- 1Lung Cancer Center, West China Hospital, Sichuan University, Chengdu, China
- 2State Key Laboratory of Biotherapy and Cancer Center, West China Hospital, Sichuan University, Chengdu, China
- 3Division of Medical Oncology, Cancer Center, West China Hospital, Sichuan University, Chengdu, China
Bispecific antibodies (bsAbs) are artificial antibodies with two distinct antigen-binding sites that can bind to different antigens or different epitopes on the same antigen. Based on a variety of technology platforms currently developed, bsAbs can exhibit different formats and mechanisms of action. The upgrading of antibody technology has promoted the development of bsAbs, which has been effectively used in the treatment of tumors. So far, 7 bsAbs have been approved for marketing in the world, and more than 200 bsAbs are in clinical and preclinical research stages. Here, we summarize the development process of bsAbs, application in tumor treatment and look forward to the challenges in future development.
1 Introduction
The development of bsAb began in 1961, Nisonoff and his colleagues linked the Fab fragments of two different rabbit antibodies by reoxidation, and proved that the product can recruit two different types of cells, and proposed the concept of bsAb (1). In 1975, Milstein and Kohler fused splenic B lymphocytes and myeloma cells from immunized mice to form hybridoma cells that could produce monoclonal antibodies (2). In 1983, Milstein et al. produced the first bsAb with an intact Immunoglobulin G (IgG) structure using two fused hybridoma cells. In 1985, Perez et al. demonstrated that bsAbs that can bind to T cell receptors and tumor-specific antigens can attract T cells to tumor sites and induce T cells to kill tumors (3, 4), enhancing antibody-dependent cell-mediated cytotoxicity (ADCC). With the continuous advancement of antibody technology, double-antibody drugs have gradually begun to appear in the market. In April 2009, the EMA approved the first therapeutic bsAb catumaxomab developed by Trion Pharma in Europe for the intraperitoneal treatment of patients with malignant ascites (5). Although catumaxomab was withdrawn from the market in 2017 due to poor sales and other reasons, research on it is still ongoing. In December 2014, the FDA approved blinatumomab developed by Amgen for the treatment of acute lymphoblastic leukemia (6), which is also the first approved drug targeting CD19. In November 2017, the FDA approved emicizumab developed by Roche for the treatment of hemophilia A (7). In May 2021, the FDA approved amivantamab, developed by Janssen, for the treatment of EGFR-mutated non-small cell lung cancer (8). In January 2022, the FDA approved faricimab, developed by Genentech, for the treatment of wet age-related macular degeneration (w-AMD) and diabetic macular edema (DME) (9). In June 2022, the FDA approved mosunetuzumab developed by Roche for the treatment of adult patients with relapsed/refractory follicular lymphoma (R/R FL) who have received at least two prior systemic therapies. China Medical Products Administration (NMPA) approved candonilimab developed by Akeso for the treatment of relapsed or metastatic cervical cancer (R/M CC) in June 2022. With the successful listing and effective clinical application of bsAbs, it has gradually become a hot spot in antibody drug research. Currently, there are more than 200 bsAbs in clinical and preclinical research stages. This article will summarize and discuss the structure type, mechanism of action, clinical application and future challenges of bispecific antibodies (Table 1).
2 Bispecific antibody
Bispecific antibodies (bsAbs) have two distinct antigen-binding sites and can recognize two distinct epitopes on the same or different antigens (10). According to the structure, bsAbs can be roughly divided into two categories: IgG-like subtypes containing an Fc region and non-IgG-like subtypes without an Fc region (11) (Figure 1).
2.1 Structures of bsAbs
2.1.1 IgG subtypes
This type of bsAb is based on the structure of full-length IgG. Because it has an Fc region, it has the advantages of good solubility and stability, long half-life (12), and enhanced tumor killing effect through ADCC and complement dependent cytotoxicity (CDC) effects. Among them, the symmetrical type has a structure and stability similar to that of natural IgG, with mature technology and high expression. However, its differences in structure and size from native antibodies can negatively affect related favorable properties such as stability and solubility, and thus may lead to impairments in physicochemical or pharmacokinetic properties (13). Due to the symmetry of the class, most formats in clinical development are characterized by the close proximity of antigen-binding sites, which may affect bispecific binding and even lead to reduced functional potency. The asymmetric type solves the technical bottleneck of Knobs-into-Holes in common light chain, realizes the bivalent binding of tumor antigens and the monovalent binding of CD3, and can reduce the toxicity of CD3 antibodies when binding tumor antigens. However, there are also problems such as long technical route and high difficulty in design process in its preparation process. There are many technical platforms for the preparation of IgG subtype bsAbs, among which the platforms for producing asymmetric bsAbs include Triomab quadroma, Knobs-into-Holes (KiH), CrossMab, DuoBody and so on. Platforms for producing symmetrical bsAbs include DVD-Ig, FynomAb, Two-in-one/DAF, etc. Only TrioMab and CrossMab are clinically validated. However, since catumaxomab has been withdrawn from the market, strictly speaking, only CrossMab has obtained clinical validation. Other platform products are in the early and mid-stage clinical trials.
2.1.1.1 Triomab quadroma
The technology platform, jointly developed by Fresenius and TriOn Pharma, is based on the somatic fusion of two different hybridoma cells to generate monoclonal antibodies with the desired specificity (14), which are structurally similar to conventional antibodies. Since quadroma produces antibodies by randomly assembling two different heavy chains and two different light chains, many different structures are produced, but only one of them is the desired functional bsAb. A chimeric quadroma technology was later developed by fusing murine and rat hybridoma cell lines (15), which enriches functional bsAbs and reduces mismatches. The bsAb catumaxomab targeting EpCAM and CD3 was developed based on this platform and consists of mouse IgG2a and rat IgG2b (5). The mechanism of Triomab quadroma is shown in Figure 2.
2.1.1.2 Knobs-into-Holes
KiH was developed by Genentech, which involves engineering the CH3 domain to create a “knob” or a “hole” on each heavy chain to promote Fc heterodimerization (16) and reduce mismatches. A small molecule amino acid in the CH3 domain is replaced with a large molecule amino acid, resulting in a knob, and a large molecule amino acid in another CH3 domain is replaced with a small molecule amino acid, resulting in a hole, and the knob binds to the hole to promote heterozygous dimerization (17). The heterodimer formation mediated by this technology can provide more than 90% of the desired product under co-expression conditions, resulting in large-scale production to meet clinical and market needs (18). While KiH technology solves the problem of heavy chain mismatches, the problem of light chain mismatches remains to be solved, and random light chain binding can cause unwanted antibodies to mix with desired antibodies. In response to this problem, researchers have proposed some methods: 1) Generate bsAbs with a common light chain (19). But this method is not applicable to all bsAbs, and specific binding may be limited; 2) Knob-containing and hole-containing half-molecules are expressed in different bacteria, respectively. This approach avoids mispairing of light chains, but expression in bacterial cells also results in the loss of key glycosylation modifications, which may affect antibody effector function (20); 3) binds CrossMab and knobs-into -holes. In CrossMab antibodies, the CH1 domain of the heavy chain was exchanged with the CL domain of the corresponding light chain to induce correct pairing of the light chain (21); 4) additional mutations were introduced in the VH-VL and CH1-CL interface. These mutations prompt the heavy chain to preferentially pair with the light chain (22), but the disadvantage is that it requires extensive mutation in conserved regions of the antibody.
2.1.1.3 CrossMab
CrossMabs were developed by Roche and are based on the intra-arm cross-exchange of the Fab domains of bispecific IgG antibodies, allowing for correct chain binding and correct heterodimerization of heavy chains via knob-into-hole or charge interaction to achieve (23–25). There are various ways of exchange, which can be Fab domains (CrossMabFab format), variable VH-VL domains in Fab fragments (CrossMabVH-VL format) and invariant CH1-CL domains (CrossMabCH1-CL format). The technology is proven and mature, becoming one of the most versatile antibody engineering techniques in industry and academia. To date, at least eight different bsAbs using CrossMab technology have entered clinical development (26), and many are in late preclinical development. The bsAb Vanucizumab developed by Roche targeting VEGF-A and angiopoietin 2 (Ang-2) is prepared by CrossMAb technology: CL and CH1 in the Fab domain of Ang-2 antibody are exchanged using CrossMAb technology, The Fab structure of VEGF antibody remains unchanged. The modified Ang-2 antibody light chain is not prone to mismatch with the heavy chain of VEGF antibody. At the same time, the “knob-into-hole” structure can promote the heterodimerization of the two heavy chains. change (27).
2.1.1.4 DuoBody
The technology platform was developed by Genmab. The DuoBody technology generates bsAbs through a controlled Fab-arm exchange redox reaction of two parental homodimeric antibodies (28). Parental mAbs are expressed and pooled separately prior to selective reduction of disulfide bonds in the hinge region. Chains are recombined to a bispecific molecule, which is facilitated by the amino acid substitutions in the CH3 domain to drive preferential chain association of a heterodimer. DuoBody preserves the structural integrity of the homodimeric mAb and preserves Fc function. This process can generate four types of bsAbs: two combinations of symmetrical unoxidized, symmetrically oxidized, and asymmetrically oxidized (29). The process of producing bsAbs using the DuoBody generally involves three basic steps: 1) separate production of monospecific antibodies with the corresponding mutations in mammalian cell culture, 2) purification according to standard procedures, 3) in specific experiments Fab-arm exchange was performed under chamber conditions, followed by another purification step. This typically yields bsAbs with heterodimer content greater than 95% (30). The bsAb Amivantamab targeting mesenchymal transition factor (MET) and epidermal growth factor receptor (EGFR) was developed using this platform for the treatment of advanced non-small cell lung cancer.
2.1.1.5 DVD-Ig
The technology platform was developed by Abbott, and its product is a bispecific tetravalent IgG-like molecule containing an Fc region and a constant region, and each arm of the molecule contains two variable domains (VDs): an external VD or VD1 composed of VH1 and VL1; and an internal VD or VD2 adjacent to CH1 and CL and composed of VH2 and VL2 (31). VD1 and VD2 are linked by flexible linkers with different binding specificities, and they can theoretically be synthesized by linking variable region sequences from any pair of mAbs (32). Linker is usually the sequence connecting VH-CH1 and VL-CL in IgG1 mAb, and it can also be the amino acid sequence or G4S sequence of IgG1 hinge region. This technique avoids mismatches between different light and heavy chains, but has the disadvantage that the affinity of the internal VD or VD2 may be reduced. The keys to the design of DVD-Ig molecules are (1): the selection of VD pairings from the parental mAb (2), the inner/outer orientation of the selected VDs, and (3) the amino acid sequence used to link the VDs (33). bsAb lutikizumab targeting IL-1 alpha and IL-1 beta was developed using the DVD-Ig technology platform for the treatment of synovitis in knee osteoarthritis and has been completed in OA (hand and knee OA) Two phase II trials were conducted.
2.1.2 Non-IgG subtypes
This type of bsAb has no Fc region and is based on the structure of a single-chain variable fragment (scFv), which has the advantages of weak immunogenicity, low dosage, small size, and strong tissue penetration (34). The disadvantages are short half-life, low expression, and unstable structure (35). scFv is composed of antibody VL and VH connected by a short peptide (linker) of 15-20 amino acids, without Fc fragment, and belongs to small molecule genetically engineered antibodies. Linker is usually 15-20 amino acids in length, usually composed of glycine (Gly) and serine (Ser), and has certain elasticity and protease resistance. Technologies for preparing bsAbs with this structure include BiTE, DART, bi-Nanobody, TandAbs, etc., and only BiTE has been clinically validated.
2.1.2.1 BiTE
BiTE is an antibody construct with 2 binding domains that recognize tumor-expressed antigens and CD3 of T cells, respectively, thereby linking endogenous T cells to tumor-expressed antigens, activating the cytotoxic potential of the patient’s own T cells to eliminate cancer without genetic modification or ex vivo expansion of T cells (36, 37). The binding domains are 2 scFv regions from a monoclonal antibody linked by a flexible peptide linker. The first scFv binding domain can be modified to target any surface antigen and thus can be targeted for different types of tumors. The second scFv binding domain was always specific for CD3 in the TCR. When BiTE molecules bind to both cytotoxic T cells and tumor cells, T cells begin to proliferate, thereby increasing the total number of effector cells and enhancing the effect of BiTE treatment (38), which then leads to tumor cell lysis. Because this does not require co-stimulation or the typical complex mechanisms of major histocompatibility, BiTE molecules can engage any T cell (39, 40). Blinatumomab is the first approved classical BiTE molecule, linking the variable regions of two monoclonal antibodies targeting CD19 on tumor cells and CD3 on T cells through a polypeptide chain, making blinatumomab largely Independent of genetic alterations or intracellular escape mechanisms (41). Because it is mainly composed of two single-chain antibodies, BiTE has a small molecular weight (55-60kDa), easily penetrates tumor tissue, and lacks the Fc segment, so its immunogenicity is low. Clinical trials have demonstrated that even at very low doses, blinatumomab can effectively recruit T cells and clear tumors, significantly improving median OS in patients with relapsed or refractory B-cell precursor acute lymphoblastic leukemia. The mechanism of BiTE is shown in Figure 3.
2.1.2.2 DART
DART is developed by MacroGenics. DART molecules are heterodimers formed by the combination of two polypeptide chains, with two unique antigen-binding sites. Its structure is to use a linker to connect the VH and VL sequences of an antibody variable region with the VL and VH sequences of another antibody variable region to form scFv, and then co-express two scFv fragments, using the antibody VH and VL domains. interact to form bispecific fragments. In addition, cysteine was introduced at the C-terminus of the two polypeptide chains, and the interchain disulfide bond was formed through the cysteine, which improved the stability of the antibody (42). Unlike BiTE, DART is designed to mimic the natural interactions within IgG molecules, maintains potency both in vitro and in vivo administration, and aggregates at a lower scale in production. DART has better structural and biological properties than BiTE, including better stability and optimal redirection of the cytotoxic effect of T cells against malignant cells. The bsAb flotetuzumab targeting CD3ϵ and CD123 was developed based on the DART platform as a rescue immunotherapy for patients with refractory acute myeloid leukemia and is currently available in Japan and in Europe through an expanded access program (43).
2.1.2.3 bi-Nanobody
The technology platform, developed by Ablynx (acquired by Sanofi in 2018), refers to the antibody structure of camelids (which only contains heavy chains and completely lacks light chains), and preserves the VH region through recombinant technology (44). Nanobody (Nanobody) is to connect the VH regions of two or more antibody molecules to achieve multi-specific binding. It has the advantages of small molecular weight, simple structure, strong tissue penetration, and can penetrate the blood-brain barrier. Sticking and clumping. But nanobodies also have the disadvantage of having a short half-life. In response to this problem, Ablynx uses half-life extension technology to combine nanobody with serum albumin, increase the molecular weight, and extend the half-life from several hours to more than 3 weeks with the help of the recycling effect of FcRn. Serum albumin can also play a role in transporting drugs to target sites. Ozoralizumab is a bi-Nanobody-based bsAb consisting of two anti-TNFα nanobodies and an anti-HSA nanobody. It is a good TNF inhibitor that inhibits arthritis progression (45) and is currently in phase II clinical studies for the treatment of rheumatoid arthritis.
2.1.2.4 TandAbs
This technology platform, developed by Affimed, produces tetravalent bsAbs containing two peptide chains that provide two binding sites for each antigen (46). The N-terminus to C-terminus of each peptide chain is arranged in the order of VL1-VH2-VL2-VH1, and the two peptide chains are paired in opposite directions to form a homodimeric molecule. Such antibodies can recruit NK cells or T cells to the surrounding tumor, thereby harnessing the innate or adaptive immune system to fight cancer. The relative molecular mass of TandAbs is about 110 kD, which is between the whole molecule antibody and BiTE (about 50 kD), and the half-life of the product can reach 23 h. The protein has no glycosylation modification, the product is more uniform, and the immunogenicity is low. The bsAb AFM13, which targets CD30 and CD16A on NK cells, was developed based on this platform. CD30 is highly expressed in Hodgkin lymphoma, and CD16A is an active receptor involved in tumor cell killing (47). A Phase I clinical trial of AFM13 for the treatment of relapsed/refractory CD30-positive Hodgkin lymphoma has been completed, and results were presented at the American Association for Cancer Research 2021 Annual Meeting, demonstrating that AFM13 treats relapsed/refractory CD30-positive Hodgkin lymphoma The objective response rate of the tumor was 100%.
2.2 Mechanism of action
The advantage of bsAb is that it can bind two different epitopes at the same time, which provides a variety of different pathways for the realization of its function, so that it can treat diseases through different mechanisms (Figure 4).
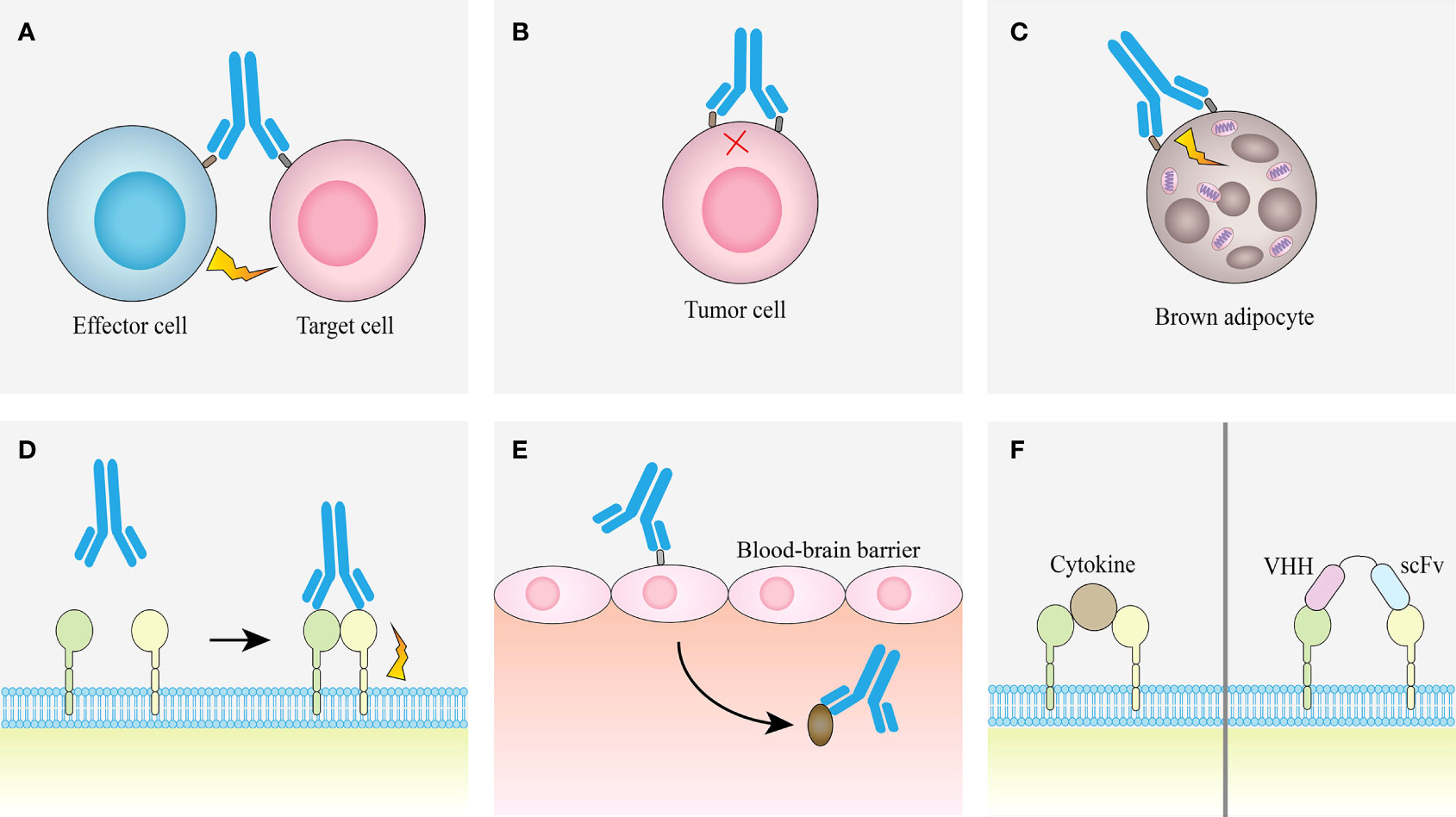
Figure 4 Examples of obligate mechanisms of action of bsAbs. (A) Linking T cells and tumor cells. (B) Receptor inhibition. (C) Receptor activation. (D) Analoging cofactor. (E) Using target to transport. (F) Surrogate cytokine agonists.
2.2.1 Linking T cells and tumor cells
This method mainly connects effector T cells and tumor cells through bsAb (48), also called T cell engaging bsAb (T-bsAb). The bsAb in this approach contains both a T-cell binding domain and a tumor-binding domain, which can bind to CD3ϵ in the CD3ϵ-TCR complex and activate T cells without antigen presentation (49) to kill tumor cells. However, only two T-bsAbs, catumaxomab and blinatumomab, are currently approved for clinical use, mainly due to difficulties in protein engineering during antibody preparation and the potential toxicity of the new constructs (50), as well as the clinically encountered association with cytokine release syndrome and neurotoxicity-related disorders (51, 52). However, studies have shown that T-bsAb has properties such as CD3 affinity screening and bystander effect, it can actively redirect internal T cells and promote their recruitment from the periphery to tumor tissues (53), and a variety of proteases accumulate in large quantities, which are beneficial for T cells and tumor cells. The formation of cytolytic synapses between target cells provides the necessary components (54). At the same time, T cells proliferate and release a variety of cytokines (55). Many mechanistic findings have been reported in preclinical studies, we are able to modulate the effects of T-bsAbs at the molecular or cellular level, and many clinical trials evaluating the efficacy and safety of T-bsAbs are ongoing. Therefore, T-bsAb is a promising emerging antibody therapy. The current state-of-the-art T-bsAb is a carcinoembryonic antigen T-cell bispecific antibody (CEA TCB) named cibisatamab (56).
2.2.1.1 Cibisatamab
Cibisatamab has a single binding site for the CD3ϵ chain on T cells and two CEA binding sites that modulate binding affinity to cancer cells with moderate to high cell surface expression of CEA (21). The introduction of a CH1-CL cross into the CD3-bound Fab formed the light chain of cibisatamab (18), while the correct association of the heavy chain was achieved by the knob-into-hole technique (23, 57). The CEA-binding agent (designated CH1A1A) used in the CEA TCB is derived from the PR1A3 antibody and possesses properties such as humanization, affinity maturation, and stabilization (58, 59). CEA, also known as carcinoembryonic antigen–related cell adhesion molecule 5 (CEACAM5) or CD66e, is a 180 to 200 kDa protein belonging to the CEACAM superfamily that is anchored to the cell surface via glycosylphosphatidylinositol (GPI), It is often highly expressed in various tumor entities (60). Preliminary results of cibisatamab in a phase Ia/Ib study in locally advanced and/or metastatic CEA-positive solid tumors reported that monotherapy produced significant antitumor activity and manageable adverse events (61). The data demonstrate that CEA TCB has favorable antitumor activity and the ability to alter the tumor microenvironment, is effective against non-inflammatory, poorly invasive tumors, and transforms non-inflammatory tumors into highly inflammatory tumors.
2.2.2 Receptor inhibition
Receptor tyrosine kinases (RTKs) are the largest class of enzyme-linked receptors, which are both growth factor receptors and enzymes that can catalyze the phosphorylation of downstream target proteins, including HER family, VEGFR family, etc. RTKs play an important regulatory role during cell proliferation, and precise regulation of the magnitude and duration of their signaling is critical for the execution of cellular programs and behaviors (62). Mutations in RTKs and their abnormal activation of intracellular signaling pathways may contribute to the development of cancer (63). Tumors can be treated by targeted inhibition of tumor receptor tyrosine kinases (RTKs), but other RTKs can escape from specific receptor inhibition and activate parallel signaling pathways, resulting in drug resistance. Therefore, bsAbs targeting multiple RTKs or their ligands can be developed to simultaneously block two or more signaling pathways to reduce tumor cell escape and overcome drug resistance. For example, zenocutuzumab is an ADCC-enhanced IgG antibody developed using Merus’ Biclonics technology and MeMo technology to target HER-2 and HER-3.
2.2.2.1 Zenocutuzumab
Zenocutuzumab is a HER2×HER3 bispecific antibody that is effective in treating tumors driven by NRG1 gene rearrangements. NRG1 rearrangements are recurrent oncogenic drivers in solid tumors. NRG1 binds to HER3, leading to heterodimerization with other HER/ERBB kinases, increasing downstream signaling and tumorigenesis, and NRG1 induces phosphorylation of HER2 and HER3 when only HER2 and HER3 are expressed (64). The HER2-HER3 dimer may be the most carcinogenic heterodimer in the ERBB family (65). Zenocutuzumab inhibits HER3 and AKT phosphorylation, induces the expression of apoptosis markers, and inhibits tumor cell growth. Zenocutuzumab binds to NRG1 or NRG1 fusion protein and prevents HER3 from interacting with NRG1 or NRG1 fusion protein. This inhibition produced robust efficacy in preclinical models and durable responses in patients with few treatment options. Zenocutuzumab is a promising treatment option in development for patients with NRG1 fusion-positive cancers. Based on this proof-of-concept, a global multicenter Phase 1/2 clinical trial in NRG1 fusion-positive cancers has been initiated (66). The FDA has granted Fast Track designation to zenocutuzumab for the treatment of patients with metastatic solid tumors harboring an NRG1 gene fusion who have progressed after receiving standard therapy.
2.2.3 Receptor activation
The treatment of some diseases requires activation of receptor signaling using agonistic antibodies, so bsAbs can also be used to activate multicomponent receptor complexes with receptors and coreceptors. For example, fibroblast growth factor 21 (FGF21) controls energy expenditure and nutrient metabolism by stimulating FGF receptor (FGFR) isoforms (1c, 2c, and 3c) that bind to the coreceptor Klothoβ (KLB) (67), the long-term use of recombinant FGF21 can improve the metabolic health of humans, but there is also a problem that the rapid molecular turnover limits the therapeutic effect. The bispecific anti-FGFR1/KLB agonist antibody BFKB8488A designed against it can treat obesity-related metabolic defects by specifically activating the FGFR1/KLB complex. Only when KLB is present on the cell surface, BFKB8488A enhances dimerization of the c-isoform of FGFR1 (FGFR1c) and stabilizes the interaction between FGFR1c and the extracellular domain of KLB protein. A clinical study in overweight human participants demonstrated that a single dose of BFKB8488A resulted in transient weight loss, sustained improvement in cardiometabolic parameters, and a trend toward reduced preference for sweetness and carbohydrate intake (68). A phase II clinical study of BFKB8488A in nonalcoholic steatohepatitis is ongoing.
2.2.4 Analoging cofactor
BsAbs can also mimic enzymes or cofactors in enzyme-substrate complexes. For example, hemophilia A is a bleeding disorder caused by a deficiency of factor VIII (FVIII), which normally acts as a cofactor for activating factor IX (FIXa) and promotes the activation of factor X (FX). The researchers designed bsAb(Mim8) as an activated FVIII mimetic. Mim8 is an anti-FIXa/anti-FX bispecific antibody whose activity is achieved in part through FIXa-stimulating activity present in the anti-FIXa arm. Preclinical experiments have shown that it has an effective hemostatic effect (69), and it has obtained an implied license for clinical trials in China. Intended to be developed for routine prophylaxis in children (<12 years of age) with hemophilia A (congenital factor VIII deficiency) with or without FVIII inhibitors to prevent or reduce the frequency of bleeding.
2.2.5 Using target to transport
A bsAb can also utilize the specificity of its first target to transport its second specific target. For example, the blood-brain barrier is a huge obstacle to developing treatments for neurological diseases. The application of bsAbs to the transferrin transport pathway can cross the blood-brain barrier and enter the immune-privileged brain regions to target pathogenic mediators that cause neurological diseases (70). By targeting the transferrin receptor (TfR), which is highly expressed on the surface of brain endothelial cells, with one of the binding arms of a bsAb, the bsAb binds to TfR and crosses the blood-brain barrier into the brain through receptor-mediated endocytosis. The second binding arm targets beta-secretase 1 (BACE1), blocking BACE1 activity in the brain. TfR×BACE1 bsAb reduces the levels of amyloid-β (Aβ) peptide, an enzymatic product of BACE1, in brain tissue and cerebrospinal fluid (71). The amyloid-β (Aβ) peptide is thought to be involved in the pathogenesis of Alzheimer’s disease, and its pathological overproduction leads to impaired memory, oxidative damage, blood-brain barrier damage, neurofibrillary tangles, and amyloid plaque formation (72).
2.2.6 Surrogate cytokine agonists
Cytokines can initiate signal transduction through receptor dimerization for immunomodulation, but their structure often influences their effects. BsAbs can dimerize cytokine receptors, thereby acting as a substitute for cytokines. Combining VHH and scFv can generate single-chain dual-specific ligands with multiple functional activities that force cytokine receptor heterodimerization and then initiate signaling. Type I IFNs have antiviral activity and can dimerize IFNAR1/IFNAR2 to activate certain signal transduction. Combining a single variable domain of a heavy chain (VHH) and a single-chain antibody fragment (scFv) in a type I IFN system to construct a surrogate agonist applied to human IFNAR1 and IFNAR2, which effectively inhibits viral replication, but does not have pro-inflammatory effects. This means that the strategy can obtain specific ligands corresponding to each receptor through screening, and it also means that the construction of dual-specific ligands is not limited to the induction of dimerization of natural receptors (73).
3 Application
The research and development of antibody drugs has always been a hot topic, among which bsAb is also a key target of corporate research and development. So far, a total of 7 bsAbs have been approved for marketing in the world, they are catumaxomab (which has been withdrawn from the market in 2017), blinatumomab, emicizumab, amivantamab, faricimab, candonilimab and mosunetuzumab (Table 2), and more than 200 bsAbs are in clinical and preclinical research stages (Tables 3, 4).
3.1 BsAbs that have been on the market
3.1.1 Catumaxomab
Catumaxomab is the world’s first commercialized monoclonal bispecific trifunctional antibody, developed by TrionPharma, produced by Triomab quadroma technology, and approved by EMA in April 2009 for intraperitoneal use in patients with malignant ascites caused by EpCAM-positive tumors treatment, but was withdrawn from the market in 2017 due to, among other reasons, poor sales. Catumaxomab consists of mouse IgG2 and rat IgG2b and targets CD3 on T cells and EpCAM on tumor cells (74). Epithelial cell adhesion molecule (EpCAM) is a 40KD type I transmembrane glycoprotein consisting of an extracellular domain (EpEX), a single transmembrane domain and an intracellular domain (EpICD) (75). Studies have shown that it plays an important role in intercellular adhesion, cell signaling, proliferation, differentiation, and the formation and maintenance of organ morphology (76). EpCAM is expressed on the entire cell surface (77) and is a very strongly and frequently expressed tumor-associated antigen (78), in ovarian, gastric, colon, pancreatic, prostate, lung and endometrial cancers. There are expressions (79). Although catumaxomab has been withdrawn from the market in 2017, its related research has not stopped. Recent studies have shown that two patients with EpCAM-positive recurrent non-muscle invasive bladder cancer were first treated with intravesical catumaxomab, which was well tolerated without any overt signs of toxicity and was Intravesical administration of catumaxomab is feasible, safe, and effective in muscle-invasive bladder cancer, thus supporting its further clinical development in this indication (80). Catumaxomab is currently undergoing clinical trials in breast, ovarian, peritoneal, non-small cell lung and gastrointestinal cancers (81).
3.1.2 Blinatumomab
Blinatumomab was developed by Amgen and was approved by the FDA in December 2014 for the treatment of Fisher chromosome-negative precursor B-cell acute lymphoblastic leukemia. Blinatumomab is a bispecific monoclonal antibody derived from recombinant mouse. It is a class of bispecific T cell engagers (BiTEs) that target the CD3 cell surface antigen on T lymphocytes and the CD19 locus on (malignant) B lymphocytes (54), enabling T cell trafficking to tumor cells And showed strong tumor killing ability. In Phase I/II clinical trials, blinatumomab was demonstrated in patients with relapsed and/or refractory (R/R) non-Hodgkin lymphoma and R/RB cell precursor acute lymphoblastic leukemia (B precursor ALL) Significant single drug activity. Cytokine release syndrome and neurologic side effects are both dose-limited, with manageable and transient adverse effects (82). However, blinatumomab also has the characteristics of possible drug resistance (83) and easy renal clearance due to its small size (84). Recent studies have shown that Among children with high-risk first-relapse B-cell acute lymphoblastic leukemia, treatment with 1 cycle of blinatumomab compared with standard intensive multidrug chemotherapy before allogeneic hematopoietic stem cell transplant resulted in an improved event-free survival at a median of 22.4 months of follow-up (85). The experimental results prove that blinatumomab has a good market prospect.
3.1.3 Emicizumab
Hemophilia A (HA) is a congenital X-linked bleeding disorder that results in complete or partial loss of activated factor VIII (FVIII) coagulation activity. Emicizumab is a recombinant humanized bispecific monoclonal antibody that restores the function of missing FVIII by bridging FIXa and FX to promote effective hemostasis in hemophilia A (HA) patients (86). Emicizumab mimics the cofactor activity of activated FVIII (FVIIIa), one arm of which binds to epidermal growth factor (EGF)-like domain 1 of FIX/FIXa, and the other arm recognizes EGF-like domain 2 of FX/FXa (87), thereby bridging FIXa and FX as a cofactor to form a sterically correct structure that facilitates the conversion of FX to FXa. Emicizumab is only functionally similar to FVIII but has a completely different molecular structure and does not bind to existing FVIII antibodies, so it is effective even in the presence of FVIII antibodies and does not induce the production of new FVIII antibodies (88). Emicizumab was developed by Roche and was approved by the FDA in November 2017 for the treatment of HA. Studies have shown that emicizumab prophylaxis is associated with a significantly lower rate of bleeding events compared with no prophylaxis in HA participants using inhibitors (89); in HA patients not using inhibitors, once a week or every 2 Once-weekly subcutaneous emicizumab prophylaxis was associated with significantly lower bleeding rates compared with no prophylaxis; more than half of the participants who received prophylaxis had no treatment-bleeding events (90). This shows that the therapeutic effect of emicizumab in HA is quite effective and has a good market prospect.
3.1.4 Amivantamab
Amivantamab, a bsAb targeting EGFR and MET (91), was developed by Janssen Biotechnology and approved by the FDA in May 2021 for patients with non-small cell lung cancer (NSCLC) whose tumors have EGFR exon 20 insertion mutations (92). Aberrant activation of EGFR and MET signaling pathways drives tumor cell growth and proliferation in lung cancer (93–96). EGFR mutations become constitutively activated signaling pathways that generate pro-survival and anti-apoptotic signals. Cancer cells with EGFR mutations become dependent on EGFR for survival, making EGFR an attractive target for anticancer therapy (97). c-MET amplification activates parallel oncogenic signaling pathways that bypass EGFR, resulting in resistance to EGFR-KI (98). Amivantamab binds to the extracellular domains of EGFR and cMet and blocks the binding of the ligand EGF to EGFR and the binding of the ligand HGF to its receptor cMet; it also induces degradation of both receptors in vivo, extending its effects to Ligand-independent receptor-driven diseases are included (99), and can bind to immune effector cells through ADCC to eliminate antigen-expressing tumor cells (100). The study showed that in patients with locally advanced or metastatic NSCLC with EGFR exon 20 insertion mutation after platinum-based chemotherapy, the overall response rate after using amivantamab was 40%, and the median duration of response was 11.1 months, indicating a good clinical effect (101).
3.1.5 Faricimab
Faricimab, which targets VEGF-A and Ang-2, was developed by Genentech and approved by the FDA in January 2022 for the treatment of wet age-related macular degeneration (wAMD) and diabetic macular edema (DME), the first approved bsAbs in this field. VEGF is an important cytokine in angiogenesis (102), and VEGF-A in particular is the target of current retinal diseases. It binds to the extracellular ligand-binding domains of VEGFR-1 and VEGFR-2, thereby activating internal signaling pathways that alter angiogenesis and vascular permeability genes (103). The Tie-2/Angiopoietin pathway plays an important role in vascular development and function (104). Activation of Tie-2 receptors by Ang-1 can maintain vascular stability to limit extravasation. Ang-2 replaces Ang-1 by binding to Tie-2 receptors and prevents its activation, thereby blocking the anti-inflammatory and anti-inflammatory effects of Ang-1. Apoptosis and tight binding support (105). Co-expression of Ang-2 and VEGF-A accelerated neovascularization in the developing retina and in ischemic retinal models. Studies have shown that Ang-2 and VEGF levels are elevated in vitreous samples from diabetic patients (106). Serum VEGF and Ang-2 levels were also higher in the proliferative diabetic retinopathy group than in the non-proliferative diabetic retinopathy group, suggesting that their levels may be associated with the progression of retinopathy (107). Preliminary 1-year results from Phase 3 trials of YOSEMITE and RHINE suggest that faricimab, which inhibits the dual action of Ang-2 and VEGF-A pathways, is designed for intraocular use to provide non-deteriorating visual gain and improved anatomical outcomes that can be achieved by This is achieved with dose adjustments up to every 16 weeks (108).
3.1.6 Mosunetuzumab
Mosunetuzumab was developed by Roche and approved by the FDA in June 2022. It is a CD20×CD3 T-cell engaging bsAb which can be used to treat adult patients with relapsed/refractory follicular lymphoma (R/R FL) who have received at least two prior systemic therapies (109). It is also the world’s first listed CD20×CD3 bsAb. CD3×CD20 is currently one of the most competitive bsAb target combinations. This type of bsAb can simultaneously bind to CD20 expressed on the surface of many B cell malignant tumors and CD3 on the surface of immune T cells, which can recruit T cells to surrounding tumor cells and activate T cells to kill tumor cells, providing tumor patients with an innovative treatment. In clinical trials, mosunetuzumab demonstrated high complete response rates, with most patients lasting at least 18 months of remission after complete responses, and was well tolerated in FL patients who had received extensive frontline therapy. A clinical development program for mosunetuzumab is underway to study the molecule as a monotherapy and in combination with other agents in patients with B-cell non-Hodgkin lymphoma, including follicular lymphoma, diffuse large B-cell lymphoma and other blood cancers.
3.1.7 Candonilimab
Candonilimab is a bsAb which targets PD-1 and CTLA-4, developed by Akeso and approved by NMPA in June 2022, for the treatment of R/M CC after failure of platinum-based chemotherapy. It is the first dual immune checkpoint inhibitor bsAb approved for marketing in the world. The median overall survival of patients with recurrent or metastatic cervical cancer treated with candonilimab was 17.51 months, and the overall survival of patients was prolonged by 8-13 months. At present, the phase III clinical study of candonilimab plus platinum-based chemotherapy with or without bevacizumab in the first-line treatment of persistent, recurrent or metastatic cervical cancer has been completed. Phase III pivotal registrational clinical study of primary adenocarcinoma, registrational/phase III clinical study of candonilimab combined with concurrent chemoradiotherapy for locally advanced cervical cancer, and the efficacy of candonilimab for adjuvant therapy after radical resection of hepatocellular carcinoma with high recurrence risk. Phase III clinical studies are in progress.
3.2 Application of bsAb in tumor immunotherapy
Over the past two decades, tumor immunotherapy has developed into the fourth pillar of cancer treatment in addition to surgery, radiotherapy and chemotherapy (110). One of the most successful immunotherapy modalities is antibody therapy (111). Among the 119 clinical and 176 preclinical bsAb projects, there are 99 clinical and 153 preclinical projects for tumor therapy. The roles of bsAb in tumor treatment are as follows.
3.2.1 Anti-angiogenesis
Angiogenesis, the formation of new blood vessels from pre-existing blood vessels, is one of the hallmarks of cancer (112). Tumor cells develop an angiogenic phenotype such that pro-angiogenic mechanisms overwhelm anti-angiogenic mechanisms. Endothelial cells grow rapidly, enabling oxygen and nutrients to reach the tumor microenvironment, supporting tumor growth and spread (113, 114). Identified pro-angiogenic molecules include VEGF, transforming growth factor-α and -β (TGF-α and -β), epidermal growth factor and so on (115). In addition, bsAb can block two or even multiple angiogenesis pathways at the same time, enhancing the anti-angiogenic effect. For example, bsAb ABL001 targeting vascular endothelial growth factor (VEGF) and Delta-like ligand4 (DLL4) competitively inhibits VEGF binding to VEGFR-2 and DLL4 to Notch1, blocking VEGF/VEGFR2 and DLL4/Notch1 in endothelial cells signaling pathway that inhibits angiogenesis. Compared with antibodies targeting VEGF alone and antibodies targeting DLL4 alone, ABL001 has shown higher potency anticancer effects in several human cancer xenograft models (116, 117), and is currently being evaluated in a phase 1 clinical study of heavy chemotherapy or targeted therapy pre-treated cancer patients (118).
3.2.2 Anti-tumorigenesis
There are high or abnormal expression of cytokine receptors in many tumors, resulting in an increase in the number of receptors or constant activation, which may lead to enhanced downstream signaling and uncontrollable cell proliferation, thereby causing cancer. Targeting oncogenic receptors is one of the widely used antitumor approaches. Most of the targeted sites of these bsAbs are HER2, HER3, EGFR, cMet, and LRP5/6. Zanidatamab is a bsAb developed by Zymeworks that can simultaneously bind two non-overlapping epitopes of HER2, ECD2 and ECD4 (119), called biparatopic binding. This unique design results in multiple mechanisms of action, including dual HER2 signaling blockade, increased cell surface HER2 protein binding and removal, and potent effector functions that lead to enhanced antitumor activity. Zymeworks is currently conducting multiple Phase 1, 2 and pivotal clinical trials worldwide to develop zanidatamab as a targeted therapy option for patients with HER2-expressing solid tumors. 1G5D2 is a bsAb targeting HER2 and HER3, and it can specifically bind to HER2 and HER3 expressed on tumor cells, thereby inhibiting AKT and ERK downstream signaling pathways and tumor cell proliferation in vitro (120), indicating that it may act as a HER2/HER3 transducer. A therapeutic candidate for expressing cancer is still in preclinical research.
3.2.3 Enhance T cell function
Tumor immunotherapy aims to improve the patient’s own anti-tumor immune response. It can enhance anti-tumor immunity by enhancing T cell helper activation signals. Common target sites include 4-1BB and OX40. Researchers developed a bsAb targeting B7-H3 and 4-1BB, which enhanced the proliferation and cytokine production of terminally differentiated CD8+ tumor-infiltrating lymphocytes, thereby enhancing antitumor immunity (121). B7-H3 (CD276) is a tumor antigen that is overexpressed in various human malignancies and tumor-associated vasculature, but not in healthy tissues. 4-1BB (CD137) is a costimulatory receptor that is widely expressed on immune cells and belongs to the tumor necrosis factor (TNF) receptor superfamily. It-mediated T cell co-stimulation can enhance T cell proliferation, cytotoxicity, and cytokine secretion, and protect T cells (122). Compared with the 4-1BB agonist mAb, the B7-H3 × 4-1BB bsAb showed more potent T-cell co-stimulatory activity and higher tumor localization in vitro. The bsAb is currently in the preclinical research stage.
3.2.4 Remove T cell inhibition
Boosting anti-tumor immunity can also be achieved by blocking T cell response inhibitory signals, commonly targeting sites including CTLA-4 and PD-1/PD-L1. We developed ATOR-1015, an IgG1 bsAb targeting OX40 and CTLA-4, that induces T cell activation and regulatory T cell (Treg) depletion in vitro. CTLA-4 is constitutively expressed on Tregs and is highly expressed in the tumor microenvironment, especially in Tregs (123). OX40 (CD134) is a co-activating member of the NGFR/TNFR superfamily expressed on activated CD4+ and CD8+ T cells, neutrophils and natural killer cells. OX40 has an important costimulatory function during T cell activation, mediating the survival and expansion of CD4+ and CD8+ T cells in a variety of animal models of autoimmunity, infectious diseases, and cancer (124). OX40 is also involved in the control of effector and memory T cell responses (125). ATOR-1015 reduces tumor growth and improves survival in several syngeneic tumor models, including bladder, colon, and pancreatic cancer models; also induces tumor-specific and long-term immune memory and enhances response to PD-1 inhibition. ATOR-1015 localizes to the tumor area, reduces the frequency of Tregs and increases the number and activation of CD8+ T cells (126). Currently, ATOR-1015 has completed the first-in-human Phase 1 clinical trial in patients with advanced and/or refractory solid malignancies.
3.2.5 Modulating tumor microenviroment
To escape immune system surveillance, tumors can suppress the function and proliferation of immune cells in the tumor microenvironment (TME) by expressing immunosuppressive molecules (eg, TGFβ, CD73) and recruiting immunosuppressive cells. Some bsAbs were developed to overcome immunosuppressive effects in the TME. For example, programmed cell death protein 1 (PD-1), an immune checkpoint expressed on T cells, inhibits T cell activity when it binds to the ligands PD-L1 or PD-L2. The PD-1-PD-L1 axis is not only an important feedback loop for immune homeostasis, but also involved in tumor immune escape (127). Transforming growth factor-β (TGF-β) is a tumor suppressor that inhibits cell proliferation and inflammation, and induces apoptosis. TGF-β is overexpressed in advanced tumors and is associated with poor prognosis (128). In TMEs with hyperactive TGF-β signaling, anti-PD-1/PD-L1 therapy has limited efficacy (129), necessitating simultaneous blockade of both PD-1/PD-L1 and TGF-β pathways. The researchers developed a bsAb YM101 targeting TGF-β and PD-L1, the world’s first anti-PD-L1 and TGF-β bispecific antibody. It can block both TGF-β and PD-L1 pathways simultaneously, and has a good antitumor effect (130), and in vivo experiments showed that the antitumor activity of YM101 was superior to that of anti-TGF-β and anti-PD-L1 monotherapy. YM101 promotes immune-inflammatory tumor formation, normalizes dysregulated antitumor immunity, and provides an immune-supportive TME. Y101D was granted an implied clinical license on May 17, 2021 for locally advanced or metastatic tumors.
3.2.6 Depletion of target cell
This type reflects the biological mechanism of most of the bsAbs currently under development, that is, by mediating effector cells or through Fc-mediated functions such as ADCC, antibody-dependent celluar phagocytosis (ADCP) and CDC, it directly targets tumor cells to achieve the purpose of clearance. Catumaxomab works through this mechanism. It uses the Triomab molecular pattern and relies on two antigen-binding arms to bind the CD3 site of cytotoxic T cells and the EpCAM site of tumor cells respectively, thereby guiding T cells to kill target cells (131–133). The bsAb AFM24 developed by Affimed targets EGFR and CD16A located on innate immune cells and can bind and redirect innate immune cells such as NK cells and macrophages to EGFR+ tumor cells (134), thereby directly killing tumor cells. AFM24 is effective against a variety of EGFR-expressing tumor cells regardless of EGFR expression level and KRAS/BRAF mutation status (135). AFM24 is currently in a Phase II clinical study for advanced solid tumors.
4 Challenges in the future
At this stage, the research and development of antibody drugs has a good momentum of development, and the research in the field of bsAb is also in full swing (Figure 5). However, there are also some risks in the clinical application of bsAbs. The higher affinity of bsAbs for CD3 may lead to activation of T cells by bsAbs before they bind to tumor cells, inducing a large release of cytokines and triggering a cytokine storm. In addition, the Fc fragment of bsAbs is immunogenic and can bind to Fc receptors on the surface of other effector cells, so full-length double antibodies are more likely to cause tumor-independent T cell activation, and in extreme cases, cytokine storms. Furthermore, the molecular structure of bsAbs does not exist in nature, which enhances the immunogenicity and elicits an immune response in the body. Therefore, the structure of bsAbs should be designed with a reasonable range of antibody affinity, which can inhibit Fc-mediated effector function as much as possible while having a more specific tumor target. Improving the humanized components of bsAbs and evaluating the immunogenicity in preclinical and clinical trials will effectively improve the safety and clinical efficacy of bsAbs.
5 Conclusions
The rapid development of antibody drugs has promoted the development of bsAbs. So far, more than 200 bsAbs are in clinical and preclinical research stages, and there are 7 bsAbs have been approved for marketing in the world. BsAbs have diverse structures, have different mechanisms of action, and are widely used in the treatment of tumors and other diseases. In the field of tumor therapy, bsAbs are widely used, and play a number of roles in anti-angiogenesis, regulation of signaling pathways, and regulation of tumor microenvironment. At present, most bsAbs are still in the clinical or preclinical research stage, and there is still a certain distance from the market. In the process of research and development, they still face many problems. With the advancement of science and technology, the research on bsAb will be further developed. It is believed that many problems faced in the research and development process will be gradually solved. We also expect that more and more bsAbs will be used in clinical treatment in the future to treat more patients with pain.
Author contributions
JK and TS: conceptualization, methodology, software, investigation, formal analysis, and writing - original draft; YZ: conceptualization, funding acquisition, resources, supervision, and writing - review and editing. All authors contributed to the article and approved the submitted version.
Funding
The study was funded by the 1·3·5 project for disciplines of excel-lence-Clinical Research Incubation Project, West China Hospital, Sichuan University (Grant No. 2019HXFH062) and from the Key R&D Projects of the Science and Technology Department of Sichuan Province (No. 2021YFS0237)
Conflict of interest
The authors declare that the research was conducted in the absence of any commercial or financial relationships that could be construed as a potential conflict of interest.
Publisher’s note
All claims expressed in this article are solely those of the authors and do not necessarily represent those of their affiliated organizations, or those of the publisher, the editors and the reviewers. Any product that may be evaluated in this article, or claim that may be made by its manufacturer, is not guaranteed or endorsed by the publisher.
References
1. Nisonoff A, Rivers MM. Recombination of a mixture of univalent antibody fragments of different specificity. Arch Biochem Biophys (1961) 93(2):460–2. doi: 10.1016/0003-9861(61)90296-X
2. Kohler G, Milstein C. Continuous cultures of fused cells secreting antibody of predefined specificity. 1975. Biotechnol (Reading Mass) (1992) 24:524–6. doi: 10.1038/256495a0
3. Perez P, Hoffman RW, Shaw S, Bluestone JA, Segal DM. Specific targeting of cytotoxic T cells by anti-T3 linked to anti-target cell antibody. Nature (1985) 316(6026):354–6. doi: 10.1038/316354a0
4. Staerz UD, Kanagawa O, Bevan MJ. Hybrid antibodies can target sites for attack by T cells. Nature (1985) 314(6012):628–31. doi: 10.1038/314628a0
5. Linke R, Klein A, Seimetz D. Catumaxomab: Clinical development and future directions. Mabs (2010) 2(2):129–36. doi: 10.4161/mabs.2.2.11221
6. Kantarjian H, Stein A, GöKbuget N, Fielding AK, Schuh AC, Ribera JM, et al. Blinatumomab versus chemotherapy for advanced acute lymphoblastic leukemia. N Engl J Med (2017) 376(9):836–47. doi: 10.1056/NEJMoa1609783
7. Oldenburg J, Mahlangu JN, Kim B, Schmitt C, Callaghan MU, Young G, et al. Emicizumab prophylaxis in hemophilia a with inhibitors. New Engl J Med (2017) 377(9):809–18. doi: 10.1056/NEJMoa1703068
8. Syed YY. Amivantamab: First approval. Drugs (2021) 81(11):1349–53. doi: 10.1007/s40265-021-01561-7
9. Nicolò M, Desideri LF, Vagge A, Traverso CE. Faricimab: An investigational agent targeting the tie-2/Angiopoietin pathway and vegf-a for the treatment of retinal diseases. Expert Opin Invest Drugs (2021) 30(3):193–200. doi: 10.1080/13543784.2021.1879791
10. Dhimolea E, Reichert JM. World bispecific antibody summit, September 27-28, 2011, Boston, ma. Mabs (2012) 4(1):4–13. doi: 10.4161/mabs.4.1.18821
11. Garber K. Bispecific antibodies rise again. Nat Rev Drug Discovery (2014) 13(11):799–801. doi: 10.1038/nrd4478
12. Kontermann RE. Strategies to extend plasma half-lives of recombinant antibodies. Biodrugs (2009) 23(2):93–109. doi: 10.2165/00063030-200923020-00003
13. Chames P, Baty D. Bispecific antibodies for cancer therapy. IDrugs (2009) 12(2):276–83. doi: 10.4161/mabs.1.6.10015
14. Zhang X, Yang Y, Fan D, Xiong D. The development of bispecific antibodies and their applications in tumor immune escape. Exp Hematol Oncol (2017) 6(1):12. doi: 10.1186/s40164-017-0072-7
15. Michael PF, Dan LS, Michael H, Ancilla WF, Parthiv JM. A systematic review of compliance with palivizumab administration for rsv immunoprophylaxis. Jmcp (2010) 16(1):46–58. doi: 10.18553/jmcp.2010.16.1.46
16. Xu Y, Lee J, Tran C, Heibeck TH, Wang WD, Yang J, et al. Production of bispecific antibodies in “Knobs-into-Holes” using a cell-free expression system. Mabs (2015) 7(1):231–42. doi: 10.4161/19420862.2015.989013
17. Ridgway JB, Presta LG, Paul C. ‘Knobs-into-Holes’ engineering of antibody Ch3 domains for heavy chain heterodimerization. Protein Eng (1996) 7):617. doi: 10.1093/protein/9.7.617
18. Atwell S, Ridgway J, Wells JA, Carter P, Atwell S, Ridgway JB, et al. Stable heterodimers from remodeling the domain interface of a homodimer using a phage display library. J Mol Biol (1997) 270(1):26–35. doi: 10.1006/jmbi.1997.1116
19. Merchant AM, Zhu Z, Yuan JQ, Goddard A, Adams CW, Presta LG, et al. An efficient route to human bispecific IgG. Nat Biotechnol (1998) 16(7):677–81. doi: 10.1038/nbt0798-677
20. Fan G, Wang Z, Hao M, Li J. Bispecific antibodies and their applications. J Hematol Oncol (2015) 8(1):130. doi: 10.1186/s13045-015-0227-0
21. Schaefer W, Regula JT, Bähner M, Schanzer J, Croasdale R, Dürr H, et al. Immunoglobulin domain crossover as a generic approach for the production of bispecific IgG antibodies. Proc Natl Acad Sci U States America (2011) 108(27):11187–92. doi: 10.1073/pnas.1019002108
22. Lewis SM, Wu X, Pustilnik A, Sereno A, Huang F, Rick HL, et al. Generation of bispecific IgG antibodies by structure-based design of an orthogonal fab interface. Nat Biotechnol (2014) 32(2):191–8. doi: 10.1038/nbt.2797
23. Carter P. Bispecific human IgG by design. J Immunol Methods (2001) 248(1-2):7–15. doi: 10.1016/s0022-1759(00)00339-2
24. Klein C, Sustmann C, Thomas M, Stubenrauch K, Croasdale R, Schanzer J, et al. Progress in overcoming the chain association issue in bispecific heterodimeric IgG antibodies. mAbs (2012) 4(6):653–63. doi: 10.4161/mabs.21379
25. Kuglstatter A, Stihle M, Neumann C, Müller C, Schaefer W, Klein C, et al. Structural differences between glycosylated, disulfide-linked heterodimeric knob-into-Hole fc fragment and its homodimeric knob-knob and hole-hole side products. Protein Engineering Design Selection (2017) 30(9):649–56. doi: 10.1093/protein/gzx041
26. Klein C, Schaefer W, Regula J, Dumontet C, Brinkmann U, Bacac M, et al. Engineering therapeutic bispecific antibodies using crossmab technology. Methods (2018) 154:21–31. doi: 10.1016/j.ymeth.2018.11.008
27. Kienast Y, Klein C, Scheuer W, Raemsch R, Lorenzon E. Ang-2-Vegf-a crossmab, a novel bispecific human IgG1 antibody blocking VEGF-a and ang-2 functions simultaneously, mediates potent anti-tumor, anti-angiogenic, and anti-metastatic efficacy. Clin Cancer Res (2013) 19(24):6730–40. doi: 10.1158/1078-0432.CCR-13-0081
28. Dennis RG, Steven JO, Adam Z, Theo R, Aran FL, Rob NJ, et al. Kinetic mechanism of controlled fab-arm exchange for the formation of bispecific immunoglobulin G1 antibodies. J Biol Chem (2018) 293(2):651–61. doi: 10.1074/jbc.RA117.000303
29. Evans AR, Capaldi MT, Goparaju G, Colter D, Shi FF, Aubert S, et al. Using bispecific antibodies in forced degradation studies to analyze the structure–function relationships of symmetrically and asymmetrically modified antibodies. mAbs (2019) 11(6):1–12. doi: 10.1080/19420862.2019.1618675
30. Hofmann T, Krah S, Sellmann C, Zielonka S, Doerner A. Greatest hits-innovative technologies for high throughput identification of bispecific antibodies. Int J Mol Sci (2020) 21(18):6551. doi: 10.3390/ijms21186551
31. Feng D, Ghayur T. Recent advancements in dvd-ig based therapeutic development. Drug Discovery Today Technol (2020) 34:1–7. doi: 10.1016/j.ddtec.2020.10.002
32. Clarissa GJ, Rohinton E, Russell AJ, Enrico D, Yingchun L, Jijie G, et al. Structure reveals function of the dual variable domain immunoglobulin (dvd-igTM) molecule. mAbs (2013) 5(3):358–63. doi: 10.4161/mabs.23977
33. Digiammarino E, Ghayur T, Liu J. Design and generation of dvd-ig molecules for dual-specific targeting. Methods Mol Biol (2012) 899:145–56. doi: 10.1007/978-1-61779-921-1_9
34. Ayyar BV, Arora S, O’Kennedy R. Coming-of-Age of antibodies in cancer therapeutics. Trends Pharmacol Sci (2016) 37(12):1009–28. doi: 10.1016/j.tips.2016.09.005
35. Demarest SJ, Glaser SM. Antibody therapeutics, antibody engineering, and the merits of protein stability. Curr Opin Drug Discovery Dev (2008) 11(5):675–87.
36. Baeuerle P, Nagorsen D. Immunomodulatory therapy of cancer with T cell-engaging bite antibody blinatumomab. Exp Cell Res (2011) 317(9):1255–60. doi: 10.1016/j.yexcr.2011.03.010
37. Baeuerle PA, Kufer P, Bargou R. Bite: Teaching antibodies to engage T-cells for cancer therapy. Curr Opin Mol Ther (2009) 11(1):22–30.
38. Klinger M, Benjamin J, Kischel R, Stienen S, Zugmaier G. Harnessing T cells to fight cancer with bite (R) antibody constructs - past developments and future directions. Immunol Rev (2016) 270(1):193–208. doi: 10.1111/imr.12393
39. Duell J, Lammers PE, Djuretic I, Chunyk AG, Alekar S, Jacobs I, et al. Bispecific antibodies in the treatment of hematologic malignancies. Clin Pharmacol Ther (2019) 106(4):781–91. doi: 10.1002/cpt.1396
40. Yuraszeck T, Kasichayanula S, Benjamin JE. Translation and clinical development of bispecific T-cell engaging antibodies for cancer treatment. Clin Pharmacol Ther (2017) 101(5):634–45. doi: 10.1002/cpt.651
41. Einsele H, Borghaei H, Orlowski RZ, Subklewe M, Roboz GJ, Zugmaier G, et al. The bite (Bispecific T-cell engager) platform: Development and future potential of a targeted immuno-oncology therapy across tumor types. Cancer (2020) 126(14):3192–201. doi: 10.1002/cncr.32909
42. Johnson S, Burke S, Ling H, Gorlatov S, Li H, Wang W, et al. Effector cell recruitment with novel fv-based dual-affinity re-targeting protein leads to potent tumor cytolysis and in vivo b-cell depletion. J Mol Biol (2010) 399(3):436–49. doi: 10.1016/j.jmb.2010.04.001
43. Maschmeyer G, Bullinger L, Garcia-Vidal C, Herbrecht R, Maertens J, Menna P, et al. Infectious complications of targeted drugs and biotherapies in acute leukemia. clinical practice guidelines by the European conference on infections in leukemia (Ecil), a joint venture of the European group for blood and marrow transplantation (Ebmt), the European organization for research and treatment of cancer (Eortc), the international immunocompromised host society (Ichs) and the European leukemia net (Eln). Leukemia (2022) 36(5):1215–26. doi: 10.1038/s41375-022-01556-7
44. Salvador JP, Vilaplana L, Marco MP. Nanobody: Outstanding features for diagnostic and therapeutic applications. Anal Bioanal Chem (2019) 411(9):1703–13. doi: 10.1007/s00216-019-01633-4
45. Ishiwatari-Ogata C, Kyuuma M, Ogata H, Yamakawa M, Iwata K, Ochi M, et al. Ozoralizumab, a humanized anti-tnf alpha Nanobody(R) compound, exhibits efficacy not only at the onset of arthritis in a human tnf transgenic mouse but also during secondary failure of administration of an anti-tnf alpha igg. Front Immunol (2022) 13:853008. doi: 10.3389/fimmu.2022.853008
46. Wang Q, Chen Y, Park J, Liu X, Betenbaugh MJ. Design and production of bispecific antibodies. Antibodies (2019) 8(3):43. doi: 10.3390/antib8030043
47. Wu J, Fu J, Zhang M, Liu D. AFM13: A first-in-Class tetravalent bispecific anti-Cd30/Cd16a antibody for nk cell-mediated immunotherapy. J Hematol Oncol (2015) 8(1):96. doi: 10.1186/s13045-015-0188-3
48. Labrijn AF, Janmaat ML, Reichert JM, Parren P. Bispecific antibodies: A mechanistic review of the pipeline. Nat Rev Drug Discovery (2019) 18(8):1–24. doi: 10.1038/s41573-019-0028-1
49. Offner S, Hofmeister R, Romaniuk A, Kufer P, Baeuerle PA. Induction of regular cytolytic T cell synapses by bispecific single-chain antibody constructs on mhc class I-negative tumor cells. Mol Immunol (2006) 43(6):763–71. doi: 10.1016/j.molimm.2005.03.007
50. Wu Z, Cheung NV. T Cell engaging bispecific antibody (T-bsab): From technology to therapeutics. Pharmacol Therapeutics: J Int Encyclopedia Pharmacol Ther (2018) 182:161–75. doi: 10.1016/j.pharmthera.2017.08.005
51. Eastwood D, Bird C, Dilger P, Hockley J, Findlay L, Poole S, et al. Severity of the Tgn1412 trial disaster cytokine storm correlated with il-2 release. Br J Clin Pharmacol (2013) 76(2):299–315. doi: 10.1111/bcp.12165
52. Teachey DT, Rheingold SR, Maude SL, Zugmaier G, Barrett DM, Seif AE, et al. Cytokine release syndrome after blinatumomab treatment related to abnormal macrophage activation and ameliorated with cytokine-directed therapy. Blood (2013) 121(26):5154–7. doi: 10.1182/blood-2013-02-485623
53. Velasquez MP, Bonifant CL, Gottschalk S. Redirecting T cells to hematological malignancies with bispecific antibodies - sciencedirect. Blood (2018) 131(1):30–8. doi: 10.1182/blood-2017-06-741058
54. Nagorsen D, Kufer P, Baeuerle PA, Bargou R. Blinatumomab: A historical perspective. Pharmacol Ther (2012) 136(3):334–42. doi: 10.1016/j.pharmthera.2012.07.013
55. Wu J, Fu J, Zhang M, Liu D. Blinatumomab: A bispecific T cell engager (Bite) antibody against Cd19/Cd3 for refractory acute lymphoid leukemia. J Hematol Oncol (2015) 8(1):104. doi: 10.1186/s13045-015-0195-4
56. Yasunaga M. T Cell bispecific antibodies: An antibody-based delivery system for inducing antitumor immunity. Pharmaceuticals (2021) 14(11):1172. doi: 10.3390/ph14111172
57. Conaghan PJ, Ashraf SQ, Tytherleigh MG, Wilding JL, Tchilian E, Bicknell D, et al. Targeted killing of colorectal cancer cell lines by a humanised IgG1 monoclonal antibody that binds to membrane-bound carcinoembryonic antigen. Br J Cancer (2008) 98(7):1217–25. doi: 10.1038/sj.bjc.6604289
58. Bacac M, Fauti T, Sam J, Colombetti S, Weinzierl T, Ouaret D, et al. A novel carcinoembryonic antigen T-cell bispecific antibody (Cea tcb) for the treatment of solid tumors. Clin Cancer Res (2016) 22(13):3286–97. doi: 10.1158/1078-0432.CCR-15-1696
59. Durbin H, Young S, Stewart LM, Wrba F, Rowan AJ, Bodmer S. An epitope on carcinoembryonic antigen defined by the clinically relevant antibody PR1A3. Proc Natl Acad Sci U States America (1994) 91(10):4313–7. doi: 10.1073/pnas.91.10.4313
60. Hammarström S. The carcinoembryonic antigen (CEA) family: Structures, suggested functions and expression in normal and malignant tissues. Semin Cancer Biol (1999) 9(2):67–81. doi: 10.1006/scbi.1998.0119
61. Segal NH, Saro J, Melero I, Ros W, Argiles G, Marabelle A, et al. Phase I studies of the novel carcinoembryonic antigen T-cell bispecific (Cea-Cd3 tcb) antibody as a single agent and in combination with atezolizumab: Preliminary efficacy and safety in patients (Pts) with metastatic colorectal cancer (Mcrc). Ann Oncol (2017) 28:1. doi: 10.1093/annonc/mdx367.036
62. Neben CL, Lo M, Jura N, Klein OD. Feedback regulation of RTK signaling in development. Dev Biol (2017) 447(1):71–89. doi: 10.1016/j.ydbio.2017.10.017
63. Lemmon MA, Schlessinger J. Cell signaling by receptor tyrosine kinases. Cell (2010) 141(7):1117–34. doi: 10.1016/j.cell.2010.06.011
64. Zhang X, Gureasko J, Shen K, Cole PA, Kuriyan J. An allosteric mechanism for activation of the kinase domain of epidermal growth factor receptor. Cell (2006) 125(6):1137–49. doi: 10.1016/j.cell.2006.05.013
65. Klapper NL, Glathe S, Vaisman N, Hynes NE, Andrews GC, Sela M, et al. The erbb-2/Her2 oncoprotein of human carcinomas may function solely as a shared coreceptor for multiple stroma-derived growth factors. Proc Natl Acad Sci U States America (1999) 96(9):4995–5000. doi: 10.1073/pnas.96.9.4995
66. Schram AM, Odintsov I, Espinosa-Cotton M, Khodos I, Sisso WJ, Mattar MS, et al. Zenocutuzumab, a Her2xher3 bispecific antibody, is effective therapy for tumors driven by Nrg1 gene rearrangements. Cancer Discovery (2022) 12(5):1233–47. doi: 10.1158/2159-8290.Cd-21-1119
67. Kliewer SA, Mangelsdorf DJ. A dozen years of discovery: Insights into the physiology and pharmacology of FGF21. Cell Metab (2019) 29(2):246–53. doi: 10.1016/j.cmet.2019.01.004
68. Baruch A, Wong C, Chinn LW, Vaze A, Sonoda J, Gelzleichter T, et al. Antibody-mediated activation of the Fgfr1/Klotho beta complex corrects metabolic dysfunction and alters food preference in obese humans. Proc Natl Acad Sci U States America (2020) 117(46):28992–9000. doi: 10.1073/pnas.2012073117
69. Ostergaard H, Lund J, Greisen PJ, Kjellev S, Henriksen A, Lorenzen N, et al. A factor viiia-mimetic bispecific antibody, Mim8, ameliorates bleeding upon severe vascular challenge in hemophilia a mice. Blood (2021) 138(14):1258–68. doi: 10.1182/blood.2020010331
70. Stanimirovic D, Kemmerich K, Haqqani AS, Farrington GK. Engineering and pharmacology of blood-brain barrier-permeable bispecific antibodies. Adv Pharmacol (San Diego Calif) (2014) 71:301–35. doi: 10.1016/bs.apha.2014.06.005
71. Yu YJ, Zhang Y, Kenrick M, Hoyte K, Luk W, Lu Y, et al. Boosting brain uptake of a therapeutic antibody by reducing its affinity for a transcytosis target. Sci Trans Med (2011) 3(84):84ra44. doi: 10.1126/scitranslmed.3002230
72. Morley JE, Farr SA. The role of amyloid-beta in the regulation of memory. Biochem Pharmacol (2014) 88(4):479–85. doi: 10.1016/j.bcp.2013.12.018
73. Michelle Y, Junming R, Qingxiang L, Caleb RG, Timothy PS, Lora KP, et al. Facile discovery of surrogate cytokine agonists. Cell (2022) 185(8):1414–30. doi: 10.1016/j.cell.2022.02.025
74. Lindhofer H, Mocikat R, Steipe B, Thierfelder S. Preferential species-restricted Heavy/Light chain pairing in Rat/Mouse quadromas. implications for a single-step purification of bispecific antibodies. J Immunol (1995) 155(1):219–25.
75. Herlyn M, Steplewski Z, Herlyn D, Koprowski H. Colorectal carcinoma-specific antigen: Detection by means of monoclonal antibodies. Proc Natl Acad Sci (1979) 76(3):1438–42. doi: 10.1073/pnas.76.3.1438
76. Schnell U, Cirulli V, Giepmans B. Epcam: Structure and function in health and disease. Bba Biomembr (2013) 1828(8):1989–2001. doi: 10.1016/j.bbamem
77. Litvinov SV, Bakker H, Gourevitch MM, Velders MP, Warnaar SO. Evidence for a role of the epithelial glycoprotein 40 (Ep-cam) in epithelial cell-cell adhesion. Cell Adhesion Communication (1994) 2(5):417. doi: 10.3109/15419069409004452
78. Went P, Lugli A, Meier S, Bundi M, Mirlacher M, Sauter G, et al. Frequent epcam protein expression in human carcinomas. Hum Pathol (2004) 35(1):122–8. doi: 10.1016/j.humpath.2003.08.026
79. Went P, Vasei M, Bubendorf L, Terracciano L, Tornillo L, Riede U, et al. Frequent high-level expression of the immunotherapeutic target ep-cam in colon, stomach, prostate and lung cancers. Br J Cancer (2006) 94(1):128. doi: 10.1038/sj.bjc.6602924
80. Ruf P, Bauer HW, Schoberth A, Kellermann C, Lindhofer H. First time intravesically administered trifunctional antibody catumaxomab in patients with recurrent non-muscle invasive bladder cancer indicates high tolerability and local immunological activity. Cancer Immunol Immunother (2021), 70(9):2727–35. doi: 10.1007/s00262-021-02930-7
81. Weidle UH, Kontermann RE, Brinkmann U. Tumor-Antigen–binding bispecific antibodies for cancer treatment. Semin Oncol (2014) 41(5):653–60. doi: 10.1053/j.seminoncol.2014.08.004
82. Goebeler ME, Bargou R. Blinatumomab: A CD19/CD3 bispecific T cell engager (Bite) with unique anti-tumor efficacy. Leuk Lymphoma (2016) 57(5):1021–32. doi: 10.3109/10428194.2016.1161185
83. Kohnke T, Krupka C, Tischer J, Knosel T, Subklewe M. Increase of PD-L1 expressing b-precursor all cells in a patient resistant to the CD19/CD3-bispecific T cell engager antibody blinatumomab. J Hematol Oncol (2015) 8:5. doi: 10.1186/s13045-015-0213-6
84. Zugmaier G, Klinger M, Schmidt M, Subklewe M. Clinical overview of anti-CD19 bite (R) and ex vivo data from anti-Cd33 BiTE (R) as examples for retargeting T cells in hematologic malignancies. Mol Immunol (2015) 67(2):58–66. doi: 10.1016/j.molimm.2015.02.033
85. Locatelli F, Zugmaier G, Rizzari C, Morris JD, Gruhn B, Klingebiel T, et al. Effect of blinatumomab vs chemotherapy on event-free survival among children with high-risk first-relapse b-cell acute lymphoblastic leukemia a randomized clinical trial. J Am Med Assoc (2021) 325(9):843–54. doi: 10.1001/jama.2021.0987
86. Blair HA. Emicizumab: A review in haemophilia a. Drugs (2019) 79(15):1697–707. doi: 10.1007/s40265-019-01200-2
87. Takehisa K, Keiko E, Tatsuhiko T, Shinya I, Tetsuhiro S, Atsushi M, et al. Factor viiia-mimetic cofactor activity of a bispecific antibody to factors IX/IXa and X/Xa, emicizumab, depends on its ability to bridge the antigens. Thromb Haemostasis (2017) 117(07):1348–57. doi: 10.1160/TH17-01-0030
88. Kitazawa T, Igawa T, Sampei Z, Muto A, Kojima T, Soeda T, et al. A bispecific antibody to factors ixa and X restores factor viii hemostatic activity in a hemophilia a model. Nat Med (2012) 18(10):1570–4. doi: 10.1038/nm.2942
89. Oldenburg J, Levy GG. Emicizumab prophylaxis in hemophilia a with inhibitors. New Engl J Med (2017) 377(22):2194–5. doi: 10.1056/NEJMc1712683
90. Mahlangu J, Oldenburg J, Paz-Priel I, Negrier C, Niggli M, Mancuso ME, et al. Emicizumab prophylaxis in patients who have hemophilia a without inhibitors. New Engl J Med (2018) 379(9):811–22. doi: 10.1056/NEJMoa1803550
91. Vijayaraghavan S, Lipfert L, Chevalier K, Bushey BS, Moores SL. Amivantamab (Jnj-61186372), an fc enhanced Egfr/Cmet bispecific antibody induces receptor downmodulation and anti-tumor activity by Monocyte/Macrophage trogocytosis. Mol Cancer Ther (2020) 19(10):2044–56. doi: 10.1158/1535-7163.MCT-20-0071
92. Dolgin E. Amivantamab ok’d for egfr-mutant nsclc. Cancer Discovery (2021) 11(7):1604. doi: 10.1158/2159-8290.Cd-nb2021-0351
93. Bean J, Brennan C, Shih JY, Riely G, Viale A, Wang L, et al. Met amplification occurs with or without T790m mutations in egfr mutant lung tumors with acquired resistance to gefitinib or erlotinib. Proc Natl Acad Sci U States America (2007) 104(52):20932–7. doi: 10.1073/pnas.0710370104
94. Birchmeier C, Birchmeier W, Gherardi E, Woude G. Met, metastasis, motility and more. Nat Rev Mol Cell Biol (2003) 4(12):915–25. doi: 10.1038/nrm1261
95. Dulak AM, Gubish CT, Stabile LP, Henry C, Siegfried JM. HGF-independent potentiation of EGFR action by c-met. Oncogene (2011) 30(33):3625–35. doi: 10.1038/onc.2011.84
96. Liu X, Yao W, Newton RC, Scherle PA. Targeting the c-met signaling pathway for cancer therapy. Expert Opin Investig Drugs (2008) 17(7):997–1011. doi: 10.1517/13543784.17.7.997
97. Hisayuki S, Li L, Takao T, Masaharu N, Makoto S, Wistuba II, et al. Clinical and biological features associated with epidermal growth factor receptor gene mutations in lung cancers. J Natl Cancer Institute (2005) 97(5):339–46. doi: 10.1093/jnci/dji055
98. Engelman JA, Zejnullahu K, Mitsudomi T, Song YC, Hyland C, Park JO, et al. Met amplification leads to gefitinib resistance in lung cancer by activating ERBB3 signaling. Science (2007) 316(5827):1039–43. doi: 10.1126/science.1141478
99. Yun J, Lee SH, Kim SY, Jeong SY, Cho BC. Antitumor activity of amivantamab (Jnj-61186372), an egfr-cmet bispecific antibody, in diverse models of egfr exon 20 insertion-driven nsclc. Cancer Discovery (2020) 10(8):1194–209. doi: 10.1158/2159-8290
100. Brazel D, Nagasaka M. Spotlight on amivantamab (Jnj-61186372) for EGFR exon 20 insertions positive non-small cell lung cancer. Lung Cancer Targets Ther (2021) 12:133–8. doi: 10.2147/lctt.S337861
101. Park K, Haura EB, Leighl NB, Mitchell P, Shu CA, Girard N, et al. Amivantamab in egfr exon 20 insertion-mutated non-Small-Cell lung cancer progressing on platinum chemotherapy: Initial results from the chrysalis phase I study. J Clin Oncol (2021) 39(30):3391–402. doi: 10.1200/jco.21.00662
102. Lien S, Lowman HB. Therapeutic anti-VEGF antibodies. Handb Exp Pharmacol (2008) 181):131–50. doi: 10.1007/978-3-540-73259-4_6
103. Samanta A, Aziz AA, Jhingan M, Singh SR, Chhablani J. Emerging therapies in neovascular age-related macular degeneration in 2020. Asia Pacific J Ophthalmol (2020) 9(3):250–9. doi: 10.1097/APO.0000000000000291
104. Gu J, Zhang Y, Han Z, Gao L, Cui J, Sun Y, et al. Targeting the ERβ/Angiopoietin-2/Tie-2 signaling-mediated angiogenesis with the FDA-approved anti-estrogen faslodex to increase the sunitinib sensitivity in RCC. Soc Sci Electronic Publishing (2020) 11(5):367. doi: 10.1038/s41419-020-2486-0
105. Jong GMD, Slager JJ, Verbon A. Systematic review of the role of angiopoietin-1 and angiopoietin-2 in plasmodium species infections: Biomarkers or therapeutic targets? Malaria J (2016) 15(1):581. doi: 10.1186/s12936-016-1624-8
106. Hussain RM, Neiweem AE, Kansara V, Harris A, Ciulla TA. Tie-2/Angiopoietin pathway modulation as a therapeutic strategy for retinal disease. Expert Opin Invest Drugs (2019) 28(10):861–9. doi: 10.1080/13543784.2019.1667333
107. Khalaf N, Helmy H, Labib H, Fahmy I, Moemen L. Role of angiopoietins and tie-2 in diabetic retinopathy. Electronic Physician (2017) 9(8):5031–5. doi: 10.19082/5031
108. Wykoff CC, Abreu F, Adamis AP, Basu K, Eichenbaum DA, Haskova Z, et al. Efficacy, durability, and safety of intravitreal faricimab with extended dosing up to every 16 weeks in patients with diabetic macular oedema (Yosemite and rhine): Two randomised, double-masked, phase 3 trials. Lancet (2022) 399(10326):741–55. doi: 10.1016/s0140-6736(22)00018-6
109. Kang C. Mosunetuzumab: First approval. Drugs (2022) 82(11):1229–34. doi: 10.1007/s40265-022-01749-5
110. Sanmamed MF, Chen L. A paradigm shift in cancer immunotherapy: From enhancement to normalization. Cell (2018) 175(2):313–26. doi: 10.1016/j.cell.2018.09.035
111. Kamakura D, Asano R, Yasunaga M. T Cell bispecific antibodies: An antibody-based delivery system for inducing antitumor immunity. Pharmaceuticals (2021) 14(11):24. doi: 10.3390/ph14111172
112. Rakesh RR, Arjan WG, Dan GD. Anti-angiogenesis for cancer revisited: Is there a role for combinations with immunotherapy? Angiogenesis (2017) 20(2):185–204. doi: 10.1007/s10456-017-9552-y
113. Dalal B, Quinn TJ, Foster L, Lin M, Matthews M, Yuhan B. Ligand-directed tumor targeting with hybrid viral phage nanoparticles - sciencedirect. Drug Targeting Stimuli Sensitive Drug Delivery Syst (2018), 483–516. doi: 10.1016/B978-0-12-813689-8.00013-6
114. Miyaji S. Current trends in cancer therapy. [Kango gijutsu]: [Nursing technique] (1970) 9:91–7.
115. Tinglu L, Guangbo K, Tingyue W, He H. Tumor angiogenesis and antiangiogenic gene therapy for cancer (Review). Oncol Lett (2018) 16(1):687–702. doi: 10.3892/ol.2018.8733
116. Kim DH, Lee S, Kang HG, Park HW, Chun KH. Synergistic antitumor activity of a Dll4/Vegf bispecific therapeutic antibody in combination with irinotecan in gastric cancer. BMB Rep (2020) 53(10):533–8. doi: 10.5483/BMBRep.2020.53.10.103
117. Lee D, Kim D, Choi YB, Kang K, Sung ES, Ahn JH, et al. Simultaneous blockade of VEGF and DLL4 by HD105, a bispecific antibody, inhibits tumor progression and angiogenesis. Mabs (2016) 8(5):892–904. doi: 10.1080/19420862.2016.1171432
118. Yeom DH, Lee YS, Ryu I, Lee S, You WK. ABL001, a bispecific antibody targeting VEGF and DLL4, with chemotherapy, synergistically inhibits tumor progression in xenograft models. Int J Mol Sci (2020) 22(1):241. doi: 10.3390/ijms22010241
119. Pant S, Ducreux M, Harding JJ, Javle MM, Jia F. A phase IIb, open-label, single-arm study of zanidatamab (ZW25) monotherapy in subjects with advanced or metastatic Her2-amplified biliary tract cancers. J Clin Oncol (2021) 39(3_suppl):TPS352–TPS. doi: 10.1200/JCO.2021.39.3_suppl.TPS352
120. Hassani D, Amiri MM, Mohammadi M, Yousefi P, Judaki MA, Mobini M, et al. A novel tumor inhibitory hybridoma monoclonal antibody with dual specificity for HER3 and HER2. Curr Res Transl Med (2021) 69(2):9. doi: 10.1016/j.retram.2021.103277
121. You G, Lee Y, Kang YW, Han WP, Lee SW. B7-H3×4-1bb bispecific antibody augments antitumor immunity by enhancing terminally differentiated CD8+ tumor-infiltrating lymphocytes. Sci Adv (2021) 7(3):eaax3160. doi: 10.1126/sciadv.aax3160
122. Myers LM, Vella AT. Interfacing T-cell effector and regulatory function through CD137 (4-1BB) Co-stimulation. Trends Immunol (2005) 26(8):440–6. doi: 10.1016/j.it.2005.06.003
123. Vargas FA, Furness AJS, Litchfield K, Joshi K, Rosenthal R, Ghorani E, et al. Fc effector function contributes to the activity of human anti-Ctla-4 antibodies. Cancer Cell (2018) 33(4):649–663.e4. doi: 10.1016/j.ccell.2018.02.010
124. Willoughby J, Griffiths J, Tews I, Cragg MS. OX40: Structure and function-what questions remain? Mol Immunol (2017) 83:13–22. doi: 10.1016/j.molimm.2017.01.006
125. Ishii N, Takahashi T, Soroosh P, Sugamura K. OX40-OX40 ligand interaction in T-Cell-Mediated immunity and immunopathology. Adv Immunol (2010) 105:63–98. doi: 10.1016/S0065-2776(10)05003-0
126. Kvarnhammar AM, Veitonmäki N, Hägerbrand K, Dahlman A, Smith KE, Fritzell S, et al. The CTLA-4 X OX40 bispecific antibody ATOR-1015 induces anti-tumor effects through tumor-directed immune activation. J Immunother Cancer (2019) 7(1):103. doi: 10.1186/s40425-019-0570-8
127. Okazaki T, Honjo T. The PD-1-PD-L pathway in immunological tolerance. Trends Immunol (2006) 27(4):195–201. doi: 10.1016/j.it.2006.02.001
128. Batlle E, Massagué J. Transforming growth factor-B signaling in immunity and cancer. Immunity (2019) 50(4):924–40. doi: 10.1016/j.immuni.2019.03.024
129. Tauriello DVF, Palomo-Ponce S, Stork D, Berenguer-Llergo A, Badia-Ramentol J, Iglesias M, et al. TGF beta drives immune evasion in genetically reconstituted colon cancer metastasis. Nature (2018) 554(7693):538–43. doi: 10.1038/nature25492
130. Yi M, Zhang J, Li A, Niu M, Wu K. The construction, expression, and enhanced anti-tumor activity of YM101: A bispecific antibody simultaneously targeting TGF-B and PD-L1. J Hematol Oncol (2021) 14(1):27. doi: 10.1186/s13045-021-01045-x
131. Heiss MM, StröHlein MA, JäGer M, Kimmig R, Burges A, Schoberth A, et al. Immunotherapy of malignant ascites with trifunctional antibodies. Int J Cancer (2010) 117(3):435–43. doi: 10.1002/ijc.21165
132. Makoto K, Masayo U, Kanako K, Hitomi M, Kai M, Hideya O, et al. Catumaxomab with activated T-cells efficiently lyses chemoresistant EpCAM-positive triple-negative breast cancer cell lines. Anticancer Res (2018) 38(7):4273–9. doi: 10.21873/anticanres.12724
133. Sebastian M. Review of catumaxomab in the treatment of malignant ascites. Cancer Manage Res (2010) 2:283–6. doi: 10.2147/CMR.S14115
134. Gabriele H, Holger JD, Erich R. (2020). Engaging innate immunity for targeting the epidermal growth factor receptor: Therapeutic options leveraging innate immunity versus adaptive immunity versus inhibition of signaling. Front Oncol (2022) 12:892212. doi: 10.3389/fonc.2022.892212
Keywords: bispecific antibody, bsAb, antibody constructs, clinical trials, tumor immunotherapy
Citation: Kang J, Sun T and Zhang Y (2022) Immunotherapeutic progress and application of bispecific antibody in cancer. Front. Immunol. 13:1020003. doi: 10.3389/fimmu.2022.1020003
Received: 15 August 2022; Accepted: 05 October 2022;
Published: 20 October 2022.
Edited by:
Chun-Jie Liu, Children’s Hospital of Philadelphia, United StatesReviewed by:
Jianguo Sun, Xinqiao Hospital, ChinaYan Xu, Peking Union Medical College Hospital (CAMS), China
Copyright © 2022 Kang, Sun and Zhang. This is an open-access article distributed under the terms of the Creative Commons Attribution License (CC BY). The use, distribution or reproduction in other forums is permitted, provided the original author(s) and the copyright owner(s) are credited and that the original publication in this journal is cited, in accordance with accepted academic practice. No use, distribution or reproduction is permitted which does not comply with these terms.
*Correspondence: Yan Zhang, emhhbmcueWFuQHNjdS5lZHUuY24=
†These authors have contributed equally to this work and share first authorship