- 1Department of Oncology, The First Affiliated Hospital of Dalian Medical University, Dalian, China
- 2Department of Oncology, Affiliated Zhongshan Hospital of Dalian University, Dalian, China
Innate immune cells in the tumor microenvironment (TME) mainly include macrophages, neutrophils, natural killer cells, dendritic cells and bone marrow derived suppressor cells. They play an anti-tumor or pro-tumor role by secreting various cytokines, chemokines and other factors, and determine the occurrence and development of tumors. Comprehending the role of innate immune cells in tumorigenesis and progression can help improve therapeutic approaches targeting innate immune cells in the TME, increasing the likelihood of favorable prognosis. In this review, we discussed the cell biology of innate immune cells, their role in tumorigenesis and development, and the current status of innate immune cell-based immunotherapy, in order to provide an overview for future research lines and clinical trials.
Introduction
The tumor microenvironment (TME) is a complex environment, mainly including tumor cells and their surrounding immune cells, tumor-related fibroblasts, vascular endothelial cells, etc. These cellular interactions enable tumor cells to evade immune surveillance, and are the cytological mechanism of tumor progression and metastasis. There is accumulating evidence that innate immune cells (macrophages, neutrophils, natural killer cells, dendritic cells and bone marrow-derived suppressor cells) and adaptive immune cells (T cells and B cells) are important components of the TME, which are involved in oncogenesis and tumor progression. In particular, innate immune cells can functionally shape their microenvironment by secreting various cytokines, chemokines, and other factors, affecting tumor survival and development. In this review, we summarized the origin and phenotype of innate immune cells in the TME, their role in tumorigenesis and development, and the immunotherapy for tumor suppression based on innate immune cells.
Macrophages
Origin and phenotype
In humans, peripheral blood monocytes form two major populations: CD14hi CD16lo and CD14lo CD16hi. In response to chemokines and growth factors produced by stromal and tumor cells in the tumor microenvironment, peripheral blood monocytes are locally recruited and differentiate into tumor-associated macrophages (TAMs). As a kind of innate immune cells in TME, TAM can be polarized into two types, M1 and M2. “M1” is induced by lipopolysaccharide (LPS) and interferon (IFN), and plays an anti-tumor effect. While type “M2” is induced by Interleukin-4 (IL-4) or Interleukin-13 (IL-13), and has a tumor-promoting effect (1). During carcinogenesis, macrophages initially exhibit antitumor M1-like polarization to eliminate more tumor cells. However, with tumor progression, macrophages polarize towards M2-like, promoting tumor development. Studies have shown that well-differentiated TAMs are associated with poor prognosis and decreased overall survival (2).
The role of TAMs in the process of tumor development
TAMs in the TME promote tumor progression in different ways, such as stimulating angiogenesis and lymphangiogenesis, inducing proliferation and epithelial-mesenchymal transition (EMT) of cancer cells, promoting destruction of basement membrane and remodeling of extracellular matrix (ECM), and inducing immunosuppression of immune cells with anti-tumor effects. For instance, M2-derived vascular endothelial growth factor-A (VEGF-A) contributes to the neovascularization and inflammatory cell recruitment at tumor sites in a mouse model of skin cancer (3). Reports about Merkel cell carcinoma, a highly malignant cutaneous neuroendocrine tumor, indicated that M2 expresses high levels of vascular endothelial growth factor-C (VEGF-C), which can promote lymphangiogenesis (4). Moreover, STAT3 and STAT6 cooperating with cathepsins secreted by macrophages, can disrupt the basement membrane and reshape the extracellular matrix (ECM), thereby enhancing tumor invasion and metastasis (5). In addition, macrophages can also inhibit the function of anti-tumor immune cells. For example, macrophages with the expression of PD-L1/PD-L2 and CD80/CD86, bind to PD-1 and CTLA4, leading to impairment of TCR signaling and suppression of cytotoxic functions of T cells, and promoting tumor evolution (6).
Although TAMs mainly play tumorigenic roles, they can exert anti-tumor effects at times. For instance, non-classically patrolled monocytes are actively recruited to lung metastases in a CX3CR 1-dependent manner, where they eliminate tumor substances, recruit and activate natural killer (NK) cells, thereby preventing tumor cell metastasis (7). Therefore, only by comprehending the role of macrophages in the TME on tumor progression, can it be better utilized in clinical treatment (Figure 1).
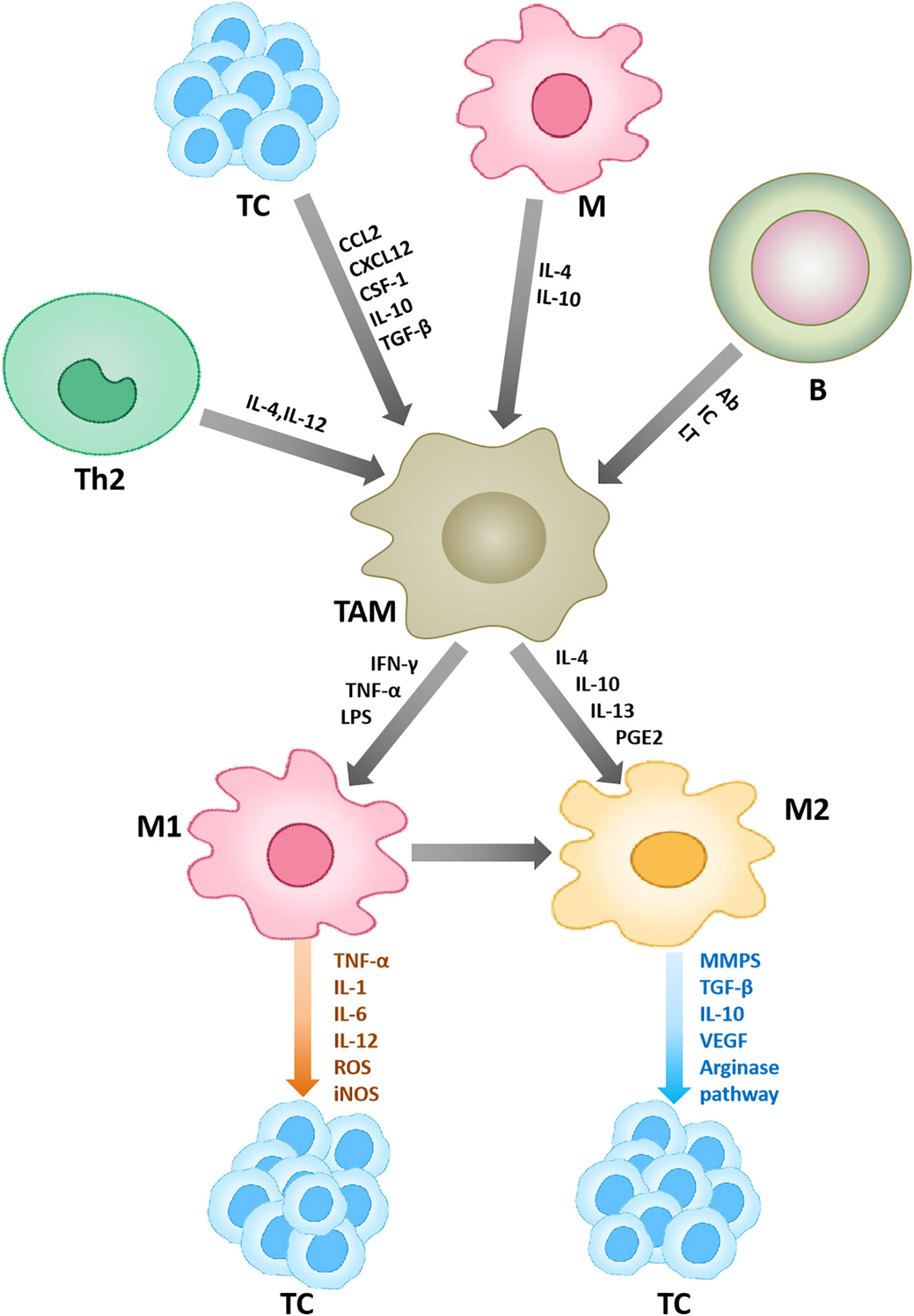
Figure 1 Macrophages in the tumor microenvironment. Other cells in the tumor microenvironment can affect the polarization and function of macrophages. Under the action of some cytokines, macrophages are polarized into two types, M1 and M2. M1 plays an anti-tumor role, and M2 plays a pro-tumor role. Ab, denotes antibodies; IC, denotes immune complexes; and LT, stands for leukotrienes.
The role of macrophages in tumor immunotherapy
At present, the researches on macrophage immunotherapy mainly include: immune checkpoint inhibitors, monoclonal antibodies, cell adoptive therapy, small molecule inhibitors, etc, aiming to directly reduce the numbers, prevent TAMs recruitment, enhance the phagocytic killing ability or target the surface molecule of TAMs.
Studies have found that certain drugs such as emactuzumab and IMC-CS4 can inhibit tumor development by reducing the number of macrophages (8, 9). In addition, primary tumors express CCL2 and CCR2, recruiting TAM. Therefore, blocking CCL2-CCR2 could inhibit TAM recruitment. CCR2 inhibitor (PF-04136309) and Dual CCR2/CCR5 antagonists (BMS-813160) have shown efficacy in preclinical data, but the results of clinical studies are dismal (10, 11). In addition, it was found that the phagocytosis of TAM was enhanced by immune checkpoint inhibitors such as PD-1/PD-L1 inhibitors, CD47 inhibitor (Hu5F9-G4), CD40 antibody (selicrelumab), and trastuzumab, reduced tumor burden (12–15). Furthermore, small molecule inhibitors inhibit tumors by targeting molecules associated with macrophages. For example, inhibitors targeting the indoleamine 2, 3-dioxygenase (IDO) molecule on the surface of macrophages have been tested in clinical trials with promising results (16). Finally, adoptive therapy can inhibit tumor progression as well. Klichinsky et al. discovered that single infusion of human chimeric antigen receptor-macrophages (CAR-Ms) reduces tumor burden and prolongs overall survival in two xenograft mouse models of solid tumors (17).
Neutrophils
Origin and phenotype
Neutrophils originate from hematopoietic stem cells and can be released from the bone marrow into the circulation after maturation. They are the most abundant leukocytes in the human circulation. In response to tissue injury and infection, neutrophils overflow from the circulation into tissues under the guidance of several cytokines. They can secrete inflammatory cytokines, release neutrophil extracellular traps (NETs), and phagocytose invading pathogenic microorganisms at the site of damage tissue (18). In the context of cancer, neutrophil migration to tumor tissue is regulated by the combined effects of granulocyte colony-stimulating factor (G-CSF), interleukin-17 (IL-17), and neutrophil chemokines. Neutrophils are transformed into tumor-associated neutrophils (TANs) after migrating into tumor tissues. TANs were identified as Ly6G+CD11b+ cells (19), classifing as either an N1 (tumor suppressing) or N2 (tumor promoting) phenotype (20).
The role of TANs in the process of tumor development
Stimulated by various factors in the TME, TANs can secrete a large number of growth factors, chemokines and cytokines to promote carcinogenesis and enhance the tumor invasion. For instance, in human pancreatic ductal adenocarcinoma (PDAC) tissues, TANs-derived transforming growth factor-beta (TGF-β) induces EMT in human lung cancer tissues through the transforming growth factor-beta (TGF-β)/Smad pathway, contributing to carcinogenesis (21, 22). In addition, in human breast cancer cell lines, neutrophils can secrete the cytokine oncostatin-M (OSM) under the induction of granulocyte-macrophage colony stimulating factor (GM-CSF), and OSM promotes vascular endothelial growth factor (VEGF) expression and increases the invasive ability of tumor cells (23, 24). On the other hand, in addition to the production of various factors, neutrophils can also release certain substances from their own stores, such as myeloperoxidase (MPO), neutrophil elastase (NE) and matrix metalloproteinases (MMPs), which affect the development of tumors. It has been reported that neutrophil-derived MPO can produce hypochlorous acid in large quantities, and induce mutations in adjacent epithelial cells by enhancing the M1dG pathway (25). Furthermore, TANs-derived MMPs, especially MMP-9, enhance tumor angiogenesis and tumor cell infiltration (26, 27).
Neutrophils also play a certain tumor suppressor in some cases. It has been shown that neutrophils can exert cytotoxic effects and inhibit tumor growth by producing H2O2 in mouse breast cancer models (28). In addition, neutrophils express Fcγ receptors that mediate the elimination of cancer cells via the antibody-dependent cell cytotoxicity (ADCC) mechanism (29, 30). For example, in the study of lymphoma treatment in mice, it was found that the neutrophil depletion reduces the efficacy of anti-CD52 monoclonal antibody (mAb) and anti-CD20 mAb (31). In addition, natural killer (NK) cells and macrophages have also been identified as potential effector cells in anti-CD20 mAb mediated tumor regression (32–34) (Figure 2).
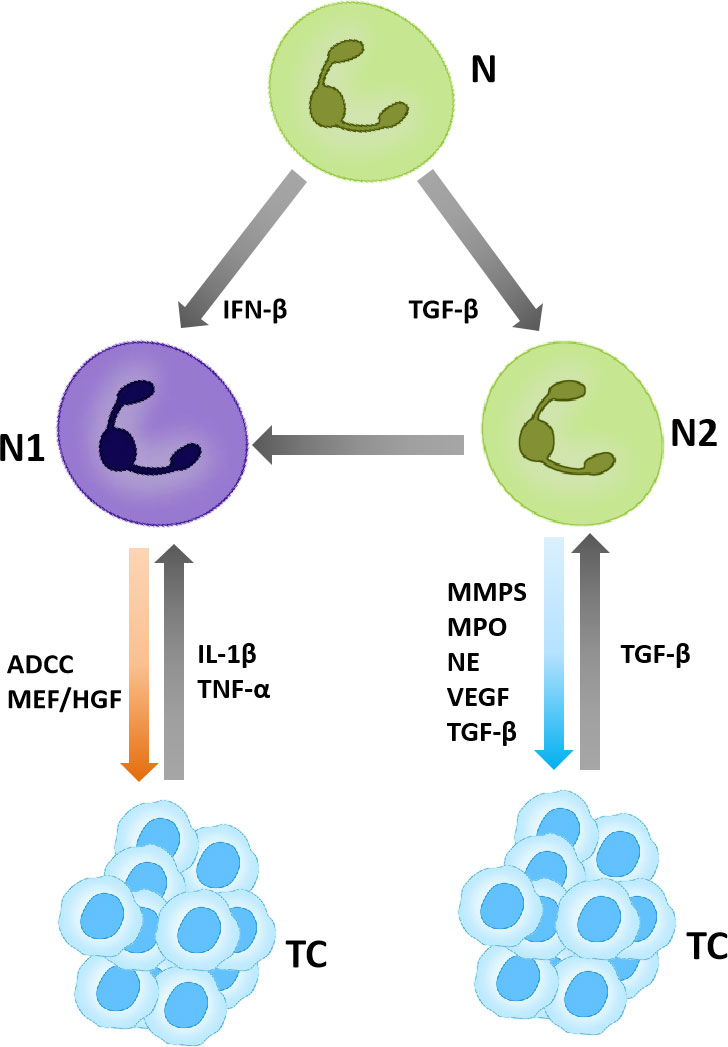
Figure 2 TANs in the tumor microenvironment. TANs are polarized into N1 and N2, in which N1 plays an anti-tumor role and N2 plays a pro-tumor role.
The role of TANs in tumor immunotherapy
TANs are one of the cellular targets of immunotherapy. Current research about targeted TANs mainly focuses on reducing the number of neutrophils, inhibiting the accumulation of neutrophils at tumor sites and changing the functional phenotype of neutrophils.
This idea of depleting neutrophil numbers has been implemented in preclinical models. However, this method will reduce the circulating neutrophils, which makes the host susceptible to infection, so it is limited in clinical application (35–37). Moreover, neutrophils will migrate toward the tumor in response to chemokines, especially CXCR2, CXCR4 and G-CSF/IL-17 axes. Therefore, targeting these molecules can reduce the accumulation of TANs at tumor sites and inhibit tumor progression. Studies indicate that inhibition of CXCR2 decreases TAN accumulation, reduces lung tumorigenesis, and inhibits PDAC metastasis in mouse models (38, 39). In addition, considering the plasticity of TAN, such as tumorigenic N2-like and antitumoral N1-like phenotypes, modification of TAN phenotype might be a more desirable anticancer therapy. This hypothesis has been supported by a mathematical model that achieves the phenotypic conversion of TANs from N2 to N1 through the use of TGF-β inhibitors for antitumor purposes (40). Finally, the suppressive effects of certain drugs targeting neutrophils, such as INCB001158 (Incyte Corporation) and IPH5401 (Innate Pharma), were found to reduce tumor burden (41, 42).
Natural killer cells
Origin and phenotype
Natural killer (NK) cells, derived from pluripotent hematopoietic stem cells in the bone marrow and developed from lymphoid progenitor cells and NK/T cell precursors, belong to the innate lymphoid cell family and play a key role in defense against viruses and tumors. NK cells can be found both in the blood and in various lymphoid and non-lymphoid organs. Traditionally, NK cells in peripheral blood can be subdivided into two main subsets: CD56brightCD16dim and CD56dimCD16bright. The former is considered immature and cytokine producers, while the latter shows more maturity and is the most cytotoxic (43).
The role of NK cells in the process of tumor development
The effect of NK cells on tumors is mainly depends on the receptors expressed on their surface. These receptors fall into two main categories: activating receptors (such as NKRs) and inhibitory receptors (such as KIRs), which control the activation of NK. In healthy cells, there is no or low expression of ligands for NK cell activating receptors, and NK cells are inhibited (44). In contrast, tumorigenic or virus-infected cells express high levels of ligands for NK cell activating receptors, and NK cells are activated (45).
In the TME, activated NK cells play a killing role on tumor cells, mainly through two mechanisms: direct killing effect (natural cytotoxicity) and ADCC pathway. In the former, NK cells can recognize activating ligands on the surface of tumor cells. In the ADCC pathway, NK cell receptors bind the Fc portion of IgG antibodies to antigenic molecules on the surface of target cells. Both of these approaches lead to phosphorylation of immunoreceptor tyrosine activation moieties (ITAMs) in the cytoplasm domain of receptors, which initiates NK cell activation. Activated NK cells can kill tumor cells by directly releasing cytotoxic granules containing granzymes and perforin or by inducing death receptor-mediated apoptosis via the expression of Fas ligand or tumor necrosis factor-related apoptosis-inducing ligand (45, 46). In addition to the ITAM pathway, there is also the PI3K pathway associated with its killing ability (47, 48). In addition to killing tumor cells in the above two ways, NK cells secrete chemokines and cytokines to play an immunomodulatory function as well. studies indicated that NK cells can also produce chemokines, C-C-motif ligands such as CCL3, CCL4, CCL5, X-C-motif chemokine ligand 1 (XCL1) and C-X-C-motif chemokine ligand 8 (CXCL8), which attract a variety of immune cells to transformed tissues and inhibit tumor progression (49). However, in addition to playing an anti-tumor role, certain subtypes of NK cells may contribute to tumor development. For example, IL6-induced overexpression of CD39 on NK cells is associated with poor prognosis in ESCC (50). In addition, some studies have shown that the poor prognosis of some cancers such as lymphoma, breast cancer and gastric cancer is related to natural killer cells, which may be related to the limited killing ability of NK cells. The detailed mechanism needs to be further explored (51–53) (Figure 3).
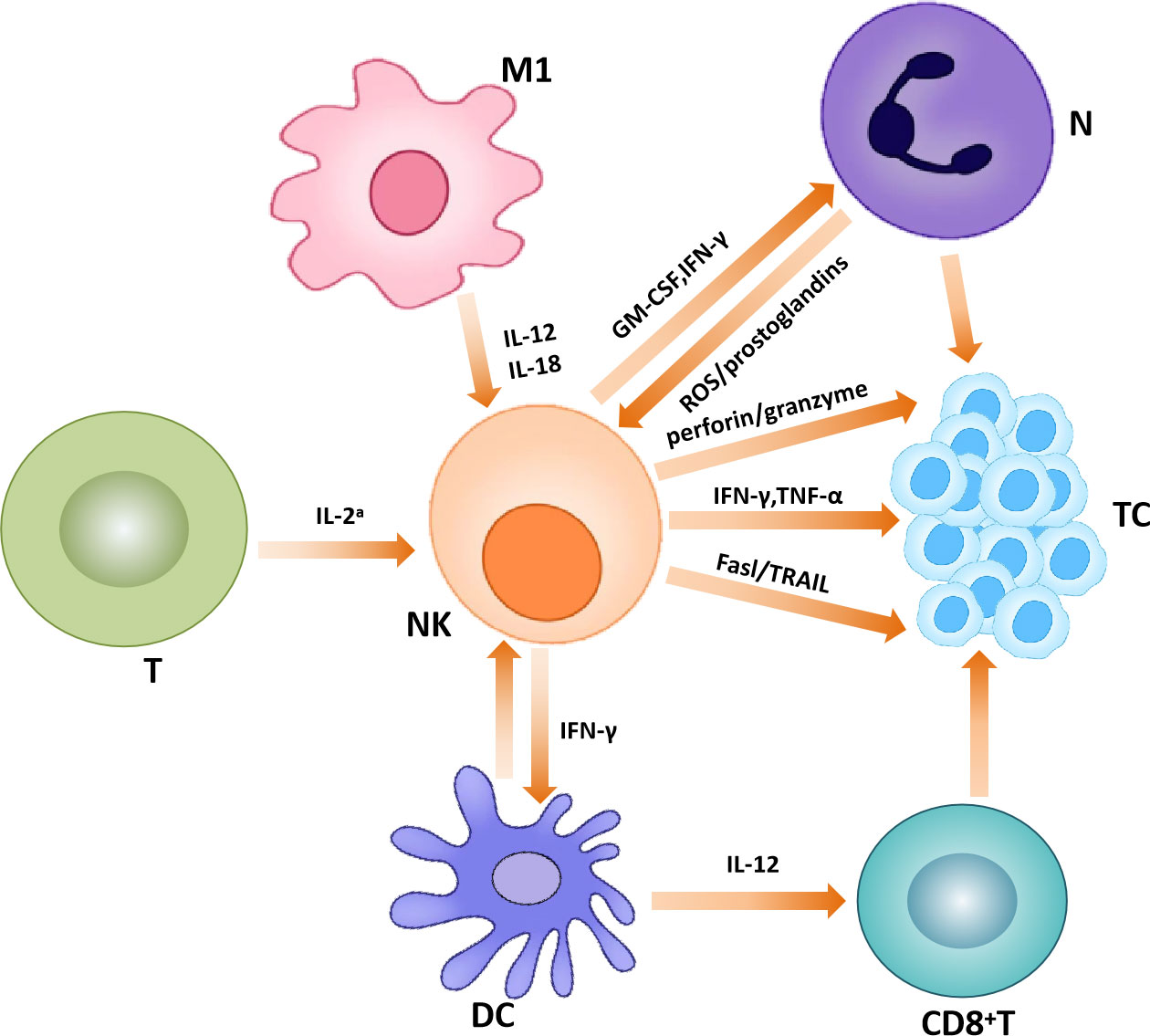
Figure 3 Natural killer cells in the tumor microenvironment. Natural killer cells interact with other immune cells to exert anti-tumor effects.
The role of NK cells in tumor immunotherapy
In recent years, the research on NK cell immunotherapy has flourished, and the latest progress mainly focuses on cytokine supplementation, monoclonal antibodies, modification of internal signaling pathways, adoptive transfer of NK cells and genetic engineering, etc.
Supplementation of cytokines such as IL-2, IL-15, IL-18 and IL-21 can improve the activity of NK cells and enhance their killing ability on tumors. For example, recombinant human IL-15 and its agonist ALT803 have great therapeutic efficacy in metastatic lung cancer (54, 55). ALT-803 combined with anti-PD-1 monoclonal antibody shows better therapeutic potential than ALT803 alone (56, 57). Furthermore, in one study, NK-cell dysregulation and cytotoxicity were restored in patients with mesothelial carcinoma treated with the monoclonal antibody against CTLA4, telemumab, indicating that blockade of CTLA4 prevents NK-cell dysfunction, slows tumor growth, and improves overall survival among patients with tumors (58). In addition, as an option to enhance autoimmunity, adoptive transfer of NK cells has been used for the treatment of certain types of cancers, such as medulloblastoma and ependymoma in children. In this study, medulloblastoma upregulates ligands of NK cell-activating receptors such as NKG2D and natural cytotoxic receptors (NCRs), thereby inducing NK cell-mediated cytotoxicity and medulloblastoma cell apoptosis, demonstrating the feasibility and safety of intraventricular infusion of autologous NK cells. It also provides a theoretical basis for local-regional delivery of NK cells (59). Finally, CAR-NK, which is genetically modified by chimeric antigen receptors (CARs) on immune cells, directly targets tumor-specific antigens, and has shown promising results in preclinical studies in ovarian, breast, prostate, and colon cancers (60).
Dendritic cells
Origin and phenotype
Dendritic cells (DCs) are typical antigen-presenting cells (APC) in the immune system, which connect the adaptive and innate immune systems to initiate and maintain T cell-mediated antitumor immune responses. DCs originate from common myeloid progenitor cells (CMPs). Driven by the expression of the transcription factor Nur77, CMPs differentiate into monocytic dendritic cells (moDCs) under inflammatory conditions (61–63). In the absence of Nur77, CMPs differentiate into common dendritic cell progenitors (CDPs), resulting in plasmacytoid dendritic cells (pDCs) and conventional dendritic cells (cDCs) (61). In addition, cDCs can be further divided into two subsets termed type I (cDC1) and type II (cDC2) conventional DCs (64). CD123, CD303, CD304, and CD45RA are expressed in pDCs. cDC1 and cDC2 express CD141, DEC205,Clec9A, XCR1 and CD1c,CD1a,CD103, respectively (65).
The role of DCs in the process of tumor development
DCs can continuously take up antigens within the TME and sense danger signals through pattern recognition receptors (PRRs) to recognize damage-related molecular patterns (DAMPs) from malignant cells. These signals enable DCs to initiate tolerance and immunogenicity properties in a coordinated, and subset-specific manner in general (66). Therefore, in order to better understand the role of DCs in the TME, further exploration is needed on the function of DC subsets.
As the most extensively studied subset of DCs, cDC1 can phagocytose exogenous antigens and other cellular debris released by tumors, and cross-present on major histocompatibility complex class I (MHC-I) to prime tumor-specific CD8+T cells (67). In addition, cDC1 can interact with natural killer (NK) cells to exert anti-tumor effects. For example, IL-12 and IL-15 produced by cDC1 can enhance the killing ability of NK cells (68). While natural killer cells produce cytokines and chemokines, such as XCL1 and CCR5, to recruit more cDC1 to TME (69). The subset of cDC2 have strong heterogeneity and functional diversity, thus their role in cancer has not been clarified. However, several researches have also indicated that cDC2 are involved in cross-presenting antigens and driving T-cell responses (70, 71). The subset of pDCs is mainly involved in antiviral responses. However, it has been found that pDCs can induce the expression of programmed cell death protein 1 ligand 1 (PD-L1) or granzyme B in malignant tumors, regulating Treg cells to drive immune tolerance (72–74). The role of MoDC in tumors is not well characterized, and may be related to cross-presentation of relevant antigens and initiation of CD8+T cell responses. In the melanoma mouse study, tumor-derived MoDCs can efficiently cross-present tumor antigens and are able to induce significant CTL proliferation (75) (Figure 4).
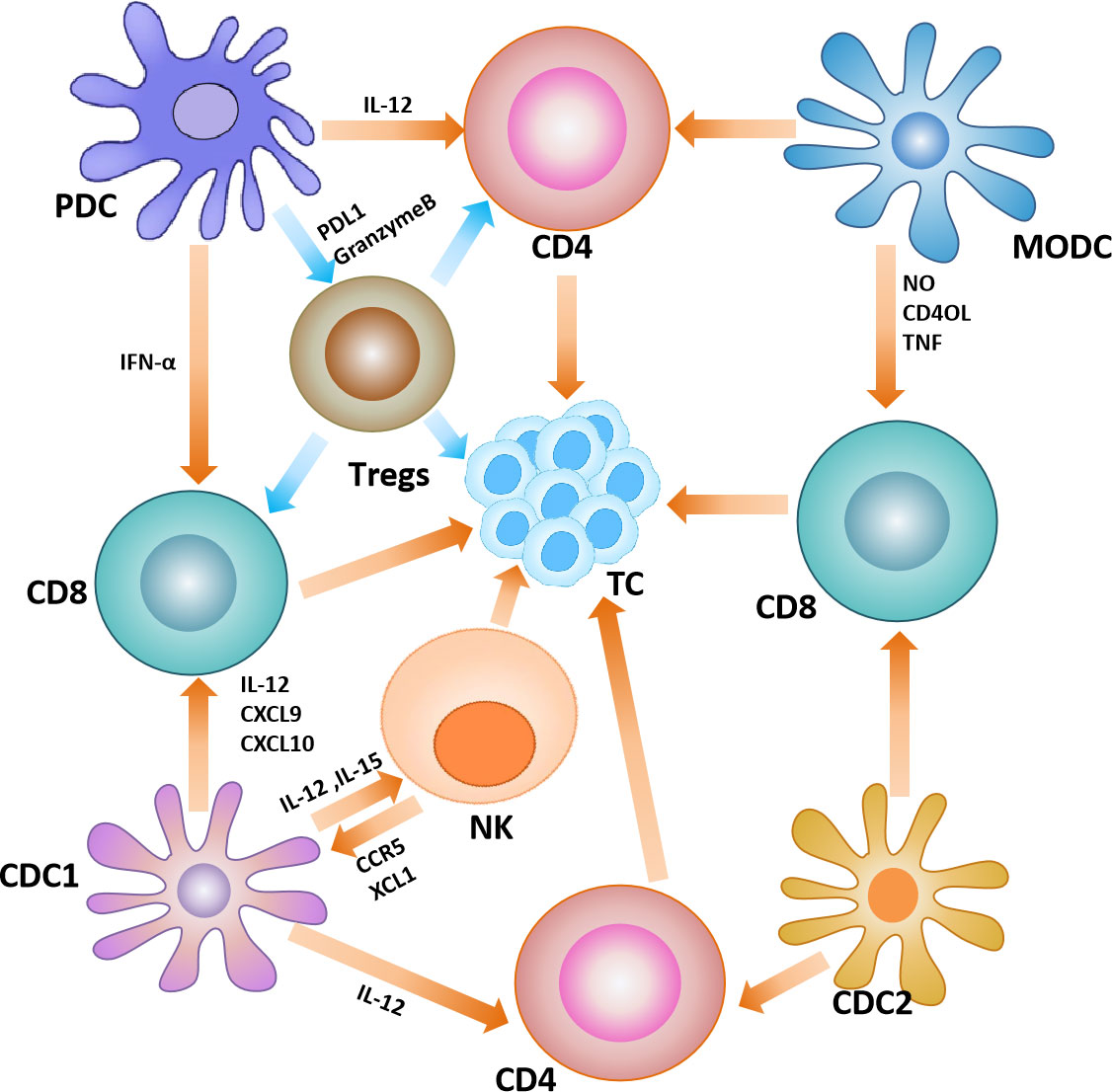
Figure 4 Dendritic cells in the tumor microenvironment. Interactions between dendritic cells and other immune cells in the tumor microenvironment, where yellow arrows represent anti-tumor effects and blue arrows represent pro-tumor effects.
The role of DCs in tumor immunotherapy
With accumulating evidence that DCs play a critical role in initiating antitumor immunity, there has been increasing interest in improving the status and numbers of DCs to suppress tumor progression. Currently, the following immune-related methods are mainly being applied: activation of DCs in vivo, blocking inhibitory signals, in vivo expansion, DC vaccines and so on.
Activation in vivo is achieved by providing exogenous activation signals, suggesting that DCs can be activated with specific agonists to shape the optimal antitumor response. Furthermore, blocking the inhibitory signals of cDCs is similar to activation in vivo. One of the most common examples is the application of VEGF inhibitors, which have been demonstrated to enhance DC maturation in patients (76, 77). In addition, tumors can be suppressed by increasing the number of DCs. For instance, systemic injection of the growth factor fms-like tyrosine kinase 3 ligand (Flt3L) has been shown in preclinical studies to cause expansion and activation of the cDC1 population, dramatically enhancing the clinical response to checkpoint and BRAF blockade and significantly delaying tumor growth (78). Alternatively, targeting DC by vaccines is another therapeutic option. The most common one is the whole-cell vaccines. For example, DCVax-L, a DC vaccine against glioblastoma, which has been tested in phase III clinical trials and has shown prolonged overall survival in patients (79). In addition, another example is that a neoantigen dendritic cell vaccine combined with anti-CD38 and CpG can produce anti-tumor immunity against immune checkpoint therapy resistant mouse lung cancer cell lines, which further develops DC vaccine therapy (80). DC vaccine is a promising immunotherapy method, and relevant clinical trials are ongoing (Table 1).
Myeloid-derived suppressor cells
Origin and phenotype
Myeloid derived suppressor cells (MDSCs) are a major regulatory cell population originating from myeloid progenitor cells that can be activated by tumor-derived factors in the TME. The diversity of phenotypic characteristics of MDSC subsets is determined by the differences in soluble factors that mobilize MDSCs. MDSCs can be roughly divided into three subgroups: polymorphonuclear MDSCs (PMN-MDSCs), monocyte MDSCs (Mo-MDSCs), and early MDSCs(E-MDSCs). The phenotypes of Mo-MDSCs were CD11b+, LY6Chi, and LY6G-; PMN-MDSCs showed CD11b+, LY6Clo, and LY6G+ phenotypes; while E-MDSCs expressed CD13-, CD14-, and CD33+ phenotypes (89). Notably, both of Mo-MDSCs and PMN-MDSCs present in the TME have enhanced inhibitory phenotypes compared with MDSCs present in peripheral lymphoid organs, which is due to the increased expression of inhibitory molecules by MDSCs in the TME.
The role of MDSCs in the process of tumor development
As one of the components of the TME, MDSCs have strong immunosuppressive potential. On the one hand, MDSCs establish an immunosuppressive TME through the production of various metabolites such as ROS and nitric oxide, which inhibit the antitumor function of T cells and NK cells. For example, MDSCs induce the production of IDO and immunosuppressive cytokines that promote tumor evolution by suppressing cytotoxic T lymphocytes, DCs and NK cells, and enhancing the effects of Tregs (90). For example, MDSC inhibits effector T cell proliferation and antitumor activity by producing ROS, arginase, and NO (91–93). MDSC inhibits the cytotoxicity of natural killer (NK) cells by inhibiting IL-2-mediated activation of NK cells or direct secretion of IL-10 (81, 82, 94). MDSC can also induce Treg differentiation and promote tumor development by secreting IL-10 and TGF-β (83). On the other hand, MDSCs contribute to tumor development by creating a premetastatic niche that promotes neovascular invasion and tumor cell growth. For instance, MDSCs can secrete an important pro-angiogenic factor (Bv8) at the tumor sites to promote tumor angiogenesis (84). In addition, in a mouse melanoma model, PMN-MDSCs were found to infiltrate primary tumors and induce epithelial-mesenchymal transition (EMT) through EGF and HGF signaling pathways to promote cancer cell spread under the induction of chemokine CXCL5 (85) (Figure 5).
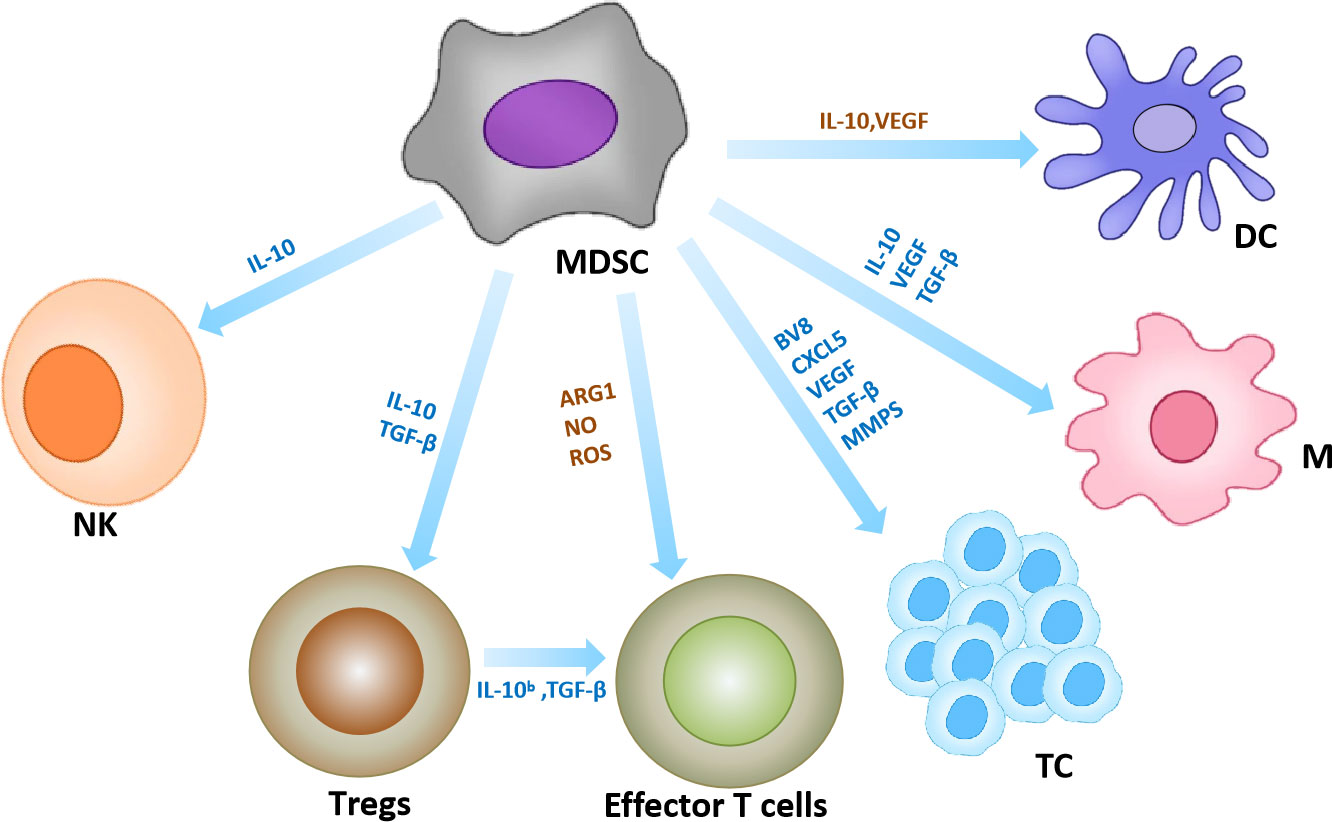
Figure 5 MDSCs in the tumor microenvironment. Interaction of MDSC with other immune cells. Yellow arrows represent anti-tumor effects and blue arrows represent pro-tumor effects. (95, 96).
The role of MDSCs in tumor immunotherapy
Since MDSCs have immune-suppressing effects in a variety of cancers, altering these cellular inhibitory effects is considered a promising approach for cancer treatment. Several strategies are being developed that can be broadly classified into four categories: inducing the differentiation of MDSCs into mature myeloid cells; reducing/consuming the number of MDSCs; blocking the development of MDSCs; inhibiting the immunosuppressive activity of MDSCs.
All-trans retinoic acid (ATRA) has been identified as a drug that induces the differentiation of MDSCs into mature myeloid cells. ATRA can bind to retinoic acid receptors and block signal transduction, leading to the differentiation and maturation of MDSCs. For example, in patients with metastatic renal cancer, ATRA combined with IL-2 resulted in significantly lower MDSCs and improved immune responses compared with untreated patients (86, 97). In addition, several chemotherapeutic agents such as 5-fluorouracil (5FU), paclitaxel, cisplatin and gemcitabine can deplete MDSCs and enhance the antitumor immune activity of T cells (87, 88, 98). Alternatively, it has also been reported that tyrosine kinase inhibitors reduce the number of MDSCs in renal cell carcinoma (RCC) patients and hepatocellular carcinoma (HCC) by blocking VEGF, c-KIT, and STAT-3 (99–101). Finally, inhibiting the immunosuppressive activity of MDSCs. For instance, cyclooxygenase 2 (COX-2)/prostaglandin E2 (PGE2) axis signaling antagonism has effectively reduced MDSCs recruitment and differentiation, and inhibited the production of MDSCs related inhibitors such as arginase 1 and ROS, thereby controlling tumor progression (102).
Conclusion and prospects
Innate immune cells in TME play an important role in the occurrence and development of tumors, and even show dual effects of tumor promotion and tumor inhibition under different microenvironments and stimulators. The tumor-promoting effect shows that they can increase the stemness of tumor cells, promote angiogenesis, induce drug resistance, inhibit immunity and promote tumor growth through phenotypic changes and secretion of certain substances. Each link may become the target for clinical treatment. We summarize some drugs and treatments that target innate immune cells. (Table 1). An in-depth comprehending on the role of innate immune cells and related molecules will not only provide important insights into the biological behavior of different types of tumors, but also provide a necessary foundation for the development of new innate immune cell-based therapies to manage and control the cancers. Although the current immunotherapy has achieved vast strides both in improving patients’ survival and their quality of life, more and more problems have emerged, such as the narrow anti-tumor spectrum, increased adverse reactions, and easy drug resistance, etc. These defects limit the practical application of immunotherapy in the clinic. Meanwhile, the complex components and heterogeneity of the TME also pose great challenges for immunotherapy. In the future, the mechanism of immune cells on tumor development should be further explored, the heterogeneity of TME should be deeply understood and studied, more accurate tumor markers should be sought, new methods of combination therapy should be explored. Thereby, formulating the more accurate and effective individualized strategies for treatment.
Data availability statement
The original contributions presented in the study are included in the article/supplementary materials. Further inquiries can be directed to the corresponding authors.
Author contributions
All authors listed have made a substantial, direct, and intellectual contribution to the work and approved it for publication.
Funding
This work was supported by XC, the host of the Capacity Construction Project of Major Clinical (specialized) Departments of Traditional Chinese Medicine of Liaoning Province (No.LNZYXZK201909), and the host of the Distinguished Professor Program of Liaoning Province. And YL, the host of the Dalian Medical Science Research Program (No.2011043).
Conflict of interest
The authors declare that the research was conducted in the absence of any commercial or financial relationships that could be construed as a potential conflict of interest.
Publisher’s note
All claims expressed in this article are solely those of the authors and do not necessarily represent those of their affiliated organizations, or those of the publisher, the editors and the reviewers. Any product that may be evaluated in this article, or claim that may be made by its manufacturer, is not guaranteed or endorsed by the publisher.
References
1. Mantovani A, Sozzani S, Locati M, Allavena P, Sica A. Macrophage Polarization:Tumor-associated macrophages as a paradigm for polarized M2 mononuclear phagocytes. Trends Immunol (2002) 23(11):549–55. doi: 10.1016/s1471-4906(02)02302-5
2. Bingle L, Brown NJ, Lewis CE. The role of tumour-associated macrophages in tumour implications for new anticancer therapies. J Pathol (2002) 196(3):254–65. doi: 10.1002/path.1027
3. Linde N, Lederle W, Depner S, van Rooijen N, Gutschalk CM, Mueller MM. Vascular endothelial growth factor-induced skin carcinogenesis depends on recruitment and alternative activation of macrophages. J Pathol (2012) 227(1):17–28. doi: 10.1002/path.3989
4. Werchau S, Toberer F, Enk A, Dammann R, Helmbold P. Merkel cell carcinoma induces lymphatic microvessel formation. J Am Acad Dermatol (2012) 67(2):215–25. doi: 10.1016/j.jaad.2011.09.002
5. Yan D, Wang HW, Bowman RL, Joyce JA. Stat3 and Stat6 signaling pathways synergize to promote cathepsin secretion from macrophages via Ire1alpha activation. Cell Rep (2016) 16(11):2914–27. doi: 10.1016/j.celrep.2016.08.035
6. Kuang DM, Zhao Q, Peng C, Xu J, Zhang JP, Wu C, et al. Activated monocytes in peritumoral stroma of hepatocellular carcinoma foster immune privilege and disease progression through pd-L1. J Exp Med (2009) 206(6):1327–37. doi: 10.1084/jem.20082173
7. Hanna RN, Cekic C, Sag D, Tacke R, Thomas GD, Nowyhed H, et al. Patrolling monocytes control tumor metastasis to the lung. Science (2015) 350(6263):985–90. doi: 10.1126/science.aac9407
8. Gomez-Roca C, Cassier P, Zamarin D, Machiels JP, Luis Perez Gracia J, Stephen Hodi F, et al. Anti-Csf-1r emactuzumab in combination with anti-Pd-L1 atezolizumab in advanced solid tumor patients naive or experienced for immune checkpoint blockade. J Immunother Cancer (2022) 10(5). doi: 10.1136/jitc-2021-004076
9. Dowlati A, Harvey RD, Carvajal RD, Hamid O, Klempner SJ, Kauh JSW, et al. Ly3022855, an anti-colony stimulating factor-1 receptor (Csf-1r) monoclonal antibody, in patients with advanced solid tumors refractory to standard therapy: Phase 1 dose-escalation trial. Invest New Drugs (2021) 39(4):1057–71. doi: 10.1007/s10637-021-01084-8
10. Noel M, O'Reilly EM, Wolpin BM, Ryan DP, Bullock AJ, Britten CD, et al. Phase 1b study of a small molecule antagonist of human chemokine (C-c motif) receptor 2 (Pf-04136309) in combination with nab-Paclitaxel/Gemcitabine in first-line treatment of metastatic pancreatic ductal adenocarcinoma. Invest New Drugs (2020) 38(3):800–11. doi: 10.1007/s10637-019-00830-3
11. Norman P. A dual CCR2/CCR5 chemokine antagonist, BMS-813160? evaluation of WO2011046916. Expert Opin Ther Pat. (2011) 21(12):1919–24. doi: 10.1517/13543776.2011.622750
12. Advani R, Flinn I, Popplewell L, Forero A, Bartlett NL, Ghosh N, et al. Cd47 blockade by Hu5f9-G4 and rituximab in non-hodgkin's lymphoma. N Engl J Med (2018) 379(18):1711–21. doi: 10.1056/NEJMoa1807315
13. Byrne KT, Betts CB, Mick R, Sivagnanam S, Bajor DL, Laheru DA, et al. Neoadjuvant selicrelumab, an agonist Cd40 antibody, induces changes in the tumor microenvironment in patients with resectable pancreatic cancer. Clin Cancer Res (2021) 27(16):4574–86. doi: 10.1158/1078-0432.CCR-21-1047
14. Gordon SR, Maute RL, Dulken BW, Hutter G, George BM, McCracken MN, et al. Pd-1 expression by tumour-associated macrophages inhibits phagocytosis and tumour immunity. Nature (2017) 545(7655):495–9. doi: 10.1038/nature22396
15. Shi Y, Fan X, Deng H, Brezski RJ, Rycyzyn M, Jordan RE, et al. Trastuzumab triggers phagocytic killing of high Her2 cancer cells in vitro and in vivo by interaction with fcgamma receptors on macrophages. J Immunol (2015) 194(9):4379–86. doi: 10.4049/jimmunol.1402891
16. Adams JL, Smothers J, Srinivasan R, Hoos A. Big opportunities for small molecules in immuno-oncology. Nat Rev Drug Discovery (2015) 14(9):603–22. doi: 10.1038/nrd4596
17. Klichinsky M, Ruella M, Shestova O, Lu XM, Best A, Zeeman M, et al. Human chimeric antigen receptor macrophages for cancer immunotherapy. Nat Biotechnol (2020) 38(8):947–53. doi: 10.1038/s41587-020-0462-y
18. Uribe-Querol E, Rosales C. Neutrophils in cancer: Two sides of the same coin. J Immunol Res (2015) 2015:983698. doi: 10.1155/2015/983698
19. Sagiv JY, Michaeli J, Assi S, Mishalian I, Kisos H, Levy L, et al. Phenotypic diversity and plasticity in circulating neutrophil subpopulations in cancer. Cell Rep (2015) 10(4):562–73. doi: 10.1016/j.celrep.2014.12.039
20. Fridlender ZG, Sun J, Kim S, Kapoor V, Cheng G, Ling L, et al. Polarization of tumor-associated neutrophil phenotype by tgf-beta: "N1" versus "N2" tan. Cancer Cell (2009) 16(3):183–94. doi: 10.1016/j.ccr.2009.06.017
21. Aoyagi Y, Oda T, Kinoshita T, Nakahashi C, Hasebe T, Ohkohchi N, et al. Overexpression of tgf-beta by infiltrated granulocytes correlates with the expression of collagen mrna in pancreatic cancer. Br J Cancer (2004) 91(7):1316–26. doi: 10.1038/sj.bjc.6602141
22. Hu P, Shen M, Zhang P, Zheng C, Pang Z, Zhu L, et al. Intratumoral neutrophil granulocytes contribute to epithelial-mesenchymal transition in lung adenocarcinoma cells. Tumour Biol (2015) 36(10):7789–96. doi: 10.1007/s13277-015-3484-1
23. Queen MM, Ryan RE, Holzer RG, Keller-Peck CR, Jorcyk CL. Breast cancer cells stimulate neutrophils to produce oncostatin m: Potential implications for tumor progression. Cancer Res (2005) 65(19):8896–904. doi: 10.1158/0008-5472.CAN-05-1734
24. Simonneau M, Frouin E, Huguier V, Jermidi C, Jégou JF, Godet J, et al. Oncostatin m is overexpressed in skin squamous-cell carcinoma and promotes tumor progression. Oncotarget (2018) 9(92):36457–73. doi: 10.18632/oncotarget.26355
25. Gungor N, Knaapen AM, Munnia A, Peluso M, Haenen GR, Chiu RK, et al. Genotoxic effects of neutrophils and hypochlorous acid. Mutagenesis (2010) 25(2):149–54. doi: 10.1093/mutage/gep053
26. Ardi VC, Kupriyanova TA, Deryugina EI, Quigley JP. Human neutrophils uniquely release TIMP-free MMP-9 to provide a potent catalytic stimulator of angiogenesis. Proc Natl Acad Sci U.S.A. (2007) 104(51):20262–7. doi: 10.1073/pnas.0706438104
27. Bekes EM, Schweighofer B, Kupriyanova TA, Zajac E, Ardi VC, Quigley JP, et al. Tumor-recruited neutrophils and neutrophil timp-free mmp-9 regulate coordinately the levels of tumor angiogenesis and efficiency of malignant cell intravasation. Am J Pathol (2011) 179(3):1455–70. doi: 10.1016/j.ajpath.2011.05.031
28. Oberg HH, Wesch D, Kalyan S, Kabelitz D. Regulatory interactions between neutrophils, tumor cells and T cells. Front Immunol (2019) 10:1690. doi: 10.3389/fimmu.2019.01690
29. Matlung HL, Babes L, Zhao XW, van Houdt M, Treffers LW, van Rees DJ, et al. Neutrophils kill antibody-opsonized cancer cells by trogoptosis. Cell Rep (2018) 23(13):3946–59.e6. doi: 10.1016/j.celrep.2018.05.082
30. Hubert P, Heitzmann A, Viel S, Nicolas A, Sastre-Garau X, Oppezzo P, et al. Antibody-dependent cell cytotoxicity synapses form in mice during tumor-specific antibody immunotherapy. Cancer Res (2011) 71(15):5134–43. doi: 10.1158/0008-5472.CAN-10-4222
31. van Egmond M, Bakema JE. Neutrophils as effector cells for antibody-based immunotherapy of cancer. Semin Cancer Biol (2013) 23(3):190–9. doi: 10.1016/j.semcancer.2012.12.002
32. Hatjiharissi E, Xu L, Santos DD, Hunter ZR, Ciccarelli BT, Verselis S, et al. Increased natural killer cell expression of CD16, augmented binding and ADCC activity to rituximab among individuals expressing the Fc{gamma}RIIIa-158 V/V and V/F polymorphism. Blood (2007) 110(7):2561–4. doi: 10.1182/blood-2007-01-070656
33. Minard-Colin V, Xiu Y, Poe JC, Horikawa M, Magro CM, Hamaguchi Y, et al. Lymphoma depletion during CD20 immunotherapy in mice is mediated by macrophage FcgammaRI, FcgammaRIII, and FcgammaRIV. Blood (2008) 112(4):1205–13. doi: 10.1182/blood-2008-01-135160
34. van der Bij GJ, Bögels M, Otten MA, Oosterling SJ, Kuppen PJ, Meijer S, et al. Experimentally induced liver metastases from colorectal cancer can be prevented by mononuclear phagocyte-mediated monoclonal antibody therapy. J Hepatol (2010) 53(4):677–85. doi: 10.1016/j.jhep.2010.04.023
35. Daley JM, Thomay AA, Connolly MD, Reichner JS, Albina JE. Use of Ly6G-specific monoclonal antibody to deplete neutrophils in mice. J Leukoc Biol (2008) 83(1):64–70. doi: 10.1189/jlb.0407247
36. Boivin G, Ancey PB, Vuillefroy de Silly R, Kalambaden P, Contat C, Petit B, et al. Anti-Ly6G binding and trafficking mediate positive neutrophil selection to unleash the anti-tumor efficacy of radiation therapy. Oncoimmunology (2021) 10(1):1876597. doi: 10.1080/2162402X.2021.1876597
37. Forero A, Bendell JC, Kumar P, Janisch L, Rosen M, Wang Q, et al. First-in-human study of the antibody DR5 agonist DS-8273a in patients with advanced solid tumors. Invest New Drugs (2017) 35(3):298–306. doi: 10.1007/s10637-016-0420-1
38. Steele CW, Karim SA, Leach JDG, Bailey P, Upstill-Goddard R, Rishi L, et al. Cxcr2 inhibition profoundly suppresses metastases and augments immunotherapy in pancreatic ductal adenocarcinoma. Cancer Cell (2016) 29(6):832–45. doi: 10.1016/j.ccell.2016.04.014
39. Gong L, Cumpian AM, Caetano MS, Ochoa CE, de la Garza MM, Lapid DJ, et al. Promoting effect of neutrophils on lung tumorigenesis is mediated by Cxcr2 and neutrophil elastase. Mol Cancer (2013) 12(1):154. doi: 10.1186/1476-4598-12-154
40. Kim Y, Lee D, Lee J, Lee S, Lawler S. Role of tumor-associated neutrophils in regulation of tumor growth in lung cancer development: A mathematical model. PloS One (2019) 14(1):e0211041. doi: 10.1371/journal.pone.0211041
41. Grobben Y, Uitdehaag JCM, Willemsen-Seegers N, Tabak WWA, de Man J, Buijsman RC, et al. Structural insights into human arginase-1 pH dependence and its inhibition by the small molecule inhibitor CB-1158. J Struct Biol X. (2019) 4:100014. doi: 10.1016/j.yjsbx.2019.100014
42. Quadrini KJ, Hegelund AC, Cortes KE, Xue C, Kennelly SM, Ji H, et al. Validation of a flow cytometry-based assay to assess C5aR receptor occupancy on neutrophils and monocytes for use in drug development. Cytometry B Clin Cytom. (2016) 90(2):177–90. doi: 10.1002/cyto.b.21260
43. Bjorkstrom NK, Ljunggren HG, Michaelsson J. Emerging insights into natural killer cells in human peripheral tissues. Nat Rev Immunol (2016) 16(5):310–20. doi: 10.1038/nri.2016.34
44. Handgretinger R, Lang P, Andre MC. Exploitation of natural killer cells for the treatment of acute leukemia. Blood (2016) 127(26):3341–9. doi: 10.1182/blood-2015-12-629055
45. Smyth MJ, Cretney E, Kelly JM, Westwood JA, Street SE, Yagita H, et al. Activation of nk cell cytotoxicity. Mol Immunol (2005) 42(4):501–10. doi: 10.1016/j.molimm.2004.07.034
46. Prager I, Watzl C. Mechanisms of natural killer cell-mediated cellular cytotoxicity. J Leukoc Biol (2019) 105(6):1319–29. doi: 10.1002/JLB.MR0718-269R
47. Li T, Yang Y, Song H, Li H, Cui A, Liu Y, et al. Activated NK cells kill hepatic stellate cells via p38/PI3K signaling in a TRAIL-involved degranulation manner. J Leukoc Biol (2019) 105(4):695–704. doi: 10.1002/JLB.2A0118-031RR
48. Cluff E, Magdaleno CC, Fernandez E, House T, Swaminathan S, Varadaraj A, et al. Hypoxia-inducible factor-1 alpha expression is induced by IL-2 via the PI3K/mTOR pathway in hypoxic NK cells and supports effector functions in NKL cells and ex vivo expanded NK cells. Cancer Immunol Immunother. (2022) 71(8):1989–2005. doi: 10.1007/s00262-021-03126-9
49. Gotthardt D, Sexl V. Stats in nk-cells: The good, the bad, and the ugly. Front Immunol (2016) 7:694. doi: 10.3389/fimmu.2016.00694
50. Zheng Y, Li Y, Tang B, Zhao Q, Wang D, Liu Y, et al. IL-6-induced CD39 expression on tumor-infiltrating NK cells predicts poor prognosis in esophageal squamous cell carcinoma. Cancer Immunol Immunother. (2020) 69(11):2371–80. doi: 10.1007/s00262-020-02629-1
51. Duault C, Kumar A, Taghi Khani A, Lee SJ, Yang L, Huang M, et al. Activated natural killer cells predict poor clinical prognosis in high-risk b- and T-cell acute lymphoblastic leukemia. Blood (2021) 138(16):1465–80. doi: 10.1182/blood.2020009871
52. Chen YY, Feng Y, Mao QS, Ma P, Liu JZ, Lu W, et al. Diagnostic and prognostic value of the peripheral natural killer cell levels in gastric cancer. Exp Ther Med (2020) 20(4):3816–22. doi: 10.3892/etm.2020.9101
53. Bouzidi L, Triki H, Charfi S, Kridis WB, Derbel M, Ayadi L, et al. Prognostic value of natural killer cells besides tumor-infiltrating lymphocytes in breast cancer tissues. Clin Breast Cancer. (2021) 21(6):e738–47. doi: 10.1016/j.clbc.2021.02.003
54. Conlon KC, Lugli E, Welles HC, Rosenberg SA, Fojo AT, Morris JC, et al. Redistribution, hyperproliferation, activation of natural killer cells and Cd8 T cells, and cytokine production during first-in-Human clinical trial of recombinant human interleukin-15 in patients with cancer. J Clin Oncol (2015) 33(1):74–82. doi: 10.1200/JCO.2014.57.3329
55. Conlon KC, Potter EL, Pittaluga S, Lee CR, Miljkovic MD, Fleisher TA, et al. IL15 by continuous intravenous infusion to adult patients with solid tumors in a phase I trial induced dramatic nk-cell subset expansion. Clin Cancer Res (2019) 25(16):4945–54. doi: 10.1158/1078-0432.CCR-18-3468
56. Romee R, Cooley S, Berrien-Elliott MM, Westervelt P, Verneris MR, Wagner JE, et al. First-in-Human phase 1 clinical study of the il-15 superagonist complex alt-803 to treat relapse after transplantation. Blood (2018) 131(23):2515–27. doi: 10.1182/blood-2017-12-823757
57. Wrangle JM, Velcheti V, Patel MR, Garrett-Mayer E, Hill EG, Ravenel JG, et al. Alt-803, an il-15 superagonist, in combination with nivolumab in patients with metastatic non-small cell lung cancer: A non-randomised, open-label, phase 1b trial. Lancet Oncol (2018) 19(5):694–704. doi: 10.1016/s1470-2045(18)30148-7
58. Sottile R, Tannazi M, Johansson MH, Cristiani CM, Calabro L, Ventura V, et al. Nk- and T-cell subsets in malignant mesothelioma patients: Baseline pattern and changes in the context of anti-Ctla-4 therapy. Int J Cancer (2019) 145(8):2238–48. doi: 10.1002/ijc.32363
59. Khatua S, Cooper LJN, Sandberg DI, Ketonen L, Johnson JM, Rytting ME, et al. Phase I study of intraventricular infusions of autologous ex vivo expanded nk cells in children with recurrent medulloblastoma and ependymoma. Neuro Oncol (2020) 22(8):1214–25. doi: 10.1093/neuonc/noaa047
60. Rezvani K, Rouce R, Liu E, Shpall E. Engineering natural killer cells for cancer immunotherapy. Mol Ther (2017) 25(8):1769–81. doi: 10.1016/j.ymthe.2017.06.012
61. Gardner A, Ruffell B. Dendritic cells and cancer immunity. Trends Immunol (2016) 37(12):855–65. doi: 10.1016/j.it.2016.09.006
62. Domínguez PM, Ardavín C. Differentiation and function of mouse monocyte-derived dendritic cells in steady state and inflammation. Immunol Rev (2010) 234(1):90–104. doi: 10.1111/j.0105-2896.2009.00876.x
63. Veglia F, Gabrilovich DI. Dendritic cells in cancer: The role revisited. Curr Opin Immunol (2017) 45:43–51. doi: 10.1016/j.coi.2017.01.002
64. Collin M, Bigley V. Human dendritic cell subsets: An update. Immunology (2018) 154(1):3–20. doi: 10.1111/imm.12888
65. Wylie B, Macri C, Mintern JD, Waithman J. Dendritic cells and cancer: From biology to therapeutic intervention. Cancers (Basel). (2019) 11(4):521. doi: 10.3390/cancers11040521
66. Joffre O, Nolte MA, Spörri R, Reis e Sousa C. Inflammatory signals in dendritic cell activation and the induction of adaptive immunity. Immunol Rev (2009) 227(1):234–47. doi: 10.1111/j.1600-065X.2008.00718.x
67. Albert ML, Sauter B, Bhardwaj N. Dendritic cells acquire antigen from apoptotic cells and induce class I-restricted ctls. Nature (1998) 392(6671):86–9. doi: 10.1038/32183
68. Mahmood S, Upreti D, Sow I, Amari A, Nandagopal S, Kung SK. Bidirectional interactions of NK cells and dendritic cells in immunotherapy: current and future perspective. Immunotherapy (2015) 7(3):301–8. doi: 10.2217/imt.14.122
69. Böttcher JP, Bonavita E, Chakravarty P, Blees H, Cabeza-Cabrerizo M, Sammicheli S, et al. NK cells stimulate recruitment of cDC1 into the tumor microenvironment promoting cancer immune control. Cell (2018) 172(5):1022–1037.e14. doi: 10.1016/j.cell.2018.01.004
70. Ruhland MK, Roberts EW, Cai E, Mujal AM, Marchuk K, Beppler C, et al. Visualizing synaptic transfer of tumor antigens among dendritic cells. Cancer Cell (2020) 37(6):786–99.e5. doi: 10.1016/j.ccell.2020.05.002
71. Binnewies M, Mujal AM, Pollack JL, Combes AJ, Hardison EA, Barry KC, et al. Unleashing type-2 dendritic cells to drive protective antitumor Cd4(+) T cell immunity. Cell (2019) 177(3):556–71.e16. doi: 10.1016/j.cell.2019.02.005
72. Diana J, Brezar V, Beaudoin L, Dalod M, Mellor A, Tafuri A, et al. Viral infection prevents diabetes by inducing regulatory T cells through NKT cell-plasmacytoid dendritic cell interplay. J Exp Med (2011) 208(4):729–45. doi: 10.1084/jem.20101692
73. Castellaneta A, Sumpter TL, Chen L, Tokita D, Thomson AW. NOD2 ligation subverts IFN-alpha production by liver plasmacytoid dendritic cells and inhibits their T cell allostimulatory activity via B7-H1 up-regulation. J Immunol (2009) 183(11):6922–32. doi: 10.4049/jimmunol.0900582
74. Jahrsdörfer B, Vollmer A, Blackwell SE, Maier J, Sontheimer K, Beyer T, et al. Granzyme b produced by human plasmacytoid dendritic cells suppresses T-cell expansion. Blood (2010) 115(6):1156–65. doi: 10.1182/blood-2009-07-235382
75. Diao J, Gu H, Tang M, Zhao J, Cattral MS. Tumor dendritic cells (Dcs) derived from precursors of conventional dcs are dispensable for intratumor ctl responses. J Immunol (2018) 201(4):1306–14. doi: 10.4049/jimmunol.1701514
76. Yu H, Pardoll D, Jove R. Stats in cancer inflammation and immunity: A leading role for Stat3. Nat Rev Cancer (2009) 9(11):798–809. doi: 10.1038/nrc2734
77. Nefedova Y, Huang M, Kusmartsev S, Bhattacharya R, Cheng P, Salup R, et al. Hyperactivation of Stat3 is involved in abnormal differentiation of dendritic cells in cancer. J Immunol (2004) 172(1):464–74. doi: 10.4049/jimmunol.172.1.464
78. Salmon H, Idoyaga J, Rahman A, Leboeuf M, Remark R, Jordan S, et al. Expansion and activation of Cd103(+) dendritic cell progenitors at the tumor site enhances tumor responses to therapeutic pd-L1 and braf inhibition. Immunity (2016) 44(4):924–38. doi: 10.1016/j.immuni.2016.03.012
79. van Willigen WW, Bloemendal M, Gerritsen WR, Schreibelt G, de Vries IJM, Bol KF. Dendritic cell cancer therapy: Vaccinating the right patient at the right time. Front Immunol (2018) 9:2265. doi: 10.3389/fimmu.2018.02265
80. Sun C, Nagaoka K, Kobayashi Y, Nakagawa H, Kakimi K, Nakajima J. Neoantigen dendritic cell vaccination combined with anti-Cd38 and cpg elicits anti-tumor immunity against the immune checkpoint therapy-resistant murine lung cancer cell line Llc1. Cancers (Basel) (2021) 13(21). doi: 10.3390/cancers13215508
81. Ibrahim ML, Klement JD, Lu C, Redd PS, Xiao W, Yang D, et al. Myeloid-derived suppressor cells produce IL-10 to elicit DNMT3b-dependent IRF8 silencing to promote colitis-associated colon tumorigenesis. Cell Rep (2018) 25(11):3036–3046.e6. doi: 10.1016/j.celrep.2018.11.050
82. Szkaradkiewicz A, Karpiński TM, Drews M, Borejsza-Wysocki M, Majewski P, Andrzejewska E. Natural killer cell cytotoxicity and immunosuppressive cytokines (IL-10, TGF-beta1) in patients with gastric cancer. J BioMed Biotechnol (2010) 2010:901564. doi: 10.1155/2010/901564
83. Gabrilovich DI, Ostrand-Rosenberg S, Bronte V. Coordinated regulation of myeloid cells by tumours. Nat Rev Immunol (2012) 12(4):253–68. doi: 10.1038/nri3175
84. Qu X, Zhuang G, Yu L, Meng G, Ferrara N. Induction of Bv8 expression by granulocyte colony-stimulating factor in Cd11b+Gr1+ cells: Key role of Stat3 signaling. J Biol Chem (2012) 287(23):19574–84. doi: 10.1074/jbc.M111.326801
85. Toh B, Wang X, Keeble J, Sim WJ, Khoo K, Wong WC, et al. Mesenchymal transition and dissemination of cancer cells is driven by myeloid-derived suppressor cells infiltrating the primary tumor. PloS Biol (2011) 9(9):e1001162. doi: 10.1371/journal.pbio.1001162
86. Nefedova Y, Fishman M, Sherman S, Wang X, Beg AA, Gabrilovich DI. Mechanism of all-trans retinoic acid effect on tumor-associated myeloid-derived suppressor cells. Cancer Res (2007) 67(22):11021–8. doi: 10.1158/0008-5472.CAN-07-2593
87. Vincent J, Mignot G, Chalmin F, Ladoire S, Bruchard M, Chevriaux A, et al. 5-fluorouracil selectively kills tumor-associated myeloid-derived suppressor cells resulting in enhanced T cell-dependent antitumor immunity. Cancer Res (2010) 70(8):3052–61. doi: 10.1158/0008-5472.CAN-09-3690
88. Sevko A, Michels T, Vrohlings M, Umansky L, Beckhove P, Kato M, et al. Antitumor effect of paclitaxel is mediated by inhibition of myeloid-derived suppressor cells and chronic inflammation in the spontaneous melanoma model. J Immunol (2013) 190(5):2464–71. doi: 10.4049/jimmunol.1202781
89. Bronte V, Brandau S, Chen SH, Colombo MP, Frey AB, Greten TF, et al. Recommendations for myeloid-derived suppressor cell nomenclature and characterization standards. Nat Commun (2016) 7:12150. doi: 10.1038/ncomms12150
90. Kolahian S, Oz HH, Zhou B, Griessinger CM, Rieber N, Hartl D. The emerging role of myeloid-derived suppressor cells in lung diseases. Eur Respir J (2016) 47(3):967–77. doi: 10.1183/13993003.01572-2015
91. Kusmartsev S, Nefedova Y, Yoder D, Gabrilovich DI. Antigen-specific inhibition of CD8+ T cell response by immature myeloid cells in cancer is mediated by reactive oxygen species. J Immunol (2004) 172(2):989–99. doi: 10.4049/jimmunol.172.2.989
92. Liu Y, Van Ginderachter JA, Brys L, De Baetselier P, Raes G, Geldhof AB. Nitric oxide-independent CTL suppression during tumor progression: association with arginase-producing (M2) myeloid cells. J Immunol (2003) 170(10):5064–74. doi: 10.4049/jimmunol.170.10.5064
93. Mazzoni A, Bronte V, Visintin A, Spitzer JH, Apolloni E, Serafini P, et al. Myeloid suppressor lines inhibit T cell responses by an NO-dependent mechanism. J Immunol (2002) 168(2):689–95. doi: 10.4049/jimmunol.168.2.689
94. Liu C, Yu S, Kappes J, Wang J, Grizzle WE, Zinn KR, et al. Expansion of spleen myeloid suppressor cells represses nk cell cytotoxicity in tumor-bearing host. Blood (2007) 109(10):4336–42. doi: 10.1182/blood-2006-09-046201
95. Zwirner NW, Domaica CI. Cytokine regulation of natural killer cell effector functions. Biofactors. (2010) 36(4):274–88. doi: 10.1002/biof.107
96. Huang B, Pan PY, Li Q, Sato AI, Levy DE, Bromberg J, et al. Gr-1+CD115+immature myeloid suppressor cells mediate the development of tumor-induced T regulatory cells and T-cell anergy in tumor-bearing host. Cancer Res. (2006) 66(2):1123–31. doi: 10.1158/0008-5472.CAN-05-1299.
97. Iclozan C, Antonia S, Chiappori A, Chen DT, Gabrilovich D. Therapeutic regulation of myeloid-derived suppressor cells and immune response to cancer vaccine in patients with extensive stage small cell lung cancer. Cancer Immunol Immunother (2013) 62(5):909–18. doi: 10.1007/s00262-013-1396-8
98. Eriksson E, Wenthe J, Irenaeus S, Loskog A, Ullenhag G. Gemcitabine reduces mdscs, tregs and tgfbeta-1 while restoring the Teff/Treg ratio in patients with pancreatic cancer. J Transl Med (2016) 14(1):282. doi: 10.1186/s12967-016-1037-z
99. Ko JS, Zea AH, Rini BI, Ireland JL, Elson P, Cohen P, et al. Sunitinib mediates reversal of myeloid-derived suppressor cell accumulation in renal cell carcinoma patients. Clin Cancer Res (2009) 15(6):2148–57. doi: 10.1158/1078-0432.CCR-08-1332
100. Fleming V, Hu X, Weber R, Nagibin V, Groth C, Altevogt P, et al. Targeting myeloid-derived suppressor cells to bypass tumor-induced immunosuppression. Front Immunol (2018) 9:398. doi: 10.3389/fimmu.2018.00398
101. Kalathil SG, Wang K, Hutson A, Iyer R, Thanavala Y. Tivozanib mediated inhibition of c-Kit/Scf signaling on tregs and mdscs and reversal of tumor induced immune suppression correlates with survival of hcc patients. Oncoimmunology (2020) 9(1):1824863. doi: 10.1080/2162402X.2020.1824863
Keywords: tumor microenvironment, immune cell, innate immune cells, anti-tumor treatment, immunotherapy
Citation: Lu C, Liu Y, Ali NM, Zhang B and Cui X (2023) The role of innate immune cells in the tumor microenvironment and research progress in anti-tumor therapy. Front. Immunol. 13:1039260. doi: 10.3389/fimmu.2022.1039260
Received: 08 September 2022; Accepted: 14 December 2022;
Published: 19 January 2023.
Edited by:
Gunes Esendagli, Hacettepe University, TurkeyReviewed by:
Yanqing Liu, Columbia University, United StatesKamalakannan Rajasekaran, Genentech Inc., United States
Copyright © 2023 Lu, Liu, Ali, Zhang and Cui. This is an open-access article distributed under the terms of the Creative Commons Attribution License (CC BY). The use, distribution or reproduction in other forums is permitted, provided the original author(s) and the copyright owner(s) are credited and that the original publication in this journal is cited, in accordance with accepted academic practice. No use, distribution or reproduction is permitted which does not comply with these terms.
*Correspondence: Xiaonan Cui, Y3huMjNAc2luYS5jb20=; Bin Zhang, emhhbmdiaW5fZGxtdUAxNjMuY29t
†These authors have contributed equally to this work