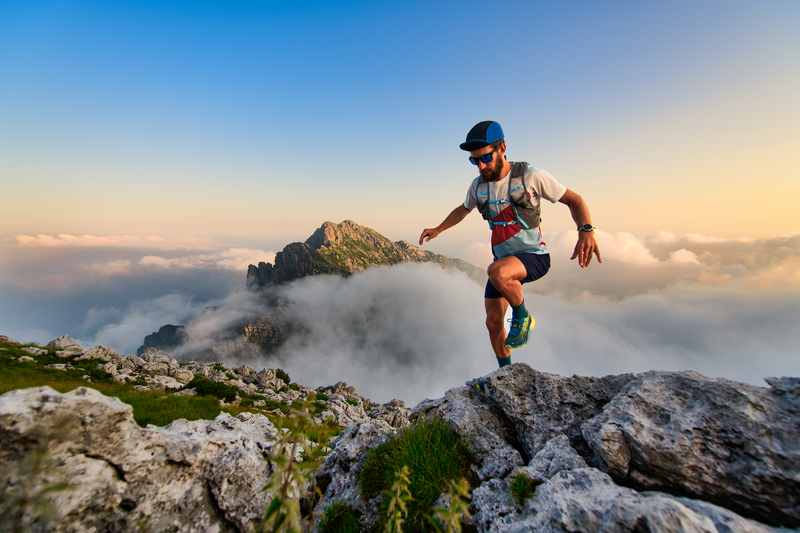
95% of researchers rate our articles as excellent or good
Learn more about the work of our research integrity team to safeguard the quality of each article we publish.
Find out more
REVIEW article
Front. Immunol. , 15 December 2021
Sec. Immunological Tolerance and Regulation
Volume 12 - 2021 | https://doi.org/10.3389/fimmu.2021.784473
This article is part of the Research Topic The Placenta, Fetomaternal Tolerance and Beyond: A Tribute to Sir Peter Medawar on the 60th Anniversary of his Nobel Prize View all 24 articles
Lectin-glycan interactions, in particular those mediated by the galectin family, regulate many processes required for a successful pregnancy. Over the past decades, increasing evidence gathered from in vitro and in vivo experiments indicate that members of the galectin family specifically bind to both intracellular and membrane bound carbohydrate ligands regulating angiogenesis, immune-cell adaptations required to tolerate the fetal semi-allograft and mammalian embryogenesis. Therefore, galectins play important roles in fetal development and placentation contributing to maternal and fetal health. This review discusses the expression and role of galectins during the course of pregnancy, with an emphasis on maternal immune adaptions and galectin-glycan interactions uncovered in the recent years. In addition, we summarize the galectin fingerprints associated with pathological gestation with particular focus on preeclampsia.
Almost fifty years ago (mid-1970s), galectins were first described as a family of evolutionarily conserved animal ß-galactoside binding lectins (1). Since then, the importance of galectins has been recognized in many biomedicine disciplines including inflammation, malignancy and reproductive biology. Galectins have extensive roles in the regulation of cell differentiation and function, but it is their ability to control the innate and adaptive immune system in healthy and disease states which has attracted significant interest. Galectins are well established regulators of lymphocytes, especially T lymphocyte development, differentiation, activation and effector function (2). Multiple members of the galectin family are widely expressed at the maternal-fetal interface where they play important roles in implantation, placental and fetal development, including in the regulation of maternal-fetal tolerance. Here, we review uterine and placental galectin expression, function, and glycan interactions at the maternal-fetal interface and highlight placental pathologies associated with an aberrant galectin signature.
Galectins are a highly evolutionarily conserved family, of which more than 20 galectins have been identified in mammals (3) and 12 in humans (4, 5). Galectins are found on many chromosomes including human chromosomes 1, 11, 14, 17, 19 and 22. A cluster of galectins found on chromosome 19 includes the placental-specific galectins (gal-13, -14, -16) (6), which are thought to be important in the formation of the highly invasive placenta found in humans. Galectins possess a characteristic carbohydrate recognition domain (CRD) and are classified according to their structure as prototype, chimera or tandem-repeat type (Figure 1) (7). Prototype galectins (gal-1, -2, -5, -7, -10, -11, -13, -14, -15 and -16) contain one CRD and typically form non-covalently linked homodimers (3). The only chimera-type galectin (gal-3) consists of a C-terminal CRD connected to a N-terminal ‘tail’ that facilitates oligomerization into trimers and pentamers (8). Tandem-repeat type galectins (gal-4, -6, -8, -9, -12) contain two distinct CRDs connected by a linker peptide (2, 3).
Figure 1 Types of galectins and general mechanisms. Shown is an illustration of the galectins structure and the functional interactions of this type of lectins with cell-surface and extracellular glycoconjugates.
Following synthesis in the cytoplasm, galectins are predominantly secreted into the extracellular space where they bind to carbohydrate ligands on the cell surface or in the extracellular matrix. Alternatively, they can be translocated to the nucleus, where they form part of the spliceosome (3). Galectins lack a classical secretion sequence; thus, galectin secretion is non-classical, bypassing the Golgi complex, likely effected by direct transport across the plasma membrane (3). Whilst the N-acetyllactosamine-enriched glycoconjugates binding capacity of galectins led to their discovery (9), galectins generally only weakly bind to β-galactoside-containing glycans (7). Due to the unique nature of their CRDs, galectins selectively bind to specific ligands, but this binding is complex, regulated in part by the physiological concentration of the lectin and multivalency and oligomeric state of the galectin and ligand (7). Indeed, the physiological ligands of many galectins are unknown. Altogether, this means that the functions of galectins are highly contextual and reflect a dynamic mechanism to regulate cell function (2).
The first member of the galectin family to be identified, gal-1, is a homodimeric protein that belongs to the prototype subgroup and is composed of two subunits of 14.5 kDa (10). Gal-1 is synthesized on cytosolic ribosomes and translocated to the extracellular compartment through a non-classical secretory pathway. Recently, it is been described that upon sensing cytosolic lipopolysaccharide (LPS), gal-1 is released from the cell by caspase-11 associated mechanisms, a process which is dependent on gasdermin D (11). Thus, gal-1 acts as a danger-associated molecules pattern (DAMP) in the extracellular space to enhance the inflammatory response elicited by intracellular LPS. However, the gal-1 secretion pathway in a physiological situation such as pregnancy and placentation has not been determined. Intracellularly, gal-1 is predominantly monomeric and participates in protein-protein interactions in a carbohydrate-independent manner (e.g. with H-Ras (12), protocadherin-24 (13) and gemin4 (14) among others). Once in the extracellular compartment, gal-1 spontaneously dimerizes and binds to numerous glycoproteins mostly in a glycan-dependent manner (15). Thus, gal-1 recognizes galactose-β1-4–N-acetyl-glucosamine [N-acetyl-lactosamine (LacNAc)] units present on the branches of N- or O-linked glycans on diverse cell surface receptors (e.g. CD45 on lymphocytes) and extracellular matrix (ECM) proteins including laminin and fibronectin (16–18) (Figure 1). Gal-1 displays broad anti-inflammatory properties and targets multiples immune cell types. This review focuses on gal-1’s significance within the maternal immune system during pregnancy and its influence on pregnancy outcome.
At the feto-maternal interface, a wide range of immune and non-immune cells synthetize and secrete gal-1 (3). Gal-1 was shown to be the most abundantly expressed in the endometrium –when compared to all other human tissues (19), where it is mainly localized to decidual stromal cells. Gal-1 expression is sex hormone-dependent, therefore its expression is increased in the late secretory phase endometrium and it is further elevated in the decidua (20). In vivo evidence also confirmed that sex hormones (estrogens and progesterone) regulate the expression of uterine gal-1, which is strongly increased during embryo implantation (21) and then sustained through pregnancy. Interestingly, in the course of the emergence of placental mammals, conserved cis motifs were gained including an estrogen responsive element in the 5′ promoter of LGALS1 (gal-1 gene) accounting for this sex steroid regulation of LGALS1 expression (19). Of importance, gal-1 is one of the most strongly expressed proteins in the decidua, mainly in decidual stromal cells, at term gestation, with decreased expression in laboring women (22). Taken together with its anti-inflammatory actions in decidual cells, measured by its inhibition of LPS-induced IL-6 production in decidua-derived mesenchymal cells (23), gal-1 may regulate decidual immune cell populations and sustain a local anti-inflammatory microenvironment that favors pregnancy maintenance, and its decreasing expression at term may allow the pro-inflammatory changes in the decidua needed for the onset of labor.
Besides maternal decidual stromal cells, another major source of gal-1 are the fetal trophoblasts as observed in various species. Early in mouse and human embryogenesis, gal-1 is detected starting in the fourth/five day of development and its expression is limited to the inner cells mass and outer cells (which includes the trophectoderm) (24, 25). The trophectoderm of the blastocyst exhibits highly differentiated functions and participates in a complex dialogue with maternal cells that enables implantation. Indeed, gal-1 expression is strong in the trophectoderm-derived giant cells (GC) and in spongiotrophoblast subsets, layers of the placenta that are involved in placental invasion and have endocrine functions (26, 27). Despite differences between mouse and human placentation, gal-1 expression is also prominent in the human trophoblast populations that carry out interstitial and endovascular invasion, and regulate the ability of extravillous trophoblasts to secrete immunoregulatory proteins such as HLA-G (25, 26, 28–30). Moreover, early-gestation chorionic villus–derived placenta mesenchymal stromal cells express prominent gal-1 on the surface and this lectin is abundant in secreted exosomes, which has been recently suggested to have neuroprotective effect (31). This opens up new avenues in the field of gal-1 as critical signaling molecule in the placenta secretome.
Decidual NK (dNK) cells are one of the best examples of how maternal immune-privileged sites shape the phenotype of leukocytes (32). dNK cells have increased gal-1 expression when compared to peripheral NK cells (33, 34). Toscano M et al. showed that gal-1 selectively controls the fate of Th1 and Th17 cells, due to the glycan-repertoire expressed by these T cells that is compatible with gal-1 binding, whereas Th2 cells are resistant to this lectin as a result of increased α2,6-sialylation of their cell surface glycoproteins (35). Based on their ability to secrete gal-1, dNK cells have been proposed to induce apoptosis of activated Th1/Th17 cells (33). It is not clear, however, whether the gal-1 expressed on dNK cells is also present in cytotoxic granulates as has been recently showed in CD8+ cytotoxic T lymphocytes (CTLs) (36). Interestingly, under inflammatory conditions such as during the course of preeclampsia, peripheral NK cell-subsets depicted a less prominent gal-1 expression, which may be responsible for the exacerbated Th1/Th17 systemic response (37). Although dNK cells generally have a less inflammatory phenotype compared to their peripheral counterparts, there is evidence for anti-viral activity of dNK cells (38).
With regards to antigen presenting cells in the decidua (e.g. dendritic cells, DCs), we found that exogenous gal-1 fine tunes the DC immunoregulatory properties (39). Specifically, gal-1 treated decidua DCs induced an IL-10 expressing Treg cell subset that is compatible with healthy gestation (39). In contrast, during a failing pregnancy, an uncontrolled Th1 response is accompanied by an immunogenic DC phenotype and decreased decidual gal-1 expression (39, 40). Although several gal-1 cell surface receptors including CD45, CD43, CD69, the pre-BCR, and vascular endothelial factor receptor 2 have been characterized, further studies are needed to identify the gal-1 receptor(s) at the feto-maternal interface responsible for the extracellular function of this lectin. In addition, characterization of the glycosylation profile of the potential decidual gal-1 ligands, will provide their functional relevance in the gal-1-glycan axis that fine tunes the immune response during gestation. Mast cells are found in mucosal and epithelial tissues including the feto-maternal interface and these cells secrete gal-1 during the course of pregnancy. Although mast cells are a small population, these cells are known to regulate vasodilation, vascular homeostasis, and angiogenesis. Therefore, the expression of gal-1 in this particular cell subtype can be related to increased angiogenesis and may indirectly regulate placentation (41).
Given the important immunomodulatory and anti-inflammatory roles of gal-1, it is unsurprising that dysregulated gal-1 production is found in complications of pregnancy associated with impaired immune tolerance including early pregnancy loss (42–44). Specifically, placental gal-1 expression and maternal blood gal-1 levels are downregulated in the first trimester in women who miscarry (25). In preeclampsia, another disease associated with inflammation, decreased maternal blood levels of gal-1 are found in the second trimester, while its increased expression in the placenta and elevated maternal blood concentrations can be detected at the time of clinical diagnosis (42–44). In murine models, loss of gal-1 causes preeclampsia-like features, exemplified by exacerbated inflammation and an anti-angiogenic maternal response, which is associated with reduced placental labyrinth area and impaired spiral artery remodeling (39, 45). Overall, these data suggest that a substantial decrease in gal-1 expression in early pregnancy may lead to the complete loss of tolerance and result in miscarriage in both species, while a lower level of inhibition of gal-1 expression at the feto-maternal interface, may enable the further progression of pregnancy but with disturbed maternal-fetal immune interactions, leading to preeclampsia and chronic rejection of the fetal semi-allograft. The observed discrepancy in gal-1 levels between the second and third trimesters in preeclampsia may reflect an initially inhibited expression of gal-1, which may result in a compensatory overexpression later in pregnancy similar to what is detected in rejected kidney allografts (46). Because preeclampsia and early and recurrent pregnancy loss (RPL) are syndromes rather than unique entities with diverse etiologies (47), is it important to emphasize that only a portion of the clinical cases exhibit the above immune/anti-angiogenic pathologies related to gal-1 dysregulation.
Galectin-2 (gal-2, encoded by the LGALS2 locus) belongs to the prototype subtype and its function at the feto-maternal interface is less understood than that of other galectins (1, 48). This lectin has been linked with pro- as well as anti-inflammatory actions (49, 50). The cellular binding sites for gal-2 are β1 integrin on T cells or closely associated glycoproteins (51). Binding of gal-2 to leukocytes results in cell-specific responses including apoptosis of activated T cells and regulation of leukocyte turnover (52, 53). In mice, gal-2 suppresses contact allergy reactions by inducting the apoptosis of activated CD8+ T cells; however, it has no significant effect on resting CD8+ T cells (54). Additionally, gal-2 has been attributed important inhibitory functions in monocyte and macrophage physiology, acting to inhibit monocyte migration and preventing macrophage-induced T cell activation (55). Thus, gal-2 was shown to shift T-cell cytokine profiles towards a Th2 phenotype, which is accompanied by downregulation of interferon (INF)-γ, tumor necrosis factor (TNF)-α and upregulation of IL-5 (52). In activated neutrophils, gal-2 induced externalization of phosphatidylserine leading to phagocytosis (53). The therapeutic potential of gal-2 has been demonstrated in acute and chronic mouse colitis disease models, where gal-2 induced a reduction in inflammation (49).
In the normal first trimester placenta, gal-2 is strongly expressed in the syncytiotrophoblasts (STB) with its cellular localization mainly found at the sites of interaction with the maternal blood. However, gal-2 expression is not restricted to the STBs as nuclear gal-2 expression has also been described in decidual cells (56). Expression of gal-2 within the placenta is reduced in pregnancy complications including miscarriages, preeclampsia and intrauterine growth restriction (IUGR) pregnancy (56–59). Preeclampsia not only causes a dysregulation of the placental gal-2 expression, but Charkiewicz et al. have shown that alterations of gal-2 levels can also be detected in the maternal circulation (60). However, whether gal-2 expression is causal for the development of miscarriage, preeclampsia or IUGR or if it is a consequence of failed trophoblast invasion is yet to be elucidated (61). Interestingly, gal-2 expression during normal pregnancy varies with fetal sex. Male placentas show more prominent gal-2 expression in the extravillous trophoblast compartment when compared to the age-matched female placentas (58). Thus, only male placentas suffering from IUGR showed a reduced gal-2 expression in this trophoblast population, whilst female placentas remained unchanged. A gender-specific role for gal-2 in the aetiology of preeclampsia and IUGR should be considered and further investigated.
DNA methylation in the LGALS2 gene may be an important mechanism to regulate gal-2 expression. It has been shown that maternal eating disorders affect offspring cord blood DNA methylation (62). In this context, offspring of women with active restrictive eating disorders in pregnancy had lower whole-genome methylation compared to offspring of women with past restrictive eating disorders (62). In addition, increased methylation at the LGALS2 locus could be identified in offspring of women with past eating disorder compared to controls (62).
Galectin 3 (gal-3, encoded by the LGALS3 locus) is the only chimera-type member of the family identified so far (8). Via its CRD, which is shaped as a cleft open at both ends, gal-3 exerts high affinity binding to poly LacNAc extensions of core 2 O- and complex N-glycans as well as to ABH blood group oligosaccharides (63).
Gal-3 is present both extracellularly and in various subcellular compartments including the cell membrane, nucleus and cytoplasm; where its unique chimeric structure allows it to interact with a variety of ligands to modulate specific processes including cell growth and survival, adhesion, migration, invasion, immune function and angiogenesis, all of which play a significant role during maternal adaptation to pregnancy. In the context of immune adaptations, a dual role in the regulation of apoptosis depending on its subcellular localization has been proposed for gal-3, promoting T cell apoptosis when secreted to the extracellular milieu (64, 65) and showing protective effects when acting intracellularly (66, 67). In addition, gal-3 has been shown to exert potent inhibitory effects on NK cell activation and function, by either binding specific core 2 O- glycan moieties on target cells (68, 69) or by directly interacting with NK cell activating receptors [i.e., NKp30, (70)]. Gal-3 is recognized as a potent modulator of innate and adaptive responses, being involved in the activation and differentiation of a variety of immune cell subsets. It can regulate several components of the acute inflammatory response including neutrophil activation and rolling (71, 72), chemoattraction of monocytes/macrophages (73) and mast cell degranulation (74). Among other mechanisms, gal-3 modulates adaptive immunity by enhancing tolerogenic (i.e., regulatory) IDO-expressing DC that support Treg expansion (75), acting extracellularly to inhibit TCR signaling at the immunological synapse (76) and by interfering with co-inhibitory receptor signaling [i.e., PD-1 and LAG-3, (77)]. Intracellularly, gal-3 appears to be critical for supporting OX-40 mediated development of memory CD8+ T cells following antigen exposure (78). Additionally, under sustained tissue damage, gal-3 can promote the transition to chronic inflammation, quenching T cell responses (64, 79) and facilitating the walling off of tissue injury with fibrogenesis and organ scarring (80). In damaged cells, the lectin can also function as a receptor for advanced glycation end products promoting accumulation of reactive oxygen species and endothelial dysfunction (81, 82), which are both considered a hallmark of preeclampsia pathogenesis. More recently, intracellular galectins including gal-3 have emerged as important mediators sensing tissue damage in the context of infections given their ability to recognize host glycans exposed to the cytosolic milieu upon rupture of endocytic vesicles or organelles (83), which in turn leads to activation of autophagy pathways and recruitment of antimicrobial factors. These functions, along with its proven ability to control immune responses through DAMP and (pathogen-associated molecular patterns) PAMP pathways (84, 85), make gal-3 an important mediator in both defense from microbial infections and development of autoimmune conditions. Determining whether these novel functions of gal-3 play a significant role during the resolution of infections at the maternal fetal interface represents an attractive subject for further research.
Despite considerable research over the past years, the functional implications of gal-3 in the context of pregnancy are only just emerging. In humans, gal-3 mRNA and protein can be detected in maternal decidual cells (86) and also in all trophoblast lineages of the placenta (87), with increased expression levels associated with differentiation of the cytotrophoblasts along the invasive extravillous trophoblast pathway (87, 88). In line with these findings, recent in vitro studies have identified gal-3 as part of the trophoblast invasion machinery (89) as well as a positive modulator of crucial trophoblast cell functions including capillary tube formation and syncytialization (88). In mice, gal-3 is detected primarily in the uterine luminal and glandular epithelium, where its timely up-regulation appears to be a requisite for successful implantation (90). A role for this lectin in driving proper placental function is further emphasized in a recent study, which demonstrated that Lgals3 deficiency is associated with impaired differentiation of trophoblast layers, enhanced decidual NK cell infiltration and cytotoxic degranulation, and defective vascularization resulting in asymmetric growth restriction due to placental insufficiency (91). Interestingly, these studies revealed a differential contribution of gal-3 to placental function depending on its source of expression, as the IUGR phenotype was only reproduced in mating models with Lgals3 deficiency of maternal origin. Furthermore, dysregulated placental expression of gal-3 associated with enhanced activation of cellular stress pathways was recently reported preceding the establishment of the maternal syndrome in an experimental model of preeclampsia superimposed on chronic hypertension (92).
During the course of pregnancy, maternal circulating gal-3 levels increase as pregnancy progresses (91). Dysregulation of systemic levels of gal-3 have been described in pregnancy complications, particularly the so-called ‘Great Obstetrical Syndromes’ including preeclampsia (42, 93), IUGR (91), preterm birth and premature rupture of membranes (94, 95), and spontaneous pregnancy loss (96), all of which are associated with disorders of deep placentation. However, an important limitation of these studies is the diversity of criteria used to establish patient cohorts, which leads to conflicting results that make it difficult to determine whether dysregulated gal-3 levels reflect a causative link or appear as consequence of the placental pathology. In this context, future studies evaluating placental biology, the regulation of vascular responses and maternal adaptations in experimental models with altered gal-3 expression represent exciting avenues of research to establish the precise physiological role of this lectin during pregnancy as well as its potential application in diagnosis and interventions in pathological settings.
Galectin-7 (gal-7) is a prototype galectin initially identified as a marker of stratified epithelia that is also expressed by many other types of epithelia and other cell types including lymphocytes (97). The LGALS7 gene is found on chromosome 19 (98), in a cluster of galectins which includes gal-4 and the placental-specific galectins gal-13, -14 and -16. In humans there is a duplicate copy of LGALS7 (LGALS7B) located adjacent to LGALS7 but on the opposite strand of chromosome 19 (99). Gal-7 is able to form homodimers with a ‘back-to-back’ organization and can be secreted despite having no cell secretion motif (97, 98). Gal-7 acts intracellularly via interactions with c-Jun N-terminal kinases, Ras or Bcl-2 and extracellularly via paracrine mechanisms to induce gene transcription, including autocrine amplification (100, 101, 102–106).
Gal-7 production is regulated by p53, TNF-α and NFĸB (97), but based on upstream promotor region sequencing it is predicted that LGALS7 and LGALS7B may each be regulated by different transcription factors (97, 99, 107). Studies to date suggest that gal-7 has key functions in epithelial cell homeostasis, including cell growth, differentiation and apoptosis as well as functions in cell adhesion, migration and immune cell regulation (101, 108;97, 105, 106, 109–116). The specific function of gal-7 likely relates to its cellular localization as gal-7 has been detected in the nuclear, cytosolic, mitochondrial and extracellular compartments (117).
Gal-7 has been suggested to play a key role in the intracellular immune response against infection. Gal-7 is recruited by Tollip to the autophagosome in HaCaT cells undergoing bacterial autophagy following group A Streptococcus infection (118). Gal-7 may also be considered an alarmin as it is released from human epithelial keratinocytes via an IL-4/IL-13/STAT6 dependent mechanism, it polarizes CD4+ T cells towards a Th1 phenotype and was found to be overexpressed in a murine model of cardiac allograft rejection (111, 115, 119).
In vivo administration of exogenous gal-7 to pregnant mice during mid-gestation results in a pro-inflammatory response with elevated placental IL-1β and IL-6 and reduced IL-10 mRNA production (120). Other reports however, indicate that gal-7 has anti-inflammatory actions treatment of PMA-stimulated Jurkat cells with gal-7 inhibits IL-2 and INF-γ production (112), gal-7 silencing elevates IL-17A-stimulated HaCaT cell secretion of IL-6 and IL-18 (107) and gal-7 is down-regulated in a mouse model of psoriasis (107). Therefore, the immune regulatory role of gal-7 may be cell-type specific and/or may depend on its cellular localization.
The absence of detectable gal-7 mRNA in term placenta (6) and the discovery that Lgals7 deficient mice are fertile and give rise to normal and fertile offspring initially suggested that gal-7 might not play an important role in female reproduction (121). More recently, immunohistochemical studies localized gal-7 to endometrial luminal and glandular epithelial cells, menstrual phase stromal cells and 1st trimester and term placental trophoblast including STB, cytotrophoblasts and extravillous trophoblasts (113, 114, 122, 123). The published reports also suggest that gal-7 localizes to uterine/decidua-resident immune cells however, the identity of the immune cells was not confirmed by dual staining (114, 122).
Exogenous gal-7 promotes endometrial epithelial cell adhesion and migration in vitro (113, 114). Gal-7 is present in menstrual fluid and in vitro studies suggest that it enhances re-epithelialization during endometrial repair after menstruation by promoting epithelial cell migration in a fibronectin/integrin dependent manner (113). Exogenous gal-7 enhances adhesion between human endometrial epithelial cells and the HTR-8/SVneo trophoblast cell line, suggesting that gal-7 may facilitate blastocyst adhesion to the uterine luminal epithelium during implantation. However, as gal-7 production is not up-regulated during the receptive phase of the menstrual cycle, it is unlikely that gal-7 is a critical mediator of blastocyst adhesion (114). Rather, somewhat paradoxically, endometrial epithelial production of gal-7 is increased in women with a history of multiple early pregnancy losses (114). Gal-7 is also abnormally elevated in maternal serum at 6 weeks gestation from women who subsequently miscarry but is not altered after week 7 of gestation (114, 124). The impact of even slightly increased gal-7 during early pregnancy may be substantial and sustained as it exhibits high stability due to the its protease resistance and increased stability following ligand binding (125). Elevated endometrial gal-7 may alter endometrial function leading to early pregnancy loss by increasing blastocyst-luminal epithelial adhesion allowing lower quality embryos to implant and altering the endometrial inflammatory environment by polarizing T cells towards a Th1 phenotype (114, 126–130).
Increased gal-7 production is found in early pregnancy chorionic villous samples (CVS; collected at 11-14 weeks gestation) from women who subsequently develop preterm preeclampsia (<37 weeks gestation) compared to women with uncomplicated pregnancies (120). A small retrospective cohort study also identified abnormally elevated gal-7 in maternal serum (collected at 10-20 weeks gestation) from women who subsequently developed preeclampsia (122). In vivo, gal-7 administration to pregnant mice causes preeclampsia-like features including hypertension, albuminuria and impaired placentation (120). Non-pregnant mice treated with gal-7 do not develop hypertension or albuminuria, demonstrating that gal-7 acts via the placenta to cause preeclampsia-like features in this model. Gal-7 regulates placental expression of many pathways thought to underlie the etiology of preeclampsia, including stimulating human placental villous production of sFlt-1-e15a, the sFlt-1 splice variant present only in the placenta of higher-order primates (120, 131). In mice, exogenous gal-7 induces a pro-inflammatory placental state (elevated IL-1β, IL-6 and reduced IL-10 mRNA) and alterations in the circulating and tissue renin-angiotensin-(aldosterone)-system (RAS) homeostasis (120, 132–137). To our knowledge this is the only mouse model of preeclampsia which causes alterations to RAS homeostasis without interventions to silence/overexpress specific RAS factors or surgically reducing uteroplacental perfusion and therefore could be a useful model to understand the role of RAS in the etiology of preeclampsia. Murine and ex vivo primary human trophoblast outgrowth experiments demonstrate that elevated placental gal-7 impairs trophoblast invasion (120) as is reported in preeclampsia (138). Gal-7’s inhibition of trophoblast invasion may occur via regulation of Pappalysin-2 and Disintegrin and metalloproteinase domain-containing protein 12 (ADAM12) (120) which are well established regulators of trophoblast migration (139–141).
Altogether these studies suggest that gal-7 likely has a role in the uterine and placental innate immune response and that it is produced at low levels during uncomplicated pregnancies. Elevated production of gal-7 during any stage of pregnancy may reflect or induce a pro-inflammatory state leading to poor pregnancy outcomes including RPL and preeclampsia.
This “tandem-repeat type” galectin encoded by the LGALS9 gene located on human chromosome 17 is a 34-39 kDa protein (142). Gal-9 undergoes post-transcriptional splicing to form many splice variants. Among tandem-repeat galectins, gal-9 has a wide expression pattern including the nuclear, cytoplasmic and extracellular compartments (143, 144). The exact mechanism of secretion for gal-9 is poorly understood, however, it is most likely to be secreted by one of the following non-classical secretory pathways: direct translocation across the plasma membrane, release via packaging in exosomes, or export via lysosomes, endosomes, and microvesicles (145, 146).
Among several identified gal-9 receptors, T-cell immunoglobulin mucin 3 (Tim-3) is the most extensively studied. TIM-3 was first discovered in 2002 on interferon IFN-γ producing CD4+ (Th1) and CD8+ T cytotoxic cells (147). TIM-3 expression was verified in various immune cells, including Th1, Th17, NK cells, NKT-like cells, γ/δ T cells, Tregs, MAIT-like cells, dendritic cells, monocytes and also on trophoblast such as giant cells (148–151). A growing body of evidence supports a role of Tim-3 signaling in shaping both the adaptive and innate immune responses (152). There is evidence that engagement of Tim-3 by its ligand gal-9 leads to the death of Th1 and Th17 cells, influencing the ability to induce T cell tolerance in both mice and humans (153–156). CD4+ T cells secrete gal-9 upon T-cell receptor activation resulting in the regulation of Th17/Treg development (157). Gal-9 suppresses Th17 cell differentiation and induces the apoptosis of Th1 and cytotoxic T cells while in mice, it has been shown to enhance regulatory T cell differentiation, suggesting immunosuppressive functions (158). Moreover IL-6 abrogates the increase of gal-9+ Th cells in vitro and indicates that the neutralization of IL-6 may be a strategy to increase gal-9+ Th cells to ameliorate Th1/Th17-skewed immunity (157). Thus, engagement of TIM-3 by gal-9 may function as a negative regulator, abrogating Th1- and Th17-driven immune responses and may modulate the Th1/Th2 balance. The outcome of this interaction could have essential roles in human pregnancy.
Gal-9 is highly expressed in the female reproductive tract and at the maternal-fetal interface (86, 159) (160). In mouse models, gal-9 plays a role in cell-to-cell interactions and the establishment of an immune-privileged local environment for implantation and early fetal development, as well as the mediation of decidual cell migration and chemotaxis (159). Both murine placental spongiotrophoblast and decidual regulatory T cells express gal-9: decidual gal-9+ Th cells are an important source of a secreted, soluble form of gal-9 (161). Endometrial stromal cells secrete gal-9 to suppress inflammatory reactions of uterine NK cells via Tim-3 [23]. The predominant gal-9 splice variant (Lgals9 D5) was found to downregulate IFN-γ production mouse dNK cells and therefore gal-9 may limit Th1 cell numbers (158). Available evidence also supports a similar role for gal-9 in human pregnancy. Gal-9 is expressed by human endometrial glandular epithelial cells (uterodomes) during the window-of-implantation. Post-implantation, gal-9 is highly produced by epithelial cells and stromal cells of the decidua during early pregnancy and by syncytiotrophoblast and cytotrophoblasts (160, 162, 163). Gal-9 is implicated in the regulation of dNK cell function and the maintenance of normal pregnancy as the expression of gal-9 by primary human trophoblasts induces the transformation of peripheral NK cells into dNK-like cells via the interaction with Tim-3 molecule (164). Treg cells increase their gal-9 expression as pregnancy progressed-coinciding with the increasing gal-9 level in maternal blood, suggesting that expression of gal-9 in the Tregs subset may have important roles in the maintenance of pregnancy (165).
The evidence discussed above suggests that gal-9 is implicated in the modulation of maternal immune tolerance to support fetal growth and development, therefore it is important to consider its role in pathologic pregnancies like spontaneous miscarriage, RPL, or preeclampsia. Normal pregnancy and cases of spontaneous abortions differ significantly in terms of endometrial gal-9 splice variant profiles in both a mouse model of spontaneous abortion (CBA/J females with DBA/2J males) and in humans (158). Placentas from abortion-prone mice had lower mRNA levels of gal-9 compared to the normal placenta (158). Tim-3 expression by dNK cells from human miscarriages and abortion-prone mouse models is also reduced compared to healthy pregnancies, and the function of Tim-3+ dNK cells has been shown to be impaired (164). Moreover, decreased Th2 cytokine and increased Th1 cytokine levels were observed in Tim-3+ dNK cells, but not in those Tim-3- dNK cells derived from human and murine miscarriages (164). These data indicate that both the frequency and the function of Tim-3+ dNK cells are abnormal in miscarriage. In addition, women with a history of RPL have lower levels of circulating gal-9 compared to healthy pregnant women and reduced Tim-3 expression by peripheral NK cells (166, 167). The dysregulation on the gal-9/Tim-3 axis is accompanied by a decrease of systemic TGF-β1 levels, which has been implicated in the upregulation of Tim-3 expression (162, 168). Besides these changes, an increase of soluble Tim-3 (sTim-3) in addition to the reduced gal-9 circulating levels has been reported in these patients, which could enhance the competitive binding of gal-9 by sTim-3 leading to failed inhibitory signals controlling inflammation (166). A therapeutic potential of recombinant (r)gal-9 has been shown in preclinical models of several diseases like transplant rejections and autoimmune condition (169). The use of rgal-9 in RPL patients might be an effective therapeutic strategy to restore maternal-fetal immune tolerance.
The gal-9/Tim-3 pathway has also been implicated in maternal systemic inflammation in early-onset preeclampsia, however further analyses are urgently needed for a better understanding of this axis (170, 171). Decreased Tim-3 expression by T cells, cytotoxic T cells, γ/δ T cells, NK cells, and CD56dim NK cells, as well as increased frequency of gal-9+ peripheral lymphocytes is detected in women with early-onset preeclampsia (148, 170). Hao et al. found that the gal-9 expression detected by immunofluorescence in decidual tissues from preeclamptic patients was significantly higher compared to the control group (171). These data suggest that the impairment of the gal-9/Tim-3 pathway can result in an enhanced systemic inflammatory response, including the activation of Th1 lymphocytes in early-onset preeclampsia. Furthermore, Li et al. demonstrated that exogenous rgal-9 administration alleviates the preeclampsia-like manifestations (characterized by insufficient trophoblast cell invasion and impaired spiral artery remodeling) in a rat model of preeclampsia induced by LPS by upregulating Tim-3 expression in decidual macrophages (172). This finding may be related to the activation of the gal-9/Tim-3 signaling pathway, which promotes decidual macrophage polarization shifting to M2 subtype (172). Thus, the gal-9/Tim-3 axis may provide a valuable target for clinical interventional immunotherapy for early-onset preeclampsia although the findings that the interaction of gal-9 with Tim-3 in innate immune cells such as monocytes and dendritic cells induces the secretion of pro-inflammatory cytokines including TNF-α and can synergize with Toll-like receptors should be taken into consideration (173). Further functional evidence is needed to better understand the in vivo extracellular functions of gal-9.
Gal-13 was discovered by Bohn et al. as Placental Protein 13 (PP13) when systematically isolating >50 pure proteins from the human placenta and characterizing them with physico-chemical methods (174). Using the purified PP13 protein and anti-PP13 antisera, Than et al. isolated and sequence analyzed the full-length cDNA encoding PP13, and described PP13 to be placenta-specifically expressed (175). They also discovered close placenta-specific galectin relatives of gal-13, and first characterized their evolution, structure, expression and functions (6, 176–182). Other groups, pioneered by Meiri et al. explored the clinical utilization of gal-13/PP13 immunoassays in maternal blood in normal and complicated pregnancies (183–186), as well as the potential use of gal-13/PP13 therapy for preeclampsia (187–193). As a result of systematic work, almost four decades of increasing international collaboration delineated the pivotal role of placental galectins in maternal-fetal immune-interactions and their promising diagnostic and therapeutic potentials for pregnancy complications.
The gene encoding gal-13 (LGALS13) is located on chromosome 19 within a cluster of galectin genes and pseudogenes, which emerged via birth-end-death evolution in anthropoid primates. Evolutionary analysis suggested that this cluster were originated from an ancestral mono-CRD galectin and underwent multiple gene duplications, rearrangements and losses as well as sequential divergence and subfunctionalization (4, 6). In humans, five genes belong to this galectin cluster, out of which three (LGALS13, LGALS14, LGALS16) are uniquely expressed by the placenta (4, 6). RNA and protein level evidences demonstrated predominant expression of these galectins in the syncytiotrophoblast but not in the underlying progenitor cytotrophoblast, with lower expression in extravillous trophoblasts, amnion epithelium and fetal endothelium (6, 177, 178, 181). The expression of these galectin genes is developmentally regulated during villous trophoblast differentiation and syncytialization, with the strongest expression observed for LGALS14 and LGALS13 due to promoter evolution (181). The insertion of a primate-specific transposable element into the upstream region of an ancestral galectin gene introduced several binding sites for transcription factors fundamental for placental gene expression, leading to the gain of placental expression of these galectins. Further promoter changes via duplication or insertion of transposable elements led to varying placental expression levels of these galectin genes (181). For gal-13, it is released from the syncytiotrophoblast into the maternal circulation by secretion or shedding of microparticles (178, 179, 183, 194, 195). Due to their similar structural and expressional characters, we may suppose that gal-14 and gal-16 behave similarly to gal-13 regarding their placenta-maternal transfer.
The placenta-specific galectins structurally belong to the “proto-type” sub-group of galectins, which have a single CRD (6, 176, 177, 196). The topologies of these CRDs revealed by homology modelling are similar to other prototype galectins, often called as “jelly-roll” structure, although their amino acid sequence has considerably diverged during evolution (4, 6, 176, 197). In fact, adaptive evolution occurred in the CRDs of newly emerged genes in the LGALS13 clade, followed by conservation of residues in descendant lineages, suggesting that placenta-specific galectins have acquired and sustained important novel functions in anthropoids (4, 6). From the eight conserved residues in the CRD, three that are key for overall sugar binding were subject to strong purifying selection, while five residues crucial for the binding of galactose or glucose moieties were replaced in several lineages following gene duplications, which have contributed to differences in their CRDs (6). In accordance with this evolutionary evidence, in vitro sugar-binding assays and in silico ligand docking simulations showed that placenta-specific galectins have sugar-binding capabilities different from other galectins (6, 176, 177, 180), which was recently also supported by X-ray crystallographic studies (198–201).
The first in vitro functional assays with recombinant placenta-specific galectins demonstrated their pro-apoptotic capability on activated T lymphocytes in a similar extent as gal-1, thus, it was proposed that these galectins emerged to reduce the danger of maternal immune attacks on the fetal semi-allograft and to confer additional immune tolerance mechanisms in anthropoid primates, supporting their hemochorial placentation during long gestation (6, 197). Later, gal-13/gal-14 were shown to increase the rate of late-apoptotic T cells irrespective of their activation status (182). Since the surface expression of CD95 was also induced by gal-13/gal-14 treatment, these galectins may increase the sensitivity of activated T cells for activation-induced cell death (182). T cytotoxic cells bound more galectins and were more susceptible to gal-13/gal-14 induced apoptosis than T helper cells, probably due to their differential glycosylation patterns (182). An interesting observation was made on extracellular gal-13 aggregates localized around decidual veins in the first trimester (202). These crystal-like aggregates were associated with immune cell-containing zones of necrosis. It was hypothesized that syncytiotrophoblast-secreted gal-13 drains from the intervillous space into decidual veins, where it forms perivenous aggregates, which attract, activate and kill maternal immune cells while facilitating local tolerance for trophoblasts to invade and convert maternal spiral arterioles.
Unlike T cells, neutrophils cultured with recombinant gal-13 do not undergo apoptosis (203). Gal-13 did not interfere either with neutrophil extracellular trap (NET) release, degranulation, phagocytosis, or bacteria-induced reactive oxygen species (ROS) response, but induced increased expression of programmed death-ligand 1 (PD-L1), hepatocyte growth factor (HGF), vascular endothelial growth factor (VEGF), matrix metalloproteinase 9 (MMP-9), and TNFα (203). Thus, gal-13 may shift neutrophils towards a placental-growth-permissive phenotype, while maintaining all their primary functions and abilities to respond to bacterial invasion (203). In addition, gal-13/gal-14 stimulated IL-8 production in non-activated T cells (182), which is interesting in the context of the pro-angiogenic effect recombinant (204), decidual neutrophil-secreted or dNK cell secreted IL-8 (205–207). Since the pro-angiogenic effects of other galectins has been established as reviewed elsewhere (208), it is tempting to hypothesize that gal-13/gal-14 may induce angiogenesis at the maternal-fetal interface via the induction of T-cell secreted IL-8. Indeed, recent in vivo studies showed that exogenous gal-13 can induce 1) the vasodilatation of rat isolated uterine arteries by activating the endothelial prostaglandin and nitric oxide (NO) pathways (188, 189); 2) expansive remodeling of uterine veins and arteries (192); 3) drop in the blood pressure of rats (187, 188); and the 4) increase of placental and pup weights (188). These functional properties of placental galectins are remarkable especially in the context what we have learnt from the placental origins of pregnancy complications, especially preeclampsia, a multifactorial syndrome, in which disturbances in trophoblast-immune cell interactions and vascular remodeling are in the center of pathologies (209, 210).
Placental expression of gal-13 has been shown to be dysregulated before and after the preeclampsia onset, as evidenced at the RNA level in CVS first trimester samples, and remains at lower expression patterns by the time of disease diagnosis (178, 179, 181, 211, 212). A similar placental down-regulation was observed for gal-14 at the third trimester, and since its regulation of expression is strongly linked to that of gal-13 (181), we hypothesize that placental gal-14 behaves similarly to gal-13 in early pregnancy. Their down-regulation may lead to disturbed immune cell interactions and reduced trophoblast invasion as well as abnormal spiral artery remodeling and angiogenesis, leading to the ischemic stress of the placenta, which are central to the pathogenesis of preterm preeclampsia. In parallel with this, lower gal-13 concentrations are detected in the maternal circulation during the first trimester of women who subsequently developed preterm preeclampsia compared to healthy pregnant women by many international clinical studies, with some earliest ones referenced here (183–186, 197, 213–216). Due to its superior biomarker properties, gal-13 was assessed to be included into first trimester risk assessment of preterm preeclampsia by these and other studies. A seemingly conflicting observation was made, with preterm preeclamptic pregnancies showing a steep increase in maternal blood gal-13 concentrations during the second trimester, reaching significantly higher levels in the third trimester compared to healthy pregnancies (197). This phenomenon was elucidated to be due to the ischemic placental stress and the consequently augmented shedding of gal-13 from the placenta into the maternal circulation via trophoblastic microvesicles (178, 179, 195, 197, 217). Thus, placental galectins, especially gal-13, turned out to be promising early biomarkers of abnormal deep placentation. Moreover, encouraging studies are being performed for the replenishment of gal-13 to restore immune balance and inhibit the development of one of the most severe obstetrical syndrome, preeclampsia.
In addition to placental galectins, the fetus-derived syncytiotrophoblast, which is the major interface with the material circulation and represents the endocrine tissue of the placenta, secretes the pregnancy-specific glycoproteins (PSGs). PSG1 or Schwangerschafts protein 1 (SP1), as it was originally called, is one of 10 human PSGs and is increasingly secreted into the maternal circulation with advancing gestation. PSGs have been studied regarding their potential for being a biomarker for pregnancy complications and trophoblastic diseases for more than four decades although specific antibodies for the different PSGs are not available (218, 219). Recently, PSG expression was also observed in a subpopulation of extravillous trophoblasts at the mRNA and protein levels (220, 221). The presence of invasive trophoblast cells and the direct contact of maternal immune cells with fetal tissue as found in species with hemochorial placentas and in equine endometrial cups is hypothesized to have favored the evolution and expansion of PSGs, which exist only in a minority of mammals (222). The number of PSG genes varies greatly; while new world monkeys have 3 PSG genes, some old world monkeys have 17 (222). Humans have 10 PSG-encoding genes and splice variants that differ by the number of Ig-like domains or length of the cytoplasmic tail have been described (223). Interestingly, copy number variations are common in the human PSG locus and while some functions are shared by all members of the family, it remains to be determined whether during evolution, some members of the family have adopted new functions and bind to different ligands (224, 225). Therefore, whether higher PSG gene dosage and expression levels of individual PSGs confers an adaptive advantage or it is detrimental during pregnancy remains unknown (226).
Within the human PSGs, PSG1 is one of the highest expressed and the best studied (222, 227, 228). PSG1 has pro-angiogenic activity and immunomodulatory actions by binding to the latency-associated peptides of the anti-inflammatory cytokines TGF-β1 and TGF-β2 resulting in their activation and increase in the number of T regulatory cells (229–232). PSG1 also interacts with two integrins, α5β1 and αIIbβ3, resulting in its ability to modulate extravillous trophoblast adhesion and migration and the interaction of platelets with fibrinogen, respectively (221, 227). We found that the concentration of PSG1 is lower in African American women diagnosed with preeclampsia when pregnant with a male fetus (233).
The high expression level of PSG1 during the third trimester permitted the isolation of native PSG1 from pooled serum of pregnant women using affinity chromatography allowing for a comprehensive glycomic and glycoproteomic investigation of the native protein (234). PSG1 has seven potential N-linked glycosylation sites across its four Ig-like domains designates as N, A1, A2 and B2 but only four sites mapping to the N, A1 and A2 domains of the protein were confirmed to be occupied by glycans (234). The presence of multiantennary and poly-N-acetyl-lactosamine (LacNac) elongated moieties with mainly alpha2,3-linked sialic acid terminals, suggested that PSG1 could interact with members of the galectin family. Because gal-1 has a well-established modulatory role in pregnancy-associated processes, the interaction between PSG1 and gal-1 has been investigated in detail (3, 39). Gal-1 binds to native and recombinant PSG1 in a carbohydrate-dependent manner, demonstrated by the inhibition of PSG1-gal-1 binding by lactose and failure of PSG1 generated in insect cells or N-acetylglucosaminlytransferase I (GnTI)-deficient cells, which carry only mannose-type glycans, to interact with gal-1. In addition, removal of N-linked oligosaccharides from the N- and A2- PSG1 domains by treatment with the amidase PNGase F prevented their binding to gal-1 (234). Changes in glycosylation could add further complexity to the regulation of important biological processes in pregnancy known to be regulated by both extracellular galectins and their ligands, including the PSGs. At present, whether glycosylation of PSGs and its ability to interact with galectins differs with gestational age or during pathological pregnancies has not been investigated. In addition, whether all members of the PSG family within a species or in the different species in which these proteins are expressed interact with gal-1 or other members of the galectin family has not been established and should be further explored.
Galectins’ extracellular functions are mainly believed to be the result of their lectin properties, but intracellular functions, which are independent of their ability to bind glycans, result from protein-protein interactions (Figure 2 and Table 1). The interaction of placental- or maternal- derived glycoproteins with galectins could potentially modulate the activity of some galectins once they are secreted from a cell. The final outcome of galectin-pregnancy specific glycoproteins interactions will depend on their concentration, that of other galectin ligands, and the affinity of the interactions. In addition, while only investigated for a few glycoproteins, the presence of specific glycan structures in proteins may vary at different times during gestation or under pathological pregnancy conditions. In this review, we have focused on the role of galectins in controlling the maternal immune adaptation to gestation; however, the galectins-glycans axis could modulate maternal immune response in the context of inflammation induced by microbes which requires further investigation. Deciphering those interactions will help to understand the critical role of glycoimmunology in the maternal adaptations and provide novel diagnostic and therapeutic targets for pregnancy complications characterized by aberrant glycosylation and imbalance of galectins.
EM and SMB planned and wrote the review article, GD and NGT edited the manuscript. NGT (human gal-1 and placental galectins), UJ (gal-2), GB (gal-3), LS (gal-9), GD (PSG) contributed to the mentioned section. NGT and SMB drafted the manuscript figures. All authors contributed to the article and approved the submitted version.
The writing of this review was supported by the Deutsche Forschungsgemeinschaft (BL1115/2-1, BL1115/4-1, Heisenberg program BL1115/7-1), and Heike Wolfgang Mühlbauer Stiftung to SMB. EM is supported by Mid-Career Fellowship, Department of Obstetrics and Gynecology, The University of Melbourne. NGT is supported by the Hungarian Academy of Sciences (Momentum LP2014-7/2014 grant) and the Hungarian National Research, Development, and Innovation Office (FIEK_16-1-2016-0005 and OTKA K124862 grants). GD is supported by the National Institutes of Health, USA (NIAID grant # R21AI156058).
The authors declare that the research was conducted in the absence of any commercial or financial relationships that could be construed as a potential conflict of interest.
All claims expressed in this article are solely those of the authors and do not necessarily represent those of their affiliated organizations, or those of the publisher, the editors and the reviewers. Any product that may be evaluated in this article, or claim that may be made by its manufacturer, is not guaranteed or endorsed by the publisher.
We deeply thank the past and present members of our laboratories who have made important contributions to this work.
1. Barondes SH, Castronovo V, Cooper DN, Cummings RD, Drickamer K, Feizi T, et al. Galectins: A Family of Animal Beta-Galactoside-Binding Lectins. Cell (1994) 76:597–8. doi: 10.1016/0092-8674(94)90498-7
2. Giovannone N, Smith LK, Treanor B, Dimitroff CJ. Galectin-Glycan Interactions as Regulators of B Cell Immunity. Front Immunol (2018) 9. doi: 10.3389/fimmu.2018.02839
3. Blois SM, Dveksler G, Vasta GR, Freitag N, Blanchard V, Barrientos G. Pregnancy Galectinology: Insights Into a Complex Network of Glycan Binding Proteins. Front Immunol (2019) 10:1166–6. doi: 10.3389/fimmu.2019.01166
4. Than NG, Romero R, Kim CJ, Mcgowen MR, Papp Z, Wildman DE. Galectins: Guardians of Eutherian Pregnancy at the Maternal-Fetal Interface. Trends Endocrinol Metab (2012) 23:23–31. doi: 10.1016/j.tem.2011.09.003
5. Than NG, Romero R, Balogh A, Karpati E, Mastrolia SA, Staretz-Chacham O, et al. Galectins: Double-Edged Swords in the Cross-Roads of Pregnancy Complications and Female Reproductive Tract Inflammation and Neoplasia. J Pathol Transl Med (2015) 49:181–208. doi: 10.4132/jptm.2015.02.25
6. Than NG, Romero R, Goodman M, Weckle A, Xing J, Dong Z, et al. A Primate Subfamily of Galectins Expressed at the Maternal Fetal Interface That Promote Immune Cell Death. Proc Natl Acad Sci (2009) 106:9731–6. doi: 10.1073/pnas.0903568106
7. Cummings RD, Liu FT, Vasta GR. “Galectins,”. In: Varki A, Cummings RD, Esko JD, Stanley P, Hart GW, Aebi M, Darvill AG, Kinoshita T, Packer NH, Prestegard JH, Schnaar RL, Seeberger PH, editors. Essentials of Glycobiology. Cold Spring Harbor NY: Cold Spring Harbor Laboratory Press (2015) Copyright 2015-2017 by The Consortium of Glycobiology Editors, La Jolla, California. All Rights Reserved. pp. 469–80.
8. Barondes SH, Cooper DN, Gitt MA, Leffler H. Galectins. Structure and Function of a Large Family of Animal Lectins. J Biol Chem (1994) 269:20807–10. doi: 10.1016/S0021-9258(17)31891-4
9. Johannes L, Jacob R, Leffler H. Galectins at a Glance. J Cell Sci (2018) 131:jcs208884. doi: 10.1242/jcs.208884
10. Lee RT, Ichikawa Y, Allen HJ, Lee YC. Binding Characteristics of Galactoside-Binding Lectin (Galaptin) From Human Spleen. J Biol Chem (1990) 265:7864–71. doi: 10.1016/S0021-9258(19)39011-8
11. Russo AJ, Vasudevan SO, Méndez-Huergo SP, Kumari P, Menoret A, Duduskar S, et al. Intracellular Immune Sensing Promotes Inflammation via Gasdermin D–driven Release of a Lectin Alarmin. Nat Immunol (2021) 22:154–65. doi: 10.1038/s41590-020-00844-7
12. Paz A, Haklai R, Elad-Sfadia G, Ballan E, Kloog Y. Galectin-1 Binds Oncogenic H-Ras to Mediate Ras Membrane Anchorage and Cell Transformation. Oncogene (2001) 20:7486–93. doi: 10.1038/sj.onc.1204950
13. Ose R, Oharaa O, Nagase T. Galectin-1 and Galectin-3 Mediate Protocadherin-24-Dependent Membrane Localization of β-Catenin in Colon Cancer Cell Line Hct116. Curr Chem Genomics (2012) 6:18–26. doi: 10.2174/1875397301206010018
14. Park JW, Voss PG, Grabski S, Wang JL, Patterson RJ. Association of Galectin-1 and Galectin-3 With Gemin4 in Complexes Containing the SMN Protein. Nucleic Acids Res (2001) 29:3595–602. doi: 10.1093/nar/29.17.3595
15. Earl LA, Bi S, Baum LG. Galectin Multimerization and Lattice Formation are Regulated by Linker Region Structure. Glycobiology (2011) 21:6–12. doi: 10.1093/glycob/cwq144
16. Cho M, Cummings RD. Galectin-1, a Beta-Galactoside-Binding Lectin in Chinese Hamster Ovary Cells. I. Physical and Chemical Characterization. J Biol Chem (1995) 270:5198–206. doi: 10.1074/jbc.270.10.5198
17. Ozeki Y, Matsui T, Yamamoto Y, Funahashi M, Hamako J, Titani K. Tissue Fibronectin is an Endogenous Ligand for Galectin-1. Glycobiology (1995) 5:255–61. doi: 10.1093/glycob/5.2.255
18. Nguyen JT, Evans DP, Galvan M, Pace KE, Leitenberg D, Bui TN, et al. CD45 Modulates Galectin-1-Induced T Cell Death: Regulation by Expression of Core 2 O-Glycans. J Immunol (2001) 167:5697–707. doi: 10.4049/jimmunol.167.10.5697
19. Than NG, Romero R, Erez O, Weckle A, Tarca AL, Hotra J, et al. Emergence of Hormonal and Redox Regulation of Galectin-1 in Placental Mammals: Implication in Maternal-Fetal Immune Tolerance. Proc Natl Acad Sci USA (2008) 105:15819–24. doi: 10.1073/pnas.0807606105
20. Mulac-Jeričević B, Šućurović S, Gulic T, Szekeres-Bartho J. The Involvement of the Progesterone Receptor in PIBF and Gal-1 Expression in the Mouse Endometrium. Am J Reprod Immunol (2019) 81:e13104. doi: 10.1111/aji.13104
21. Choe YS, Shim C, Choi D, Lee CS, Lee KK, Kim K. Expression of Galectin-1 mRNA in the Mouse Uterus Is Under the Control of Ovarian Steroids During Blastocyst Implantation. Mol Reprod Dev (1997) 48:261–6. doi: 10.1002/(SICI)1098-2795(199710)48:2<261::AID-MRD14>3.0.CO;2-0
22. El-Azzamy H, Balogh A, Romero R, Xu Y, Lajeunesse C, Plazyo O, et al. Characteristic Changes in Decidual Gene Expression Signature in Spontaneous Term Parturition. J Pathol Transl Med (2017) 51:264–83. doi: 10.4132/jptm.2016.12.20
23. Gómez-Chávez F, Castro-Leyva V, Espejel-Núñez A, Zamora-Mendoza RG, Rosas-Vargas H, Cancino-Díaz JC, et al. Galectin-1 Reduced the Effect of LPS on the IL-6 Production in Decidual Cells by Inhibiting LPS on the Stimulation of Iκbζ. J Reprod Immunol (2015) 112:46–52. doi: 10.1016/j.jri.2015.07.002
24. Colnot C, Ripoche MA, Scaerou F, Foulis D, Poirier F. Galectins in Mouse Embryogenesis. Biochem Soc Trans (1996) 24:141–6. doi: 10.1042/bst0240141
25. Tirado-Gonzalez I, Freitag N, Barrientos G, Shaikly V, Nagaeva O, Strand M, et al. Galectin-1 Influences Trophoblast Immune Evasion and Emerges as a Predictive Factor for the Outcome of Pregnancy. Mol Hum Reprod (2013) 19:43–53. doi: 10.1093/molehr/gas043
26. Tang M, You J, Wang W, Lu Y, Hu X, Wang C, et al. Impact of Galectin-1 on Trophoblast Stem Cell Differentiation and Invasion in In Vitro Implantation Model. Reprod Sci (2018) 25:700–11. doi: 10.1177/1933719117725816
27. You JL, Wang W, Tang MY, Ye YH, Liu AX, Zhu YM. A Potential Role of Galectin-1 in Promoting Mouse Trophoblast Stem Cell Differentiation. Mol Cell Endocrinol (2018) 470:228–39. doi: 10.1016/j.mce.2017.11.003
28. Vicovac L, Jankovic M, Cuperlovic M. Galectin-1 and -3 in Cells of the First Trimester Placental Bed. Hum Reprod (1998) 13:730–5. doi: 10.1093/humrep/13.3.730
29. Kolundžić N, Bojić-Trbojević Ž., Kovačević T, Stefanoska I, Kadoya T, Vićovac L. Galectin-1 Is Part of Human Trophoblast Invasion Machinery–a Functional Study In Vitro. PloS One (2011) 6:e28514. doi: 10.1371/journal.pone.0028514
30. Barrientos G, Freitag N, Tirado-Gonzalez I, Unverdorben L, Jeschke U, Thijssen VL, et al. Involvement of Galectin-1 in Reproduction: Past, Present and Future. Hum Reprod Update (2014) 20:175–93. doi: 10.1093/humupd/dmt040
31. Kumar P, Becker JC, Gao K, Carney RP, Lankford L, Keller BA, et al. Neuroprotective Effect of Placenta-Derived Mesenchymal Stromal Cells: Role of Exosomes. FASEB journal: Off Publ Fed Am Societies Exp Biol (2019) 33:5836–49. doi: 10.1096/fj.201800972R
32. Sanguansermsri D, Pongcharoen S. Pregnancy Immunology: Decidual Immune Cells. Asian Pac J Allergy Immunol (2008) 26:171–81.
33. Kopcow HD, Rosetti F, Leung Y, Allan DS, Kutok JL, Strominger JL. T Cell Apoptosis at the Maternal-Fetal Interface in Early Human Pregnancy, Involvement of Galectin-1. Proc Natl Acad Sci USA (2008) 105:18472–7. doi: 10.1073/pnas.0809233105
34. Kopcow HD, Eriksson M, Mselle TF, Damrauer SM, Wira CR, Sentman CL, et al. Human Decidual NK Cells From Gravid Uteri and NK Cells From Cycling Endometrium are Distinct NK Cell Subsets. Placenta (2010) 31:334–8. doi: 10.1016/j.placenta.2010.01.003
35. Toscano MA, Bianco GA, Ilarregui JM, Croci DO, Correale J, Hernandez JD, et al. Differential Glycosylation of TH1, TH2 and TH-17 Effector Cells Selectively Regulates Susceptibility to Cell Death. Nat Immunol (2007) 8:825–34. doi: 10.1038/ni1482
36. Clemente T, Vieira NJ, Cerliani JP, Adrain C, Luthi A, Dominguez MR, et al. Proteomic and Functional Analysis Identifies Galectin-1 as a Novel Regulatory Component of the Cytotoxic Granule Machinery. Cell Death & Dis (2017) 8:e3176–6. doi: 10.1038/cddis.2017.506
37. Molvarec A, Blois SM, Stenczer B, Toldi G, Tirado-Gonzalez I, Ito M, et al. Peripheral Blood Galectin-1-Expressing T and Natural Killer Cells in Normal Pregnancy and Preeclampsia. Clin Immunol (2011) 139:48–56. doi: 10.1016/j.clim.2010.12.018
38. Quillay H, El Costa H, Duriez M, Marlin R, Cannou C, Madec Y, et al. NK Cells Control HIV-1 Infection of Macrophages Through Soluble Factors and Cellular Contacts in the Human Decidua. Retrovirology (2016) 13:39. doi: 10.1186/s12977-016-0271-z
39. Blois SM, Ilarregui JM, Tometten M, Garcia M, Orsal AS, Cordo-Russo R, et al. A Pivotal Role for Galectin-1 in Fetomaternal Tolerance. Nat Med (2007) 13:1450–7. doi: 10.1038/nm1680
40. Blois SM, Kammerer U, Alba Soto C, Tometten MC, Shaikly V, Barrientos G, et al. Dendritic Cells: Key to Fetal Tolerance? Biol Reprod (2007) 77:590–8. doi: 10.1095/biolreprod.107.060632
41. Woidacki K, Popovic M, Metz M, Schumacher A, Linzke N, Teles A, et al. Mast Cells Rescue Implantation Defects Caused by C-Kit Deficiency. Cell Death & Dis (2013) 4:e462–2. doi: 10.1038/cddis.2012.214
42. Jeschke U, Mayr D, Schiessl B, Mylonas I, Schulze S, Kuhn C, et al. Expression of Galectin-1, -3 (Gal-1, Gal-3) and the Thomsen-Friedenreich (TF) Antigen in Normal, IUGR, Preeclamptic and HELLP Placentas. Placenta (2007) 28:1165–73. doi: 10.1016/j.placenta.2007.06.006
43. Than NG, Erez O, Wildman DE, Tarca AL, Edwin SS, Abbas A, et al. Severe Preeclampsia Is Characterized by Increased Placental Expression of Galectin-1. J Matern Fetal Neonatal Med (2008) 21:429–42. doi: 10.1080/14767050802041961
44. Hirashima C, Ohkuchi A, Nagayama S, Suzuki H, Takahashi K, Ogoyama M, et al. Galectin-1 as a Novel Risk Factor for Both Gestational Hypertension and Preeclampsia, Specifially its Expression at a Low Level in the Second Trimester and a High Level After Onset. Hypertens Res (2018) 41:45–52. doi: 10.1038/hr.2017.85
45. Freitag N, Tirado-Gonzalez I, Barrientos G, Herse F, Thijssen VL, Weedon-Fekjaer SM, et al. Interfering With Gal-1-Mediated Angiogenesis Contributes to the Pathogenesis of Preeclampsia. Proc Natl Acad Sci USA (2013) 110:11451–6. doi: 10.1073/pnas.1303707110
46. Van Der Leij J, Van Den Berg A, Blokzijl T, Harms G, Van Goor H, Zwiers P, et al. Dimeric Galectin-1 Induces IL-10 Production in T-Lymphocytes: An Important Tool in the Regulation of the Immune Response. J Pathol (2004) 204:511–8. doi: 10.1002/path.1671
47. Than NG, Romero R, Hillermann R, Cozzi V, Nie G, Huppertz B. Prediction of Preeclampsia – A Workshop Report. Placenta (2008) 29:83–5. doi: 10.1016/j.placenta.2007.10.008
48. Cooper DN, Barondes SH. God Must Love Galectins; He Made So Many of Them. Glycobiology (1999) 9:979–84. doi: 10.1093/glycob/9.10.979
49. Paclik D, Berndt U, Guzy C, Dankof A, Danese S, Holzloehner P, et al. Galectin-2 Induces Apoptosis of Lamina Propria T Lymphocytes and Ameliorates Acute and Chronic Experimental Colitis in Mice. J Mol Med (Berl) (2008) 86:1395–406. doi: 10.1007/s00109-007-0290-2
50. Yildirim C, Vogel DY, Hollander MR, Baggen JM, Fontijn RD, Nieuwenhuis S, et al. Galectin-2 Induces a Proinflammatory, Anti-Arteriogenic Phenotype in Monocytes and Macrophages. PloS One (2015) 10:e0124347. doi: 10.1371/journal.pone.0124347
51. Sedlář A, Trávníčková M, Bojarová P, Vlachová M, Slámová K, Křen V, et al. Interaction Between Galectin-3 and Integrins Mediates Cell-Matrix Adhesion in Endothelial Cells and Mesenchymal Stem Cells. Int J Mol Sci (2021) 22:5144. doi: 10.3390/ijms22105144
52. Sturm A, Lensch M, Andre S, Kaltner H, Wiedenmann B, Rosewicz S, et al. Human Galectin-2: Novel Inducer of T Cell Apoptosis With Distinct Profile of Caspase Activation. J Immunol (2004) 173:3825–37. doi: 10.4049/jimmunol.173.6.3825
53. Stowell SR, Karmakar S, Stowell CJ, Dias-Baruffi M, Mcever RP, Cummings RD. Human Galectin-1, -2, and -4 Induce Surface Exposure of Phosphatidylserine in Activated Human Neutrophils But Not in Activated T Cells. Blood (2007) 109:219–27. doi: 10.1182/blood-2006-03-007153
54. Loser K, Sturm A, Voskort M, Kupas V, Balkow S, Auriemma M, et al. Galectin-2 Suppresses Contact Allergy by Inducing Apoptosis in Activated CD8+ T Cells. J Immunol (2009) 182:5419–29. doi: 10.4049/jimmunol.0802308
55. Paclik D, Werner L, Guckelberger O, Wiedenmann B, Sturm A. Galectins Distinctively Regulate Central Monocyte and Macrophage Function. Cell Immunol (2011) 271:97–103. doi: 10.1016/j.cellimm.2011.06.003
56. Hutter S, Martin N, Von Schonfeldt V, Messner J, Kuhn C, Hofmann S, et al. Galectin 2 (Gal-2) Expression is Downregulated on Protein and mRNA Level in Placentas of Preeclamptic (PE) Patients. Placenta (2015) 36:438–45. doi: 10.1016/j.placenta.2015.01.198
57. Ristich V, Liang S, Zhang W, Wu J, Horuzsko A. Tolerization of Dendritic Cells by HLA-G. Eur J Immunol (2005) 35:1133–42. doi: 10.1002/eji.200425741
58. Hutter S, Knabl J, Andergassen U, Hofmann S, Kuhn C, Mahner S, et al. Placental Expression Patterns of Galectin-1, Galectin-2, Galectin-3 and Galectin-13 in Cases of Intrauterine Growth Restriction (IUGR). Int J Mol Sci (2016) 17:523. doi: 10.3390/ijms17040523
59. Unverdorben L, Haufe T, Santoso L, Hofmann S, Jeschke U, Hutter S. Prototype and Chimera-Type Galectins in Placentas With Spontaneous and Recurrent Miscarriages. Int J Mol Sci (2016) 17:644. doi: 10.3390/ijms17050644
60. Charkiewicz K, Goscik J, Raba G, Laudanski P. Syndecan 4, Galectin 2, and Death Receptor 3 (DR3) as Novel Proteins in Pathophysiology of Preeclampsia. J Matern Fetal Neonatal Med (2019) 34(18):2965–70. doi: 10.1080/14767058.2019.1676410
61. Naljayan MV, Karumanchi SA. New Developments in the Pathogenesis of Preeclampsia. Adv Chronic Kidney Dis (2013) 20:265–70. doi: 10.1053/j.ackd.2013.02.003
62. Kazmi N, Gaunt TR, Relton C, Micali N. Maternal Eating Disorders Affect Offspring Cord Blood DNA Methylation: A Prospective Study. Clin Epigenet (2017) 9:120. doi: 10.1186/s13148-017-0418-3
63. Seetharaman J, Kanigsberg A, Slaaby R, Leffler H, Barondes SH, Rini JM. X-Ray Crystal Structure of the Human Galectin-3 Carbohydrate Recognition Domain at 2.1-A Resolution. J Biol Chem (1998) 273:13047–52. doi: 10.1074/jbc.273.21.13047
64. Fukumori T, Takenaka Y, Yoshii T, Kim HR, Hogan V, Inohara H, et al. CD29 and CD7 Mediate Galectin-3-Induced Type II T-Cell Apoptosis. Cancer Res (2003) 63:8302–11.
65. Karlsson A, Christenson K, Matlak M, Bjorstad A, Brown KL, Telemo E, et al. Galectin-3 Functions as an Opsonin and Enhances the Macrophage Clearance of Apoptotic Neutrophils. Glycobiology (2009) 19:16–20. doi: 10.1093/glycob/cwn104
66. Moon BK, Lee YJ, Battle P, Jessup JM, Raz A, Kim HR. Galectin-3 Protects Human Breast Carcinoma Cells Against Nitric Oxide-Induced Apoptosis: Implication of Galectin-3 Function During Metastasis. Am J Pathol (2001) 159:1055–60. doi: 10.1016/S0002-9440(10)61780-4
67. Yu F, Finley RL Jr, Raz A, Kim HR. Galectin-3 Translocates to the Perinuclear Membranes and Inhibits Cytochrome C Release From the Mitochondria. A Role for Synexin in Galectin-3 Translocation. J Biol Chem (2002) 277:15819–27. doi: 10.1074/jbc.M200154200
68. Tsuboi S, Sutoh M, Hatakeyama S, Hiraoka N, Habuchi T, Horikawa Y, et al. A Novel Strategy for Evasion of NK Cell Immunity by Tumours Expressing Core2 O-Glycans. EMBO J (2011) 30:3173–85. doi: 10.1038/emboj.2011.215
69. Suzuki Y, Sutoh M, Hatakeyama S, Mori K, Yamamoto H, Koie T, et al. MUC1 Carrying Core 2 O-Glycans Functions as a Molecular Shield Against NK Cell Attack, Promoting Bladder Tumor Metastasis. Int J Oncol (2012) 40:1831–8. doi: 10.3892/ijo.2012.1411
70. Wang W, Guo H, Geng J, Zheng X, Wei H, Sun R, et al. Tumor-Released Galectin-3, a Soluble Inhibitory Ligand of Human NKp30, Plays an Important Role in Tumor Escape From NK Cell Attack. J Biol Chem (2014) 289:33311–9. doi: 10.1074/jbc.M114.603464
71. Yamaoka A, Kuwabara I, Frigeri LG, Liu FT. A Human Lectin, Galectin-3 (Epsilon Bp/Mac-2), Stimulates Superoxide Production by Neutrophils. J Immunol (1995) 154:3479–87.
72. Kuwabara I, Liu FT. Galectin-3 Promotes Adhesion of Human Neutrophils to Laminin. J Immunol (1996) 156:3939–44.
73. Sano H, Hsu DK, Yu L, Apgar JR, Kuwabara I, Yamanaka T, et al. Human Galectin-3 is a Novel Chemoattractant for Monocytes and Macrophages. J Immunol (2000) 165:2156–64. doi: 10.4049/jimmunol.165.4.2156
74. Frigeri LG, Zuberi RI, Liu FT. Epsilon BP, a Beta-Galactoside-Binding Animal Lectin, Recognizes IgE Receptor (Fc Epsilon RI) and Activates Mast Cells. Biochemistry (1993) 32:7644–9. doi: 10.1021/bi00081a007
75. Volarevic V, Zdravkovic N, Harrell CR, Arsenijevic N, Fellabaum C, Djonov V, et al. Galectin-3 Regulates Indoleamine-2,3-Dioxygenase-Dependent Cross-Talk Between Colon-Infiltrating Dendritic Cells and T Regulatory Cells and May Represent a Valuable Biomarker for Monitoring the Progression of Ulcerative Colitis. Cells (2019) 8:709. doi: 10.3390/cells8070709
76. Gilson RC, Gunasinghe SD, Johannes L, Gaus K. Galectin-3 Modulation of T-Cell Activation: Mechanisms of Membrane Remodelling. Prog Lipid Res (2019) 76:101010. doi: 10.1016/j.plipres.2019.101010
77. Kouo T, Huang L, Pucsek AB, Cao M, Solt S, Armstrong T, et al. Galectin-3 Shapes Antitumor Immune Responses by Suppressing CD8+ T Cells via LAG-3 and Inhibiting Expansion of Plasmacytoid Dendritic Cells. Cancer Immunol Res (2015) 3:412–23. doi: 10.1158/2326-6066.CIR-14-0150
78. Amani MF, Rolig AS, Redmond WL. Intracellular Galectin-3 Is Essential for OX40-Mediated Memory CD8(+) T Cell Development. J Immunol (2020) 205:1857–66. doi: 10.4049/jimmunol.1901052
79. Morgan R, Gao G, Pawling J, Dennis JW, Demetriou M, Li B. N-Acetylglucosaminyltransferase V (Mgat5)-Mediated N-Glycosylation Negatively Regulates Th1 Cytokine Production by T Cells. J Immunol (2004) 173:7200–8. doi: 10.4049/jimmunol.173.12.7200
80. Henderson NC, Sethi T. The Regulation of Inflammation by Galectin-3. Immunol Rev (2009) 230:160–71. doi: 10.1111/j.1600-065X.2009.00794.x
81. Vlassara H, Li YM, Imani F, Wojciechowicz D, Yang Z, Liu FT, et al. Identification of Galectin-3 as a High-Affinity Binding Protein for Advanced Glycation End Products (AGE): A New Member of the AGE-Receptor Complex. Mol Med (1995) 1:634–46. doi: 10.1007/BF03401604
82. Luis C, Costa R, Rodrigues I, Castela A, Coelho P, Guerreiro S, et al. Xanthohumol and 8-Prenylnaringenin Reduce Type 2 Diabetes-Associated Oxidative Stress by Downregulating Galectin-3. Porto BioMed J (2019) 4:e23. doi: 10.1016/j.pbj.0000000000000023
83. Hong MH, Weng IC, Li FY, Lin WH, Liu FT. Intracellular Galectins Sense Cytosolically Exposed Glycans as Danger and Mediate Cellular Responses. J BioMed Sci (2021) 28:16. doi: 10.1186/s12929-021-00713-x
84. Sato S, Bhaumik P, St-Pierre G, Pelletier I. Role of Galectin-3 in the Initial Control of Leishmania Infection. Crit Rev Immunol (2014) 34:147–75. doi: 10.1615/CritRevImmunol.2014010154
85. Vasta GR, Feng C, Gonzalez-Montalban N, Mancini J, Yang L, Abernathy K, et al. Functions of Galectins as ‘Self/Non-Self’-Recognition and Effector Factors. Pathog Dis (2017) 75:ftx046. doi: 10.1093/femspd/ftx046
86. Von Wolff M, Wang X, Gabius HJ, Strowitzki T. Galectin Fingerprinting in Human Endometrium and Decidua During the Menstrual Cycle and in Early Gestation. Mol Hum Reprod (2005) 11:189–94. doi: 10.1093/molehr/gah144
87. Maquoi E, Van Den Brule FA, Castronovo V, Foidart JM. Changes in the Distribution Pattern of Galectin-1 and Galectin-3 in Human Placenta Correlates With the Differentiation Pathways of Trophoblasts. Placenta (1997) 18:433–9. doi: 10.1016/S0143-4004(97)80044-6
88. Freitag N, Tirado-Gonzalez I, Barrientos G, Cohen M, Daher S, Goldman-Wohl D, et al. The Chimera-Type Galectin-3 is a Positive Modulator of Trophoblast Functions With Dysregulated Expression in Gestational Diabetes Mellitus. Am J Reprod Immunol (2020) 84:e13311. doi: 10.1111/aji.13311
89. Bojic-Trbojevic Z, Jovanovic Krivokuca M, Vilotic A, Kolundzic N, Stefanoska I, Zetterberg F, et al. Human Trophoblast Requires Galectin-3 for Cell Migration and Invasion. Sci Rep (2019) 9:2136. doi: 10.1038/s41598-018-38374-w
90. Yang H, Lei C, Zhang W. Expression of Galectin-3 in Mouse Endometrium and Its Effect During Embryo Implantation. Reprod BioMed Online (2012) 24:116–22. doi: 10.1016/j.rbmo.2011.09.003
91. Freitag N, Tirado-Gonzalez I, Barrientos G, Powell KL, Boehm-Sturm P, Koch SP, et al. Galectin-3 Deficiency in Pregnancy Increases the Risk of Fetal Growth Restriction (FGR) via Placental Insufficiency. Cell Death Dis (2020) 11:560. doi: 10.1038/s41419-020-02791-5
92. Blois SM, Prince PD, Borowski S, Galleano M, Barrientos G. Placental Glycoredox Dysregulation Associated With Disease Progression in an Animal Model of Superimposed Preeclampsia. Cells (2021) 10:800. doi: 10.3390/cells10040800
93. Pankiewicz K, Szczerba E, Fijalkowska A, Szamotulska K, Szewczyk G, Issat T, et al. The Association Between Serum Galectin-3 Level and its Placental Production in Patients With Preeclampsia. J Physiol Pharmacol (2020) 71:845–56. doi: 10.26402/jpp.2020.6.08
94. Stefanoska I, Tadic J, Vilotic A, Jovanovic Krivokuca M, Abu Rabi T, Vicovac L. Histological Chorioamnionitis in Preterm Prelabor Rupture of the Membranes is Associated With Increased Expression of Galectin-3 by Amniotic Epithelium. J Matern Fetal Neonatal Med (2017) 30:2232–6. doi: 10.1080/14767058.2016.1243100
95. Kaya B, Turhan U, Sezer S, Kaya S, Dag I, Tayyar A. Maternal Serum Galectin-1 and Galectin-3 Levels in Pregnancies Complicated With Preterm Prelabor Rupture of Membranes. J Matern Fetal Neonatal Med (2020) 33:861–8. doi: 10.1080/14767058.2019.1637409
96. Xiao Q, Zeng FL, Tang GY, Lei CY, Zou XX, Liu XL, et al. Expression of Galectin-3 and Apoptosis in Placental Villi From Patients With Missed Abortion During Early Pregnancy. Exp Ther Med (2019) 17:2623–31. doi: 10.3892/etm.2019.7227
97. Advedissian T, Deshayes F, Viguier M. Galectin-7 in Epithelial Homeostasis and Carcinomas. Int J Mol Sci (2017) 18:2760. doi: 10.3390/ijms18122760
98. Madsen P, Rasmussen HH, Flint T, Gromov P, Kruse TA, Honoré B, et al. Cloning, Expression, and Chromosome Mapping of Human Galectin-7. J Biol Chem (1995) 270:5823–9. doi: 10.1074/jbc.270.11.5823
99. Kaltner H, Raschta A-S, Manning JC, Gabius HJ. Copy-Number Variation of Functional Galectin Genes: Studying Animal Galectin-7 (P53-Induced Gene 1 in Man) and Tandem-Repeat-Type Galectins-4 and -9. Glycobiology (2013) 23:1152–63. doi: 10.1093/glycob/cwt052
100. Kuwabara I, Kuwabara Y, Yang R-Y, Schuler M, Green DR, Zuraw BL, et al. Galectin-7 Exhibits Pro-Apoptotic Function Through JNK Activation and Mitochondrial Cytochrome Crelease. J Biol Chem (2002) 277:3487–97. doi: 10.1074/jbc.M109360200
101. Demers M, Magnaldo T, St-Pierre Y. A Novel Function for Galectin-7: Promoting Tumorigenesis by Up-Regulating MMP-9 Gene Expression. Cancer Res (2005) 65:5205–10. doi: 10.1158/0008-5472.CAN-05-0134
102. Rabinovich GA, Toscano MA. Turning ‘Sweet’ on Immunity: Galectin-Glycan Interactions in Immune Tolerance and Inflammation. Nat Rev Immunol (2009) 9:338–52. doi: 10.1038/nri2536
103. Villeneuve C, Baricault L, Canelle L, Barboule N, Racca C, Monsarrat B, et al. Mitochondrial Proteomic Approach Reveals Galectin-7 as a Novel BCL-2 Binding Protein in Human Cells. Mol Biol Cell (2011) 22:999–1013. doi: 10.1091/mbc.e10-06-0534
104. Bibens-Laulan N, St-Pierre Y. Intracellular Galectin-7 Expression in Cancer Cell Results From an Autocrine Transcriptional Mechanism and Endocytosis of Extracellular Galectin-7. PloS One (2017) 12:e0187194. doi: 10.1371/journal.pone.0187194
105. Guo J-P, Li X-G. Galectin-7 Promotes the Invasiveness of Human Oral Squamous Cell Carcinoma Cells via Activation of ERK and JNK Signaling. Oncol Lett (2017) 13:1919–24. doi: 10.3892/ol.2017.5649
106. Sun X, Zhang W. Silencing of Gal-7 Inhibits TGF-β1-Induced Apoptosis of Human Airway Epithelial Cells Through JNK Signaling Pathway. Exp Cell Res (2019) 375:100–5. doi: 10.1016/j.yexcr.2018.12.017
107. Chen H-L, Lo C-H, Huang C-C, Lu M-P, Hu P-Y, Chen C-S, et al. Galectin-7 Downregulation in Lesional Keratinocytes Contributes to Enhanced IL-17A Signaling and Skin Pathology in Psoriasis. J Clin Invest (2021) 131:e130740. doi: 10.1172/JCI130740
108. Cao Z, Said N, Amin S, Wu HK, Bruce A, Garate M, et al. Galectins-3 and -7, But Not Galectin-1, Play a Role in Re-Epithelialization of Wounds. J Biol Chem (2002) 277:42299–305. doi: 10.1074/jbc.M200981200
109. Park JE, Chang WY, Cho M. Induction of Matrix Metalloproteinase-9 by Galectin-7 Through P38 MAPK Signaling in HeLa Human Cervical Epithelial Adenocarcinoma Cells. Oncol Rep (2009) 22:1371–9. doi: 10.3892/or_00000577
110. Lee J-S, Lee YS, Jeon B, Jeon YJ, Too H, Kim T-Y. EC-SOD Induces Apoptosis Through COX-2 and Galectin-7 in the Epidermis. J Dermatol Sci (2012) 65:126–33. doi: 10.1016/j.jdermsci.2011.12.013
111. Luo Z, Ji Y, Zhou H, Huang X, Fang J, Guo H, et al. Galectin-7 in Cardiac Allografts in Mice: Increased Expression Compared With Isografts and Localization in Infiltrating Lymphocytes and Vascular Endothelial Cells. Transplant Proc (2013) 45:630–4. doi: 10.1016/j.transproceed.2012.12.005
112. Yamaguchi T, Hiromasa K, Kabashima-Kubo R, Yoshioka M, Nakamura M. Galectin-7, Induced by Cis-Urocanic Acid and Ultraviolet B Irradiation, Down-Modulates Cytokine Production by T Lymphocytes. Exp Dermatol (2013) 22:832–49. doi: 10.1111/exd.12268
113. Evans J, Yap J, Gamage T, Salamonsen LA, Dimitriadis E, Menkhorst EM. Galectin-7 is Important for Nomal Uterine Repair Following Menstruation. Mol Hum Reprod (2014) 20:787–98. doi: 10.1093/molehr/gau032
114. Menkhorst EM, Gamage T, Cuman C, Kaitu’u-Lino TJ, Tong S, Dimitriadis E. Galectin-7 Acts as an Adhesion Molecule During Implantation and Increased Expression is Associated With Miscarriage. Placenta (2014) 35:195–201. doi: 10.1016/j.placenta.2014.01.004
115. Luo Z, Ji Y, Tian D, Zhang Y, Chang S, Yang C, et al. Galectin-7 Promotes Proliferation and Th1/2 Cells Polarization Toward Th1 in Activated CD4+ T Cells by Inhibiting The Tgfβ/Smad3 Pathway. Mol Immunol (2018) 101:80–5. doi: 10.1016/j.molimm.2018.06.003
116. Menkhorst E, Griffith M, Van Sinderen M, Niven K, Dimitriadis E. Galectin-7 is Elevated in Endometroid (Type 1) Endometrial Cancer and Promotes Cell Migration. Oncol Lett (2018) 16:4721–8. doi: 10.3892/ol.2018.9193
117. Saussez S, Kiss R. Galectin-7. Cell Mol Life Sci (2006) 63:686–97. doi: 10.1007/s00018-005-5458-8
118. Lin C-Y, Nozawa T, Minowa-Nozawa A, Toh H, Hikichi M, Iibushi J, et al. Autophagy Receptor Tollip Facilitates Bacterial Autophagy by Recruiting Galectin-7 in Response to Group A Streptococcus Infection. Front Cell Infect Microbiol (2020) 10:583137. doi: 10.3389/fcimb.2020.583137
119. Umayahara T, Shimauchi T, Iwasaki M, Sakabe JI, Aoshima M, Nakazawa S, et al. Protective Role of Galectin-7 for Skin Barrier Impairment in Atopic Dermatitis. Clin Exp Allergy (2020) 50:922–31. doi: 10.1111/cea.13672
120. Menkhorst E, Zhou W, Santos L, Delforce S, So T, Rainczuk K, et al. Galectin-7 Impairs Placentation and Causes Preeclampsia Features in Mice. Hypertension (2020) 76:1185–94. doi: 10.1161/HYPERTENSIONAHA.120.15313
121. Gendronneau G, Sidhy SS, Delacour D, Dang T, Calonne C, Houzelstein D, et al. Galectin-7 in the Control of Epidermal Homeostasis After Injury. Mol Biol Cell (2008) 19:5541–9. doi: 10.1091/mbc.e08-02-0166
122. Menkhorst E, Koga K, Van Sinderen M, Dimitriadis E. Galectin-7 Serum Levels are Altered Prior to the Onset of Pre-Eclampsia. Placenta (2014) 35:281–5. doi: 10.1016/j.placenta.2014.01.009
123. Unverdorben L, Jesche U, Santoso L, Hofmann S, Kuhn C, Arck P, et al. Comparative Analyses on Expression of Galectins1-4, 7-10 and 12 in First Trimester Placenta, Decidua and Isolated Trophoblast Cells In Vitro. Histol Histopathol (2016) 31:1095–111. doi: 10.14670/HH-11-739
124. Cagli F, Uysal G, Oz L, Akkaya H, Aksoy H, Ulku Karakilic E, et al. Serum Galectin 7 is Not Useful to Predict Abortion in the First Trimester. Fetal Pediatr Pathol (2018) 37:141–6. doi: 10.1080/15513815.2018.1453001
125. Cummings RD, Liu F-T. “Galectins,”. In: Varki A, Cummings RD, Esko JD, Freeze HH, Stanley P, Bertozzi CR, Hart GW, Etzler ME, editors. Essentials of Glycobiology, 2 ed. Cold Spring Harbor (NY: Cold Spring Harbor Laboratory Press (2009).
126. Saito S, Nakashima A, Shima T, Ito M. Th1/Th2/Th17 and Regulatory T-Cell Paradigm in Pregnancy. Am J Reprod Immunol (2010) 63:601–10. doi: 10.1111/j.1600-0897.2010.00852.x
127. Teklenburg G, Salker M, Molokhia M, Lavery S, Trew G, Aojanepong T, et al. Natural Selection of Human Embryos: Decidualizing Endometrial Stromal Cells Serve as Sensors of Embryo Quality Upon Implantation. PloS One (2010) 5:e10258. doi: 10.1371/journal.pone.0010258
128. Inada K, Shima T, Nakashima A, Aoki K, Ito M, Saito S. Characterization of Regulatory T Cells in Decidua of Miscarriage Cases With Abnormal or Normal Fetal Chromosomal Content. J Reprod Immunol (2013) 97:104–11. doi: 10.1016/j.jri.2012.12.001
129. Brosens JJ, Salker M, Teklenburg G, Nautiyal J, Salter S, Lucas ES, et al. Uterine Selection of Human Embryos at Implantation. Sci Rep (2014) 4:3894. doi: 10.1038/srep03894
130. Dimitriadis E, Menkhorst E, Saito S, Kutteh W, Brosens J. Recurrent Pregnancy Loss. Nat Rev Dis Primers (2020) 2020:98. doi: 10.1038/s41572-020-00228-z
131. Palmer K, Tong S, Kaitu’u-Lino TJ. Placental-Specific sFLT-1: Role in Pre-Eclamptic Pathophysiology and its Translational Possibilities for Clinical Prediction and Diagnosis. Mol Hum Reprod (2017) 23:69–78. doi: 10.1093/molehr/gaw077
132. Herse F, Dechend R, Harsem N, Wallukat G. Dysregulation of the Circulating and Tissue-Based Renin-Angiotensin System in Preeclampsia. Hypertension (2007) 49:604–11. doi: 10.1161/01.HYP.0000257797.49289.71
133. Amash A, Holcberg G, Sapir O, Huleilel M. Placental Secretion of Interleukin-1 and Interleukin-1 Receptor Antagonist in Preeclampsia: Effect of Magnesium Sulfate. J Interferon & Cytokine Res (2012) 32:432–41. doi: 10.1089/jir.2012.0013
134. Bernardi FC, Felisberto F, Vuolo F, Petronilho F, Souza DR, Luciano TF, et al. Oxidative Damage, Inflammation, and Toll-Like Receptor 4 Pathway are Increased in Preeclamptic Patients: A Case-Control Study. Oxid Med Cell Longevity (2012) 2012:636419. doi: 10.1155/2012/636419
135. Zhang Z, Gao Y, Zhang L, Jia L, Wang P, Zhang L, et al. Alterations of IL-6, IL-6R and Gp130 in Early and Late Onset Severe Preeclampsia. Hypertens Pregnancy (2013) 32:270–80. doi: 10.3109/10641955.2013.798332
136. Daneva AM, Hadzi-Lega M, Stefanovic M. Correlation of the System of Cytokines in Moderate and Severe Preeclampsia. Clin Exp Obstetrics & Gynecol (2016) 43:220–4.
137. Lumbers ER, Delforce SJ, Arthurs AL, Pringle KG. Causes and Consequences of the Dysregulated Maternal Renin-Angiotensin System in Preeclampsia. Front Endocrinol (2019) 10. doi: 10.3389/fendo.2019.00563
138. Burton G, Redman CW, Roberts JM, Moffett A. Preeclampsia: Pathophysiology and Clinical Implications. BMJ (2019) 366:2381. doi: 10.1136/bmj.l2381
139. Aghababaei M, Perdu S, Irvine K, Beristain AG. A Disintegrin and Mettaloproteinase 12 Localizes to Invasive Trophoblast, Promotes Cell Invasion and Directs Column Outgrowth in Early Placental Development. Mol Hum Reprod (2014) 20:235–49. doi: 10.1093/molehr/gat084
140. Biadasiewicz K, Fock V, Dekan S, Proestling K, Velicky P, Haider S, et al. Extravillous Trophoblast-Associated ADAM12 Exerts Pro-Invasive Properties Including Induction of Integrin Beta 1-Mediated Cellular Spreading. Biol Reprod (2014) 90:101. doi: 10.1095/biolreprod.113.115279
141. Winship A, Koga K, Menkhorst E, Van Sinderen M, Rainczuk K, Nagai M, et al. Interleukin-11 Alters Placentation and Causes Preeclampsia Features in Mice. PNAS (2015) 112:15928. doi: 10.1073/pnas.1515076112
142. Wada J, Kanwar YS. Identification and Characterization of Galectin-9, a Novel Beta-Galactoside-Binding Mammalian Lectin. J Biol Chem (1997) 272:6078–86. doi: 10.1074/jbc.272.9.6078
143. Thijssen VL, Hulsmans S, Griffioen AW. The Galectin Profile of the Endothelium: Altered Expression and Localization in Activated and Tumor Endothelial Cells. Am J Pathol (2008) 172:545–53. doi: 10.2353/ajpath.2008.070938
144. Matsuura A, Tsukada J, Mizobe T, Higashi T, Mouri F, Tanikawa R, et al. Intracellular Galectin-9 Activates Inflammatory Cytokines in Monocytes. Genes Cells (2009) 14:511–21. doi: 10.1111/j.1365-2443.2009.01287.x
145. Popa SJ, Stewart SE, Moreau K. Unconventional Secretion of Annexins and Galectins. Semin Cell Dev Biol (2018) 83:42–50. doi: 10.1016/j.semcdb.2018.02.022
146. Bänfer S, Jacob R. Galectins in Intra- and Extracellular Vesicles. Biomolecules (2020) 10:1232. doi: 10.3390/biom10091232
147. Monney L, Sabatos CA, Gaglia JL, Ryu A, Waldner H, Chernova T, et al. Th1-Specific Cell Surface Protein Tim-3 Regulates Macrophage Activation and Severity of an Autoimmune Disease. Nature (2002) 415:536–41. doi: 10.1038/415536a
148. Miko E, Szereday L, Barakonyi A, Jarkovich A, Varga P, Szekeres-Bartho J. Immunoactivation in Preeclampsia: Vdelta2+ and Regulatory T Cells During the Inflammatory Stage of Disease. J Reprod Immunol (2009) 80:100–8. doi: 10.1016/j.jri.2009.01.003
149. Zhao J, Lei Z, Liu Y, Li B, Zhang L, Fang H, et al. Human Pregnancy Up-Regulates Tim-3 in Innate Immune Cells for Systemic Immunity. J Immunol (2009) 182:6618–24. doi: 10.4049/jimmunol.0803876
150. Li F, Dang J, Jiang M, He M, Yang M, Li J, et al. Upregulation of Tim-3 Expression at Feto-Maternal Interface may Explain Embryo Survival in the CBAxDBA/2 Model of Abortion. Am J Reprod Immunol (2018) 79:e12775. doi: 10.1111/aji.12775
151. Meggyes M, Szanto J, Lajko A, Farkas B, Varnagy A, Tamas P, et al. The Possible Role of CD8+/Vα7.2+/CD161++ T (MAIT) and CD8+/Vα7.2+/CD161(lo) T (MAIT-Like) Cells in the Pathogenesis of Early-Onset Pre-Eclampsia. Am J Reprod Immunol (2018) 79:e12805. doi: 10.1111/aji.12805
152. Tang D, Lotze MT. Tumor Immunity Times Out: TIM-3 and HMGB1. Nat Immunol (2012) 13:808–10. doi: 10.1038/ni.2396
153. Sabatos CA, Chakravarti S, Cha E, Schubart A, Sánchez-Fueyo A, Zheng XX, et al. Interaction of Tim-3 and Tim-3 Ligand Regulates T Helper Type 1 Responses and Induction of Peripheral Tolerance. Nat Immunol (2003) 4:1102–10. doi: 10.1038/ni988
154. Zhu C, Anderson AC, Schubart A, Xiong H, Imitola J, Khoury SJ, et al. The Tim-3 Ligand Galectin-9 Negatively Regulates T Helper Type 1 Immunity. Nat Immunol (2005) 6:1245–52. doi: 10.1038/ni1271
155. Seki M, Oomizu S, Sakata KM, Sakata A, Arikawa T, Watanabe K, et al. Galectin-9 Suppresses the Generation of Th17, Promotes the Induction of Regulatory T Cells, and Regulates Experimental Autoimmune Arthritis. Clin Immunol (2008) 127:78–88. doi: 10.1016/j.clim.2008.01.006
156. Wang F, Wan L, Zhang C, Zheng X, Li J, Chen ZK. Tim-3-Galectin-9 Pathway Involves the Suppression Induced by CD4+CD25+ Regulatory T Cells. Immunobiology (2009) 214:342–9. doi: 10.1016/j.imbio.2008.10.007
157. Oomizu S, Arikawa T, Niki T, Kadowaki T, Ueno M, Nishi N, et al. Cell Surface Galectin-9 Expressing Th Cells Regulate Th17 and Foxp3+ Treg Development by Galectin-9 Secretion. PloS One (2012) 7:e48574. doi: 10.1371/journal.pone.0048574
158. Heusschen R, Freitag N, Tirado-Gonzalez I, Barrientos G, Moschansky P, Munoz-Fernandez R, et al. Profiling Lgals9 Splice Variant Expression at the Fetal-Maternal Interface: Implications in Normal and Pathological Human Pregnancy. Biol Reprod (2013) 88:22. doi: 10.1095/biolreprod.112.105460
159. Shimizu Y, Kabir-Salmani M, Azadbakht M, Sugihara K, Sakai K, Iwashita M. Expression and Localization of Galectin-9 in the Human Uterodome. Endocr J (2008) 55:879–87. doi: 10.1507/endocrj.K08E-111
160. Popovici RM, Krause MS, Germeyer A, Strowitzki T, Von Wolff M. Galectin-9: A New Endometrial Epithelial Marker for the Mid- and Late-Secretory and Decidual Phases in Humans. J Clin Endocrinol & Metab (2005) 90:6170–6. doi: 10.1210/jc.2004-2529
161. Meggyes M, Lajko A, Palkovics T, Totsimon A, Illes Z, Szereday L, et al. Feto-Maternal Immune Regulation by TIM-3/Galectin-9 Pathway and PD-1 Molecule in Mice at Day 14.5 of Pregnancy. Placenta (2015) 36:1153–60. doi: 10.1016/j.placenta.2015.07.124
162. Sun J, Yang M, Ban Y, Gao W, Song B, Wang Y, et al. Tim-3 Is Upregulated in NK Cells During Early Pregnancy and Inhibits NK Cytotoxicity Toward Trophoblast in Galectin-9 Dependent Pathway. PloS One (2016) 11:e0147186. doi: 10.1371/journal.pone.0147186
163. Enninga E, Harrington SM, Creedon DJ, Ruano R, Markovic SN, Dong H, et al. Immune Checkpoint Molecules Soluble Program Death Ligand 1 and Galectin-9 Are Increased in Pregnancy. Am J Reprod Immunol (2018) 79:e12795. doi: 10.1111/aji.12795
164. Li YH, Zhou WH, Tao Y, Wang SC, Jiang YL, Zhang D, et al. The Galectin-9/Tim-3 Pathway is Involved in the Regulation of NK Cell Function at the Maternal-Fetal Interface in Early Pregnancy. Cell Mol Immunol (2016) 13:73–81. doi: 10.1038/cmi.2014.126
165. Meggyes M, Miko E, Polgar B, Bogar B, Farkas B, Illes Z, et al. Peripheral Blood TIM-3 Positive NK and CD8+ T Cells Throughout Pregnancy: TIM-3/Galectin-9 Interaction and Its Possible Role During Pregnancy. PloS One (2014) 9:e92371. doi: 10.1371/journal.pone.0092371
166. Wu M, Zhu Y, Zhao J, Ai H, Gong Q, Zhang J, et al. Soluble Costimulatory Molecule Stim3 Regulates the Differentiation of Th1 and Th2 in Patients With Unexplained Recurrent Spontaneous Abortion. Int J Clin Exp Med (2015) 8:8812–9.
167. Wyatt MA, Baumgarten SC, Weaver AL, Van Oort CC, Fedyshyn B, Ruano R, et al. Evaluating Markers of Immune Tolerance and Angiogenesis in Maternal Blood for an Association With Risk of Pregnancy Loss. J Clin Med (2021) 10:3579. doi: 10.3390/jcm10163579
168. Li Y, Zhang J, Zhang D, Hong X, Tao Y, Wang S, et al. Tim-3 Signaling in Peripheral NK Cells Promotes Maternal-Fetal Immune Tolerance and Alleviates Pregnancy Loss. Sci Signal (2017) 10:eaah4323. doi: 10.1126/scisignal.aah4323
169. Hu X, Zhu Q, Wang Y, Wang L, Li Z, Mor G, et al. Newly Characterized Decidual Tim-3+ Treg Cells are Abundant During Early Pregnancy and Driven by IL-27 Coordinately With Gal-9 From Trophoblasts. Hum Reprod (2020) 35:2454–66. doi: 10.1093/humrep/deaa223
170. Miko E, Meggyes M, Bogar B, Schmitz N, Barakonyi A, Varnagy A, et al. Involvement of Galectin-9/TIM-3 Pathway in the Systemic Inflammatory Response in Early-Onset Preeclampsia. PloS One (2013) 8:e71811. doi: 10.1371/journal.pone.0071811
171. Hao H, He M, Li J, Zhou Y, Dang J, Li F, et al. Upregulation of the Tim-3/Gal-9 Pathway and Correlation With the Development of Preeclampsia. Eur J Obstet Gynecol Reprod Biol (2015) 194:85–91. doi: 10.1016/j.ejogrb.2015.08.022
172. Li Z-H, Wang L-L, Liu H, Muyayalo KP, Huang X-B, Mor G, et al. Galectin-9 Alleviates LPS-Induced Preeclampsia-Like Impairment in Rats via Switching Decidual Macrophage Polarization to M2 Subtype. Front Immunol (2019) 9. doi: 10.3389/fimmu.2018.03142
173. Anderson AC, Anderson DE, Bregoli L, Hastings WD, Kassam N, Lei C, et al. Promotion of Tissue Inflammation by the Immune Receptor Tim-3 Expressed on Innate Immune Cells. Science (2007) 318:1141–3. doi: 10.1126/science.1148536
174. Bohn H, Kraus W, Winckler W. Purification and Characterization of Two New Soluble Placental Tissue Proteins (PP13 and PP17). Oncodev Biol Med (1983) 4:343–50.
175. Than NG, Sumegi B, Than GN, Berente Z, Bohn H. Isolation and Sequence Analysis of a cDNA Encoding Human Placental Tissue Protein 13 (PP13), a New Lysophospholipase, Homologue of Human Eosinophil Charcot-Leyden Crystal Protein. Placenta (1999) 20:703–10. doi: 10.1053/plac.1999.0436
176. Visegrady B, Than NG, Kilar F, Sumegi B, Than GN, Bohn H. Homology Modelling and Molecular Dynamics Studies of Human Placental Tissue Protein 13 (Galectin-13). Protein Eng (2001) 14:875–80. doi: 10.1093/protein/14.11.875
177. Than NG, Pick E, Bellyei S, Szigeti A, Burger O, Berente Z, et al. Functional Analyses of Placental Protein 13/Galectin-13. Eur J Biochem (2004) 271:1065–78. doi: 10.1111/j.1432-1033.2004.04004.x
178. Than NG, Abdul Rahman O, Magenheim R, Nagy B, Fule T, Hargitai B, et al. Placental Protein 13 (Galectin-13) has Decreased Placental Expression But Increased Shedding and Maternal Serum Concentrations in Patients Presenting With Preterm Pre-Eclampsia and HELLP Syndrome. Virchows Arch (2008) 453:387–400. doi: 10.1007/s00428-008-0658-x
179. Balogh A, Pozsgay J, Matko J, Dong Z, Kim CJ, Varkonyi T, et al. Placental Protein 13 (PP13/galectin-13) Undergoes Lipid Raft-Associated Subcellular Redistribution in the Syncytiotrophoblast in Preterm Preeclampsia and HELLP Syndrome. Am J Obstet Gynecol (2011) 205:156 e151–114. doi: 10.1016/j.ajog.2011.03.023
180. Than NG, Romero R, Meiri H, Erez O, Xu Y, Tarquini F, et al. PP13, Maternal ABO Blood Groups and the Risk Assessment of Pregnancy Complications. PloS One (2011) 6:e21564. doi: 10.1371/journal.pone.0021564
181. Than NG, Romero R, Xu Y, Erez O, Xu Z, Bhatti G, et al. Evolutionary Origins of the Placental Expression of Chromosome 19 Cluster Galectins and Their Complex Dysregulation in Preeclampsia. Placenta (2014) 35:855–65. doi: 10.1016/j.placenta.2014.07.015
182. Balogh A, Toth E, Romero R, Parej K, Csala D, Szenasi NL, et al. Placental Galectins Are Key Players in Regulating the Maternal Adaptive Immune Response. Front Immunol (2019) 10:1240. doi: 10.3389/fimmu.2019.01240
183. Burger O, Pick E, Zwickel J, Klayman M, Meiri H, Slotky R, et al. Placental Protein 13 (PP-13): Effects on Cultured Trophoblasts, and its Detection in Human Body Fluids in Normal and Pathological Pregnancies. Placenta (2004) 25:608–22. doi: 10.1016/j.placenta.2003.12.009
184. Nicolaides KH, Bindra R, Turan OM, Chefetz I, Sammar M, Meiri H, et al. A Novel Approach to First-Trimester Screening for Early Pre-Eclampsia Combining Serum PP-13 and Doppler Ultrasound. Ultrasound Obstet Gynecol (2006) 27:13–7. doi: 10.1002/uog.2686
185. Chafetz I, Kuhnreich I, Sammar M, Tal Y, Gibor Y, Meiri H, et al. First-Trimester Placental Protein 13 Screening for Preeclampsia and Intrauterine Growth Restriction. Am J Obstet Gynecol (2007) 197:35 e31–37. doi: 10.1016/j.ajog.2007.02.025
186. Romero R, Kusanovic JP, Than NG, Erez O, Gotsch F, Espinoza J, et al. First-Trimester Maternal Serum PP13 in the Risk Assessment for Preeclampsia. Am J Obstet Gynecol (2008) 199:122 e121–122 e111. doi: 10.1016/j.ajog.2008.01.013
187. Gizurarson S, Huppertz B, Osol G, Skarphedinsson JO, Mandala M, Meiri H. Effects of Placental Protein 13 on the Cardiovascular System in Gravid and non-Gravid Rodents. Fetal Diagn Ther (2013) 33:257–64. doi: 10.1159/000345964
188. Gizurarson S, Sigurdardottir ER, Meiri H, Huppertz B, Sammar M, Sharabi-Nov A, et al. Placental Protein 13 Administration to Pregnant Rats Lowers Blood Pressure and Augments Fetal Growth and Venous Remodeling. Fetal Diagn Ther (2016) 39:56–63. doi: 10.1159/000381914
189. Drobnjak T, Gizurarson S, Gokina NI, Meiri H, Mandala M, Huppertz B, et al. Placental Protein 13 (PP13)-Induced Vasodilation of Resistance Arteries From Pregnant and Nonpregnant Rats Occurs via Endothelial-Signaling Pathways. Hypertens Pregnancy (2017) 36:186–95. doi: 10.1080/10641955.2017.1295052
190. Meiri H, Osol G, Cetin I, Gizurarson S, Huppertz B. Personalized Therapy Against Preeclampsia by Replenishing Placental Protein 13 (PP13) Targeted to Patients With Impaired PP13 Molecule or Function. Comput Struct Biotechnol J (2017) 15:433–46. doi: 10.1016/j.csbj.2017.09.002
191. Drobnjak T, Meiri H, Mandala M, Huppertz B, Gizurarson S. Pharmacokinetics of Placental Protein 13 After Intravenous and Subcutaneous Administration in Rabbits. Drug Des Devel Ther (2018) 12:1977–83. doi: 10.2147/DDDT.S167926
192. Drobnjak T, Jonsdottir AM, Helgadottir H, Runolfsdottir MS, Meiri H, Sammar M, et al. Placental Protein 13 (PP13) Stimulates Rat Uterine Vessels After Slow Subcutaneous Administration. Int J Womens Health (2019) 11:213–22. doi: 10.2147/IJWH.S188303
193. Sammar M, Drobnjak T, Mandala M, Gizurarson S, Huppertz B, Meiri H. Galectin 13 (PP13) Facilitates Remodeling and Structural Stabilization of Maternal Vessels During Pregnancy. Int J Mol Sci (2019) 20:3192. doi: 10.3390/ijms20133192
194. Huppertz B, Sammar M, Chefetz I, Neumaier-Wagner P, Bartz C, Meiri H. Longitudinal Determination of Serum Placental Protein 13 During Development of Preeclampsia. Fetal Diagn Ther (2008) 24:230–6. doi: 10.1159/000151344
195. Sammar M, Dragovic R, Meiri H, Vatish M, Sharabi-Nov A, Sargent I, et al. Reduced Placental Protein 13 (PP13) in Placental Derived Syncytiotrophoblast Extracellular Vesicles in Preeclampsia - A Novel Tool to Study the Impaired Cargo Transmission of the Placenta to the Maternal Organs. Placenta (2018) 66:17–25. doi: 10.1016/j.placenta.2018.04.013
196. Modenutti CP, Capurro JIB, Di Lella S, Marti MA. The Structural Biology of Galectin-Ligand Recognition: Current Advances in Modeling Tools, Protein Engineering, and Inhibitor Design. Front Chem (2019) 7:823. doi: 10.3389/fchem.2019.00823
197. Than NG, Balogh A, Romero R, Karpati E, Erez O, Szilagyi A, et al. Placental Protein 13 (PP13) - A Placental Immunoregulatory Galectin Protecting Pregnancy. Front Immunol (2014) 5:348. doi: 10.3389/fimmu.2014.00348
198. Su J, Cui L, Si Y, Song C, Li Y, Yang T, et al. Resetting the Ligand Binding Site of Placental Protein 13/Galectin-13 Recovers its Ability to Bind Lactose. Biosci Rep (2018) 38:BSR20181787. doi: 10.1042/BSR20181787
199. Su J, Wang Y, Si Y, Gao J, Song C, Cui L, et al. Galectin-13, a Different Prototype Galectin, Does Not Bind Beta-Galacto-Sides and Forms Dimers via Intermolecular Disulfide Bridges Between Cys-136 and Cys-138. Sci Rep (2018) 8:980. doi: 10.1038/s41598-018-19465-0
200. Si Y, Li Y, Yang T, Li X, Ayala GJ, Mayo KH, et al. Structure-Function Studies of Galectin-14, an Important Effector Molecule in Embryology. FEBS J (2021) 288:1041–55. doi: 10.1111/febs.15441
201. Si Y, Yao Y, Jaramillo Ayala G, Li X, Han Q, Zhang W, et al. Human Galectin-16 has a Pseudo Ligand Binding Site and Plays a Role in Regulating C-Rel-Mediated Lymphocyte Activity. Biochim Biophys Acta Gen Subj (2021) 1865:129755. doi: 10.1016/j.bbagen.2020.129755
202. Kliman HJ, Sammar M, Grimpel YI, Lynch SK, Milano KM, Pick E, et al. Placental Protein 13 and Decidual Zones of Necrosis: An Immunologic Diversion That May Be Linked to Preeclampsia. Reprod Sci (2012) 19:16–30. doi: 10.1177/1933719111424445
203. Vokalova L, Balogh A, Toth E, Van Breda SV, Schafer G, Hoesli I, et al. Placental Protein 13 (Galectin-13) Polarizes Neutrophils Toward an Immune Regulatory Phenotype. Front Immunol (2020) 11:145. doi: 10.3389/fimmu.2020.00145
204. Li A, Dubey S, Varney ML, Dave BJ, Singh RK. IL-8 Directly Enhanced Endothelial Cell Survival, Proliferation, and Matrix Metalloproteinases Production and Regulated Angiogenesis. J Immunol (2003) 170:3369–76. doi: 10.4049/jimmunol.170.6.3369
205. Amsalem H, Kwan M, Hazan A, Zhang J, Jones RL, Whittle W, et al. Identification of a Novel Neutrophil Population: Proangiogenic Granulocytes in Second-Trimester Human Decidua. J Immunol (2014) 193:3070–9. doi: 10.4049/jimmunol.1303117
206. Lash GE, Ernerudh J. Decidual Cytokines and Pregnancy Complications: Focus on Spontaneous Miscarriage. J Reprod Immunol (2015) 108:83–9. doi: 10.1016/j.jri.2015.02.003
207. Gamliel M, Goldman-Wohl D, Isaacson B, Gur C, Stein N, Yamin R, et al. Trained Memory of Human Uterine NK Cells Enhances Their Function in Subsequent Pregnancies. Immunity (2018) 48:951–962 e955. doi: 10.1016/j.immuni.2018.03.030
208. Blois SM, Conrad ML, Freitag N, Barrientos G. Galectins in Angiogenesis: Consequences for Gestation. J Reprod Immunol (2015) 108:33–41. doi: 10.1016/j.jri.2014.12.001
209. Chaiworapongsa T, Chaemsaithong P, Yeo L, Romero R. Pre-Eclampsia Part 1: Current Understanding of its Pathophysiology. Nat Rev Nephrol (2014) 10:466–80. doi: 10.1038/nrneph.2014.102
210. Leavey K, Bainbridge SA, Cox BJ. Large Scale Aggregate Microarray Analysis Reveals Three Distinct Molecular Subclasses of Human Preeclampsia. PloS One (2015) 10:e0116508. doi: 10.1371/journal.pone.0116508
211. Sekizawa A, Purwosunu Y, Yoshimura S, Nakamura M, Shimizu H, Okai T, et al. PP13 mRNA Expression in Trophoblasts From Preeclamptic Placentas. Reprod Sci (2009) 16:408–13. doi: 10.1177/1933719108328615
212. Than NG, Romero R, Tarca AL, Kekesi KA, Xu Y, Xu Z, et al. Integrated Systems Biology Approach Identifies Novel Maternal and Placental Pathways of Preeclampsia. Front Immunol (2018) 9:1661. doi: 10.3389/fimmu.2018.01661
213. Spencer K, Cowans NJ, Chefetz I, Tal J, Meiri H. First-Trimester Maternal Serum PP-13, PAPP-A and Second-Trimester Uterine Artery Doppler Pulsatility Index as Markers of Pre-Eclampsia. Ultrasound Obstet Gynecol (2007) 29:128–34. doi: 10.1002/uog.3876
214. Gonen R, Shahar R, Grimpel YI, Chefetz I, Sammar M, Meiri H, et al. Placental Protein 13 as an Early Marker for Pre-Eclampsia: A Prospective Longitudinal Study. BJOG (2008) 115:1465–72. doi: 10.1111/j.1471-0528.2008.01902.x
215. Akolekar R, Syngelaki A, Beta J, Kocylowski R, Nicolaides KH. Maternal Serum Placental Protein 13 at 11-13 Weeks of Gestation in Preeclampsia. Prenat Diagn (2009) 29:1103–8. doi: 10.1002/pd.2375
216. Khalil A, Cowans NJ, Spencer K, Goichman S, Meiri H, Harrington K. First Trimester Maternal Serum Placental Protein 13 for the Prediction of Pre-Eclampsia in Women With a Priori High Risk. Prenat Diagn (2009) 29:781–9. doi: 10.1002/pd.2287
217. Sammar M, Nisemblat S, Fleischfarb Z, Golan A, Sadan O, Meiri H, et al. Placenta-Bound and Body Fluid PP13 and its mRNA in Normal Pregnancy Compared to Preeclampsia, HELLP and Preterm Delivery. Placenta (2011) 32(Suppl):S30–36. doi: 10.1016/j.placenta.2010.09.006
218. Bohn H, Schmidtberger R, Zilg H. Isolation of the Pregnancy Specific Beta-Glycoprotein (SP1) and Antigen-Related Proteins by Means of Immunoadsorption. Blut (1976) 32:103–13. doi: 10.1007/BF00995937
219. Burton GJ, Fowden AL. The Placenta: A Multifaceted, Transient Organ. Philos Trans R Soc Lond B Biol Sci (2015) 370:20140066. doi: 10.1098/rstb.2014.0066
220. Liu Y, Fan X, Wang R, Lu X, Dang Y-L, Wang H, et al. Single-Cell RNA-Seq Reveals the Diversity of Trophoblast Subtypes and Patterns of Differentiation in the Human Placenta. Cell Res (2018) 28:819–32. doi: 10.1038/s41422-018-0066-y
221. Rattila S, Dunk CEE, Im M, Grichenko O, Zhou Y, Yanez-Mo M, et al. Interaction of Pregnancy-Specific Glycoprotein 1 With Integrin Alpha5beta1 Is a Modulator of Extravillous Trophoblast Functions. Cells (2019) 8:1369. doi: 10.3390/cells8111369
222. Zimmermann W, Kammerer R. The Immune-Modulating Pregnancy-Specific Glycoproteins Evolve Rapidly and Their Presence Correlates With Hemochorial Placentation in Primates. BMC Genomics (2021) 22:128. doi: 10.1186/s12864-021-07413-8
223. Teglund S, Olsen A, Khan WN, Frängsmyr L, Hammarström S. The Pregnancy-Specific Glycoprotein (PSG) Gene Cluster on Human Chromosome 19: Fine Structure of the 11 PSG Genes and Identification of 6 New Genes Forming a Third Subgroup Within the Carcinoembryonic Antigen (CEA) Family. Genomics (1994) 23:669–84. doi: 10.1006/geno.1994.1556
224. Chen H, Chan WY, Chen CL, Mansfield BC, Chou JY. The Carboxyl-Terminal Domain of the Human Pregnancy-Specific Glycoprotein Specifies Intracellular Retention and Stability. J Biol Chem (1993) 268:22066–75. doi: 10.1016/S0021-9258(20)80649-8
225. Warren J, Im M, Ballesteros A, Ha C, Moore T, Lambert F, et al. Activation of Latent Transforming Growth Factor-β1, a Conserved Function for Pregnancy-Specific Beta 1-Glycoproteins. Mol Hum Reprod (2018) 24:602–12. doi: 10.1093/molehr/gay044
226. Chang CL, Semyonov J, Cheng PJ, Huang SY, Park JI, Tsai HJ, et al. Widespread Divergence of the CEACAM/PSG Genes in Vertebrates and Humans Suggests Sensitivity to Selection. PloS One (2013) 8:e61701. doi: 10.1371/journal.pone.0061701
227. Shanley DK, Kiely PA, Golla K, Allen S, Martin K, O’riordan RT, et al. Pregnancy-Specific Glycoproteins Bind Integrin Alphaiibbeta3 and Inhibit the Platelet-Fibrinogen Interaction. PloS One (2013) 8:e57491. doi: 10.1371/journal.pone.0057491
228. Moore T, Dveksler GS. Pregnancy-Specific Glycoproteins: Complex Gene Families Regulating Maternal-Fetal Interactions. Int J Dev Biol (2014) 58:273–80. doi: 10.1387/ijdb.130329gd
229. Lisboa FA, Warren J, Sulkowski G, Aparicio M, David G, Zudaire E, et al. Pregnancy-Specific Glycoprotein 1 Induces Endothelial Tubulogenesis Through Interaction With Cell Surface Proteoglycans. J Biol Chem (2011) 286:7577–86. doi: 10.1074/jbc.M110.161810
230. Blois SM, Sulkowski G, Tirado-Gonzalez I, Warren J, Freitag N, Klapp BF, et al. Pregnancy-Specific Glycoprotein 1 (PSG1) Activates TGF-Beta and Prevents Dextran Sodium Sulfate (DSS)-Induced Colitis in Mice. Mucosal Immunol (2014) 7:3448–58. doi: 10.1038/mi.2013.53
231. Warren J, Im M, Ballesteros A, Ha C, Moore T, Lambert F, et al. Activation of Latent Transforming Growth Factor-Beta1, a Conserved Function for Pregnancy-Specific Beta 1-Glycoproteins. Mol Hum Reprod (2018) 24:602–12. doi: 10.1093/molehr/gay044
232. Rattila S, Kleefeldt F, Ballesteros A, Beltrame JS, M LR, Ergun S, et al. Pro-Angiogenic Effects of Pregnancy-Specific Glycoproteins in Endothelial and Extravillous Trophoblast Cells. Reproduction (2020) 160:737–50. doi: 10.1530/REP-20-0169
233. Rattila S, Dunk CEE, Im M, Grichenko O, Zhou Y, Yanez-Mo M, et al. Interaction of Pregnancy-Specific Glycoprotein 1 With Integrin α5β1 Is a Modulator of Extravillous Trophoblast Functions. Cells (2019) 8:1369. doi: 10.3390/cells8111369
234. Mendoza M, Lu D, Ballesteros A, Blois SM, Abernathy K, Feng C, et al. Glycan Characterization of Pregnancy-Specific Glycoprotein 1 and Its Identification as a Novel Galectin-1 Ligand. Glycobiology (2020) 30(11):895–909. doi: 10.1093/glycob/cwaa034
Keywords: galectins, pregnancy, pregnancy specific proteins, glycoimmunology, placenta
Citation: Menkhorst E, Than NG, Jeschke U, Barrientos G, Szereday L, Dveksler G and Blois SM (2021) Medawar’s PostEra: Galectins Emerged as Key Players During Fetal-Maternal Glycoimmune Adaptation. Front. Immunol. 12:784473. doi: 10.3389/fimmu.2021.784473
Received: 27 September 2021; Accepted: 15 November 2021;
Published: 15 December 2021.
Edited by:
Kenneth Beaman, Rosalind Franklin University of Medicine and Science, United StatesCopyright © 2021 Menkhorst, Than, Jeschke, Barrientos, Szereday, Dveksler and Blois. This is an open-access article distributed under the terms of the Creative Commons Attribution License (CC BY). The use, distribution or reproduction in other forums is permitted, provided the original author(s) and the copyright owner(s) are credited and that the original publication in this journal is cited, in accordance with accepted academic practice. No use, distribution or reproduction is permitted which does not comply with these terms.
*Correspondence: Sandra M. Blois, cy5ibG9pc0B1a2UuZGU=
Disclaimer: All claims expressed in this article are solely those of the authors and do not necessarily represent those of their affiliated organizations, or those of the publisher, the editors and the reviewers. Any product that may be evaluated in this article or claim that may be made by its manufacturer is not guaranteed or endorsed by the publisher.
Research integrity at Frontiers
Learn more about the work of our research integrity team to safeguard the quality of each article we publish.