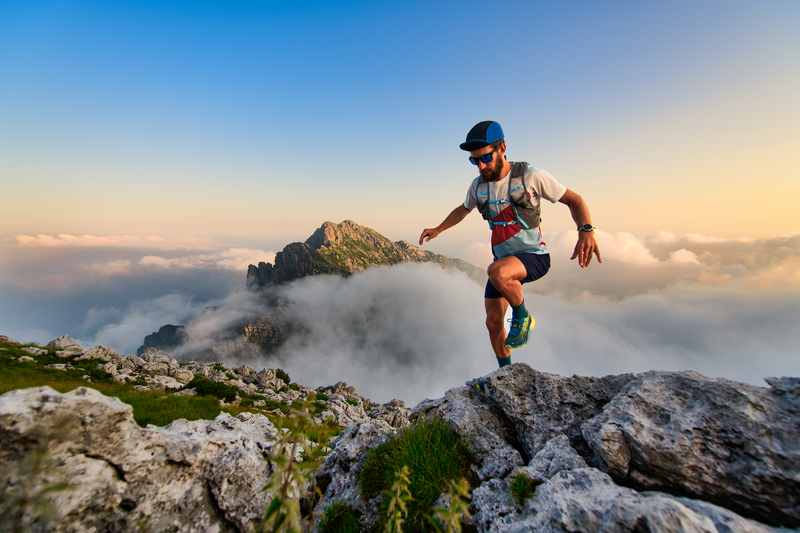
95% of researchers rate our articles as excellent or good
Learn more about the work of our research integrity team to safeguard the quality of each article we publish.
Find out more
MINI REVIEW article
Front. Immunol. , 20 October 2022
Sec. Cancer Immunity and Immunotherapy
Volume 13 - 2022 | https://doi.org/10.3389/fimmu.2022.1036298
This article is part of the Research Topic Community Series in Novel Insights into Immunotherapy Targeting Tumor Microenvironment in Ovarian Cancer, volume I View all 13 articles
Epithelial ovarian cancer (EOC) ranks as the second most common cause of gynecologic cancer death. The conventional treatment for patients with EOC is postoperative therapy along with platinum chemotherapy. However, a more efficient treatment regimen is of great need for these patients diagnosed with advanced disease (FIGO stages III–IV), whose survival is approximately 29%. Immunotherapy seems to be an encouraging therapeutic strategy for EOC. Given the crucial role in the complicated interactions between tumor cells and other cells, the tumor microenvironment (TME) influences the response to immunotherapy. In this review, we discuss feasible strategies for EOC immunotherapy by exploiting the reciprocity of cancer cells and the constituents of the TME.
Epithelial ovarian cancer (EOC) recognized by its high occurrence and poor prognosis (1), ranks as the second most common cause of gynecologic cancer death (2). The conventional treatment for EOC patients is postoperative therapy along with platinum chemotherapy (3). However, survival is dismal since over two-thirds of patients are diagnosed with advanced disease (FIGO stages III–IV) (4), and the survival rate for advanced stages is about 29% (5). Thus, a more effective treatment is of great need for these patients. Currently, immunotherapy is an encouraging treatment for various cancers (6). Immunotherapy agents are used to activate effector and cytotoxic T cells that respond to cancer cells through natural mechanisms, many of which are suppressed during cancer progression (7). Poor response to immunotherapy in ovarian tumors was associated with low expression of programmed cell death ligand 1 (PD-L1) (8). Therefore, it is urgent to explore the cells in the TME and their effects on the response of immune checkpoint inhibitors (ICIs).
The tumor microenvironment (TME), which is made up of vessels, immune infiltration and extracellular matrix (ECM), promotes cancer growth, invasion and metastasis (9). Understanding the interplay between cancer cells and various immune cells in the TME such as T lymphocytes, dendritic cells (DCs), tumor associated macrophages (TAMs) and natural killer (NK) cells, could explain the pathogenesis and explore novel therapies for EOC (10) (11). Immune editing, defined as the dual function of the immune system, can suppress and/or promote tumor growth (12). Studying the dual function of immune cells in the TME can suppress the key pathways that inhibit antitumor responses, and promising therapies will be discovered (13). PD-1 and CTLA-4 expressed on T cells are the basis of immune checkpoint immunotherapy (14). Additionally, immunosuppressive molecules in the TME such as indoleamine-2,3-dioxygenase (IDO), interleukin-10 (IL-10) and prostaglandin E2 (PGE2), can also be targets of immunotherapies (15).
In this review, the effect of the TME in immunotherapies and progress in EOC immunotherapy will be discussed.
T-lymphocytes in the TME contain tumor infiltrating lymphocytes (TILs) and regulatory T cells (Tregs). Tregs have been shown to weaken antitumor immunity indicating poor prognosis in patients with EOC (16). Studies have revealed that increases in tumor Treg cells represent a low survival rate of EOC (16), while other studies show their association with a pleasing clinical outcome biomarker in colorectal cancer (17). Immunosuppressive mechanisms regulated by Tregs leading to immunological tolerance and ignorance of cancer are as follows: 1) releasing soluble or membranous repressive cytokines such as interleukin-10 (IL-10), interleukin-35 (IL-35) and transforming growth factor-β (TGF-β), which can kill effector T cells (18). 2) high expression of granzymes and perforin mediates the cytolysis of NK cells and cytotoxic CD8+ T cells (19). 3) interfering with effector T cell metabolism by reducing IL-2, which is competitively consumed by T cells, and increasing adenosine which is an inhibitory molecule (20). 4) inducing DC tolerance by expressing CTLA-4 and ligands CD80 and/or CD86 on DCs that can generate immunosuppressive tryptophan metabolites, lymphocyte activation gene 3 (LAG3) molecules can suppress MHC II molecules on DCs (21). In view of the key immunosuppressive effect of Tregs, several agents have been explored that directly target markers such as CTLA-4 and IL-2 (20, 22).
Tumor-associated macrophages (TAMs) are recruited from monocytes in blood and resident peritoneal macrophages, and these are major infiltrating immune cells in the TME (23). Given the important heterogeneity and plasticity, TAMs contain two groups: anti-tumorigenic M1 type and pro-tumorigenic M2 type (24). In the TME, the most pro-tumorigenic M2-like phenotype (25) is critical for cancer angiogenesis, invasion and metastasis through different kinds of cytokines, chemokines, growth factors, and proteases (26, 27). Vascular endothelial growth factor A (VEGF-A), a pro-angiogenetic chemokine and protease secreted by TAMs promotes tumor angiogenesis (26, 28). By producing epidermal growth factor (EGF), TAMs promote cancer cell proliferation (29). Moreover, TAMs exhibit immunosuppressive effects through secreting IL-10, TGF-β, CCL2 and arginase (30, 31). Current studies targeting TAMs mainly include: 1) suppressing M2-like TAMs via inhibiting the recruitment of TAMs and exhausting TAMs 2) activating M1-like TAMs by strengthening the repolarization of M2 macrophages into M1 macrophages (32, 33). For inhibition of the recruitment of TAMs, the CCL2/CCR2 axis barricade which is has been found to be helpful in a mouse ovarian cancer model (34), and disrupting the CXCL12/CXCR4 axis which prolongs the survival of a tumor mouse model (35) seem to be an encouraging therapy. The decrease in TAMs caused by inhibiting the CSF-1/CSF-1R pathway has been proven to strengthen the tumor suppressive effect of docetaxel (36). In EOC, trabectedin can effectively deplete macrophages by inducing apoptosis of macrophages and thus play an antitumor role in ovarian cancer (37). Paclitaxel treats ovarian cancer by reprogramming the M2 to the M1 phenotype through TLR4 in gene expression analysis (38). Furthermore, another macrophage-directed therapy targets PD-L1 on TAMs which is involved in tumor immune escape mechanisms (39, 40).
Myeloid-derived suppressor cells (MDSCs) are a subpopulation of immunoregulatory immature myeloid cells that increase in multiple pathologic situations (41). MDSCs have been implicated in suppressing T cells and impacting other cells in the TME (42). The mechanisms of suppressing T cells include the following: 1) accelerating the depletion of T cell essential amino acids such as L-cysteine, L-arginine and L-tryptophan which are critical for T cell activity (43–45). 2) expressing PD-L1 interacting with PD-1 on T cells to suppress the antitumor effect of T cells and thus promote immune evasion (46). 3) producing ROS and NO which are toxic to T cells (47). Moreover, MDSCs boost the activation of Tregs via IL-10 and TGFβ in the need of CD40 (48, 49). MDSCs can be targeted by various strategies: 1) reduction of MDSCs, 2) inhibition of the recruitment of MDSCs, 3) suppression of MDSC function and 4) induction of MDSCs to differentiate into non‐suppressive cells (50). Thus, immunotherapies in combination with targeting MDSCs could be a major strategy. Phosphodiesterase‐5 (PDE‐5) inhibitors targeting arginase 1 (ARG1) and iNOS restabilize the immunosuppressive response of T cells (51). Synthetic triterpenoids activate the Nrf2 gene which modulates antioxidant enzymes and nitroaspirin, inhibiting iNOS and ROS production and thus relieving the oxidative stress caused by MDSCs (52, 53). Furthermore, STAT3 inhibitors combined with immune checkpoint blockade have been shown to be beneficial in lymphoma (54). In addition, blocking COX‐2 which is correlated with the expression of ARG1 can also be a promising approach to attenuate MDSC function (55). A schematic illustration of how suppressive immune cells affect the antitumor immune response in the TME is shown in Figure 1.
Figure 1 Immunosuppressive cells in the TME. Immune suppressive cells mainly contain Tregs, TAMs and MDSCs. Tregs restrain antitumor effector T cells including (i) secretion of repressive cytokines such as IL-10, IL-35 and TGF-β; (ii)induction of cytolysis via releasing granzymes and perforins; (iii) metabolic disturbance through adenosine production. Also, Tregs induce antigen presenting cell dysfunction for a tolerant phenotype. TAMs can (i) secrete EGF and MMPs to promote tumor progression; (ii) produce VEGF to aid in tumor angiogenesis; (iii) secrete IL-10 and TGF-β to induce Treg activation. MDSCs suppress T cells through (i) secretion of IL-10 and TGF-β which induce Tregs; (ii) production of NO and ROS which induce cytolysis and inhibit T cell activation; (iii) depletion of essential amino acids which play a crucial role in T cell activation and proliferation.
TILs contain CD8+ T and CD4+ T lymphocytes, especially CD8+ TILs which represent a good prognosis of EOC (56). CD8 + T cells recognize and kill pathogenic infections or cancer cells through perforin and granzyme (57). In addition to destroying cancer cells directly, CD8+ T cells suppress tumor vascularization via secreting IFN-γ which suppresses the development of cancer. Emerging evidence has revealed that CD8+ T cells in the TME are often beneficial to survival in ovarian cancer patients (58). Furthermore, CD4+ T cells are divided into different subtypes which include T helper 1 (Th1) cells, a group of cells that provide cytokines such as IL-2 and IFN-γ to support the antitumoral effect of CD8+ T cells (59). Moreover, high expression of CCL5 released by CD4+ T cells benefits the activation of DCs and thus induces an antitumor response (60). Hence, increases in Th1 cells within the TME are related to significant outcomes in a variety of cancers (61). As mentioned above, not all T cells function as antitumor effectors such as Tregs and Th17 cells. Thus, immunotherapies targeting the main impaired antitumor T effector cells are considered optimistic therapeutic approaches (62, 63). Recently, major advances have been made in developing PD-1 therapy which can potentiate the efficacy of CD8+ T cell based immunotherapy (64). T cells express PD-1 while other cells such as Tregs, TAMs, and cancer cells express PD-L1. Consequently, blockade targeting PD-1 or PD-L1 can suppress activation of T cell and breakdown immune tolerance and thus potentially mobilize immunity in tumors (65, 66).
NK cells are the most efficient antineoplastic effectors and do not need any prior sensitization or HLA-independent tumor target recognition (67, 68). NK cells encompass two main populations with CD56bright/CD16– functioning to produce IFN-γ and TNF-α cytokines, while CD56dim/CD16+ killing tumor cells directly via releasing perforin/granzyme or through TRAIL pathways (69). However, NK cells usually exhibit dysfunction in the TME, such as reduced proliferation, decreased secretion of cytotoxic molecules and abnormal expression of immune checkpoints (70). Studies have revealed that PD-L1 on tumor cells can inhibit PD-1 expression on NK cells, thereby promoting immune escape from cancer (71). A series of molecules such as IDO and PGE2 secreted by fibroblasts were shown to suppress the expression of the activating receptor NKG2D and thus mediate immune escape (72). Several immunotherapeutic strategies based on NK cells are currently being explored including adaptive NK cells, cytokines, antibodies and ICIs (68). Remarkably, adaptive NK cell therapy induced by various cytokines exhibits enhanced antitumor effects in ovarian cancer (73). Currently, therapy based on cytokines such as IL-2 and IL-15 is shown to vigorously increase NK cells (74, 75). Although antibody-based immunotherapy is not the gold standard treatment for ovarian cancer patients, antigens on tumor cells including NY-ESO-1 and MUC1 have attracted great attention (76). Furthermore, evidence has revealed that PD-1 and CD96/TIGIT inhibitors potentiate the tumor lysis mediated by NK cells (77).
DCs capture and process antigens to T cells and secrete inflammatory cytokines to induce pathogen-specific T-cell effects (78). Generally, DCs submit exogenous antigen peptides to CD4+ T cells through MHC II molecules and endogenous antigens to CD8+ T cells with MHC I molecules, and strengthen CD4+ and CD8+ T cell activity via presenting exogenous antigens (79, 80). However, the tumor microenvironment inhibits DC maturation through immunosuppressive factors including VEGF, IL-6, IL-10 and TGF-β, as well as repressive molecules including IDO (81, 82). Nevertheless, upregulating suppressive receptors such as PD-L1 and LAG3 induces T cell exhaustion thereby limiting the immune response (83). IDO produced by DCs restrains the function of NK cell and CD8+ T cell leading to an abated immune response (84). Therapeutic schemes targeting DCs have attracted great attention. Clinical studies revealed that IDO1 inhibitors combined with chemotherapy or ICIs elicit tumor regression (85). Collectively, potential DC based immune therapy seems to be an encouraging target against ovarian cancer. Furthermore, with such an effective ability for Ag presentation and T cell activation, DCs were tested as cancer vaccines that can produce “trained” DCs carrying tumor antigens and thus potentially induce strong antitumor T-cell effects (86, 87). In particular, it is well documented that DC vaccines combined with ICIs may result in synergistic effects (88, 89). A schematic illustration of how immune active cells exhibit dysfunction in the TME is shown in Figure 2.
Figure 2 Immunoactivated cells in the TME. Immune activated cells include CD8+ and CD4+ T lymphocytes, NK cells and DCs. Th1 cell which is a subtype of CD4+ T cells express CCL5 to induce DC activation and produce IL-2 and IFN-γ to assist CD8+ T cells. CD8+ T cells recognize antigens expressed on MHC class I on cancer cells leading to the cytotoxic killing of cancer cells via granzymes and perforins. CD8+ T cell dysfunction is mediated by elevated inhibitory ligand PD-L1 and B7 molecules on cancer cells. NK cell dysfunction mechanisms include (i) PGE2 and IDO derived from CAFs downregulate the expression of activating receptor NKG2D on DCs; (ii) inhibitory ligand PD-L1 and B7 molecules expressed by cancer cells restrain NK cell function by combining with PD-1 and CTLA-4. The mechanism of impaired DC function includes (i) overexpression of PD-1 ligands and LAG3 on DCs results in T cell exhaustion; (ii) IL-6, IL-10 and TGF-β produced by cancer cells alter DC maturation and migration capacity.
CAFs derived from mesenchymal stem cells are crucial components of stromal cell types (90, 91). CAFs produce proteins, paracrine cytokines and various ECMs that contribute to shaping the tumor microenvironment (92, 93). Classical growth factors secreted by CAFs include the following: 1) TGF-β regulates the interaction between cancer and stroma thereby facilitating tumor initiation and progression (94). 2) Epidermal growth factor (EGF) maintains the expression of ATC integrin α5 (ITGA5) which can promote the early peritoneal spread of HGSOC (95). 3) Hepatocyte growth factor (HGF) contributes to proliferation via the c-Met/PI3K/Akt and GRP78 pathways (96). 4) CXCL12, IL6, and VEGFA induced by CAFs result in the epithelial-to-mesenchymal transition (EMT) which can promote peritoneal metastasis of ovarian cancer (97). 5) CAFs activate MMPs to assist in the growth, invasion, and metastasis of tumors (98). 6) Lipoma-preferred partner (LPP) which has been proven to increase the focal adhesion and stress fiber formation of ECs contributes to ovarian cancer chemoresistance (99). Therapeutic strategies targeting CAFs fall into several aspects, one of which involves TGF-β inhibitors which were shown to improve the overall survival of EOC in a mouse model with peritoneal metastasis (100). Imatinib, an inhibitor targeting PDGF-D produced by fibroblasts was found to suppress ovarian cancer cell growth (101).
Cancer-associated adipocytes (CAAs) are documented to promote stromal reshaping and the invasion of cancer cells through interacting with ovarian cancer cells in the TME (102). It has been proven that metastases appear in the omentum which is made up of adipocytes in most patients with ovarian cancer (103). Adipocytes secrete various molecules such as metabolites, MMPs, enzymes and growth factors supporting tumor cell progression (104). Furthermore, coculture studies found that CAAs boost the oxidation of cancer cells, indicating that CAAs supply energy to maintain the ovarian cancer cells proliferation (105). Additionally, CAAs produce adipokines that can promote tumor development. Among them, leptin stimulates the migration and invasion abilities of ovarian cancer cells through the JAK/Stat3, PI3K/Akt and RhoA/ROCK pathways (106). FABP4, a lipid chaperone protein is a key regulator in ovarian cancer metastasis (107). Molecules including IL-6, IL8 and TNF-α secreted by CAAs have also been proven to aid in the growth and invasion of breast tumor cells (108). Collectively, the reciprocation between CAAs and cancer cells results in the metastasis of tumor cells. Overall, therapeutic strategies specifically targeting lipid metabolism and transport such as FABP4 inhibitors in ovarian cancer are full of hope (107).
Endothelial cells (ECs) impact the process of cancer growth and invasion (109). Angiogenesis is known to be a process regulated by the interplay of angiogenic activators and inhibitors. During tumor progression, oxygen deficiency and accumulation of metabolic products lead to hypoxia and acidity in the TME (110). Hypoxia in the TME induces the production of hypoxia-inducible factors (HIFs), which promote pro-angiogenic factors secreted by ECs, thereby promoting angiogenesis (111). In the course of angiogenesis, factors produced by cancer cells contain VEGF, platelet derived growth factor (PDGF), fibroblast growth factor 2 (FGF-2) and angiopoietins (112). VEGF, a chemokine secreted into malignant ascites contributes to the genesis of tumor blood via signaling with VEGF receptor-2 (113). To stabilize and increase the maturation of endothelial cell channels, pericytes express PDGFR-β which can interact with PDGF-B (114). Furthermore, FGF-2 promotes the production of MMPs, collagenase and plasminogen activator resulting in vascularization (115). In addition, FGF expression has been proven to be responsible for resistance to VEGF targeted therapies (116). Angiopoietin 1 and 2 (Ang1/2) has been found to promote the proliferation and survival of ECs via binding to the Tie-2 receptor (117). Thus, considerable attention is being paid to exploring therapeutic strategies to block the angiogenic signaling pathway. Bevacizumab as the most studied anti-VEGF monoclonal antibody has been demonstrated to positively increase PFS with cisplatin-based chemotherapy in several randomized phase III trials and has been recognized as the standard treatment in EOC (118, 119). Tyrosine kinase inhibitors (TKIs) such as pazopanib and cediranib are promising VEGFR targeting agents for ovarian cancer patients (120, 121). Trebananib, an inhibitor that targets non-VEGF signaling has meaningful effects on PFS when used in combination with paclitaxel in recurrent ovarian cancer through binding to Ang1/2 (122).
It is obviously that ICIs have revolutionized immunotherapy and brought concrete benefits to many patients. However, the response rate is unsatisfactory with different anti-PD-1 or PD-L1 agents since EOC is known to have a high immunosuppressive TME and low expression of PD-L1 (8). Novel combination therapies are currently evolving. Inhibitor of PD-1 in combination with CTLA-4 increased the frequency of tumor infiltration by effector T cells as well as uniquely decreased the frequency of Tregs in tumors (123). Evidence from clinical trials found that this combination blockade therapy is effective. The NRG-GY003 study showed that in recurrent epithelial ovarian cancer, nivolumab combined with ipilimumab led to an effective response rate of 31.4% while nivolumab alone is 12.2%. In addition, the mPFS was 2 months in the monotherapy group and 3.9 months in the combination treatment group (124). Combining nivolumab with ipilimumab produced higher response rates and longer PFS in EOC than nivolumab alone, but is still limited, so more combination clinical studies such as NCT02834013 and NCT03508570 are underway.
In a mouse model of intraperitoneal ovarian cancer, compared with single drug therapy of AMD3100 (CXCR4 antagonist) and αPD-1, the antitumor efficacy of combined therapy in inhibiting tumor growth and prolonging the survival time of mice was significantly improved (35). This provides strong preclinical evidence for ovarian cancer combination therapy, but to date, no clinical trials related to ovarian cancer have been carried out. It is worth noting that in a phase IIa trial, disease control with BL8040 plus pembrolizumab was 34.5% in 29 patients. 22 patients were treated with BL8040 plus pembrolizumab plus chemotherapy. The results exhibited an effective response rate of 32% and a disease control rate of 77% (125). These data all suggest that the combination of CXCR4 antagonists and PD-1 inhibitors can amplify the antitumor effect of chemotherapy.
In recent years, transmembrane protein triggering receptor expressed on myeloid cells 2 (TREM2) has gained great attention in anti-PD-1 resistant therapy since its enriched expression on TAMs in EOC (126). In a model of invasive ovarian cancer in situ, anti-TREM2mAb therapy can drive effective antitumor immunity (126). This finding indicates that TREM2 is a potential immunotherapy target when ICIs are ineffective and TAMs are rich in the tumor microenvironment. A phase I clinical trial (NCT04691375) to evaluate the single drug anti-TREM2 and anti-TREM combined with pembrolizumab in solid tumors which include ovarian cancer is underway.
Evidence has shown that PD-1 blockade in combination with a VEGF-A inhibitor can potentiate antitumor efficiency via increases in CD8+ and CD4+ T cells, and decreases in MDSCs and Tregs (127, 128). In a single-arm phase 2 clinical study, 38 women with recurrent epithelial ovarian cancer were screened for intravenous treatment with nivolumab and bevacizumab. The results showed that the ORR was 28.9%, among which 40.0% were platinum sensitive patients and 16.7% were platinum resistant patients (129). These data indicate that the combining nivolumab with bevacizumab is effective and feasible in ovarian cancer patients, especially those who are sensitive to platinum.
Poly-(ADP)-ribose polymerase inhibitors (PARPi), known as synthetic lethal agents in tumors with BRCA1/BRCA2 mutations are rapidly evolving (130). Combining PARPis with PD-1/PD-L1 blockades is a promising therapy that can synthetically enhance the antitumor effect (131). A phase II clinical study of MEDIOLA found that 32 patients received olaparib combined with durvalumab, and the overall disease control rate was 81% (132). The final result of the study showed that the OS of 31 patients treated with olaparib combined with durvalumab and bevacizumab was 31.9 months compared with that of 23.2 months in the two-drug group. In addition, the DCR of the two-drug group at 56 weeks was 9.4%, and that of the three-drug group was 38.7%, which indicated that the three-drug treatment mode was superior to the two-drug treatment mode for platinum-sensitive recurrent non-gBRCA ovarian cancer patients (133). The three-drug regimen has been applied to a third-phase clinical study of first-line maintenance therapy (NCT03737643).
Immune checkpoint immunotherapy has been the most prominent therapeutic strategy for successfully treating different kinds of cancers. However, the response rate in EOC is low since the immunosuppressive tumor microenvironment could limit the efficiency of ICIs. It is urgent to improve the effect of immunotherapy for EOC. Novel targets have been described and targeting approaches combined with ICs have already impacted the clinical outcomes of ovarian cancer. Targeting immune subtypes such as TAMs, Tregs, CAFs or angiogenesis could contribute to potentiating the antitumor effect of ICs. However, there are still many details to explore and discuss. In summary, the constituents within the TME should all be considered to explore novel combinations that contribute to achieving maximal benefits in EOC.
YW and LZ wrote the original manuscript. YB drew the figure. XM and LW corrected the original draft. All authors contributed to the article and approved the submitted version.
The authors declare that the research was conducted in the absence of any commercial or financial relationships that could be construed as a potential conflict of interest.
All claims expressed in this article are solely those of the authors and do not necessarily represent those of their affiliated organizations, or those of the publisher, the editors and the reviewers. Any product that may be evaluated in this article, or claim that may be made by its manufacturer, is not guaranteed or endorsed by the publisher.
EOC, epithelial ovarian cancer; TME, tumor microenvironment; PD-1, programmed cell death 1; PD-L1, programmed cell death ligand 1; ECM, extracellular matrix; DCs, dendritic cells; TAMs, tumor associated macrophages; NK, natural killer; ICIs, immune checkpoint inhibitors; Tregs, regulatory T cells; IL-10, interleukin-10; IL-35, interleukin-35; TGF-β, transforming growth factor-β; IDO, indoleamine-2,3-dioxygenase; PGE2, prostaglandin E2; LAG3, lymphocyte activation gene 3; VEGF-A, vascular endothelial growth factor A; EGF, epidermal growth factor; MDSCs, myeloid-derived suppressor cells; ARG1, arginase 1; CAFs, cancer associated fibroblasts; CAAs, cancer-associated adipocytes; HGF, hepatocyte growth factor; PDGF, platelet derived growth factor; FGF-2, fibroblast growth factor 2.
1. Momenimovahed Z, Tiznobaik A, Taheri S, Salehiniya H. Ovarian cancer in the world: epidemiology and risk factors. Int J Women's Health (2019) 11:287:99. doi: 10.2147/IJWH.S197604
2. Lheureux S, Braunstein M, Oza AM. Epithelial ovarian cancer: Evolution of management in the era of precision medicine. CA: Cancer J For Clin (2019) 69(4):3125. doi: 10.3322/caac.21559
3. Kuroki L, Guntupalli SR. Treatment of epithelial ovarian cancer. BMJ (Clinical Res ed) (2020) 371:m3773. doi: 10.1136/bmj.m3773
4. Baci D, Bosi A, Gallazzi M, Rizzi M, Noonan DM, Poggi A, et al. The ovarian cancer tumor immune microenvironment (TIME) as target for therapy: A focus on innate immunity cells as therapeutic effectors. Int J Mol Sci (2020) 21(9):3125. doi: 10.3390/ijms21093125
5. Kouba S, Ouldamer L, Garcia C, Fontaine D, Chantome A, Vandier C, et al. Lipid metabolism and calcium signaling in epithelial ovarian cancer. Cell Calcium (2019) 81:38–50. doi: 10.1016/j.ceca.2019.06.002
6. Pardoll DM. The blockade of immune checkpoints in cancer immunotherapy. Nat Rev Cancer (2012) 12(4):252–64. doi: 10.1038/nrc3239
7. Riley RS, June CH, Langer R, Mitchell MJ. Delivery technologies for cancer immunotherapy. Nat Rev Drug Discovery (2019) 18(3):175–96. doi: 10.1038/s41573-018-0006-z
8. Chardin L, Leary A. Immunotherapy in ovarian cancer: Thinking beyond PD-1/PD-L1. Front Oncol (2021) 11:795547. doi: 10.3389/fonc.2021.795547
9. Meurette O, Mehlen P. Notch signaling in the tumor microenvironment. Cancer Cell (2018) 34(4):536–48. doi: 10.1016/j.ccell.2018.07.009
10. Pogge von Strandmann E, Reinartz S, Wager U, Müller R. Tumor-host cell interactions in ovarian cancer: Pathways to therapy failure. Trends In Cancer (2017) 3(2):137–48. doi: 10.1016/j.trecan.2016.12.005
11. O'Donnell JS, Teng MWL, Smyth MJ. Cancer immunoediting and resistance to T cell-based immunotherapy. Nat Rev Clin Oncol (2019) 16(3):151–67. doi: 10.1038/s41571-018-0142-8
12. Schreiber RD, Old LJ, Smyth MJ. Cancer immunoediting: integrating immunity's roles in cancer suppression and promotion. Sci (New York NY) (2011) 331(6024):1565–70. doi: 10.1126/science.1203486
13. Yang Y. Cancer immunotherapy: harnessing the immune system to battle cancer. J Clin Invest (2015) 125(9):3335–7. doi: 10.1172/JCI83871
14. Granier C, De Guillebon E, Blanc C, Roussel H, Badoual C, Colin E, et al. Mechanisms of action and rationale for the use of checkpoint inhibitors in cancer. ESMO Open (2017) 2(2):e000213. doi: 10.1136/esmoopen-2017-000213
15. Kandalaft LE, Odunsi K, Coukos G. Immunotherapy in ovarian cancer: Are we there yet? J Clin Oncol (2019) 37(27):2460–71. doi: 10.1200/JCO.19.00508
16. Curiel TJ, Coukos G, Zou L, Alvarez X, Cheng P, Mottram P, et al. Specific recruitment of regulatory T cells in ovarian carcinoma fosters immune privilege and predicts reduced survival. Nat Med (2004) 10(9):942–9. doi: 10.1038/nm1093
17. Sakaguchi S, Mikami N, Wing JB, Tanaka A, Ichiyama K, Ohkura N. Regulatory T cells and human disease. Annu Rev Immunol (2020) 38:541–66. doi: 10.1146/annurev-immunol-042718-041717
18. Vignali DA, Collison LW, Workman CJ. How regulatory T cells work. Nat Rev Immunol (2008) 8(7):523–32. doi: 10.1038/nri2343
19. Cao X, Cai SF, Fehniger TA, Song J, Collins LI, Piwnica-Worms DR, et al. Granzyme b and perforin are important for regulatory T cell-mediated suppression of tumor clearance. Immunity (2007) 27(4):635–46. doi: 10.1016/j.immuni.2007.08.014
20. Facciabene A, Motz GT, Coukos G. T-Regulatory cells: key players in tumor immune escape and angiogenesis. Cancer Res (2012) 72(9):2162–71. doi: 10.1158/0008-5472.CAN-11-3687
21. Li C, Jiang P, Wei S, Xu X, Wang J. Regulatory T cells in tumor microenvironment: new mechanisms, potential therapeutic strategies and future prospects. Mol Cancer (2020) 19(1):116. doi: 10.1158/1557-3125.HIPPO19-B11
22. Drerup JM, Deng Y, Pandeswara SL, Padrón ÁS, Reyes RM, Zhang X, et al. CD122-selective IL2 complexes reduce immunosuppression, promote treg fragility, and sensitize tumor response to PD-L1 blockade. Cancer Res (2020) 80(22):5063–75. doi: 10.1158/0008-5472.CAN-20-0002
23. Colvin EK. Tumor-associated macrophages contribute to tumor progression in ovarian cancer. Front Oncol (2014) 4:137. doi: 10.3389/fonc.2014.00137
24. Gupta V, Yull F, Khabele D. Bipolar tumor-associated macrophages in ovarian cancer as targets for therapy. Cancers (Basel) (2018) 10(10):366. doi: 10.3390/cancers10100366
25. Rogers TL, Holen I. Tumour macrophages as potential targets of bisphosphonates. J Transl Med (2011) 9:177. doi: 10.1186/1479-5876-9-177
26. Pollard JW. Tumour-educated macrophages promote tumour progression and metastasis. Nat Rev Cancer (2004) 4(1):71–8. doi: 10.1038/nrc1256
27. Okła K, Wertel I, Polak G, Surówka J, Wawruszak A, Kotarski J. Tumor-associated macrophages and myeloid-derived suppressor cells as immunosuppressive mechanism in ovarian cancer patients: Progress and challenges. Int Rev Immunol (2016) 35(5):372–85. doi: 10.1080/08830185.2016.1206097
28. Fiani ML, Barreca V, Sargiacomo M, Ferrantelli F, Manfredi F, Federico M. Exploiting manipulated small extracellular vesicles to subvert immunosuppression at the tumor microenvironment through mannose Receptor/CD206 targeting. Int J Mol Sci (2020) 21(17):6318. doi: 10.3390/ijms21176318
29. Carroll MJ, Kapur A, Felder M, Patankar MS, Kreeger PK. M2 macrophages induce ovarian cancer cell proliferation via a heparin binding epidermal growth factor/matrix metalloproteinase 9 intercellular feedback loop. Oncotarget (2016) 7(52):86608–20. doi: 10.18632/oncotarget.13474
30. Hagemann T, Wilson J, Kulbe H, Li NF, Leinster DA, Charles K, et al. Macrophages induce invasiveness of epithelial cancer cells via NF-kappa b and JNK. J Immunol (2005) 175(2):1197–205. doi: 10.4049/jimmunol.175.2.1197
31. Beltraminelli T, De Palma M. Biology and therapeutic targeting of tumour-associated macrophages. J Pathol (2020) 250(5):573–92. doi: 10.1002/path.5403
32. Mantovani A, Marchesi F, Malesci A, Laghi L, Allavena P. Tumour-associated macrophages as treatment targets in oncology. Nat Rev Clin Oncol (2017) 14(7):399–416. doi: 10.1038/nrclinonc.2016.217
33. Zhang S-Y, Song X-Y, Li Y, Ye L-L, Zhou Q, Yang W-B. Tumor-associated macrophages: A promising target for a cancer immunotherapeutic strategy. Pharmacol Res (2020) 161:105111. doi: 10.1016/j.phrs.2020.105111
34. Moisan F, Francisco EB, Brozovic A, Duran GE, Wang YC, Chaturvedi S, et al. Enhancement of paclitaxel and carboplatin therapies by CCL2 blockade in ovarian cancers. Mol Oncol (2014) 8(7):1231–9. doi: 10.1016/j.molonc.2014.03.016
35. Zeng Y, Li B, Liang Y, Reeves PM, Qu X, Ran C, et al. Dual blockade of CXCL12-CXCR4 and PD-1-PD-L1 pathways prolongs survival of ovarian tumor-bearing mice by prevention of immunosuppression in the tumor microenvironment. FASEB J (2019) 33(5):6596–608. doi: 10.1096/fj.201802067RR
36. Lu X, Meng T. Depletion of tumor-associated macrophages enhances the anti-tumor effect of docetaxel in a murine epithelial ovarian cancer. Immunobiology (2019) 224(3):355–61. doi: 10.1016/j.imbio.2019.03.002
37. D'Incalci M, Galmarini CM. A review of trabectedin (ET-743): a unique mechanism of action. Mol Cancer Ther (2010) 9(8):2157–63. doi: 10.1158/1535-7163.MCT-10-0263
38. Wanderley CW, Colón DF, Luiz JPM, Oliveira FF, Viacava PR, Leite CA, et al. Paclitaxel reduces tumor growth by reprogramming tumor-associated macrophages to an M1 profile in a TLR4-dependent manner. Cancer Res (2018) 78(20):5891–900. doi: 10.1158/0008-5472.CAN-17-3480
39. Jiang X, Wang J, Deng X, Xiong F, Ge J, Xiang B, et al. Role of the tumor microenvironment in PD-L1/PD-1-mediated tumor immune escape. Mol Cancer (2019) 18(1):10. doi: 10.1186/s12943-018-0928-4
40. Kryczek I, Zou L, Rodriguez P, Zhu G, Wei S, Mottram P, et al. B7-H4 expression identifies a novel suppressive macrophage population in human ovarian carcinoma. J Exp Med (2006) 203(4):871–81. doi: 10.1084/jem.20050930
41. Youn J-I, Gabrilovich DI. The biology of myeloid-derived suppressor cells: the blessing and the curse of morphological and functional heterogeneity. Eur J Immunol (2010) 40(11):2969–75. doi: 10.1002/eji.201040895
42. Parker KH, Beury DW, Ostrand-Rosenberg S. Myeloid-derived suppressor cells: Critical cells driving immune suppression in the tumor microenvironment. Adv In Cancer Res (2015) 128:95–139. doi: 10.1016/bs.acr.2015.04.002
43. Srivastava MK, Sinha P, Clements VK, Rodriguez P, Ostrand-Rosenberg S. Myeloid-derived suppressor cells inhibit T-cell activation by depleting cystine and cysteine. Cancer Res (2010) 70(1):68–77. doi: 10.1158/0008-5472.CAN-09-2587
44. Bronte V, Zanovello P. Regulation of immune responses by l-arginine metabolism. Nat Rev Immunol (2005) 5(8):641–54. doi: 10.1038/nri1668
45. Mezrich JD, Fechner JH, Zhang X, Johnson BP, Burlingham WJ, Bradfield CA. An interaction between kynurenine and the aryl hydrocarbon receptor can generate regulatory T cells. J Immunol (2010) 185(6):3190–8. doi: 10.4049/jimmunol.0903670
46. Juneja VR, McGuire KA, Manguso RT, LaFleur MW, Collins N, Haining WN, et al. PD-L1 on tumor cells is sufficient for immune evasion in immunogenic tumors and inhibits CD8 T cell cytotoxicity. J Exp Med (2017) 214(4):895–904. doi: 10.1084/jem.20160801
47. Groth C, Hu X, Weber R, Fleming V, Altevogt P, Utikal J, et al. Immunosuppression mediated by myeloid-derived suppressor cells (MDSCs) during tumour progression. Br J Cancer (2019) 120(1):16–25. doi: 10.1038/s41416-018-0333-1
48. Pan P-Y, Ma G, Weber KJ, Ozao-Choy J, Wang G, Yin B, et al. Immune stimulatory receptor CD40 is required for T-cell suppression and T regulatory cell activation mediated by myeloid-derived suppressor cells in cancer. Cancer Res (2010) 70(1):99–108. doi: 10.1158/0008-5472.CAN-09-1882
49. Hoechst B, Ormandy LA, Ballmaier M, Lehner F, Krüger C, Manns MP, et al. A new population of myeloid-derived suppressor cells in hepatocellular carcinoma patients induces CD4(+)CD25(+)Foxp3(+) T cells. Gastroenterology (2008) 135(1):234–43. doi: 10.1053/j.gastro.2008.03.020
50. Mabuchi S, Sasano T, Komura N. Targeting myeloid-derived suppressor cells in ovarian cancer. Cells (2021) 10(2):329. doi: 10.3390/cells10020329
51. Serafini P, Meckel K, Kelso M, Noonan K, Califano J, Koch W, et al. Phosphodiesterase-5 inhibition augments endogenous antitumor immunity by reducing myeloid-derived suppressor cell function. J Exp Med (2006) 203(12):2691–702. doi: 10.1084/jem.20061104
52. Ohl K, Tenbrock K. Reactive oxygen species as regulators of MDSC-mediated immune suppression. Front In Immunol (2018) 9:2499. doi: 10.3389/fimmu.2018.02499
53. De Santo C, Serafini P, Marigo I, Dolcetti L, Bolla M, Del Soldato P, et al. Nitroaspirin corrects immune dysfunction in tumor-bearing hosts and promotes tumor eradication by cancer vaccination. Proc Natl Acad Sci U.S.A. (2005) 102(11):4185–90. doi: 10.1073/pnas.0409783102
54. Poschke I, Mougiakakos D, Hansson J, Masucci GV, Kiessling R. Immature immunosuppressive CD14+HLA-DR-/low cells in melanoma patients are Stat3hi and overexpress CD80, CD83, and DC-sign. Cancer Res (2010) 70(11):4335–45. doi: 10.1158/0008-5472.CAN-09-3767
55. Veltman JD, Lambers MEH, van Nimwegen M, Hendriks RW, Hoogsteden HC, Aerts JGJV, et al. COX-2 inhibition improves immunotherapy and is associated with decreased numbers of myeloid-derived suppressor cells in mesothelioma. celecoxib influences MDSC function. BMC Cancer (2010) 10:464. doi: 10.1186/1471-2407-10-464
56. Santoiemma PP, Powell DJ. Tumor infiltrating lymphocytes in ovarian cancer. Cancer Biol Ther (2015) 16(6):807–20. doi: 10.1080/15384047.2015.1040960
57. Farhood B, Najafi M, Mortezaee K. CD8 cytotoxic T lymphocytes in cancer immunotherapy: A review. J Cell Physiol (2019) 234(6):8509–21. doi: 10.1002/jcp.27782
58. Sato E, Olson SH, Ahn J, Bundy B, Nishikawa H, Qian F, et al. Intraepithelial CD8+ tumor-infiltrating lymphocytes and a high CD8+/regulatory T cell ratio are associated with favorable prognosis in ovarian cancer. Proc Natl Acad Sci U.S.A. (2005) 102(51):18538–43. doi: 10.1073/pnas.0509182102
59. Quail DF, Joyce JA. Microenvironmental regulation of tumor progression and metastasis. Nat Med (2013) 19(11):1423–37. doi: 10.1038/nm.3394
60. Nesbeth YC, Martinez DG, Toraya S, Scarlett UK, Cubillos-Ruiz JR, Rutkowski MR, et al. CD4+ T cells elicit host immune responses to MHC class II-negative ovarian cancer through CCL5 secretion and CD40-mediated licensing of dendritic cells. J Immunol (2010) 184(10):5654–62. doi: 10.4049/jimmunol.0903247
61. Tsiatas ML, Gyftaki R, Liacos C, Politi E, Rodolakis A, Dimopoulos M-A, et al. Study of T lymphocytes infiltrating peritoneal metastases in advanced ovarian cancer: associations with vascular endothelial growth factor levels and prognosis in patients receiving platinum-based chemotherapy. Int J Gynecol Cancer (2009) 19(8):1329–34. doi: 10.1111/IGC.0b013e3181b7a40e
62. Hwang W-T, Adams SF, Tahirovic E, Hagemann IS, Coukos G. Prognostic significance of tumor-infiltrating T cells in ovarian cancer: A meta-analysis. Gynecol Oncol (2012) 124(2):192–8. doi: 10.1016/j.ygyno.2011.09.039
63. Rodriguez GM, Galpin KJC, McCloskey CW, Vanderhyden BC. The tumor microenvironment of epithelial ovarian cancer and its influence on response to immunotherapy. Cancers (Basel) (2018) 10(8):242. doi: 10.3390/cancers10080242
64. Topalian SL, Drake CG, Pardoll DM. Immune checkpoint blockade: a common denominator approach to cancer therapy. Cancer Cell (2015) 27(4):450–61. doi: 10.1016/j.ccell.2015.03.001
65. Coukos G, Tanyi J, Kandalaft LE. Opportunities in immunotherapy of ovarian cancer. Ann Oncol (2016) 27 Suppl 1:i11–i5. doi: 10.1093/annonc/mdw084
66. Iwai Y, Ishida M, Tanaka Y, Okazaki T, Honjo T, Minato N. Involvement of PD-L1 on tumor cells in the escape from host immune system and tumor immunotherapy by PD-L1 blockade. Proc Natl Acad Sci U.S.A. (2002) 99(19):12293–7. doi: 10.1073/pnas.192461099
67. Drakes ML, Stiff PJ. Regulation of ovarian cancer prognosis by immune cells in the tumor microenvironment. Cancers (Basel) (2018) 10(9):302. doi: 10.3390/cancers10090302
68. Uppendahl LD, Dahl CM, Miller JS, Felices M, Geller MA. Natural killer cell-based immunotherapy in gynecologic malignancy: A review. Front In Immunol (2017) 8:1825. doi: 10.3389/fimmu.2017.01825
69. Nersesian S, Glazebrook H, Toulany J, Grantham SR, Boudreau JE. Naturally killing the silent killer: NK cell-based immunotherapy for ovarian cancer. Front In Immunol (2019) 10:1782. doi: 10.3389/fimmu.2019.01782
70. Worzfeld T, Pogge von Strandmann E, Huber M, Adhikary T, Wagner U, Reinartz S, et al. The unique molecular and cellular microenvironment of ovarian cancer. Front Oncol (2017) 7:24. doi: 10.3389/fonc.2017.00024
71. Pesce S, Greppi M, Tabellini G, Rampinelli F, Parolini S, Olive D, et al. Identification of a subset of human natural killer cells expressing high levels of programmed death 1: A phenotypic and functional characterization. J Allergy Clin Immunol (2017) 139(1):335–46. doi: 10.1016/j.jaci.2016.04.025
72. Li T, Yang Y, Hua X, Wang G, Liu W, Jia C, et al. Hepatocellular carcinoma-associated fibroblasts trigger NK cell dysfunction via PGE2 and IDO. Cancer Lett (2012) 318(2):154–61. doi: 10.1016/j.canlet.2011.12.020
73. Greppi M, Tabellini G, Patrizi O, Candiani S, Decensi A, Parolini S, et al. Strengthening the AntiTumor NK cell function for the treatment of ovarian cancer. Int J Mol Sci (2019) 20(4):890. doi: 10.3390/ijms20040890
74. Pillet A-H, Bugault F, Thèze J, Chakrabarti LA, Rose T. A programmed switch from IL-15- to IL-2-dependent activation in human NK cells. J Immunol (2009) 182(10):6267–77. doi: 10.4049/jimmunol.0801933
75. Silva RD, Yoshida A, Cardozo D, Jales R, Paust S, Derchain S, et al. Natural killer cells response to IL-2 stimulation is distinct between ascites with the presence or absence of malignant cells in ovarian cancer patients. Int J Mol Sci (2017) 18(5):856. doi: 10.3390/ijms18050856
76. Mantia-Smaldone GM, Corr B, Chu CS. Immunotherapy in ovarian cancer. Hum Vaccines Immunother (2012) 8(9):1179–91. doi: 10.4161/hv.20738
77. Chan CJ, Martinet L, Gilfillan S, Souza-Fonseca-Guimaraes F, Chow MT, Town L, et al. The receptors CD96 and CD226 oppose each other in the regulation of natural killer cell functions. Nat Immunol (2014) 15(5):431–8. doi: 10.1038/ni.2850
78. Constantino J, Gomes C, Falcão A, Neves BM, Cruz MT. Dendritic cell-based immunotherapy: a basic review and recent advances. Immunol Res (2017) 65(4):798–810. doi: 10.1007/s12026-017-8931-1
79. Dudek AM, Martin S, Garg AD, Agostinis P. Immature, semi-mature, and fully mature dendritic cells: Toward a DC-cancer cells interface that augments anticancer immunity. Front In Immunol (2013) 4:438. doi: 10.3389/fimmu.2013.00438
80. Sabado RL, Balan S, Bhardwaj N. Dendritic cell-based immunotherapy. Cell Res (2017) 27(1):74–95. doi: 10.1038/cr.2016.157
81. Lin H, Wei S, Hurt EM, Green MD, Zhao L, Vatan L, et al. Host expression of PD-L1 determines efficacy of PD-L1 pathway blockade-mediated tumor regression. J Clin Invest (2018) 128(2):805–15. doi: 10.1172/JCI96113
82. Motta JM, Rumjanek VM. Sensitivity of dendritic cells to microenvironment signals. J Immunol Res (2016) 2016:4753607. doi: 10.1155/2016/4753607
83. Bastien JP, Minguy A, Dave V, Roy DC. Cellular therapy approaches harnessing the power of the immune system for personalized cancer treatment. Semin Immunol (2019) 42:101306. doi: 10.1016/j.smim.2019.101306
84. Munn DH, Mellor AL. IDO in the tumor microenvironment: Inflammation, counter-regulation, and tolerance. Trends In Immunol (2016) 37(3):193–207. doi: 10.1016/j.it.2016.01.002
85. Beatty GL, O'Dwyer PJ, Clark J, Shi JG, Bowman KJ, Scherle PA, et al. First-in-Human phase I study of the oral inhibitor of indoleamine 2,3-Dioxygenase-1 epacadostat (INCB024360) in patients with advanced solid malignancies. Clin Cancer Res (2017) 23(13):3269–76. doi: 10.1158/1078-0432.CCR-16-2272
86. Butterfield LH. Dendritic cells in cancer immunotherapy clinical trials: are we making progress? Front In Immunol (2013) 4:454. doi: 10.3389/fimmu.2013.00454
87. Bol KF, Schreibelt G, Gerritsen WR, de Vries IJM, Figdor CG. Dendritic cell-based immunotherapy: State of the art and beyond. Clin Cancer Res (2016) 22(8):1897–906. doi: 10.1158/1078-0432.CCR-15-1399
88. Flies DB, Higuchi T, Harris JC, Jha V, Gimotty PA, Adams SF. Immune checkpoint blockade reveals the stimulatory capacity of tumor-associated CD103(+) dendritic cells in late-stage ovarian cancer. Oncoimmunology (2016) 5(8):e1185583. doi: 10.1080/2162402X.2016.1185583
89. van Gulijk M, Dammeijer F, Aerts JGJV, Vroman H. Combination strategies to optimize efficacy of dendritic cell-based immunotherapy. Front In Immunol (2018) 9:2759. doi: 10.3389/fimmu.2018.02759
90. Orimo A, Weinberg RA. Stromal fibroblasts in cancer: a novel tumor-promoting cell type. Cell Cycle (Georgetown Tex) (2006) 5(15):1597–601. doi: 10.4161/cc.5.15.3112
91. Desbois M, Wang Y. Cancer-associated fibroblasts: Key players in shaping the tumor immune microenvironment. Immunol Rev (2021) 302(1):241–58. doi: 10.1111/imr.12982
92. Erez N, Glanz S, Raz Y, Avivi C, Barshack I. Cancer associated fibroblasts express pro-inflammatory factors in human breast and ovarian tumors. Biochem Biophys Res Commun (2013) 437(3):397–402. doi: 10.1016/j.bbrc.2013.06.089
93. Yeung T-L, Leung CS, Li F, Wong SST, Mok SC. Targeting stromal-cancer cell crosstalk networks in ovarian cancer treatment. Biomolecules (2016) 6(1):3. doi: 10.3390/biom6010003
94. Bhowmick NA, Chytil A, Plieth D, Gorska AE, Dumont N, Shappell S, et al. TGF-beta signaling in fibroblasts modulates the oncogenic potential of adjacent epithelia. Sci (New York NY) (2004) 303(5659):848–51. doi: 10.1126/science.1090922
95. Jiang Y, Wang C, Zhou S. Targeting tumor microenvironment in ovarian cancer: Premise and promise. Biochim Et Biophys Acta Rev On Cancer (2020) 1873(2):188361. doi: 10.1016/j.bbcan.2020.188361
96. Deying W, Feng G, Shumei L, Hui Z, Ming L, Hongqing W. CAF-derived HGF promotes cell proliferation and drug resistance by up-regulating the c-Met/PI3K/Akt and GRP78 signalling in ovarian cancer cells. Biosci Rep (2017) 37(2):BSR20160470. doi: 10.1042/BSR20160470
97. Dasari S, Fang Y, Mitra AK. Cancer associated fibroblasts: Naughty neighbors that drive ovarian cancer progression. Cancers (2018) 10(11):406. doi: 10.3390/cancers10110406
98. Najafi M, Farhood B, Mortezaee K. Extracellular matrix (ECM) stiffness and degradation as cancer drivers. J Cell Biochem (2019) 120(3):2782–90. doi: 10.1002/jcb.27681
99. Leung CS, Yeung T-L, Yip K-P, Wong K-K, Ho SY, Mangala LS, et al. Cancer-associated fibroblasts regulate endothelial adhesion protein LPP to promote ovarian cancer chemoresistance. J Clin Invest (2018) 128(2):589–606. doi: 10.1172/JCI95200
100. Yamamura S, Matsumura N, Mandai M, Huang Z, Oura T, Baba T, et al. The activated transforming growth factor-beta signaling pathway in peritoneal metastases is a potential therapeutic target in ovarian cancer. Int J Cancer (2012) 130(1):20–8. doi: 10.1002/ijc.25961
101. Matei D, Chang DD, Jeng M-H. Imatinib mesylate (Gleevec) inhibits ovarian cancer cell growth through a mechanism dependent on platelet-derived growth factor receptor alpha and akt inactivation. Clin Cancer Res (2004) 10(2):681–90. doi: 10.1158/1078-0432.CCR-0754-03
102. Xiong Y, McDonald LT, Russell DL, Kelly RR, Wilson KR, Mehrotra M, et al. Hematopoietic stem cell-derived adipocytes and fibroblasts in the tumor microenvironment. World J Stem Cells (2015) 7(2):253–65. doi: 10.4252/wjsc.v7.i2.253
103. Nieman KM, Kenny HA, Penicka CV, Ladanyi A, Buell-Gutbrod R, Zillhardt MR, et al. Adipocytes promote ovarian cancer metastasis and provide energy for rapid tumor growth. Nat Med (2011) 17(11):1498–503. doi: 10.1038/nm.2492
104. Wu Q, Li B, Li J, Sun S, Yuan J, Sun S. Cancer-associated adipocytes as immunomodulators in cancer. biomark Res (2021) 9(1):2. doi: 10.1186/s40364-020-00257-6
105. Clark R, Krishnan V, Schoof M, Rodriguez I, Theriault B, Chekmareva M, et al. Milky spots promote ovarian cancer metastatic colonization of peritoneal adipose in experimental models. Am J Pathol (2013) 183(2):576–91. doi: 10.1016/j.ajpath.2013.04.023
106. Kato S, Abarzua-Catalan L, Trigo C, Delpiano A, Sanhueza C, García K, et al. Leptin stimulates migration and invasion and maintains cancer stem-like properties in ovarian cancer cells: An explanation for poor outcomes in obese women. Oncotarget (2015) 6(25):21100–19. doi: 10.18632/oncotarget.4228
107. Mukherjee A, Chiang C-Y, Daifotis HA, Nieman KM, Fahrmann JF, Lastra RR, et al. Adipocyte-induced FABP4 expression in ovarian cancer cells promotes metastasis and mediates carboplatin resistance. Cancer Res (2020) 80(8):1748–61. doi: 10.1158/0008-5472.CAN-19-1999
108. Guerrero J, Tobar N, Cáceres M, Espinoza L, Escobar P, Dotor J, et al. Soluble factors derived from tumor mammary cell lines induce a stromal mammary adipose reversion in human and mice adipose cells. possible role of TGF-beta1 and TNF-alpha. Breast Cancer Res Treat (2010) 119(2):497–508. doi: 10.1007/s10549-009-0491-1
109. Bussard KM, Mutkus L, Stumpf K, Gomez-Manzano C, Marini FC. Tumor-associated stromal cells as key contributors to the tumor microenvironment. Breast Cancer Res (2016) 18(1):84. doi: 10.1186/s13058-016-0740-2
110. Anderson NM, Simon MC. The tumor microenvironment. Curr Biol (2020) 30(16):R921–R5. doi: 10.1016/j.cub.2020.06.081
111. Shih H-J, Chang H-F, Chen C-L, Torng P-L. Differential expression of hypoxia-inducible factors related to the invasiveness of epithelial ovarian cancer. Sci Rep (2021) 11(1):22925. doi: 10.1038/s41598-021-02400-1
112. Lugano R, Ramachandran M, Dimberg A. Tumor angiogenesis: causes, consequences, challenges and opportunities. Cell Mol Life Sci CMLS (2020) 77(9):1745–70. doi: 10.1007/s00018-019-03351-7
113. Mesiano S, Ferrara N, Jaffe RB. Role of vascular endothelial growth factor in ovarian cancer: inhibition of ascites formation by immunoneutralization. Am J Pathol (1998) 153(4):1249–56. doi: 10.1016/S0002-9440(10)65669-6
114. Gaengel K, Genové G, Armulik A, Betsholtz C. Endothelial-mural cell signaling in vascular development and angiogenesis. Arterioscler Thromb Vasc Biol (2009) 29(5):630–8. doi: 10.1161/ATVBAHA.107.161521
115. Turner N, Grose R. Fibroblast growth factor signalling: from development to cancer. Nat Rev Cancer (2010) 10(2):116–29. doi: 10.1038/nrc2780
116. Incio J, Ligibel JA, McManus DT, Suboj P, Jung K, Kawaguchi K, et al. Obesity promotes resistance to anti-VEGF therapy in breast cancer by up-regulating IL-6 and potentially FGF-2. Sci Transl Med (2018) 10(432):eaag0945. doi: 10.1126/scitranslmed.aag0945
117. Lim D, Do Y, Kwon BS, Chang W, Lee M-S, Kim J, et al. Angiogenesis and vasculogenic mimicry as therapeutic targets in ovarian cancer. BMB Rep (2020) 53(6):291–8. doi: 10.5483/BMBRep.2020.53.6.060
118. Monk BJ, Minion LE, Coleman RL. Anti-angiogenic agents in ovarian cancer: past, present, and future. Ann Oncol (2016) 27(Suppl 1):i33–i9. doi: 10.1093/annonc/mdw093
119. Rossi L, Verrico M, Zaccarelli E, Papa A, Colonna M, Strudel M, et al. Bevacizumab in ovarian cancer: A critical review of phase III studies. Oncotarget (2017) 8(7):12389–405. doi: 10.18632/oncotarget.13310
120. Ledermann JA, Embleton AC, Raja F, Perren TJ, Jayson GC, Rustin GJS, et al. Cediranib in patients with relapsed platinum-sensitive ovarian cancer (ICON6): a randomised, double-blind, placebo-controlled phase 3 trial. Lancet (2016) 387(10023):1066–74. doi: 10.1016/S0140-6736(15)01167-8
121. du Bois A, Floquet A, Kim JW, Rau J, del Campo JM, Friedlander M, et al. Incorporation of pazopanib in maintenance therapy of ovarian cancer. J Clin Oncol (2014) 32(30):3374–82. doi: 10.1200/JCO.2014.55.7348
122. Monk BJ, Poveda A, Vergote I, Raspagliesi F, Fujiwara K, Bae D-S, et al. Anti-angiopoietin therapy with trebananib for recurrent ovarian cancer (TRINOVA-1): a randomised, multicentre, double-blind, placebo-controlled phase 3 trial. Lancet Oncol (2014) 15(8):799–808. doi: 10.1016/S1470-2045(14)70244-X
123. Homicsko K, Duraiswamy J, Doucey M-A, Coukos G. Combine and conquer: Double CTLA-4 and PD-1 blockade combined with whole tumor antigen vaccine cooperate to eradicate tumors. Cancer Res (2016) 76(23):6765–7. doi: 10.1158/0008-5472.CAN-16-2868
124. Zamarin D, Burger RA, Sill MW, Powell DJ, Lankes HA, Feldman MD, et al. Randomized phase II trial of nivolumab versus nivolumab and ipilimumab for recurrent or persistent ovarian cancer: An NRG oncology study. J Clin Oncol (2020) 38(16):1814–23. doi: 10.1200/JCO.19.02059
125. Bockorny B, Semenisty V, Macarulla T, Borazanci E, Wolpin BM, Stemmer SM, et al. BL-8040, a CXCR4 antagonist, in combination with pembrolizumab and chemotherapy for pancreatic cancer: the COMBAT trial. Nat Med (2020) 26(6):878–85. doi: 10.1038/s41591-020-0880-x
126. Binnewies M, Pollack JL, Rudolph J, Dash S, Abushawish M, Lee T, et al. Targeting TREM2 on tumor-associated macrophages enhances immunotherapy. Cell Rep (2021) 37(3):109844. doi: 10.1016/j.celrep.2021.109844
127. Kusmartsev S, Eruslanov E, Kübler H, Tseng T, Sakai Y, Su Z, et al. Oxidative stress regulates expression of VEGFR1 in myeloid cells: link to tumor-induced immune suppression in renal cell carcinoma. J Immunol (2008) 181(1):346–53. doi: 10.4049/jimmunol.181.1.346
128. Wallin JJ, Bendell JC, Funke R, Sznol M, Korski K, Jones S, et al. Atezolizumab in combination with bevacizumab enhances antigen-specific T-cell migration in metastatic renal cell carcinoma. Nat Commun (2016) 7:12624. doi: 10.1038/ncomms12624
129. Liu JF, Herold C, Gray KP, Penson RT, Horowitz N, Konstantinopoulos PA, et al. Assessment of combined nivolumab and bevacizumab in relapsed ovarian cancer: A phase 2 clinical trial. JAMA Oncol (2019) 5(12):1731–8. doi: 10.1001/jamaoncol.2019.3343
130. Vanacker H, Harter P, Labidi-Galy SI, Banerjee S, Oaknin A, Lorusso D, et al. PARP-inhibitors in epithelial ovarian cancer: Actual positioning and future expectations. Cancer Treat Rev (2021) 99:102255. doi: 10.1016/j.ctrv.2021.102255
131. Ghisoni E, Imbimbo M, Zimmermann S, Valabrega G. Ovarian cancer immunotherapy: Turning up the heat. Int J Mol Sci (2019) 20(12):2927. doi: 10.3390/ijms20122927
132. Domchek SM, Postel-Vinay S, Im S-A, Park YH, Delord J-P, Italiano A, et al. Olaparib and durvalumab in patients with germline BRCA-mutated metastatic breast cancer (MEDIOLA): An open-label, multicentre, phase 1/2, basket study. Lancet Oncol (2020) 21(9):1155–64. doi: 10.1016/S1470-2045(20)30324-7
133. Banerjee S, Imbimbo M, Roxburgh P, Kim JW, Kim MH, Plummer R, et al. 529MO phase II study of olaparib plus durvalumab with or without bevacizumab (MEDIOLA): Final analysis of overall survival in patients with non-germline BRCA-mutated platinum-sensitive relapsed ovarian cancer. Ann Oncol (2022) 33:S788–S9. doi: 10.1016/j.annonc.2022.07.657
Keywords: tumor microenvironment, immunotherapy, immune cells, stromal cells, combination therapy
Citation: Wang Y, Zhang L, Bai Y, Wang L and Ma X (2022) Therapeutic implications of the tumor microenvironment in ovarian cancer patients receiving PD-1/PD-L1 therapy. Front. Immunol. 13:1036298. doi: 10.3389/fimmu.2022.1036298
Received: 04 September 2022; Accepted: 27 September 2022;
Published: 20 October 2022.
Edited by:
Haitao Wang, National Cancer Institute (NIH), United StatesReviewed by:
Yi Liu, Nanjing Medical University, ChinaCopyright © 2022 Wang, Zhang, Bai, Wang and Ma. This is an open-access article distributed under the terms of the Creative Commons Attribution License (CC BY). The use, distribution or reproduction in other forums is permitted, provided the original author(s) and the copyright owner(s) are credited and that the original publication in this journal is cited, in accordance with accepted academic practice. No use, distribution or reproduction is permitted which does not comply with these terms.
*Correspondence: Li Wang, d2FuZ2xpMzcwMjkxOTJAc2luYS5jb20=; Xuelei Ma, ZHJtYXh1ZWxlaUBnbWFpbC5jb20=
†These authors share first authorship
Disclaimer: All claims expressed in this article are solely those of the authors and do not necessarily represent those of their affiliated organizations, or those of the publisher, the editors and the reviewers. Any product that may be evaluated in this article or claim that may be made by its manufacturer is not guaranteed or endorsed by the publisher.
Research integrity at Frontiers
Learn more about the work of our research integrity team to safeguard the quality of each article we publish.