- 1College of Veterinary Medicine, South China Agricultural University, Guangzhou, China
- 2Key Laboratory of Zoonosis Prevention and Control of Guangdong Province, Guangzhou, China
- 3China Institute of Veterinary Drug Control, Beijing, China
Classical swine fever (CSF), caused by the classical swine fever virus (CSFV), is a highly contagious and fatal viral disease, posing a significant threat to the swine industry. Heat shock protein 90 kDa alpha class A member 1 (HSP90AA1) is a very conservative chaperone protein that plays an important role in signal transduction and viral proliferation. However, the role of HSP90AA1 in CSFV infection is unknown. In this study, we found that expression of HSP90AA1 could be promoted in PK-15 and 3D4/2 cells infected by CSFV. Over-expression of HSP90AA1 could inhibit CSFV replication and functional silencing of HSP90AA1 gene promotes CSFV replication. Further exploration revealed that HSP90AA1 interacted with CSFV NS5A protein and reduced the protein levels of NS5A. Since NS5A has an important role in CSFV replication and is closely related to type I IFN and NF-κB response, we further analyzed whether HSP90AA1 affects CSFV replication by regulating type I IFN and NF-κB pathway responses. Our research found HSP90AA1 positively regulated type I IFN response by promoting STAT1 phosphorylation and nuclear translocation processes and promoted the nuclear translocation processes of p-P65. However, CSFV infection antagonizes the activation of HSP90AA1 on JAK/STAT and NF-κB pathway. In conclusion, our study found that HSP90AA1 overexpression significantly inhibited CSFV replication and may inhibit CSFV replication by interacting with NS5A and activating JAK/STAT and NF-κB signaling pathways. These results provide new insights into the mechanism of action of HSP90AA1 in CSFV infection, which abundant the candidate library of anti-CSFV.
Introduction
Classical swine fever (CSF), caused by the classical swine fever virus (CSFV), is a highly contagious disease in pigs (1), and listed as a notifiable disease by the World Organization for Animal Health (OIE) (2, 3). CSFV, which belongs to the genus Pestivirus within the Flaviviridae family, is an enveloped virus containing a single-stranded, positive-sense RNA genome of approximately 12.3 kb (4, 5). The genome encodes a poly protein that is processed into 4 structural proteins (C、Erns、E1 and E2) and 8 non-structural proteins (Npro、p7、NS2、NS3、NS4A、NS4B、NS5A and NS5B) by protease of the virus and host cells (6, 7). These structural and non-structural proteins have been proposed to play diverse roles in proliferation and virulence. Among these proteins, the essential roles of NS5A protein in regulation of viral replication are getting increasing attention with the deepening of research.
CSFV NS5A protein consists of 496 amino acid (aa) residues, with a molecule of mass 55 kDa (8, 9). In spite of the exact function of NS5A is still unknown, many researches on it seem to imply that the NS5A protein is an important tool of CSFV to generate a favorable environment for viral replication. CSFV NS5A protein could interact with a variety of host proteins. For instance, a few studies indicate that NS5A protein interacts with eukaryotic translation initiation factor 3 subunit E (eIF3E), ras-related protein 18 (Rab-18), glucose-regulated protein 78 (GRP78), heat shock protein 70 (Hsp70) and so on to facilitate viral replication (10–13). Recent studies revealed that NS5A induces autophagy to enhance replication of CSFV (14, 15). It has also been shown that CSFV NS5A protein could inhibit the secretion of inflammatory cytokines by suppressing the NF-κB pathway (16). Although the effect of CSFV NS5A on type I IFNs pathway is not well understood, sufficient studies have shown that many flaviviruses NS5 have an antagonism of type I IFN (17). For example, Japanese encephalitis virus (JEV) NS5 could competitively bind to the nuclear transport proteins KPNA3 and KPNA4, which inhibited the nuclear translocation of IRF3 and NF-κB, resulting in the suppression of type I IFN (18). The NS5A protein of hepatitis C virus (HCV) which also belongs to the Flaviviridae family, suppresses type I interferon signaling and the phosphorylation of STAT1 (19). Moreover, a study also reveals that binding of the NS5 to Hsp90 could disrupt the interaction of Hsp90 with Janus kinase (JAK), which can help flaviviruses to broadly inhibit JAK/STAT pathway (20).
As is well known, type I IFN (IFN-α/β) is one of the critical lines of defense against viral infections widely (21, 22). IFN-dependent anti-viral response, is initiated by intracellular signaling cascade through the Janus protein kinase (JAK) family members, JAK and Tyk2 (23, 24). The binding of type I IFNs with the receptor (IFNAR) trigger JAK and STATs phosphorylation (25, 26). Subsequently, the phosphorylated STATs dimerize and translocate to the nucleus where they bind to IFN-response elements (ISRE) in ISG promoters to activate transcription of ISGs (24, 26, 27). The antiviral response induced by IFN/JAK/STAT could prevent viral replication directly and quickly (28). ISGs those are amplified effect factors of the IFN signaling cascades have been proved to block various steps of the viral life (26, 29). These ISG-encoded proteins could act directly on the virus to limit viral infection (22, 29). For instance, IFN-α inducible Mx2 could inhibit HBV replication and RNA transcription (30). Studies have shown that ISG15, Mx1 and OAS could inhibit CSFV replication (31, 32).
NF-κB pathway also plays an important role in the control of immunity, inflammation and other processes (33). The binding of viral pathogen-associated molecular patterns (PAMPs) to their receptor host pathogen recognition receptors (PRRs) triggers natural immunity and activates IFN regulatory factor (IRF) family members as well as NF-κB and thus promotes the expression of downstream ISGs (34). The transcription factor NF-κB proteins consist of the Rel family of proteins which include RelA (P65), RelB, c-Rel, p105/p50 (NF-κB1) and p100/p52 (NF-κB2) (35). In most resting cells, the NF-κB protein binds to its inhibitory protein IκB and maintains inactive in the cytoplasm (36). When is activated, the IκB phosphorylates and degrads rapidly, which in turn exposes NF-κB to the nuclear localization sequence (NLS) before translocating to the nucleus to drive the corresponding gene transcription (33). Activation of the NF-κB signaling pathway also play a key role to restrict virus replication (37). Studies have shown that activation of NF-κB is necessary for the generation of ROS to limit HSV-1 replication (38), and may affect HBV viral replication levels by regulating antiviral immunity (39). However, CSFV NS5A inhibits NF-κB nuclear translocation and NF-κB activity (16).
Heat shock protein 90 (HSP90), which family includes HSP90α, HSP90β, glucose-regulated protein 94 (GRP94) and tumor necrosis factor receptor-associated protein 1 (TRAP1) isoforms, is an essential molecular chaperon that is highly conserved in evolution (40, 41). It is involved in diverse biological processes such as virus infection, immune regulation, signal transduction. (42, 43). Many viruses depend on cellular HSP90 to complete their life cycles, especially depend on HSP90α and HSP90β isoforms (44). Studies have revealed that HSP90 is vital for the reverse transcriptase viability of Hepatitis B Virus (HBV), which is essentially required to initiate and maintain HBV reverse transcription (45, 46). In addition to direct binding to viral proteins, HSP90 can also affect the viral infection by regulating the expression of cytokines and antigen presentation (47). It is worth pointing out that HSP90 is required by JAK and IKK to enhance kinase activity, which promotes activation and nuclear translocation of STAT and NF-κB (43, 48).
In previous studies, we found that HSP90AB1 interacts with CSFV NS5A protein (15). However, it is currently unknown whether one of the HSP90 family members, HSP90AA1, also interacts with NS5A and the association of HSP90AA1 with JAK/STAT and NF-κB affects CSFV replication. Therefore, we sought to explore the regulatory effect of HSP90AA1 on JAK/STAT and NF-κB in CSFV infection.
Here, we found that there is a regulatory relationship between CSFV infection and the expression of HSP90AA1. The over-expression of HSP90AA1 inhibits CSFV replication. Further, HSP90AA1 interacts with viral protein NS5A and decreased the protein levels of NS5A. Mechanistically, our results show that over-expression of HSP90AA1 could activate JAK/STAT and NF-κB signaling pathways, which antagonize CSFV infection. Thus, HSP90AA1 is a key host factor limiting CSFV proliferation, it could interact with CSFV NS5A protein and its upregulation could activate host cell antiviral responses.
Material and methods
Cells, virus, and plasmids
Porcine alveolar macrophages 3D4/2 were cultured in RPMI 1640 medium (Thermo Fisher, 11875500) with 10% fetal bovine serum (FBS) (Thermo Fisher, 10100147). The swine kidney cell line PK-15 (ATCC, CCL-33) and human embryonic kidney cell line HEK293T (ATCC, CRL-1573) were cultured in Dulbecco’s minimal essential medium (Thermo Fisher, 11965092) with 8% FBS. All cells were cultured at 37°C in a 5% CO2 incubator. The CSFV strain (Shimen) used in this study was stored in our laboratory and was propagated in PK-15 cells. pMD18-T-NS5B, p3×Flag-CMV10, pEGFP-C3, pEGFP-NS4A and pEGFP-NS5A were deposited in our laboratory. Lipo3000 (Thermo Fisher, L3000075) reagent was used for transient transfection of plasmids.
Virus titration by indirect immunofluorescence assay (IFA)
The PK-15 cells were transferred to a 96-well plate, and CSFV virus solution was inoculated when the mono-layer cells reached 70%~80% confluence. The CSFV virus solution was inoculated in 1.5 mL centrifuge tubes with DMEM for 10-fold serial dilution (10-1 to 10-7), and 4 wells were repeated for each dilution.
IFA was used to determine CSFV titers in the culture supernatant. With 48-hour post infection (hpi), cells were washed three times with PBS and fixed with pre-chilled absolute ethanol (200 μL/well) at -20°C for 20 minutes. Following three washes with PBS, the cells were dried at room temperature for about 10 min to completely evaporate the absolute ethanol. The cells were incubated CSFV E2 antibody (JBT, 9011) at 4°C overnight (in dilute E2 protein antibody with PBS at a ratio of 1:200 (40 μL/well). After five washes, the cells were incubated with FITC-labeled or Alexa488-labeled goat anti-mouse IgG antibody (Beyotime, A0428) at 37°C for 1 h. After five washes, Immunofluorescence was observed using a fluorescence microscope (Nikon, Japan). Mock-infected cells were used as controls to establish background staining levels.
Quantitative real-time RT-PCR (qPCR)
The relative mRNA expression of HSP90AA1, ISGs and IFN-α was tested by RT-PCR using specific primers (Table 1). Total RNA from cells was extracted using Total RNA Kit I (OMEGA, R6834) and Viral RNA extraction using Viral RNA Kit (OMEGA, R6874). Subsequently, the cDNA was synthesized by reverse transcription using the HiScript II Q RT SuperMix for qPCR (Vazyme, R223-01). RT-PCR was performed with ChamQ Universal SYBR qPCR Master Mix (Vazyme, Q711-02) according to the manufacturer’s protocol. Relative quantification of mRNA levels was conducted using ΔΔCT method and β-actin as an internal reference gene. Calculation of the gene copy numbers of CSFV was carried out using the absolute quantification method. A standard curve generated from the amplification results of the standard (10-fold serial dilution of the pMD18-T-NS5B plasmid of known concentration) was used to calculate the CSFV gene copy numbers.
Western blot analysis
Cell lysates were prepared in radioimmunoprecipitation (RIPA) (Beyotime, P0013) buffer with protease and phosphatase inhibitor cocktail (Beyotime, P1050). The protein concentration was determined with Pierce™ BCA Protein Assay Kit (Thermo Fisher, 23225). The samples were separated by 10% or 12.5% SDS-PAGE that prepared with PAGE Gel Rapid Prep Kit (Jacob enzyme Biotech, PG112) followed by transfer onto polyvinylidene difluoride (PVDF) membranes. After blocking with 5% skim milk at room temperature for 1 h, the membranes were incubated with primary antibodies overnight at 4°C. Primary antibodies used include mouse anti-HSP90AA1 monoclonal antibody (mAb) (Santa Cruz, sc-515081), mouse anti-phospho-STAT1 polyclonal antibody (pAb) (Santa Cruz, sc-136229), mouse anti-tubulin mAb (Beyotime, AT819), mouse anti-Flag mAb (Beyotime, AF5051), rabbit anti-GFP mAb (Beyotime, AF1483), rabbit anti-STAT1 pAb (Beyotime, AF0288), rabbit anti-phospho-JAK1 pAb (Beyotime, AF5857), rabbit anti-P65 mAb (Abmart, AF1234), rabbit anti-JAK1 mAb (Abmart, AT8190), rabbit anti-IκBα mAb (Abmart, T55026S), rabbit anti-phospho-IκBα mAb (Abmart, T57246S), rabbit anti-OAS2 pAb (Sangon Biotech, D121064) and rabbit anti-ISG15 pAb (Sangon Biotech, D225264). After three washes with PBST, the membranes were incubated with horseradish peroxidase (HRP)-conjugated goat anti-mouse IgG (Beyotime, A0216) secondary antibody at 37°C in a thermal shaker for 1 h. Finally, the results were visualized by ECL chemiluminescence kit (Jacob enzyme Biotech, SQ201) and X-ray film exposure (Tanon, China).
Plasmid construction and synthesis of small interfering RNA
HSP90AA1 PCR amplification primers were designed according to the HSP90AA1 sequence (NM_213973.2) published by NCBI using Primer Premier 5. HSP90AA1 was amplified by PCR from PK-15 cells cDNA and cloned into p3×Flag CMV10. The siRNA targeting HSP90AA1 and a negative control siNC were designed and synthesized by Sangon Biotech. All primers and sequence of siRNA were listed in Table 1.
Immunoprecipitation
The p3×Flag-HSP90AA1 was co-transfected into 293T cells with pEGFP-NS5A or pEGFP-NS4A using Lipofectamine™ 3000, respectively. Controls were represented by cells cotransfected with p3×Flag-HSP90AA1 and pEGFP, p3×Flag-CMV and pEGFP-NS5A. Cells were harvested at 24h after plasmid transfection using Western blot and IP lysis buffer (Beyotime, p0013) containing a protease inhibitor. After centrifugation for 10 min at 4°C, a part of the supernatant was boiled for 10 min with loading buffer (Beyotime, P0015L) as whole cell extracts (Input). The remaining lysate, used for IP experiment, was first incubated with Protein A+G at 4°C with slow rotation for 4h, and then incubated with the corresponding Flag or GFP antibody at 4°C overnight. After incubation, samples were centrifuged at 4°C for 2 min (2000 g/min). The supernatant was discarded and the precipitate was washed five times with pre-cooled PBS. Finally, loading buffer was added to the precipitate and boiled for 10 minutes to perform Western blot experiments with the input samples.
Confocal microscopy
The HEK-293T cells were cultured in laser confocal dishes to 60% confluence. The p3×Flag-HSP90AA1 was co-transfected into 293T cells with pEGFP-NS5A using Lipofectamine™ 3000, respectively. While setting two controls: 293T cells respectively cotransfected with p3×Flag-HSP90AA1 and pEGFP, p3×Flag-CMV and pEGFP. After culturing for 24 h, the cells were washed twice with PBS and fixed with pre-cooled absolute ethanol at room temperature for 10 minutes. Absolute ethanol was discarded and the cells were washed 3 times with PBS, then permeabilized with 0.1% Triton X-100 for 10 min at room temperature and discarded. Cells were washed 3 times with PBS and incubated with goat anti-mouse Flag antibody (Beyotime, AF5051) overnight at 4°C (antibody was diluted 1:200 in PBS), then washed 3 times with PBS and incubated with Cy3-labeled fluorescent secondary antibody (Beyotime, A0521) at 37°C for 1 Hour. The nucleus was counterstained with DAPI for 10 min. Finally, anti-fluorescence quencher was added dropwise to the cells. Observation of cell fluorescence signals under a laser confocal microscope.
In the experiment of the effect of HSP90AA1 on the phosphorylation and nuclear translocation of STAT1 and P65, p3×Flag-HSP90AA1 was transfected into PK-15 cells and 3D4/2 cells. Primary antibodies include rabbit anti-p-STAT1 pAb (Bioss, bs-3427R), rabbit-anti-p-P65 pAb (Affinity Biosciences, AF2006) and mouse anti-Flag mAb. Fluorescent secondary antibodies include FITC-labeled goat anti-mouse IgG (Beyotime, A0568) and Cy3-labeled goat anti-rabbit IgG (Beyotime, A0516).
Results
CSFV infection Up-regulates HSP90AA1 expression
To explore whether CSFV infection affects the expression of HSP90AA1, CSFV-infected (MOI=1) and CSFV-uninfected PK-15 and 3D4/2 cells were harvested at 24 hpi and 48 hpi to detect the mRNA and levels of HSP90AA1. The results showed that there was a significant increase in mRNA and protein levels of HSP90AA1 in PK-15 and 3D4/2 cells compared with control cells (Figure 1). The results show that CSFV infection prompted HSP90AA1 expression.
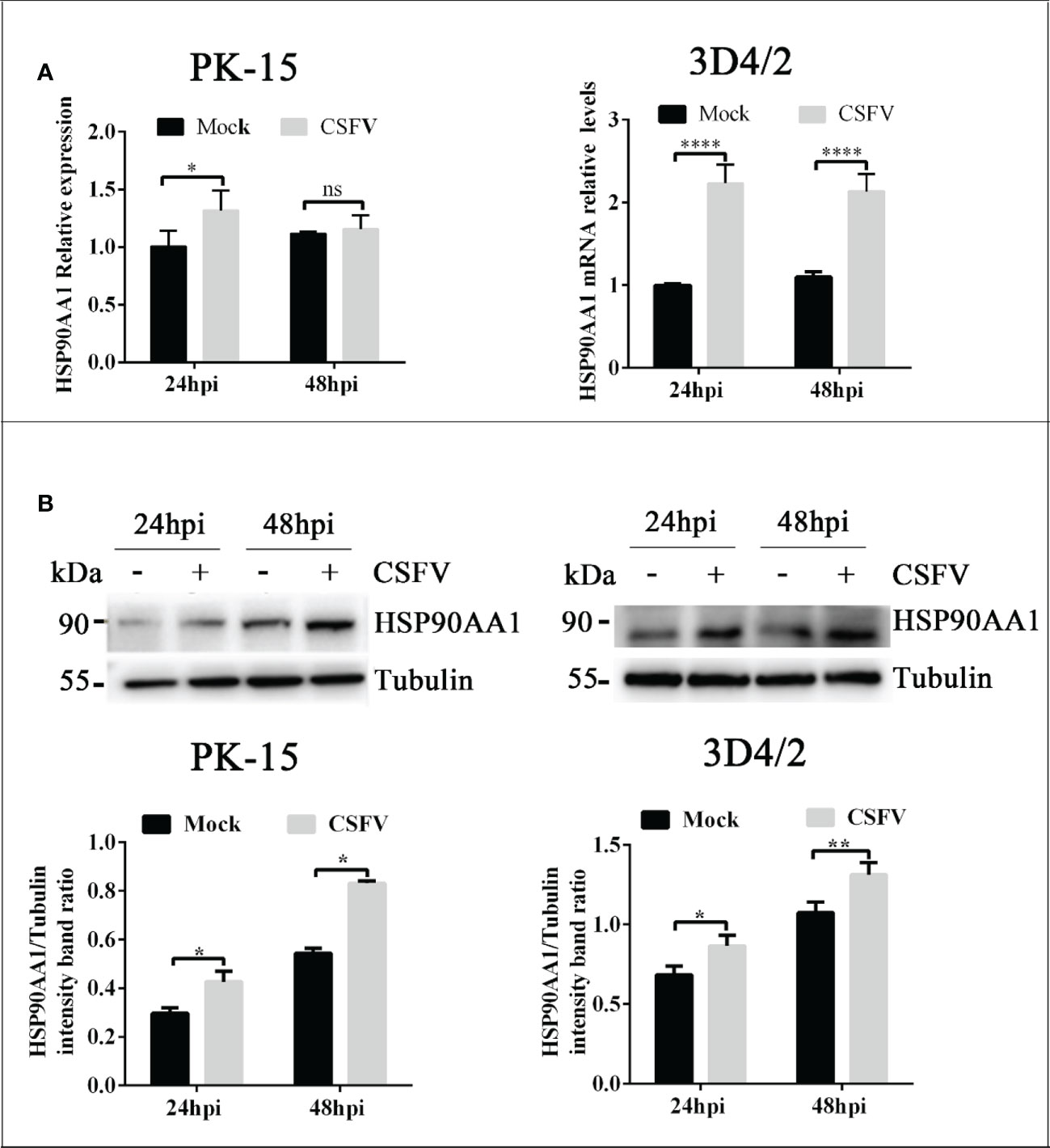
Figure 1 CSFV infection Up-regulates HSP90AA1 expression. (A) HSP90AA1 mRNA relative levels in PK-15 and 3D4/2 cells was analyzed by qRT-PCR; (B) Western blot showing HSP90AA1 protein expression and the relative protein levels of HSP90AA1 in PK-15 and 3D4/2 cells were estimated by histograms representing density readings of the gel bands with Image J, and the ratios were calculated relative to Tubulin control. (*p < 0.05, **p < 0.01 and ****p < 0.0001 calculated using two-way ANOVA, ns, not significant).
HSP90AA1 overexpression decreases CSFV replication
In order to determine the effect of HSP90AA1 on CSFV replication, PK-15 and 3D4/2 cells was transfected with p3×Flag-HSP90AA1 and then infected with CSFV (MOI=1). The replication of CSFV was detected by qRT-PCR, Western blot and IFA, respectively. The results showed that over-expression of HSP90AA1 inhibited CSFV replication in PK-15 and 3D4/2 cells. (Figure 2).
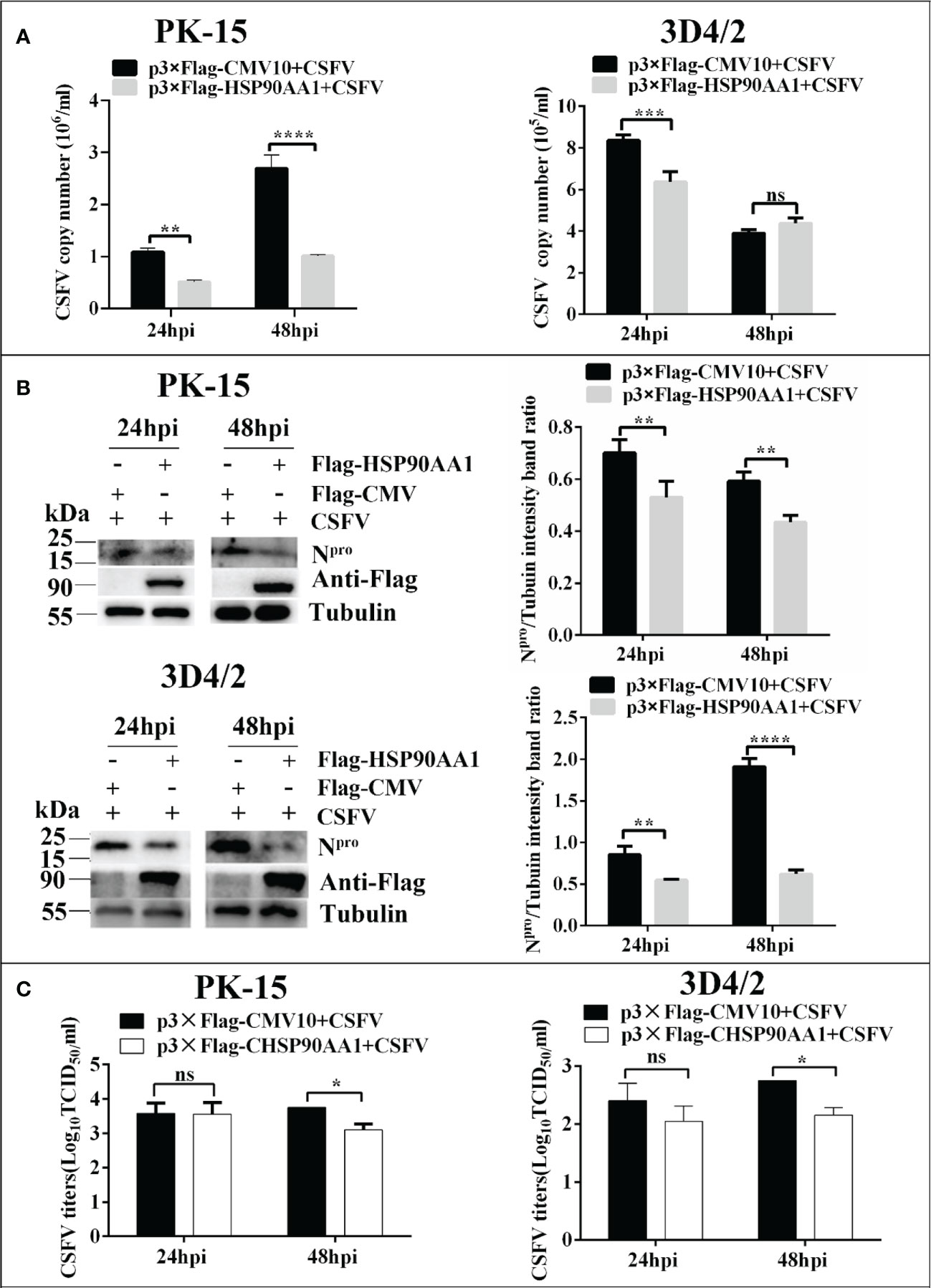
Figure 2 Over-expression of HSP90AA1 inhibits CSFV replication. (A) CSFV NS5B copy numbers were determined by RT-PCR at 24 and 48 hpi in HSP90AA1-overexpression PK-15 and 3D4/2 cells. (B) Western blot for HSP90AA1 and CSFV Npro expression in HSP90AA1-overexpression PK-15 and 3D4/2 cells, and the relative protein levels of Npro in HSP90AA1-overexpression PK-15 and 3D4/2 cells were estimated by histograms representing density readings of the gel bands with Image J, and the ratios were calculated relative to Tubulin control. (C) Infectious progeny viral titers in supernatants from HSP90AA1-overexpressing PK-15 and 3D4/2 cells. Viral titers from the supernatants collected at 24 and 48 hpi were determined and expressed as TCID50/ml. (*p < 0.05, **p < 0.01, ***p < 0.001 and ****p < 0.0001 calculated using two-way ANOVA. ns, not significant).
HSP90AA1 inhibition promotes CSFV replication
To investigate whether silencing HSP90AA1 expression affects CSFV replication, small interfering RNA targeting HSP90AA1 (siRNA-HSP90AA1) was transfected into PK-15 and 3D4/2 cells and then the cells were infected with CSFV (MOI=1). Similarly, the replication of CSFV was detected by qRT-PCR, Western blot and IFA, respectively. The results showed that silencing of HSP90AA1 gene function increased CSFV replication in PK-15 and 3D4/2 cells (Figure 3).
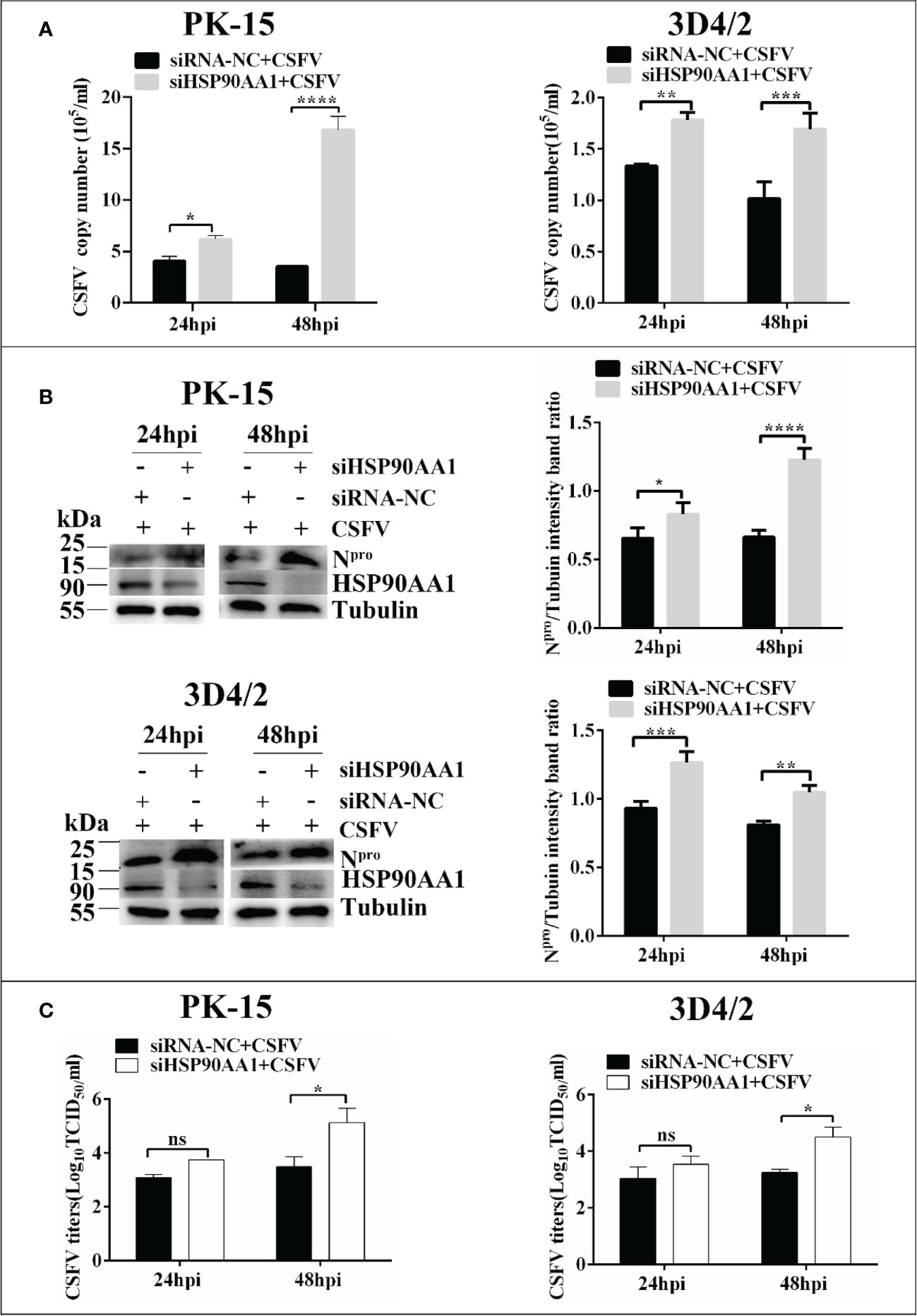
Figure 3 Knockdown of HSP90AA1 promoted CSFV replication. (A) RT-PCR determined CSFV NS5B copy numbers at 24 and 48 hpi in HSP90AA1 knockdown PK-15 and 3D4/2 cells. (B) Western blot for HSP90AA1 and CSFV Npro expression in HSP90AA1 knock-downed PK-15 and 3D4/2 cells, and the relative levels of Npro in HSP90AA1 knock-downed PK-15 and 3D4/2 cells were estimated by histograms representing density readings of the gel bands with Image J, and the ratios were calculated relative to tubulin control. (C) Infectious progeny viral titers in supernatants from HSP90AA1-knockdown PK-15 and 3D4/2 cells. Viral titers from the supernatant collected at 24 and 48 hpi were determined and expressed as TCID50/ml. (*p < 0.05, **p < 0.01, ***p < 0.001 and ****p < 0.0001 calculated using two-way ANOVA, ns, not significant).
HSP90AA1 interacts with CSFV NS5A protein
Our previous works have found that CSFV NS5A protein interacted with HSP90 by liquid chromatography-mass spectrometry (15). Due to that HSP90AA1 was one of the members of the HSP90 family, this study further confirms the interaction of HSP90AA1 with CSFV NS5A protein. The results of confocal laser microscopiclaser showed that CSFV NS5A co-localized with HSP90AA1 in the cytoplasm in 293T, PK-15 and 3D4/2 cells (Figures 4A, C, D). And the results of co-immunoprecipitation experiments showed that HSP90AA1 interacted with CSFV NS5A protein in 293T cells (Figure 4B).
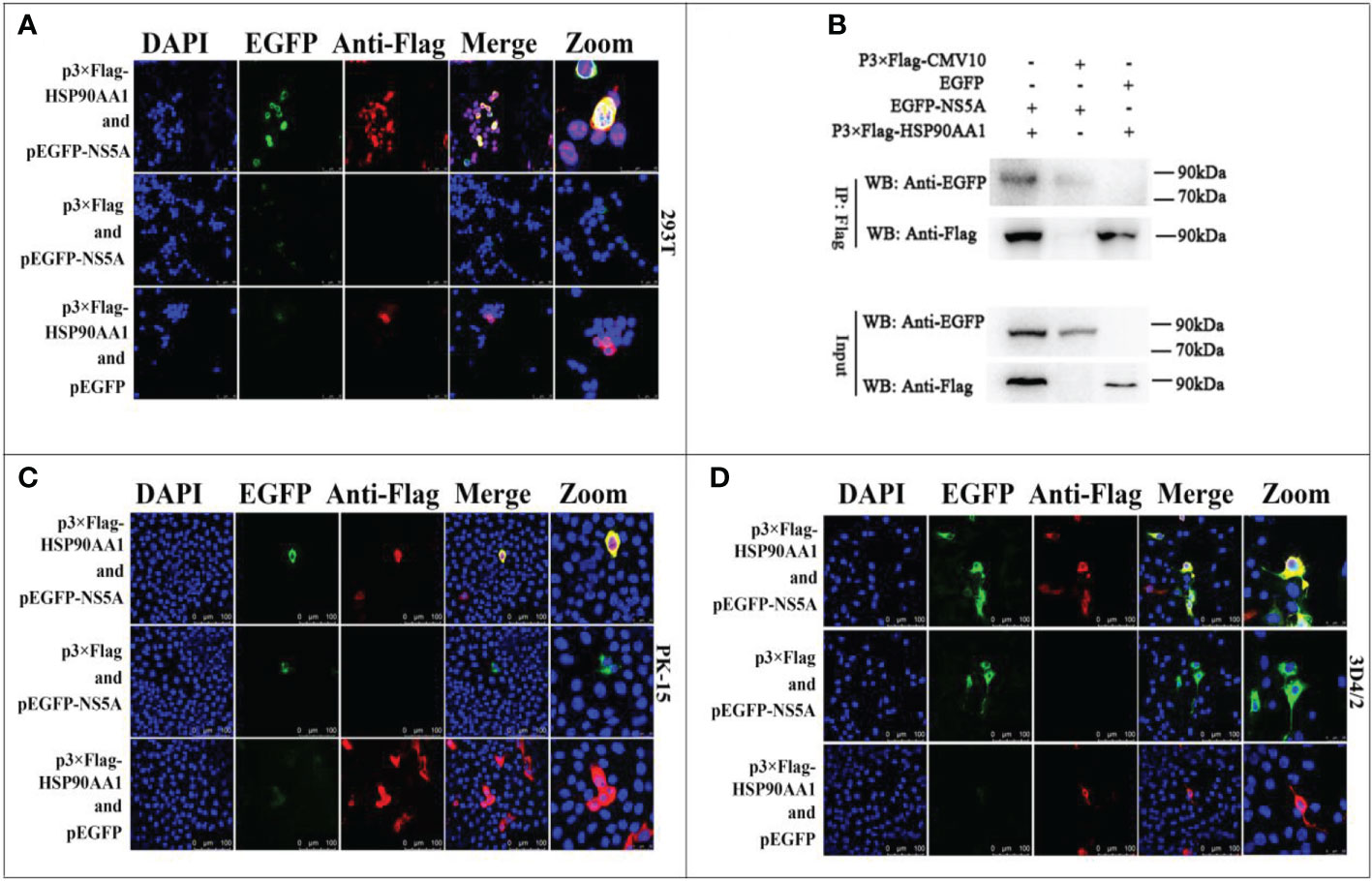
Figure 4 Validation for the interaction of HSP90AA1 with CSFV NS5A protein. (A) Identification of the colocalization of HSP90AA1 with NS5A protein in 293T cells. 293T cells co-expressing pEGFP-NS5A and p3×Flag-HSP90AA1 were analyzed by laser-scanning confocal microscopy. 293T Cells were co-transfected with pEGFP and p3×Flag-HSP90AA1 and pEGFP-NS5A with p3×Flag-CMV as negative controls. (B) Exogenous Co-IP analysis of NS5A with HSP90AA1 in 293T cells. 293T cells were co-transfected with p3×Flag-HSP90AA1 and EGFP-NS5A. 293T Cells were co-transfected with pEGFP and p3×Flag-HSP90AA1 and pEGFP-NS5A with p3×Flag-CMV as negative controls. (C, D) Identification of the colocalization of HSP90AA1 with NS5A protein in PK and 3D4/2 cells. PK-15 and 3D4/2 cells co-expressing pEGFP-NS5A and p3×Flag-HSP90AA1 were analyzed by laser-scanning confocal microscopy. PK-15 and 3D4/2 Cells were co-transfected with pEGFP and p3×Flag-HSP90AA1 and pEGFP-NS5A with p3×Flag-CMV as negative controls.
HSP90AA1 reduces the protein levels of CSFV NS5A
To investigate effects of HSP90AA1 on CSFV NS5A protein levels, we co-transfected pEGFP-NS5A with different amounts of p3×Flag-HSP90AA1 into PK-15 and 3D4/2 cells, and the cells were harvested to assess protein levels of fusion protein EGFP-NS5A by Western blot. Meanwhile, co-transfected NS4A was used as a control. We found that the protein levels of NS5A decreased gradually with the increasing expression of HSP90AA1 in PK-15 and 3D4/2 cells without dose-dependent inhibition of NS4A (Figure 5). It suggested that HSP90AA1 was not required to maintain the stability of CSFV NS5A. On the contrary, it can specifically reduce the protein levels of CSFV NS5A.
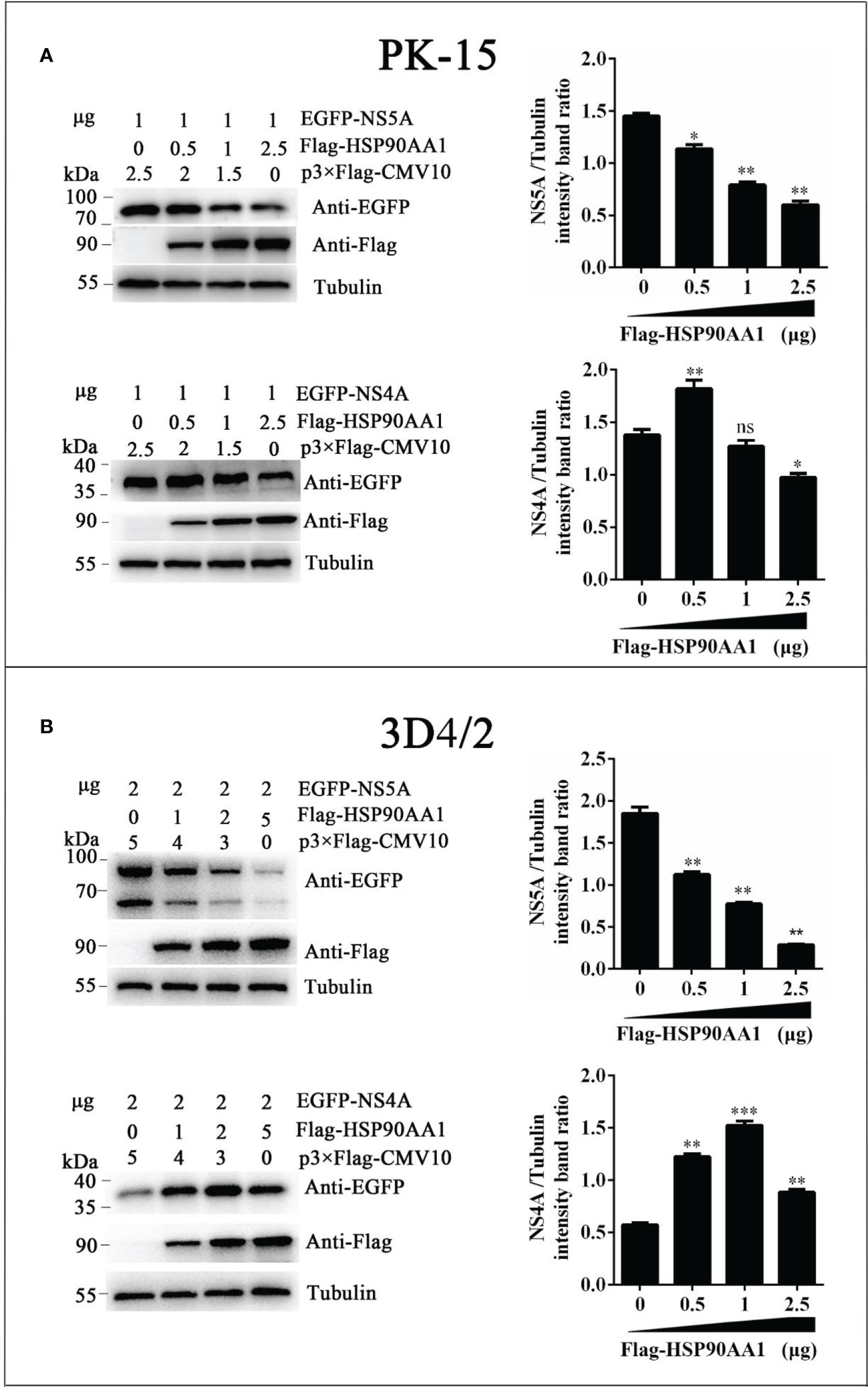
Figure 5 HSP90AA1 reduces the protein levels of CSFV NS5A. (A, B) EGFP-NS5A or EGFP-NS4A protein expression in HSP90AA1-expressing PK-15 and 3D4/2 cells. PK-15 and 3D4/2 cells were co-transfected with pEGFP-NS5A or EGFP-NS4A with a different amount of p3×Flag-HSP90AA1. Western blot analyzed EGFP-NS5A or EGFP-NS4A protein expression at 24 hours post co-transfected. Tubulin served as an internal control. The relative levels of EGFP-NS5A or EGFP-NS4A were estimated by histograms representing density reading of the gel bands with Image J, and the ratios were calculated relative to tubulin control. (*p < 0.05, **p < 0.01 and ***p < 0.001 calculated using one-way ANOVA, ns, not significant).
HSP90AA1 over-expression activates STAT1 and P65
To study the effect of HSP90AA1 on JAK/STAT and NF-κB signaling pathways, we detected the phosphorylation and nuclear translocation of STAT1 and P65 in HSP90AA1-overexpressing PK-15 and 3D4/2 cells by laser confocal microscopy. As a result, it was observed that higher levels of p-STAT1 in HSP90AA1-overexpressing PK-15 and 3D4/2 cells than that observed in control cells. And p-STAT1 was mostly distributed in the nucleus of HSP90AA1-overexpressing PK-15 while p-STAT1 in control cells was mainly distributed in the cytoplasm (Figure 6A). In addition, the nuclear distribution of p-P65 in HSP90AA1-overexpressing PK-15 and 3D4/2 cells was more than that in control cells (Figure 6B). These results indicated that HSP90AA1 over-expression promoted nuclear translocation of p-STAT1 and p-P65.
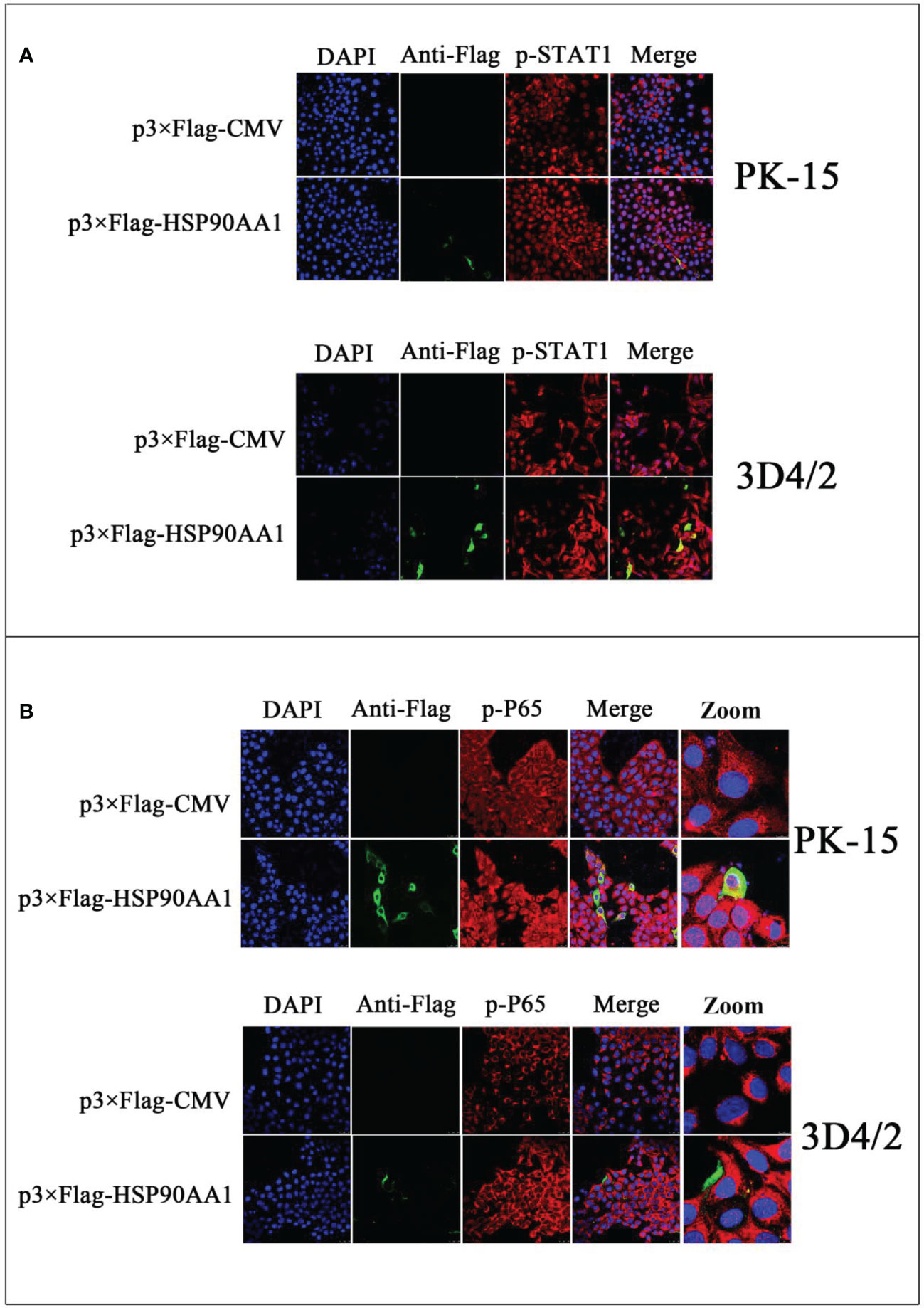
Figure 6 HSP90AA1 over-expression activates STAT1 and P65 in PK-15 and 3D4/2 cells. (A) HSP90AA1 over-expression affected the phosphorylation of STAT1 and nuclear translocation of p-STAT1 in PK-15 and 3D4/2 cells were observed by laser confocal microscopy. (B) HSP90AA1 over-expression affected the nuclear translocation of p-P65 in PK-15 and 3D4/2 cells were observed by laser confocal microscopy.
HSP90AA1 over-expression does not promote the expressions of ISGs and IFN-α under CSFV infection
To investigate the effect of HSP90AA1 on the regulation of JAK/STAT and NF-κB pathways under CSFV infection, we first analyzed the expression levels of ISGs and IFN-α in HSP90AA1-overexpressing PK-15 and 3D4/2 cells which were infected with CSFV (MOI=1). The cells were harvested at 24 hpi and 48 hpi to detect the mRNA relative levels of ISGs and IFN-α by RT-PCR. The results showed that over-expression of HSP90AA1 could significantly promote the expressions of ISGs (ISG-15, OAS2 and Mx1) in PK-15 and 3D4/2 cells and significantly promote the expressions of IFN-α in 3D4/2 cells (Figure 7). However, HSP90AA1 Over-expression does not promote the expressions of ISGs under CSFV infection (Figure 7). This suggests that the facilitation effect of HSP90AA1 on ISGs is diminished or even disappeared after CSFV infection.
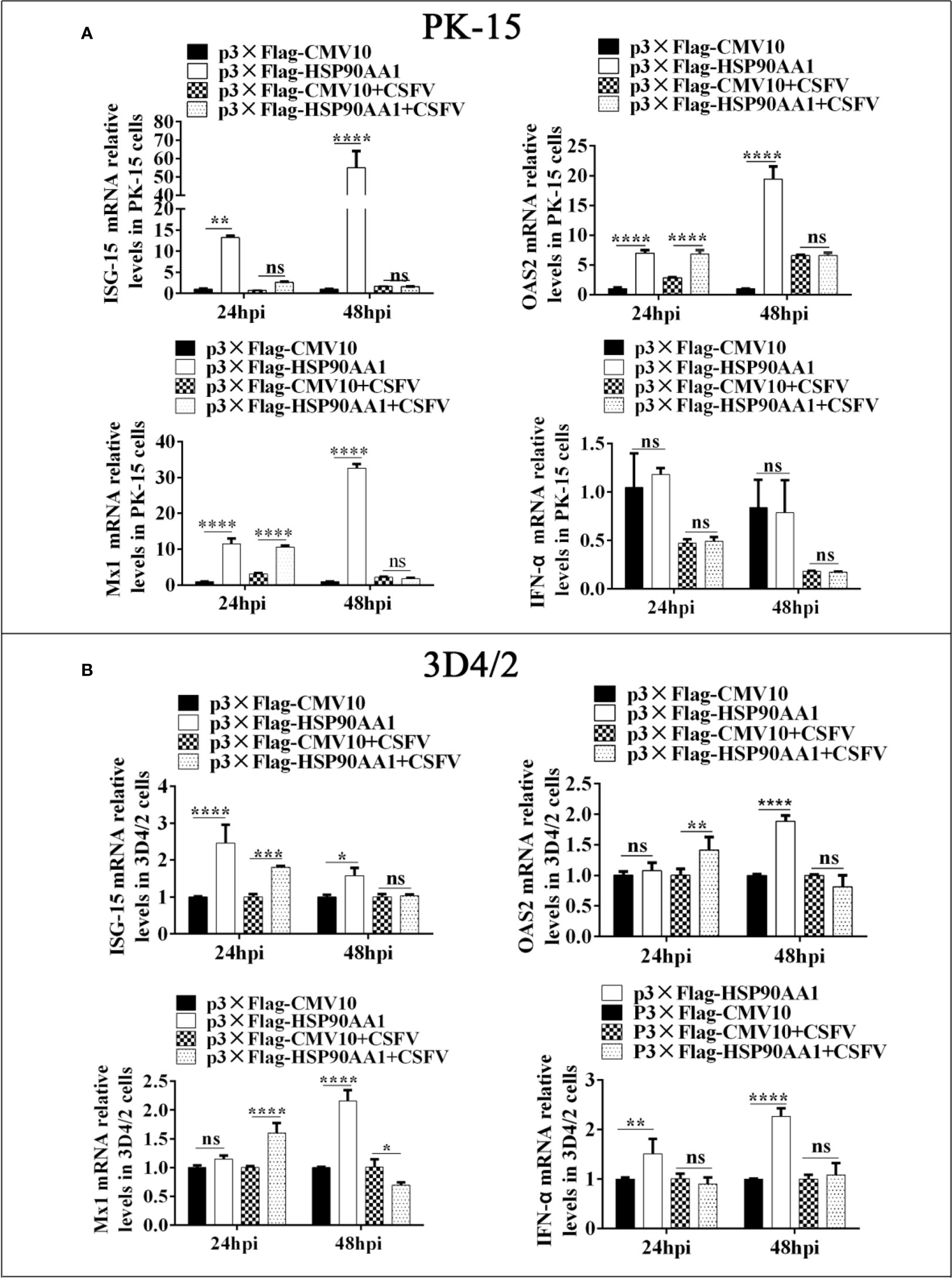
Figure 7 HSP90AA1 over-expression does not promote the expressions of ISGs and IFN-α under CSFV Infection. (A, B) Relative mRNA levels of ISGs and IFN-α in PK-15 and 3D4/2 cells were determined by RT-PCR. Cells were transfected with p3×Flag-HSP90AA1 first and then infected with CSFV (MOI=1). Cells were harvested at 24hpi and 48hpi. Total cellular RNA was extracted to determine relative mRNA expression levels of ISGs and IFN-α. (*p < 0.05, **p < 0.01, ***p < 0.001 and ****p < 0.0001 calculated using two-way ANOVA, ns, not significant).
CSFV infection antagonizes the activation of HSP90AA1 on JAK/STAT and NF-κB pathway
To further verify the effects of HSP90AA1 on JAK/STAT and NF-κB pathway under CSFV infection, we detected changes in the levels of proteins related to above two pathways from CSFV infection in HSP90AA1 over-expression or knock-down PK-15 and 3D4/2 cells. The results showed that HSP90AA1 activated JAK/STAT pathway, while CSFV infection attenuates activation of HSP90AA1 on JAK/STAT pathway (Figure 8). HSP90AA1 significantly promoted the phosphorylation of IκBα, but had no significant effect on the protein expressions of P65 and IκBα, while CSFV infection inhibited the phosphorylation of HSP90AA1 on IκBα (Figure 9). These results showed that CSFV infection antagonizes the activation of HSP90AA1 on JAK/STAT and NF-κB pathway. It is suggested that HSP90AA1 may inhibit CSFV replication via activating JAK/STAT and NF-κB pathway.
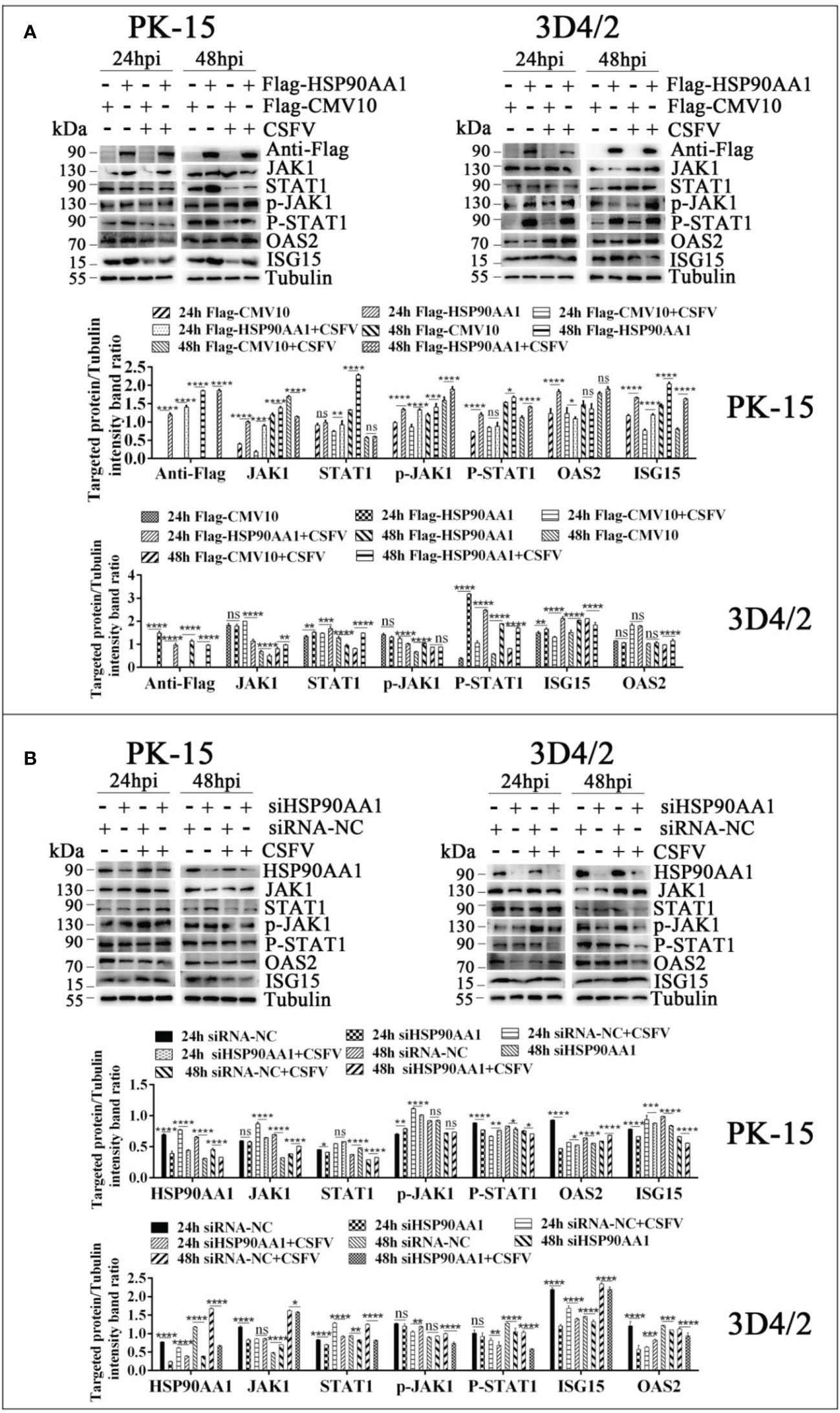
Figure 8 CSFV infection antagonizes the activation of HSP90AA1 on JAK/STAT pathway. (A, B) Western blot for JAK1, STAT1, p-JAK1, p-STAT1, OAS2, ISG-15 and HSP90AA1 expression in HSP90AA1-overexpression or knock-downed PK-15 and 3D4/2 cells. Cells were infected with CSFV (MOI=1) after transfection p3×Flag-HSP90AA1 or siHSP90AA1. The cells were not infected with CSFV as a control. Cells were harvested at 24hpi and 48hpi served to Western blot. The relative levels of proteins were estimated by histograms representing density reading of the gel bands with Image J, and the ratios were calculated relative to tubulin control. (*p < 0.05, **p < 0.01, ***p < 0.001 and ****p < 0.0001 calculated using two-way ANOVA, ns, not significant).
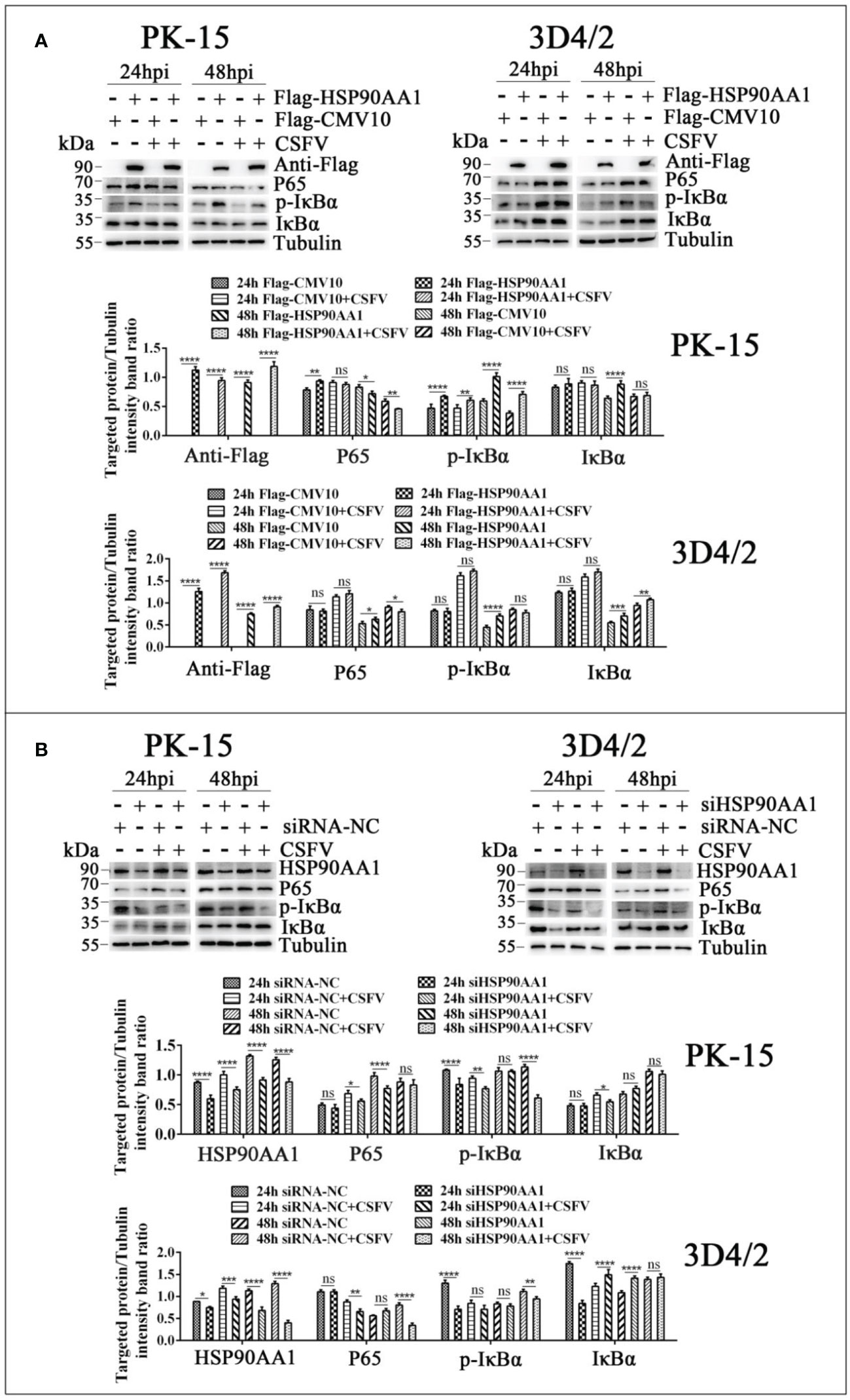
Figure 9 CSFV infection antagonizes the activation of HSP90AA1 on NF-κB pathway. (A, B) Western blot for P65, p-IκBα and IκBα in HSP90AA1-overexpression or knock-downed PK-15 and 3D4/2 cells. Cells were infected with CSFV (MOI=1) after transfection p3×Flag-HSP90AA1 or siHSP90AA1, and the cells were not infected with CSFV as a control. Cells were harvested at 24hpi and 48hpi served to Western blot. The relative levels of proteins were estimated by histograms representing density reading of the gel bands with Image J, and the ratios were calculated relative to tubulin control. (*p < 0.05, **p < 0.01, ***p < 0.001 and ****p < 0.0001 calculated using two-way ANOVA, ns, not significant).
Discussion
The production of type I IFNs are triggered by virus infection, which in turn activates the synthesis of interferon-stimulated genes to limit viral proliferation (49). The activation of JAK/STAT and NF-κB signaling pathway plays a key role in establishing the antiviral state (17, 29). However, CSFV suppresses the production of type I IFN, which facilitates its massive replication and persistent infection in tropic cells (15, 50). Moreover, like other members of the Flaviviridae family, CSFV NS5A also plays a critical role in regulation of type I IFN response (15, 16). In the current study, we found that HSP90AA1 could inhibit CSFV replication as a host antiviral factor. Further research found that HSP90AA1 may inhibit CSFV replication by inhibiting CSFV NS5A protein expression in a dose-dependent manner and activating JAK/STAT and NF-κB signaling pathway.
The critical role of HSP90 in type I IFN response has been well described (51, 52). HSP90 inhibition can lead to dysregulation of JAK/STAT pathway activation and suppress the activation of NF-κB (53, 54). Our results highlight that HSP90AA1 positively regulates type I IFN response by promoting the stability of JAK, and STAT1 phosphorylation and nuclear translocation processes. It also promotes the activation of NF-kB signaling pathway. However, the promotion of type I IFN and ISGs by HSP90AA1 was inhibited in the CSFV-infected state. We hypothesize that HSP90AA1 is not the main molecule for CSFV to escape innate immunity, there are still other key proteins that help CSFV to escape innate immunity, but HSP90AA1 is important for establishing an intracellular antiviral immune state, which helps to limit viral infection.
Like other members of the Flaviviridae family, CSFV infection significantly inhibits the activation of the JAK/STAT signaling pathway (50, 55). Although the mechanism of the inhibition is not fully elucidated, many studies suggest that flavivirus nonstructural protein NS5 plays an important role in this process (56–58). Justin A. et al. proposed that the binding of the flavivirus nonstructural protein NS5 to HSP90 resulting in an imbalance in JAK/STAT pathway signal transduction (20). Our results show that HSP90AA1 is not necessary to maintain NS5A stability, but rather dose-dependently inhibits the protein levels of NS5A. It suggests that HSP90AA1 can act as a key host restriction factor to limit CSFV replication by inhibiting the CSFV NS5A protein.
It seems to be evident that HSP90 is able to enhance the homeostasis of signal transduction proteins in stressful stress states (42, 59). However, there are also distinct effects of HSP90 in different viral infections. It has been shown that HSP90AA1 can promote rabies virus (RABV) proliferation by binding to P protein (60). Enterovirus 71 (EV71) in human rhabdomyosarcoma enters cells by binding to HSP90β on the surface of cells and the cytoplasmic HSP90β can prevent viral proteins from being degraded by proteasome (61). However, our results revealed that CSFV infection induces upregulation of HSP90AA1 expression and that HSP90AA1 overexpression activates innate immunity to in turn inhibit CSFV replication. We hypothesize that inhibition of type I interferon response by CSFV infection may lead to the accumulation of HSP90AA1, which has a regulatory effect on innate immunity. Although upregulation of HSP90AA1 did not alter the inhibition of the type I interferon response by CSFV, it may act as a negative feedback signal to promote cell survival and protect cellular proteins from the risk of damage. Moreover, our study found that HSP90 is not essential for protein stabilization of CSFV NS5A of the Flaviviridae family, but restricts CSFV infection by activating the type I IFN signaling pathway. It shares similarities with the involvement of HSP90 in the regulation of cellular and inflammatory factors during flavivirus infection (20). It suggests that the role of HSP90 in flavivirus infection may be specific. It remains relevant to explore the important mechanisms of HSP90 action during flavivirus infection.
The important role of molecular chaperone proteins like HSP70 and HSP90 in viral infections has been studied extensively and heat shock proteins are very conserved in evolution (62, 63). Therefore, some researchers have also suggested that inhibitors of the above two chaperone proteins can be used as broad-spectrum antiviral drugs (64, 65). However, the mechanisms of regulation of viral homeostasis and regulation of intracellular protein homeostasis by molecular chaperones are complex and even opposite for different viral infection processes. So, it is very necessary to investigate the mechanism of interaction between heat shock proteins and flavivirus proteins and the effects of the interaction on immune and inflammatory. It can provide new ideas to elucidate the molecular mechanism of viral infection and help to screening of broad-spectrum anti-flavivirus drugs.
Data availability statement
The datasets presented in this study can be found in online repositories. The names of the repository/repositories and accession number(s) can be found in the article/supplementary material.
Author contributions
CL, SF, JF, and JC conceived and designed the study. CL, MeZ, XLiu, FZ, LZo, ZZ, WZ, JS, and LZh performed the experiments. CL, SF, XLi, XC, YL, and JF analyzed the data. CL, SF, MiZ, LY, and JC wrote the manuscript. All authors read and agreed upon the final manuscript.
Funding
This work was supported by the Program of National Natural Science Foundation of China (No.321728243、No.32102643), the Science and Technology Program of Guangzhou, China, (No. 202206010161), the Key Research Projects of Universities in Guangdong Province (No.2019KZDXM026), Quality and Efficiency Improvement Project of South China Agricultural University(No.C18).
Conflict of interest
The authors declare that the research was conducted in the absence of any commercial or financial relationships that could be construed as a potential conflict of interest.
Publisher’s note
All claims expressed in this article are solely those of the authors and do not necessarily represent those of their affiliated organizations, or those of the publisher, the editors and the reviewers. Any product that may be evaluated in this article, or claim that may be made by its manufacturer, is not guaranteed or endorsed by the publisher.
References
1. Wei Q, Liu Y, Zhang G. Research progress and challenges in vaccine development against classical swine fever virus. Viruses (2021) 13(3):445. doi: 10.3390/v13030445
2. Edwards S, Fukusho A, Lefevre PC, Lipowski A, Pejsak Z, Roehe P, et al. Classical swine fever: The global situation. Veterinary Microbiol (2000) 73(2-3):103–19. doi: 10.1016/S0378-1135(00)00138-3
3. Liu C, Liu Y, Cheng Y, Zhang Y, Zhang J, Liang X, et al. The ESCRT-I subunit tsg101 plays novel dual roles in entry and replication of classical swine fever virus. J Virol (2021) 95(6):e01928–20. doi: 10.1128/JVI.01928-20
4. Ruggli N, Tratschin JD, Schweizer M, McCullough KC, Hofmann MA, Summerfield A. Classical swine fever virus interferes with cellular antiviral defense: Evidence for a novel function of n(pro). J Virol (2003) 77(13):7645–54. doi: 10.1128/JVI.77.13.7645-7654.2003
5. Tautz N, Tews BA, Meyers G. The molecular biology of pestiviruses. Adv Virus Res (2015) 93:47–160. doi: 10.1016/bs.aivir.2015.03.002
6. Elbers K, Tautz N, Becher P, Stoll D, Rümenapf T, Thiel HJ. Processing in the pestivirus E2-NS2 region: Identification of proteins p7 and E2p7. J Virol (1996) 70(6):4131–5. doi: 10.1128/jvi.70.6.4131-4135.1996
7. Ruggli N, Summerfield A, Fiebach AR, Guzylack-Piriou L, Bauhofer O, Lamm CG, et al. Classical swine fever virus can remain virulent after specific elimination of the interferon regulatory factor 3-degrading function of npro. J Virol (2009) 83(2):817–29. doi: 10.1128/JVI.01509-08
8. Reed KE, Gorbalenya AE, Rice CM. The NS5A/NS5 proteins of viruses from three genera of the family flaviviridae are phosphorylated by associated Serine/Threonine kinases. J Virol (1998) 72(7):6199–206. doi: 10.1128/JVI.72.7.6199-6206.1998
9. Sheng C, Zhu Z, Yu J, Wan L, Wang Y, Chen J, et al. Characterization of NS3, NS5A and NS5B of classical swine fever virus through mutation and complementation analysis. Veterinary Microbiol (2010) 140(1-2):72–80. doi: 10.1016/j.vetmic.2009.07.026
10. Liu X, Wang X, Wang Q, Luo M, Guo H, Gong W, et al. The eukaryotic translation initiation factor 3 subunit e binds to classical swine fever virus NS5A and facilitates viral replication. Virol (New York N.Y.) (2018) 515:11–20. doi: 10.1016/j.virol.2017.11.019
11. Okamoto T, Nishimura Y, Ichimura T, Suzuki K, Miyamura T, Suzuki T, et al. Hepatitis c virus RNA replication is regulated by FKBP8 and Hsp90. EMBO J (2006) 25(20):5015–25. doi: 10.1038/sj.emboj.7601367
12. Zhang L, Zhao D, Jin M, Song M, Liu S, Guo K, et al. Rab18 binds to classical swine fever virus NS5A and mediates viral replication and assembly in swine umbilical vein endothelial cells[J]. Virulence (2020) 11(1):489–501. doi: 10.1080/21505594.2020.1767356
13. Chengcheng Z, Fuxi Z, Mengjiao G, Baoyang R, Xuefeng W, Yantao W, et al. CSFV protein NS5A activates the unfolded protein response to promote viral replication. Virology (2020) 541:75–84. doi: 10.1016/j.virol.2019.12.006
14. Zhang C, Wang X, Sun J, Guo M, Zhang X, Wu Y. Autophagy induced by the n-terminus of the classic swine fever virus nonstructural protein 5A protein promotes viral replication. Front Microbiol (2021) 12:733385. doi: 10.3389/fmicb.2021.733385
15. Xie B, Zhao M, Song D, Wu K, Yi L, Li W, et al. Induction of autophagy and suppression of type I IFN secretion by CSFV. Autophagy (2021) 17(4):925–47. doi: 10.1080/15548627.2020.1739445
16. Dong XY, Tang SQ. Classical swine fever virus NS5A protein changed inflammatory cytokine secretion in porcine alveolar macrophages by inhibiting the NF-kappaB signaling pathway. Virol J (2016) 13:101. doi: 10.1186/s12985-016-0545-z
17. Latanova A, Starodubova E, Karpov V. Flaviviridae nonstructural proteins: The role in molecular mechanisms of triggering inflammation. Viruses (2022) 14(8):1808. doi: 10.3390/v14081808
18. Ye J, Chen Z, Li Y, Zhao Z, He W, Zohaib A, et al. Japanese Encephalitis virus NS5 inhibits type i interferon (IFN) production by blocking the nuclear translocation of IFN regulatory factor 3 and NF-κB. J Virol (2017) 91(8):e00039–17. doi: 10.1128/JVI.00039-17
19. Kumthip K, Chusri P, Jilg N, Zhao L, Fusco DN, Zhao H, et al. Hepatitis c virus NS5A disrupts STAT1 phosphorylation and suppresses type I interferon signaling. J Virol (2012) 86(16):8581–91. doi: 10.1128/JVI.00533-12
20. Roby JA, Esser-Nobis K, Dewey-Verstelle EC, Fairgrieve MR, Schwerk J, Lu AY, et al. Flavivirus nonstructural protein NS5 dysregulates HSP90 to broadly inhibit JAK/STAT signaling. Cells (2020) 9(4):899. doi: 10.3390/cells9040899
21. Wan Y, Cao W, Han T, Ren S, Feng J, Chen T, et al. Inducible Rubicon facilitates viral replication by antagonizing interferon production. Cell Mol Immunol (2017) 14(7):607–20. doi: 10.1038/cmi.2017.1
22. Yuan H, You J, You H, Zheng C. Herpes simplex virus 1 UL36USP antagonizes type i interferon-mediated antiviral innate immunity. J Virol (2018) 92(19):e01161–18. doi: 10.1128/JVI.01161-18
23. Ezeonwumelu IJ, Garcia-Vidal E, Ballana E. JAK-STAT pathway: A novel target to tackle viral infections. Viruses (2021) 13(12):2379. doi: 10.3390/v13122379
24. Schindler C, Levy DE, Decker T. JAK-STAT signaling: From interferons to cytokines. J Biol Chem (2007) 282(28):20059–63. doi: 10.1074/jbc.R700016200
25. Kessler DS, Veals SA, Fu X, Levy DE. Interferon-α regulates nuclear translocation and DNA-binding affinity of ISGF3, a multimeric transcriptional activator. Genes Dev (1990) 4(10):1753–65. doi: 10.1101/gad.4.10.1753
26. Rojas JM, Alejo A, Martin V, Sevilla N. Viral pathogen-induced mechanisms to antagonize mammalian interferon (IFN) signaling pathway. Cell Mol Life Sci (2021) 78(4):1423–44. doi: 10.1007/s00018-020-03671-z
27. Darnell JE. STATs and gene regulation. Science (1997) 277(5332):1630–5. doi: 10.1126/science.277.5332.1630
28. Nan Y, Wu C, Zhang Y. Interplay between janus Kinase/Signal transducer and activator of transcription signaling activated by type i interferons and viral antagonism. Front Immunol (2017) 8:1758. doi: 10.3389/fimmu.2017.01758
29. Zhu H, Zheng C. The race between host antiviral innate immunity and the immune evasion strategies of herpes simplex virus 1. Microbiol Mol Biol Rev (2020) 84(4):e00099–20. doi: 10.1128/MMBR.00099-20
30. Wang YX, Niklasch M, Liu T, Wang Y, Shi B, Yuan W, et al. Interferon-inducible MX2 is a host restriction factor of hepatitis b virus replication. J Hepatol (2020) 72(5):865–76. doi: 10.1016/j.jhep.2019.12.009
31. Zhou J, Chen J, Zhang XM, Gao Z, Liu C, Zhang Y, et al. Porcine mx1 protein inhibits classical swine fever virus replication by targeting nonstructural protein NS5B. J Virol (2018) 92(7):e02147–17. doi: 10.1128/JVI.02147-17
32. Li C, Wang Y, Zheng H, Dong W, Lv H, Lin J, et al. Antiviral activity of ISG15 against classical swine fever virus replication in porcine alveolar macrophages via inhibition of autophagy by ISGylating BECN1. Veterinary Res (2020) 51(1):22. doi: 10.1186/s13567-020-00753-5
33. Hayden MS, Ghosh S. Shared principles in NF-kappaB signaling. Cell (2008) 132(3):344–62. doi: 10.1016/j.cell.2008.01.020
34. Cai C, Tang Y, Zhai J, Zheng C. The RING finger protein family in health and disease. Signal transduction targeted Ther (2022) 7(1):1–23. doi: 10.1038/s41392-022-01152-2
35. Barnabei L, Laplantine E, Mbongo W, Rieux-Laucat F, Weil R. NF-kappaB: At the borders of autoimmunity and inflammation. Front Immunol (2021) 12:716469. doi: 10.3389/fimmu.2021.716469
36. Baeuerle PA, Baltimore D. IκB: A specific inhibitor of the NF-κB transcription factor. Science (1988) 242(4878):540–6. doi: 10.1126/science.3140380
37. Jensen S, Thomsen AR. Sensing of RNA viruses: A review of innate immune receptors involved in recognizing RNA virus invasion. J Virol (2012) 86(6):2900–10. doi: 10.1128/JVI.05738-11
38. Marino-Merlo F, Papaianni E, Frezza C, Pedatella S, De Nisco M, Macchi B, et al. NF-kappaB-Dependent production of ROS and restriction of HSV-1 infection in u937 monocytic cells. Viruses (2019) 11(5):428. doi: 10.3390/v11050428
39. Lu X, Chen Q, Liu H, Zhang X. Interplay between non-canonical NF-kappaB signaling and hepatitis b virus infection. Front Immunol (2021) 12:730684. doi: 10.3389/fimmu.2021.730684
40. Maloney A, Workman P. HSP90 as a new therapeutic target for cancer therapy: The story unfolds. Expert Opin On Biol Ther (2005) 2(1):3–24. doi: 10.1517/14712598.2.1.3
41. Serwetnyk MA, Blagg B. The disruption of protein-protein interactions with co-chaperones and client substrates as a strategy towards Hsp90 inhibition. Acta Pharm Sin B (2021) 11(6):1446–68. doi: 10.1016/j.apsb.2020.11.015
42. Saibil H. Chaperone machines for protein folding, unfolding and disaggregation. Nat Rev Mol Cell Biol (2013) 14(10):630–42. doi: 10.1038/nrm3658
43. Schopf FH, Biebl MM, Buchner J. The HSP90 chaperone machinery. Nat Rev Mol Cell Biol (2017) 18(6):345–60. doi: 10.1038/nrm.2017.20
44. Geller R, Taguwa S, Frydman J. Broad action of Hsp90 as a host chaperone required for viral replication. Biochim Biophys Acta (BBA) - Mol Cell Res (2012) 1823(3):698–706. doi: 10.1016/j.bbamcr.2011.11.007
45. Hu J. Hepadnavirus assembly and reverse transcription require a multi-component chaperone complex which is incorporated into nucleocapsids. EMBO J (1997) 16(1):59–68. doi: 10.1093/emboj/16.1.59
46. Hu J, Seeger C. Hsp90 is required for the activity of a hepatitis b virus reverse transcriptase. Proc Natl Acad Sci (1996) 93(3):1060–4. doi: 10.1073/pnas.93.3.1060
47. Joshi P, Maidji E, Stoddart CA. Inhibition of heat shock protein 90 prevents HIV rebound. J Biol Chem (2016) 291(19):10332–46. doi: 10.1074/jbc.M116.717538
48. Echeverría PC, Bernthaler A, Dupuis P, Mayer B, Picard D. An interaction network predicted from public data as a discovery tool: Application to the hsp90 molecular chaperone machine. PloS One (2011) 6(10):e26044. doi: 10.1371/journal.pone.0026044
49. Nie Y, Ran Y, Zhang HY, Huang ZF, Pan ZY, Wang SY, et al. GPATCH3 negatively regulates RLR-mediated innate antiviral responses by disrupting the assembly of VISA signalosome. PloS Pathog (2017) 13(4):e1006328. doi: 10.1371/journal.ppat.1006328
50. Goraya MU, Ziaghum F, Chen S, Raza A, Chen Y, Chi X. Role of innate immunity in pathophysiology of classical swine fever virus infection. Microb Pathog (2018) 119:248–54. doi: 10.1016/j.micpath.2018.04.020
51. Shang L, Tomasi TB. The heat shock protein 90-CDC37 chaperone complex is required for signaling by types I and II interferons. J Biol Chem (2006) 281(4):1876–84. doi: 10.1074/jbc.M509901200
52. Bocchini CE, Kasembeli MM, Roh SH, Tweardy DJ. Contribution of chaperones to STAT pathway signaling. JAKSTAT (2014) 3(3):e970459. doi: 10.4161/21623988.2014.970459
53. Schoof N, von Bonin F, Trumper L, Kube D. HSP90 is essential for jak-STAT signaling in classical Hodgkin lymphoma cells. Cell Communication Signaling (2009) 7:17. doi: 10.1186/1478-811X-7-17
54. Nollen EA, Morimoto RI. Chaperoning signaling pathways: Molecular chaperones as stress-sensing 'heat shock' proteins. J Cell Sci (2002) 115(Pt 14):2809–16. doi: 10.1242/jcs.115.14.2809
55. Liniger M, Gerber M, Renzullo S, García-Nicolás O, Ruggli N. TNF-mediated inhibition of classical swine fever virus replication is IRF1-, NF-κB- and JAK/STAT signaling-dependent. Viruses (2021) 13(10):2017. doi: 10.3390/v13102017
56. Grant A, Ponia SS, Tripathi S, Balasubramaniam V, Miorin L, Sourisseau M, et al. Zika virus targets human STAT2 to inhibit type i interferon signaling. Cell Host Microbe (2016) 19(6):882–90. doi: 10.1016/j.chom.2016.05.009
57. Best SM, Pierson TC. The many faces of the flavivirus NS5 protein in antagonism of type i interferon signaling. J Virol (2017) 91(3):e01970–16. doi: 10.1128/JVI.01970-16
58. Laurent-Rolle M, Boer EF, Lubick KJ, Wolfinbarger JB, Carmody AB, Rockx B, et al. The NS5 protein of the virulent West Nile virus NY99 strain is a potent antagonist of type I interferon-mediated JAK-STAT signaling. J Virol (2010) 84(7):3503–15. doi: 10.1128/JVI.01161-09
59. Balchin D, Hayer-Hartl M, Hartl FU. In vivo aspects of protein folding and quality control. Sci (American Assoc Advancement Science) (2016) 353(6294):42. doi: 10.1126/science.aac4354
60. Xu Y, Liu F, Liu J, Wang D, Yan Y, Ji S, et al. The co-chaperone Cdc37 regulates the rabies virus phosphoprotein stability by targeting to Hsp90AA1 machinery. Sci Rep (2016) 6(1):27123. doi: 10.1038/srep27123
61. Tsou Y, Lin Y, Chang H, Lin HY, Shao HY, Yu SL, et al. Heat shock protein 90: Role in enterovirus 71 entry and assembly and potential target for therapy. PloS One (2013) 8(10):e77133. doi: 10.1371/journal.pone.0077133
62. Lubkowska A, Pluta W, Strońska A, Lalko A. Role of heat shock proteins (HSP70 and HSP90) in viral infection. Int J Mol Sci (2021) 22(17):9366. doi: 10.3390/ijms22179366
63. Robert J. Evolution of heat shock protein and immunity. Dev Comp Immunol (2003) 27(6-7):449–64. doi: 10.1016/S0145-305X(02)00160-X
64. Taguwa S, Yeh M, Rainbolt TK, Nayak A, Shao H, Gestwicki JE, et al. Zika virus dependence on host Hsp70 provides a protective strategy against infection and disease. Cell Rep (Cambridge) (2019) 26(4):906–20. doi: 10.1016/j.celrep.2018.12.095
Keywords: CSFV, HSP90AA1, NS5A, JAK/STAT, NF-κB
Citation: Liu C, Zhao W, Su J, Chen X, Zhao F, Fan J, Li X, Liu X, Zou L, Zhang M, Zhang Z, Zhang L, Fan S, Li Y, Zhao M, Chen J and Yi L (2022) HSP90AA1 interacts with CSFV NS5A protein and regulates CSFV replication via the JAK/STAT and NF-κB signaling pathway. Front. Immunol. 13:1031868. doi: 10.3389/fimmu.2022.1031868
Received: 30 August 2022; Accepted: 10 October 2022;
Published: 02 November 2022.
Edited by:
Chenhe Su, Wistar Institute, United StatesReviewed by:
Yuexiu Zhang, The Ohio State University, United StatesGongguan Liu, Nanjing Agricultural University, China
Biao Qiu, NewYork-Presbyterian, United States
Copyright © 2022 Liu, Zhao, Su, Chen, Zhao, Fan, Li, Liu, Zou, Zhang, Zhang, Zhang, Fan, Li, Zhao, Chen and Yi. This is an open-access article distributed under the terms of the Creative Commons Attribution License (CC BY). The use, distribution or reproduction in other forums is permitted, provided the original author(s) and the copyright owner(s) are credited and that the original publication in this journal is cited, in accordance with accepted academic practice. No use, distribution or reproduction is permitted which does not comply with these terms.
*Correspondence: Lin Yi, yilin@scau.edu.cn; Jinding Chen, jdchen@scau.edu.cn