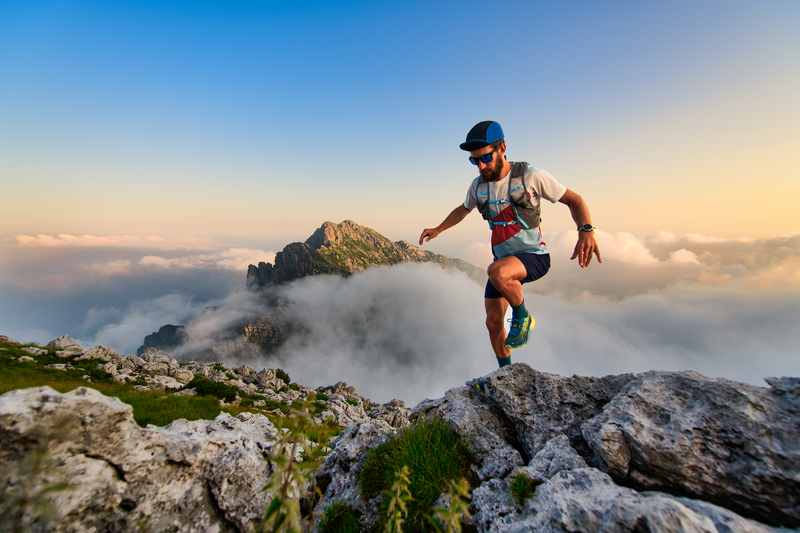
95% of researchers rate our articles as excellent or good
Learn more about the work of our research integrity team to safeguard the quality of each article we publish.
Find out more
REVIEW article
Front. Immunol. , 06 October 2022
Sec. Cancer Immunity and Immunotherapy
Volume 13 - 2022 | https://doi.org/10.3389/fimmu.2022.1034903
This article is part of the Research Topic Community Series in Novel Insights into Immunotherapy Targeting Tumor Microenvironment in Ovarian Cancer, volume I View all 13 articles
Ovarian cancer, one of the most common gynecological malignancies, is characterized by high mortality and poor prognosis. Cytoreductive surgery and chemotherapy remain the mainstay of ovarian cancer treatment, and most women experience recurrence after standard care therapies. There is compelling evidence that ovarian cancer is an immunogenic tumor. For example, the accumulation of tumor-infiltrating lymphocytes is associated with increased survival, while increases in immunosuppressive regulatory T cells are correlated with poor clinical outcomes. Therefore, immunotherapies targeting components of the tumor microenvironment have been gradually integrated into the existing treatment options, including immune checkpoint blockade, adoptive cell therapy, and cancer vaccines. Immunotherapies have changed guidelines for maintenance treatment and established a new paradigm in ovarian cancer treatment. Despite single immunotherapies targeting DNA repair mechanisms, immune checkpoints, and angiogenesis bringing inspiring efficacy, only a subset of patients can benefit much from it. Thus, the multi-immunotherapy investigation remains an active area for ovarian cancer treatment. The current review provides an overview of various clinically oriented forms of multi-immunotherapy and explores potentially effective combinational therapies for ovarian cancer.
Ovarian cancer is the most lethal gynecological malignancy, of which epithelial ovarian cancer (EOC) is the most prevalent subtype. Most EOC patients are diagnosed with advanced stage accompanied with tumor spread to the peritoneal cavity. Current frontline treatments include debulking surgery, platinum-taxane maintenance chemotherapy, and recently developed targeted agents and immunotherapy. Despite aggressive treatment, the 5-year survival rate for women diagnosed with stage III or IV disease is still less than 25% (1). Most patients would suffer a recurrence after the initial response to therapy and almost all of them resistance to chemotherapy and leading to the death.
Growing evidence suggests that ovarian cancer is immunogenic cancer. There has been a significant increase in understanding of molecular and genetic changes in the ovarian cancer microenvironment. Thus, various immunotherapies target the tumor microenvironment (TME) and attempt to address the challenges posed by the highly immunosuppressive TME (2). Current immunotherapy for ovarian cancer includes immune checkpoint blockade, adoptive cell therapy, cancer vaccine, oncolytic virus and so on (Figure 1). Despite several of them achieving inspiring efficacy in the clinic, such as PARP inhibitors. Only a tiny fraction of patients benefited from them, and most of them would eventually suffer a recurrence or progression. With the limited efficacy brought by studies testing single-agent immunotherapy in recurrent ovarian cancer, optimism has resurfaced around the possibility that combinational therapy would deliver the better outcome expected by the community. In this review, we summarize the progress of clinical developments in multi-immunotherapies for ovarian cancer and briefly discuss the future directions of combinational therapies in ovarian cancer.
Figure 1 Immunotherapies in ovarian cancer. Created with BioRender.com.
The TME comprises the extracellular matrix (ECM) and stromal cells. The ECM consists of water, proteoglycans, minerals, and fibrous proteins secreted by resident cells in an interlocking network (3). The ECM plays a critical role during tumorigenesis, affecting cell migration, invasion, and metastasis. Besides, stromal rearrangement plays a supportive role during the malignancy progresses and eventually, the tumoral and stromal changes aggravate each other and promote a dynamic reciprocity cycle (4). The matrix-centric, stromal-targeted cancer therapies developed as the ECM is altered at the biochemical, architectural, biomechanical, and topographical levels (5). Stromal cells in the TME include cancer-associated adipocytes, mesothelial cells, fibroblasts, and immune cells. Immune cells include tumor-infiltrating lymphocytes (TILs), Tregs, neutrophils, macrophages, dendritic cells (DCs), natural killer (NK) cells, myeloid-derived suppressor cells (MDSCs), polymorphonuclear neutrophils (PMNs), and so on (6, 7) (Figure 2). The tumor-permissive TME is achieved by reprogramming host cells to support tumor phenotypes and functions (6). The metastatic tropism of cancer cells to the omentum, characterized by highly vascularized immune cell structures called milky spots, plays a critical role in the generation of the metastatic TME in the intraperitoneal cavity (6). In addition, not only components in the TME communicate and impact each other, but also ovarian cancer cells communicate with TME through various signaling pathways, such as STATs family pathway, IL-6 pathway, and NF-KB pathway (1). Several factors are associated with response to immunotherapy, including T cell exhaustion, PD-L1 status, microsatellite instability, mismatch repair deficiency, Tumor mutation burden (TMB), CD8+ positivity, T cell infiltration and so on (8). Thus, immunotherapies target TME developed, current immunotherapies target ovarian cancer TME including CAFs targeting therapy, anti-angiogenesis therapy, immune checkpoint inhibitors (ICIs), oncolytic virus and so on (9). Tumors responsive to ICIs are usually called hot tumors, which depends on T cells’ infiltration. On the contrary, cold tumors usually do not respond to ICIs, which is characterized by poor T cell infiltration (10). Besides, the effectiveness of immunotherapy is associated with baseline immune responses and unleashing of pre-existing immunity. Thus, combinational immunotherapies may boost weak antitumor immunity, enhance tumor antigens cross-presentation, and promotes T cell priming and infiltration (11).
Figure 2 Tumor microenvironment in ovarian cancer. Created with BioRender.com. TIL: Tumor-infiltrating lymphocytes (TILs), APC: Antigen-presenting cell, MDSC: Myeloid-derived suppressor cells (MDSCs), Treg: Regulatory T, CSC: cancer stem cell.
There are at least five recognized pathways that exist for DNA repair: direct repair, mismatch repair (MMR), nucleotide excision repair (NER), base excision repair (BER), and double-strand break (DSB) recombinational repair. DSB occurs by non-homologous end-joining and high-fidelity homologous recombination repair, which is much more error prone (12). Besides, germline aberrations in critical DNA repair and DNA-damage response (DDR) genes contribute to cancer susceptibility syndromes, including BRCA1, BRCA2, BLM, FANCA, TP53, RAD51C, and MSH2. After exposure to carcinogens, the generation of DNA damage increases the risk of cancer. Therefore, genomic instability is a recognized hallmark of cancer (13). Various agents are developed to target different processes during DNA repair, including PARP inhibitors, NER inhibitors, BER inhibitors, DDR kinases inhibitors, inhibitors targeting termini recognition, end bridging, DNA-end processing, and DNA ligation, inhibitors targeting homology directed repair and Rad51 (14). We will focus on PARPi-based combinational therapies, as it is most widely studied in ovarian cancer.
The poly (ADP-ribose) polymerase (PARP) is a recognized sensor of DNA damage, which is known for its role in DNA BER and DNA single-strand breaks (SSB) repair. The role of PARP in DSB repair is less elucidated (13). PARP inhibitors have been a new targeted treatment for ovarian cancer, particularly in women with BRCA1 and BRCA2 mutation or patients without a functional homologous recombination repair pathway (15). Homologous recombination deficient cells are susceptible to PARP inhibitors. BRCA1 and BRCA2 are tumor suppressor genes. They are associated with fundamental roles in DNA repair by forming a homologous recombination repair complex (16). Several PARP inhibitors are approved by the US Food and Drug Administration (FDA) or studied in clinical trials, including olaparib, niraparib, rucaparib, veliparib, and talazoparib (17). On March 27, 2017, niraparib was approved by the US FDA. The approval is based on the results of NOVA (NCT01847274) (18). On April 6, 2018, the US FDA approved rucaparib for the maintenance treatment. The approval relies on ARIEL3 (NCT01968213) (19, 20). Based on the results of SOLO-1 (NCT01844986), on December 19, 2018, the US FDA approved olaparib for the maintenance treatment of adult patients with germline or somatic BRCA-mutated (gBRCAm or sBRCAm) who exhibited either a complete or partial response to first-line platinum-based chemotherapy (21). Nevertheless, a recent clinical trial indicated that the efficacy of platinum-based subsequent chemotherapy seems to be reduced in BRCA1/2-mutated patients with platinum-sensitive relapsed ovarian cancer (PSROC) compared to patients who haven’t received PARPi therapy (22). Despite the inspiring benefits PARPi brought, lots of limits still exist. Future studies should focus more on combinations that can enhance the effect of PARPi, benefit patients with non-HRD tumors, mitigate toxicity, and overcome PARPi resistance (23). Therefore, the combination of PARPi and other immunotherapies are developed, especially antiangiogenic agents and immune checkpoint inhibition.
Angiogenesis plays a vital role in normal ovarian physiology as well as in ovarian cancer pathogenesis. Tumor progression and growth largely depend on angiogenesis, as tumor could not grow beyond 1-2 mm if the neovascularization cannot meet the requirements of nutrients and oxygen. Thus, antiangiogenic agents have been incorporated into the therapy regimen for ovarian cancer. Vascular endothelial growth factor (VEGF) and VEGF receptor (VEGFR) are primarily explored in clinical settings, and this pathway contributes to malignant ascites and tumor progression (24). Besides, it is also shown that overexpressed VEGF is correlated with tumor staging and prognosis (25). Plenty of angiogenesis inhibitors are being investigated, including Bevacizumab, Aflibercept, Nintedanib, Cediranib, Pazopanib, Sunitinib, Sorafenib, and Trebananib (26). Approved by the FDA, Bevacizumab exhibited modest efficacy, and most patients developed acquired resistance. Therefore, the combination of PARPi and angiogenesis inhibitors are reasonable and meaningful.
There are two purposes for combining PARPi and angiogenesis inhibitors. Firstly, PARPi could decrease angiogenesis (27). Secondly, both VEGF3 inhibitors and hypoxia induce the downregulation of HRD proteins (28, 29). On May 8, 2020, the indication of olaparib was expanded to combination therapy with bevacizumab for first-line maintenance treatment of HRD-positive advanced ovarian cancer (30). The approval was based on the PAOLA-1 trial, which revealed that combined therapy of bevacizumab and olaparib provided a significant progression-free survival (PFS) benefit in HRD-positive patients, regardless of whether the patient had the BRCA mutation (31). More combinational strategies are being studied. In a patient-derived ovarian cancer xenografts (OC-PDXs) model, the combination of PARPi Olaparib and VEGFR inhibitor cediranib reduced the growth of all OC-PDXs independent of BRCA status (32). In 2014, a phase 2 study revealed that Cediranib plus Olaparib could prolong PFS (33). Later, a phase 3 clinical study NRG-GY004 showed that combining Cediranib and Olaparib did not prolong PFS compared with chemotherapy and resulted in reduced patient-reported outcomes (PRO) (34). Besides, other combinational strategies are being investigated too. Compared to monotherapy, niraparib plus bevacizumab significantly increased the PFS of platinum-sensitive recurrent ovarian cancer, while a more extensive scale phase 3 clinical trial is planned (35, 36). More preclinical and clinical studies are needed to provide information about the most appropriate combination strategy and which subset of patients in what clinical setting benefit most.
In addition to antiangiogenic agents, PARPi was combined with other targeted immunotherapies, such as PD-1/PD-L1 inhibitors, WEE-1 inhibitors, ataxia-telangiectasia-mutated-and-Rad3-related kinase (ATR) inhibitors, MEK inhibitors, and so on (37). Plenty of studies regarding PARPi and PD-1/PD-L1 combinational therapy are completed or ongoing. Olaparib, niraparib, rucaparib, and talazoparib are combined with anti-PD-1 antibodies (nivolumab, pembrolizumab) and anti-PD-L1 antibodies (durvalumab, atezolizumab, avelumab) (38). PARPi and PD-1/PD-L1 antibodies demonstrated synergistic antitumor activities in animal models regardless of BRCA mutation status, which is achieved by blockade of single-stranded DNA damage repair and activation of the STING-dependent immune response. Moreover, PARPi induces an immunostimulatory micromilieu in ovarian cancer, thereby complementing the activity of PD-1/PD-L1 blockade (39, 40). A phase 2 clinical trial revealed that a combination of olaparib and durvalumab showed modest efficacy whereas blockade of VEGF/VEGFR would be necessary to improve the combination (41). PARPi was also combined with many other ICB in ovarian cancer, such as inhibitors target phosphatidylinositol-4,5- bisphosphate 3-kinase (PI3K) (42, 43), V-akt murine thymoma viral oncogene homolog (AKT) (44), ATR (45, 46), heat shock protein 90 (HSP90) (47, 48), checkpoint kinase 1 (CHK1) (49), cytotoxic T-lymphocyte-associated protein 4 (CTLA-4) (50), salt-inducible kinase 2 (SIK2) (51), insulin-like growth factor-1 receptor (IGF-1R) (52). However, most of the combinations are still in preclinical or phase 1 clinical studies, and a larger scale of clinical studies is needed to further evaluate the efficacy. In addition, the natural compound alantolactone (ALT) could inhibit the thioredoxin reductase, thus inducing ROS accumulation and oxidative DNA damage in cancer cells. A combination of pro-oxidative agent ALT and Olaparib induced tumor regression, which broadened the application of PARP inhibitors (53).
Other agents targeting DNA repair are much less investigated in ovarian cancer. Some studies report their application in other types of cancers as previously reviewed (14). More data are needed on ovarian cancer.
Adoptive cell therapy (ACT) mainly refers to chimeric antigen receptor (CAR)-modified T cells, T-cell receptor (TCR)-engineered T cells, natural TILs, CAR-NK cells, and CAR-macrophages. ACT has achieved a remarkable revolution in the hematological tumor. Nevertheless, for solid tumors, including ovarian cancer, ACT seems insufficient to elicit significant antitumor activity. In ovarian cancer, CAR-T cells target folate-receptor alpha (FRα), mesothelin, MUC-1, and HER2 have been widely investigated. However, no satisfactory therapeutic efficacy has been observed so far. The low avidity and heterogeneous expression of targetable membrane antigens and difficulties in CAT-T cell infiltration and survival are the key obstacles (54). Novel targets or combinational therapies are expected to solve these problems. For instance, CAR-T cells targeting the Mullerian inhibiting substance type 2 receptor (MISIIR), B7-H3, Epithelial cell adhesion molecule (EpCAM), C-X-C chemokine receptor 1 (CXCR1), or C-X-C chemokine receptor 2 (CXCR2), 5T4 significantly controlled tumor growth in vivo (55–59). Apart from CAR-T therapy, other ACT, including TCR-T and CAR-NK, are also under investigation. TCR-T therapy is MHC restricted and relies on the presentation of the MHC complex. Unlike CAR-T therapy, whose target antigens are only cell surface proteins, TCR-T could recognize both intracellular antigen fragments and surface proteins as long as MHC molecules present them. In ovarian cancer, TCR-T targeting melanoma-associated antigen 4 (MAGE-A4) and New York esophageal-1 (NY-ESO-1) are in early clinical trials (60). CAR-NK targeting folate receptor alpha (αFR) (61), glypican-3 (GPC3) (62), human leukocyte antigen G (HLA-G) (63), CD44 (64), CD24 (65), CD133 (66), MSLN (67) have achieved therapeutic efficacy in preclinical studies. More clinical data are needed to verify their efficacy in ovarian cancer patients.
As we mentioned, a common mechanism of tumor escape from single-target CAR-T cells is the downregulation and mutational loss of the targeted antigen. Thus, targeting multiple antigens may improve the efficacy of CAR-T cells. Several bispecific CAR-T products are under investigation. For instance, Zhen et found that folate receptor 1 (FOLR1) and mesothelin (MSLN) are specifically highly expressed in ovarian cancer cells by screening the GEO database. Therefore, they established tandem CAR-T cells target both FOLR1 and MSLN, and the tandem CAR-T cells exhibited enhanced antitumor activity and prolonged mouse survival compared to single-target CAR-T cells (68). Besides, MSLN CAR-T-secreting anti-CD40 antibody had a more powerful cytotoxic effect on ovarian tumor (69). Dual targeting tumor-associated glycoprotein 72 (TAG-72) and CD47 are effective in ovarian cancer model (70). CAR-T cells targeting PDL1 and MUC16 also demonstrated more potent antitumor efficacy than single-target CAR-T cells (71). Dual CAR-T cells targeting NKG2D and PD-1 ligands exhibited inspiring efficacy in treating metastatic peritoneal tumors (72). In the clinic, CAR-T cells targeting MSLN and PD-1 combined with apatinib exhibited potent therapeutic efficacy in one patient with refractory EOC (73). To summarize, most bispecific CAR-T therapies in ovarian cancer are still in the preclinical stages. Future studies should search for more specific and practical targets in the clinic.
According to the modest efficacy of CAR-T in ovarian cancer, several agents are applied to enhance CAR-T cells’ efficacy. Firstly, the efficacy of ICIs limited by a lack of a tumor-reactive microenvironment. CAR-T cells may provide the necessary tumor-targeting immune infiltrate. Conversely, ICIs counteract the immunosuppressive environment that undermines optimal CAR-T cell efficacy (74). Thus, combining ICI with CAR-T could be a promising strategy. By loading anti-HER2 or anti-EGFR bispecific antibodies, CD19-CAR-T and activated T cells showed comparable specific cytotoxicity against ovarian cancer cells (75). In addition, arm CAR-T cells with therapeutic cytokines. For instance, IL-12 secreting 4H11-28z CAR-T cells showed enhanced proliferation and antitumor ability compared to 4H11-28z CAR-T cells only (76). Besides, pretreatment of ovarian cancer cells with histone deacetylase inhibitor sodium valproate (VPA) could upregulate NKG2DL expression in ovarian cancer cells expressing low to moderate NKG2DL. Consequently, chimeric NKG2D CAR-T cells exhibited better efficacy by enhanced immune recognition (77). In some papers, upregulation or downregulation of certain receptors could enhance CAR-T cells’ efficacy. Co-expressing of CXCR2 enhanced homing and efficacy of CAR-T cells targeting the integrin αvβ6 (78). Besides, adenosine 2A receptors (A2aRs) disruption improved the efficacy of CAR-T cells targeting MSLN (79). As we mentioned before, poor T cell infiltration contributes to the failure of CAR-T therapy. Therefore, to improve T cell infiltration in ovarian cancer, a vascular disrupting agent (VDA) called combretastatin A-4 phosphate (CA4P) was combined with CAR-T cells and results indicated that CA4P enhanced the efficacy of CAR-T cells and could be an effective antitumor agent candidate in treating solid tumor (80). In addition, a substantial body of work suggests that the accumulation of adenosine in the TME contributed to the failure of immunotherapies. As a result, adenosine deaminase 1 (ADA) overexpression improved CAR-T cells’ antitumor ability in ovarian cancer (81). In summary, CAR-T-associated combinational therapy is still preclinical studies, and more reasonable and effective combinational strategies are being exploited.
CAR-NK, TCR-T and CAR-macrophage therapy are alternate cell-based therapies. Cancer-testis antigens (CTA) are developed as targets for TCR-T, including MAGE-A4 and NY-ES0-1 (60). CAR-NK offers some significant advantages compared to CAR-T, such as better safety, multiple cytotoxic mechanisms, and high feasibility for “off-the-shelf” manufacturing (82). CAR-NK against human leukocyte antigen G (HLA-G) inhibited tumor growth in vitro and in vivo, and such efficacy was enhanced when combined with chemotherapeutic agents (63). Besides, CXCR1 expression could enhance the antitumor efficacy of NKG2D CAR-NK, which provided a novel strategy for improving the therapeutic efficacy of NK cells (83). CAR-Macrophage own unique advantages. CAR-macrophage could significantly immerse in the TME, and direct kill tumor cells as well as enhance T cell function. In addition, CAR-macrophage has fewer non-tumor toxicities compared to CAR-T (84). Most CAR-macrophage therapies are in the preclinical stage, including CAR-macrophage targeting CD19, CD22, HER2, CCR7 and so on. Only several phase 1 clinical trials for solid tumors are ongoing (85). In ovarian cancer, reports of CAR-NK, TCR-T, and CAR-macrophage are rare. More data from preclinical and clinical studies are needed to prove the safety and antitumor efficacy.
A single application of cancer vaccine in ovarian cancer is under exploration, such as peptide vaccine, whole tumor cell vaccine, cancer stem cells (CSCs), antigen-presenting cell (APC) vaccine, DNA/RNA vaccine, bacteria vaccine and so on. Most of them augment antitumor immunity in ovarian cancer patients. Nevertheless, clinical data only revealed modest efficacy in most patients. Therapeutic efficacy in more patients is testable (86–92). Despite most cancer vaccines only achieving moderate efficacy in other malignancies, combining cancer vaccines and other immunotherapies may broaden its application and elevate efficacy. For instance, murine ovarian cancer cell ID8 was spray dried and made into a microparticulate vaccine. The microparticulate ovarian cancer vaccine exhibited the most efficacious in inhibiting tumor growth when administered with interleukins (93). Adding immunomodulator agents such as IL-12 may augment the efficacy of cell-based cancer vaccine (94). In a phase 2 trial, a multiepitope FRα vaccine called TPIV200 was combined with PD-L1 inhibitor durvalumab in treating advanced platinum-resistant ovarian cancer. The combination was safe and elicited robust FRα-specific immune responses (95). Dual blockade of PD-1 and CTLA-4 enhanced efficacy of the GVAX vaccine in ovarian cancer models through activation of CD4 and CD8 T cells, secretion of cytokines, and inhibition of Treg cells (96). Besides, immunostimulatory adjuvant could elevate the efficacy of cancer vaccines. For instance, cowpea mosaic virus (CPMV) co-delivered with irradiated ovarian cancer cells elicited prophylactic efficacy and immunologic memory responses in mice models (97). 21 recurrent high-grade serous ovarian cancer (HGSOC) patients were treated with a polyvalent antigen-KLH plus OPT-821 vaccine and bevacizumab. Results indicated that the combinational therapy was well-tolerated. Although immunogenic responses were not associated with improved survival, researchers discovered that increased IL-18 correlated with improved PFS while increased PDGF was associated with worse OS (98). Gemogenovatucel-T (Vigil) is an autologous whole tumor cell vaccine transfected with GM-CSF gene and silenced of furin, the critical convertase responsible for activation of TGFβ-1 and TGFβ-2. The vigil was well-tolerated, but the primary endpoint was not met (99). A combination of vigil and a PD-L1 blocking antibody atezolizumab was safe. Further clinical exploration was justified (100). Apart from peptide and irradiated tumor cell vaccine, DC vaccine was combined with ex vivo-stimulated autologous T cells. Six patients were enrolled in this study. They received bevacizumab plus autologous DC pulsed with tumor lysate supernatants, followed by lymphodepletion and adoptive transfer of autologous vaccine-primed and CD3/CD28-stimulated T cells. Four patients benefit from the therapy, including two partial responses (PR) and two stable disease (SD) (101). Combining human monocytes and IFN-α2a and IFN-γ mediated potent antitumor effect in ovarian cancer (102). Immuno-modulators, including anti-CD40Ab and TLR3 ligand—poly(I:C), could enhance the antitumor effect of a DNA vaccine encoding MSLN and antigen-specific connective tissue growth factor (CTGF) (103). CPMV in situ vaccination combined with CD47-blocking antibody promoted macrophage activity and enhanced T cell function in ovarian cancer model (104). To summarize, most cancer vaccines could not wholly eradicate established tumors. They exhibit better therapeutic effects when tumor volume is small and the vaccine is given in an adjuvant setting (105).
Dual inhibition of PD-1/PD-L1 exhibited better efficacy in ovarian cancer compared to single-target. Bispecific targeting of PD-1 and PD-L1 induced superior cellular changes in T and NK cells compared to monospecific targeting (106). Besides, A soluble form of the PD-1 receptor (sPD-1) neutralized both PD-L1 and PD-L2 and achieved better efficacy. PD-L2 blockade facilitates ICB resistance through incomplete blockade of the PD-1 signaling pathway (107).
More inhibitors simultaneously target two signaling pathways to enhance the antitumor effects. APCS-540, a newly developed inhibitor targeting glycogen synthase kinase 3 beta (GSK3B) and histone deacetylases (HDACs), inhibited tumor growth and prolonged survival in an ovarian cancer model (108). Another inhibitor, Istiratumab, bispecific targets IGF-1R and epidermal growth factor receptor 3 (ErbB3). Istiratumab could be a candidate for treating chemotherapy-resistant ovarian cancer (109). Besides, MSC2363318A is a newly developed inhibitor targeting AKT1, AKT3, and P70S6K. Yes-associated protein (YAP1) could be a marker that predicts ovarian tumors’ sensitivity to MSC2363318A (110). HKMTI-1-005 simultaneously inhibited the histone methyltransferase G9A and EZH2, which elicited antitumor efficacy in HGSOC (111). Several papers focus on the pro-tumorigenic microenvironment induced by chemotherapy. Tumor cell debris produced by platinum- and taxane-based chemotherapy stimulates a “surge” of macrophage-derived proinflammatory cytokines and bioactive lipids. A dual cyclooxygenase-2 (COX-2) and soluble epoxide hydrolase (sEH) inhibitor PTUPB decreased proinflammatory cytokines and lipids in the TME and delayed ovarian tumor growth (112).
When certain ICI works, it is possible that a compensatory signaling pathway was induced, providing an idea of the dual blockade. As one of the most widely applicated inhibitors, PD-1/PD-L1 inhibitors are combined with various inhibitors. Dual blockade of CXCL12-CXCR4 and PD1-PDL1 enhanced antitumor effects compared with the single blockade, which was associated with increased effector T cells infiltration and function, increased memory T cells, and decreased Treg cells in the TME (113). Dual blockade of PD-1 and CTLA-4 elicited antitumor efficacy in preclinical studies (114). A combination of PD-1 inhibitor Nivolumab and CTLA-4 inhibitor Ipilimumab in EOC patients resulted in superior responses and longer PFS (115). PD-1 inhibitor LY3300054 and CHK1 inhibitor prexasertib combinational therapy were tolerable and demonstrated preliminary efficacy in HGSOC patients (116). PD-L1 inhibitor atezolizumab and VEGF inhibitor bevacizumab achieved durable responses and/or disease stabilization in some platinum-resistant ovarian cancer patients (117). High expression of CXCL13 predicted a more prolonged survival and facilitated the maintenance of CXCR5+CD8+ T cells. Besides, CXCL13, combined with anti-PD-1 therapy, significantly retarded ovarian tumor growth (118). Combining cyclin-dependent kinases 4 and 6 (CDK4/6) inhibitor abemaciclib and anti-PD-1 therapy may have a better promise for poorly immune-infiltrated ovarian cancer (119).
Despite that more than 60% of ovarian cancers are positive for the estrogen receptor (ER), ER-targeted treatment in ovarian cancer was disappointing. Src is also activated in most ovarian cancers. It was found that estrogen could activate Src to phosphorylate p27, thus promoting its degradation and increasing cell-cycle progression. Combinational ER and Src blockade therapy by fulvestrant and saracatinib increased cell-cycle arrest, induced autophagy, and inhibited ovarian cancer growth in vivo (120, 121). Apart from Src inhibitor, MEK inhibitor selumetinib could also reverse antiestrogen resistance in ER-positive HGSOC. Besides, MAPK overexpression predicted poor prognosis and may help identify MEK inhibitor-responsive cancer (122).
Although the EGFR signaling pathway is usually activated and associated with a poor prognosis, clinical results of EGFR inhibition in recurrent ovarian cancer patients are disappointing. An article revealed that STAT3 activation might contribute to resistance to EGFR inhibition. Therefore, combined inhibition of EGFR and JAK/STAT3 had synergistic antitumor effects, whereas combinational inhibition of other pathways, including AKT/mTOR, MEK, and SRC, was relatively less effective (123). 12 patients received intraperitoneal cisplatin, intraperitoneal TLR3 ligand rintatolimad, and oral COX-2 blocker celecoxib. The study revealed that the combination was safe and tolerable. A phase 2 clinical trial would be tested (124). The insulin growth factor 1 (IGF-1) expression was elevated in two ovarian cancer models treated with bevacizumab. Dual blockade of IGF-1 and VEGF resulted in increased tumor growth inhibition (125). Delta-like ligand 4 (Dll4), one of the Notch ligands, is overexpressed in ovarian cancer. Dual blockade of Dll4 and VEGF markedly reduced ovarian cancer cell growth (126). Overexpression of BCL2L1 was associated with platinum resistance to multiple anti-cancer agents in ovarian cancer. Dual inhibition of FGFR4 and BCL-xL demonstrated potent efficacy and tolerable toxicity (127). Forkhead domain inhibitor-6 (FDI-6) is a forkhead box protein M1 (FOXM1). FDI-6 inhibition elicited the upregulation of N-Ras, phosphoprotein kinase Cδ (p-PKCδ), and HER3. Combination FDI-6 with tipifarnib (N-Ras inhibitor), rottlerin (p-PKCδ inhibitor), or sapitinib (HER3 inhibitor) decreased the survival of cancer cells (128). Src and MAPK are activated in HGSOC. Dual blockade of Src and MAPK by saracatinib and selumetinib inhibited ovarian tumor growth and targeted tumor initiating stem-like cells (129). Dual inhibition of DNA methylation and histone H3 lysine 9 dimethylation by 5-aza-CdR and G9Ai increased viral mimicry and served as a basis for this combination strategy (130). Combined inhibition of MEK and BCL-2/XL had therapeutic efficacy in HGSOC models, and BIM protein was a biomarker of responsiveness (131). Dual inhibition of PI3K/mTOR and RAS/ERK by PF-04691502 and PD-0325901 showed robust synergistic antitumor efficacy (132).
Targeting agents participating in cancer cell metabolism are being explored. Dual inhibition of glycolysis and glutaminolysis could be a promising therapeutic strategy in ovarian cancer (133). Similarly, A triphenylphosphonium-modified terpyridine platinum (II) complex (TTP) inhibited multiple mitochondrial and glycolytic bioenergetics, thus inducing a hypometabolic state in several cancers, including ovarian cancer (134).
Besides EOC, research on other types of ovarian cancer was much less. The PI3K and murine double minute 2 (MDM2) upregulation predict a worse outcome in clear cell ovarian carcinoma (CCOC). Dual inhibition of PI3K and MDM2 by DS-7423 and RG7112 significantly reduced CCOC growth (135).
Although ICIs have changed the practice of cancer treatment and prognosis, the application of ICIs for ovarian cancer is limited. Adding cytotoxic cytokines or neutralizing immunosuppressive cytokines may augment the efficacy. IL-10 in the TME sustained the immunosuppression in ovarian cancer. Therefore, IL-10 neutralization enhanced the antitumor efficacy of PD-1 blockade, and the combinational therapy prolonged survival and decreased tumor burden through T cell and B cell immunity in mice (136). Besides, active immunotherapy precedes administrated of ICI. Thus, promoting T cell maturation and resistance to the cytotoxic effects of the Bcl-2 inhibitor (137).
Oncolytic viruses are gene-modified or naturally occurring viruses that selectively replicate and destroy cancer cells without harming the normal tissues (138). Adenovirus, herpes simplex virus (HSV), poxvirus, and measles virus are the most well-known oncolytic viruses in cancer therapy (105, 139). The oncolytic virus is combined chiefly with ICB in ovarian cancer. For example, oncolytic Maraba virus and PD-1 blockade combination mediated heterogeneous radiologic patterns through non-invasive MRI scanning (140). Plant virus CPMV nanoparticles conjugated with anti-PD-1 peptide had superior efficacy against metastatic ovarian cancer compared to adding free anti-PD-1 peptide (141). Oncolytic vaccinia virus therapy in ovarian cancer induced expression of PD-L1 in cancer cells and immune cells. Therefore, combining therapy of oncolytic vaccinia virus and PD-L1 blockade could synergistically enhance therapeutic efficacy (142).
Moreover, oncolytic viruses could be genetically modified to express exogenous cytokines or proteins. A modified Vaccinia Ankara vaccine expressing wild-type human p53 (p53MVA) promoted T cell responses, and combination with gemcitabine or other agents was expected to exhibit superior clinical responses (143). In addition, the oncolytic vaccinia virus (VV) engineered to express a fusion protein of IL-15 and IL-15Ralpha was named vvDD-IL15-Rα. A combination of vvDD-IL15-Rα and PD-1 blockade exhibited a dramatic tumor regression (144). Mice were pretreated with three homologous thrombospondin type 1 repeat domains (3TSR) alone or followed by combination with a fusogenic oncolytic Newcastle disease virus (NDV). 3TSR could normalize tumor vasculature, thus enhancing NDV delivery and trafficking of immune cells to the tumor core. The combinational therapy resulted in a most significant reduction in tumor volume and ascites accumulation (145).
Oncolytic viruses are also combined with other immunogenic agents. The oncolytic vaccinia virus (OVV) was enhanced by MEK inhibitor PD0325901 and trametinib in doxorubicin-resistant ovarian cancer (146). Microtubule destabilizing agents (MDAs) could sensitize tumors to oncolytic virus therapy. The combination of trastuzumab emtansine and oncolytic vesicular stomatitis virus (VSVΔ51) demonstrated that a viral-sensitizing molecule could enhance oncolytic virus efficacy (147). Infection of RNA virus induced upregulation of heat shock protein 70 (HSP70). HSP70 increased measles virus cytotoxicity. HSP90 inhibitors could upregulate HSP70, therefore increasing the efficacy of measles virotherapy (148). Furthermore, modulating interferon modulators by JAK1/2 inhibitor ruxolitinib could overcome partial resistance of an oncolytic vesicular stomatitis virus variant pseudotyped with the nonneurotropic glycoprotein (VSV-GP) (149).
The combination of two types of viruses demonstrated enhanced efficacy. For example, infection with Semliki Forest virus-ovalbumin (SFV-OVA) followed by infection with vaccinia virus-ovalbumin (VV-OVA) induced an enhanced antitumor efficacy through a combination of viral oncolysis and antigen-specific immunity (150).
A limitation of recombinant oncolytic virus therapy is the viral clearance by neutralizing antibodies. Therefore, a study found that cyclooxygenase-2 (Cox-2) inhibitors may circumvent this limitation. Cox-2 inhibitors successfully inhibited the generation of neutralizing antibodies and exhibited more effective antitumor efficacy when combined with the vaccinia virus in ovarian cancer (151). Another obstacle to viral therapy is that oncolytic viruses are large particles. Thus, it is difficult to efficient extravasation from tumor blood vessels. A study proved that the oncolytic sindbis virus target tumor cells by the laminin receptor. Therefore, modulating vascular leakiness by VEGF or metronomic chemotherapy could enhance specific targeting and delivery of sindbis viral vectors (152). Combination of adeno-associated virus (AAV) expressing 3TSR and Fc3TSR and bevacizumab extended mice survival, suggesting a further investigation of such a combination (153). The application of adenoviruses is limited by rapid, systemic cytokine release and consequently inflammatory toxicity. To overcome this obstacle, researchers used β3 integrin to significantly reduce toxicity without compromising antitumor efficacy (154).
Chemotherapy combined with cytoreductive surgery is the mainstay treatment for ovarian cancer. Although the majority of people initially respond to platinum-based chemotherapy, most patients would suffer a recurrence within 5 years. Currently, most clinical studies regarding immunotherapies are applied to patients who previously received chemotherapy, as we discussed before (37). Resistance to platinum agents and PARP inhibitors is one of the main obstacles to ovarian cancer therapy (155). Thus, it’s urgent to explore novel targets or combinational strategies. RNA sequencing and panel DNA sequencing revealed that neoadjuvant chemotherapy induces genomic and transcriptomic changes, and combined treatment of AP-1 or SIK2 inhibitors with carboplatin or paclitaxel showed synergistic effects (156). RNA sequencing analysis also suggested that stress promoted chemoresistance, which provided targets to overcome chemo resistance (157). In addition, targeting LRRC15 could inhibit metastatic dissemination through β1-integrin/FAK signaling (158). Apart from preclinical studies, several clinical trials revealed that MEK inhibitor trametinib, Wee1 inhibitor adavosertib, and CDK4/6 inhibitor ribociclib showed preliminary efficacy in ovarian cancer (159–161). Overall, a single application of immunotherapy is unlikely to have a dramatically effect in ovarian cancer. Understanding the interplay between signal pathways may provide a better combined therapy of chemotherapy and immunotherapy.
Poor aqueous solubilities limited the application of several drugs. Nanoplatforms could help solve the barrier. Diblock copolymer nanoplatforms were used to formulate micelles through the solvent evaporation method. A dual drug loaded micelles (DDM) containing chetomin and everolimus targeted HIF and mTOR. The DDM significantly inhibited angiogenesis and induced apoptosis compared to the individual micells (162). Besides, ovarian tumor cells overexpress low-density lipoprotein receptors (LDLr). Thus, LDL-encapsulated cholesterol-conjugated heat shock protein 27 (HSP27) and human epidermal growth factor receptor 2 (HER2) dual inhibitor specifically targeted and inhibited ovarian cancer cells (163).
Radiotherapy was nearly abandoned in ovarian cancer due to its modest efficacy and toxicity. However, recent studies revealed that a low dose of radiotherapy might reprogram the tumor microenvironment and reverse tumor immune desertification and resistance to immunotherapy (164). Low-dose radiotherapy plays a role in immune modulation and tumor microenvironment reprogramming rather than direct tumor killing. Although radiotherapy could promote antitumor immunity, including tumor antigen presentation and T cell recruitment, immune suppressive cells, including Tregs and MDSCs, are also activated. Therefore, radiotherapy combined with immunotherapy may promote the activity of favorable immune cells and elevate antitumor efficacies (164). Low dose radiotherapy (LDRT) triggered T cell infiltration in an IFN-dependent manner in ovarian cancer patients with immune-desert tumors when combined with immune checkpoint blockade (165). In a preclinical setting, radiation therapy combined with immunostimulatory CPMV elicited significant tumor retardation and increased TIL in the TME (166). Radiotherapy combined with immunotherapy in other types of cancers, including melanoma, lung cancer, and colon cancer, is under plenty of preclinical and clinical studies, providing a basis for application in ovarian cancer (164).
Ovarian cancer, especially epithelial ovarian cancer, is typically diagnosed at an advanced stage. Patients who experience a recurrence within six months after the end of platinum-based chemotherapy are characterized by poor prognosis, which needs a novel and effective treatment modality (167). Multi-immunotherapies are expected to prolong the survival and improve the prognosis, plenty of clinical trials are investigating their efficacy in ovarian cancer (Table 1). Immunotherapy could be strengthened through several points. Firstly, it is recommended that all women with newly diagnosed ovarian cancer should be offered genetic testing. Approximately 10%-20% of ovarian cancers are related to germline mutations. Besides, relatives of women with genetic mutations are recommended to have gene testing (168). In addition, several preclinical and early clinical data suggested that toll-like receptor 7 (TLR7) and TLR8 agonists could activate DCs, monocytes, macrophages, and fibroblasts. TLR7/8 agonists also promoted proinflammatory cytokines and chemokines secretion, including IL-6. Thus, activation of TLR7/8 may be a potential target (169). Moreover, RNA-associated therapy aroused researchers’ attention. Long non-coding RNAs (lncRNAs) are critical regulators in ovarian cancer occurrence and progression (170). RNA-binding proteins (RBPs), a class of endogenous proteins that bind to mRNA, regulate a series of pathological processes in ovarian cancer (171). Therefore, both lncRNAs and RBPs could be a potential therapeutic target (172–178). Non-coding RNA miR-146b simultaneously inhibited EGFR and IL6-STAT3 signal pathways, resulting in a more excellent suppression of ovarian cancer cell migration (179). Another non-coding RNA, HOTAIR, was overexpressed in ovarian cancer stem cells (OCSCs). Inhibition of HOTAIR and DNA methylation help eradicate OCSCs and block disease recurrence (180). In addition, several natural agents could target multiple signaling pathways. For instance, berberine was proved to target both EGFR and ErbB2. Berberine inhibited migration and invasion of ovarian cancer cells (181).
To conclude, multi-immunotherapies of ovarian cancer are far from fully elucidated. Future studies should focus on fully recognizing immunogenic characteristics, developing biomarkers, and selecting eligible patients. Multi-immunotherapy is supposed to combine immunotherapies rationally while minimizing toxicities.
XYH wrote the initial draft of manuscript. CB and XZ revised the manuscript. TY reviewed and approved content. All authors contributed to the article and approved the submitted version.
This research was funded by the National Natural Science Foundation of China, grant number No. 81821002; Sichuan Science and Technology Program, grant number 2021YJ0011.
Images were created with Biorender.com.
The authors declare that the research was conducted in the absence of any commercial or financial relationships that could be construed as a potential conflict of interest.
All claims expressed in this article are solely those of the authors and do not necessarily represent those of their affiliated organizations, or those of the publisher, the editors and the reviewers. Any product that may be evaluated in this article, or claim that may be made by its manufacturer, is not guaranteed or endorsed by the publisher.
1. Jiang Y, Wang C, Zhou S. Targeting tumor microenvironment in ovarian cancer: Premise and promise. Biochim Biophys Acta Rev Cancer (2020) 1873(2):188361. doi: 10.1016/j.bbcan.2020.188361
2. Rodriguez GM, Galpin KJC, McCloskey CW, Vanderhyden BC. The tumor microenvironment of epithelial ovarian cancer and its influence on response to immunotherapy. Cancers (Basel) (2018) 10(8):242. doi: 10.3390/cancers10080242
3. Walker C, Mojares E, Del Río Hernández A. Role of extracellular matrix in development and cancer progression. Int J Mol Sci (2018) 19(10):3028. doi: 10.3390/ijms19103028
4. Malik R, Lelkes PI, Cukierman E. Biomechanical and biochemical remodeling of stromal extracellular matrix in cancer. Trends Biotechnol (2015) 33(4):230–6. doi: 10.1016/j.tibtech.2015.01.004
5. Cox TR. The matrix in cancer. Nat Rev Cancer (2021) 21(4):217–38. doi: 10.1038/s41568-020-00329-7
6. Baci D, Bosi A, Gallazzi M, Rizzi M, Noonan DM, Poggi A, et al. The ovarian cancer tumor immune microenvironment (TIME) as target for therapy: A focus on innate immunity cells as therapeutic effectors. Int J Mol Sci (2020) 21(9):3125. doi: 10.3390/ijms21093125
7. Ghoneum A, Almousa S, Warren B, Abdulfattah AY, Shu J, Abouelfadl H, et al. Exploring the clinical value of tumor microenvironment in platinum-resistant ovarian cancer. Semin Cancer Biol (2021) 77:83–98. doi: 10.1016/j.semcancer.2020.12.024
8. Meric-Bernstam F, Larkin J, Tabernero J, Bonini C. Enhancing anti-tumour efficacy with immunotherapy combinations. Lancet (2021) 397(10278):1010–22. doi: 10.1016/S0140-6736(20)32598-8
9. Yang Y, Yang Y, Yang J, Zhao X, Wei X. Tumor microenvironment in ovarian cancer: Function and therapeutic strategy. Front Cell Dev Biol (2020) 8:758. doi: 10.3389/fcell.2020.00758
10. Liu YT, Sun ZJ. Turning cold tumors into hot tumors by improving T-cell infiltration. Theranostics (2021) 11(11):5365–86. doi: 10.7150/thno.58390
11. Galon J, Bruni D. Approaches to treat immune hot, altered and cold tumours with combination immunotherapies. Nat Rev Drug Discov (2019) 18(3):197–218. doi: 10.1038/s41573-018-0007-y
12. Plummer R. Perspective on the pipeline of drugs being developed with modulation of DNA damage as a target. Clin Cancer Res (2010) 16(18):4527–31. doi: 10.1158/1078-0432.CCR-10-0984
13. Brown JS, O'Carrigan B, Jackson SP, Yap TA. Targeting DNA repair in cancer: Beyond PARP inhibitors. Cancer Discov (2017) 7(1):20–37. doi: 10.1158/2159-8290.CD-16-0860
14. Gavande NS, VanderVere-Carozza PS, Hinshaw HD, Jalal SI, Sears CR, Pawelczak KS, et al. DNA Repair targeted therapy: The past or future of cancer treatment? Pharmacol Ther (2016) 160:65–83. doi: 10.1016/j.pharmthera.2016.02.003
15. LaFargue CJ, Dal Molin GZ, Sood AK, Coleman RL. Exploring and comparing adverse events between PARP inhibitors. Lancet Oncol (2019) 20(1):e15–28. doi: 10.1016/S1470-2045(18)30786-1
16. Kurnit KC, Avila M, Hinchcliff EM, Coleman RL, Westin SN. PARP inhibition in the ovarian cancer patient: Current approvals and future directions. Pharmacol Ther (2020) 213:107588. doi: 10.1016/j.pharmthera.2020.107588
17. Mirza MR, Coleman RL, González-Martín A, Moore KN, Colombo N, Ray-Coquard I, et al. The forefront of ovarian cancer therapy: update on PARP inhibitors. Ann Oncol (2020) 31(9):1148–59. doi: 10.1016/j.annonc.2020.06.004
18. Mirza MR, Monk BJ, Herrstedt J, Oza AM, Mahner S, Redondo A, et al. Niraparib maintenance therapy in platinum-sensitive, recurrent ovarian cancer. N Engl J Med (2016) 375(22):2154–64. doi: 10.1056/NEJMoa1611310
19. Coleman RL, Oza AM, Lorusso D, Aghajanian C, Oaknin A, Dean A, et al. Rucaparib maintenance treatment for recurrent ovarian carcinoma after response to platinum therapy (ARIEL3): a randomised, double-blind, placebo-controlled, phase 3 trial. Lancet (2017) 390(10106):1949–61. doi: 10.1016/S0140-6736(17)32440-6
20. Ledermann JA, Oza AM, Lorusso D, Aghajanian C, Oaknin A, Dean A, et al. Rucaparib for patients with platinum-sensitive, recurrent ovarian carcinoma (ARIEL3): post-progression outcomes and updated safety results from a randomised, placebo-controlled, phase 3 trial. Lancet Oncol (2020) 21(5):710–22. doi: 10.1016/S1470-2045(20)30061-9
21. Moore K, Colombo N, Scambia G, Kim BG, Oaknin A, Friedlander M, et al. Maintenance olaparib in patients with newly diagnosed advanced ovarian cancer. N Engl J Med (2018) 379(26):2495–505. doi: 10.1056/NEJMoa1810858
22. Frenel JS, Kim JW, Aryal N, Asher R, Berton D, Vidal L, et al. Efficacy of subsequent chemotherapy for patients with BRCA1/2-mutated recurrent epithelial ovarian cancer progressing on olaparib versus placebo maintenance: post-hoc analyses of the SOLO2/ENGOT ov-21 trial. Ann Oncol (2022) S0923-7534(22)01740-9. doi: 10.1016/j.annonc.2022.06.011
23. Burki TK. Veliparib for advanced ovarian cancer. Lancet Oncol (2019) 20(11):e616. doi: 10.1016/S1470-2045(19)30630-8
24. Monk BJ, Minion LE, Coleman RL. Anti-angiogenic agents in ovarian cancer: past, present, and future. Ann Oncol (2016) 27 Suppl 1(Suppl 1):i33–9. doi: 10.1093/annonc/mdw093
25. Nusrat O, Belotte J, Fletcher NM, Memaj I, Saed MG, Diamond MP, et al. The role of angiogenesis in the persistence of chemoresistance in epithelial ovarian cancer. Reprod Sci (2016) 23(11):1484–92. doi: 10.1177/1933719116645191
26. Singh N, Badrun D, Ghatage P. State of the art and up-and-coming angiogenesis inhibitors for ovarian cancer. Expert Opin Pharmacother (2020) 21(13):1579–90. doi: 10.1080/14656566.2020.1775813
27. Tentori L, Lacal PM, Muzi A, Dorio AS, Leonetti C, Scarsella M, et al. Poly(ADP-ribose) polymerase (PARP) inhibition or PARP-1 gene deletion reduces angiogenesis. Eur J Cancer (2007) 43(14):2124–33. doi: 10.1016/j.ejca.2007.07.010
28. Lim JJ, Yang K, Taylor-Harding B, Wiedemeyer WR, Buckanovich RJ. VEGFR3 inhibition chemosensitizes ovarian cancer stemlike cells through down-regulation of BRCA1 and BRCA2. Neoplasia (2014) 16(4):343–53.e1-2. doi: 10.1016/j.neo.2014.04.003
29. Bindra RS, Gibson SL, Meng A, Westermark U, Jasin M, Pierce AJ, et al. Hypoxia-induced down-regulation of BRCA1 expression by E2Fs. Cancer Res (2005) 65(24):11597–604. doi: 10.1158/0008-5472.CAN-05-2119
30. Arora S, Balasubramaniam S, Zhang H, Berman T, Narayan P, Suzman D, et al. FDA Approval summary: Olaparib monotherapy or in combination with bevacizumab for the maintenance treatment of patients with advanced ovarian cancer. Oncologist (2021) 26(1):e164–72. doi: 10.1002/onco.13551
31. Ray-Coquard I, Pautier P, Pignata S, Pérol D, González-Martín A, Berger R, et al. Olaparib plus bevacizumab as first-line maintenance in ovarian cancer. N Engl J Med (2019) 381(25):2416–28. doi: 10.1056/NEJMoa1911361
32. Bizzaro F, Fuso Nerini I, Taylor MA, Anastasia A, Russo M, Damia G, et al. VEGF pathway inhibition potentiates PARP inhibitor efficacy in ovarian cancer independent of BRCA status. J Hematol Oncol (2021) 14(1):186. doi: 10.1186/s13045-021-01196-x
33. Liu JF, Barry WT, Birrer M, Lee JM, Buckanovich RJ, Fleming GF, et al. Combination cediranib and olaparib versus olaparib alone for women with recurrent platinum-sensitive ovarian cancer: a randomised phase 2 study. Lancet Oncol (2014) 15(11):1207–14. doi: 10.1016/S1470-2045(14)70391-2
34. Liu JF, Brady MF, Matulonis UA, Miller A, Kohn EC, Swisher EM, et al. Olaparib with or without cediranib versus platinum-based chemotherapy in recurrent platinum-sensitive ovarian cancer (NRG-GY004): A randomized, open-label, phase III trial. J Clin Oncol (2022) 40(19):2138–47. doi: 10.1200/JCO.21.02011
35. Mirza MR, Åvall Lundqvist E, Birrer MJ, dePont Christensen R, Nyvang GB, Malander S, et al. Niraparib plus bevacizumab versus niraparib alone for platinum-sensitive recurrent ovarian cancer (NSGO-AVANOVA2/ENGOT-ov24): a randomised, phase 2, superiority trial. Lancet Oncol (2019) 20(10):1409–19. doi: 10.1016/S1470-2045(19)30515-7
36. Konstantinopoulos PA, Waggoner S, Vidal GA, Mita M, Moroney JW, Holloway R, et al. Single-arm phases 1 and 2 trial of niraparib in combination with pembrolizumab in patients with recurrent platinum-resistant ovarian carcinoma. JAMA Oncol (2019) 5(8):1141–9. doi: 10.1001/jamaoncol.2019.1048
37. Alvarez Secord A, O'Malley DM, Sood AK, Westin SN, Liu JF. Rationale for combination PARP inhibitor and antiangiogenic treatment in advanced epithelial ovarian cancer: A review. Gynecol Oncol (2021) 162(2):482–95. doi: 10.1016/j.ygyno.2021.05.018
38. Lee EK, Konstantinopoulos PA. Combined PARP and immune checkpoint inhibition in ovarian cancer. Trends Cancer (2019) 5(9):524–8. doi: 10.1016/j.trecan.2019.06.004
39. Wang Z, Sun K, Xiao Y, Feng B, Mikule K, Ma X, et al. Niraparib activates interferon signaling and potentiates anti-PD-1 antibody efficacy in tumor models. Sci Rep (2019) 9(1):1853. doi: 10.1038/s41598-019-38534-6
40. Shen J, Zhao W, Ju Z, Wang L, Peng Y, Labrie M, et al. PARPi triggers the STING-dependent immune response and enhances the therapeutic efficacy of immune checkpoint blockade independent of BRCAness. Cancer Res (2019) 79(2):311–9. doi: 10.1158/0008-5472.CAN-18-1003
41. Lampert EJ, Zimmer A, Padget M, Cimino-Mathews A, Nair JR, Liu Y, et al. Combination of PARP inhibitor olaparib, and PD-L1 inhibitor durvalumab, in recurrent ovarian cancer: a proof-of-Concept phase II study. Clin Cancer Res (2020) 26(16):4268–79. doi: 10.1158/1078-0432.CCR-20-0056
42. Wang D, Li C, Zhang Y, Wang M, Jiang N, Xiang L, et al. Combined inhibition of PI3K and PARP is effective in the treatment of ovarian cancer cells with wild-type PIK3CA genes. Gynecol Oncol (2016) 142(3):548–56. doi: 10.1016/j.ygyno.2016.07.092
43. Konstantinopoulos PA, Barry WT, Birrer M, Westin SN, Cadoo KA, Shapiro GI, et al. Olaparib and α-specific PI3K inhibitor alpelisib for patients with epithelial ovarian cancer: a dose-escalation and dose-expansion phase 1b trial. Lancet Oncol (2019) 20(4):570–80. doi: 10.1016/S1470-2045(18)30905-7
44. Westin SN, Labrie M, Litton JK, Blucher A, Fang Y, Vellano CP, et al. Phase ib dose expansion and translational analyses of olaparib in combination with capivasertib in recurrent endometrial, triple-negative breast, and ovarian cancer. Clin Cancer Res (2021) 27(23):6354–65. doi: 10.1158/1078-0432.CCR-21-1656
45. Kim H, Xu H, George E, Hallberg D, Kumar S, Jagannathan V, et al. Combining PARP with ATR inhibition overcomes PARP inhibitor and platinum resistance in ovarian cancer models. Nat Commun (2020) 11(1):3726. doi: 10.1038/s41467-020-17127-2
46. Biegała Ł, Gajek A, Marczak A, Rogalska A. PARP inhibitor resistance in ovarian cancer: Underlying mechanisms and therapeutic approaches targeting the ATR/CHK1 pathway. Biochim Biophys Acta Rev Cancer (2021) 1876(2):188633. doi: 10.1016/j.bbcan.2021.188633
47. Gabbasov R, Benrubi ID, O'Brien SW, Krais JJ, Johnson N, Litwin S, et al. Targeted blockade of HSP90 impairs DNA-damage response proteins and increases the sensitivity of ovarian carcinoma cells to PARP inhibition. Cancer Biol Ther (2019) 20(7):1035–45. doi: 10.1080/15384047.2019.1595279
48. Konstantinopoulos PA, Cheng SC, Supko JG, Polak M, Wahner-Hendrickson AE, Ivy SP, et al. Combined PARP and HSP90 inhibition: preclinical and phase 1 evaluation in patients with advanced solid tumours. Br J Cancer (2022) 126(7):1027–36. doi: 10.1038/s41416-021-01664-8
49. Do KT, Kochupurakkal B, Kelland S, de Jonge A, Hedglin J, Powers A, et al. Phase 1 combination study of the CHK1 inhibitor prexasertib and the PARP inhibitor olaparib in high-grade serous ovarian cancer and other solid tumors. Clin Cancer Res (2021) 27(17):4710–6. doi: 10.1158/1078-0432.CCR-21-1279
50. Higuchi T, Flies DB, Marjon NA, Mantia-Smaldone G, Ronner L, Gimotty PA, et al. CTLA-4 blockade synergizes therapeutically with PARP inhibition in BRCA1-deficient ovarian cancer. Cancer Immunol Res (2015) 3(11):1257–68. doi: 10.1158/2326-6066.CIR-15-0044
51. Lu Z, Mao W, Yang H, Santiago-O'Farrill JM, Rask PJ, Mondal J, et al. SIK2 inhibition enhances PARP inhibitor activity synergistically in ovarian and triple-negative breast cancers. J Clin Invest (2022) 132(11):e146471. doi: 10.1172/JCI146471
52. Beauchamp MC, Knafo A, Yasmeen A, Carboni JM, Gottardis MM, Pollak MN, et al. BMS-536924 sensitizes human epithelial ovarian cancer cells to the PARP inhibitor, 3-aminobenzamide. Gynecol Oncol (2009) 115(2):193–8. doi: 10.1016/j.ygyno.2009.07.009
53. Wang H, Zhang S, Song L, Qu M, Zou Z. Synergistic lethality between PARP-trapping and alantolactone-induced oxidative DNA damage in homologous recombination-proficient cancer cells. Oncogene (2020) 39(14):2905–20. doi: 10.1038/s41388-020-1191-x
54. Moreno V, Hernandez T, de Miguel M, Doger B, Calvo E. Adoptive cell therapy for solid tumors: Chimeric antigen receptor T cells and beyond. Curr Opin Pharmacol (2021) 59:70–84. doi: 10.1016/j.coph.2021.05.004
55. Rodriguez-Garcia A, Sharma P, Poussin M, Boesteanu AC, Minutolo NG, Gitto SB, et al. CAR T cells targeting MISIIR for the treatment of ovarian cancer and other gynecologic malignancies. Mol Ther (2020) 28(2):548–60. doi: 10.1016/j.ymthe.2019.11.028
56. Du H, Hirabayashi K, Ahn S, Kren NP, Montgomery SA, Wang X, et al. Antitumor responses in the absence of toxicity in solid tumors by targeting B7-H3 via chimeric antigen receptor T cells. Cancer Cell (2019) 35(2):221–237.e8. doi: 10.1016/j.ccell.2019.01.002
57. Fu J, Shang Y, Qian Z, Hou J, Yan F, Liu G, et al. Chimeric antigen receptor-T (CAR-T) cells targeting epithelial cell adhesion molecule (EpCAM) can inhibit tumor growth in ovarian cancer mouse model. J Vet Med Sci (2021) 83(2):241–7. doi: 10.1292/jvms.20-0455
58. Jin L, Tao H, Karachi A, Long Y, Hou AY, Na M, et al. CXCR1- or CXCR2-modified CAR T cells co-opt IL-8 for maximal antitumor efficacy in solid tumors. Nat Commun (2019) 10(1):4016. doi: 10.1038/s41467-019-11869-4
59. Owens GL, Sheard VE, Kalaitsidou M, Blount D, Lad Y, Cheadle EJ, et al. Preclinical assessment of CAR T-cell therapy targeting the tumor antigen 5T4 in ovarian cancer. J Immunother (2018) 41(3):130–40. doi: 10.1097/CJI.0000000000000203
60. Wu JWY, Dand S, Doig L, Papenfuss AT, Scott CL, Ho G, et al. T-Cell receptor therapy in the treatment of ovarian cancer: A mini review. Front Immunol (2021) 12:672502. doi: 10.3389/fimmu.2021.672502
61. Ao X, Yang Y, Li W, Tan Y, Guo W, Ao L, et al. Anti-αFR CAR-engineered NK-92 cells display potent cytotoxicity against αFR-positive ovarian cancer. J Immunother (2019) 42(8):284–96. doi: 10.1097/CJI.0000000000000286
62. Ueda T, Kumagai A, Iriguchi S, Yasui Y, Miyasaka T, Nakagoshi K, et al. Non-clinical efficacy, safety and stable clinical cell processing of induced pluripotent stem cell-derived anti-glypican-3 chimeric antigen receptor-expressing natural killer/innate lymphoid cells. Cancer Sci (2020) 111(5):1478–90. doi: 10.1111/cas.14374
63. Jan CI, Huang SW, Canoll P, Bruce JN, Lin YC, Pan CM, et al. Targeting human leukocyte antigen G with chimeric antigen receptors of natural killer cells convert immunosuppression to ablate solid tumors. J Immunother Cancer (2021) 9(10):e003050. doi: 10.1136/jitc-2021-003050
64. Klapdor R, Wang S, Morgan MA, Zimmermann K, Hachenberg J, Büning H, et al. NK cell-mediated eradication of ovarian cancer cells with a novel chimeric antigen receptor directed against CD44. Biomedicines (2021) 9(10):1339. doi: 10.3390/biomedicines9101339
65. Klapdor R, Wang S, Morgan M, Dörk T, Hacker U, Hillemanns P, et al. Characterization of a novel third-generation anti-CD24-CAR against ovarian cancer. Int J Mol Sci (2019) 20(3):660. doi: 10.3390/ijms20030660
66. Klapdor R, Wang S, Hacker U, Büning H, Morgan M, Dörk T, et al. Improved killing of ovarian cancer stem cells by combining a novel chimeric antigen receptor-based immunotherapy and chemotherapy. Hum Gene Ther (2017) 28(10):886–96. doi: 10.1089/hum.2017.168
67. Cao B, Liu M, Wang L, Liang B, Feng Y, Chen X, et al. Use of chimeric antigen receptor NK-92 cells to target mesothelin in ovarian cancer. Biochem Biophys Res Commun (2020) 524(1):96–102. doi: 10.1016/j.bbrc.2020.01.053
68. Liang Z, Dong J, Yang N, Li SD, Yang ZY, Huang R, et al. Tandem CAR-T cells targeting FOLR1 and MSLN enhance the antitumor effects in ovarian cancer. Int J Biol Sci (2021) 17(15):4365–76. doi: 10.7150/ijbs.63181
69. Zhang Y, Wang P, Wang T, Fang Y, Ding Y, Qian Q. Chimeric antigen receptor T cells engineered to secrete CD40 agonist antibodies enhance antitumor efficacy. J Transl Med (2021) 19(1):82. doi: 10.1186/s12967-021-02750-4
70. Shu R, Evtimov VJ, Hammett MV, Nguyen NN, Zhuang J, Hudson PJ, et al. Engineered CAR-T cells targeting TAG-72 and CD47 in ovarian cancer. Mol Ther Oncolytics (2021) 20:325–41. doi: 10.1016/j.omto.2021.01.002
71. Li T, Wang J. Therapeutic effect of dual CAR-T targeting PDL1 and MUC16 antigens on ovarian cancer cells in mice. BMC Cancer (2020) 20(1):678. doi: 10.1186/s12885-020-07180-x
72. Jiang G, Ng YY, Tay JCK, Du Z, Xiao L, Wang S, et al. Dual CAR-T cells to treat cancers co-expressing NKG2D and PD1 ligands in xenograft models of peritoneal metastasis. Cancer Immunol Immunother (2022) Online ahead of print. doi: 10.1007/s00262-022-03247-9
73. Fang J, Ding N, Guo X, Sun Y, Zhang Z, Xie B, et al. αPD-1-mesoCAR-T cells partially inhibit the growth of advanced/refractory ovarian cancer in a patient along with daily apatinib. J Immunother Cancer (2021) 9(2):e001162. doi: 10.1136/jitc-2020-001162
74. Grosser R, Cherkassky L, Chintala N, Adusumilli PS. Combination immunotherapy with CAR T cells and checkpoint blockade for the treatment of solid tumors. Cancer Cell (2019) 36(5):471–82. doi: 10.1016/j.ccell.2019.09.006
75. Thakur A, Scholler J, Schalk DL, June CH, Lum LG, et al. Enhanced cytotoxicity against solid tumors by bispecific antibody-armed CD19 CAR T cells: a proof-of-concept study. J Cancer Res Clin Oncol (2020) 146(8):2007–16. doi: 10.1007/s00432-020-03260-4
76. Koneru M, Purdon TJ, Spriggs D, Koneru S, Brentjens RJ. IL-12 secreting tumor-targeted chimeric antigen receptor T cells eradicate ovarian tumors in vivo. Oncoimmunology (2015) 4(3):e994446. doi: 10.4161/2162402X.2014.994446
77. Song DG, Ye Q, Santoro S, Fang C, Best A, Powell DJ, et al. Chimeric NKG2D CAR-expressing T cell-mediated attack of human ovarian cancer is enhanced by histone deacetylase inhibition. Hum Gene Ther (2013) 24(3):295–305. doi: 10.1089/hum.2012.143
78. Whilding LM, Halim L, Draper B, Parente-Pereira AC, Zabinski T, Davies DM, et al. CAR T-cells targeting the integrin αvβ6 and Co-expressing the chemokine receptor CXCR2 demonstrate enhanced homing and efficacy against several solid malignancies. Cancers (Basel) (2019) 11(5):674. doi: 10.3390/cancers11050674
79. Liu G, Zhang Q, Liu G, Li D, Zhang L, Gu Z, et al. Disruption of adenosine 2A receptor improves the anti-tumor function of anti-mesothelin CAR T cells both in vitro and in vivo. Exp Cell Res (2021) 409(1):112886. doi: 10.1016/j.yexcr.2021.112886
80. Deng C, Zhao J, Zhou S, Dong J, Cao J, Gao J, et al. The vascular disrupting agent CA4P improves the antitumor efficacy of CAR-T cells in preclinical models of solid human tumors. Mol Ther (2020) 28(1):75–88. doi: 10.1016/j.ymthe.2019.10.010
81. Qu Y, Dunn ZS, Chen X, MacMullan M, Cinay G, Wang HY, et al. Adenosine deaminase 1 overexpression enhances the antitumor efficacy of chimeric antigen receptor-engineered T cells. Hum Gene Ther (2022) 33(5-6):223–36. doi: 10.1089/hum.2021.050
82. Xie G, Dong H, Liang Y, Ham JD, Rizwan R, Chen J. CAR-NK cells: A promising cellular immunotherapy for cancer. EBioMedicine (2020) 59:102975. doi: 10.1016/j.ebiom.2020.102975
83. Ng YY, Tay JCK, Wang S. CXCR1 expression to improve anti-cancer efficacy of intravenously injected CAR-NK cells in mice with peritoneal xenografts. Mol Ther Oncolytics (2020) 16:75–85. doi: 10.1016/j.omto.2019.12.006
84. Chen Y, Yu Z, Tan X, Jiang H, Xu Z, Fang Y, et al. CAR-macrophage: A new immunotherapy candidate against solid tumors. BioMed Pharmacother (2021) 139:111605. doi: 10.1016/j.biopha.2021.111605
85. Pan K, Farrukh H, Chittepu V, Xu H, Pan CX, Zhu Z, et al. CAR race to cancer immunotherapy: from CAR T, CAR NK to CAR macrophage therapy. J Exp Clin Cancer Res (2022) 41(1):119. doi: 10.1186/s13046-022-02327-z
86. Adams SF, Grimm AJ, Chiang CL, Mookerjee A, Flies D, Jean S, et al. Rapid tumor vaccine using toll-like receptor-activated ovarian cancer ascites monocytes. J Immunother Cancer (2020) 8(2):e000875. doi: 10.1136/jitc-2020-000875
87. Kalli KR, Block MS, Kasi PM, Erskine CL, Hobday TJ, Dietz A, et al. Folate receptor alpha peptide vaccine generates immunity in breast and ovarian cancer patients. Clin Cancer Res (2018) 24(13):3014–25. doi: 10.1158/1078-0432.CCR-17-2499
88. Morisaki T, Hikichi T, Onishi H, Morisaki T, Kubo M, Hirano T, et al. Intranodal administration of neoantigen peptide-loaded dendritic cell vaccine elicits epitope-specific T cell responses and clinical effects in a patient with chemorefractory ovarian cancer with malignant ascites. Immunol Invest (2021) 50(5):562–79. doi: 10.1080/08820139.2020.1778721
89. Cecil DL, Liao JB, Dang Y, Coveler AL, Kask A, Yang Y, et al. Immunization with a plasmid DNA vaccine encoding the n-terminus of insulin-like growth factor binding protein-2 in advanced ovarian cancer leads to high-level type I immune responses. Clin Cancer Res (2021) 27(23):6405–12. doi: 10.1158/1078-0432.CCR-21-1579
90. Wu D, Yu X, Wang J, Hui X, Zhang Y, Cai Y, et al. Ovarian cancer stem cells with high ROR1 expression serve as a new prophylactic vaccine for ovarian cancer. J Immunol Res (2019) 2019:9394615. doi: 10.1155/2019/9394615
91. Fucikova J, Hensler M, Kasikova L, Lanickova T, Pasulka J, Rakova J, et al. An autologous dendritic cell vaccine promotes anticancer immunity in patients with ovarian cancer with low mutational burden and cold tumors. Clin Cancer Res (2022) 28(14):3053–65. doi: 10.1158/1078-0432.CCR-21-4413
92. Sinnathamby G, Lauer P, Zerfass J, Hanson B, Karabudak A, Krakover J, et al. Priming and activation of human ovarian and breast cancer-specific CD8+ T cells by polyvalent listeria monocytogenes-based vaccines. J Immunother (2009) 32(8):856–69. doi: 10.1097/CJI.0b013e3181b0b125
93. Tawde SA, Chablani L, Akalkotkar A, D'Souza MJ. Evaluation of microparticulate ovarian cancer vaccine via transdermal route of delivery. J Control Release (2016) 235:147–54. doi: 10.1016/j.jconrel.2016.05.058
94. Chang MC, Chen YL, Chiang YC, Chen TC, Tang YC, Chen CA, et al. Mesothelin-specific cell-based vaccine generates antigen-specific immunity and potent antitumor effects by combining with IL-12 immunomodulator. Gene Ther (2016) 23(1):38–49. doi: 10.1038/gt.2015.85
95. Zamarin D, Walderich S, Holland A, Zhou Q, Iasonos AE, Torrisi JM, et al. Safety, immunogenicity, and clinical efficacy of durvalumab in combination with folate receptor alpha vaccine TPIV200 in patients with advanced ovarian cancer: a phase II trial. J Immunother Cancer (2020) 8(1):e000829. doi: 10.1136/jitc-2020-000829
96. Duraiswamy J, Kaluza KM, Freeman GJ, Coukos G. Dual blockade of PD-1 and CTLA-4 combined with tumor vaccine effectively restores T-cell rejection function in tumors. Cancer Res (2013) 73(12):3591–603. doi: 10.1158/0008-5472.CAN-12-4100
97. Stump CT, Ho G, Mao C, Veliz FA, Beiss V, Fields J, et al. Remission-stage ovarian cancer cell vaccine with cowpea mosaic virus adjuvant prevents tumor growth. Cancers (Basel) (2021) 13(4):627. doi: 10.3390/cancers13040627
98. Kahn RM, Ragupathi G, Zhou QC, Iasonos A, Kravetz S, Hensley ML, et al. Long-term outcomes of patients with recurrent ovarian cancer treated with a polyvalent vaccine with bevacizumab combination. Cancer Immunol Immunother (2022) Online ahead of print. doi: 10.1007/s00262-022-03225-1
99. Rocconi RP, Grosen EA, Ghamande SA, Chan JK, Barve MA, Oh J, et al. Gemogenovatucel-T (Vigil) immunotherapy as maintenance in frontline stage III/IV ovarian cancer (VITAL): a randomised, double-blind, placebo-controlled, phase 2b trial. Lancet Oncol (2020) 21(12):1661–72. doi: 10.1016/S1470-2045(20)30533-7
100. Rocconi RP, Stevens EE, Bottsford-Miller JN, Ghamande SA, Elder J, DeMars LL, et al. Proof of principle study of sequential combination atezolizumab and vigil in relapsed ovarian cancer. Cancer Gene Ther (2022) 29(3-4):369–82. doi: 10.1038/s41417-021-00317-5
101. Kandalaft LE, Powell DJ, Jr. , Chiang CL, Tanyi J, Kim S, et al. Autologous lysate-pulsed dendritic cell vaccination followed by adoptive transfer of vaccine-primed ex vivo co-stimulated T cells in recurrent ovarian cancer. Oncoimmunology (2013) 2(1):e22664. doi: 10.4161/onci.22664
102. Nakashima H, Miyake K, Clark CR, Bekisz J, Finbloom J, Husain SR, et al. Potent antitumor effects of combination therapy with IFNs and monocytes in mouse models of established human ovarian and melanoma tumors. Cancer Immunol Immunother (2012) 61(7):1081–92. doi: 10.1007/s00262-011-1152-x
103. Chen YL, Chang MC, Chiang YC, Lin HW, Sun NY, Chen CA, et al. Immuno-modulators enhance antigen-specific immunity and anti-tumor effects of mesothelin-specific chimeric DNA vaccine through promoting DC maturation. Cancer Lett (2018) 425:152–63. doi: 10.1016/j.canlet.2018.03.032
104. Wang C, Steinmetz NF. CD47 blockade and cowpea mosaic virus nanoparticle in situ vaccination triggers phagocytosis and tumor killing. Adv Healthc Mater (2019) 8(8):e1801288. doi: 10.1002/adhm.201801288
105. Hu X, Zhou W, Pi R, Zhao X, Wang W. Genetically modified cancer vaccines: Current status and future prospects. Med Res Rev (2022) 42(4):1492–517. doi: 10.1002/med.21882
106. Wan C, Keany MP, Dong H, Al-Alem LF, Pandya UM, Lazo S, et al. Enhanced efficacy of simultaneous PD-1 and PD-L1 immune checkpoint blockade in high-grade serous ovarian cancer. Cancer Res (2021) 81(1):158–73. doi: 10.1158/0008-5472.CAN-20-1674
107. Miao YR, Thakkar KN, Qian J, Kariolis MS, Huang W, Nandagopal S, et al. Neutralization of PD-L2 is essential for overcoming immune checkpoint blockade resistance in ovarian cancer. Clin Cancer Res (2021) 27(15):4435–48. doi: 10.1158/1078-0432.CCR-20-0482
108. Taylan E, Zayou F, Murali R, Karlan BY, Pandol SJ, Edderkaoui M, et al. Dual targeting of GSK3B and HDACs reduces tumor growth and improves survival in an ovarian cancer mouse model. Gynecol Oncol (2020) 159(1):277–84. doi: 10.1016/j.ygyno.2020.07.005
109. Camblin AJ, Tan G, Curley MD, Yannatos I, Iadevaia S, Rimkunas V, et al. Dual targeting of IGF-1R and ErbB3 as a potential therapeutic regimen for ovarian cancer. Sci Rep (2019) 9(1):16832. doi: 10.1038/s41598-019-53322-y
110. Previs RA, Armaiz-Pena GN, Ivan C, Dalton HJ, Rupaimoole R, Hansen JM, et al. Role of YAP1 as a marker of sensitivity to dual AKT and P70S6K inhibition in ovarian and uterine malignancies. J Natl Cancer Inst (2017) 109(7):djw296. doi: 10.1093/jnci/djw296
111. Spiliopoulou P, Spear S, Mirza H, Garner I, McGarry L, Grundland-Freile F, et al. Dual G9A/EZH2 inhibition stimulates antitumor immune response in ovarian high-grade serous carcinoma. Mol Cancer Ther (2022) 21(4):522–34. doi: 10.1158/1535-7163.MCT-21-0743
112. Gartung A, Yang J, Sukhatme VP, Bielenberg DR, Fernandes D, Chang J, et al. Suppression of chemotherapy-induced cytokine/lipid mediator surge and ovarian cancer by a dual COX-2/sEH inhibitor. Proc Natl Acad Sci USA (2019) 116(5):1698–703. doi: 10.1073/pnas.1803999116
113. Zeng Y, Li B, Liang Y, Reeves PM, Qu X, Ran C, et al. Dual blockade of CXCL12-CXCR4 and PD-1-PD-L1 pathways prolongs survival of ovarian tumor-bearing mice by prevention of immunosuppression in the tumor microenvironment. FASEB J (2019) 33(5):6596–608. doi: 10.1096/fj.201802067RR
114. Duraiswamy J, Freeman G, Coukos G. Replenish the source within: Rescuing tumor-infiltrating lymphocytes by double checkpoint blockade. Oncoimmunology (2013) 2(10):e25912. doi: 10.4161/onci.25912
115. Zamarin D, Burger RA, Sill MW, Powell DJ, Jr. , Lankes HA, et al. Randomized phase II trial of nivolumab versus nivolumab and ipilimumab for recurrent or persistent ovarian cancer: An NRG oncology study. J Clin Oncol (2020) 38(16):1814–23. doi: 10.1200/JCO.19.02059
116. Do KT, Manuszak C, Thrash E, Giobbie-Hurder A, Hu J, Kelland S, et al. Immune modulating activity of the CHK1 inhibitor prexasertib and anti-PD-L1 antibody LY3300054 in patients with high-grade serous ovarian cancer and other solid tumors. Cancer Immunol Immunother (2021) 70(10):2991–3000. doi: 10.1007/s00262-021-02910-x
117. Moroney JW, Powderly J, Lieu CH, Bendell JC, Eckhardt SG, Chang CW, et al. Safety and clinical activity of atezolizumab plus bevacizumab in patients with ovarian cancer: A phase ib study. Clin Cancer Res (2020) 26(21):5631–7. doi: 10.1158/1078-0432.CCR-20-0477
118. Yang M, Lu J, Zhang G, Wang Y, He M, Xu Q, et al. CXCL13 shapes immunoactive tumor microenvironment and enhances the efficacy of PD-1 checkpoint blockade in high-grade serous ovarian cancer. J Immunother Cancer (2021) 9(1):e001136. doi: 10.1136/jitc-2020-001136
119. Zhang QF, Li J, Jiang K, Wang R, Ge JL, Yang H, et al. CDK4/6 inhibition promotes immune infiltration in ovarian cancer and synergizes with PD-1 blockade in a b cell-dependent manner. Theranostics (2020) 10(23):10619–33. doi: 10.7150/thno.44871
120. Simpkins F, Hevia-Paez P, Sun J, Ullmer W, Gilbert CA, da Silva T, et al. Src inhibition with saracatinib reverses fulvestrant resistance in ER-positive ovarian cancer models in vitro and in vivo. Clin Cancer Res (2012) 18(21):5911–23. doi: 10.1158/1078-0432.CCR-12-1257
121. Li L, Li X, Han X, Yang T, Fu J, Zhang Y, et al. An ovarian cancer model with positive ER: Reversion of ER antagonist resistance by src blockade. Oncol Rep (2014) 32(3):943–50. doi: 10.3892/or.2014.3284
122. Hew KE, Miller PC, El-Ashry D, Sun J, Besser AH, Ince TA, et al. MAPK activation predicts poor outcome and the MEK inhibitor, selumetinib, reverses antiestrogen resistance in ER-positive high-grade serous ovarian cancer. Clin Cancer Res (2016) 22(4):935–47. doi: 10.1158/1078-0432.CCR-15-0534
123. Wen W, Wu J, Liu L, Tian Y, Buettner R, Hsieh MY, et al. Synergistic anti-tumor effect of combined inhibition of EGFR and JAK/STAT3 pathways in human ovarian cancer. Mol Cancer (2015) 14:100. doi: 10.1186/s12943-015-0366-5
124. Orr B, Mahdi H, Fang Y, Strange M, Uygun I, Rana M, et al. Phase I trial combining chemokine-targeting with loco-regional chemoimmunotherapy for recurrent, platinum-sensitive ovarian cancer shows induction of CXCR3 ligands and markers of type 1 immunity. Clin Cancer Res (2022) 28(10):2038–49. doi: 10.1158/1078-0432.CCR-21-3659
125. Shao M, Hollar S, Chambliss D, Schmitt J, Emerson R, Chelladurai B, et al. Targeting the insulin growth factor and the vascular endothelial growth factor pathways in ovarian cancer. Mol Cancer Ther (2012) 11(7):1576–86. doi: 10.1158/1535-7163.MCT-11-0961
126. Huang J, Hu W, Hu L, Previs RA, Dalton HJ, Yang XY, et al. Dll4 inhibition plus aflibercept markedly reduces ovarian tumor growth. Mol Cancer Ther (2016) 15(6):1344–52. doi: 10.1158/1535-7163.MCT-15-0144
127. Guo T, Gu C, Li B, Xu C. Dual inhibition of FGFR4 and BCL-xL inhibits multi-resistant ovarian cancer with BCL2L1 gain. Aging (Albany NY) (2021) 13(15):19750–9. doi: 10.18632/aging.203386
128. Lee DW, Lee W, Kwon M, Lee HN. Dual inhibition of FOXM1 and its compensatory signaling pathway decreased the survival of ovarian cancer cells. Oncol Rep (2021) 45(1):390–400. doi: 10.3892/or.2020.7845
129. Simpkins F, Jang K, Yoon H, Hew KE, Kim M, Azzam DJ, et al. Dual src and MEK inhibition decreases ovarian cancer growth and targets tumor initiating stem-like cells. Clin Cancer Res (2018) 24(19):4874–86. doi: 10.1158/1078-0432.CCR-17-3697
130. Liu M, Thomas SL, DeWitt AK, Zhou W, Madaj ZB, Ohtani H, et al. Dual inhibition of DNA and histone methyltransferases increases viral mimicry in ovarian cancer cells. Cancer Res (2018) 78(20):5754–66. doi: 10.1158/0008-5472.CAN-17-3953
131. Iavarone C, Zervantonakis IK, Selfors LM, Palakurthi S, Liu JF, Drapkin R, et al. Combined MEK and BCL-2/X(L) inhibition is effective in high-grade serous ovarian cancer patient-derived xenograft models and BIM levels are predictive of responsiveness. Mol Cancer Ther (2019) 18(3):642–55. doi: 10.1158/1535-7163.MCT-18-0413
132. Sheppard KE, Cullinane C, Hannan KM, Wall M, Chan J, Barber F, et al. Synergistic inhibition of ovarian cancer cell growth by combining selective PI3K/mTOR and RAS/ERK pathway inhibitors. Eur J Cancer (2013) 49(18):3936–44. doi: 10.1016/j.ejca.2013.08.007
133. Sun L, Yin Y, Clark LH, Sun W, Sullivan SA, Tran AQ, et al. Dual inhibition of glycolysis and glutaminolysis as a therapeutic strategy in the treatment of ovarian cancer. Oncotarget (2017) 8(38):63551–61. doi: 10.18632/oncotarget.18854
134. Wang K, Zhu C, He Y, Zhang Z, Zhou W, Muhammad N, et al. Restraining cancer cells by dual metabolic inhibition with a mitochondrion-targeted Platinum(II) complex. Angew Chem Int Ed Engl (2019) 58(14):4638–43. doi: 10.1002/anie.201900387
135. Makii C, Ikeda Y, Oda K, Uehara Y, Nishijima A, Koso T, et al. Anti-tumor activity of dual inhibition of phosphatidylinositol 3-kinase and MDM2 against clear cell ovarian carcinoma. Gynecol Oncol (2019) 155(2):331–9. doi: 10.1016/j.ygyno.2019.08.028
136. Lamichhane P, Karyampudi L, Shreeder B, Krempski J, Bahr D, Daum J, et al. IL10 release upon PD-1 blockade sustains immunosuppression in ovarian cancer. Cancer Res (2017) 77(23):6667–78. doi: 10.1158/0008-5472.CAN-17-0740
137. Kim PS, Jochems C, Grenga I, Donahue RN, Tsang KY, Gulley JL, et al. Pan-Bcl-2 inhibitor, GX15-070 (obatoclax), decreases human T regulatory lymphocytes while preserving effector T lymphocytes: a rationale for its use in combination immunotherapy. J Immunol (2014) 192(6):2622–33. doi: 10.4049/jimmunol.1301369
138. Fukuhara H, Ino Y, Todo T. Oncolytic virus therapy: A new era of cancer treatment at dawn. Cancer Sci (2016) 107(10):1373–9. doi: 10.1111/cas.13027
139. Mondal M, Guo J, He P, Zhou D. Recent advances of oncolytic virus in cancer therapy. Hum Vaccin Immunother (2020) 16(10):2389–402. doi: 10.1080/21645515.2020.1723363
140. McGray AJR, Huang RY, Battaglia S, Eppolito C, Miliotto A, Stephenson KB, et al. Oncolytic maraba virus armed with tumor antigen boosts vaccine priming and reveals diverse therapeutic response patterns when combined with checkpoint blockade in ovarian cancer. J Immunother Cancer (2019) 7(1):189. doi: 10.1186/s40425-019-0641-x
141. Gautam A, Beiss V, Wang C, Wang L, Steinmetz NF. Plant viral nanoparticle conjugated with anti-PD-1 peptide for ovarian cancer immunotherapy. Int J Mol Sci (2021) 22(18):9733. doi: 10.3390/ijms22189733
142. Liu Z, Ravindranathan R, Kalinski P, Guo ZS, Bartlett DL. Rational combination of oncolytic vaccinia virus and PD-L1 blockade works synergistically to enhance therapeutic efficacy. Nat Commun (2017) 8:14754. doi: 10.1038/ncomms14754
143. Hardwick NR, Frankel P, Ruel C, Kilpatrick J, Tsai W, Kos F, et al. p53-reactive T cells are associated with clinical benefit in patients with platinum-resistant epithelial ovarian cancer after treatment with a p53 vaccine and gemcitabine chemotherapy. Clin Cancer Res (2018) 24(6):1315–25. doi: 10.1158/1078-0432.CCR-17-2709
144. Kowalsky SJ, Liu Z, Feist M, Berkey SE, Ma C, Ravindranathan R, et al. Superagonist IL-15-Armed oncolytic virus elicits potent antitumor immunity and therapy that are enhanced with PD-1 blockade. Mol Ther (2018) 26(10):2476–86. doi: 10.1016/j.ymthe.2018.07.013
145. Matuszewska K, Santry LA, van Vloten JP, AuYeung AWK, Major PP, Lawler J, et al. Combining vascular normalization with an oncolytic virus enhances immunotherapy in a preclinical model of advanced-stage ovarian cancer. Clin Cancer Res (2019) 25(5):1624–38. doi: 10.1158/1078-0432.CCR-18-0220
146. Lee S, Yang W, Kim DK, Kim H, Shin M, Choi KU, et al. Inhibition of MEK-ERK pathway enhances oncolytic vaccinia virus replication in doxorubicin-resistant ovarian cancer. Mol Ther Oncolytics (2022) 25:211–24. doi: 10.1016/j.omto.2022.04.006
147. Arulanandam R, Taha Z, Garcia V, Selman M, Chen A, Varette O, et al. The strategic combination of trastuzumab emtansine with oncolytic rhabdoviruses leads to therapeutic synergy. Commun Biol (2020) 3(1):254. doi: 10.1038/s42003-020-0972-7
148. Liu C, Erlichman C, McDonald CJ, Ingle JN, Zollman P, Iankov I, et al. Heat shock protein inhibitors increase the efficacy of measles virotherapy. Gene Ther (2008) 15(14):1024–34. doi: 10.1038/gt.2008.30
149. Dold C, Rodriguez Urbiola C, Wollmann G, Egerer L, Muik A, Bellmann L, et al. Application of interferon modulators to overcome partial resistance of human ovarian cancers to VSV-GP oncolytic viral therapy. Mol Ther Oncolytics (2016) 3:16021. doi: 10.1038/mto.2016.21
150. Zhang YQ, Tsai YC, Monie A, Wu TC, Hung CF. Enhancing the therapeutic effect against ovarian cancer through a combination of viral oncolysis and antigen-specific immunotherapy. Mol Ther (2010) 18(4):692–9. doi: 10.1038/mt.2009.318
151. Chang CL, Ma B, Pang X, Wu TC, Hung CF. Treatment with cyclooxygenase-2 inhibitors enables repeated administration of vaccinia virus for control of ovarian cancer. Mol Ther (2009) 17(8):1365–72. doi: 10.1038/mt.2009.118
152. Tseng JC, Granot T, DiGiacomo V, Levin B, Meruelo D. Enhanced specific delivery and targeting of oncolytic sindbis viral vectors by modulating vascular leakiness in tumor. Cancer Gene Ther (2010) 17(4):244–55. doi: 10.1038/cgt.2009.70
153. Stegelmeier AA, Santry LA, Guilleman MM, Matuszewska K, Minott JA, Yates JGE, et al. AAV-vectored expression of the vascular normalizing agents 3TSR and Fc3TSR, and the anti-angiogenic bevacizumab extends survival in a murine model of end-stage epithelial ovarian carcinoma. Biomedicines (2022) 10(2):362. doi: 10.3390/biomedicines10020362
154. Browne A, Tookman LA, Ingemarsdotter CK, Bouwman RD, Pirlo K, Wang Y, et al. Pharmacological inhibition of β3 integrin reduces the inflammatory toxicities caused by oncolytic adenovirus without compromising anticancer activity. Cancer Res (2015) 75(14):2811–21. doi: 10.1158/0008-5472.CAN-14-3761
155. Park JY, Lee JY, Lee YY, Shim SH, Suh DH, Kim JW. Major clinical research advances in gynecologic cancer in 2021. J Gynecol Oncol (2022) 33(2):e43. doi: 10.3802/jgo.2022.33.e43
156. Javellana M, Eckert MA, Heide J, Zawieracz K, Weigert M, Ashley S, et al. Neoadjuvant chemotherapy induces genomic and transcriptomic changes in ovarian cancer. Cancer Res (2022) 82(1):169–76. doi: 10.1158/0008-5472.CAN-21-1467
157. Zhang K, Erkan EP, Jamalzadeh S, Dai J, Andersson N, Kaipio K, et al. Longitudinal single-cell RNA-seq analysis reveals stress-promoted chemoresistance in metastatic ovarian cancer. Sci Adv (2022) 8(8):eabm1831. doi: 10.1126/sciadv.abm1831
158. Ray U, Jung DB, Jin L, Xiao Y, Dasari S, Sarkar Bhattacharya S, et al. Targeting LRRC15 inhibits metastatic dissemination of ovarian cancer. Cancer Res (2022) 82(6):1038–54. doi: 10.1158/0008-5472.CAN-21-0622
159. Gershenson DM, Miller A, Brady WE, Paul J, Carty K, Rodgers W, et al. Trametinib versus standard of care in patients with recurrent low-grade serous ovarian cancer (GOG 281/LOGS): an international, randomised, open-label, multicentre, phase 2/3 trial. Lancet (2022) 399(10324):541–53. doi: 10.1016/S0140-6736(21)02175-9
160. Moore KN, Chambers SK, Hamilton EP, Chen LM, Oza AM, Ghamande SA, et al. Adavosertib with chemotherapy in patients with primary platinum-resistant ovarian, fallopian tube, or peritoneal cancer: An open-label, four-arm, phase II study. Clin Cancer Res (2022) 28(1):36–44. doi: 10.1158/1078-0432.CCR-21-0158
161. Coffman LG, Orellana TJ, Liu T, Frisbie LG, Normolle D, Griffith K, et al. Phase I trial of ribociclib with platinum chemotherapy in ovarian cancer. JCI Insight (2022) 7(18):e160573. doi: 10.1172/jci.insight.160573
162. Doddapaneni BS, Al-Fatease AM, Rao DA, Alani AWG. Dual-drug loaded micelle for combinatorial therapy targeting HIF and mTOR signaling pathways for ovarian cancer treatment. J Control Release (2019) 307:272–81. doi: 10.1016/j.jconrel.2019.06.036
163. Alhadad LJ, Harisa GI, Alanazi FK. Design and encapsulation of anticancer dual HSP27 and HER2 inhibitor into low density lipoprotein to target ovarian cancer cells. Saudi Pharm J (2020) 28(4):387–96. doi: 10.1016/j.jsps.2020.01.020
164. Herrera FG, Irving M, Kandalaft LE, Coukos G. Rational combinations of immunotherapy with radiotherapy in ovarian cancer. Lancet Oncol (2019) 20(8):e417–33. doi: 10.1016/S1470-2045(19)30401-2
165. Herrera FG, Ronet C, Ochoa de Olza M, Barras D, Crespo I, Andreatta M, et al. Low-dose radiotherapy reverses tumor immune desertification and resistance to immunotherapy. Cancer Discov (2022) 12(1):108–33. doi: 10.1158/2159-8290.CD-21-0003
166. Patel R, Czapar AE, Fiering S, Oleinick NL, Steinmetz NF. Radiation therapy combined with cowpea mosaic virus nanoparticle in situ vaccination initiates immune-mediated tumor regression. ACS Omega (2018) 3(4):3702–7. doi: 10.1021/acsomega.8b00227
167. Bogani G, Lopez S, Mantiero M, Ducceschi M, Bosio S, Ruisi S, et al. Immunotherapy for platinum-resistant ovarian cancer. Gynecol Oncol (2020) 158(2):484–8. doi: 10.1016/j.ygyno.2020.05.681
168. Kurnit KC, Fleming GF, Lengyel E. Updates and new options in advanced epithelial ovarian cancer treatment. Obstet Gynecol (2021) 137(1):108–21. doi: 10.1097/AOG.0000000000004173
169. Ray-Coquard I, Lorusso D. Immunotherapy and epithelial ovarian cancer: a double-edged sword? Ann Oncol (2017) 28(5):909–10. doi: 10.1093/annonc/mdx102
170. Wang JY, Lu AQ, Chen LJ. LncRNAs in ovarian cancer. Clin Chim Acta (2019) 490:17–27. doi: 10.1016/j.cca.2018.12.013
171. Wu J, Wu Y, Guo Q, Wang S, Wu X. RNA-Binding proteins in ovarian cancer: a novel avenue of their roles in diagnosis and treatment. J Transl Med (2022) 20(1):37. doi: 10.1186/s12967-022-03245-6
172. Wang J, Liu L. MiR-149-3p promotes the cisplatin resistance and EMT in ovarian cancer through downregulating TIMP2 and CDKN1A. J Ovarian Res (2021) 14(1):165. doi: 10.1186/s13048-021-00919-5
173. Xu H, Wang X, Zhang Y, Zheng W, Zhang H. GATA6-AS1 inhibits ovarian cancer cell proliferation and migratory and invasive abilities by sponging miR-19a-5p and upregulating TET2. Oncol Lett (2021) 22(4):718. doi: 10.3892/ol.2021.12979
174. Chen H, Liu Y, Liu P, Dai Q, Wang P. LINC01094 promotes the invasion of ovarian cancer cells and regulates the wnt/β-catenin signaling pathway by targeting miR-532-3p. Exp Ther Med (2021) 22(5):1228. doi: 10.3892/etm.2021.10662
175. Jiang R, Zhang H, Zhou J, Wang J, Xu Y, Zhang H, et al. Inhibition of long non-coding RNA XIST upregulates microRNA-149-3p to repress ovarian cancer cell progression. Cell Death Dis (2021) 12(2):145. doi: 10.1038/s41419-020-03358-0
176. Liu HR, Zhao J. Effect and mechanism of miR-217 on drug resistance, invasion and metastasis of ovarian cancer cells through a regulatory axis of CUL4B gene silencing/inhibited wnt/β-catenin signaling pathway activation. Eur Rev Med Pharmacol Sci (2021) 25(1):94–107. doi: 10.26355/eurrev_202101_24353
177. Zhu FJ, Li JZ, Wang LL. MicroRNA-1-3p inhibits the growth and metastasis of ovarian cancer cells by targeting DYNLT3. Eur Rev Med Pharmacol Sci (2020) 24(17):8713–21. doi: 10.26355/eurrev_202009_22808
178. Zuo Y, Zheng W, Tang Q, Liu J, Wang S, Xin C. miR−576−3p overexpression enhances cisplatin sensitivity of ovarian cancer cells by dysregulating PD−L1 and cyclin D1. Mol Med Rep (2021) 23(1):81. doi: 10.3892/mmr.2020.11719
179. Yan M, Han M, Yang X, Shen R, Wang H, Zhang L, et al. Dual inhibition of EGFR and IL-6-STAT3 signalling by miR-146b: a potential targeted therapy for epithelial ovarian cancer. J Enzyme Inhib Med Chem (2021) 36(1):1905–15. doi: 10.1080/14756366.2021.1963240
180. Wang W, Fang F, Ozes A, Nephew KP. Targeting ovarian cancer stem cells by dual inhibition of HOTAIR and DNA methylation. Mol Cancer Ther (2021) 20(6):1092–101. doi: 10.1158/1535-7163.MCT-20-0826
Keywords: ovarian cancer, immunotherapy, multi-immunotherapy, immune checkpoint inhibitor, adoptive cell therapy, cancer vaccine, oncolytic virus
Citation: Hu X, Bian C, Zhao X and Yi T (2022) Efficacy evaluation of multi-immunotherapy in ovarian cancer: From bench to bed. Front. Immunol. 13:1034903. doi: 10.3389/fimmu.2022.1034903
Received: 02 September 2022; Accepted: 26 September 2022;
Published: 06 October 2022.
Edited by:
Qi Zhao, University of Macau, ChinaReviewed by:
Yiqing Zhao, Case Western Reserve University, United StatesCopyright © 2022 Hu, Bian, Zhao and Yi. This is an open-access article distributed under the terms of the Creative Commons Attribution License (CC BY). The use, distribution or reproduction in other forums is permitted, provided the original author(s) and the copyright owner(s) are credited and that the original publication in this journal is cited, in accordance with accepted academic practice. No use, distribution or reproduction is permitted which does not comply with these terms.
*Correspondence: Tao Yi, WWl0YW9Ac2N1LmVkdS5jbg==
Disclaimer: All claims expressed in this article are solely those of the authors and do not necessarily represent those of their affiliated organizations, or those of the publisher, the editors and the reviewers. Any product that may be evaluated in this article or claim that may be made by its manufacturer is not guaranteed or endorsed by the publisher.
Research integrity at Frontiers
Learn more about the work of our research integrity team to safeguard the quality of each article we publish.