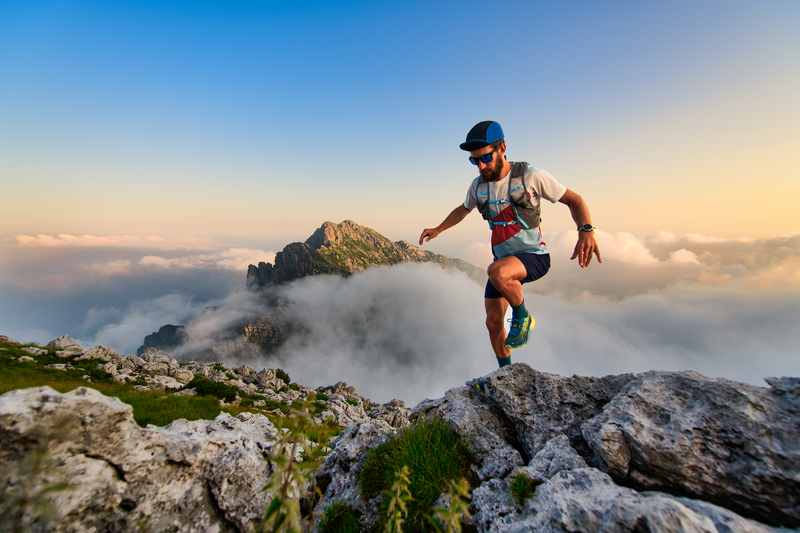
95% of researchers rate our articles as excellent or good
Learn more about the work of our research integrity team to safeguard the quality of each article we publish.
Find out more
MINI REVIEW article
Front. Immunol. , 28 September 2022
Sec. Cytokines and Soluble Mediators in Immunity
Volume 13 - 2022 | https://doi.org/10.3389/fimmu.2022.1000996
This article is part of the Research Topic Women in Cytokines and Soluble Mediators in Immunity View all 63 articles
Acute myeloid leukemia (AML) is a highly heterogeneous malignancy of the blood and bone marrow, characterized by clonal expansion of myeloid stem and progenitor cells and rapid disease progression. Chemotherapy has been the first-line treatment for AML for more than 30 years. Application of recent high-throughput next-generation sequencing technologies has revealed significant molecular heterogeneity to AML, which in turn has motivated efforts to develop new, targeted therapies. However, due to the high complexity of this disease, including multiple driver mutations and the coexistence of multiple competing tumorigenic clones, the successful incorporation of these new agents into clinical practice remains challenging. These continuing difficulties call for the identification of innovative therapeutic approaches that are effective for a larger cohort of AML patients. Recent studies suggest that chronic immune stimulation and aberrant cytokine signaling act as triggers for AML initiation and progression, facets of the disease which might be exploited as promising targets in AML treatment. However, despite the greater appreciation of cytokine profiles in AML, the exact functions of cytokines in AML pathogenesis are not fully understood. Therefore, unravelling the molecular basis of the complex cytokine networks in AML is a prerequisite to develop new therapeutic alternatives based on targeting cytokines and their receptors.
Acute Myeloid Leukemia (AML) is a highly aggressive and heterogenous hematological cancer characterized by the accumulation of molecular and cytogenetic mutations within hematopoietic stem and/or progenitor cells (HSPCs), leading to the establishment of leukemic stem cells (LSCs). LSCs are the source of immature myeloid progenitor cells, so-called myeloblasts or leukemic blasts, which accumulate in the bone marrow (BM), displace normal HSPCs, impair normal hematopoiesis, and eventually spread into the peripheral blood (PB), lymph nodes, liver, spleen, testes, and central nervous system (1–4). Whereas many AML patients follow an aggressive clinical course with an overall 5-year survival rate of only 28%, individual patient survival strongly depends on the underlying tumor-driving genetic alterations and individual risk factors, including age, gender, prior chemotherapy, radiation exposure and genetic predisposition (1, 2, 5–8). However, irrespective of the molecular driver mutations initiating the disease, AML onset and development always go hand in hand with significant remodeling of the BM into a tumor-promoting microenvironment that supports and protects LSCs at the expense of normal HSPCs (9–15). In this review we discuss how cytokine signaling networks contribute to these maladaptations, fuel AML tumorigenesis and progression, and enable chemoresistance and immune evasion. We further shed some light on promising therapeutic approaches targeting cytokine signaling to irradicate the LSC population and prevent relapse after chemotherapy.
Within the healthy BM microenvironment, hematopoietic stem cells (HSCs) are normally maintained in a delicate balance between quiescence, self-renewal, and differentiation to ensure life-long steady-state hematopoiesis and replenishment of the blood effector cell population under stress conditions such as infection, acute and chronic inflammation, aging or bleeding (16). During infection and inflammation, an array of cytokines, including Interleukin (IL)-1β (17, 18), IL-3 (19, 20), IL-6 (21–23), Tumor necrosis factor-α (TNF-α) (24–27) and Interferon (IFN) (28, 29) together with hematopoietic growth factors (HGFs) such as M-CSF, G-CSF and GM-CSF (17, 30), orchestrates the switch from steady-state to emergency hematopoiesis (31–33). In patients with preleukemic and leukemic conditions, including AML, the tight regulation of these cytokines is impaired, leading to aberrant cytokine secretion (32–38). Studies evaluating pro- and anti-inflammatory cytokine and growth factor levels in serum revealed that GM-CSF, IL-1β, IL-3, IL-4, IL-5, IL-6, IL-8, IL-10, IL-12p70, IL-27, IL-35, osteopontin and stem cell factor (SCF) are upregulated in all or in distinct AML patient groups compared to age-matched controls (39–43). In contrast to most cytokines, TRAIL and TGF-β levels are decreased in the serum of AML patients (39, 43, 44). To gain insights into the specific functions of individual cytokines and growth factors in AML, numerous studies have characterized the effects of recombinant cytokines and HGFs on proliferation and colony formation of primary AML cells and cell lines in vitro, thereby establishing ex vivo AML cell culture conditions (see Table 1).
Suggestive of a supportive feedback loop, some patient-derived LSCs and blasts can produce a variety of cytokines (e.g., IL-1β, IL-1α, IL-6, GM-CSF, and TNF-α) and proliferate in vitro without the addition of exogenous cytokines and HGFs (99–101). In particular, IL-1β was shown to act as an autocrine growth factor for AML blasts by inducing the production of HGFs and cytokines, including GM-CSF and IL-6 (50, 59, 99, 102–105). Moreover, IL-1-stimulated AML blast cells secreted increased levels of TNF-α, which synergized with IL-3- or GM-CSF-induced AML cell proliferation and colony formation (106). Of note, increased autonomous and/or HGF- and cytokine-induced in vitro proliferation of patient-derived leukemic cells correlated with negative clinical outcomes, including lower complete remission rates, higher risk for relapse, and shorter AML patient survival (107–109). In vivo, however, LSC and blast growth, survival, and protection from therapeutic agents do not exclusively rely on endogenous cytokine signaling, but strongly depend on AML cell interactions with the leukemic BM microenvironment and the latter’s provisioning of supporting ligands and soluble factors, including cytokines (Figure 1), some of which are discussed in the following sections (110).
Figure 1 Cytokines supporting AML progression. Osteoblasts, myeloblasts and mesenchymal stromal cells (MSCs) secrete osteopontin. This in turn promotes AML cell proliferation and disease progression. CXCL12 is mainly secreted by perivascular stromal cells (PSCs), and osteoblasts and promotes growth and survival of AML blasts cell via the chemokine receptor CXCR4. IL-1β acts on myeloblasts and HSPCs, which express the IL-1 receptor (IL-1R) as well as the IL-1 receptor accessor protein (IL-1RAP), thereby enhancing IL-1β production, AML cell proliferation and survival. IL-1 signaling can be blocked by Canakinumab, a human monoclonal antibody targeting IL-1β. IL-8 is constitutively produced by AML myeloblasts and acts in an autocrine way. MSCs and myeloblasts are potent sources of IL-6, which can be blocked by IL-6-blocking antibodies such as Siltuximab. Created with Biorender.com.
Osteopontin, a secreted matrix glycoprotein produced by many cell types (e.g., stomal, endothelial, epithelial and immune cells), is crucial for the regulation and/or induction of inflammation, angiogenesis, proliferation, migration, and apoptosis throughout the body. During normal hematopoiesis, osteopontin is predominantly produced by osteoblasts within in the endosteal BM region, to guide and maintain healthy HSCs within supportive niches (111). Interestingly, PB and BM osteopontin levels were significantly increased in AML patients compared to healthy controls, and high osteopontin BM levels were associated with reduced overall and event-free survival (82, 83). Osteopontin was not, however, exclusively expressed by cells of the osteolineage within the leukemic BM, although it was strongly expressed by AML blasts (82). Additionally, it was shown that AML patient-derived mesenchymal stromal cells (MSCs) or healthy MSCs co-cultured with AML cells undergo osteogenic differentiation and produce increased amounts of osteopontin (112). Functionally, osteopontin was shown to upregulate AKT, mTOR, PTEN, and β-catenin mRNA expression in AML cells in vitro (113) and increase AML LSC self-renewal, proliferation, and expression of anti-apoptotic and cell-cycle-associated genes, thereby leading to accelerated disease progression in an MLL-AF9 driven AML mouse model (84). Direct targeting of osteopontin is difficult due to its ubiquitous expression and, so far, has been limited to approaches utilizing RNAi or blocking antibodies and aptamers in breast cancer models. Although delivered without specificity to cell type, these initial treatment studies confirm the antitumoral effect of osteopontin inhibition (114) and call for testing in AML disease models.
Due to its pleiotropic effects, IL-1-mediated signaling is recognized as a central hub between inflammation and cancer, including leukemia development and progression (32, 115–119). In AML patients, multiple studies have reported increased levels of IL-1β and IL-1 receptors as well as decreased levels of interleukin-1 receptor antagonist (IL-1RA) in PB and BM (41, 42, 56, 120). In an MLL-AF9-driven leukemic mouse model, chronic exposure to IL-1β accelerated leukemia progression and impaired normal hematopoiesis by modulating stromal niche support. Using both in vitro and in vivo AML models it was shown that depletion or deletion of IL-1RA resulted in reduced expansion of AML progenitor cells and partially restored normal hematopoiesis (121). This was confirmed by targeting IL-1 receptor signaling via inhibition of p38 MAPK, which enabled normal HSPCs to expand in the presence of IL-1β (41). Of note, in vivo AML development was curbed by knockout of IL-1 receptor in the MLL-AF9 mouse model but was increased in FLT3-ITD-driven leukemic mice (122), suggesting different dependencies on IL-1 signaling. Additionally, it was shown that AML HSPCs express high levels of IL-1 receptor accessory protein (IL-1RAP), which contributed to increased IL-1β production, AML cell proliferation and survival, but reduced normal hematopoiesis. This phenotype was further promoted when co-culturing AML CD34+ HSPCs on MSCs (123, 124). Inhibition of IL-1RAP signaling antagonized this effect and enabled HSC proliferation in the presence of AML cell-conditioned media (124). In line with these AML cell-supporting functions, gene expression analysis revealed reduced overall survival (OS) of AML patients who expressed high levels of IL-1RAP (123). These observations suggest an important role for the IL-1β signaling pathway in the pathogenesis of AML and encourage studies to evaluate the therapeutic effects of targeting IL-1 signaling (117, 120). Multiple US Food and Drug Administration (FDA)-approved IL-1 blockers [Anakinra (Kineret); Rilonacept (Arcalyst); Canakinumab (Ilaris)] are already available. In particular, the effect of Canakinumab is being intensely evaluated in the CANTOS trail (NCT01327846), a randomized, double-blind, placebo-controlled phase 3 study involving 10,061 patients with solid tumors as well as hematological malignancies like chronic myelomonocytic leukemia (CMML) and myelodysplastic syndrome (MDS) (125, 126). However, additional studies will be required to fully understand the therapeutic value of targeting IL-1 and in particular IL-1β in hematological malignancies, including AML.
IL-6 is a potent pro-inflammatory cytokine which is crucial for a rapid and coordinated immune response during infections and tissue injuries, but also helps to maintain the hematopoietic system (127–129). Deregulated expression of IL-6 is associated with inflammatory and autoimmune diseases as well as skewed hematopoiesis and leukemia (104, 127, 130). In AML patients with reduced OS, blood and BM serum levels of IL-6 are increased (39, 53, 131). Further studies confirm these findings and suggest that IL-6 levels correlate with poor prognosis, rapid disease progression, and resistance to chemotherapy (39, 132–134). The combined assessment of PB IL-6 and FLT3-ligand levels during AML induction therapy revealed that patients with persistent high IL‐6 levels display lower survival rates compared to patients with decreasing IL-6 and increasing FLT3-ligand levels (134). Similarly, low IL-6 levels accompanied by high IL-10 levels have been linked to better prognosis (39). Although AML blasts are clearly exposed to microenvironment-derived (135–137) and self-produced IL-6 (104), it is disputed how IL-6 contributes to AML progression. Curiously, all AML samples express the IL-6 receptor but only a subset responds to IL-6 treatment in vitro (48, 67, 69, 70, 131, 138). However, multiple studies suggest that IL-6-induced STAT3 signaling promotes AML by inducing chemoresistance (132, 135, 139). Hou and colleagues showed that BM MSCs promote chemoresistance against daunorubicin and cytosine arabinoside (Ara-c) by increasing IL-6 secretion and activation of STAT3 signaling and the oxidative phosphorylation metabolic pathway in AML cells (135). Zhang et al. showed that IL-6-induced STAT3 signaling promotes CD36 expression, CD36-mediated uptake of fatty acids, and chemoresistance against Ara-c (139). Several IL-6 or IL-6 receptor-blocking antibodies have demonstrated promising results in (pre-) clinical studies for the treatment of cancers, chronic inflammation, and autoimmune diseases (130). While Siltuximab (CNTO 328; IL-6-blocking antibody) has been proposed as a treatment option for myelodysplastic syndrome (MDS) and multiple myeloma and is FDA-approved for the treatment of idiopathic multicentric Castleman’s disease (140), Siltuximab in the AML setting has so far only been investigated in an AML xenograft mouse model that mimics end-stage BM failure. In that study, Siltuximab treatment antagonized AML-induced anemia and BM failure and prolonged mouse OS (141).
IL-8 (CXCL8) belongs to the CXC family of chemokines and is best known for its role as a chemoattractant for neutrophils (142). While production of IL-8 can be induced by various stimuli, including lipopolysaccharide, IL-1, and TNF in healthy cells, many tumor cells express IL-8 constitutively (142) or in a hypoxia-, acidosis-, or chemotherapy-induced manner, leading to anti-apoptotic and growth-supporting MAPK, PI3K, FAK and SRC18 signaling (143). In AML, constitutive production of IL-8 has been observed in both AML cell lines and primary AML samples, together with expression of functional IL-8 receptors [IL-8RA (CXCR1) and IL-8RB (CXCR2)] (144–146). Interestingly, AML cell-derived IL-8 also signals in a paracrine manner and affects neighboring non-leukemic cells in the BM microenvironment. Hypoxia-induced IL-8 secretion by AML cells resulted in increased migration of MSCs into the leukemic BM niche (147). MSCs, in turn, prevent apoptosis and confer drug resistance on leukemic cells by up-regulation of anti-apoptotic proteins and secretion of growth factors, cytokines, and extracellular vesicles (148, 149). Importantly, it has been shown that IL-8 production and secretion by MSCs, fibroblasts, and endothelial cells is induced or increased upon their co-culture with AML cells, thereby contributing to reduced apoptosis and increased proliferation and chemoresistance of the AML cells (150–152). In patients, this bidirectional signaling seems to result in elevated IL-8 levels in PB and BM levels (72), which additionally might contribute to impaired neutrophil migration and hematopoiesis (153). However, further confirmation, especially in the context of AML, is required. Inhibition of the IL-8–IL-8R axis has been proposed as a novel therapeutic intervention targeting the aberrant leukemic BM microenvironment. Blocking the IL-8 pathway with neutralizing antibodies has been shown to restore the sensitivity of malignant cells to chemotherapeutics and reduce AML cell proliferation (150, 151). Using knockdown or pharmacological inhibition approaches, Schinke and colleagues showed that inhibition of IL-8RB-mediated signaling leads to a significant reduction in proliferation and G0/G1 cell cycle arrest in several leukemic cell lines and primary MDS/AML samples (119).
The chemotactic cytokine (chemokine) CXCL12, also referred to as SDF-1, is secreted by a variety of cells including stromal cells, fibroblasts, and epithelial cells (154). CXCL12 initiates signaling by binding to its receptors CXCR4 and CXCR7 and plays a crucial role in regulating hematopoiesis (proliferation, differentiation, survival) and hematopoietic cell trafficking to and within the BM (95, 155), but also contributes to tumor growth, survival, metastasis, vascularization, and chemoresistance of several types of cancer (95, 156–160). In AML, low expression of CXCL12, high expression of CXCR4 and low to intermediate expression of CXCR7 have been measured on AML blasts in comparison to normal HSPCs (92–94). Interestingly, decreased CXCL12 and increased CXCR4 expression by AML blasts was associated with reduced patient relapse-free and overall survival OS (161–164). Within the healthy BM, CXCL12 is mainly secreted by perivascular stromal cells [mesenchymal stem and CXCL12-abundant reticular (CAR) cells], endothelial cells, and osteoblasts, thereby guiding, retaining, and regulating HSPCs to and within supportive BM niches (16). Within the AML BM microenvironment, it has not yet been determined which and to what extent cell populations produce and secrete CXCL12. In vitro, CXCL12 was shown to promote AML cell growth, survival, and chemoresistance (95–98) by activating or inducing the pro-survival proteins PI3K/AKT, MAP3K/ERK1/2, MYC, Bcl-2, and Bcl-XL (93, 96, 165). In vivo, however, while the deletion of CXCR4 in AML MLL-AF9+ HSPCs prolonged leukemic mouse survival, deletion of CXCL12 within the AML microenvironment did not alter the development and progression of the disease (166). This surprising finding suggests that CXCR4 signaling can support AML cells in a CXCL12-independent manner. Nevertheless, blocking the CXCL12/CXCR4 axis represents an attractive therapeutic strategy and several CXCR4 and CXCL12 inhibitors have been developed and used in preclinical and clinical models to induce the mobilization of the AML cells from the BM into the circulation with the aim of increasing their exposure to chemotherapeutic agents (95, 167). Plerixaflor (NCT01319864, NCT01352650, NCT01027923), LY2510924 (NCT02652871), and PF-06747143 (NCT02954653) are among the antagonists that have been under Phase 1 clinical trials to test for safety, tolerability and clinical activity, either alone or in combination with standard chemotherapy in AML patients.
Interferon-γ (IFN-γ) is one of the lead cytokines of cellular immunity. It is mainly secreted by activated lymphocytes (168) and orchestrates tumor defense by regulating AML blast survival and apoptosis (118). While T cells obtained from AML patients at primary diagnosis exhibit increased IFN-γ production, strongly reduced levels of IFN-γ were observed in CD8+ T cells from patients who developed relapsed AML after allogeneic HCT (allo-HCT), whereas patients without relapse did not show reduced IFN-γ production (169). This suggests that lower IFN-γ levels may elevate the risk of relapse. An early phase 1 trial was recently started to evaluate the potential of IFN-γ treatment in AML patients with reoccurring disease after allo-HCT (NCT04628338). However, manipulation of IFN-γ levels in AML patients should be carefully assessed, because systemic administration of IFN-γ is limited by rapid IFN-γ clearance and insufficient distribution to tumor sites. Moreover, while IFN-γ can restore T cell-mediated anti-cancer immunity and the surface expression of HLA class II molecules, the loss of which has been shown to impair AML recognition by donor T cells (170), IFN-γ is also capable of promoting PD-L1 and PD-L2 expression in AML (171, 172). Indeed, high expression of PD-L1 and PD-L2 is associated with poor OS in AML patients (173, 174). Binding of PD-L1/PD-L2 to the receptor PD-1 increases T cell exhaustion, promotes effector T cell apoptosis, induces the resistance to effector T cell-mediated killing (175) and increases the conversion and development of Tregs which have strong immune-suppressive abilities (176). Thus, the potential induction of PD-L1 and PD-L2 by IFN-γ may have unfavorable consequences, because the PD-1/PD-L1/PD-L2 axis helps the tumor to maintain an immunosuppressive microenvironment, thereby promoting immune evasion and survival of cancer cells (177). In addition to T cells, innate lymphoid cells type I (ILC1s) are another potent source of IFN-γ in healthy individuals. ILCs are important players of innate immune responses by reacting promptly to signals, or inducer cytokines, expressed by tissue-resident cells. ILC1s function as a first line of defense against intracellular pathogens, such as viruses, and tumors (178). By secreting IFN-γ, healthy ILC1s induce apoptosis and block differentiation by modulating JAK-STAT or PI3K/AKT signaling. However, in AML, ILC1s exhibit reduced IFN-γ secretion and lose their ability to suppress the development of LSCs and antagonize AML progression (179). ILC1s thus seem pivotal as an anti-cancer immune cell, and administration of ex vivo-expanded ILC1s could provide a new immunotherapeutic approach to ensure that IFN-y levels are locally increased within leukemic niches. Importantly, this approach would significantly decrease toxicity for AML patients in comparison to systemic delivery of IFN-y (179).
IL-4 is a signature cytokine of type II inflammation and regulates many aspects of Th2-mediated immunity (180). In epithelial cancers, IL-4 is generally considered to have pro-tumorigenic and pro-metastatic functions, suggesting that inhibition of the IL-4/IL-4R axis may be beneficial to limiting diseases (181, 182). Yet, in hematological cancers, a tumor-promoting role of IL-4 is controversial. Already in the early 1990s there were studies reporting that IL-4 might also have tumor-limiting functions, by suppressing IL-1-induced proliferation of AML cells (60, 64, 65). More recent findings substantiate those earlier observations and show that IL-4 has the potential to inhibit survival of AML cell lines as well as patient-derived AML cells, irrespective of their cytogenetic status and French-American-British (FAB) subtype, without affecting normal HSPCs. Anti-leukemic effects of IL-4 are at least partially dependent on STAT6 and Caspase-3, which agrees with the crucial role of STAT6 in mediating IL-4’s effects downstream of the IL-4 receptor (183). In addition, IL-4-induced STAT6, in cooperation with the nuclear receptor protein proliferator-activated receptor gamma (PPARγ), upregulates the expression of prostaglandins. In particular, COX (cyclooxygenase)-dependent prostaglandins, so-called CyPGs, play an important role in apoptosis (184). After stimulation by IL-4, these lipid mediators are increasingly produced via the COX/prostaglandin axis, which leads to activation of p53 and caspase-3 and subsequently stimulates apoptosis of leukemic cells (185). The fact that IL-4 treatment specifically acts on AML blasts, but does not affect HSCs, even upon long-term treatment, makes IL-4 an interesting candidate for therapeutic intervention in AML. Yet, despite its promising role as an anti-leukemic cytokine, IL-4 additionally promotes the differentiation of immune cells, including M2 macrophages, which are regarded as having a leukemia-supporting phenotype. M2 macrophages release various cytokines and growth factors that promote blast survival and proliferation, induce proangiogenic effects and can directly inhibit CD8+ T cell-mediated killing of blast cells (186, 187). Therefore, more detailed studies are required to assess the value of IL-4 as an antileukemic molecule.
IL-10, an anti-inflammatory cytokine produced by several immune cells, is crucial for limiting immune responses and damage caused by long-lasting inflammation (188). In AML patients, significantly higher levels of plasma IL-10 are observed (53, 56, 73) which directly correlate with prolonged overall patient survival, event-free survival and higher complete remission rates (39, 189, 190). In vitro, IL-10 treatment of AML blasts inhibited spontaneous AML blast proliferation and colony formation by negatively affecting the production and secretion of pro-leukemic cytokines (IL-1α, IL-1β, TNF-α, GM-CSF, GM-CSF, and IL-6) (74–76, 191). However, contrary to these findings, there is also evidence that IL-10 together with IL-35 — the latter a CD4+ and CD8+ T cell-suppressing and T regulatory cell (Treg)-supporting cytokine upregulated in AML (118) — promotes AML cell proliferation, survival and chemoresistance. So far, ICOS1+ and PD1+ Treg cells as well as BM-MSCs have been suggested as a source for IL-10 in the AML microenvironment (176, 192), contributing to the establishment of an IL-10-induced immunosuppressive and anti-inflammatory niche which ensures LSC survival and stemness (193, 194). Therefore, despite the direct correlation between IL-10 serum level and prolonged patient survival and treatment response (39, 189), antagonizing IL-10 signaling could support current chemotherapeutic approaches to irradicate LSCs and decrease the patient relapse rate (195). However, so far, no combinatory studies have been performed. Interestingly, Chen et al. recently highlighted the IL-10 receptor as a potential candidate for AML immunotherapy as it is significantly upregulated on AML cells in patients and is required for LSC stemness. CAR-T cells harboring an IL-10 peptide structure within their antigen-binding domain were shown to recognize and bind to the IL-10 receptor of multiple AML cell lines (MV4-11, Kasumi-1, U937, THP-1 and MOLM-13) and primary AML cells, thereby inducing the CAR T-cell mediated killing of these cells in vitro and in vivo (196). Although the function of IL-10 may be patient-dependent, immunomodulatory agents that block IL-10 could offer an interesting approach for treatment of AML.
More than a decade ago, IL-12p70 was shown to inhibit the angiogenic potential but not the survival or proliferation of AML cells (77) and to increase T-cell proliferation and cytotoxicity against leukemic cells in vitro (78, 79). Multiple in vitro co-culture studies overexpressing IL-12p70 in dendritic cells have confirmed these findings (197, 198) and paved the way for initial immunotherapies using genetically modified dendritic cells (phase 1 clinical trial NCT01734304) (199), thereby trying to avoid the toxicity of systemic administration of IL-12. Recently, another elegant therapeutic approach based on transplantation of genetically modified AML blasts constitutively expressing IL-12 in a vaccine-like manner was successfully established in murine and human cells (197) and is currently under investigation in a phase 1 clinical trial (NCT02483312).
Despite intensive research leading to new and targeted therapeutic strategies for AML in recent years, the prognosis for a large proportion of patients remains poor. Due to the highly heterogeneous nature of AML, current therapies often only eliminate specific subclones but cannot permanently halt disease progression. As in many other cancers, chronic inflammation, characterized by the release of pro-inflammatory cytokines and growth factors, which significantly influence the interaction of tumor and immune cells in the tumor microenvironment, can also be observed in AML. On the one hand these cytokines may ensure survival of cancer cells by promoting tumor cell proliferation while inhibiting the antitumor immune responses; on the other hand, some cytokines contribute to cancer cell elimination by supporting the body’s own immunological defense mechanisms. Therapeutic application of cytokines, or therapies that specifically target cytokines and/or their receptors, may provide new avenues for the treatment of AML patients in the coming years. In particular, IL-1β, IL-6 and CXCL12 might be promising new druggable targets. However, due to the pleiotropic effects of most cytokines, which control both tumor growth and anti-tumor immune responses, we are faced with the challenge of establishing new therapies, which on the one hand inhibit tumor growth and at the same time specifically enhance the anti-tumor response. The use of combination therapies, in which potential inhibitors of inflammatory cytokines are combined with other therapeutic molecules and agents, may prove promising for this purpose.
Conceptualization, JH-H, ML, PWK, writing—original draft preparation, ML and PWK, writing—review and editing, JH-H, visualization, ML and funding acquisition, JH-H. All authors contributed to the article and approved the submitted version.
This work was supported by the County of Salzburg, Cancer Cluster Salzburg [grant number 20102-P1601064-FPR01-2017], the Austrian Science Fund (FWF) [grant numbers W1213 and P33969], the Biomed Center Salzburg (project 20102-F1901165-KZP), the European Interreg project EPIC (grant number ITAT1054), and by the Priority program ACBN, University of Salzburg.
The authors declare that the research was conducted in the absence of any commercial or financial relationships that could be construed as a potential conflict of interest.
All claims expressed in this article are solely those of the authors and do not necessarily represent those of their affiliated organizations, or those of the publisher, the editors and the reviewers. Any product that may be evaluated in this article, or claim that may be made by its manufacturer, is not guaranteed or endorsed by the publisher.
1. Dohner H, Weisdorf DJ, Bloomfield CD. Acute myeloid leukemia. N Engl J Med (2015) 373(12):1136–52. doi: 10.1056/NEJMra1406184
2. Estey E, Döhner H. Acute myeloid leukaemia. Lancet (London England) (2006) 368(9550):1894–907. doi: 10.1016/S0140-6736(06)69780-8
3. Pelcovits A, Niroula R. Acute myeloid leukemia: A review. Rhode Island Med J (2013) (2020) 103(3):38–40.
4. Papayannidis C, Sartor C, Marconi G, Fontana MC, Nanni J, Cristiano G, et al. Acute myeloid leukemia mutations: Therapeutic implications. Int J Mol Sci (2019) 20(11):2721. doi: 10.3390/ijms20112721
5. Noone AM HN, Krapcho M, Miller D, Brest A, Yu M, Ruhl J, Tatalovich Z, Mariotto A, Lewis DR, Chen HS, Feuer EJ, Cronin KA eds. SEER cancer statistics review, 1975-2015. Bethesda, MD: National Cancer Institute (2018). Available at: https://seer.cancer.gov/csr/1975_2015/.
6. Prada-Arismendy J, Arroyave JC, Rothlisberger S. Molecular biomarkers in acute myeloid leukemia. Blood Rev (2017) 31(1):63–76. doi: 10.1016/j.blre.2016.08.005
7. De Kouchkovsky I, Abdul-Hay M. Acute myeloid leukemia: a comprehensive review and 2016 update Blood Cancer J (2016) 6(7):e441. doi: 10.1038/bcj.2016.50
8. Narayanan D, Weinberg OK. How I investigate acute myeloid leukemia. Int J Lab Hematol (2020) 42(1):3–15. doi: 10.1111/ijlh.13135
9. Krause DS, Scadden DT. A hostel for the hostile: the bone marrow niche in hematologic neoplasms Haematologica. (2015) 100(11):1376–87. doi: 10.3324/haematol.2014.113852
10. Lane SW, Scadden DT, Gilliland DG. The leukemic stem cell niche: current concepts and therapeutic opportunities. Blood (2009) 114(6):1150–7. doi: 10.1182/blood-2009-01-202606
11. Schepers K, Campbell TB, Passegué E. Normal and leukemic stem cell niches: insights and therapeutic opportunities. Cell Stem Cell (2015) 16(3):254–67. doi: 10.1016/j.stem.2015.02.014
12. Bhatia R, McGlave PB, Dewald GW, Blazar BR, Verfaillie CM. Abnormal function of the bone marrow microenvironment in chronic myelogenous leukemia: role of malignant stromal macrophages. Blood (1995) 85(12):3636–45. doi: 10.1182/blood.V85.12.3636.bloodjournal85123636
13. Somervaille TC, Cleary ML. Identification and characterization of leukemia stem cells in murine MLL-AF9 acute myeloid leukemia. Cancer Cell (2006) 10(4):257–68. doi: 10.1016/j.ccr.2006.08.020
14. Reynaud D, Pietras E, Barry-Holson K, Mir A, Binnewies M, Jeanne M, et al. IL-6 controls leukemic multipotent progenitor cell fate and contributes to chronic myelogenous leukemia development. Cancer Cell (2011) 20(5):661–73. doi: 10.1016/j.ccr.2011.10.012
15. Schemionek M, Spieker T, Kerstiens L, Elling C, Essers M, Trumpp A, et al. Leukemic spleen cells are more potent than bone marrow-derived cells in a transgenic mouse model of CML. Leukemia (2012) 26(5):1030–7. doi: 10.1038/leu.2011.366
16. Krenn PW, Montanez E, Costell M, Fässler R. Integrins, anchors and signal transducers of hematopoietic stem cells during development and in adulthood. Curr Top Dev Biol (2022) 149:203–61. doi: 10.1016/bs.ctdb.2022.02.009
17. Boettcher S, Manz MG. Regulation of inflammation- and infection-driven hematopoiesis. Trends Immunol (2017) 38(5):345–57. doi: 10.1016/j.it.2017.01.004
18. Pietras EM, Mirantes-Barbeito C, Fong S, Loeffler D, Kovtonyuk LV, Zhang S, et al. Chronic interleukin-1 exposure drives haematopoietic stem cells towards precocious myeloid differentiation at the expense of self-renewal. Nat Cell Biol (2016) 18(6):607–18. doi: 10.1038/ncb3346
19. Weber GF, Chousterman BG, He S, Fenn AM, Nairz M, Anzai A, et al. Interleukin-3 amplifies acute inflammation and is a potential therapeutic target in sepsis. Science (2015) 347(6227):1260–5. doi: 10.1126/science.aaa4268
21. Ishihara K, Hirano T. IL-6 in autoimmune disease and chronic inflammatory proliferative disease. Cytokine Growth Factor Rev (2002) 13(4-5):357–68. doi: 10.1016/S1359-6101(02)00027-8
22. Reynaud D, Pietras E, Barry-Holson K, Mir A, Binnewies M, Jeanne M, et al. IL-6 controls leukemic multipotent progenitor cell fate and contributes to chronic myelogenous leukemia development. Cancer Cell (2011) 20(5):661–73. doi: 10.1016/j.ccr.2011.10.012
23. Maeda K, Baba Y, Nagai Y, Miyazaki K, Malykhin A, Nakamura K, et al. IL-6 blocks a discrete early step in lymphopoiesis. Blood (2005) 106(3):879–85. doi: 10.1182/blood-2005-02-0456
24. Tian T, Wang M, Ma D. TNF-alpha, a good or bad factor in hematological diseases? Stem Cell Investig (2014) 1:12. doi: 10.3978/j.issn.2306-9759.2014.04.02.
25. Selleri C, Maciejewski JP, Sato T, Young NS. Interferon-gamma constitutively expressed in the stromal microenvironment of human marrow cultures mediates potent hematopoietic inhibition. Blood. (1996) 87(10):4149–57. doi: 10.1182/blood.V87.10.4149.bloodjournal87104149
26. Jacobsen SE, Ruscetti FW, Dubois CM, Keller JR. Tumor necrosis factor alpha directly and indirectly regulates hematopoietic progenitor cell proliferation: role of colony-stimulating factor receptor modulation. J Exp Med (1992) 175(6):1759–72. doi: 10.1084/jem.175.6.1759
27. Rezzoug F, Huang Y, Tanner MK, Wysoczynski M, Schanie CL, Chilton PM, et al. TNF-alpha is critical to facilitate hemopoietic stem cell engraftment and function. J Immunol (2008) 180(1):49–57. doi: 10.4049/jimmunol.180.1.49
28. Baldridge MT, King KY, Boles NC, Weksberg DC, Goodell MA. Quiescent haematopoietic stem cells are activated by IFN-gamma in response to chronic infection. Nature (2010) 465(7299):793–7. doi: 10.1038/nature09135
29. Essers MA, Offner S, Blanco-Bose WE, Waibler Z, Kalinke U, Duchosal MA, et al. IFNalpha activates dormant haematopoietic stem cells in vivo. Nature (2009) 458(7240):904–8. doi: 10.1038/nature07815
30. Ushach I, Zlotnik A. Biological role of granulocyte macrophage colony-stimulating factor (GM-CSF) and macrophage colony-stimulating factor (M-CSF) on cells of the myeloid lineage. J Leukoc Biol (2016) 100(3):481–9. doi: 10.1189/jlb.3RU0316-144R
31. Mirantes C, Passegue E, Pietras EM. Pro-inflammatory cytokines: emerging players regulating HSC function in normal and diseased hematopoiesis. Exp Cell Res (2014) 329(2):248–54. doi: 10.1016/j.yexcr.2014.08.017
32. Hemmati S, Haque T, Gritsman K. Inflammatory signaling pathways in preleukemic and leukemic stem cells. Front Oncol (2017) 7:265. doi: 10.3389/fonc.2017.00265
33. King KY, Goodell MA. Inflammatory modulation of HSCs: viewing the HSC as a foundation for the immune response. Nat Rev Immunol (2011) 11(10):685–92. doi: 10.1038/nri3062
34. Camacho V, McClearn V, Patel S, Welner RS. Regulation of normal and leukemic stem cells through cytokine signaling and the microenvironment. Int J Hematol (2017) 105(5):566–77. doi: 10.1007/s12185-017-2184-6
35. Vilchis-Ordoñez A, Contreras-Quiroz A, Vadillo E, Dorantes-Acosta E, Reyes-López A, Quintela-Nuñez del Prado HM, et al. Bone marrow cells in acute lymphoblastic leukemia create a proinflammatory microenvironment influencing normal hematopoietic differentiation fates. BioMed Res Int (2015) 2015:386165. doi: 10.1155/2015/386165
36. Hoermann G, Greiner G, Valent P. Cytokine regulation of microenvironmental cells in myeloproliferative neoplasms. Mediators Inflamm (2015) 2015:869242. doi: 10.1155/2015/869242
37. Kiss C, Benko I, Kovács P. Leukemic cells and the cytokine patchwork. Pediatr Blood Cancer (2004) 42(2):113–21. doi: 10.1002/pbc.10436
38. Pietras EM. Inflammation: a key regulator of hematopoietic stem cell fate in health and disease. Blood (2017) 130(15):1693–8. doi: 10.1182/blood-2017-06-780882
39. Sanchez-Correa B, Bergua JM, Campos C, Gayoso I, Arcos MJ, Banas H, et al. Cytokine profiles in acute myeloid leukemia patients at diagnosis: survival is inversely correlated with IL-6 and directly correlated with IL-10 levels. Cytokine (2013) 61(3):885–91. doi: 10.1016/j.cyto.2012.12.023
40. Ahmed HA, Maklad AM, Khaled SA, Elyamany A. Interleukin-27 and interleukin-35 in de novo acute myeloid leukemia: expression and significance as biological markers. J Blood Med (2019) 10:341–9. doi: 10.2147/JBM.S221301
41. Carey A, DKt E, CA E, Newell L, Traer E, BC M, et al. Identification of interleukin-1 by functional screening as a key mediator of cellular expansion and disease progression in acute myeloid leukemia. Cell Rep (2017) 18(13):3204–18. doi: 10.1016/j.celrep.2017.03.018
42. Tao M, Li B, Nayini J, Andrews CB, Huang RW, Devemy E, et al. SCF, IL-1beta, IL-1ra and GM-CSF in the bone marrow and serum of normal individuals and of AML and CML patients. Cytokine (2000) 12(6):699–707. doi: 10.1006/cyto.2000.0666
43. Elbaz O, Shaltout A. Implication of granulocyte-macrophage colony stimulating factor (GM-CSF) and interleukin-3 (IL-3) in children with acute myeloid leukaemia (AML); malignancy. Hematology (2001) 5(5):383–8.
44. Islam M, Mohamed EH, Esa E, Kamaluddin NR, Zain SM, Yusoff YM, et al. Circulating cytokines and small molecules follow distinct expression patterns in acute myeloid leukaemia. Br J Cancer (2017) 117(10):1551–6. doi: 10.1038/bjc.2017.316
45. Carlo-Stella C, Mangoni L, Almici C, Frassoni F, Fiers W, Rizzoli V. Growth of CD34+ acute myeloblastic leukemia colony-forming cells in response to recombinant hematopoietic growth factors. Leukemia (1990) 4(8):561–6.
46. Delwel R, Salem M, Pellens C, Dorssers L, Wagemaker G, Clark S, et al. Growth regulation of human acute myeloid leukemia: effects of five recombinant hematopoietic factors in a serum-free culture system. Blood (1988) 72(6):1944–9. doi: 10.1182/blood.V72.6.1944.1944
47. Pebusque MJ, Fay C, Lafage M, Sempere C, Saeland S, Caux C, et al. Recombinant human IL-3 and G-CSF act synergistically in stimulating the growth of acute myeloid leukemia cells. Leukemia (1989) 3(3):200–5.
48. Suzuki T, Morio T, Tohda S, Nagata K, Yamashita Y, Imai Y, et al. Effects of interleukin-6 and granulocyte colony-stimulating factor on the proliferation of leukemic blast progenitors from acute myeloblastic leukemia patients. Jpn J Cancer Res (1990) 81(10):979–86. doi: 10.1111/j.1349-7006.1990.tb03335.x
49. Kassem NM, Ayad AM, El Husseiny NM, El-Demerdash DM, Kassem HA, Mattar MM. Role of granulocyte-macrophage colony-stimulating factor in acute myeloid Leukemia/Myelodysplastic syndromes. J Glob Oncol (2018) 4:1–6. doi: 10.1200/JGO.2017.009332
50. Hoang T, Haman A, Goncalves O, Letendre F, Mathieu M, Wong GG, et al. Interleukin 1 enhances growth factor-dependent proliferation of the clonogenic cells in acute myeloblastic leukemia and of normal human primitive hemopoietic precursors. J Exp Med (1988) 168(2):463–74. doi: 10.1084/jem.168.2.463
51. Carter A, Silvian-Draxler I, Tatarsky I. Effect of interleukin-1, tumor necrosis factor-alpha, and interferon-alpha on the blast cells of acute myeloblastic leukemia. Am J Hematol (1992) 40(4):245–51. doi: 10.1002/ajh.2830400402
52. Sissolak G, Hoffbrand AV, Mehta AB, Ganeshaguru K. Effects of interferon-alpha (IFN) on the expression of interleukin 1-beta (IL-1), interleukin 6 (IL-6), granulocyte-macrophage colony-stimulating factor (GM-CSF) and tumor necrosis factor-alpha (TNF) in acute myeloid leukemia (AML) blasts. Leukemia. (1992) 6(11):1155–60.
53. Sun YX, Kong HL, Liu CF, Yu S, Tian T, Ma DX, et al. The imbalanced profile and clinical significance of T helper associated cytokines in bone marrow microenvironment of the patients with acute myeloid leukemia. Hum Immunol (2014) 75(2):113–8. doi: 10.1016/j.humimm.2013.11.014
54. Corradi G, Bassani B, Simonetti G, Sangaletti S, Vadakekolathu J, Fontana MC, et al. Release of IFN-γ by acute myeloid leukemia cells remodels bone marrow immune microenvironment by inducing regulatory T cells. Clin Cancer Res (2022) 28(14):3141–55. doi: 10.1158/1078-0432.CCR-21-3594
55. Ersvaer E, Skavland J, Ulvestad E, Gjertsen BT, Bruserud Ø. Effects of interferon gamma on native human acute myelogenous leukaemia cells. Cancer Immunol Immunother. (2007) 56(1):13–24. doi: 10.1007/s00262-006-0159-1
56. Tsimberidou AM, Estey E, Wen S, Pierce S, Kantarjian H, Albitar M, et al. The prognostic significance of cytokine levels in newly diagnosed acute myeloid leukemia and high-risk myelodysplastic syndromes. Cancer. (2008) 113(7):1605–13. doi: 10.1002/cncr.23785
57. Yin M, Gopal V, Banavali S, Gartside P, Preisler H. Effects of an IL-1 receptor antagonist on acute myeloid leukemia cells. Leukemia (1992) 6(9):898–901.
58. Estrov Z, Kurzrock R, Estey E, Wetzler M, Ferrajoli A, Harris D, et al. Inhibition of acute myelogenous leukemia blast proliferation by interleukin-1 (IL-1) receptor antagonist and soluble IL-1 receptors. Blood (1992) 79(8):1938–45. doi: 10.1182/blood.V79.8.1938.1938
59. Cozzolino F, Rubartelli A, Aldinucci D, Sitia R, Torcia M, Shaw A, et al. Interleukin 1 as an autocrine growth factor for acute myeloid leukemia cells. Proc Natl Acad Sci USA. (1989) 86(7):2369–73. doi: 10.1073/pnas.86.7.2369
60. Wagteveld AJ, Esselink MT, Limburg P, Halie MR, Vellenga E. The effects of IL-1 beta and IL-4 on the proliferation and endogenous secretion of growth factors by acute myeloblastic leukemic cells. Leukemia (1992) 6(10):1020–4.
61. Delwel R, Dorssers L, Touw I, Wagemaker G, Lowenberg B. Human recombinant multilineage colony stimulating factor (interleukin-3): stimulator of acute myelocytic leukemia progenitor cells in vitro. Blood (1987) 70(1):333–6. doi: 10.1182/blood.V70.1.333.333
62. Lemoli RM, Gulati SC, Strife A, Lambek C, Perez A, Clarkson BD. Proliferative response of human acute myeloid leukemia cells and normal marrow enriched progenitor cells to human recombinant growth factors IL-3, GM-CSF and G-CSF alone and in combination. Leukemia (1991) 5(5):386–91.
63. Santoli D, Yang YC, Clark SC, Kreider BL, Caracciolo D, Rovera G. Synergistic and antagonistic effects of recombinant human interleukin (IL) 3, IL-1 alpha, granulocyte and macrophage colony-stimulating factors (G-CSF and m-CSF) on the growth of GM-CSF-dependent leukemic cell lines. J Immunol (1987) 139(10):3348–54.
64. Tuyt LM, Dokter WH, Esselink MT, Vellenga E. Divergent effects of IL-10 and IL-4 on the proliferation and growth factor secretion by acute myeloblastic leukemic cells. Eur Cytokine Netw (1995) 6(4):231–5.
65. Akashi K. The role of interleukin-4 in the negative regulation of leukemia cell growth. Leuk Lymphoma (1993) 9(3):205–9. doi: 10.3109/10428199309147371
66. Negaard HF, Iversen N, Bowitz-Lothe IM, Sandset PM, Steinsvik B, Ostenstad B, et al. Increased bone marrow microvascular density in haematological malignancies is associated with differential regulation of angiogenic factors. Leukemia (2009) 23(1):162–9. doi: 10.1038/leu.2008.255
67. Sugiyama H, Inoue K, Ogawa H, Yamagami T, Soma T, Miyake S, et al. The expression of IL-6 and its related genes in acute leukemia. Leuk Lymphoma (1996) 21(1-2):49–52. doi: 10.3109/10428199609067579
68. Hoang T, Haman A, Goncalves O, Wong GG, Clark SC. Interleukin-6 enhances growth factor-dependent proliferation of the blast cells of acute myeloblastic leukemia. Blood (1988) 72(2):823–6. doi: 10.1182/blood.V72.2.823.823
69. Säily M, Koistinen P, Zheng A, Savolainen ER. Signaling through interleukin-6 receptor supports blast cell proliferation in acute myeloblastic leukemia Eur J Haematol (1998) 61(3):190–6. doi: 10.1111/j.1600-0609.1998.tb01083.x.
70. Lopez M, Maroc N, Kerangueven F, Bardin F, Courcoul M, Lavezzi C, et al. Coexpression of the genes for interleukin 6 and its receptor without apparent involvement in the proliferation of acute myeloid leukemia cells. Exp Hematol (1991) 19(8):797–803.
71. Suzuki T, Bessho M, Hirashma K, Tohda S, Nagata K, Imai Y, et al. Interleukin-6 reduces the optimal growth in vitro of leukemic blast progenitors from acute myeloblastic leukemia patients. Acta Haematol (1992) 87(1-2):63–8. doi: 10.1159/000204718
72. Çelik H, Lindblad KE, Popescu B, Gui G, Goswami M, Valdez J, et al. Highly multiplexed proteomic assessment of human bone marrow in acute myeloid leukemia. Blood Adv (2020) 4(2):367–79. doi: 10.1182/bloodadvances.2019001124
73. Wu H, Li P, Shao N, Ma J, Ji M, Sun X, et al. Aberrant expression of treg-associated cytokine IL-35 along with IL-10 and TGF-β in acute myeloid leukemia. Oncol Lett (2012) 3(5):1119–23. doi: 10.3892/ol.2012.614
74. Westermann F, Kube D, Haier B, Bohlen H, Engert A, Zuehlsdorf M, et al. Interleukin 10 inhibits cytokine production of human AML cells. Ann Oncol (1996) 7(4):397–404. doi: 10.1093/oxfordjournals.annonc.a010607
75. Bruserud O, Tore Gjertsen B, Brustugun OT, Bassøe CF, Nesthus I, Espen Akselsen P, et al. Effects of interleukin 10 on blast cells derived from patients with acute myelogenous leukemia. Leukemia (1995) 9(11):1910–20.
76. Asano Y, Shibata S, Kobayashi S, Okamura S, Niho Y. Interleukin-10 inhibits the autocrine growth of leukemic blast cells from patients with acute myeloblastic leukemia. Int J Hematol (1997) 66(4):445–50. doi: 10.1016/S0925-5710(97)00070-4
77. Ferretti E, Di Carlo E, Cocco C, Ribatti D, Sorrentino C, Ognio E, et al. Direct inhibition of human acute myeloid leukemia cell growth by IL-12. Immunol Lett (2010) 133(2):99–105. doi: 10.1016/j.imlet.2010.08.002
78. Orleans-Lindsay JK, Deru A, Craig JI, Prentice HG, Lowdell MW. In vitro co-stimulation with anti-CD28 synergizes with IL-12 in the generation of T cell immune responses to leukaemic cells; a strategy for ex-vivo generation of CTL for immunotherapy. Clin Exp Immunol (2003) 133(3):467–75. doi: 10.1046/j.1365-2249.2003.02235.x
79. Pan L, Ohnishi K, Zhang WJ, Yoshida H, Maksumova L, Muratkhodjaev F, et al. In vitro IL-12 treatment of peripheral blood mononuclear cells from patients with leukemia or myelodysplastic syndromes: increase in cytotoxicity and reduction in WT1 gene expression. Leukemia (2000) 14(9):1634–41. doi: 10.1038/sj.leu.2401872
80. Tao Q, Pan Y, Wang Y, Wang H, Xiong S, Li Q, et al. Regulatory T cells-derived IL-35 promotes the growth of adult acute myeloid leukemia blasts. Int J Cancer (2015) 137(10):2384–93. doi: 10.1002/ijc.29563
81. Wang J, Tao Q, Wang H, Wang Z, Wu F, Pan Y, et al. Elevated IL-35 in bone marrow of the patients with acute myeloid leukemia. Hum Immunol (2015) 76(9):681–6. doi: 10.1016/j.humimm.2015.09.020
82. Liersch R, Gerss J, Schliemann C, Bayer M, Schwöppe C, Biermann C, et al. Osteopontin is a prognostic factor for survival of acute myeloid leukemia patients. Blood (2012) 119(22):5215–20. doi: 10.1182/blood-2011-11-389692
83. Lee CY, Tien HF, Hou HA, Chou WC, Lin LI. Marrow osteopontin level as a prognostic factor in acute myeloid leukaemia. Br J Haematol (2008) 141(5):736–9. doi: 10.1111/j.1365-2141.2008.07082.x
84. Zhou J, Chen X, Zhou P, Sun X, Chen Y, Li M, et al. Osteopontin is required for the maintenance of leukemia stem cells in acute myeloid leukemia. Biochem Biophys Res Commun (2022) 600:29–34. doi: 10.1016/j.bbrc.2022.02.022
85. Carlesso N, Pregno P, Bresso P, Gallo E, Pileri A, Zsebo KM, et al. Human recombinant stem cell factor stimulates in vitro proliferation of acute myeloid leukemia cells and expands the clonogenic cell pool. Leukemia (1992) 6(7):642–8.
86. Hassan HT, Zander A. Stem cell factor as a survival and growth factor in human normal and malignant hematopoiesis. Acta Haematol (1996) 95(3-4):257–62. doi: 10.1159/000203893
87. Bendall LJ, Makrynikola V, Hutchinson A, Bianchi AC, Bradstock KF, Gottlieb DJ. Stem cell factor enhances the adhesion of AML cells to fibronectin and augments fibronectin-mediated anti-apoptotic and proliferative signals. Leukemia (1998) 12(9):1375–82. doi: 10.1038/sj.leu.2401136
88. Wu Y, Chen P, Huang HF, Huang MJ, Chen YZ. Reduction of transforming growth factor-β1 expression in leukemia and its possible role in leukemia development. Leuk Lymphoma (2012) 53(1):145–51. doi: 10.3109/10428194.2011.603446
89. Nara N, Tohda S, Nagata K, Suzuki T, Yamashita Y. Inhibition of the in vitro growth of blast progenitors from acute myeloblastic leukemia patients by transforming growth factor-beta (TGF-beta). Leukemia (1989) 3(8):572–7.
90. Tessier N, Hoang T. Transforming growth factor beta inhibits the proliferation of the blast cells of acute myeloblastic leukemia. Blood (1988) 72(1):159–64. doi: 10.1182/blood.V72.1.159.159
91. Zhou X, Zhou S, Li B, Li Q, Gao L, Li D, et al. Transmembrane TNF-α preferentially expressed by leukemia stem cells and blasts is a potent target for antibody therapy. Blood (2015) 126(12):1433–42. doi: 10.1182/blood-2015-01-624833
92. Sison EA, Magoon D, Li L, Annesley CE, Rau RE, Small D, et al. Plerixafor as a chemosensitizing agent in pediatric acute lymphoblastic leukemia: efficacy and potential mechanisms of resistance to CXCR4 inhibition. Oncotarget (2014) 5(19):8947–58. doi: 10.18632/oncotarget.2407
93. Yu X, Munoz-Sagredo L, Streule K, Muschong P, Bayer E, Walter RJ, et al. CD44 loss of function sensitizes AML cells to the BCL-2 inhibitor venetoclax by decreasing CXCL12-driven survival cues. Blood (2021) 138(12):1067–80. doi: 10.1182/blood.2020006343
94. Wang SS, Xu ZJ, Jin Y, Ma JC, Xia PH, Wen X, et al. Clinical and prognostic relevance of CXCL12 expression in acute myeloid leukemia. PeerJ (2021) 9:e11820. doi: 10.7717/peerj.11820
95. Mehrpouri M. The contributory roles of the CXCL12/CXCR4/CXCR7 axis in normal and malignant hematopoiesis: A possible therapeutic target in hematologic malignancies. Eur J Pharmacol (2022) 920:174831. doi: 10.1016/j.ejphar.2022.174831
96. Cho BS, Kim HJ, Konopleva M. Targeting the CXCL12/CXCR4 axis in acute myeloid leukemia: from bench to bedside. Korean J Intern Med (2017) 32(2):248–57. doi: 10.3904/kjim.2016.244
97. Peled A, Klein S, Beider K, Burger JA, Abraham M. Role of CXCL12 and CXCR4 in the pathogenesis of hematological malignancies. Cytokine (2018) 109:11–6. doi: 10.1016/j.cyto.2018.02.020
98. Ladikou EE, Chevassut T, Pepper CJ, Pepper AG. Dissecting the role of the CXCL12/CXCR4 axis in acute myeloid leukaemia. Br J Haematol (2020) 189(5):815–25. doi: 10.1111/bjh.16456
99. Rodriguez-Cimadevilla JC, Beauchemin V, Villeneuve L, Letendre F, Shaw A, Hoang T. Coordinate secretion of interleukin-1 beta and granulocyte-macrophage colony-stimulating factor by the blast cells of acute myeloblastic leukemia: role of interleukin-1 as an endogenous inducer. Blood (1990) 76(8):1481–9. doi: 10.1182/blood.V76.8.1481.1481
100. Russell NH. Autocrine growth factors and leukaemic haemopoiesis. Blood Rev (1992) 6(3):149–56. doi: 10.1016/0268-960X(92)90026-M
101. Murohashi I, Tohda S, Suzuki T, Nagata K, Yamashita Y, Nara N. Autocrine growth mechanisms of the progenitors of blast cells in acute myeloblastic leukemia Blood (1989) 74(1):35–41. doi: 10.1182/blood.V74.1.35.35
102. Delwel R, Schipper P, van Buitenen C, van Agthoven T, Touw I, Löwenberg B. Comparative analysis of IL-1 regulated and spontaneous growth of acute myeloid leukemia in vitro. Bone marrow Transplant (1990) 6 Suppl 1:22–6.
103. Bradbury D, Rogers S, Reilly IA, Kozlowski R, Russell NH. Role of autocrine and paracrine production of granulocyte-macrophage colony-stimulating factor and interleukin-1 beta in the autonomous growth of acute myeloblastic leukaemia cells–studies using purified CD34-positive cells. Leukemia (1992) 6(6):562–6.
104. Oster W, Cicco NA, Klein H, Hirano T, Kishimoto T, Lindemann A, et al. Participation of the cytokines interleukin 6, tumor necrosis factor-alpha, and interleukin 1-beta secreted by acute myelogenous leukemia blasts in autocrine and paracrine leukemia growth control. J Clin Invest (1989) 84(2):451–7. doi: 10.1172/JCI114186
105. Beauchemin V, Villeneuve L, Rodriguez-Cimadevilla JC, Rajotte D, Kenney JS, Clark SC, et al. Interleukin-6 production by the blast cells of acute myeloblastic leukemia: regulation by endogenous interleukin-1 and biological implications. J Cell Physiol (1991) 148(3):353–61. doi: 10.1002/jcp.1041480305
106. Delwel R, van Buitenen C, Salem M, Oosterom R, Touw I, Löwenberg B. Hemopoietin-1 activity of interleukin-1 (IL-1) on acute myeloid leukemia colony-forming cells (AML-CFU) in vitro: IL-1 induces production of tumor necrosis factor-alpha which synergizes with IL-3 or granulocyte-macrophage colony-stimulating factor. Leukemia (1990) 4(8):557–60.
107. Tsuzuki M, Ezaki K, Maruyama F, Ino T, Kojima H, Okamoto M, et al. Proliferative effects of several hematopoietic growth factors on acute myelogenous leukemia cells and correlation with treatment outcome. Leukemia (1997) 11(12):2125–30. doi: 10.1038/sj.leu.2400870
108. Russell NH, Hunter AE, Bradbury D, Zhu YM, Keith F. Biological features of leukaemic cells associated with autonomous growth and reduced survival in acute myeloblastic leukaemia. Leuk Lymphoma (1995) 16(3-4):223–9. doi: 10.3109/10428199509049761
109. Lowenberg B, van Putten WL, Touw IP, Delwel R, Santini V. Autonomous proliferation of leukemic cells in vitro as a determinant of prognosis in adult acute myeloid leukemia N Engl J Med (1993) 328(9):614–9. doi: 10.1056/NEJM199303043280904
110. Pimenta DB, Varela VA, Datoguia TS, Caraciolo VB, Lopes GH, Pereira WO. The bone marrow microenvironment mechanisms in acute myeloid leukemia. Front Cell Dev Biol (2021) 9:764698. doi: 10.3389/fcell.2021.764698
111. Nilsson SK, Johnston HM, Whitty GA, Williams B, Webb RJ, Denhardt DT, et al. Osteopontin, a key component of the hematopoietic stem cell niche and regulator of primitive hematopoietic progenitor cells. Blood (2005) 106(4):1232–9. doi: 10.1182/blood-2004-11-4422
112. Battula VL, Le PM, Sun JC, Nguyen K, Yuan B, Zhou X, et al. AML-induced osteogenic differentiation in mesenchymal stromal cells supports leukemia growth. JCI Insight (2017) 2(13):e90036. doi: 10.1172/jci.insight.90036
113. Zahed Panah M, Nikbakht M, Sajjadi SM, Rostami S, Norooznezhad AH, Kamranzadeh Fumani H, et al. Anti-apoptotic effects of osteopontin via the up-regulation of AKT/mTOR/β-catenin loop in acute myeloid leukemia cells. Int J Hematol Oncol Stem Cell Res (2017) 11(2):148–57.
114. Bandopadhyay M, Bulbule A, Butti R, Chakraborty G, Ghorpade P, Ghosh P, et al. Osteopontin as a therapeutic target for cancer. Expert Opin Ther Targets (2014) 18(8):883–95. doi: 10.1517/14728222.2014.925447
115. Mantovani A, Dinarello CA, Molgora M, Garlanda C. Interleukin-1 and related cytokines in the regulation of inflammation and immunity. Immunity (2019) 50(4):778–95. doi: 10.1016/j.immuni.2019.03.012
116. Mantovani A, Ponzetta A, Inforzato A, Jaillon S. Innate immunity, inflammation and tumour progression: double-edged swords. J Intern Med (2019) 285(5):524–32. doi: 10.1111/joim.12886
117. Arranz L, Arriero MDM, Villatoro A. Interleukin-1beta as emerging therapeutic target in hematological malignancies and potentially in their complications. Blood Rev (2017) 31(5):306–17. doi: 10.1016/j.blre.2017.05.001
118. Binder S, Luciano M, Horejs-Hoeck J. The cytokine network in acute myeloid leukemia (AML): A focus on pro- and anti-inflammatory mediators. Cytokine Growth Factor Rev (2018) 43:8–15. doi: 10.1016/j.cytogfr.2018.08.004
119. Zhang W, Borcherding N, Kolb R. IL-1 signaling in tumor microenvironment. Adv Exp Med Biol (2020) 1240:1–23. doi: 10.1007/978-3-030-38315-2_1
120. de Mooij CEM, Netea MG, van der Velden W, Blijlevens NMA. Targeting the interleukin-1 pathway in patients with hematological disorders. Blood (2017) 129(24):3155–64. doi: 10.1182/blood-2016-12-754994
121. Wang Y, Sun X, Yuan S, Hou S, Guo T, Chu Y, et al. Interleukin-1β inhibits normal hematopoietic expansion and promotes acute myeloid leukemia progression via the bone marrow niche. Cytotherapy (2020) 22(3):127–34. doi: 10.1016/j.jcyt.2020.01.001
122. Höckendorf U, Yabal M, Herold T, Munkhbaatar E, Rott S, Jilg S, et al. RIPK3 restricts myeloid leukemogenesis by promoting cell death and differentiation of leukemia initiating cells. Cancer Cell (2016) 30(1):75–91. doi: 10.1016/j.ccell.2016.06.002
123. Barreyro L, Will B, Bartholdy B, Zhou L, Todorova TI, Stanley RF, et al. Overexpression of IL-1 receptor accessory protein in stem and progenitor cells and outcome correlation in AML and MDS. Blood (2012) 120(6):1290–8. doi: 10.1182/blood-2012-01-404699
124. De Boer B, Sheveleva S, Apelt K, Vellenga E, Mulder AB, Huls G, et al. The IL1-IL1RAP axis plays an important role in the inflammatory leukemic niche that favors acute myeloid leukemia proliferation over normal hematopoiesis. Haematologica (2020) 106(12):3067–78. doi: 10.3324/haematol.2020.254987
125. Svensson EC, Madar A, Campbell CD, He Y, Sultan M, Healey ML, et al. TET2-driven clonal hematopoiesis and response to canakinumab: An exploratory analysis of the CANTOS randomized clinical trial. JAMA Cardiol (2022) 7(5):521–8. doi: 10.1001/jamacardio.2022.0386
126. Wong CC, Baum J, Silvestro A, Beste MT, Bharani-Dharan B, Xu S, et al. Inhibition of IL1β by canakinumab may be effective against diverse molecular subtypes of lung cancer: An exploratory analysis of the CANTOS trial. Cancer Res (2020) 80(24):5597–605. doi: 10.1158/0008-5472.CAN-19-3176
127. Tanaka T, Narazaki M, Kishimoto T. IL-6 in inflammation, immunity, and disease. Cold Spring Harbor Perspect Biol (2014) 6(10):a016295. doi: 10.1101/cshperspect.a016295
128. Leary AG, Ikebuchi K, Hirai Y, Wong GG, Yang YC, Clark SC, et al. Synergism between interleukin-6 and interleukin-3 in supporting proliferation of human hematopoietic stem cells: comparison with interleukin-1 alpha. Blood (1988) 71(6):1759–63. doi: 10.1182/blood.V71.6.1759.1759
129. Ikebuchi K, Wong GG, Clark SC, Ihle JN, Hirai Y, Ogawa M. Interleukin 6 enhancement of interleukin 3-dependent proliferation of multipotential hemopoietic progenitors. Proc Natl Acad Sci USA (1987) 84(24):9035–9. doi: 10.1073/pnas.84.24.9035
130. Yao X, Huang J, Zhong H, Shen N, Faggioni R, Fung M, et al. Targeting interleukin-6 in inflammatory autoimmune diseases and cancers. Pharmacol Ther (2014) 141(2):125–39. doi: 10.1016/j.pharmthera.2013.09.004
131. Inoue K, Sugiyama H, Ogawa H, Yamagami T, Azuma T, Oka Y, et al. Expression of the interleukin-6 (IL-6), IL-6 receptor, and gp130 genes in acute leukemia. Blood (1994) 84(8):2672–80. doi: 10.1182/blood.V84.8.2672.2672
132. Stevens AM, Miller JM, Munoz JO, Gaikwad AS, Redell MS. Interleukin-6 levels predict event-free survival in pediatric AML and suggest a mechanism of chemotherapy resistance. Blood Adv (2017) 1(18):1387–97. doi: 10.1182/bloodadvances.2017007856
133. Saadi MI, Ramzi M, Hosseinzadeh M, Ebrahimi N, Owjfard M, Abdolyousefi EN, et al. Expression levels of il-6 and il-18 in acute myeloid leukemia and its relation with response to therapy and acute GvHD after bone marrow transplantation. Indian J Surg Oncol (2021) 12(3):465–71. doi: 10.1007/s13193-021-01358-w
134. Peterlin P, Gaschet J, Guillaume T, Garnier A, Eveillard M, Le Bourgeois A, et al. A new cytokine-based dynamic stratification during induction is highly predictive of survivals in acute myeloid leukemia. Cancer Med (2021) 10(2):642–8. doi: 10.1002/cam4.3648
135. Hou D, Wang B, You R, Wang X, Liu J, Zhan W, et al. Stromal cells promote chemoresistance of acute myeloid leukemia cells via activation of the IL-6/STAT3/OXPHOS axis. Ann Transl Med (2020) 8(21):1346. doi: 10.21037/atm-20-3191
136. Reikvam H, Brenner AK, Hagen KM, Liseth K, Skrede S, Hatfield KJ, et al. The cytokine-mediated crosstalk between primary human acute myeloid cells and mesenchymal stem cells alters the local cytokine network and the global gene expression profile of the mesenchymal cells. Stem Cell Res (2015) 15(3):530–41. doi: 10.1016/j.scr.2015.09.008
137. O’Hagan-Wong K, Nadeau S, Carrier-Leclerc A, Apablaza F, Hamdy R, Shum-Tim D, et al. Increased IL-6 secretion by aged human mesenchymal stromal cells disrupts hematopoietic stem and progenitor cells’ homeostasis. Oncotarget (2016) 7(12):13285–96. doi: 10.18632/oncotarget.7690
138. Säily M, Koistinen P, Savolainen M, Rantala M, Savolainen ER. Expression of interleukin-6 (IL-6) receptor gene in acute myeloblastic leukemia and response of leukemic cells to exogenous IL-6. A Comp study between Cell line Cells corresponding native Cells Growth Factors (1998) 15(4):243–57. doi: 10.3109/08977199809017481
139. Zhang Y, Guo H, Zhang Z, Lu W, Zhu J, Shi J. IL-6 promotes chemoresistance via upregulating CD36 mediated fatty acids uptake in acute myeloid leukemia. Exp Cell Res (2022) 415(1):113112. doi: 10.1016/j.yexcr.2022.113112
140. Chen R, Chen B. Siltuximab (CNTO 328): a promising option for human malignancies. Drug Des Devel Ther (2015) 9:3455–8. doi: 10.2147/DDDT.S86438
141. Zhang TY, Dutta R, Benard B, Zhao F, Yin R, Majeti R. IL-6 blockade reverses bone marrow failure induced by human acute myeloid leukemia. Sci Transl Med (2020) 12(538):eaax5104. doi: 10.1126/scitranslmed.aax5104
142. Xie K. Interleukin-8 and human cancer biology. Cytokine Growth Factor Rev (2001) 12(4):375–91. doi: 10.1016/S1359-6101(01)00016-8
143. Campbell LM, Maxwell PJ, Waugh DJ. Rationale and means to target pro-inflammatory interleukin-8 (CXCL8) signaling in cancer. Pharm (Basel. (2013) 6(8):929–59. doi: 10.3390/ph6080929
144. Vinante F, Rigo A, Vincenzi C, Ricetti MM, Marrocchella R, Chilosi M, et al. IL-8 mRNA expression and IL-8 production by acute myeloid leukemia cells. Leukemia (1993) 7(10):1552–6.
145. Tobler A, Moser B, Dewald B, Geiser T, Studer H, Baggiolini M, et al. Constitutive expression of interleukin-8 and its receptor in human myeloid and lymphoid leukemia. Blood (1993) 82(8):2517–25. doi: 10.1182/blood.V82.8.2517.2517
146. Schinke C, Giricz O, Li W, Shastri A, Gordon S, Barreyro L, et al. IL8-CXCR2 pathway inhibition as a therapeutic strategy against MDS and AML stem cells. Blood (2015) 125(20):3144–52. doi: 10.1182/blood-2015-01-621631
147. Kuett A, Rieger C, Perathoner D, Herold T, Wagner M, Sironi S, et al. IL-8 as mediator in the microenvironment-leukaemia network in acute myeloid leukaemia Sci Rep (2015) 5:18411. doi: 10.1038/srep18411
148. Konopleva M, Konoplev S, Hu W, Zaritskey AY, Afanasiev BV, Andreeff M. Stromal cells prevent apoptosis of AML cells by up-regulation of anti-apoptotic proteins. Leukemia (2002) 16(9):1713–24. doi: 10.1038/sj.leu.2402608
149. Nefedova Y, Landowski TH, Dalton WS. Bone marrow stromal-derived soluble factors and direct cell contact contribute to de novo drug resistance of myeloma cells by distinct mechanisms. Leukemia (2003) 17(6):1175–82. doi: 10.1038/sj.leu.2402924
150. Ryningen A, Wergeland L, Glenjen N, Gjertsen BT, Bruserud O. In vitro crosstalk between fibroblasts and native human acute myelogenous leukemia (AML) blasts via local cytokine networks results in increased proliferation and decreased apoptosis of AML cells as well as increased levels of proangiogenic interleukin 8. Leukemia Res (2005) 29(2):185–96. doi: 10.1016/j.leukres.2004.06.008
151. Vijay V, Miller R, Vue GS, Pezeshkian MB, Maywood M, Ast AM, et al. Interleukin-8 blockade prevents activated endothelial cell mediated proliferation and chemoresistance of acute myeloid leukemiaLeukemia Res (2019) 84:106180. doi: 10.1016/j.leukres.2019.106180
152. Abdul-Aziz AM, Shafat MS, Mehta TK, Di Palma F, Lawes MJ, Rushworth SA, et al. MIF-induced stromal PKCβ/IL8 is essential in human acute myeloid leukemia. Cancer Res (2017) 77(2):303–11. doi: 10.1158/0008-5472.CAN-16-1095
153. Simonet WS, Hughes TM, Nguyen HQ, Trebasky LD, Danilenko DM, Medlock ES. Long-term impaired neutrophil migration in mice overexpressing human interleukin-8. J Clin Invest (1994) 94(3):1310–9. doi: 10.1172/JCI117450
154. Shi Y, Riese DJ. 2nd. Role CXCL12/CXCR4/CXCR7 Chemokine Axis Cancer. Front Pharmacol (2020) 11:574667. doi: 10.3389/fphar.2020.574667
155. Teicher BA, Fricker SP. CXCL12 (SDF-1)/CXCR4 pathway in cancer. Clin Cancer Res (2010) 16(11):2927–31. doi: 10.1158/1078-0432.CCR-09-2329
156. Khare T, Bissonnette M, Khare S. CXCL12-CXCR4/CXCR7 axis in colorectal cancer: Therapeutic target in preclinical and clinical studies. Int J Mol Sci (2021) 22(14):7371. doi: 10.3390/ijms22147371
157. Liao YX, Zhou CH, Zeng H, Zuo DQ, Wang ZY, Yin F, et al. The role of the CXCL12-CXCR4/CXCR7 axis in the progression and metastasis of bone sarcomas (Review). Int J Mol Med (2013) 32(6):1239–46. doi: 10.3892/ijmm.2013.1521
158. Daniel SK, Seo YD, Pillarisetty VG. The CXCL12-CXCR4/CXCR7 axis as a mechanism of immune resistance in gastrointestinal malignancies. Semin Cancer Biol (2020) 65:176–88. doi: 10.1016/j.semcancer.2019.12.007
159. Nazari A, Khorramdelazad H, Hassanshahi G. Biological/pathological functions of the CXCL12/CXCR4/CXCR7 axes in the pathogenesis of bladder cancer. Int J Clin Oncol (2017) 22(6):991–1000. doi: 10.1007/s10147-017-1187-x
160. Chen N, Jiang X, Wang J, Wu T, Cheng B, Xia J. CXCL12-CXCR4/CXCR7 axis contributes to cell motilities of oral squamous cell carcinoma. Tumour Biol (2016) 37(1):567–75. doi: 10.1007/s13277-015-3803-6
161. Spoo AC, Lübbert M, Wierda WG, Burger JA. CXCR4 is a prognostic marker in acute myelogenous leukemia. Blood (2007) 109(2):786–91. doi: 10.1182/blood-2006-05-024844
162. Du W, Lu C, Zhu X, Hu D, Chen X, Li J, et al. Prognostic significance of CXCR4 expression in acute myeloid leukemia. Cancer Med (2019) 8(15):6595–603. doi: 10.1002/cam4.2535
163. Zhao H, Guo L, Zhao H, Zhao J, Weng H, Zhao B. CXCR4 over-expression and survival in cancer: a system review and meta-analysis. Oncotarget (2015) 6(7):5022–40. doi: 10.18632/oncotarget.3217
164. Ahn JY, Seo K, Weinberg OK, Arber DA. The prognostic value of CXCR4 in acute myeloid leukemia. Appl Immunohistochem Mol Morphol (2013) 21(1):79–84. doi: 10.1097/PAI.0b013e3182606f4d
165. Chen Y, Jacamo R, Konopleva M, Garzon R, Croce C, Andreeff M. CXCR4 downregulation of let-7a drives chemoresistance in acute myeloid leukemia. J Clin Invest (2013) 123(6):2395–407. doi: 10.1172/JCI66553
166. Ramakrishnan R, Peña-Martínez P, Agarwal P, Rodriguez-Zabala M, Chapellier M, Högberg C, et al. CXCR4 signaling has a CXCL12-independent essential role in murine MLL-AF9-Driven acute myeloid leukemia. Cell Rep (2020) 31(8):107684. doi: 10.1016/j.celrep.2020.107684
167. Cancilla D, Rettig MP, DiPersio JF. Targeting CXCR4 in AML and ALL. Front Oncol (2020) 10:1672. doi: 10.3389/fonc.2020.01672
168. Castro F, Cardoso AP, Gonçalves RM, Serre K, Oliveira MJ. Interferon-gamma at the crossroads of tumor immune surveillance or evasion. Front Immunol (2018) 9:847. doi: 10.3389/fimmu.2018.00847
169. Uhl FM, Chen S, O’Sullivan D, Edwards-Hicks J, Richter G, Haring E, et al. Metabolic reprogramming of donor T cells enhances graft-versus-leukemia effects in mice and humans. Sci Transl Med (2020) 12(567):eabb8969. doi: 10.1126/scitranslmed.abb8969
170. Toffalori C, Zito L, Gambacorta V, Riba M, Oliveira G, Bucci G, et al. Immune signature drives leukemia escape and relapse after hematopoietic cell transplantation. Nat Med (2019) 25(4):603–11. doi: 10.1038/s41591-019-0400-z
171. Garcia-Diaz A, Shin DS, Moreno BH, Saco J, Escuin-Ordinas H, Rodriguez GA, et al. Interferon receptor signaling pathways regulating PD-L1 and PD-L2 expression. Cell Rep (2017) 19(6):1189–201. doi: 10.1016/j.celrep.2017.04.031
172. Berthon C, Driss V, Liu J, Kuranda K, Leleu X, Jouy N, et al. In acute myeloid leukemia, B7-H1 (PD-L1) protection of blasts from cytotoxic T cells is induced by TLR ligands and interferon-gamma and can be reversed using MEK inhibitors. Cancer Immunol Immunother (2010) 59(12):1839–49. doi: 10.1007/s00262-010-0909-y
173. Chen C, Liang C, Wang S, Chio CL, Zhang Y, Zeng C, et al. Expression patterns of immune checkpoints in acute myeloid leukemia. J Hematol Oncol (2020) 13(1):28. doi: 10.1186/s13045-020-00853-x
174. Haroun F, Solola SA, Nassereddine S, Tabbara I. PD-1 signaling and inhibition in AML and MDS. Ann Hematol (2017) 96(9):1441–8. doi: 10.1007/s00277-017-3051-5
175. Xu L, Liu L, Yao D, Zeng X, Zhang Y, Lai J, et al. PD-1 and TIGIT are highly Co-expressed on CD8(+) T cells in AML patient bone marrow. Front Oncol (2021) 11:686156. doi: 10.3389/fonc.2021.686156
176. Dong Y, Han Y, Huang Y, Jiang S, Huang Z, Chen R, et al. PD-L1 is expressed and promotes the expansion of regulatory T cells in acute myeloid leukemia. Front Immunol (2020) 11:1710. doi: 10.3389/fimmu.2020.01710
177. Yang X, Ma L, Zhang X, Huang L, Wei J. Targeting PD-1/PD-L1 pathway in myelodysplastic syndromes and acute myeloid leukemia. Exp Hematol Oncol (2022) 11(1):11. doi: 10.1186/s40164-022-00263-4
178. Vivier E, Artis D, Colonna M, Diefenbach A, Di Santo JP, Eberl G, et al. Innate lymphoid cells: 10 years on. Cell (2018) 174(5):1054–66. doi: 10.1016/j.cell.2018.07.017
179. Li Z, Ma R, Ma S, Tian L, Lu T, Zhang J, et al. ILC1s control leukemia stem cell fate and limit development of AML. Nat Immunol (2022) 23(5):718–30. doi: 10.1038/s41590-022-01198-y
180. Junttila IS. Tuning the cytokine responses: An update on interleukin (IL)-4 and IL-13 receptor complexes. Front Immunol (2018) 9:888. doi: 10.3389/fimmu.2018.00888
181. Bankaitis KV, Fingleton B. Targeting IL4/IL4R for the treatment of epithelial cancer metastasis. Clin Exp Metastasis (2015) 32(8):847–56. doi: 10.1007/s10585-015-9747-9
182. Li Z, Jiang J, Wang Z, Zhang J, Xiao M, Wang C, et al. Endogenous interleukin-4 promotes tumor development by increasing tumor cell resistance to apoptosis. Cancer Res (2008) 68(21):8687–94. doi: 10.1158/0008-5472.CAN-08-0449
183. Peña-Martínez P, Eriksson M, Ramakrishnan R, Chapellier M, Högberg C, Orsmark-Pietras C, et al. Interleukin 4 induces apoptosis of acute myeloid leukemia cells in a Stat6-dependent manner. Leukemia (2018) 32(3):588–96. doi: 10.1038/leu.2017.261
184. Finch ER, Tukaramrao DB, Goodfield LL, Quickel MD, Paulson RF, Prabhu KS. Activation of PPARγ by endogenous prostaglandin J(2) mediates the antileukemic effect of selenium in murine leukemia. Blood (2017) 129(13):1802–10. doi: 10.1182/blood-2016-08-736405
185. Qian F, Arner BE, Kelly KM, Annageldiyev C, Sharma A, Claxton DF, et al. Interleukin-4 treatment reduces leukemia burden in acute myeloid leukemia FASEB J (2022) 36(5):e22328. doi: 10.1096/fj.202200251R
186. Miari KE, Guzman ML, Wheadon H, Williams MTS. Macrophages in acute myeloid leukaemia: Significant players in therapy resistance and patient outcomes. Front Cell Dev Biol (2021) 9:692800. doi: 10.3389/fcell.2021.692800
187. Pan Y, Yu Y, Wang X, Zhang T. Tumor-associated macrophages in tumor immunity. Front Immunol (2020) 11:583084. doi: 10.3389/fimmu.2020.583084
188. Saraiva M, O’Garra A. The regulation of IL-10 production by immune cells. Nat Rev Immunol (2010) 10(3):170–81. doi: 10.1038/nri2711
189. Kornblau SM, McCue D, Singh N, Chen W, Estrov Z, Coombes KR. Recurrent expression signatures of cytokines and chemokines are present and are independently prognostic in acute myelogenous leukemia and myelodysplasia. Blood (2010) 116(20):4251–61. doi: 10.1182/blood-2010-01-262071
190. Dong Q, Li G, Fozza C, Wang S, Yang S, Sang Y, et al. Levels and clinical significance of regulatory b cells and T cells in acute myeloid leukemia. BioMed Res Int (2020) 2020:7023168. doi: 10.1155/2020/7023168
191. Bruserud O. IL-4, IL-10 and IL-13 in acute myelogenous leukemia. Cytokines Cell Mol Ther (1998) 4(3):187–98.
192. Han Y, Dong Y, Yang Q, Xu W, Jiang S, Yu Z, et al. Acute myeloid leukemia cells express ICOS ligand to promote the expansion of regulatory T cells. Front Immunol (2018) 9:2227. doi: 10.3389/fimmu.2018.02227
193. Musuraca G, De Matteis S, Napolitano R, Papayannidis C, Guadagnuolo V, Fabbri F, et al. IL-17/IL-10 double-producing T cells: new link between infections, immunosuppression and acute myeloid leukemia. J Transl Med (2015) 13:229. doi: 10.1186/s12967-015-0590-1
194. Xu Y, Mou J, Wang Y, Zhou W, Rao Q, Xing H, et al. Regulatory T cells promote the stemness of leukemia stem cells through IL10 cytokine-related signaling pathway. Leukemia (2022) 36(2):403–15. doi: 10.1038/s41375-021-01375-2
195. Jimbu L, Mesaros O, Neaga A, Nanut AM, Tomuleasa C, Dima D, et al. The potential advantage of targeting both PD-L1/PD-L2/PD-1 and IL-10-IL-10R pathways in acute myeloid leukemia. Pharm (Basel) (2021) 14(11):1105. doi: 10.3390/ph14111105
196. Chen N, Xu Y, Mou J, Rao Q, Xing H, Tian Z, et al. Targeting of IL-10R on acute myeloid leukemia blasts with chimeric antigen receptor-expressing T cells. Blood Cancer J (2021) 11(8):144. doi: 10.1038/s41408-021-00536-x
197. Huang J, Liu Y, Au BC, Barber DL, Arruda A, Schambach A, et al. Preclinical validation: LV/IL-12 transduction of patient leukemia cells for immunotherapy of AML. Mol Ther Methods Clin Dev (2016) 3:16074. doi: 10.1038/mtm.2016.74
198. Curti A, Pandolfi S, Aluigi M, Isidori A, Alessandrini I, Chiodoni C, et al. Interleukin-12 production by leukemia-derived dendritic cells counteracts the inhibitory effect of leukemic microenvironment on T cells. Exp Hematol (2005) 33(12):1521–30. doi: 10.1016/j.exphem.2005.08.005
Keywords: acute myeloid leukemia, cytokine signaling, inflammation, tumor microenvironment, cytokine inhibitors
Citation: Luciano M, Krenn PW and Horejs-Hoeck J (2022) The cytokine network in acute myeloid leukemia. Front. Immunol. 13:1000996. doi: 10.3389/fimmu.2022.1000996
Received: 22 July 2022; Accepted: 14 September 2022;
Published: 28 September 2022.
Edited by:
Giselle Penton-Rol, Center for Genetic Engineering and Biotechnology (CIGB), CubaReviewed by:
Andrea Biondi, University of Milano-Bicocca, ItalyCopyright © 2022 Luciano, Krenn and Horejs-Hoeck. This is an open-access article distributed under the terms of the Creative Commons Attribution License (CC BY). The use, distribution or reproduction in other forums is permitted, provided the original author(s) and the copyright owner(s) are credited and that the original publication in this journal is cited, in accordance with accepted academic practice. No use, distribution or reproduction is permitted which does not comply with these terms.
*Correspondence: Jutta Horejs-Hoeck, anV0dGEuaG9yZWpzX2hvZWNrQHBsdXMuYWMuYXQ=
Disclaimer: All claims expressed in this article are solely those of the authors and do not necessarily represent those of their affiliated organizations, or those of the publisher, the editors and the reviewers. Any product that may be evaluated in this article or claim that may be made by its manufacturer is not guaranteed or endorsed by the publisher.
Research integrity at Frontiers
Learn more about the work of our research integrity team to safeguard the quality of each article we publish.