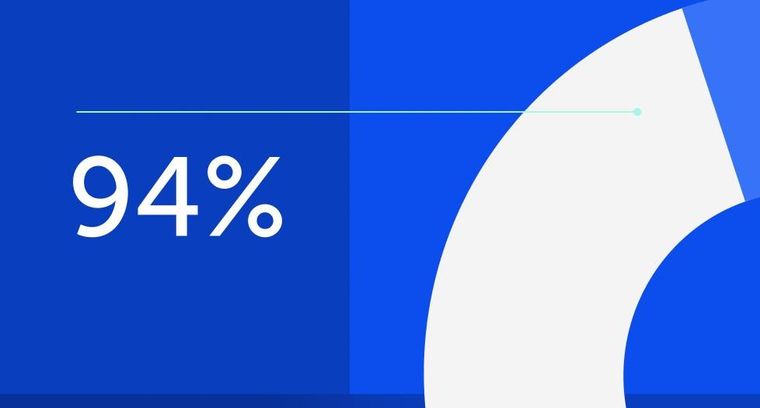
94% of researchers rate our articles as excellent or good
Learn more about the work of our research integrity team to safeguard the quality of each article we publish.
Find out more
ORIGINAL RESEARCH article
Front. Immunol., 04 January 2022
Sec. Microbial Immunology
Volume 12 - 2021 | https://doi.org/10.3389/fimmu.2021.794627
This article is part of the Research TopicUnderstanding anti-Trypanosomatid immune responses: the key for developing protective strategies against themView all 5 articles
Leishmania infantum, the etiological agent of canine leishmaniosis (CanL) in Europe, was responsible of the largest outbreak of human leishmaniosis in Spain. The parasite infects and survives within myeloid lineage cells, causing a potentially fatal disease if left untreated. The only treatment option relies on chemotherapy, although immunotherapy strategies are being considered as novel approaches to prevent progression of the disease. To this aim, a deeper characterization of the molecular mechanisms behind the immunopathogenesis of leishmaniosis is necessary. Thus, we evaluated, for the first time, the host immune response during L. infantum infection through transcriptome sequencing of the popliteal lymph nodes aspirates of dogs with CanL. Differential expression and weighted gene co-expression network analyses were performed, resulting in the identification of 5,461 differentially expressed genes (DEGs) and four key modules in sick dogs, compared to controls. As expected, defense response was the highest enriched biological process in the DEGs, with six genes related to immune response against pathogens (CHI3L1, SLPI, ACOD1, CCL5, MPO, BPI) included among the ten most expressed genes; and two of the key co-expression modules were associated with regulation of immune response, which also positively correlated with clinical stage and blood monocyte concentration. In particular, sick dogs displayed significant changes in the expression of Th1, Th2, Th17 and Tr1 cytokines (e. g. TNF-α, IFN-γ, IL-21, IL-17, IL-15), markers of T cell and NK cell exhaustion (e. g. LAG3, CD244, Blimp-1, JUN), and B cell, monocyte and macrophage disrupted functionality (e. g. CD40LG, MAPK4, IL-1R, NLRP3, BCMA). In addition, we found an overexpression of XBP1 and some other genes involved in endoplasmic reticulum stress and the IRE1 branch of the unfolded protein response, as well as one co-expression module associated with these processes, which could be induced by L. infantum to prevent host cell apoptosis and modulate inflammation-induced lymphangiogenesis at lymph nodes. Moreover, 21 lncRNAs were differentially expressed in sick dogs, and one key co-expression module was associated with chromatin organization, suggesting that epigenetic mechanisms could also contribute to dampening host immune response during natural L. infantum infection in the lymph nodes of dogs suffering from clinical leishmaniosis.
Leishmaniosis is a zoonotic disease caused by Leishmania spp. and transmitted by blood-sucking phlebotomine sand flies. Despite affecting 200,000–400,000 people annually and causing an estimated 20,000–40,000 deaths per year, leishmaniosis is still one of the most neglected diseases in the world (1–3). L. infantum was identified as the causative agent of the largest outbreak of human leishmaniosis in Spain (4), which is also the aetiological agent of canine leishmaniosis (CanL) in Europe. This protozoan is an obligate intracellular parasite that lives within myeloid lineage cells. It is capable of reprogramming the host microenvironment to invade and persist within the mammalian host cells, causing a systemic, chronic, and potentially fatal disease if left untreated (5–8).
Treatment of this zoonotic disease is a major challenge as the only option relies on chemotherapy (9, 10), and the emerging anti-leishmanial drug resistances, coupled with long duration of treatments and drug toxicity, further limit its efficacy (11). Immunotherapy, in conjunction with anti-leishmanial drugs (immunochemotherapy), could result in a synergic effect with activation of the protective immunity of the host and direct action of drugs against the parasite. Thus, immunotherapy and/or immunochemotherapy might be a promising alternative approach for treating leishmaniosis (12–15). In this regard, the use of vaccines as immunomodulatory agents could also help to reduce the parasite burden in infected dogs (13). However, a deeper characterization and a better understanding of the complex molecular mechanisms behind the immunopathogenesis of L. infantum infection are necessary to successfully develop efficient immunomodulatory drugs and treatment strategies.
Currently, it is widely accepted that a delicate balance between inflammatory and regulatory responses is required to achieve the immune control of L. infantum (12). Specifically, control of the infection relies on a successful macrophage activation via interferon-γ (IFN-γ), produced mainly by proinflammatory CD4+ T helper type 1 (Th1) cells and natural killer (NK) cells stimulated by interleukin (IL)-12, that promotes leishmanicidal activity mediated by nitric oxide (NO) (12, 15–17).
In contrast, the parasite’s survival is associated with a predominant immunosuppressive response mediated by CD4+ T regulatory type 1 (Tr1) cells and populations of regulatory B cells. These cells produce IL-10 and transforming growth factor-β (TGF-β) (18), decreasing the proliferation of Th1 cells producing IFN-γ, and then resulting in a lack of M1 macrophage activation and parasite killing (12, 19, 20), which might be also correlated with an increase on the alternatively activated macrophages (M2). M2 macrophage polarization will result in the induction of IL-10 and the inhibition of proinflammatory signals (5). Additionally, it has been shown that an incomplete activation or exhaustion of CD8+ cytotoxic T lymphocytes (CTLs) and NK cells could also limit IFN-γ production and contribute to a more severe immunological imbalance, favoring the parasite persistence (21).
Other cells, such as neutrophils, are additional components involved in the immune response during Leishmania infection. Although they are the first cells recruited to the infection site, phagocytosis of Leishmania amastigotes by neutrophils could lead to both parasite elimination, via neutrophil oxidative burst, or to prolonged parasite survival within the parasitophorous vacuole, avoiding inflammatory signals (12, 22, 23). Furthermore, neutrophils release networks of extracellular fibers composed of chromatin and granular proteins, also known as neutrophil extracellular traps (NETs) (24), that may help some Leishmania species to escape from extracellular effector immune mechanisms and to stimulate M2 macrophages.
Even though these host-parasite interactions during Leishmania infection have been well-studied in various species, research and knowledge gaps remain, mainly regarding the precise mechanisms involved in the immune evasion of the parasite. Recent advances in next-generation sequencing, specifically transcriptomics, help to overcome the drawbacks of traditional methods and allow to expand the knowledge about the immunopathogenesis of diseases (25–28). Furthermore, emerging evidence suggests that intracellular pathogens can modulate or even hijack their host gene expression processes through non-coding RNA-mediated regulatory mechanisms as an additional strategy to dampen the host immune response (29, 30). Non-coding RNAs (ncRNAs), which consist of microRNAs (miRNAs) and long ncRNAs (lncRNAs), are transcripts that do not encode proteins but still act as global and crucial biological regulators (29–31). In particular, the expression of lncRNAs, defined as transcripts longer than 200 nucleotides with no protein-coding potential, are either up- or down-regulated during infections, enhancing the host immunity, or even promoting pathogen invasion or replication within the host cells (29). Thus, they could be involved in the immune evasion during L. infantum infection.
Here, we performed transcriptome analyses for deep profiling of molecular basis of the host immune response during L. infantum infection in the dog. Our main goal was to evaluate gene expression signatures in the popliteal lymph nodes of dogs with CanL compared to controls. Our data showed that L. infantum infection induces strong transcriptional changes in this tissue, which could regulate host immunity at multiple levels to promote parasite persistence. These results provide new insights into the underlying mechanisms behind CanL and pinpoint potential targets for novel therapeutic strategies.
We recruited client-owned dogs with a diagnosis of CanL of any age, breed, or gender attending to the Consultant of Infectious diseases at the Complutense Veterinary Teaching Hospital of Madrid, Spain. Informed consent was obtained from each dog’s owner before enrollment in the study. The main inclusion criteria were a positive serology result for L. infantum by immunofluorescence antibody assay (IFA) plus a positive cytology and a PCR result obtained from bone marrow or lymph node aspirates, as well as presenting with clinical signs or clinicopathological abnormalities associated with clinical stages II-III, according to LeishVet guidelines (32). Animals were excluded if they met any of the following conditions: (1) current or recent (past 90 days) treatment for CanL (e.g., allopurinol, methylglucamine antimoniate, miltefosine) or immunomodulators, such as domperidone, ciclosporin and/or corticosteroids; (2) current or recent (past 90 days) use of any kind of special diet or supplements to improve their immune response; (3) vaccinated against CanL; (4) current or recent (past 90 days) use of any drugs except flea’s, heartworm, and/or tick prevention; (5) evidence of secondary immune-mediated disease (e.g., neoplasia, other infectious diseases) based upon imaging studies or infectious disease serology/PCR testing; (6) under one year of age; (7) pregnant or lactating females. For each case, we recorded breed, age, sex, clinical signs, relevant laboratory values and clinical stage, based on LeishVet guidelines (32).
Healthy control dogs were also recruited considering the following inclusion criteria: (1) negative serology result for L. infantum by IFA test; (2) negative cytology and/or PCR result obtained from bone marrow or lymph node aspirates; (3) an unremarkable physical examination performed by a veterinarian; (4) absence of clinicopathological abnormalities. Exclusion criteria for control dogs were the same as for infected/sick dogs. For each control dog, we recorded breed, age, and sex.
In order to perform aspirates from popliteal lymph nodes, hairs were removed from the skin over the site of puncture and asepsis was done with an alcoholic solution of 2% chlorhexidine. The lymph node aspirates were done using a 25 x 20 mm needle and a 10 ml syringe (Figure 1A). The lymph node samples (approximately 50 µl) were preserved in 200 µl of RNAlater (Qiagen, Valencia, CA) and stored according to the manufacturer’s guidelines. For infected/sick dogs, lymph node aspirates were collected prior to any therapeutic intervention.
Figure 1 (A) Overview of the study workflow (created with BioRender.com). (B) Principal component analysis of the samples showing PC1 and PC2. (C) Heatmap of the most variable genes expressed in dogs with CanL vs. healthy dogs. Expression profiles for the 1,500 genes with the highest variability that shown significant expression changes in dogs with CanL (right) and healthy dogs (left). Red represents genes overrepresented in CanL samples, and blue indicates genes overrepresented in controls. (D) Volcano plot where mean log2FC is plotted against the –log10 FDR adjusted P-values for all expressed genes. Each point represents a gene, and those with FDR < 1x10-26 and/or log2FC > 7 are displayed as triangles. Genes that reach the cut-off values (FDR < 0.05 and absolute log2FC > 0.5) are highlighted. Labels are displayed for the most significant (FDR < 10-8 and absolute log2FC > 2.5) protein-coding genes.
Total RNA was extracted from the lymph node samples with the RNeasy mini kit (Qiagen) with on-column DNase I treatment according to the manufacturer’s protocol (Figure 1A). Concentration and integrity of extracted RNA were measured with a NanoDrop ND-1000 Spectrophotometer (NanoDrop Technologies, Wilmington, DE) and an Agilent 2100 Expert Bioanalyzer (Agilent, Santa Clara, CA), respectively. Samples with an RNA Integrity Number (RIN) < 7 were excluded from downstream analyses. Approximately 1 μg of RNA from each dog was submitted for library preparation and sequencing at the DNA Link Sequencing Lab (Republic of Korea). cDNA libraries were constructed using the TruSeq Stranded Total RNA library Preparation Kit (Illumina, San Diego, CA), and a minimum of 5 Gb RNA-seq data per sample were generated using a NovaSeq 6000 System (Illumina) in paired-end read, 100 bp run mode. Base calling was done by Real Time Analysis (Illumina), and the output was demultiplexed and converted to FASTQ format with Bcl2fastq (version 2.20; Illumina).
Raw paired-end reads were checked for a possible low base score, Illumina sequencing adapters and PCR contaminations with FastQC v0.11.9 (33). Illumina sequencing adapters and low quality sequences were removed with Trimmomatic v0.39 (34).
Illumina-RNA sequencing reads were pseudo-aligned to the Ensembl 98 CanFam 3.1 reference transcriptome (35) using Salmon v1.1.0 (36). Expression per gene were summarized using tximport v1.14.2 (37) in R v4.1.0 (38) and Bioconductor v3.13 (39).
Genes with a mean raw count lower than 5 across all samples were removed for downstream analyses. Raw gene counts were normalized and variance stabilized for exploratory data analysis using principal component analysis (PCA) of the 1,500 most variable genes.
To estimate the abundance of immune cell subtypes and account for potential tissue heterogeneity (Figure 1A), we used the human validated signature matrix LM22 to deconvolute the bulk lymph node gene expression mixture matrix of orthologous human genes, in Fragments Per Kilobase of exon model per Million reads, with CIBERSORTx online tool (40). The LM22 matrix contains a total of 547 genes for distinguishing 22 hematopoietic cell subsets, including seven T cell types, naïve and memory B cells, plasma cells, NK cells and myeloid subsets. It was generated using Affymetrix HGU133A microarray data (41), but it has been rigorously tested for the application to RNA-Seq data and immune monitoring when samples cannot be immediately processed (40–42), even for non-human specimens (43–45). The fractions of immune cell types were compared across different groups using Wilcoxon test, and considering a statistical significance threshold of p-value ≤ 0.05.
Differential expression analysis was performed using DESeq2 v1.26.0 (46) between uninfected versus infected samples (Figure 1A), considering as a significant expression change when the False Discovery Rate (FDR) ≤ 0.05. Additional gene annotation was obtained using biomaRt v2.42.1 (47) to access canine reference transcriptome (CanFam 3.1), such as biotype (e.g. protein-coding or non-coding RNA) and gene names.
Gene co-expression analysis was performed to identified highly co-regulated modules across infected samples (clinical stages II-III) using WGCNA package v1.46 (48) (Figure 1A). Briefly, we built a gene co-expression network of genes with a mean of normalized counts across samples ≥ 7 by calculating Pearson’s correlations between their expression values. Subsequently, a weighted adjacency matrix was established by raising the co-expression similarity to apply a soft threshold power (β) of 12 to fit a scale-free network model (49) (Figure S1). The topological overlap measure (TOM) and its corresponding dissimilarity (1-TOM) were calculated using the adjacency matrix, and (1-TOM) was used as a distance between genes for hierarchical clustering analysis.
Modules were defined using DynamicTreeCut algorithm (48) with the following parameters: deep split = 2, cut height = 0.25, minimal module size = 30 genes. Therefore, modules can be defined as clusters of highly interconnected genes –with high topological overlap– and each of them will be identified with a color. The module-trait relationships were also estimated by calculating the correlations between Module Eigengenes (ME), a summarized expression profile of the module and inter-connected with intra-modular genes, and clinical features of patients (e.g. clinical stage, age, sex, haematological and biochemical parameters) (Table S1). In addition, Pearson’s correlation between expression profile of each gene and ME was calculated to identify the Module Membership (MM). Modules were potential biologically interesting and significantly associated with the variables when they had a correlation > 0.6 and a p-value ≤ 0.01 with clinical traits.
Gene Significance (GS), defined as the absolute correlation between the gene and the trait, was used to quantify associations of individual genes with clinical traits. The Module Significance (MS), defined as the average absolute GS of all the genes involved in the module, was calculated to evaluate the association strength.
Functional enrichment analyses for biological processes in the Gene Ontology (GO) were performed either for differentially expressed genes and genes within modules using enrichGO and gseGO functions of clusterProfiler v4.1 (50) (Figure 1A). We used all expressed genes as background, and only annotated genes were included in the analyses. Later, multiple testing correction was applied using Benjamini-Hochberg method and selecting only as significantly enriched those GO terms with an adjusted p-value ≤ 0.05. The most significant GO categories of biological process were used to characterize the key modules.
A total of 18 dogs met the inclusion criteria and were recruited for this study. Ten of these dogs were affected with CanL, and the other were healthy non-infected dogs which served as controls. The median age of animals included was 5 years, ranging from 1 to 12 years. The most represented dog breed was mixed breed, followed by American Staffordshire Terrier. Seven patients were clinically classified as stage II (moderate disease), and only three cases were in clinical stage III (severe disease). Complete clinical data is shown in (Table 1). Seven of the infected/sick dogs had been treated with leishmanicidal and/or leishmaniostatic drugs at some point before they were included in the study. However, none of them had received any treatment for CanL within the last 90 days prior to inclusion.
After mapping to canine transcriptome, relationships between samples were evaluated by PCA, which explained 63% and 10% sample variability (the first and second principal component, respectively). The distribution of the samples was divided into two clusters and no outlier samples were detected (Figures 1B, C).
All samples were significant (p-value < 0.05) at the deconvolution for immune cell subset identification and considered acceptable for further analysis. CanL samples had higher proportions of plasma cells, CD8+ T cells, γδ T cells, monocytes and M1 macrophages, and lower levels of memory B cells, resting memory CD4+ T cells, naïve CD4+ T cells, follicular T helper cells, activated dendritic cells, resting NK cells and eosinophils than control samples. In addition, lymph nodes of dogs with CanL in stage III showed higher amounts of activated NK cells and tended to present higher proportions of M0 macrophages (Figure 2).
Figure 2 The landscape of the cell composition in the lymph node aspirates based on their RNA-seq data. (A) Relative proportion of each immune cell type across samples was inferred by CIBERSORTx. (B) Violin and dot plots of immune cell populations from deconvolution analysis that displayed significant differences between groups, as estimated by Wilcoxon test. Significant differences between clinical stages II and III are highlighted in red. *p-value <0.05, **p-value <0.01, ***p-value <0.001.
A total of 20,772 genes were expressed in lymph nodes, of which 14,134 had a normalized counts across samples ≥ 5 (Table S2 and Figure 1D). Comparison of gene expression between case and control samples revealed 5,461 significantly differentially expressed genes (Table S2); 2,689 had a positive log2 fold change (log2FC) and 2,772 had a negative log2FC. In addition, 132 of the 20,772 genes were lncRNAs, of which 21 were significantly differentially expressed.
To understand the functional role of relevant genes during CanL, gene ontology (GO) enrichment analysis were performed to explore the biological functions of the 2,689 DEGs with a positive log2FC and the 2,772 DEGs with a negative log2FC. Complete results of functional enrichment analyses are provided in (Table S3).
In the DEGs with higher expression in CanL group, the enriched GO terms in biological processes were related to defense and immune responses (Figures 3, 4 and Table S3), including: humoral immune response, mononuclear cell migration, myeloid leukocyte activation and phagocytosis, among others; moreover they are also associated with response to endoplasmic reticulum (ER) stress and unfolded protein response (UPR), mediated by the activation of ER stress sensor inositol-requiring transmembrane kinase/endonuclease 1 (IRE1).
Figure 3 GO enrichment of the differentially expressed genes with positive log2FC. (A) All enriched GO categories of biological process in the differentially expressed genes with positive log2FC are displayed. The significance levels (FDR p-value) are represented by the color saturation, the size of the dots represents the number of genes in the gene set associated with the GO term and the gene ratio is represented by the horizontal bar length. (B) Gene expression heatmaps of the four most significant GO terms enriched in the differentially expressed genes with positive log2FC. Red through blue color indicates high to low expression.
Figure 4 Th1, Th2, Th17, Tr1 gene expression signatures observed in the lymph nodes. Violin and dot plots of selected genes involved in Th1, Th2, Th17 and Tr1 immune responses during canine leishmaniosis. *p-value <0.05, **p-value <0.01, ***p-value <0.001.
In the DEGs with lower expression in CanL group, cell-cell adhesion via plasma membrane adhesion molecules, transmembrane transport and cell-cell signaling, including trans-synaptic signaling, were the most enriched GO terms in biological processes (Table S3).
Weighted gene co-expression network analysis was performed on 12,155 genes, using a soft threshold power (β) of 12 (scale free R2 = 0.85) to ensure a scale-free network (Figure S1). Initially, 187 modules were partitioned by dynamic tree cutting. Then, the eigengenes of each module (ME) were identified and merged into 16 main modules by applying a merging distance threshold of 0.45, which is a strict cut-off value that supported the reliability of the module divisions (Figure S2). Finally, 4 of these modules containing 6,835 expressed genes were associated with clinical traits related to the infection (correlation > 0.6 and a p-value ≤ 0.01) (Figure 5A and Table 2), and were considered key modules associated with CanL and worthy of further exploration, including: A module containing 3,706 genes; B module containing 2,302 genes; C module containing 779 genes and D module containing 48 genes (Table 2). Module eigengenes of A, C and D modules correlated positively with blood monocyte concentration, GPT levels and clinical stage, respectively, while B module correlated inversely with blood monocyte concentration (Figure 5A).
Figure 5 (A) Heatmap of the correlation of WGCNA modules with clinical traits. Red through blue color indicates positive to negative correlation. HCT, hematocrit; HGB, hemoglobin; RBC, red blood cells; MCV, mean corpuscular volume; MCH, mean corpuscular hemoglobin; MCHC, mean corpuscular hemoglobin concentration; PLT, platelets; WBC, white blood cells; NEU_S, segmented neutrophils; NEU_B, band neutrophils; LYM, lymphocytes; MON, monocytes; EOS, eosinophils; GLU, glucose; CREA, creatinine; PT, total protein; GPT, glutamate pyruvate transaminase enzyme; UPC, urine protein,creatinine ratio; IFA, indirect immunofluorescent assay; ALB, albumin; ALPHA_1, ALPHA_2, BETA, GAMMA, serum globulin fractions; A/G, albumin,globulin ratio. (B) GO enrichment of the A, B, C and D modules. The top 10 enriched GO categories of biological process were selected with clusterProfiler package to avoid redundant GO terms (showCategory = 10 and simplify = 0.5, 0.65, 0.95 and 0.75 for A (blue4), B (navajowhite1), C (chocolate2) and D (yellow2) modules, respectively). The significance levels (FDR p-value) are represented by the color saturation, the size of the dots represents the number of genes in the gene set associated with the GO term and the gene ratio is represented by the horizontal bar length.
Table 2 Summary information of the key co-expression modules detected in the lymph nodes of dogs suffering from CanL.
The key modules were characterized based on the most significant GO categories for biological processes (Figure 5B and Table S3). Thus, the A module was associated with cell cycle process, ER stress and UPR; the B module with the regulation of immune response, leukocyte differentiation, activation and adhesion; the C module with chromatin organization; and the D module with the regulation of B cell apoptotic process (Table 2).
In this study, we integrated whole transcriptome profiling and bioinformatics analysis for identifying regulatory pathways in canine lymph nodes associated with L. infantum natural infection. This approach allowed us to get a deeper understanding of the molecular mechanisms underlying the immunopathogenesis of CanL and identified four key modules associated with the disease, as well as several candidate genes which may contribute to CanL. To the best of our knowledge, this is the first study in the dog that evaluates the impact of L. infantum natural infection on the immune response at whole transcriptome level.
The ER is an organelle responsible for the synthesis, folding and modification of proteins (51). However, the capacity of ER functions can be exceeded under certain circumstances, such as infections by intracellular parasites (52, 53), and lead to ER stress due to the accumulation of misfolded proteins (54, 55). This triggers the UPR, one of the main protective mechanisms of the ER to resolve stress and dysfunction, which is also necessary for the physiological function of the innate immune system (56).
In this study, ER stress and UPR signaling, specifically the IRE1 and ER-associated degradation (ERAD) pathways, were found: a) associated with CanL samples (Figure 3); b) linked to module A from the WGCNA analysis; c) correlated with higher blood monocyte concentrations in sick dogs (Figure 5 and Table 2); d) which also tended to correlate with increased monocyte abundances by cell deconvolution analysis (p-value = 0.074); and most important e) with increased M0 macrophage proportions in dogs in stage III of disease (p-value = 0.067) (Figure 2). The IRE1 branch of the ER stress response is a key signaling pathway in modulating innate and adaptive immune responses (56, 57). It is highly expressed in macrophages, T cells, plasma cells, dendritic cells, and NK cells in response to external stimuli, and regulates the production of proinflammatory cytokines through the activation of the X-box binding protein 1 (XBP1) (57–59). Thus, we could hypothesize that dysregulation of UPR may contribute to the inflammatory/regulatory imbalance during clinical leishmaniosis.
The role of ER stress in macrophages infected by L. infantum was evaluated by Galluzzi et al. (60), who showed a significant up-regulation of XBP1, suggesting that the parasite could promote survival of host cells by inducing a mild ER stress response. Similarly, we confirmed for the first time in naturally infected dogs a significant induction of ER stress and UPR, with an increased expression of XBP1. Other genes with a significantly higher expression involved in the IRE1 branch were ERN1, EDEM1, DNAJB9, WIPI1, HYOU1 and PDIA5. Therefore, IRE1 could be a critical pathway implicated in the immunopathogenesis of CanL.
Vascular endothelial growth factor-A (VEGFA) had also a significantly positive log2FC. Endothelial growth factor family members induce changes in the vascular network during inflammation. In fact, VEGFA can mediate inflammation-induced lymphangiogenesis and have intense effects on lymph nodes (61–65). IRE1 powerfully regulates VEGFA expression under various stress conditions (66–68), and infected macrophages harboring Leishmania donovani release extracellular vesicles that induce endothelial cells to secrete VEGFA (69). Weinkopff et al. (70) also found that infection with Leishmania major increases the expression of VEGFA and VEGF receptor-2 (VEGFR-2) at the site of infection, and it correlated with lesion size and parasite burden, suggesting that VEGFA-dependent lymphangiogenesis could be a mechanism that restricts tissue inflammation and contributes to the severity of leishmaniosis.
Defense response is the most relevant biological process modified in the lymph nodes of dogs with CanL, and is highly enriched in the DEGs with positive log2FC (Figure 3), with six of the ten higher expressed DEGs (CHI3L1, SLPI, ACOD1, CCL5, MPO and BPI) involved in immune responses against pathogens (71–76). Moreover, we observed a significantly decreased expression of tumor necrosis factor-α (TNF-α) (Figure 4). This cytokine is an essential component of the Th1 response, as it contributes to trigger nitric oxide production in activated macrophages, and therefore, its deficit may increase the severity of leishmaniosis (77). However, a significant increase in the expression of IFN-γ, the canonical Th1 cytokine, was found in the CanL group (Figure 4). The expression of some key factors for IFN-γ production were significantly increased too, including T-box expressed in T cells (TBX21), eomesodermin (EOMES), AP-1 transcription factor subunit (JUN), Src kinase (SRC), β1 and β2 subunits of the IL12 receptor (IL12RB1; IL12RB2) (Figure 4). Nevertheless, even with high IFN-γ levels, the host may fail to control the infection, probably due to an incomplete response to IFN-γ (78).
Although the expression of IL-10 was slightly elevated, the IL-6 receptor (IL6R) and the subunit α of the IL-10 receptor (IL10RA) were the only Th2 interleukin-related molecules significantly increased in the CanL group. In contrast, IL-4 and IL-13 showed a tendency to decrease in infected dogs (Figure 4). Alves et al. (79) detected a significant increase of TGF-β and IL-10 expression in the lymph nodes of dogs with CanL, but their expression remained stable in our study, with dogs in stage III displaying just a slightly higher expression of IL-10 than those in stage II. The expression of Th2 cytokines probably increase in very severe CanL (clinical stage IV). Thus, it could not be detected here, as we only included dogs with moderate to severe disease (clinical stages II-III). In fact, we found the expression of GATA3 significantly decreased in the CanL group (Figure 4). Among other functions, this transcription factor is critical for the induction of Th2 cytokine production and growth of Th2 cells (80), supporting a lack of Th2 response and a less relevant role of Th2 cells in the lymph nodes at these stages of the infection. However, this hypothesis could not be confirmed, since the clinical staging was not performed by Alves et al. (79), and the transcriptional changes we observed may not directly correlate with cell functionality.
Along with the dysregulated expression of pro- and anti-inflammatory interleukins, significant changes in the expression of some chemokines and chemokine receptors were also detected: CCL3, CCL4, CCL5, CCL8, CCL22, CXCL10, CCR1, CCR2 and CCR5. These chemokines play a vital role in determining the Th1/Th2-mediated responses (81), and can represent a potential prophylactic and therapeutic target to promote immune clearance of the parasite in CanL, specially CCL5, as it is one of the ten most expressed DEGs.
Th17 cells are an additional type of CD4+ T helper cells contributing to defense response, mainly through the production of IL-17. This interleukin synergizes with CCL3 and acts as a potent activator of neutrophils (82–85). Therefore, the continual production of IL-17 during clinical CanL may lead to an over-recruitment of neutrophils to inflammatory sites (86), which could result in the slightly higher expression of IL-8 we observed. The overexpression of IL-8 may promote parasite persistence, as this cytokine induces a massive and long-lasting accumulation of neutrophils (87, 88), where the parasite may survive (12, 22, 23, 89). However, in an experimental model of canine L. infantum infection, IL-17 transcription was reduced in lymph nodes, suggesting that the hyperinflammatory response generated at the beginning of the infection was suppressed as the disease progressed (90). This is also in accordance with our results, since three related members of the IL-17 gene family (IL17A, IL17B and IL17C), as well as IL-22, had a significantly lower expression in the case group (Figure 4).
The peripherally derived regulatory T cell subset CD4+ CD25- Foxp3- type 1 regulatory T (Tr1) cells are induced by chronic activation of CD4+ T cells by antigens in the presence of tolerogenic conditions (91–93). These Tr1 cells suppress the host immunity and down-regulate the activation and proliferation of effector T cells, including Th1 (12, 92–94). Increased activation of IL-10 producing Tr1 cells has been shown in chronic cutaneous leishmaniosis in humans, as well as in murine visceral leishmaniosis (95–98), although no changes in the expression of IL-10 and TGF-β were detected in CanL (99).
Interestingly, we observed a significantly lower expression of CD25 (IL2RA) and FOXP3 (Figure 4), which could be consistent with an increase in the number of Tr1 cells in the lymph nodes of CanL dogs, with no variation in the expression of IL-10 and TGF-β. Furthermore, the expression of several Tr1 markers and transcription factors were also significantly higher (LAG3, IRF1, IRF4, CCR5, BATF, MAF, PRDM1, ENTPD1 and IL21) (Figure 4). Many of these genes were included within the B module, which negatively correlated with the concentration of circulating blood monocytes and was enriched in GO terms related to lymphocyte activation and proliferation (Figure 5). As it was previously described, L. infantum may induce the activation of Tr1 cells to suppress the inflammatory response of the host (91, 93, 100), and we hypothesize that this suppression could potentially reduce the abundance of the blood monocyte population, although further research is needed to confirm it.
T cell exhaustion is a state of dysfunction triggered by a prolonged antigen exposure during many chronic infections that prevents optimal control of pathogens, including intracellular parasites (101–105). Indeed, T cell exhaustion has already been described in CanL, where T cell proliferation and functionality decreased as disease progresses (106). CD8+ T cells typically show an impaired cytotoxic activity, and lose the ability to produce IL-2 and TNF during the first stages of exhaustion, while severe exhaustion may lead to a completely lack of the ability to produce IFN-γ, CCL chemokines or to degranulate. More severe CD8+ exhaustion correlates with higher antigen load and loss of help from CD4+ T cells (105, 107). Here, we observed a lower expression of TNF and IL-2 receptor, as well as a higher expression of several transcriptional markers previously associated with the T cell exhaustion process, such as: surface inhibitory receptors and their ligands (LAG3, CD244, CD160, Fas and Fas ligand, TRAIL, and four TNF receptors), IL-10 receptor, and some downstream transcription factors (Blimp-1, EOMES, BATF and JUN) (102, 105), together with the Tr1 transcriptional markers mentioned above. In addition, LAG3, TRAIL and Blimp-1 (PRDM1) were also detected in the A co-expression module, which was significantly associated with cell cycle processes. However, these transcriptional factors can be expressed by other cell types and could exert additional functions.
On the other hand, we found that the expression of IL-15 was slightly but significantly increased in the case group. In lymph nodes, this cytokine is produced by APCs and it promotes the development and function of NK cells, priming them for cytolytic activity and production of IFN-γ (108–110). In fact, we found that the proportion of activated NK cells was significantly higher in the lymph nodes of dogs with CanL in stage III than in stage II (Figure 2). Although we noted overexpression of genes related to cytotoxic activity of NK cells, including Fas (FAS), granzyme A (GZMA), granzyme B (GZMB), perforin 1 (PRF1), natural killer receptor 2B4 (CD244) and Killer Cell Lectin Like Receptor K1 (KLRK1), the expression of CD69, a marker of NK cell activation (111, 112), was lower in CanL dogs, compared with the control group.
These results are compatible with an exhausted phenotype or bystander activation of CTLs and NK cells during CanL, which could lead to incomplete activation of these cells, linked with lower production of Th1 cytokines and enhanced cytotoxic molecule expression. Similar changes are frequently observed in chronic infections involving high levels of persistent antigen (94, 107). In fact, a strong induction of cytotoxic transcriptional signature associated with CTL and NK cell senescence was found in cutaneous leishmaniasis lesions (113). This cytolytic transcriptional signature correlated with treatment outcome (114), and it was also found in the blood of L. braziliensis patients (115), suggesting that dysfunctional states of T and NK cells may have a major role in the immunopathology of Leishmania infections.
Monocytes and macrophages are the final host cells for Leishmania and, therefore, these cells are crucial to disease progression (116, 117). Here, we found that the B module was inversely correlated with the concentration of circulating blood monocytes (Figure 5A and Table 2), which could be partially explained by the fact that it was functionally enriched in leukocyte adhesion GO terms, as shown in Figure 5B. A high number of DEGs with positive log2FC were also involved in mononuclear cell migration, specifically in monocyte chemotaxis (Figure 3), such as AIF1, CCL3, CCL4, CCR2, ICAM1, ICAM2, RAP1A, ITGB2, SELPG (118). We hypothesis that the increased expression of these genes in lymph nodes may promote the extravasation of monocytes from blood vessels and their migration into infected tissues, where their interaction with the parasite could lead to their activation into functional macrophages (12).
Although IFN-γ is critical for this activation and control of macrophage infection by Leishmania species, mainly by inducing the release of NO, the complete activation of macrophages to effector cells requires CD40-CD40 ligand (CD40LG) interactions (12, 119). However, the expressions of CD40 and CD40LG were both decreased in the CanL group, although only CD40L (CD40LG) reached a statistically significant value (Figure 4). For instance, CD40LG, TNF-α and IFN-γ were all detected in the B co-expression module, which was associated with the regulation of immune response and correlated with blood monocyte concentration, suggesting that the expression of these genes may influence the dynamics of monocytes and macrophages during L. infantum infection.
In addition to CD40-CD40L, the activation of mitogen-activated protein kinases (MAPKs) is needed to induce the production of proinflammatory cytokines and NO in macrophages (120–122). Interestingly, the disruption of the MAPK signaling pathway is frequently observed during Leishmania infections (6, 123–125) and we found MAPK4 among the ten DEGs with the lowest expression.
Another potential mechanism contributing to dampen specific immune activation during L infantum infection in the lymph nodes of dogs suffering from CanL may be the higher expression of serine leucocyte proteinase inhibitor (SLPI) they displayed, as it is a potent myeloid-derived anti-inflammatory and microbicidal molecule that targets monocytes and macrophages to modulate innate and adaptive immune responses (126–128), and has been shown to dysregulate the M1/M2 response during other Leishmania infections (129).
Moreover, we observed a higher expression of the IL-1 receptor antagonist and a lower expression of the Nod-like receptor protein 3 in the CanL group. These genes has been previously shown to contribute to the suppression of inflammatory responses and NO production via signaling through IL-1R, favoring the parasite survival in macrophages (130–132). However, additional mechanisms affecting these or other molecules cannot be ruled out, such as receptor instabilities, post-translational modifications, diminished DNA binding activity of transcription factors or the influence of Leishmania exosomes on immune cells, among others (133, 6, 134).
Overall, our results may suggest that the upstream signaling events leading to the production of IFN-γ are expressed in the lymph nodes of dogs with CanL, but we did not find any significant variations in the expression of inducible nitric oxide synthase (iNOS) or arginase 1 (Arg1), the two main enzymes involved in NO metabolism during the infection of macrophages by Leishmania spp. (5, 135, 136).
On the other hand, it is worth noting that solute carrier family 11 member 1 (SLC11A1), formerly known as natural resistance-associated macrophage protein 1 (NRAMP1), was identified among the overexpressed DEGs involved phagosome maturation (137). This gene has been widely investigated for its potential role in susceptibility to leishmaniosis (138), as it pumps the metal ions out of the parasitophorous vacuole (139) to deprive the parasite of iron and block its development (140).
Impaired humoral immunity could play a critical role in the progression of CanL, as hypergammaglobulinemia, nonspecific polyclonal antibodies and circulating immune complexes correlate with clinical progression of the disease (12, 141–143). However, participation of B cells in the immunopathogenesis of leishmaniosis is not only related to antibody production, but also to their functions as regulatory and APCs (144).
Human visceral leishmaniosis is associated with an increased expression of Blimp-1, which dampen the antigen presentation machinery in B cells and promotes their differentiation into plasma cells, leading to the observed hypergammaglobulinemia during clinical disease (143). The overexpression of two anti-apoptotic and survival signals for plasma cells are also key in the immunopathogenesis of visceral leishmaniosis: B-cell maturation antigen (BCMA) and transmembrane activator, calcium modulator and cyclophilin ligand interactor (TACI) (143, 145, 146). Our results were in line with these findings, as the three markers (Blimp-1, BCMA and TACI) were highly expressed in the CanL group, and hypergammaglobulinemia was observed in these patients (Table S1), which also presented higher proportions of plasma cells (Figure 2). Furthermore, we detected a co-expression module, the D module, associated with the regulation of B cell apoptotic process and significantly correlated with the clinical stage of CanL (Figure 5 and Table 2), suggesting that B cell homeostasis could be a key factor in the progression of CanL. All these provided clues regarding the involvement of B cells in promoting leishmaniosis, as they may have compromised abilities and would produce high antibody titers, which are detrimental during the chronic infection.
Finally, it is worth noting that lncRNAs might participate in the immunopathogenesis of CanL, as they are critical regulators of gene transcription during immune response through regulating protein-protein interactions or via their ability to assemble with RNA and DNA (147–150). Accordingly, we found that changes in the expression of several lncRNAs were significantly associated with CanL, including an antimicrobial peptide NK-lysin-like (LOC608395) and JUN, which play immunomodulatory roles (151, 152). In particular, JUN could be a key immune regulator during L. infantum infection, since it is the second most differentially expressed lncRNA in the lymph nodes of dogs with CanL and regulates proinflammatory cytokines, chemokines and NO production (152), essential to achieve parasite control.
Chromatin organization is also crucial for transcriptional regulation in the immune system (153, 154). In fact, the immune response induced by antigen exposure led to an increase in the level of chromatin activation and RNA content in the popliteal lymph nodes of dogs (155), suggesting that modification in the chromatin structure is essential to mount an effective immune response. Therefore, regulation of chromatin organization could be targeted by intracellular parasites to evade their host defense mechanisms. For instance, some parasites provoke changes in the chromatin states through sequence-specific DNA-binding proteins or ncRNAs to inhibit inflammatory responses (156). Here, we identified a significant association between the C co-expression module and the chromatin organization GO term (Figure 5B and Table 2). This module was also correlated with age and glutamate-pyruvate transaminase (GPT) serum levels (Figure 5A), which is not surprising, as aging influences both chromatin structure (157) and liver function (158). Then, changes in chromatin organization are likely to contribute to shaping the immune response during Leishmania infection, and age could impact this immune modulation, although the precise underlaying mechanisms involved in the pathogenesis cannot be established at this stage of the study.
In summary, we identified 5,461 differentially expressed genes and four key modules involved in several biological processes related to immune responses in dogs with CanL. Altogether, these data showed that L. infantum infection induces strong transcriptional changes in canine lymph nodes. These alterations could regulate host immunity at multiple levels to promote parasite persistence, such as: increasing host cell survival through the expression of the IRE1 branch of the UPR; dysregulating the expression of cytokines which are key in determining Th1, Th2, Th17 and Tr1-mediated responses; fostering T cell and NK cell exhaustion processes; and disrupting monocyte, macrophage and B cell activation and functionality. Furthermore, L. infantum infection could influence gene transcription by modulating lncRNA’s expression profiles and chromatin organization. Further investigation into these biological processes may lead to new immunomodulatory strategies to control Leishmania infections. Future studies are also warranted to further characterize the role of differentially expressed lncRNAs in the immunopathogenesis of CanL, as they display the potential to be targets for immunotherapy.
The datasets presented in this study can be found in online repositories. The names of the repository/repositories and accession number(s) can be found below: https://www.ebi.ac.uk/ena, PRJEB47771.
The animal study was reviewed and approved by the Experimentation and Animal Welfare Committee of the Complutense University. Written informed consent was obtained from the owners for the participation of their animals in this study.
CS, AR-P, NS, SD and GM conceived the project. CS, NS, and SD acquired funding. CS and GM acquired samples and clinical information of the patients. CS conducted the experiment. CS and AR-P performed the bioinformatic analyses, data visualization and wrote the original draft. All authors reviewed and approved the final version of the manuscript.
CS acknowledges the financial support for her Predoctoral Fellowship for Research Personnel in Training UCM of the Universidad Complutense de Madrid (Spain) and Banco Santander (CT42/18-CT43/18). AR-P has been supported by the Research Program “Atracción de Talento de la Comunidad de Madrid” (2017-T2/BMD-5532), and granted by the Regional Programme of Research and Technological Innovation for Young Doctors UCM-CAM (PR65/19- 22460).
The authors declare that the research was conducted in the absence of any commercial or financial relationships that could be construed as a potential conflict of interest.
All claims expressed in this article are solely those of the authors and do not necessarily represent those of their affiliated organizations, or those of the publisher, the editors and the reviewers. Any product that may be evaluated in this article, or claim that may be made by its manufacturer, is not guaranteed or endorsed by the publisher.
The authors would like to thank all the dogs and their owners who agreed to participate in this study. We would also like to thank J. Sarquis and A. Montoya from the Consultant of Infectious diseases of the Complutense Veterinary Teaching Hospital, who assisted with sample collection.
The Supplementary Material for this article can be found online at: https://www.frontiersin.org/articles/10.3389/fimmu.2021.794627/full#supplementary-material
1. Dantas-Torres F, Miró G, Baneth G, Bourdeau P, Breitschwerdt E, Capelli G, et al. Canine Leishmaniasis Control in the Context of One Health. Emerg Infect Dis (2019) 25:1–4. doi: 10.3201/eid2512.190164
2. Burza S, Croft SL, Boelaert M. Leishmaniasis. Lancet (2018) 392:951–70. doi: 10.1016/S0140-6736(18)31204-2
3. Alvar J, Vélez ID, Bern C, Herrero M, Desjeux P, Cano J, et al. Leishmaniasis Worldwide and Global Estimates of Its Incidence. PLoS One (2012) 7:e35671. doi: 10.1371/journal.pone.0035671
4. Arce A, Estirado A, Ordobas M, Sevilla S, García N, Moratilla L, et al. Re-Emergence of Leishmaniasis in Spain: Community Outbreak in Madrid, Spain to 2012. Eurosurveillance (2013) 18:20546. doi: 10.2807/1560-7917.ES2013.18.30.20546
5. Conceição-Silva F, Morgado FN. Leishmania Spp-Host Interaction: There Is Always an Onset, But Is There an End? Front Cell Infect Microbiol (2019) 9:330. doi: 10.3389/fcimb.2019.00330
6. Mahanta A, Ganguli P, Barah P, Sarkar RR, Sarmah N, Phukan S, et al. Integrative Approaches to Understand the Mastery in Manipulation of Host Cytokine Networks by Protozoan Parasites With Emphasis on Plasmodium and Leishmania Species. Front Immunol (2018) 9:296. doi: 10.3389/fimmu.2018.00296
7. Martínez-López M, Soto M, Iborra S, Sancho D. Leishmania Hijacks Myeloid Cells for Immune Escape. Front Microbiol (2018) 9:883. doi: 10.3389/fmicb.2018.00883
8. Bogdan C. Mechanisms and Consequences of Persistence of Intracellular Pathogens: Leishmaniasis as an Example. Cell Microbiol (2008) 10:1221–34. doi: 10.1111/j.1462-5822.2008.01146.x
9. Sundar S, Singh A. Chemotherapeutics of Visceral Leishmaniasis: Present and Future Developments. Parasitology (2018) 145:481–9. doi: 10.1017/S0031182017002116
10. Miró G, Petersen C, Cardoso L, Bourdeau P, Baneth G, Solano-Gallego L, et al. Novel Areas for Prevention and Control of Canine Leishmaniosis. Trends Parasitol (2017) 33:718–30. doi: 10.1016/j.pt.2017.05.005
11. Ponte-Sucre A, Gamarro F, Dujardin J-C, Barrett MP, López-Vélez R, García-Hernández R, et al. Drug Resistance and Treatment Failure in Leishmaniasis: A 21st Century Challenge. PLoS Negl Trop Dis (2017) 11:e0006052. doi: 10.1371/journal.pntd.0006052
12. Toepp AJ, Petersen CA. The Balancing Act: Immunology of Leishmaniosis. Res Vet Sci (2020) 130:19–25. doi: 10.1016/j.rvsc.2020.02.004
13. Gonçalves AAM, Leite JC, Resende LA, Mariano RM, da Silveira SP, de Melo-Júnior OAO, et al. An Overview of Immunotherapeutic Approaches Against Canine Visceral Leishmaniasis: What Has Been Tested on Dogs and a New Perspective on Improving Treatment Efficacy. Front Cell Infect Microbiol (2019) 9:427. doi: 10.3389/fcimb.2019.00427
14. Baxarias M, Martínez-Orellana P, Baneth G, Solano-Gallego L. Immunotherapy in Clinical Canine Leishmaniosis: A Comparative Update. Res Vet Sci (2019) 125:218–26. doi: 10.1016/j.rvsc.2019.06.009
15. Roatt BM, Aguiar-Soares RD, de O, Coura-Vital W, Ker HG, das Moreira ND, et al. Immunotherapy and Immunochemotherapy in Visceral Leishmaniasis: Promising Treatments for This Neglected Disease. Front Immunol (2014) 5:272. doi: 10.3389/fimmu.2014.00272
16. Brandonisio O, Panaro MA, Fumarola L, Sisto M, Leogrande D, Acquafredda A, et al. Macrophage Chemotactic Protein-1 and Macrophage Inflammatory Protein-1α Induce Nitric Oxide Release and Enhance Parasite Killing in Leishmania Infantum-Infected Human Macrophages. Clin Exp Med (2002) 5:125–9. doi: 10.1007/s102380200017
17. Bogdan C, Röllinghoff M, Diefenbach A. The Role of Nitric Oxide in Innate Immunity. Immunol Rev (2000) 10:17–26. doi: 10.1034/j.1600-065X.2000.917307.x
18. Roncarolo MG, Bacchetta R, Bordignon C, Narula S, Levings MK. Type 1 T Regulatory Cells: Tr1 Cells. Immunol Rev (2001) 182:68–79. doi: 10.1034/j.1600-065X.2001.1820105.x
19. Solano-Gallego L, Montserrat-Sangrà S, Ordeix L, Martínez-Orellana P. Leishmania Infantum-Specific Production of IFN-γ and IL-10 in Stimulated Blood From Dogs With Clinical Leishmaniosis. Parasit Vectors (2016) 9:317. doi: 10.1186/s13071-016-1598-y
20. Esch KJ, Juelsgaard R, Martinez PA, Jones DE, Petersen CA. Programmed Death 1–Mediated T Cell Exhaustion During Visceral Leishmaniasis Impairs Phagocyte Function. J.I (2013) 191:5542–50. doi: 10.4049/jimmunol.1301810
21. Gautam S, Kumar R, Singh N, Singh AK, Rai M, Sacks D, et al. CD8 T Cell Exhaustion in Human Visceral Leishmaniasis. J Infect Dis (2014) 209:290–9. doi: 10.1093/infdis/jit401
22. Regli IB, Passelli K, Hurrell BP, Tacchini-Cottier F. Survival Mechanisms Used by Some Leishmania Species to Escape Neutrophil Killing. Front Immunol (2017) 8:1558. doi: 10.3389/fimmu.2017.01558
23. de Menezes JP, Saraiva EM, da Rocha-Azevedo B. The Site of the Bite: Leishmania Interaction With Macrophages, Neutrophils and the Extracellular Matrix in the Dermis. Parasit Vectors (2016) 9:264. doi: 10.1186/s13071-016-1540-3
24. Sollberger G, Tilley DO, Zychlinsky A. Neutrophil Extracellular Traps: The Biology of Chromatin Externalization. Dev Cell (2018) 44:542–53. doi: 10.1016/j.devcel.2018.01.019
25. Sanz CR, Sevane N, Pérez-Alenza MD, Valero-Lorenzo M, Dunner S. Polymorphisms in Canine Immunoglobulin Heavy Chain Gene Cluster: A Double-Edged Sword for Diabetes Mellitus in the Dog. Anim Genet (2021) 52:333–41. doi: 10.1111/age.13047
26. Shadab M, Das S, Banerjee A, Sinha R, Asad M, Kamran M, et al. RNA-Seq Revealed Expression of Many Novel Genes Associated With Leishmania Donovani Persistence and Clearance in the Host Macrophage. Front Cell Infect Microbiol (2019) 9:17. doi: 10.3389/fcimb.2019.00017
27. Veras PST, Ramos PIP, de Menezes JPB. In Search of Biomarkers for Pathogenesis and Control of Leishmaniasis by Global Analyses of Leishmania-Infected Macrophages. Front Cell Infect Microbiol (2018) 8:326. doi: 10.3389/fcimb.2018.00326
28. Bragato JP, Melo LM, Venturin GL, Rebech GT, Encarna L. Relationship of Peripheral Blood Mononuclear Cells miRNA Expression and Parasitic Load in Canine Visceral Leishmaniasis. PLoS One (2018) 16:e0206876. doi: 10.1371/journal.pone.0206876
29. Shirahama S, Miki A, Kaburaki T, Akimitsu N. Long Non-Coding RNAs Involved in Pathogenic Infection. Front Genet (2020) 11:454. doi: 10.3389/fgene.2020.00454
30. Fernandes J, Acuña S, Aoki J, Floeter-Winter L, Muxel S. Long Non-Coding RNAs in the Regulation of Gene Expression: Physiology and Disease. ncRNA (2019) 5:17. doi: 10.3390/ncrna5010017
31. Le Béguec C, Wucher V, Lagoutte L, Cadieu E, Botherel N, Hédan B, et al. Characterisation and Functional Predictions of Canine Long Non-Coding RNAs. Sci Rep (2018) 8:13444. doi: 10.1038/s41598-018-31770-2
32. Solano-Gallego L, Miró G, Koutinas A, Cardoso L, Pennisi MG, Ferrer L, et al. LeishVet Guidelines for the Practical Management of Canine Leishmaniosis. Parasit Vectors (2011) 4:86. doi: 10.1186/1756-3305-4-86
33. Andrews S. FastQC: A Quality Control Tool for High Throughput Sequence Data. UK: F1000Research (2015).
34. Bolger AM, Lohse M, Usadel B. Trimmomatic: A Flexible Trimmer for Illumina Sequence Data. Bioinformatics (2014) 30:2114–20. doi: 10.1093/bioinformatics/btu170
35. Cunningham F, Achuthan P, Akanni W, Allen J, Amode MR, Armean IM, et al. Ensembl 2019. Nucleic Acids Res (2019) 47:D745–51. doi: 10.1093/nar/gky1113
36. Patro R, Duggal G, Love MI, Irizarry RA, Kingsford C. Salmon Provides Fast and Bias-Aware Quantification of Transcript Expression. Nat Methods (2017) 14:417–9. doi: 10.1038/nmeth.4197
37. Soneson C, Love MI, Robinson MD. Differential Analyses for RNA-Seq: Transcript-Level Estimates Improve Gene-Level Inferences. F1000Research (2016) 4:1521. doi: 10.12688/f1000research.7563.2
38. R Core Team. R: A Language and Environment for Statistical Computing. Vienna, Austria: R foundation for Statistical Computing (2021).
39. Huber W, Carey V, Gentleman R, Anders S, Carlson M, Carvalho B, et al. Orchestrating High-Throughput Genomic Analysis With Bioconductor. Nat Methods (2015) 12(2):115–21. doi: 10.1038/nmeth.3252
40. Newman AM, Steen CB, Liu CL, Gentles AJ, Chaudhuri AA, Scherer F, et al. Determining Cell Type Abundance and Expression From Bulk Tissues With Digital Cytometry. Nat Biotechnol (2019) 37(7):773–82. doi: 10.1038/s41587-019-0114-2
41. Newman AM, Liu CL, Green MR, Gentles AJ, Feng W, Xu Y, et al. Robust Enumeration of Cell Subsets From Tissue Expression Profiles. Nat Methods (2015) 12(5):453–7. doi: 10.1038/nmeth.3337
42. Chen B, Khodadoust MS, Liu CL, Newman AM, Alizadeh AA. Profiling Tumor Infiltrating Immune Cells With CIBERSORT. Methods Mol Biol (2018) 1711:243–59. doi: 10.1007/978-1-4939-7493-1_12
43. Amin SB, Anderson KJ, Boudreau CE, Martinez-Ledesma E, Kocakavuk E, Johnson KC, et al. Comparative Molecular Life History of Spontaneous Canine and Human Gliomas. Cancer Cell (2020) 37(2):243–57.e7. doi: 10.1016/j.ccell.2020.01.004
44. Wisdom AJ, Mowery YM, Hong CS, Himes JE, Nabet BY, Qin X, et al. Single Cell Analysis Reveals Distinct Immune Landscapes in Transplant and Primary Sarcomas That Determine Response or Resistance to Immunotherapy. Nat Commun (2020) 11(1):6410. doi: 10.1038/s41467-020-19917-0
45. Tuit S, Salvagno C, Kapellos TS, Hau CS, Seep L, Oestreich M, et al. Transcriptional Signature Derived From Murine Tumor-Associated Macrophages Correlates With Poor Outcome in Breast Cancer Patients. Cell Rep (2019) 29(5):1221–35.e5. doi: 10.1016/j.celrep.2019.09.067
46. Love MI, Huber W, Anders S. Moderated Estimation of Fold Change and Dispersion for RNA-Seq Data With Deseq2. Genome Biol (2014) 15:550. doi: 10.1186/s13059-014-0550-8
47. Durinck S, Spellman PT, Birney E, Huber W. Mapping Identifiers for the Integration of Genomic Datasets With the R/Bioconductor Package biomaRt. Nat Protoc (2009) 4:1184–91. doi: 10.1038/nprot.2009.97
48. Langfelder P, Horvath S. WGCNA: An R Package for Weighted Correlation Network Analysis. BMC Bioinf (2008) 9:559. doi: 10.1186/1471-2105-9-559
49. Zhang B, Horvath S. A General Framework for Weighted Gene Co-Expression Network Analysis. Stat Appl Genet Mol Biol (2005) 4. doi: 10.2202/1544-6115.1128
50. Yu G, Wang L-G, Han Y, He Q-Y. Clusterprofiler: An R Package for Comparing Biological Themes Among Gene Clusters. OMICS: A J Integr Biol (2012) 16:284–7. doi: 10.1089/omi.2011.0118
51. Braakman I, Bulleid NJ. Protein Folding and Modification in the Mammalian Endoplasmic Reticulum. Annu Rev Biochem (2011) 80:71–99. doi: 10.1146/annurev-biochem-062209-093836
52. Dias-Teixeira KL, Pereira RM, Silva JS, Fasel N, Aktas BH, Lopes UG. Unveiling the Role of the Integrated Endoplasmic Reticulum Stress Response in Leishmania Infection – Future Perspectives. Front Immunol (2016) 7:283. doi: 10.3389/fimmu.2016.00283
53. Inácio P, Zuzarte-Luís V, Ruivo MT, Falkard B, Nagaraj N, Rooijers K, et al. Parasite-Induced ER Stress Response in Hepatocytes Facilitates Plasmodium Liver Stage Infection. EMBO Rep (2015) 16:955–64. doi: 10.15252/embr.201439979
54. Cubillos-Ruiz JR, Bettigole SE, Glimcher LH. Tumorigenic and Immunosuppressive Effects of Endoplasmic Reticulum Stress in Cancer. Cell (2017) 168:692–706. doi: 10.1016/j.cell.2016.12.004
55. Yoshida H. ER Stress and Diseases: ER Stress and Diseases. FEBS J (2007) 274:630–58. doi: 10.1111/j.1742-4658.2007.05639.x
56. Di Conza G, Ho P-C. ER Stress Responses: An Emerging Modulator for Innate Immunity. Cells (2020) 9:695. doi: 10.3390/cells9030695
57. Dong H, Adams NM, Xu Y, Cao J, Allan DSJ, Carlyle JR, et al. The IRE1 Endoplasmic Reticulum Stress Sensor Activates Natural Killer Cell Immunity in Part by Regulating C-Myc. Nat Immunol (2019) 20:865–78. doi: 10.1038/s41590-019-0388-z
58. Cubillos-Ruiz JR, Silberman PC, Rutkowski MR, Chopra S, Perales-Puchalt A, Song M, et al. ER Stress Sensor XBP1 Controls Anti-Tumor Immunity by Disrupting Dendritic Cell Homeostasis. Cell (2015) 161:1527–38. doi: 10.1016/j.cell.2015.05.025
59. Martinon F, Chen X, Lee A-H, Glimcher LH. TLR Activation of the Transcription Factor XBP1 Regulates Innate Immune Responses in Macrophages. Nat Immunol (2010) 11:411–8. doi: 10.1038/ni.1857
60. Galluzzi L, Diotallevi A, De Santi M, Ceccarelli M, Vitale F, Brandi G, et al. Leishmania Infantum Induces Mild Unfolded Protein Response in Infected Macrophages. PLoS One (2016) 11:e0168339. doi: 10.1371/journal.pone.0168339
61. Wuest TR, Carr DJJ. VEGF-A Expression by HSV-1–Infected Cells Drives Corneal Lymphangiogenesis. J Exp Med (2010) 207:101–15. doi: 10.1084/jem.20091385
62. Kataru RP, Jung K, Jang C, Yang H, Schwendener RA, Baik JE, et al. Critical Role of CD11b+ Macrophages and VEGF in Inflammatory Lymphangiogenesis, Antigen Clearance, and Inflammation Resolution. Blood (2009) 113:5650–9. doi: 10.1182/blood-2008-09-176776
63. Halin C, Tobler NE, Vigl B, Brown LF, Detmar M. VEGF-A Produced by Chronically Inflamed Tissue Induces Lymphangiogenesis in Draining Lymph Nodes. Blood (2007) 110:10. doi: 10.1182/blood-2007-01-066811
64. Cursiefen C, Chen L, Borges LP, Jackson D, Cao J, Radziejewski C, et al. VEGF-A Stimulates Lymphangiogenesis and Hemangiogenesis in Inflammatory Neovascularization via Macrophage Recruitment. J Clin Invest (2004) 113:1040–50. doi: 10.1172/JCI20465
65. Nagy JA, Vasile E, Feng D, Sundberg C, Brown LF, Detmar MJ, et al. Vascular Permeability Factor/Vascular Endothelial Growth Factor Induces Lymphangiogenesis as Well as Angiogenesis. J Exp Med (2002) 196:1497–506. doi: 10.1084/jem.20021244
66. Duan Q, Ni L, Wang P, Chen C, Yang L, Ma B, et al. Deregulation of XBP 1 Expression Contributes to Myocardial Vascular Endothelial Growth Factor-A Expression and Angiogenesis During Cardiac Hypertrophy In Vivo. Aging Cell (2016) 15:625–33. doi: 10.1111/acel.12460
67. Ghosh R, Lipson KL, Sargent KE, Mercurio AM, Hunt JS, Ron D, et al. Transcriptional Regulation of VEGF-A by the Unfolded Protein Response Pathway. PLoS One (2010) 5:e9575. doi: 10.1371/journal.pone.0009575
68. Pereira ER, Liao N, Neale GA, Hendershot LM. Transcriptional and Post-Transcriptional Regulation of Proangiogenic Factors by the Unfolded Protein Response. J Periodontol (2010) 5:e12521. doi: 10.1371/journal.pone.0012521
69. Gioseffi A, Hamerly T, Van K, Zhang N, Dinglasan RR, Yates PA, et al. Leishmania -Infected Macrophages Release Extracellular Vesicles That Can Promote Lesion Development. Life Sci Alliance (2020) 3:e202000742. doi: 10.26508/lsa.202000742
70. Weinkopff T, Konradt C, Christian DA, Discher DE, Hunter CA, Scott P. Leishmania Major Infection–Induced VEGF-A/VEGFR-2 Signaling Promotes Lymphangiogenesis That Controls Disease. J.I (2016) 197:1823–31. doi: 10.4049/jimmunol.1600717
71. Wu R, Chen F, Wang N, Tang D, Kang R. ACOD1 in Immunometabolism and Disease. Cell Mol Immunol (2020) 17:822–33. doi: 10.1038/s41423-020-0489-5
72. Davenport B, Eberlein J, Nguyen TT, Victorino F, van der Heide V, Kuleshov M, et al. Chemokine Signatures of Pathogen-Specific T Cells II: Memory T Cells in Acute and Chronic Infection. J.I (2020) 205:2188–206. doi: 10.4049/jimmunol.2000254
73. Breyne K, Steenbrugge J, Demeyere K, Lee CG, Elias JA, Petzl W, et al. Immunomodulation of Host Chitinase 3-Like 1 During a Mammary Pathogenic Escherichia Coli Infection. Front Immunol (2018) 9:1143. doi: 10.3389/fimmu.2018.01143
74. Bülow S, Zeller L, Werner M, Toelge M, Holzinger J, Entzian C, et al. Bactericidal/Permeability-Increasing Protein Is an Enhancer of Bacterial Lipoprotein Recognition. Front Immunol (2018) 9:2768. doi: 10.3389/fimmu.2018.02768
75. Theeß W, Sellau J, Steeg C, Klinke A, Baldus S, Cramer JP, et al. Myeloperoxidase Attenuates Pathogen Clearance During Plasmodium Yoelii Nonlethal Infection. Infect Immun (2017) 85:e00475–16. doi: 10.1128/IAI.00475-16
76. Gomez SA, Argüelles CL, Guerrieri D, Tateosian NL, Amiano NO, Slimovich R, et al. Secretory Leukocyte Protease Inhibitor: A Secreted Pattern Recognition Receptor for Mycobacteria. Am J Respir Crit Care Med (2009) 179:247–53. doi: 10.1164/rccm.200804-615OC
77. Osero BO, Aruleba RT, Brombacher F, Hurdayal R. Unravelling the Unsolved Paradoxes of Cytokine Families in Host Resistance and Susceptibility to Leishmania Infection. Cytokine: X (2020) 2:100043. doi: 10.1016/j.cytox.2020.100043
78. Ansari NA, Saluja S, Salotra P. Elevated Levels of Interferon-γ, Interleukin-10, and Interleukin-6 During Active Disease in Indian Kala Azar. Clin Immunol (2006) 119:339–45. doi: 10.1016/j.clim.2006.01.017
79. Alves CF, de Amorim IFG, Moura EP, Ribeiro RR, Alves, Cibele F, Michalick MS, et al. Expression of IFN-γ, TNF-α, IL-10 and TGF-β in Lymph Nodes Associates With Parasite Load and Clinical Form of Disease in Dogs Naturally Infected With Leishmania (Leishmania) Chagasi. Vet Immunol Immunopathol (2009) 128:349–58. doi: 10.1016/j.vetimm.2008.11.020
80. Zhu J, Yamane H, Cote-Sierra J, Guo L, Paul WE. GATA-3 Promotes Th2 Responses Through Three Different Mechanisms: Induction of Th2 Cytokine Production, Selective Growth of Th2 Cells and Inhibition of Th1 Cell-Specific Factors. Cell Res (2006) 16:3–10. doi: 10.1038/sj.cr.7310002
81. Oghumu S, Lezama-Dávila CM, Isaac-Márquez AP, Satoskar AR. Role of Chemokines in Regulation of Immunity Against Leishmaniasis. Exp Parasitol (2010) 126:389–96. doi: 10.1016/j.exppara.2010.02.010
82. Gonçalves-de-Albuquerque S, da C, Pessoa-e-Silva R, Trajano-Silva LAM, de Goes TC, de Morais RCS, et al. The Equivocal Role of Th17 Cells and Neutrophils on Immunopathogenesis of Leishmaniasis. Front Immunol (2017) 8:1437. doi: 10.3389/fimmu.2017.01437
83. Charmoy M, Auderset F, Allenbach C, Tacchini-Cottier F. The Prominent Role of Neutrophils During the Initial Phase of Infection by Leishmania Parasites. J Biomed Biotechnol (2010) 2010:1–8. doi: 10.1155/2010/719361
84. Charmoy M, Brunner-Agten S, Aebischer D, Auderset F, Launois P, Milon G, et al. Neutrophil-Derived CCL3 Is Essential for the Rapid Recruitment of Dendritic Cells to the Site of Leishmania Major Inoculation in Resistant Mice. PLoS Pathog (2010) 6:e1000755. doi: 10.1371/journal.ppat.1000755
85. Tacchini-Cottier F, Zweifel C, Belkaid Y, Mukankundiye C, Vasei M, Launois P, et al. An Immunomodulatory Function for Neutrophils During the Induction of a CD4 + Th2 Response in BALB/c Mice Infected With Leishmania Major. J Immunol (2000) 165:2628–36. doi: 10.4049/jimmunol.165.5.2628
86. de Nicolato RC, de Abreu RT, Roatt BM, de Aguiar-Soares RDO, Reis LES, das Carvalho MG, et al. Clinical Forms of Canine Visceral Leishmaniasis in Naturally Leishmania Infantum–Infected Dogs and Related Myelogram and Hemogram Changes. PLoS One (2013) 8:e82947. doi: 10.1371/journal.pone.0082947
87. Zeilhofer HU, Schorr W. Role of Interleukin-8 in Neutrophil Signaling. Curr Opin Hematol (2000) 7:178–82. doi: 10.1097/00062752-200005000-00009
88. Bickel M. The Role of Interleukin-8 in Inflammation and Mechanisms of Regulation. (1993) 64(5 Suppl):456–60.
89. Aga E, Katschinski DM, van Zandbergen G, Laufs H, Hansen B, Müller K, et al. Inhibition of the Spontaneous Apoptosis of Neutrophil Granulocytes by the Intracellular Parasite Leishmania Major. J Immunol (2002) 169:898–905. doi: 10.4049/jimmunol.169.2.898
90. Hosein S, Rodríguez-Cortés A, Blake DP, Allenspach K, Alberola J, Solano-Gallego L. Transcription of Toll-Like Receptors 2, 3, 4 and 9, FoxP3 and Th17 Cytokines in a Susceptible Experimental Model of Canine Leishmania Infantum Infection. PLoS One (2015) 10:e0140325. doi: 10.1371/journal.pone.0140325
91. Roncarolo MG, Gregori S, Bacchetta R, Battaglia M, Gagliani N. The Biology of T Regulatory Type 1 Cells and Their Therapeutic Application in Immune-Mediated Diseases. Immunity (2018) 49:1004–19. doi: 10.1016/j.immuni.2018.12.001
92. Costa DL, Cardoso TM, Queiroz A, Milanezi CM, Bacellar O, Carvalho EM, et al. Tr-1–Like CD4+CD25–CD127–/lowFOXP3– Cells Are the Main Source of Interleukin 10 in Patients With Cutaneous Leishmaniasis Due to Leishmania Braziliensis. J Infect Dis (2015) 211:708–18. doi: 10.1093/infdis/jiu406
93. Zeng H, Zhang R, Jin B, Chen L. Type 1 Regulatory T Cells: A New Mechanism of Peripheral Immune Tolerance. Cell Mol Immunol (2015) 12:566–71. doi: 10.1038/cmi.2015.44
94. Rouse BT, Sehrawat S. Immunity and Immunopathology to Viruses: What Decides the Outcome? Nat Rev Immunol (2010) 10:514–26. doi: 10.1038/nri2802
95. Rodrigues OR, Marques C, Soares-Clemente M, Ferronha MH, Santos-Gomes GM. Identification of Regulatory T Cells During Experimental Leishmania Infantum Infection. Immunobiology (2009) 214:101–11. doi: 10.1016/j.imbio.2008.07.001
96. Anderson CF, Oukka M, Kuchroo VJ, Sacks D. CD4+CD25–Foxp3– Th1 Cells Are the Source of IL-10–Mediated Immune Suppression in Chronic Cutaneous Leishmaniasis. J Exp Med (2007) 204:285–97. doi: 10.1084/jem.20061886
97. Nylén S, Maurya R, Eidsmo L, Manandhar KD, Sundar S, Sacks D. Splenic Accumulation of IL-10 mRNA in T Cells Distinct From CD4+CD25+ (Foxp3) Regulatory T Cells in Human Visceral Leishmaniasis. J Exp Med (2007) 204:805–17. doi: 10.1084/jem.20061141
98. Stäger S, Maroof A, Zubairi S, Sanos SL, Kopf M, Kaye PM. Distinct Roles for IL-6 and IL-12p40 in Mediating Protection Againstleishmania Donovani and the Expansion of IL-10+ CD4+ T Cells. Eur J Immunol (2006) 36:1764–71. doi: 10.1002/eji.200635937
99. Strauss-Ayali D, Baneth G, Jaffe CL. Splenic Immune Responses During Canine Visceral Leishmaniasis. Vet Res (2007) 38:547–64. doi: 10.1051/vetres:2007015
100. Wu Y, Chang Y-M, Stell AJ, Priestnall SL, Sharma E, Goulart MR, et al. Phenotypic Characterisation of Regulatory T Cells in Dogs Reveals Signature Transcripts Conserved in Humans and Mice. Sci Rep (2019) 9:13478. doi: 10.1038/s41598-019-50065-8
101. Pérez-Antón E, Thomas MC, Lopez Lopez MC. T-Cell Exhaustion Process During Chronic Infection Caused by Intracellular Trypanosomatids. Ars Pharm (2019) 60:65–78. doi: 10.30827/ars.v60i2.9432
102. Hwang S, Cobb DA, Bhadra R, Youngblood B, Khan IA. Blimp-1–Mediated CD4 T Cell Exhaustion Causes CD8 T Cell Dysfunction During Chronic Toxoplasmosis. J Exp Med (2016) 213:1799–818. doi: 10.1084/jem.20151995
103. Illingworth J, Butler NS, Roetynck S, Mwacharo J, Pierce SK, Bejon P, et al. Chronic Exposure to Plasmodium Falciparum Is Associated With Phenotypic Evidence of B and T Cell Exhaustion. J.I (2013) 190:1038–47. doi: 10.4049/jimmunol.1202438
104. Butler NS, Moebius J, Pewe LL, Traore B, Doumbo OK, Tygrett LT, et al. Therapeutic Blockade of PD-L1 and LAG-3 Rapidly Clears Established Blood-Stage Plasmodium Infection. Nat Immunol (2012) 13:188–95. doi: 10.1038/ni.2180
106. Boggiatto PM, Ramer-Tait AE, Metz K, Kramer EE, Gibson-Corley K, Mullin K, et al. Immunologic Indicators of Clinical Progression During Canine L Eishmania Infantum Infection. Clin Vaccine Immunol (2010) 17:267–73. doi: 10.1128/CVI.00456-09
107. Virgin HW, Wherry EJ, Ahmed R. Redefining Chronic Viral Infection. Cell (2009) 138:30–50. doi: 10.1016/j.cell.2009.06.036
108. Lucas M, Schachterle W, Oberle K, Aichele P. Natural Killer Cell-Mediated Control of Infections Requires Production of Interleukin 15 by Type I IFN-Triggered Dendritic Cells. Blood (2008) 28:4210–9. doi: 10.1016/j.immuni.2007.03.006
109. Ma A, Koka R, Burkett P. Diverse Functions of IL-2, IL-15, AND IL-7 in Lymphoid Homeostasis. Rev Immunol (2006) 25:657–79. doi: 10.1146/annurev.immunol.24.021605.090727
110. Williams NS, Klem J, Puzanov I. Natural Killer Cell Differentiation: Insights From Knockout and Transgenic Mouse Models and In Vitro Systems. Immunol Rev (1998) 16:47–61. doi: 10.1111/j.1600-065X.1998.tb01229.x
111. Hood SP, Pockley AG. Phenotype and Function of Activated Natural Killer Cells From Patients With Prostate Cancer: Patient-Dependent Responses to Priming and IL-2 Activation. Front Immunol (2019) 9:20. doi: 10.3389/fimmu.2018.03169
112. Bogdan C, Schleicher U. Monocyte-Derived Signals Activate Human Natural Killer Cells in Response to Leishmania Parasites. Front Immunol (2018) 9:18. doi: 10.3389/fimmu.2018.00024
113. Fantecelle CH, Covre LP, Garcia de Moura R, Guedes H, Amorim CF, Scott P, et al. Transcriptomic Landscape of Skin Lesions in Cutaneous Leishmaniasis Reveals a Strong CD8+ T Cell Immunosenescence Signature Linked to Immunopathology. Immunology (2021) 164(4):754–65. doi: 10.1111/imm.13410
114. Amorim CF, Novais FO, Nguyen BT, Misic AM, Carvalho LP, Carvalho EM, et al. Variable Gene Expression and Parasite Load Predict Treatment Outcome in Cutaneous Leishmaniasis. Sci Trans Med (2019) 11(519):eaax4204. doi: 10.1126/scitranslmed.aax4204
115. Amorim CF, Novais FO, Nguyen BT, Nascimento MT, Lago J, Lago AS, et al. Localized Skin Inflammation During Cutaneous Leishmaniasis Drives a Chronic, Systemic IFN-γ Signature. PLoS Neglect Trop Dis (2021) 15(4):e0009321. doi: 10.1371/journal.pntd.0009321
116. Singh N, Kumar R, Chauhan SB, Engwerda C, Sundar S. Peripheral Blood Monocytes With an Antiinflammatory Phenotype Display Limited Phagocytosis and Oxidative Burst in Patients With Visceral Leishmaniasis. J Infect Dis (2018) 12:1130–41. doi: 10.1093/infdis/jiy228
117. Tomiotto-Pellissier F, da Silva Bortoleti BT, Assolini JP, Gonçalves MD, Machado Carloto AC, Miranda-Sapla MM, et al. Macrophage Polarization in Leishmaniasis: Broadening Horizons. Front Immunol (2018) 9:12. doi: 10.3389/fimmu.2018.02529
118. Lorenowicz MJ, van Gils J, de Boer M, Hordijk PL, Fernandez-Borja M. Epac1-Rap1 Signaling Regulates Monocyte Adhesion and Chemotaxis. J Leukocyte Biol (2006) 80:1542–52. doi: 10.1189/jlb.0506357
119. Tang T, Cheng X, Truong B, Sun L, Yang X, Wang H. Molecular Basis and Therapeutic Implications of CD40/CD40L Immune Checkpoint. Pharmacol Ther (2021) 219:107709. doi: 10.1016/j.pharmthera.2020.107709
120. Kuriakose S, Onyilagha C, Singh R, Olayinka-Adefemi F, Jia P, Uzonna JE. TLR-2 and MyD88-Dependent Activation of MAPK and STAT Proteins Regulates Proinflammatory Cytokine Response and Immunity to Experimental Trypanosoma Congolense Infection. Front Immunol (2019) 10:2673. doi: 10.3389/fimmu.2019.02673
121. Kim EK, Choi E-J. Compromised MAPK Signaling in Human Diseases: An Update. Arch Toxicol (2015) 89:867–82. doi: 10.1007/s00204-015-1472-2
122. Schoenborn JR, Wilson CB. Regulation of Interferon-Gamma During Innate and Adaptive Immune Responses. Adv Immunol (2007) 96:41–101. doi: 10.1016/S0065-2776(07)96002-2
123. Faria MS, Reis FCG, Lima APCA. Toll-Like Receptors in Leishmania Infections: Guardians or Promoters? J Parasitol Res (2012) 2012:930257. doi: 10.1155/2012/930257
124. Shio MT, Hassani K, Isnard A, Ralph B, Contreras I, Gomez MA, et al. Host Cell Signalling and Leishmania Mechanisms of Evasion. J Trop Med (2012) 2012:819512. doi: 10.1155/2012/819512
125. Bhardwaj S, Srivastava N, Sudan R, Saha B. Leishmania Interferes With Host Cell Signaling to Devise a Survival Strategy. J BioMed Biotechnol (2010) 2010:109189. doi: 10.1155/2010/109189
126. Guerrieri D, Tateosian NL, Maffía PC, Reiteri RM, Amiano NO, Costa MJ, et al. Serine Leucocyte Proteinase Inhibitor-Treated Monocyte Inhibits Human CD4+ Lymphocyte Proliferation. Immunology (2011) 133:434–41. doi: 10.1111/j.1365-2567.2011.03451.x
127. Vroling AB, Konijn T, Samsom JN, Kraal G. The Production of Secretory Leukocyte Protease Inhibitor by Dendritic Cells. Mol Immunol (2011) 48:630–6. doi: 10.1016/j.molimm.2010.11.002
128. Hiemstra PS. Novel Roles of Protease Inhibitors in Infection and Inflammation. Biochem Soc Trans (2002) 30:116–20.
129. McCartney-Francis N, Jin W, Belkaid Y, McGrady G, Wahl SM. Aberrant Host Defense Against Leishmania Major in the Absence of SLPI. J Leukocyte Biol (2014) 96:917–29. doi: 10.1189/jlb.4A0612-295RR
130. Lecoeur H, Prina E, Rosazza T, Kokou K, N’Diaye P, Aulner N, et al. Targeting Macrophage Histone H3 Modification as a Leishmania Strategy to Dampen the NF-κb/NLRP3-Mediated Inflammatory Response. Cell Rep (2020) 30:1870–82.e4. doi: 10.1016/j.celrep.2020.01.030
131. Swanson KV, Deng M, Ting JP-Y. The NLRP3 Inflammasome: Molecular Activation and Regulation to Therapeutics. Nat Rev Immunol (2019) 19:477–89. doi: 10.1038/s41577-019-0165-0
132. Lima-Junior DS, Costa DL, Carregaro V, Cunha LD, Silva ALN, Mineo TWP, et al. Inflammasome-Derived IL-1β Production Induces Nitric Oxide-Mediated Resistance to Leishmania. Nat Med (2013) 19:909–15. doi: 10.1038/nm.3221
133. Wu Z, Wang L, Li J, Wang L, Wu Z, Sun X. Extracellular Vesicle-Mediated Communication Within Host-Parasite Interactions. Front Immunol (2019) 9:3066. doi: 10.3389/fimmu.2018.03066
134. Atayde VD, Hassani K, da Silva Lira Filho A, Borges AR, Adhikari A, Martel C, et al. Leishmania Exosomes and Other Virulence Factors: Impact on Innate Immune Response and Macrophage Functions. Cell Immunol (2016) 309:7–18. doi: 10.1016/j.cellimm.2016.07.013
135. Tomiotto-Pellissier F, Miranda-Sapla MM, Silva TF, Bortoleti BT, da S, Gonçalves MD, et al. Murine Susceptibility to Leishmania Amazonensis Infection Is Influenced by Arginase-1 and Macrophages at the Lesion Site. Front Cell Infect Microbiol (2021) 11:687633. doi: 10.3389/fcimb.2021.687633
136. Paduch K, Debus A, Rai B, Schleicher U, Bogdan C. Resolution of Cutaneous Leishmaniasis and Persistence of Leishmania Major in the Absence of Arginase 1. J.I (2019) 202:1453–64. doi: 10.4049/jimmunol.1801249
137. Cuellar-Mata P, Jabado N, Liu J, Furuya W, Finlay BB, Gros P, et al. Nramp1 Modifies the Fusion of Salmonella Typhimurium-Containing Vacuoles With Cellular Endomembranes in Macrophages *. J Biol Chem (2002) 277:2258–65. doi: 10.1074/jbc.M105508200
138. Braliou GG, Kontou PI, Boleti H, Bagos PG. Susceptibility to Leishmaniasis is Affected by Host SLC11A1 Gene Polymorphisms: A Systematic Review and Meta-Analysis. Parasitol Res (2019) 118:2329–42. doi: 10.1007/s00436-019-06374-y
139. Blackwell JM, Searle S, Mohamed H, White JK. Divalent Cation Transport and Susceptibility to Infectious and Autoimmune Disease: Continuation of the Ity/Lsh/Bcg/Nramp1/Slc11a1 Gene Story. Immunol Lett (2003) 85:197–203. doi: 10.1016/s0165-2478(02)00231-6
140. Cassat JE, Skaar EP. Iron in Infection and Immunity. Cell Host Microbe (2013) 13:509–19. doi: 10.1016/j.chom.2013.04.010
141. Goncalves R, Christensen SM, Mosser DM. Humoral Immunity in Leishmaniasis – Prevention or Promotion of Parasite Growth? Cytokine: X (2020) 2:100046. doi: 10.1016/j.cytox.2020.100046
142. Parody N, Cacheiro-Llaguno C, Osuna C, Renshaw-Calderón A, Alonso C, Carnés J. Circulating Immune Complexes Levels Correlate With the Progression of Canine Leishmaniosis in Naturally Infected Dogs. Vet Parasitol (2019) 274:108921. doi: 10.1016/j.vetpar.2019.108921
143. Singh B, Singh OP, Singh N, Singh SS, Sundar S. Abnormal B-Cell Subset and Blimp-1–Mediated Humoral Responses Associated With Visceral Leishmaniasis Pathogenesis. Am J Trop Med Hyg (2019) 100:816–21. doi: 10.4269/ajtmh.18-0350
144. Firmino-Cruz L, Decote-Ricardo D, Gomes DC, de O, Morrot A, Freire-de-Lima CG, et al. How to B(e)-1 Important Cell During Leishmania Infection. Front Cell Infect Microbiol (2020) 9:424. doi: 10.3389/fcimb.2019.00424
145. Carpenter RO, Evbuomwan MO, Pittaluga S, Rose JJ, Raffeld M, Yang S, et al. B-Cell Maturation Antigen is a Promising Target for Adoptive T-Cell Therapy of Multiple Myeloma. Clin Cancer Res (2013) 19:2048–60. doi: 10.1158/1078-0432.CCR-12-2422
146. Rickert RC, Jellusova J, Miletic AV. Signaling by the Tumor Necrosis Factor Receptor Superfamily in B-Cell Biology and Disease. Immunol Rev (2011) 244:115–33. doi: 10.1111/j.1600-065X.2011.01067.x
147. Ghafouri-Fard S, Abak A, Tavakkoli Avval S, Shoorei H, Taheri M, Samadian M. The Impact of Non-Coding RNAs on Macrophage Polarization. BioMed Pharmacother (2021) 142:112112. doi: 10.1016/j.biopha.2021.112112
148. Liu Y, Wang X, Yang F, Zheng Y, Ye T, Yang L. Immunomodulatory Role and Therapeutic Potential of Non-Coding RNAs Mediated by Dendritic Cells in Autoimmune and Immune Tolerance-Related Diseases. Front Immunol (2021) 12:678918. doi: 10.3389/fimmu.2021.678918
149. Bayer-Santos E, Marini MM, da Silveira JF. Non-Coding RNAs in Host–Pathogen Interactions: Subversion of Mammalian Cell Functions by Protozoan Parasites. Front Microbiol (2017) 8:474. doi: 10.3389/fmicb.2017.00474
150. Heward JA, Lindsay MA. Long non-Coding RNAs in the Regulation of the Immune Response. Trends Immunol (2014) 35:408–19. doi: 10.1016/j.it.2014.07.005
151. Acosta J, Roa F, González-Chavarría I, Astuya A, Maura R, Montesino R, et al. In Vitro Immunomodulatory Activities of Peptides Derived From Salmo Salar NK-Lysin and Cathelicidin in Fish Cells. Fish Shellfish Immunol (2019) 88:587–94. doi: 10.1016/j.fsi.2019.03.034
152. Atsaves V, Leventaki V, Rassidakis GZ, Claret FX. AP-1 Transcription Factors as Regulators of Immune Responses in Cancer. Cancers (Basel) (2019) 11:1037. doi: 10.3390/cancers11071037
153. Davis SZ, Hollin T, Lenz T, Roch KGL. Three-Dimensional Chromatin in Infectious Disease—A Role for Gene Regulation and Pathogenicity? PLoS Pathog (2021) 17:e1009207. doi: 10.1371/journal.ppat.1009207
154. Smale ST, Tarakhovsky A, Natoli G. Chromatin Contributions to the Regulation of Innate Immunity. Annu Rev Immunol (2014) 32:489–511. doi: 10.1146/annurev-immunol-031210-101303
155. Golovatskĭ AS. [Degree of Chromatin Activity and the RNA Level in Lymphocytes of Popliteal Lymph Nodes of Dogs After Administration of an Antigen]. Arkh Anat Gistol Embriol (1990) 98:61–6.
156. Cheeseman K, Weitzman JB. Host–parasite Interactions: An Intimate Epigenetic Relationship. Cell Microbiol (2015) 17:1121–32. doi: 10.1111/cmi.12471
157. Saul D, Kosinsky RL. Epigenetics of Aging and Aging-Associated Diseases. Int J Mol Sci (2021) 22:401. doi: 10.3390/ijms22010401
Keywords: canine leishmaniosis, immunomodulation, parasite immune evasion, unfolded protein response, cytokine, T cell exhaustion, RNA-seq, lncRNA
Citation: Sanz CR, Miró G, Sevane N, Reyes-Palomares A and Dunner S (2022) Modulation of Host Immune Response during Leishmania infantum Natural Infection: A Whole-Transcriptome Analysis of the Popliteal Lymph Nodes in Dogs. Front. Immunol. 12:794627. doi: 10.3389/fimmu.2021.794627
Received: 13 October 2021; Accepted: 10 December 2021;
Published: 04 January 2022.
Edited by:
Silvia Ines Cazorla, Centro de Referencia para Lactobacilos (CERELA) (CONICET), ArgentinaReviewed by:
Maria Laura Belaunzaran, Consejo Nacional de Investigaciones Científicas y Técnicas (CONICET), ArgentinaCopyright © 2022 Sanz, Miró, Sevane, Reyes-Palomares and Dunner. This is an open-access article distributed under the terms of the Creative Commons Attribution License (CC BY). The use, distribution or reproduction in other forums is permitted, provided the original author(s) and the copyright owner(s) are credited and that the original publication in this journal is cited, in accordance with accepted academic practice. No use, distribution or reproduction is permitted which does not comply with these terms.
*Correspondence: Carolina R. Sanz, Y2Fyb3JvMDdAdWNtLmVz
Disclaimer: All claims expressed in this article are solely those of the authors and do not necessarily represent those of their affiliated organizations, or those of the publisher, the editors and the reviewers. Any product that may be evaluated in this article or claim that may be made by its manufacturer is not guaranteed or endorsed by the publisher.
Research integrity at Frontiers
Learn more about the work of our research integrity team to safeguard the quality of each article we publish.