, Wei Q, Lin Q, Fang J, Wang H, Kwok H, et al. Anti-spike igg causes severe acute lung injury by skewing macrophage responses during acute sars-cov infection. JCI Insight (2019) 4(4):e123158. doi: 10.1172/jci.insight.123158
59. Yip MS, Leung HL, Li PH, Cheung CY, Dutry I, Li D, et al. Antibody-dependent enhancement of sars coronavirus infection and its role in the pathogenesis of sars. Hong Kong Med J (2016) 22(3 Suppl 4):25–31.
60. Jafarzadeh A, Chauhan P, Saha B, Jafarzadeh S, Nemati M. Contribution of monocytes and macrophages to the local tissue inflammation and cytokine storm in covid-19: Lessons from sars and mers, and potential therapeutic interventions. Life Sci (2020) 257:118102. doi: 10.1016/j.lfs.2020.118102
61. Feng Z, Diao B, Wang R, Wang G, Wang C, Tan Y, et al. The novel severe acute respiratory syndrome coronavirus 2 (Sars-Cov-2) directly decimates human spleens and lymph nodes. medRxiv (2020). doi: 10.1101/2020.03.27.20045427
62. Szekely L, Bozoky B, Bendek M, Ostad M, Lavignasse P, Haag L, et al. Pulmonary stromal expansion and intra-alveolar coagulation are primary causes of covid-19 death. Heliyon (2021) 7(5):e07134. doi: 10.1016/j.heliyon.2021.e07134
63. Ramos da Silva S, Ju E, Meng W, Paniz Mondolfi AE, Dacic S, Green A, et al. Broad severe acute respiratory syndrome coronavirus 2 cell tropism and immunopathology in lung tissues from fatal coronavirus disease 2019. J Infect Dis (2021) 223(11):1842–54. doi: 10.1093/infdis/jiab195
64. Pontelli MC, Castro IA, Martins RB, Veras FP, Serra L, Nascimento DC, et al. Sars-Cov-2 productively infects primary human immune system cells in vitro and in covid-19 patients. J Mol Cell Biol (2022) 14(4):mjac021. doi: 10.1093/jmcb/mjac021
65. Martínez-Colón GJ, Ratnasiri K, Chen H, Jiang S, Zanley E, Rustagi A, et al. Sars-Cov-2 infects human adipose tissue and elicits an inflammatory response consistent with severe covid-19. bioRxiv (2021). doi: 10.1101/2021.10.24.465626
66. Junqueira C, Crespo Â, Ranjbar S, de Lacerda LB, Lewandrowski M, Ingber J, et al. Fcγr-mediated sars-Cov-2 infection of monocytes activates inflammation. Nature (2022) 606(7914):576–84. doi: 10.1038/s41586-022-04702-4
67. Lv J, Wang Z, Qu Y, Zhu H, Zhu Q, Tong W, et al. Distinct uptake, amplification, and release of sars-Cov-2 by M1 and M2 alveolar macrophages. Cell Discov (2021) 7(1):24. doi: 10.1038/s41421-021-00258-1
68. Zheng J, Wang Y, Li K, Meyerholz DK, Allamargot C, Perlman S. Severe acute respiratory syndrome coronavirus 2-induced immune activation and death of monocyte-derived human macrophages and dendritic cells. J Infect Dis (2021) 223(5):785–95. doi: 10.1093/infdis/jiaa753
69. Boumaza A, Gay L, Mezouar S, Bestion E, Diallo AB, Michel M, et al. Monocytes and macrophages, targets of severe acute respiratory syndrome coronavirus 2: The clue for coronavirus disease 2019 immunoparalysis. J Infect Dis (2021) 224(3):395–406. doi: 10.1093/infdis/jiab044
70. Ferreira AC, Soares VC, de Azevedo-Quintanilha IG, Dias S, Fintelman-Rodrigues N, Sacramento CQ, et al. Sars-Cov-2 engages inflammasome and pyroptosis in human primary monocytes. Cell Death Discov (2021) 7(1):43. doi: 10.1038/s41420-021-00428-w
71. Rodrigues TS, de Sa KSG, Ishimoto AY, Becerra A, Oliveira S, Almeida L, et al. Inflammasomes are activated in response to sars-Cov-2 infection and are associated with covid-19 severity in patients. J Exp Med (2021) 218(3):e20201707. doi: 10.1084/jem.20201707
72. Shen XR, Li Q, Li HL, Wang X, Wang Q, Zheng XS, et al. Antibody-dependent enhancement of sars-Cov-2 infection of human immune cells: In vitro assessment provides insight in covid-19 pathogenesis. Viruses (2021) 13(12):2483. doi: 10.3390/v13122483
73. Laurent P, Yang C, Rendeiro AF, Nilsson-Payant BE, Carrau L, Chandar V, et al. Sensing of sars-Cov-2 by pdcs and their subsequent production of ifn-I contribute to macrophage-induced cytokine storm during covid-19. Sci Immunol (2022) 7(75):eadd4906. doi: 10.1126/sciimmunol.add4906
74. Wang Z, Deng T, Zhang Y, Niu W, Nie Q, Yang S, et al. Ace2 can act as the secondary receptor in the fcgammar-dependent ade of sars-Cov-2 infection. iScience (2022) 25(1):103720. doi: 10.1016/j.isci.2021.103720
75. Wang S, Wang J, Yu X, Jiang W, Chen S, Wang R, et al. Antibody-dependent enhancement (Ade) of sars-Cov-2 pseudoviral infection requires fcgammariib and virus-antibody complex with bivalent interaction. Commun Biol (2022) 5(1):262. doi: 10.1038/s42003-022-03207-0
76. Parihar A, Eubank TD, Doseff AI. Monocytes and macrophages regulate immunity through dynamic networks of survival and cell death. J Innate Immun (2010) 2(3):204–15. doi: 10.1159/000296507
77. Mosig S, Rennert K, Krause S, Kzhyshkowska J, Neunubel K, Heller R, et al. Different functions of monocyte subsets in familial hypercholesterolemia: Potential function of Cd14+ Cd16+ monocytes in detoxification of oxidized ldl. FASEB J (2009) 23(3):866–74. doi: 10.1096/fj.08-118240
78. Yang J, Zhang L, Yu C, Yang XF, Wang H. Monocyte and macrophage differentiation: Circulation inflammatory monocyte as biomarker for inflammatory diseases. biomark Res (2014) 2(1):1. doi: 10.1186/2050-7771-2-1
79. Patel AA, Zhang Y, Fullerton JN, Boelen L, Rongvaux A, Maini AA, et al. The fate and lifespan of human monocyte subsets in steady state and systemic inflammation. J Exp Med (2017) 214(7):1913–23. doi: 10.1084/jem.20170355
80. Underhill DM, Gordon S, Imhof BA, Nunez G, Bousso P. Elie metchnikoff (1845-1916): Celebrating 100 years of cellular immunology and beyond. Nat Rev Immunol (2016) 16(10):651–6. doi: 10.1038/nri.2016.89
81. Larionova I, Tuguzbaeva G, Ponomaryova A, Stakheyeva M, Cherdyntseva N, Pavlov V, et al. Tumor-associated macrophages in human breast, colorectal, lung, ovarian and prostate cancers. Front Oncol (2020) 10:566511. doi: 10.3389/fonc.2020.566511
82. Rendra E, Riabov V, Mossel DM, Sevastyanova T, Harmsen MC, Kzhyshkowska J. Reactive oxygen species (Ros) in macrophage activation and function in diabetes. Immunobiology (2019) 224(2):242–53. doi: 10.1016/j.imbio.2018.11.010
83. Russo S, Kwiatkowski M, Govorukhina N, Bischoff R, Melgert BN. Meta-inflammation and metabolic reprogramming of macrophages in diabetes and obesity: The importance of metabolites. Front Immunol (2021) 12:746151. doi: 10.3389/fimmu.2021.746151
84. Sun X, Li Y, Deng Q, Hu Y, Dong J, Wang W, et al. Macrophage polarization, metabolic reprogramming, and inflammatory effects in ischemic heart disease. Front Immunol (2022) 13:934040. doi: 10.3389/fimmu.2022.934040
85. Torres-Castro I, Arroyo-Camarena UD, Martinez-Reyes CP, Gomez-Arauz AY, Duenas-Andrade Y, Hernandez-Ruiz J, et al. Human monocytes and macrophages undergo M1-type inflammatory polarization in response to high levels of glucose. Immunol Lett (2016) 176:81–9. doi: 10.1016/j.imlet.2016.06.001
86. Moganti K, Li F, Schmuttermaier C, Riemann S, Kluter H, Gratchev A, et al. Hyperglycemia induces mixed M1/M2 cytokine profile in primary human monocyte-derived macrophages. Immunobiology (2017) 222(10):952–9. doi: 10.1016/j.imbio.2016.07.006
87. Manjarrez-Reyna AN, Martinez-Reyes CP, Aguayo-Guerrero JA, Mendez-Garcia LA, Esquivel-Velazquez M, Leon-Cabrera S, et al. Native low-density lipoproteins act in synergy with lipopolysaccharide to alter the balance of human monocyte subsets and their ability to produce il-1 beta, Ccr2, and Cx3cr1 in vitro and in vivo: Implications in atherogenesis. Biomolecules (2021) 11(8):1169. doi: 10.3390/biom11081169
88. Xu L, Yan X, Zhao Y, Wang J, Liu B, Yu S, et al. Macrophage polarization mediated by mitochondrial dysfunction induces adipose tissue inflammation in obesity. Int J Mol Sci (2022) 23(16):9252. doi: 10.3390/ijms23169252
89. Puschel GP, Klauder J, Henkel J. Macrophages, low-grade inflammation, insulin resistance and hyperinsulinemia: A mutual ambiguous relationship in the development of metabolic diseases. J Clin Med (2022) 11(15):9252. doi: 10.3390/jcm11154358
90. Viurcos-Sanabria R, Escobedo G. Immunometabolic bases of type 2 diabetes in the severity of covid-19. World J Diabetes (2021) 12(7):1026–41. doi: 10.4239/wjd.v12.i7.1026
91. Kzhyshkowska J, Venketaraman V, Escobedo G. Editorial: Immunometabolic mechanisms underlying the severity of covid-19. Front Immunol (2022) 13:977907. doi: 10.3389/fimmu.2022.977907
92. Mitsui Y, Suzuki T, Kuniyoshi K, Inamo J, Yamaguchi K, Komuro M, et al. M2c macrophages hijacked by sars-Cov-2 cause covid-19 severity. bioRxiv (2022). doi: 10.1101/2022.09.30.510331
93. Knoll R, Schultze JL, Schulte-Schrepping J. Monocytes and macrophages in covid-19. Front Immunol (2021) 12:720109. doi: 10.3389/fimmu.2021.720109
94. Kosyreva A, Dzhalilova D, Lokhonina A, Vishnyakova P, Fatkhudinov T. The role of macrophages in the pathogenesis of sars-Cov-2-Associated acute respiratory distress syndrome. Front Immunol (2021) 12:682871. doi: 10.3389/fimmu.2021.682871
95. Cabeza-Cabrerizo M, Cardoso A, Minutti CM, Pereira da Costa M, Reis e Sousa C. Dendritic cells revisited. Annu Rev Immunol (2021) 39:131–66. doi: 10.1146/annurev-immunol-061020-053707
96. Zhan Y, Chow KV, Soo P, Xu Z, Brady JL, Lawlor KE, et al. Plasmacytoid dendritic cells are short-lived: Reappraising the influence of migration, genetic factors and activation on estimation of lifespan. Sci Rep (2016) 6(1):25060. doi: 10.1038/srep25060
97. Law HK, Cheung CY, Ng HY, Sia SF, Chan YO, Luk W, et al. Chemokine up-regulation in sars-Coronavirus-Infected, monocyte-derived human dendritic cells. Blood (2005) 106(7):2366–74. doi: 10.1182/blood-2004-10-4166
98. Law HK, Cheung CY, Sia SF, Chan YO, Peiris JS, Lau YL. Toll-like receptors, chemokine receptors and death receptor ligands responses in sars coronavirus infected human monocyte derived dendritic cells. BMC Immunol (2009) 10:35. doi: 10.1186/1471-2172-10-35
99. McDonald D. Dendritic cells and hiv-1 trans-infection. Viruses (2010) 2(8):1704–17. doi: 10.3390/v2081704
100. Yang ZY, Huang Y, Ganesh L, Leung K, Kong WP, Schwartz O, et al. Ph-dependent entry of severe acute respiratory syndrome coronavirus is mediated by the spike glycoprotein and enhanced by dendritic cell transfer through dc-sign. J Virol (2004) 78(11):5642–50. doi: 10.1128/JVI.78.11.5642-5650.2004
101. Jones DD, Wilmore JR, Allman D. Cellular dynamics of memory b cell populations: Igm+ and igg+ memory b cells persist indefinitely as quiescent cells. J Immunol (2015) 195(10):4753–9. doi: 10.4049/jimmunol.1501365
102. Kam YW, Kien F, Roberts A, Cheung YC, Lamirande EW, Vogel L, et al. Antibodies against trimeric s glycoprotein protect hamsters against sars-cov challenge despite their capacity to mediate fcgammarii-dependent entry into b cells in vitro. Vaccine (2007) 25(4):729–40. doi: 10.1016/j.vaccine.2006.08.011
103. Lee HJ, Woo Y, Hahn TW, Jung YM, Jung YJ. Formation and maturation of the phagosome: A key mechanism in innate immunity against intracellular bacterial infection. Microorganisms (2020) 8(9):1298. doi: 10.3390/microorganisms8091298
104. Levin R, Grinstein S, Canton J. The life cycle of phagosomes: Formation, maturation, and resolution. Immunol Rev (2016) 273(1):156–79. doi: 10.1111/imr.12439
105. Nguyen JA, Yates RM. Better together: Current insights into phagosome-lysosome fusion. Front Immunol (2021) 12:636078. doi: 10.3389/fimmu.2021.636078
106. Prabhakara C, Godbole R, Sil P, Jahnavi S, Gulzar SE, van Zanten TS, et al. Strategies to target sars-Cov-2 entry and infection using dual mechanisms of inhibition by acidification inhibitors. PLoS Pathog (2021) 17(7):e1009706. doi: 10.1371/journal.ppat.1009706
107. Kreutzberger AJB, Sanyal A, Saminathan A, Bloyet LM, Stumpf S, Liu Z, et al. Sars-Cov-2 requires acidic ph to infect cells. Proc Natl Acad Sci USA (2022) 119(38):e2209514119. doi: 10.1073/pnas.2209514119
108. Park MD. Macrophages: A Trojan horse in covid-19? Nat Rev Immunol (2020) 20(6):351. doi: 10.1038/s41577-020-0317-2
109. Percivalle E, Sammartino JC, Cassaniti I, Arbustini E, Urtis M, Smirnova A, et al. Macrophages and monocytes: "Trojan horses" in covid-19. Viruses (2021) 13(11):2178. doi: 10.3390/v13112178
110. Jorgensen I, Miao EA. Pyroptotic cell death defends against intracellular pathogens. Immunol Rev (2015) 265(1):130–42. doi: 10.1111/imr.12287
111. Frank D, Vince JE. Pyroptosis versus necroptosis: Similarities, differences, and crosstalk. Cell Death Differ (2019) 26(1):99–114. doi: 10.1038/s41418-018-0212-6
112. Winsor N, Krustev C, Bruce J, Philpott DJ, Girardin SE. Canonical and noncanonical inflammasomes in intestinal epithelial cells. Cell Microbiol (2019) 21(11):e13079. doi: 10.1111/cmi.13079
113. Shrivastava G, Leon-Juarez M, Garcia-Cordero J, Meza-Sanchez DE, Cedillo-Barron L. Inflammasomes and its importance in viral infections. Immunol Res (2016) 64(5-6):1101–17. doi: 10.1007/s12026-016-8873-z
114. Kuriakose T, Kanneganti TD. Pyroptosis in antiviral immunity. Curr Top Microbiol Immunol (2019). Springer, Berlin/Heidelberg, Germany. doi: 10.1007/82_2019_189
115. Broz P, Dixit VM. Inflammasomes: Mechanism of assembly, regulation and signalling. Nat Rev Immunol (2016) 16(7):407–20. doi: 10.1038/nri.2016.58
116. Ma J, Zhu F, Zhao M, Shao F, Yu D, Ma J, et al. Sars-Cov-2 nucleocapsid suppresses host pyroptosis by blocking gasdermin d cleavage. EMBO J (2021) 40(18):e108249. doi: 10.15252/embj.2021108249
117. Cheung CY, Poon LL, Ng IH, Luk W, Sia SF, Wu MH, et al. Cytokine responses in severe acute respiratory syndrome coronavirus-infected macrophages in vitro: Possible relevance to pathogenesis. J Virol (2005) 79(12):7819–26. doi: 10.1128/JVI.79.12.7819-7826.2005
118. Zhang Y, Dong Z, Song W. Nlrp3 inflammasome as a novel therapeutic target for alzheimer's disease. Signal Transduct Target Ther (2020) 5(1):37. doi: 10.1038/s41392-020-0145-7
119. Takada A, Kawaoka Y. Antibody-dependent enhancement of viral infection: Molecular mechanisms and in vivo implications. Rev Med Virol (2003) 13(6):387–98. doi: 10.1002/rmv.405
120. Tirado SM, Yoon KJ. Antibody-dependent enhancement of virus infection and disease. Viral Immunol (2003) 16(1):69–86. doi: 10.1089/088282403763635465
121. Taylor A, Foo SS, Bruzzone R, Dinh LV, King NJ, Mahalingam S. Fc receptors in antibody-dependent enhancement of viral infections. Immunol Rev (2015) 268(1):340–64. doi: 10.1111/imr.12367
122. Kulkarni R. Antibody-dependent enhancement of viral infections. Springer, Berlin/Heidelberg, Germany (2019). doi: 10.1007/978-981-15-1045-8_2
123. Ajmeriya S, Kumar A, Karmakar S, Rana S, Singh H. Neutralizing antibodies and antibody-dependent enhancement in covid-19: A perspective. J Indian Institute Sci (2022) 102(2):671–87. doi: 10.1007/s41745-021-00268-8
124. Yang. Antibody-dependent enhancement: ″Evil″ antibodies favorable for viral infections. Viruses (2022) 14(8):1739. doi: 10.3390/v14081739
125. Cloutier M, Nandi M, Ihsan AU, Chamard HA, Ilangumaran S, Ramanathan S. Ade and hyperinflammation in sars-Cov2 infection- comparison with dengue hemorrhagic fever and feline infectious peritonitis. Cytokine (2020) 136:155256. doi: 10.1016/j.cyto.2020.155256
126. Shukla AK, Misra S. Antibody-dependent enhancement of virus infection and disease: Implications in covid-19. J Basic Clin Physiol Pharmacol (2022) 33(1):13–6. doi: 10.1515/jbcpp-2021-0264
127. Zhou Y, Liu Z, Li S, Xu W, Zhang Q, Silva IT, et al. Enhancement versus neutralization by sars-Cov-2 antibodies from a convalescent donor associates with distinct epitopes on the rbd. Cell Rep (2021) 34(5):108699. doi: 10.1016/j.celrep.2021.108699
128. Sanchez-Zuno GA, Matuz-Flores MG, Gonzalez-Estevez G, Nicoletti F, Turrubiates-Hernandez FJ, Mangano K, et al. A review: Antibody-dependent enhancement in covid-19: The not so friendly side of antibodies. Int J Immunopathol Pharmacol (2021) 35:20587384211050199. doi: 10.1177/20587384211050199
129. Wieczorek L, Zemil M, Merbah M, Dussupt V, Kavusak E, Molnar S, et al. Evaluation of antibody-dependent fc-mediated viral entry, as compared with neutralization, in sars-Cov-2 infection. Front Immunol (2022) 13:901217. doi: 10.3389/fimmu.2022.901217
130. Pang T, Cardosa MJ, Guzman MG. Of cascades and perfect storms: The immunopathogenesis of dengue haemorrhagic fever-dengue shock syndrome (Dhf/Dss). Immunol Cell Biol (2007) 85(1):43–5. doi: 10.1038/sj.icb.7100008
131. Bournazos S, Gupta A, Ravetch JV. The role of igg fc receptors in antibody-dependent enhancement. Nat Rev Immunol (2020) 20(10):633–43. doi: 10.1038/s41577-020-00410-0
132. Zanella I, Degli Antoni M, Marchese V, Castelli F, Quiros-Roldan E. Non-neutralizing antibodies: Deleterious or propitious during sars-Cov-2 infection? Int Immunopharmacol (2022) 110:108943. doi: 10.1016/j.intimp.2022.108943
133. Junker F, Gordon J, Qureshi O. Fc gamma receptors and their role in antigen uptake, presentation, and T cell activation. Front Immunol (2020) 11:1393. doi: 10.3389/fimmu.2020.01393
134. Yuan FF, Tanner J, Chan PK, Biffin S, Dyer WB, Geczy AF, et al. Influence of fcgammariia and mbl polymorphisms on severe acute respiratory syndrome. Tissue Antigens (2005) 66(4):291–6. doi: 10.1111/j.1399-0039.2005.00476.x
135. Hayes JM, Wormald MR, Rudd PM, Davey GP. Fc gamma receptors: Glycobiology and therapeutic prospects. J Inflamm Res (2016) 9:209–19. doi: 10.2147/JIR.S121233
136. Guilliams M, Bruhns P, Saeys Y, Hammad H, Lambrecht BN. The function of fcgamma receptors in dendritic cells and macrophages. Nat Rev Immunol (2014) 14(2):94–108. doi: 10.1038/nri3582
137. Maverakis E, Kim K, Shimoda M, Gershwin ME, Patel F, Wilken R, et al. Glycans in the immune system and the altered glycan theory of autoimmunity: A critical review. J Autoimmun (2015) 57:1–13. doi: 10.1016/j.jaut.2014.12.002
138. Nimmerjahn F, Ravetch JV. Fcγ receptors as regulators of immune responses. Nat Rev Immunol (2008) 8(1):34–47. doi: 10.1038/nri2206
139. Wan Y, Shang J, Sun S, Tai W, Chen J, Geng Q, et al. Molecular mechanism for antibody-dependent enhancement of coronavirus entry. J Virol (2020) 94(5):e02015-19. doi: 10.1128/JVI.02015-19
140. Falconer DJ, Subedi GP, Marcella AM, Barb AW. Antibody fucosylation lowers the Fcgammariiia/Cd16a affinity by limiting the conformations sampled by the N162-glycan. ACS Chem Biol (2018) 13(8):2179–89. doi: 10.1021/acschembio.8b00342
141. Larsen MD, de Graaf EL, Sonneveld ME, Plomp HR, Nouta J, Hoepel W, et al. Afucosylated igg characterizes enveloped viral responses and correlates with covid-19 severity. Science (2021) 371(6532):eabc8378. doi: 10.1126/science.abc8378
142. Oosterhoff JJ, Larsen MD, van der Schoot CE, Vidarsson G. Afucosylated igg responses in humans - structural clues to the regulation of humoral immunity. Trends Immunol (2022) 43(10):800–14. doi: 10.1016/j.it.2022.08.001
143. Chakraborty S, Gonzalez J, Edwards K, Mallajosyula V, Buzzanco AS, Sherwood R, et al. Proinflammatory igg fc structures in patients with severe covid-19. Nat Immunol (2021) 22(1):67–73. doi: 10.1038/s41590-020-00828-7
144. Hoepel W, Chen HJ, Geyer CE, Allahverdiyeva S, Manz XD, de Taeye SW, et al. High titers and low fucosylation of early human anti-Sars-Cov-2 igg promote inflammation by alveolar macrophages. Sci Transl Med (2021) 13(596):eabf8654. doi: 10.1126/scitranslmed.abf8654
145. Chakraborty S, Gonzalez JC, Sievers BL, Mallajosyula V, Chakraborty S, Dubey M, et al. Early non-neutralizing, afucosylated antibody responses are associated with covid-19 severity. Sci Transl Med (2022) 14(635):eabm7853. doi: 10.1126/scitranslmed.abm7853
146. Matsumiya S, Yamaguchi Y, Saito J, Nagano M, Sasakawa H, Otaki S, et al. Structural comparison of fucosylated and nonfucosylated fc fragments of human immunoglobulin G1. J Mol Biol (2007) 368(3):767–79. doi: 10.1016/j.jmb.2007.02.034
147. Cambay F, Raymond C, Brochu D, Gilbert M, Tu TM, Cantin C, et al. Impact of Igg1 n-glycosylation on their interaction with fc gamma receptors. Curr Res Immunol (2020) 1:23–37. doi: 10.1016/j.crimmu.2020.06.001
148. Shields RL, Lai J, Keck R, O'Connell LY, Hong K, Meng YG, et al. Lack of fucose on human Igg1 n-linked oligosaccharide improves binding to human fcgamma riii and antibody-dependent cellular toxicity. J Biol Chem (2002) 277(30):26733–40. doi: 10.1074/jbc.M202069200
149. Kapur R, Kustiawan I, Vestrheim A, Koeleman CA, Visser R, Einarsdottir HK, et al. A prominent lack of Igg1-fc fucosylation of platelet alloantibodies in pregnancy. Blood (2014) 123(4):471–80. doi: 10.1182/blood-2013-09-527978
150. Wuhrer M, Porcelijn L, Kapur R, Koeleman CA, Deelder A, de Haas M, et al. Regulated glycosylation patterns of igg during alloimmune responses against human platelet antigens. J Proteome Res (2009) 8(2):450–6. doi: 10.1021/pr800651j
151. Aster RH. Core fucosylation and igg function in nait. Blood (2014) 123(4):463–4. doi: 10.1182/blood-2013-12-539965
152. Bournazos S, Vo HTM, Duong V, Auerswald H, Ly S, Sakuntabhai A, et al. Antibody fucosylation predicts disease severity in secondary dengue infection. Science (2021) 372(6546):1102–5. doi: 10.1126/science.abc7303
153. Thulin NK, Brewer RC, Sherwood R, Bournazos S, Edwards KG, Ramadoss NS, et al. Maternal anti-dengue igg fucosylation predicts susceptibility to dengue disease in infants. Cell Rep (2020) 31(6):107642. doi: 10.1016/j.celrep.2020.107642
154. Larsen MD, Lopez-Perez M, Dickson EK, Ampomah P, Tuikue Ndam N, Nouta J, et al. Afucosylated plasmodium falciparum-specific igg is induced by infection but not by subunit vaccination. Nat Commun (2021) 12(1):5838. doi: 10.1038/s41467-021-26118-w
155. Van Coillie J, Pongracz T, Rahmöller J, Chen H-J, Geyer C, van Vlught LA, et al. The Bnt162b2 mrna sars-Cov-2 vaccine induces transient afucosylated Igg1 in naive but not antigen-experienced vaccinees. bioRxiv (2022). doi: 10.1101/2022.02.14.480353
156. Okuya K, Hattori T, Saito T, Takadate Y, Sasaki M, Furuyama W, et al. Multiple routes of antibody-dependent enhancement of sars-Cov-2 infection. Microbiol Spectr (2022) 10(2):e0155321. doi: 10.1128/spectrum.01553-21
157. Wang SF, Tseng SP, Yen CH, Yang JY, Tsao CH, Shen CW, et al. Antibody-dependent sars coronavirus infection is mediated by antibodies against spike proteins. Biochem Biophys Res Commun (2014) 451(2):208–14. doi: 10.1016/j.bbrc.2014.07.090
158. Guérin P, Yahi N, Azzaz F, Chahinian H, Sabatier J-M, Fantini J. Structural dynamics of the sars-Cov-2 spike protein: A 2-year retrospective analysis of sars-Cov-2 variants (from alpha to omicron) reveals an early divergence between conserved and variable epitopes. Molecules (2022) 27(12):3851. doi: 10.3390/molecules27123851
159. Garcia-Beltran WF, Lam EC, Astudillo MG, Yang D, Miller TE, Feldman J, et al. Covid-19-Neutralizing antibodies predict disease severity and survival. Cell (2021) 184(2):476–88.e11. doi: 10.1016/j.cell.2020.12.015
160. Yang ZY, Werner HC, Kong WP, Leung K, Traggiai E, Lanzavecchia A, et al. Evasion of antibody neutralization in emerging severe acute respiratory syndrome coronaviruses. Proc Natl Acad Sci USA (2005) 102(3):797–801. doi: 10.1073/pnas.0409065102
161. Mantegazza AR, Magalhaes JG, Amigorena S, Marks MS. Presentation of phagocytosed antigens by mhc class I and ii. Traffic (2013) 14(2):135–52. doi: 10.1111/tra.12026
162. Nechipurenko YD, Anashkina AA, Matveeva OV. Change of antigenic determinants of sars-Cov-2 virus s-protein as a possible cause of antibody-dependent enhancement of virus infection and cytokine storm. Biophysics (Oxf) (2020) 65(4):703–9. doi: 10.1134/S0006350920040119
163. Nechipurenko YD, Semyonov DA, Lavrinenko IA, Lagutkin DA, Generalov EA, Zaitceva AY, et al. The role of acidosis in the pathogenesis of severe forms of covid-19. Biol (Basel) (2021) 10(9):852. doi: 10.3390/biology10090852
164. Jimenez L, Campos Codo A, Sampaio VS, Oliveira AER, Ferreira LKK, Davanzo GG, et al. Acid ph increases sars-Cov-2 infection and the risk of death by covid-19. Front Med (Lausanne) (2021) 8:637885. doi: 10.3389/fmed.2021.637885
165. Shevel E. Conditions favoring increased covid-19 morbidity and mortality: Their common denominator and its early treatment. Mo Med (2021) 118(2):113–5. Available at: https://pubmed.ncbi.nlm.nih.gov/33840847/.
Keywords: SARS-CoV-2, ADE, immune cells infection, phagocyte infection, macrophage infection, monocyte infection, pyroptosis, COVID-19 pathogenesis
Citation: Matveeva O, Nechipurenko Y, Lagutkin D, Yegorov YE and Kzhyshkowska J (2022) SARS-CoV-2 infection of phagocytic immune cells and COVID-19 pathology: Antibody-dependent as well as independent cell entry. Front. Immunol. 13:1050478. doi: 10.3389/fimmu.2022.1050478
Received: 21 September 2022; Accepted: 08 November 2022;
Published: 01 December 2022.
Edited by:
Daxing Gao, University of Science and Technology of China, ChinaReviewed by:
Gregory Dekaban, Western University, CanadaCopyright © 2022 Matveeva, Nechipurenko, Lagutkin, Yegorov and Kzhyshkowska. This is an open-access article distributed under the terms of the Creative Commons Attribution License (CC BY). The use, distribution or reproduction in other forums is permitted, provided the original author(s) and the copyright owner(s) are credited and that the original publication in this journal is cited, in accordance with accepted academic practice. No use, distribution or reproduction is permitted which does not comply with these terms.
*Correspondence: Olga Matveeva, b2xnYS5tYXR2ZWV2YUBnbWFpbC5jb20=; Julia Kzhyshkowska, anVsaWEua3poeXNoa293c2thQG1lZG1hLnVuaS1oZWlkZWxiZXJnLmRl
Disclaimer: All claims expressed in this article are solely those of the authors and do not necessarily represent those of their affiliated organizations, or those of the publisher, the editors and the reviewers. Any product that may be evaluated in this article or claim that may be made by its manufacturer is not guaranteed or endorsed by the publisher.
Research integrity at Frontiers
Learn more about the work of our research integrity team to safeguard the quality of each article we publish.