- 1Laboratório de Imunobiologia, Departamento de Microbiologia, Imunologia e Parasitologia, Universidade Federal de Santa Catarina, Florianópolis, Brazil
- 2Department of Pathology, University of Cambridge, Cambridge, United Kingdom
- 3Laboratório de Farmacologia e Bioquímica do Câncer, Departamento de Farmacologia, Universidade Federal de Santa Catarina, Florianópolis, Brazil
Zika virus (ZIKV) is a single-strand RNA mosquito-borne flavivirus with significant public health impact. ZIKV infection induces double-strand DNA breaks (DSBs) in human neural progenitor cells that may contribute to severe neuronal manifestations in newborns. The DNA-PK complex plays a critical role in repairing DSBs and in the innate immune response to infection. It is unknown, however, whether DNA-PK regulates ZIKV infection. Here we investigated the role of DNA-PKcs, the catalytic subunit of DNA-PK, during ZIKV infection. We demonstrate that DNA-PKcs restricts the spread of ZIKV infection in human epithelial cells. Increased ZIKV replication and spread in DNA-PKcs deficient cells is related to a notable decrease in transcription of type I and III interferons as well as IFIT1, IFIT2, and IL6. This was shown to be independent of IRF1, IRF3, or p65, canonical transcription factors necessary for activation of both type I and III interferon promoters. The mechanism of DNA-PKcs to restrict ZIKV infection is independent of DSB. Thus, these data suggest a non-canonical role for DNA-PK during Zika virus infection, acting downstream of IFNs transcription factors for an efficient antiviral immune response.
Introduction
Zika virus (ZIKV) is a single-strand RNA mosquito-borne flavivirus (1). First isolated in 1947 in Africa, ZIKV caught public health attention in 2007 with the first viral outbreak in Pacific Islands, from where it spread to South America in 2015 (2–5). In 2016, Zika disease was declared to be a worldwide public health emergency due to severe neurological manifestations in newborns (6, 7). The neurological complications are associated with the tropism of ZIKV for human neural progenitor cells which results in growth arrest, DNA double-strand breaks (DSBs), and cell death (8, 9). DSBs are the most cytotoxic type of DNA lesions that rapidly activate DNA-damage repair response, orchestrated in part by the DNA-dependent protein kinase (DNA-PK) complex (10, 11). However, the relevance of DNA-PK in restricting ZIKV infection is unknown.
DNA-PK is a multifunctional protein complex consisting of Ku70, Ku80, and the catalytic subunit (DNA-PKcs) which are involved, among other functions, in DNA damage repair, V(D)J recombination of lymphocytes receptors, transcriptional regulation, DNA replication, and RNA metabolism (11–13). Present in the cytoplasm and nuclei, DNA-PK also functions as an intracellular DNA receptor critical for primary immune response against DNA virus infections by inducing interferon (IFN)-I and IFN-III expression (14–19). IFNs induce the transcription of interferon-stimulated genes (ISGs) that are critical for inhibition of viral replication cycle (20–22).
During RNA virus infections, IFN-I and IFN-III are mainly induced by intracellular RNA sensing receptors such as retinoic acid-inducible gene I (RIG-I) and melanoma differentiation-associated protein 5 (MDA5) (23). For instance, ZIKV RNA genome detection is mediated by RIG-I, leading to activation and nuclear translocation of the transcription factors belonging to the interferon regulatory factor (IRF) and nuclear factor-kappa B (NF-κB) families (24–35). IRFs and NF-κB bind to interferon-stimulated response element (ISRE) and NF-κB motifs, respectively, both present in the promoter region responsible for IFN-I and IFN-III genes (32, 36).
Several studies associate DNA-PK complex with ZIKV or other flaviviruses, such as Dengue virus (DENV). Vetter et al. (37) described DNA-PK localization and activation as a very early marker of DENV infection (37). DENV infection causes DNA-PK subunits relocation to the nucleoli, which may regulate RNA splicing (37–39). In addition, human cells with Ku80 protein partially depleted, reduce the interferon response induced by DENV (37). The DNA-PK complex is also associated with both ZIKV and DENV genomic RNA in human cells, with unknown effects (40). In this context and considering ZIKV can induce DSB (8, 9), we investigated whether DNA-PKcs affects ZIKV infection and triggers antiviral immune response pathways.
We found that DNA-PKcs restricts ZIKV spread in human epithelial cells. In the course of infection, DNA-PKcs is required for IFN-related gene transcription, independent of transcription factors IRF1, IRF3, or p65 (NF-κB subunit). In addition, DNA-PKcs role during ZIKV infection was DSB independent. This study provides information about the DNA-PKcs dynamics on the antiviral immune response during ZIKV infection and may contribute to new therapeutic strategies.
Materials and methods
Cell culture and reagents
A549 (ATCC®, CCL-185™), RPE (ATCC, CRL-2302), Vero (ATCC®, CCL-81™), and derived cell lines were maintained in DMEM-F12 (GIBCO, 12400-024) containing 5% fetal bovine serum (FBS – GIBCO, 12657-029) supplemented with 1 U/mL penicillin/streptomycin (Sigma, P4333). C6/36 (ATCC®, CRL-1660™) cells were maintained at 28 °C, in L-15 (Sigma, L4386) containing 5% FBS supplemented with 0.26% tryptose phosphate broth (GIBCO, 18050-39), and 1 U/mL penicillin/streptomycin. Both cell lines were routinely tested for mycoplasma contamination. Cells were treated with 0.5 or 1 µM of NU7441 (BioGems, 5039598), 100 ng/mL human TNF (Peprotech, 300-01A), and 3 µM etoposide (Sigma, E1383).
Virus infection
ZIKV strain BR2015/15261 (41) was kindly provided by Dra. Claudia N. Duarte dos Santos (Fiocruz-PR, Brazil). Viral stocks were purified from infected C6/36 cells supernatants and titrated by plaque assay on Vero cells. This virus was used for infection for indicated times and multiplicity of infection (m.o.i.).
Flow cytometry
Cells were detached from the plate with trypsin-EDTA (GIBCO, 25300-062), centrifuged at 460 x g for 5 min, washed in saline solution, and stained with Zombie NIR™ fixable viability kit (Biolegend, 423105) at the dilution 1:2000 for 20 min at room temperature. Cells were then fixed with 3% paraformaldehyde (PFA – Sigma, P6148) for 20 min followed by staining with FITC-conjugated flavivirus E protein antibody (anti-4G2) (provided from Fiocruz-PR, Brazil) in permeabilization buffer (0.25% saponin - Vetec, 1364) for 40 min. All cells were washed with saline solution and acquired on BD FACSVerse with FACSuite software. Analysis was performed using FlowJo software v. 10.1 (TreeStar). The gating strategy used for flow cytometry is available in Supplementary Figure 2.
MTT assay
Cells were incubated with 0.5 mg/mL MTT reagent (Amresco, 793) for 3 hours at 37 °C and 5% CO2, followed by incubation of DMSO for formazan crystals extraction. Measures were performed at 570 nm abs on a Biotek spectrophotometer using Gen5 1.10 software.
Immunofluorescence
Cells were seeded onto a plate containing a 15 mm glass coverslip, followed by ZIKV infection at determining time and concentration. Cells were then fixed with 3% PFA for 20 min followed by permeabilization with PBS containing 0.1% Triton X-100 (Amresco, 694) and 2% bovine serum albumin (BSA – Inlab, 1870) for 5 min. Primary antibodies (Supplementary Table I) were diluted in PBS with 2% BSA, and incubated for 1 hour at room temperature, followed by detection by secondary antibodies (Supplementary Table II), diluted in PBS with 2% BSA, and incubated for 1 hour at room temperature. Then, cell nuclei were stained with DAPI (Molecular probes, D3571) for 15 minutes and mounted with Mowiol (Sigma, 81381). Pictures were obtained on an Olympus BX41 microscope and processed on Q-capture Pro 5.1 software (Q-imaging). For transcription factors translocation into the nucleus, cells were counted on at least 20 infection plaque from each sample, in biological triplicates using ImageJ software.
Real-time quantitative PCR
Total RNA was extracted from cells using TRIzol reagent (Ambion, 15596026) according to manufacturer’s instructions. RT-PCR was performed with M-MLV Reverse Transcriptase (Promega, M170A) using 500 ng of RNA. For qPCR reaction, 2 µL of 1:20 diluted cDNA was used in a final volume of 10 µL, and 0.1 µM forward and reverse primers (Supplementary Table III), performed with GoTaq qPCR Master Mix (Promega, A600A). GAPDH was used as the housekeeping gene. Data were obtained on the StepOne Plus Real-Time PCR system (Applied BioSystems) and analyzed with StepOne Software v2.1. Relative mRNA expression was calculated by 2-ΔΔCT method.
Immunoblotting
Immunoblotting was used for characterization of A549PRKDC-/- cells. Cells were lysed using a lysis buffer containing protease inhibitor (Mini Protease Inhibitor Tablets – Roche, 5056489001). The samples were incubated for 30 min at 4 °C, being briefly vortexed each 10 min followed by centrifugation at 13.000 x g for 10 min at 4 °C. The supernatant was transferred to a new tube and the total proteins were quantified using Pierce BCA protein assay kit (Thermo, 23225). From total proteins, 20 µg were transferred to polyacrylamide gel electrophoresis for protein separation and then transferred to nitrocellulose 0.22 µm blotting membranes. The membranes were blocked in 5% non-fat milk in TBS containing 0.1% Tween 20 (TBST) for 1 hour at room temperature. Membranes were then probed with primary antibodies (Supplementary Table IV) diluted in TBST containing 5% BSA, at 4 °C shaking overnight. Membranes were washed with TBST and incubated in secondary antibodies (Supplementary Table V) for 1 hour at room temperature. Then, membranes were washed and chemiluminescence developed using ECL substrate (Pierce, 34577). Tubulin was normalized as the reference control.
CRISPR/Cas9
A549PRKDC-/- and RPEPRKDC-/- cells were generated with a pair of sgRNA guides (Guide1: GATCACGCCGCCAGTCTCCA; Guide2: CAGACATCTGAACAACTTTA). The guides were inserted in pX458 plasmids containing Cas9 encoded genes plus GFP sequence for clone isolation. The cells were transfected with pX458/Guide using lipofectamine 3000 reagent (Invitrogen, L3000-008) following manufacturer’s instructions. For clone selection and expansion, the fluorescent cells were isolated into a 96-well plate using BDMelody cell sorter (BD). Knockout cell line clones were confirmed by immunofluorescence and immunoblotting assays.
Data processing and statistical analyses
Data derived from the experiments were processed using GraphPad Prism nine software. The data were analyzed according to experimental settings using unpaired two-tailed Student’s t-test or two-way ANOVA, with Sidak’s correction where necessary.
Results
DNA-PKcs is required for control of ZIKV infection
DNA-PK complex plays a critical role in DNA damage repair such as DSB as well as in antiviral immune response (10, 11, 14, 16–19). It was demonstrated that ZIKV can induce DSB in neural progenitor cells (8, 9). However, the role of the catalytic subunit of DNA-PK in controlling ZIKV infection is currently unknown. To determine the role of DNA-PKcs during ZIKV infection, we used CRISPR/Cas9 editing of the PRKDC gene to generate DNA-PKcs-deficient A549 and RPE epithelial cells, referred to as A549PRKDC-/- (Figures S1A, B) and RPEPRKDC-/- (Figure S1C). We observed an increase of infectious ZIKV particles released from A549PRKDC-/- (Figure 1A) and RPEPRKDC-/- (Figure 1B) compared with wild-type (WT) cells as well as a significant increase in intracellular ZIKV RNA (Figures 1C, D). In the absence of DNA-PKcs, ZIKV spread to adjacent cells, as measured by plaque area size (Figures 1E, F), as well as the percentage of infected cells (Figure 1G, Figure S2) and dead cells (Figure 1H) are also increased. Altogether, these results indicate DNA-PKcs is required for full control of ZIKV infection in both A549 and RPE cells.
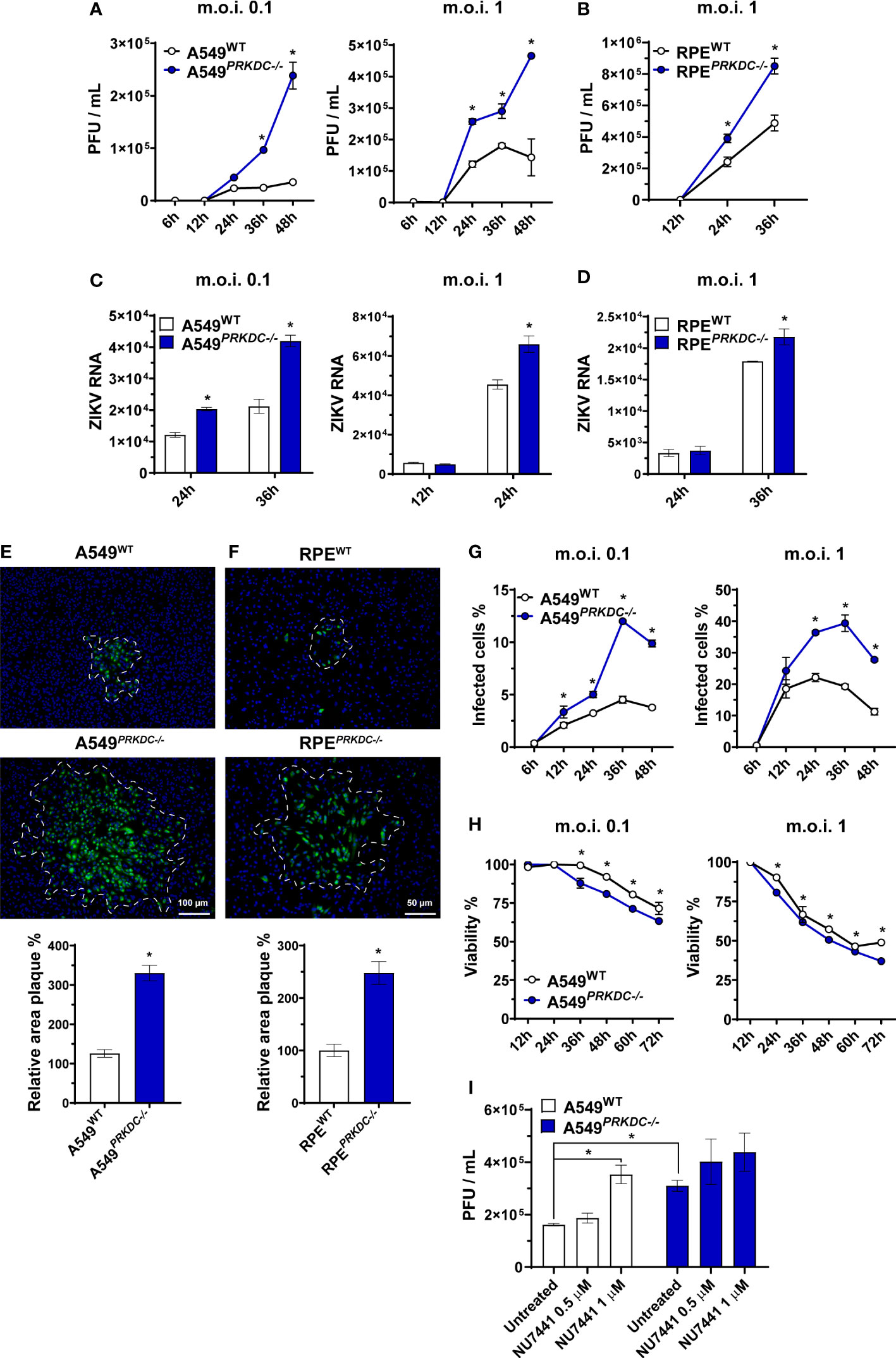
Figure 1 DNA-PKcs is critical for control of ZIKV infection. Virus replication measured by plaque assay, expressed as plaque-forming units per mL (PFU/mL), on (A) A549WT and A549PRKDC-/- or (B) RPEWT and RPEPRKDC-/- cells infected with ZIKV at indicated m.o.i. and time. RT-qPCR analysis to measure ZIKV RNA in (C) A549WT and A549PRKDC-/- or (D) RPEWT and RPEPRKDC-/- cells infected at indicated m.o.i. and time. (E) A549WT and A549PRKDC-/- or (F) RPEWT and RPEPRKDC-/- cells infected with 50 PFU of ZIKV at 48 hours in semi-solid medium, then ZIKV-E protein (green) was stained for immunofluorescence analysis, and the relative area of infection percentage was measured using the ImageJ software. The cell nuclei were stained with DAPI (blue). (G) Percentage of ZIKV-infected A549WT and A549PRKDC-/- cells at indicated m.o.i. and time, analyzed by flow cytometry. (H) Viability analysis by MTT assay of ZIKV-infected A549WT and A549PRKDC-/- cells relative to uninfected cells (mock) at indicated m.o.i. and time. (I) A549WT and A549PRKDC-/- were pretreated with NU7441 (0.5 and 1 µM) at 24 hours followed by ZIKV infection (m.o.i. 1) at 24 hours. We used two-way ANOVA with Sidak’s correction in (A–D, G, H), and unpaired two-tailed Student’s t-test was used in (E, F). * p<0.05, n = 3, error bars ± SEM.
To evaluate whether the DNA-PKcs kinase function is required for ZIKV control, we treated A549 cells with NU7441, a DNA-PKcs inhibitor (42, 43), 24 hours before infecting with ZIKV (Figure 1I). Similar to what we observed in PRKDC-/- cells, NU7441 pre-treatment increased ZIKV infection in WT, but not in A549PRKDC-/- cells. These results suggest DNA-PKcs kinase function is necessary for control of ZIKV infection.
ZIKV infection does not induce double-strand DNA breaks in A549 cells
IFN-I is induced by DNA-PKcs through its DNA sensing function following DSB (44). Furthermore, ZIKV infection induces DSB in neural progenitor cells as observed by γH2A.X histone phosphorylation (8, 9). Thus, we evaluated whether ZIKV infection induces DSB in A549 cells, leading to a source of immunostimulatory DNA to activate DNA-PKcs. To assess this, the A549WT and A549PRKDC-/- cells as well as A549 cells pre-treated with 1 µM NU7441 were infected with ZIKV (m.o.i. 1) at 24 hours. As a positive control, cells were treated with 3 µM etoposide, a topoisomerase II inhibitor, for 12 hours (Figures 2A, B). As expected, etoposide treatment increased the number of γH2A.X foci (green) per cell, being enhanced in A549PRKDC-/- or in NU7441-treated cells. However, the γH2A.X foci levels were similar in A549 cells infected with ZIKV (red) compared with uninfected cells. These results suggest that DNA-PKcs controls the ZIKV infection independent of any DSB-induced response.
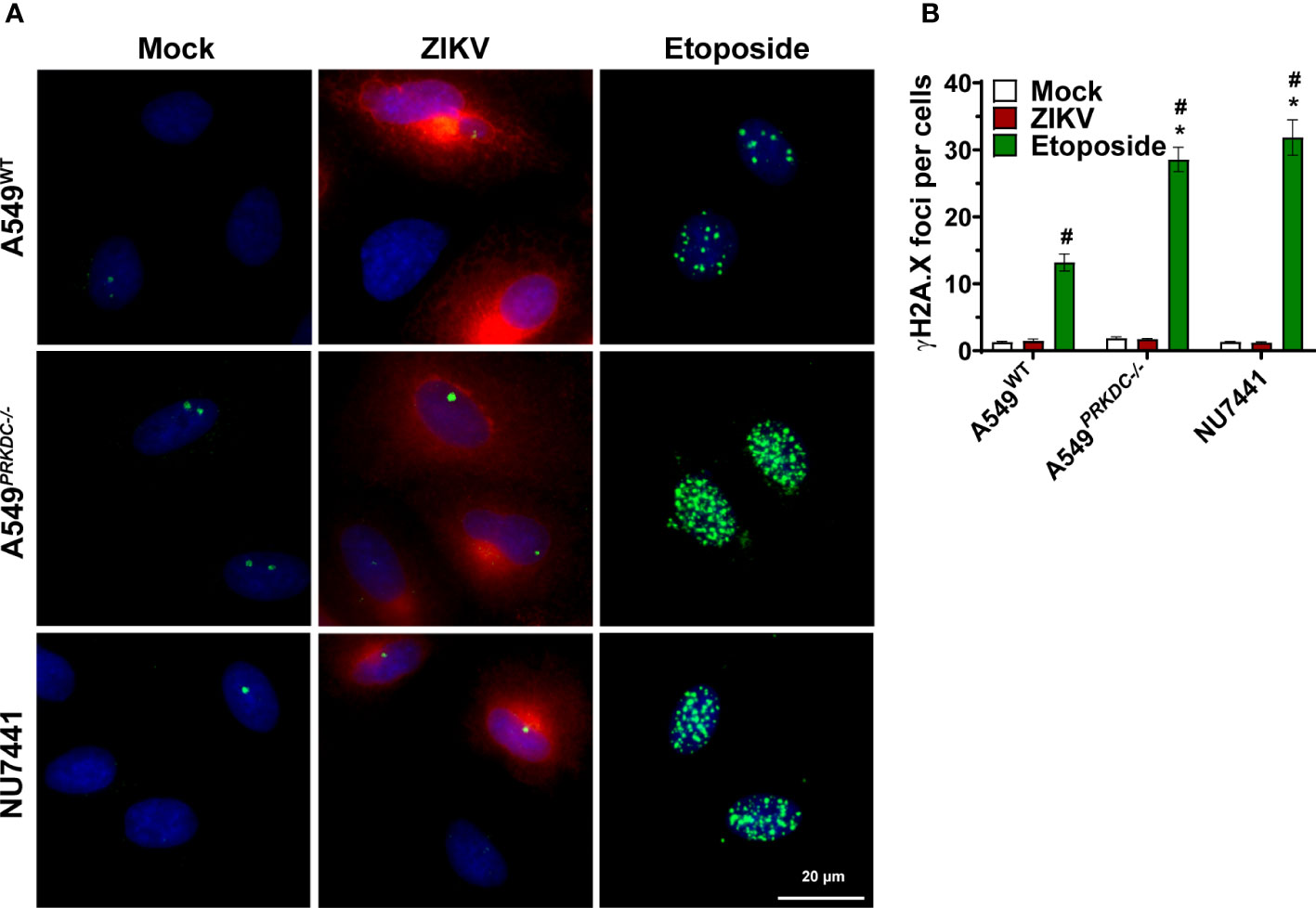
Figure 2 ZIKV infection does not induce DSB in A549 cells. A549WT, A549PRKDC-/- and 1 µM NU7441 pre-treated A549WT infected with ZIKV (m.o.i. 1) at 24 hours. Stimulation with 3 µM etoposide for 12 hours was used as a DSB positive control. (A) Immunofluorescence to analyze γH2AX (green) in the ZIKV-infected cells (red, ZIKV-E protein). The cell nuclei were stained with DAPI (blue). (B) Percentage of γH2AX foci per cell showed in (A). *Compared with WT cells; #Compared with mock. We used two-way ANOVA with Sidak’s correction. * or # p<0.05, n = 3, error bars ± SEM.
DNA-PKcs is required for IFN-I and IFN-III genes transcription during ZIKV infection
RIG-I is the major ZIKV RNA sensor, leading to induction of IFN-I and IFN-III transcription, crucial for an efficient antiviral immune response (33, 34). To evaluate whether RIG-I orchestrates ZIKV sensing in A549 cells, we infected A549WT and A549RIGI-/- with m.o.i. 1 for 24 hours to measure the induction of IFN-related genes transcription (Figure 3A). We observed A549 lacking RIG-I failed to induce IFN-I, IFN-III, ISGs, and IL6 transcriptions during ZIKV infection, confirming that RIG-I is necessary for activation of antiviral immune response. Next, we investigated whether DNA-PKcs is required for activating the IFNs pathways downstream RIG-I during ZIKV infection. We performed a gene expression analysis of IFN-I, IFN-III, and ISGs in A549WT and A549PRKDC-/- cells infected with ZIKV at an m.o.i. 0.1 (Figure 3B) and m.o.i. 1 (Figure 3C). During ZIKV infection we observed a reduction of IFNL1, IFIT1, and IFIT2, but not IFNB, IFIT3, and ISG15 transcription in the absence of DNA-PKcs. Similarly, in RPE cells, where ZIKV infection does not induce IFNL1 transcription, the absence of DNA-PKcs decreased IFNB and IFIT2, but not ISG15 transcription (Figure S3A). In addition, proinflammatory gene transcription induced after RIG-I activation such as IL6 and NFKBIA is independent of DNA-PKcs in A549 cells upon ZIKV infection (Figure 3D), but dependent in RPE cells lacking DNA-PKcs (Figure S3B). Altogether, these results suggest crosstalk between RIG-I and DNA-PKcs during ZIKV infection, which results in an efficient antiviral immune response in A549 and RPE cells.
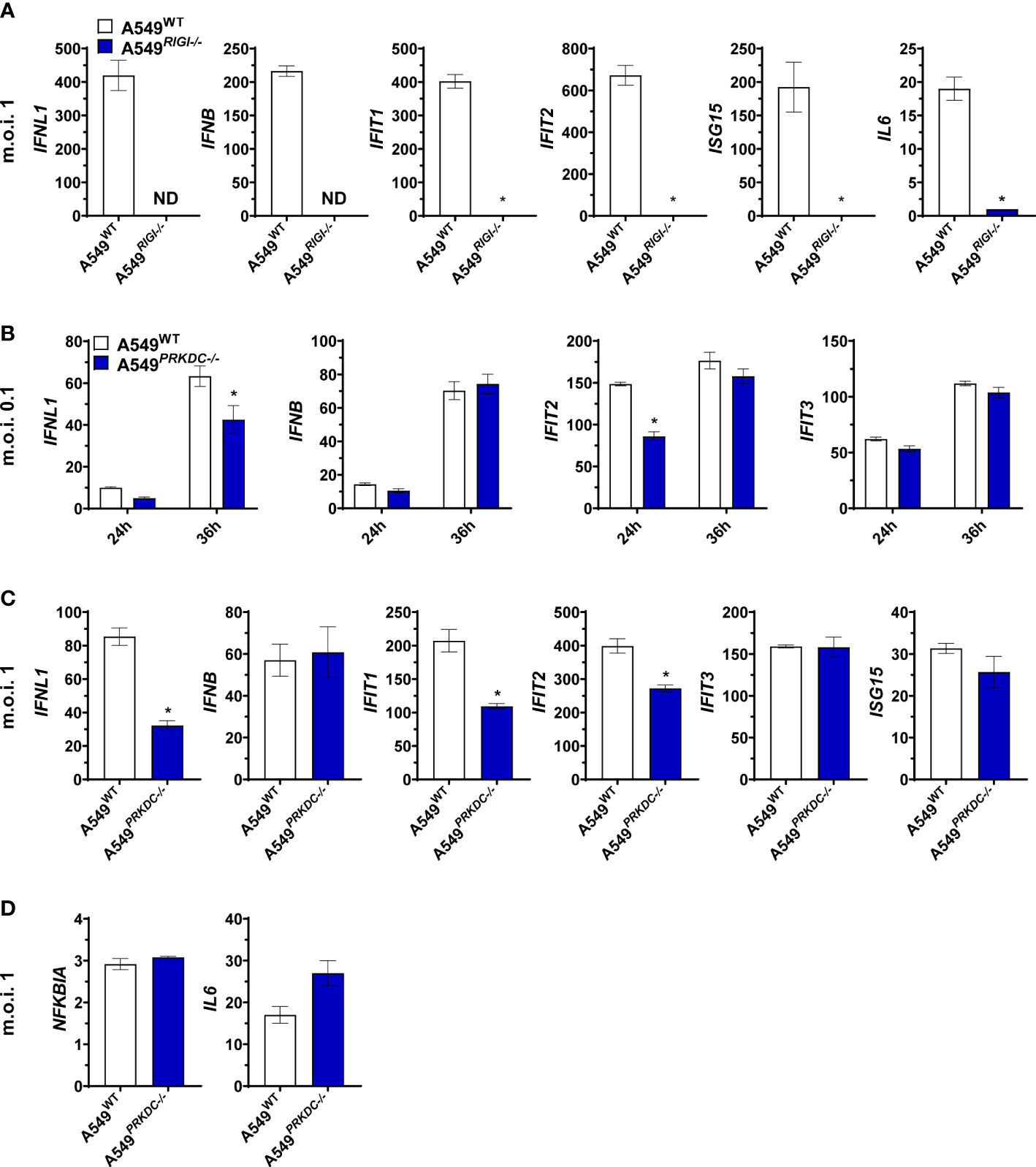
Figure 3 DNA-PKcs regulates interferon-related genes during ZIKV infection. (A) RT-qPCR to measure the expression of mRNA for indicated genes on A549WT and A549RIGI-/- cells infected with ZIKV m.o.i. 1 for 24 hours. (B) RT-qPCR to measure the expression of mRNA for indicated genes on A549WT and A549PRKDC-/- cells infected with ZIKV m.o.i. 0.1 at the indicated time or (C) m.o.i. 1 at 24 hours. (D) Expression of mRNA for NFKBIA and IL6, determined by RT-qPCR on A549WT and A549PRKDC-/- cells infected with ZIKV m.o.i. 1 at 24 hours. We used unpaired two-tailed Student’s t-test in (A, C, D), and two-way ANOVA with Sidak’s correction in (B). ND: Non-detected. * p<0.05, n = 3, error bars ± SEM.
IRF1, IRF3, and p65 nuclear accumulation during ZIKV infection is DNA-PKcs independent
We evaluated the crosstalk between RIG-I and DNA-PKcs during ZIKV infection analyzing the IFN-I/III-inducing transcription factors including IRF1, IRF3, IRF5, IRF7, and p65 (NF-κB subunit) (24, 25, 27, 29–31, 35). We infected A549 cells with ZIKV and measured the accumulation of the transcription factors in the nucleus. We observed that ZIKV infection induces IRF1 and IRF3, but not IRF5 and IRF7 nuclear accumulation (Figures 4A–D and Figures S4A, B). As expected, A549RIGI-/- cells failed to induce IRF3 and IRF1 nuclear accumulation, confirming that RIG-I is the major ZIKV intracellular sensor (Figures 4A, B). However, the activation of the transcription factors was independent of DNA-PKcs, showing a similar nuclear location percentage of IRF3 and IRF1 (Figure 4C, D). Similarly, the induction of p65 nuclei accumulation was independent of DNA-PKcs (Figure 4E). Interestingly, while IRF3 nuclei translocation occurred exclusively in infected cells, we observed that IRF1 accumulation in the nuclei occurred mostly in bystander cells, confirmed by ZIKV dsRNA staining, which shows the early stage of virus infection (Figure S4C). Altogether, these results suggest that upon ZIKV infection, DNA-PKcs is necessary for enhancement of IFNs and ISGs transcription, acting downstream activation of transcription factors.
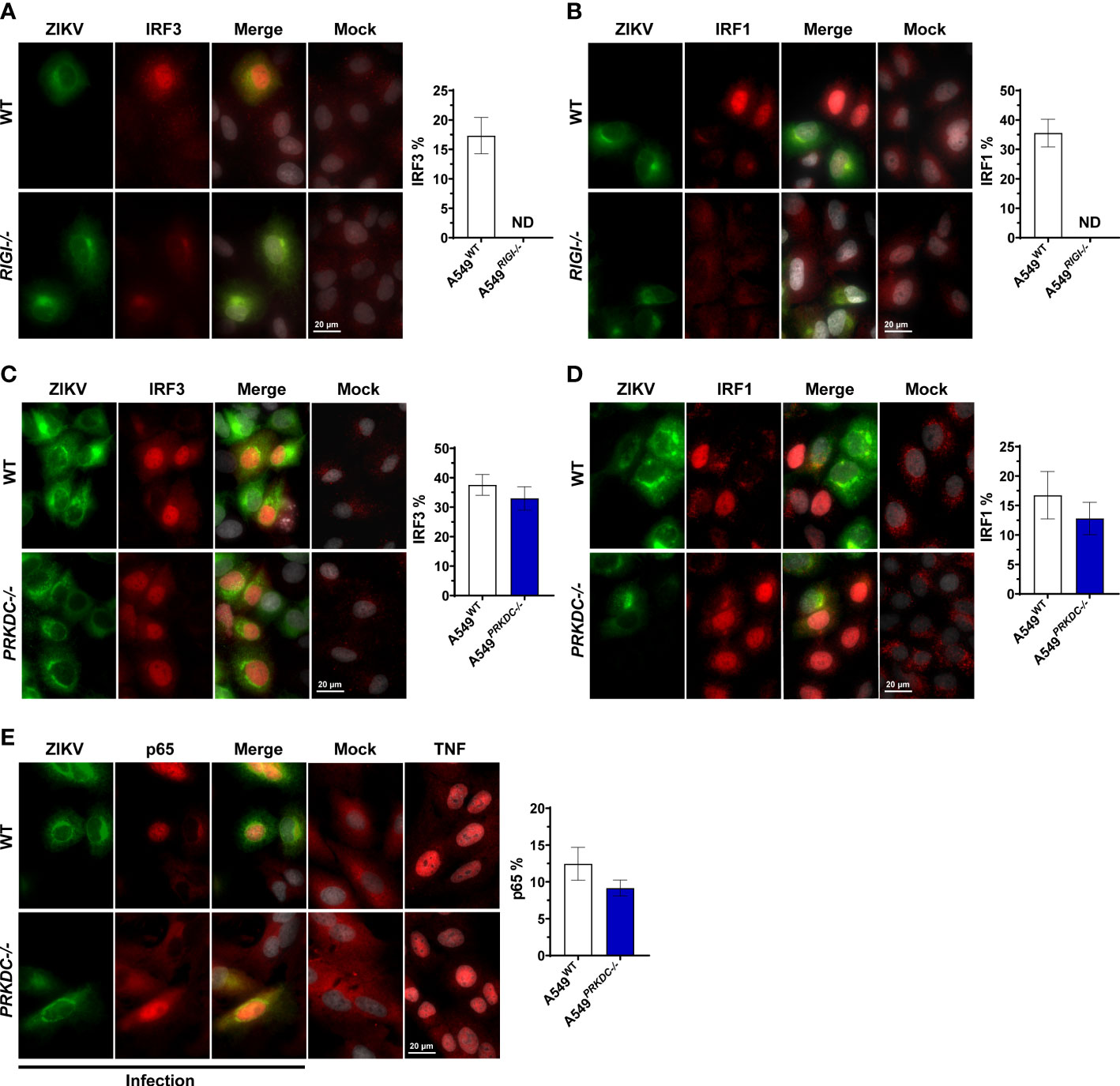
Figure 4 ZIKV induces IRF1, IRF3, and p65 nuclei accumulation independent of DNA-PKcs. Immunofluorescence analysis for localization of endogenous (A) IRF3 (red) and (B) IRF1 (red) on A549WT and A549RIGI-/- cells infected with ZIKV m.o.i. 1 (green, ZIKV-E protein) at 36 hours (left panel), and quantified by scoring cells with nuclear staining (right panel, n = 3, counts of at least 30 nuclei per slide). (C–E) Immunofluorescence analysis for localization of endogenous (C) IRF3 (red), (D) IRF1 (red), and (E) p65 (red) on A549WT and A549PRKDC-/- cells infected with ZIKV m.o.i. 1 (green, ZIKV-E protein) at 24 hours (left panel), and quantified by scoring cells with nuclear staining (right panel, n = 3, counts of at least 30 nuclei per slide). TNF was used as a positive control in (E). Cell nuclei were stained with DAPI (grey). We used unpaired two-tailed Student’s t-test. N = 3, error bars ± SEM. ND: non-detected.
Discussion
The DNA-PK complex senses viral DNA with an unknown impact on ZIKV infection. Here we demonstrate that DNA-PKcs, the catalytic subunit of the DNA-PK complex, is critical for control of ZIKV infection by a non-canonical mechanism.
We analyzed different infection profiles such as quantifying viable ZIKV, intracellular ZIKV-RNA, ZIKV spreading to adjacent cells, and ZIKV-infected cells. We showed that absence of DNA-PKcs increases susceptibility to ZIKV infection in two different human epithelial cell lineages, allowing a faster virus spreading. Moreover, we showed that the DNA-PKcs mechanism for controlling ZIKV infection depends on its kinase function. Previous studies have associated DNA-PK complex with infection of flaviviruses. For instance, Vetter et al. (37) demonstrated that Ku70 and Ku80 knockdown in Huh7 cells are insufficient to impact DENV infection, differing from our findings with ZIKV (37). The difference could be explained due to a partial knockdown of the Ku components or the use of different cell lines and flavivirus. In addition, depletion of each DNA-PK subunit separately varies on number of differentially expressed genes (45). Hence, instead of Ku proteins, only the catalytic subunit may be critical for control of infection.
ZIKV infection induces DSB in human neural stem cells (8, 9), which may implicate DNA-PK activation (46). However, our findings showed that ZIKV does not increase H2A.X phosphorylated foci, a DSB marker, in human epithelial (A549) cells. Hence, ZIKV does not induce DSB in A549 cells, and the role of DNA-PKcs in controlling viral infection is independent of DSB repair response. In addition, we showed that either inhibition of DNA-PKcs kinase function or loss of whole protein has increased DSB sensitivity to etoposide, a genotoxic stressor, as suggested in previous studies (43, 47).
We showed that DNA-PKcs is necessary for an effective antiviral response against ZIKV infection which may explain its role in restricting the infection. The effect of DNA-PKcs on IFN-I and IFN-III transcriptions differs between cell types. Similar to our findings, Vetter et al. (37) demonstrated that inhibition of Ku80 protein expression decreases IFNB transcription in DENV-infected cells (37). It is important to notice that DNA-PKcs antiviral response might differ between humans and mice and virus species (19). These could explain why the antiviral response against other RNA viruses is DNA-PKcs independent (14). ZIKV infection might induce mitochondrial DNA release, activating DNA sensors such as DNA-PK or cGAS, which results in IFN-I and IFN-III transcription (14, 48–50). However, we observed abrogation of the IFNs transcription in A549RIGI-/- cells infected with ZIKV, suggesting only RNA sensing pathway is activated during the infection.
Our data show that ZIKV infection induces nuclear accumulation of the main transcription factors involved in IFN-I and IFN-III transcription independently of DNA-PKcs. These findings implicate that DNA or RNA sensing pathways upstream of the transcription factors are not affected by DNA-PKcs during ZIKV infection. A prior study suggested DNA-PK complex is not required for IRF3 nor p65 nuclear translocation under RNA virus infection or Poly(I:C) stimulation (14). Furthermore, we showed ZIKV infection fails to induce IRF1 and IRF3 accumulation to the nucleus in absence of the RNA sensor RIG-I, suggesting DNA-PKcs is not a ZIKV sensor receptor. Our findings confirmed that RIG-I is the major nucleic acid sensor activated by ZIKV, as previously demonstrated (33, 34), and suggests novel crosstalk between DNA-PKcs and RIG-I pathway downstream to transcription factors. One possibility is during ZIKV infection, DNA-PKcs acts in the nucleus regulating IFN transcription. The DNA-PK is known to be a regulator of the transcriptome and RNA metabolism (13, 38, 51–55). DNA-PKcs is described to phosphorylate RNA polymerase II, which might enhance the transcription of some viral genomes such as Hepatitis B virus and Human immunodeficiency virus (52, 55–57). Genotoxic stress or viral infections such as DENV induce DNA-PKcs localization to the nucleolus, where it acts as a regulator of pre-mRNA splicing (13, 37, 38). The regulation of RNA metabolism by DNA-PKcs should be explored in the future in the context of ZIKV infections.
Overall, these findings provide a role of DNA-PKcs in control of ZIKV virus infection beyond its DNA sensing function or DSB repair response. Future work should consider whether this conclusion can be generalized to different RNA viruses. This study will advance our understanding of the antiviral immune response and may contribute to new therapeutic approaches.
Data availability statement
The original contributions presented in the study are included in the article/Supplementary Materials. Further inquiries can be directed to the corresponding author.
Author contributions
DP, AZ-F, AB, BF and DM designed experiments and analyzed data. DP, GD, LG, DB, and DM performed experiments. GD, AZ-F, BT, HT and BF contributed with critical reagents/tools. DP and DM wrote the manuscript. All authors contributed to the article and approved the submitted version.
Funding
Conselho Nacional de Desenvolvimento Científico e Tecnológico PhD studentship 141906/2017-0 to DP; Wellcome Trust PhD Studentship 203778/Z/16/Z to HT and BF, a UKRI/BBSRC research project grant BB/S001336/1 to BF.
Acknowledgments
We thank Laboratório Multiusuário de Estudos em Biologia (LAMEB) for technical assistance. We thank the laboratory of immunobiology (LIM) members for their helpful discussions.
Conflict of interest
The authors declare that the research was conducted in the absence of any commercial or financial relationships that could be construed as a potential conflict of interest.
Publisher’s note
All claims expressed in this article are solely those of the authors and do not necessarily represent those of their affiliated organizations, or those of the publisher, the editors and the reviewers. Any product that may be evaluated in this article, or claim that may be made by its manufacturer, is not guaranteed or endorsed by the publisher.
Supplementary material
The Supplementary Material for this article can be found online at: https://www.frontiersin.org/articles/10.3389/fimmu.2022.1042463/full#supplementary-material
References
1. Masmejan S, Musso D, Vouga M, Pomar L, Dashraath P, Stojanov M, et al. Zika virus. Pathogens (2020) 9:898. doi: 10.3390/pathogens9110898
2. Cardoso CW, Paploski IAD, Kikuti M, Rodrigues MS, Silva MMO, Campos GS, et al. Outbreak of exanthematous illness associated with zika, chikungunya, and dengue viruses, Salvador, Brazil. Emerg Infect Dis (2015) 21:2274–6. doi: 10.3201/eid2112.151167
3. Musso D, Nilles EJ, Cao-Lormeau V-M. Rapid spread of emerging zika virus in the pacific area. Clin Microbiol Infection (2014) 20:O595–6. doi: 10.1111/1469-0691.12707
4. Duffy MR, Chen T-H, Hancock WT, Powers AM, Kool JL, Lanciotti RS, et al. Zika virus outbreak on yap island, federated states of Micronesia. New Engl J Med (2009) 360:2536–43. doi: 10.1056/NEJMoa0805715
5. Dick GWA, Kitchen SF, Haddow AJ. Zika virus (I). isolations and serological specificity. Trans R Soc Trop Med Hyg (1952) 46:509–20. doi: 10.1016/0035-9203(52)90042-4
6. Gulland A. Zika virus is a global public health emergency, declares WHO. BMJ (2016) 352:i657. doi: 10.1136/bmj.i657
7. Lazear HM, Diamond MS. Zika virus: New clinical syndromes and its emergence in the Western hemisphere. J Virol (2016) 90:4864–75. doi: 10.1128/JVI.00252-16
8. Hammack C, Ogden SC, Madden JC, Medina A, Xu C, Phillips E, et al. Zika virus infection induces DNA damage response in human neural progenitors that enhances viral replication. J Virol (2019) 93(20):e00638–19. doi: 10.1128/JVI.00638-19
9. Devhare P, Meyer K, Steele R, Ray RB, Ray R. Zika virus infection dysregulates human neural stem cell growth and inhibits differentiation into neuroprogenitor cells. Cell Death Dis (2017) 8:e3106–6. doi: 10.1038/cddis.2017.517
10. Callén E, Jankovic M, Wong N, Zha S, Chen H-T, Difilippantonio S, et al. Essential role for DNA-PKcs in DNA double-strand break repair and apoptosis in ATM-deficient lymphocytes. Mol Cell (2009) 34:285–97. doi: 10.1016/j.molcel.2009.04.025
11. Anderson CW. DNA Damage and the DNA-activated protein kinase. Trends Biochem Sci (1993) 18:433–7. doi: 10.1016/0968-0004(93)90144-C
12. Nussenzweig A, Chen C, da Costa Soares V, Sanchez M, Sokol K, Nussenzweig MC, et al. Requirement for Ku80 in growth and immunoglobulin V(D)J recombination. Nature (1996) 382:551–5. doi: 10.1038/382551a0
13. Abbasi S, Schild-Poulter C. Mapping the Ku interactome using proximity-dependent biotin identification in human cells. J Proteome Res (2019) 18:1064–77. doi: 10.1021/acs.jproteome.8b00771
14. Ferguson BJ, Mansur DS, Peters NE, Ren H, Smith GL. DNA-PK is a DNA sensor for IRF-3-dependent innate immunity. Elife (2012) 14:1–e00047. doi: 10.7554/eLife.00047
15. Kotula E, Faigle W, Berthault N, Dingli F, Loew D, Sun J-S, et al. DNA-PK target identification reveals novel links between DNA repair signaling and cytoskeletal regulation. PLoS One (2013) 8:e80313. doi: 10.1371/journal.pone.0080313
16. Karpova AY, Trost M, Murray JM, Cantley LC, Howley PM. Interferon regulatory factor-3 is an in vivo target of DNA-PK. Proc Natl Acad Sci (2002) 99:2818–23. doi: 10.1073/pnas.052713899
17. Zhang X, Brann TW, Zhou M, Yang J, Oguariri RM, Lidie KB, et al. Cutting edge: Ku70 is a novel cytosolic DNA sensor that induces type III rather than type I IFN. J Immunol (2011) 186:4541–5. doi: 10.4049/jimmunol.1003389
18. Sui H, Zhou M, Imamichi H, Jiao X, Sherman BT, Lane HC, et al. STING is an essential mediator of the Ku70-mediated production of IFN-λ1 in response to exogenous DNA. Sci Signal (2017) 10(488):eaah5054. doi: 10.1126/scisignal.aah5054
19. Burleigh K, Maltbaek JH, Cambier S, Green R, Gale M, James RC, et al. Human DNA-PK activates a STING-independent DNA sensing pathway. Sci Immunol (2020) 19(430):eaba4219. doi: 10.1126/sciimmunol.aba4219
20. Lanford RE, Guerra B, Lee H, Chavez D, Brasky KM, Bigger CB. Genomic response to interferon-α in chimpanzees: Implications of rapid downregulation for hepatitis c kinetics. Hepatology (2006) 43:961–72. doi: 10.1002/hep.21167
21. Schoggins JW, Wilson SJ, Panis M, Murphy MY, Jones CT, Bieniasz P, et al. A diverse range of gene products are effectors of the type I interferon antiviral response. Nature (2011) 472:481–5. doi: 10.1038/nature09907
22. Mostafavi S, Yoshida H, Moodley D, LeBoité H, Rothamel K, Raj T, et al. Parsing the interferon transcriptional network and its disease associations. Cell (2016) 164:564–78. doi: 10.1016/j.cell.2015.12.032
23. Yoneyama M, Kikuchi M, Matsumoto K, Imaizumi T, Miyagishi M, Taira K, et al. Shared and unique functions of the DExD/H-box helicases RIG-I, MDA5, and LGP2 in antiviral innate immunity. J Immunol (2005) 175:2851–8. doi: 10.4049/jimmunol.175.5.2851
24. Dou L, Liang H-F, Geller DA, Chen Y-F, Chen X-P. The regulation role of interferon regulatory factor-1 gene and clinical relevance. Hum Immunol (2014) 75:1110–4. doi: 10.1016/j.humimm.2014.09.015
25. Panda D, Gjinaj E, Bachu M, Squire E, Novatt H, Ozato K, et al. IRF1 maintains optimal constitutive expression of antiviral genes and regulates the early antiviral response. Front Immunol (2019) 10:1019. doi: 10.3389/fimmu.2019.01019
26. Au WC, Moore PA, Lowther W, Juang YT, Pitha PM. Identification of a member of the interferon regulatory factor family that binds to the interferon-stimulated response element and activates expression of interferon-induced genes. Proc Natl Acad Sci (1995) 92:11657–61. doi: 10.1073/pnas.92.25.11657
27. Juang Y-T, Lowther W, Kellum M, Au W-C, Lin R, Hiscott J, et al. Primary activation of interferon a and interferon b gene transcription by interferon regulatory factor 3. Proc Natl Acad Sci (1998) 95:9837–42. doi: 10.1073/pnas.95.17.9837
28. Lin R, Heylbroeck C, Pitha PM, Hiscott J. Virus-dependent phosphorylation of the IRF-3 transcription factor regulates nuclear translocation, transactivation potential, and proteasome-mediated degradation. Mol Cell Biol (1998) 18:2986–96. doi: 10.1128/MCB.18.5.2986
29. Marie I, Durbin J, Levy D. Differential viral induction of distinct interferon-alpha genes by positive feedback through interferon regulatory factor-7. EMBO J (1998) 17:6660–9. doi: 10.1093/emboj/17.22.6660
30. Andrilenas KK, Ramlall V, Kurland J, Leung B, Harbaugh AG, Siggers T. DNA-Binding landscape of IRF3, IRF5 and IRF7 dimers: implications for dimer-specific gene regulation. Nucleic Acids Res (2018) 46:2509–20. doi: 10.1093/nar/gky002
31. Barnes BJ, Moore PA, Pitha PM. Virus-specific activation of a novel interferon regulatory factor, IRF-5, results in the induction of distinct interferon α genes. J Biol Chem (2001) 276:23382–90. doi: 10.1074/jbc.M101216200
32. Visvanathan K v, Goodbourn S. Double-stranded RNA activates binding of NF-kappa b to an inducible element in the human beta-interferon promoter. EMBO J (1989) 8:1129–38. doi: 10.1002/j.1460-2075.1989.tb03483.x
33. Chazal M, Beauclair G, Gracias S, Najburg V, Simon-Lorière E, Tangy F, et al. RIG-I recognizes the 5′ region of dengue and zika virus genomes. Cell Rep (2018) 24:320–8. doi: 10.1016/j.celrep.2018.06.047
34. Schilling M, Bridgeman A, Gray N, Hertzog J, Hublitz P, Kohl A, et al. RIG-I plays a dominant role in the induction of transcriptional changes in zika virus-infected cells, which protect from virus-induced cell death. Cells (2020) 9:1476. doi: 10.3390/cells9061476
35. Lenardo MJ, Fan C-M, Maniatis T, Baltimore D. The involvement of NF-κB in β-interferon gene regulation reveals its role as widely inducible mediator of signal transduction. Cell (1989) 57:287–94. doi: 10.1016/0092-8674(89)90966-5
36. Levy DE, Kessler DS, Pine R, Reich N, Darnell JE. Interferon-induced nuclear factors that bind a shared promoter element correlate with positive and negative transcriptional control. Genes Dev (1988) 2:383–93. doi: 10.1101/gad.2.4.383
37. Vetter ML, Rodgers MA, Patricelli MP, Yang PL. Chemoproteomic profiling identifies changes in DNA-PK as markers of early dengue virus infection. ACS Chem Biol (2012) 7:2019–26. doi: 10.1021/cb300420z
38. Liu S, Shao Y, Wang Q, Zhai Y, Li X. Genotoxic stress causes the accumulation of DNA-dependent protein kinase catalytic subunit phosphorylated at serine 2056 at nuclear speckles and alters pre- mRNA alternative splicing. FEBS Open Bio (2019) 9:304–14. doi: 10.1002/2211-5463.12569
39. Miao M, Yu F, Wang D, Tong Y, Yang L, Xu J, et al. Proteomics profiling of host cell response via protein expression and phosphorylation upon dengue virus infection. Virol Sin (2019) 34:549–62. doi: 10.1007/s12250-019-00131-2
40. Ooi YS, Majzoub K, Flynn RA, Mata MA, Diep J, Li JK, et al. An RNA-centric dissection of host complexes controlling flavivirus infection. Nat Microbiol (2019) 4:2369–82. doi: 10.1038/s41564-019-0518-2
41. Strottmann DM, Zanluca C, Mosimann ALP, Koishi AC, Auwerter NC, Faoro H, et al. Genetic and biological characterisation of zika virus isolates from different Brazilian regions. Mem Inst Oswaldo Cruz (2019) 114:e190150. doi: 10.1590/0074-02760190150
42. Leahy JJJ, Golding BT, Griffin RJ, Hardcastle IR, Richardson C, Rigoreau L, et al. Identification of a highly potent and selective DNA-dependent protein kinase (DNA-PK) inhibitor (NU7441) by screening of chromenone libraries. Bioorg Med Chem Lett (2004) 14:6083–7. doi: 10.1016/j.bmcl.2004.09.060
43. Zhao Y, Thomas HD, Batey MA, Cowell IG, Richardson CJ, Griffin RJ, et al. Preclinical evaluation of a potent novel DNA-dependent protein kinase inhibitor NU7441. Cancer Res (2006) 66:5354–62. doi: 10.1158/0008-5472.CAN-05-4275
44. Morales AJ, Carrero JA, Hung PJ, Tubbs AT, Andrews JM, Edelson BT, et al. A type I IFN-dependent DNA damage response regulates the genetic program and inflammasome activation in macrophages. Elife (2017) 6:e24655. doi: 10.7554/eLife.24655
45. Shadrina O, Garanina I, Anisenko A, Kireev I, Gottikh M. Transcriptome analysis of HEK 293T cells revealed different significance of the depletion of DNA-dependent protein kinase subunits, Ku70, Ku80, and DNA-PKcs. Biochimie (2022) 199:139–49. doi: 10.1016/j.biochi.2022.04.004
46. Gu Y, Jin S, Gao Y, Weaver DT, Alt FW. Ku70-deficient embryonic stem cells have increased ionizing radiosensitivity, defective DNA end-binding activity, and inability to support V(D)J recombination. Proc Natl Acad Sci (1997) 94:8076–81. doi: 10.1073/pnas.94.15.8076
47. Yang L, Yang X, Tang Y, Zhang D, Zhu L, Wang S, et al. Inhibition of DNA-PK activity sensitizes A549 cells to X-ray irradiation by inducing the ATM-dependent DNA damage response. Mol Med Rep (2018) 17(6):7545–7552. doi: 10.3892/mmr.2018.8828
48. Sun L, Wu J, Du F, Chen X, Chen ZJ. Cyclic GMP-AMP synthase is a cytosolic DNA sensor that activates the type I interferon pathway. Sci (1979) (2013) 339:786–91. doi: 10.1126/science.1232458
49. White MJ, McArthur K, Metcalf D, Lane RM, Cambier JC, Herold MJ, et al. Apoptotic caspases suppress mtDNA-induced STING-mediated type I IFN production. Cell (2014) 159:1549–62. doi: 10.1016/j.cell.2014.11.036
50. West AP, Khoury-Hanold W, Staron M, Tal MC, Pineda CM, Lang SM, et al. Mitochondrial DNA stress primes the antiviral innate immune response. Nature (2015) 520:553–7. doi: 10.1038/nature14156
51. Zhang S, Schlott B, Görlach M, Grosse F. DNA-Dependent protein kinase (DNA-PK) phosphorylates nuclear DNA helicase II/RNA helicase a and hnRNP proteins in an RNA-dependent manner. Nucleic Acids Res (2004) 32:1–10. doi: 10.1093/nar/gkg933
52. Dvir A, Peterson SR, Knuth MW, Lu H, Dynan WS. Ku Autoantigen is the regulatory component of a template-associated protein kinase that phosphorylates RNA polymerase II. Proc Natl Acad Sci (1992) 89:11920–4. doi: 10.1073/pnas.89.24.11920
53. Song Z, Xie Y, Guo Z, Han Y, Guan H, Liu X, et al. Genome-wide identification of DNA-PKcs-associated RNAs by RIP-seq. Signal Transduct Target Ther (2019) 4:22. doi: 10.1038/s41392-019-0057-6
54. Song L, Yu M, Jin R, Gu M, Wang Z, Hou D, et al. Long-read sequencing annotation of the transcriptome in DNA-PK inactivated cells. Front Oncol (2022) 12:941638. doi: 10.3389/fonc.2022.941638
55. Peterson SR, Jesch SA, Chamberlin TN, Dvir A, Rabindran SK, Wu C, et al. Stimulation of the DNA-dependent protein kinase by RNA polymerase II transcriptional activator proteins. J Biol Chem (1995) 270:1449–54. doi: 10.1074/jbc.270.3.1449
56. Zicari S, Sharma AL, Sahu G, Dubrovsky L, Sun L, Yue H, et al. DNA Dependent protein kinase (DNA-PK) enhances HIV transcription by promoting RNA polymerase II activity and recruitment of transcription machinery at HIV LTR. Oncotarget (2020) 11:699–726. doi: 10.18632/oncotarget.27487
Keywords: Zika virus, interferon, DNA-PKcs, double-strand DNA breaks, infection
Citation: Patricio DdO, Dias GBM, Granella LW, Trigg B, Teague HC, Bittencourt D, Báfica A, Zanotto-Filho A, Ferguson B and Mansur DS (2022) DNA-PKcs restricts Zika virus spreading and is required for effective antiviral response. Front. Immunol. 13:1042463. doi: 10.3389/fimmu.2022.1042463
Received: 12 September 2022; Accepted: 27 September 2022;
Published: 13 October 2022.
Edited by:
Sergio C. Oliveira, Federal University of Minas Gerais, BrazilReviewed by:
Tomozumi Imamichi, National Cancer Institute at Frederick (NIH), United StatesCarlos Maluquer De Motes, University of Surrey, United Kingdom
Copyright © 2022 Patricio, Dias, Granella, Trigg, Teague, Bittencourt, Báfica, Zanotto-Filho, Ferguson and Mansur. This is an open-access article distributed under the terms of the Creative Commons Attribution License (CC BY). The use, distribution or reproduction in other forums is permitted, provided the original author(s) and the copyright owner(s) are credited and that the original publication in this journal is cited, in accordance with accepted academic practice. No use, distribution or reproduction is permitted which does not comply with these terms.
*Correspondence: Daniel Santos Mansur, ZGFuaWVsLm1hbnN1ckB1ZnNjLmJy; bWFuc3VyZHNAZ29vZ2xlbWFpbC5jb20=