- Department of Critical Care Medicine, Union Hospital, Tongji Medical College, Huazhong University of Science and Technology, Wuhan, China
Sepsis is a life-threatening organ dysfunction resulting from dysregulated host responses to infection. Macrophages play significant roles in host against pathogens and the immunopathogenesis of sepsis, such as phagocytosis of pathogens, secretion of cytokines, and phenotype reprogramming. However, the rapid progression of sepsis impairs macrophage function, and conventional antimicrobial and supportive treatment are not sufficient to restore dysregulated macrophages roles. Nanoparticles own unique physicochemical properties, surface functions, localized surface plasmon resonance phenomenon, passive targeting in vivo, good biocompatibility and biodegradability, are accessible for biomedical applications. Once into the body, NPs are recognized by host immune system. Macrophages are phagocytes in innate immunity dedicated to the recognition of foreign substances, including nanoparticles, with which an immune response subsequently occurs. Various design strategies, such as surface functionalization, have been implemented to manipulate the recognition of nanoparticles by monocytes/macrophages, and engulfed by them to regulate their function in sepsis, compensating for the shortcomings of sepsis traditional methods. The review summarizes the mechanism of nanomaterials targeting macrophages and recent advances in nanomedicine targeting macrophages in sepsis, which provides good insight for exploring macrophage-based nano-management in sepsis.
Introduction
Sepsis is a life-threatening organ dysfunction associated with dysregulated host response to infection (1, 2). Sepsis kills as many as one in four similar to acute myocardial infarction, stroke, or multiple injuries and ranks third in diverse disease mortality (1, 2). There are approximately 48.9 million sepsis patients worldwide, with a mortality rate of 19.7% (3). However, due to its complex pathogenesis, targeted therapies still need to be explored (2).
Monocytes/macrophages are important players in the pathogenesis of sepsis (4, 5). Induced by pathogens and cytokines in the environment, macrophages differentiate to diverse functional phenotypes and perform different functions, including killing of pathogenic microorganisms, cytokine and chemokine production (6). During sepsis, the complicated pathogenesis brings changes in macrophage function, which include phenotype reprogramming, alterations in inflammatory signaling pathways, macrophage overactivation causes inflammatory factor storm, etc (7, 8). These changes complicate the pathophysiological status of clinical patients, leading to the dramatically reduced effects of conventional gene therapy and drugs (9–14).
Nanomaterials are a class of materials consisting of organic or/and inorganic particles with a size of about 1 to 100 nm, and the representative classes of nanomaterials includes polymeric materials, liposomes, biomimetic materials, exosomes and metal/inorganic materials and so on (15), it has been considered a promising tool in sepsis treatment. Nanomaterials can be used as drug carriers transport inflammation-modifying drugs, because it can enhance drug targeting delivery and bioavailability, modulating pro-/anti-inflammatory roles (16). For macrophage, nanomaterials act as contrast and diagnostic devices that can detect the physicochemical properties of macrophage phagosomes and realize macrophage labelling, imaging, and long-term follow-up (17–22), the features of nanomaterials may help to improve the diagnostic and therapeutic techniques for patients with sepsis, by such as targeting macrophage activation, modulating inflammatory pathways, reprogramming macrophage polarization, etc (23–26). However, their characteristics and functional mechanisms targeting macrophages during sepsis have not been addressed fully in sepsis (16, 27). Herein, we review the following: i) the rationality of nanomaterials targeting macrophages; ii) the mechanisms for nanomaterials or technologies targeting sepsis-associated macrophages; and iii) the prospects of nanomaterials for the diagnosis and management of sepsis.
The dysregulated function of macrophages in sepsis
During early sepsis, lipopolysaccharides (LPS) is recognized by toll-like receptor 4 (TLR4) of macrophages, which activate the nuclear factor-κB (NF-κB) pathway and mitogen-activated protein kinase (MAPK) pathway, causing inflammation-active mediators (such as IL-1, IL-6, IL-18, TNF-α) releasing and facilitating the clearance of pathogenic microorganisms (28). However, excessive activated macrophages cause a cascade of amplified inflammatory responses, such as “cytokine storm”, impairing host immune function and mediating organ dysfunction (29). Meanwhile, macrophage reprogramming, also known as LPS tolerance, causes a reduction in the ability of macrophage to release pro-inflammatory cytokines participating in sepsis immunosuppression (30–34). LPS tolerent macrophage show decresed expression of costimulatory molecules (CD86, etc.), decreased expression of MHC-II-like molecules, and elevated CTLA4 expression, resulting in an antigen-presenting deficiency, decreased ability to produce IL-6, TNF-α and IFN-γ, and an increased ability to produce IL-10 and TGF-β, which induces immunosuppression (29, 35–44). In addition, pathogens induce macrophage apoptosis, pyroptosis, necroptosis, and parthanatos that make it impossible for immune cells to proliferate effectively, thus making it difficult for the host to effectively respond to pathogens (33, 45). The above dysregulated macrophage function, including macrophage overactivation, macrophage phenotypic reprogramming, and programmed macrophage death can be regulated by nanomaterials to achieve macrophage-targeted therapy in sepsis.
The rationality of nanomaterial targeting macrophage
NPs are synthesized by chemical reduction, wet chemical methods, ligand-mediated self-assembly, electrostatic assembly, polymer encapsulation, and nanoprecipitation and so on (46, 47).Nanomaterials have unique advantages over ordinary drugs, which include tunable properties (e.g., structural size and composition, carried charge and surface chemical properties), surface functions (e.g., target ligands and molecules) and specific binding features (48, 49). The core of nanomaterials is nanoparticles, which are particles of nanoscale size (from 1nm to 100nm) (47). Engineered NPs are classified into polymeric NPs, liposomes, biomimetic NPs, exosomes and metal/inorganic NPs (47, 50). Different material compositions (such as SPIONs, metallic fabrics, and organic materials), surface coatings (such as positively/negatively charged on the surface or coated by PEG) and shapes (such as nanospheres, nanorods, nanostars, nanocubes, nanodisks, etc.) give NPs different properties that affect the efficiency and mode of action of nanomaterials into cells (47, 51) (Figure 1).
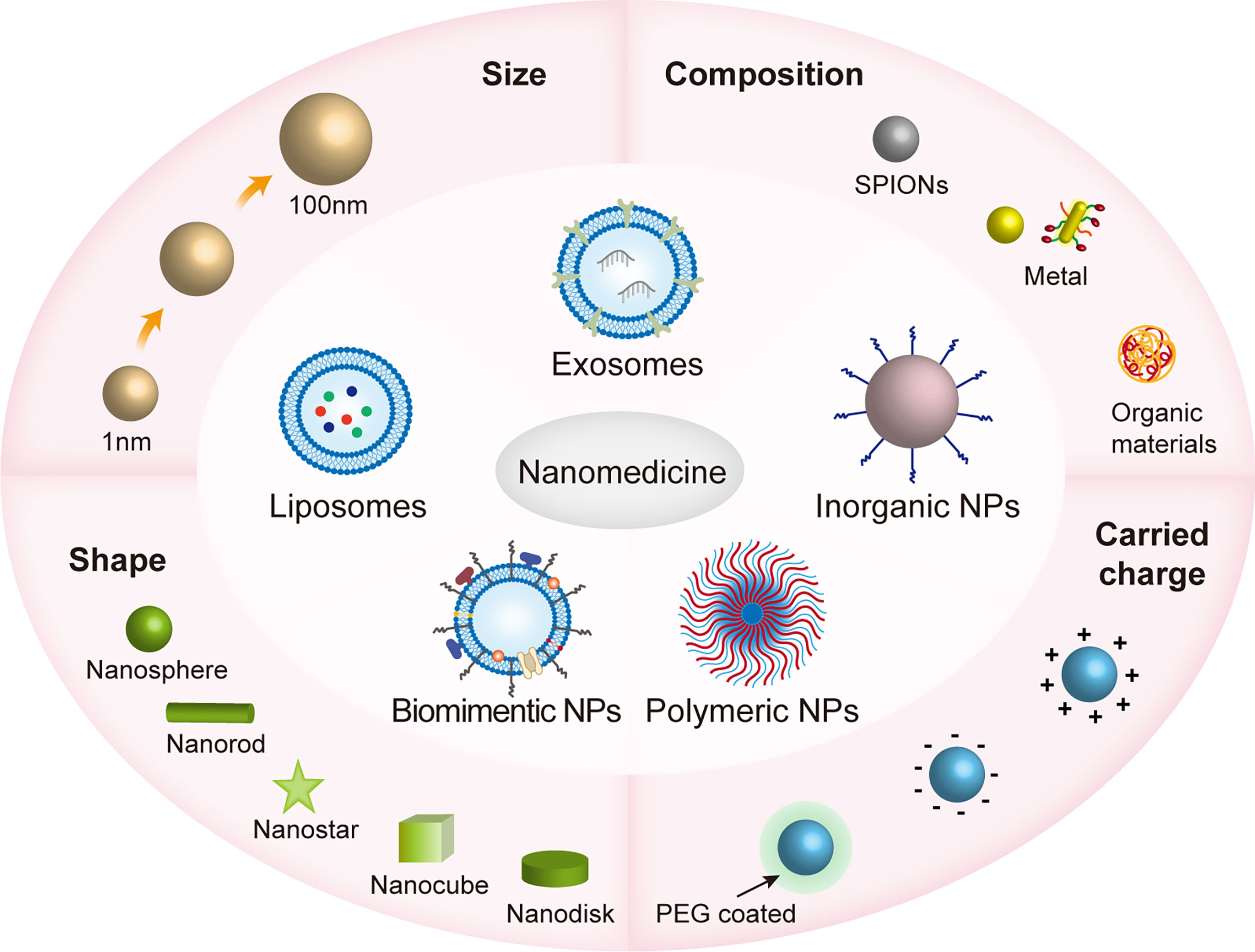
Figure 1 The various properties of nanomaterials in nanomedicines. Nanomaterials are subdivided into polymorphic nanoparticles (NPs), liposomes, biomimetic NPs, inorganic NPs, and cell-derived exosomes, which range in size from approximately 1 to 100 nm and have different shapes, including nanospheres, nanorods, nanostars, nanocubes, nanodisks, etc.; consist of different materials, including SPIONs, metallic fabrics, and organic materials; and are both positively/negatively charged on the surface or coated by PEG. These physicochemical properties influence how efficiently nanomaterials enter the cell and the mode of action that affects cellular activity. The size and shape of the nanomaterial can determine the way it enters the cell, such as clathrin-mediated endocytosis (120nm), and clathrin- and caveolae-independent endocytosis (~60nm) (51).. The surface charge of nanoparticles affects the cell membrane state, for example, the binding of negatively charged nanoparticles to cellular lipid bilayers causes local gelation, while the binding of positively charged nanoparticles to cellular lipid bilayers causes the flow of phospholipid bilayers (52). Polyethylene glycolization reduces premature removal of NPs from the cycle (53).
Polymeric NPs not only protect “antigen” from enzymatic digestion but also have APC targeting, easy surface modification, biodegradable, nontoxic and nonimmunogenic features. Drugs can be loaded on the surface or inside polymeric nanospheres and nanocapsules to avoid enzymatic digestion while crossing biological barriers to the target region (54). Liposomes compose phospholipids and cholesterol and can encapsulate both lipid-soluble and water-soluble drugs, preventing the drug from rapidly degrading and reducing adverse reactions by preventing direct contact with the systemic circulation (52, 53). Based on the properties of receptor-ligand binding, target cell-specifical ligands can be assembled on the surface of liposomes to facilitate receptor-mediated liposome endocytosis and promote the entry of liposomes and their loaded drugs into target cells (52, 53). Biomimetic NPs are NPs formed by attaching natural ligands or functional components, such as cell membranes, to the surface of engineered NPs (16). Cell membrane coating nanotechnology has been developed to synthesize biomimetic NPs by covering the surface of synthesized NPs with cell membranes prepared using techniques such as osmotic pressure difference, chemical interference, electroporation, and ultrasonic treatment (55). Macrophage membrane-coated biomimetic NPs combine the unique biochemical functions of macrophages that can achieve targeted drug delivery with low immunogenicity (16, 56). Exosomes defined as a type of extracellular vehicles between 30 and 150 nm can transfer the encapsulated biomolecules (such as DNA, RNA, proteins, lipids and metabolites) from the donor cell to the recipient cell, thus triggering cell phenotypic changes participating in a variety of immune responses (57–63). Exosomes possess the advantages of inherent stability, high delivery efficiency, and ability to cross biological barriers (62, 64–66). Inorganic nanomaterials have superior optical and magnetic characteristics and a high surface-area-to-volume ratio, thus making them ideal for molecular detection, drug delivery, immunomodulation, etc. For example, nanoscale noble metals (e.g., AuNPs) exposed to light exhibit localized surface plasmon resonance (LSPR) phenomena, resulting in the improved sensitivity of molecular detection (67, 68). Moreover, cerium nanoparticles significantly attenuated the total superoxide flux in macrophages (69).
Through nanoprecipitation, emulsion polymerization, electroporation, film dispersion, ultrasonic dispersion, reverse-phase evaporation method, etc., scientists can program the physicochemical properties of NPs. Engineered NPs are both for loading drugs targeting macrophages to improve their bioavailability and for modifying the structure of nanomaterials to modulate macrophage function by supramolecular chemistry. NPs exerting their intracellular or extracellular biological activities after being recognized and endocytosed by macrophages (Figure 2) (46, 47, 55, 70). Plasma contains various proteins, which can bind to the nanoparticles (NPs), and once NPs enter the host and contact with plasma proteins, they acquire a new biological characteristic called protein corona (PC) (71). PC changes the physicochemical properties of NPs, including surface charge, size, aggregation state and antigenic epitopes; and these changes preferentially in turn affect the pharmacokinetics, biodistribution and therapeutic effects of NPs (71). Binding to certain proteins lead to recognition of NPs by the mononuclear phagocyte system (MPS) (72). For example, IgG, fibrinogen and complement proteins, promote the uptake of NPs by the MPS utilizing the corresponding receptors expressed on the phagocyte surface, such as scavenger receptor on Kupffer cell. In addition, IgG and complement C3b promote the uptake of NPs by monocytes (73).
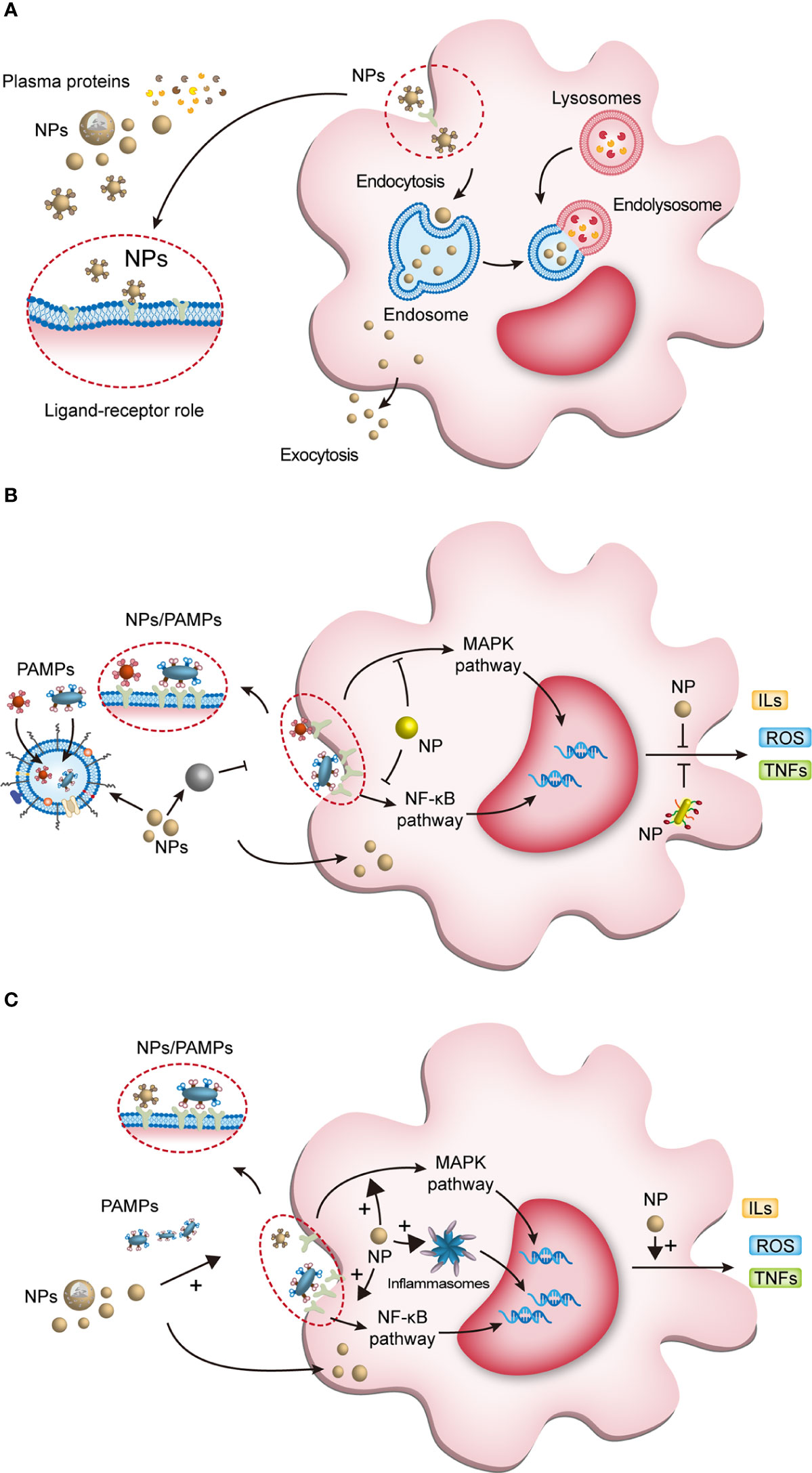
Figure 2 The interaction of NPs with macrophages and NPs modulating macrophage anti/pro-inflammatory function. (A) Once NPs enter the body, they bind to plasma proteins and enter macrophages. Some endosomes containing NPs degrade the processed NPs and release them extracellularly to exert active effects; the other endosomes fuse with lysosomes to form endolysosomes, which exert intracellular effects. (B) First, NPs can eliminate macrophage activation by phagocytosis and confinement of pathogen-associated molecular pattern molecules (PAMPs); second, they inhibit PAMPs interacting with pattern recognition receptors (PRRs); third, NPs entering the cytoplasm inhibit the transduction of inflammatory signaling pathways; and finally, NPs inhibit the release of active products of inflammatory pathways and control the cell and tissue damage caused by overactivated macrophages. (C) NPs modulate macrophage proinflammatory activity. NPs can enhance PRR activation to initiate macrophage inflammation. After entering the cytoplasm, NPs activate downstream pathways and inflammasomes to induce proinflammatory factor production. NPs, nanoparticles; PAMPs, pathogen-associated molecular pattern molecules; ROS, reactive oxygen species; ILs, interleukins; TNFs, tumor necrosis factors; MAPK, mitogen-activated protein kinase; NF-κB, nuclear factor-kappa B.
Nanomaterials are successfully used in diverse diseases (Table 1), especially sepsis (Table 2) (56, 85, 116). Nanomaterials targeting macrophages mainly lie in the therapeutic aspect, including as drug carrier or nanodrug to regulate macrophage anti-inflammatory/pro-inflammatory function (Figure 2), macrophage reprogramming (Figure 3) and programmed macrophage death (Figure 4) (16, 22, 112, 113, 115, 117). In the regulation of macrophage anti-inflammatory/pro-inflammatory function, PAMPs and pattern recognition receptor (PRR) shows great potential (35). The process of recognition and phagocytosis of nanomaterials by macrophages approximates the mutual recognition of PAMPs and PRRs (35). NP delivery platforms in combination with PAMPs or their synthetic mimics hold great promise in immunomodulatory therapy using synthetic or natural polymers, lipid-polymer hybrids and self-assembled compounds to constitute nanodelivery systems that capture or adsorb TLR ligands and modulate innate immune responses (118, 119). CpG sequences are typical PAMPs, which when bound to PLG can be widely recognized and phagocytosed by antigen-presenting cells (APCs), including macrophages, to enhance the host immune response (120). TLR receptors of dendritic cells and monocytes have been shown to recognize alginate-coated chitosan nanogels, affecting the TLR ligands Pam3Cys-SK4 or CpG-ODN involved in the regulation of their immune function, inducing the release of IL1-β, IL-6, TNF-α, and IFN-α (121). After enter the macrophages, NPs which load immunomodulatory drugs can promote/inhibit NK-κB/MAPK pathway to modulate macrophage function (84, 122).What’s more, PRRs assemble into inflammasomes after detecting pathogenic microorganisms or DAMPs in the cytoplasmic matrix of the host cell (123). In macrophages, PRRs assemble into inflammasomes upon detection of pathogenic microorganisms and danger signals in the cytoplasmic matrix of host cells. Silica nanoparticles (SiO (2) NPs) Silica enters the cell and generates ROS, which activate the inflammasome, including caspase-1, ASC multimerization, and promote IL-1β and IL-18 expression in macrophages (124, 125). Multi-walled carbon nanotubes (MWCNTs) and asbestos induce NLPR3 inflammasome activation in macrophages, and this activation depends on reactive oxygen species (ROS) production, histone B activity, P2X7 receptors, and Src and Syk tyrosine kinases (126). In addition, the specific deposition of imaging agents in macrophages can be detected with the aid of an imaging device, enabling time- and space-specific monitoring of macrophages (Figure 5).
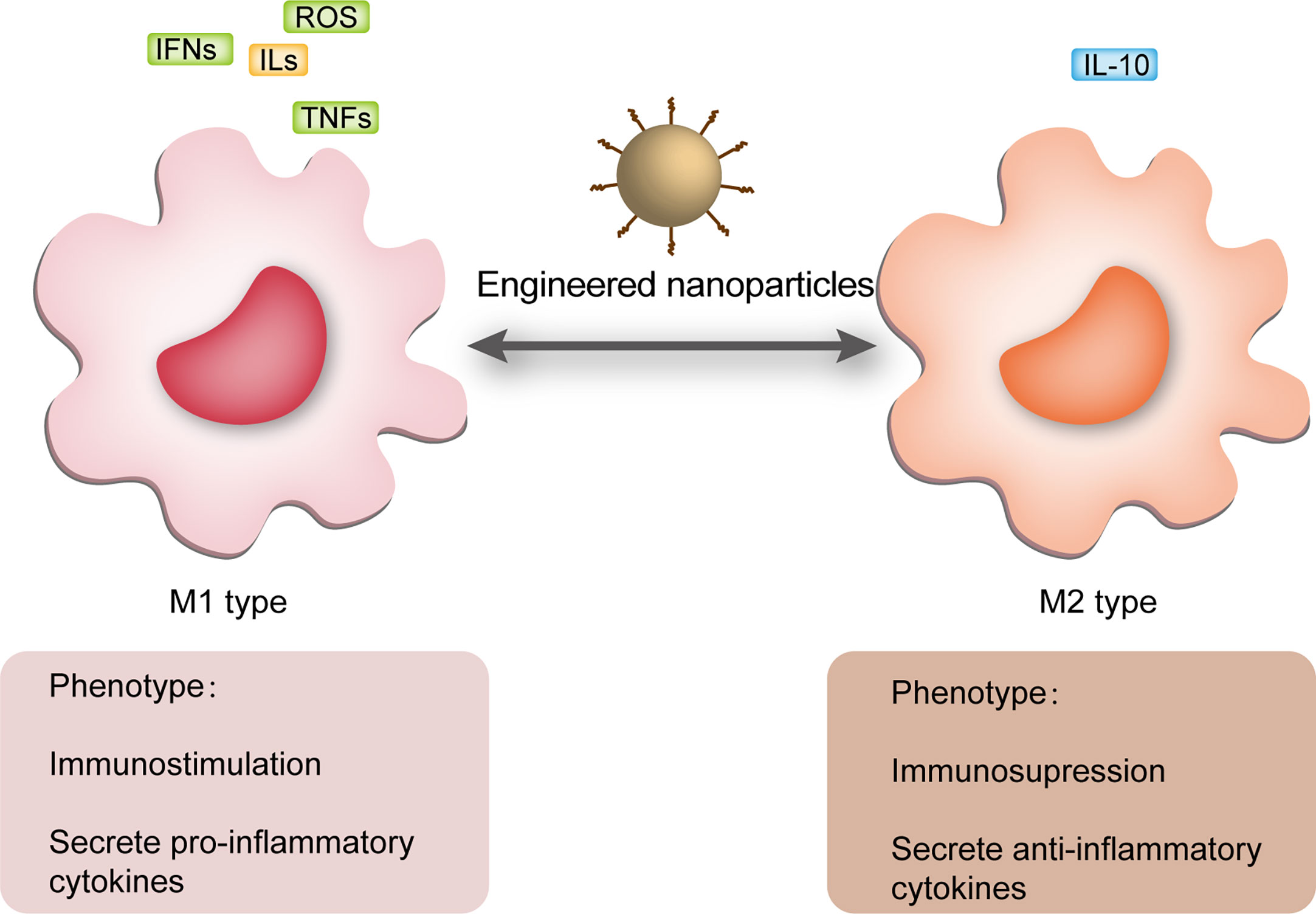
Figure 3 NPs regulated macrophage polarization. M1 macrophages usually act in the sepsis cytokine storm state and release a large number of pro-inflammatory mediators, including ROS, IFNs, ILs and TNFs, while M2 macrophages usually appear in the immune paralysis phase and secrete anti-inflammatory mediators, the most characteristic of which is IL-10. During periods of inflammatory overstimulation, sustained release of pro-inflammatory mediators from M1 induces damage to the organism, while massive activation of M2 macrophages during periods of immune paralysis increases the risk of secondary infection. NPs can regulate macrophage polarization in different periods of sepsis improving its prognosis. NPs, nanoparticles; ROS, reactive oxygen species; ILs, interleukins; IFNs, interferons; TNFs, tumor necrosis factors.
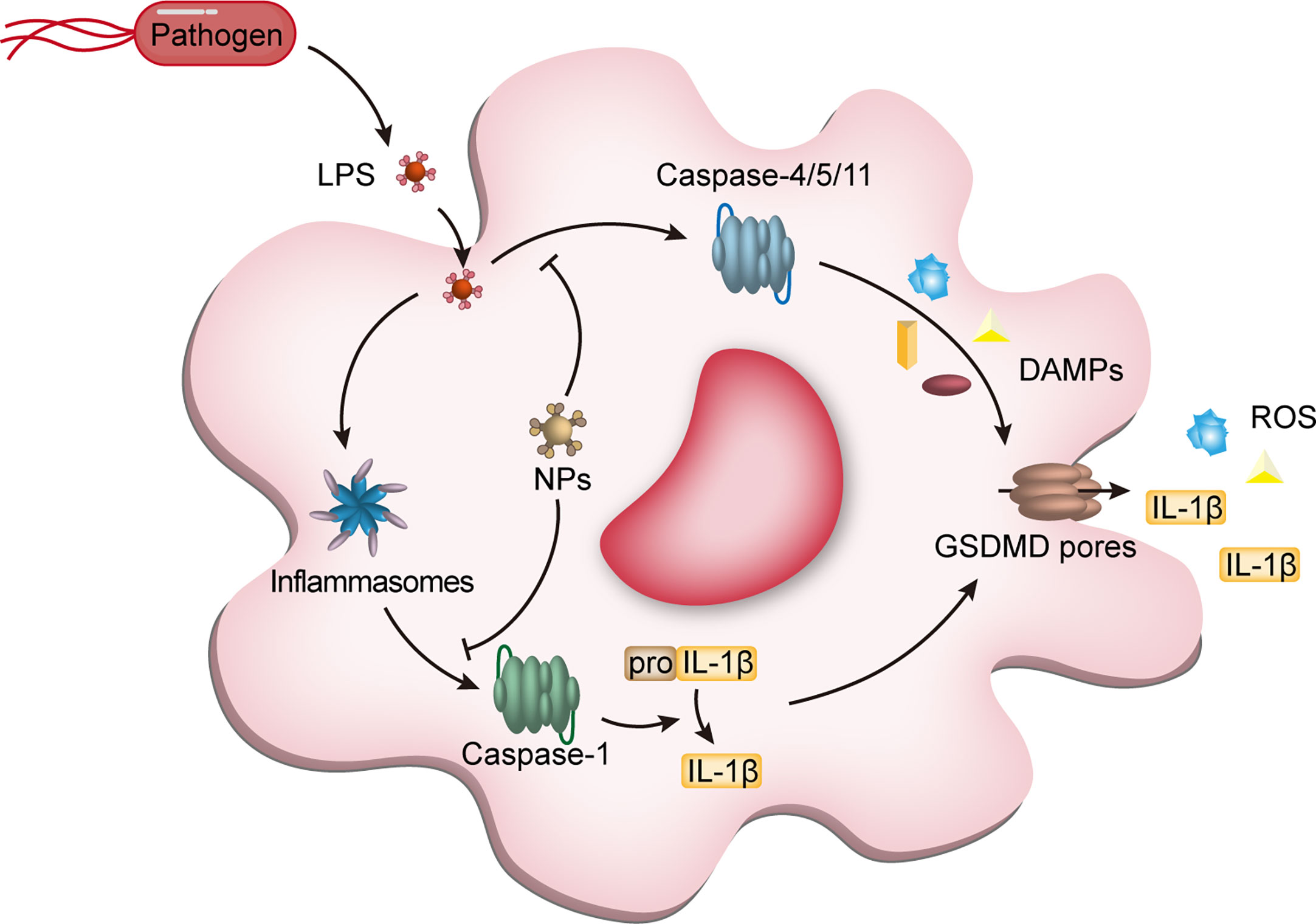
Figure 4 NPs interfere with macrophage pyroptosis. Pyroptosis, a class of programmed cell death dependent on pro-inflammatory caspases (caspase-1,-4,-5 from humans and caspase-1 and -11 from mice) and gasdermin D (GSDMD), is an important type of programmed macrophage death. After recognition of LPS derived from pathogens by macrophage intracellular receptors, activation of caspases triggers the cleavage of GSDMD and IL-1β, which accumulates in the cell membrane to form pores causing cell membrane collapse, accompanied by the release of inflammatory cytokines, including IL-1β, ultimately leading to cell death. The NPs entering the cells can block the activation of caspase-1 and caspase4/5/11, reducing the release of DAMPs and avoiding unnecessary tissue and cell damage. NPs, nanoparticles; LPS, lipopolysaccharides; IL-1β, interleukin-1β; DAMPs, damage-associated molecular pattern molecules; GSDMD, gasdermin D.
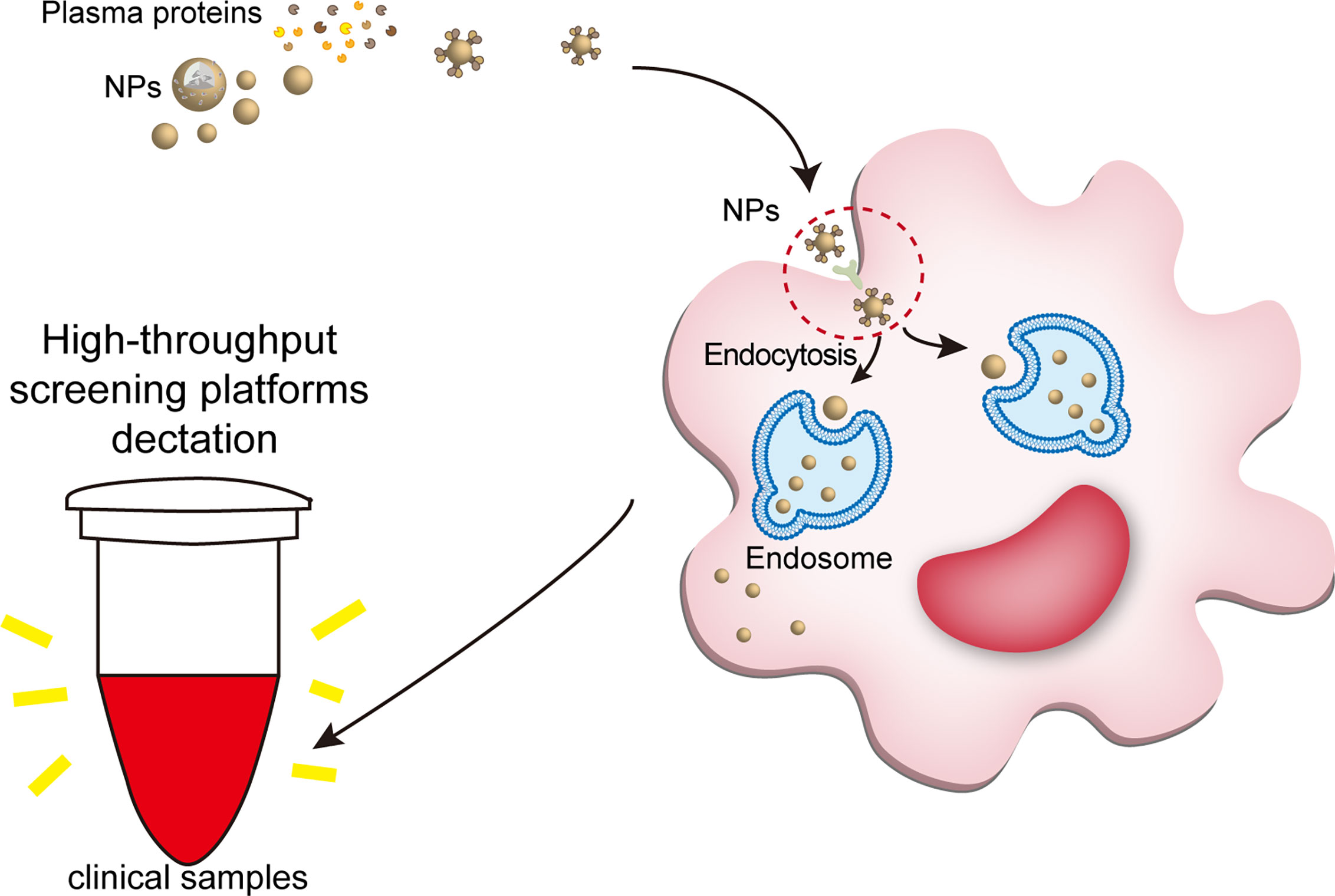
Figure 5 NPs engulfed by macrophages can be detected by clinical tools. The large number of proteins bound to the NP can trigger immediate recognition by macrophages. Ligand-receptor role is a part of way that plasma protein helps NPs enter macrophages. When nanomaterials interact with biological fluids, after the formation of a protein corona, their main requirement is to interact with cell membranes (especially the molecule in the cell membranes surface) to show their biological effects, such as ligand-receptor role. For example, IgG, fibrinogen and complement proteins, promote the uptake of NPs by the macrophages utilizing the corresponding receptors expressed on the phagocyte surface, such as scavenger receptor on Kupffer cell. Once the NPs are endocytosed into macrophages, the nanotracer in macrophages of the samples can be tested by high-throughput screening platforms, such as optical nanosensor and other nano-detector. NPs, nanoparticles.
Nano drug carriers targeting macrophage
Drugs can be encapsulated and sequestered by NPs or covalently attached to the surface of NPs, enhancing drug-targeted delivery and release and/or improving drug biodistribution and/or bioavailability to modulate the anti-inflammatory/pro-inflammatory activity of macrophages, macrophage reprogramming and macrophage pyroptosis (17). In addition, the release time and site of the modified nanodrug can be controlled after being triggered by environmental physicochemical properties (e.g., pH and enzyme action), thus regulating the eff ects of the drug in plasma and cells (49, 127).
Polymeric NPs as drug carriers target macrophages
Polymeric NPs can facilitate targeted drug delivery due to easy surface modification, biodegradable, nontoxic and nonimmunogenic features (128–130).
Polymeric NPs promote macrophage anti-inflammatory activity in sepsis overactivation stage. Chitosan, as a kind of polymeric NPs, improves drug delivery efficiency and controlled release (22). For example, Hongsa et al. designed a modified biotin-quat 188-chitosan (Bi-QCS) and collagen nanodrug carrier (Bi-QCS-AuNPS@collagen) wrapped in AuNPs surface (79). Bi-QCS significantly improved the uptake of loaded drugs by macrophages, and chitosan improves physicochemical stability, controls drug release and promotes its anti-inflammatory activity (79). Compared with conventional AuNPs, Bi-QCS-AuNPs@collagen has higher drug loading and promotes apparent anti-inflammatory role in RAW264.7 macrophage (79). In addition, reactive oxygen species (ROS) and pH-sensitive polymeric chitosan/alginate hydrogel NPs loaded with curcumin effectively avoid the hydrolysis of digestive fluid and directly target macrophages to exert anti-inflammatory effects via TLR4-MAPK/NF-κB pathway inhibition; among them, chondroitin sulfate promotes macrophage targeting of NPs, while chitosan/alginate hydrogel protects NPs from being destroyed by digestive juices (122). A nanocomposite synthesized by chitosan and antimicrobial peptides (AMPs) significantly inhibited NF-κB/MAPK pathway activation by LPS in RAW264.7 macrophages as well (131). Moreover, chitosan was developed to carry NF-κB/p65 antisense oligonucleotides that target macrophages to inhibit NF-κB/p65 signaling and downstream release levels of inflammatory factors such as IL-1, IL-6 and TNF-, in LPS-stimulated RAW264.7 macrophages (92). Rajendrakumar et al. developed a mannosylated disulfide cross-linked polyethyleneimine (ssPEI) (Msp)-encapsulated bovine serum albumin-reduced manganese dioxide (MSPAM) nanocomplex that effectively avoided organ damage caused by macrophages in a sepsis model (109). Hydrophilic bovine serum albumin-reduced manganese dioxide (BM) NPs self-assembled with cationic mannose cross-linked polyethyleneimine (Msp) from MSPAM nanocomplexes scavenged H2O2, inhibited HIF1α expression and reduced serum TNF-α and IL-6 (109).
Polymeric NPs promote macrophage pro-inflammatory activity in sepsis immunosuppression stage. Apoptosis, endotoxin tolerance, metabolic reprogramming, and changes in inflammatory pathway are involved in the immunosuppressive state of sepsis (37). In the immunosuppression stage, the host often dies due to organ dysfunction (132), and nanomaterials can induce macrophage proinflammatory reaction to improve the survival rate of patients (95). iE-DAP is a drug that promotes intracellular receptor NOD1 activation and induces pro-inflammatory factor gene expression, but cannot be internalized by macrophage. After be encapsulated by poly(3-hydroxybutyrate-co-3-hydroxyvalerate) (PHBV), iE-DAP-PHBV can be effectively internalized into macrophages activateing NOD1 signaling to induce activation of the NF-κB pathway and secrete IL-6 and TNF-α against inflammation (84). Zhao et al. loaded monphosphatidyl lipid A (MPLA) and muramyl dipeptide (MDP) into poly(lactide-co-glycolide) (PLGA) NPs and combined them with alginate (ALG) to develop two-phase release immunostimulatory composite NPs (MDP+P-M@ALG). MDP+P-M@ALG improves macrophage phagocytic and bactericidal functions, the survival of CLP-induced sepsis mouse models and the resistance of surviving mice to secondary infections, providing long-term sepsis protection (24).
Polymeric NPs regulate macrophage reprogramming. Macrophages activated in inflammation are generally divided into two types, pro-inflammatory M1 and anti-inflammatory M2 macrophages (133). NP can change the inflammatory environment by regulating the activated macrophage state and thus treat diseases (77). For example, Jiang et al. prepared chitosan-based nanoparticles (CN) loaded with tripolyphosphate that dynamically regulated M1-M2 macrophage reprogramming. In M1-like macrophages, CN decreased CD86 and iNOS expression, and increased Arg-1 and IL-10 expression; in M2-like macrophages, CN decreased Arg-1 expression, and increased CD86, iNOS and TNF-α expression. The biphasic polarization was achieved by STAT-1/STAT-6 signaling pathway transformation. Therefore, CN alter macrophage polarization homeostasis and thus can be used for treating sepsis (134).
Polymeric NPs inhibit macrophage pyroptosis. Pyroptosis is a caspases-mediated cell death, which GSDMD accumulates in cell membrane to form pores causing cell membrane collapse, inducing the release of lots of cytokines, including IL-1β, ultimately leading to dramatically abnormal activation of immune cells (135). NPs can serve as carriers prevent its occurrence (115). For example, Ou et al. prepared a disulfiram-lactoferrin nanocomplex (DSF-LF NPs), a naturally occurring powerful antibacterial and anti-inflammatory protein, with DSF, a drug that inhibits gasdermin D (GSDMD)-induced pyroptosis (115). LF binds specifically to low-density lipoprotein receptor-related protein-associated protein (LRP-1) and promotes phagocytosis of NPs by macrophages, and has immunomodulatory effects (115). Utilizing the immunosuppressive activity of LF and DSF, DSF-LF NPs effectively inhibit macrophage pyroptosis and proinflammatory cytokine release process with significant efficacy in LPS-induced sepsis (115). In addition, a siHMGB1 liponanocomplex can be engulfed by macrophage via the mannose receptor to form endolysosomes. Endolysosomes can release active factors to silence the transcription of high mobility group box protein 1 (HMGB1), thus inhibiting pyroptosis (136).
Liposomes as drug carriers target macrophages
Liposomes are widely used as drug carriers for small molecule, peptide, protein, gene and antibody delivery due to their high drug encapsulation, low drug toxicity, good targeting, good biocompatibility, biodegradability, and optimized biodegradability pharmacokinetic properties (77, 137–139). Liposomes are phagocytosed by macrophages after entering the body through intravenous injection, forming a natural aggregation effect and realizing macrophage targeting (140).
Liposomes promote macrophage anti-inflammatory activity in sepsis overactivation stage. Liposomes loaded guanabenz regulates macrophage anti-inflammatory activity through eukaryotic initiation factor 2 (eIF2α) dependent signaling, which downregulates IL-6 and cyclooxygenase 2 (COX-2) and also through eIF2α non-dependent signaling, which downregulates IL1β, TNFα, significantly reduced the cytokines secreted by macrophages (94).
Liposomes promote macrophage pro-inflammatory activity in sepsis immunosuppression stage. Hou et al. constructed an antimicrobial peptide, cathepsin B mRNA (AMP-cat B mRNA), encoding AMP-IB367 and Cat-B, which was encapsulated in vitamin liposomes. The vitamin liposomes promote the accumulation of NPs in the lysosomes of macrophages (107). Such macrophages assembled with AMP-cat-B@VLMP could eliminate MDR bacteria in septic mice in an immunosuppressive state (107), providing an alternative strategy to overcome sepsis caused by multidrug-resistant bacteria. Moreover, wheat germ agglutinin (WGA)-modified liposomes encapsulating clarithromycin is used for bacterial target delivery and enhancement of host immune defense by improving the uptake of bacteria by macrophages and inhibiting bacteria growth (141).
Liposomes regulate macrophage reprogramming. M2 macrophages treated with PEGylated liposomes containing IFN-γ expressed elevated NO and decreased arginase levels, suggesting that such liposomes enhanced the targeted delivery of drugs to macrophages and promoted M2 to M1 polarization (78).
Biomimetic macrophage NPs
Biomimetic macrophage membrane-coated NPs can cross biological barriers, enable the cargo to precisely target the lesion and avoid immune recognition (80, 142, 143).
Biomimetic NPs can both inhibit and promote macrophage phagocytosis. Wang et al. reported a biomimetic NP (MM/RAPNPs) that coats macrophage membranes on the surface of PLGA NPs assembled with rapamycin (RAPNPs) (56). Due to the MM coating, MM/RAPNPs, possessing good biocompatibility, biosafety, and targeting properties, effectively inhibited macrophage phagocytosis in vitro (144) and efficiently targeted aggregation to lesions in vivo (56). CD47, a ligand for signal-regulated protein-α (SIRPα) on macrophages (145), upon binding to SIRPα, SIRPα activates phosphatase-1 (SHP-1), which contains the Src homology 2 domain, to regulate intracellular signaling and inhibit cellular phagocytosis (146). Related studies reported that magnetic NPs (gCM-MNs) encapsulated by gene-edited cell membranes effectively blocked the CD47-SIRPα signaling pathway and could elicit robust macrophage phagocytosis (85).
Biomimetic NPs promote macrophage anti-inflammatory activity in sepsis overactivation stage. Lu et al. developed a biomimetic nanomedicine (MM-CEP/NLCs) containing cefadroxil (CEP) nanolipid carriers (NLCs) inside and MM encapsulated outside. Due to its biocompatibility and targeting, biomimetic macrophage membrane allows effective accumulation of MM-CEP/NLCs in lung inflammation, achieving sustained drug release and circulation and therapeutic lung inflammation effects (81).
Biomimetic NPs eliminate PAMPs. Macrophage-mimetic NPs (MΦ-NPs) combine polymeric cores with macrophage cell membranes, possessing LPS binding sites (e.g., CD126, CD14 and TLR4) with long circulation times and low toxicity (147, 148). MΦ-NPs can capture and eliminate LPS and damage-associated molecular pattern molecules (DAMPs), reducing the free LPS level in the serum and overexcitation of immune cells and alleviating LPS-induced sepsis in mice (23, 147, 148).
Biomimetic NPs regulate macrophage reprogramming. Engineered macrophages carrying nanodrugs containing oxaliplatin prodrug and photosensitizer induce conversion of M2 macrophages to M1 macrophages as evidenced by increasing of iNOS (M1 marker) and decreasing of Arg-1 (M2 marker), which realized by macrophage-mimetic NP-mediated, light-triggered accurate delivery of drugs (80).
Exosomes derived from macrophages serve as drug carriers
Exosomes exhibit low immunogenicity, excellent biocompatibility, and immune inertness, and can carry various drugs.
Exosomes regulate macrophage reprogramming. Pei et al. designed an EM-PLGA@Dnmt3aos smart silencer by isolating natural exosomes from M2 macrophages and centrifugation encapsulating a PLGA@Dnmt3aos smart silencer (103). Long non-coding RNAs (lncRNAs) were differentially expressed in M1/M2 macrophages (103). Among them, DNA methyltransferase 3A, opposite strand (Dnmt3aos) is a known lncRNA located on the antisense strand of DNA methyltransferase 3A (Dnmt3a), which highly expressed in M2 macrophages and regulates the expression of Dnmt3a. Smart silencers consist of three small interfering RNAs (siRNAs) and three antisense oligonucleotides (ASOs) that play an important role in mediating sequence-specific silencing of a given target gene. When PLGA@Dnmt3aos-smart silencer encapsulated by M2 macrophage-derived exosomal membranes was injected into allergic asthmatic mice, it effectively targeted M2 macrophages in the lungs and significantly inhibited the production of pro-inflammatory cytokines, demonstrating strong permeability, effective drug delivery, robust targeting, high stability and safety of the exosomes (103). Intercellular adhesion molecule 1 (ICAM-1)/lymphocyte function-associated antigen 1 (LFA-1), and vascular cell adhesion molecule 1 (VCAM-1)/very late antigen 4 (VLA-4), specifically bind to each other (149). ICAM-1 and VCAM-1 are only expressed by macrophages activated by LPS, and LFA-1 and VLA-4 are upregulated in exosomes derived from M2 macrophages, thus enabling targeted recognition of M2-derived exosomes with LPS-activated macrophages (150). The use of exosomes derived from M2 macrophages will encapsulate the plasmid DNA encoding IL-10, avoid the degradation of plasmid DNA by nucleases and adverse reactions of plasmid DNA (150). The exosomes realize the targeted transporting to M1 macrophage, and enhance the reprogramming of the M1 type to the M2 type macrophages, which was demonstrated by the upregulation of IL-10 and IL-4 and the downregulation of IL-1β and TNF-α (150).
Inorganic NPs as drug carriers target macrophages
The tunable optical and electronic properties, simple synthesis techniques, and biocompatibility of carbon nanomaterials make them promising for applications in in vitro and in vivo biosensing, bioimaging, and drug delivery (151, 152).Metal NPs are representative of inorganic NPs, which offer considerable advantages as therapeutic platforms due to their high drug-carrying capacity, low immunogenicity, and biotunable targeting properties (153).
Inorganic NPs promote macrophage anti-inflammatory activity in sepsis overactivation stage. Currently, scientists combine ginsenoside compound K (CK) and peptide CopA3 on gold NPs (GNP-CK-CopA3) targeting RAW264.7 macrophages to decrease LPS-induced NF-κB/MAPK pathway activation (93). Gold NPs improve CK and CopA3 delivery efficiency (93). Pretreatment of RAW264.7 cells with GNP-CK-CopA3for 1 h followed by stimulation with LPS for 2 h resulted in significant inhibition of protein IκBα and p38 MAP phosphorylation and degradation in macrophages, indicating that GNP-CK-CopA3 inhibits macrophage anti-inflammatory activity (93).
In organic NPs promote macrophage pro-inflammatory activity. Steckiewicz et al. reported that AgNPs loaded with chlorhexidine or metronidazole enhance the antimicrobial roles, and IL-1β expression of RAW264.7 macrophages when compared with conventional chlorhexidine or metronidazole, demonstrating that AgNPs are effective cargo carriers (154).
Inorganic NPs regulate macrophage reprogramming. For example, mangostin-functionalized gold NPs (MGF-AuNPs) were applied to target the NF-kB pathway in splenic macrophages and regulated M2 polarization to M1, which was illustrated by a 10-fold elevation in IL-12, a 50-fold upregulation of TNF-α, and a twofold decrease in IL-6 and IL-10 (101). In sepsis, superparamagnetic iron oxide (SPIO) of γ-Fe2O3 NPs, which serve as an antibacterial agent, regulated macrophage reprogramming dependent on TRAF1 protein expressed by mesenchymal stem cells to treat septic liver injury (113).
It was concluded that NPs are excellent drug carriers to improve the traditional sepsis therapy efficacy. Equally important, it needs to be emphasized that the functional NPs should be selected properly for sepsis patients in different immune states (such as pro-inflammatory NPs for the immune paralysis state), and the selection of inappropriate NPs will exert adverse effects on the organism (such as anti-inflammatory NPs for the immune paralysis state).
Nano-molecular drugs targeting macrophage
In addition to being used as drug carriers, nanomaterial itself can be used as macrophage immunomodulator.
NPs inhibit macrophage phagocytosis. As early as 2013, Kodali et al. reported that silica and SPIO NPs could diminish the phagocytic activity of macrophages toward S. pneumoniae (90). SPIO have a common recognition receptor with Streptococcus pneumoniae-class a macrophage scavenger receptor (SR-A). SR-A binds SPIO by the charge interaction between the anionic group on the surface of nanoparticles and the lysine rich region of the receptor collagen like (CL) domain. Transcriptional reprogramming induced by SPIO leads to decreased phagocytosis of pathogens by macrophages. Additionally, Palomba et al. combined the natural fatty acid methyl palmitate with albumin to constitute a stable spherical NP capable of inducing macrophages into a dormant state and inhibiting their phagocytosis (91). The albumin acts as a structural support and methyl palmitate regulates the internalization ability of macrophages (91).
NPs inhibit inflammatory pathways. As an antioxidant, CeO2 NPs is biosafe and can effectively intervene in disease processes (155). CeO2NPs synthesized by biological and materials engineering effectively reduce the superoxide flux of the mitochondrial electron transport chain (METC) and plasma membrane nicotinamide adenine dinucleotide phosphate (NADPH) oxidase (NOX), which regulate the antioxidant activity of macrophages (69). Moreover, CeO2NPs reduce MAP kinase/NF-kB-mediated signaling pathway activation by attenuating LPS induced IKB- α dilapidation and the nuclear translocation of NF KB/p65 (25, 26, 108). Macrophages exposed to CeO2NPs show downregulation of LPS-induced cytokine release (IL-1β, IL-6, TNF-α, HMGB1) (26, 108). Cargo-free loaded immunomodulatory NPs (iNPs) can interact with macrophages to regulate inflammatory processes (110, 111, 156). Furthermore, it was shown that cargo-free loaded iNPs reduced LPS-induced NF-κB p65 and MAPK p38 activation (156). This immunomodulatory property of cargo-free loaded iNPs is converted to a survival advantage in a lethal dose of LPS-induced sepsis mouse model (111). Therefore, nanomaterials inhibit their phagocytosis and inflammatory pathway activation, which are used to inhibit the overactivation of macrophages in sepsis.
NPs promote macrophage phagocytosis. A study reported that multiwalled carbon nanotubes (MWCNTs) mediate the activation of alveolar and parenchymal macrophages by CD40 and CD80 upregulation (82). Additionally, MWCNTs were recognized by the T-cell immunoglobulin mucin 4 (Tim4) receptor of macrophages, induced activation of the macrophage NLRP3 inflammasome, and enhanced phagocytosis of macrophages (83).
NPs promote inflammatory pathway. Silica NPs, iron oxide NPs (IONPs), and PLGA NPs can mediate the secretion of TNF-α by macrophages, which are involved in proinflammatory processes (86, 157). Moreover, an emerging nanomaterial called carbon dots (CDs) can target macrophages in lung tissue and induce macrophage endoplasmic reticulum stress (87). After coculture, macrophages phagocytosed CDs to induce NLRP3 inflammasome activation and proinflammatory cytokine secretion, which was proven by increased IL-1β and IL-8 (87). Additionally, polystyrene spheres denatured by amine treatment with a size of 60 nm can induce ROS production in macrophages by 20 μg/ml (88). Moreover, a nano copolymer can be endocytosed by macrophages to induce ROS production (89).Together, NPs could play a critical immunomodulatory role in the immunosuppressed state of sepsis.
NPs promote macrophage reprogramming. Peled et al. designed drug-free amphiphilic polymeric NPs generated by the self-assembly of hydrolyzed galactomannan (hGM)-linked copolymers (95). The drug-free amphiphilic polymeric NPs can be recognized by macrophage surface receptors (e.g. lectin-like receptors) polarize M1 macrophages to the M2 macrophage, as confirmed by the downregulation of the M1 marker (CD80) and the upregulation of M2 markers (CD163 and CD206) (95). Zhao et al. constructed Fe3O4@C/MnO2 NPs, which show promising photothermal functions and magnetic and catalytic activities, and can be implemented to induce M2-type macrophages to polarize to M1-type macrophages (158).Therefore, active intervention of engineered NPs in the M1-M2 macrophage polarization process could be applied to sepsis therapies.
NPs monitoring macrophage function
The high-throughput platform is a monitoring platform that can use the biosensor developed by engineers to achieve continuous monitoring of cell behavior (159, 160). Currently, developed sensors are used to monitor macrophage function (74, 75). Monitor macrophage immune status. NPs are recognized and bound to receptors on the cell surface, initiating phagocytosis by macrophages and finally forming phagosomes (161). Recently, Law et al. designed an optical nanosensor that feeds back information about the environmental pH by monitoring changes in the Raman spectrum of p-mercaptobenzoic acid (p-MBA) to probe macrophage phagosome function (74). The optical nanosensor (p-MBA-NP) uses p-MBA-functionalized AuNPs as material and measures pH in macrophage phagosomes, which can be measured by changes in Raman spectra caused by the response of carboxyl groups to hydrogen ion concentrations in the environment, representing a new and precise means to evaluate macrophage function (74).
Monitor macrophage immunotherapy effect. Nanotechnology enables real-time monitoring of the physicochemical properties of macrophages and is used to observe the response to immunotherapy. For instance, a noninvasive imaging nitric oxide (NO) nanodetector allows real-time monitoring for macrophage immunotherapy (75). The detector promotes the assembly of NO imaging probes with colipids to construct a NO nanoreporter (NO-NR) liposome NP system, which monitors NO production during M1-M2 polarization in real time, reflecting the macrophage immunotherapeutic response (75).
Monitor macrophage temporal and spatial location. Apart from observation for therapeutic effect, marking macrophage locations is of great importance (162). As early as 2010, Wong et al. implemented nanomaterials for sepsis monitoring based on the phagocytosis of macrophages through quantitative susceptibility mapping (QSM) magnetic resonance (MRI) imaging to quantify iron (106). Once “Feridex”, a class of superparamagnetic iron oxide NP contrast agents, enters the body, they will be rapidly swallowed by Kupffer cells. Quantification of “Feridex” taken up by Kupffer cells by QSM MRI and linking the result to the immune response in sepsis progression may enable monitoring sepsis status (106). In addition, spherical dextran NP 64Cu-Macrin assembled from nontoxic polydextrose is used as a nanotracer for positron emission tomography (PET) for quantitative noninvasive assessment of cardiocpulmonary macrophages. This nanotracer can be used to investigate the spatiotemporal dynamics of macrophages in sepsis and act as an imaging biomarker for macrophages (Figure 5) (105). In 2021, Raja et al. developed chemically modified cellulose nanocrystal (CNC) derivatives by covalently linking PEGylated biotin and a perylene diimide (PDI)-based near-infrared organic dye to label and image J774A.1 macrophages in a dose-dependent manner, which realize the monitoring macrophage localization (76).
Therefore, nanomaterials could not only detect the localization of macrophages for the determination of tissue and organ damage severity but also assess the functional status of macrophages, which shows broad potential in the study and real-time monitoring of sepsis.
Challenges and prospects of nanomedicine in sepsis
As a life-threatening pathophysiological syndrome, sepsis has a complex pathogenesis, in which the involvement of macrophages is particularly critical. The complicated pathophysiology of sepsis changes the phenotype and function of macrophages and induces macrophage exhaustion. At present, the drugs targeting macrophage function and the detection of macrophage function are still insufficient (4).Nanomaterials are promising candidates for targeting macrophages in sepsis. As drug carriers, NPs encapsulate and sequester active ingredients, enhancing macrophage-targeted time and specific delivery and/or improving drug biodistribution and/or bioavailability. In addition, nanomaterials modified by supramolecular structures can modulate macrophage function (17). For example, NPs can inhibit/enhance macrophage phagocytosis (90, 91) and suppress/promote its inflammatory pathways and cytokines secretion (82–84, 87, 89, 92, 93, 109, 163). NPs mediate M1-2 macrophage reprogramming as well (164, 165). Therefore, nanomaterials have the potential to treat macrophage-associated diseases, especially sepsis. Except for treatment, numerous clinical monitoring technologies of NPs are emerging, such as electrochemical and immunosensors for identifying infections, organ dysfunction, and immune dysregulation state (51, 166, 167). Detecting the localization of macrophages via nanomaterials can determine the severity of organ and tissue damage, thereby monitoring the progression of various macrophage-related diseases in real time (76). Although indirectly recognizing the pro-/anti-inflammatory cytokines’ lack of specificity, it provides directable roles in observing the inflammatory state of macrophage-associated diseases (51, 168–171). Furthermore, finding sepsis-specific biomarkers remains a legacy challenge. There is no doubt that the introduction of nanotechnology into preclinical studies in sepsis-associated macrophage therapeutics has made remarkable progress and has become a prospect for clinical applications.
Many challenges remain in this field. First, there is a lack of studies that have reported NPs targeting the epigenetic alterations of sepsis-associated macrophages. In LPS-stimulated macrophages, chromatin reorganization of enhancer regions was enhanced compared with that in resting macrophages (172, 173). The molecular mechanisms underlying the epigenetic regulatory effects of LPS include upregulation of the histone demethylase KDM6B via NF-κB initiation (8) and accumulation of histone deacetylase at the promoters of IL-1β and TNF, which lead to altered gene transcription (174). Thus, storing damaged macrophage function by regulating epigenetic alterations may be a hotpot. Second, a related study showed that intestinal microflora disruption may be harmful to macrophage phagocytosis promoting sepsis (175). Macrophages in lung tissues from gut microbiota-deficient mice show altered cellular responses and metabolic pathways (175), which also provides prospective for sepsis-managing gut microbiology. Third, macrophages produce extracellular traps (ETs) in response to various microorganisms and have similar characteristics to neutrophil ETs, which could be further explored in relation to nanomedicine (176). Fourth, metabolic changes in macrophages are also integral to the progression of sepsis. Moreover, stress erythrophagocytosis by the monocyte/macrophage system in the spleen could induce immunosuppression in sepsis via the STAT1 pathway (177). Consequently, there are many difficulties that can be further explored in the future. Furthermore, nanomaterials are widely applied for therapeutic interventions, but relatively few are designed to monitor macrophage function. Achieving effective monitoring of immune function in sepsis greatly guides subsequent treatment. Thus, the detection of the macrophage state needs to be achieved at a deeper level.
Conclusion
To conclude, sepsis is a highly heterogeneous and clinically refractory syndrome. Based on the functional diversity and plasticity of macrophages, nanomedicine has achieved excellent breaks in the management of sepsis. However, the design of sepsis-state responsive nanotherapies interacting with the diversity and plasticity of macrophages is a clinical component that needs to be further explored. There is no question that the exploration process requires multidisciplinary collaboration among critical care medicine, immunology, molecular biology, biochemistry, pharmacology, and materials science.
Author contributions
All authors listed have made a substantial, direct and intellectual contribution to the work, and approved it for publication. CS, JX, CG, WZ and XF wrote the review and designed the figures. YS revised the manuscript. All authors contributed to the article and approved the submitted version.
Funding
This work was supported by National Natural Science Foundation of China (No. 81772047, 81971818 82002026 and 82272217).
Acknowledgments
Thanks for researchers who contribute to the exploration for nanotechnology in medicine.
Conflict of interest
The authors declare that the research was conducted in the absence of any commercial or financial relationships that could be construed as a potential conflict of interest.
Publisher’s note
All claims expressed in this article are solely those of the authors and do not necessarily represent those of their affiliated organizations, or those of the publisher, the editors and the reviewers. Any product that may be evaluated in this article, or claim that may be made by its manufacturer, is not guaranteed or endorsed by the publisher.
Glossary
References
1. Deutschman CS, Tracey KJ. Sepsis: current dogma and new perspectives. Immun (2014) 40(4):463–75. doi: 10.1016/j.immuni.2014.04.001
2. Rhee C, Jones TM, Hamad Y, Pande A, Varon J, O'Brien C, et al. Prevalence, underlying causes, and preventability of sepsis-associated mortality in US acute care hospitals. JAMA Netw Open (2019) 2(2):e187571. doi: 10.1001/jamanetworkopen.2018.7571
3. Rudd KE, Johnson SC, Agesa KM, Shackelford KA, Tsoi D, Kievlan DR, et al. Global, regional, and national sepsis incidence and mortality, 1990-2017: analysis for the global burden of disease study. Lancet (2020) 395(10219):200–11. doi: 10.1016/S0140-6736(19)32989-7
4. van der Poll T, van de Veerdonk FL, Scicluna BP, Netea MG. The immunopathology of sepsis and potential therapeutic targets. Nat Rev Immunol (2017) 17(7):407–20. doi: 10.1038/nri.2017.36
5. Locati M, Curtale G, Mantovani A. Diversity, mechanisms, and significance of macrophage plasticity. Annu Rev Pathol (2020) 15:123–47. doi: 10.1146/annurev-pathmechdis-012418-012718
6. Shapouri-Moghaddam A, Mohammadian S, Vazini H, Taghadosi M, Esmaeili SA, Mardani F, et al. Macrophage plasticity, polarization, and function in health and disease. J Cell Physiol (2018) 233(9):6425–40. doi: 10.1002/jcp.26429
7. Deng M, Tang Y, Li W, Wang X, Zhang R, Zhang X, et al. The endotoxin delivery protein HMGB1 mediates caspase-11-Dependent lethality in sepsis. Immunity (2018) 49(4):740–53 e7. doi: 10.1016/j.immuni.2018.08.016
8. De Santa F, Totaro MG, Prosperini E, Notarbartolo S, Testa G, Natoli G. The histone H3 lysine-27 demethylase Jmjd3 links inflammation to inhibition of polycomb-mediated gene silencing. Cell (2007) 130(6):1083–94. doi: 10.1016/j.cell.2007.08.019
9. Rivers E, Nguyen B, Havstad S, Ressler J, Muzzin A, Knoblich B, et al. Early goal-directed therapy in the treatment of severe sepsis and septic shock. N Engl J Med (2001) 345(19):1368–77. doi: 10.1056/NEJMoa010307
10. Ferrer R, Martin-Loeches I, Phillips G, Osborn TM, Townsend S, Dellinger RP, et al. Empiric antibiotic treatment reduces mortality in severe sepsis and septic shock from the first hour: results from a guideline-based performance improvement program. Crit Care Med (2014) 42(8):1749–55. doi: 10.1097/CCM.0000000000000330
11. Sterling SA, Miller WR, Pryor J, Puskarich MA, Jones AE. The impact of timing of antibiotics on outcomes in severe sepsis and septic shock: A systematic review and meta-analysis. Crit Care Med (2015) 43(9):1907–15. doi: 10.1097/CCM.0000000000001142
12. Casaer MP, Mesotten D, Hermans G, Wouters PJ, Schetz M, Meyfroidt G, et al. Early versus late parenteral nutrition in critically ill adults. N Engl J Med (2011) 365(6):506–17. doi: 10.1056/NEJMoa1102662
13. Gotts JE, Matthay MA. Sepsis: pathophysiology and clinical management. BMJ (2016) 353:i1585. doi: 10.1136/bmj.i1585
14. Marshall JC. Why have clinical trials in sepsis failed? Trends Mol Med (2014) 20(4):195–203. doi: 10.1016/j.molmed.2014.01.007
15. Makabenta JMV, Nabawy A, Li CH, Schmidt-Malan S, Patel R, Rotello VM. Nanomaterial-based therapeutics for antibiotic-resistant bacterial infections. Nat Rev Microbiol (2021) 19(1):23–36. doi: 10.1038/s41579-020-0420-1
16. Luo G, Zhang J, Sun Y, Wang Y, Wang H, Cheng B, et al. Nanoplatforms for sepsis management: Rapid Detection/Warning, pathogen elimination and restoring immune homeostasis. Nanomicro Lett (2021) 13:88. doi: 10.1007/s40820-021-00598-3
17. Shi J, Kantoff PW, Wooster R, Farokhzad OC. Cancer nanomedicine: progress, challenges and opportunities. Nat Rev Cancer (2017) 17(1):20–37. doi: 10.1038/nrc.2016.108
18. Jiang W, Yuan H, Chan CK, von Roemeling CA, Yan Z, Weissman IL, et al. Lessons from immuno-oncology: a new era for cancer nanomedicine? Nat Rev Drug Discovery (2017) 16(6):369–70. doi: 10.1038/nrd.2017.34
19. Lobatto ME, Fuster V, Fayad ZA, Mulder WJ. Perspectives and opportunities for nanomedicine in the management of atherosclerosis. Nat Rev Drug Discovery (2011) 10(11):835–52. doi: 10.1038/nrd3578
20. Yang Y, Guo L, Wang Z, Liu P, Liu X, Ding J, et al. Targeted silver nanoparticles for rheumatoid arthritis therapy via macrophage apoptosis and re-polarization. Biomaterials (2021) 264:120390. doi: 10.1016/j.biomaterials.2020.120390
21. Veiseh O, Tang BC, Whitehead KA, Anderson DG, Langer R. Managing diabetes with nanomedicine: challenges and opportunities. Nat Rev Drug Discovery (2015) 14(1):45–57. doi: 10.1038/nrd4477
22. Yang M, Li J, Gu P, Fan X. The application of nanoparticles in cancer immunotherapy: Targeting tumor microenvironment. Bioact Mater (2021) 6(7):1973–87. doi: 10.1016/j.bioactmat.2020.12.010
23. Wu B, Lin L, Zhou F, Wang X. Precise engineering of neutrophil membrane coated with polymeric nanoparticles concurrently absorbing of proinflammatory cytokines and endotoxins for management of sepsis. Bioprocess Biosyst Eng. (2020) 43(11):2065–74. doi: 10.1007/s00449-020-02395-5
24. Zhao H, Lv X, Huang J, Huang S, Zhou H, Wang H, et al. Two-phase releasing immune-stimulating composite orchestrates protection against microbial infections. Biomaterials (2021) 277:121106. doi: 10.1016/j.biomaterials.2021.121106
25. Selvaraj V, Manne ND, Arvapalli R, Rice KM, Nandyala G, Fankenhanel E, et al. Effect of cerium oxide nanoparticles on sepsis induced mortality and NF-kappaB signaling in cultured macrophages. Nanomed (Lond) (2015) 10(8):1275–88. doi: 10.2217/nnm.14.205
26. Selvaraj V, Nepal N, Rogers S, Manne ND, Arvapalli R, Rice KM, et al. Inhibition of MAP kinase/NF-kB mediated signaling and attenuation of lipopolysaccharide induced severe sepsis by cerium oxide nanoparticles. Biomaterials (2015) 59:160–71. doi: 10.1016/j.biomaterials.2015.04.025
27. Pant A, Mackraj I, Govender T. Advances in sepsis diagnosis and management: a paradigm shift towards nanotechnology. J BioMed Sci (2021) 28(1):6. doi: 10.1186/s12929-020-00702-6
28. Foster SL, Hargreaves DC, Medzhitov R. Gene-specific control of inflammation by TLR-induced chromatin modifications. Nature (2007) 447(7147):972–8. doi: 10.1038/nature05836
29. Chousterman BG, Swirski FK, Weber GF. Cytokine storm and sepsis disease pathogenesis. Semin Immunopathol (2017) 39(5):517–28. doi: 10.1007/s00281-017-0639-8
30. Tang D, Kang R, Berghe TV, Vandenabeele P, Kroemer G. The molecular machinery of regulated cell death. Cell Res (2019) 29(5):347–64. doi: 10.1038/s41422-019-0164-5
31. Sica A, Mantovani A. Macrophage plasticity and polarization: in vivo veritas. J Clin Invest (2012) 122(3):787–95. doi: 10.1172/JCI59643
32. Rackov G, Hernandez-Jimenez E, Shokri R, Carmona-Rodriguez L, Manes S, Alvarez-Mon M, et al. p21 mediates macrophage reprogramming through regulation of p50-p50 NF-kappaB and IFN-beta. J Clin Invest (2016) 126(8):3089–103. doi: 10.1172/JCI83404
33. Robinson N, Ganesan R, Hegedus C, Kovacs K, Kufer TA, Virag L. Programmed necrotic cell death of macrophages: Focus on pyroptosis, necroptosis, and parthanatos. Redox Biol (2019) 26:101239. doi: 10.1016/j.redox.2019.101239
34. van der Poll T, Shankar-Hari M, Wiersinga WJ. The immunology of sepsis. Immunity (2021) 54(11):2450–64. doi: 10.1016/j.immuni.2021.10.012
35. Tang D, Kang R, Coyne CB, Zeh HJ, Lotze MT. PAMPs and DAMPs: signal 0s that spur autophagy and immunity. Immunol Rev (2012) 249(1):158–75. doi: 10.1111/j.1600-065X.2012.01146.x
36. Netea MG, Balkwill F, Chonchol M, Cominelli F, Donath MY, Giamarellos-Bourboulis EJ, et al. A guiding map for inflammation. Nat Immunol (2017) 18(8):826–31. doi: 10.1038/ni.3790
37. Venet F, Monneret G. Advances in the understanding and treatment of sepsis-induced immunosuppression. Nat Rev Nephrol (2018) 14(2):121–37. doi: 10.1038/nrneph.2017.165
38. Kumar V. Targeting macrophage immunometabolism: Dawn in the darkness of sepsis. Int Immunopharmacol (2018) 58:173–85. doi: 10.1016/j.intimp.2018.03.005
39. Schultze JL, Schmidt SV. Molecular features of macrophage activation. Semin Immunol (2015) 27(6):416–23. doi: 10.1016/j.smim.2016.03.009
40. Guo RF, Ward PA. Role of C5a in inflammatory responses. Annu Rev Immunol (2005) 23:821–52. doi: 10.1146/annurev.immunol.23.021704.115835
41. Joffre J, Hellman J, Ince C, Ait-Oufella H. Endothelial responses in sepsis. Am J Respir Crit Care Med (2020) 202(3):361–70. doi: 10.1164/rccm.201910-1911TR
42. Zhang H, Zeng L, Xie M, Liu J, Zhou B, Wu R, et al. TMEM173 drives lethal coagulation in sepsis. Cell Host Microbe (2020) 27(4):556–70 e6. doi: 10.1016/j.chom.2020.02.004
43. Tang Y, Wang X, Li Z, He Z, Yang X, Cheng X, et al. Heparin prevents caspase-11-dependent septic lethality independent of anticoagulant properties. Immunity (2021) 54(3):454–67 e6. doi: 10.1016/j.immuni.2021.01.007
44. Shannon O. The role of platelets in sepsis. Res Pract Thromb Haemost (2021) 5(1):27–37. doi: 10.1002/rth2.12465
45. Wang J, Sahoo M, Lantier L, Warawa J, Cordero H, Deobald K, et al. Caspase-11-dependent pyroptosis of lung epithelial cells protects from melioidosis while caspase-1 mediates macrophage pyroptosis and production of IL-18. PloS Pathog (2018) 14(5):e1007105. doi: 10.1371/journal.ppat.1007105
46. Boraschi D, Italiani P, Palomba R, Decuzzi P, Duschl A, Fadeel B, et al. Nanoparticles and innate immunity: new perspectives on host defence. Semin Immunol (2017) 34:33–51. doi: 10.1016/j.smim.2017.08.013
47. Kinnear C, Moore TL, Rodriguez-Lorenzo L, Rothen-Rutishauser B, Petri-Fink A. Form follows function: Nanoparticle shape and its implications for nanomedicine. Chem Rev (2017) 117(17):11476–521. doi: 10.1021/acs.chemrev.7b00194
48. Drexler KE. Molecular engineering: An approach to the development of general capabilities for molecular manipulation. Proc Natl Acad Sci U S A (1981) 78(9):5275–8. doi: 10.1073/pnas.78.9.5275
49. Sadikot RT. The potential role of nano- and micro-technology in the management of critical illnesses. Adv Drug Delivery Rev (2014) 77:27–31. doi: 10.1016/j.addr.2014.07.004
50. Pelaz B, Alexiou C, Alvarez-Puebla RA, Alves F, Andrews AM, Ashraf S, et al. Diverse applications of nanomedicine. ACS Nano (2017) 11(3):2313–81. doi: 10.1021/acsnano.6b06040
52. Allen TM, Cullis PR. Liposomal drug delivery systems: from concept to clinical applications. Adv Drug Delivery Rev (2013) 65(1):36–48. doi: 10.1016/j.addr.2012.09.037
53. Zahednezhad F, Saadat M, Valizadeh H, Zakeri-Milani P, Baradaran B. Liposome and immune system interplay: Challenges and potentials. J Control Release (2019) 305:194–209. doi: 10.1016/j.jconrel.2019.05.030
54. Vauthier C, Bouchemal K. Methods for the preparation and manufacture of polymeric nanoparticles. Pharm Res (2009) 26(5):1025–58. doi: 10.1007/s11095-008-9800-3
55. Rothen-Rutishauser BM, Schurch S, Haenni B, Kapp N, Gehr P. Interaction of fine particles and nanoparticles with red blood cells visualized with advanced microscopic techniques. Environ Sci Technol (2006) 40(14):4353–9. doi: 10.1021/es0522635
56. Wang Y, Zhang K, Li T, Maruf A, Qin X, Luo L, et al. Macrophage membrane functionalized biomimetic nanoparticles for targeted anti-atherosclerosis applications. Theranostics (2021) 11(1):164–80. doi: 10.7150/thno.47841
57. Mathieu M, Martin-Jaular L, Lavieu G, Thery C. Specificities of secretion and uptake of exosomes and other extracellular vesicles for cell-to-cell communication. Nat Cell Biol (2019) 21(1):9–17. doi: 10.1038/s41556-018-0250-9
58. Hashemian SM, Pourhanifeh MH, Fadaei S, Velayati AA, Mirzaei H, Hamblin MR. Non-coding RNAs and exosomes: Their role in the pathogenesis of sepsis. Mol Ther Nucleic Acids (2020) 21:51–74. doi: 10.1016/j.omtn.2020.05.012
59. Valadi H, Ekstrom K, Bossios A, Sjostrand M, Lee JJ, Lotvall JO. Exosome-mediated transfer of mRNAs and microRNAs is a novel mechanism of genetic exchange between cells. Nat Cell Biol (2007) 9(6):654–9. doi: 10.1038/ncb1596
60. Yanez-Mo M, Siljander PR, Andreu Z, Zavec AB, Borras FE, Buzas EI, et al. Biological properties of extracellular vesicles and their physiological functions. J Extracell Vesicles (2015) 4:27066. doi: 10.3402/jev.v4.27066
61. Kalluri R, LeBleu VS. The biology, function, and biomedical applications of exosomes. Science (2020) 367(6478):eaau6977. doi: 10.1126/science.aau6977
62. Guo W, Li Y, Pang W, Shen H. Exosomes: A potential therapeutic tool targeting communications between tumor cells and macrophages. Mol Ther (2020) 28(9):1953–64. doi: 10.1016/j.ymthe.2020.06.003
63. Yan W, Jiang S. Immune cell-derived exosomes in the cancer-immunity cycle. Trends Cancer (2020) 6(6):506–17. doi: 10.1016/j.trecan.2020.02.013
64. Wu P, Zhang B, Ocansey DKW, Xu W, Qian H. Extracellular vesicles: A bright star of nanomedicine. Biomaterials (2021) 269:120467. doi: 10.1016/j.biomaterials.2020.120467
65. Tian T, Zhang HX, He CP, Fan S, Zhu YL, Qi C, et al. Surface functionalized exosomes as targeted drug delivery vehicles for cerebral ischemia therapy. Biomaterials (2018) 150:137–49. doi: 10.1016/j.biomaterials.2017.10.012
66. Zhu Q, Heon M, Zhao Z, He M. Microfluidic engineering of exosomes: editing cellular messages for precision therapeutics. Lab Chip. (2018) 18(12):1690–703. doi: 10.1039/c8lc00246k
67. Boisselier E, Astruc D. Gold nanoparticles in nanomedicine: preparations, imaging, diagnostics, therapies and toxicity. Chem Soc Rev (2009) 38(6):1759–82. doi: 10.1039/b806051g
68. Lee JH, Cho HY, Choi HK, Lee JY, Choi JW. Application of gold nanoparticle to plasmonic biosensors. Int J Mol Sci (2018) 19(7):2021. doi: 10.3390/ijms19072021
69. Li YR, Zhu H. Nanoceria potently reduce superoxide fluxes from mitochondrial electron transport chain and plasma membrane NADPH oxidase in human macrophages. Mol Cell Biochem (2021) 476(12):4461–70. doi: 10.1007/s11010-021-04246-7
70. Aderem A, Underhill DM. Mechanisms of phagocytosis in macrophages. Annu Rev Immunol (1999) 17:593–623. doi: 10.1146/annurev.immunol.17.1.593
71. Corbo C, Molinaro R, Tabatabaei M, Farokhzad OC, Mahmoudi M. Personalized protein corona on nanoparticles and its clinical implications. Biomater Sci (2017) 5(3):378–87. doi: 10.1039/c6bm00921b
72. Chen D, Ganesh S, Wang W, Amiji M. Plasma protein adsorption and biological identity of systemically administered nanoparticles. Nanomed (Lond) (2017) 12(17):2113–35. doi: 10.2217/nnm-2017-0178
73. Docter D, Westmeier D, Markiewicz M, Stolte S, Knauer SK, Stauber RH. The nanoparticle biomolecule corona: lessons learned - challenge accepted? Chem Soc Rev (2015) 44(17):6094–121. doi: 10.1039/c5cs00217f
74. Law SM, Stanfield SJ, Hardisty GR, Dransfield I, Campbell CJ, Gray RD. Human cystic fibrosis monocyte derived macrophages display no defect in acidification of phagolysosomes when measured by optical nanosensors. J Cyst Fibros (2020) 19(2):203–10. doi: 10.1016/j.jcf.2019.09.003
75. Ramesh A, Kumar S, Brouillard A, Nandi D, Kulkarni A. A nitric oxide (NO) nanoreporter for noninvasive real-time imaging of macrophage immunotherapy. Adv Mater (2020) 32(24):e2000648. doi: 10.1002/adma.202000648
76. Raja S, Hamouda AEI, de Toledo MAS, Hu C, Bernardo MP, Schalla C, et al. Functionalized cellulose nanocrystals for cellular labeling and bioimaging. Biomacromolecules (2021) 22(2):454–66. doi: 10.1021/acs.biomac.0c01317
77. Chang X, Xing L, Wang Y, Zhou TJ, Shen LJ, Jiang HL. Nanoengineered immunosuppressive therapeutics modulating M1/M2 macrophages into the balanced status for enhanced idiopathic pulmonary fibrosis therapy. Nanoscale (2020) 12(16):8664–78. doi: 10.1039/d0nr00750a
78. Kateh Shamshiri M, Jaafari MR, Badiee A. Preparation of liposomes containing IFN-gamma and their potentials in cancer immunotherapy: In vitro and in vivo studies in a colon cancer mouse model. Life Sci (2021) 264:118605. doi: 10.1016/j.lfs.2020.118605
79. Hongsa N, Thinbanmai T, Luesakul U, Sansanaphongpricha K, Muangsin N. A novel modified chitosan/collagen coated-gold nanoparticles for 5-fluorouracil delivery: Synthesis, characterization, in vitro drug release studies, anti-inflammatory activity and in vitro cytotoxicity assay. Carbohydr Polym (2022) 277:118858. doi: 10.1016/j.carbpol.2021.118858
80. Huang Y, Guan Z, Dai X, Shen Y, Wei Q, Ren L, et al. Engineered macrophages as near-infrared light activated drug vectors for chemo-photodynamic therapy of primary and bone metastatic breast cancer. Nat Commun (2021) 12(1):4310. doi: 10.1038/s41467-021-24564-0
81. Lu C, Zheng J, Ding Y, Meng Y, Tan F, Gong W, et al. Cepharanthine loaded nanoparticles coated with macrophage membranes for lung inflammation therapy. Drug Deliv (2021) 28(1):2582–93. doi: 10.1080/10717544.2021.2009936
82. Beyeler S, Steiner S, Wotzkow C, Tschanz SA, Adhanom Sengal A, Wick P, et al. Multi-walled carbon nanotubes activate and shift polarization of pulmonary macrophages and dendritic cells in an in vivo model of chronic obstructive lung disease. Nanotoxicology (2020) 14(1):77–96. doi: 10.1080/17435390.2019.1663954
83. Omori S, Tsugita M, Hoshikawa Y, Morita M, Ito F, Yamaguchi SI, et al. Tim4 recognizes carbon nanotubes and mediates phagocytosis leading to granuloma formation. Cell Rep (2021) 34(6):108734. doi: 10.1016/j.celrep.2021.108734
84. Cabana-Brunod M, Herrera PA, Marquez-Miranda V, Llancalahuen FM, Duarte Y, Gonzalez-Nilo D, et al. Development of a PHBV nanoparticle as a peptide vehicle for NOD1 activation. Drug Deliv (2021) 28(1):1020–30. doi: 10.1080/10717544.2021.1923862
85. Rao L, Zhao SK, Wen C, Tian R, Lin L, Cai B, et al. Activating macrophage-mediated cancer immunotherapy by genetically edited nanoparticles. Adv Mater (2020) 32(47):e2004853. doi: 10.1002/adma.202004853
86. Saborano R, Wongpinyochit T, Totten JD, Johnston BF, Seib FP, Duarte IF. Metabolic reprogramming of macrophages exposed to silk, poly(lactic-co-glycolic acid), and silica nanoparticles. Adv Healthc Mater (2017) 6(14):1601240. doi: 10.1002/adhm.201601240
87. Weiss M, Fan J, Claudel M, Lebeau L, Pons F, Ronzani C. Combined In vitro and In vivo approaches to propose a putative adverse outcome pathway for acute lung inflammation induced by nanoparticles: A study on carbon dots. Nanomaterials (Basel) (2021) 11(1):180. doi: 10.3390/nano11010180
88. Chiu HW, Xia T, Lee YH, Chen CW, Tsai JC, Wang YJ. Cationic polystyrene nanospheres induce autophagic cell death through the induction of endoplasmic reticulum stress. Nanoscale (2015) 7(2):736–46. doi: 10.1039/c4nr05509h
89. Eidi H, Joubert O, Nemos C, Grandemange S, Mograbi B, Foliguet B, et al. Drug delivery by polymeric nanoparticles induces autophagy in macrophages. Int J Pharm (2012) 422(1-2):495–503. doi: 10.1016/j.ijpharm.2011.11.020
90. Kodali V, Littke MH, Tilton SC, Teeguarden JG, Shi L, Frevert CW, et al. Dysregulation of macrophage activation profiles by engineered nanoparticles. ACS Nano (2013) 7(8):6997–7010. doi: 10.1021/nn402145t
91. Palomba R, di Francesco M, di Francesco V, Piccardi F, Catelani T, Ferreira M, et al. Boosting nanomedicine performance by conditioning macrophages with methyl palmitate nanoparticles. Mater Horiz. (2021) 8(10):2726–41. doi: 10.1039/d1mh00937k
92. Ma L, Shen CA, Gao L, Li DW, Shang YR, Yin K, et al. Anti-inflammatory activity of chitosan nanoparticles carrying NF-kappaB/p65 antisense oligonucleotide in RAW264.7 macropghage stimulated by lipopolysaccharide. Colloids Surf B Biointerfaces (2016) 142:297–306. doi: 10.1016/j.colsurfb.2016.02.031
93. Liu Y, Perumalsamy H, Kang CH, Kim SH, Hwang JS, Koh SC, et al. Intracellular synthesis of gold nanoparticles by gluconacetobacter liquefaciens for delivery of peptide CopA3 and ginsenoside and anti-inflammatory effect on lipopolysaccharide-activated macrophages. Artif Cells Nanomed Biotechnol (2020) 48(1):777–88. doi: 10.1080/21691401.2020.1748639
94. Tian M, Ticer T, Wang Q, Walker S, Pham A, Suh A, et al. Adipose-derived biogenic nanoparticles for suppression of inflammation. Small (2020) 16(10):e1904064. doi: 10.1002/smll.201904064
95. Peled E, Sosnik A. Amphiphilic galactomannan nanoparticles trigger the alternative activation of murine macrophages. J Control Release (2021) 339:473–83. doi: 10.1016/j.jconrel.2021.10.017
96. Toita R, Kawano T, Murata M, Kang JH. Bioinspired macrophage-targeted anti-inflammatory nanomedicine: A therapeutic option for the treatment of myocarditis. Mater Sci Eng C Mater Biol Appl (2021) 131:112492. doi: 10.1016/j.msec.2021.112492
97. Sharifiaghdam M, Shaabani E, Sharifiaghdam Z, De Keersmaecker H, Lucas B, Lammens J, et al. Macrophage reprogramming into a pro-healing phenotype by siRNA delivered with LBL assembled nanocomplexes for wound healing applications. Nanoscale (2021) 13(36):15445–63. doi: 10.1039/d1nr03830c
98. Guo M, Wang S, Guo Q, Hou B, Yue T, Ming D, et al. NIR-responsive spatiotemporally controlled cyanobacteria micro-nanodevice for intensity-modulated chemotherapeutics in rheumatoid arthritis. ACS Appl Mater Interfaces (2021) 13(16):18423–31. doi: 10.1021/acsami.0c20514
99. Wei Y, Wang Z, Yang J, Xu R, Deng H, Ma S, et al. Reactive oxygen species / photothermal therapy dual-triggered biomimetic gold nanocages nanoplatform for combination cancer therapy via ferroptosis and tumor-associated macrophage repolarization mechanism. J Colloid Interface Sci (2022) 606(Pt 2):1950–65. doi: 10.1016/j.jcis.2021.09.160
100. Guo Z, He H, Zhang Y, Rao J, Yang T, Li T, et al. Heavy-Atom-Modulated supramolecular assembly increases antitumor potency against malignant breast tumors via tunable cooperativity. Adv Mater (2021) 33(2):e2004225. doi: 10.1002/adma.202004225
101. Khoobchandani M, Khan A, Katti KK, Thipe VC, Al-Yasiri AY, MohanDoss DKD, et al. Green nanotechnology of MGF-AuNPs for immunomodulatory intervention in prostate cancer therapy. Sci Rep (2021) 11(1):16797. doi: 10.1038/s41598-021-96224-8
102. Xu B, Cui Y, Wang W, Li S, Lyu C, Wang S, et al. Immunomodulation-enhanced nanozyme-based tumor catalytic therapy. Adv Mater (2020) 32(33):e2003563. doi: 10.1002/adma.202003563
103. Pei W, Li X, Bi R, Zhang X, Zhong M, Yang H, et al. Exosome membrane-modified M2 macrophages targeted nanomedicine: Treatment for allergic asthma. J Control Release (2021) 338:253–67. doi: 10.1016/j.jconrel.2021.08.024
104. Nie W, Wu G, Zhang J, Huang LL, Ding J, Jiang A, et al. Responsive exosome nano-bioconjugates for synergistic cancer therapy. Angew Chem Int Ed Engl (2020) 59(5):2018–22. doi: 10.1002/anie.201912524
105. Nahrendorf M, Hoyer FF, Meerwaldt AE, van Leent MMT, Senders ML, Calcagno C, et al. Imaging cardiovascular and lung macrophages with the positron emission tomography sensor (64)Cu-macrin in mice, rabbits, and pigs. Circ Cardiovasc Imaging (2020) 13(10):e010586. doi: 10.1161/CIRCIMAGING.120.010586
106. Wong R, Shou J, Wang Y. Probing sepsis and sepsis-like conditions using untargeted SPIO nanoparticles. Annu Int Conf IEEE Eng Med Biol Soc (2010) 2010:3053–6. doi: 10.1109/IEMBS.2010.5626123
107. Hou X, Zhang X, Zhao W, Zeng C, Deng B, McComb DW, et al. Vitamin lipid nanoparticles enable adoptive macrophage transfer for the treatment of multidrug-resistant bacterial sepsis. Nat Nanotechnol (2020) 15(1):41–6. doi: 10.1038/s41565-019-0600-1
108. Chen G, Xu Y. Biosynthesis of cerium oxide nanoparticles and their effect on lipopolysaccharide (LPS) induced sepsis mortality and associated hepatic dysfunction in male sprague dawley rats. Mater Sci Eng C Mater Biol Appl (2018) 83:148–53. doi: 10.1016/j.msec.2017.11.014
109. Rajendrakumar SK, Revuri V, Samidurai M, Mohapatra A, Lee JH, Ganesan P, et al. Peroxidase-mimicking nanoassembly mitigates lipopolysaccharide-induced endotoxemia and cognitive damage in the brain by impeding inflammatory signaling in macrophages. Nano Lett (2018) 18(10):6417–26. doi: 10.1021/acs.nanolett.8b02785
110. Getts DR, Terry RL, Getts MT, Deffrasnes C, Muller M, van Vreden C, et al. Therapeutic inflammatory monocyte modulation using immune-modifying microparticles. Sci Transl Med (2014) 6(219):219ra7. doi: 10.1126/scitranslmed.3007563
111. Lasola JJM, Cottingham AL, Scotland BL, Truong N, Hong CC, Shapiro P, et al. Immunomodulatory nanoparticles mitigate macrophage inflammation via inhibition of PAMP interactions and lactate-mediated functional reprogramming of NF-kappaB and p38 MAPK. Pharmaceutics (2021) 13(11):1841. doi: 10.3390/pharmaceutics13111841
112. Taratummarat S, Sangphech N, Vu CTB, Palaga T, Ondee T, Surawut S, et al. Gold nanoparticles attenuates bacterial sepsis in cecal ligation and puncture mouse model through the induction of M2 macrophage polarization. BMC Microbiol (2018) 18(1):85. doi: 10.1186/s12866-018-1227-3
113. Xu Y, Liu X, Li Y, Dou H, Liang H, Hou Y. SPION-MSCs enhance therapeutic efficacy in sepsis by regulating MSC-expressed TRAF1-dependent macrophage polarization. Stem Cell Res Ther (2021) 12(1):531. doi: 10.1186/s13287-021-02593-2
114. Xu Y, Li Y, Liu X, Pan Y, Sun Z, Xue Y, et al. SPIONs enhances IL-10-producing macrophages to relieve sepsis via Cav1-Notch1/HES1-mediated autophagy. Int J Nanomed (2019) 14:6779–97. doi: 10.2147/IJN.S215055
115. Ou AT, Zhang JX, Fang YF, Wang R, Tang XP, Zhao PF, et al. Disulfiram-loaded lactoferrin nanoparticles for treating inflammatory diseases. Acta Pharmacol Sin (2021) 42(11):1913–20. doi: 10.1038/s41401-021-00770-w
116. Ren H, He Y, Liang J, Cheng Z, Zhang M, Zhu Y, et al. Role of liposome size, surface charge, and PEGylation on rheumatoid arthritis targeting therapy. ACS Appl Mater Interfaces (2019) 11(22):20304–15. doi: 10.1021/acsami.8b22693
117. Wang C, Zhang Y, Dong Y. Lipid nanoparticle-mRNA formulations for therapeutic applications. Acc Chem Res (2021) 54(23):4283–93. doi: 10.1021/acs.accounts.1c00550
118. Hafner AM, Corthesy B. Merkle HP. particulate formulations for the delivery of poly(I:C) as vaccine adjuvant. Adv Drug Delivery Rev (2013) 65(10):1386–99. doi: 10.1016/j.addr.2013.05.013
119. Lynn GM, Laga R, Darrah PA, Ishizuka AS, Balaci AJ, Dulcey AE, et al. In vivo characterization of the physicochemical properties of polymer-linked TLR agonists that enhance vaccine immunogenicity. Nat Biotechnol (2015) 33(11):1201–10. doi: 10.1038/nbt.3371
120. Malyala P, O'Hagan DT, Singh M. Enhancing the therapeutic efficacy of CpG oligonucleotides using biodegradable microparticles. Adv Drug Delivery Rev (2009) 61(3):218–25. doi: 10.1016/j.addr.2008.12.009
121. Demoulins T, Bassi I, Thomann-Harwood L, Jandus C, Kaeuper P, Simon HU, et al. Alginate-coated chitosan nanogel capacity to modulate the effect of TLR ligands on blood dendritic cells. Nanomedicine (2013) 9(6):806–17. doi: 10.1016/j.nano.2013.01.002
122. Xu H, Luo R, Dong L, Pu X, Chen Q, Ye N, et al. pH/ROS dual-sensitive and chondroitin sulfate wrapped poly (beta-amino ester)-SA-PAPE copolymer nanoparticles for macrophage-targeted oral therapy for ulcerative colitis. Nanomedicine (2022) 39:102461. doi: 10.1016/j.nano.2021.102461
123. Kumar S, Ingle H, Prasad DV, Kumar H. Recognition of bacterial infection by innate immune sensors. Crit Rev Microbiol (2013) 39(3):229–46. doi: 10.3109/1040841X.2012.706249
124. Shirasuna K, Karasawa T, Takahashi M. Exogenous nanoparticles and endogenous crystalline molecules as danger signals for the NLRP3 inflammasomes. J Cell Physiol (2019) 234(5):5436–50. doi: 10.1002/jcp.27475
125. Liu X, Lu B, Fu J, Zhu X, Song E, Song Y. Amorphous silica nanoparticles induce inflammation via activation of NLRP3 inflammasome and HMGB1/TLR4/MYD88/NF-kb signaling pathway in HUVEC cells. J Hazard Mater (2021) 404(Pt B):124050. doi: 10.1016/j.jhazmat.2020.124050
126. Palomaki J, Valimaki E, Sund J, Vippola M, Clausen PA, Jensen KA, et al. Long, needle-like carbon nanotubes and asbestos activate the NLRP3 inflammasome through a similar mechanism. ACS Nano (2011) 5(9):6861–70. doi: 10.1021/nn200595c
127. Bertrand N, Grenier P, Mahmoudi M, Lima EM, Appel EA, Dormont F, et al. Mechanistic understanding of in vivo protein corona formation on polymeric nanoparticles and impact on pharmacokinetics. Nat Commun (2017) 8(1):777. doi: 10.1038/s41467-017-00600-w
128. Kamaly N, Yameen B, Wu J, Farokhzad OC. Degradable controlled-release polymers and polymeric nanoparticles: Mechanisms of controlling drug release. Chem Rev (2016) 116(4):2602–63. doi: 10.1021/acs.chemrev.5b00346
129. Ulbrich K, Hola K, Subr V, Bakandritsos A, Tucek J, Zboril R. Targeted drug delivery with polymers and magnetic nanoparticles: Covalent and noncovalent approaches, release control, and clinical studies. Chem Rev (2016) 116(9):5338–431. doi: 10.1021/acs.chemrev.5b00589
130. Ekladious I, Colson YL, Grinstaff MW. Polymer-drug conjugate therapeutics: advances, insights and prospects. Nat Rev Drug Discovery (2019) 18(4):273–94. doi: 10.1038/s41573-018-0005-0
131. Haitao Y, Yifan C, Mingchao S, Shuaijuan H. A novel polymeric nanohybrid antimicrobial engineered by antimicrobial peptide MccJ25 and chitosan nanoparticles exerts strong antibacterial and anti-inflammatory activities. Front Immunol (2021) 12:811381. doi: 10.3389/fimmu.2021.811381
132. Boomer JS, To K, Chang KC, Takasu O, Osborne DF, Walton AH, et al. Immunosuppression in patients who die of sepsis and multiple organ failure. JAMA (2011) 306(23):2594–605. doi: 10.1001/jama.2011.1829
133. Ivashkiv LB. Epigenetic regulation of macrophage polarization and function. Trends Immunol (2013) 34(5):216–23. doi: 10.1016/j.it.2012.11.001
134. Jiang L, Wang Y, Wei X, Yang L, Liu S, Wang Y, et al. Improvement in phenotype homeostasis of macrophages by chitosan nanoparticles and subsequent impacts on liver injury and tumor treatment. Carbohydr Polym (2022) 277:118891. doi: 10.1016/j.carbpol.2021.118891
135. Sborgi L, Ruhl S, Mulvihill E, Pipercevic J, Heilig R, Stahlberg H, et al. GSDMD membrane pore formation constitutes the mechanism of pyroptotic cell death. EMBO J (2016) 35(16):1766–78. doi: 10.15252/embj.201694696
136. Zhou JE, Sun L, Liu L, Jia Y, Han Y, Shao J, et al. Hepatic macrophage targeted siRNA lipid nanoparticles treat non-alcoholic steatohepatitis. J Control Release (2022) 343:175–86. doi: 10.1016/j.jconrel.2022.01.038
137. Luo G, Yang Q, Yao B, Tian Y, Hou R, Shao A, et al. Slp-coated liposomes for drug delivery and biomedical applications: potential and challenges. Int J Nanomed (2019) 14:1359–83. doi: 10.2147/IJN.S189935
138. Di J, Xie F, Xu Y. When liposomes met antibodies: Drug delivery and beyond. Adv Drug Delivery Rev (2020) 154-155:151–62. doi: 10.1016/j.addr.2020.09.003
139. Al Saqr A, Aldawsari MF, Alrbyawi H, Poudel I, Annaji M, Mulabagal V, et al. Co-Delivery of hispolon and doxorubicin liposomes improves efficacy against melanoma cells. AAPS PharmSciTech (2020) 21(8):304. doi: 10.1208/s12249-020-01846-2
140. Shah S, Dhawan V, Holm R, Nagarsenker MS, Perrie Y. Liposomes: Advancements and innovation in the manufacturing process. Adv Drug Delivery Rev (2020) 154-155:102–22. doi: 10.1016/j.addr.2020.07.002
141. Meng Y, Hou X, Lei J, Chen M, Cong S, Zhang Y, et al. Multi-functional liposomes enhancing target and antibacterial immunity for antimicrobial and anti-biofilm against methicillin-resistant staphylococcus aureus. Pharm Res (2016) 33(3):763–75. doi: 10.1007/s11095-015-1825-9
142. Chen Z, Wang Z, Gu Z. Bioinspired and biomimetic nanomedicines. Acc Chem Res (2019) 52(5):1255–64. doi: 10.1021/acs.accounts.9b00079
143. Khatoon N, Zhang Z, Zhou C, Chu M. Macrophage membrane coated nanoparticles: a biomimetic approach for enhanced and targeted delivery. Biomater Sci (2022) 10(5):1193–208. doi: 10.1039/d1bm01664d
144. Li Y, Che J, Chang L, Guo M, Bao X, Mu D, et al. CD47- and integrin alpha4/beta1-Comodified-Macrophage-Membrane-Coated nanoparticles enable delivery of colchicine to atherosclerotic plaque. Adv Healthc Mater (2022) 11(4):e2101788. doi: 10.1002/adhm.202101788
145. Brown EJ, Frazier WA. Integrin-associated protein (CD47) and its ligands. Trends Cell Biol (2001) 11(3):130–5. doi: 10.1016/s0962-8924(00)01906-1
146. Gresham HD, Dale BM, Potter JW, Chang PW, Vines CM, Lowell CA, et al. Negative regulation of phagocytosis in murine macrophages by the src kinase family member, fgr. J Exp Med (2000) 191(3):515–28. doi: 10.1084/jem.191.3.515
147. Thamphiwatana S, Angsantikul P, Escajadillo T, Zhang Q, Olson J, Luk BT, et al. Macrophage-like nanoparticles concurrently absorbing endotoxins and proinflammatory cytokines for sepsis management. Proc Natl Acad Sci U S A (2017) 114(43):11488–93. doi: 10.1073/pnas.1714267114
148. Shen S, Han F, Yuan A, Wu L, Cao J, Qian J, et al. Engineered nanoparticles disguised as macrophages for trapping lipopolysaccharide and preventing endotoxemia. Biomaterials (2019) 189:60–8. doi: 10.1016/j.biomaterials.2018.10.029
149. Yusuf-Makagiansar H, Anderson ME, Yakovleva TV, Murray JS, Siahaan TJ. Inhibition of LFA-1/ICAM-1 and VLA-4/VCAM-1 as a therapeutic approach to inflammation and autoimmune diseases. Med Res Rev (2002) 22(2):146–67. doi: 10.1002/med.10001
150. Li H, Feng Y, Zheng X, Jia M, Mei Z, Wang Y, et al. M2-type exosomes nanoparticles for rheumatoid arthritis therapy via macrophage re-polarization. J Control Release (2022) 341:16–30. doi: 10.1016/j.jconrel.2021.11.019
151. Panwar N, Soehartono AM, Chan KK, Zeng S, Xu G, Qu J, et al. Nanocarbons for biology and medicine: Sensing, imaging, and drug delivery. Chem Rev (2019) 119(16):9559–656. doi: 10.1021/acs.chemrev.9b00099
152. Schaak RE, Williams ME. Full disclosure: the practical side of nanoscale total synthesis. ACS Nano (2012) 6(10):8492–7. doi: 10.1021/nn304375v
153. Lee HP, Gaharwar AK. Light-responsive inorganic biomaterials for biomedical applications. Adv Sci (Weinh) (2020) 7(17):2000863. doi: 10.1002/advs.202000863
154. Steckiewicz KP, Cieciorski P, Barcinska E, Jaskiewicz M, Narajczyk M, Bauer M, et al. Silver nanoparticles as chlorhexidine and metronidazole drug delivery platforms: Their potential use in treating periodontitis. Int J Nanomed (2022) 17:495–517. doi: 10.2147/IJN.S339046
155. Casals E, Zeng M, Parra-Robert M, Fernandez-Varo G, Morales-Ruiz M, Jimenez W, et al. Cerium oxide nanoparticles: Advances in biodistribution, toxicity, and preclinical exploration. Small (2020) 16(20):e1907322. doi: 10.1002/smll.201907322
156. Casey LM, Kakade S, Decker JT, Rose JA, Deans K, Shea LD, et al. Cargo-less nanoparticles program innate immune cell responses to toll-like receptor activation. Biomaterials (2019) 218:119333. doi: 10.1016/j.biomaterials.2019.119333
157. Ying H, Ruan Y, Zeng Z, Bai Y, Xu J, Chen S. Iron oxide nanoparticles size-dependently activate mouse primary macrophages via oxidative stress and endoplasmic reticulum stress. Int Immunopharmacol (2022) 105:108533. doi: 10.1016/j.intimp.2022.108533
158. Zhao X, Guo K, Zhang K, Duan S, Chen M, Zhao N, et al. Orchestrated yolk-shell nanohybrids regulate macrophage polarization and dendritic cell maturation for oncotherapy with augmented antitumor immunity. Adv Mater (2022) 34(9):e2108263. doi: 10.1002/adma.202108263
159. Sarkar J, Kumar A. Recent advances in biomaterial-based high-throughput platforms. Biotechnol J (2021) 16(2):e2000288. doi: 10.1002/biot.202000288
160. Park K, Lee Y, Seo J. Recent advances in high-throughput platforms with engineered biomaterial microarrays for screening of cell and tissue behavior. Curr Pharm Des (2018) 24(45):5458–70. doi: 10.2174/1381612825666190207093438
161. Patel S, Kim J, Herrera M, Mukherjee A, Kabanov AV, Sahay G. Brief update on endocytosis of nanomedicines. Adv Drug Delivery Rev (2019) 144:90–111. doi: 10.1016/j.addr.2019.08.004
162. Kang SH, Shin YS, Lee DH, Park IS, Kim SK, Ryu D, et al. Interactions of nanoparticles with macrophages and feasibility of drug delivery for asthma. Int J Mol Sci (2022) 23(3):1622. doi: 10.3390/ijms23031622
163. Yu Y, Duan J, Yu Y, Li Y, Liu X, Zhou X, et al. Silica nanoparticles induce autophagy and autophagic cell death in HepG2 cells triggered by reactive oxygen species. J Hazard Mater (2014) 270:176–86. doi: 10.1016/j.jhazmat.2014.01.028
164. Novakovic B, Habibi E, Wang SY, Arts RJW, Davar R, Megchelenbrink W, et al. Beta-glucan reverses the epigenetic state of LPS-induced immunological tolerance. Cell (2016) 167(5):1354–68 e14. doi: 10.1016/j.cell.2016.09.034
165. Saeed S, Quintin J, Kerstens HH, Rao NA, Aghajanirefah A, Matarese F, et al. Epigenetic programming of monocyte-to-macrophage differentiation and trained innate immunity. Science (2014) 345(6204):1251086. doi: 10.1126/science.1251086
166. Kumar S, Tripathy S, Jyoti A, Singh SG. Recent advances in biosensors for diagnosis and detection of sepsis: A comprehensive review. Biosens Bioelectron (2019) 124-125:205–15. doi: 10.1016/j.bios.2018.10.034
167. Claxton A, Papafilippou L, Hadjidemetriou M, Kostarelos K, Dark P. The challenge of recognising sepsis: Future nanotechnology solutions. J Intensive Care Soc (2020) 21(3):241–6. doi: 10.1177/1751143719896554
168. Srinivasan B, Li Y, Jing Y, Xu Y, Yao X, Xing C, et al. A detection system based on giant magnetoresistive sensors and high-moment magnetic nanoparticles demonstrates zeptomole sensitivity: potential for personalized medicine. Angew Chem Int Ed Engl (2009) 48(15):2764–7. doi: 10.1002/anie.200806266
169. Wang G, Huang H, Zhang G, Zhang X, Fang B, Wang L. Dual amplification strategy for the fabrication of highly sensitive interleukin-6 amperometric immunosensor based on poly-dopamine. Langmuir (2011) 27(3):1224–31. doi: 10.1021/la1033433
170. Wang G, He X, Chen L, Zhu Y, Zhang X. Ultrasensitive IL-6 electrochemical immunosensor based on au nanoparticles-graphene-silica biointerface. Colloids Surf B Biointerfaces (2014) 116:714–9. doi: 10.1016/j.colsurfb.2013.11.015
171. Khosravi F, Loeian SM, Panchapakesan B. Ultrasensitive label-free sensing of IL-6 based on PASE functionalized carbon nanotube micro-arrays with RNA-aptamers as molecular recognition elements. Biosensors (Basel) (2017) 7(2):17. doi: 10.3390/bios7020017
172. Smale ST, Tarakhovsky A, Natoli G. Chromatin contributions to the regulation of innate immunity. Annu Rev Immunol (2014) 32:489–511. doi: 10.1146/annurev-immunol-031210-101303
173. Ghisletti S, Barozzi I, Mietton F, Polletti S, De Santa F, Venturini E, et al. Identification and characterization of enhancers controlling the inflammatory gene expression program in macrophages. Immunity (2010) 32(3):317–28. doi: 10.1016/j.immuni.2010.02.008
174. Liu TF, Yoza BK, El Gazzar M, Vachharajani VT, McCall CE. NAD+-dependent SIRT1 deacetylase participates in epigenetic reprogramming during endotoxin tolerance. J Biol Chem (2011) 286(11):9856–64. doi: 10.1074/jbc.M110.196790
175. Schuijt TJ, Lankelma JM, Scicluna BP, de Sousa e Melo F, Roelofs JJ, de Boer JD, et al. The gut microbiota plays a protective role in the host defence against pneumococcal pneumonia. Gut (2016) 65(4):575–83. doi: 10.1136/gutjnl-2015-309728
176. Doster RS, Rogers LM, Gaddy JA, Aronoff DM. Macrophage extracellular traps: A scoping review. J Innate Immun (2018) 10(1):3–13. doi: 10.1159/000480373
Keywords: sepsis, nanomaterials, macrophages, nanotargeted therapy, nanodiagnosis
Citation: Song C, Xu J, Gao C, Zhang W, Fang X and Shang Y (2022) Nanomaterials targeting macrophages in sepsis: A promising approach for sepsis management. Front. Immunol. 13:1026173. doi: 10.3389/fimmu.2022.1026173
Received: 23 August 2022; Accepted: 28 November 2022;
Published: 09 December 2022.
Edited by:
Christelle Faveeuw, INSERM U1019 Centre d’Infection et Immunité de Lille (CIIL), FranceReviewed by:
Yaqing Qie, Mayo Clinic Florida, United StatesPhilipp Starkl, Medical University of Vienna, Austria
Copyright © 2022 Song, Xu, Gao, Zhang, Fang and Shang. This is an open-access article distributed under the terms of the Creative Commons Attribution License (CC BY). The use, distribution or reproduction in other forums is permitted, provided the original author(s) and the copyright owner(s) are credited and that the original publication in this journal is cited, in accordance with accepted academic practice. No use, distribution or reproduction is permitted which does not comply with these terms.
*Correspondence: You Shang, eW91X3NoYW5naHVzdEAxNjMuY29t
†These authors have contributed equally to this work