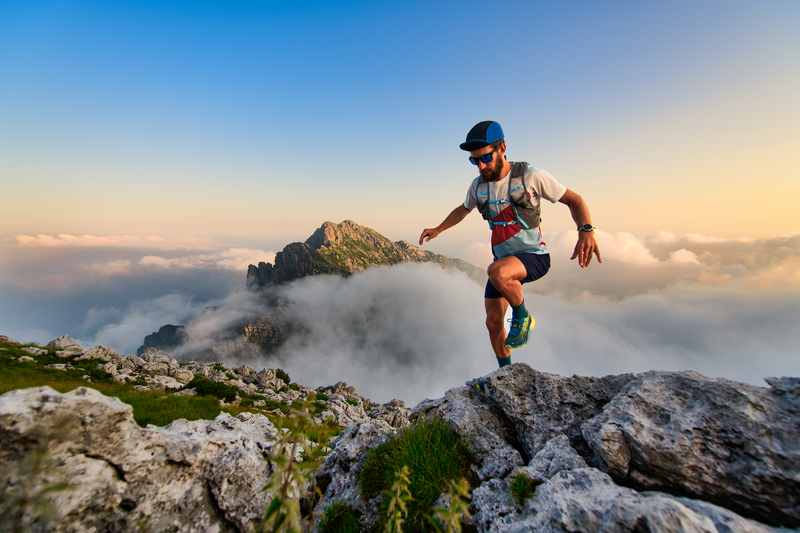
94% of researchers rate our articles as excellent or good
Learn more about the work of our research integrity team to safeguard the quality of each article we publish.
Find out more
REVIEW article
Front. Immunol. , 11 October 2022
Sec. Cancer Immunity and Immunotherapy
Volume 13 - 2022 | https://doi.org/10.3389/fimmu.2022.1016059
This article is part of the Research Topic Therapeutic Targeting Of MDSC In The Tumor And Immune Microenvironment View all 10 articles
The success of immunotherapeutic approaches in hematological cancers is partially hampered by the presence of an immunosuppressive microenvironment. Myeloid-derived suppressor cells (MDSC) are key components of this suppressive environment and are frequently associated with tumor cell survival and drug resistance. Based on their morphology and phenotype, MDSC are commonly subdivided into polymorphonuclear MDSC (PMN-MDSC or G-MDSC) and monocytic MDSC (M-MDSC), both characterized by their immunosuppressive function. The phenotype, function and prognostic value of MDSC in hematological cancers has been intensively studied; however, the therapeutic targeting of this cell population remains challenging and needs further investigation. In this review, we will summarize the prognostic value of MDSC and the different attempts to target MDSC (or subtypes of MDSC) in hematological cancers. We will discuss the benefits, challenges and opportunities of using MDSC-targeting approaches, aiming to enhance anti-tumor immune responses of currently used cellular and non-cellular immunotherapies.
The tumor microenvironment is a complex and dynamic network of distinct cell types (1–3). The composition of the environment is variable between different tumor types; however, it typically includes stromal cells, blood vessels, immune cells and extracellular matrix (4). Myeloid-derived suppressor cells (MDSC), tumor associated macrophages (TAM) and regulatory T-cell (Treg) are major components of the microenvironment and are critical drivers of immunosuppression, creating a tumor-promoting and drug resistant niche (5, 6).
MDSC are a heterogeneous population of immature myeloid cells and are generated in the bone marrow (BM) by myelopoiesis (7). Under healthy conditions, the precursor cells can terminally differentiate into mature dendritic cells, granulocytes or macrophages. However, in pathological circumstances including cancer, the differentiation of precursor cells is partially blocked, leading to an accumulation of an immature myeloid cell population, defined as MDSC (8, 9). MDSC are known to accumulate during cancer progression and promote tumor immune escape through multiple mechanisms including (i) the expression of enzymes [e.g., arginase (Arg), nitric oxide synthase (NOS), indoleamine 2,3-dioxygenase (IDO)], (ii) the release of reactive oxygen species (ROS), (iii) sequestering of cystine (↓ extracellular pool of cysteine), (iv) the interaction and stimulation of other immunosuppressive cell types (e.g., Treg) and (v) the secretion of immunosuppressive cytokines (e.g., IL-6, IL-10, TGF-β) (10–13).
In hematological malignancies, the presence and accumulation of MDSC is often correlated with a poor prognosis, however the optimal strategy to specifically eliminate MDSC or alter their suppressive function remains challenging (14). Immunotherapy emerged as one of the most promising treatment options for almost all types of hematological cancers and is primarily focused on the modulation/stimulation of T-cell using monoclonal antibodies, bispecific T-cell engagers, cell therapies, vaccines and immune checkpoint inhibitors (e.g., PD-1-, LAG-3-, CTLA-4-blocking antibodies) (15). In this regard, therapeutic strategies to tackle immunosuppressive cell types (including MDSC, TAM and Tregs) became an interesting option to increase anti-tumor immune responses and overcome the occurrence of drug resistance to currently used or investigated cancer immunotherapies.
MDSC are commonly subdivided into two groups: monocytic MDSC (M-MDSC) and granulocytic (or polymorphonuclear) MDSC (G-MDSC). The phenotype and morphology of M-MDSC is very similar to monocytes, G-MDSC and neutrophils also share common characteristics (e.g., arginase-mediated arginine depletion) (16–18). Despite the morphological and phenotypical similarities, functional differences between steady-state neutrophils and G-MDSC are described including a higher activity of arginase, myeloperoxidase (MPO), and ROS; reduced expression of CD16 and CD62L; and less granules in G-MDSC compared to neutrophils (17, 19). In recent years, it became clear that M-MDSC and G-MDSC also utilize distinct mechanisms to suppress the immune system. M-MDSC hamper T-cell responses in a STAT1/3- and iNOS-dependent manner, which is associated with increased NO and immunosuppressive cytokine production (IL-10, TGF-β). The effect of G-MDSC, on the other hand, is attributed to an antigen-specific induction of T-cell tolerance by STAT3 activity and increased expression of Arg-1, ROS, peroxynitrite and prostaglandin E2 (8).
In humans, the distinction between MDSC and monocytes/neutrophils can be made based on density gradient and phenotypic markers (e.g., expression HLA-DR), however the distinction between these subtypes in mice is much more challenging and therefore the nature and uniqueness of the MDSC populations continues to be a matter of debate. In murine models, MDSC are phenotypically defined as CD11b+GR1+ and further subdivided into CD11b+Ly6G−Ly6Clow for M-MDSC and CD11b+Ly6G+Ly6Clow for G-MDSC. In humans, both subtypes are distinguished based on the following phenotypic markers: CD11b+CD14+CD15−CD33+HLA-DR−/low for M-MDSC and CD11b+CD14−CD15+CD33+(CD66b+) for G-MDSC (17, 18). More recently, in humans, a third “early-stage” MDSC subset (eMDSC) has been identified, characterized as Lin− (CD3/14/15/19/56) HLA-DR−CD33+. This subset comprises immature progenitor and precursor cells with colony-forming activity, however its exact function and contribution to immune suppression remains unclear (20). Various reviews described the presence and immunosuppressive function of MDSC in hematological malignancies, however below we aimed to provide a brief and structured overview about the main findings on MDSC subsets and their prognostic value in different hematological cancers as this is particularly important in the context of therapeutic strategies (Table 1) (14, 20, 47–50).
Table 1 Summary of MDSC representative phenotype and their prognostic role in different hematological cancers.
Acute Myeloid Leukemia (AML) represents the most common myeloid malignancy and is characterized by the expansion of immature myeloid progenitors or blasts in the BM and peripheral blood (PB) (51). In AML, distinct MDSC subsets have been characterized and specifically the circulating M-MDSC subset (defined as CD14+HLA-DRlow) appeared to be elevated and correlated with a poor prognosis in AML patients (21, 22). In addition, eMDSC (CD33+CD11b+HLA-DR−/LowCD14−CD15−) were also increased in the PB of AML patients, however its impact of prognosis remains unknown (23). Interestingly, Sun et al. observed a correlation between the total number of MDSC in the BM (CD33+CD11b+HLA-DRlow/−) and minimal residual disease (MRD) (determined by flow cytometry), as MDSC levels in the high MRD group (MRD > 1×10−2) was significantly higher than that in the middle (1x10−2 > MRD > 1×10−4) and the low (MRD < 1×10−4) MRD groups (24).
Chronic Myeloid Leukemia (CML) is a hematopoietic stem cell malignancy characterized by the acquisition of the t (9, 48) chromosomal translocation leading to expression of the BCR/ABL oncogene (52). Both M-MDSC (CD14+HLA-DR−) and G-MDSC (CD11b+CD33+CD14-HLA-DR−) were increased in the PB of CML patients compared to healthy controls and treatment with the tyrosine kinase inhibitor imatinib decreased the MDSC percentages to normal levels (25, 53). Although higher levels of G-MDSC could be detected in high-risk patients (based on Sokal score) compared to low-risk patients, its impact on prognosis needs to be further elucidated (26).
In precursor B cell Acute Lymphoblastic Leukemia (B-ALL), a malignancy of precursor B cells with the highest incidence among children, elevated levels of G-MDSC (CD45+CD19−HLA-DR−CD11b+CD33+CD15+) in the PB and BM of newly diagnosed patients has been observed (27). Similar to the findings in AML, a correlation could be observed between the G-MDSC levels, in the BM and PB, and MRD status of B-ALL patients at diagnosis. In addition, the frequency of G-MDSC correlated positively with other prognostic indicators including the percentage of CD20+ cells and blast cells (14, 27, 28).
Chronic Lymphocytic Leukemia (CLL) arises from the clonal expansion of CD5+ B lymphocytes in the BM (54). A study of 49 CLL patients demonstrated an upregulation of CD14+HLA-DRlow/− M-MDSC compared to healthy patients (29). In addition, the elevated levels of M-MDSC were significantly correlated with tumor progression and a poor prognosis of CLL patients (30). The negative impact of M-MDSC (CD14+CD11b+CD15−HLA-DR−/low) on the clinical outcome of CLL patients was also confirmed by Kowalska et al. (31). In contrast, the study by Ferrer et al. found a significant increase in the G-MDSC (HLA-DRlowCD11b+CD33+CD15+) number of CLL patients which was associated with a poor clinical outcome. While CLL-derived G-MDSC suppressed T-cell growth in vitro, M-MDSC were less immunosuppressive due to the presence of TNFα and were defined as a more immunostimulatory subtype. The authors concluded that the G-MDSC appeared to be the preferred subtype to target, since they more effectively induce immune suppression in CLL patients (32).
Diffuse large B cell lymphoma (DLBCL) is the most common type of non-Hodgkin lymphoma (NHL) hat develops from the B lymphocytes. Azzaoui et al. observed an increase in M-MDSC (CD14+HLA-DRlow) and G-MDSC (Lin-HLA-DR-CD33+CD11b+) populations in DLBCL patients, however the M-MDSC were the only subset that could be correlated with the International Prognostic Index and event-free survival (33). This observation was confirmed by Wang et al. who demonstrated a significant increase in the circulating M-MDSC (CD14+CD33+HLA-DR−/low) of newly diagnosed and relapsed DLBCL patients and found that the level of M-MDSC could be used as a biomarker for poor prognosis of DLBCL patients (34).
The presence of Reed-Sternberg cells is a specific hallmark of Hodgkin lymphoma (HL). Romano et al. demonstrated that all circulating MDSC subsets (CD11b+CD33+CD14−CD34+HLA-DR− or immature MDSC, CD11b+CD33+CD14−HLA-DR− or G-MDSC, CD14+HLA-DRlow/− or M-MDSC) were increased in HL patients compared to normal subjects. Higher MDSC percentages were present in non-responders and CD34+ immature MDSC were predictive for a short progression-free survival in HL patients (35).
More recently, a study in B-NHL patients including CLL, DLBCL, marginal zone lymphoma (MZL), high‐grade B‐cell lymphoma (HGBL), mantle‐cell lymphoma (MCL), primary central nervous system lymphoma (PCNSL) and follicular lymphoma (FL) patients was carried out to investigate the impact of MDSC number and subsets (CD14+CD33+HLA‐DR−/low for M‐MDSC, CD10‐HLA‐DR−/low for G‐MDSC) on B-NHL patient’s prognosis. A significant increase could be observed in the levels of M-MDSC and G-MDSC in the diverse types of B-NHL compared to healthy donors. MDSC levels were closely associated with disease progression (tumor stage, LDH levels) and both subsets were defined as effective indicators of poor prognosis in B-NHL patients (36, 55).
Multiple myeloma (MM) is a plasma cell malignancy in which monoclonal plasma cells proliferate in the BM (56). Controversial results were reported regarding the MDSC levels and subtypes present in MM patients. One of the first studies demonstrated elevated levels of M-MDSC (CD14+HLA-DR−/low) in MM patients at diagnosis compared to healthy controls (57). In addition, M-MDSC levels were correlated with tumor progression and MDSC levels could be considered as an indicator for the efficacy of therapy (37, 38). A study by Bae et al. recently confirmed the independent adverse prognostic impact of PB derived M-MDSC in patients with MM and suggested the analysis of M-MDSC as a prognostic marker in clinical practice (39). In the context of autologous stem cell transplantation (ASCT), lower M-MDSC levels were associated with a longer time to progression. Interestingly, pre-ASCT derived M-MDSC strongly inhibited the in vitro cytotoxic effect of melphalan; which could be reduced by the blockade of colony-stimulating factor 1 receptor (CSF1R) (40). However, more recent studies demonstrate a significant increase of G-MDSC (CD11b+CD33+HLA‐DR−/lowCD14-CD15+) in BM and PB of MM patients compared to monoclonal gammopathy of undetermined significance (MGUS), smoldering MM patients and healthy controls, while no significance could be observed for M-MDSC (41–44). The increase in G-MDSC was also associated with MM disease activity and could be used to predict the response to immunomodulatory agent lenalidomide (45). Perez et al. also observed a correlation between the clinical significance, immunosuppressive potential, and transcriptional network of well-defined neutrophil subsets. In addition, they suggested a set of optimal markers (CD11b/CD13/CD16) for accurate monitoring of G-MDSC in MM patients (46).
In past years, some specific and various unspecific strategies have been investigated to either modulate the MDSC suppressive function, affect their differentiation/maturation potential, block MDSC development or deplete this cell population in the tumor microenvironment. Below, and in Figure 1 and Table 2, we will summarize all strategies that have been tested in the context of hematological cancers.
Figure 1 The landscape of MDSC-targeting strategies in hematological cancers. Multiple MDSC-targeting approaches were evaluated in hematological cancers to restore the anti-tumor immune response, including: (A) depleting MDSC populations through low-dose chemotherapy agents, mAbs, peptibodies, brentuximab vedotin, epigenetic compounds, CD33/CD3-bispecific T-cell engaging (BiTE®) antibody, LXR agonist RGX-104, Immunomodulatory drugs et al; (B) attenuating the immunosuppressive mechanisms of MDSC by immune checkpoint inhibitors, tyrosine kinase inhibitors, MRIAN, notch inhibitors, S100A9 inhibitors, STAT3 inhibitors, phosphodiesterase-5 inhibitors, histamine hydrochloride; arginase inhibitors; (C) inducing the differentiation of MDSC into mature myeloid cells by all-trans-retinoic acid (ATRA) to reduce MDSC population and remove their immunosuppression; (D) inhibiting MDSC accumulation in the tumor microenvironment by palmitoyltransferase inhibitor and zoledronic acid. mAb, monoclonal antibody; BiTE, bi-specific T-cell engagers; 5-FU, 5-fluorouracil; LXR, activation of liver X receptor; STAT3, signal transducer and activator of transcription 3; NOX2, NADPH oxidase 2; ATRA, all-trans-retinoic acid; DC, dendritic cell.
5-Fluorouracil and Gemcitabine, both chemotherapeutic compounds routinely used in the clinic for the treatment of cancer, have been described to decrease the number of MDSC in preclinical mouse models of hematological cancers (58–60). Due to the low selectivity and dose-dependent toxicity, various encapsulated gemcitabine formulations have been developed and examined for safety and tumor-directed toxicity. Sasso et al. demonstrated that low dose gemcitabine-loaded lipid nanocapsules efficiently targeted the M-MDSC subset and relieved tumor-associated immunosuppression in vitro and in vivo using the E.G7-OVA lymphoma model. The efficient uptake of the nanocapsules into the M-MDSC subset was attributed to a mechanism called ‘macropinocytosis’. Moreover, authors found that preconditioning with low dose gemcitabine-loaded lipid nanocapsules enhanced the efficacy of adoptive T-cell therapy in the E.G7-OVA tumor model, further illustrating its potential as immune modulating therapy in cancer (61).
Daratumumab is an anti-CD38 monoclonal antibody, FDA approved in 2015 for the treatment of relapsed/refractory MM patients. Besides the ubiquitous expression of CD38 on MM cells, CD38 antigen is also expressed by other cell types including MDSC and regulatory B cells (62). Krejcik et al. demonstrated that in vitro generated G-MDSC (CD11b+CD14–HLA–DR–CD15+CD33+) expressed elevated CD38 and were highly sensitive to daratumumab-mediated ADCC/CDC compared with the isotype control. Findings were confirmed in patients treated with a combination of lenalidomide, dexamethasone with or without daratumumab. Using western blot analysis, a selective reduction of M-MDSC was observed in patients treated with the triple combination compared to patients treated with dexamethasone and lenalidomide (93). Data obtained by Cohen et al. further supported the daratumumab-mediated depletion of M-MDSC using a combination of daratumumab and anti-PD-1 monoclonal antibody cetrelimab in relapsed/refractory MM patients (63).
Using a competitive peptide phage display platform, candidate peptides were identified that specifically bind to MDSC derived from EL4 mice, a murine lymphoblastic tumor model. Peptides were fused with the Fc portion of mouse IgG2b to generate MDSC-specific peptibodies. In vivo studies in lymphoma models including A20, EL4 and E.G7-OVA demonstrated that the peptibodies were able to deplete intra-tumoral MDSC, without affecting other inflammatory cell types (e.g., dendritic cells and T-cell). In contrast to anti-GR1 depleting antibodies which preferentially eliminate G-MDSC, peptibodies were able to deplete both M-MDSC and G-MDSC subsets. Peptibodies significantly delayed tumor growth in EL4 mice and alarmins S100A8/S100A9 were identified as potential candidate targets expressed by the MDSC (64, 94).
Brentuximab Vedotin (BV) is an antibody-drug conjugate designed to selectively deliver monomethylauristatin E, a microtubule-disrupting agent, to CD30-expressing cells. The compound has been FDA approved in 2018 for the treatment of patients with previously untreated stage III or IV classical HL in combination with chemotherapy (95). Although it remains unclear whether CD30 is expressed or not on MDSC subsets, Romano et al. demonstrated that BV reduced the absolute number of three MDSC subtypes (CD11b+CD33+CD14−CD34+HLA-DR−; M-MDSC and G-MDSC) coinciding with reduced soluble Arg-1 levels and restored the entire T-cell populations in HL patients; indicating its therapeutic use as MDSC targeting agent (65).
5-aza-2′-deoxycytidine, also known as decitabine (DAC), has been shown to act as an irreversible inhibitor of DNA methyltransferases and induces gene-specific DNA demethylation when administered at a low dose (96). Besides the reactivation of tumor suppressor genes through demethylation, DAC exerts pleiotropic effects on the tumor immune microenvironment including the upregulation of MHC-I/MHC-II expression levels, the increased expression of co-stimulatory molecules and the targeting of immunosuppressive cell types. The effect of DAC on MDSC subtypes was analyzed in leukemia (WEHI-3), lymphoma (EL4) and MM (MPC11) models in vitro and in vivo. DAC treatment induced MDSC apoptosis (CD11b+GR1+) in vitro and increased T-cell activation in leukemia and lymphoma models. In the MCP11 MM model, DAC inhibited MM cell proliferation and induced an autologous T-cell immune response by depleting the M-MDSC subset in the MM BM microenvironment (66, 67).
Histone deacetylase (HDAC) inhibitors (e.g. entinostat, valproic acid, vorinostat) are another class of epigenetic compounds and were also reported to reduce MDSC levels or inhibit MDSC suppressive capacity in solid tumor models (97). Treatment of BM mononuclear cells of MM patients with ACY241, an HDAC6 selective inhibitor, significantly reduced the HLA-DRlow/-CD11b+CD33+ MDSC population, while it augments the immune response as evidenced by increased perforin/CD107a expression, IFN-γ/IL-2/TNF-α production and antigen-specific central memory cytotoxic T lymphocytes (68).
AMG 330 is the first BiTE® developed against CD33, an antigen that is not only expressed on the majority of AML-blasts, but also on M-MDSC (98). Jitschin et al. observed an increase in the percentage of HLA-DRlow (CD14+CD11b+) M-MDSC, that co-express CD33, in newly diagnosed AML patients compared to healthy controls. In the presence of AMG 330, T-cell were able to eliminate CD33+IDO+ in vitro generated MDSC. Adding MDSC to co-cultures of T-cell and AML cells resulted in reduced AML-blast killing, while the addition of an IDO inhibitor promoted the AMG 330-mediated clearance of AML-blasts. Data suggest a dual anti-tumor effect of AMG 330 through increased T-cell mediated cytotoxicity against AML blasts and CD33+ MDSC (69). Another study by Cheng et al. evaluated the effects of AMV 564, a novel bivalent CD33/CD3 T-cell engager and showed immunodepletion of MDSC and anti-tumor activity using primary samples of myelodysplastic syndrome (MDS) patients and a disseminated leukemia mouse model (70).
Liver-X nuclear receptors (LXR) are members of the nuclear hormone receptor family that drive, among others, the transcriptional activation of ApoE. Masoud et al. observed that an LXR agonist RGX-104 induces apoptosis of MDSC and enhances T-cell activation in solid tumor models (71). RGX-104 is currently evaluated in an ongoing phase 1 clinical trial in patients with metastatic solid cancers or lymphomas that have progressed on standard therapies (NTC02922764). Blood sample analysis revealed a depletion of G-MDSC and increased T-cell activation after treatment of cancer patients with RGX-104.
Immunomodulatory drugs (IMiDs), including lenalidomide and pomalidomide, are a group of drugs that are derivatives from thalidomide and are routinely used in the treatment of MM (99). Kuwahara-Ota et al. examined the impact of IMiDs on MDSC in vitro and found a significant reduction in MDSC level upon coculture of MM-derived PB mononuclear cells and human MM cell lines, with pomalidomide being more potent than lenalidomide (72). However, clinical evidence supporting this hypothesis is missing as lenalidomide-treated patients showed a higher abundance of CD14+CD15+ MDSC. Moreover, in vitro findings by Görgun et al. demonstrated that lenalidomide could not overcome MDSC-mediated T-cell suppression in MM (100). In the A20 lymphoma tumor model, a lenalidomide-associated reduction in systemic MDSC number and increased immune activation has been observed, further illustrating the controversy regarding the impact of lenalidomide on MDSC populations, depending on the used tumor model and type.
In MM, the immune checkpoint PD-L1 was significantly higher expressed on the G-MDSC subset of BM and PB-derived MM patients (newly diagnosed and relapsed) compared to G-MDSC of MGUS and healthy individuals (42). Although some studies in solid tumors suggest that PD-L1 blocking could partially restore the MDSC suppressive function, Ahn and colleagues could not observe any effect of a PD-L1 blocking antibody on splenic MDSC number or subsets in the MOPC-315 immunocompetent MM model (101–104). To fully elucidate whether PD-L1 expression on MDSC is linked to its suppressive function, additional studies are required in MM models that allow BM-derived MDSC investigation as well.
V-domain Ig suppressor of T-cell activation (VISTA) or PD-1H is a novel checkpoint regulator that is predominantly expressed in the hematopoietic compartment, and particularly within the myeloid lineage (105). In solid tumors, inhibition of VISTA resulted in improved anti-tumor immune responses in vivo and currently clinical trials are ongoing to assess its therapeutic potential in advanced solid tumor malignancies (NCT05082610, NCT04475523) (106). In AML, VISTA was found to be highly expressed on monocytes (CD45intCD11b+CD14high/low) and myeloid leukemia blasts (CD45in vs. SCC). VISTA expression on PB-derived MDSC (CD11b+CD33+ HLA-DR−) was significantly higher in AML patients compared to healthy controls. In addition, siRNA mediated knockdown of VISTA in MDSC resulted in increased T-cell proliferation in vitro and diminished the MDSC-mediated suppression of CD8+ T-cell. Strikingly, the authors observed a strong correlation between VISTA-expressing MDSC and PD-1 expressing T-cells (including CD4, CD8 and Treg), indicating a link between both checkpoints to suppress the immune system in AML patients (73). In another study, VISTA-expressing murine myeloid leukemia cells were injected into wild type and PD-1H (VISTA) knock out mice. Authors observed a reduction in AML cell growth in PD-1H knock out mice, which was further diminished by the administration of PD-1H blocking antibodies. These data suggest that VISTA expression on both the host cells and AML cells are involved in the cancer immune evasion. Moreover, epigenetic modulation using DAC further increased the overall survival of PD-1H knock out mice, indicating the potential of combining both compounds in clinical setting (74).
Ibrutinib is a first-in-class oral irreversible inhibitor of Bruton Tyrosine Kinase (BTK), a critical enzyme in the B-cell receptor signaling cascade, and is highly effective in the treatment of CLL, MCL and Waldenstrom’s macroglobulinaemia. BTK has been described to be expressed by MDSC and treatment with ibrutinib was found to affect the MDSC generation and function in solid tumor models, indicating its therapeutic potential to increase immune-based therapies (107, 108). A study by Ferrer et al. demonstrated that G-MDSC were the preferential subset to target in CLL patients to increase T-cell function. Three months ibrutinib therapy of CLL patients resulted in a significant decline of G-MDSC, while M-MDSC and monocytes remained unaffected. While ibrutinib had no direct effect on the T-cell suppressive activity, it skewed the T-cell differentiation to T helper 1 cells in the presence of MDSC, indicating a change from an immunosuppressive towards a more immune effective state (32).
The effect of other tyrosine kinase inhibitors including imatinib, nilotinib and dasatinib on MDSC levels was evaluated in CML patients. All compounds induced a significant reduction in G‐MDSC at 3–6 months and 9–12 months of treatment. However, the M-MDSC subset was not significantly changed during imatinib and nilotinib therapy and was only reduced in dasatinib‐treated patients. Interestingly, a significant correlation was found between the major molecular response (MMR) values and number of persistent M‐MDSC at 12 months of dasatinib treatment, indicating its prognostic value in these patients (75, 76).
Metabolic Reprogramming Immunosurveillance Activation Nanomedicine (MRIAN) is an L-phenylalanine polymer, developed to target the immunosuppressive BM microenvironment by inhibiting MDSC. MRIAN reduced ROS levels and induced MDSC differentiation towards functional immune cells (e.g., macrophages, natural killer cells, dendritic cells). In T-ALL mice, MRIAN significantly improved the T-cell number and function by inhibiting MDSC. Studies also demonstrated an enhanced cellular uptake of MRIAN in T-ALL cells and MDSC compared to normal hematopoietic cells and progenitors. MRIAN assembled to doxorubicin (MRIAN-Dox) demonstrated an enhanced anti-tumor efficacy and reduced toxicity profile (including cardiotoxicity and myeloablation side effects) in T-ALL mice; indicating its therapeutic potential as metabolic modifier to target MDSC (77).
The Notch signaling pathway has been identified to play a key role in MDSC accumulation (109–111). In transgenic mice overexpressing ADAM10, a Notch processing enzyme, an accumulation of systemic CD11b+Gr1+ MDSC was found (78). A study by Grazioli et al. observed an expansion of MDSC in a transgenic mouse model of Notch3-dependent T-ALL. Interestingly, using both in vitro and in vivo experiments, they found that CD4+CD8+ T-cell (derived from the Notch3-transgenic mice) were the drivers of MDSC accumulation, through a mechanism that was dependent on both Notch and IL6. Conversely, anti-Gr1-mediated depletion of MDSCs in T-ALL-bearing mice significantly reduced the proliferation and expansion of malignant T-cell. These data were confirmed by coculturing human Notch-dependent T-ALL cell lines and healthy donor derived PB mononuclear cells in vitro, resulting in increased CD14+HLA-DRlow/neg MDSC accumulation and T-cell suppression; effects that were not observed with T-ALL cells that did not express Notch1- or Notch3-activated protein (79).
Another therapeutic approach to alter Notch signaling is the use of anti-Jagged blocking antibodies. Sierra et al. assessed the anti-tumor and immunogenic effect of CTX014, a humanized IgG1 blocking antibody, cross-reactive for both mouse and human Jagged1 and 2, in solid and hematological tumor models. Surprisingly, results demonstrated an increase of CD11b+GR1+ MDSC in tumors of mice treated with anti-Jagged therapy compared to vehicle. Data suggested that anti-Jagged therapy triggered an anti-tumor immune response through induction of immunogenic MDSC-like cells. Anti-tumor and immunogenic effects of anti-Jagged therapy was evaluated in an E.G7-OVA T-cell lymphoma model in combination with adoptive T-cell transfer of OT-I cells. Results showed that anti-Jagged therapy could overcome tumor-induced immune tolerance and increased the effect of the T-cell based immunotherapy (80).
In solid tumors, targeting Notch using γ-secretase inhibitors significantly increased the MDSC number in preclinical cancer models (112). There was a specific increase in the G-MDSC subset and a downregulation of CD80, CD115 and CD124 markers, all associated with MDSC suppressive function. Using short hairpin constructs against RBP-J, Notch signaling was attenuated in BM cells and this resulted in reduced MDSC suppressive capacity. In addition, injection of RBP-J-deficient MDSC in tumor-bearing mice significantly reduced the tumor growth compared to controls (79, 113).
Altogether, these studies revealed a role of Notch signaling in the accumulation and suppressive function of MDSC in tumor-bearing mice. However, whether the effect is direct, indirect or a combination of both remains to be elucidated.
S100A9 is a calcium-binding protein, mainly secreted by granulocytes and monocytes, and has been reported to be essential for MDSC survival and accumulation in tumor-bearing mice including MM and lymphoma models (114). In MM, our group demonstrated the expression of S100A9 and its receptor TLR4 in both monocytic and granulocytic MDSC subsets. S100A9 acted as a chemoattractant for MM cells in vitro and induced the expression of pro-inflammatory cytokines by MDSC (e.g., TNFα, IL-6, IL-10). Targeting the interaction of S100A9 and its receptors using ABR-238901 did not affect MDSC accumulation, but significantly reduced cytokine expression by MDSC. Moreover, anti-angiogenic and anti-MM effects were observed in vivo using a combination therapy of ABR-238901 and bortezomib (81). Recently, we also investigated the effects of S100A9 inhibitor tasquinimod, currently evaluated in clinical trial for relapsed/refractory MM patients and observed a clear reduction in the M-MDSC subset in vivo (NCT04405167). In addition, tasquinimod abolished the immunosuppressive activity of in vitro generated MDSC, illustrating its potential as an immunotherapeutic compound (82).
Although STAT3 activation is known to play a pivotal role in MDSC accumulation and function, the effects of STAT3 inhibitors on MDSC activity is rather controversial (115, 116). AZD1480, a small-molecule inhibitor of JAK1/2 kinase, significantly decreased MDSC number and delayed tumor growth in MO4 melanoma-bearing mice. Despite a decrease in MDSC percentage, Maenhout et al. observed an enhanced MDSC-suppressive capacity and impaired T-cell proliferation and IFN-γ secretion upon treatment with AZD1480 (117). AZD9150, a next-generation antisense oligonucleotide inhibitor of STAT3, also demonstrated potent anti-tumor effects of lymphoma cell lines and in preclinical lymphoma models (83). The inhibitor was evaluated in a small group of non-HL patients and three out of four patients showed a decrease in the circulating G-MDSC population and an increase in CD4+ and CD8+ T-cell (118). Napabucasin, another STAT3 inhibitor, was also found to abrogate the MDSC suppressive function in solid tumors and exhibited potent cytotoxicity against NHL cell lines (119, 120). However, napabucasin-mediated MDSC-targeting and modulation has not been investigated in hematological cancers so far.
Phosphodiesterase-5 (PDE5) inhibitors (e.g., sildenafil, tadalafil, vardenafil), particularly used for nonmalignant conditions in the clinic, have been found to increase anti-tumor immune responses by altering the MDSC suppressive function and restoring anti-tumor immunity (121). Using the A20 lymphoma model, it has been found that IL4Rα expression on MDSC correlated with tumor progression and could be inhibited using sildenafil. In addition, sildenafil reduced lymphoma-induced T-cell anergy and expansion of regulatory Treg (84). A case report of a patient with end-stage relapsed/refractory MM showed that the addition of tadalafil to its treatment regimen (lenalidomide, clarithromycin, dexamethasone) reduced the MDSC suppressive activity, as illustrated by a reduction in IL4Rα+, iNOS, Arg-1 and ROS. Interestingly, the changes in MDSC function were more pronounced in the BM compared to the blood and were associated with an increase in T-cell function (↑ IFNγ expression). With the administration of tadalafil, the patient could tolerate the combination of lenalidomide and dexamethasone and achieved a very good partial response (+/- 90% reduction in tumor burden) (85). Although a clinical trial was initiated combining tadalafil, dexamethasone and lenalidomide in MM patients who were refractory to lenalidomide-based regimens, the study was terminated at an early stage due to a lack of response. The limited efficacy could be explained by the low number of MDSC present in the patients at the time of enrollment, potentially attributed to the pre-treatment with lenalidomide (86). Further studies are required to investigate the impact of PDE-5 inhibitors in patients with elevated MDSC levels.
Histamine hydrochloride (HDC) is a NOX2 inhibitor and is known to inhibit the immunosuppressive function of myeloid cells by reducing ROS production (122). Low-dose IL-2 combined with HDC is approved in Europe for remission maintenance in adult AML patients. Grauers et al. further unraveled the impact of HDC on MDSC number and function using the EL4 lymphoma tumor model. HDC significantly reduced MDSC number in vivo and altered the MDSC-induced immunosuppression of T-cells ex vivo. Moreover, using Nox2 knock out mice and GR1-depleting antibodies, it has been suggested that HDC exerted its anti-tumor effects by targeting the NOX2+ GR1+ cells in vivo. Finally, authors also observed an enhanced anti-tumor efficacy using the combination of HDC and anti-PD-1 antibodies in the EL4 lymphoma model. HDC-mediated effects on MDSC were further evaluated using blood samples of AML patients that received HDC in conjunction with low-dose IL-2 for relapse prevention (NCT01347996) (87). HDC/IL-2 therapy resulted in a significant reduction in the frequency and absolute counts of M-MDSC, and this strong reduction significantly predicted the leukemia-free survival.
Arginase is a key enzyme involved in the immunosuppressive function of G-MDSC. Romano et al. demonstrated that Arg-1 is mainly expressed by G-MDSC in MM, and that both Arg-1 and G-MDSC are reduced after treatment with lenalidomide in vivo (45). Interestingly, Vonwirth et al. demonstrated that arginase inhibition, using nor-NOHA or CB-1158, could reduce T-cell anergy of MM patients in the presence of supernatant derived from polymorphonuclear neutrophil granulocytes (~G-MDSC). In preclinical solid tumor models, arginase inhibitor CB-1158 inhibited MDSC-mediated immunosuppression, increased T-cell proliferation and activity, and reduced tumor growth in vivo (89). A first-in-human phase 1 study in solid tumors demonstrated that CB-1158 was well tolerated and achieved on-target inhibition as illustrated by the increase in plasma arginine (16, 88). Moreover, arginase inhibition has been proposed as an interesting adjuvant therapy by Mussai et al. in leukemia patients. Inhibition of the arginine metabolism by L-NMMA and L-NOHA enhanced the proliferation and cytotoxicity of anti-NY-ESO (AML associated cancer-testis antigen) T-cells against epigenetically-treated AML blasts. In addition, it could also boost the anti-CD33 Chimeric Antigen Receptor T-cell cytotoxicity against AML, further illustrating its potential as adjunct therapy in hematological cancers (89).
All-trans-retinoic acid (ATRA), a vitamin A derivative, has been described as an inducer of myeloid cell differentiation and maturation, reducing MDSC number and inducing activation of immune responses in preclinical hematological and solid tumor models (90). In acute promyelocytic leukemia (APL) patients, peripheral ‘group 2 innate lymphoid cells’ (ILC2s) were found to be increased and hyperactivated, and in turn activated M-MDSC (CD14+CD33+) through IL-13 secretion. Using patient samples and APL mice, authors demonstrated that ATRA-treatment reversed the increase in ILC2 induced M-MDSC, accompanied by an increase in T-cell function in vitro and in vivo (91).
Unfortunately, due to a poor solubility and fast drug metabolism, the clinical application of ATRA has been limited. Recently, a drug encapsulated liposome formulation L-ATRA has been developed with sustained release properties. In vitro treatment of myeloid leukemia cell lines HL-60 and NB4 resulted in increased expression of myeloid differentiation markers CD11b and CD11c, illustrating its therapeutic potential to target MDSC (123).
It has been shown that CD14+HLA-DRlow M-MDSC accumulate in newly diagnosed AML patients. Tohumeken et al. found that AML-derived extracellular vesicles were taken up by conventional monocytes in vitro which subsequently underwent MDSC differentiation. Apparently, the presence of palmitoylated proteins on the surface of AML-derived extracellular vesicles was responsible for the activation of TLR2/Akt/mTOR signaling and accumulation of MDSC. TLR2 neutralizing antibodies, mTOR inhibitor rapamycin or palmitoyltransferases inhibitor 2-BP abolished the generation of MDSC, indicating its potential therapeutic application as MDSC-targeted therapies (92).
Zoledronic acid is a bisphosphonate used for the treatment of MM associated hypercalcemia and bone metastasis in solid tumors (124). Although no information is available on the MDSC-targeting potential of zoledronic acid in hematological malignancies, Porembka et al. observed a reduced MDSC accumulation and improved anti-tumor immune response in pancreatic cancer models (125). These data suggest that zoledronic acid might exert a dual role as anti-MM therapy, impacting on the bone disease and the accumulation of immunosuppressive cell types.
Although not yet tested in hematological malignancies, other specific/unspecific MDSC-targeting approaches might be considered in the future. For example, an indoleamine-pyrrole 2,3-dioxygenase (IDO) peptide vaccine has been developed and significantly decreased IDO-expressing MDSC. The peptide vaccine delayed tumor progression in solid tumors inoculated with either IDO+ or IDO- tumor cells, indicating the therapeutic effect was partially mediated by targeting of the immunosuppressive environment (126).
Consistent with the results obtained using AMG 330 and AMV 564, CD33-directed therapy with gemtuzumab ozogamicin demonstrated MDSC depleting capacity in solid tumor models. CD33 was expressed on blood and tissue-derived MDSC of patients across different cancer subtypes, indicating its broad therapeutic potential (127).
The past years, immune checkpoint inhibitors and chimeric antigen receptor (CAR) T-cell therapies emerged as concomitant approaches to treat hematological cancers. However, the presence of immunosuppressive MDSC influences their efficacy. A study in large B-cell lymphoma patients receiving axicabtagene ciloleucel (axi-cel), a CD19-directed CART-cell therapy, demonstrated a clear association between poor CART-cell expansion and PB M-MDSC (128). Combinatorial approaches using CART- therapy or immune checkpoint inhibitors with MDSC-targeting agents (e.g., ATRA, gemtuzumab ozogamicin, AMV 564) clearly enhanced the anti-tumor efficacy in solid tumor models (127–129). These results imply the importance of using a similar approach in the treatment of hematological cancers.
Despite the controversy surrounding the nature and uniqueness of MDSC, there is no exists about their value as a therapeutic target in hematological cancers. MDSC contribute to tumor cell survival, immunosuppression and drug resistance; however, strategies to specifically eliminate this cell population or block their development are rather limited. Differences in analysis, tumor models, disease stages and treatment-related changes certainly contributed to the complexity to identify unique markers and specific approaches to tackle this cell type and reverse their immunosuppressive capacity. Further developments and applications of single-cell multi-omics will provide unique insights about the MDSC phenotypical markers and subsets, hopefully leading to a more specific MDSC-targeting approach in future. In addition, as MDSC are key regulators of immunosuppression, they contribute to the reduced effectiveness of current immunotherapeutic approaches including CAR-T therapy and immune checkpoint inhibitors. Specific targeting of these cell types in combination with other immunotherapies should be evaluated in clinical trials as this approach might be the key to increase anti-tumor immune responses and improve patient’s outcome.
RF and KDV developed the design and arguments for the paper, drafted the manuscript and designed the figures. AM, NB, EM, EB, KV, KM, and KB revised the manuscript. All authors contributed to the article and approved the submitted version.
This work was supported by Fonds voor Wetenschappelijk Onderzoek (FWO), Wetenschappelijk Fonds Willy Gepts (WFWG), and Strategic Research Programme (SRP48). KDV is a postdoctoral fellow of FWO (12I0921N).
The authors declare that the research was conducted in the absence of any commercial or financial relationships that could be construed as a potential conflict of interest.
All claims expressed in this article are solely those of the authors and do not necessarily represent those of their affiliated organizations, or those of the publisher, the editors and the reviewers. Any product that may be evaluated in this article, or claim that may be made by its manufacturer, is not guaranteed or endorsed by the publisher.
1. Farc O, Cristea V. An overview of the tumor microenvironment, from cells to complex networks (Review). Exp Ther Med (2021) 21(1):96. doi: 10.3892/etm.2020.9528
2. García-Ortiz A, Rodríguez-García Y, Encinas J, Maroto-Martín E, Castellano E, Teixidó J, et al. The role of tumor microenvironment in multiple myeloma development and progression. Cancers (Basel) (2021) 13(2):217. doi: 10.3390/cancers13020217
3. Simioni C, Conti I, Varano G, Brenna C, Costanzi E, Neri LM. The complexity of the tumor microenvironment and its role in acute lymphoblastic leukemia: Implications for therapies. Front Oncol (2021) 11:673506. doi: 10.3389/fonc.2021.673506
4. Anderson NM, Simon MC. The tumor microenvironment. Curr Biol (2020) 30(16):R921–r5. doi: 10.1016/j.cub.2020.06.081
5. Engblom C, Pfirschke C, Pittet MJ. The role of myeloid cells in cancer therapies. Nat Rev Cancer (2016) 16(7):447–62. doi: 10.1038/nrc.2016.54
6. Ohue Y, Nishikawa H. Regulatory T (Treg) cells in cancer: Can treg cells be a new therapeutic target? Cancer Sci (2019) 110(7):2080–9. doi: 10.1111/cas.14069
7. Gabrilovich DI, Nagaraj S. Myeloid-derived suppressor cells as regulators of the immune system. Nat Rev Immunol (2009) 9(3):162–74. doi: 10.1038/nri2506
8. Gabrilovich DI, Ostrand-Rosenberg S, Bronte V. Coordinated regulation of myeloid cells by tumours. Nat Rev Immunol (2012) 12(4):253–68. doi: 10.1038/nri3175
9. Li K, Shi H, Zhang B, Ou X, Ma Q, Chen Y, et al. Myeloid-derived suppressor cells as immunosuppressive regulators and therapeutic targets in cancer. Signal Transduction Targeted Ther (2021) 6(1):362. doi: 10.1038/s41392-021-00670-9
10. Tang H, Li H, Sun Z. Targeting myeloid-derived suppressor cells for cancer therapy. Cancer Biol Med (2021) 18(4):992–1009. doi: 10.20892/j.issn.2095-3941.2020.0806
11. Gabrilovich DI. The dawn of myeloid-derived suppressor cells: Identification of arginase I as the mechanism of immune suppression. Cancer Res (2021) 81(15):3953–5. doi: 10.1158/0008-5472.Can-21-1237
12. Holmgaard RB, Zamarin D, Li Y, Gasmi B, Munn DH, Allison JP, et al. Tumor-expressed ido recruits and activates mdscs in a treg-dependent manner. Cell Rep (2015) 13(2):412–24. doi: 10.1016/j.celrep.2015.08.077
13. Ohl K, Tenbrock K. Reactive oxygen species as regulators of mdsc-mediated immune suppression. Front Immunol (2018) 9:2499. doi: 10.3389/fimmu.2018.02499
14. Bizymi N, Bjelica S, Kittang AO, Mojsilovic S, Velegraki M, Pontikoglou C, et al. Myeloid-derived suppressor cells in hematologic diseases: Promising biomarkers and treatment targets. Hemasphere (2019) 3(1):e168. doi: 10.1097/hs9.0000000000000168
15. Noh JY, Seo H, Lee J, Jung H. Immunotherapy in hematologic malignancies: Emerging therapies and novel approaches. Int J Mol Sci (2020) 21(21):1–23. doi: 10.3390/ijms21218000
16. Vonwirth V, Bülbül Y, Werner A, Echchannaoui H, Windschmitt J, Habermeier A, et al. Inhibition of arginase 1 liberates potent T cell immunostimulatory activity of human neutrophil granulocytes. Front Immunol (2020) 11:617699. doi: 10.3389/fimmu.2020.617699
17. Bronte V, Brandau S, Chen S-H, Colombo MP, Frey AB, Greten TF, et al. Recommendations for myeloid-derived suppressor cell nomenclature and characterization standards. Nat Commun (2016) 7(1):12150. doi: 10.1038/ncomms12150
18. Bergenfelz C, Leandersson K. The generation and identity of human myeloid-derived suppressor cells. Front Oncol (2020) 10:109. doi: 10.3389/fonc.2020.00109
19. Youn JI, Collazo M, Shalova IN, Biswas SK, Gabrilovich DI. Characterization of the nature of granulocytic myeloid-derived suppressor cells in tumor-bearing mice. J Leukoc Biol (2012) 91(1):167–81. doi: 10.1189/jlb.0311177
20. Gunes EG, Rosen ST, Querfeld C. The role of myeloid-derived suppressor cells in hematologic malignancies. Curr Opin Oncol (2020) 32(5):518–26. doi: 10.1097/cco.0000000000000662
21. Pyzer AR, Stroopinsky D, Rajabi H, Washington A, Tagde A, Coll M, et al. Muc1-mediated induction of myeloid-derived suppressor cells in patients with acute myeloid leukemia. Blood (2017) 129(13):1791–801. doi: 10.1182/blood-2016-07-730614
22. Wang H, Tao Q, Wang Z, Zhang Q, Xiao H, Zhou M, et al. Circulating monocytic myeloid-derived suppressor cells are elevated and associated with poor prognosis in acute myeloid leukemia. J Immunol Res (2020) 2020:7363084. doi: 10.1155/2020/7363084
23. Lv J, Zhao Y, Zong H, Ma G, Wei X, Zhao Y. Increased levels of circulating monocytic- and early-stage myeloid-derived suppressor cells (Mdsc) in acute myeloid leukemia. Clin Lab (2021) 67(3):1. doi: 10.7754/Clin.Lab.2020.200719
24. Sun H, Li Y, Zhang ZF, Ju Y, Li L, Zhang BC, et al. Increase in myeloid-derived suppressor cells (Mdscs) associated with minimal residual disease (Mrd) detection in adult acute myeloid leukemia. Int J Hematol (2015) 102(5):579–86. doi: 10.1007/s12185-015-1865-2
25. Giallongo C, Parrinello N, Tibullo D, La Cava P, Romano A, Chiarenza A, et al. Myeloid derived suppressor cells (Mdscs) are increased and exert immunosuppressive activity together with polymorphonuclear leukocytes (Pmns) in chronic myeloid leukemia patients. PloS One (2014) 9(7):e101848. doi: 10.1371/journal.pone.0101848
26. Christiansson L, Söderlund S, Svensson E, Mustjoki S, Bengtsson M, Simonsson B, et al. Increased level of myeloid-derived suppressor cells, programmed death receptor ligand 1/Programmed death receptor 1, and soluble Cd25 in sokal high risk chronic myeloid leukemia. PloS One (2013) 8(1):e55818. doi: 10.1371/journal.pone.0055818
27. Liu YF, Chen YY, He YY, Wang JY, Yang JP, Zhong SL, et al. Expansion and activation of granulocytic, myeloid-derived suppressor cells in childhood precursor b cell acute lymphoblastic leukemia. J Leukoc Biol (2017) 102(2):449–58. doi: 10.1189/jlb.5MA1116-453RR
28. Salem ML, El-Shanshory MR, Abdou SH, Attia MS, Sobhy SM, Zidan MF, et al. Chemotherapy alters the increased numbers of myeloid-derived suppressor and regulatory T cells in children with acute lymphoblastic leukemia. Immunopharmacol Immunotoxicol (2018) 40(2):158–67. doi: 10.1080/08923973.2018.1424897
29. Liu J, Zhou Y, Huang Q, Qiu L. Cd14(+)Hla-Dr(Low/-) expression: A novel prognostic factor in chronic lymphocytic leukemia. Oncol Lett (2015) 9(3):1167–72. doi: 10.3892/ol.2014.2808
30. Zarobkiewicz M, Kowalska W, Chocholska S, Tomczak W, Szymańska A, Morawska I, et al. High m-mdsc percentage as a negative prognostic factor in chronic lymphocytic leukaemia. Cancers (Basel) (2020) 12(9):1–30. doi: 10.3390/cancers12092614
31. Kowalska W, Bojarska-Junak A. Monocytic mdsc as a source of immunosuppressive cytokines in chronic lymphocytic leukemia (Cll) microenvironment. Folia Histochemica Cytobiologica (2020) 58(1):25–36. doi: 10.5603/FHC.a2020.0006
32. Ferrer G, Jung B, Chiu PY, Aslam R, Palacios F, Mazzarello AN, et al. Myeloid-derived suppressor cell subtypes differentially influence T-cell function, T-helper subset differentiation, and clinical course in cll. Leukemia (2021) 35(11):3163–75. doi: 10.1038/s41375-021-01249-7
33. Azzaoui I, Uhel F, Rossille D, Pangault C, Dulong J, Le Priol J, et al. T-Cell defect in diffuse Large b-cell lymphomas involves expansion of myeloid-derived suppressor cells. Blood (2016) 128(8):1081–92. doi: 10.1182/blood-2015-08-662783
34. Wang Z, Jiang R, Li Q, Wang H, Tao Q, Zhai Z. Elevated m-mdscs in circulation are indicative of poor prognosis in diffuse Large b-cell lymphoma patients. J Clin Med (2021) 10(8):1–11. doi: 10.3390/jcm10081768
35. Romano A, Parrinello NL, Vetro C, Forte S, Chiarenza A, Figuera A, et al. Circulating myeloid-derived suppressor cells correlate with clinical outcome in Hodgkin lymphoma patients treated up-front with a risk-adapted strategy. Br J Haematol (2015) 168(5):689–700. doi: 10.1111/bjh.13198
36. Wang Y, Wang J, Zhu F, Wang H, Yi L, Huang K, et al. Elevated circulating myeloid-derived suppressor cells associated with poor prognosis in b-cell non-hodgkin's lymphoma patients. Immun Inflammation Dis (2022) 10(5):e616. doi: 10.1002/iid3.616
37. Brimnes MK, Vangsted AJ, Knudsen LM, Gimsing P, Gang AO, Johnsen HE, et al. Increased level of both Cd4+Foxp3+ regulatory T cells and Cd14+Hla-Dr⁻/Low myeloid-derived suppressor cells and decreased level of dendritic cells in patients with multiple myeloma. Scand J Immunol (2010) 72(6):540–7. doi: 10.1111/j.1365-3083.2010.02463.x
38. Wang Z, Zhang L, Wang H, Xiong S, Li Y, Tao Q, et al. Tumor-induced Cd14+Hla-Dr–/Low myeloid-derived suppressor cells correlate with tumor progression and outcome of therapy in multiple myeloma patients. Cancer Immunology Immunotherapy (2015) 64(3):389–99. doi: 10.1007/s00262-014-1646-4
39. Bae MH, Park CJ, Suh C. Increased monocytic myeloid-derived suppressor cells in whole blood predict poor prognosis in patients with plasma cell myeloma. J Clin Med (2021) 10(20):1–13. doi: 10.3390/jcm10204717
40. Lee SE, Lim JY, Kim TW, Ryu DB, Park SS, Jeon YW, et al. Different role of circulating myeloid-derived suppressor cells in patients with multiple myeloma undergoing autologous stem cell transplantation. J Immunother Cancer (2019) 7(1):35. doi: 10.1186/s40425-018-0491-y
41. Giallongo C, Tibullo D, Parrinello NL, La Cava P, Di Rosa M, Bramanti V, et al. Granulocyte-like myeloid derived suppressor cells (G-mdsc) are increased in multiple myeloma and are driven by dysfunctional mesenchymal stem cells (Msc). Oncotarget (2016) 7(52):85764–75. doi: 10.18632/oncotarget.7969
42. Bae J, Accardi F, Hideshima T, Tai Y-T, Prabhala R, Shambley A, et al. Targeting Lag3/Gal-3 to overcome immunosuppression and enhance anti-tumor immune responses in multiple myeloma. Leukemia (2022) 36(1):138–54. doi: 10.1038/s41375-021-01301-6
43. Ai L, Mu S, Sun C, Fan F, Yan H, Qin Y, et al. Myeloid-derived suppressor cells endow stem-like qualities to multiple myeloma cells by inducing pirna-823 expression and Dnmt3b activation. Mol Cancer (2019) 18(1):88. doi: 10.1186/s12943-019-1011-5
44. Favaloro J, Liyadipitiya T, Brown R, Yang S, Suen H, Woodland N, et al. Myeloid derived suppressor cells are numerically, functionally and phenotypically different in patients with multiple myeloma. Leuk Lymphoma (2014) 55(12):2893–900. doi: 10.3109/10428194.2014.904511
45. Romano A, Parrinello NL, La Cava P, Tibullo D, Giallongo C, Camiolo G, et al. Pmn-mdsc and arginase are increased in myeloma and may contribute to resistance to therapy. Expert Rev Mol Diagn (2018) 18(7):675–83. doi: 10.1080/14737159.2018.1470929
46. Perez C, Botta C, Zabaleta A, Puig N, Cedena MT, Goicoechea I, et al. Immunogenomic identification and characterization of granulocytic myeloid-derived suppressor cells in multiple myeloma. Blood (2020) 136(2):199–209. doi: 10.1182/blood.2019004537
47. Yu S, Ren X, Li L. Myeloid-derived suppressor cells in hematologic malignancies: Two sides of the same coin. Exp Hematol Oncol (2022) 11(1):43. doi: 10.1186/s40164-022-00296-9
48. Betsch A, Rutgeerts O, Fevery S, Sprangers B, Verhoef G, Dierickx D, et al. Myeloid-derived suppressor cells in lymphoma: The good, the bad and the ugly. Blood Rev (2018) 32(6):490–8. doi: 10.1016/j.blre.2018.04.006
49. Bewersdorf JP, Zeidan AM. Myeloid-derived suppressor cells: A grey eminence in the aml tumor microenvironment? Expert Rev Anticancer Ther (2022) 22(3):239–41. doi: 10.1080/14737140.2022.2030227
50. Palumbo GA, Parrinello NL, Giallongo C, D'Amico E, Zanghì A, Puglisi F, et al. Monocytic myeloid derived suppressor cells in hematological malignancies. Int J Mol Sci (2019) 20(21):1–14. doi: 10.3390/ijms20215459
51. Döhner H, Weisdorf DJ, Bloomfield CD. Acute myeloid leukemia. New Engl J Med (2015) 373(12):1136–52. doi: 10.1056/NEJMra1406184
52. Houshmand M, Simonetti G, Circosta P, Gaidano V, Cignetti A, Martinelli G, et al. Chronic myeloid leukemia stem cells. Leukemia (2019) 33(7):1543–56. doi: 10.1038/s41375-019-0490-0
53. Lv M, Wang K, Huang X-j. Myeloid-derived suppressor cells in hematological malignancies: Friends or foes. J Hematol Oncol (2019) 12(1):105. doi: 10.1186/s13045-019-0797-3
54. Darwiche W, Gubler B, Marolleau JP, Ghamlouch H. Chronic lymphocytic leukemia b-cell normal cellular counterpart: Clues from a functional perspective. Front Immunol (2018) 9:683. doi: 10.3389/fimmu.2018.00683
55. Papafragkos I, Markaki E, Kalpadakis C, Verginis P. Decoding the myeloid-derived suppressor cells in lymphoid malignancies. J Clin Med (2021) 10(16):1–20. doi: 10.3390/jcm10163462
56. van de Donk NWCJ, Pawlyn C, Yong KL. Multiple myeloma. Lancet (2021) 397(10272):410–27. doi: 10.1016/S0140-6736(21)00135-5
57. Leone P, Solimando AG, Malerba E, Fasano R, Buonavoglia A, Pappagallo F, et al. Actors on the scene: Immune cells in the myeloma niche. Front Oncol (2020) 10:599098. doi: 10.3389/fonc.2020.599098
58. Vincent J, Mignot G, Chalmin F, Ladoire S, Bruchard M, Chevriaux A, et al. 5-fluorouracil selectively kills tumor-associated myeloid-derived suppressor cells resulting in enhanced T cell-dependent antitumor immunity. Cancer Res (2010) 70(8):3052–61. doi: 10.1158/0008-5472.Can-09-3690
59. De Veirman K, Van Ginderachter JA, Lub S, De Beule N, Thielemans K, Bautmans I, et al. Multiple myeloma induces mcl-1 expression and survival of myeloid-derived suppressor cells. Oncotarget (2015) 6(12):10532–47. doi: 10.18632/oncotarget.3300
60. Zhu XJ, Yang ZF, Zhou JY, Liu L, Sun XM, Fan ZF, et al. Progression of Large lymphoma is significantly impeded with a combination of gemcitabine chemotherapy and dendritic cells intra-tumor vaccination. PloS One (2015) 10(7):e0132799. doi: 10.1371/journal.pone.0132799
61. Sasso MS, Lollo G, Pitorre M, Solito S, Pinton L, Valpione S, et al. Low dose gemcitabine-loaded lipid nanocapsules target monocytic myeloid-derived suppressor cells and potentiate cancer immunotherapy. Biomaterials (2016) 96:47–62. doi: 10.1016/j.biomaterials.2016.04.010
62. Krejcik J, Casneuf T, Nijhof IS, Verbist B, Bald J, Plesner T, et al. Daratumumab depletes Cd38+ immune regulatory cells, promotes T-cell expansion, and skews T-cell repertoire in multiple myeloma. Blood (2016) 128(3):384–94. doi: 10.1182/blood-2015-12-687749
63. Cohen YC, Oriol A, Wu KL, Lavi N, Vlummens P, Jackson C, et al. Daratumumab with cetrelimab, an anti-Pd-1 monoclonal antibody, in Relapsed/Refractory multiple myeloma. Clin Lymphoma Myeloma Leuk (2021) 21(1):46–54.e4. doi: 10.1016/j.clml.2020.08.008
64. Qin H, Lerman B, Sakamaki I, Wei G, Cha SC, Rao SS, et al. Generation of a new therapeutic peptide that depletes myeloid-derived suppressor cells in tumor-bearing mice. Nat Med (2014) 20(6):676–81. doi: 10.1038/nm.3560
65. Romano A, Parrinello NL, Chiarenza A, Motta G, Tibullo D, Giallongo C, et al. Immune off-target effects of brentuximab vedotin in Relapsed/Refractory Hodgkin lymphoma. Br J Haematol (2019) 185(3):468–79. doi: 10.1111/bjh.15801
66. Zhou J, Yao Y, Shen Q, Li G, Hu L, Zhang X. Demethylating agent decitabine disrupts tumor-induced immune tolerance by depleting myeloid-derived suppressor cells. J Cancer Res Clin Oncol (2017) 143(8):1371–80. doi: 10.1007/s00432-017-2394-6
67. Zhou J, Shen Q, Lin H, Hu L, Li G, Zhang X. Decitabine shows potent anti-myeloma activity by depleting monocytic myeloid-derived suppressor cells in the myeloma microenvironment. J Cancer Res Clin Oncol (2019) 145(2):329–36. doi: 10.1007/s00432-018-2790-6
68. Bae J, Hideshima T, Tai YT, Song Y, Richardson P, Raje N, et al. Histone deacetylase (Hdac) inhibitor Acy241 enhances anti-tumor activities of antigen-specific central memory cytotoxic T lymphocytes against multiple myeloma and solid tumors. Leukemia (2018) 32(9):1932–47. doi: 10.1038/s41375-018-0062-8
69. Jitschin R, Saul D, Braun M, Tohumeken S, Völkl S, Kischel R, et al. Cd33/Cd3-bispecific T-cell engaging (Bite®) antibody construct targets monocytic aml myeloid-derived suppressor cells. J Immunother Cancer (2018) 6(1):116. doi: 10.1186/s40425-018-0432-9
70. Cheng P, Chen X, Dalton R, Calescibetta A, So T, Gilvary D, et al. Immunodepletion of mdsc by Amv564, a novel bivalent, bispecific Cd33/Cd3 T cell engager, ex vivo in mds and melanoma. Mol Ther (2022) 30(6):2315–26. doi: 10.1016/j.ymthe.2022.02.005
71. Tavazoie MF, Pollack I, Tanqueco R, Ostendorf BN, Reis BS, Gonsalves FC, et al. Lxr/Apoe activation restricts innate immune suppression in cancer. Cell (2018) 172(4):825–40.e18. doi: 10.1016/j.cell.2017.12.026
72. Kuwahara-Ota S, Shimura Y, Steinebach C, Isa R, Yamaguchi J, Nishiyama D, et al. Lenalidomide and pomalidomide potently interfere with induction of myeloid-derived suppressor cells in multiple myeloma. Br J Haematol (2020) 191(5):784–95. doi: 10.1111/bjh.16881
73. Wang L, Jia B, Claxton DF, Ehmann WC, Rybka WB, Mineishi S, et al. Vista is highly expressed on mdscs and mediates an inhibition of T cell response in patients with aml. Oncoimmunology (2018) 7(9):e1469594. doi: 10.1080/2162402x.2018.1469594
74. Kim TK, Han X, Wang J, Sanmamed M, Zhang T, Halene S, et al. Pd-1h (Vista) induces immune evasion in acute myeloid leukemia. Blood (2017) 130(Supplement 1):2658. doi: 10.1182/blood.V130.Suppl_1.2658.2658
75. Chang YC, Chiang YH, Hsu K, Chuang CK, Kao CW, Chang YF, et al. Activated naïve Γδ T cells accelerate deep molecular response to bcr-abl inhibitors in patients with chronic myeloid leukemia. Blood Cancer J (2021) 11(11):182. doi: 10.1038/s41408-021-00572-7
76. Giallongo C, Parrinello NL, La Cava P, Camiolo G, Romano A, Scalia M, et al. Monocytic myeloid-derived suppressor cells as prognostic factor in chronic myeloid leukaemia patients treated with dasatinib. J Cell Mol Med (2018) 22(2):1070–80. doi: 10.1111/jcmm.13326
77. Li C, You X, Xu X, Wu B, Liu Y, Tong T, et al. A metabolic reprogramming amino acid polymer as an immunosurveillance activator and leukemia targeting drug carrier for T-cell acute lymphoblastic leukemia. Adv Sci (Weinh) (2022) 9(9):e2104134. doi: 10.1002/advs.202104134
78. Gibb DR, Saleem SJ, Kang DJ, Subler MA, Conrad DH. Adam10 overexpression shifts lympho- and myelopoiesis by dysregulating site 2/Site 3 cleavage products of notch. J Immunol (2011) 186(7):4244–52. doi: 10.4049/jimmunol.1003318
79. Grazioli P, Orlando A, Giordano N, Noce C, Peruzzi G, Abdollahzadeh B, et al. Notch-signaling deregulation induces myeloid-derived suppressor cells in T-cell acute lymphoblastic leukemia. Front Immunol (2022) 13:809261. doi: 10.3389/fimmu.2022.809261
80. Sierra RA, Trillo-Tinoco J, Mohamed E, Yu L, Achyut BR, Arbab A, et al. Anti-jagged immunotherapy inhibits mdscs and overcomes tumor-induced tolerance. Cancer Res (2017) 77(20):5628–38. doi: 10.1158/0008-5472.Can-17-0357
81. De Veirman K, De Beule N, Maes K, Menu E, De Bruyne E, De Raeve H, et al. Extracellular S100a9 protein in bone marrow supports multiple myeloma survival by stimulating angiogenesis and cytokine secretion. Cancer Immunol Res (2017) 5(10):839–46. doi: 10.1158/2326-6066.Cir-17-0192
82. Fan R, Satilmis H, Vandewalle N, De Bruyne E, Menu E, Chantry AD, et al. Tasquinimod targets immunosuppressive myeloid cells, increases osteogenesis and has direct anti-myeloma effects by inhibiting c-myc expression in vitro and in vivo. Blood (2021) 138:1594. doi: 10.1182/blood-2021-147744
83. Hong D, Kurzrock R, Kim Y, Woessner R, Younes A, Nemunaitis J, et al. Azd9150, a next-generation antisense oligonucleotide inhibitor of Stat3 with early evidence of clinical activity in lymphoma and lung cancer. Sci Transl Med (2015) 7(314):314ra185. doi: 10.1126/scitranslmed.aac5272
84. Serafini P, Mgebroff S, Noonan K, Borrello I. Myeloid-derived suppressor cells promote cross-tolerance in b-cell lymphoma by expanding regulatory T cells. Cancer Res (2008) 68(13):5439–49. doi: 10.1158/0008-5472.Can-07-6621
85. Noonan KA, Ghosh N, Rudraraju L, Bui M, Borrello I. Targeting immune suppression with Pde5 inhibition in end-stage multiple myeloma. Cancer Immunol Res (2014) 2(8):725–31. doi: 10.1158/2326-6066.Cir-13-0213
86. Ghosh N, Rudraraju L, Ye X, Noonan K, Huff CA, Borrello IM. Administration of an oral Pde5 inhibitor, tadalafil in conjunction with a lenalidomide containing regimen in patients with multiple myeloma. Blood (2013) 122(21):1959–. doi: 10.1182/blood.V122.21.1959.1959
87. Grauers Wiktorin H, Nilsson MS, Kiffin R, Sander FE, Lenox B, Rydström A, et al. Histamine targets myeloid-derived suppressor cells and improves the anti-tumor efficacy of pd-1/Pd-L1 checkpoint blockade. Cancer Immunol Immunother (2019) 68(2):163–74. doi: 10.1007/s00262-018-2253-6
88. Papadopoulos KP, Tsai FY-C, Bauer TM, Muigai L, Liang Y, Bennett MK, et al. Cx-1158-101: A first-in-Human phase 1 study of cb-1158, a small molecule inhibitor of arginase, as monotherapy and in combination with an anti-Pd-1 checkpoint inhibitor in patients (Pts) with solid tumors. J Clin Oncol (2017) 35(15_suppl):3005. doi: 10.1200/JCO.2017.35.15_suppl.3005
89. Mussai F, Wheat R, Sarrou E, Booth S, Stavrou V, Fultang L, et al. Targeting the arginine metabolic brake enhances immunotherapy for leukaemia. Int J Cancer (2019) 145(8):2201–8. doi: 10.1002/ijc.32028
90. Nefedova Y, Fishman M, Sherman S, Wang X, Beg AA, Gabrilovich DI. Mechanism of all-trans retinoic acid effect on tumor-associated myeloid-derived suppressor cells. Cancer Res (2007) 67(22):11021–8. doi: 10.1158/0008-5472.Can-07-2593
91. Trabanelli S, Chevalier MF, Martinez-Usatorre A, Gomez-Cadena A, Salomé B, Lecciso M, et al. Tumour-derived Pgd2 and Nkp30-B7h6 engagement drives an immunosuppressive Ilc2-mdsc axis. Nat Commun (2017) 8(1):593. doi: 10.1038/s41467-017-00678-2
92. Tohumeken S, Baur R, Böttcher M, Stoll A, Loschinski R, Panagiotidis K, et al. Palmitoylated proteins on aml-derived extracellular vesicles promote myeloid-derived suppressor cell differentiation Via Tlr2/Akt/Mtor signaling. Cancer Res (2020) 80(17):3663–76. doi: 10.1158/0008-5472.Can-20-0024
93. Casneuf T, Adams HC 3rd, van de Donk N, Abraham Y, Bald J, Vanhoof G, et al. Deep immune profiling of patients treated with lenalidomide and dexamethasone with or without daratumumab. Leukemia (2021) 35(2):573–84. doi: 10.1038/s41375-020-0855-4
94. De Cicco P, Ercolano G, Ianaro A. The new era of cancer immunotherapy: Targeting myeloid-derived suppressor cells to overcome immune evasion. Front Immunol (2020) 11:1680. doi: 10.3389/fimmu.2020.01680
95. Angelopoulou MK, Vassilakopoulos TP, Batsis I, Sakellari I, Gkirkas K, Pappa V, et al. Brentuximab vedotin in Relapsed/Refractory Hodgkin lymphoma. Hellenic Experience. Hematol Oncol (2018) 36(1):174–81. doi: 10.1002/hon.2383
96. Diesch J, Zwick A, Garz AK, Palau A, Buschbeck M, Götze KS. A clinical-molecular update on azanucleoside-based therapy for the treatment of hematologic cancers. Clin Epigenet (2016) 8:71. doi: 10.1186/s13148-016-0237-y
97. Cui Y, Cai J, Wang W, Wang S. Regulatory effects of histone deacetylase inhibitors on myeloid-derived suppressor cells. Front Immunol (2021) 12:690207. doi: 10.3389/fimmu.2021.690207
98. Laszlo GS, Gudgeon CJ, Harrington KH, Dell'Aringa J, Newhall KJ, Means GD, et al. Cellular determinants for preclinical activity of a novel Cd33/Cd3 bispecific T-cell engager (Bite) antibody, amg 330, against human aml. Blood (2014) 123(4):554–61. doi: 10.1182/blood-2013-09-527044
99. Chang X, Zhu Y, Shi C, Stewart AK. Mechanism of immunomodulatory drugs' action in the treatment of multiple myeloma. Acta Biochim Biophys Sin (Shanghai) (2014) 46(3):240–53. doi: 10.1093/abbs/gmt142
100. Görgün GT, Whitehill G, Anderson JL, Hideshima T, Maguire C, Laubach J, et al. Tumor-promoting immune-suppressive myeloid-derived suppressor cells in the multiple myeloma microenvironment in humans. Blood (2013) 121(15):2975–87. doi: 10.1182/blood-2012-08-448548
101. Ballbach M, Dannert A, Singh A, Siegmund DM, Handgretinger R, Piali L, et al. Expression of checkpoint molecules on myeloid-derived suppressor cells. Immunol Lett (2017) 192:1–6. doi: 10.1016/j.imlet.2017.10.001
102. Lu C, Redd PS, Lee JR, Savage N, Liu K. The expression profiles and regulation of pd-L1 in tumor-induced myeloid-derived suppressor cells. Oncoimmunology (2016) 5(12):e1247135. doi: 10.1080/2162402x.2016.1247135
103. Noman MZ, Desantis G, Janji B, Hasmim M, Karray S, Dessen P, et al. Pd-L1 is a novel direct target of hif-1α, and its blockade under hypoxia enhanced mdsc-mediated T cell activation. J Exp Med (2014) 211(5):781–90. doi: 10.1084/jem.20131916
104. Ahn JH, Lee BH, Kim SE, Kwon BE, Jeong H, Choi JR, et al. A novel anti-Pd-L1 antibody exhibits antitumor effects on multiple myeloma in murine models Via antibody-dependent cellular cytotoxicity. Biomol Ther (Seoul) (2021) 29(2):166–74. doi: 10.4062/biomolther.2020.131
105. Hosseinkhani N, Derakhshani A, Shadbad MA, Argentiero A, Racanelli V, Kazemi T, et al. The role of V-domain ig suppressor of T cell activation (Vista) in cancer therapy: Lessons learned and the road ahead. Front Immunol (2021) 12:676181. doi: 10.3389/fimmu.2021.676181
106. Tagliamento M, Agostinetto E, Borea R, Brandão M, Poggio F, Addeo A, et al. Vista: A promising target for cancer immunotherapy? Immunotargets Ther (2021) 10:185–200. doi: 10.2147/itt.S260429
107. Stiff A, Trikha P, Wesolowski R, Kendra K, Hsu V, Uppati S, et al. Myeloid-derived suppressor cells express bruton's tyrosine kinase and can be depleted in tumor-bearing hosts by ibrutinib treatment. Cancer Res (2016) 76(8):2125–36. doi: 10.1158/0008-5472.Can-15-1490
108. Varikuti S, Singh B, Volpedo G, Ahirwar DK, Jha BK, Saljoughian N, et al. Ibrutinib treatment inhibits breast cancer progression and metastasis by inducing conversion of myeloid-derived suppressor cells to dendritic cells. Br J Cancer (2020) 122(7):1005–13. doi: 10.1038/s41416-020-0743-8
109. Grazioli P, Felli MP, Screpanti I, Campese AF. The mazy case of notch and immunoregulatory cells. J Leukoc Biol (2017) 102(2):361–8. doi: 10.1189/jlb.1VMR1216-505R
110. Cheng P, Kumar V, Liu H, Youn JI, Fishman M, Sherman S, et al. Effects of notch signaling on regulation of myeloid cell differentiation in cancer. Cancer Res (2014) 74(1):141–52. doi: 10.1158/0008-5472.Can-13-1686
111. Saleem SJ, Conrad DH. Hematopoietic cytokine-induced transcriptional regulation and notch signaling as modulators of mdsc expansion. Int Immunopharmacol (2011) 11(7):808–15. doi: 10.1016/j.intimp.2011.03.010
112. Moore G, Annett S, McClements L, Robson T. Top notch targeting strategies in cancer: A detailed overview of recent insights and current perspectives. Cells (2020) 9(6):1–46. doi: 10.3390/cells9061503
113. Wang S-H, Lu Q-Y, Guo Y-H, Song Y-Y, Liu P-J, Wang Y-C. The blockage of notch signalling promoted the generation of polymorphonuclear myeloid-derived suppressor cells with lower immunosuppression. Eur J Cancer (2016) 68:90–105. doi: 10.1016/j.ejca.2016.08.019
114. Cheng P, Corzo CA, Luetteke N, Yu B, Nagaraj S, Bui MM, et al. Inhibition of dendritic cell differentiation and accumulation of myeloid-derived suppressor cells in cancer is regulated by S100a9 protein. J Exp Med (2008) 205(10):2235–49. doi: 10.1084/jem.20080132
115. Condamine T, Gabrilovich DI. Molecular mechanisms regulating myeloid-derived suppressor cell differentiation and function. Trends Immunol (2011) 32(1):19–25. doi: 10.1016/j.it.2010.10.002
116. Dufait I, Van Valckenborgh E, Menu E, Escors D, De Ridder M, Breckpot K. Signal transducer and activator of transcription 3 in myeloid-derived suppressor cells: An opportunity for cancer therapy. Oncotarget (2016) 7(27):42698–715. doi: 10.18632/oncotarget.8311
117. Maenhout SK, Du Four S, Corthals J, Neyns B, Thielemans K, Aerts JL. Azd1480 delays tumor growth in a melanoma model while enhancing the suppressive activity of myeloid-derived suppressor cells. Oncotarget (2014) 5(16):6801–15. doi: 10.18632/oncotarget.2254
118. Reilley MJ, McCoon P, Cook C, Lyne P, Kurzrock R, Kim Y, et al. Stat3 antisense oligonucleotide Azd9150 in a subset of patients with heavily pretreated lymphoma: Results of a phase 1b trial. J Immunother Cancer (2018) 6(1):119. doi: 10.1186/s40425-018-0436-5
119. Bitsch R, Kurzay A, Özbay Kurt F, de la Torre C, Lasser S, Lepper A, et al. Stat3 inhibitor napabucasin abrogates mdsc immunosuppressive capacity and prolongs survival of melanoma-bearing mice. J Immunother Cancer (2022) 10(3):1–12. doi: 10.1136/jitc-2021-004384
120. Li X, Wei Y, Wei X. Napabucasin, a novel inhibitor of Stat3, inhibits growth and synergises with doxorubicin in diffuse Large b-cell lymphoma. Cancer Lett (2020) 491:146–61. doi: 10.1016/j.canlet.2020.07.032
121. Serafini P, Meckel K, Kelso M, Noonan K, Califano J, Koch W, et al. Phosphodiesterase-5 inhibition augments endogenous antitumor immunity by reducing myeloid-derived suppressor cell function. J Exp Med (2006) 203(12):2691–702. doi: 10.1084/jem.20061104
122. Mellqvist UH, Hansson M, Brune M, Dahlgren C, Hermodsson S, Hellstrand K. Natural killer cell dysfunction and apoptosis induced by chronic myelogenous leukemia cells: Role of reactive oxygen species and regulation by histamine. Blood (2000) 96(5):1961–8. doi: 10.1182/blood.V96.5.1961
123. Zheng A, Xie F, Shi S, Liu S, Long J, Xu Y. Sustained drug release from liposomes for the remodeling of systemic immune homeostasis and the tumor microenvironment. Front Immunol (2022) 13:829391. doi: 10.3389/fimmu.2022.829391
124. Pozzi S, Raje N. The role of bisphosphonates in multiple myeloma: Mechanisms, side effects, and the future. Oncologist (2011) 16(5):651–62. doi: 10.1634/theoncologist.2010-0225
125. Porembka MR, Mitchem JB, Belt BA, Hsieh CS, Lee HM, Herndon J, et al. Pancreatic adenocarcinoma induces bone marrow mobilization of myeloid-derived suppressor cells which promote primary tumor growth. Cancer Immunol Immunother (2012) 61(9):1373–85. doi: 10.1007/s00262-011-1178-0
126. Nandre R, Verma V, Gaur P, Patil V, Yang X, Ramlaoui Z, et al. Ido vaccine ablates immune-suppressive myeloid populations and enhances antitumor effects independent of tumor cell ido status. Cancer Immunol Res (2022) 10(5):571–80. doi: 10.1158/2326-6066.Cir-21-0457
127. Fultang L, Panetti S, Ng M, Collins P, Graef S, Rizkalla N, et al. Mdsc targeting with gemtuzumab ozogamicin restores T cell immunity and immunotherapy against cancers. EBioMedicine (2019) 47:235–46. doi: 10.1016/j.ebiom.2019.08.025
128. Jain MD, Zhao H, Wang X, Atkins R, Menges M, Reid K, et al. Tumor interferon signaling and suppressive myeloid cells are associated with car T-cell failure in Large b-cell lymphoma. Blood (2021) 137(19):2621–33. doi: 10.1182/blood.2020007445
Keywords: hematological cancers, myeloid-derived suppressor cells, immunotherapies, multiple myeloma, leukemia, lymphoma
Citation: Fan R, De Beule N, Maes A, De Bruyne E, Menu E, Vanderkerken K, Maes K, Breckpot K and De Veirman K (2022) The prognostic value and therapeutic targeting of myeloid-derived suppressor cells in hematological cancers. Front. Immunol. 13:1016059. doi: 10.3389/fimmu.2022.1016059
Received: 10 August 2022; Accepted: 16 September 2022;
Published: 11 October 2022.
Edited by:
Nicola Giuliani, University of Parma, ItalyReviewed by:
Francesco Di Raimondo, University of Catania, ItalyCopyright © 2022 Fan, De Beule, Maes, De Bruyne, Menu, Vanderkerken, Maes, Breckpot and De Veirman. This is an open-access article distributed under the terms of the Creative Commons Attribution License (CC BY). The use, distribution or reproduction in other forums is permitted, provided the original author(s) and the copyright owner(s) are credited and that the original publication in this journal is cited, in accordance with accepted academic practice. No use, distribution or reproduction is permitted which does not comply with these terms.
*Correspondence: Kim De Veirman, S2ltLkRlLlZlaXJtYW5AdnViLmJl
Disclaimer: All claims expressed in this article are solely those of the authors and do not necessarily represent those of their affiliated organizations, or those of the publisher, the editors and the reviewers. Any product that may be evaluated in this article or claim that may be made by its manufacturer is not guaranteed or endorsed by the publisher.
Research integrity at Frontiers
Learn more about the work of our research integrity team to safeguard the quality of each article we publish.