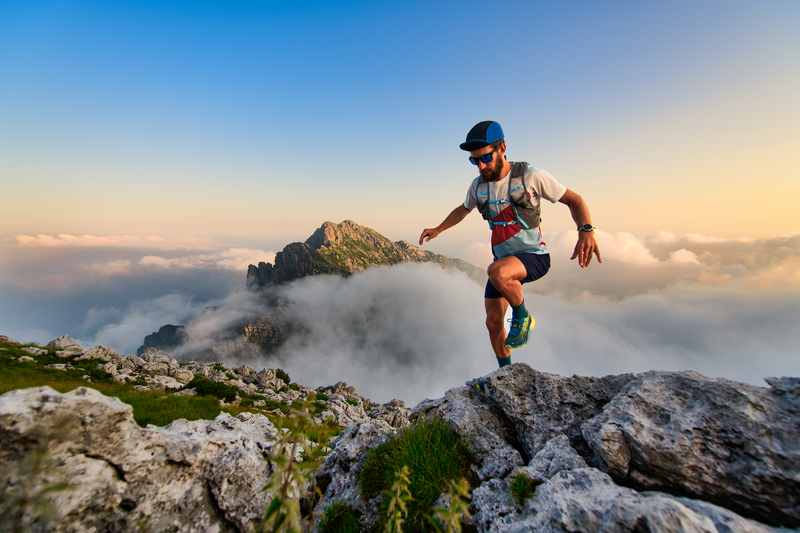
95% of researchers rate our articles as excellent or good
Learn more about the work of our research integrity team to safeguard the quality of each article we publish.
Find out more
REVIEW article
Front. Immunol. , 21 November 2022
Sec. Cancer Immunity and Immunotherapy
Volume 13 - 2022 | https://doi.org/10.3389/fimmu.2022.1049340
This article is part of the Research Topic Updates on Toll-Like Receptors in Cancer Immunity and Immunotherapy View all 7 articles
Toll-like receptors (TLRs) are pattern recognition receptors, originally discovered to stimulate innate immune reactions against microbial infection. TLRs also play essential roles in bridging the innate and adaptive immune system, playing multiple roles in inflammation, autoimmune diseases, and cancer. Thanks to the immune stimulatory potential of TLRs, TLR-targeted strategies in cancer treatment have proved to be able to regulate the tumor microenvironment towards tumoricidal phenotypes. Quantities of pre-clinical studies and clinical trials using TLR-targeted strategies in treating cancer have been initiated, with some drugs already becoming part of standard care. Here we review the structure, ligand, signaling pathways, and expression of TLRs; we then provide an overview of the pre-clinical studies and an updated clinical trial watch targeting each TLR in cancer treatment; and finally, we discuss the challenges and prospects of TLR-targeted therapy.
Toll-like receptors (TLRs) are type I transmembrane glycoproteins (1) with evolutionarily conserved structures, existing in a wide variety of species from plants to vertebrates (2). Since the discovery in 1996 of Toll receptor protein that contributes to Drosophila’s anti-fungal response and embryonic development (3, 4), 13 functionally active homologs of Toll receptor-TLRs, have been identified in humans and mice, of which TLR1-9 and TLR11 are conserved in both species (5–7). However, TLR11 is non-functional in human, presented only by a pseudogene (8).TLR12 and 13 do not exist in humans, while TLR10 in mice is non-functional due to a retrovirus insertion (6).
Pattern recognition receptors (PRRs) are key components of innate immunity because of their ability to sense infection, elicit intracellular signaling cascades and initiate immune responses that ultimately eliminate pathogens and infected cells (9). As a group of important PRRs (3), TLRs recognize diverse microbial pathogens (e.g., lipids, peptides, carbohydrates, and nucleic acids) by their conserved molecular patterns, indicated as pathogen-associated molecular patterns (PAMPs), and initiate immune responses (10, 11). Almost all cells of the immune system (e.g., macrophages, B lymphocytes, dendritic cells, mast cells, neutrophils, etc.) as well as epithelial cells, endothelial cells, adipocytes, and cardiomyocytes recognize pathogens via TLRs (9). Recognition of microbial products by TLRs activates the innate immune response and triggers the activation of downstream signaling pathways in which myeloid differentiation factor 88 (MyD88) and toll-IL-1 receptor structural domain (TRIF) lead to the activation of NF-κB and subsequent transcription of pro-inflammatory cytokines including tumor necrosis factor-α (TNF-α), IL-1 and IL-6 (12). TLRs also recognize conserved molecular structures of host-derived molecules, often referred to as damage-associated molecular patterns (DAMPs) (13), derived after cell death and extracellular matrix (ECM) degradation from tissue damage caused by trauma or infection. TLRs have also been found to play critical roles in many other activities, including adaptive immune responses (14), differentiation and development (15, 16), tissue regeneration (17–19), cell cycle regulation (20, 21), and metabolism (22, 23).
As a group of regulators of various cellular functions, it is unsurprising that TLRs also exert their versatility in the process of carcinogenesis and tumor development, where their functions are augmented or dysregulated, resulting in either anti-tumor or pro-tumor responses (24–26). The exploitation of TLR anti-tumor activities has shown great promise in cancer immunotherapies, with some synthesized TLR agonists already approved by FDA for clinical use (27); however, problems still exist relating to their use, including limited translation rate from bench to bedside, possible immunosuppression induced by TLR agonists, and safety issues (28, 29).
In this article, we review the basic features of TLRs. We report on the applications of TLR-targeted treatment in anti-cancer therapies in clinical trials, encompassing various combinatory therapeutic strategies. Lastly, we review the challenges facing TLR-targeted therapy at present.
TLRs are membrane-spanning proteins (3) with an extracellular N-terminal domain consisting of 19-25 tandem leucine-rich repeat motifs (2), displaying a horseshoe tertiary structure (30, 31), which is responsible for ligand recognition (2). Next to the ectodomain is a transmembrane region (3), connecting the intracellular portion: the Toll/IL-1 receptor (TIR) domain in the C-terminal tail, which is homologous to IL-1 receptor family’s cytoplasmic region (32) and required for downstream signaling (10).
All the TLRs are synthesized in the endoplasmic reticulum (ER), then transferred to Golgi apparatus, and finally migrate to the plasma membrane or intracellular endosomes (33).
Human TLRs can be classified into two groups by sub-cellular localization: 1) the cell surface TLRs, TLR1, 2, 4, 5, 6, and 10, which reside on the plasma membrane (4), traveling to phagosomes when activated (34), 2) the intracellular TLRs, TLR7, 8, 9, which are expressed on endosomes inside the cells, with the LRR domain facing the inner cavity of the compartment, sampling their ligands (31). TLR3 are also primarily expressed on endosomes, yet it has been reported to express on the cell surface of fibroblasts, epithelial cells, and macrophages as well (35, 36).
In the ligand-binding, namely, activated status, TLRs tend to be dimerized (31). TLR2 alternatively forms heterodimers with TLR1, 6, or 10 (37, 38); each combination is associated with the specificity for recognizing a particular group of ligands, making TLR2 the most competent TLR in sensing a diverse range of PAMPs. Other TLRs tend to form homodimers (Figure 1) (39).
Figure 1 TLR ligands and signaling pathways Physiologically, TLRs are expressed on the cell membrane (TLR 1, 2, 4, 5, 6, 10) or the endosome (TLR3, 7, 8, 9). TLRs recognize a wide range of pathogen-associated molecular patterns (PAMPs). Upon ligation, dimerized TLRs can activate either MyD88 or TRIF pathways. TLR4 can activate MyD88 (with adaptor protein TIRAP) and TRIF (with adaptor protein TRAM) pathways; TLR3 activates TRIF alone, and TLR1/2, TLR2/6, TLR2/10, TLR5 as well as TLR7-9 activate MyD88 alone, all without the need of an adaptor protein. As a result, type I IFNs are induced via IRF3 and IRF7 activation and inflammatory cytokines via NF-kB, MAPKs, and IRF5 stimulation, which thus initiates a wide range of inflammatory and immune activities, giving the basis for therapeutic exploration on TLRs in tumor therapies. (This figure is created with BioRender.com).
TLRs do not necessarily distinguish between self and non-self, but they respond to “danger signals” in general; as long as the “signals” are encompassed within certain molecular patterns, both no matter whether exogenous or endogenous ligands (40).
TLR ligands (TLRLs) primarily fall into three categories (1): natural exogenous ligands (PAMPs) (2); natural endogenous ligands (DAMPs and secreted ligands) (3); synthesized agents.
Exogenous ligands, namely, PAMPs originate from broadly expressed components in bacteria, fungi, protozoa, parasites, and viruses (10, 41, 42); they are common and genetically conserved because of their significance to microbial survival and infectivity (1, 43). As sentinels of the host’s defense system, TLRs sense PAMPs as invading dangers and directly activate the innate immune system (44), meanwhile indirectly activating the adaptive immune system (43), thereby providing indispensable protection against pathogens. Studies on mice lacking each TLR have shown that each TLR has a different function in PAMP recognition and immune response (45). Cell surface TLRs mainly recognize microbial membrane components such as lipids, lipoproteins, and proteins. TLRs expressed in intracellular components, such as endosomes, recognize viral or bacterial nucleic acids (46, 47). Specifically, TLR1/2 recognizes triacylated lipopeptides from Gram negative bacteria (38, 39), TLR2/6 recognizes diacylated lipopeptide from mycoplasma (38, 48), and TLR2/10 recognizes microbial components shared by TLR1 (37). TLR3 ligands are double-stranded RNAs (dsRNAs) emerging from cytosol virus replication (13, 39). TLR4 binds lipopolysaccharide (LPS) from Gram negative bacteria (39, 49) and fungal mannanes (48).TLR5 ligates with bacterial flagellin (13).TLR7 and 8 ligands are both single-stranded RNAs (ssRNAs) from viruses (13, 39).TLR9 recognizes nonmethylated CpG motifs normally found in bacterial and viral DNA (13, 39).
On the other hand, endogenously derived DAMPs from cells and ECM also activate TLRs. DAMPs are generated from cellular components or fragments of extracellular macromolecules, which are typicallyinaccessible to TLR ligand-binding regions in homeostasis, yet exposed to TLRs in the case of cell necrosis (passive release), apoptosis (pulsative release) or extracellular matrix degradation (5, 16, 43, 50) following trauma, inflammation and tissue remodeling during development (43). Specifically, TLR2 recognizes HMGB1, β-defensin-3, serum amyloid A, and biglycan (51);TLR4 recognizes HMGB1, fibrinogen, saturated fatty acids, and hyaluronic acid fragments (51);TLR3 recognizes mRNA (52);TLR7 and TLR8 recognize ssRNA (53) and antiphospholipid antibody (54);TLR9 recognizes IgG-chromatin complexes (55).
In the tumor microenvironment (TME), DAMPs can be abundantly released by solid tumors (5, 56) and have a significant impact in the TME by adopting mechanisms of tissue repair [such as cell proliferation (57, 58), angiogenesis (56), and tissue remodeling (59)], contributing to tumor progression. Alternatively, just as PAMPs, DAMPs can also act as stimulators of immune responses, showing anticancer potential in vitro as well (56).
Synthetic TLRLs on the other hand have varied levels of similarity to natural ligands (41), designed for the purpose of disease treatment by manipulating different aspects of TLR functions. The synthetic TLRLs are in active pre-clinical and clinical investigation. The advances of TLRLs, including synthetic TLRLs, in therapeutic research are reviewed in the following section 5 and Tables 1–3.
TLR signaling is initiated after TLR-ligand binding, which causes conformational changes, leading to the recruitment of four major adaptor proteins (Figure 1) (41). Each of these proteins contain a TIR domain that is homologous to the intracellular region of TLRs; all TLRs transmit signals through at least one of those proteins: myeloid differentiation factor 88 (MyD88), TIR domain-containing adaptor protein (TIRAP, also identified as MyD88 adaptor-like protein, MAL), TIR domain-containing adaptor protein-inducing interferon (IFN)-β (TRIF, also identified as TIR domain-containing adaptor molecule 1, TICAM1), and TRIF-related adaptor molecule (TRAM, also identified as TICAM2 and TIR-containing protein, TIRP) (1, 10, 41, 224, 225). A fifth TIR-domain exists, containing adaptor protein- sterile alpha and HEAT/armadillo motif protein (SARM), but contrary to the other four adaptors with functions of TLR signaling stimulation, it negatively regulates TLR signaling by inhibiting the downstream of TRIF pathway (225, 226).
TLR signaling can be divided into two pathways according to the TIR-containing adaptor used: the MyD88 pathway (MyD88-dependent pathway) and the TRIF pathway (MyD88-independent pathway) (227). TLR4 is the only TLR that activates both pathways; TLR3 relies only on the TRIF pathway; the remaining TLRs, TLR1-2, TLR5-10, activate solely the MyD88 pathway (10, 24, 37, 227). When initiating these pathways, some TLRs require an accompanying adaptor molecule. TLR4 and TLR2 (after dimerized with TLR1 or TLR6 but not TLR10) require TIRAP to recruit MyD88. TLR4 requires TRAM to bridge to TRIF (227–229). However, TLR5 and TLR7-9 can independently interact with MyD88 (227), and TLR3 depends solely on TRIF (228, 230).
In the MyD88 pathway, a series of downstream molecules are sequentially activated following recruitment of MyD88, including but not limited to interleukin-1 receptor-associated kinases (IRAKs), tumor necrosis factor (TNF) receptor-associated factor 6 (TRAF6), interferon (IFN) regulatory factor 5 (IRF5), receptor-interacting protein kinase 1 (RIP1), nuclear factor κB (NF-κB) inhibitor (IκB) kinase (IKK) complex, finally leading to the activation of mitogen-activated protein kinases (MAPKs) (ERK, JNK, p38) and NF-κB, which induces a wide range of pro-inflammatory cytokines (227, 228). In addition, in a cell-specific manner, following TLR7-9 ligation, the stimulated MyD88 pathway in plasmacytoid dendritic cells (pDCs) can activate IRF7, which subsequently leads to IFNα production (227).
In the TRIF pathway, IRF3 is activated and translocated into the nucleus, consequently resulting in IFNβ induction (227, 228). TLR3 and TLR4 can signal through the IRF3/IFNβ pathway (231, 232). In addition, TRIF can also stimulate RIP1 and TRAF6, leading to activation of MAPKs and NF-κB to induce pro-inflammatory cytokine production (227, 228).
Besides typeIIFN (IFNα, IFNβ) and inflammatory cytokines (TNFα, interleukin-1 (IL-1), IL-6, IL-10, IL-12, etc.), TLRs also induce many different chemokines (IL-8, macrophage inflammatory protein (MIP)-1, IP-10, etc.) and microRNAs (miRNAs) (10, 41, 233–235). In cells, TLR activation may lead to apoptotic, proliferative, or differentiative responses (41). In tissues, TLR signaling can result in inflammation, immune responses, and tissue repair (236).
In humans, TLRs have a broad expression in various tissues, with the most diversity in locations involved in immune function, such as the spleen and peripheral blood, and locations in constant contact with microbes, such as the lung and the gastroenterological tract (237). Other locations expressing TLRs include the female reproductive tract, urinary tract, skin, neural system, and vascular system (238–240).
Specific to cell types, TLRs can typically be found in immune cells, such as DCs, monocytes, macrophages, granulocytes, NK cells, mast cells, and lymphocytes. Other non-immune cells also express TLRs, including endothelial cells, epithelial cells, fibroblasts, glial cells, astrocytes, keratinocytes, vascular smooth muscle cells, and sperm cells (3, 10, 33, 238, 239, 241, 242).
Recent evidence has shown that TLRs are expressed in diverse tumor cell populations (including tumor stem cells) together with cancer-associated fibroblasts, tumor-associated macrophages, myeloid-derived suppressor cells (MDSCs), regulatory T cells (Tregs), and adipocytes in the TME, participating in both promotion and inhibition in tumor growth (4, 10, 243).
During the process of malignant transformation, the level of TLR expression tend to elevate in transformed cells (including tumor cells) (24, 238, 244); meanwhile, the expression of TLR2, 4, 5, which is normally on cell membrane, increases in cytosol in a diffused manner (25, 245).
The expression patterns of TLRs and the downstream molecules are varied and regulated by multiple factors (237). Understanding these factors is vital for better exploitation in pre-clinical/translational research in TLR-targeted anti-tumor therapies.
TLR expression varies among distinctive species. For example, in humans, TLR9 is primarily expressed in B cells, whereas in mice, it can be found in B cells, monocytes, macrophages, and plasmacytoid DCs (pDCs) (246). Thus, cross-species interpretation of TLR research data should be carried out with caution.
Cell differentiation and activation status can also influence TLR expression. The differentiated human monocytic cell line THP-1 expresses increased TLR1, 4, 6, 7, 8, and MyD88 compared to undifferentiated THP-1 cells (33). E. coli-stimulated monocytes and granulocytes present different expression profiles of TLR1-10 (237). Phorbol myristate acetate/ionomycin-activated cytotoxic T cells (CTLs) showed increased TLR2 expression compared to unstimulated CTLs (247).
Ligands are another major factor affecting TLR expression. A specific TLR agonist may influence the expression of the TLR that it is ligating to and other TLRs as well. For example, the TLR4 agonist LPS elevated TLR1-8 expression in THP-1 cells, not just TLR4 (237). In addition, different ligands may differentially regulate the expression of a particular TLR. While TLR3 expression is upregulated by LPS, it is down-regulated by synthetic bacterial lipopeptide - a TLR2 agonist (237). Hence, cell localization related to potential ligand accessibility can impact TLR expression (39). As the case with TLR2 expression in B cells, only a restricted portion of peripheral blood B cells express TLR2 (248), but different developmental stages of tonsillar B cells all express TLR2 (249).
Cytokines are also engaged in the regulation of TLR expression. Many cytokines have been reported to increase TLRs expression level, including IL-6 and IFN-γ (237).
TLR activation can initiate negative feedback on TLR expression (237), and there is also internal balance among different expression of different TLRs (250, 251).
The factors discussed above indicate a complex network regulating TLR expression. This results in varied spectrums of TLR expression in respective cell groups and varied TLR-mediated responses, contributing to the heterogeneity of neoplasia, while also providing the basis for understanding the evidence for both anti-tumor and pro-tumor TLR-mediated activities. TLRs expressing in cancer cells could lead to tumor cell proliferation and induce cytokines that suppress immune activities; TLRs expressing on immune cells, however, could elicit Th1-based immunity, immune memory, and suppress tumor metastasis (252). The TLR expression in different tumor tissues is associated with patients’ outcome. Urban-Wojciuk et al. (252) have reviewed the association. In their review, the same TLR expressing in different types of cancers, could show opposite clinical correlations. TLR5, 7, 8, and 9 correlate with better clinical outcomes (high immune cell marker expression and/or longer survival), while TLR4, 7, and 9 are associated with poorer outcomes (advanced tumor stage, poor differenciation, and shorter survival) (252).
TLR-targeted therapies exert anti-tumor effects mainly by exploiting the potential of TLRs in the enhancement of both innate and adaptive immunity, and the induction of apoptosis in TLR-expressing tumor cells (41).
In this section, we introduce advances in pre-clinical (mainly from the past decade) as well as clinical research in tumor therapies targeting TLR. The TLR-targeting strategies and treatment modalities applied in both pre-clinical and clinical tumor therapeutic studies are summarized in Figure 2.
Figure 2 TLR-targeting Strategies and Combined Treatment Modalities in Tumor Therapies Current strategies targeting TLRs in tumor therapies encompass the development of novel TLR agonists, inactivated virus, engineered bacteria, functional RNAs expressing the TLR and/or its ligand, vaccine conjugates, modified T cells with active motifs of the TLR, and TLR antagonism. There is a trend of incorporating nanotechnology into TLR drug manufacturing and delivery to improve treatment efficacy and safety. Increasing evidence demonstrates the insufficiency of single intervention in circumventing immunosuppression incurred by tumor progression, hence leading to both pre-clinical and clinical research focusing on multi-agent and multi-modality treatment. (This figure is created with BioRender.com).
Pre-clinically, classic TLR agonists are being further explored in novel treatment strategies, and newly synthesized or naturally extracted TLR-stimulating agents are reported for improved immune activation ability and lowered toxicity; novel strategies of TLR agonism, aside from TLR agonists, have also been investigated (Table 1).
There have been many clinical trials completed involving TLRs, and some with promising results (Table 2). The ongoing clinical trials are continuously pushing the boundary of TLR-targeted therapy from bench to bedside (Table 3). Recent advances in clinical trials focusing on therapeutic interventions of tumors were summarized from critically reviewing clinicaltrials.gov and ncbi.nlm.nih.gov/pubmed.
In tumors, TLR2 has been found to enhance T cell immunity (253, 254) and suppress the accumulation of immunosuppressive T regulatory cells (Tregs) (62) and MDSCs (255). TLR2 also induces the anti-tumor M1-like macrophage polarization (66). The immune stimulating potential of TLR2 has been utilized in both basic and clinical therapeutic research.
Recently, there has been pre-clinical data (Table 1) demonstrating tumor inhibition effects by different TLR2L, including triacylated lipopeptides, synthetic chemical compounds, glucomannan polysaccharide, naturally extracted compound, and inactivated virus. In those studies, TLR2Ls are either investigated alone or in combination with tumor vaccines, immune checkpoint inhibition (ICB), chemotherapy, photodynamic therapy(PDT), or adoptive cell transfusion (ACT). In general, enhanced immune activation and suppressed tumor cell viability were observed in these studies (Table 1).
The number of clinical trials with sole TLR2 agonism in tumor immunotherapy is limited. It is noteworthy that Amplivant, the modified Pam3CSK4, can conjugate synthetic long peptides (SLPs) and elicit stronger DC and T cell stimulation in preclinical models compared to Pam3CSK4 (63). In 2021 the first-in-human phase I clinical trial NCT02821494 showed HPV16 E6 SLP-conjugated Amplivant induced robust HPV16-specific T cell immunity with good safety profile (187).XS15 is a TLR1/2 agonist, applied as an adjuvant to a multi-peptide vaccine scheme is in currently active phase I trial NCT04688385 (256).
TLR2 agonism has been applied in chimeric antigen receptor T cell (CAR-T) technology. In trial NCT02822326, a TLR2 Toll/IL-1 domain is incorporated in CAR-T, and the results showed that the tumor-targeting CD19-CAR-T2 cells augmented anti-leukemia responses in relapsed or refractory B cell acute lymphoblastic leukemia (B-ALL) patients with extramedullary involvement, competent in the eradication of extramedullary leukemia cells (257). Another similar phase I trial NCT04049513 is currently investigating the efficacy of 1928T2z CAR-T cells in treating B cell lymphoma.
In general, the Amplivant-SLP compounds are promising for further research for their early clinical data in terms of safety and efficacy of immune stimulation. Also, they have a great potential in treating different tumors as SLPs can be highly variable.
Clinical trials with multi-TLR activation, including TLR2, are discussed in the below sections.
TLR3 actively regulates multiple aspects of tumor development. Either directly or indirectly, TLR3 triggers both innate and adaptive immune responses (258, 259);, it suppresses tumor cell proliferation and promotes tumor cell apoptosis (260–262); it regulates tumor angiogenesis (263); it has also been found to enhance chemosensitivity (70) and radiosensitivity (264). By initiating these mechanisms, TLR3 agonists have shown promising therapeutic value.
In recent years, pre-clinical investigations of TLR3-targeted therapy (Table 1) have focused on combination therapy of TLR3 agonism with other treatment modalities, including chemotherapy, tumor vaccines, and ICB. There have also been studies examining nanoparticle incorporated TLR3 targeted therapies. Poly (I:C) has been used in most studies as the TLR3-targeting agent, while ARNAX, a new DNA-capped dsRNA, has also been applied. These treatment strategies aim to improve immune stimulation and reduce treatment side effects, thus achieving better therapeutic results.
Three TLR3 agonists, Poly ICLC (Hiltonol), Rintatolimod (Ampligen), and BO-112 have progressed for assessment in clinical trials (Tables 2 and 3) as cancer treatments.
Poly ICLC is carboxymethylcellulose, polyinosinic-polycytidylic acid, and poly-L-lysine double-stranded RNA, administratedintratumorally (i.t.), intramuscularly (i.m.), or subcutaneously (s.c.) in cancer treatments of different malignant stages, including primary, recurrent, and metastatic. The various types of cancer involved range from hematopoietic malignancies, skin cancers, sarcoma, squamous cell carcinomas, breast cancer, neurological malignancies, gastroenterological tumors, female reproductive tract malignancies, and urinary tumors (Tables 2 and 3). In these cancer treatments, Poly ICLC is mostly used in combination with traditional therapies (surgery, chemotherapy, or radiotherapy), immunotherapies (tumor vaccine, immune co-stimulation, or ICB), or other synthesized anti-tumor agents. Most of the trials are active or undergoing recruitment. One completed phase II trial (NCT02423863) aiming at multiple solid tumors applied Poly ICLC intratumorally (i.t.) or intramuscularly (i.m.), showing good tolerability with local and systemic immune responses, achieving clinical benefit (188). Two trials investigating melanoma have also been completed with available results: in phase I/II trial NCT01079741, poly ICLC was combined with NY-ESO-1 protein vaccine and Montanide to treat melanoma, effectively activating humoral and T cell immunity in patients. In phase I trial NCT01585350, poly ICLC together with peptide vaccine and incomplete Freund’s adjuvant (IFA) markedly enhanced T cell responses (190). Phase I trial NCT01834248 also combined poly ICLC with NY-ESO-1 vaccine and Decitabine in treating myelodysplastic syndrome, which showed success in the induction of NY-ESO-1-specific cytotoxic CD4+ and CD8+ T cells as well as CD141Hi cDCs (265). The completed phase I trial NCT03380871 combined Hiltonol with a tumor vaccine, pembrolizumab, and chemotherapy to treat NSCLC, which showed a good safety profile and immune stimulation. Two completed phase I trials investigating either Hiltonol combined with tumor vaccines (NCT02721043) or Hiltonol with a tumor vaccine and Nivolumab (NCT02897765), all showing good treatment safety profile and immunogenicity. In particular, the latter treatment strategy induced intra-tumoral chemotaxis of cytotoxic T cells (191). The phase I trial NCT02544880 recently completed, where Hiltonol and Mucin1 were combined to make the MUC1 vaccine; together with influenza vaccine and PDE5 inhibitor Tadalafil, MUC1 vaccine has been used to treat solid tumors. Published interim results showed that the combination of MUC1 vaccine and Tadalafil was well tolerated and induced immune activation in HNSCC patients, despite the implication of immune evasion after the treatment was also observed (192). However, trial NCT01532960, where Hiltonol was combined with a tumor vaccine in treating breast cancer, was terminated due to the lack of immune stimulation by of Hiltonol for the vaccine (266). Phase II trial NCT02873819, phase I/II trial NCT02643303, and phase I trial NCT03358719 usingHiltonol in combinatory cancer therapies have recently closed, and are awaiting results. In general, Hitonol is well tolerated and can induce immune responses; however, there is a lack of robustdata presenting its competence in bringing clinical benefit.
Rintatolimod is a modified poly (I:C), PolyI: PolyC12U, administratedintravenously (i.v.) or intraperitoneally (i.p.) in gynecological cancers, breast cancer, and colorectal carcinoma. In clinical trials, Rintatolimod is applied used in combination with chemotherapy, a tumor vaccine, ICB, or other immune-modulatory therapies. Phase I/II trial NCT01312389 and phase II trial NCT03403634 using Rintatolimod have been completed, however the results are not yet publicly available.
BO-112 is a nanoplexed form of poly (I:C), which is currently under investigation in melanoma with pembrolizumab (NCT04570332).
In conclusion, pre-clinical data of TLR3 agonists provide promising perspectives for them to move from bench to bedside, yet more research and trials are needed in optimizing TLR3Ls’ clinical effectiveness.
In tumor studies, TLR4 has been reported to enhance the function of antigen-presenting cells (APCs) (267), increase the production of pro-inflammatory cytokines as well as IFNs (268–270), and boost cytotoxic responses of CTLs and NK cells (267, 271). These immune stimulating mechanisms can be initiated by TLR4 agonists in cancer treatment.
Recently, lipopolysaccharide, lipid A derivatives, polysaccharides, and protein TLR4 agonists were investigated in therapeutic studies (Table 1). These TLR4 agonists have been mostly used in combination with tumor vaccines, including DNA-, peptide- and DC-based vaccines; they have also been used in combinationwith radiotherapy and different kinds of immune-stimulating agents. Notably, many TLR4 agonists are engineered into nanoparticles, which have improved drug delivery efficiency and reduced off-target effects (88–91).
Several TLR4 agonists have completed clinical studies(Table 2), including LPS, GLA-SE(G100), IDC-G305, GSK1795091 (CRX-601), and the TriMix DC vaccine.
The completed phase I trial NCT01585350, investigated LPS in combination with a peptide vaccine and incomplete Freund’s adjuvant (IFA) to treat melanoma, which showed that this treatment was well tolerated and stimulated marked T cell responses (190).
GLA-SE is a glucopyranosyl lipid A-stable oil-in-water emulsion, tested in lymphoma, skin cancers, sarcoma, lung cancer, and colorectal cancer, either applied alone or in combination with surgery, chemotherapy, radiotherapy, ICB, or a tumor vaccine. In these trials, GLA-SE is administered i.t. or i.m. A phase I trial NCT02035657 applying GLA-SE in treatment against Merkel cell carcinoma has been completed. Treatment withintratumoral GLA-SE as an adjuvant to surgery and radiotherapy demonstrated safety and feasibility in Merkle cell carcinoma with increased intratumoral infiltration of CD8+ and CD4+ T cells, activation of immune-related genes, and local tumor regression (193).
IDC-G305 is a combination of NY-ESO-1 recombinant protein and GLA-SE, that has been tested in melanoma, ovarian cancer, renal cell cancer, and non-small cell lung cancer(NSCLC) (NCT02015416). This phase I trial showed that intramuscular administration of IDC-G305 was well-tolerated and can generate antigen-specific immunity (195). On the contrary, another two phase I trials NCT02387125 and NCT02609984, where NY-ESO-1 recombinant protein-GLA-SE was used with a DC-targeting tumor vaccine in treating solid tumors including sarcoma, melanoma, NSCLC, and ovarian cancer, were terminated because of lack of efficacy.
GSK1795091, a synthetic aminoalkyl glucosaminide 4-phosphate, in conjunction with anti-PD-1 pembrolizumab was investigated in advanced solid tumor treatment in trial NCT03447314; the results of this trial are yet to come.
TriMix DCs are autologous DCs incorporating mRNA encoding, CD40 ligand, CD70, and a constitutively active TLR4. The phase I/II trial NCT01530698 showed TriMix DCs, further electroporated with a tumor antigent encoding mRNA, administered intranodally, was safe but with only narrow immunological and clinical response.
In general, recent clinical trials usingTLR4 agonists did show clinical or immune stimulating efficacy in solid tumor treatment. However, there are contradictory results about the same agent in different trials. For those agents that showed promising results in early phase trials, future studies should pay attention to the optimal treatment conditions, including the therapy combination strategy and the route of administration.
TLR5 can be activated by bacterial flagellin and plays a key role in the immune homeostasis of pathogen-host interactions (272, 273). In addition, TLR5 is associated with decreased tumor cell proliferation, invasion, and metastasis (274, 275). Several drugs targeting TLR5 are under investigation.
Recently, protein-based TLR5 agonists have been investigated pre-clinically: in combination with a tumor vaccine (96), incorporated into the adoptive cell therapy (97), or used alone (98). All yielded enhanced therapeutic effects (Table 1).
Entolimod and Mobilan are administrated in two phase I trials (Table 2). Entolimod, namely, CBLB502 is a derivative of Salmonella flagellin. In trial NCT01527136, i.m. or s.c. administration of Entolimod was usedto treat locally advanced or metastatic solid tumors that cannot be removed by surgery. Results of this trial are yet to be available. While having the advantage of avoiding the dangerous inflammatory “cytokine storm” (276), Entolimod has a drawback that it can induce a rapid antibody neutralization (277),leading to its efficacy limitation. Pharmacological modification could be one solution to this problem. Mett et al. (277) identified and eliminated the epitopes leading to Entolimod’s neutralizing immunogenicity and refined its structure to get GP532 as a new TLR5 agonist. GP532 reduced neutralizing antibody response while preserving pro-inflammatory capacity. However, the anti-tumor potential of GP532 has not been verified in any therapeutic studies yet.
To extend the application of TLR5 agonism in TLR5 negative tumors, an adenovirus carrying TLR5 receptor and its agonist’s gene called Mobilan (M-VM3) was designed (276). In preclinical prostate cancer models, Mobilan displayed promising therapeutic efficacy (276).In phase I clinical trial NCT02654938, Mobilan was used intratumorally to treat prostate cancer; no results are available yet. A similar study showed Mobilan was well-tolerated in prostate cancer patients (278); however, more data is needed to verify its clinical benefit.
TLR 7 and 8 serve as important sentinels in response to infection, which makes them irreplaceable in the activation of mammalian innate immune cells (279). These two receptors have also been found to participate in the regulation of the host adaptive immunity (280–283). The emergence of novel small molecular agents targeting these two receptors in the last decade has greatly facilitated cancer immunotherapy studies by adopting their immune activation potential.
Since many agonists exert dual activation to both TLR7 and TLR8, we will summarize TLR7 and TLR8 agonists together in one section.
Following pre-clinical investigations TLR7 or TLR7/8 agonists can be classified by molecular nature into imidazoquinoline and its derivatives, novel synthetic small molecules, and RNA-based agents (Table 1). These agonists are studied either with traditional therapies, such as chemotherapy, radiotherapy, surgery, or novel therapies, such as tumor vaccines, immune checkpoint blockade, adoptive cell therapy, and photothermal therapy (Table 1). It is noteworthy that nanotechnology has become a hot research topic in the drug delivery of therapies applying TLR7/8 agonists. TLR7/8 agonists are incorporated into multi-functional nanoparticles that overcome drug solubility limitations (120, 122) or improve tumor-specific environment-targeting ability (115, 124, 125), thus improving drug delivery efficiency.
A number of TLR7 agonists that have undergone clinical investigation include imiquimod, TMX-101, RNA-LPX, BNT411, DSP-0509, LHC165, SHR2150, TQ-A3334, and RO7119929 (Tables 2 and 3).
Imiquimod has already been clinically applied in the treatment for genital warts (284), low-risk superficial basal cell carcinoma (285), and Bowen’s disease (cutaneous squamous cell carcinoma in situ) (286).In clinical trials initiated in the decade, Imiquimod has been utilized in a wide range of clinical trials targeting different tumor types. There are studies attempting to widen the utilization of Imiquimod based on the current standard of care.
Many clinical studies of Imiquimod focus on Skin cancer. In basal cell carcinoma, a phase III trial (NCT05212246) is going to use Imiquimod as a single treatment in high risk populations to prevent tumor occurrence; imiquimod is also applied in two active trials in combination with curettage (NCT02242929, phase III), and sonidegib together with surgery (NCT03534947, phase II) to treat basal cell carcinoma. In an active phase I trial (NCT03370406), Imiquimod together with 5-fluorouracil is applied to treat squamous cell carcinoma. Two active phase I trial are investigating melanoma, where Imiquimod is combined with either Pembrolizumab(NCT03276832) or tumor vaccine(NCT04072900). A completed phase III trial NCT02385188 found imiquimod together with paracetamol and lidocaine induced 82.6% response rate in vulvar Paget disease but 80% of patients needed painkiller to mitigate side effects (197).
Imiquimod is also studied in intraepithelial neoplasia. In grade 2/3 cervical intraepithelial neoplasia (CIN), a phase II/III trial (NCT02130323) showed that Imiquimod induced lower HPV clearance rate compared to large loop excision (43% vs 64%), which made conization remain the standard care when intervention is needed (199).However, trial NCT03233412 showed that when adjuvant to the loop excision procedure, weekly application of imiquimod can promote regression of high-grade squamous intraepithelial lesions (200). Two active trials are investigating Imiquimod combining HPV vaccine (phase II, NCT02864147) or topical Fluorouracil (phase I, NCT03196180) in treating CIN2/3. In vaginal intraepithelial neoplasia (VIN), the results of the phase III trial NCT01861535demonstrated that: Imiquimod is safe and effective in treating VIN and should be considered as a first-line treatment alternative to surgery (198).In the active phase III trial NCT02059499, single use of Imiquimod is applied in HIV patients who have anal intraepithelial neoplasia.
In glioma, results of phase I trial NCT02454634 recently published in Nature (202) showed that topical Imiquimod combined with IDH1 peptide vaccine induced 93.3% immune responses in IDH1R132H-mutated patients while adverse effects are within grade I. The immune responses induced by the treatment were represented by enhanced IL-17 production and increased IDH1-specific T cells (202). Trial NCT00899574 focused on breast carcinoma; the results showed that single topical use of imiquimod proved beneficial for breast cancer metastasis to skin/chest wall and has been well-tolerated; additionally, this trial proved that imiquimod could induced a pro-immunogenic TME (201). There are also completed phase I/II trials using Imiquimod with radiotherapy, chemotherapy or tumor vaccine to treat breast cancer (NCT01421017, NCT02276300).
In prostate cancer, Imiquimod is applied topically with different tumor vaccines (NCT02293707, NCT02234921). The phase II trial NCT02293707 showed the combination of Imiquimod, telomerase peptide-based vaccine, and Montanide ISA-51 VG was safe in prostate cancer patients. The number of treatments was positively associated with immunological response (204). Bladder cancer is another type of malignancy where Imiquimod displayed its therapeutic potential. TMX-101 is the intravesical formulation for Imiquimod; single use of TMX-101 induced significant increase in urinary cytokines, including IL-6, IL-18, IL-1β, IL-1 receptor antagonist (IL-1ra), and vascular endothelial growth factor (VEGF), in non-muscle invasive bladder cancer, despite accompanied with mild adverse effects (phase II, NCT01731652) (205).
In general, Imiquimod showedits potential in cancer treatment, and also an increasing number of active trials using it has been initiated.
A trial with RNA-LPX (NCT02410733) showed early results with effective quick induction of IFNα and IP-10, and the induction of de novo antigen-specific T-cell responses. In this study, i.v. administration of RNA-LPX proved to be well-tolerated (116). SHR2150 and RO7119929 are oral TLR7 agonists, currently in trials in combination with other therapies in metastatic solid tumors (SHR2150, NCT04588324), and liver/biliary tract cancer (RO7119929, NCT04338685). BNT411 and DSP-0509 are synthetic small molecular TLR7 agonists administered i.v. that are being investigated in multiple types of tumors. LHC165 is a benzonapthyridine TLR7 agonist, which has been used in combination with anti-PD-1 (PDR001) to treat solid tumors.
TLR8 agonist VTX-2337, also known as Motolimod, has been tested in ovarian cancer and squamous cell cancer patients combined with chemotherapy, ICB, or small molecular inhibitors; in most studies, Motolimod was applied subcutaneously. Four studies have since completed with published results. In the first trial, a phase I trial (NCT01294293) with different histological subtypes of ovarian cancer, Motolimod was combined with doxorubicin, showing 15% patients with a complete response and 53% with stable disease (210). A similar phase II trial (NCT01666444), however, showed that the supplement of Motolimod to doxorubicin did not improve clinical outcomes in patients with recurrent or persistent epithelial ovarian, fallopian tube or primary peritoneal cancer, compared with placebo (207). Motolimod was demonstrated to augment clinical responses in patients with HNSCC of advanced stages, who have also been treated with the epidermal growth factor receptor inhibitor cetuximab (phase I trial NCT01334177) (209). However, in a trial of recurrent or metastatic SCCHN patients, the addition of Motolimod to platinum-based chemotherapy and cetuximab did not improve progression free survival (PFS) or OS; nevertheless, remarkable benefit was presented in HPV-positive patients and those with injection site reaction, suggesting the application potential of Motolimod in subset- and biomarker-selected patients (phase II trial NCT01836029) (287). Recently, a strong TLR8 agonist, combined with a HER2 monoclonal antibody was designed (sbt-6050), aiming to activate myeloid cells with the presence of HER2 positive tumor cells, and it is under investigation now in HER2 positive solid tumors with pembrolizumab (NCT04460456).
In terms of dual TLR7/8 agonists, Resiquimod, TransCon, 852A, BDB001, BDB018, NKTR-262, and BDC-1001 have undergone clinical investigation. Resiquimod has been examined by topical use in melanoma and brain tumors in combination treatment with a tumor vaccine and/or other adjuvants, however the addition of Resiquimod to NY-ESO-1 protein vaccine and IFA failed to elicit steady antigen-specific CD8+ T cell response (NCT00821652) (206). TransCon is a type of Resiquimod prodrug, now under investigation in combination with pembrolizumab in solid tumors (NCT04799054). 852A is also an imidazoquinoline TLR7/8 agonist, which has previously been tested in multiple tumors types (including renal cell carcinoma, melanoma, lung cancer, hematologic malignancies, breast cancer, ovarian cancer, and cervical cancer), showing acceptable tolerability, evident immune stimulation, and clinical benefit in some patients (288–291). The i.v. administered TLR7/8 agonist BDB001 and its refined analog BDB018 (designed to enhance immune stimulation while preserving the safety profile of BDB001) are both investigated in solid tumor treatment (NCT03486301, NCT04196530, NCT04840394). BDC-1001 is an immune stimulating antibody conjugate of an anti-HER2 monoclonal antibody with a TLF7/8 dual agonist, currently recruiting a trial enrolling patients with HER2 positive solid tumors (NCT04278144).
In summary, TLR7/8 agonists have drawn most research attention among other TLR ligands; despite that preclinical data indicate promising prospects, none of the TLR7/8 agonists has gained regulatory approval to treat cancer in human except Imiquimod. Future study could focus on optimizing delivery routes, administration schedule, and the vaccine itself (where vaccines are applied) (292).
TLR9 is preferentially expressed on the endosomal membrane of B cells and plasmacytoid dendritic cells (pDCs), and its primary ligand is unmethylated cytidine phosphoguanosine (CpG) oligonucleotides (ODNs) (293). TLR9 activation can lead to potent activation of innate (133, 294, 295) and adaptive immunity (both cell and humoral immunity) (133).
TLR9 agonists previously evaluated in pre-clinical studies are all DNA-based agents containing non-methylated CpG motifs (Table 1). Most of these agonists are CpG ODNs, while some have more complex DNA structures designed for better protection from drug degradation and enhanced TLR9 stimulation capacity (164–166). The therapeutic enhancing effect of TLR9 agonists has been studied with chemotherapy, photodynamic therapy, radiofrequency ablation, vaccines, ICB, and other immune stimulatory agents. (Table 1) Interestingly, in two studies (139, 140) the CpG motif has been used as myeloid cell targeting sequence, incorporated into decoy ODNs that contain STAT3-specific sequence, which can inhibit STAT3 transcription. Both studies showed the decoy ODNs effectively suppressed tumors in animal models of hematological malignancies. Similar to TLR7/8 agonists, many studies have also looked into the design of nanoparticles for TLR9 agonist drug delivery. These versatile nanoparticles have improved the ability for TME-targeting (159), specific cell-targeting (148, 150, 163), and even sub-cellular compartment-targeting (147); they have also protected against drug degradation (152, 156); plus, some conjugated to drug tracers to monitor drug tracking after administration in vivo (152, 155).
Currently the most clinically investigated TLR9 agonists (Tables 2, 3) are SD-101, CMP-001, IMO-2125. SD-101 is a class C CpG oligonucleotide, applied intratumorally in trials for advanced (metastatic, refractory, or recurrent) malignancies such as pancreatic adenocarcinoma, prostate cancer, liver metastatic uveal melanoma, and multiple lymphoma subtypes. In these early phase trials, SD-101 will be administered in combination with ICB, radiotherapy, small molecular inhibitors, or an anti-OX40 antibody.
Two phase I/II trials applying SD-101 in combination with radiotherapy and immunotherapy in treating lymphoma and other solid tumors just completed, and the results are not yet available. CMP-001, also named as Vidutolimod, is a short DNA piece packaged in protein, mimicking the virus structure. It has been administratedsubcutaneously or intratumorally with ICB, an OX40 agonist, an IgG2 agonist, surgery, and radiotherapy in multiple types of primary and metastatic solid tumors. The completed phase I trial NCT03084640 is completed, which combinedPembrolizumab with CMP-001, and compared the safety together with response rates of CMP-001 administered in different routes in intreating melanoma. Another completed phase I trial NCT03438318 combined CMP-001 with Atezolizumab and radiotherapy to treat NSCLC. In both of the above trials, CMP-001 elicited antitumor-related transcriptional signatures (2022 AACR Abstract #: LB107); more results from trial on CMP-001 are yet to be published. IMO-2125, also known as Tilsotolimod, a synthetic phosphorothioate oligodeoxyribonucleotides, has shown a good safety profile with effective activation of dendritic cells, induction of type I IFN signaling, and up-regulation of multiple immune checkpoint pathways in a phase I trial (NCT03052205) (213), where IMO-2125 was usedas a monotherapy administrated intratumorally in refractory solid tumors. However, a phase III trial (NCT03445533), where IMO-2125 and Ipilimumab were combined for treating melanoma, was terminated for lack of efficacy. Other phase I to II trials with intratumoral administration of IMO-2125 are actively recruiting, either alone or with the combination of ICB in solid tumors (Table 3).
MGN1703 (Lefitolimod) is a DNA-based molecular alternative to a CpG-ODN, administratedsubcutaneously in trials and currently under investigation in combination with anti-CTLA4 in advanced cancers (NCT02668770). In trial NCT02200081, MGN1703 was used with platinum-based chemotherapy in advanced stage small-cell lung cancer where it was well tolerated but showed no impact on the main efficacy of treatment and overall survival of patients (214). DUK-CPG-001 a CpG-based TLR9 agonist is being evaluated in the treatment of myeloid and lymphoid malignancies combined with donor lymphocyte infusion enriched with NK cells in a phase II trial (NCT02452697).
Other TLR9 agonists, all CpG-based, have been assessed in clinical trials, with results available. Inhaled DV281 was tested with anti-PD-1 in advanced NSCLC, with no results publicly available yet (NCT03326752). CpG-MCL vaccine, combining CpG7909 and autologous mantle cell lymphoma-derived tumor cells, was studied to treat mantle cell lymphoma in combination with immunochemotherapy and an autologous hematopoietic stem cell transplant (NCT00490529). The trial showed moderate response to the vaccine among patients (40% developed anti-tumor CD8 T cell response, associated with favorable clinical outcomes), good treatment efficacy (89% were minimal residual disease negative), and a good safety profile (211). CpG7910 was applied in combinationwith local radiotherapy to treat recurrent low-grade lymphoma in a phase I/II trial (NCT00185965), where local administration of this agent systemically induced tumor-reactive memory CD8+ T cells. This regimen showed clinical feasibility (212). EMD 1201081 combined with EGFR inhibitor, however, showed no improvement to the clinical efficacy of HNSCC treatment (215).
In summary, despite that there are trials showing the immunogenicity and good tolerability of CpG-based agents, low translational rate remains an issue. This may due to the difference in TLR9 expression in animal models and humans (296). Pre-clinical models of human origins are necessary, such as primary tumor organoids. Also, one safety concern is that CpG motifs are potential to induce autoreactivity (297). There need to be trials with larger sample sizes, longer follow-up durations, and stratified patient subgroups to verify the safety and the suitable patient groups that can benefit from CpG ODN adjuvanted therapies.
In addition to single TLR activation, multi-TLR agonism is also under both pre-clinical and clinical investigation for many cancers. Multi-TLR agonism can be realized by a single agent or by a combination of several TLR agonists.
Preclinically, the most studied treatment modality with multi-TLR activation is with tumor vaccines (Table 1). There is also a trend of applying nanotechnology in drug delivery in multi-TLR stimulating treatments for improved APC-targeting (173, 179) and drug tracing (170).
In clinical trials, Bacillus Calmette-Guérin (BCG), an attenuated live Mycobacterium bovis, is the most studied multi-TLR agonist. It can activate TLR2, TLR4, and TLR9 (298); it was originally applied as a vaccine against tuberculosis and later broadened its application as an adjuvant in the standard care of a part of bladder cancer patients (298). Currently, BCG is still investigated in bladder carcinoma patients in combination with other drug treatments, including an anti-PD-1/PD-L1 antibody, small molecule inhibitors, and a neoantigen encoding gene vaccine. A completed trial NCT02753309 combined rapamycin with BCG in bladder cancer treatment, which showed good tolerability in high-grade non-muscle invasive bladder cancer patients and induction of antigen-specific γδ T cell response as well as urinary cytokine production (219). BCG has also been assessed for the treatment of upper or lower urinary tract carcinoma. In treating urinary tract urothelial carcinoma, the trial NCT00794950 investigated combination therapy of BCG and sunitinib, a novel anti-angiogenesis drug. This trial has since been completed, and results showed the combination treatment was associated with a lower rate of progression and recurrence with an acceptable safety profile (218). Besides malignancies in the urinary system, BCG combinedwith chemotherapy, radiofrequency ablation, and GM-CSF have been evaluated in the treatment of liver metastatic colorectal cancer (NCT04062721).
OM-174 is a lipid A analog, which activates both TLR2 and TLR4. In a phase I trial (NCT01800812) with solid tumor patients, OM-174 as a monotherapy was well tolerated and proved to be effective for the induction of IL-8, IL-10, TNF-α, and IL-6 (despite that TNF-αand IL-6 decreased progressively in repeated treatment). Progressively increased NK cells and NK cell activity were observed in patients who received the highest dose, 1000 μg/m2 (217). AS-15 is an adjuvant system, comprised of a TLR4 agonism system (MPL and QS-21) and a TLR9 agonist (CpG 7909) in a liposomal formulation. In the completed phase II trial NCT01266603, AS-15 was combined with the MAGE-A3 tumor antigen and high dose IL-2 to treat metastatic melanoma. This treatment achieved 25% response rate, which was associated with increased infiltrating T cells in the primary tumor tissue (222).
TLR3 agonist poly-ICIC and TLR7/8 agonist Resiquimod have been combined with tumor vaccines in the treatment of melanoma (NCT02126579) and advanced tumors refractory to conventional treatment (NCT00948961). In the completed trial NCT00948961, poly-ICIC and/or Resiquimod combined with NY-ESO-1-targeting DC cell vaccine. The combination therapy induced NY-ESO-1–specific IgG titers in 79% patients and NY-ESO-1–specific T cell responses in 56% patients, leading to disease stabilization and tumor regression in 15/70 patients (220).
A phase I trial NCT00068510, where poly-ICLC or imiquimod used in conjunction with an autologous tumor-pulsed DC vaccine, has reported that this regimen was safe and effective in prolonging survival for glioblastoma patients (221).
Considering the versatility of TLRs in cancer, some of which can promote tumor growth, there is also rationale for exploring TLR antagonists as a cancer therapy.
In several types of cancers arising from the digestive system, pre-clinical studies have demonstrated the therapeutic potential of TLR antagonists. OPN-301, an inhibitory anti-TLR2 antibody, suppressed tumor initiation and growth in a gastric animal model, which was associated with gene suppression of CXCL2 and TNF-α (183). Another two studies reported that IRS-954, an inhibitory DNA sequence against TLR7 and 9, and chloroquine, which inhibits TLR7 and 9 activation, significantly impeded tumor development and growth in vivo and in vitro in liver cancer (184) and cholangiocarcinoma models (185).
A subset of diffuse large B cell lymphoma patients carry the MyD88 L265P mutation, associated with overactivation of the TLR7/9/MyD88 pathway (186). A TLR7/8/9 antagonist IMO-8400 was previously usedin this subset of lymphoma patients in a completed clinical trial NCT02252146, which, showed limited therapeutic efficacy. Similarly, trial NCT02092909, studying IMO-8400 in treating Waldenstrom’s macroglobulinemia, also showed insufficient efficacy of IMO-8400, and hence it was terminated.
Recently, another synthesized ODN-based TLR7/9 antagonist HJ901 was shown to significantly inhibit tumor cell proliferation and tumor growth in cell lines or animal models carrying the MyD88 L265P mutation (186). However, these data warrant further investigation before it can be translated to the establishment of an early phase trial.
There are obviously fewer published studies on anti-cancer TLR antagonists, which generally showed less efficacy than TLR agonists.
As TLRs regulate a wide range of functions, initiating signaling pathways that may lead to both anti-tumor and pro-tumor effects, there is a concern regarding whether the application of TLR agonists will promote tumor growth or whether the anti-tumor effect will be counteracted by the pro-tumor effect they simultaneously initiated. For example, Theodoraki and colleagues have found that TLR3 agonists stimulate the TLR3-TRAF3/IRF3 pathway, which leads to the production of CTL attractants and activate MAVS/helicase pathway, which elicits Treg chemotaxis (299).
Two directions may be feasible for further investigation into this challenge. On one hand, choosing the agonist that specifically activates the anti-tumor pathways but leaves no effect on the pro-tumor pathway could help (Figure 3). Theodoraki et al. (299) further discovered the difference of pathway stimulation potential in different TLR3 agonists: dsRNA Sendai Virus, Poly (I:C), and Rintatolimod. Rintatolimod can activate the TLR3 pathway only, resulting in the induction of CTL attracting cytokines IFNa, ISG-60, and CXCL10, and avoid the initiation of MAVS/helicase pathway, which then prevents Treg accumulation. In the future, more focus to elucidate how drug conformation of TLR-targeting agents influences downstream signaling is necessary, which can then guide future drug synthesis or discovery.
Figure 3 Challenges and Future Perspective of TLR-targeting tumor therapies Due to the versatile nature of TLRs, simultaneous activation of both anti-tumor and pro-tumour effects can arise; future work may involve combination therapy and further exploration of the association between ligand conformation and specific pathway activation as well as the development of pathway-specific drugs. To resolve the inefficacy and toxicity of TLR-targeting drugs, identifying an appropriate patient population is of great significance; combination therapy again can be considered; systemic drug administration can shift to localized delivery; the incorporation of polymer and nanotechnology is an emerging field. TLR tolerance could be a major obstacle preventing the TLR-targeting therapies from achieving their full potential; thus, more effort should be put into studying cyclical changes in TLR-TLRL interaction and investigating the optimal treatment dose and schedule. (This figure is created with BioRender.com).
On the other hand, combination therapy is of utmost significance in TLR-targeted therapy. Combined therapies can enhance therapeutic effects in many cases and potentially diminish the pro-tumor impact elicited by TLR agonists. Feng et al. (300) discovered that poly (I:C) could inhibit HCC cell proliferation and lead to cell apoptosis while promoting cell migration and invasion at the same time. When combined with the toad venom constituent bufalin, cell migration and invasion were inhibited, whereas cell apoptosis and suppressed proliferation remained unaffected (300), indicating the potential of combination therapy of the two in eliminating poly (I:C)-initiated pro-tumor effect.
Despite numerous promising results emerging from pre-clinical research with TLR-targeted therapies, many failed to advance to clinical implementation. Lack of efficacy and toxicity has been an issue for TLR-targeting therapy (28, 301).
To address this, first, precise identification and investigation of patient sub-populations that respond to TLR-targeting strategies is important (Figure 3). Some treatments may not have shown significant efficacy in the overall study population, but do in a subset of patients. Monk et al. (207) and Ferris et al. (208) both found in their clinical trial studies that adding Motolimod to chemotherapy did not improve survival in the overall patient population. In contrast, the sub-population with injection site reactions did significantly benefit from Motolimod. The evidence above suggests 1) lack of significance in the overall analysis does not reject the potential of a certain TLR-targeting drug; 2) there are certain groups of patients that may be more responsive to TLR-targeting agents than others. Future work calls for more translational studies incorporated into the trial designs to examine any associations between treatment responsiveness and patient characteristics, including gene expression patterns, biomarker levels, local treatment reaction, etc., thus providing clues for the screening of the target patient population.
Secondly, both lab and clinical research data support that the optimal treatment potency of immunotherapy requires combinatory approaches (27, 28), which continues to be the trend for TLR-targeted therapy. As discussed in section 5 (Tables 1–3), many studies showed that the combination of TLR agonists with other therapies enhanced tumor retardation compared to a single treatment alone. However, there is still a gap in our knowledge regarding the timing and dosing to be applied to each treatment to achieve the optimal therapeutic result. For example, in the combinatory treatment of poly (I:C) and radiation in Lewis lung carcinoma models, Yoshida et al. (264) found using poly (I:C) one day ahead of radiotherapy yielded better tumor suppression than to use after radiation.
Pharmaceutical modification of targeting agents has great potential to improve efficacy and lower toxicity as well. Furthermore, polymer and nanoparticle formulations incorporating TLR agonists are promising avenues to explore as they may potentially increase the specificity of targeting and prevent the drug from early degradation, thereby reducing systemic toxicity. Those formulations can now be designed to load multiple agents and be multi-functional (Figure 3), including but not limited to pH-specific drug-releasing (115, 124), in vivo drug tracing (152, 155), and tumor hypoxia relief (106). The advancement of nanotechnology utilizedin TLR-targeted therapy has been reviewed comprehensively elsewhere (302). However, careful attention should be paid to these new biomaterials as the bioactivities they may incur in vivo remain to be fully elucidated. Meanwhile, reducing the financial and timing costs is also a formidable challenge to face.
Besides refinement of the drug delivery system, choosing a localized delivery route may also have an influence on efficacy. This can avoid systemic inflammation to a great extent and improve intratumoral efficacy (300), especially for solid tumors.
TLR tolerance arises due to the unresponsiveness or hyporesponsiveness of TLRs upon TLR agonist stimulation after repeated, prolonged, or chronic activation, which also includes TLR cross-tolerance, where a pre-used TLR agonist incurs tolerance of another TLR (303, 304). There have been studies showing TLR tolerance in TLR2, 3, 4, 5, 7/8, and 9 (304–309). This mechanism physiologically prevents uncontrolled inflammation and autoimmunity, thus protecting healthy tissues from inflammatory damage (303, 304). In cancer, however, such tolerance leads to impaired tumoricidal effect.
To overcome this phenomenon, Bourquin et al. (310) found cycles of repeated low dose TLR7/8 agonist injection within 24h with intervals every five days, compared to one high dose injection every three days, significantly suppressed tumor growth in a mouse model (310), indicating the potential of circumventing TLR tolerance by adjusting treatment dose and time. Similarly, Tsitoura et al. (311) found the minimum and optimal dosing interval to maintain a TLR agonists’ pharmacological responsiveness. Future research needs to further explore the cyclical changes in specific TLR-TLRL induced responsiveness and consider cross-tolerance as new cancer therapies incorporating multi-TLR agonism continue to emerge (Figure 3).
The pattern recognition receptor family of TLRs, widely expressed on both healthy cells and tumor cells, play versatile roles in both physical and pathological conditions. Each TLR influences multiple aspects of tumor development, with both anti- and pro-tumor potentials.
TLR-targeted cancer therapies have been widely studied, with some TLR agonists (Imiquimod and BCG) approved for clinical use to treat cancers (312). Pre-clinical studies are currently focusing on developing novel TLR-targeting agents, incorporating nanotechnology into TLR drug manufacturing and delivery, and novel approaches activating TLRs apart from using agonists (e.g. inactivated virus (67), engineered bacteria (175), and modified T cells (97)).(Figure 2) Increasing evidence demonstrates the insufficiency of single intervention in circumventing immunosuppression incurred by tumor progression, hence leading to both pre-clinical and clinical research focusing on multi-agent and multi-modality treatment at present (312). (Figure 2) In addition, TLR antagonists also showed therapeutic potential in pre-clinical models with tumors in the digestive system, but the potency of this group of agents in human remains to be fully elucidated.
Future research into TLR-targeting therapies must tackle several challenges, including the control of simultaneous activation of both anti- and pro-tumor effect elicited by TLR activation, TLR tolerance, and insufficient efficacy and toxicity. Additionally, due to the heterogenous nature of different types of tumors and the consequent influence on TLR activities in the TME, better research models, which preserve the important genetic mutations that affect tumor growth and treatment response, should be developed (313). Instead of using cell lines, bulk tumors derived from the real patient could be an ideal source for research models (252), from where the primary TME cell components– tumor cells, stromal cells, and immune cells–can be extracted.
Furthermore, with the trend of precision medicine and the deepening of research, there is a need for patient stratification according to their disease profile and treatment responsiveness to treat each patient with the most suitable strategy, providing them with the optimal benefit both therapeutically and economically. We speculate that addressing the challenges described above will elevateTLR-targeted therapies to their full potential and augment the efficacy of immunotherapies for patients.
YY and HL drafted the manuscript and organized the tables. YY designed the figures. XZ raised the article topic and designed the original manuscript structure. CF and PC modified the manuscript and proofread the language. All authors contributed to the article and approved the submitted version.
This work was supported by the National Natural Science Foundation of China (No. 81821002), National Natural Science Foundation of China (No.81902662), and Sichuan Science and Technology Program 2021YJ0011.
The authors declare that the research was conducted in the absence of any commercial or financial relationships that could be construed as a potential conflict of interest.
All claims expressed in this article are solely those of the authors and do not necessarily represent those of their affiliated organizations, or those of the publisher, the editors and the reviewers. Any product that may be evaluated in this article, or claim that may be made by its manufacturer, is not guaranteed or endorsed by the publisher.
1. Rakoff-Nahoum S, Medzhitov R. Toll-like receptors and cancer. Nat Rev Cancer (2009) 9(1):57–63. doi: 10.1038/nrc2541
2. Loiarro M, Ruggiero V, Sette C. Targeting Tlr/Il-1r signalling in human diseases. Mediators Inflammation (2010) 2010:674363. doi: 10.1155/2010/674363
3. Gnjatic S, Sawhney NB, Bhardwaj N. Toll-like receptor agonists: Are they good adjuvants? Cancer J (Sudbury Mass) (2010) 16(4):382–91. doi: 10.1097/PPO.0b013e3181eaca65
4. Chang ZL. Important aspects of toll-like receptors, ligands and their signaling pathways. Inflammation research: Off J Eur Histamine Res Soc [et al] (2010) 59(10):791–808. doi: 10.1007/s00011-010-0208-2
5. Patidar A, Selvaraj S, Sarode A, Chauhan P, Chattopadhyay D, Saha B. Damp-Tlr-Cytokine axis dictates the fate of tumor. Cytokine (2018) 104:114–23. doi: 10.1016/j.cyto.2017.10.004
6. Kawai T, Akira S. The role of pattern-recognition receptors in innate immunity: Update on toll-like receptors. Nat Immunol (2010) 11(5):373–84. doi: 10.1038/ni.1863
7. Anwar MA, Shah M, Kim J, Choi S. Recent clinical trends in toll-like receptor targeting therapeutics. Medicinal Res Rev (2019) 39(3):1053–90. doi: 10.1002/med.21553
8. Roach JC, Glusman G, Rowen L, Kaur A, Purcell MK, Smith KD, et al. The evolution of vertebrate toll-like receptors. Proc Natl Acad Sci U.S.A. (2005) 102(27):9577–82. doi: 10.1073/pnas.0502272102
9. Takeuchi O, Akira S. Pattern recognition receptors and inflammation. Cell (2010) 140(6):805–20. doi: 10.1016/j.cell.2010.01.022
10. Bayraktar R, Bertilaccio MTS, Calin GA. The interaction between two worlds: Micrornas and toll-like receptors. Front Immunol (2019) 10:1053. doi: 10.3389/fimmu.2019.01053
11. Bourquin C, Pommier A, Hotz C. Harnessing the immune system to fight cancer with toll-like receptor and rig-I-Like receptor agonists. Pharmacol Res (2019) 154:104192. doi: 10.1016/j.phrs.2019.03.001
12. Takeda K, Akira S. Tlr Signaling Pathways. Seminars in immunology (2004) 16(1):3–9. doi: 10.1016/j.smim.2003.10.003
13. Hennessy EJ, Parker AE, O’Neill LA. Targeting toll-like receptors: Emerging therapeutics? Nat Rev Drug Discovery (2010) 9(4):293–307. doi: 10.1038/nrd3203
14. Iwasaki A, Medzhitov R. Regulation of adaptive immunity by the innate immune system. Sci (New York NY) (2010) 327(5963):291–5. doi: 10.1126/science.1183021
15. Hua Z, Hou B. Tlr signaling in b-cell development and activation. Cell Mol Immunol (2013) 10(2):103–6. doi: 10.1038/cmi.2012.61
16. Haage V, Elmadany N, Roll L, Faissner A, Gutmann DH, Semtner M, et al. Tenascin c regulates multiple microglial functions involving Tlr4 signaling and Hdac1. Brain behavior Immun (2019) 81:470–83. doi: 10.1016/j.bbi.2019.06.047
17. Seki E, Tsutsui H, Iimuro Y, Naka T, Son G, Akira S, et al. Contribution of toll-like Receptor/Myeloid differentiation factor 88 signaling to murine liver regeneration. Hepatol (Baltimore Md) (2005) 41(3):443–50. doi: 10.1002/hep.20603
18. Chen Y, Sun R. Toll-like receptors in acute liver injury and regeneration. Int Immunopharmacol (2011) 11(10):1433–41. doi: 10.1016/j.intimp.2011.04.023
19. Kim D, Chen R, Sheu M, Kim N, Kim S, Islam N, et al. Noncoding dsrna induces retinoic acid synthesis to stimulate hair follicle regeneration Via Tlr3. Nat Commun (2019) 10(1):2811. doi: 10.1038/s41467-019-10811-y
20. Hasan UA, Trinchieri G, Vlach J. Toll-like receptor signaling stimulates cell cycle entry and progression in fibroblasts. J Biol Chem (2005) 280(21):20620–7. doi: 10.1074/jbc.M500877200
21. Hari P, Millar FR, Tarrats N, Birch J, Quintanilla A, Rink CJ, et al. The innate immune sensor toll-like receptor 2 controls the senescence-associated secretory phenotype. Sci Adv (2019) 5(6):eaaw0254. doi: 10.1126/sciadv.aaw0254
22. Mogilenko DA, Haas JT, L’Homme L, Fleury S, Quemener S, Levavasseur M, et al. Metabolic and innate immune cues merge into a specific inflammatory response Via the upr. Cell (2019) 177(5):1201–16.e19. doi: 10.1016/j.cell.2019.03.018
23. Krawczyk CM, Holowka T, Sun J, Blagih J, Amiel E, DeBerardinis RJ, et al. Toll-like receptor-induced changes in glycolytic metabolism regulate dendritic cell activation. Blood (2010) 115(23):4742–9. doi: 10.1182/blood-2009-10-249540
24. Pradere JP, Dapito DH, Schwabe RF. The yin and yang of toll-like receptors in cancer. Oncogene (2014) 33(27):3485–95. doi: 10.1038/onc.2013.302
25. Huang B, Zhao J, Unkeless JC, Feng ZH, Xiong H. Tlr signaling by tumor and immune cells: A double-edged sword. Oncogene (2008) 27(2):218–24. doi: 10.1038/sj.onc.1210904
26. Conroy H, Marshall NA, Mills KH. Tlr ligand suppression or enhancement of treg cells? a double-edged sword in immunity to tumours. Oncogene (2008) 27(2):168–80. doi: 10.1038/sj.onc.1210910
27. Smith M, Garcia-Martinez E, Pitter MR, Fucikova J, Spisek R, Zitvogel L, et al. Trial watch: Toll-like receptor agonists in cancer immunotherapy. Oncoimmunology (2018) 7(12):e1526250. doi: 10.1080/2162402x.2018.1526250
28. Guha M. Anticancer tlr agonists on the ropes. Nat Rev Drug Discovery (2012) 11(7):503–5. doi: 10.1038/nrd3775
29. Lu H. Tlr agonists for cancer immunotherapy: Tipping the balance between the immune stimulatory and inhibitory effects. Front Immunol (2014) 5:83. doi: 10.3389/fimmu.2014.00083
30. Carpenter S, O’Neill LA. Recent insights into the structure of toll-like receptors and post-translational modifications of their associated signalling proteins. Biochem J (2009) 422(1):1–10. doi: 10.1042/BJ20090616
31. Lee BL, Barton GM. Trafficking of endosomal toll-like receptors. Trends Cell Biol (2014) 24(6):360–9. doi: 10.1016/j.tcb.2013.12.002
32. Hedayat M, Takeda K, Rezaei N. Prophylactic and therapeutic implications of toll-like receptor ligands. Medicinal Res Rev (2012) 32(2):294–325. doi: 10.1002/med.20214
33. Patra MC, Shah M, Choi S. Toll-like receptor-induced cytokines as immunotherapeutic targets in cancers and autoimmune diseases. Semin Cancer Biol (2019) 64:61–82. doi: 10.1016/j.semcancer.2019.05.002
34. Blander JM, Medzhitov R. On regulation of phagosome maturation and antigen presentation. Nat Immunol (2006) 7(10):1029–35. doi: 10.1038/ni1006-1029
35. Murakami Y, Fukui R, Motoi Y, Kanno A, Shibata T, Tanimura N, et al. Roles of the cleaved n-terminal Tlr3 fragment and cell surface Tlr3 in double-stranded rna sensing. J Immunol (Baltimore Md: 1950) (2014) 193(10):5208–17. doi: 10.4049/jimmunol.1400386
36. Matsumoto M, Seya T. Tlr3: Interferon induction by double-stranded rna including Poly(I:C). Advanced Drug delivery Rev (2008) 60(7):805–12. doi: 10.1016/j.addr.2007.11.005
37. Guan Y, Ranoa DR, Jiang S, Mutha SK, Li X, Baudry J, et al. Human tlrs 10 and 1 share common mechanisms of innate immune sensing but not signaling. J Immunol (Baltimore Md: 1950) (2010) 184(9):5094–103. doi: 10.4049/jimmunol.0901888
38. Takeuchi O, Sato S, Horiuchi T, Hoshino K, Takeda K, Dong Z, et al. Cutting edge: Role of toll-like receptor 1 in mediating immune response to microbial lipoproteins. J Immunol (Baltimore Md: 1950) (2002) 169(1):10–4. doi: 10.4049/jimmunol.169.1.10
39. Mansell A, Jenkins BJ. Dangerous liaisons between interleukin-6 cytokine and toll-like receptor families: A potent combination in inflammation and cancer. Cytokine Growth factor Rev (2013) 24(3):249–56. doi: 10.1016/j.cytogfr.2013.03.007
40. Khan AA, Khan Z, Warnakulasuriya S. Cancer-associated toll-like receptor modulation and insinuation in infection susceptibility: Association or coincidence? Ann oncology: Off J Eur Soc Med Oncol (2016) 27(6):984–97. doi: 10.1093/annonc/mdw053
41. Kanzler H, Barrat FJ, Hessel EM, Coffman RL. Therapeutic targeting of innate immunity with toll-like receptor agonists and antagonists. Nat Med (2007) 13(5):552–9. doi: 10.1038/nm1589
42. Khajeh Alizadeh Attar M, Anwar MA, Eskian M, Keshavarz-Fathi M, Choi S, Rezaei N. Basic understanding and therapeutic approaches to target toll-like receptors in cancerous microenvironment and metastasis. Medicinal Res Rev (2018) 38(5):1469–84. doi: 10.1002/med.21480
43. Medvedev AE. Toll-like receptor polymorphisms, inflammatory and infectious diseases, allergies, and cancer. J Interferon Cytokine research: Off J Int Soc Interferon Cytokine Res (2013) 33(9):467–84. doi: 10.1089/jir.2012.0140
44. Takeda K, Akira S. Toll-like receptors in innate immunity. Int Immunol (2005) 17(1):1–14. doi: 10.1093/intimm/dxh186
45. Satoh T, Akira S. Toll-like receptor signaling and its inducible proteins. Microbiol Spectr (2016) 4(6):4.6.41. doi: 10.1128/microbiolspec.MCHD-0040-2016
46. Blasius AL, Beutler B. Intracellular toll-like receptors. Immunity (2010) 32(3):305–15. doi: 10.1016/j.immuni.2010.03.012
47. Fitzgerald KA, Kagan JC. Toll-like receptors and the control of immunity. Cell (2020) 180(6):1044–66. doi: 10.1016/j.cell.2020.02.041
48. LaRue H, Ayari C, Bergeron A, Fradet Y. Toll-like receptors in urothelial cells–targets for cancer immunotherapy. Nat Rev Urol (2013) 10(9):537–45. doi: 10.1038/nrurol.2013.153
49. Wardill HR, Gibson RJ, Logan RM, Bowen JM. Tlr4/Pkc-mediated tight junction modulation: A clinical marker of chemotherapy-induced gut toxicity? Int J Cancer (2014) 135(11):2483–92. doi: 10.1002/ijc.28656
50. Basith S, Manavalan B, Yoo TH, Kim SG, Choi S. Roles of toll-like receptors in cancer: A double-edged sword for defense and offense. Arch pharmacal Res (2012) 35(8):1297–316. doi: 10.1007/s12272-012-0802-7
51. Piccinini AM, Midwood KS. Dampening inflammation by modulating tlr signalling. Mediators Inflammation (2010) 2010:672395. doi: 10.1155/2010/672395
52. Kariko K, Ni HP, Capodici J, Lamphier M, Weissman D. Mrna is an endogenous ligand for toll-like receptor 3. J Biol Chem (2004) 279(13):12542–50. doi: 10.1074/jbc.M310175200
53. Vollmer J, Tluk S, Schmitz C, Hamm S, Jurk M, Forsbach A, et al. Immune stimulation mediated by autoantigen binding sites within small nuclear rnas involves toll-like receptors 7 and 8. J Exp Med (2005) 202(11):1575–85. doi: 10.1084/jem.20051696
54. Hurst J, Prinz N, Lorenz M, Bauer S, Chapman J, Lackner KJ, et al. Tlr7 and Tlr8 ligands and antiphospholipid antibodies show synergistic effects on the induction of il-1beta and caspase-1 in monocytes and dendritic cells. Immunobiology (2009) 214(8):683–91. doi: 10.1016/j.imbio.2008.12.003
55. Leadbetter EA, Rifkin IR, Hohlbaum AM, Beaudette BC, Shlomchik MJ, Marshak-Rothstein A. Chromatin-igg complexes activate b cells by dual engagement of igm and toll-like receptors. Nature (2002) 416(6881):603–7. doi: 10.1038/416603a
56. Lotfi R, Eisenbacher J, Solgi G, Fuchs K, Yildiz T, Nienhaus C, et al. Human mesenchymal stem cells respond to native but not oxidized damage associated molecular pattern molecules from necrotic (Tumor) material. Eur J Immunol (2011) 41(7):2021–8. doi: 10.1002/eji.201041324
57. Szczepanski MJ, Czystowska M, Szajnik M, Harasymczuk M, Boyiadzis M, Kruk-Zagajewska A, et al. Triggering of toll-like receptor 4 expressed on human head and neck squamous cell carcinoma promotes tumor development and protects the tumor from immune attack. Cancer Res (2009) 69(7):3105–13. doi: 10.1158/0008-5472.Can-08-3838
58. Fonte E, Agathangelidis A, Reverberi D, Ntoufa S, Scarfo L, Ranghetti P, et al. Toll-like receptor stimulation in splenic marginal zone lymphoma can modulate cell signaling, activation and proliferation. Haematologica (2015) 100(11):1460–8. doi: 10.3324/haematol.2014.119933
59. Micera A, Balzamino BO, Di Zazzo A, Biamonte F, Sica G, Bonini S. Toll-like receptors and tissue remodeling: The Pro/Cons recent findings. J Cell Physiol (2016) 231(3):531–44. doi: 10.1002/jcp.25124
60. Cen X, Zhu G, Yang J, Yang J, Guo J, Jin J, et al. Tlr1/2 specific small-molecule agonist suppresses leukemia cancer cell growth by stimulating cytotoxic T lymphocytes. Advanced Sci (Weinheim Baden-Wurttemberg Germany) (2019) 6(10):1802042. doi: 10.1002/advs.201802042
61. Quach H, Rotival M, Pothlichet J, Loh YE, Dannemann M, Zidane N, et al. Genetic adaptation and neandertal admixture shaped the immune system of human populations. Cell (2016) 167(3):643–56.e17. doi: 10.1016/j.cell.2016.09.024
62. Sharma N, Vacher J, Allison JP. Tlr1/2 ligand enhances antitumor efficacy of ctla-4 blockade by increasing intratumoral treg depletion. Proc Natl Acad Sci U.S.A. (2019) 116(21):10453–62. doi: 10.1073/pnas.1819004116
63. Zom GG, Willems M, Khan S, van der Sluis TC, Kleinovink JW, Camps MGM, et al. Novel Tlr2-binding adjuvant induces enhanced T cell responses and tumor eradication. J immunotherapy Cancer (2018) 6(1):146. doi: 10.1186/s40425-018-0455-2
64. Wang Y, Su L, Morin MD, Jones BT, Mifune Y, Shi H, et al. Adjuvant effect of the novel Tlr1/Tlr2 agonist diprovocim synergizes with anti-Pd-L1 to eliminate melanoma in mice. Proc Natl Acad Sci U.S.A. (2018) 115(37):E8698–e706. doi: 10.1073/pnas.1809232115
65. Feng Y, Mu R, Wang Z, Xing P, Zhang J, Dong L, et al. A toll-like receptor agonist mimicking microbial signal to generate tumor-suppressive macrophages. Nat Commun (2019) 10(1):2272. doi: 10.1038/s41467-019-10354-2
66. Meng Y, Qu Y, Wu W, Chen L, Sun L, Tai G, et al. Galactan isolated from cantharellus cibarius modulates antitumor immune response by converting tumor-associated macrophages toward M1-like phenotype. Carbohydr polymers (2019) 226:115295. doi: 10.1016/j.carbpol.2019.115295
67. Samudio I, Rezvani K, Shaim H, Hofs E, Ngom M, Bu L, et al. Uv-inactivated hsv-1 potently activates nk cell killing of leukemic cells. Blood (2016) 127(21):2575–86. doi: 10.1182/blood-2015-04-639088
68. Salem ML, Attia ZI, Galal SM. Acute inflammation induces immunomodulatory effects on myeloid cells associated with anti-tumor responses in a tumor mouse model. J advanced Res (2016) 7(2):243–53. doi: 10.1016/j.jare.2015.06.001
69. Aznar MA, Planelles L, Perez-Olivares M, Molina C, Garasa S, Etxeberria I, et al. Immunotherapeutic effects of intratumoral nanoplexed poly I:C. J immunotherapy Cancer (2019) 7(1):116. doi: 10.1186/s40425-019-0568-2
70. Ding L, Ren J, Zhang D, Li Y, Huang X, Ji J, et al. The Tlr3 agonist inhibit drug efflux and sequentially consolidates low-dose cisplatin-based chemoimmunotherapy while reducing side effects. Mol Cancer Ther (2017) 16(6):1068–79. doi: 10.1158/1535-7163.MCT-16-0454
71. Alkurdi L, Virard F, Vanbervliet B, Weber K, Toscano F, Bonnin M, et al. Release of c-flip brake selectively sensitizes human cancer cells to Tlr3-mediated apoptosis. Cell Death Dis (2018) 9(9):874. doi: 10.1038/s41419-018-0850-0
72. Zemek RM, De Jong E, Chin WL, Schuster IS, Fear VS, Casey TH, et al. Sensitization to immune checkpoint blockade through activation of a Stat1/Nk axis in the tumor microenvironment. Sci Transl Med (2019) 11(501):eaav7816. doi: 10.1126/scitranslmed.aav7816
73. Azuma M, Takeda Y, Nakajima H, Sugiyama H, Ebihara T, Oshiumi H, et al. Biphasic function of Tlr3 adjuvant on tumor and spleen dendritic cells promotes tumor T cell infiltration and regression in a vaccine therapy. Oncoimmunology (2016) 5(8):e1188244. doi: 10.1080/2162402x.2016.1188244
74. Nimanong S, Ostroumov D, Wingerath J, Knocke S, Woller N, Gurlevik E, et al. Cd40 signaling drives potent cellular immune responses in heterologous cancer vaccinations. Cancer Res (2017) 77(8):1918–26. doi: 10.1158/0008-5472.Can-16-2089
75. Chen YL, Chang MC, Chiang YC, Lin HW, Sun NY, Chen CA, et al. Immuno-modulators enhance antigen-specific immunity and anti-tumor effects of mesothelin-specific chimeric DNA vaccine through promoting dc maturation. Cancer Lett (2018) 425:152–63. doi: 10.1016/j.canlet.2018.03.032
76. Zhao J, Zhang Z, Xue Y, Wang G, Cheng Y, Pan Y, et al. Anti-tumor macrophages activated by ferumoxytol combined or surface-functionalized with the Tlr3 agonist poly (I: C) promote melanoma regression. Theranostics (2018) 8(22):6307–21. doi: 10.7150/thno.29746
77. Liu L, He H, Liang R, Yi H, Meng X, Chen Z, et al. Ros-inducing micelles sensitize tumor-associated macrophages to Tlr3 stimulation for potent immunotherapy. Biomacromolecules (2018) 19(6):2146–55. doi: 10.1021/acs.biomac.8b00239
78. Kim SY, Noh YW, Kang TH, Kim JE, Kim S, Um SH, et al. Synthetic vaccine nanoparticles target to lymph node triggering enhanced innate and adaptive antitumor immunity. Biomaterials (2017) 130:56–66. doi: 10.1016/j.biomaterials.2017.03.034
79. Rahimian S, Fransen MF, Kleinovink JW, Christensen JR, Amidi M, Hennink WE, et al. Polymeric nanoparticles for Co-delivery of synthetic long peptide antigen and poly ic as therapeutic cancer vaccine formulation. J Controlled release: Off J Controlled Release Soc (2015) 203:16–22. doi: 10.1016/j.jconrel.2015.02.006
80. Luo Z, Wang C, Yi H, Li P, Pan H, Liu L, et al. Nanovaccine loaded with poly I:C and Stat3 sirna robustly elicits anti-tumor immune responses through modulating tumor-associated dendritic cells in vivo. Biomaterials (2015) 38:50–60. doi: 10.1016/j.biomaterials.2014.10.050
81. Takeda Y, Kataoka K, Yamagishi J, Ogawa S, Seya T, Matsumoto M. A Tlr3-specific adjuvant relieves innate resistance to pd-L1 blockade without cytokine toxicity in tumor vaccine immunotherapy. Cell Rep (2017) 19(9):1874–87. doi: 10.1016/j.celrep.2017.05.015
82. Nelson MH, Bowers JS, Bailey SR, Diven MA, Fugle CW, Kaiser AD, et al. Toll-like receptor agonist therapy can profoundly augment the antitumor activity of adoptively transferred Cd8(+) T cells without host preconditioning. J immunotherapy Cancer (2016) 4:6. doi: 10.1186/s40425-016-0110-8
83. Atukorale PU, Raghunathan SP, Raguveer V, Moon TJ, Zheng C, Bielecki PA, et al. Nanoparticle encapsulation of synergistic immune agonists enables systemic codelivery to tumor sites and ifnbeta-driven antitumor immunity. Cancer Res (2019) 79(20):5394–406. doi: 10.1158/0008-5472.Can-19-0381
84. Haro MA, Dyevoich AM, Phipps JP, Haas KM. Activation of b-1 cells promotes tumor cell killing in the peritoneal cavity. Cancer Res (2019) 79(1):159–70. doi: 10.1158/0008-5472.Can-18-0981
85. Tang AC, Rahavi SM, Fung SY, Lu HY, Yang H, Lim CJ, et al. Combination therapy with proteasome inhibitors and tlr agonists enhances tumour cell death and il-1beta production. Cell Death Dis (2018) 9(2):162. doi: 10.1038/s41419-017-0194-1
86. Gableh F, Saeidi M, Hemati S, Hamdi K, Soleimanjahi H, Gorji A, et al. Combination of the toll like receptor agonist and alpha-galactosylceramide as an efficient adjuvant for cancer vaccine. J Biomed Sci (2016) 23:16. doi: 10.1186/s12929-016-0238-3
87. Zamani P, Navashenaq JG, Nikpoor AR, Hatamipour M, Oskuee RK, Badiee A, et al. Mpl nano-liposomal vaccine containing P5 Her2/Neu-derived peptide pulsed padre as an effective vaccine in a mice tubo model of breast cancer. J Controlled release: Off J Controlled Release Soc (2019) 303:223–36. doi: 10.1016/j.jconrel.2019.04.019
88. Boks MA, Ambrosini M, Bruijns SC, Kalay H, van Bloois L, Storm G, et al. Mpla incorporation into dc-targeting glycoliposomes favours anti-tumour T cell responses. J Controlled release: Off J Controlled Release Soc (2015) 216:37–46. doi: 10.1016/j.jconrel.2015.06.033
89. Zhuang X, Wu T, Zhao Y, Hu X, Bao Y, Guo Y, et al. Lipid-enveloped zinc phosphate hybrid nanoparticles for codelivery of h-2k(B) and h-2d(B)-Restricted antigenic peptides and monophosphoryl lipid a to induce antitumor immunity against melanoma. J Controlled release: Off J Controlled Release Soc (2016) 228:26–37. doi: 10.1016/j.jconrel.2016.02.035
90. Guo Y, Wang D, Song Q, Wu T, Zhuang X, Bao Y, et al. Erythrocyte membrane-enveloped polymeric nanoparticles as nanovaccine for induction of antitumor immunity against melanoma. ACS Nano (2015) 9(7):6918–33. doi: 10.1021/acsnano.5b01042
91. Wafa EI, Geary SM, Ross KA, Goodman JT, Narasimhan B, Salem AK. Pentaerythritol-based lipid a bolsters the antitumor efficacy of a polyanhydride particle-based cancer vaccine. Nanomedicine: nanotechnology biology Med (2019) 21:102055. doi: 10.1016/j.nano.2019.102055
92. Xu L, Kwak M, Zhang W, Zeng L, Lee PC, Jin JO. Rehmannia glutinosa polysaccharide induces toll-like receptor 4 dependent spleen dendritic cell maturation and anti-cancer immunity. Oncoimmunology (2017) 6(7):e1325981. doi: 10.1080/2162402x.2017.1325981
93. Villanueva L, Silva L, Llopiz D, Ruiz M, Iglesias T, Lozano T, et al. The toll like receptor 4 ligand cold-inducible rna-binding protein as vaccination platform against cancer. Oncoimmunology (2018) 7(4):e1409321. doi: 10.1080/2162402x.2017.1409321
94. Kim YS, Park HJ, Park JH, Hong EJ, Jang GY, Jung ID, et al. A novel function of Api5 (Apoptosis inhibitor 5), Tlr4-dependent activation of antigen presenting cells. Oncoimmunology (2018) 7(10):e1472187. doi: 10.1080/2162402x.2018.1472187
95. Wei F, Yang D, Tewary P, Li Y, Li S, Chen X, et al. The alarmin Hmgn1 contributes to antitumor immunity and is a potent immunoadjuvant. Cancer Res (2014) 74(21):5989–98. doi: 10.1158/0008-5472.Can-13-2042
96. Lee SE, Hong SH, Verma V, Lee YS, Duong TN, Jeong K, et al. Flagellin is a strong vaginal adjuvant of a therapeutic vaccine for genital cancer. Oncoimmunology (2016) 5(2):e1081328. doi: 10.1080/2162402x.2015.1081328
97. Geng D, Kaczanowska S, Tsai A, Younger K, Ochoa A, Rapoport AP, et al. Tlr5 ligand-secreting T cells reshape the tumor microenvironment and enhance antitumor activity. Cancer Res (2015) 75(10):1959–71. doi: 10.1158/0008-5472.Can-14-2467
98. Brackett CM, Kojouharov B, Veith J, Greene KF, Burdelya LG, Gollnick SO, et al. Toll-like receptor-5 agonist, entolimod, suppresses metastasis and induces immunity by stimulating an nk-Dendritic-Cd8+ T-cell axis. Proc Natl Acad Sci U.S.A. (2016) 113(7):E874–83. doi: 10.1073/pnas.1521359113
99. Doorduijn EM, Sluijter M, Salvatori DC, Silvestri S, Maas S, Arens R, et al. Cd4(+) T cell and nk cell interplay key to regression of mhc class I(Low) tumors upon Tlr7/8 agonist therapy. Cancer Immunol Res (2017) 5(8):642–53. doi: 10.1158/2326-6066.Cir-16-0334
100. Soong RS, Song L, Trieu J, Knoff J, He L, Tsai YC, et al. Toll-like receptor agonist imiquimod facilitates antigen-specific Cd8+ T-cell accumulation in the genital tract leading to tumor control through ifngamma. Clin Cancer research: an Off J Am Assoc Cancer Res (2014) 20(21):5456–67. doi: 10.1158/1078-0432.Ccr-14-0344
101. Wei J, Long Y, Guo R, Liu X, Tang X, Rao J, et al. Multifunctional polymeric micelle-based chemo-immunotherapy with immune checkpoint blockade for efficient treatment of orthotopic and metastatic breast cancer. Acta Pharm Sin B (2019) 9(4):819–31. doi: 10.1016/j.apsb.2019.01.018
102. Nuhn L, De Koker S, Van Lint S, Zhong Z, Catani JP, Combes F, et al. Nanoparticle-conjugate Tlr7/8 agonist localized immunotherapy provokes safe antitumoral responses. Advanced materials (Deerfield Beach Fla) (2018) 30(45):e1803397. doi: 10.1002/adma.201803397
103. Xu J, Xu L, Wang C, Yang R, Zhuang Q, Han X, et al. Near-Infrared-Triggered photodynamic therapy with multitasking upconversion nanoparticles in combination with checkpoint blockade for immunotherapy of colorectal cancer. ACS Nano (2017) 11(5):4463–74. doi: 10.1021/acsnano.7b00715
104. Ge R, Liu C, Zhang X, Wang W, Li B, Liu J, et al. Photothermal-activatable Fe3o4 superparticle nanodrug carriers with pd-L1 immune checkpoint blockade for anti-metastatic cancer immunotherapy. ACS Appl materials interfaces (2018) 10(24):20342–55. doi: 10.1021/acsami.8b05876
105. Chen Q, Xu L, Liang C, Wang C, Peng R, Liu Z. Photothermal therapy with immune-adjuvant nanoparticles together with checkpoint blockade for effective cancer immunotherapy. Nat Commun (2016) 7:13193. doi: 10.1038/ncomms13193
106. Chen Q, Chen J, Yang Z, Xu J, Xu L, Liang C, et al. Nanoparticle-enhanced radiotherapy to trigger robust cancer immunotherapy. Advanced materials (Deerfield Beach Fla) (2019) 31(10):e1802228. doi: 10.1002/adma.201802228
107. Yang R, Xu J, Xu L, Sun X, Chen Q, Zhao Y, et al. Cancer cell membrane-coated adjuvant nanoparticles with mannose modification for effective anticancer vaccination. ACS Nano (2018) 12(6):5121–9. doi: 10.1021/acsnano.7b09041
108. Zhou Z, Yu X, Zhang J, Tian Z, Zhang C. Tlr7/8 agonists promote nk-dc cross-talk to enhance nk cell anti-tumor effects in hepatocellular carcinoma. Cancer Lett (2015) 369(2):298–306. doi: 10.1016/j.canlet.2015.09.017
109. Kumai T, Lee S, Cho HI, Sultan H, Kobayashi H, Harabuchi Y, et al. Optimization of peptide vaccines to induce robust antitumor Cd4 T-cell responses. Cancer Immunol Res (2017) 5(1):72–83. doi: 10.1158/2326-6066.Cir-16-0194
110. Hosoya T, Sato-Kaneko F, Ahmadi A, Yao S, Lao F, Kitaura K, et al. Induction of oligoclonal Cd8 T cell responses against pulmonary metastatic cancer by a phospholipid-conjugated Tlr7 agonist. Proc Natl Acad Sci U.S.A. (2018) 115(29):E6836–e44. doi: 10.1073/pnas.1803281115
111. Narayanan JSS, Ray P, Hayashi T, Whisenant TC, Vicente D, Carson DA, et al. Irreversible electroporation combined with checkpoint blockade and Tlr7 stimulation induces antitumor immunity in a murine pancreatic cancer model. Cancer Immunol Res (2019) 7(10):1714–26. doi: 10.1158/2326-6066.Cir-19-0101
112. Vascotto F, Petschenka J, Walzer KC, Vormehr M, Brkic M, Strobl S, et al. Intravenous delivery of the toll-like receptor 7 agonist Sc1 confers tumor control by inducing a Cd8+ T cell response. Oncoimmunology (2019) 8(7):1601480. doi: 10.1080/2162402x.2019.1601480
113. Wiedemann GM, Jacobi SJ, Chaloupka M, Krachan A, Hamm S, Strobl S, et al. A novel Tlr7 agonist reverses nk cell anergy and cures rma-s lymphoma-bearing mice. Oncoimmunology (2016) 5(7):e1189051. doi: 10.1080/2162402x.2016.1189051
114. Zhu J, He S, Du J, Wang Z, Li W, Chen X, et al. Local administration of a novel toll-like receptor 7 agonist in combination with doxorubicin induces durable tumouricidal effects in a murine model of T cell lymphoma. J Hematol Oncol (2015) 8:21. doi: 10.1186/s13045-015-0121-9
115. Huang Z, Gan J, Long Z, Guo G, Shi X, Wang C, et al. Targeted delivery of let-7b to reprogramme tumor-associated macrophages and tumor infiltrating dendritic cells for tumor rejection. Biomaterials (2016) 90:72–84. doi: 10.1016/j.biomaterials.2016.03.009
116. Kranz LM, Diken M, Haas H, Kreiter S, Loquai C, Reuter KC, et al. Systemic rna delivery to dendritic cells exploits antiviral defence for cancer immunotherapy. Nature (2016) 534(7607):396–+. doi: 10.1038/nature18300
117. Pilch Z, Tonecka K, Braniewska A, Sas Z, Skorzynski M, Boon L, et al. Antitumor activity of Tlr7 is potentiated by Cd200r antibody leading to changes in the tumor microenvironment. Cancer Immunol Res (2018) 6(8):930–40. doi: 10.1158/2326-6066.Cir-17-0454
118. Cheadle EJ, Lipowska-Bhalla G, Dovedi SJ, Fagnano E, Klein C, Honeychurch J, et al. A Tlr7 agonist enhances the antitumor efficacy of obinutuzumab in murine lymphoma models Via nk cells and Cd4 T cells. Leukemia (2017) 31(7):1611–21. doi: 10.1038/leu.2016.352
119. Park CG, Hartl CA, Schmid D, Carmona EM, Kim HJ, Goldberg MS. Extended release of perioperative immunotherapy prevents tumor recurrence and eliminates metastases. Sci Transl Med (2018) 10(433):eaar1916. doi: 10.1126/scitranslmed.aar1916
120. Lu R, Groer C, Kleindl PA, Moulder KR, Huang A, Hunt JR, et al. Formulation and preclinical evaluation of a toll-like receptor 7/8 agonist as an anti-tumoral immunomodulator. J Controlled release: Off J Controlled Release Soc (2019) 306:165–76. doi: 10.1016/j.jconrel.2019.06.003
121. Schmid D, Park CG, Hartl CA, Subedi N, Cartwright AN, Puerto RB, et al. T Cell-targeting nanoparticles focus delivery of immunotherapy to improve antitumor immunity. Nat Commun (2017) 8(1):1747. doi: 10.1038/s41467-017-01830-8
122. Rodell CB, Arlauckas SP, Cuccarese MF, Garris CS, Ahmed RLMS, Kohler RH, et al. Tlr7/8-Agonist-Loaded nanoparticles promote the polarization of tumour-associated macrophages to enhance cancer immunotherapy. Nat Biomed Eng (2018) 2(8):578–+. doi: 10.1038/s41551-018-0236-8
123. Kim SY, Kim S, Kim JE, Lee SN, Shin IW, Shin HS, et al. Lyophilizable and multifaceted toll-like receptor 7/8 agonist-loaded nanoemulsion for the reprogramming of tumor microenvironments and enhanced cancer immunotherapy. ACS Nano (2019) 13:12671–86. doi: 10.1021/acsnano.9b04207
124. Kim H, Sehgal D, Kucaba TA, Ferguson DM, Griffith TS, Panyam J. Acidic ph-responsive polymer nanoparticles as a Tlr7/8 agonist delivery platform for cancer immunotherapy. Nanoscale (2018) 10(44):20851–62. doi: 10.1039/c8nr07201a
125. Kim H, Niu L, Larson P, Kucaba TA, Murphy KA, James BR, et al. Polymeric nanoparticles encapsulating novel Tlr7/8 agonists as immunostimulatory adjuvants for enhanced cancer immunotherapy. Biomaterials (2018) 164:38–53. doi: 10.1016/j.biomaterials.2018.02.034
126. Persano S, Guevara ML, Li Z, Mai J, Ferrari M, Pompa PP, et al. Lipopolyplex potentiates anti-tumor immunity of mrna-based vaccination. Biomaterials (2017) 125:81–9. doi: 10.1016/j.biomaterials.2017.02.019
127. Liu M, O’Connor RS, Trefely S, Graham K, Snyder NW, Beatty GL. Metabolic rewiring of macrophages by cpg potentiates clearance of cancer cells and overcomes tumor-expressed Cd47-mediated ‘Don’t-Eat-Me’ signal. Nat Immunol (2019) 20(3):265–75. doi: 10.1038/s41590-018-0292-y
128. Lin YC, Hsu CY, Huang SK, Fan YH, Huang CH, Yang CK, et al. Induction of liver-specific intrahepatic myeloid cells aggregation expands Cd8 T cell and inhibits growth of murine hepatoma. Oncoimmunology (2018) 7(12):e1502129. doi: 10.1080/2162402x.2018.1502129
129. Benbenishty A, Gadrich M, Cottarelli A, Lubart A, Kain D, Amer M, et al. Prophylactic Tlr9 stimulation reduces brain metastasis through microglia activation. PloS Biol (2019) 17(3):e2006859. doi: 10.1371/journal.pbio.2006859
130. Sagiv-Barfi I, Czerwinski DK, Levy S, Alam IS, Mayer AT, Gambhir SS, et al. Eradication of spontaneous malignancy by local immunotherapy. Sci Trans Med (2018) 10(426):eaan4488. doi: 10.1126/scitranslmed.aan4488
131. Sagiv-Barfi I, Kohrt HE, Burckhardt L, Czerwinski DK, Levy R. Ibrutinib enhances the antitumor immune response induced by intratumoral injection of a Tlr9 ligand in mouse lymphoma. Blood (2015) 125(13):2079–86. doi: 10.1182/blood-2014-08-593137
132. Jordan M, Waxman DJ. Cpg-1826 immunotherapy potentiates chemotherapeutic and anti-tumor immune responses to metronomic cyclophosphamide in a preclinical glioma model. Cancer Lett (2016) 373(1):88–96. doi: 10.1016/j.canlet.2015.11.029
133. Gallotta M, Assi H, Degagné É, Kannan SK, Coffman RL, Guiducci C. Inhaled Tlr9 agonist renders lung tumors permissive to pd-1 blockade by promoting optimal Cd4(+) and Cd8(+) T-cell interplay. Cancer Res (2018) 78(17):4943–56. doi: 10.1158/0008-5472.Can-18-0729
134. Wang S, Campos J, Gallotta M, Gong M, Crain C, Naik E, et al. Intratumoral injection of a cpg oligonucleotide reverts resistance to pd-1 blockade by expanding multifunctional Cd8+ T cells. Proc Natl Acad Sci U.S.A. (2016) 113(46):E7240–e9. doi: 10.1073/pnas.1608555113
135. Marabelle A, Kohrt H, Sagiv-Barfi I, Ajami B, Axtell RC, Zhou G, et al. Depleting tumor-specific tregs at a single site eradicates disseminated tumors. J Clin Invest (2013) 123(6):2447–63. doi: 10.1172/jci64859
136. Nie Y, He J, Shirota H, Trivett AL, Yang D, DM K, et al. Blockade of Tnfr2 signaling enhances the immunotherapeutic effect of cpg odn in a mouse model of colon cancer. Sci Signaling (2018) 11(511):eaan0790. doi: 10.1126/scisignal.aan0790
137. Xu A, Zhang L, Yuan J, Babikr F, Freywald A, Chibbar R, et al. Tlr9 agonist enhances radiofrequency ablation-induced ctl responses, leading to the potent inhibition of primary tumor growth and lung metastasis. Cell Mol Immunol (2019) 16(10):820–32. doi: 10.1038/s41423-018-0184-y
138. Behm B, Di Fazio P, Michl P, Neureiter D, Kemmerling R, Hahn EG, et al. Additive antitumour response to the rabbit Vx2 hepatoma by combined radio frequency ablation and toll like receptor 9 stimulation. Gut (2016) 65(1):134–43. doi: 10.1136/gutjnl-2014-308286
139. Zhang Q, Hossain DM, Duttagupta P, Moreira D, Zhao X, Won H, et al. Serum-resistant cpg-Stat3 decoy for targeting survival and immune checkpoint signaling in acute myeloid leukemia. Blood (2016) 127(13):1687–700. doi: 10.1182/blood-2015-08-665604
140. Zhao X, Zhang Z, Moreira D, Su YL, Won H, Adamus T, et al. B cell lymphoma immunotherapy using Tlr9-targeted oligonucleotide Stat3 inhibitors. Mol therapy: J Am Soc Gene Ther (2018) 26(3):695–707. doi: 10.1016/j.ymthe.2018.01.007
141. Fend L, Gatard-Scheikl T, Kintz J, Gantzer M, Schaedler E, Rittner K, et al. Intravenous injection of mva virus targets Cd8+ lymphocytes to tumors to control tumor growth upon combinatorial treatment with a Tlr9 agonist. Cancer Immunol Res (2014) 2(12):1163–74. doi: 10.1158/2326-6066.Cir-14-0050
142. Humbert M, Guery L, Brighouse D, Lemeille S, Hugues S. Intratumoral cpg-b promotes antitumoral neutrophil, cdc, and T-cell cooperation without reprograming tolerogenic pdc. Cancer Res (2018) 78(12):3280–92. doi: 10.1158/0008-5472.Can-17-2549
143. Babaer D, Amara S, McAdory BS, Johnson O, Myles EL, Zent R, et al. Oligodeoxynucleotides odn 2006 and M362 exert potent adjuvant effect through tlr-9/-6 synergy to exaggerate mammaglobin-a peptide specific cytotoxic Cd8+T lymphocyte responses against breast cancer cells. Cancers (2019) 11(5):672. doi: 10.3390/cancers11050672
144. Kramer K, Shields NJ, Poppe V, Young SL, Walker GF. Intracellular cleavable cpg oligodeoxynucleotide-antigen conjugate enhances anti-tumor immunity. Mol therapy: J Am Soc Gene Ther (2017) 25(1):62–70. doi: 10.1016/j.ymthe.2016.10.001
145. Domingos-Pereira S, Galliverti G, Hanahan D, Nardelli-Haefliger D. Carboplatin/Paclitaxel, E7-vaccination and intravaginal cpg as tri-therapy towards efficient regression of genital Hpv16 tumors. J immunotherapy Cancer (2019) 7(1):122. doi: 10.1186/s40425-019-0593-1
146. Duraiswamy J, Freeman GJ, Coukos G. Therapeutic pd-1 pathway blockade augments with other modalities of immunotherapy T-cell function to prevent immune decline in ovarian cancer. Cancer Res (2013) 73(23):6900–12. doi: 10.1158/0008-5472.Can-13-1550
147. Haabeth OAW, Blake TR, McKinlay CJ, Waymouth RM, Wender PA, Levy R. Mrna vaccination with charge-altering releasable transporters elicits human T cell responses and cures established tumors in mice. Proc Natl Acad Sci U.S.A. (2018) 115(39):E9153–e61. doi: 10.1073/pnas.1810002115
148. Han S, Wang W, Wang S, Wang S, Ju R, Pan Z, et al. Multifunctional biomimetic nanoparticles loading baicalin for polarizing tumor-associated macrophages. Nanoscale (2019) 11:20206–20. doi: 10.1039/c9nr03353j
149. Mochizuki S, Morishita H, Kobiyama K, Aoshi T, Ishii KJ, Sakurai K. Immunization with antigenic peptides complexed with beta-glucan induces potent cytotoxic T-lymphocyte activity in combination with cpg-odns. J Controlled release: Off J Controlled Release Soc (2015) 220(Pt A):495–502. doi: 10.1016/j.jconrel.2015.11.008
150. Yoshizaki Y, Yuba E, Sakaguchi N, Koiwai K, Harada A, Kono K. Ph-sensitive polymer-modified liposome-based immunity-inducing system: Effects of inclusion of cationic lipid and cpg-DNA. Biomaterials (2017) 141:272–83. doi: 10.1016/j.biomaterials.2017.07.001
151. Guo L, Yan DD, Yang D, Li Y, Wang X, Zalewski O, et al. Combinatorial photothermal and immuno cancer therapy using chitosan-coated hollow copper sulfide nanoparticles. ACS Nano (2014) 8(6):5670–81. doi: 10.1021/nn5002112
152. Zhu G, Liu Y, Yang X, Kim YH, Zhang H, Jia R, et al. DNA-Inorganic hybrid nanovaccine for cancer immunotherapy. Nanoscale (2016) 8(12):6684–92. doi: 10.1039/c5nr08821f
153. Lin SI, Huang MH, Chang YW, Chen IH, Roffler S, Chen BM, et al. Chimeric peptide containing both b and T cells epitope of tumor-associated antigen L6 enhances anti-tumor effects in hla-A2 transgenic mice. Cancer Lett (2016) 377(2):126–33. doi: 10.1016/j.canlet.2016.04.031
154. Lu Y, Yang Y, Gu Z, Zhang J, Song H, Xiang G, et al. Glutathione-depletion mesoporous organosilica nanoparticles as a self-adjuvant and Co-delivery platform for enhanced cancer immunotherapy. Biomaterials (2018) 175:82–92. doi: 10.1016/j.biomaterials.2018.05.025
155. Ruiz-de-Angulo A, Zabaleta A, Gomez-Vallejo V, Llop J, Mareque-Rivas JC. Microdosed lipid-coated (67)Ga-magnetite enhances antigen-specific immunity by image tracked delivery of antigen and cpg to lymph nodes. ACS Nano (2016) 10(1):1602–18. doi: 10.1021/acsnano.5b07253
156. Munakata L, Tanimoto Y, Osa A, Meng J, Haseda Y, Naito Y, et al. Lipid nanoparticles of type-a cpg D35 suppress tumor growth by changing tumor immune-microenvironment and activate Cd8 T cells in mice. J Controlled release: Off J Controlled Release Soc (2019) 313:106–19. doi: 10.1016/j.jconrel.2019.09.011
157. Yan S, Rolfe BE, Zhang B, Mohammed YH, Gu W, Xu ZP. Polarized immune responses modulated by layered double hydroxides nanoparticle conjugated with cpg. Biomaterials (2014) 35(35):9508–16. doi: 10.1016/j.biomaterials.2014.07.055
158. Wu C, Guan X, Xu J, Zhang Y, Liu Q, Tian Y, et al. Highly efficient cascading synergy of cancer photo-immunotherapy enabled by engineered graphene quantum Dots/Photosensitizer/Cpg oligonucleotides hybrid nanotheranostics. Biomaterials (2019) 205:106–19. doi: 10.1016/j.biomaterials.2019.03.020
159. Zhong X, Zhang Y, Tan L, Zheng T, Hou Y, Hong X, et al. An aluminum adjuvant-integrated nano-mof as antigen delivery system to induce strong humoral and cellular immune responses. J Controlled release: Off J Controlled Release Soc (2019) 300:81–92. doi: 10.1016/j.jconrel.2019.02.035
160. Kadiyala P, Li D, Nunez FM, Altshuler D, Doherty R, Kuai R, et al. High-density lipoprotein-mimicking nanodiscs for chemo-immunotherapy against glioblastoma multiforme. ACS Nano (2019) 13(2):1365–84. doi: 10.1021/acsnano.8b06842
161. Ahmed KK, Geary SM, Salem AK. Surface engineering tumor cells with adjuvant-loaded particles for use as cancer vaccines. J Controlled release: Off J Controlled Release Soc (2017) 248:1–9. doi: 10.1016/j.jconrel.2016.12.036
162. Zeng Q, Li H, Jiang H, Yu J, Wang Y, Ke H, et al. Tailoring polymeric hybrid micelles with lymph node targeting ability to improve the potency of cancer vaccines. Biomaterials (2017) 122:105–13. doi: 10.1016/j.biomaterials.2017.01.010
163. Xu X, Jin Z, Liu Y, Gong H, Sun Q, Zhang W, et al. Carbohydrate-based adjuvants activate tumor-specific Th1 and Cd8(+) T-cell responses and reduce the immunosuppressive activity of mdscs. Cancer Lett (2019) 440-441:94–105. doi: 10.1016/j.canlet.2018.10.013
164. Kapp K, Volz B, Curran MA, Oswald D, Wittig B, Schmidt M. Enandim - a novel family of l-Nucleotide-Protected Tlr9 agonists for cancer immunotherapy. J immunotherapy Cancer (2019) 7(1):5. doi: 10.1186/s40425-018-0470-3
165. Koo JE, Shin SW, Um SH, Lee JY. X-Shaped DNA potentiates therapeutic efficacy in colitis-associated colon cancer through dual activation of Tlr9 and inflammasomes. Mol Cancer (2015) 14:104. doi: 10.1186/s12943-015-0369-2
166. Volz B, Schmidt M, Heinrich K, Kapp K, Schroff M, Wittig B. Design and characterization of the tumor vaccine Mgn1601, allogeneic fourfold gene-modified vaccine cells combined with a tlr-9 agonist. Mol Ther oncolytics (2016) 3:15023. doi: 10.1038/mto.2015.23
167. Rosalia RA, Cruz LJ, van Duikeren S, Tromp AT, Silva AL, Jiskoot W, et al. Cd40-targeted dendritic cell delivery of plga-nanoparticle vaccines induce potent anti-tumor responses. Biomaterials (2015) 40:88–97. doi: 10.1016/j.biomaterials.2014.10.053
168. Caisova V, Li L, Gupta G, Jochmanova I, Jha A, Uher O, et al. The significant reduction or complete eradication of subcutaneous and metastatic lesions in a pheochromocytoma mouse model after immunotherapy using mannan-bam, tlr ligands, and anti-Cd40. Cancers (2019) 11(5):654. doi: 10.3390/cancers11050654
169. Shojaei H, Oberg HH, Juricke M, Marischen L, Kunz M, Mundhenke C, et al. Toll-like receptors 3 and 7 agonists enhance tumor cell lysis by human gammadelta T cells. Cancer Res (2009) 69(22):8710–7. doi: 10.1158/0008-5472.Can-09-1602
170. Bocanegra Gondan AI, Ruiz-de-Angulo A, Zabaleta A, Gomez Blanco N, Cobaleda-Siles BM, Garcia-Granda MJ, et al. Effective cancer immunotherapy in mice by polyic-imiquimod complexes and engineered magnetic nanoparticles. Biomaterials (2018) 170:95–115. doi: 10.1016/j.biomaterials.2018.04.003
171. Le Noci V, Tortoreto M, Gulino A, Storti C, Bianchi F, Zaffaroni N, et al. Poly(I:C) and cpg-odn combined aerosolization to treat lung metastases and counter the immunosuppressive microenvironment. Oncoimmunology (2015) 4(10):e1040214. doi: 10.1080/2162402x.2015.1040214
172. Le Noci V, Sommariva M, Tortoreto M, Zaffaroni N, Campiglio M, Tagliabue E, et al. Reprogramming the lung microenvironment by inhaled immunotherapy fosters immune destruction of tumor. Oncoimmunology (2016) 5(11):e1234571. doi: 10.1080/2162402x.2016.1234571
173. Silva JM, Zupancic E, Vandermeulen G, Oliveira VG, Salgado A, Videira M, et al. In vivo delivery of peptides and toll-like receptor ligands by mannose-functionalized polymeric nanoparticles induces prophylactic and therapeutic anti-tumor immune responses in a melanoma model. J Controlled release: Off J Controlled Release Soc (2015) 198:91–103. doi: 10.1016/j.jconrel.2014.11.033
174. Zhang L, Dewan V, Yin H. Discovery of small molecules as multi-Toll-Like receptor agonists with proinflammatory and anticancer activities. J medicinal Chem (2017) 60(12):5029–44. doi: 10.1021/acs.jmedchem.7b00419
175. Zheng JH, Nguyen VH, Jiang S-N, Park S-H, Tan W, Hong SH, et al. Two-step enhanced cancer immunotherapy with engineered salmonella typhimurium secreting heterologous flagellin. Sci Trans Med (2017) 9(376):eaak9537. doi: 10.1126/scitranslmed.aak9537
176. Zhu D, Hu C, Fan F, Qin Y, Huang C, Zhang Z, et al. Co-Delivery of antigen and dual agonists by programmed mannose-targeted cationic lipid-hybrid polymersomes for enhanced vaccination. Biomaterials (2019) 206:25–40. doi: 10.1016/j.biomaterials.2019.03.012
177. Zhang L, Wu S, Qin Y, Fan F, Zhang Z, Huang C, et al. Targeted codelivery of an antigen and dual agonists by hybrid nanoparticles for enhanced cancer immunotherapy. Nano Lett (2019) 19(7):4237–49. doi: 10.1021/acs.nanolett.9b00030
178. Zhu M, Ding X, Zhao R, Liu X, Shen H, Cai C, et al. Co-Delivery of tumor antigen and dual toll-like receptor ligands into dendritic cell by silicon microparticle enables efficient immunotherapy against melanoma. J Controlled release: Off J Controlled Release Soc (2018) 272:72–82. doi: 10.1016/j.jconrel.2018.01.004
179. Sainz V, Moura LIF, Peres C, Matos AI, Viana AS, Wagner AM, et al. Alpha-galactosylceramide and peptide-based nano-vaccine synergistically induced a strong tumor suppressive effect in melanoma. Acta biomaterialia (2018) 76:193–207. doi: 10.1016/j.actbio.2018.06.029
180. Kuai R, Sun X, Yuan W, Ochyl LJ, Xu Y, Hassani Najafabadi A, et al. Dual tlr agonist nanodiscs as a strong adjuvant system for vaccines and immunotherapy. J Controlled release: Off J Controlled Release Soc (2018) 282:131–9. doi: 10.1016/j.jconrel.2018.04.041
181. Macedo R, Rochefort J, Guillot-Delost M, Tanaka K, Le Moignic A, Noizat C, et al. Intra-cheek immunization as a novel vaccination route for therapeutic vaccines of head and neck squamous cell carcinomas using plasmo virus-like particles. Oncoimmunology (2016) 5(7):e1164363. doi: 10.1080/2162402x.2016.1164363
182. Sato-Kaneko F, Yao S, Ahmadi A, Zhang SS, Hosoya T, Kaneda MM, et al. Combination immunotherapy with tlr agonists and checkpoint inhibitors suppresses head and neck cancer. JCI Insight (2017) 2(18):e93397. doi: 10.1172/jci.insight.93397
183. Tye H, Kennedy CL, Najdovska M, McLeod L, McCormack W, Hughes N, et al. Stat3-driven upregulation of Tlr2 promotes gastric tumorigenesis independent of tumor inflammation. Cancer Cell (2012) 22(4):466–78. doi: 10.1016/j.ccr.2012.08.010
184. Mohamed FE, Al-Jehani RM, Minogue SS, Andreola F, Winstanley A, Olde Damink SW, et al. Effect of toll-like receptor 7 and 9 targeted therapy to prevent the development of hepatocellular carcinoma. Liver international: Off J Int Assoc Study Liver (2015) 35(3):1063–76. doi: 10.1111/liv.12626
185. Mohamed FEZ, Jalan R, Minogue S, Andreola F, Habtesion A, Hall A, et al. Inhibition of Tlr7 and Tlr9 reduces human cholangiocarcinoma cell proliferation and tumor development. Digestive Dis Sci (2021) 67:1806–21. doi: 10.1007/s10620-021-06973-9
186. An B, Zhu S, Li T, Wu J, Zang G, Lv X, et al. A dual Tlr7/Tlr9 inhibitor Hj901 inhibits abc-dlbcl expressing the Myd88 L265p mutation. Front Cell Dev Biol (2020) 8:262. doi: 10.3389/fcell.2020.00262
187. Speetjens FM, Welters MJP, Slingerland M, de Vos van Steenwijk P, Roozen ICFM, Boekestijn S, et al. Phase I trial to determine safety and immunogenicity of amplivant, a synthetic toll-like receptor 2 ligand, conjugated to two Hpv16 E6 synthetic long peptides. J Clin Oncol (2021) 39(15_suppl):2614. doi: 10.1200/JCO.2021.39.15_suppl.2614
188. Kyi C, Roudko V, Sabado R, Saenger Y, Loging W, Mandeli J, et al. Therapeutic immune modulation against solid cancers with intratumoral poly-iclc: A pilot trial. Clin Cancer research: an Off J Am Assoc Cancer Res (2018) 24(20):4937–48. doi: 10.1158/1078-0432.Ccr-17-1866
189. Awad MM, Govindan R, Spigel DR, Garon EB, Kohler V, Vyasamneni R, et al. Abstract 73: A personal neoantigen vaccine neo-Pv-01 in combination with chemotherapy and pembrolizumab induces broad De novo immune responses in first-line non-squamous nsclc: Associations with clinical outcomes. Cancer Res (2021) 81(13_Supplement):73. doi: 10.1158/1538-7445.Am2021-73
190. Melssen MM, Petroni GR, Chianese-Bullock KA, Wages NA, Grosh WW, Varhegyi N, et al. A multipeptide vaccine plus toll-like receptor agonists lps or polyiclc in combination with incomplete freund’s adjuvant in melanoma patients. J immunotherapy Cancer (2019) 7(1):163. doi: 10.1186/s40425-019-0625-x
191. Ott PA, Hu-Lieskovan S, Chmielowski B, Govindan R, Naing A, Bhardwaj N, et al. A phase ib trial of personalized neoantigen therapy plus anti-Pd-1 in patients with advanced melanoma, non-small cell lung cancer, or bladder cancer. Cell (2020) 183(2):347–62.e24. doi: 10.1016/j.cell.2020.08.053
192. Weed DT, Zilio S, Reis IM, Sargi Z, Abouyared M, Gomez-Fernandez CR, et al. The reversal of immune exclusion mediated by tadalafil and an anti-tumor vaccine also induces Pdl1 upregulation in recurrent head and neck squamous cell carcinoma: Interim analysis of a phase I clinical trial. Front Immunol (2019) 10:1206. doi: 10.3389/fimmu.2019.01206
193. Bhatia S, Miller NJ, Lu H, Longino NV, Ibrani D, Shinohara MM, et al. Intratumoral G100, a Tlr4 agonist, induces antitumor immune responses and tumor regression in patients with merkel cell carcinoma. Clin Cancer research: an Off J Am Assoc Cancer Res (2019) 25(4):1185–95. doi: 10.1158/1078-0432.Ccr-18-0469
194. Seo YD, Zhou J, Morse K, Patino J, Mackay S, Kim EY, et al. Effect of intratumoral (It) injection of the toll-like receptor 4 (Tlr4) agonist G100 on a clinical response and Cd4 T-cell response locally and systemically. J Clin Oncol. (2018) 36:71. doi: 10.1200/JCO.2018.36.5_suppl.71
195. Mahipal A, Ejadi S, Gnjatic S, Kim-Schulze S, Lu H, Ter Meulen JH, et al. First-in-Human phase 1 dose-escalating trial of G305 in patients with advanced solid tumors expressing ny-Eso-1. Cancer immunology immunotherapy: CII (2019) 68(7):1211–22. doi: 10.1007/s00262-019-02331-x
196. Bol KF, Figdor CG, Aarntzen EH, Welzen ME, van Rossum MM, Blokx WA, et al. Intranodal vaccination with mrna-optimized dendritic cells in metastatic melanoma patients. Oncoimmunology (2015) 4(8):e1019197–e. doi: 10.1080/2162402X.2015.1019197
197. van der Linden M, Meeuwis K, van Hees C, van Dorst E, Bulten J, Bosse T, et al. P191 paget trial: Topical 5% imiquimod for vulvar paget disease, first results of clinical efficacy. Int J Gynecologic Cancer (2019) 29(Suppl 4):A170. doi: 10.1136/ijgc-2019-ESGO.248
198. Trutnovsky G, Reich O, Joura EA, Holter M, Ciresa-König A, Widschwendter A, et al. Topical imiquimod versus surgery for vulvar intraepithelial neoplasia: A multicentre, randomised, phase 3, non-inferiority trial. Lancet (London England) (2022) 399(10337):1790–8. doi: 10.1016/s0140-6736(22)00469-x
199. Polterauer S, Reich O, Widschwendter A, Hadjari L, Bogner G, Reinthaller A, et al. Topical imiquimod compared with conization to treat cervical high-grade squamous intraepithelial lesions: Multicenter, randomized controlled trial. Gynecologic Oncol (2022) 165(1):23–9. doi: 10.1016/j.ygyno.2022.01.033
200. Fonseca BO, Possati-Resende JC, Salcedo MP, Schmeler KM, Accorsi GS, Fregnani J, et al. Topical imiquimod for the treatment of high-grade squamous intraepithelial lesions of the cervix: A randomized controlled trial. Obstet Gynecol (2021) 137(6):1043–53. doi: 10.1097/aog.0000000000004384
201. Adams S, Kozhaya L, Martiniuk F, Meng TC, Chiriboga L, Liebes L, et al. Topical Tlr7 agonist imiquimod can induce immune-mediated rejection of skin metastases in patients with breast cancer. Clin Cancer research: an Off J Am Assoc Cancer Res (2012) 18(24):6748–57. doi: 10.1158/1078-0432.Ccr-12-1149
202. Platten M, Bunse L, Wick A, Bunse T, Le Cornet L, Harting I, et al. A vaccine targeting mutant Idh1 in newly diagnosed glioma. Nature (2021) 592(7854):463–8. doi: 10.1038/s41586-021-03363-z
203. Platten M, Schilling D, Bunse L, Wick A, Bunse T, Riehl D, et al. A mutation-specific peptide vaccine targeting Idh1r132h in patients with newly diagnosed malignant astrocytomas: A first-in-Man multicenter phase I clinical trial of the German neurooncology working group (Noa-16). J Clin Oncol (2018) 36(15_suppl):2001. doi: 10.1200/JCO.2018.36.15_suppl.2001
204. Filaci G, Fenoglio D, Nolè F, Zanardi E, Tomasello L, Aglietta M, et al. Telomerase-based Gx301 cancer vaccine in patients with metastatic castration-resistant prostate cancer: A randomized phase ii trial. Cancer immunology immunotherapy: CII (2021) 70(12):3679–92. doi: 10.1007/s00262-021-03024-0
205. Donin NM, Chamie K, Lenis AT, Pantuck AJ, Reddy M, Kivlin D, et al. A phase 2 study of tmx-101, intravesical imiquimod, for the treatment of carcinoma in situ bladder cancer. Urologic Oncol (2017) 35(2):39. doi: 10.1016/j.urolonc.2016.09.006
206. Sabado RL, Pavlick A, Gnjatic S, Cruz CM, Vengco I, Hasan F, et al. Resiquimod as an immunologic adjuvant for ny-Eso-1 protein vaccination in patients with high-risk melanoma. Cancer Immunol Res (2015) 3(3):278–87. doi: 10.1158/2326-6066.Cir-14-0202
207. Monk BJ, Brady MF, Aghajanian C, Lankes HA, Rizack T, Leach J, et al. A phase 2, randomized, double-blind, placebo- controlled study of chemo-immunotherapy combination using motolimod with pegylated liposomal doxorubicin in recurrent or persistent ovarian cancer: A gynecologic oncology group partners study. Ann oncology: Off J Eur Soc Med Oncol (2017) 28(5):996–1004. doi: 10.1093/annonc/mdx049
208. Ferris RL, Saba NF, Gitlitz BJ, Haddad R, Sukari A, Neupane P, et al. Effect of adding motolimod to standard combination chemotherapy and cetuximab treatment of patients with squamous cell carcinoma of the head and neck: The Active8 randomized clinical trial. JAMA Oncol (2018) 4(11):1583–8. doi: 10.1001/jamaoncol.2018.1888
209. Dietsch GN, Lu H, Yang Y, Morishima C, Chow LQ, Disis ML, et al. Coordinated activation of toll-like Receptor8 (Tlr8) and Nlrp3 by the Tlr8 agonist, vtx-2337, ignites tumoricidal natural killer cell activity. PloS One (2016) 11(2):e0148764. doi: 10.1371/journal.pone.0148764
210. Monk BJ, Facciabene A, Brady WE, Aghajanian CA, Fracasso PM, Walker JL, et al. Integrative development of a Tlr8 agonist for ovarian cancer chemoimmunotherapy. Clin Cancer research: an Off J Am Assoc Cancer Res (2017) 23(8):1955–66. doi: 10.1158/1078-0432.Ccr-16-1453
211. Frank MJ, Khodadoust MS, Czerwinski DK, Haabeth OAW, Chu MP, Miklos DB, et al. Autologous tumor cell vaccine induces antitumor T cell immune responses in patients with mantle cell lymphoma: A phase I/Ii trial. J Exp Med (2020) 217(9):e20191712. doi: 10.1084/jem.20191712
212. Brody JD, Ai WZ, Czerwinski DK, Torchia JA, Levy M, Advani RH, et al. In situ vaccination with a Tlr9 agonist induces systemic lymphoma regression: A phase I/Ii study. J Clin Oncol (2010) 28(28):4324–32. doi: 10.1200/jco.2010.28.9793
213. Babiker H, Borazanci E, Subbiah V, Maguire O, Rahimian S, Minderman H, et al. 1195p - safety, efficacy, and immune effects of intratumoral tilsotolimod in patients with refractory solid tumours: Updated results from illuminate-101. Ann Oncol (2019) 30:v487. doi: 10.1093/annonc/mdz253.021
214. Thomas M, Ponce-Aix S, Navarro A, Riera-Knorrenschild J, Schmidt M, Wiegert E, et al. Immunotherapeutic maintenance treatment with toll-like receptor 9 agonist lefitolimod in patients with extensive-stage small-cell lung cancer: Results from the exploratory, controlled, randomized, international phase ii impulse study. Ann oncology: Off J Eur Soc Med Oncol (2018) 29(10):2076–84. doi: 10.1093/annonc/mdy326
215. Ruzsa A, Sen M, Evans M, Lee LW, Hideghety K, Rottey S, et al. Phase 2, open-label, 1:1 randomized controlled trial exploring the efficacy of emd 1201081 in combination with cetuximab in second-line cetuximab-naive patients with recurrent or metastatic squamous cell carcinoma of the head and neck (R/M scchn). Investigational New Drugs (2014) 32(6):1278–84. doi: 10.1007/s10637-014-0117-2
216. Garon E, Spira AI, Johnson M, Bazhenova L, Leach J, Candia A, et al. Abstract Ct224: Phase Ib/Ii, open label, multicenter study of inhaled Dv281, a toll-like receptor 9 agonist, in combination with nivolumab in patients with advanced or metastatic non small cell lung cancer (Nsclc). Cancer Res (2019) 79(13 Supplement):CT224–CT. doi: 10.1158/1538-7445.Am2019-ct224
217. Isambert N, Fumoleau P, Paul C, Ferrand C, Zanetta S, Bauer J, et al. Phase I study of om-174, a lipid a analogue, with assessment of immunological response, in patients with refractory solid tumors. BMC Cancer (2013) 13:172. doi: 10.1186/1471-2407-13-172
218. Helfand AM, Lee CT, Hafez K, Hussain M, Liebert M, Daignault S, et al. Phase ii clinical trial of intravesical bacillus calmette-guerin (Bcg) followed by sunitinib for the treatment of high-risk nonmuscle-invasive bladder cancer (Nmibc). J Clin Oncol (2015) 33(7_suppl):293. doi: 10.1200/jco.2015.33.7_suppl.293
219. Ji N, Mukherjee N, Reyes RM, Gelfond J, Javors M, Meeks JJ, et al. Rapamycin enhances bcg-specific Γδ T cells during intravesical bcg therapy for non-muscle invasive bladder cancer: A randomized, double-blind study. J immunotherapy Cancer (2021) 9(3):e001941. doi: 10.1136/jitc-2020-001941
220. Dhodapkar MV, Sznol M, Zhao B, Wang D, Carvajal RD, Keohan ML, et al. Induction of antigen-specific immunity with a vaccine targeting ny-Eso-1 to the dendritic cell receptor Dec-205. Sci Trans Med (2014) 6(232):232ra51. doi: 10.1126/scitranslmed.3008068
221. Prins RM, Soto H, Konkankit V, Odesa SK, Eskin A, Yong WH, et al. Gene expression profile correlates with T-cell infiltration and relative survival in glioblastoma patients vaccinated with dendritic cell immunotherapy. Clin Cancer Res (2011) 17(6):1603–15. doi: 10.1158/1078-0432.Ccr-10-2563
222. McQuade JL, Homsi J, Torres-Cabala CA, Bassett R, Popuri RM, James ML, et al. A phase ii trial of recombinant mage-A3 protein with immunostimulant As15 in combination with high-dose interleukin-2 (Hdil2) induction therapy in metastatic melanoma. BMC Cancer (2018) 18(1):1274. doi: 10.1186/s12885-018-5193-9
223. Neil E, Eaton A, Lunn S, Nelson K, Greengard E, Moertel C, et al. Ctim-15. first-in-Human phase 1 study of Cd200 activation receptor-ligand (Cd200ar-l) and allogeneic tumor lysate vaccine immunotherapy for recurrent glioblastoma: Initial results from an ongoing clinical trial. Neuro-oncology (2021) 23(Supplement_6):vi52–vi3. doi: 10.1093/neuonc/noab196.207
224. Andreakos E, Sacre SM, Smith C, Lundberg A, Kiriakidis S, Stonehouse T, et al. Distinct pathways of lps-induced nf-kappa b activation and cytokine production in human myeloid and nonmyeloid cells defined by selective utilization of Myd88 and Mal/Tirap. Blood (2004) 103(6):2229–37. doi: 10.1182/blood-2003-04-1356
225. Fitzgerald KA, Rowe DC, Golenbock DT. Endotoxin recognition and signal transduction by the Tlr4/Md2-complex. Microbes Infect (2004) 6(15):1361–7. doi: 10.1016/j.micinf.2004.08.015
226. Carty M, Goodbody R, Schroder M, Stack J, Moynagh PN, Bowie AG. The human adaptor sarm negatively regulates adaptor protein trif-dependent toll-like receptor signaling. Nat Immunol (2006) 7(10):1074–81. doi: 10.1038/ni1382
227. Jenkins KA, Mansell A. Tir-containing adaptors in toll-like receptor signalling. Cytokine (2010) 49(3):237–44. doi: 10.1016/j.cyto.2009.01.009
228. Kawai T, Akira S. Tlr signaling. Semin Immunol (2007) 19(1):24–32. doi: 10.1016/j.smim.2006.12.004
229. Hasan U, Chaffois C, Gaillard C, Saulnier V, Merck E, Tancredi S, et al. Human Tlr10 is a functional receptor, expressed by b cells and plasmacytoid dendritic cells, which activates gene transcription through Myd88. J Immunol (Baltimore Md: 1950) (2005) 174(5):2942–50. doi: 10.4049/jimmunol.174.5.2942
230. Schroder M, Bowie AG. Tlr3 in antiviral immunity: Key player or bystander? Trends Immunol (2005) 26(9):462–8. doi: 10.1016/j.it.2005.07.002
231. Zhao W, Qi J, Wang L, Zhang M, Wang P, Gao C. Ly294002 inhibits Tlr3/4-mediated ifn-B production Via inhibition of Irf3 activation with a Pi3k-independent mechanism. FEBS Lett (2012) 586(6):705–10. doi: 10.1016/j.febslet.2012.01.016
232. Eskan MA, Rose BG, Benakanakere MR, Lee MJ, Kinane DF. Sphingosine 1-phosphate 1 and Tlr4 mediate ifn-beta expression in human gingival epithelial cells. J Immunol (Baltimore Md: 1950) (2008) 180(3):1818–25. doi: 10.4049/jimmunol.180.3.1818
233. Bala S, Petrasek J, Mundkur S, Catalano D, Levin I, Ward J, et al. Circulating micrornas in exosomes indicate hepatocyte injury and inflammation in alcoholic, drug-induced, and inflammatory liver diseases. Hepatol (Baltimore Md) (2012) 56(5):1946–57. doi: 10.1002/hep.25873
234. Galli R, Paone A, Fabbri M, Zanesi N, Calore F, Cascione L, et al. Toll-like receptor 3 (Tlr3) activation induces microrna-dependent reexpression of functional rarbeta and tumor regression. Proc Natl Acad Sci U.S.A. (2013) 110(24):9812–7. doi: 10.1073/pnas.1304610110
235. Luster AD. The role of chemokines in linking innate and adaptive immunity. Curr Opin Immunol (2002) 14(1):129–35. doi: 10.1016/s0952-7915(01)00308-9
236. Sabroe I, Parker LC, Dower SK, Whyte MK. The role of tlr activation in inflammation. J Pathol (2008) 214(2):126–35. doi: 10.1002/path.2264
237. Zarember KA, Godowski PJ. Tissue expression of human toll-like receptors and differential regulation of toll-like receptor mrnas in leukocytes in response to microbes, their products, and cytokines. J Immunol (2002) 168(2):554–61. doi: 10.4049/jimmunol.168.2.554
238. Ridnour LA, Cheng RY, Switzer CH, Heinecke JL, Ambs S, Glynn S, et al. Molecular pathways: Toll-like receptors in the tumor microenvironment–poor prognosis or new therapeutic opportunity. Clin Cancer research: an Off J Am Assoc Cancer Res (2013) 19(6):1340–6. doi: 10.1158/1078-0432.Ccr-12-0408
239. Fellner L, Irschick R, Schanda K, Reindl M, Klimaschewski L, Poewe W, et al. Toll-like receptor 4 is required for alpha-synuclein dependent activation of microglia and astroglia. Glia (2013) 61(3):349–60. doi: 10.1002/glia.22437
240. Ayari C, Bergeron A, LaRue H, Menard C, Fradet Y. Toll-like receptors in normal and malignant human bladders. J Urol (2011) 185(5):1915–21. doi: 10.1016/j.juro.2010.12.097
241. Kadowaki N, Ho S, Antonenko S, Malefyt RD, Kastelein RA, Bazan F, et al. Subsets of human dendritic cell precursors express different toll-like receptors and respond to different microbial antigens. J Exp Med (2001) 194(6):863–9. doi: 10.1084/jem.194.6.863
242. Fujita Y, Mihara T, Okazaki T, Shitanaka M, Kushino R, Ikeda C, et al. Toll-like receptors (Tlr) 2 and 4 on human sperm recognize bacterial endotoxins and mediate apoptosis. Hum Reprod (Oxford England) (2011) 26(10):2799–806. doi: 10.1093/humrep/der234
243. Chen X, Cheng F, Liu Y, Zhang L, Song L, Cai X, et al. Toll-like receptor 2 and toll-like receptor 4 exhibit distinct regulation of cancer cell stemness mediated by cell death-induced high-mobility group box 1. EBioMedicine (2019) 40:135–50. doi: 10.1016/j.ebiom.2018.12.016
244. Yu L, Chen S. Toll-like receptors expressed in tumor cells: Targets for therapy. Cancer immunology immunotherapy: CII (2008) 57(9):1271–8. doi: 10.1007/s00262-008-0459-8
245. Jouhi L, Renkonen S, Atula T, Makitie A, Haglund C, Hagstrom J. Different toll-like receptor expression patterns in progression toward cancer. Front Immunol (2014) 5:638. doi: 10.3389/fimmu.2014.00638
246. Duthie MS, Windish HP, Fox CB, Reed SG. Use of defined tlr ligands as adjuvants within human vaccines. Immunol Rev (2011) 239(1):178–96. doi: 10.1111/j.1600-065X.2010.00978.x
247. Sobek V, Birkner N, Falk I, Wurch A, Kirschning CJ, Wagner H, et al. Direct toll-like receptor 2 mediated Co-stimulation of T cells in the mouse system as a basis for chronic inflammatory joint disease. Arthritis Res Ther (2004) 6(5):R433–46. doi: 10.1186/ar1212
248. Ganley-Leal LM, Liu X, Wetzler LM. Toll-like receptor 2-mediated human b cell differentiation. Clin Immunol (2006) 120(3):272–84. doi: 10.1016/j.clim.2006.04.571
249. Mansson A, Adner M, Hockerfelt U, Cardell LO. A distinct toll-like receptor repertoire in human tonsillar b cells, directly activated by pamcsk, r-837 and cpg-2006 stimulation. Immunology (2006) 118(4):539–48. doi: 10.1111/j.1365-2567.2006.02392.x
250. Wang J, Shao Y, Bennett TA, Shankar RA, Wightman PD, Reddy LG. The functional effects of physical interactions among toll-like receptors 7, 8, and 9. J Biol Chem (2006) 281(49):37427–34. doi: 10.1074/jbc.M605311200
251. Fukui R, Saitoh S, Matsumoto F, Kozuka-Hata H, Oyama M, Tabeta K, et al. Unc93b1 biases toll-like receptor responses to nucleic acid in dendritic cells toward DNA- but against rna-sensing. J Exp Med (2009) 206(6):1339–50. doi: 10.1084/jem.20082316
252. Urban-Wojciuk Z, Khan MM, Oyler BL, Fåhraeus R, Marek-Trzonkowska N, Nita-Lazar A, et al. The role of tlrs in anti-cancer immunity and tumor rejection. Front Immunol (2019) 10:2388. doi: 10.3389/fimmu.2019.02388
253. Lowe EL, Crother TR, Rabizadeh S, Hu B, Wang H, Chen S, et al. Toll-like receptor 2 signaling protects mice from tumor development in a mouse model of colitis-induced cancer. PloS One (2010) 5(9):e13027. doi: 10.1371/journal.pone.0013027
254. Asprodites N, Zheng L, Geng D, Velasco-Gonzalez C, Sanchez-Perez L, Davila E. Engagement of toll-like receptor-2 on cytotoxic T-lymphocytes occurs in vivo and augments antitumor activity. FASEB journal: Off Publ Fed Am Societies Exp Biol (2008) 22(10):3628–37. doi: 10.1096/fj.08-108274
255. Li S, Sun R, Chen Y, Wei H, Tian Z. Tlr2 limits development of hepatocellular carcinoma by reducing Il18-mediated immunosuppression. Cancer Res (2015) 75(6):986–95. doi: 10.1158/0008-5472.CAN-14-2371
256. Nelde A, Maringer Y, Bilich T, Salih HR, Roerden M, Heitmann JS, et al. Immunopeptidomics-guided warehouse design for peptide-based immunotherapy in chronic lymphocytic leukemia. Front Immunol (2021) 12:705974. doi: 10.3389/fimmu.2021.705974
257. Weng J, Lai P, Qin L, Lai Y, Jiang Z, Luo C, et al. A novel generation 1928zt2 car T cells induce remission in extramedullary relapse of acute lymphoblastic leukemia. J Hematol Oncol (2018) 11(1):25. doi: 10.1186/s13045-018-0572-x
258. Matsumoto M, Takeda Y, Tatematsu M, Seya T. Toll-like receptor 3 signal in dendritic cells benefits cancer immunotherapy. Front Immunol (2017) 8:1897. doi: 10.3389/fimmu.2017.01897
259. Takemura R, Takaki H, Okada S, Shime H, Akazawa T, Oshiumi H, et al. Polyi:C-induced, Tlr3/Rip3-dependent necroptosis backs up immune effector-mediated tumor elimination in vivo. Cancer Immunol Res (2015) 3(8):902–14. doi: 10.1158/2326-6066.Cir-14-0219
260. Fan L, Zhou P, Hong Q, Chen AX, Liu GY, Yu KD, et al. Toll-like receptor 3 acts as a suppressor gene in breast cancer initiation and progression: A two-stage association study and functional investigation. Oncoimmunology (2019) 8(6):e1593801. doi: 10.1080/2162402x.2019.1593801
261. Yoneda K, Sugimoto K, Shiraki K, Tanaka J, Beppu T, Fuke H, et al. Dual topology of functional toll-like receptor 3 expression in human hepatocellular carcinoma: Differential signaling mechanisms of Tlr3-induced nf-kappab activation and apoptosis. Int J Oncol (2008) 33(5):929–36. doi: 10.3892/ijo_00000080
262. Gambara G, Desideri M, Stoppacciaro A, Padula F, De Cesaris P, Starace D, et al. Tlr3 engagement induces irf-3-Dependent apoptosis in androgen-sensitive prostate cancer cells and inhibits tumour growth in vivo. J Cell Mol Med (2015) 19(2):327–39. doi: 10.1111/jcmm.12379
263. Bergé M, Bonnin P, Sulpice E, Vilar J, Allanic D, Silvestre JS, et al. Small interfering rnas induce target-independent inhibition of tumor growth and vasculature remodeling in a mouse model of hepatocellular carcinoma. Am J Pathol (2010) 177(6):3192–201. doi: 10.2353/ajpath.2010.100157
264. Yoshida S, Shime H, Takeda Y, Nam JM, Takashima K, Matsumoto M, et al. Toll-like receptor 3 signal augments radiation-induced tumor growth retardation in a murine model. Cancer Sci (2018) 109(4):956–65. doi: 10.1111/cas.13543
265. Griffiths EA, Srivastava P, Matsuzaki J, Brumberger Z, Wang ES, Kocent J, et al. Ny-Eso-1 vaccination in combination with decitabine induces antigen-specific T-lymphocyte responses in patients with myelodysplastic syndrome. Clin Cancer research: an Off J Am Assoc Cancer Res (2018) 24(5):1019–29. doi: 10.1158/1078-0432.Ccr-17-1792
266. Dillon PM, Petroni GR, Smolkin ME, Brenin DR, Chianese-Bullock KA, Smith KT, et al. A pilot study of the immunogenicity of a 9-peptide breast cancer vaccine plus poly-iclc in early stage breast cancer. J immunotherapy Cancer (2017) 5(1):92. doi: 10.1186/s40425-017-0295-5
267. Apetoh L, Ghiringhelli F, Tesniere A, Obeid M, Ortiz C, Criollo A, et al. Toll-like receptor 4-dependent contribution of the immune system to anticancer chemotherapy and radiotherapy. Nat Med (2007) 13(9):1050–9. doi: 10.1038/nm1622
268. Naseemuddin M, Iqbal A, Nasti TH, Ghandhi JL, Kapadia AD, Yusuf N. Cell mediated immune responses through Tlr4 prevents dmba-induced mammary carcinogenesis in mice. Int J Cancer (2012) 130(4):765–74. doi: 10.1002/ijc.26100
269. Okamoto M, Oshikawa T, Tano T, Ohe G, Furuichi S, Nishikawa H, et al. Involvement of toll-like receptor 4 signaling in interferon-gamma production and antitumor effect by streptococcal agent ok-432. J Natl Cancer Inst (2003) 95(4):316–26. doi: 10.1093/jnci/95.4.316
270. Yusuf N, Nasti TH, Long JA, Naseemuddin M, Lucas AP, Xu H, et al. Protective role of toll-like receptor 4 during the initiation stage of cutaneous chemical carcinogenesis. Cancer Res (2008) 68(2):615–22. doi: 10.1158/0008-5472.CAN-07-5219
271. D’Agostini C, Pica F, Febbraro G, Grelli S, Chiavaroli C, Garaci E. Antitumour effect of om-174 and cyclophosphamide on murine B16 melanoma in different experimental conditions. Int Immunopharmacol (2005) 5(7-8):1205–12. doi: 10.1016/j.intimp.2005.02.013
272. Hussain S, Johnson CG, Sciurba J, Meng X, Stober VP, Liu C, et al. Tlr5 participates in the Tlr4 receptor complex and promotes Myd88-dependent signaling in environmental lung injury. Elife (2020) 9:e50458. doi: 10.7554/eLife.50458
273. Faber E, Tedin K, Speidel Y, Brinkmann MM, Josenhans C. Functional expression of Tlr5 of different vertebrate species and diversification in intestinal pathogen recognition. Sci Rep (2018) 8(1):11287. doi: 10.1038/s41598-018-29371-0
274. Jiang W, Han Y, Liang T, Zhang C, Gao F, Hou G. Down-regulation of toll-like receptor 5 (Tlr5) increased vegfr expression in triple negative breast cancer (Tnbc) based on radionuclide imaging. Front Oncol (2021) 11:708047. doi: 10.3389/fonc.2021.708047
275. Shi D, Liu W, Zhao S, Zhang C, Liang T, Hou G. Tlr5 is a new reporter for triple-negative breast cancer indicated by radioimmunoimaging and fluorescent staining. J Cell Mol Med (2019) 23(12):8305–13. doi: 10.1111/jcmm.14707
276. Mett V, Komarova EA, Greene K, Bespalov I, Brackett C, Gillard B, et al. Mobilan: A recombinant adenovirus carrying toll-like receptor 5 self-activating cassette for cancer immunotherapy. Oncogene (2018) 37(4):439–49. doi: 10.1038/onc.2017.346
277. Mett V, Kurnasov OV, Bespalov IA, Molodtsov I, Brackett CM, Burdelya LG, et al. A deimmunized and pharmacologically optimized toll-like receptor 5 agonist for therapeutic applications. Commun Biol (2021) 4(1):466–. doi: 10.1038/s42003-021-01978-6
278. Eremina NV, Kazey VI, Mishugin SV, Leonenkov RV, Pushkar DY, Mett VL, et al. First-in-Human study of anticancer immunotherapy drug candidate mobilan: Safety, pharmacokinetics and pharmacodynamics in prostate cancer patients. Oncotarget (2020) 11(14):1273–88. doi: 10.18632/oncotarget.27549
279. McGowan DC. Latest advances in small molecule tlr 7/8 agonist drug research. Curr Top Med Chem (2019) 19(24):2228–38. doi: 10.2174/1568026619666191009165418
280. Spaner DE, Shi Y, White D, Mena J, Hammond C, Tomic J, et al. Immunomodulatory effects of toll-like receptor-7 activation on chronic lymphocytic leukemia cells. Leukemia (2006) 20(2):286–95. doi: 10.1038/sj.leu.2404061
281. Clark RA, Huang SJ, Murphy GF, Mollet IG, Hijnen D, Muthukuru M, et al. Human squamous cell carcinomas evade the immune response by down-regulation of vascular e-selectin and recruitment of regulatory T cells. J Exp Med (2008) 205(10):2221–34. doi: 10.1084/jem.20071190
282. Michaelis KA, Norgard MA, Zhu X, Levasseur PR, Sivagnanam S, Liudahl SM, et al. The Tlr7/8 agonist R848 remodels tumor and host responses to promote survival in pancreatic cancer. Nat Commun (2019) 10(1):4682. doi: 10.1038/s41467-019-12657-w
283. Ye J, Ma C, Hsueh EC, Dou J, Mo W, Liu S, et al. Tlr8 signaling enhances tumor immunity by preventing tumor-induced T-cell senescence. EMBO Mol Med (2014) 6(10):1294–311. doi: 10.15252/emmm.201403918
284. Park IU, Introcaso C, Dunne EF. Human papillomavirus and genital warts: A review of the evidence for the 2015 centers for disease control and prevention sexually transmitted diseases treatment guidelines. Clin Infect diseases: an Off Publ Infect Dis Soc America (2015) 61 Suppl 8:S849–55. doi: 10.1093/cid/civ813
285. Peris K, Fargnoli MC, Garbe C, Kaufmann R, Bastholt L, Seguin NB, et al. Diagnosis and treatment of basal cell carcinoma: European consensus-based interdisciplinary guidelines. Eur J Cancer (Oxford England: 1990) (2019) 118:10–34. doi: 10.1016/j.ejca.2019.06.003
286. Patel GK, Goodwin R, Chawla M, Laidler P, Price PE, Finlay AY, et al. Imiquimod 5% cream monotherapy for cutaneous squamous cell carcinoma in situ (Bowen’s disease): A randomized, double-blind, placebo-controlled trial. J Am Acad Dermatol (2006) 54(6):1025–32. doi: 10.1016/j.jaad.2006.01.055
287. Chow LQM, Morishima C, Eaton KD, Baik CS, Goulart BH, Anderson LN, et al. Phase ib trial of the toll-like receptor 8 agonist, motolimod (Vtx-2337), combined with cetuximab in patients with recurrent or metastatic scchn. Clin Cancer research: an Off J Am Assoc Cancer Res (2017) 23(10):2442–50. doi: 10.1158/1078-0432.Ccr-16-1934
288. Dummer R, Hauschild A, Becker JC, Grob JJ, Schadendorf D, Tebbs V, et al. An exploratory study of systemic administration of the toll-like receptor-7 agonist 852a in patients with refractory metastatic melanoma. Clin Cancer research: an Off J Am Assoc Cancer Res (2008) 14(3):856–64. doi: 10.1158/1078-0432.Ccr-07-1938
289. Geller MA, Cooley S, Argenta PA, Downs LS, Carson LF, Judson PL, et al. Toll-like receptor-7 agonist administered subcutaneously in a prolonged dosing schedule in heavily pretreated recurrent breast, ovarian, and cervix cancers. Cancer immunology immunotherapy: CII (2010) 59(12):1877–84. doi: 10.1007/s00262-010-0914-1
290. Weigel BJ, Cooley S, DeFor T, Weisdorf DJ, Panoskaltsis-Mortari A, Chen W, et al. Prolonged subcutaneous administration of 852a, a novel systemic toll-like receptor 7 agonist, to activate innate immune responses in patients with advanced hematologic malignancies. Am J Hematol (2012) 87(10):953–6. doi: 10.1002/ajh.23280
291. Dudek AZ, Yunis C, Harrison LI, Kumar S, Hawkinson R, Cooley S, et al. First in human phase I trial of 852a, a novel systemic toll-like receptor 7 agonist, to activate innate immune responses in patients with advanced cancer. Clin Cancer research: an Off J Am Assoc Cancer Res (2007) 13(23):7119–25. doi: 10.1158/1078-0432.Ccr-07-1443
292. Frega G, Wu Q, Le Naour J, Vacchelli E, Galluzzi L, Kroemer G, et al. Trial watch: Experimental Tlr7/Tlr8 agonists for oncological indications. Oncoimmunology (2020) 9(1):1796002. doi: 10.1080/2162402x.2020.1796002
293. Kumagai Y, Takeuchi O, Akira S. Tlr9 as a key receptor for the recognition of DNA. Advanced Drug delivery Rev (2008) 60(7):795–804. doi: 10.1016/j.addr.2007.12.004
294. Kang TH, Mao CP, Kim YS, Kim TW, Yang A, Lam B, et al. Tlr9 acts as a sensor for tumor-released DNA to modulate anti-tumor immunity after chemotherapy. J immunotherapy Cancer (2019) 7(1):260. doi: 10.1186/s40425-019-0738-2
295. Krieg AM. Toll-like receptor 9 (Tlr9) agonists in the treatment of cancer. Oncogene (2008) 27(2):161–7. doi: 10.1038/sj.onc.1210911
296. Kayraklioglu N, Horuluoglu B, Klinman DM. Cpg oligonucleotides as vaccine adjuvants. Methods Mol Biol (2021) 2197:51–85. doi: 10.1007/978-1-0716-0872-2_4
297. Leonard JP, Link BK, Emmanouilides C, Gregory SA, Weisdorf D, Andrey J, et al. Phase I trial of toll-like receptor 9 agonist pf-3512676 with and following rituximab in patients with recurrent indolent and aggressive non hodgkin’s lymphoma. Clin Cancer research: an Off J Am Assoc Cancer Res (2007) 13(20):6168–74. doi: 10.1158/1078-0432.Ccr-07-0815
298. van Puffelen JH, Keating ST, Oosterwijk E, van der Heijden AG, Netea MG, Joosten LAB, et al. Trained immunity as a molecular mechanism for bcg immunotherapy in bladder cancer. Nat Rev Urol (2020) 17(9):513–25. doi: 10.1038/s41585-020-0346-4
299. Theodoraki MN, Yerneni S, Sarkar SN, Orr B, Muthuswamy R, Voyten J, et al. Helicase-driven activation of nfκb-Cox2 pathway mediates the immunosuppressive component of dsrna-driven inflammation in the human tumor microenvironment. Cancer Res (2018) 78(15):4292–302. doi: 10.1158/0008-5472.Can-17-3985
300. Feng Y, Chen Y, Meng Y, Cao Q, Liu Q, Ling C, et al. Bufalin suppresses migration and invasion of hepatocellular carcinoma cells elicited by poly (I:C) therapy. Oncoimmunology (2018) 7(5):e1426434. doi: 10.1080/2162402x.2018.1426434
301. Galluzzi L, Vacchelli E, Eggermont A, Fridman WH, Galon J, Sautès-Fridman C, et al. Trial watch. Oncoimmunology (2012) 1(5):699–739. doi: 10.4161/onci.20696
302. Tran TH, Tran TTP, Truong DH, Nguyen HT, Pham TT, Yong CS, et al. Toll-like receptor-targeted particles: A paradigm to manipulate the tumor microenvironment for cancer immunotherapy. Acta biomaterialia (2019) 94:82–96. doi: 10.1016/j.actbio.2019.05.043
303. Kondo T, Kawai T, Akira S. Dissecting negative regulation of toll-like receptor signaling. Trends Immunol (2012) 33(9):449–58. doi: 10.1016/j.it.2012.05.002
304. Bhagchandani S, Johnson JA, Irvine DJ. Evolution of toll-like receptor 7/8 agonist therapeutics and their delivery approaches: From antiviral formulations to vaccine adjuvants. Advanced Drug delivery Rev (2021) 175:113803. doi: 10.1016/j.addr.2021.05.013
305. Bagchi A, Herrup EA, Warren HS, Trigilio J, Shin HS, Valentine C, et al. Myd88-dependent and Myd88-independent pathways in synergy, priming, and tolerance between tlr agonists. J Immunol (Baltimore Md: 1950) (2007) 178(2):1164–71. doi: 10.4049/jimmunol.178.2.1164
306. Michaelis KA, Norgard MA, Levasseur PR, Olson B, Burfeind KG, Buenafe AC, et al. Persistent toll-like receptor 7 stimulation induces behavioral and molecular innate immune tolerance. Brain behavior Immun (2019) 82:338–53. doi: 10.1016/j.bbi.2019.09.004
307. Hayashi T, Gray CS, Chan M, Tawatao RI, Ronacher L, McGargill MA, et al. Prevention of autoimmune disease by induction of tolerance to toll-like receptor 7. Proc Natl Acad Sci U.S.A. (2009) 106(8):2764–9. doi: 10.1073/pnas.0813037106
308. Butcher SK, O’Carroll CE, Wells CA, Carmody RJ. Toll-like receptors drive specific patterns of tolerance and training on restimulation of macrophages. Front Immunol (2018) 9:933(933). doi: 10.3389/fimmu.2018.00933
309. Nahid MA, Satoh M, Chan EK. Microrna in tlr signaling and endotoxin tolerance. Cell Mol Immunol (2011) 8(5):388–403. doi: 10.1038/cmi.2011.26
310. Bourquin C, Hotz C, Noerenberg D, Voelkl A, Heidegger S, Roetzer LC, et al. Systemic cancer therapy with a small molecule agonist of toll-like receptor 7 can be improved by circumventing tlr tolerance. Cancer Res (2011) 71(15):5123. doi: 10.1158/0008-5472.CAN-10-3903
311. Tsitoura D, Ambery C, Price M, Powley W, Garthside S, Biggadike K, et al. Early clinical evaluation of the intranasal Tlr7 agonist Gsk2245035: Use of translational biomarkers to guide dosing and confirm target engagement. Clin Pharmacol Ther (2015) 98(4):369–80. doi: 10.1002/cpt.157
312. Vanpouille-Box C, Hoffmann JA, Galluzzi L. Pharmacological modulation of nucleic acid sensors - therapeutic potential and persisting obstacles. Nat Rev Drug Discovery (2019) 18:845–67. doi: 10.1038/s41573-019-0043-2
Keywords: toll-like receptors, cancer, targeted therapy, immunotherapy, clinical trials
Citation: Yang Y, Li H, Fotopoulou C, Cunnea P and Zhao X (2022) Toll-like receptor-targeted anti-tumor therapies: Advances and challenges. Front. Immunol. 13:1049340. doi: 10.3389/fimmu.2022.1049340
Received: 20 September 2022; Accepted: 31 October 2022;
Published: 21 November 2022.
Edited by:
Laura Schuettpelz, Washington University in St. Louis, United StatesReviewed by:
Aleksandra Nita-Lazar, National Institute of Allergy and Infectious Diseases (NIH), United StatesCopyright © 2022 Yang, Li, Fotopoulou, Cunnea and Zhao. This is an open-access article distributed under the terms of the Creative Commons Attribution License (CC BY). The use, distribution or reproduction in other forums is permitted, provided the original author(s) and the copyright owner(s) are credited and that the original publication in this journal is cited, in accordance with accepted academic practice. No use, distribution or reproduction is permitted which does not comply with these terms.
*Correspondence: Xia Zhao, eGlhLXpoYW9AMTI2LmNvbQ==
†These authors have contributed equally to this work
‡ORCID: Christina Fotopoulou, orcid.org/0000-0001-6375-9645
Paula Cunnea2, orcid.org/0000-0002-3699-4911
Disclaimer: All claims expressed in this article are solely those of the authors and do not necessarily represent those of their affiliated organizations, or those of the publisher, the editors and the reviewers. Any product that may be evaluated in this article or claim that may be made by its manufacturer is not guaranteed or endorsed by the publisher.
Research integrity at Frontiers
Learn more about the work of our research integrity team to safeguard the quality of each article we publish.