- 1ZIK plasmatis, Leibniz Institute for Plasma Science and Technology (INP), Greifswald, Germany
- 2Department of General, Thoracic, Vascular, and Visceral Surgery, Greifswald University Medical Center, Greifswald, Germany
- 3Department of Oral and Maxillofacial Surgery, Plastic Surgery, Greifswald University Medical Center, Greifswald, Germany
Considering cancer not only as malignant cells on their own but as a complex disease in which tumor cells interact and communicate with their microenvironment has motivated the establishment of clinically relevant 3D models in past years. Technological advances gave rise to novel bioengineered models, improved organoid systems, and microfabrication approaches, increasing scientific importance in preclinical research. Notwithstanding, mammalian in vivo models remain closest to mimic the patient’s situation but are limited by cost, time, and ethical constraints. Herein, the in ovo model bridges the gap as an advanced model for basic and translational cancer research without the need for ethical approval. With the avian embryo being a naturally immunodeficient host, tumor cells and primary tissues can be engrafted on the vascularized chorioallantoic membrane (CAM) with high efficiencies regardless of species-specific restrictions. The extraembryonic membranes are connected to the embryo through a continuous circulatory system, readily accessible for manipulation or longitudinal monitoring of tumor growth, metastasis, angiogenesis, and matrix remodeling. However, its applicability in immunoncological research is largely underexplored. Dual engrafting of malignant and immune cells could provide a platform to study tumor-immune cell interactions in a complex, heterogenic and dynamic microenvironment with high reproducibility. With some caveats to keep in mind, versatile methods for in and ex ovo monitoring of cellular and molecular dynamics already established in ovo are applicable alike. In this view, the present review aims to emphasize and discuss opportunities and limitations of the chicken embryo model for pre-clinical research in cancer and cancer immunology.
Introduction
As early as 1911, Rous and Murphy were the first to transplant primary tumor tissue on the highly vascularized chicken embryo CAM, demonstrating the rapid growth of Rous sarcomas early after engrafting. Starting from those findings, the in ovo model gained emerging interest as an alternative for costly, time-consuming mammalian in vivo models in pre-clinical oncological research (1). For centuries a drawback in rodent models, tumor cells and tissues can be engrafted at high efficiencies without species-specific restrictions due to the embryo’s natural immunodeficiency (2, 3). By that oncogenesis can be studied in a humanized system with widespread adoption. Developing tumors perform neo-vascularization and matrix deposition, mimicking the complex tumor microenvironment largely limited in conventional in vitro models (4). The extraembryonic membranes are connected to the embryo through a continuous circulatory system, readily accessible for manipulation and versatile visualization techniques to study tumor growth, metastasis and angiogenesis in and ex ovo, deep mechanistic insights at cellular and molecular levels can be achieved after tumor excision. Ethical approval is omitted if experiments are terminated at embryonic day 14 in most countries, facilitating screenings of pharmacological or physics-based therapies with high reproducibility at large scales supporting the 3Rs principle (5).
In spite of a valuable history in pre-clinical oncology, the applicability of the tumor chorioallantoic membrane (TUM-CAM) model in immunoncological research is largely underexplored. Only few studies have taken advantage of a naturally immunodeficient host to study tumor-immune interactions without species-specific restrictions in ovo. Rodent models have yielded fundamental insights into key aspects of the human immune system, including its dual role in elimination and guarding of malignant cells. As such, the discovery that culture supernatants of activated T cells can boost the reactivity of previously generated cytotoxic T lymphocytes gave rise to the first, IL-2 based, immunotherapy already 30 years ago (6–8) and new waves of excitement are dedicated to recent advances in antibody and cell based therapy approaches. On the other hand, it is undisputable that cancer cells are excellent maskers, hijacking and coopting immune and stromal cells residing in their microenvironment to aid in tumor progression and metastasis. Tumor-immune cell interactions evolve in a highly dynamic, heterogenic environment, and mammalian models remain state-of-the-art reflecting the patient’s situation. However, establishment of clinically relevant, cost and time efficient 3D models for basic and translational immunooncological research at larger scales is urgently needed. The TUM-CAM model is ideal to bridge this gap.
The present review aims to summarize, highlight and emphasize opportunities and limitations of the chicken embryo model for its use in pre-clinical (immuno-)oncological research. Moreover, its potential for tailored treatments based on patient-derived xenografts (PDX) in the context of personalized medicine is discussed.
The in ovo model in cancer research
The in ovo model has served as an alternative for mammalian tumor models to investigate characteristics of tumor growth, metastasis, and efficacy of cancer therapies in preclinical oncological research for more than a century. Grafting of tumor cell suspensions but also murine and human primary tissues has been successfully applied to address a broad range of scientific questions with diverse methodological read-outs (Table 1).
General experimental procedure and ethical guidelines
Several techniques have been established to engraft tumor cells and tissues on the vascularized CAM, with main differences between in ovo and shell-less ex ovo approaches. The latter offers better accessibility of the CAM but increases drop-out rates due to frequent rupture of the yolk membrane and contamination, requiring high levels of experience (36, 37). With respect to its prevalent use in cancer research, the present review provides a step-by-step protocol of the general experimental procedure in ovo.
Specific pathogen-free (SPF) fertilized chicken eggs are placed in a specialized breeding incubator equipped with a turning unit in horizontal position. Commercial hatcheries turn eggs once every hour but should be at least 3 times a day to prevent embryo adhesion to the shell. Breeding conditions should be at 37°C and 65% humidity throughout the experimental period. After six days of incubation, the pointed pole of the eggs can be punctured using a 20 G cannula to create an air hole for subsequent tumor inoculation. The hole is covered with an air permeable plaster, and eggs are placed back in the static unit of the incubator, now in vertical position. Prior puncturing, fertilization, and viability can be easily verified by candling the egg using a bright flashlight. Unfertilized, dead, or contaminated eggs should be removed at any time throughout the experimental period to avoid cross-contaminations. Sterile conditions are not necessarily needed for any intervention but might help to preserve high viability during long-term incubation due to reduced infection risks. On day 7, the egg shell is windowed at the punctured side with a diameter of approximately 2 cm. Caution should be drawn to the highly vascularized CAM. A larger hole can facilitate tumor inoculation and, e.g., in vivo monitoring of tumor growth, but raises the risk of damaging the CAM and reducing viability rates due to severe bleeding and contaminations. Next, a filter paper of the size of approximately 1 cm x 1 cm is soaked in diethylether and placed shortly (≤ 1s) central on the visible CAM as local vessel arrosion has been shown to facilitate tumor formation (38, 39). Immediately after, tumor cells can be inoculated in a silicon ring (inner diameter ≥ 5 mm) placed on the CAM. Engrafting of 1-2 mio. cells per egg is commonly reported in the literature, but a titration to optimize numbers specifically for different cell lines is recommended (Table 2). Solid tumor formation of cell suspensions is improved if cells are resuspended in a hydrogel mixture. A pre-manufactured gel is easy to handle while minimizing basal toxicity, but self-made, e.g., collagen mixtures are feasible alike (9). Again, the hole is covered with a transparent, air-permeable foil, and eggs are incubated for a minimum of three days to allow solid tumor formation prior to treatment on day 11. The tumor-chorioallantoic membrane (TUM-CAM) model has been investigated as a benchmark model for an array of oncological therapies, including various chemotherapeutics (44, 45), targeted (23) and checkpoint therapies (46), oncolytic viruses (30), radiotherapy (47), photodynamic therapy (48), and medical gas plasmas (49). Administration of single and several therapy cycles paralleled by continuous monitoring of tumor growth (view chapter 2.2) is feasible. By that, the TUM-CAM model is a versatile but likewise relatively simple, low-cost model that allows screening of pharmacological or physics-based therapies in a short time. Embryos are sacrificed on day 14, and excised tumors and additional tissue samples can be analyzed in various versatile downstream assays (Figure 1).
As the chicken embryo is not considered as living animal until day 17 of ontogenesis, the TUM-CAM model does not require administrative procedures for obtaining ethical approval obligatory for common animal models. Experiments are terminated before development of regions in the central nervous system that are associated with experience of pain. The National Institute of Health (50), as well as the Institutional Animal Care and Use Committee (IACUC) (51), established that chick embryos lack pain perception before reaching day 14 of gestation and can therefore be used without any ethical restriction.
Monitoring of tumor growth, invasion, and metastasis
Tumor growth as the primary objective in screening studies or mechanistic knock-out models has been assessed by endpoint analysis by means of size, volume, or tumor weight measurements after excision at the simplest. As a major limitation, repeated tumor monitoring is unfeasible in this case. More versatile methods applicable also for longitudinal monitoring include two- or three-dimensional imaging or the evaluation of surrogate parameters based on bioluminescence. The latter requires grafting of genetically engineered cell lines equipped with luciferase reporter genes (17). Upon administration of the respective substrate luciferin, luciferase-expressing tumor cells emit light, allowing to evaluate the tumor growth as a linear function of light emission detected with appropriate optical detection systems. Due to excellent light penetration and biodistribution of the substrate, bioluminescent imaging could also serve to investigate distant metastasis in chick embryo organs but might be limited to shell-less ex ovo approaches. Along similar lines, fluorescent-tagged tumor cells have been engrafted (14, 52). Three-dimensional visualization of tumor development has been achieved using advanced imaging techniques such as micro-computed tomography (CT) (53) and magnetic resonance imaging (MRI) (20). However, time expenditure, high costs, and technical demand are obvious limitations. In addition, the high density of the egg shell causes radio-opacity with obligate need for contrast agent administration (54). High levels of experience are needed for intravascular injections in the small CAM vessels due to a high risk of excessive bleeding, causing increased drop-out rates. Several research groups have focused on noninvasive, three-dimensional imaging such as repeated ultrasonography (24). If accessible, this imaging technology has low operating costs and is time-efficient without need for application of additional imaging agents (Figure 2).
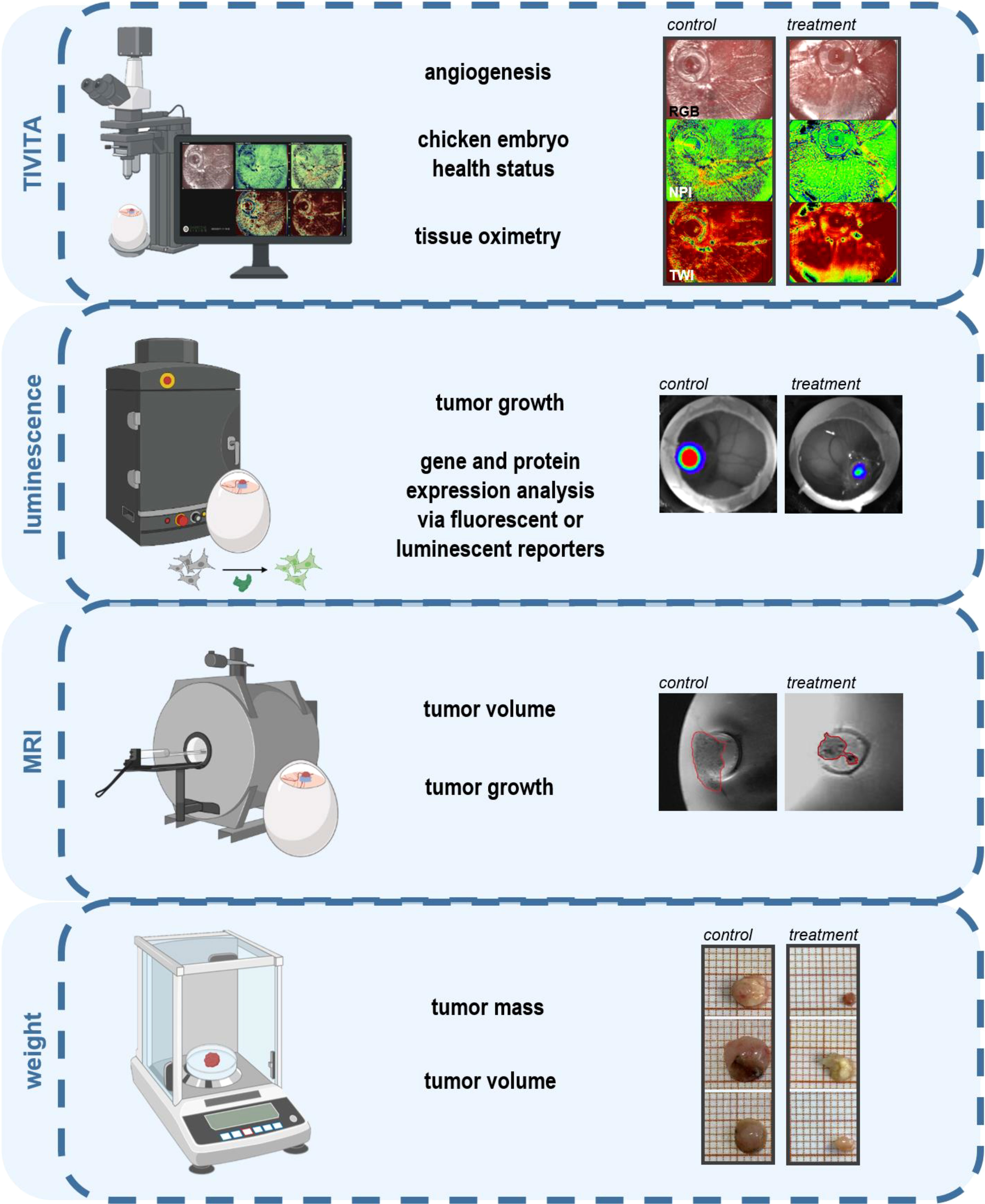
Figure 2 Intravital monitoring of angiogenesis, tumor growth, and assessment of tumor weight (11, 13).
As the extraembryonic membranes are connected with the avian embryo through a continuous circulatory system readily accessible for manipulation, the in ovo model represents a comprehensive experimental system that recapitulates all steps in the metastatic cascade. While available 3D in vitro models mimic only poorly the structure of blood vessels, particularly small vessels such as capillaries and post-capillary venules, where cancer invasion is believed to take place, rodent models are often limited by the scarcity of metastasizing tumors. The highly vascularized nature of the CAM provides by contrast a platform to study both experimental and spontaneous metastasis. Cancer cell invasion, extravasation, and metastatic colonization have been investigated in ovo by morphological assessment (55), selective outgrowth of individual metastasized cells, and detection of microscopic tumor colonies (56). Moreover, biomarkers including human urokinase plasminogen activator (uPA) activity (57) and others related to epithelial-mesenchymal transition (EMT) have been evaluated using various methods, including enzyme-linked immunosorbent assay (ELISA), tissue staining, fluorescence-activated cell sorting (FACS) and polymerase chain reaction (PCR) (26). Cancer cell metastasis based on tumor cell grafting on the CAM has provided valuable information regarding the complex series of events involved in the metastatic cascade. After penetration of the chorionic epithelium, invasion of the mesenchyme below, and blood vessels (58), tumor cells have been shown to survive in the circulation (59), arrest in the vasculature, and proliferate in distinct organs after extravasation (60, 61). As a major drawback in standard mouse models of experimental metastasis, the majority of injected tumor cells perishes in the microcirculation before extravasation can be observed. In the chicken CAM metastasis model, 80% of tumor cells injected in the allantoic vein have been shown to survive and extravasate after 1-3 days following migration to the vicinity of preexisting vessels (62). Longitudinal monitoring has been achieved by establishing an intravital microscopy platform for high-resolution time-lapse imagery of human tumor growth, cell migration, and extravasation in ovo. After luminal labeling of avian endothelial cells via intravenous injection of fluorescent Lens culinaris agglutinin and visualization of blood flow using fluorescent dextran, fluorescent human epidermoid carcinoma Hep3-GFP cells were injected into the vitelline vein to visualize tumor cell extravasation and the role of invadopodia in metastatic processes over a 24 h period (60). Spontaneous metastasis after tumor cell grafting of the CAM has been investigated, e.g., by injection of labeled cells that can be traced in organs, blood, and amnion in and ex ovo (63). In a study investigating two isogenic fibrosarcoma cell lines with differing intravasation capacities, HT-1080-hi/diss, and HT-1080–lo/diss, topically applied highly disseminating cells appeared localized under the CAM ectoderm already 24 h after inoculation (64). Circulating cancer cells have been detected using fiber optic array scanning technology (FAST). Therefore, peripheral blood was distributed on glass slides and immunostained with anti-human CD44 mAb 29-7. Invasion of tumor cells in the circulatory system was first detected 3 days after tumor cell grafting with the highest frequency observed on day 4 (65), which coincides with the onset of intravasation determined in the distal CAM by qPCR (64). Given proof of metastatic colonization in distinct organs is partially limited by the low amount of tumor cells that needs to be detected. Most tumor cells are not able to produce macroscopic visible colonies within the short experimental time. However, as the human genome is uniquely enriched in Alu sequences (frequency 5%), metastasized human tumor cells can be detected and quantified by sensitive Alu PCR assays (66, 67) which enables detection quantification of a minimum of 50 cells (61).
Tumor angiogenesis and vascular remodeling
Expanding tumors hijack physiological angiogenesis to deliver adequate oxygen and nutrient supply, enable waste disposal, and facilitate dissemination of cancer cells to distant sides. During the avascular phase early in tumor progression, the tumor size is largely limited to 1-2 mm. Nutrient deprivation can trigger an ‘‘angiogenic switch’’, resulting in vascular branching and proliferation of endothelial cells, enabling the tumor to grow beyond a restricted size by ensuring sustained energy supply (68). Deregulation of angiogenesis as a hallmark of cancer (69) plays a major role in disease progression, and inhibition of tumor angiogenesis was introduced as a therapeutic strategy more than 50 years ago (70). In 2003, a clinical trial demonstrated prolonged survival of colorectal cancer patients in combination regimes with humanized neutralizing antibodies (bevacizumab, approved by FDA 2005) targeting vascular endothelial growth factor (VEGF), providing proof-of-concept of the successful use of anti-angiogenic therapies in oncology. Likewise, several tyrosine kinase inhibitors designed to target pro-angiogenetic signaling (e.g., sunitinib, approved by FDA 2006) are applied in the treatment of gastrointestinal neoplasms, renal cell carcinoma, and glioblastoma at the front line setting. As a major drawback, hypoxia in the context of aberrant perfusion has been linked to increased resistance to conventional chemotherapeutics, radiotherapy, and immunotherapy, raising the need to unravel further signaling pathways and molecules involved in vascular remodeling and characteristics of vascular structure in the tumor microenvironment.
Conventional in vitro assays, including the aortic ring or endothelial tube forming model, have contributed significantly to underlying mechanisms of (patho-) physiological angiogenesis but are limited in reflecting heterogeneous and malformed vessels building the tumor microvasculature, as well as the complexity of the TME. The highly vascularized CAM as the avian gas exchange organ is considered ideal for angiogenic studies. Pharmacological screenings of pro- and anti-angiogenic compounds have long been performed in ovo, with emerging interest in its use in angiogenic research in pre-clinical oncology. After tumor inoculation, tumor xenografts become visible within 2-3 days and are readily supplied with blood vessels of CAM origin that penetrate deep into the tissue. Several qualitative and quantitative approaches have been described to evaluate angiogenic responses in ovo. Recent advances in imaging technologies, as well as improvements in contrast and imaging agents, allow for visualization of vascular perfusion and selective labeling of vascular structures at microscopic levels. Histological and immunohistochemical analyses are considered the reference standard, including hematoxylin-eosin (HE) stainings, staining of vascular and endothelial markers such as CD31 (47) and von Willebrand factor (vWF) (71), or highlighting the vasculature via injection of Sambuco negro agglutinin (SNA) which binds specifically to chicken endothelium (72). In ovo polymer injection followed by micro-CT has moreover been described for three-dimensional vascular remodeling (71). Besides endpoint analyses, versatile methods have been employed for longitudinal monitoring of vascular dynamics. Under controlled light conditions, digital images can be taken and analyzed using image analysis platforms such as Image J (73). Viral nanoparticles have been used for intravital monitoring of vascular structures and fluid dynamics to a depth of 500 µm over 72 h and were retained even after subsequent pathological tissue analysis (74). Hyperspectral imaging has been applied (13) to quantify superficial and deeper tissue oxygenation related to hemoglobin content and water content in tissues based on mathematical modeling of spectral band intensities (Figure 2). Without the need for intravascular application of imaging agents, pre-described ultrasonography can be extended by Doppler mode measurements of vascular structure (24), however largely limited to monitoring of large vessels due to tissue clutter. A more comprehensive characterization of slow-flow vessels in microvasculature has been achieved by high-frequency ultrasensitive ultrasound microvessel (UMI) imaging and validated after administration of two FDA-approved anti-angiogenic agents in a model of renal cell carcinoma (23). As a major limitation, distinguishing tumor-related neoangiogenesis from innate embryonic neovascularization or increased vascular density due to rearrangement of existing vessels can affect data interpretation (62). Timing of angiogenic studies is crucial to avoid respective confounding factors. As the endothelial mitotic index and the general complexity of the CAM decreases around embryonic day (ED) 11, angiogenic studies are widely performed between ED10/11 until ED14/15 (1).
Further perspectives: Investigating the hallmarks of cancer at cellular and molecular levels
Evaluation of tumor growth, metastasis, and vascular remodeling in ovo can give important implications for the therapeutic efficacy and safety of novel agents or the influence of future targets based on knock-in/knock-out models. Notwithstanding, once the tumor is excised, an unlimited field of feasible downstream assays opens up (Figure 3). By that, detailed characterization of classical hallmarks of cancer can be achieved to provide mechanistic insights underlying oncogenesis or therapeutic effects (Figure 4).
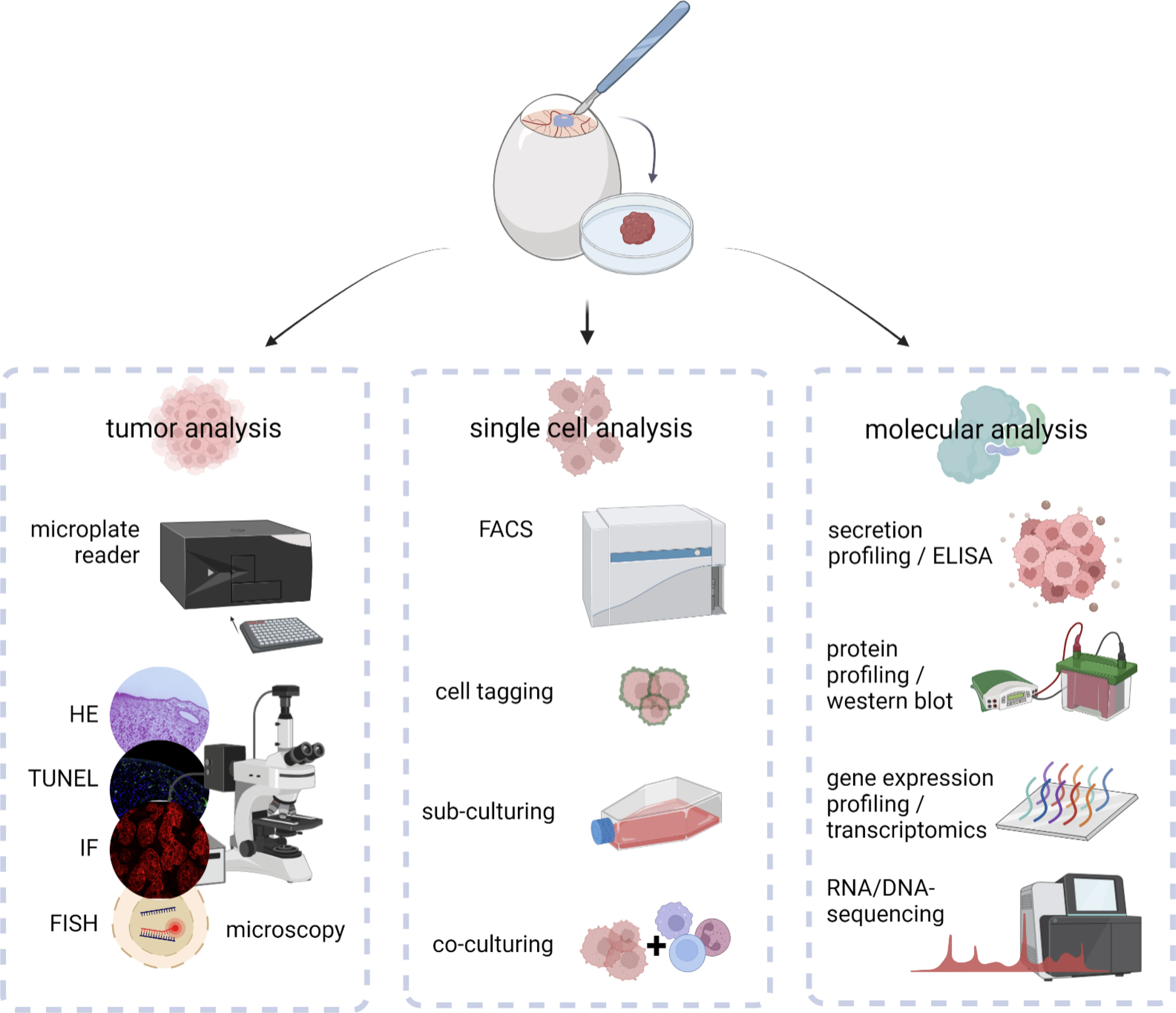
Figure 3 Downstream analysis of excised in ovo-grown tumors at macroscopic, cellular, and molecular levels.
Excised tumors can be embedded for further histological, immunohistochemical, or –fluorescent staining. By that, the architecture of the tumor microenvironment can be evaluated using conventional HE stainings (27), endothelial surface markers for evaluation of tumor perfusion (view chapter 2.3), or selective staining of chick and xenograft cells to evaluate their spatial distribution within the tumor (9). Staining of cleaved caspase 3 or TdT-mediated dUTP-biotin nick end labeling (TUNEL) (29) has been used to evaluate cell death in tissues combined with Ki-67 as a biomarker reflecting the proliferative state (15, 47). Various surface markers of interest, including integrins related to EMT (view chapter 2.2) (26), the immunogenicity of cell death, myeloid (28), and classical checkpoints (75) (view chapter 3.3) as well as diagnostic (9, 15, 35, 45) and predictive biomarkers (14, 31, 73) can be assessed. Tumor dissociation using adequate dissociation kits can be used to validate previous results at single cell levels using flow cytometry after intra- and extracellular staining with high throughput, including intracellular ROS levels, translocation, and phosphorylation of transcription factors, and an unlimited range of surface markers (13). Characterization of the TME can moreover be achieved using ELISA or bead-based multiplex assays for quantification of cytokine, chemokine, and growth factor release (26). As a consequence of aggressive growth, tumor cells exhibit a deregulated metabolism with shifts in carbon consumption characterized by high glycolytic activity, referred to as the Warburg effect (76). In the context of metabolic reprogramming during embryonal development, adaptions characteristic for aerobic glycolysis in cancer have been investigated by uptake measurements of fluorescent glucose analogs, such as 2-NBDG, basal oxygen consumption rates, and extracellular acidification rates using Seahorse technology, or conventional lactate and glucose uptake assay kits in ovo recently (77). Several studies have further focused on metabolic profiling via mass spectrometry (33, 34) or positron emission tomography (PET) imaging for evaluation of glucose metabolism and protein synthesis (53). At the simplest, the metabolic activity can be assessed by conventional colorimetric or fluorescence-based methods, including resazurin, MTT, and WST assays before or after tumor dissociation (10). While non-malignant cells exhibit a limited number of divisions, cancer cells bypass this barrier by hijacking telomerases to extend the length of their telomeres. Replicative immortality can be evaluated in ovo by sub-culturing of excised tumors after dissociation in the context of microtissues, spheroids, or conventional colony formation assays (78). Molecular insights into the mechanistic action of novel therapeutic agents, oncogenesis, and tumor progression have been achieved using western blot, PCR, and transcriptomics (79). Antibody panels with high specificity for chicken tissues based on the complete characterization of the chick genome enable to investigate interactions between xenograft and chicken tissues and help to distinguish between both.
Aspects in cancer immunology
Experimental studies in animal models, rodents in particular, have yielded fundamental insights into key aspects of the development and regulation of the human hematopoietic and immune systems. However, as 65 million years of evolution may suggest, many aspects of mammalian biological systems, particularly their immune systems, display distinct differences (80). As therapeutic approaches become ever more sophisticated and specifically targeted, it becomes increasingly important to address the limitations of extrapolating pre-clinical discoveries to humans using animal models that more closely recapitulate human biological systems. Since the early 2000s, key advances have been made in the development of immunodeficient mice for generating humanized mice based on the mutant IL2rγ gene introduced in non-obese diabetic (NOD)/severe combined immunodeficiency (SCID) (81) and RAG1/2null mice (82). Xenografting of human primary hematopoietic cells and tissues generating a functional human immune system became feasible, opening up novel avenues in basic and translational preclinical research, permitting insights into cause and cure of human diseases, including cancer immunity. As a major drawback, rodent models, and humanized mice in particular, are accompanied by ethical, cost, and efficiency constraints, limiting widespread adoption and pre-clinical investigation at larger scales. As a naturally immunodeficient host, the chick embryo model has served as an ideal preclinical alternative to rodent tumor models for almost a century. Engrafting of human immortalized cell lines and patient-derived xenografts already featuring a complex and unique microenvironment has successfully been applied. However, few studies have taken advantage of a naturally immunodeficient host to study tumor-immune responses without species-specific restrictions in ovo.
Development of the avian immune system
Chicken embryos are naturally immunodeficient, enabling functional analysis of exogenously applied molecules, engrafted cells, or tissues without species-specific restrictions. Knowledge on the avian immune system and its responses still lag behind better studied mammalian biomedical systems, but the chicken genome sequence provided far better understanding in the past years. Development of the innate immune system takes place from ED3 until ED16. Avian macrophages are present in the circulatory system from ED4 and liver and spleen after ED12, where they become functional around ED14 (83). Studies on chicken macrophage receptor repertoires indicated the expression of scavenger, complement, Fc, C-type lectin, and mannose receptors, all essential for antigen recognition. During ontogenesis, embryonic macrophages play an important role in guiding the avian lymphoid system (84) and phagocytose cellular debris (85). As in humans, IFNγ can induce polarization into the pro-inflammatory M1 phenotype, while IL4 induces M2 polarization, involved in tissue repair and angiogenesis via secretion of IL10, TGFβ and VEGF (86, 87). Many aspects of mature dendritic cells (DCs), including their subsets, morphology, surface receptors and dedicated functions, share remarkable similarities between chickens and humans (88, 89). Their precursors have been detected in the avian thymus by ED11, but the time frame of maturation is still subject of investigations (88). Strikingly, while other secondary lymphoid organs vary only slightly in structure and morphology, birds lack encapsulated lymph nodes and the location of avian antigen presentation to lymphocytes remains unclear until now. Analog to mammalian neutrophils, chick embryos develop heterophils (90) containing lysosomal and non-lysosomal enzymes important for pathogen defense. Yet it is unclear whether heterophils represent a homogenous population or a subgroup of cells with different functionalities (91). Functional Natural Killer (NK) cells have been detected in the embryonic spleen starting from ED14. NK cell frequency in peripheral blood lymphocytes is lower in chicken (0,5-1%) compared to humans (2-5%) (92, 93) but identification of several receptors homologue to human KIR, NKp46 and LILR (91, 94) suggested the chicken NK cell biology generally to be close to mammals (95).
Primary lymphoid organs include the thymus and bursa of Fabricius, which are colonized by hematopoietic stem cells to become immunologically competent T and B cells before re-entering the circulation and colonizing peripheral lymphoid organs, broadly similar to mammalian immune systems. Early lymphoid cells deriving from the yolk sac and spleen are present in the thymus starting from ED8 and the bursa of Fabricius on ED11. Like mammals, avian T cells recognize MHC presented antigens via the heterodimeric T cell receptor (TCR), subdivided in αβ and γδ TCRs. Precursor hematopoietic cells enter the thymus in three waves, until TCR-γδ + and TCR-αβ1+ mature T cells migrate to the spleen by ED15 and ED19, respectively (96). Mature B cells leave the bursa of Fabricius only post-hatch (97). Generation of the avian antibody repertoire relies on somatic gene conversion, a process taking place during bursal development, as chickens only have a single copy of functional variable (V) and joining (J) segments for both chains of immunoglobulins (95, 98, 99), representing a major difference compared to mammals.
Although the avian immune system can respond to tumor cells by infiltration of monocytes and inflammatory-like cells such as avian heterophils, it is incapable of mounting an immune response before ED18. A non-specific inflammatory reaction has been reported if the experiment extends beyond ED15 but is dampened if the xenografts are implanted early during development when the avian immune system is still immature (100). Notwithstanding, due to ethical restrictions, in ovo experiments are widely terminated before cell-mediated immunity occurs.
Addressing cancer immunity in ovo
The history of immune-oncological research in ovo is surprisingly short. Until now, two comprehensive studies present in the literature addressed tumor-immune cell interactions related to immune contexture in pancreatic ductal carcinoma (43) and reactive oxygen species-based therapy approaches in ovo (101) (Table 3). Other studies focused on the role of immune cells in inflammation-induced angiogenesis, partially translating results to the angiogenic switch in cancer (view chapter 2.3).
In this view, Naldini and colleagues provided evidence that osteopontin (OPN) up-regulation in endothelial cells could represent a mechanism of amplifying growth factor-induced neovascularization via mononuclear phagocyte recruitment and increased levels of monocyte-derived pro-angiogenic cytokines. Administration of supernatants of human OPN-treated monocytes was highly angiogenic when delivered on the CAM but completely abrogated by neutralizing human anti-IL1 antibodies (102). Likewise, Huang and colleagues validated the anti-inflammatory and pro-angiogenic properties of human mesenchymal stem cell-derived exosomesmiRNA-21-5p for their application to promote ischemic tissue repair (103) and Pacini and colleagues investigated the role of human Gc protein-derived macrophage activating factor (GcMAF) in the context of deregulated angiogenesis in cancer in ovo (104). Ardi and colleagues addressed the contribution of human neutrophils and separately administered human neutrophil-derived MMP9 during the angiogenic switch in cancer in ovo (105), while Bansal and colleagues engrafted bone marrow-derived cells, peripheral blood mononuclear cells, and splenocytes isolated from C57BL/6 mice in the presence of various angiogenic growth factors in the context of choroidal neovascularization in acute macular degeneration (106). Although only partially related to oncogenesis, those findings provided evidence that hematopoietic precursors and mature immune cells of both myeloid and lymphoid lineage could successfully be implanted on the CAM, shaping a complex microenvironment through matrix and vascular remodeling, emphasizing the applicability to study tumor-immune cell interactions in ovo.
In the course of studying plasticity of tumor-associated macrophages, Khabipov and colleagues were the first to engraft immortalized RAW264.7 murine macrophages in a coculture model of pancreatic cancer in ovo. In a hydrogel onplant, murine PDA6606 pancreatic cancer cells were inoculated either with non-stimulated (naïve, M0) or pre-stimulated (polarized; M2) RAW264.7 macrophages at a 1:1 effector-target ratio, with 1 mio. cells, respectively. Pre-stimulated macrophages were generated by exposing naïve RAW264.7 cells to pre-conditioned PDA6606 supernatants 1:2 in fresh medium for 72 h. Interestingly, MRI measurements revealed pre-stimulated macrophages to increase PDA6606 tumor growth compared to monoculture tumors, while naïve did not. The authors hypothesized that the short time span (72 h) limited M2 polarization in naïve macrophages during tumor development (view chapter 3.3). Flow-cytometric analysis of dissociated PDA6606:RAW264.7(pre-stimulated) co-culture tumors confirmed the M2-phenotype of the latter, while tissue sections emphasized their role in promoting angiogenesis and matrix remodeling in pancreatic cancer (43). The second study focused on redox-based effects of oxidant-enriched carrier solutions for adjuvant peritoneal lavage in the context of peritoneal carcinomatosis. In vitro, the approach increased immunogenicity and uptake of three human carcinoma cell lines by monocyte-derived dendritic cells. The authors used the in ovo model as a screening platform to validate the therapeutic efficacy observed in vitro in a physiologically more complex model. Here, human monocyte-derived dendritic cells isolated from healthy donors were engrafted 1:1 with the immortalized tumor cell lines and grown on the CAM for 7 days. Reduction in tumor burden correlated well with results obtained in a model of peritoneal carcinomatosis in mice, emphasizing the translational relevance of the chicken embryo model for pre-clinical studies (101). A widely different approach was employed by Wang and colleagues, who took advantage of homologies in the PD1/PDL1 axis between chickens and humans and proposed the TUM-CAM model as an alternative for immunooncological drug development. Clinically approved checkpoint inhibitors mitigated tumor growth in ovo and partially restored T cell-mediated tumor toxicity of chicken lymphocytes in vitro (75). However, appropriate controls and quantification of crucial mechanistic insights, including checkpoint antibody binding assays, were largely missing, questioning the scientific relevance of such findings. Furthermore, tumors were excised at ED18, only shortly after mature T cells could be detected in the avian circulatory system (view chapter 3.2).
Obstacles or chance?
The caveat of immunooncological research in the chicken embryo model relies in the definition of an appropriate study design depending on the scientific question and careful data interpretation with respect to model-specific characteristics. Comprehensive studies are needed to establish and evaluate eligible protocols to investigate cancer immunity in ovo. Key steps, including reasonable timing of immune cell grafting and convenient routes, co-administration of cytokines and growth factors, and lineage-dependent differences have to be clarified. In some cases, additional irradiation to destroy the developing avian immune system might be worth considering.
Importantly, the majority of in ovo experiments end, due to ethical restrictions in most countries, with ED14, before mature T and B cells, macrophages, and NK cells have been detected in the avian circulation. The onset of DC maturation is yet unknown (83, 95). Future studies have to clarify the role of remaining host immune cell precursors in the outcome and evaluation of tested immunotherapies in ovo. Likewise, it is conceivable that evolutionarily conserved mechanisms or higher homology protein structures are more involved in the control of tumor growth in this system due to higher ligand/receptor cross-reactivity compared to species-specific mechanisms. Many of the hallmarks of cancer recapitulate unicellular modalities, and their onset is suggested to correlate inversely with the chronological sequence in which the respective genes evolved (107, 108). Comparative gene expression profiling helped to identify the repertoire of avian immune receptors, surface-expressed antigens, cytokines, and growth factors and link them to mammalian orthologs in the past. Cross-reactivity between cytokines from different species has been shown to appear with an apparent threshold of around 60% amino acid identity, in dependence on the folding family. However, despite similar biological activities, chicken cytokines have only 25 – 35% amino acid identity with their mammalian counterparts (109, 110). The role of cross-reactivity in the xenogeneic system remains unclear as of now and has to be elucidated in future studies. In analog to the different models of humanization in mice, this review suggests the dual engraftment of human immune and tumor cells in fertilized chicken embryos as a xenogeneic system to study immunotherapies in a more comprehensive but cost-efficient semi in vivo model. This would include the engraftment of human leucocytes, similar to the human peripheral blood leucocyte (Hu-PBL) model in mice. In the Hu-PBL model, a major caveat remains the variable efficacy of different immune cell subpopulations to be engrafted in humanized mice. As such, only low levels of human B lymphocytes and myeloid cells have generally been detected in the circulatory system, most likely due to the lack of human cytokines required for survival (111). Along similar lines, the longevity and functionality of different human immune cell subpopulations and the need for coadministration of relevant human cytokines to ensure their survival has to be evaluated in ovo.
As a major drawback, the short experimental time span dictates limitations in long-term evaluation of cancer immunity in ovo. As such, the dual role of the immune system in the context of immunoediting cannot be addressed. Rare tumor cell variants persisting after the elimination and equilibrium phase become clinically apparent if altering their response to immunoselection pressures in the escape phase (112). Here, the humanized chicken embryo model can provide insights in underlying mechanisms or the efficacy of novel therapeutic strategies using the broad range of applicable read-outs discussed in this review (view chapters 2.2-2.4). For instance, downregulation or loss of tumor antigens, antigen-presenting machinery, or lack of costimulatory molecules results in ineffective priming and activation of DCs and CD8+ T cells in the tumor microenvironment. Therapeutic approaches aim to turn the tide by induction of immunogenic cell death (113), engineering T cells to recognize specific antigens on the surface of cancer cells (114), or administration of adjuvants such as CD40 agonists and anti-CD137 antibodies (115) and can readily be explored in ovo. Another obstacle with tumor cells is their ability to upregulate resistance mechanisms against cytotoxic effectors of immunity, e.g., via STAT3 signaling or increased expression of pro-survival and growth factor genes, including Bcl-2, Her2, EGFR, and c-kit (116, 117). Targeted therapies that find and kill cancer cells by homing in on molecular changes in respective oncogene and growth receptor pathways can likewise be administered and evaluated in ovo. Moreover, specific molecular changes can be identified to develop novel agents targeting transformed cells in the future. Despite fooling the immune system in dangerous hide and seek, cancer cells hijack their surrounding environment to create an immune barrier, cause immune cell malfunction, or even co-opt and modify innocent bystanders to produce tumor promoting growth factors, chemokines and matrix-degrading enzymes. In this view, therapies targeting cytokines (e.g., VEGF, TGFβ), metabolic factors (e.g., adenosine, PGEE2), or blockage of inhibitory receptors (e.g., CTLA4, PD1, TIM3) on effector cells can be considered. Investigation of functional orientation, density, and spatial distribution further allows for correlation of complex immune contexture with tumor growth and outcome (Figure 5).
Overall, extensive research is needed to clarify if the in ovo model represents a faithful avatar for evaluating immunotherapies in preclinical oncological research. Nonetheless, considering previous research in humanized in ovo models of inflammation-induced angiogenesis and latest reports studying tumor-immune cell interactions in ovo, it is conceivable that fertilized chicken embryos could represent a comprehensive research model in immunooncological research in the future.
Outlook
A major advantage relies in the CAM’s easy accessibility and low rejection rate to facilitate increasing the model’s complexity by engrafting 3D spheroids (118, 119) or multicellular organoids. For instance, mature organoids derived from human-induced pluripotent stem cells (PSCs) rapidly connected to the vascular network of the chick embryo after transferring them on the CAM (120), and PSC–derived inner ear and kidney organoids demonstrated the model’s potential to optimize developmental maturity and functionality of organoids based on vascularization (121, 122). Along similar lines, grafting of tumor organoids, also combined with immune cells and/or fibroblasts, reflecting tissue heterogeneity in cancer to a greater extent, can be employed (123).
Engrafting patient-derived xenografts (PDX) could provide improved diagnostic opportunities, tailoring medical treatments in the context of personalized medicine. PDXs preserve many features of primary tumor biology and heterogeneity, including genetic, proteomic, morphological, and pharmacologic characteristics. Moreover, PDXs are characterized by their unique immunological history with cancer-specific immune evasion mechanisms and substantial heterogeneity in (immune) microenvironments (124), reflecting the clinical situation more accurately. Transplantation of human glioblastoma biopsies on the avian CAM emphasized their ability to recapitulate features of the primary tumor, including cellular polymorphism and infiltrating immune cells (125). Likewise, bladder, prostate, and nasopharyngeal carcinoma-derived xenografts could mimic tumor biology, growth, angiogenesis, extracellular matrix interaction, and metastasis as found in the patient (126, 127). Immunohistochemistry, analysis of mutational load, mRNA, and microRNA expression profiling unveiled primary PDAC tumors grown on the CAM to share histopathological and genetic characteristics with the parent tumors (18). Despite solid tumors, tumor cell engraftment and distribution of intravenously injected CD34+ leukemic cells were validated by transcript detection via reverse transcription polymerase chain reaction (RT-PCR) in blood, bone marrow, spleen, and liver from embryos (128). Circulating cancer stem cells were engrafted to analyze aggressiveness and proliferation capacity of primary tumors, closely resembling its complex structure for pre-clinical drug screenings and biomarker discovery (129). Re-grafting and repetitive passaging of human colorectal liver metastases on the CAM, paralleled by immunophenotyping and evaluation of invasiveness, enabled long-term monitoring of PDX tumors, displaying an interesting approach to study tumor progression in ovo (130) (Figure 6).
Needless to say, the chicken embryo model can clearly not be suggested as a complete replacement for conventional pre-clinical animal models. Despite other advantages, humanized mice models aim, e.g., for genetic modifications of HLA expression to ensure appropriate antigen presentation in peripheral tissues or transgenic expression of human cytokines to improve development and function of transplanted cells at large time scales, which widely outperforms the limits of studying oncogenesis and cancer immunity in ovo. On the contrary, the well-vascularized CAM provides high efficiency of tissue grafting, bridging the gap between in vitro and complex but costly mammalian in vivo models while supporting the 3Rs guidelines. With some major limitations and caveats to keep in mind, the humanized chicken chorioallantoic tumor model could serve as an alternative for pre-clinical immunooncological drug screenings and basic immunological research. It provides a quick, reproducible and effective evaluation of different therapeutic options and has, based on PDX, the potential for development of tailored treatments, including personalized immunotherapy (131).
Author contributions
SB designed the review; LM and JB designed the figures; LM and JB performed experiments; all authors wrote the manuscript draft and reviewed the draft. All authors contributed to the article and approved the submitted version.
Funding
Funding was received by the German Federal Ministry of Education and Research (BMBF, grant numbers to SB: 03Z22DN11 and 03Z22Di1).
Acknowledgments
Figure design was supported by the biorender.com platform.
Conflict of interest
The authors declare that the research was conducted in the absence of any commercial or financial relationships that could be construed as a potential conflict of interest.
Publisher’s note
All claims expressed in this article are solely those of the authors and do not necessarily represent those of their affiliated organizations, or those of the publisher, the editors and the reviewers. Any product that may be evaluated in this article, or claim that may be made by its manufacturer, is not guaranteed or endorsed by the publisher.
References
1. Chu PY, Koh AP, Antony J, Huang RY, et al. Applications of the chick chorioallantoic membrane as an alternative model for cancer studies. Cells Tissues Organs (2022) 211(2):222–37. doi: 10.1159/000513039
2. Ribatti D. The chick embryo chorioallantoic membrane as a model for tumor biology. Exp Cell Res (2014) 328(2):314–24. doi: 10.1016/j.yexcr.2014.06.010
3. DeBord LC, Pathak RR, Villaneuva M, Liu HC, Harrington DA, Yu WD, et al. The chick chorioallantoic membrane (CAM) as a versatile patient-derived xenograft (PDX) platform for precision medicine and preclinical research. Am J Cancer Res (2018) 8(8):1642–60.
4. Lokman NA, Elder AS, Ricciardelli C, Oehler MK. Chick chorioallantoic membrane (CAM) assay as an in vivo model to study the effect of newly identified molecules on ovarian cancer invasion and metastasis. Int J Mol Sci (2012) 13(8):9959–70. doi: 10.3390/ijms13089959
5. Kue CS, Tan KY, Lam ML, Lee HB. Chick embryo chorioallantoic membrane (CAM): an alternative predictive model in acute toxicological studies for anti-cancer drugs. Exp Anim (2015) 64(2):129–38. doi: 10.1538/expanim.14-0059
6. Rosenberg SA, Yang JC, Topalian SL, Schwartzentruber DJ, Weber JS, Parkinson DR, et al. Treatment of 283 consecutive patients with metastatic melanoma or renal cell cancer using high-dose bolus interleukin 2. JAMA (1994) 271(12):907–13. doi: 10.1001/jama.1994.03510360033032
7. Rosenberg SA, Yannelli JR, Yang JC, Topalian SL, Schwartzentruber DJ, Weber JS, et al. Treatment of patients with metastatic melanoma with autologous tumor-infiltrating lymphocytes and interleukin 2. J Natl Cancer Inst (1994) 86(15):1159–66. doi: 10.1093/jnci/86.15.1159
8. Fyfe G, Fisher RI, Rosenberg SA, Sznol M, Parkinson DR, Louie AC, et al. Results of treatment of 255 patients with metastatic renal cell carcinoma who received high-dose recombinant interleukin-2 therapy. J Clin Oncol (1995) 13(3):688–96. doi: 10.1200/JCO.1995.13.3.688
9. Bekeschus S, Freund E, Spadola C, Privat-Maldonado A, Hackbarth C, Bogaerts A, et al. Risk assessment of kINPen plasma treatment of four human pancreatic cancer cell lines with respect to metastasis. Cancers (Basel) (2019) 11(9):1237. doi: 10.3390/cancers11091237
10. Khabipov A, Freund E, Liedtke KR, Kading A, Riese J, van der Linde J, et al. Murine macrophages modulate their inflammatory profile in response to gas plasma-inactivated pancreatic cancer cells. Cancers (2021) 13(11):2525. doi: 10.3390/cancers13112525
11. Miebach L, Freund E, Clemen R, Weltmann KD, Metelmann HR, von Woedtke T, et al. Conductivity augments ROS and RNS delivery and tumor toxicity of an argon plasma jet. Free Radic Biol Med (2022) 180:210–9. doi: 10.1016/j.freeradbiomed.2022.01.014
12. Kunz P, Schenker A, Sahr H, Lehner B, Fellenberg J. Optimization of the chicken chorioallantoic membrane assay as reliable in vivo model for the analysis of osteosarcoma. PLoS One (2019) 14(4):e0215312. doi: 10.1371/journal.pone.0215312
13. Miebach L, Freund E, Horn S, Niessner F, Sagwal SK, von Woedtke T, et al. Tumor cytotoxicity and immunogenicity of a novel V-jet neon plasma source compared to the kINPen. Sci Rep (2021) 11(1):136. doi: 10.1038/s41598-020-80512-w
14. Martinho O, Granja S, Jaraquemada T, Caeiro C, Miranda-Goncalves V, Honavar M, et al. Downregulation of RKIP is associated with poor outcome and malignant progression in gliomas. PloS One (2012) 7(1):e30769. doi: 10.1371/journal.pone.0030769
15. Ghaffari-Tabrizi-Wizsy N, Passegger CA, Nebel L, Krismer F, Herzer-Schneidhofer G, Schwach G, et al. The avian chorioallantoic membrane as an alternative tool to study medullary thyroid cancer. Endocr Connect (2019) 8(5):462–7. doi: 10.1530/EC-18-0431
16. Chandrika M, Chua PJ, Muniasamy U, Huang RYJ, Thike AA, Ng C.T, et al. Prognostic significance of phosphoglycerate dehydrogenase in breast cancer. Breast Cancer Res Treat (2021) 186(3):655–65. doi: 10.1007/s10549-021-06123-9
17. Kuan SL, Fischer S, Hafner S, Wang T, Syrovets T, Liu W, et al. Boosting antitumor drug efficacy with chemically engineered multidomain proteins. Adv Sci (Weinh) (2018) 5(8):1701036. doi: 10.1002/advs.201701036
18. Rovithi M, Avan A, Funel N, Leon LG, Gomez VE, Wurdinger T, et al. Development of bioluminescent chick chorioallantoic membrane (CAM) models for primary pancreatic cancer cells: a platform for drug testing. Sci Rep (2017) 7:44686. doi: 10.1038/srep44686
19. Jefferies B, Lenze F, Sathe A, Truong N, Anton M, von Eisenhart-Rothe R, et al. Non-invasive imaging of engineered human tumors in the living chicken embryo. Sci Rep (2017) 7(1):4991. doi: 10.1038/s41598-017-04572-1
20. Zuo Z, Syrovets T, Genze F, Abaei A, Ma G, Simmet T, et al. High-resolution MRI analysis of breast cancer xenograft on the chick chorioallantoic membrane. NMR BioMed (2015) 28(4):440–7. doi: 10.1002/nbm.3270
21. Winter G, Koch ABF, Loffler J, Jelezko F, Linden M, Li H, et al. In vivo PET/MRI imaging of the chorioallantoic membrane. Front Phys (2020) 8:151. doi: 10.3389/fphy.2020.00151
22. Zuo Z, Syrovets T, Wu Y, Hafner S, Vernikouskaya I, Liu W, et al. The CAM cancer xenograft as a model for initial evaluation of MR labelled compounds. Sci Rep (2017) 7:46690. doi: 10.1038/srep46690
23. Eckrich J, Kugler P, Buhr CR, Ernst BP, Mendler S, Baumgart J, et al. Monitoring of tumor growth and vascularization with repetitive ultrasonography in the chicken chorioallantoic-membrane-assay. Sci Rep (2020) 10(1):18585. doi: 10.1038/s41598-020-75660-y
24. Radu-Cosnita AD, Nesiu A, Berzava PL, Cerbu S, Cosma A, Comsa S, et al. Anti-chloride intracellular channel protein 1 (CLIC1) antibodies induce tumour necrosis and angiogenesis inhibition on in vivo experimental models of human renal cancer. Anticancer Res (2022) 42(3):1313–25. doi: 10.21873/anticanres.15599
25. Huang C, Lowerison MR, Lucien F, Gong P, Wang D, Song P, et al. Noninvasive contrast-free 3D evaluation of tumor angiogenesis with ultrasensitive ultrasound microvessel imaging. Sci Rep (2019) 9(1):4907. doi: 10.1038/s41598-019-41373-0.
26. Freund E, Spadola C, Schmidt A, Privat-Maldonado A, Bogaerts A, von Woedtke T, et al. Risk evaluation of EMT and inflammation in metastatic pancreatic cancer cells following plasma treatment. Front Phys (2020) 8(450). doi: 10.3389/fphy.2020.569618
27. Partecke LI, Evert K, Haugk J, Doering F, Normann L, Diedrich S, et al. Tissue tolerable plasma (TTP) induces apoptosis in pancreatic cancer cells in vitro and in vivo. BMC Cancer (2012) 12(1):473. doi: 10.1186/1471-2407-12-473
28. Lin A, Razzokov J, Verswyvel H, Privat-Maldonado A, De Backer J, Yusupov M, et al. Oxidation of innate immune checkpoint CD47 on cancer cells with non-thermal plasma. Cancers (Basel) (2021) 13(3):579. doi: 10.3390/cancers13030579
29. Martinho O, Pinto F, Granja S, Miranda-Goncalves V, Moreira MA, Ribeiro LF, et al. RKIP inhibition in cervical cancer is associated with higher tumor aggressive behavior and resistance to cisplatin therapy. PloS One (2013) 8(3):e59104. doi: 10.1371/journal.pone.0059104
30. Gilson P, Couvet M, Vanwonterghem L, Henry M, Vollaire J, Baulin V, et al. The pyrrolopyrimidine colchicine-binding site agent PP-13 reduces the metastatic dissemination of invasive cancer cells in vitro and in vivo. Biochem Pharmacol (2019) 160:1–13. doi: 10.1016/j.bcp.2018.12.004
31. Kanwar N, Carmine-Simmen K, Nair R, Wang C, Moghadas-Jafari S, Blaser H, et al. Amplification of a calcium channel subunit CACNG4 increases breast cancer metastasis. EBioMedicine (2020) 52:102646. doi: 10.1016/j.ebiom.2020.102646
32. Krutzke L, Allmendinger E, Hirt K, Kochanek S. Chorioallantoic membrane tumor model for evaluating oncolytic viruses. Hum Gene Ther (2020) 31(19-20):1100–13. doi: 10.1089/hum.2020.045
33. Melin C, Perraud A, Christou N, Bibes R, Cardot P, Jauberteau MO, et al. New ex-ovo colorectal-cancer models from different SdFFF-sorted tumor-initiating cells. Anal Bioanal Chem (2015) 407(28):8433–43. doi: 10.1007/s00216-015-9029-z
34. Achkar IW, Kader S, Dib SS, Junejo K, Al-Bader SB, Hayat S, et al. Metabolic signatures of tumor responses to doxorubicin elucidated by metabolic profiling in ovo. Metabolites (2020) 10(7). doi: 10.3390/metabo10070268
35. Peulen O, Gonzalez A, Peixoto P, Turtoi A, Mottet D, Delvenne P, et al. The anti-tumor effect of HDAC inhibition in a human pancreas cancer model is significantly improved by the simultaneous inhibition of cyclooxygenase 2. PLoS One (2013) 8(9):e75102. doi: 10.1371/journal.pone.0075102
36. Nowak-Sliwinska P, Segura T, Iruela-Arispe ML. The chicken chorioallantoic membrane model in biology, medicine and bioengineering. Angiogenesis (2014) 17(4):779–804. doi: 10.1007/s10456-014-9440-7
37. Kohli N, Sawadkar P, Ho S, Sharma V, Snow M, Powell S, et al. Pre-screening the intrinsic angiogenic capacity of biomaterials in an optimised ex ovo chorioallantoic membrane model. J Tissue Eng (2020) 11:2041731420901621. doi: 10.1177/2041731420901621
38. Armstrong PB, Quigley JP, Sidebottom E. Transepithelial invasion and intramesenchymal infiltration of the chick embryo chorioallantois by tumor cell lines. Cancer Res (1982) 42(5):1826–37.
39. Dani SU, Espindola R. A model system for testing gene vectors using murine tumor cells on the chorioallantoic membrane of the chick embryo. Genet Mol Res (2002) 1(2):167–75.
40. Shaw P, Kumar N, Hammerschmid D, Privat-Maldonado A, Dewilde S, Bogaerts A, et al. Synergistic effects of melittin and plasma treatment: A promising approach for cancer therapy. Cancers (Basel) (2019) 11(8):1109. doi: 10.3390/cancers11081109
41. Liedtke KR, Freund E, Hermes M, Oswald S, Heidecke CD, Partecke LI, et al. Gas plasma-conditioned ringer's lactate enhances the cytotoxic activity of cisplatin and gemcitabine in pancreatic cancer in vitro and in ovo. Cancers (Basel) (2020) 12(1):123. doi: 10.3390/cancers12010123
42. Privat-Maldonado A, Verloy R, Cardenas Delahoz E, Lin A, Vanlanduit S, Smits E, et al. Cold atmospheric plasma does not affect stellate cells phenotype in pancreatic cancer tissue in ovo. Int J Mol Sci (2022) 23(4). doi: 10.3390/ijms23041954
43. Khabipov A, Kading A, Liedtke KR, Freund E, Partecke LI, Bekeschus S, et al. RAW 264.7 macrophage polarization by pancreatic cancer cells - a model for studying tumour-promoting macrophages. Anticancer Res (2019) 39(6):2871–82. doi: 10.21873/anticanres.13416
44. Busch M, Papior D, Stephan H, Dunker N. Characterization of etoposide- and cisplatin-chemoresistant retinoblastoma cell lines. Oncol Rep (2018) 39(1):160–172. doi: 10.3892/or.2017.6100
45. Merlos Rodrigo MA, Casar B, Michalkova H, Jimenez Jimenez AM, Heger Z, Adam V, et al. Extending the applicability of in ovo and ex ovo chicken chorioallantoic membrane assays to study cytostatic activity in neuroblastoma cells. Front Oncol (2021) 11:707366. doi: 10.3389/fonc.2021.707366
46. Marcion G, Hermetet F, Neiers F, Uyanik B, Dondaine L, Dias AMM, et al. Nanofitins targeting heat shock protein 110: An innovative immunotherapeutic modality in cancer. Int J Cancer (2021) 148(12):3019–31. doi: 10.1002/ijc.33485
47. Kleibeuker EA, Ten Hooven MA, Castricum KC, Honeywell R, Griffioen AW, Verheul HM, et al. Optimal treatment scheduling of ionizing radiation and sunitinib improves the antitumor activity and allows dose reduction. Cancer Med (2015) 4(7):1003–15. doi: 10.1002/cam4.441
48. Honda N, Kariyama Y, Hazama H, Ishii T, Kitajima Y, Inoue K, et al. Optical properties of tumor tissues grown on the chorioallantoic membrane of chicken eggs: tumor model to assay of tumor response to photodynamic therapy. J BioMed Opt (2015) 20(12):125001. doi: 10.1117/1.JBO.20.12.125001
49. Liedtke KR, Diedrich S, Pati O, Freund E, Flieger R, Heidecke CD, et al. Cold physical plasma selectively elicits apoptosis in murine pancreatic cancer cells in vitro and in ovo. Anticancer Res (2018) 38(10):5655–63. doi: 10.21873/anticanres.12901
50. National Institute of Health. The public health service responds to commonly asked questions (1991). Available at: https://grants.nih.gov/grants/olaw/references/ilar91.htm.Office of Laboratory Animal Welfare
51. IACUC. Policy on protocol approval for use ofChicken embryos and eggs. An Association of New England Medical Center and Tufts (2001).
52. Tayoun T, Faugeroux V, Oulhen M, Deas O, Michels J, Brulle-Soumare L, et al. Targeting genome integrity dysfunctions impedes metastatic potency in non-small cell lung cancer circulating tumor cell-derived explants. JCI Insight (2022) 7(11):e155804. doi: 10.1172/jci.insight.155804
53. Warnock G, Turtoi A, Blomme A, Bretin F, Bahri MA, Lemaire C, et al. In vivo PET/CT in a human glioblastoma chicken chorioallantoic membrane model: a new tool for oncology and radiotracer development. J Nucl Med (2013) 54(10):1782–8. doi: 10.2967/jnumed.112.117150
54. Henning AL, Jiang MX, Yalcin HC, Butcher JT. Quantitative three-dimensional imaging of live avian embryonic morphogenesis via micro-computed tomography. Dev Dyn (2011) 240(8):1949–57. doi: 10.1002/dvdy.22694
55. Brooks PC, Lin JM, French DL, Quigley JP. Subtractive immunization yields monoclonal antibodies that specifically inhibit metastasis. J Cell Biol (1993) 122(6):1351–9. doi: 10.1083/jcb.122.6.1351
56. MacDonald IC, Schmidt EE, Morris VL, Chambers AF, Groom AC. Intravital videomicroscopy of the chorioallantoic microcirculation: a model system for studying metastasis. Microvasc Res (1992) 44(2):185–99. doi: 10.1016/0026-2862(92)90079-5
57. Gordon JR, Quigley JP. Early spontaneous metastasis in the human epidermoid carcinoma HEp3/chick embryo model: Contribution of incidental colonization. Int J Cancer (1986) 38(3):437–44. doi: 10.1002/ijc.2910380321
58. Scher C, Haudenschild C, Klagsbrun M. The chick chorioallantoic membrane as a model system for the study of tissue invasion by viral transformed cells. Cell (1976) 8(3):373–82. doi: 10.1016/0092-8674(76)90149-5
59. Zijlstra A, Lewis J, Degryse B, Stuhlmann H, Quigley JP, et al. The inhibition of tumor cell intravasation and subsequent metastasis via regulation of in vivo tumor cell motility by the tetraspanin CD151. Cancer Cell (2008) 13(3):221–34. doi: 10.1016/j.ccr.2008.01.031
60. Leong HS, Robertson AE, Stoletov K, Leith SJ, Chin CA, Chien AE, et al. Invadopodia are required for cancer cell extravasation and are a therapeutic target for metastasis. Cell Rep (2014) 8(5):1558–70. doi: 10.1016/j.celrep.2014.07.050
61. Kim J, Yu W, Kovalski K, Ossowski L. Requirement for specific proteases in cancer cell intravasation as revealed by a novel semiquantitative PCR-based assay. Cell (1998) 94(3):353–62. doi: 10.1016/S0092-8674(00)81478-6
62. Ribatti D. The chick embryo chorioallantoic membrane (CAM). a multifaceted experimental model. Mech Dev (2016) 141:70–7. doi: 10.1016/j.mod.2016.05.003
63. Swadi RR, Sampat K, Herrmann A, Losty PD, See V, Moss DJ. CDK inhibitors reduce cell proliferation and reverse hypoxia-induced metastasis of neuroblastoma tumours in a chick embryo model. Sci Rep (2019) 9(1):9136. doi: 10.1038/s41598-019-45571-8
64. Deryugina EI, Zijlstra A, Partridge JJ, Kupriyanova TA, Madsen MA, Papagiannakopoulos T, et al. Unexpected effect of matrix metalloproteinase down-regulation on vascular intravasation and metastasis of human fibrosarcoma cells selected in vivo for high rates of dissemination. Cancer Res (2005) 65(23):10959–69. doi: 10.1158/0008-5472.CAN-05-2228
65. Krivacic RT, Ladanyi A, Curry DN, Hsieh HB, Kuhn P, Bergsrud DE, et al. A rare-cell detector for cancer. Proc Natl Acad Sci U.S.A. (2004) 101(29):10501–4. doi: 10.1073/pnas.0404036101
66. Mira E, Lacalle RA, Gomez-Mouton C, Leonardo E, Manes S. Quantitative determination of tumor cell intravasation in a real-time polymerase chain reaction-based assay. Clin Exp Metastasis (2002) 19(4):313–8. doi: 10.1023/A:1015563031769
67. Zijlstra A, Mellor R, Panzarella G, Aimes RT, Hooper JD, Marchenko ND, et al. A quantitative analysis of rate-limiting steps in the metastatic cascade using human-specific real-time polymerase chain reaction. Cancer Res (2002) 62(23):7083–92.
68. Weis SM, Cheresh DA. Tumor angiogenesis: Molecular pathways and therapeutic targets. Nat Med (2011) 17(11):1359–70. doi: 10.1038/nm.2537
69. Hanahan D, Weinberg RA. The hallmarks of cancer. Cell (2000) 100(1):57–70. doi: 10.1016/S0092-8674(00)81683-9
71. Ames JJ, Henderson T, Liaw L, Brooks P.C. Methods for analyzing tumor angiogenesis in the chick chorioallantoic membrane model. Methods Mol Biol (2016) 1406:255–69. doi: 10.1007/978-1-4939-3444-7_22
72. Fergelot P, Bernhard JC, Soulet F, Kilarski WW, Leon C, Courtois N, et al. The experimental renal cell carcinoma model in the chick embryo. Angiogenesis (2013) 16(1):181–94. doi: 10.1007/s10456-012-9311-z
73. Belugali Nataraj N, Salimath BP. Crosstalk between VEGF and novel angiogenic protein regulates tumor angiogenesis and contributes to aggressiveness of breast carcinoma. Cell Signal (2013) 25(1):277–94. doi: 10.1016/j.cellsig.2012.09.013
74. Leong HS, Steinmetz NF, Ablack A, Destito G, Zijlstra A, Stuhlmann H, et al. Intravital imaging of embryonic and tumor neovasculature using viral nanoparticles. Nat Protoc (2010) 5(8):1406–17. doi: 10.1038/nprot.2010.103
75. Wang Y, Rousset X, Prunier C, Garcia P, Dosda E, Leplus E, et al. PD-1/PD-L1 checkpoint inhibitors are active in the chicken embryo model and show antitumor efficacy in vivo. Cancers (Basel) (2022) 14(13). doi: 10.3390/cancers14133095
76. Warburg O. On the origin of cancer cells. Science (1956) 123(3191):309–14. doi: 10.1126/science.123.3191.309
77. Bhattacharya D, Azambuja AP, Simoes-Costa M. Metabolic reprogramming promotes neural crest migration via Yap/Tead signaling. Dev Cell (2020), 53(2) 199–211.e6. doi: 10.1016/j.devcel.2020.03.005
78. Akbari Z, Saadati F, Mahdikia H, Freund E, Abbasvandi F, Shokri B, et al. Antitumor effects in gas plasma-treated patient-derived microtissues–an adjuvant therapy for ulcerating breast cancer? Appl Sci (2021) 11(10):4527. doi: 10.3390/app11104527
79. Saidi A, Javerzat S, Bellahcene A, De Vos J, Bello L, Castronovo V, et al. Experimental anti-angiogenesis causes upregulation of genes associated with poor survival in glioblastoma. Int J Cancer (2008) 122(10):2187–98. doi: 10.1002/ijc.23313
80. Mestas J, Hughes CC. Of mice and not men: Differences between mouse and human immunology. J Immunol (2004) 172(5):2731–8. doi: 10.4049/jimmunol.172.5.2731
81. Ito M, Hiramatsu H, Kobayashi K, Suzue K, Kawahata M, Hioki K, et al. NOD/SCID/gamma(c)(null) mouse: an excellent recipient mouse model for engraftment of human cells. Blood (2002) 100(9):3175–82. doi: 10.1182/blood-2001-12-0207
82. Traggiai E, Chicha L, Mazzucchelli L, Bronz L, Piffaretti JC, Lanzavecchia A, et al. Development of a human adaptive immune system in cord blood cell-transplanted mice. Science (2004) 304(5667):104–7. doi: 10.1126/science.1093933
83. Qureshi MA. Avian macrophage and immune response: An overview. Poult Sci (2003) 82(5):691–8. doi: 10.1093/ps/82.5.691
84. Houssaint E. Cell lineage segregation during bursa of fabricius ontogeny. J Immunol (1987) 138(11):3626–34.
85. Cuadros MA, Coltey P, Carmen Nieto M, Martin C. Demonstration of a phagocytic cell system belonging to the hemopoietic lineage and originating from the yolk sac in the early avian embryo. Development (1992) 115(1):157–68. doi: 10.1242/dev.115.1.157
86. Cui L, Ma Y, Liang Y, Zhang Y, Chen Z, Wang Z, et al. Polarization of avian macrophages upon avian flavivirus infection. Vet Microbiol (2021) 256:109044. doi: 10.1016/j.vetmic.2021.109044
87. Peng L, van den Biggelaar R, Jansen CA, Haagsman HP, Veldhuizen EJA. A method to differentiate chicken monocytes into macrophages with proinflammatory properties. Immunobiology (2020) 225(6):152004. doi: 10.1016/j.imbio.2020.152004
88. Nagy N, Bodi I, Olah I. Avian dendritic cells: Phenotype and ontogeny in lymphoid organs. Dev Comp Immunol (2016) 58:47–59. doi: 10.1016/j.dci.2015.12.020
89. Vu Manh TP, Marty H, Sibille P, Le Vern Y, Kaspers B, Dalod M, et al. Existence of conventional dendritic cells in gallus gallus revealed by comparative gene expression profiling. J Immunol (2014) 192(10):4510–7. doi: 10.4049/jimmunol.1303405
90. Zijlstra A, Seandel M, Kupriyanova TA, Partridge JJ, Madsen MA, Hahn-Dantona EA, et al. Proangiogenic role of neutrophil-like inflammatory heterophils during neovascularization induced by growth factors and human tumor cells. Blood (2006) 107(1):317–27. doi: 10.1182/blood-2005-04-1458
91. Kaiser P, Balic A. The avian immune system. In: Sturkie's avian physiology. Academic Press (2015). p. 403–4018.
92. Perera Molligoda Arachchige AS. Human NK cells: From development to effector functions. Innate Immun (2021) 27(3):212–29. doi: 10.1177/17534259211001512
93. Juul-Madsen HR, Viertlböeck B, Härtle S, Smith A.L, Göbel TW. Innate immune responses. Avian Immunol (2014) p:121–47. doi: 10.1016/B978-0-12-396965-1.00007-8
94. Neulen ML, Viertlboeck BC, Straub C, Gobel TW. Identification of novel chicken CD4(+) CD3(-) blood population with NK cell like features. Dev Comp Immunol (2015) 49(1):72–8. doi: 10.1016/j.dci.2014.11.012
95. Garcia P, Wang Y, Viallet J, Macek Jilkova Z. The chicken embryo model: A novel and relevant model for immune-based studies. Front Immunol (2021) 12:791081. doi: 10.3389/fimmu.2021.791081
96. Chen CH, Pickel J.M, Lahti J.M, Cooper MD. Surface marker on avian immune cells. In: Sharma JM, editor. Avian cellular immunology. Routledge (1991). p. 1–22.
97. Le Douarin NM, Houssaint E, Jotereau FV, Belo M. Origin of hemopoietic stem cells in embryonic bursa of fabricius and bone marrow studied through interspecific chimeras. Proc Natl Acad Sci U S A (1975) 72(7):2701–5. doi: 10.1073/pnas.72.7.2701
98. Parra D, Takizawa F, Sunyer JO. Evolution of b cell immunity. Annu Rev Anim Biosci (2013) 1:65–97. doi: 10.1146/annurev-animal-031412-103651
99. Ko KH, Lee IK, Kim G, Gu MJ, Kim HY, Park BC, et al. Changes in bursal b cells in chicken during embryonic development and early life after hatching. Sci Rep (2018) 8(1):16905. doi: 10.1038/s41598-018-34897-4
100. Leene W, Duyzings MJ, van Steeg C. Lymphoid stem cell identification in the developing thymus and bursa of fabricius of the chick. Z Zellforsch Mikrosk Anat (1973) 136(4):521–33. doi: 10.1007/BF00307368
101. Miebach L, Freund E, Clemen R, Kersting S, Partecke LI, Bekeschus S. Gas plasma–oxidized sodium chloride acts via hydrogen peroxide in a model of peritoneal carcinomatosis. Proc Natl Acad Sci (2022) 119(31). doi: 10.1073/pnas.2200708119
102. Naldini A, Leali D, Pucci A, Morena E, Carraro F, Nico B, et al. Cutting edge: IL-1beta mediates the proangiogenic activity of osteopontin-activated human monocytes. J Immunol (2006) 177(7):4267–70. doi: 10.4049/jimmunol.177.7.4267
103. Huang C, Luo W, Wang Q, Ye Y, Fan J, Lin L, et al. Human mesenchymal stem cells promote ischemic repairment and angiogenesis of diabetic foot through exosome miRNA-21-5p. Stem Cell Res (2021) 52:102235. doi: 10.1016/j.scr.2021.102235
104. Pacini S, Morucci G, Punzi T, Gulisano M, Ruggiero M. Gc protein-derived macrophage-activating factor (GcMAF) stimulates cAMP formation in human mononuclear cells and inhibits angiogenesis in chick embryo chorionallantoic membrane assay. Cancer Immunol Immunother (2011) 60(4):479–85. doi: 10.1007/s00262-010-0953-7
105. Ardi VC, Kupriyanova TA, Deryugina EI, Quigley JP. Human neutrophils uniquely release TIMP-free MMP-9 to provide a potent catalytic stimulator of angiogenesis. Proc Natl Acad Sci U S A (2007) 104(51):20262–7. doi: 10.1073/pnas.0706438104
106. Bansal A, Csaky KG, Ponce ML. The origin of xenotransplanted putative endothelial progenitor cells in a modified chick chorioallantoic membrane (CAM) angiogenesis assay., in ARVO anual meeting. In: Investigative ophthalmology & visual science (2004). p. 1872.
107. Lineweaver CH, Bussey KJ, Blackburn AC, Davies PCW. Cancer progression as a sequence of atavistic reversions. Bioessays (2021) 43(7):e2000305. doi: 10.1002/bies.202000305
108. Bussey KJ, Davies PCW. Reverting to single-cell biology: The predictions of the atavism theory of cancer. Prog Biophys Mol Biol (2021) 165:49–55. doi: 10.1016/j.pbiomolbio.2021.08.002
109. Shields LE, Jennings J, Liu Q, Lee J, Ma W, Blecha F, et al. Cross-species genome-wide analysis reveals molecular and functional diversity of the unconventional interferon-omega subtype. Front Immunol (2019) 10:1431. doi: 10.3389/fimmu.2019.01431
110. Scheerlinck JP. Functional and structural comparison of cytokines in different species. Vet Immunol Immunopathol (1999) 72(1-2):39–44. doi: 10.1016/S0165-2427(99)00115-4
111. De La Rochere P, Guil-Luna S, Decaudin D, Azar G, Sidhu SS, Piaggio E, et al. Humanized mice for the study of immuno-oncology. Trends Immunol (2018) 39(9):748–63. doi: 10.1016/j.it.2018.07.001
112. Dunn GP, Bruce AT, Ikeda H, Old LJ, Schreiber RD. Cancer immunoediting: from immunosurveillance to tumor escape. Nat Immunol (2002) 3(11):991–8. doi: 10.1038/ni1102-991
113. Obeid M, Tesniere A, Ghiringhelli F, Fimia GM, Apetoh L, Perfettini JL, et al. Calreticulin exposure dictates the immunogenicity of cancer cell death. Nat Med (2007) 13(1):54–61. doi: 10.1038/nm1523
114. Depil S, Duchateau P, Grupp SA, Mufti G, Poirot L. 'Off-the-shelf' allogeneic CAR T cells: development and challenges. Nat Rev Drug Discovery (2020) 19(3):185–99.
115. Tai YT, Li X, Tong X, Santos D, Otsuki T, Catley L, et al. Human anti-CD40 antagonist antibody triggers significant antitumor activity against human multiple myeloma. Cancer Res (2005) 65(13):5898–906. doi: 10.1158/0008-5472.CAN-04-4125
116. Potti A, Moazzam N, Langness E, Sholes K, Tendulkar K, Koch M, et al. Immunohistochemical determination of HER-2/neu, c-kit (CD117), and vascular endothelial growth factor (VEGF) overexpression in malignant melanoma. J Cancer Res Clin Oncol (2004) 130(2):80–6. doi: 10.1007/s00432-003-0509-8
117. Disis ML, Wallace DR, Gooley TA, Dang Y, Slota M, Lu H, et al. Concurrent trastuzumab and HER2/neu-specific vaccination in patients with metastatic breast cancer. J Clin Oncol (2009) 27(28):4685–92. doi: 10.1200/JCO.2008.20.6789
118. De Magalhaes N, Liaw LH, Berns M. An instruction on the in vivo shell-less chorioallantoic membrane 3-dimensional tumor spheroid model. Cytotechnology (2010) 62(3):279–83. doi: 10.1007/s10616-010-9279-8
119. Elberskirch L, Le Harzic R, Scheglmann D, Wieland G, Wiehe A, Mathieu-Gaedke M, et al. A HET-CAM based vascularized intestine tumor model as a screening platform for nano-formulated photosensitizers. Eur J Pharm Sci (2022) 168:106046. doi: 10.1016/j.ejps.2021.106046
120. Schmidt S, Alt Y, Deoghare N, Krüger S, Kern A, Rockel AF, et al. A blood vessel organoid model recapitulating aspects of vasculogenesis, angiogenesis and vessel wall maturation. Organoids (2022) 1(1):41–53. doi: 10.3390/organoids1010005
121. Moeinvaziri F, Shojaei A, Haghparast N, Yakhkeshi S, Nemati S, Hassani SN, et al. Towards maturation of human otic hair cell-like cells in pluripotent stem cell-derived organoid transplants. Cell Tissue Res (2021) 386(2):321–33. doi: 10.1007/s00441-021-03510-y
122. Kaisto S, Saarela U, Donges L, Raykhel I, Skovorodkin I, Vainio SJ, et al. Optimization of renal organoid and organotypic culture for vascularization, extended development, and improved microscopy imaging. J Vis Exp (2020) 157. doi: 10.3791/60995
123. Drost J, Clevers H. Organoids in cancer research. Nat Rev Cancer (2018) 18(7):407–18. doi: 10.1038/s41568-018-0007-6
124. Thelen M, Wennhold K, Lehmann J, Garcia-Marquez M, Klein S, Kochen E, et al. Cancer-specific immune evasion and substantial heterogeneity within cancer types provide evidence for personalized immunotherapy. NPJ Precis Oncol (2021) 5(1):52. doi: 10.1038/s41698-021-00196-x
125. Balčiūnienė N, Tamasauskas A, Valanciute A, Deltuva V, Vaitiekaitis G, Gudinaviciene I, et al. Histology of human glioblastoma transplanted on chicken chorioallantoic membrane. Medicina (2009) 45(2). doi: 10.3390/medicina45020016
126. Hu J, Ishihara M, Chin AI, Wu L. Establishment of xenografts of urological cancers on chicken chorioallantoic membrane (CAM) to study metastasis. Precis Clin Med (2019) 2(3):140–51. doi: 10.1093/pcmedi/pbz018
127. Xiao X, Zhou X, Ming H, Zhang J, Huang G, Zhang Z, et al. Chick chorioallantoic membrane assay: A 3D animal model for study of human nasopharyngeal carcinoma. PLoS One (2015) 10(6):e0130935. doi: 10.1371/journal.pone.0130935
128. Farhat A, Ali-Deeb E, Sulaiman A, Aljamali M. Reinforcing the utility of chick embryo model to in vivo evaluate engraftment of human leukemic stem cells. J Egypt Natl Canc Inst (2018) 30(1):1–5. doi: 10.1016/j.jnci.2018.01.005
129. Pizon M, Schott D, Pachmann U, Schobert R, Pizon M, Wozniak M, et al. Chick chorioallantoic membrane (CAM) assays as a model of patient-derived xenografts from circulating cancer stem cells (cCSCs) in breast cancer patients. Cancers (Basel) (2022) 14(6). doi: 10.3390/cancers14061476
130. Ceausu RA, Ciolofan A, Blidisel A, Cretu OM, Cimpean AM, Raica M, et al. Liver metastatic colorectal tumor cells change their phenotype during consecutive passages on chick embryo chorioallantoic membrane: Lessons from the Lab to the clinic. In Vivo (2021) 35(5):2711–8. doi: 10.21873/invivo.12555
Keywords: CAM, cancer immunity, patient-derived xenografts, oncology, macrophages
Citation: Miebach L, Berner J and Bekeschus S (2022) In ovo model in cancer research and tumor immunology. Front. Immunol. 13:1006064. doi: 10.3389/fimmu.2022.1006064
Received: 28 July 2022; Accepted: 13 September 2022;
Published: 29 September 2022.
Edited by:
Ibrahim Ozbolat, The Pennsylvania State University (PSU), United StatesReviewed by:
Sergej Tomić, Institute for the Application of Nuclear Energy (INEP), SerbiaDomenico RIBATTI, University of Bari Aldo Moro, Italy
Copyright © 2022 Miebach, Berner and Bekeschus. This is an open-access article distributed under the terms of the Creative Commons Attribution License (CC BY). The use, distribution or reproduction in other forums is permitted, provided the original author(s) and the copyright owner(s) are credited and that the original publication in this journal is cited, in accordance with accepted academic practice. No use, distribution or reproduction is permitted which does not comply with these terms.
*Correspondence: Sander Bekeschus, c2FuZGVyLmJla2VzY2h1c0BpbnAtZ3JlaWZzd2FsZC5kZQ==