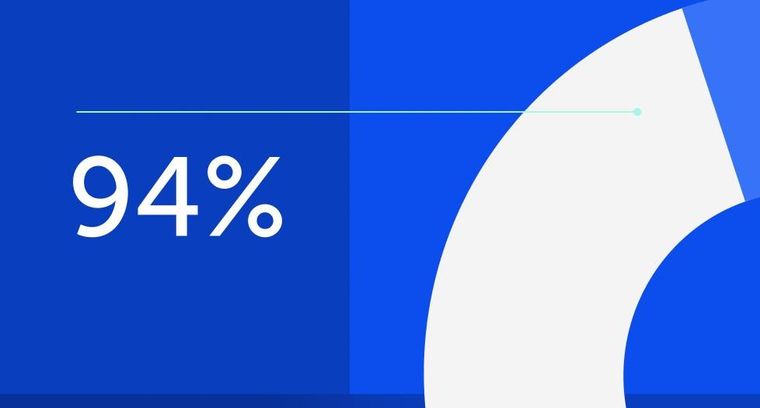
94% of researchers rate our articles as excellent or good
Learn more about the work of our research integrity team to safeguard the quality of each article we publish.
Find out more
REVIEW article
Front. Immunol., 28 October 2022
Sec. Cancer Immunity and Immunotherapy
Volume 13 - 2022 | https://doi.org/10.3389/fimmu.2022.1027385
This article is part of the Research TopicT-Cell Directed Therapies in Multiple MyelomaView all 9 articles
Despite advances in treatment for multiple myeloma, the majority of patients ultimately develop relapsed disease marked by immune evasion and resistance to standard therapy. Immunotherapy has emerged as a powerful tool for tumor-directed cytotoxicity with the unique potential to induce immune memory to reduce the risk of relapse. Understanding the specific mechanisms of immune dysregulation and dysfunction in advanced myeloma is critical to the development of further therapies that produce a durable response. Adoptive cellular therapy, most strikingly CAR T cell therapy, has demonstrated dramatic responses in the setting of refractory disease. Understanding the factors that contribute to immune evasion and the mechanisms of response and resistance to therapy will be critical to developing the next generation of adoptive cellular therapies, informing novel combination therapy, and determining the optimal time to incorporate immune therapy in the treatment of myeloma.
Multiple myeloma (MM) is a plasma cell malignancy characterized by clonal proliferation of terminally differentiated B cells in the context of an immunosuppressive milieu that permits immune escape (1, 2). There have been remarkable treatment advances for both front-line and relapsed and refractory (R/R) disease that have dramatically improved survival in recent years, including the use of anti-CD38 monoclonal antibody therapy, proteasome inhibitors, and immunomodulatory imide drugs. However, current therapies are not curative, with 5-year survival less than 60%, and most patients ultimately relapse with resistant disease (3–5). As such, a critical area of recent discovery lies in novel immunotherapeutic approaches to target the malignant plasma cell clone and overcome the immunosuppressive tumor microenvironment, which has the potential to treat previously refractory disease and create a more durable response to therapy. Here, we will review mechanisms of immune dysregulation in MM as well as current advances in immune and cellular immunotherapy, including adoptive cell transfer, T cell engagers, checkpoint inhibition, and vaccine therapy.
Immune dysfunction and evasion in myeloma is mediated by multiple cytokine and cellular signaling pathways which decrease immune effector cell function and create a suppressive bone marrow microenvironment. Immune dysregulation in the bone marrow is driven in part by soluble components such as transforming growth factor (TGF)-β, interleukin (IL)-10, IL-6, and prostaglandin E2, which are produced by the malignant plasma cell and by other suppressive cell populations in the tumor microenvironment, including regulatory T cells, myeloid derived suppressor cells (MDSCs), and bone marrow stromal cells (6). Additionally, upregulation of negative checkpoint pathways, including the PD-1/PD-L1 pathway, contributes to impaired T cell mediated killing of the malignant clone. PD-L1 is highly expressed on myeloma cells, and PD-1 has been shown to be upregulated on circulating T cells isolated from patients with advanced MM (7). These and other pathways drive T cell dysfunction, including impaired cytotoxic T lymphocyte activation, reduced CD4 T cells, increased T regulatory cells, and hallmarks of T cell exhaustion, which have been described even in monoclonal gammopathy of undetermined significance (MGUS) (6, 8, 9). Critical aspects underlying this process include T cell senescence and ineffective antigen presentation by tumor and dendritic cells (DCs) (9–12). Indeed, DCs isolated from MM patients are decreased in number and profoundly dysfunctional (6, 13). Factors contributing to DC dysfunction include IL-6–mediated inhibition, decreased expression of human leukocyte antigen (HLA), high levels of PD-L1 expression, and decreased expression of co-stimulatory molecules (6, 10, 11). Innate immunity is further impaired by suppression of NK cell function by MM and tumor evasion of NK cell surveillance (14). Corresponding changes in the surrounding microenvironment include increased presence of immunosuppressive cells such as regulatory T cells, regulatory B cells, MDSCs, tumor-associated macrophages (TAMs), and mesenchymal stem cells (MSCs) (6, 15, 16). In particular, increased presence of MDSCs has been associated with worse outcomes in MM (16–18).
As understanding of these complex interactions disrupting immune equilibrium has expanded, immunotherapy has emerged as a strategy to overcome the immunosuppressive tumor microenvironment and promote host anti-tumor immunity in myeloma (19). These efforts have focused on the activation and expansion of effector cells which can target and attack malignant cells as well as creation of a durable memory response to confer long term remission and protection against tumor relapse (20).
The potential role of immunotherapy for MM was initially established with the graft-versus-myeloma effect of allogeneic hematopoietic cell transplantation (allo-SCT). Allo-SCT has been shown to induce long-term remission and potential cure in a subset of MM patients, which is thought to be due to anti-myeloma activity of graft alloreactive lymphocytes (2, 21–23). The efficacy of this approach was further underscored by success of donor lymphocyte infusion (DLI) for patients who relapsed after allo-SCT (24). However, significant toxicities including infection, graft-versus-host disease (GVHD), and treatment-related mortality have limited its use, particularly with the introduction of newer induction and maintenance regimens in MM leading to improved response rates (25). To reduce treatment-related morbidity and mortality and increase the pool of allo-SCT eligible patients, non-myeloablative or reduced-intensity conditioning (RIC) allo-SCT has similarly been used (25–27); however, this strategy has been associated with increased risk of relapse, highlighting the importance of initial high-dose cytotoxic conditioning for durable response and remission (2, 25). High-dose chemotherapy with autologous transplant followed by RIC allo-SCT has been evaluated to potentially balance these two approaches, but studies have demonstrated some late relapses as well as unclear efficacy or reduced survival when compared with tandem autologous transplantation (2, 23, 25). While allo-SCT may still have a potentially curative role in a curated subset of patient (28), risks related to infection and GVHD remain significant. Nonetheless, the potency of the graft-versus-myeloma effect speaks to the unique potential for the immune system to overcome resistance, and fuels the field of immunotherapy which strives to more specifically target the malignant plasma cell clone.
Chimeric antigen receptor (CAR) T cell therapy has demonstrated dramatic response rates in patients with advanced myeloma, resulting in the FDA approval of two CAR T cell products for patients with R/R disease (idecabtagene vicleucel and ciltacabtagene autoloeucel). CAR T cells are generated by transducing autologous T cells with the CAR construct, consisting of an antigen binding site, generally a single variable chain targeting a tumor antigen expressed on the malignant clone, a costimulatory domain, and the ζ-chain of the T cell receptor (TCR) complex. Upon ligation, the receptor signals through the ζ-chain of the TCR complex as well as a costimulatory molecule, most commonly CD28 or 4-1BB, to induce CAR T expansion and anti-tumor immunity through targeted lysis of malignant cells (29, 30). The inflammatory potential, persistence, and toxicity of the CAR T construct are impacted by choice of costimulatory signaling (29, 30). Specifically, CD28 is associated with a terminal effector cell phenotype with more rapid expansion, robust cytokine production, and early-onset CRS, while 4-1BB pushes cells toward a memory phenotype with slowed expansion, but with increased persistence and reduced exhaustion (31–34). CAR T toxicity can include on-target/off-tumor effects on non-malignant cells expressing the antigen of interest. More commonly, toxicity is driven by dysregulated immune hyperactivation and cytokine production, including TNFα, IL-6, and IL-1α, which lead to cytokine release syndrome (CRS), a syndrome of systemic inflammation characteristically including fever and capillary leak, as well as neurotoxicity (34).
In MM, CAR T cells were initially studied targeting a variety of antigens, including CD19, CD138, light chains, NKG2D, and the Lewis Y antigen, with mixed results (35–40). Specifically, initial efforts to target a population of myeloma-propagating cells using an anti-CD19 CAR T product concurrently with autologous transplant significantly increased progression-free survival (PFS) in a small subset of responders (35, 36). However, the most robust and promising outcomes in patients with R/R disease have been shown with CAR constructs targeting B-cell maturation antigen (BCMA), with key trials highlighted in Table 1. BCMA is a member of the tumor necrosis factor superfamily expressed preferentially on mature B cells that is crucial for survival of plasma cells and has limited expression on hematopoietic stem cells or other tissues, making it an ideal therapeutic target (47, 48). An initial trial of 16 patients with R/R myeloma treated with an anti-BCMA CAR T clone using a CD28 costimulatory domain showed an overall response (OR) rate of 81%; however, this construct induced high rates of toxicity, with 94% of patients developing CRS and 38% grade 3 or above (41). To reduce toxicity, idecabtagene vicleucel (ide-cel) was developed with a 4-1BB costimulatory domain (42, 43). In a phase 2 study of 128 patients with R/R myeloma who had received at least 3 prior therapies, patients treated with ide-cel had an OR of 73%, complete response (CR) of 33%, and PFS of 8.8 months (43). While patients had high rates of CRS overall (84%), only 5% developed grade 3 or above CRS, and 18% of patients developed neurotoxicity including just 3% with grade 3 or higher reactions, suggesting a more tolerable toxicity profile. Notably, CAR T clones persisted for at least 12 months in 36% of patients, suggesting the potential for prolonged disease control (43).
To increase avidity of the CAR T clone, ciltacabtagene autoleucel (cilta-cel) was designed with two distinct BCMA-targeting antibodies as well as a 4-1BB costimulatory domain (44). In a multicenter phase 1/2 trial of 97 patients with R/R myeloma, cilta-cel showed an OR of 97% and CR of 67%. Significantly, 1-year overall survival (OS) was 89% and PFS 77%, with median duration of response not reached at median follow up of 12.4 months; further studies are ongoing for long term outcomes. The toxicity profile was similar to ide-cel, with high incidence of CRS (95%) but low rates of severe disease (4%), and similar rates of neurotoxicity (21% overall, 9% severe) (44). Finally, other targets have been identified including G-protein coupled receptor, class C group 5 member D (GPCR5D), a hair follicle protein that is upregulated in myeloma cells with a similar distribution to BCMA (49). Preliminary results from an ongoing phase 1 study of an anti-GPRC5D CAR OriCAR-017 (NCT05016778) were recently presented in 9 patients with R/R myeloma, including patients previously treated with anti-BCMA CAR T therapies. Initial data showed a 100% OR rate, with 100% incidence of CRS but 0 grade 3 events (45).
Despite their transformational role in treatment of R/R disease and the potential for durable remission, emergence of CAR T resistance remains a significant challenge. Multiple mechanisms of CAR T resistance have been described, including emergence of antigen-negative clones, immune clearance or poor survival of the CAR T cells, and CAR T cell exhaustion (50–53). In MM specifically, biallelic loss of BCMA expression has been shown in a subset of patients with recurrence after CAR T therapy (54, 55). One method to combat BCMA loss is the use of γ-secretase inhibitors to increase membrane BCMA expression. γ-secretase cleaves membrane-bound BCMA to allow shedding from plasma cells, and the resulting soluble BCMA can further limit CAR T cell recognition of myeloma cells (56, 57). A phase 1 study of the γ-secretase inhibitor JSMD194 in conjunction with anti-BCMA CAR T cells is currently underway (NCT03502577). Another strategy to reduce effects of antigenic loss on efficacy is development of CAR T cells simultaneously targeting multiple tumor antigens, such as BCMA with CD38 (58, 59), CS1 (60), or GPRC5D (49, 61, 62), as well as a novel approach of an anti-BCMA CAR with a CD38 chimeric costimulatory receptor to further drive lymphocyte activation (63), with continued studies ongoing.
Another significant hurdle in durable response to CAR therapy is limited survival of the CAR clone, and methods are under investigation to promote the persistence of the CAR T product, including optimizing manufacturing to increase selection of stem and central memory T cell subpopulations (64–66). As such, the bb21217 construct was created, which contains the same CAR design as ide-cel with addition of the PI3K inhibitor bb007, which increases the proportion of memory-type T cells (46). In preliminary data presented at the ASH 2021 national meeting from an ongoing phase 1 study (NCT03274219), 72 patients treated with bb21217 showed an OR rate of 69%, CR of 28%, and a median duration of response of 17 months. Toxicity was similar to ide-cel with a CRS incidence of 75% (4% grade ≥3) and neurotoxicity in 15% of patients (4% grade ≥3). Significantly, the CAR T clone was detectable in 81% and 60% of patients as 6 and 12 months, respectively (46). Additional methods to improve CAR T cell persistence and reduce functional exhaustion currently under development in preclinical models include the use of fully humanized variable chains to reduce immunogenicity and rejection of cells containing the CAR construct (67–70), combination costimulation with novel signaling pathways such as ICOS (71), cytokine manipulation to promote T cell activation and epitope spreading (72), combination therapy with immunomodulatory agents such as lenalidomide (73, 74), and blocking TGF-β responsiveness to inhibit tumor-induced immunosuppression (75).
Finally, underlying dysfunction of patient-derived T cells may limit clinical efficacy of the manufactured CAR T product. CAR T therapy is primarily used in patients with R/R disease, and autologous lymphocytes have therefore been exposed to the tumor’s immunosuppressive milieu as well as multiple lines of therapy prior to transducing the CAR construct (76). The composition of endogenous T cells used in manufacturing the CAR product, including the CD4:CD8 ratio and presence of central memory and stem cell memory T cells, has been shown to affect CAR T expansion, anti-tumor activity, and clinical response in myeloma and other B cell malignancies (77–79). One strategy to combat this is collection of T cells for CAR production at early stages of disease; in particular, one group showed T cells isolated from G-CSF-treated patients at the time of cell harvest for autologous stem cell transplant (SCT) showed appropriate expansion and anti-tumor activity in vitro and in mice (76).
In summary, the dramatic response rates observed following BMCA-directed CAR T cell therapy has added a vital tool to the treatment of patients with relapsed disease, and has had a meaningful impact on survival of patients with refractory myeloma. Limitations include lack of persistence of the CAR T cell population and the emergence of antigen negative variants. Strategies to overcome these mechanisms of resistance are a major focus of ongoing research and include the development of dual-targeting CARs and novel combinatorial approaches. In addition, the time needed to generate autologous CAR T cell products poses challenges to patients with aggressive advanced disease. Allogeneic CARs have the potential to overcome this limitation by providing an off the shelf product using allogeneic lymphocytes from healthy donors, as discussed below.
Given the success of autologous CAR constructs, allogeneic CAR T cells are being explored, with multiple possible advantages over autologous cells. Allogeneic products address multiple logistical challenges with manufacturing and quality and can be immediately available as an “off-the-shelf” option for therapeutic use in patients with unstable disease (80). Additionally, allogeneic CARs rely on functional T cells from healthy donors, which may avoid the challenges discussed above of dysfunctional T cells derived from patients with R/R disease. In preclinical studies, BCMA-targeted CAR T cells from healthy donors showed increased T cell expansion and memory populations, increased cytotoxicity in vitro, and decreased checkpoint marker expression compared with cells generated from patients with R/R disease (81). The primary drawback of this approach is concern for rejection limiting the persistence of the allogeneic CAR product as well as possible GVHD, and prior attempts to reduce off-target effects have included deletion of the native T cell receptor (TCR) or use of non-αβ T cells (80). An ongoing phase 1 trial (NCT04093596) for R/R myeloma treated with ALLO-715, an allogeneic anti-BCMA CAR T cell with a disrupted TCR and CD52 gene to reduce risk of GVHD, was presented at the ASH 2021 national meeting. 26 patients treated at higher cell doses had an OR rate of 62% at median follow up of 7.4 months with CRS incidence of 52% (2% grade 3) in the overall cohort (82), though expanded studies with further follow up are likely needed to assess the incidence and severity of GVHD. While allogeneic CAR T cells show promise as an off-the-shelf immunotherapeutic for patients with MM, the risks of GVHD, complexities of gene editing including the risk of genetic mutations, and the host immune clearance of the allogeneic CAR product limiting expansion and persistence in vivo remain challenges to be overcome.
Marrow-infiltrating lymphocytes (MILs) are T cells directly exposed to malignant cells in the marrow microenvironment. These cells home to and survive in the marrow and contain a high proportion of tumor-specific cytotoxic lymphocytes (83). In an initial trial in MM, MILs were harvested and expanded ex vivo and reinfused in 25 patients after autologous SCT (84). Patients showed an OR rate of 54% with 27% CR, with a significant increase in PFS and OS in the subset of patients with significant disease reduction (84). Further studies are underway of MILs in combination with other standard and vaccine-based therapies.
TCR-modified T cells (TCR T cells) are native T cells with edited TCRs to recognize a specific tumor antigen presented on the major histocompatibility complex (85). While CAR T cells exclusively bind cell surface proteins, TCR T cells allow for targeting of both intracellular and extracellular antigens. However, the major barrier to development is identification and generalizability of appropriate targets, as they are specific to each patient’s tumor antigens and HLA profile (85). In multiple myeloma, TCR T cells have been developed primarily targeting cancer testis antigens, such as NY-ESO-1, which is overexpressed in about one third of myeloma patients (86). A phase 1/2 trial of TCR T cells recognizing a shared peptide from NY-ESO-1 and the testis antigen LAGE-1 presented on HLA-A*0201 was administered to 20 patients after autologous SCT and showed an 80% response rate, 70% near CR at 100 days, estimated PFS of 19.1 months, and no evidence of CRS (87). Cytotoxicity in this model was shown to be further augmented in vitro with PD-1 blockade (88), and a phase 1 study of an NY-ESO-1/LAGE-1 T cell with CRISPR deletion of the native TCR and endogenous PD-1 gene showed initial feasibility (89). However, despite the lack of CRS, off-target toxicity of TCR T cells towards similar epitopes from other proteins as well as on-target/off-tumor toxicity directed against shared tumor antigens in other tissues remains a significant concern. Notably, an engineered affinity-enhanced HLA-A*01-restricted MAGE-A3-targeted T cell induced cardiogenic shock and death from myocardial infiltration in both treated patients in an initial study, likely due to cross-reactivity with epitopes from the native cardiac protein titin (90). While TCR T cells may represent a promising avenue to target an expanded set of intracellular antigens in a subset of patients with specific HLA phenotypes, further work is needed to appropriately identify effective targets while limiting alloreactivity towards normal tissues.
Adoptive NK cell therapy represents an additional promising avenue for anti-tumor immunity. NK cells possess multiple advantages over T cells, including cytotoxic activity without prior antigen exposure or HLA restriction and reduced risk of GVHD. In a phase 1 study, 12 myeloma patients received cord blood-derived NK cells in association with autologous SCT. The study showed an OR of 83% and near CR in 67% of the cohort, detectable NK cells in vivo at 6 months, and no evidence of GVHD (91), with a phase 2 study underway (NCT01729091). CRISPR-edited NK cells with reduced expression of the inhibitory KLRC1 locus showed improved anti-myeloma cytolytic activity in vitro, and further study is needed for the clinical utility of ex vivo NK cell engineering in myeloma (92). Additionally, CAR NK cells are being developed with modified targeted antibodies bound to NK cell signaling proteins. Further, NK cells from an allogeneic donor may allow for rapid production of an off-the-shelf CAR NK cell product, do not need to be individualized for each patient, and do not require CRISPR gene editing as do allogeneic CAR T cells in order to mitigate the risk of GVHD (93, 94). In murine models, CAR-NK cells targeting CD138 and CS-1 have showed some efficacy, with further preclinical work needed (95, 96).
Immune checkpoint blockade through the PD-1/PD-L1 or CTLA4 axes has had remarkable clinical efficacy in other malignancies in reducing immune dysregulation in the tumor microenvironment and re-activating native adaptive immunity. PD-L1 is highly expressed on malignant plasma cells and is associated with resistance to therapy and relapse, suggesting it may be an important pathway in mediating immune dysregulation (7, 97–99). However, pharmacologic PD-1 blockade in R/R myeloma did not show clinical benefit when used as monotherapy (100). Additionally, two phase 3 randomized trials of PD-1 blockade combined with immunomodulatory agents (lenalidomide/pomalidomide) were stopped prematurely due increased toxicity without a signal of improved efficacy, and there are ongoing concerns about the safety of checkpoint inhibitors in MM (101, 102). Further work is needed to define the potential therapeutic role of checkpoint inhibitors in myeloma, including in combination with other immune-based therapies such as vaccines (103, 104) and adoptive cell therapy (60, 105, 106).
To reduce immune evasion and dysregulation, bispecific T cell engagers (BiTEs) have been developed in multiple hematologic malignancies. These antibodies bind a specific tumor antigen as well as CD3 to induce colocalization of T cells with neoplastic cells in the context of T cell activation. BiTE antibodies utilize the native T cell repertoire and can induce a polyclonal response with expansion of memory populations; however, they are therefore dependent on native T cell quality and function, which may be impaired in the setting of malignancy (9).
In MM, BiTE development has centered on BCMA as a plasma cell marker. There are multiple CD3/BCMA constructs currently under investigation, with some early evidence of efficacy and durable response in phase 1 trials (107–112), and multiple phase 1/2 trials ongoing (NCT03145181, NCT03287908, NCT03933735, NCT03486067, NCT03761108). In a study of the AMG 420 compound in 42 patients with R/R disease, the OR rate was 70% with median response of 9 months in patients who received the target dose, though with 48% of patients developing grade 3 adverse events (107). A phase 1/2 trial of teclistamab in 165 patients showed an OR of 63%, CR of 39%, and median PFS of 11.3 months at median follow up of 14.1 months. Patients had a 72% incidence of CRS (0.6% grade ≥3) and 15% neurotoxicity (0 grade 3) (113). As noted above, γ-secretase inhibitors increase membrane BCMA expression, and they have been shown to augment the efficacy of an anti-BCMA BiTE in vitro, suggesting they may be effective as complementary agents (114). Multiple other BiTE targets are under investigation in preclinical and early clinical studies, including GPRC5D (NCT03399799) (115), FcRH5 (NCT03275103) (116), and CD38 (117). BiTEs represent a highly exciting therapy, as evidenced by promising response rates in the setting of advanced myeloma. Understanding how to best incorporate BiTE therapy earlier in the disease course and how to sequence various BCMA directed therapies is an area of ongoing investigation. Importantly, using anti-BCMA BiTEs as a partner in combination therapy, including with CAR T cells, immunomodulatory drugs, and vaccines, has the potential to further improve outcomes for patients with MM.
Cancer vaccine therapy utilizes peptide or cell-based methodologies to facilitate presentation of tumor-specific antigenic targets with the goal of activating and expanding cytotoxic T cells to target malignant cells. Vaccines have the potential to induce a polyclonal response to capture the heterogeneity of the tumor as well as induce a memory response to mitigate risk of disease relapse (118, 119). Challenges in vaccine development and clinical efficacy include identification of appropriate antigenic targets that are sufficiently immunogenic and specific to the malignant clone as well as effective presentation by antigen presenting cells in the context of costimulation (119).
Early efforts in immunization in myeloma have focused on peptide-based vaccine strategies targeting upregulated or aberrantly expressed proteins by the myeloma clone to induce tumor-specific immunity, with selected trials highlighted in Table 2. These vaccines have generally induced T cell and interferon responses but have not markedly improved clinical outcomes. An anti-MUC1 signal peptide vaccine, ImMucin, led to myeloma-specific immunity and at least disease stabilization in most patients (120). RHAMM-R3 peptide vaccination resulted in immunologic and biochemical responses in patients with myeloma overexpressing RHAMM (121, 122). Vaccination against the anti-apoptotic Bcl-2 family proteins generated immune responses in relapsed myeloma patients in a phase 1 clinical trial (123). Idiotypic DNA vaccines targeting the myeloma-specific immunoglobulin were also noted to engender immune responses in a phase 1 clinical trial (124). A recent phase 2 randomized study of an idiotype vaccine paired with adoptive transfer of ex-vivo expanded, vaccine-specific autologous T cells showed increased markers of functional immune activation and memory generation, though without significant difference in CR or 3-year PFS (125). Additionally, to decrease the risk of off-target toxicity, cancer-testis antigens such as NY-ESO-1 and MAGE-A3, proteins normally restricted to fetal development or germ cells but aberrantly re-expressed in malignant cells, have been targeted, with immunization leading to durable immune responses in proof-of-concept studies (129, 130).
Despite some immune efficacy noted above, there are multiple major limitations of antigen peptide-based vaccination, including potential resistance by antigenic escape and downregulation of target antigens. To combat this, multiepitope vaccines have been trialed, and one such strategy targeting XBP1, CD138, and CS1 peptides in smoldering myeloma induced a robust myeloma-specific immune response in vitro (131). In a nonrandomized clinical trial, patients demonstrated a vaccine-specific T cell response that was further enhanced when administered in conjunction with lenalidomide, though with few significant clinical responses (126). Another concern with peptide vaccines is limited immunogenicity of self-antigens despite overexpression by the tumor given thymic deletion of T cells with high affinity for these antigens during development (132). As a note, this decreased activity of the native T cell repertoire in response to self-antigens is likely why vaccination against cancer testis antigens NY-ESO-1 or MAGE-A3 is well tolerated, while infusion of ex-vivo engineered TCR T cells targeting these same antigens led to significant alloreactivity and toxicity, as discussed above. Alternatively, novel protein sequences and foreign antigens created by tumor-specific mutations, or neoantigens, may induce robust T cell recruitment and anti-tumor immune response. Interestingly, neoantigen burden in myeloma has been found to correlate with inferior survival and associated upregulation of T-cell suppression pathway genes, creating an opportunity for neoantigen-based vaccination as a vehicle for activation of suppressed T cells and host anti-tumor immunity (133–135). While neoantigens may be patient or tumor-specific, shared neoantigens can be identified from common oncogenic driver mutations that can be feasibly targeted by vaccines. In myeloma, common shared neoantigens such as PKD1, PRKDC, and NRAS are being further characterized in the laboratory, and in preclinical studies, a neoantigen-based vaccine targeting MOPC315 has been shown to induce anti-tumor immunity (136, 137). Further research is needed in neoantigen identification and immunogenicity to determine the potential of neoantigen-based vaccines as a therapeutic strategy in myeloma.
In addition to selecting an optimal tumor-specific antigen, promoting a method of antigen uptake and presentation is key in inducing robust and durable anti-tumor immunity and mitigating the effects of immune tolerance within the tumor microenvironment. As such, a variety of DC-based vaccines strategies have been developed to ensure antigen presentation in the context of DC-mediated costimulation. In clinical trials, these strategies most commonly involve reinfusion of ex vivo DCs pulsed with tumor antigens, tumor cell lysate, or apoptotic bodies, with the goal of DC loading with varied tumor antigens to reduce risk of antigenic escape (138, 139). Idiotype-pulsed DCs were associated with prolonged survival following autologous SCT (127). Additionally, a study using DCs loaded with irradiated autologous MM cells was well tolerated and resulted in immune responses and disease stabilization in a phase 1 trial of patients with R/R myeloma (128).
An additional approach is manipulation of patient-derived tumor cells to express GM-CSF, thereby promoting DC migration to the site of vaccination. This strategy, also known as GVAX, was initially applied in acute myeloid leukemia (140, 141). The GVAX platform has been employed in combination with lenalidomide in myeloma patients in near complete remission. In a proof-of-principle study, over 50% of treated patients reached CR, with median PFS not yet reached at median follow up of 5 years and with persistent immunologic responses in all patients (142).
Our group has developed a personalized vaccine strategy wherein autologous DCs are fused with patient-derived tumor cells, which creates a hybridoma expressing a wide variety of tumor-specific neoantigens in the context of increased costimulation. In a phase 1 trial evaluating this DC/myeloma fusion vaccine, vaccination was well tolerated and resulted in expansion of CD4 and CD8 myeloma-reactive T cells and disease stabilization in the majority of patients (143). In a subsequent phase 2 trial following autologous SCT, vaccination significantly increased CD4 and CD8 myeloma-specific T cells, with a CR rate of 47% and a 2-year PFS of 57% (144). In light of these encouraging results, a randomized multicenter trial of the DC/myeloma fusion vaccine versus lenalidomide maintenance alone after autologous SCT is currently in progress (NCT02728102).
Immune-based therapies have demonstrated exciting results in patients with MM. Ongoing areas of research focus remain understanding and overcoming mechanisms of resistance, optimizing combinatorial approaches, and identifying biomarkers of resistance and response. The potency of CAR T cell therapy has been demonstrated in the setting of advanced disease, and it is currently being investigated in the setting of high-risk disease in the newly diagnosed setting. In newly diagnosed disease, the current treatment landscape for myeloma is such that with immunomodulatory agents, proteasome inhibitors, and CD38-targeting antibodies, one can achieve deep responses. Incorporating immune based therapy has the potential to eradicate minimal residual disease to promote immune surveillance and protect from relapse. Understanding and addressing mechanisms of resistance and biomarkers of response will be critical toward designing the appropriate combinatorial approach, choosing the optimal sequence of therapy, and ultimately, developing a curative therapeutic approach to the treatment of MM.
All authors listed have made a substantial, direct, and intellectual contribution to the work and approved it for publication.
Author JR has the following conflicts of interest: Attivare Therapeutics- consultant; Bioclinica- consultant; Parexelconsultant Imaging endpoints- consultant: BMS- advisory board, research funding; Karyopharm-DSMB; Celgene - research funding; Sanofi - research funding.
The remaining authors declare that the research was conducted in the absence of any commercial or financial relationships that could be construed as a potential conflict of interest.
All claims expressed in this article are solely those of the authors and do not necessarily represent those of their affiliated organizations, or those of the publisher, the editors and the reviewers. Any product that may be evaluated in this article, or claim that may be made by its manufacturer, is not guaranteed or endorsed by the publisher.
1. Boussi L, Niesvizky R. Advances in immunotherapy in multiple myeloma. Curr Opin Oncol (2017) 29(6):460–6. doi: 10.1097/CCO.0000000000000407
2. Rosenblatt J, Avigan D. Cellular immunotherapy for multiple myeloma. Cancer J (2019) 25(1):38–44. doi: 10.1097/PPO.0000000000000356
3. Robak P, Drozdz I, Szemraj J, Robak T. Drug resistance in multiple myeloma. Cancer Treat Rev (2018) 70:199–208. doi: 10.1016/j.ctrv.2018.09.001
4. Costa LJ, Brill IK, Omel J, Godby K, Kumar SK, Brown EE. Recent trends in multiple myeloma incidence and survival by age, race, and ethnicity in the united states. Blood Adv (2017) 1(4):282–7. doi: 10.1182/bloodadvances.2016002493
5. Surveillance, Epidemiology, and End Results (SEER) Program. SEER*Stat database: Cancer stat facts: Myeloma. National Cancer Institute (2022). https://seer.cancer.gov/statfacts/html/mulmy.html.
6. Tamura H. Immunopathogenesis and immunotherapy of multiple myeloma. Int J Hematol (2018) 107(3):278–85. doi: 10.1007/s12185-018-2405-7
7. Rosenblatt J, Avigan D. Targeting the PD-1/PD-L1 axis in multiple myeloma: a dream or a reality? Blood (2017) 129(3):275–9. doi: 10.1182/blood-2016-08-731885
8. Bailur JK, McCachren SS, Doxie DB, Shrestha M, Pendleton K, Nooka AK, et al. Early alterations in stem-like/resident T cells, innate and myeloid cells in the bone marrow in preneoplastic gammopathy. JCI Insight (2019) 5(11): e127807. doi: 10.1172/jci.insight.127807
9. Cohen AD, Raje N, Fowler JA, Mezzi K, Scott EC, Dhodapkar MV. How to train your T cells: Overcoming immune dysfunction in multiple myeloma. Clin Cancer Res (2020) 26(7):1541–54. doi: 10.1158/1078-0432.CCR-19-2111
10. Brimnes MK, Svane IM, Johnsen HE. Impaired functionality and phenotypic profile of dendritic cells from patients with multiple myeloma. Clin Exp Immunol (2006) 144(1):76–84. doi: 10.1111/j.1365-2249.2006.03037.x
11. Ratta M, Fagnoni F, Curti A, Vescovini R, Sansoni P, Oliviero B, et al. Dendritic cells are functionally defective in multiple myeloma: the role of interleukin-6. Blood (2002) 100(1):230–7. doi: 10.1182/blood.v100.1.230
12. Suen H, Brown R, Yang S, Weatherburn C, Ho PJ, Woodland N, et al. Multiple myeloma causes clonal T-cell immunosenescence: identification of potential novel targets for promoting tumour immunity and implications for checkpoint blockade. Leukemia (2016) 30(8):1716–24. doi: 10.1038/leu.2016.84
13. Pessoa de Magalhães RJ, Vidriales MB, Paiva B, Fernandez-Gimenez C, García-Sanz R, Mateos MV, et al. Analysis of the immune system of multiple myeloma patients achieving long-term disease control by multidimensional flow cytometry. Haematologica (2013) 98(1):79–86. doi: 10.3324/haematol.2012.067272
14. Clara JA, Childs RW. Harnessing natural killer cells for the treatment of multiple myeloma. Semin Oncol (2022) 49(1):69–85. doi: 10.1053/j.seminoncol.2022.01.004
15. André T, Najar M, Stamatopoulos B, Pieters K, Pradier O, Bron D, et al. Immune impairments in multiple myeloma bone marrow mesenchymal stromal cells. Cancer Immunol Immunother. (2015) 64(2):213–24. doi: 10.1007/s00262-014-1623-y
16. Ramachandran IR, Martner A, Pisklakova A, Condamine T, Chase T, Vogl T, et al. Myeloid-derived suppressor cells regulate growth of multiple myeloma by inhibiting T cells in bone marrow. J Immunol (2013) 190(7):3815–23. doi: 10.4049/jimmunol.1203373
17. Lv M, Wang K, Huang XJ. Myeloid-derived suppressor cells in hematological malignancies: friends or foes. J Hematol Oncol (2019) 12(1):105. doi: 10.1186/s13045-019-0797-3
18. Veglia F, Perego M, Gabrilovich D. Myeloid-derived suppressor cells coming of age. Nat Immunol (2018) 19(2):108–19. doi: 10.1038/s41590-017-0022-x
19. Ohmine K, Uchibori R. Novel immunotherapies in multiple myeloma. Int J Hematol (2022) 115(6):799–810. doi: 10.1007/s12185-022-03365-1
20. Dhodapkar MV, Dhodapkar KM. Immune modulation in hematologic malignancies. Semin Oncol (2015) 42(4):617–25. doi: 10.1053/j.seminoncol.2015.05.009
21. Bird JM, Russell NH, Samson D. Minimal residual disease after bone marrow transplantation for multiple myeloma: evidence for cure in long-term survivors. Bone Marrow Transplant. (1993) 12(6):651–4.
22. Tarantolo S, Bierman PJ. Allogeneic transplantation for the treatment of multiple myeloma. In: Laughlin MJ, Lazarus HM, editors. Allogeneic stem cell transplantation: Clinical research and practice. Totowa, NJ: Humana Press (2003). p. 69–81.
23. Greil C, Engelhardt M, Finke J, Wäsch R. Allogeneic stem cell transplantation in multiple myeloma. Cancers (Basel). (2021) 14(1): 55. doi: 10.3390/cancers14010055
24. Lokhorst HM, Schattenberg A, Cornelissen JJ, van Oers MH, Fibbe W, Russell I, et al. Donor lymphocyte infusions for relapsed multiple myeloma after allogeneic stem-cell transplantation: predictive factors for response and long-term outcome. J Clin Oncol (2000) 18(16):3031–7. doi: 10.1200/JCO.2000.18.16.3031
25. Dhakal B, Vesole DH, Hari PN. Allogeneic stem cell transplantation for multiple myeloma: is there a future? Bone Marrow Transplant (2016) 51(4):492–500. doi: 10.1038/bmt.2015.325
26. Giralt S, Aleman A, Anagnostopoulos A, Weber D, Khouri I, Anderlini P, et al. Fludarabine/melphalan conditioning for allogeneic transplantation in patients with multiple myeloma. Bone Marrow Transplant. (2002) 30(6):367–73. doi: 10.1038/sj.bmt.1703652
27. Kröger N, Schwerdtfeger R, Kiehl M, Sayer HG, Renges H, Zabelina T, et al. Autologous stem cell transplantation followed by a dose-reduced allograft induces high complete remission rate in multiple myeloma. Blood (2002) 100(3):755–60. doi: 10.1182/blood-2002-01-0131
28. Greil C, Engelhardt M, Ihorst G, Schoeller K, Bertz H, Marks R, et al. Allogeneic transplantation of multiple myeloma patients may allow long-term survival in carefully selected patients with acceptable toxicity and preserved quality of life. Haematologica (2019) 104(2):370–9. doi: 10.3324/haematol.2018.200881
29. Feins S, Kong W, Williams EF, Milone MC, Fraietta JA. An introduction to chimeric antigen receptor (CAR) T-cell immunotherapy for human cancer. Am J Hematol (2019) 94(S1):S3–s9. doi: 10.1002/ajh.25418
30. Jayaraman J, Mellody MP, Hou AJ, Desai RP, Fung AW, Pham AHT, et al. CAR-T design: Elements and their synergistic function. EBioMedicine (2020) 58:102931. doi: 10.1016/j.ebiom.2020.102931
31. Kawalekar OU, O'Connor RS, Fraietta JA, Guo L, McGettigan SE, Posey AD Jr., et al. Distinct signaling of coreceptors regulates specific metabolism pathways and impacts memory development in CAR T cells. Immunity (2016) 44(2):380–90. doi: 10.1016/j.immuni.2016.01.021
32. Salter AI, Ivey RG, Kennedy JJ, Voillet V, Rajan A, Alderman EJ, et al. Phosphoproteomic analysis of chimeric antigen receptor signaling reveals kinetic and quantitative differences that affect cell function. Sci Signal (2018) 11(544): eaat6753. doi: 10.1126/scisignal.aat6753
33. Long AH, Haso WM, Shern JF, Wanhainen KM, Murgai M, Ingaramo M, et al. 4-1BB costimulation ameliorates T cell exhaustion induced by tonic signaling of chimeric antigen receptors. Nat Med (2015) 21(6):581–90. doi: 10.1038/nm.3838
34. Brudno JN, Kochenderfer JN. Recent advances in CAR T-cell toxicity: Mechanisms, manifestations and management. Blood Rev (2019) 34:45–55. doi: 10.1016/j.blre.2018.11.002
35. Garfall AL, Maus MV, Hwang WT, Lacey SF, Mahnke YD, Melenhorst JJ, et al. Chimeric antigen receptor T cells against CD19 for multiple myeloma. N Engl J Med (2015) 373(11):1040–7. doi: 10.1056/NEJMoa1504542
36. Garfall AL, Stadtmauer EA, Hwang WT, Lacey SF, Melenhorst JJ, Krevvata M, et al. Anti-CD19 CAR T cells with high-dose melphalan and autologous stem cell transplantation for refractory multiple myeloma. JCI Insight (2018) 3(8):e120505. doi: 10.1172/jci.insight.120505
37. Peinert S, Prince HM, Guru PM, Kershaw MH, Smyth MJ, Trapani JA, et al. Gene-modified T cells as immunotherapy for multiple myeloma and acute myeloid leukemia expressing the Lewis y antigen. Gene Ther (2010) 17(5):678–86. doi: 10.1038/gt.2010.21
38. Guo B, Chen M, Han Q, Hui F, Dai H, Zhang W, et al. CD138-directed adoptive immunotherapy of chimeric antigen receptor (CAR)-modified T cells for multiple myeloma. J Cell Immunother (2016) 2(1):28–35. doi: 10.1016/j.jocit.2014.11.001
39. Ramos CA, Savoldo B, Torrano V, Ballard B, Zhang H, Dakhova O, et al. Clinical responses with T lymphocytes targeting malignancy-associated κ light chains. J Clin Invest. (2016) 126(7):2588–96. doi: 10.1172/JCI86000
40. Baumeister SH, Murad J, Werner L, Daley H, Trebeden-Negre H, Gicobi JK, et al. Phase I trial of autologous CAR T cells targeting NKG2D ligands in patients with AML/MDS and multiple myeloma. Cancer Immunol Res (2019) 7(1):100–12. doi: 10.1158/2326-6066.CIR-18-0307
41. Brudno JN, Maric I, Hartman SD, Rose JJ, Wang M, Lam N, et al. T Cells genetically modified to express an anti-B-Cell maturation antigen chimeric antigen receptor cause remissions of poor-prognosis relapsed multiple myeloma. J Clin Oncol (2018) 36(22):2267–80. doi: 10.1200/JCO.2018.77.8084
42. Raje N, Berdeja J, Lin Y, Siegel D, Jagannath S, Madduri D, et al. Anti-BCMA CAR T-cell therapy bb2121 in relapsed or refractory multiple myeloma. N Engl J Med (2019) 380(18):1726–37. doi: 10.1056/NEJMoa1817226
43. Munshi NC, Anderson LD Jr., Shah N, Madduri D, Berdeja J, Lonial S, et al. Idecabtagene vicleucel in relapsed and refractory multiple myeloma. N Engl J Med (2021) 384(8):705–16. doi: 10.1056/NEJMoa2024850
44. Berdeja JG, Madduri D, Usmani SZ, Jakubowiak A, Agha M, Cohen AD, et al. Ciltacabtagene autoleucel, a b-cell maturation antigen-directed chimeric antigen receptor T-cell therapy in patients with relapsed or refractory multiple myeloma (CARTITUDE-1): A phase 1b/2 open-label study. Lancet (2021) 398(10297):314–24. doi: 10.1016/S0140-6736(21)00933-8
45. Huang H, Hu Y, Zhang M, Ding X, Tang Y, He X, et al. Phase I open-label single arm study of GPRC5D CAR T-cells (OriCAR-017) in patients with relapsed/refractory multiple myeloma (POLARIS). J Clin Oncol (2022) 40(16_suppl):8004. doi: 10.1200/JCO.2022.40.16_suppl.8004
46. Raje NS, Shah N, Jagannath S, Kaufman JL, Siegel DS, Munshi NC, et al. Updated clinical and correlative results from the phase I CRB-402 study of the BCMA-targeted CAR T cell therapy bb21217 in patients with relapsed and refractory multiple myeloma. Blood (2021) 138(Supplement 1):548. doi: 10.1182/blood-2021-146518
47. Shah N, Chari A, Scott E, Mezzi K, Usmani SZ. B-cell maturation antigen (BCMA) in multiple myeloma: rationale for targeting and current therapeutic approaches. Leukemia (2020) 34(4):985–1005. doi: 10.1038/s41375-020-0734-z
48. O'Connor BP, Raman VS, Erickson LD, Cook WJ, Weaver LK, Ahonen C, et al. BCMA is essential for the survival of long-lived bone marrow plasma cells. J Exp Med (2004) 199(1):91–8. doi: 10.1084/jem.20031330
49. Smith EL, Harrington K, Staehr M, Masakayan R, Jones J, Long TJ, et al. GPRC5D is a target for the immunotherapy of multiple myeloma with rationally designed CAR T cells. Sci Transl Med (2019) 11(485): eaau7746. doi: 10.1126/scitranslmed.aau7746
50. Shah NN, Fry TJ. Mechanisms of resistance to CAR T cell therapy. Nat Rev Clin Oncol (2019) 16(6):372–85. doi: 10.1038/s41571-019-0184-6
51. Cheng J, Zhao L, Zhang Y, Qin Y, Guan Y, Zhang T, et al. Understanding the mechanisms of resistance to CAR T-cell therapy in malignancies. Front Oncol (2019) 9:1237. doi: 10.3389/fonc.2019.01237
52. van de Donk N, Themeli M, Usmani SZ. Determinants of response and mechanisms of resistance of CAR T-cell therapy in multiple myeloma. Blood Cancer Discovery (2021) 2(4):302–18. doi: 10.1158/2643-3230.BCD-20-0227
53. Rodríguez-Lobato LG, Ganzetti M, Fernández de Larrea C, Hudecek M, Einsele H, Danhof S. CAR T-cells in multiple myeloma: State of the art and future directions. Front Oncol (2020) 10:1243. doi: 10.3389/fonc.2020.01243
54. Samur MK, Fulciniti M, Aktas Samur A, Bazarbachi AH, Tai Y-T, Prabhala R, et al. Biallelic loss of BCMA as a resistance mechanism to CAR T cell therapy in a patient with multiple myeloma. Nat Commun (2021) 12(1):868. doi: 10.1038/s41467-021-21177-5
55. Da Vià MC, Dietrich O, Truger M, Arampatzi P, Duell J, Heidemeier A, et al. Homozygous BCMA gene deletion in response to anti-BCMA CAR T cells in a patient with multiple myeloma. Nat Med (2021) 27(4):616–9. doi: 10.1038/s41591-021-01245-5
56. Laurent SA, Hoffmann FS, Kuhn PH, Cheng Q, Chu Y, Schmidt-Supprian M, et al. γ-secretase directly sheds the survival receptor BCMA from plasma cells. Nat Commun (2015) 6:7333. doi: 10.1038/ncomms8333
57. Pont MJ, Hill T, Cole GO, Abbott JJ, Kelliher J, Salter AI, et al. γ-secretase inhibition increases efficacy of BCMA-specific chimeric antigen receptor T cells in multiple myeloma. Blood (2019) 134(19):1585–97. doi: 10.1182/blood.2019000050
58. Mei H, Li C, Jiang H, Zhao X, Huang Z, Jin D, et al. A bispecific CAR-T cell therapy targeting BCMA and CD38 in relapsed or refractory multiple myeloma. J Hematol Oncol (2021) 14(1):161. doi: 10.1186/s13045-021-01170-7
59. Li C, Mei H, Hu Y, Guo T, Liu L, Jiang H, et al. A bispecific CAR-T cell therapy targeting bcma and CD38 for relapsed/refractory multiple myeloma: updated results from a phase 1 dose-climbing trial. Blood (2019) 134:930. doi: 10.1182/blood-2019-130340
60. Zah E, Nam E, Bhuvan V, Tran U, Ji BY, Gosliner SB, et al. Systematically optimized BCMA/CS1 bispecific CAR-T cells robustly control heterogeneous multiple myeloma. Nat Commun (2020) 11(1):2283. doi: 10.1038/s41467-020-16160-5
61. Mailankody S, Diamonte C, Fitzgerald L, Kane P, Wang X, Sikder DS, et al. Phase I first-in-Class trial of MCARH109, a G protein coupled receptor class c group 5 member d (GPRC5D) targeted CAR T cell therapy in patients with relapsed or refractory multiple myeloma. Blood (2021) 138:827. doi: 10.1182/blood-2021-153204
62. Fernández de Larrea C, Staehr M, Lopez AV, Ng KY, Chen Y, Godfrey WD, et al. Defining an optimal dual-targeted CAR T-cell therapy approach simultaneously targeting BCMA and GPRC5D to prevent BCMA escape-driven relapse in multiple myeloma. Blood Cancer Discovery (2020) 1(2):146–54. doi: 10.1158/2643-3230.BCD-20-0020
63. Katsarou A, Sjöstrand M, Naik J, Mansilla-Soto J, Kefala D, Kladis G, et al. Combining a CAR and a chimeric costimulatory receptor enhances T cell sensitivity to low antigen density and promotes persistence. Sci Transl Med (2021) 13(623):eabh1962. doi: 10.1126/scitranslmed.abh1962
64. Sommermeyer D, Hudecek M, Kosasih PL, Gogishvili T, Maloney DG, Turtle CJ, et al. Chimeric antigen receptor-modified T cells derived from defined CD8+ and CD4+ subsets confer superior antitumor reactivity in vivo. Leukemia (2016) 30(2):492–500. doi: 10.1038/leu.2015.247
65. Biasco L, Izotova N, Rivat C, Ghorashian S, Richardson R, Guvenel A, et al. Clonal expansion of T memory stem cells determines early anti-leukemic responses and long-term CAR T cell persistence in patients. Nat Cancer. (2021) 2(6):629–42. doi: 10.1038/s43018-021-00207-7
66. Dancy E, Garfall AL, Cohen AD, Fraietta JA, Davis M, Levine BL, et al. Clinical predictors of T cell fitness for CAR T cell manufacturing and efficacy in multiple myeloma. Blood (2018) 132:1886. doi: 10.1182/blood-2018-99-115319
67. Smith EL, Staehr M, Masakayan R, Tatake IJ, Purdon TJ, Wang X, et al. Development and evaluation of an optimal human single-chain variable fragment-derived BCMA-targeted CAR T cell vector. Mol Ther (2018) 26(6):1447–56. doi: 10.1016/j.ymthe.2018.03.016
68. Mikkilineni L, Manasanch EE, Vanasse D, Brudno JN, Mann J, Sherry R, et al. Deep and durable remissions of relapsed multiple myeloma on a first-in-Humans clinical trial of T cells expressing an anti-B-Cell maturation antigen (BCMA) chimeric antigen receptor (CAR) with a fully-human heavy-Chain-Only antigen recognition domain. Blood (2020) 136:50–1. doi: 10.1182/blood-2020-138839
69. Wang D, Wang J, Hu G, Wang W, Xiao Y, Cai H, et al. A phase 1 study of a novel fully human BCMA-targeting CAR (CT103A) in patients with relapsed/refractory multiple myeloma. Blood (2021) 137(21):2890–901. doi: 10.1182/blood.2020008936
70. Green DJ, Pont M, Sather BD, Cowan AJ, Turtle CJ, Till BG, et al. Fully human bcma targeted chimeric antigen receptor T cells administered in a defined composition demonstrate potency at low doses in advanced stage high risk multiple myeloma. Blood (2018) 132(Supplement 1):1011. doi: 10.1182/blood-2018-99-117729
71. Guedan S, Posey AD Jr., Shaw C, Wing A, Da T, Patel PR, et al. Enhancing CAR T cell persistence through ICOS and 4-1BB costimulation. JCI Insight (2018) 3(1): e96976. doi: 10.1172/jci.insight.96976
72. Yeku OO, Brentjens RJ. Armored CAR T-cells: utilizing cytokines and pro-inflammatory ligands to enhance CAR T-cell anti-tumour efficacy. Biochem Soc Trans (2016) 44(2):412–8. doi: 10.1042/BST20150291
73. Works M, Soni N, Hauskins C, Sierra C, Baturevych A, Jones JC, et al. Anti-b-cell maturation antigen chimeric antigen receptor T cell function against multiple myeloma is enhanced in the presence of lenalidomide. Mol Cancer Ther (2019) 18(12):2246–57. doi: 10.1158/1535-7163.MCT-18-1146
74. Wang X, Walter M, Urak R, Weng L, Huynh C, Lim L, et al. Lenalidomide enhances the function of CS1 chimeric antigen receptor-redirected T cells against multiple myeloma. Clin Cancer Res (2018) 24(1):106–19. doi: 10.1158/1078-0432.CCR-17-0344
75. Kloss CC, Lee J, Zhang A, Chen F, Melenhorst JJ, Lacey SF, et al. Dominant-negative TGF-β receptor enhances PSMA-targeted human CAR T cell proliferation and augments prostate cancer eradication. Mol Ther (2018) 26(7):1855–66. doi: 10.1016/j.ymthe.2018.05.003
76. Battram AM, Oliver-Caldés A, Suárez-Lledó M, Lozano M, Bosch ICM, Martínez-Cibrián N, et al. T Cells isolated from G-CSF-treated multiple myeloma patients are suitable for the generation of BCMA-directed CAR-T cells. Mol Ther Methods Clin Dev (2022) 26:207–23. doi: 10.1016/j.omtm.2022.06.010
77. Cohen AD, Garfall AL, Stadtmauer EA, Melenhorst JJ, Lacey SF, Lancaster E, et al. B cell maturation antigen-specific CAR T cells are clinically active in multiple myeloma. J Clin Invest. (2019) 129(6):2210–21. doi: 10.1172/JCI126397
78. Turtle CJ, Hanafi LA, Berger C, Gooley TA, Cherian S, Hudecek M, et al. CD19 CAR-T cells of defined CD4+:CD8+ composition in adult b cell ALL patients. J Clin Invest. (2016) 126(6):2123–38. doi: 10.1172/JCI85309
79. Fraietta JA, Lacey SF, Orlando EJ, Pruteanu-Malinici I, Gohil M, Lundh S, et al. Determinants of response and resistance to CD19 chimeric antigen receptor (CAR) T cell therapy of chronic lymphocytic leukemia. Nat Med (2018) 24(5):563–71. doi: 10.1038/s41591-018-0010-1
80. Depil S, Duchateau P, Grupp SA, Mufti G, Poirot L. 'Off-the-shelf' allogeneic CAR T cells: development and challenges. Nat Rev Drug Discovery (2020) 19(3):185–99. doi: 10.1038/s41573-019-0051-2
81. Metelo AM, Jozwik A, Luong LA, Dominey-Foy D, Graham C, Attwood C, et al. Allogeneic anti-BCMA CAR T cells are superior to multiple myeloma-derived CAR T cells in preclinical studies and may be combined with gamma secretase inhibitors. Cancer Res Commun (2022) 2(3):158–71. doi: 10.1158/2767-9764.CRC-21-0157
82. Mailankody S, Liedtke M, Sidana S, Matous JV, Chhabra S, Oluwole OO, et al. Universal updated phase 1 data validates the feasibility of allogeneic anti-BCMA ALLO-715 therapy for Relapsed/Refractory multiple myeloma. Blood (2021) 138(Supplement 1):651. doi: 10.1182/blood-2021-145572
83. Noonan KA, Borrello IM. Marrow infiltrating lymphocytes: Their role in adoptive immunotherapy. Cancer J (2015) 21(6):501–5. doi: 10.1097/PPO.0000000000000159
84. Noonan KA, Huff CA, Davis J, Lemas MV, Fiorino S, Bitzan J, et al. Adoptive transfer of activated marrow-infiltrating lymphocytes induces measurable antitumor immunity in the bone marrow in multiple myeloma. Sci Transl Med (2015) 7(288):288ra78. doi: 10.1126/scitranslmed.aaa7014
85. Füchsl F, Krackhardt AM. Adoptive cellular therapy for multiple myeloma using CAR- and TCR-transgenic T cells: Response and resistance. Cells (2022) 11(3): 410. doi: 10.3390/cells11030410
86. Andrade VC, Vettore AL, Felix RS, Almeida MS, Carvalho F, Oliveira JS, et al. Prognostic impact of cancer/testis antigen expression in advanced stage multiple myeloma patients. Cancer Immun (2008) 8:2. doi: 10.1182/blood.V110.11.4733.4733
87. Rapoport AP, Stadtmauer EA, Binder-Scholl GK, Goloubeva O, Vogl DT, Lacey SF, et al. NY-ESO-1-specific TCR-engineered T cells mediate sustained antigen-specific antitumor effects in myeloma. Nat Med (2015) 21(8):914–21. doi: 10.1038/nm.3910
88. Moon EK, Ranganathan R, Eruslanov E, Kim S, Newick K, O'Brien S, et al. Blockade of programmed death 1 augments the ability of human T cells engineered to target NY-ESO-1 to control tumor growth after adoptive transfer. Clin Cancer Res (2016) 22(2):436–47. doi: 10.1158/1078-0432.CCR-15-1070
89. Stadtmauer EA, Fraietta JA, Davis MM, Cohen AD, Weber KL, Lancaster E, et al. CRISPR-engineered T cells in patients with refractory cancer. Science (2020) 367(6481): eaba7365. doi: 10.1126/science.aba7365
90. Linette GP, Stadtmauer EA, Maus MV, Rapoport AP, Levine BL, Emery L, et al. Cardiovascular toxicity and titin cross-reactivity of affinity-enhanced T cells in myeloma and melanoma. Blood (2013) 122(6):863–71. doi: 10.1182/blood-2013-03-490565
91. Shah N, Li L, McCarty J, Kaur I, Yvon E, Shaim H, et al. Phase I study of cord blood-derived natural killer cells combined with autologous stem cell transplantation in multiple myeloma. Br J Haematol (2017) 177(3):457–66. doi: 10.1111/bjh.14570
92. Bexte T, Alzubi J, Reindl LM, Wendel P, Schubert R, Salzmann-Manrique E, et al. CRISPR-Cas9 based gene editing of the immune checkpoint NKG2A enhances NK cell mediated cytotoxicity against multiple myeloma. Oncoimmunology (2022) 11(1):2081415. doi: 10.1080/2162402X.2022.2081415
93. Basar R, Daher M, Rezvani K. Next-generation cell therapies: the emerging role of CAR-NK cells. Blood Adv (2020) 4(22):5868–76. doi: 10.1182/bloodadvances.2020002547
94. Liu E, Marin D, Banerjee P, Macapinlac HA, Thompson P, Basar R, et al. Use of CAR-transduced natural killer cells in CD19-positive lymphoid tumors. N Engl J Med (2020) 382(6):545–53. doi: 10.1056/NEJMoa1910607
95. Jiang H, Zhang W, Shang P, Zhang H, Fu W, Ye F, et al. Transfection of chimeric anti-CD138 gene enhances natural killer cell activation and killing of multiple myeloma cells. Mol Oncol (2014) 8(2):297–310. doi: 10.1016/j.molonc.2013.12.001
96. Chu J, Deng Y, Benson DM, He S, Hughes T, Zhang J, et al. CS1-specific chimeric antigen receptor (CAR)-engineered natural killer cells enhance in vitro and in vivo antitumor activity against human multiple myeloma. Leukemia (2014) 28(4):917–27. doi: 10.1038/leu.2013.279
97. Yousef S, Marvin J, Steinbach M, Langemo A, Kovacsovics T, Binder M, et al. Immunomodulatory molecule PD-L1 is expressed on malignant plasma cells and myeloma-propagating pre-plasma cells in the bone marrow of multiple myeloma patients. Blood Cancer J (2015) 5(3):e285. doi: 10.1038/bcj.2015.7
98. Ishibashi M, Tamura H, Sunakawa M, Kondo-Onodera A, Okuyama N, Hamada Y, et al. Myeloma drug resistance induced by binding of myeloma B7-H1 (PD-L1) to PD-1. Cancer Immunol Res (2016) 4(9):779–88. doi: 10.1158/2326-6066.CIR-15-0296
99. Tamura H, Ishibashi M, Yamashita T, Tanosaki S, Okuyama N, Kondo A, et al. Marrow stromal cells induce B7-H1 expression on myeloma cells, generating aggressive characteristics in multiple myeloma. Leukemia (2013) 27(2):464–72. doi: 10.1038/leu.2012.213
100. Lesokhin AM, Ansell SM, Armand P, Scott EC, Halwani A, Gutierrez M, et al. Nivolumab in patients with relapsed or refractory hematologic malignancy: Preliminary results of a phase ib study. J Clin Oncol (2016) 34(23):2698–704. doi: 10.1200/JCO.2015.65.9789
101. Usmani SZ, Schjesvold F, Oriol A, Karlin L, Cavo M, Rifkin RM, et al. Pembrolizumab plus lenalidomide and dexamethasone for patients with treatment-naive multiple myeloma (KEYNOTE-185): a randomised, open-label, phase 3 trial. Lancet Haematol (2019) 6(9):e448–e58. doi: 10.1016/S2352-3026(19)30109-7
102. Mateos MV, Blacklock H, Schjesvold F, Oriol A, Simpson D, George A, et al. Pembrolizumab plus pomalidomide and dexamethasone for patients with relapsed or refractory multiple myeloma (KEYNOTE-183): a randomised, open-label, phase 3 trial. Lancet Haematol (2019) 6(9):e459–e69. doi: 10.1016/S2352-3026(19)30110-3
103. Rosenblatt J, Glotzbecker B, Mills H, Vasir B, Tzachanis D, Levine JD, et al. PD-1 blockade by CT-011, anti-PD-1 antibody, enhances ex vivo T-cell responses to autologous dendritic cell/myeloma fusion vaccine. J Immunother. (2011) 34(5):409–18. doi: 10.1097/CJI.0b013e31821ca6ce
104. Hallett WH, Jing W, Drobyski WR, Johnson BD. Immunosuppressive effects of multiple myeloma are overcome by PD-L1 blockade. Biol Blood Marrow Transplant. (2011) 17(8):1133–45. doi: 10.1016/j.bbmt.2011.03.011
105. Cherkassky L, Morello A, Villena-Vargas J, Feng Y, Dimitrov DS, Jones DR, et al. Human CAR T cells with cell-intrinsic PD-1 checkpoint blockade resist tumor-mediated inhibition. J Clin Invest. (2016) 126(8):3130–44. doi: 10.1172/JCI83092
106. Rafiq S, Yeku OO, Jackson HJ, Purdon TJ, van Leeuwen DG, Drakes DJ, et al. Targeted delivery of a PD-1-blocking scFv by CAR-T cells enhances anti-tumor efficacy in vivo. Nat Biotechnol (2018) 36(9):847–56. doi: 10.1038/nbt.4195
107. Topp MS, Duell J, Zugmaier G, Attal M, Moreau P, Langer C, et al. Anti-B-Cell maturation antigen BiTE molecule AMG 420 induces responses in multiple myeloma. J Clin Oncol (2020) 38(8):775–83. doi: 10.1200/JCO.19.02657
108. Usmani SZ, Garfall AL, van de Donk N, Nahi H, San-Miguel JF, Oriol A, et al. Teclistamab, a b-cell maturation antigen × CD3 bispecific antibody, in patients with relapsed or refractory multiple myeloma (MajesTEC-1): a multicentre, open-label, single-arm, phase 1 study. Lancet (2021) 398(10301):665–74. doi: 10.1016/S0140-6736(21)01338-6
109. Harrison SJ, Minnema MC, Lee HC, Spencer A, Kapoor P, Madduri D, et al. A phase 1 first in human (FIH) study of AMG 701, an anti-B-Cell maturation antigen (BCMA) half-life extended (HLE) BiTE® (bispecific T-cell engager) molecule, in Relapsed/Refractory (RR) multiple myeloma (MM). Blood (2020) 136:28–9. doi: 10.1182/blood-2020-134063
110. Rodriguez C, D'Souza A, Shah N, Voorhees PM, Buelow B, Vij R, et al. Initial results of a phase I study of TNB-383B, a BCMA x CD3 bispecific T-cell redirecting antibody, in relapsed/refractory multiple myeloma. Blood (2020) 136:43–4. doi: 10.1182/blood-2020-139893
111. Costa LJ, Wong SW, Bermúdez A, de la Rubia J, Mateos M-V, Ocio EM, et al. First clinical study of the b-cell maturation antigen (BCMA) 2+1 T cell engager (TCE) CC-93269 in patients (Pts) with Relapsed/Refractory multiple myeloma (RRMM): Interim results of a phase 1 multicenter trial. Blood (2019) 134:143. doi: 10.1182/blood-2019-122895
112. Madduri D, Rosko A, Brayer J, Zonder J, Bensinger WI, Li J, et al. REGN5458, a BCMA x CD3 bispecific monoclonal antibody, induces deep and durable responses in patients with relapsed/refractory multiple myeloma (RRMM). Blood (2020) 136:41–2. doi: 10.1182/blood-2020-139192
113. Moreau P, Garfall AL, van de Donk N, Nahi H, San-Miguel JF, Oriol A, et al. Teclistamab in relapsed or refractory multiple myeloma. N Engl J Med (2022) 387(6):495–505. doi: 10.1056/NEJMoa2203478
114. Chen H, Yu T, Lin L, Xing L, Cho SF, Wen K, et al. γ-secretase inhibitors augment efficacy of BCMA-targeting bispecific antibodies against multiple myeloma cells without impairing T-cell activation and differentiation. Blood Cancer J (2022) 12(8):118. doi: 10.1038/s41408-022-00716-3
115. Chari A, Berdeja JG, Oriol A, van de Donk NW, Rodriguez P, Askari E, et al. A phase 1, first-in-human study of talquetamab, a G protein-coupled receptor family c group 5 member d (GPRC5D) x CD3 bispecific antibody, in patients with relapsed and/or refractory multiple myeloma (RRMM). Blood (2020) 136:40–1. doi: 10.1182/blood-2020-133873
116. Cohen AD, Harrison SJ, Krishnan A, Fonseca R, Forsberg PA, Spencer A, et al. Initial clinical activity and safety of BFCR4350A, a FcRH5/CD3 T-cell-engaging bispecific antibody, in relapsed/refractory multiple myeloma. Blood (2020) 136:42–3. doi: 10.1182/blood-2020-136985
117. Zuch de Zafra CL, Fajardo F, Zhong W, Bernett MJ, Muchhal US, Moore GL, et al. Targeting multiple myeloma with AMG 424, a novel anti-CD38/CD3 bispecific T-cell-recruiting antibody optimized for cytotoxicity and cytokine release. Clin Cancer Res (2019) 25(13):3921–33. doi: 10.1158/1078-0432.CCR-18-2752
118. Chung C. Role of immunotherapy in targeting the bone marrow microenvironment in multiple myeloma: An evolving therapeutic strategy. Pharmacotherapy (2017) 37(1):129–43. doi: 10.1002/phar.1871
119. Avigan D, Rosenblatt J. Vaccine therapy in hematologic malignancies. Blood (2018) 131(24):2640–50. doi: 10.1182/blood-2017-11-785873
120. Carmon L, Avivi I, Kovjazin R, Zuckerman T, Dray L, Gatt ME, et al. Phase I/II study exploring ImMucin, a pan-major histocompatibility complex, anti-MUC1 signal peptide vaccine, in multiple myeloma patients. Br J Haematol (2015) 169(1):44–56. doi: 10.1111/bjh.13245
121. Greiner J, Schmitt A, Giannopoulos K, Rojewski MT, Götz M, Funk I, et al. High-dose RHAMM-R3 peptide vaccination for patients with acute myeloid leukemia, myelodysplastic syndrome and multiple myeloma. Haematologica (2010) 95(7):1191–7. doi: 10.3324/haematol.2009.014704
122. Schmitt M, Schmitt A, Rojewski MT, Chen J, Giannopoulos K, Fei F, et al. RHAMM-R3 peptide vaccination in patients with acute myeloid leukemia, myelodysplastic syndrome, and multiple myeloma elicits immunologic and clinical responses. Blood (2008) 111(3):1357–65. doi: 10.1182/blood-2007-07-099366
123. Jørgensen NG, Ahmad SM, Abildgaard N, Straten PT, Svane IM, Andersen MH, et al. Peptide vaccination against multiple myeloma using peptides derived from anti-apoptotic proteins: a phase I trial. Stem Cell Investig (2016) 3:95. doi: 10.21037/sci.2016.11.09
124. McCann KJ, Godeseth R, Chudley L, Mander A, Di Genova G, Lloyd-Evans P, et al. Idiotypic DNA vaccination for the treatment of multiple myeloma: safety and immunogenicity in a phase I clinical study. Cancer Immunol Immunother. (2015) 64(8):1021–32. doi: 10.1007/s00262-015-1703-7
125. Qazilbash MH, Saini NY, Cha SC, Wang Z, Stadtmauer EA, Baladandayuthapani V, et al. A randomized phase 2 trial of idiotype vaccination and adoptive autologous T-cell transfer in patients with multiple myeloma. Blood (2022) 139(9):1289–301. doi: 10.1182/blood.2020008493
126. Nooka AK, Wang ML, Yee AJ, Kaufman JL, Bae J, Peterkin D, et al. Assessment of safety and immunogenicity of PVX-410 vaccine with or without lenalidomide in patients with smoldering multiple myeloma: A nonrandomized clinical trial. JAMA Oncol (2018) 4(12):e183267. doi: 10.1001/jamaoncol.2018.3267
127. Lacy MQ, Mandrekar S, Dispenzieri A, Hayman S, Kumar S, Buadi F, et al. Idiotype-pulsed antigen-presenting cells following autologous transplantation for multiple myeloma may be associated with prolonged survival. Am J Hematol (2009) 84(12):799–802. doi: 10.1002/ajh.21560
128. Jung SH, Lee HJ, Lee YK, Yang DH, Kim HJ, Rhee JH, et al. A phase I clinical study of autologous dendritic cell therapy in patients with relapsed or refractory multiple myeloma. Oncotarget (2017) 8(25):41538–48. doi: 10.18632/oncotarget.14582
129. Batchu RB, Moreno AM, Szmania SM, Bennett G, Spagnoli GC, Ponnazhagan S, et al. Protein transduction of dendritic cells for NY-ESO-1-based immunotherapy of myeloma. Cancer Res (2005) 65(21):10041–9. doi: 10.1158/0008-5472.CAN-05-1383
130. Szmania S, Gnjatic S, Tricot G, Stone K, Zhan F, Moreno A, et al. Immunization with a recombinant MAGE-A3 protein after high-dose therapy for myeloma. J Immunother. (2007) 30(8):847–54. doi: 10.1097/CJI.0b013e318158fcff
131. Bae J, Prabhala R, Voskertchian A, Brown A, Maguire C, Richardson P, et al. A multiepitope of XBP1, CD138 and CS1 peptides induces myeloma-specific cytotoxic T lymphocytes in T cells of smoldering myeloma patients. Leukemia (2015) 29(1):218–29. doi: 10.1038/leu.2014.159
132. Xing Y, Hogquist KA. T-Cell tolerance: central and peripheral. Cold Spring Harb Perspect Biol (2012) 4(6): a006957. doi: 10.1101/cshperspect.a006957
133. Dong C, Cesarano A, Bombaci G, Reiter JL, Yu CY, Wang Y, et al. Intron retention-induced neoantigen load correlates with unfavorable prognosis in multiple myeloma. Oncogene (2021) 40(42):6130–8. doi: 10.1038/s41388-021-02005-y
134. Miller A, Asmann Y, Cattaneo L, Braggio E, Keats J, Auclair D, et al. High somatic mutation and neoantigen burden are correlated with decreased progression-free survival in multiple myeloma. Blood Cancer J (2017) 7(9):e612. doi: 10.1038/bcj.2017.94
135. Sabdia MB, Patch AM, Tsang H, Gandhi MK. Neoantigens - the next frontier in precision immunotherapy for b-cell lymphoproliferative disorders. Blood Rev (2022) 56:100969. doi: 10.1016/j.blre.2022.100969
136. Bekri S, Rodney-Sandy R, Gruenstein D, Mei A, Bogen B, Castle J, et al. Neoantigen vaccine-induced CD4 T cells confer protective immunity in a mouse model of multiple myeloma through activation of CD8 T cells against non-vaccine, tumor-associated antigens. J Immunother Cancer (2022) 10(2): e003572. doi: 10.1136/jitc-2021-003572
137. Perumal D, Imai N, Laganà A, Finnigan J, Melnekoff D, Leshchenko VV, et al. Mutation-derived neoantigen-specific T-cell responses in multiple myeloma. Clin Cancer Res (2020) 26(2):450–64. doi: 10.1158/1078-0432.CCR-19-2309
138. Courrèges MC, Benencia F, Conejo-García JR, Zhang L, Coukos G. Preparation of apoptotic tumor cells with replication-incompetent HSV augments the efficacy of dendritic cell vaccines. Cancer Gene Ther (2006) 13(2):182–93. doi: 10.1038/sj.cgt.7700888
139. Mastelic-Gavillet B, Balint K, Boudousquie C, Gannon PO, Kandalaft LE. Personalized dendritic cell vaccines-recent breakthroughs and encouraging clinical results. Front Immunol (2019) 10:766–. doi: 10.3389/fimmu.2019.00766
140. DeAngelo DJ, Alyea EP, Borrello IM, Levitsky HI, Stock W, Sher D, et al. Posttransplant immunotherapy with a GM-CSF-Based tumor vaccine (GVAX®) following autologous stem cell transplant (ASCT) for acute myeloid leukemia (AML). Blood (2004) 104(11):441. doi: 10.1182/blood.V104.11.441.441
141. Dranoff G, Jaffee E, Lazenby A, Golumbek P, Levitsky H, Brose K, et al. Vaccination with irradiated tumor cells engineered to secrete murine granulocyte-macrophage colony-stimulating factor stimulates potent, specific, and long-lasting anti-tumor immunity. Proc Natl Acad Sci U S A. (1993) 90(8):3539–43. doi: 10.1073/pnas.90.8.3539
142. Biavati L, Huff CA, Ferguson A, Sidorski A, Stevens MA, Rudraraju L, et al. An allogeneic multiple myeloma GM-CSF-Secreting vaccine with lenalidomide induces long-term immunity and durable clinical responses in patients in near complete remission. Clin Cancer Res (2021) 27(24):6696–708. doi: 10.1158/1078-0432.CCR-21-1916
143. Rosenblatt J, Vasir B, Uhl L, Blotta S, Macnamara C, Somaiya P, et al. Vaccination with dendritic cell/tumor fusion cells results in cellular and humoral antitumor immune responses in patients with multiple myeloma. Blood (2011) 117(2):393–402. doi: 10.1182/blood-2010-04-277137
144. Rosenblatt J, Avivi I, Vasir B, Uhl L, Munshi NC, Katz T, et al. Vaccination with dendritic cell/tumor fusions following autologous stem cell transplant induces immunologic and clinical responses in multiple myeloma patients. Clin Cancer Res (2013) 19(13):3640–8. doi: 10.1158/1078-0432.CCR-13-0282
Keywords: multiple myeloma, immunotherapy, CAR T therapy, adoptive cell therapy, cancer vaccines
Citation: Boussi LS, Avigan ZM and Rosenblatt J (2022) Immunotherapy for the treatment of multiple myeloma. Front. Immunol. 13:1027385. doi: 10.3389/fimmu.2022.1027385
Received: 26 August 2022; Accepted: 12 October 2022;
Published: 28 October 2022.
Edited by:
Beatriz Martín-Antonio, University Hospital Fundación Jiménez Díaz, SpainReviewed by:
Jean-Sébastien Delisle, Hôpital Maisonneuve-Rosemont, CanadaCopyright © 2022 Boussi, Avigan and Rosenblatt. This is an open-access article distributed under the terms of the Creative Commons Attribution License (CC BY). The use, distribution or reproduction in other forums is permitted, provided the original author(s) and the copyright owner(s) are credited and that the original publication in this journal is cited, in accordance with accepted academic practice. No use, distribution or reproduction is permitted which does not comply with these terms.
*Correspondence: Jacalyn Rosenblatt, anJvc2VuYjFAYmlkbWMuaGFydmFyZC5lZHU=
†These authors have contributed equally to this work and share first authorship
Disclaimer: All claims expressed in this article are solely those of the authors and do not necessarily represent those of their affiliated organizations, or those of the publisher, the editors and the reviewers. Any product that may be evaluated in this article or claim that may be made by its manufacturer is not guaranteed or endorsed by the publisher.
Research integrity at Frontiers
Learn more about the work of our research integrity team to safeguard the quality of each article we publish.