- 1Scientific Research Centre, The Seventh Affiliated Hospital, Sun Yat-sen University, Shenzhen, China
- 2School of Medicine, Sun Yat-sen University, Shenzhen, China
- 3School of Medicine, Johns Hopkins University, Baltimore, MD, United States
Immune cells are capable of influencing tumor progression in the tumor microenvironment (TME). Meanwhile, one mechanism by which tumor modulate immune cells function is through extracellular vesicles (EVs), which are cell-derived extracellular membrane vesicles. EVs can act as mediators of intercellular communication and can deliver nucleic acids, proteins, lipids, and other signaling molecules between cells. In recent years, studies have found that EVs play a crucial role in the communication between tumor cells and immune cells. Innate immunity is the first-line response of the immune system against tumor progression. Therefore, tumor cell-derived EVs (TDEVs) which modulate the functional change of innate immune cells serve important functions in the context of tumor progression. Emerging evidence has shown that TDEVs dually enhance or suppress innate immunity through various pathways. This review aims to summarize the influence of TDEVs on macrophages, dendritic cells, neutrophils, and natural killer cells. We also summarize their further effects on the progression of tumors, which may provide new ideas for developing novel tumor therapies targeting EVs.
1 Introduction
Extracellular vesicles (EVs) usually refer to a group of heterogeneous, cell-released, double-membraned 150-1000 nm structure vesicles (1, 2). To address the considerable discrepancy in the definitions of EV subtypes, the International Society for Extracellular Vesicles (ISEV) suggested using “small EVs” (sEVs) for EVs under 200nm and “medium/large EVs” (m/lEVs) for EVs larger than 200nm in the position paper published in 2018 (1). In this article, the term “EVs” represents all cell-derived extracellular membrane vesicles (though most studies investigate sEVs).
The biogenesis of EVs has been well characterized (3). It is known that EVs originate from endosomes that are divided into 3 parts: early endosomes, late endosomes, and recycling endosomes. Invaginated vesicles first form intraluminal vesicles and integrate their cargoes into early endosomes. This cargo can consist of proteins, nucleic acids, and lipids, etc. Endosomes that internalize cargo to be recycled are classified as “recycling endosomes”. The rest of the early endosomes are subsequently transformed into late endosomes (4), also called multi-vesicular bodies (MVBs) (5), while dividing their cargo into vesicles budding in the lumen of the late endosomes. Four multi-protein complexes, endosomal sorting complexes required for transport (ESCRT) 0, I, II, and III, are involved in this step (6–8). ESCRT-0 has an association with cargo gathering in a ubiquitin-dependent process. The recruitment of ESCRT-I and II promotes endosomal membrane budding and ESCRT III is essential for completing budding. If the cargoes are destined to be degraded, the late endosomes will fuse with lysosomes and their cargoes will be digested. Other late endosomes carrying contents that are fated to be exported fuse with the plasma membrane and their internal vesicles are exported as EVs. Some ESCRT-independent mechanisms of EV biogenesis have also been reported, such as the biogenesis mechanism related to ceramide signaling pathways (9, 10).
Loaded with specific proteins, nucleic acids, and lipids, EVs play an important role in intercellular communication. In the 1990s, Raposo et al. reported that highly enriched MHC II molecules from Epstein-Barre Virus (EBV)-transformed B cell-derived EVs provoked certain CD4+ T cell clones, demonstrating EVs to be the critical mediator of intercellular communication (11). The binding specificity of EVs and recipient cells may be endowed by ligands expressed on their membranes. Thus, EVs can be used as potential targets of biomarker testing and transporters of drugs and genes.
Numerous recent studies have found that EVs are closely related to cancer progression (12–14). Tumor-derived EVs (TDEVs) take part in the formation of the tumor microenvironment (TME), epithelial-mesenchymal transition (EMT), angiogenesis, and metastasis. EMT and angiogenesis are both crucial processes for tumor metastasis and are regulated by EVs. For example, by interacting with endothelial cells TDEVs display a capacity for promoting angiogenesis by transporting activated epidermal growth factor receptor (EGFR) (15). Moreover, EVs facilitate tumor metastasis by mediating extracellular matrix (ECM) remodeling. Matrix metalloproteinase (MMP) is the major performing protein in tissue remodeling (16) and EVs derived from glioblastoma tumors have been found to increase the expression of MMP14 RNA in microglia (17). It has been found that macrophage migration inhibitory factor (MIF) existing in EVs derived from pancreatic ductal adenocarcinoma (PDAC) formed a pre-metastatic niche in the liver thus advancing liver metastasis (18). In addition, TDEVs can deliver their cargo to cancer cells, immune cells, and stromal cells. TDEVs exert a dual effect on tumor development by inhibiting natural killer (NK) cytotoxicity, mediating neutrophil differentiation, and influencing dendritic cell function. In many cases, TDEVs stimulate the pro-inflammatory M2 phenotype differentiation of macrophages and create an immunosuppressive microenvironment in tumor tissue. PD-L1 packed in TDEVs prevents T cell activation and stops tumor cells from being identified and killed (19, 20). These studies all provide evidence that TDEVs are engaged in many processes of tumor progression that affect immune cell functions.
Innate immune cells can be divided into classical innate immune cells, innate lymphoid cells (ILCs), and innate-like lymphocytes (ILLs). Classical innate immune cells include monocytes, macrophages, conventional dendritic cells, granulocytes, and mast cells. Here we review the studies focusing on the modulatory functions of TDEVs on four kinds of innate immune cells: macrophages, dendritic cells, neutrophils of granulocytes, and natural killer (NK) of ILCs.
Macrophages are differentiated from monocytes that migrate to tissues and organs under the influence of chemokines such as monocyte chemoattractant protein 1 (MCP-1). Induced by pathogens or different types of cytokines in the local microenvironment, monocytes differentiate into two macrophage subsets with different functional properties: type-1 macrophage (M1, classically activated macrophage) and type-2 macrophage (M2, alternatively activated macrophage). Compared with M1, M2 commonly accounts for a larger proportion of TME in solid tumors and results in tumor immune escape (21). Macrophages perform a variety of important functions including: phagocytosis and sterilization, inflammatory reaction, antigen-presenting, and immune regulation. Thus, macrophages are fundamental for the development and progression of tumors.
Dendritic cells (DCs) are functionally sorted as conventional DCs (cDCs), plasmacytoid DCs (pDCs), and monocyte-derived inflammatory DCs (moDCs) while the last type only appears during inflammation (22). cDCs generally participate in immunity as antigen-presenting cells (APC), acting as a bridge between adaptive and innate immune systems. They are essential for the induction and maintenance of anti-tumor immunity. In TME the antigen-presenting function is impaired (23). Depending on distinct environmental signals, tumor-infiltrating dendritic cells (TIDC) display either anti-tumor or pro-tumor functions. In most cases, TIDCs exhibit a tolerogenic phenotype under the impact of immune-suppressive factors like vascular endothelial growth factor (VEGF), IL10, TGFβ, and prostaglandin E2 (PGE2), subduing Th1-activating ability while enhancing Th2 and Treg responding (24). As a special lineage of DCs, pDCs are poor in antigen presentation and strong in IFN production. They contribute to tumor growth probably by activating Treg and forming an immune-subversive environment (25).
Neutrophils account for 70-80% of peripheral granulocytes. They possess a high generation rate of 1×107 per minute but are short-lived (about 2-3 days in circulation). Within the cancer framework, neutrophils show N1 and N2 phenotypes, respectively acting as tumor suppressors and tumor promoters (22). Neutrophils are pro-inflammatory in the early stages of the tumor, but gradually display the immunosuppressive phenotype as the tumor progresses (26). They produce reactive oxygen/nitrogen species (ROS/RNS) to regulate inflammation, secret neutrophil elastase (NE) and MMP8/9 to accelerate invasion, release Oncostatin-M to encourage angiogenesis, and make PGE2 to promote tumor development (27).
NK cells are a subset of ILCs. There is a plethora of evidence indicating that NK receptor NKG2D assists the immune system in recognizing tumors. NK cells kill target cells and restrain primary tumor progression through various pathways including: antibody-dependent cell-mediated cytotoxicity (ADCC), Fas/FasL pathway, perforin/granzyme pathway, and release of cytokines such as TNF (28). Nevertheless, the killing efficiency of NK is limited due to the presence of TGF-β in plasma of both solid and hematological tumors (29–31). TGF-β can reduce the expression of activating receptors (including NKG2D, NKp30 and NKp46) and upregulate the expression of the inhibitory receptor NKG2A (29–31).
In the following sections we discuss the diverse impacts of TDEVs on innate immune cells, which including macrophages, dendritic cells, neutrophils, and NK cells. We further summarize the effects of these regulated immune cells on tumor progression. We will focus on the cargoes of TDEVs which modulate innate immunity is the promising novel targets for tumor diagnosis and treatment.
2 Macrophage
Macrophages have multiple functions, including phagocytosis and sterilization, and participation in inflammatory reactions, presenting antigens, and immune regulation (32). They recognize antigenic foreign bodies through surface pattern recognition receptors (PRR) and opsonic receptors, swallowing pathogens into the cell through receptor-mediated endocytosis. Sterilization is conducted by reactive oxygen intermediate (ROI) and reactive nitrogen intermediate (RNI), or directly by lysosomes and accumulated lactic acid. IgG Fc receptors on the surface of activated macrophages mediate ADCC to kill tumor cells or virus-infected cells (33). Activated macrophages also synthesize and secret chemokines and cytokines to participate in the inflammatory response. M1 and M2 macrophages have distinct secretion profiles (34). M1 macrophages tend to release chemokines (such as CCL2, CCL3, and CXCL8) and pro-inflammatory cytokines (such as IL-6, TNF-α, and IL-1β (35–37), while M2 macrophages produce anti-inflammatory factors like IL-10 and TGF-β more (34, 38). Peripheral organ-resident macrophages swallow pathogens, process them into small immunogenic peptides, present peptide fragments to the cell surface for recognition by CD4+ Th cells and trigger adaptive immune response (39, 40). Macrophages play a dual role in tumor immunity (41). On the one hand, macrophages induce specific anti-tumor responses by presenting antigens as professional APCs. Activated macrophages kill tumor cells through non-specific phagocytosis or ADCC, and also indirectly by secreting TNF, NO, and other cytotoxic factors. On the other hand, macrophages are polarized into TAMs under the influence of certain molecules released by tumor cells, promoting tumor development.
2.1 TDEVs regulate the polarization of macrophage
It is well known that macrophages participate in innate immune responses (42). CD14 and CD68 are the characteristic surface markers of the human macrophages (43–46). Circulating monocytes become macrophages when infiltrating into tissues. Based on the expression of specific surface markers, monocyte-derived macrophages can be polarized and divided into two phenotypes including M1 and M2 (47). In humans, M1 macrophages specifically express CD64, CD86, MARCO, CXCL9, CXCL10, CXCL11, NOS2, and SOCS1 (46, 48–51) on the surface, while M2 macrophages are identified by expressing TGM2, CD23, CD163, CD206, ARG1 and CCL22 (44, 46, 48, 49, 52–54). M1 macrophages, also known as classically activated macrophages, are induced by Th1 cytokines such as IFN-γ, IL-1β, and LPS. They secrete pro-inflammatory cytokines and function as anti-tumor cells. M1 macrophages bear the antigen-presenting ability so that they can activate adaptive immunity and bring about tissue damage (39, 40, 42, 55, 56). Secreting inhibitory factors like IL-4, IL-10, and IL-13, M2 macrophages show pro-tumor activity by promoting local immunosuppression, angiogenesis, and metastasis (57, 58). Tumor-associated macrophages (TAM), taking up 50% of the host infiltrating cells in TME, are usually considered as M2 phenotype (59). Meanwhile, there are many subsets in between that have yet to be clarified, expressing both M1 and M2 markers (60–62). Studies have shown that the polarization of macrophages in TME towards M1 or M2 can be promoted by contents in TDEVs. In Table 1 we summarize previous studies that investigate TDEVs cargoes in regulating the polarization of the macrophage, including miRNAs, lncRNAs, circRNAs, and proteins.
2.1.1 miRNAs cargo in TDEVs
Tumor-derived miRNAs assist in M2 polarization, thereby enhancing tumor proliferation through intercellular dialogue. The PTEN/PI3K/AKT signaling pathway is a common mechanism by which miRNAs regulate macrophage polarization. In macrophages, activating the PI3K/AKT pathway leads to the activation of signal transducer and activator of transcription 3 (STAT3), which is crucial for the differentiation of macrophages into M2 phenotype (102, 103). For example, miR−21 is carried in human bladder T24 cancer cell-derived EVs and promotes macrophages to M2 polarization, which through inhibited PI3K/AKT dephosphorylation leads to increase STAT3 activation (63). The miRNAs (miR-25-3p, miR-130b-3p, miR-425-5p) transferred from colorectal cancer (CRC) to macrophages via EVs can enhance M2 polarization by adjusting PTEN through the activation of PI3K/Akt signaling pathway, which finally leads to tumor EMT, angiogenesis, and liver metastasis (64). The PTEN/PI3K/AKT signaling pathway is also the target of miR-301a-3p from esophageal squamous cancer cell-derived EVs (65) and miR-222 from Adriamycin-resistant MCF-7 breast cancer cell-derived EVs (66) to induce M2 polarization. Chen et al. have reported that highly enriched miR-19b-3p in lung adenocarcinoma-derived EVs promoted M2 polarization through the Hippo pathway (67). MiR-19b-3p targets PTPRD, suppresses the PTPRD-mediated dephosphorylation of STAT3, activates STAT3, and induces polarization (67). In addition, Yan et al. have found that cervical cancer cell-secreted EVs transported miR-423-3p to stimulate macrophage M2 polarization by blocking the expression of CDK4 mRNA (68).
EVs produced by hypoxic tumor cells are enclosed with miR-21 and promote the transformation from monocyte to M2-polarized macrophage, and form an immunosuppression environment in TME (69). The same effect of EV miR-21 has also been observed in head and neck squamous cell carcinoma (HNSCC) and bladder cancer (63, 70). Moreover, breast cancer cell-derived EVs carrying miR-138-5p suppress M1 polarization and upgrade M2 polarization through the inhibition of epigenetic factor lysine demethylase 6B (KDM6B) expression in a suspension coculture system comprising breast cancer cells and macrophages (71).
Notably, some miRNAs in EVs are capable of suppressing tumor progression. Non-small cell lung carcinoma (NSCLC) cell-derived extracellular vesicular miR-770 has been confirmed to inhibit M2 macrophage polarization by targeting MAP3K1, which in turn prevents tumor invasion (72). MiR-130 originating from breast cancer cells leads to a reprogramming from M2 macrophages to M1 macrophages while the upregulation of M1 specific markers and downregulation of M2 specific markers were tested. After reprogramming, the phagocytic function of macrophages is enhanced and the ability to metastasize is impaired (73). Furthermore, miR-9-enriched EVs derived from HPV + HNSCC promote M1 phenotype polarization and then improve the radiosensitivity of HNSCC (74).
2.1.2 lncRNAs cargo in TDEVs
lncRNAs are RNAs longer than 200 nucleotides with a relatively restricted protein-coding capacity (104). lncRNAs can promote M2 polarization in many ways. Chen et al. found that the knockdown of lncRNA PCAT6 could prevent its positive effect on M2 polarization and in turn accelerated NSCLC development (75). lncARSR-containing EVs derived from renal cell carcinoma achieve a similar effect via the STAT3 pathway (76). MiR-147a/ARID3A axis is activated under hypoxia condition by hepatocellular carcinoma (HCC)-derived EVs delivering lncRNA HMMR-AS1 (77). Mechanistically, HMMR-AS1 interacts with miR-147a to reduce ARID3A degradation after which M2 macrophage polarization and the development of HCC are promoted (77). In addition, lncRNA TP73-AS1 is highly expressed in nasopharyngeal carcinoma cell-derived EVs, which binds to miR-342-3p, promotes the M2 polarization of macrophages, and reinforces the motility and microtubule formation of macrophages (78). Furthermore, FGD5-AS1 enriched in pancreatic cancer cell-derived EVs mediates M2 polarization of macrophages by activating STAT3/NF-κB pathway (79).
Interestingly, previous studies have found that lncRNAs can bind with miRNA to remove them from circulation and then promote tumor growth. For example, lncRNA ELFN1-AS1 exists in osteosarcoma cell-derived EVs sponge miR-138-5p and miR-1291 to suppress the M2 polarization (80). The down-regulation of miR-875-3p reached by lncRNA HCG18 in gastric cancer cell-derived EVs facilitates the M2 polarization of macrophages (81).
In addition to regulating macrophage polarization, lncRNAs also cause chemoresistance. It has been confirmed that glioblastoma cell-derived extracellular vesicular lnc-TALC induces temozolomide resistance by binding to ENO1 and phosphorylating p38 MAPK (105). LncRNA PART1 in esophageal squamous cell carcinoma cell-derived EVs competitively combines with miR-129 to raise the expression of Bcl-2 in esophageal squamous cell carcinoma cells, thus inducing the drug resistance of tumor cells to gefitinib (106). This indicates that lncRNA in EVs may serve as a promising therapeutic target for cancer patients.
2.1.3 circRNAs cargo in TDEVs
Emerging studies indicate that circRNAs play a critical role in tumor-induced immune responses. CircRNAs sometimes serve as miRNA sponges, binding with them and removing them from circulation. For instance, esophageal squamous cancer cell-derived hsa-circ-0048117 is transmitted to macrophages, works as a miR-140 sponge, and mediates M2 polarization (82). Hsa_circ_0017252-containing EVs of gastric cancer inhibits M2 polarization and tumor development through sponging miR-17-5p (83).
Various signaling pathways are involved in the regulation of macrophage polarization. Tumor-derived circFARSA delivered by EVs regulates M2 polarization via PTEN/PI3K/AKT pathway to raise the metastatic potential of NSCLC (84). Circ_0001142 carried by breast cancer cell-released EVs influences macrophages’ autophagy and polarization via circ_0001142/miR-361-3p/PIK3CB pathway (85). Circ_0001142, targeting PIK3CB, is capable of activating the PI3K/AKT - an effect that can be reversed by miR-361-3p (85). There are other signaling pathways circRNAs interfere with, resulting in M2 polarization and tumor progression, such as the miR-124-3p/EZH2 axis targeted by circPVT1 in EVs and miR-620/JAK1/STAT3 axis targeted by circSAFB2 in EVs. Lung cancer cell-derived circPVT1 increases EZH2 expression by downregulating miR-124-3p expression so that macrophages are induced to polarize towards an M2-like phenotype (86). CircSAFB2 in renal cell carcinoma cell-derived EVs functioned as a miR-620 sponge while JAK1 and STAT3 protein levels were tested markedly lower after co-culturing with miR-620 mimics (87). This correlates with the result that renal cell carcinoma cell-derived EVs induce macrophages express a higher level of JAK1 and STAT3 protein, and miR-620 can prevent the JAK1 and STAT3 expression (87). In addition, circNEIL3 has been shown to contribute to tumor progression by stabilizing the oncogenic protein IGF2BP3, which is packaged by hnRNPA2B1 in glioma cells and transported to TAMs (88).
2.1.4 Protein cargo in TDEVs
Previous studies have found that proteins carried by TDEVs affect the polarization of macrophages in multiple ways. Park et al. reported a 3-4-fold increase of total EV-containing protein per cell under hypoxia conditions in melanoma cell lines (B16-F0 and A375), skin squamous cell carcinoma cell line (A431), and lung cancer cell line (A549). Several abundant proteins such as CSF-1, MCP-1/CCL2, EMAP2/AIMP1, and LTA4H were detected in these EVs, which help in monocyte/macrophage recruitment and M2 polarization (89). Diffuse large B-cell lymphoma-derived EVs are enriched in gp130, which functions as the activator of the STAT3 signaling pathway to stimulate downstream targets like BCL2, SURVIVIN, and BAX to promote M2 polarization (90). Similarly, leptin existing in the EVs derived from gallbladder cancer boosts M2 macrophage polarization by activating the STAT3 signaling pathway as well (91). On the contrary, protein tyrosine phosphatase receptor type O (PTPRO) in EVs produced by breast cancer cells induces M1 polarization via inactivating the STAT signaling pathway and then inhibits tumor migration (92). In addition, nasopharyngeal carcinoma-derived EVs containing RNF126 induce the M2 polarization of macrophages and contribute to the invasion and metastasis of tumors. Yu et al. have demonstrated that RNF126 degrades PTEN and provokes the PI3K/AKT pathway to regulate macrophage polarization (93). Furthermore, HNSCC-derived EVs carrying Anillin, actin-binding protein (ANLN), induced M2 polarization of macrophages via PTEN/PI3K/AKT signaling pathway (94).
The T-cell immunoglobulin and mucin domain 3 (TIM-3), also known as HAVCR2, has been proved to express in activated Th1 cells, Tregs, macrophages, dendritic cells, NK cells, and tumor cells (107, 108). Cheng Z. et al. found that the TIM-3 in osteosarcoma cells-derived EVs promoted M2 polarization, tumor invasion, metastasis, and EMT (95). The underlying mechanism is that TIM-3 increases the expression of N-cadherin and Vimentin, but decreases that of E-cadherin in infiltrated monocytes (95). While Li et al. also demonstrated that TIM-3 enriched in melanoma cell-derived EVs facilitated M2 type differentiation but the mechanism remained elusive (96).
It is well known that the plasma membrane-associated receptors play an important role in the function of immune cells. The surface receptors can also be packed in TDEVs and exert an influence on macrophages in different manners. Yuan et al. revealed the mechanism underlying endoplasmic reticulum stress and tumor development. They found that endoplasmic reticulum stress led oral squamous cell carcinoma (OSCC) to produce EVs loaded with PD-L1 and up-regulate the expression of PD-L1 in macrophages, thus driving the M2 macrophage polarization (97). In addition, ICAM-1 enriched in PDAC-derived EVs binds to CD11c on the surface of macrophages. Besides inducing M2 phenotype differentiation, these EVs also up-regulate the secretion of pro-tumoral molecules like VEGF, MCP-1, IL-6, IL-1β, MMP-9, and TNFα in macrophages exposed (98). Moreover, evidence indicates that prostate cancer cell-derived EVs loading CXCL14 promotes M2 polarization through activating NF-κB signaling, which is a key regulator of macrophage function and tumor progression (99, 100). Prostate cancer cells release two kinds of EVs, including αvβ6 (a surface receptor of the integrin family) positive and negative expression EVs. The αvβ6-positive EVs promote the M2 type differentiation of peripheral blood mononuclear cells, while the negative ones prevent this effect (101).
2.2 TDEVs regulate the pro-inflammatory responses of macrophage
Macrophages can secret pro-inflammatory factors to regulate fibrosis, metabolism, cellular debris, and T cell function directly or indirectly (109). For example, IL-6 released from macrophages dominates in phosphorylating STAT3 and further promotes tumor growth and metastasis (110, 111). The level of pro-inflammatory cytokines can be up-regulated by TDEVs in gastric cancer, breast cancer, lung cancer, CRC, melanoma, and OSCC (112–119), leading to tumorigenesis and metastasis further. Inflammatory cytokines array showed that the expression of IL-6, CCL2, GCSF, and TNF-α was augmented by TDEVs, along with the phosphorylation of transcription factor NF-kB, which indicates that TDEVs stimulate the secretion of pro-inflammatory cytokines via NF-κB signaling pathway in gastric cancer, lung cancer, breast cancer and melanoma (112–115, 120). Various components of EVs exerting regulatory effects are being studied. For example, palmitoylated proteins on the surface of EVs are identified as a key factor in binding with macrophage surface protein Toll-like receptor (TLR) 2 to further phosphorylate NF-κB and activate inflammatory responses (113) (Figure 1). In addition, miRNAs also play an important role in modulating pro-inflammatory cytokines release. Lung cancer-derived EVs transporting miR-16, -21, -29a bind to TLR7/8 on the surface of macrophages to elicit phosphorylated activation of NF-κB, which induces an increase in transcription of pro-inflammatory cytokines including IL-6 (115). Similarly, miR-25-3p in breast cancer-derived EVs binding with TLR7/8 increases the expression of IL-6 and phosphorylated NF-κB (116). Moreover, oncogenic miR-183-5p in breast cancer-derived EVs are engulfed by macrophages and downregulate target gene PPP2CA expression by combining with the binding sequence which leads to a decrease in dephosphorylation of p65, consequently promoting IL-1β, IL-6, and TNF-α secretion (121).
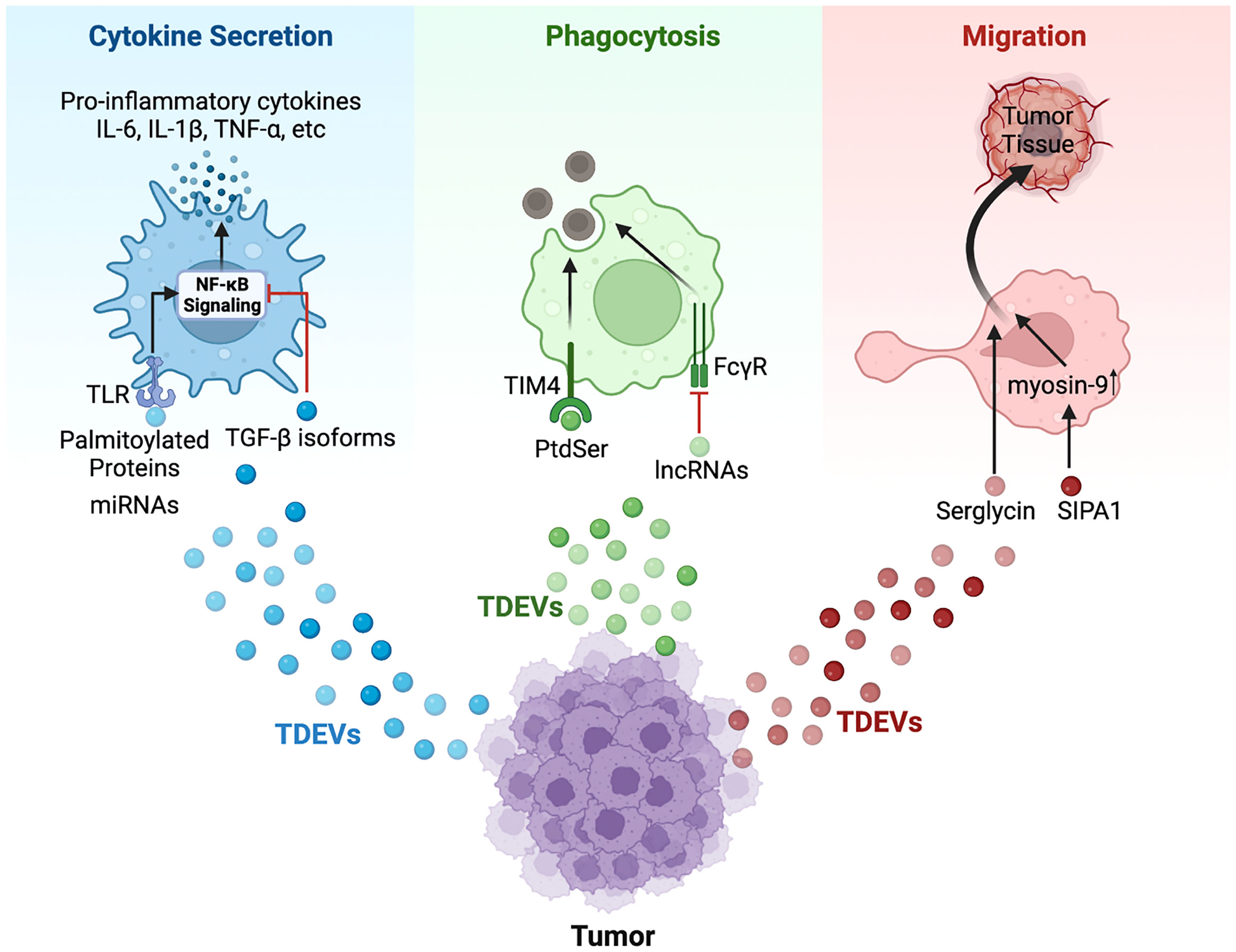
Figure 1 Schematic model of TDEVs in regulating the functions of macrophage. TDEVs can modulate the cytokine secretion, phagocytosis, and migration of macrophages via various signaling pathways. For cytokine secretion (Blue): Palmitoylated proteins carried by gastric cancer-derived EVs bind with TLR2 on the surface of macrophages to further activate NF-κB signaling, leading to an elevated level of pro-inflammatory cytokines including IL-6, IL-1β, TNF-α and so on. Similarly, miRNAs in EVs secreted by lung cancer and breast cancer bind with TLR7/8 to induce pro-inflammatory cytokines secretion via the NF-κB pathway. However, HNSCC-derived EVs cargo TGF-β isoform inhibits NF-κB signaling to down-regulate the expression of pro-inflammatory cytokines. For phagocytosis (Green): PtdSer in apoptotic tumor-derived EVs binds with receptors such as TIM-4 to promote the phagocytosis of apoptotic cells by macrophages. However, lncRNA TUC399 contained in HCC-derived EVs down-regulates the FcγR-mediated phagocytosis. For migration (Red): TDEVs from myeloma containing serglycin can augment macrophage migration, and SIPA1 in breast cancer-derived EVs induces elevated expression of myosin-9 in macrophages, which contributes to migration.
The STAT3 pathway is engaged in the modulation by TDEVs. EVs released by endoplasmic reticulum-stressed liver cancer cells upregulate IL-6, IL-10, and MCP-1 levels but downregulate TNF-α levels in macrophages with an increase in p-JAK2 and p-STAT3 (119). Gp130 (IL-6 receptor) is carried by TDEVs and interacts with macrophages to induce the phosphorylation and translocation of STAT3 to the nucleus, leading to an elevated expression of IL-6 and a shape of the pro-tumor cancer environment in several human breast cancer cell lines (MDA-MB231, MDA-MB-468, Hs578T, and MCF7) (118).
2.3 TDEVs regulate the anti-inflammatory responses of macrophage
TDEVs present a double-sided sword: they participate in activation of inflammatory responses, but they can downregulate the expression of pro-inflammatory cytokines as well. HREV-positive EVs derived from two CRC cell lines (SK-CO1 and Caco-2) lead to a lower level of pro-inflammatory cytokine IL-1β and a higher level of anti-inflammatory cytokine IL-10 in the zebrafish model with a positive correlation between the concentration of HERV-positive EVs and anti-inflammatory responses (122). HNSCC-derived EVs down-regulate macrophage release of IL-1β, indicating that HNSCC-derived EVs block the activation of inflammatory responses. TGF-β isoforms composition is hypothesized to be the key factor via inhibiting the NF-κB signaling pathway (123) (Figure 1). Previous studies have also indicated that miRNAs play an important role in the modulation of cytokines. Li J. et al. found that EVs treated under hypoxia and released by lung cancer cells down-regulate the expression of pro-inflammatory cytokines IL-6 and IL-1A through cargo containing miR101 while targeting CDK8 and SUB1 (124). Moreover, lncRNAs act as the mediator of cytokines secretion through TDEVs. Li X. et al. found that HCC-derived EVs containing lncRNA TUC339 were engulfed by macrophage (THP-1 cell) and downregulate the secretion of IL-1β and TNF-α (125).
2.4 TDEVs regulate the macrophages phagocytotic function
Macrophages, as phagocytes, engulf apoptotic cells and debris that trigger immune responses to exert an anti-tumor effect (109). Previous studies have indicated that the phagocytotic activity of macrophages can be downregulated by TDEVs. EVs from metastatic osteosarcoma (K7M3 and DLM8) reduce the phagocytic function of alveolar macrophages via promoting TGF-β2 secretion, while there is no significant change in phagocytosis of macrophages taking up non-metastatic osteosarcoma (K7 and Dunn) -derived EVs (126). Along these same lines research conducted by Li X. and colleagues showed that HCC-derived EVs enriched in lncRNA TUC339 led to decreased FcγR-mediated phagocytosis in macrophages were found in (125). Furthermore, Gregory C.D. et al. found that apoptotic TDEVs contained phosphatidylserine (PtdSer) to bind with proteins such as T cell immunoglobulin and mucin domain-4 (TIM-4), which had a positive relation to phagocytosis of apoptotic cells (127) (Figure 1). These studies shed light on the relationship between phagocytosis in macrophages and TDEVs, however, the specific mechanism is still unclear.
2.5 TDEVs modulate the macrophages migration
During cancer development, macrophages migrate out of circulation and into the tumor milieu, triggering inflammation and tumor metastasis (128). EVs released by PDAC elicit migration to the liver of bone marrow-derived cells including macrophages, which follows an elevated level of TGF-β released by Kupffer cells (18). Myeloma-derived EVs with serglycin engulfed by macrophages augment migration as compared with serglycin-null EVs (129). In addition, it is reported that EVs from breast cancer cells (MDA-MB-231) expressing high levels of signal-induced proliferation-associated 1 (SIPA1) promote the migration of macrophages to tumor tissue (130) (Figure 1). SIPA1 binds to the promoter of the target gene MYH9 to upregulate the transcription of MYH9, and the enrichment of myosin-9 in EVs contributes to macrophage migration (Figure 1).
3 Dendritic cells
Dendritic cells (DCs), derived from hematopoietic stem cells, are identified as a vital kind of innate immune cells. As APCs, DCs recognize and swallow pathogens, subsequently presenting to immune cells like T cells to activate immune responses, by corresponding receptors and co-stimulatory molecules on the surface (131). In addition, DCs also secrete cytokines and chemokines capable of modulating the microenvironment and tumor development. Various functions of DCs are regulated by TDEVs, and interestingly they are capable of causing both anti-tumor and pro-tumor effects under certain conditions. DCs are divided into three subsets, classical DC (cDC), plasmacytoid DC (pDC), and monocyte-derived DC (mo-DC) (132). The former two are derived from common dendritic cell progenitors (CDPs), and mo-DC are derived from monocytes. Mature DCs express a higher level of functional molecules including co-stimulatory molecules (CD40, CD80, CD86), MHC II, pro-inflammatory cytokines, and CCR7 comparing to immature DCs, via the stimulus with GM-CSF, IFN-γ, IL-4 and pathogens (133).
3.1 Anti-tumor effect of DCs on responses to TDEVs
TDEVs augment anti-tumor activity by promoting the function of DCs in many cancers like melanoma, HCC, and colon carcinoma (120, 134, 135). It has been found that tumor antigen (TA) carried by EVs are a key factor in this process. HCC cell-derived EVs inhibit tumor growth by transferring HCC antigens and antigenic chaperones to DCs, which induces cytolysis and increases IFN-γ expression but decreases IL-10 and TGF-β expression. Furthermore, DCs treated with HCC cell-derived EVs activate T-cell immunity by presenting antigens (135). Similarly, EVs containing tumor antigen ErbB2 promotes the activation of CD8+T cell by DC (136). Melanoma cell-derived EVs carrying tumor-associated antigens (TAAs) promote DCs to express maturation marker CD86 and raise the anti-tumor activity in mouse models (137). In addition to whole-tumor antigens, MHC-I peptide complexes are also transferred to DCs by TDEVs in melanoma, resulting in the activation of cytotoxic T-lymphocytes (CTL) (138). The specific mechanism for this is still under investigation. The only known study to date is by Squadrito M.L. et al. who found that CRC-derived EVs internalized by DCs promoted the presentation of tumor antigens mediated by MHC-I, and extracellular vesicle-internalizing receptor (EVIR) played an important role in the binding and internalization of TDEVs by DCs (134) (Figure 2).
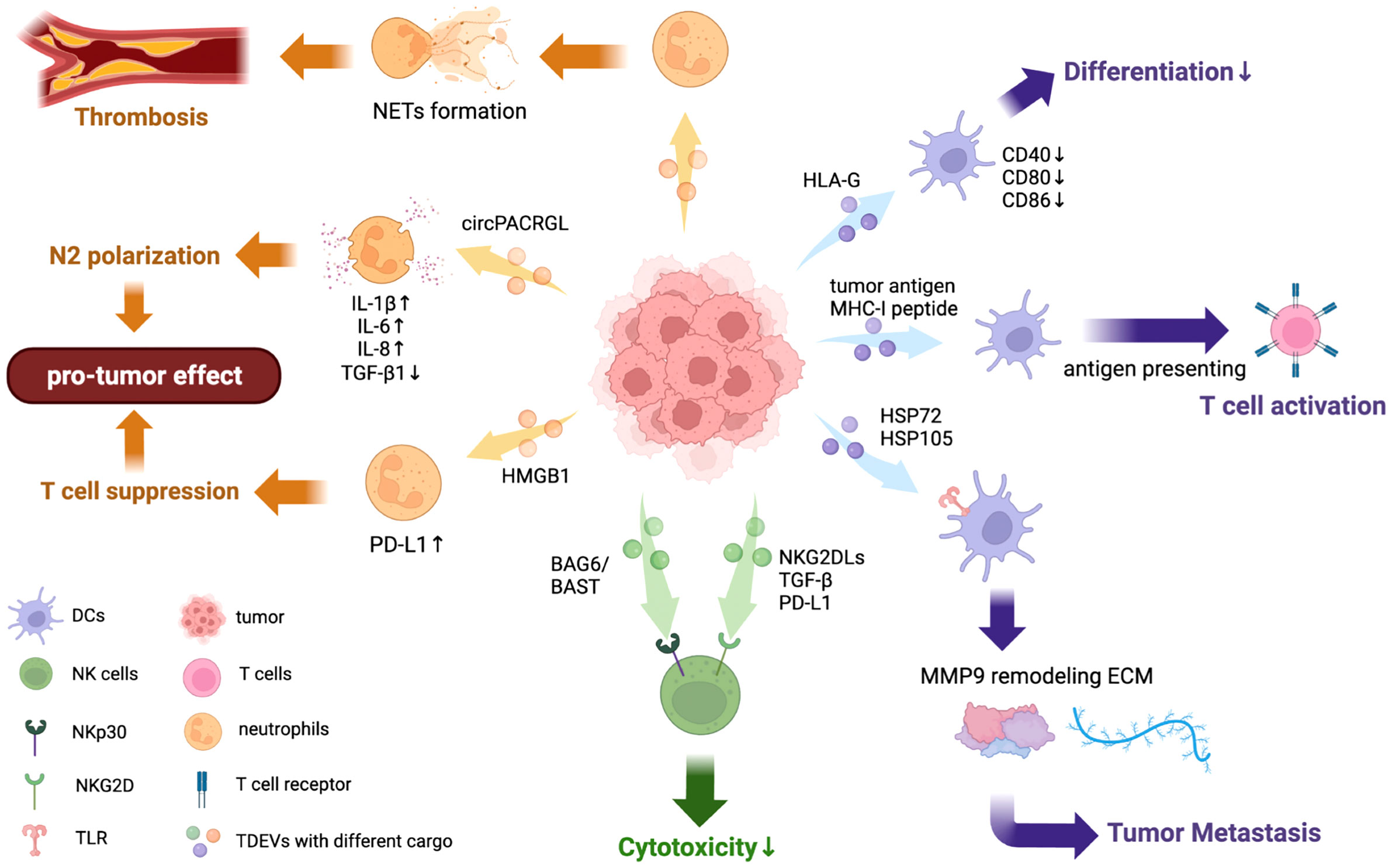
Figure 2 Effects of TDEVs in regulating DCs, neutrophils, and NK cell functions. TDEVs can interact with innate immune cells including DCs, neutrophils, and NK cells, exerting a dual effect in regulating their functions. TDEVs to DCs: EVs containing tumor antigen from HCC can transfer it to DCs and further activate T cell immunity, indicating its anti-tumor effect. TDEVs can impair DCs differentiation and maturation to suppress immune responses in some cancers, HLA-G is identified as a key factor in this negative regulation. Some TDEVs contain HSP72 and HSP105 bind with TLR on DCs to increase MMP9 expression, reorganizing ECM and contributing to tumor invasion. TDEVs to neutrophils: circRNA PACRGL in CRC-derived EVs are engulfed by neutrophils and down-regulate TGF-β1 expression, further inducing polarization to the N2 phenotype. TDEVs can also induce NET formation by neutrophils in a dose-dependent way, which further contributes to thrombosis. TDEVs containing HMGB1 can upregulate PD-L1 on neutrophils to suppress T cell immunity, exerting a pro-tumor effect. TDEVs to NK cells: BAG6/BAT3 on the surface of TDEVs can bind with NKp30 to suppress NK cytotoxicity via the nSmase2-dependent pathway. NKG2DLs, TGF-β, and other immunosuppressive proteins from TDEVs bind with NKG2Ds on the surface of NK cells to block NK cytotoxicity.
3.2 Immunosuppress effect of DCs on responses to TDEVs
A decrease in pro-inflammatory cytokine secretion but an increase in anti-inflammatory cytokines can be detected in DCs stimulated with TDEVs. In Lewis lung cancer (LLC) and breast cancer, surface markers including CD80, MHC-II, and CD86 are downregulated by TDEVs, indicating that DCs are immature. In addition, the cytokine expression in DCs is regulated by TDEVs as well. There is a decrease in TNF-α, IL-6, and IL-12, but an increase in Arginase I while the levels of IL-10 and IL-12p40 do not change significantly. In addition, chemokine receptor expression (which is essential for the migration of DCs) is inhibited by LLC cell-derived EVs (139). The expression of IL-6 is increased by TDEVs in breast cancer, lung cancer, and melanoma in various ways (140). HSP72 and HSP105 proteins on melanoma-derived EVs surface bind with TLR2 and TLR4 on DCs, causing the phosphorylation of ERK, JNK, p38, and NF-kB to induce expression of IL-6. IL-6 induces STAT phosphorylation to bind in the MMP9 promoter site and elevates the transcription of MMP9. Due to the function of reorganizing extracellular matrix by MMP9 (141), tumor cells invade other organs. PGE2 carried by prostate cancer-derived EVs binds to receptors EP2/EP4 on DCs to upregulate the CD73 expression, while the adenosine monophosphate (AMP)-depends on expressions of IL-12 and TNF-α decrease subsequently (142). However, the underlying mechanism still needs further investigation.
Notably, microRNAs in TDEVs can interfere with the function of DCs. MiR-212-3p carried by pancreatic cancer cell-derived EVs inhibited the expression of regulatory factor X-associated protein (RFXAP) to decrease MHC II on DCs and induce immune tolerance (143), while miR-203 induced the downregulation of TLR4 and cytokines in DCs such as TNF-α and IL-12 in pancreatic cancer (113). In head and neck cancer, TDEVs disrupt the maturation, viability, and migration of mono-CDs targeted by 133 miRNAs including miR-16, miR-23b, miR-24. CD80 and HLA-DR expression have been shown to exhibit a decrease in DCs after incubating with HNSCC cell-derived EVs (144) (Figure 2).
3.3 TDEVs modulate the differentiation of DCs
Wieckowski E. et al. found that antigen-processing machinery (APM) components including MIB1, IMP7, Tapasin, and Calreticulin were downregulated in monocytes after stimulation with TDEVs, indicating impaired differentiation from monocytes to DCs (145). Surface proteins that demonstrate the maturation of DCs decrease with the stimulation of TDEVs in melanoma, lung cancer, renal cancer, breast cancer, and thymoma (139, 146–149). Expressions of markers such as CD40, α5 integrin, CD80, CD86, and HLA-DR are downregulated in monocyte-derived DCs after co-incubation with renal cancer derived-EVs carrying HLA-G, which can be inhibited by anti-HLA-G-antibody. This confirmed the negative-regulatory role of EVs with HLA-G in DCs differentiation (146) (Figure 2). Hendrik Gassmann and colleagues proposed a model where Ewing sarcoma-derived EVs carrying RNA and protein activate myeloid cell pathology and induce the secretion of pro-inflammatory cytokines such as IL-6, IL-8, and TNF, which modulates the differentiation of myeloid cells into semi-mature DCs and impairs T cell activation (150) (Figure 2). Moreover, the role of modulating differentiation by IL-6 was also examined in the breast cancer (148). Glioma-derived EVs down-regulate the expression of IL-12p70 in immortalized DCs, which orchestrates the maturation and differentiation of DCs (151).
4 Neutrophils
Neutrophils, abundant in circulation, are indispensable for an immune response due to their dual role of both affecting innate immunity and modulating adaptive immunity (152). The complicated function of neutrophils in the innate immune response includes forming neutrophil extracellular traps (NETs), polarization to a different state, phagocytosis, co-regulation with T cells, and so on. Interestingly, NETs are a double-sided sword in the immune response. On the one hand, they neutralize and ensnare microbiotics to against infection. On the other hand, they have adverse such as promoting thrombosis, tumor metastasis, and inflammation that causes organ and vascular damage (Figure 2). In addition, neutrophils also exert a dual effect on tumors by polarization to N1 or N2 phenotypes. In TME, stimulators including TGF-β and IFN-β respectively switch the phenotype of tumor-associated neutrophils (TANs) into N1 and N2 phenotypes (153) (Figure 2). The N1 phenotype shows an anti-tumor effect via enhancing apoptosis and secreting pro-inflammatory cytokines, while the N2 phenotype promotes tumor development and suppresses immune responses (154) (Figure 2).
4.1 TDEVs promote neutrophils NETs formation
TDEVs engulfed by neutrophils target NETs to promote thrombosis. A previous study has shown that tumor microparticles carrying tissue factor (TF) promoted cancer-associated deep vein thrombosis (DVT) initiation by adhering to NETs in a mouse model with pancreatic cancer (155). Ana C. Leal et al. showed that 4T1 murine breast tumor derived-EVs contribute to the prothrombotic state via inducing NETs formation by neutrophils stimulated by G-CSF in the murine breast cancer model (Figure 2). Moreover, there is a dose-dependent procoagulant property of 4T1 derived-EVs and this progress relies on the ability to recruit TDEVs by NETs (156). Exposure to TDEVs which bear gDNA induces TF activation in leukocytes, along with the upregulation of IL-8 (157). However, the function that TDEVs stimulated NETs promoting tumor growth is still under investigation.
4.2 TDEVs modulate neutrophils polarization
In addition to stimulating the formation of NETs, TDEVs play an important role in regulating the polarization of neutrophils. In CRC, TDEVs carrying oncogene circPACRGL promote the differentiation of neutrophils from N1 to N2 by regulating the miR-142-3p/miR-506-30-TGF-β1 axis (158). circRNA PACRGL swallowed by neutrophils binds to miR-142-30/miR-506-3p to inhibit the post-transcriptional control of mature mRNA and therefore TGF-b1 expression is downregulated, which induces neutrophils into the N2 phenotype (Figure 2). In addition, TDEVs induce neutrophil polarization to the N2 phenotype via the NF-κB pathway in gastric cancer and CRC (159, 160). Gastric cancer-cell derived EVs carrying high mobility group box-1 (HMGB1) bind with TLR or receptor for advanced glycation end products (RAGE) to activate NF-κB signaling, which induces the phosphorylation of downstream proteins including p65, STAT, and ERK and upregulates the expression of inflammatory factors such as IL-1β, IL-6, IL-8, OSM, and TNFα (Figure 2). Moreover, these pro-tumor effects of EVs can be blocked by NF-κB inhibitors (159). In HCC, TDEVs regulate the phenotype of neutrophils into N2, but the exact mechanism needs further investigation (161). Taken together, these data suggest that TDEVs induce neutrophils to polarize into the pro-tumor state of the N2 phenotype in various signaling pathways.
4.3 TDEVs regulate neutrophils’ other functions
Other functions of neutrophils can also be regulated by TDEVs. For example, the lifespan of neutrophils was prolonged by EVs from CRC stem cells by modulating the expression of IL-1β via the NF-κB signaling axis. EVs with tri-phosphate RNAs, acting as pathogen-associated molecular pattern (PAMP) molecules, interact with PRRs and activate the NF-κB pathway with elevated expression of nuclear p65 and IL-1β [20]. In addition, PD-L1 on neutrophils is upregulated by gastric cancer cell-derived EVs, activated by HMGB1 via phosphorylating STAT3 and downstream molecules, which suppresses T-cell immunity to have a pro-tumor influence (162) (Figure 2). More changes in neutrophil function by TDEVs need further investigation.
5 NK cells
NK cells are a subset of type 1 ILCs with surface markers CD3- and CD19-, but are CD56+ and CD16+. They originate from common lymphoid progenitor (CLP) cells in the bone marrow and are widely distributed in the blood, peripheral lymphoid tissue, liver, spleen, and other organs, accounting for 5-10% of peripheral blood mononuclear cells (163, 164). Activating and inhibitory receptors are co-expressed on the surface of NK cells, which can bind to MHC I molecules expressed on the surface of one’s cells. NK cells also express NKG2D and natural cytotoxicity receptors (NCR) (NKp30, NKp44, and NKp46) (165). They are activating receptors that do not interact with MHC I. Cancerous cells decrease the expression of MHC I and cause a loss of inhibitory receptor function, referred to as the “missing-self” mode. Meanwhile, tumor cells overexpress ligands of NKG2D and NCR, providing sufficient targets for activating receptors via the “induced-self” mode (166, 167). NK cells are activated through the above two modes and kill tumor cells by releasing perforin, granzyme, TNF-α, or FasL (168). In addition, as a group of type 1 ILCs, NK cells synthesize and secret IFN-γ to play a role in the anti-infection and immune regulation (169, 170).
In most cases, TDEVs exert an influence on NK cells through NKG2D ligands (MICA, MICB, ULBP-1, ULBP-2, or ULBP-3) existing on the surface of EVs. NKG2DLs downregulate NKG2Ds floating on the surface of NK cells and block cell activation, resulting in the damage of NK cytotoxicity (171, 172) (Figure 2). Besides NKG2DLs, TGF-β1 (173, 174) and some other immunosuppressive proteins (PD-L1, CD39, CD73, FasL, LAP-TGFβ, TRAIL, CTLA-4) (175–177) are common cargoes of TDEVs, which act the same way as NKG2DLs do (Figure 2). TGF-β in EVs has another mechanism to inhibit the activation of NK cells: interacting with its receptors on the surface of NK, activating the TGFβ-Smad2/3 pathway, and promoting the phosphorylation of Smad2/3 (178, 179). Furthermore, soluble ligand BAG6/BAT3 was found to exist in chronic lymphocytic leukemia patients’ blood (180). Once BAG6/BAT3 is expressed on the surface of EVs it interacts with the activating receptor NKp30 of NK cells and induces cell stress through the nSmase2-dependent pathway to suppress NK cytotoxicity (180).
Some cytokines in TDEVs have a dual effect on NK cells. For example, genetically modified myeloid leukemia cell line K562 expresses IL-15, IL-18, and 4-1BBL (TNFSF9) on the surface of its EVs. These proteins stimulate NK cells to proliferate and enhance the cytotoxicity of NK cells within 4 hours. However, as time goes by (48 hours), the cytokines reduce NK cytotoxicity via the inhibition of activated receptors (NKG2D、NKp44) and the promotion of inhibitory receptors (NKG2A) (181). Moloudizargari et al. also reported the bifacial effect of myeloma derived-EVs on NK cells (181, 182).
The RNA component in TDEVs is also participating in regulating NK cells’ function. CRC-derived EVs containing lncRNA SNHG10 increase INHBC expression and then suppress the activation of NK (183). MiRNA-378a-3p in EVs is induced in tumors undergoing radiotherapy, leading to the reduction of granzyme-B secretion and loss of activity in NK cells (184). EVs from HCC cells deliver circUHRF and affect NK cells through three different ways to achieve immunosuppression and drug resistance. Firstly, decreasing IFN-γ and TNF-α secretion of NK cells. Secondly, degrading miR-449c-5p to foster the expression of TIM-3. Based on the fact that circRNAs usually work as miRNA sponges, studies indicated that circUHRF and miR-449c-5p may target each other in human NK-92 cells, thus impairing their function. Finally, there is a finding that the high levels of circUHRF1 in EVs can increase the connection with limited NK cell proportion and tumor infiltration (185).
6 Conclusions and perspectives
In recent years, EVs have become a popular topic in cancer and immunity research due to their complicated functions in regulating TME and their important role in mediating cell-to-cell communication (186, 187). Almost all cells including immune cells and cancer cells can generate EVs with common or specific cargoes which interact with recipient cells to further affect their functions and tumorigenesis (188, 189). In this review, we summarize the regulation of innate immune cells including macrophages, DCs, NK cells, and neutrophils by TDEVs (Figures 1, 2). Overall, TDEVs exert a dual effect on immune responses and tumor development. For example, the secretion of pro-inflammation cytokines by macrophages and cytotoxicity of NK cells is either up-regulated or down-regulated by TDEVs. Moreover, TDEVs induce macrophage polarization to different states (functions as pro-tumor or anti-tumor), further influencing tumorigenesis, metastasis, and so on. Previous studies have demonstrated that the specific function of TDEVs mainly depends on their bioactive cargoes and tumor stage. For instance, at the beginning of tumorigenesis, EVs stimulate TAM and up-regulate cytokines that beneficial for angiogenesis and tumor metastasis. However, in the context of metastasis, anti-tumor responses such as cytotoxicity and phagocytosis are promoted by TDEVs. Based on these previous findings, the expression of innate immune cell markers and related molecules can be used as step-change indicators of diagnosis and prognosis in clinical treatment (190).
Various surface proteins and cargo components in TDEVs have been found to play an essential role in the regulation of macrophage polarization. Of the cargo components, most of the studies so far have been on miRNAs (Table 1). Other RNAs, proteins, and cytokines also target recipient cells to modulate their functions via direct binding with receptors or regulating corresponding gene expression. While the specific mechanism is still not completely known, NF-κB pathway and PTEN/PI3K/AKT pathways have been recognized as crucial signaling pathways in regulating immune responses. Therefore, key factor inhibitors can pharmacologically antagonize the effect of TDEVs. Moreover, there are also numerous studies concerning modulation of the formation, circulation, and absorption of TDEVs in anticancer therapies (191).
Although existing studies have provided many insights about the regulation of innate immunity by TDEVs, there are still many limitations. Firstly, the studies investigating TDEVs’ modulation of the function of innate immune cells like mast cells, eosinophils, and basophils remain inadequate. Secondly, more detailed mechanisms of TDEVs functions on innate immunity are still under investigation, and the results of these studies will be vital for identifying new cancer targets. Additionally, the majority of current studies are in vitro experiments, and more in vivo investigations are supposed to be conducted to better demonstrate the effects of TDEVs in cancer progression (192). TDEVs mediating intercellular communication between cancer and innate immune cells is a promising field, which can shed a spotlight on novel cancer therapies. We hope more investigations will be conducted to promote this progression.
Author contributions
ZL conceived and designed this review. SW and JS draft the manuscript. SW, JS, RD, and ZL revised and edited the manuscript. All authors approved the final version of the manuscript. All authors contributed to the article and approved the submitted version.
Funding
This work was supported by the Research Start-up Fund of the Seventh Affiliated Hospital, Sun Yat-sen University (ZSQYBRJH0021 to ZL); Fundamental and Applied Basic Research Fund of Guangdong Province (2021A1515110512 to ZL); General project of Shenzhen Science and Technology Innovation Committee (JCYJ20210324134612035 to ZL); Fundamental Research Funds for the Central Universities, Sun Yat-sen University (22qntd3703 to ZL).
Conflict of interest
The authors declare that the research was conducted in the absence of any commercial or financial relationships that could be construed as a potential conflict of interest.
Publisher’s note
All claims expressed in this article are solely those of the authors and do not necessarily represent those of their affiliated organizations, or those of the publisher, the editors and the reviewers. Any product that may be evaluated in this article, or claim that may be made by its manufacturer, is not guaranteed or endorsed by the publisher.
Glossary
References
1. Thery C, Witwer KW, Aikawa E, Alcaraz MJ, Anderson JD, Andriantsitohaina R, et al. Minimal information for studies of extracellular vesicles 2018 (Misev2018): A position statement of the international society for extracellular vesicles and update of the Misev2014 guidelines. J Extracell Vesicles (2018) 7(1):1535750. doi: 10.1080/20013078.2018.1535750
2. Colombo M, Raposo G, Thery C. Biogenesis, secretion, and intercellular interactions of exosomes and other extracellular vesicles. Annu Rev Cell Dev Biol (2014) 30:255–89. doi: 10.1146/annurev-cellbio-101512-122326
3. Akers JC, Gonda D, Kim R, Carter BS, Chen CC. Biogenesis of extracellular vesicles (Ev): Exosomes, microvesicles, retrovirus-like vesicles, and apoptotic bodies. J Neurooncol (2013) 113(1):1–11. doi: 10.1007/s11060-013-1084-8
4. Stoorvogel W, Strous GJ, Geuze HJ, Oorschot V, Schwartz AL. Late endosomes derive from early endosomes by maturation. Cell (1991) 65(3):417–27. doi: 10.1016/0092-8674(91)90459-c
5. Sotelo JR, Porter KR. An electron microscope study of the rat ovum. J Biophys Biochem Cytol (1959) 5(2):327–42. doi: 10.1083/jcb.5.2.327
6. Hurley JH, Hanson PI. Membrane budding and scission by the escrt machinery: It’s all in the neck. Nat Rev Mol Cell Biol (2010) 11(8):556–66. doi: 10.1038/nrm2937
7. Babst M, Katzmann DJ, Estepa-Sabal EJ, Meerloo T, Emr SD. Escrt-iii: An endosome-associated heterooligomeric protein complex required for mvb sorting. Dev Cell (2002) 3(2):271–82. doi: 10.1016/s1534-5807(02)00220-4
8. Wollert T, Hurley JH. Molecular mechanism of multivesicular body biogenesis by escrt complexes. Nature (2010) 464(7290):864–9. doi: 10.1038/nature08849
9. Trajkovic K, Hsu C, Chiantia S, Rajendran L, Wenzel D, Wieland F, et al. Ceramide triggers budding of exosome vesicles into multivesicular endosomes. Science (2008) 319(5867):1244–7. doi: 10.1126/science.1153124
10. Fang Y, Wu N, Gan X, Yan W, Morrell JC, Gould SJ. Higher-order oligomerization targets plasma membrane proteins and hiv gag to exosomes. PloS Biol (2007) 5(6):e158. doi: 10.1371/journal.pbio.0050158
11. Raposo G, Nijman HW, Stoorvogel W, Liejendekker R, Harding CV, Melief CJ, et al. B lymphocytes secrete antigen-presenting vesicles. J Exp Med (1996) 183(3):1161–72. doi: 10.1084/jem.183.3.1161
12. You Y, Shan Y, Chen J, Yue H, You B, Shi S, et al. Matrix metalloproteinase 13-containing exosomes promote nasopharyngeal carcinoma metastasis. Cancer Sci (2015) 106(12):1669–77. doi: 10.1111/cas.12818
13. Yue S, Mu W, Erb U, Zoller M. The tetraspanins Cd151 and Tspan8 are essential exosome components for the crosstalk between cancer initiating cells and their surrounding. Oncotarget (2015) 6(4):2366–84. doi: 10.18632/oncotarget.2958
14. Yoshizaki T, Kondo S, Wakisaka N, Murono S, Endo K, Sugimoto H, et al. Pathogenic role of Epstein-Barr virus latent membrane protein-1 in the development of nasopharyngeal carcinoma. Cancer Lett (2013) 337(1):1–7. doi: 10.1016/j.canlet.2013.05.018
15. Al-Nedawi K, Meehan B, Kerbel RS, Allison AC, Rak J. Endothelial expression of autocrine vegf upon the uptake of tumor-derived microvesicles containing oncogenic egfr. Proc Natl Acad Sci U.S.A. (2009) 106(10):3794–9. doi: 10.1073/pnas.0804543106
16. Kessenbrock K, Plaks V, Werb Z. Matrix metalloproteinases: Regulators of the tumor microenvironment. Cell (2010) 141(1):52–67. doi: 10.1016/j.cell.2010.03.015
17. de Vrij J, Maas SL, Kwappenberg KM, Schnoor R, Kleijn A, Dekker L, et al. Glioblastoma-derived extracellular vesicles modify the phenotype of monocytic cells. Int J Cancer (2015) 137(7):1630–42. doi: 10.1002/ijc.29521
18. Costa-Silva B, Aiello NM, Ocean AJ, Singh S, Zhang H, Thakur BK, et al. Pancreatic cancer exosomes initiate pre-metastatic niche formation in the liver. Nat Cell Biol (2015) 17(6):816–26. doi: 10.1038/ncb3169
19. Chen G, Huang AC, Zhang W, Zhang G, Wu M, Xu W, et al. Exosomal pd-L1 contributes to immunosuppression and is associated with anti-Pd-1 response. Nature (2018) 560(7718):382–6. doi: 10.1038/s41586-018-0392-8
20. Poggio M, Hu T, Pai CC, Chu B, Belair CD, Chang A, et al. Suppression of exosomal pd-L1 induces systemic anti-tumor immunity and memory. Cell (2019) 177(2):414–27.e13. doi: 10.1016/j.cell.2019.02.016
21. Quatromoni JG, Eruslanov E. Tumor-associated macrophages: Function, phenotype, and link to prognosis in human lung cancer. Am J Transl Res (2012) 4(4):376–89.
22. Hinshaw DC, Shevde LA. The tumor microenvironment innately modulates cancer progression. Cancer Res (2019) 79(18):4557–66. doi: 10.1158/0008-5472.CAN-18-3962
23. Wu D. Innate and adaptive immune cell metabolism in tumor microenvironment. Adv Exp Med Biol (2017) 1011:211–23. doi: 10.1007/978-94-024-1170-6_7
24. Motta JM, Rumjanek VM. Sensitivity of dendritic cells to microenvironment signals. J Immunol Res (2016) 2016:4753607. doi: 10.1155/2016/4753607
25. Le Mercier I, Poujol D, Sanlaville A, Sisirak V, Gobert M, Durand I, et al. Tumor promotion by intratumoral plasmacytoid dendritic cells is reversed by Tlr7 ligand treatment. Cancer Res (2013) 73(15):4629–40. doi: 10.1158/0008-5472.CAN-12-3058
26. Fridlender ZG, Albelda SM. Tumor-associated neutrophils: Friend or foe? Carcinogenesis (2012) 33(5):949–55. doi: 10.1093/carcin/bgs123
27. Schauer C, Janko C, Munoz LE, Zhao Y, Kienhofer D, Frey B, et al. Aggregated neutrophil extracellular traps limit inflammation by degrading cytokines and chemokines. Nat Med (2014) 20(5):511–7. doi: 10.1038/nm.3547
28. Langers I, Renoux VM, Thiry M, Delvenne P, Jacobs N. Natural killer cells: Role in local tumor growth and metastasis. Biologics (2012) 6:73–82. doi: 10.2147/BTT.S23976
29. Lee JC, Lee KM, Kim DW, Heo DS. Elevated tgf-Beta1 secretion and down-modulation of Nkg2d underlies impaired nk cytotoxicity in cancer patients. J Immunol (2004) 172(12):7335–40. doi: 10.4049/jimmunol.172.12.7335
30. Rouce RH, Shaim H, Sekine T, Weber G, Ballard B, Ku S, et al. The tgf-Beta/Smad pathway is an important mechanism for nk cell immune evasion in childhood b-acute lymphoblastic leukemia. Leukemia (2016) 30(4):800–11. doi: 10.1038/leu.2015.327
31. Castriconi R, Cantoni C, Della Chiesa M, Vitale M, Marcenaro E, Conte R, et al. Transforming growth factor beta 1 inhibits expression of Nkp30 and Nkg2d receptors: Consequences for the nk-mediated killing of dendritic cells. Proc Natl Acad Sci U.S.A. (2003) 100(7):4120–5. doi: 10.1073/pnas.0730640100
32. Varol C, Mildner A, Jung S. Macrophages: Development and tissue specialization. Annu Rev Immunol (2015) 33:643–75. doi: 10.1146/annurev-immunol-032414-112220
33. Gordon S. Phagocytosis: An immunobiologic process. Immunity (2016) 44(3):463–75. doi: 10.1016/j.immuni.2016.02.026
34. Mantovani A, Sica A, Sozzani S, Allavena P, Vecchi A, Locati M. The chemokine system in diverse forms of macrophage activation and polarization. Trends Immunol (2004) 25(12):677–86. doi: 10.1016/j.it.2004.09.015
35. Mills EL, Kelly B, Logan A, Costa ASH, Varma M, Bryant CE, et al. Succinate dehydrogenase supports metabolic repurposing of mitochondria to drive inflammatory macrophages. Cell (2016) 167(2):457–70.e13. doi: 10.1016/j.cell.2016.08.064
36. Tannahill GM, Curtis AM, Adamik J, Palsson-McDermott EM, McGettrick AF, Goel G, et al. Succinate is an inflammatory signal that induces il-1beta through hif-1alpha. Nature (2013) 496(7444):238–42. doi: 10.1038/nature11986
37. Rodriguez-Prados JC, Traves PG, Cuenca J, Rico D, Aragones J, Martin-Sanz P, et al. Substrate fate in activated macrophages: A comparison between innate, classic, and alternative activation. J Immunol (2010) 185(1):605–14. doi: 10.4049/jimmunol.0901698
38. Schultze JL, Schmidt SV. Molecular features of macrophage activation. Semin Immunol (2015) 27(6):416–23. doi: 10.1016/j.smim.2016.03.009
39. Fujiwara N, Kobayashi K. Macrophages in inflammation. Curr Drug Targets Inflammation Allergy (2005) 4(3):281–6. doi: 10.2174/1568010054022024
40. Wynn TA, Chawla A, Pollard JW. Macrophage biology in development, homeostasis and disease. Nature (2013) 496(7446):445–55. doi: 10.1038/nature12034
41. Tamura R, Tanaka T, Yamamoto Y, Akasaki Y, Sasaki H. Dual role of macrophage in tumor immunity. Immunotherapy (2018) 10(10):899–909. doi: 10.2217/imt-2018-0006
42. Wang N, Liang H, Zen K. Molecular mechanisms that influence the macrophage M1-M2 polarization balance. Front Immunol (2014) 5:614. doi: 10.3389/fimmu.2014.00614
43. Barros MH, Hauck F, Dreyer JH, Kempkes B, Niedobitek G. Macrophage polarisation: An immunohistochemical approach for identifying M1 and M2 macrophages. PloS One (2013) 8(11):e80908. doi: 10.1371/journal.pone.0080908
44. Holness CL, Simmons DL. Molecular cloning of Cd68, a human macrophage marker related to lysosomal glycoproteins. Blood (1993) 81(6):1607–13. doi: 10.1182/blood.V81.6.1607.1607
45. Khazen W, M’Bika JP, Tomkiewicz C, Benelli C, Chany C, Achour A, et al. Expression of macrophage-selective markers in human and rodent adipocytes. FEBS Lett (2005) 579(25):5631–4. doi: 10.1016/j.febslet.2005.09.032
46. Stoger JL, Gijbels MJ, van der Velden S, Manca M, van der Loos CM, Biessen EA, et al. Distribution of macrophage polarization markers in human atherosclerosis. Atherosclerosis (2012) 225(2):461–8. doi: 10.1016/j.atherosclerosis.2012.09.013
47. Mosser DM, Edwards JP. Exploring the full spectrum of macrophage activation. Nat Rev Immunol (2008) 8(12):958–69. doi: 10.1038/nri2448
48. Gensel JC, Kopper TJ, Zhang B, Orr MB, Bailey WM. Predictive screening of M1 and M2 macrophages reveals the immunomodulatory effectiveness of post spinal cord injury azithromycin treatment. Sci Rep (2017) 7:40144. doi: 10.1038/srep40144
49. Xu N, Tang XH, Pan W, Xie ZM, Zhang GF, Ji MH, et al. Spared nerve injury increases the expression of microglia M1 markers in the prefrontal cortex of rats and provokes depression-like behaviors. Front Neurosci (2017) 11:209. doi: 10.3389/fnins.2017.00209
50. Wang Y, Zhang Y, Wang Z, Zhang J, Qiao RR, Xu M, et al. Optical/Mri dual-modality imaging of M1 macrophage polarization in atherosclerotic plaque with Marco-targeted upconversion luminescence probe. Biomaterials (2019) 219:119378. doi: 10.1016/j.biomaterials.2019.119378
51. Jablonski KA, Amici SA, Webb LM, Ruiz-Rosado Jde D, Popovich PG, Partida-Sanchez S, et al. Novel markers to delineate murine M1 and M2 macrophages. PloS One (2015) 10(12):e0145342. doi: 10.1371/journal.pone.0145342
52. Jaguin M, Houlbert N, Fardel O, Lecureur V. Polarization profiles of human m-Csf-Generated macrophages and comparison of M1-markers in classically activated macrophages from gm-csf and m-csf origin. Cell Immunol (2013) 281(1):51–61. doi: 10.1016/j.cellimm.2013.01.010
53. Roszer T. Understanding the mysterious M2 macrophage through activation markers and effector mechanisms. Mediators Inflammation (2015) 2015:816460. doi: 10.1155/2015/816460
54. Gordon EJ, Rao S, Pollard JW, Nutt SL, Lang RA, Harvey NL. Macrophages define dermal lymphatic vessel calibre during development by regulating lymphatic endothelial cell proliferation. Development (2010) 137(22):3899–910. doi: 10.1242/dev.050021
55. Verreck FA, de Boer T, Langenberg DM, Hoeve MA, Kramer M, Vaisberg E, et al. Human il-23-Producing type 1 macrophages promote but il-10-Producing type 2 macrophages subvert immunity to (Myco)Bacteria. Proc Natl Acad Sci U.S.A. (2004) 101(13):4560–5. doi: 10.1073/pnas.0400983101
56. Bouhlel MA, Derudas B, Rigamonti E, Dievart R, Brozek J, Haulon S, et al. Ppargamma activation primes human monocytes into alternative M2 macrophages with anti-inflammatory properties. Cell Metab (2007) 6(2):137–43. doi: 10.1016/j.cmet.2007.06.010
57. Mantovani A, Marchesi F, Malesci A, Laghi L, Allavena P. Tumour-associated macrophages as treatment targets in oncology. Nat Rev Clin Oncol (2017) 14(7):399–416. doi: 10.1038/nrclinonc.2016.217
58. Allavena P, Sica A, Solinas G, Porta C, Mantovani A. The inflammatory micro-environment in tumor progression: The role of tumor-associated macrophages. Crit Rev Oncol Hematol (2008) 66(1):1–9. doi: 10.1016/j.critrevonc.2007.07.004
59. Genard G, Wera AC, Huart C, Le Calve B, Penninckx S, Fattaccioli A, et al. Proton irradiation orchestrates macrophage reprogramming through nfkappab signaling. Cell Death Dis (2018) 9(7):728. doi: 10.1038/s41419-018-0757-9
60. Martinez FO, Gordon S. The M1 and M2 paradigm of macrophage activation: Time for reassessment. F1000Prime Rep (2014) 6:13. doi: 10.12703/P6-13
61. Murray PJ, Allen JE, Biswas SK, Fisher EA, Gilroy DW, Goerdt S, et al. Macrophage activation and polarization: Nomenclature and experimental guidelines. Immunity (2014) 41(1):14–20. doi: 10.1016/j.immuni.2014.06.008
62. Xue J, Schmidt SV, Sander J, Draffehn A, Krebs W, Quester I, et al. Transcriptome-based network analysis reveals a spectrum model of human macrophage activation. Immunity (2014) 40(2):274–88. doi: 10.1016/j.immuni.2014.01.006
63. Lin F, Yin HB, Li XY, Zhu GM, He WY, Gou X. Bladder cancer cellsecreted exosomal Mir21 activates the Pi3k/Akt pathway in macrophages to promote cancer progression. Int J Oncol (2020) 56(1):151–64. doi: 10.3892/ijo.2019.4933
64. Wang D, Wang X, Si M, Yang J, Sun S, Wu H, et al. Exosome-encapsulated mirnas contribute to Cxcl12/Cxcr4-induced liver metastasis of colorectal cancer by enhancing M2 polarization of macrophages. Cancer Lett (2020) 474:36–52. doi: 10.1016/j.canlet.2020.01.005
65. Shou Y, Wang X, Chen C, Liang Y, Yang C, Xiao Q, et al. Exosomal mir-301a-3p from esophageal squamous cell carcinoma cells promotes angiogenesis by inducing M2 polarization of macrophages Via the Pten/Pi3k/Akt signaling pathway. Cancer Cell Int (2022) 22(1):153. doi: 10.1186/s12935-022-02570-6
66. Chen WX, Wang DD, Zhu B, Zhu YZ, Zheng L, Feng ZQ, et al. Exosomal mir-222 from adriamycin-resistant mcf-7 breast cancer cells promote macrophages M2 polarization Via Pten/Akt to induce tumor progression. Aging (Albany NY) (2021) 13(7):10415–30. doi: 10.18632/aging.202802
67. Chen J, Zhang K, Zhi Y, Wu Y, Chen B, Bai J, et al. Tumor-derived exosomal mir-19b-3p facilitates M2 macrophage polarization and exosomal Linc00273 secretion to promote lung adenocarcinoma metastasis Via hippo pathway. Clin Transl Med (2021) 11(9):e478. doi: 10.1002/ctm2.478
68. Yan X, Zhang S, Jia J, Yang J, Song Y, Duan H. Exosomal mir-423-3p inhibits macrophage M2 polarization to suppress the malignant progression of cervical cancer. Pathol Res Pract (2022) 235:153882. doi: 10.1016/j.prp.2022.153882
69. Xiao L, He Y, Peng F, Yang J, Yuan C. Endometrial cancer cells promote M2-like macrophage polarization by delivering exosomal mirna-21 under hypoxia condition. J Immunol Res (2020) 2020:9731049. doi: 10.1155/2020/9731049
70. Hsieh CH, Tai SK, Yang MH. Snail-overexpressing cancer cells promote M2-like polarization of tumor-associated macrophages by delivering mir-21-Abundant exosomes. Neoplasia (2018) 20(8):775–88. doi: 10.1016/j.neo.2018.06.004
71. Xun J, Du L, Gao R, Shen L, Wang D, Kang L, et al. Cancer-derived exosomal mir-138-5p modulates polarization of tumor-associated macrophages through inhibition of Kdm6b. Theranostics (2021) 11(14):6847–59. doi: 10.7150/thno.51864
72. Liu J, Luo R, Wang J, Luan X, Wu D, Chen H, et al. Tumor cell-derived exosomal mir-770 inhibits M2 macrophage polarization Via targeting Map3k1 to inhibit the invasion of non-small cell lung cancer cells. Front Cell Dev Biol (2021) 9:679658. doi: 10.3389/fcell.2021.679658
73. Moradi-Chaleshtori M, Shojaei S, Mohammadi-Yeganeh S, Hashemi SM. Transfer of mirna in tumor-derived exosomes suppresses breast tumor cell invasion and migration by inducing M1 polarization in macrophages. Life Sci (2021) 282:119800. doi: 10.1016/j.lfs.2021.119800
74. Tong F, Mao X, Zhang S, Xie H, Yan B, Wang B, et al. Hpv + hnscc-derived exosomal mir-9 induces macrophage M1 polarization and increases tumor radiosensitivity. Cancer Lett (2020) 478:34–44. doi: 10.1016/j.canlet.2020.02.037
75. Chen Y, Hong C, Qu J, Chen J, Qin Z. Knockdown of lncrna Pcat6 suppresses the growth of non-small cell lung cancer cells by inhibiting macrophages M2 polarization Via mir-326/Klf1 axis. Bioengineered (2022) 13(5):12834–46. doi: 10.1080/21655979.2022.2076388
76. Zhang W, Zheng X, Yu Y, Zheng L, Lan J, Wu Y, et al. Renal cell carcinoma-derived exosomes deliver lncarsr to induce macrophage polarization and promote tumor progression Via Stat3 pathway. Int J Biol Sci (2022) 18(8):3209–22. doi: 10.7150/ijbs.70289
77. Wang X, Zhou Y, Dong K, Zhang H, Gong J, Wang S. Exosomal lncrna hmmr-As1 mediates macrophage polarization through mir-147a/Arid3a axis under hypoxia and affects the progression of hepatocellular carcinoma. Environ Toxicol (2022) 37(6):1357–72. doi: 10.1002/tox.23489
78. Yao H, Tian L, Yan B, Yang L, Li Y. Lncrna Tp73-As1 promotes nasopharyngeal carcinoma progression through targeting mir-342-3p and M2 polarization Via exosomes. Cancer Cell Int (2022) 22(1):16. doi: 10.1186/s12935-021-02418-5
79. He Z, Wang J, Zhu C, Xu J, Chen P, Jiang X, et al. Exosome-derived Fgd5-As1 promotes tumor-associated macrophage M2 polarization-mediated pancreatic cancer cell proliferation and metastasis. Cancer Lett (2022) 548:215751. doi: 10.1016/j.canlet.2022.215751
80. Wang B, Wang X, Li P, Niu X, Liang X, Liu G, et al. Osteosarcoma cell-derived exosomal Elfn1-As1 mediates macrophage M2 polarization Via sponging mir-138-5p and mir-1291 to promote the tumorgenesis of osteosarcoma. Front Oncol (2022) 12:881022. doi: 10.3389/fonc.2022.881022
81. Xin L, Wu Y, Liu C, Zeng F, Wang JL, Wu DZ, et al. Exosome-mediated transfer of lncrna Hcg18 promotes M2 macrophage polarization in gastric cancer. Mol Immunol (2021) 140:196–205. doi: 10.1016/j.molimm.2021.10.011
82. Lu Q, Wang X, Zhu J, Fei X, Chen H, Li C. Hypoxic tumor-derived exosomal Circ0048117 facilitates M2 macrophage polarization acting as mir-140 sponge in esophageal squamous cell carcinoma. Onco Targets Ther (2020) 13:11883–97. doi: 10.2147/OTT.S284192
83. Song J, Xu X, He S, Wang N, Bai Y, Li B, et al. Exosomal Hsa_Circ_0017252 attenuates the development of gastric cancer Via inhibiting macrophage M2 polarization. Hum Cell (2022) 35(5):1499–511. doi: 10.1007/s13577-022-00739-9
84. Chen T, Liu Y, Li C, Xu C, Ding C, Chen J, et al. Tumor-derived exosomal circfarsa mediates M2 macrophage polarization Via the Pten/Pi3k/Akt pathway to promote non-small cell lung cancer metastasis. Cancer Treat Res Commun (2021) 28:100412. doi: 10.1016/j.ctarc.2021.100412
85. Lu C, Shi W, Hu W, Zhao Y, Zhao X, Dong F, et al. Endoplasmic reticulum stress promotes breast cancer cells to release exosomes Circ_0001142 and induces M2 polarization of macrophages to regulate tumor progression. Pharmacol Res (2022) 177:106098. doi: 10.1016/j.phrs.2022.106098
86. Liu Y, Li L, Song X. Exosomal Circpvt1 derived from lung cancer promotes the progression of lung cancer by targeting mir-124-3p/Ezh2 axis and regulating macrophage polarization. Cell Cycle (2022) 21(5):514–30. doi: 10.1080/15384101.2021.2024997
87. Huang X, Wang J, Guan J, Zheng Z, Hao J, Sheng Z, et al. Exosomal Circsafb2 reshaping tumor environment to promote renal cell carcinoma progression by mediating M2 macrophage polarization. Front Oncol (2022) 12:808888. doi: 10.3389/fonc.2022.808888
88. Pan Z, Zhao R, Li B, Qi Y, Qiu W, Guo Q, et al. Ewsr1-induced Circneil3 promotes glioma progression and exosome-mediated macrophage immunosuppressive polarization Via stabilizing Igf2bp3. Mol Cancer (2022) 21(1):16. doi: 10.1186/s12943-021-01485-6
89. Park JE, Dutta B, Tse SW, Gupta N, Tan CF, Low JK, et al. Hypoxia-induced tumor exosomes promote M2-like macrophage polarization of infiltrating myeloid cells and microrna-mediated metabolic shift. Oncogene (2019) 38(26):5158–73. doi: 10.1038/s41388-019-0782-x
90. Ling HY, Yang Z, Wang PJ, Sun Y, Ju SG, Li J, et al. Diffuse Large b-cell lymphoma-derived exosomes push macrophage polarization toward M2 phenotype Via Gp130/Stat3 signaling pathway. Chem Biol Interact (2022) 352:109779. doi: 10.1016/j.cbi.2021.109779
91. Zhao S, Liu Y, He L, Li Y, Lin K, Kang Q, et al. Gallbladder cancer cell-derived exosome-mediated transfer of leptin promotes cell invasion and migration by modulating Stat3-mediated M2 macrophage polarization. Anal Cell Pathol (Amst) (2022) 2022:9994906. doi: 10.1155/2022/9994906
92. Dong H, Xie C, Jiang Y, Li K, Lin Y, Pang X, et al. Tumor-derived exosomal protein tyrosine phosphatase receptor type O polarizes macrophage to suppress breast tumor cell invasion and migration. Front Cell Dev Biol (2021) 9:703537. doi: 10.3389/fcell.2021.703537
93. Yu C, Xue B, Li J, Zhang Q. Tumor cell-derived exosome Rnf126 affects the immune microenvironment and promotes nasopharyngeal carcinoma progression by regulating pten ubiquitination. Apoptosis (2022) 27(7-8):590–605. doi: 10.1007/s10495-022-01738-9
94. Guo E, Mao X, Wang X, Guo L, An C, Zhang C, et al. Alternatively spliced anln isoforms synergistically contribute to the progression of head and neck squamous cell carcinoma. Cell Death Dis (2021) 12(8):764. doi: 10.1038/s41419-021-04063-2
95. Cheng Z, Wang L, Wu C, Huang L, Ruan Y, Xue W. Tumor-derived exosomes induced M2 macrophage polarization and promoted the metastasis of osteosarcoma cells through Tim-3. Arch Med Res (2021) 52(2):200–10. doi: 10.1016/j.arcmed.2020.10.018
96. Li X, Liu Y, Yang L, Jiang Y, Qian Q. Tim-3 shuttled by Mv3 cells-secreted exosomes inhibits Cd4(+) T cell immune function and induces macrophage M2 polarization to promote the growth and metastasis of melanoma cells. Transl Oncol (2022) 18:101334. doi: 10.1016/j.tranon.2021.101334
97. Yuan Y, Jiao P, Wang Z, Chen M, Du H, Xu L, et al. Endoplasmic reticulum stress promotes the release of exosomal pd-L1 from head and neck cancer cells and facilitates M2 macrophage polarization. Cell Commun Signal (2022) 20(1):12. doi: 10.1186/s12964-021-00810-2
98. Linton SS, Abraham T, Liao J, Clawson GA, Butler PJ, Fox T, et al. Tumor-promoting effects of pancreatic cancer cell exosomes on thp-1-Derived macrophages. PloS One (2018) 13(11):e0206759. doi: 10.1371/journal.pone.0206759
99. Tian HY, Liang Q, Shi Z, Zhao H. Exosomal Cxcl14 contributes to M2 macrophage polarization through nf-kappab signaling in prostate cancer. Oxid Med Cell Longev (2022) 2022:7616696. doi: 10.1155/2022/7616696
100. Biswas SK, Lewis CE. Nf-kappab as a central regulator of macrophage function in tumors. J Leukoc Biol (2010) 88(5):877–84. doi: 10.1189/jlb.0310153
101. Lu H, Bowler N, Harshyne LA, Craig Hooper D, Krishn SR, Kurtoglu S, et al. Exosomal Alphavbeta6 integrin is required for monocyte M2 polarization in prostate cancer. Matrix Biol (2018) 70:20–35. doi: 10.1016/j.matbio.2018.03.009
102. Tacke RS, Tosello-Trampont A, Nguyen V, Mullins DW, Hahn YS. Extracellular hepatitis c virus core protein activates Stat3 in human Monocytes/Macrophages/Dendritic cells Via an il-6 autocrine pathway. J Biol Chem (2011) 286(12):10847–55. doi: 10.1074/jbc.M110.217653
103. Sica A, Bronte V. Altered macrophage differentiation and immune dysfunction in tumor development. J Clin Invest (2007) 117(5):1155–66. doi: 10.1172/JCI31422
104. Dong R, Zhang B, Tan B, Lin N. Long non-coding rnas as the regulators and targets of macrophage M2 polarization. Life Sci (2021) 266:118895. doi: 10.1016/j.lfs.2020.118895
105. Li Z, Meng X, Wu P, Zha C, Han B, Li L, et al. Glioblastoma cell-derived lncrna-containing exosomes induce microglia to produce complement C5, promoting chemotherapy resistance. Cancer Immunol Res (2021) 9(12):1383–99. doi: 10.1158/2326-6066.CIR-21-0258
106. Kang M, Ren M, Li Y, Fu Y, Deng M, Li C. Exosome-mediated transfer of lncrna Part1 induces gefitinib resistance in esophageal squamous cell carcinoma Via functioning as a competing endogenous rna. J Exp Clin Cancer Res (2018) 37(1):171. doi: 10.1186/s13046-018-0845-9
107. Wing JB, Tay C, Sakaguchi S. Control of regulatory T cells by Co-signal molecules. Adv Exp Med Biol (2019) 1189:179–210. doi: 10.1007/978-981-32-9717-3_7
108. Li X, Lu H, Gu Y, Zhang X, Zhang G, Shi T, et al. Tim-3 suppresses the killing effect of Vgamma9vdelta2t cells on colon cancer cells by reducing perforin and granzyme b expression. Exp Cell Res (2020) 386(1):111719. doi: 10.1016/j.yexcr.2019.111719
109. DeNardo DG, Ruffell B. Macrophages as regulators of tumour immunity and immunotherapy. Nat Rev Immunol (2019) 19(6):369–82. doi: 10.1038/s41577-019-0127-6
110. Iliopoulos D, Hirsch HA, Struhl K. An epigenetic switch involving nf-kappab, Lin28, let-7 microrna, and Il6 links inflammation to cell transformation. Cell (2009) 139(4):693–706. doi: 10.1016/j.cell.2009.10.014
111. Jinushi M, Chiba S, Yoshiyama H, Masutomi K, Kinoshita I, Dosaka-Akita H, et al. Tumor-associated macrophages regulate tumorigenicity and anticancer drug responses of cancer Stem/Initiating cells. Proc Natl Acad Sci U.S.A. (2011) 108(30):12425–30. doi: 10.1073/pnas.1106645108
112. Wu L, Zhang X, Zhang B, Shi H, Yuan X, Sun Y, et al. Exosomes derived from gastric cancer cells activate nf-kappab pathway in macrophages to promote cancer progression. Tumour Biol (2016) 37(9):12169–80. doi: 10.1007/s13277-016-5071-5
113. Chow A, Zhou W, Liu L, Fong MY, Champer J, Van Haute D, et al. Macrophage immunomodulation by breast cancer-derived exosomes requires toll-like receptor 2-mediated activation of nf-kappab. Sci Rep (2014) 4:5750. doi: 10.1038/srep05750
114. Czernek L, Duchler M. Functions of cancer-derived extracellular vesicles in immunosuppression. Arch Immunol Ther Exp (Warsz) (2017) 65(4):311–23. doi: 10.1007/s00005-016-0453-3
115. Fabbri M, Paone A, Calore F, Galli R, Gaudio E, Santhanam R, et al. Micrornas bind to toll-like receptors to induce prometastatic inflammatory response. Proc Natl Acad Sci U.S.A. (2012) 109(31):E2110–6. doi: 10.1073/pnas.1209414109
116. Li Z, He F, Yang Z, Cao X, Dai S, Zou J, et al. Retracted article: Exosomal mir-25-3p derived from hypoxia tumor mediates il-6 secretion and stimulates cell viability and migration in breast cancer. RSC Adv (2019) 9(3):1451–9. doi: 10.1039/c8ra06750c
117. Cheng AN, Cheng LC, Kuo CL, Lo YK, Chou HY, Chen CH, et al. Mitochondrial lon-induced mtdna leakage contributes to pd-L1-Mediated immunoescape Via sting-ifn signaling and extracellular vesicles. J Immunother Cancer (2020) 8(2):e001372. doi: 10.1136/jitc-2020-001372
118. Ham S, Lima LG, Chai EPZ, Muller A, Lobb RJ, Krumeich S, et al. Breast cancer-derived exosomes alter macrophage polarization Via Gp130/Stat3 signaling. Front Immunol (2018) 9:871. doi: 10.3389/fimmu.2018.00871
119. He C, Hua W, Liu J, Fan L, Wang H, Sun G. Exosomes derived from endoplasmic reticulum-stressed liver cancer cells enhance the expression of cytokines in macrophages Via the Stat3 signaling pathway. Oncol Lett (2020) 20(1):589–600. doi: 10.3892/ol.2020.11609
120. Marton A, Vizler C, Kusz E, Temesfoi V, Szathmary Z, Nagy K, et al. Melanoma cell-derived exosomes alter macrophage and dendritic cell functions in vitro. Immunol Lett (2012) 148(1):34–8. doi: 10.1016/j.imlet.2012.07.006
121. Guo J, Duan Z, Zhang C, Wang W, He H, Liu Y, et al. Mouse 4t1 breast cancer cell-derived exosomes induce proinflammatory cytokine production in macrophages Via mir-183. J Immunol (2020) 205(10):2916–25. doi: 10.4049/jimmunol.1901104
122. Ferrari L, Cafora M, Rota F, Hoxha M, Iodice S, Tarantini L, et al. Extracellular vesicles released by colorectal cancer cell lines modulate innate immune response in zebrafish model: The possible role of human endogenous retroviruses. Int J Mol Sci (2019) 20(15):3669. doi: 10.3390/ijms20153669
123. Bottino L, Rodrigues-Junior DM, Farias IS, Branco LM, Iyer NG, de Albuquerque GE, et al. Extracellular vesicles derived from head and neck squamous cells carcinoma inhibit Nlrp3 inflammasomes. Curr Res Immunol (2021) 2:175–83. doi: 10.1016/j.crimmu.2021.10.005
124. Li J, Xu P, Wu D, Guan M, Weng X, Lu Y, et al. Hypoxic stress suppresses lung tumor-secreted exosomal Mir101 to activate macrophages and induce inflammation. Cell Death Dis (2021) 12(8):776. doi: 10.1038/s41419-021-04030-x
125. Li X, Lei Y, Wu M, Li N. Regulation of macrophage activation and polarization by hcc-derived exosomal lncrna Tuc339. Int J Mol Sci (2018) 19(10):2958. doi: 10.3390/ijms19102958
126. Wolf-Dennen K, Gordon N, Kleinerman ES. Exosomal communication by metastatic osteosarcoma cells modulates alveolar macrophages to an M2 tumor-promoting phenotype and inhibits tumoricidal functions. Oncoimmunology (2020) 9(1):1747677. doi: 10.1080/2162402X.2020.1747677
127. Gregory CD, Dransfield I. Apoptotic tumor cell-derived extracellular vesicles as important regulators of the onco-regenerative niche. Front Immunol (2018) 9:1111. doi: 10.3389/fimmu.2018.01111
128. Gao WJ, Liu JX, Liu MN, Yao YD, Liu ZQ, Liu L, et al. Macrophage 3d migration: A potential therapeutic target for inflammation and deleterious progression in diseases. Pharmacol Res (2021) 167:105563. doi: 10.1016/j.phrs.2021.105563
129. Purushothaman A, Bandari SK, Chandrashekar DS, Jones RJ, Lee HC, Weber DM, et al. Chondroitin sulfate proteoglycan serglycin influences protein cargo loading and functions of tumor-derived exosomes. Oncotarget (2017) 8(43):73723–32. doi: 10.18632/oncotarget.20564
130. Feng L, Weng J, Yao C, Wang R, Wang N, Zhang Y, et al. Extracellular vesicles derived from Sipa1(High) breast cancer cells enhance macrophage infiltration and cancer metastasis through myosin-9. Biol (Basel) (2022) 11(4):543. doi: 10.3390/biology11040543
131. Balan S, Saxena M, Bhardwaj N. Dendritic cell subsets and locations. Int Rev Cell Mol Biol (2019) 348:1–68. doi: 10.1016/bs.ircmb.2019.07.004
132. Collin M, Bigley V. Human dendritic cell subsets: An update. Immunology (2018) 154(1):3–20. doi: 10.1111/imm.12888
133. Iwasaki A, Medzhitov R. Control of adaptive immunity by the innate immune system. Nat Immunol (2015) 16(4):343–53. doi: 10.1038/ni.3123
134. Squadrito ML, Cianciaruso C, Hansen SK, De Palma M. Evir: Chimeric receptors that enhance dendritic cell cross-dressing with tumor antigens. Nat Methods (2018) 15(3):183–6. doi: 10.1038/nmeth.4579
135. Rao Q, Zuo B, Lu Z, Gao X, You A, Wu C, et al. Tumor-derived exosomes elicit tumor suppression in murine hepatocellular carcinoma models and humans in vitro. Hepatology (2016) 64(2):456–72. doi: 10.1002/hep.28549
136. Napoletano C, Rughetti A, Landi R, Pinto D, Bellati F, Rahimi H, et al. Immunogenicity of allo-vesicle carrying Erbb2 tumor antigen for dendritic cell-based anti-tumor immunotherapy. Int J Immunopathol Pharmacol (2009) 22(3):647–58. doi: 10.1177/039463200902200310
137. Ito T, Sugiura K, Hasegawa A, Ouchi W, Yoshimoto T, Mizoguchi I, et al. Microbial antigen-presenting extracellular vesicles derived from genetically modified tumor cells promote antitumor activity of dendritic cells. Pharmaceutics (2021) 13(1):57. doi: 10.3390/pharmaceutics13010057
138. Wolfers J, Lozier A, Raposo G, Regnault A, Thery C, Masurier C, et al. Tumor-derived exosomes are a source of shared tumor rejection antigens for ctl cross-priming. Nat Med (2001) 7(3):297–303. doi: 10.1038/85438
139. Ning Y, Shen K, Wu Q, Sun X, Bai Y, Xie Y, et al. Tumor exosomes block dendritic cells maturation to decrease the T cell immune response. Immunol Lett (2018) 199:36–43. doi: 10.1016/j.imlet.2018.05.002
140. Shen Y, Guo D, Weng L, Wang S, Ma Z, Yang Y, et al. Tumor-derived exosomes educate dendritic cells to promote tumor metastasis Via Hsp72/Hsp105-Tlr2/Tlr4 pathway. Oncoimmunology (2017) 6(12):e1362527. doi: 10.1080/2162402X.2017.1362527
141. Augoff K, Hryniewicz-Jankowska A, Tabola R, Stach K. Mmp9: A tough target for targeted therapy for cancer. Cancers (Basel) (2022) 14(7):1847. doi: 10.3390/cancers14071847
142. Salimu J, Webber J, Gurney M, Al-Taei S, Clayton A, Tabi Z. Dominant immunosuppression of dendritic cell function by prostate-Cancer-Derived exosomes. J Extracell Vesicles (2017) 6(1):1368823. doi: 10.1080/20013078.2017.1368823
143. Ding G, Zhou L, Qian Y, Fu M, Chen J, Chen J, et al. Pancreatic cancer-derived exosomes transfer mirnas to dendritic cells and inhibit rfxap expression Via mir-212-3p. Oncotarget (2015) 6(30):29877–88. doi: 10.18632/oncotarget.4924
144. de Paula Silva E, Marti LC, Andreghetto FM, de Sales RO, Hoberman M, Dos Santos Dias B, et al. Extracellular vesicles cargo from head and neck cancer cell lines disrupt dendritic cells function and match plasma micrornas. Sci Rep (2021) 11(1):18534. doi: 10.1038/s41598-021-97753-y
145. Wieckowski E, Whiteside TL. Human tumor-derived vs dendritic cell-derived exosomes have distinct biologic roles and molecular profiles. Immunol Res (2006) 36(1-3):247–54. doi: 10.1385/IR:36:1:247
146. Grange C, Tapparo M, Tritta S, Deregibus MC, Battaglia A, Gontero P, et al. Role of hla-G and extracellular vesicles in renal cancer stem cell-induced inhibition of dendritic cell differentiation. BMC Cancer (2015) 15:1009. doi: 10.1186/s12885-015-2025-z
147. Valenti R, Huber V, Filipazzi P, Pilla L, Sovena G, Villa A, et al. Human tumor-released microvesicles promote the differentiation of myeloid cells with transforming growth factor-Beta-Mediated suppressive activity on T lymphocytes. Cancer Res (2006) 66(18):9290–8. doi: 10.1158/0008-5472.CAN-06-1819
148. Yu S, Liu C, Su K, Wang J, Liu Y, Zhang L, et al. Tumor exosomes inhibit differentiation of bone marrow dendritic cells. J Immunol (2007) 178(11):6867–75. doi: 10.4049/jimmunol.178.11.6867
149. Yang C, Kim SH, Bianco NR, Robbins PD. Tumor-derived exosomes confer antigen-specific immunosuppression in a murine delayed-type hypersensitivity model. PloS One (2011) 6(8):e22517. doi: 10.1371/journal.pone.0022517
150. Gassmann H, Schneider K, Evdokimova V, Ruzanov P, Schober SJ, Xue B, et al. Ewing Sarcoma-derived extracellular vesicles impair dendritic cell maturation and function. Cells (2021) 10(8):2081. doi: 10.3390/cells10082081
151. Sheybani ND, Batts AJ, Mathew AS, Thim EA, Price RJ. Focused ultrasound hyperthermia augments release of glioma-derived extracellular vesicles with differential immunomodulatory capacity. Theranostics (2020) 10(16):7436–47. doi: 10.7150/thno.46534
152. Rosales C. Neutrophils at the crossroads of innate and adaptive immunity. J Leukoc Biol (2020) 108(1):377–96. doi: 10.1002/JLB.4MIR0220-574RR
153. Raftopoulou S, Valadez-Cosmes P, Mihalic ZN, Schicho R, Kargl J. Tumor-mediated neutrophil polarization and therapeutic implications. Int J Mol Sci (2022) 23(6):3218. doi: 10.3390/ijms23063218
154. Shaul ME, Fridlender ZG. Cancer-related circulating and tumor-associated neutrophils - subtypes, sources and function. FEBS J (2018) 285(23):4316–42. doi: 10.1111/febs.14524
155. Thomas GM, Brill A, Mezouar S, Crescence L, Gallant M, Dubois C, et al. Tissue factor expressed by circulating cancer cell-derived microparticles drastically increases the incidence of deep vein thrombosis in mice. J Thromb Haemost (2015) 13(7):1310–9. doi: 10.1111/jth.13002
156. Leal AC, Mizurini DM, Gomes T, Rochael NC, Saraiva EM, Dias MS, et al. Tumor-derived exosomes induce the formation of neutrophil extracellular traps: Implications for the establishment of cancer-associated thrombosis. Sci Rep (2017) 7(1):6438. doi: 10.1038/s41598-017-06893-7
157. Chennakrishnaiah S, Meehan B, D’Asti E, Montermini L, Lee TH, Karatzas N, et al. Leukocytes as a reservoir of circulating oncogenic DNA and regulatory targets of tumor-derived extracellular vesicles. J Thromb Haemost (2018) 16(9):1800–13. doi: 10.1111/jth.14222
158. Shang A, Gu C, Wang W, Wang X, Sun J, Zeng B, et al. Exosomal circpacrgl promotes progression of colorectal cancer Via the mir-142-3p/Mir-506-3p- tgf-Beta1 axis. Mol Cancer (2020) 19(1):117. doi: 10.1186/s12943-020-01235-0
159. Zhang X, Shi H, Yuan X, Jiang P, Qian H, Xu W. Tumor-derived exosomes induce N2 polarization of neutrophils to promote gastric cancer cell migration. Mol Cancer (2018) 17(1):146. doi: 10.1186/s12943-018-0898-6
160. Hwang WL, Lan HY, Cheng WC, Huang SC, Yang MH. Tumor stem-like cell-derived exosomal rnas prime neutrophils for facilitating tumorigenesis of colon cancer. J Hematol Oncol (2019) 12(1):10. doi: 10.1186/s13045-019-0699-4
161. Han Q, Zhao H, Jiang Y, Yin C, Zhang J. Hcc-derived exosomes: Critical player and target for cancer immune escape. Cells (2019) 8(6):558. doi: 10.3390/cells8060558
162. Ludwig N, Yerneni SS, Azambuja JH, Gillespie DG, Menshikova EV, Jackson EK, et al. Tumor-derived exosomes promote angiogenesis Via adenosine A2b receptor signaling. Angiogenesis (2020) 23(4):599–610. doi: 10.1007/s10456-020-09728-8
163. Cherrier DE, Serafini N, Di Santo JP. Innate lymphoid cell development: A T cell perspective. Immunity (2018) 48(6):1091–103. doi: 10.1016/j.immuni.2018.05.010
164. Zhang Y, Wallace DL, de Lara CM, Ghattas H, Asquith B, Worth A, et al. In vivo kinetics of human natural killer cells: The effects of ageing and acute and chronic viral infection. Immunology (2007) 121(2):258–65. doi: 10.1111/j.1365-2567.2007.02573.x
165. Kumar S. Natural killer cell cytotoxicity and its regulation by inhibitory receptors. Immunology (2018) 154(3):383–93. doi: 10.1111/imm.12921
166. Kim S, Poursine-Laurent J, Truscott SM, Lybarger L, Song YJ, Yang L, et al. Licensing of natural killer cells by host major histocompatibility complex class I molecules. Nature (2005) 436(7051):709–13. doi: 10.1038/nature03847
167. Malmberg KJ, Carlsten M, Bjorklund A, Sohlberg E, Bryceson YT, Ljunggren HG. Natural killer cell-mediated immunosurveillance of human cancer. Semin Immunol (2017) 31:20–9. doi: 10.1016/j.smim.2017.08.002
168. Wu SY, Fu T, Jiang YZ, Shao ZM. Natural killer cells in cancer biology and therapy. Mol Cancer (2020) 19(1):120. doi: 10.1186/s12943-020-01238-x
169. Luetke-Eversloh M, Cicek BB, Siracusa F, Thom JT, Hamann A, Frischbutter S, et al. Nk cells gain higher ifn-gamma competence during terminal differentiation. Eur J Immunol (2014) 44(7):2074–84. doi: 10.1002/eji.201344072
170. Vivier E, Raulet DH, Moretta A, Caligiuri MA, Zitvogel L, Lanier LL, et al. Innate or adaptive immunity? the example of natural killer cells. Science (2011) 331(6013):44–9. doi: 10.1126/science.1198687
171. Clayton A, Mitchell JP, Court J, Linnane S, Mason MD, Tabi Z. Human tumor-derived exosomes down-modulate Nkg2d expression. J Immunol (2008) 180(11):7249–58. doi: 10.4049/jimmunol.180.11.7249
172. Hedlund M, Nagaeva O, Kargl D, Baranov V, Mincheva-Nilsson L. Thermal- and oxidative stress causes enhanced release of Nkg2d ligand-bearing immunosuppressive exosomes in Leukemia/Lymphoma T and b cells. PloS One (2011) 6(2):e16899. doi: 10.1371/journal.pone.0016899
173. Hong CS, Sharma P, Yerneni SS, Simms P, Jackson EK, Whiteside TL, et al. Circulating exosomes carrying an immunosuppressive cargo interfere with cellular immunotherapy in acute myeloid leukemia. Sci Rep (2017) 7(1):14684. doi: 10.1038/s41598-017-14661-w
174. Szczepanski MJ, Szajnik M, Welsh A, Whiteside TL, Boyiadzis M. Blast-derived microvesicles in sera from patients with acute myeloid leukemia suppress natural killer cell function Via membrane-associated transforming growth factor-Beta1. Haematologica (2011) 96(9):1302–9. doi: 10.3324/haematol.2010.039743
175. Hong CS, Danet-Desnoyers G, Shan X, Sharma P, Whiteside TL, Boyiadzis M. Human acute myeloid leukemia blast-derived exosomes in patient-derived xenograft mice mediate immune suppression. Exp Hematol (2019) 76:60–6.e2. doi: 10.1016/j.exphem.2019.07.005
176. Ludwig S, Floros T, Theodoraki MN, Hong CS, Jackson EK, Lang S, et al. Suppression of lymphocyte functions by plasma exosomes correlates with disease activity in patients with head and neck cancer. Clin Cancer Res (2017) 23(16):4843–54. doi: 10.1158/1078-0432.CCR-16-2819
177. Sharma P, Diergaarde B, Ferrone S, Kirkwood JM, Whiteside TL. Melanoma cell-derived exosomes in plasma of melanoma patients suppress functions of immune effector cells. Sci Rep (2020) 10(1):92. doi: 10.1038/s41598-019-56542-4
178. Zhao J, Schlosser HA, Wang Z, Qin J, Li J, Popp F, et al. Tumor-derived extracellular vesicles inhibit natural killer cell function in pancreatic cancer. Cancers (Basel) (2019) 11(6):874. doi: 10.3390/cancers11060874
179. Xia Y, Zhang Q, Zhen Q, Zhao Y, Liu N, Li T, et al. Negative regulation of tumor-infiltrating nk cell in clear cell renal cell carcinoma patients through the exosomal pathway. Oncotarget (2017) 8(23):37783–95. doi: 10.18632/oncotarget.16354
180. Reiners KS, Topolar D, Henke A, Simhadri VR, Kessler J, Sauer M, et al. Soluble ligands for nk cell receptors promote evasion of chronic lymphocytic leukemia cells from nk cell anti-tumor activity. Blood (2013) 121(18):3658–65. doi: 10.1182/blood-2013-01-476606
181. Li Q, Huang Q, Huyan T, Wang Y, Huang Q, Shi J. Bifacial effects of engineering tumour cell-derived exosomes on human natural killer cells. Exp Cell Res (2018) 363(2):141–50. doi: 10.1016/j.yexcr.2017.12.005
182. Moloudizargari M, Redegeld F, Asghari MH, Mosaffa N, Mortaz E. Long-chain polyunsaturated omega-3 fatty acids reduce multiple myeloma exosome-mediated suppression of nk cell cytotoxicity. Daru (2020) 28(2):647–59. doi: 10.1007/s40199-020-00372-7
183. Huang Y, Luo Y, Ou W, Wang Y, Dong D, Peng X, et al. Exosomal lncrna Snhg10 derived from colorectal cancer cells suppresses natural killer cell cytotoxicity by upregulating inhbc. Cancer Cell Int (2021) 21(1):528. doi: 10.1186/s12935-021-02221-2
184. Briand J, Garnier D, Nadaradjane A, Clement-Colmou K, Potiron V, Supiot S, et al. Radiotherapy-induced overexpression of exosomal mirna-378a-3p in cancer cells limits natural killer cells cytotoxicity. Epigenomics (2020) 12(5):397–408. doi: 10.2217/epi-2019-0193
185. Zhang PF, Gao C, Huang XY, Lu JC, Guo XJ, Shi GM, et al. Cancer cell-derived exosomal Circuhrf1 induces natural killer cell exhaustion and may cause resistance to anti-Pd1 therapy in hepatocellular carcinoma. Mol Cancer (2020) 19(1):110. doi: 10.1186/s12943-020-01222-5
186. Ho J, Chaiswing L, St Clair DK. Extracellular vesicles and cancer therapy: Insights into the role of oxidative stress. Antioxidants (Basel) (2022) 11(6):1194. doi: 10.3390/antiox11061194
187. Li Y, Zhao W, Wang Y, Wang H, Liu S. Extracellular vesicle-mediated crosstalk between pancreatic cancer and stromal cells in the tumor microenvironment. J Nanobiotechnol (2022) 20(1):208. doi: 10.1186/s12951-022-01382-0
188. Becker A, Thakur BK, Weiss JM, Kim HS, Peinado H, Lyden D. Extracellular vesicles in cancer: Cell-to-Cell mediators of metastasis. Cancer Cell (2016) 30(6):836–48. doi: 10.1016/j.ccell.2016.10.009
189. Lipinski S, Tiemann K. Extracellular vesicles and their role in the spatial and temporal expansion of tumor-immune interactions. Int J Mol Sci (2021) 22(7). doi: 10.3390/ijms22073374
190. Catoni C, Di Paolo V, Rossi E, Quintieri L, Zamarchi R. Cell-secreted vesicles: Novel opportunities in cancer diagnosis, monitoring and treatment. Diagnostics (Basel) (2021) 11(6):1118. doi: 10.3390/diagnostics11061118
191. Kosaka N, Yoshioka Y, Fujita Y, Ochiya T. Versatile roles of extracellular vesicles in cancer. J Clin Invest (2016) 126(4):1163–72. doi: 10.1172/JCI81130
Keywords: macrophages, dendritic cells, neutrophils, natural killer cells, tumor-derived extracellular vesicles (TDEVs), tumor progression
Citation: Wang S, Sun J, Dastgheyb RM and Li Z (2022) Tumor-derived extracellular vesicles modulate innate immune responses to affect tumor progression. Front. Immunol. 13:1045624. doi: 10.3389/fimmu.2022.1045624
Received: 15 September 2022; Accepted: 18 October 2022;
Published: 02 November 2022.
Edited by:
Alexei Gratchev, Russian Cancer Research Center NN Blokhin, RussiaReviewed by:
Raphael Pollock, The Ohio State University, United StatesCarlos Cabañas, Spanish National Research Council (CSIC), Spain
Copyright © 2022 Wang, Sun, Dastgheyb and Li. This is an open-access article distributed under the terms of the Creative Commons Attribution License (CC BY). The use, distribution or reproduction in other forums is permitted, provided the original author(s) and the copyright owner(s) are credited and that the original publication in this journal is cited, in accordance with accepted academic practice. No use, distribution or reproduction is permitted which does not comply with these terms.
*Correspondence: Zhigang Li, bGl6aGcyNUBtYWlsLnN5c3UuZWR1LmNu
†These authors have contributed equally to this work