- 1Division of Allergy and Immunology and Center for Food Allergy, Department of Pediatrics, University of Rochester School of Medicine and Dentistry and Golisano Children’s Hospital, Rochester, NY, United States
- 2Department of Microbiology and Immunology and Department of Biostatistics and Computational Biology, University of Rochester School of Medicine and Dentistry, Rochester, NY, United States
- 3Medical Scientist Training Program, University of Rochester School of Medicine and Dentistry, Rochester, NY, United States
- 4Department of Public Health Sciences & Environmental Medicine, University of Rochester School of Medicine and Dentistry, Rochester, NY, United States
- 5Division of Neonatology and Division of Gastroenterology, Hepatology and Nutrition, Department of Pediatrics, University of California, San Diego, La Jolla, CA, United States
- 6Mother-Milk-Infant Center of Research Excellence (MOMI CORE), University of California, San Diego, La Jolla, CA, United States
- 7Department of Genetics and Genomic Sciences, Icahn Institute for Data Science and Genomic Technology, Precision Immunology Institue, Icahn School of Medicine at Mount Sinai, New York, New York, NY, United States
- 8Division of Allergy, Immunology, and Rheumatology, Department of Medicine, University of Rochester School of Medicine and Dentistry, Rochester, NY, United States
Background: In addition to farming exposures in childhood, maternal farming exposures provide strong protection against allergic disease in their children; however, the effect of farming lifestyle on human milk (HM) composition is unknown.
Objective: This study aims to characterize the maternal immune effects of Old Order Mennonite (OOM) traditional farming lifestyle when compared with Rochester (ROC) families at higher risk for asthma and allergic diseases using HM as a proxy.
Methods: HM samples collected at median 2 months of lactation from 52 OOM and 29 ROC mothers were assayed for IgA1 and IgA2 antibodies, cytokines, endotoxin, HM oligosaccharides (HMOs), and targeted fatty acid (FA) metabolites. Development of early childhood atopic diseases in children by 3 years of age was assessed. In addition to group comparisons, systems level network analysis was performed to identify communities of multiple HM factors in ROC and OOM lifestyle.
Results: HM contains IgA1 and IgA2 antibodies broadly recognizing food, inhalant, and bacterial antigens. OOM HM has significantly higher levels of IgA to peanut, ovalbumin, dust mites, and Streptococcus equii as well TGF-β2, and IFN-λ3. A strong correlation occurred between maternal antibiotic use and levels of several HMOs. Path-based analysis of HMOs shows lower activity in the path involving lactoneohexaose (LNH) in the OOM as well as higher levels of lacto-N-neotetraose (LNnT) and two long-chain FAs C-18OH (stearic acid) and C-23OH (tricosanoic acid) compared with Rochester HM. OOM and Rochester milk formed five different clusters, e.g., butyrate production was associated with Prevotellaceae, Veillonellaceae, and Micrococcaceae cluster. Development of atopic disease in early childhood was more common in Rochester and associated with lower levels of total IgA, IgA2 to dust mite, as well as of TSLP.
Conclusion: Traditional, agrarian lifestyle, and antibiotic use are strong regulators of maternally derived immune and metabolic factors, which may have downstream implications for postnatal developmental programming of infant’s gut microbiome and immune system.
Introduction
Asthma and atopic diseases including food allergy, eczema, and rhinoconjunctivitis have increased exponentially over recent decades of industrialization and urbanization and are now the most common chronic medical conditions affecting children in the USA and are a global public health concern (1). Although heredity is a strong determinant of an allergic constitution, environmental factors, including a Western lifestyle, microbial exposures, and diet likely play a role in the rise of atopic diseases, which are characterized by Th2 responses. Cumulative data to date suggest that living on farms is associated with a major reduction in the risk of asthma and atopic diseases, including studies from Europe (2–4) and North America among the Amish (5, 6) and the Old Order Mennonites (OOM) (7–9). The individual factors that appear to be associated with this “farm-life effect” include consumption of unpasteurized farm milk (2, 10, 11) and exposure to farm animals, stables (2, 3) common in traditional one-family farms, but not in communal farms (6).
It is less appreciated that the temporal window for the immunomodulatory effects of a farming lifestyle extends from early postnatal to the prenatal period. Maternal microbial exposures during pregnancy have a strong and independent protective impact on atopic sensitization and allergic disease in their children (2–4, 12). This protection was associated with maternal exposure to farm animals and unboiled farm milk (3, 10), increased T regulatory cells (Tregs) in cord blood (13), and altered expression of receptors of the innate immunity (TLR2, TLR4, CD14) in school-aged children (3). These data suggest a maternal farmlife effect on protection against allergic diseases, but to date, only a single study has assessed the maternal, postnatal farming lifestyle effect related to human milk composition. In the European “Protection against Allergy-Study in Rural Environments” (PASTURE) cohort, IgA levels were higher in the milk of mothers with contact to farm animals and cats than in those unexposed during pregnancy (14).
Human milk contains immunologically active factors such as immune cells, cytokines, chemokines and hormones, immunoglobulins, critical growth factors, active enzymes including peroxidases and lysozymes, lactoferrin, saturated fatty acids, poly-unsaturated fatty acids (PUFAs) and additional secretory components, soluble CD14, TLR2, and tumor necrosis factor (TNF) receptor, along with maternal diet-derived food antigens (15). The role for maternal milk components in regulation of breastfed immunity is best demonstrated in mouse models, in which secretory breast milk IgA plays a role in the longterm gut homeostasis by regulating microbiota and host gene expression (16), and IgG immune complexes are shown to induce food-specific tolerance in offspring (17). Transforming growth factor (TGF)-β in murine milk plays a role in development of tolerance in the offspring (18). Epidermal growth factor (EGF) not only prevents dissemination of a gut-resident pathogen by inhibiting goblet cell-mediated bacterial translocation (19) but may also prevent food allergen uptake during breastfeeding, which may have implication for development of tolerance (20). Using human samples and infant cohorts, we have shown that human milk IgA controls excessive intestinal uptake of food antigens, which is associated with protection against food allergies in the offspring (21), as are higher levels of TGF-β1, along with IL-10 and IL-6 (22). In addition to providing immunostimulatory factors for the breastfed infant, breastfeeding significantly influences the development of the infant microbiome composition (23, 24), which influences gut dysbiosis in infancy. Early childhood precedes the development of multisensitization and atopic disease (25), including food allergy (26, 27) and asthma (28). One way breastfeeding impacts infant’s gut microbiota composition includes human milk oligosaccharides (HMOs), complex glycans that provide the main substrate for infant’s gut microbiota, particularly for Bifidobacteria and some Bacteroides (29, 30), and HMO compositional differences were detected in children protected against food allergy (31–33) and IgE-associated eczema (34). In addition, human milk contains microbiota, but the data are conflicting to which extent human milk may act as a source of bacterial species that colonize the infant gut (35–41). Human milk is also a rich source of short-chain fatty acids (SCFAs) (42), the function of which is unknown.
Breastfeeding provides continued exposure to the mother’s immune system during the crucial window of the first few months of life, when the infant’s immune system is continuously developing. However, the immunomodulatory composition of human milk characterized so far demonstrates a great deal of variability between mothers, which implies that the benefit of breastfeeding to the breastfed infants may differ between dyads. For the first time, we sought to characterize the differences in the multiple maternally derived immune and metabolic factors in human milk, including a broad characterization of specificity of IgA1 and IgA2, comparing farming and nonfarming lifestyles. Specifically, we here use the OOM as a model population of a lifestyle dating back 100 years. We hypothesized that because their lifestyle includes exposure to farm animals, homegrown food and unpasteurized milk, home births, and large family sizes and is limited in exposure to modern commodities such as cars and use of antibiotics, their human milk would have a different composition. Assessment of human milk composition can serve as a proxy for maternal immunity, the impact of which on allergic sensitization in the offspring starts in utero and expands postnatally through breastfeeding.
Methods
Study Population
For this cross-sectional study, we recruited a cohort of infants at low risk and those at high risk for allergies, as described before (41). The low-risk OOM of the Western NY reside in Penn Yan 65 mi southeast of Rochester and were recruited by a nurse midwife among prenatal visits in her clinic. Rochester urban and suburban mothers were recruited prenatally from the University of Rochester Medical Center (URMC) and Rochester, NY Obstetric practices. The OOM are a branch of the Mennonite Protestant tradition and of Swiss-German ancestry. They have a lifestyle incorporating growing up on a farm, having large families, exposure to numerous pets and farm animals, consumption of raw milk, low rate of smoking, antibiotic utilization, and infant vaccinations. They use a horse and buggy for transportation, grow most of their food, and many live on a dairy farm. OOM have very low rates of asthma, rhinitis, and eczema, e.g., the rate of asthma in OOM is only 1.8% compared with 12.0% in the general US population, by NHANES data (7). A follow-up questionnaire indicated that food allergies impact <2% of the OOM compared with 6.5% of the NHANES children (8). Only infants who were born full term (>36 weeks of gestation), with birth weight of >2,000 g, and generally healthy born to mothers without chronic infection, inflammatory disease, or known immunodeficiency or metabolic disease were included. Our protocol was approved by the Institutional Review Board of the University of Rochester Medical Center (RSRB52971).
Altogether, 65 OOM and 39 Rochester women were recruited pre- or immediately postnatally. Among them, human milk samples were received in 52 OOM and 29 Rochester mothers, collected between 2 weeks and 6 months of age. Details on study procedures and sampling are shown on Figure 1. The median duration of lactation at collection of human milk samples was 59 days (IQR 19).
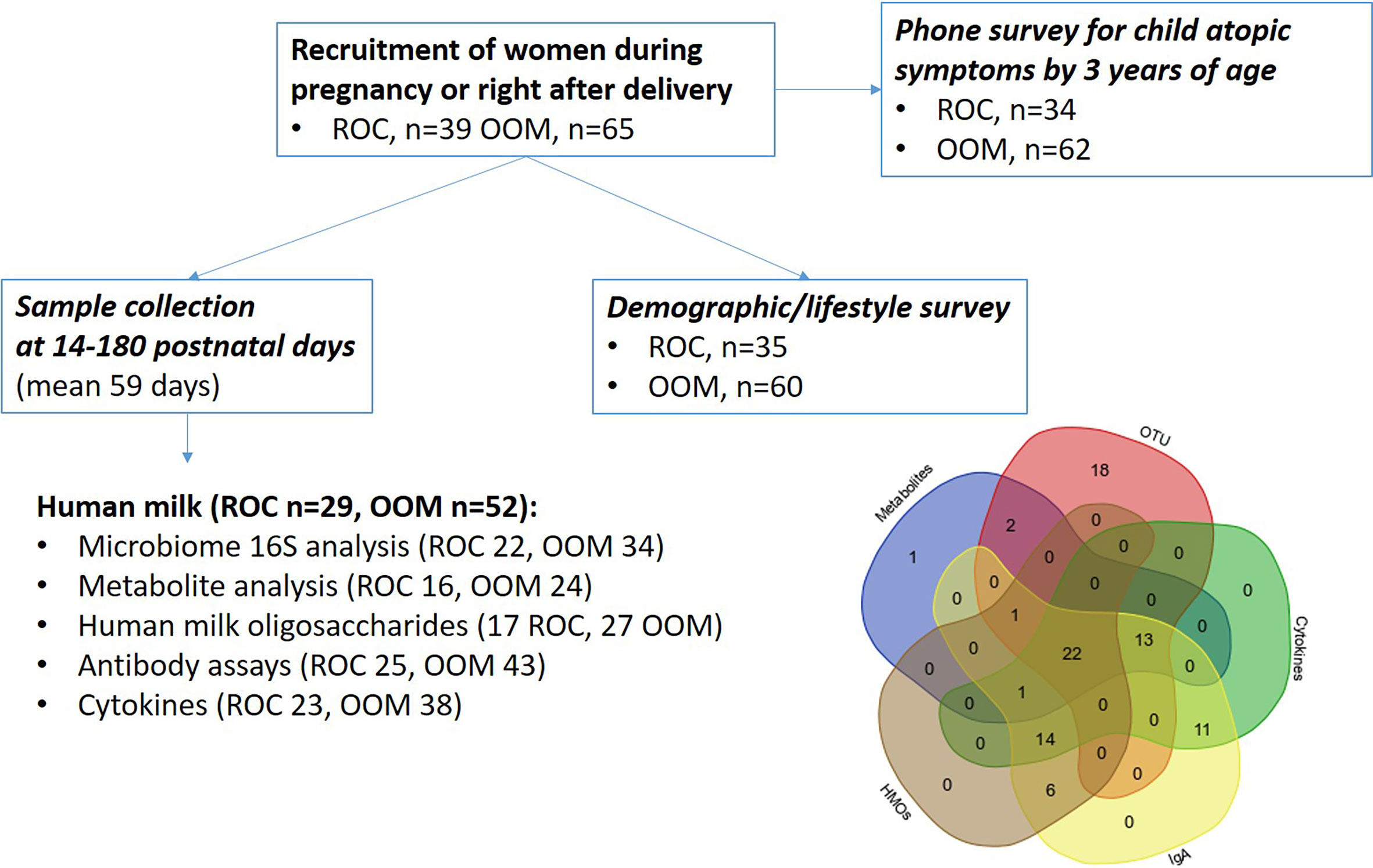
Figure 1 Schematic describing the cohort, samples, and assays of mother-infant pairs. OOM, Old Order Mennonite; ROC, Rochester.
Follow-Up of Children for Atopic Symptoms
Presence of possible/likely atopic disease by the age of 3 years was defined through a telephone screening for possible allergic symptoms by a research coordinator, and a follow-up phone survey was performed by a pediatric allergist (KJ). We queried physician diagnosis not only of atopic dermatitis, allergic rhinitis, food allergy, and asthma but also symptoms consistent with atopic disease due to concerns of lower healthcare utilization by the OOM. The latter include chronic/remitting pruritic rash in a distribution age-typical for atopic eczema that had been treated by over-the-counter (OTC) or prescription steroidal creams or other anti-inflammatory topical treatments commonly indicated for atopic dermatitis; chronic or recurring symptoms of rhinorrhea/congestion/sneezing treated with antihistamines; symptoms suggestive of IgE-mediated food allergy and allergic proctocolitis; and recurrent wheezing episodes. Intermittent wheezing episodes associated with viral infections alone were not determined indicative of asthma due to young age.
Maternal Prenatal Exposures
We collected data on maternal race and ethnicity, BMI, occupation, use of antibiotics during pregnancy, history of allergic diseases (asthma, eczema, allergic rhinitis, and food allergy), exposure to indoor and outdoor animals, consumption of unpasteurized milk and home-grown foods, the use of household cleaning agents, home heating and water sources and personal care products, and infant’s race and ethnicity, sex, mode, and place of birth, as well as the number of older siblings, initiation and duration of breastfeeding.
Samples Collection and Processing
Human milk samples were collected in the morning, in UV-irradiated, sterile collection cups by sterile manual breast pumps (Harmony Breastpump, Medela) or by manual expression, wearing gloves. Foremilk was collected after cleaning the breast with Castille soap. All the samples were frozen at −20°C immediately upon collection until being transferred to −80°C within 4 weeks for long-term storage.
Total and Specific IgA in Human Milk
Total IgA was measured using commercially available ELISA kit (Bethyl laboratories, Montgomery, TX, USA). For food-specific IgA, hen’s egg ovalbumin and bovine casein and β-lactoglobulin (BLG, Sigma, St. Loius, MO, USA) and defatted crude peanut extract were used as antigens. Microtiter plates (Nunc MaxiSorb, ThermoFisher Scientific, Waltham, MA, USA) were coated at a concentration of 20 µg/ml in phosphate-buffered saline (PBS) and incubated at 4°C overnight. After washing with PBS-0.05% Tween (PBS-T), plates were blocked with 1% bovine serum albumin (BSA) in PBS-T for 1 h at room temperature followed by washing with PBS-T. Human milk was diluted 1:5,000 for total IgA, 1:4 for specific IgA, and 1:2 for specific IgG. Purified human IgA (Bethyl Laboratories, Montgomery, TX, USA) was used as a standard curve for IgA and IgG. After washing, bound IgA antibody was detected using 1:100,000 diluted, HRP-conjugated goat-anti-human IgA for 1 h at room temperature. After washing, 100 µl of TMB substrate solution was applied to the plates, and the reaction was stopped and the optical density was measured at 450 nm.
To measure aeroallergen-specific IgA responses, we used a commercially available Luminex kit (Indoor Technologies, Charlottesville, VA, USA) including Alternaria mold, Bet v1 (birch), Phl p5 (timothy), Can f1 (dog), Mus m1 (mouse), Rat n1 (rat), Der p1 and Der f1 (dust mites), and Fel d1 (cat), which was modified for use as an IgA assay using secondary antibody with IgA specificity (Bethyl Laboratories, Mongomery, TX, USA).
To quantitate human milk IgA response to select bacterial species, we used whole bacteria (43) in a Luminex assay. Seven bacterial species were selected based on differential presence of IgA coating similarly as shown by Planer et al. (44), including gut commensals Escheria coli, Enterobacter cloacae, Enterococcus faecalis, Morganella morganii, Lactobacillus reuteri, Salmonella enterica spp. typhimurium, and Streptococcus equii, a horse respiratory pathogen. Bacterial species were grown in culture and used in whole bacteria ELISA to quantitatively measure specific IgA. Specificity was demonstrated by competitive inhibition of binding by the same bacteria and lack of competitive inhibition by other bacteria in the panel (data not shown) (Supplementary Table 1).
Cytokines in Human Milk
Human milk cytokines were measured in milk supernatants by multiplex kits (Milliplex, Millipore, Billerica, MA, USA) and read on a Luminex 200 Multiplex analyzer (Luminex, Austin, TX, USA) after centrifugation and removal of fat layer as previously described (21). The following cytokines were measured: Th17 kit: interferon-gamma, IL-10, IL-12P70, IL-13, IL-17A, IL-9, IL-1β, IL-2, IL-23, IL-5, IL-6, IL-31, and Inflammation kit: IL-27, gp130/sIL-6Rβ, IL-34, IL-26, IL-19, IL-20, IL-29/IFN-Gamma1, IL-35, IL-32, BAFF/TNFSF13B, IL-11, APRIL/TNFSF13, IFN-β, sCD163, Pentraxin-3, LIGHT/TNFSF14, TSLP, sCD30/TNFRSF8, TWEAK/TNFSF12, Osteocalcin, IL-28A/IFN-Gamma2, sTNF-R2, Chitinase3-like 1, and sTNF-R1. TGF-β1, TGF-β2, and TGF-β3 were measured using Bio-Plex TGF-β kit (Bio-Rad, Billerica, MA, USA) from samples acid activated to release the latent form (Supplementary Table 1).
HMO Composition
Analysis of HMO composition and original data has been previously described by us (41). In brief, we measured the absolute concentrations of 19 most abundant HMOs (see Supplementary Table 2) by HPLC including type 1 and type 2 chains, branching, all types of fucosylation (α1-2, α1-3, and α1-4), which will allow determination of the mother’s Secretor and Lewis status, as well as all types of sialylation (α2-3 and terminal and internal α2-6).
Human Milk Microbiome
Results and analysis of human milk and infant gut microbiota has been previously described (41, BioProject accession number PRJNA746977). In brief, genomic DNA from human milk samples was extracted using phenol-chloroform technique. Milk extraction was done using pelleted bacteria combined with fat layer in order to capture bacterial species that associate with fat globules and do not pellet during centrifugation. The V4 region of the 16S rRNA DNA gene was amplified in triplicate from all samples, pooled and sequenced on the Illumina MiSeq platform (paired-end 250bp) following standard protocols (45, 46), and data OTU level analyzed in previous publication was used (46).
Human Milk Metabolites
Methods and original data have been previously published by us (41). In brief, human milk samples were analyzed for the concentrations of a comprehensive panel of 69 fatty acids (FAs) ranging in chain length from 2 to 26. The analysis was carried out at the Metabolic Invention Center (TMIC) at the University of Victoria, Alberta, Canada using a standard-based quantitation of 3-aminophenyl-derivatized FAs on UHPLC-MS/MS instrument (47). The data were manually annotated to Human Metabolome Database (HMDB) IDs. The data is available in the Supplementary Table 3.
Human Milk Endotoxin
Endotoxin levels were measured by Limulus Amebocyte Lysate (LAL) chromogenic endotoxin quantification kit (Thermo Fisher Scientific) following manufacturer’s instructions.
Statistical Analysis of Milk Factors
Cytokines and IgA were log10 transformed. The normality was tested using Shapiro-Wilk test for all HMOs, cytokines, short-chain fatty acids, and IgA levels. Rochester women and OOM were compared by t-test when Shapiro-Wilk test was not significant (p > 0.05) and Wilcoxon signed-rank test when Shapiro-Wilk test was significant (p < 0.05) in univariate analysis. Multivariate analysis was performed using generalized linear models with Gaussian error distribution when the Shapiro-Wilk test was nonsignificant and Quantile regression when the Shapiro-Wilk test was significant. The association among features were measured by Pearson’s correlation. All analyses were performed in R. To identify a differential activity of HMO cascades, CLIPPER was used (48).
Milk IgA, metabolites, microbiome, and HMOs were integrated, and differential network analysis was performed using xMWAS (49). For microbiome family level, OTU were used. Briefly, xMWAS constructs a matrix of pairwise correlation analysis using sparse partial least squares then ranks and filters the top association scores by p-value and association score. The edge lists are merged to a global network upon which community detection algorithms are utilized to group nodes into communities. Furthermore, centrality scores are calculated for each node in each group separately, then compared to generate differences between groups. The igraph package in R is used to generate a multidata integrative network (50). Community detection is performed using the multilevel community detection method (51).
Results
Maternal and Lifestyle Characteristics
Our questionnaires show OOM mothers have significantly lower rates of self-reported allergic rhinitis, atopic dermatitis, and food allergy and higher rates of exposure to farm animals, dogs, farm milk, and barns. They have higher rates of vaginal and home births and use of bleach and low rates of perinatal antibiotics and exposure to pesticides (Table 1). Previous report from this cohort reported some of the similar findings (41).
Human Milk IgA Antibodies Differ Based on Lifestyle and Are Elevated in the OOM
Whereas, total IgA levels were comparable (Figure 2A) specific IgA1 and IgA2 levels were measured to nine common aeroallergens and three food antigens (milk, egg, and peanut). IgA2 was significantly elevated in OOM milk to dust mite (p = 0.04), peanut (p = 0.02), and egg ovalbumin (p = 0.02) (Figures 2B–D). Among nine bacterial species used in antibody assays, levels of IgA1 to S. equis were higher (p = 0.002) and those to E. cloacae were lower (p = 0.01) in the OOM compared with Rochester mothers’ milk (Figures 2E, F). Several other specific IgA antibodies appeared to be elevated in the OOM as well, but differences did not reach statistical significance.
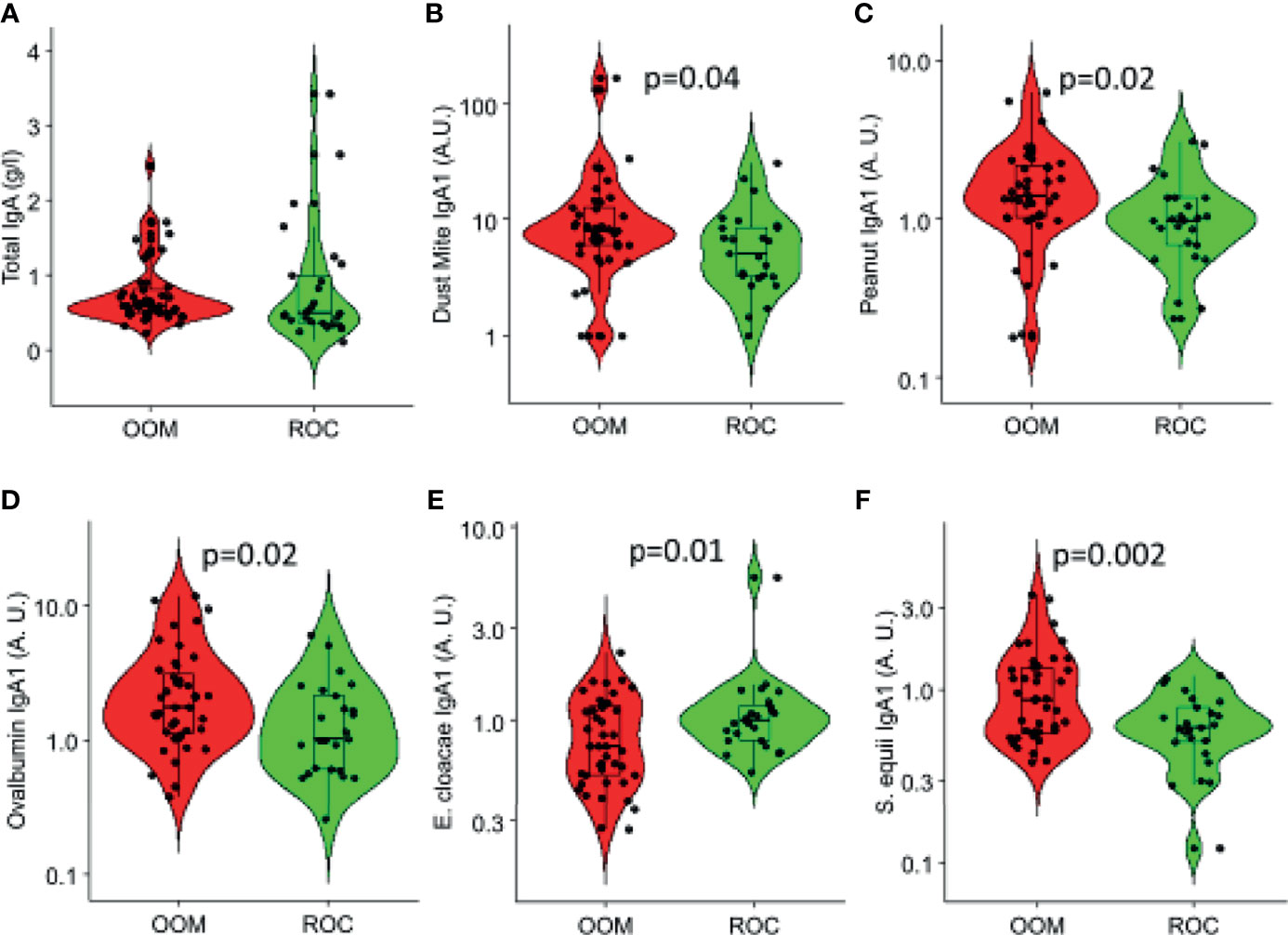
Figure 2 Total and specific IgA responses in human milk that are significantly different between the OOM (red) and Rochester (green) mothers. (A) Total IgA and IgA specific to (B) dust mite (Der p1 and Der f1), (C) peanut, (D) ovalbumin, (E) E. cloacae, and (F) S. equii are shown. Antigen-specific IgAs are normalized between 0 and 1.
Comparison of cumulative levels of IgA1 and IgA2 across OOM and ROC indicated that IgA1 was expanded more in OOM (Figure 3B) whereas IgA2 was expanded more in Rochester (Figure 3D). Hierachial cluster analysis of IgA1 showed that aeroallergen-specific IgA1 antibodies all clustered together as did bacteria-specific IgA1 except for two bacteria (Figure 3A). Peanut-specific IgA1 clustered with aeroantigen IgA1 but the BLG-, OVA-, or casein-specific IgAs did not cluster with either one of the two clusters, neither did they cluster with each other. For IgA2, there was only one cluster, which included aeroallergen IgA2 and peanut, similar to IgA1 (Figure 3C). Food-specific IgA2 antibodies did not cluster with each similar to IgA1. Our initial results on a small number of samples showed that bacterial IgA2 was barely detectable, and therefore not further assessed.
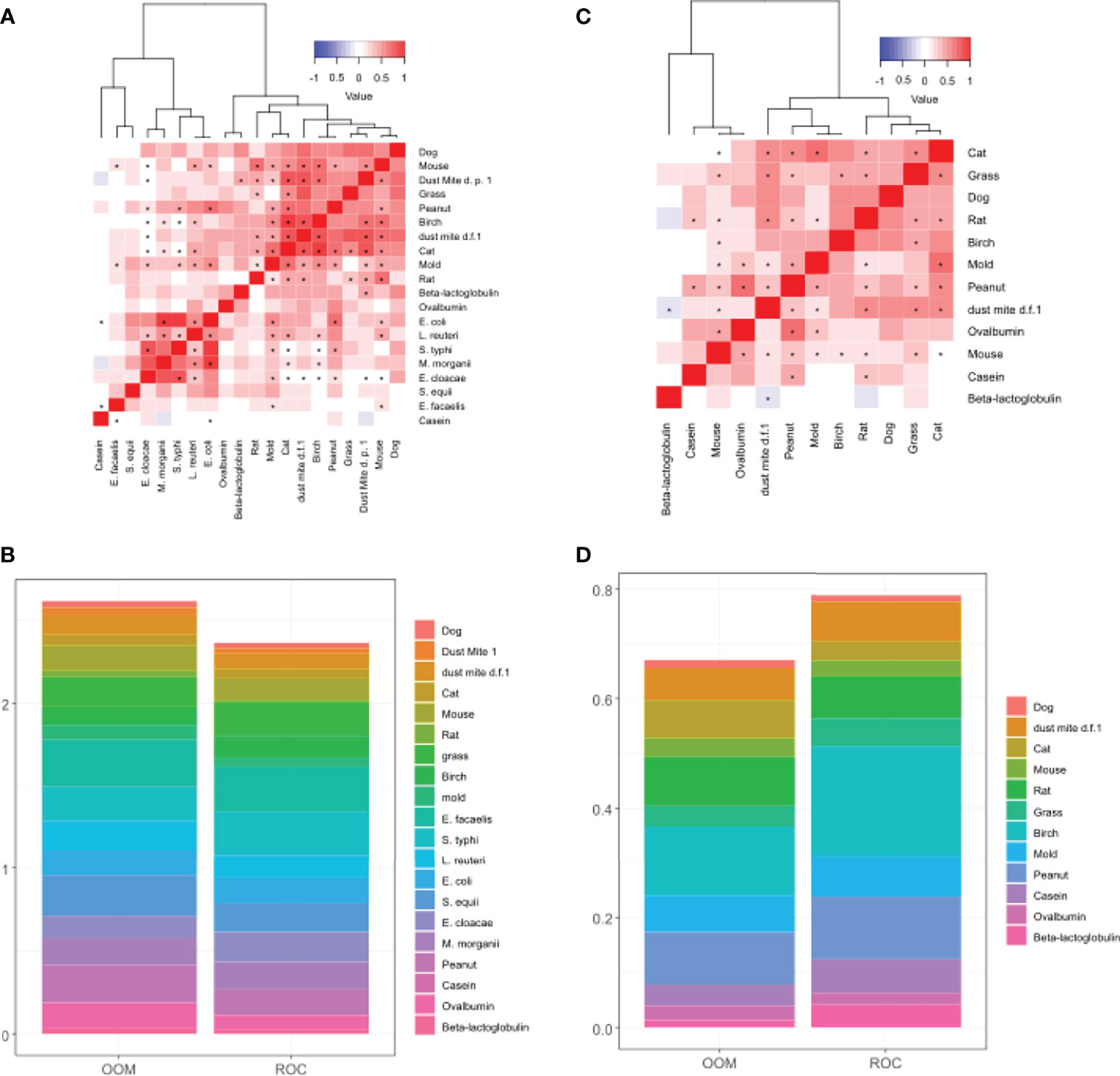
Figure 3 Characterization of IgA1 and IgA2 responses in human milk. Hierachial clustering of IgA1 levels depicting correlation between antigen-specific IgA1 (A) and IgA2 levels (C). (B) Stacked bar plot of IgA1 levels normalized between 0 and 1 depicting higher accumulated levels of IgA1 in OOM. (D) Stacked bar plot of IgA2 levels normalized between 0 and 1 depicting higher accumulated levels of IgA2 in Rochester. IgA2 levels to bacteria antigens were too low to graph. D.p., Dermatophagoides pteronyssinus; d.f, Dermatophagoides farina. * indicates statistically significant correlation with p<0.001.
In a multivariate analysis incorporating BMI, lifestyle, antibiotic use, age, atopy, cats, dogs, and parity, only casein-specific IgA1 remained significantly associated with lifestyle and was higher in Rochester mothers (Table 2). BLG-specific IgA2 was negatively associated with parity. IgA1 to cat and mouse were significantly negatively and positively associated with mother’s atopy, respectively. IgA1 to S. equi was significantly higher in cat owners.
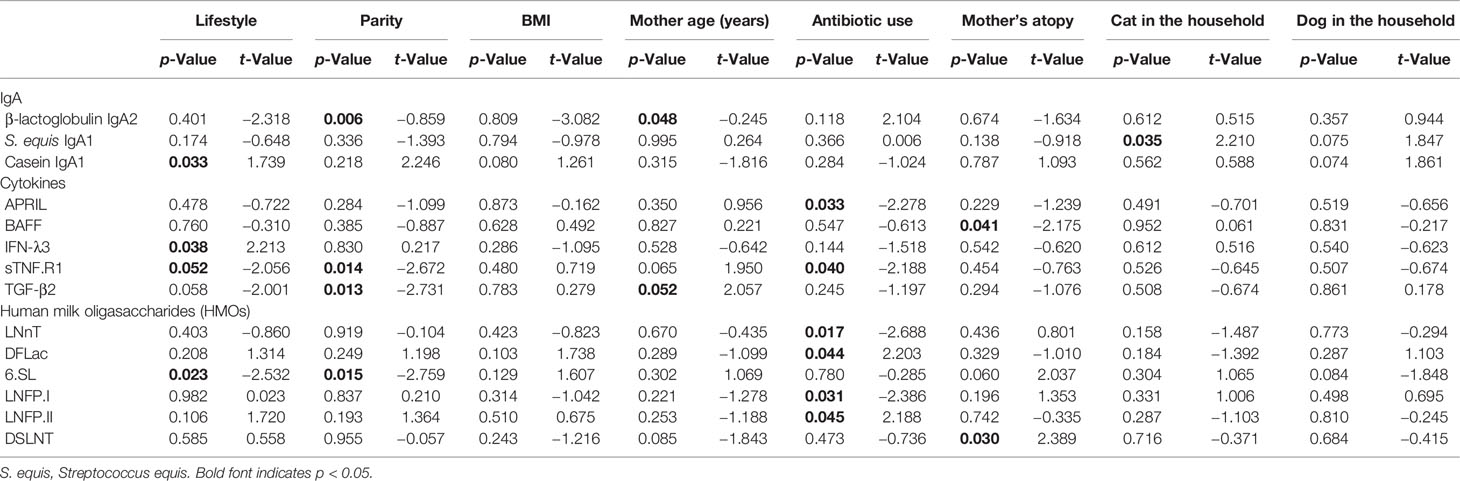
Table 2 Multivariate analysis incorporating specific IgA levels, BMI, lifestyle, antibiotic use, age, atopy, cats, dogs, and parity.
Characterization of Human Milk Cytokines Shows Higher Levels of IgA-Producing Cytokines in OOM
The levels of IFN-λ3 (p =0.03) and TGF-β2 (p = 0.04) were higher in the milk of OOM mothers compared with Rochester mothers (Figures 4A, B). In a multivariate analysis incorporating BMI, lifestyle, antibiotic use, age, atopy, cats, dogs, and parity, IFN-λ3 and sTNFR1 were found to be significantly higher and lower in OOM compared with Rochester, respectively (Table 2). sTNFR1 and TGF-β2 were also significantly lower with higher parity. In addition, functionally related cytokines showed similar trends suggesting biological mechanisms associated with lifestyle and other covariables. As an example, IL-6, TGF-β1, and TGF-β2, cytokines involved in IgA production, are grouped together along with other cytokines involved in IgA production including TSLP, APRIL, and TGF-β3 (Figure 4C). TWEAK and BAFF formed a separate cluster.
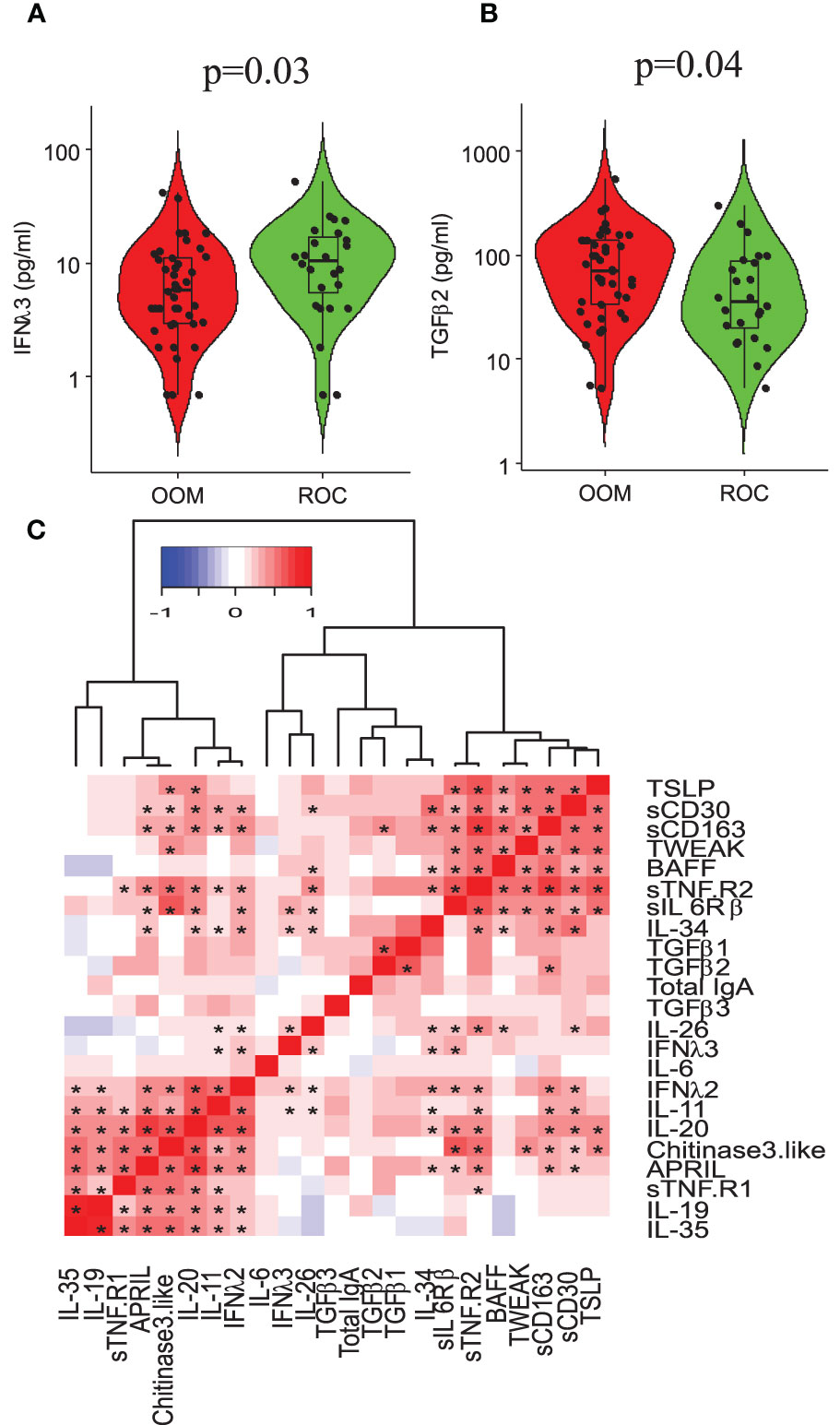
Figure 4 Cytokine profiling of human milk and its association with total IgA levels. Distributions of (A) IL-6 (p = 0.02, 0.05 by Wilcoxon signed-rank test) and (B) TGF-β2 (p = 0.05) in OOM and ROC population shown on logarithmic scale to the base 10. (C) Hierarchial cluster analysis showing correlation between a panel of cytokines. Asterisk, adjusting for mother’s atopy in multivariate analysis.
Specific HMOs Are Correlated With Lifestyle and Maternal Use of Antibiotics
Levels of LNnT and DFLNT were significantly elevated in milk of OOM mothers (p = 0.02 and p = 0.05, respectively), and levels of LNFPIII were higher in the milk of Rochester mothers (p = 0.04) (Figures 5A–C). In a multivariate analysis incorporating BMI, lifestyle, antibiotic use, age, atopy, cats, dogs, and parity, a strong correlation occurred between maternal antibiotic use and levels of several HMOs (Table 2). Moreover, after controlling for other variables, 6’SL was significantly higher in OOM compared with Rochester mothers. Finally, to investigate whether certain metabolic transitions are different between OOM and Rochester groups, we performed a topological analysis by CLIPPER using HMO biosynthetic pathway. The metabolic transitions starting from LNH to FDSLNH were significantly higher in Rochester mothers (Figure 5D).
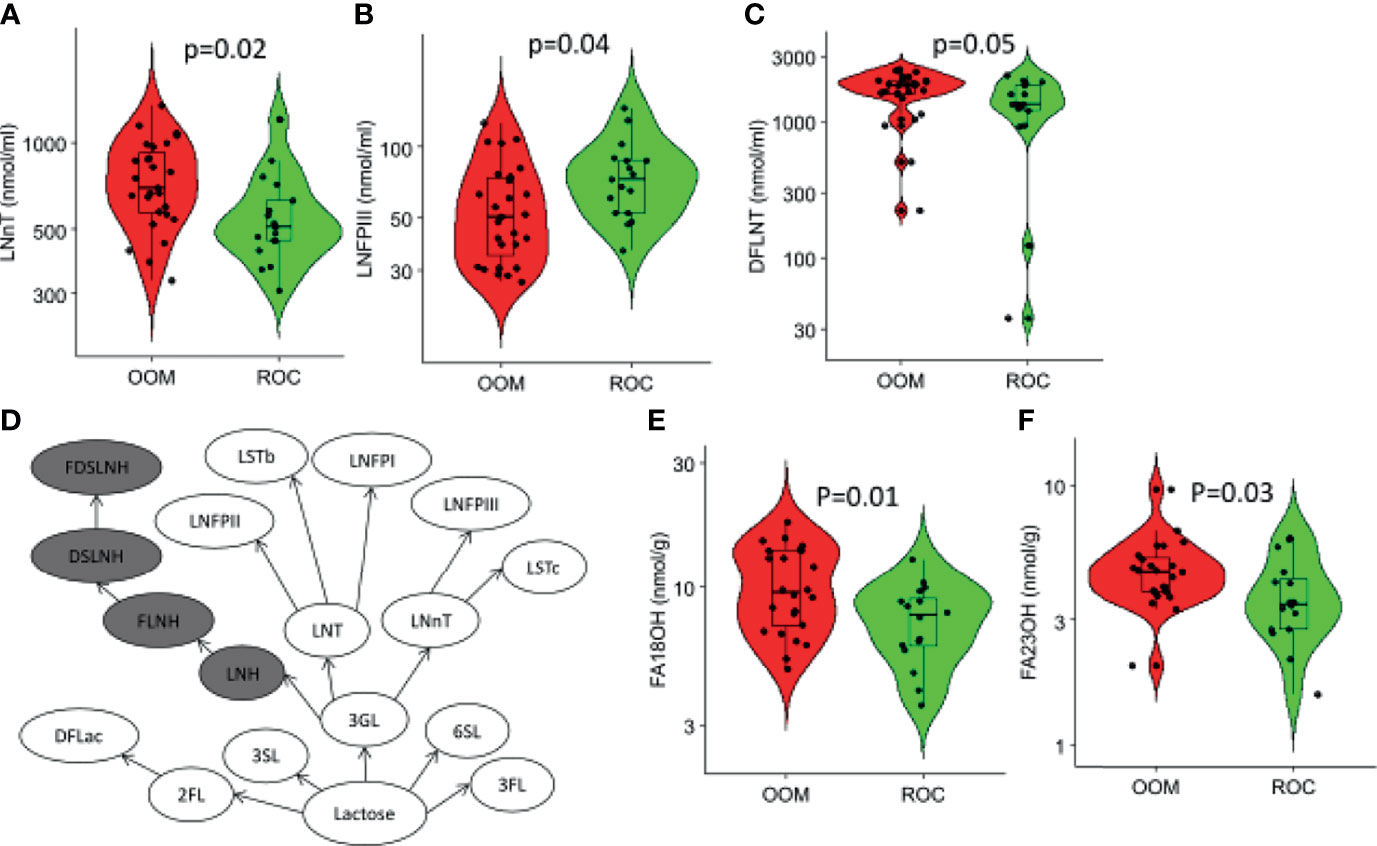
Figure 5 Characterization of human milk oligosaccharides (HMOs) and fatty acid metabolites that are different in Rochester and OOM population. Shown are the three HMOs between the OOM and Rochester mothers by the t-test (A) LNnT, (B) LNFPIII, and (C) DFLNT. (D) Path-based analysis was performed to find differences in the OOM vs. Rochester. The highlighted path has significantly lower (p = 6e−10) activity in OOM. The difference in the pathways is based on homoscedacity. In other words, the paths that have HMOs with different variance across two groups are selected. (E, F) Fatty acids that were significantly different between the OOM and Rochester.
Characterization of Milk Metabolites Between Lifestyles
Characterization of short-, medium-, and long- chain fatty acids in human milk from OOM and Rochester communities revealed only two long-chain fatty acids both of which are elevated in OOM milk (Figures 5E, F). Those are C-18OH (stearic acid) and C-23OH (tricosanoic acid).
Endotoxin
Endotoxin levels were undetectable in most samples, and there were no significant differences between the groups.
Integration of Milk Microbiota With Milk Factors
To identify milk factors associated with milk microbiota, we performed differential network analysis measuring associations across data types (Figure 6). OOM- and ROC-specific networks were identified when HMOs, IgA, and metabolites were combined with microbiota. Community detection was performed to identify topological modules composed of related biomolecules (52, 53). The number of communities are optimized as previously described (51, 54). OOM milk was characterized by five communities which we compare with Rochester milk communities. All associations described below are significant at p < 0.05. Cluster OOM1: total IgA was associated with several medium to long-chain fatty acids in OOM but not in Rochester milk. Cluster OOM2: previously identified milk microbiota Prevotellaceae, Veillonellaceae, and Micrococcaceae are grouped with several fatty acides including butyrate and HMOs including DFLNT, LNH, FLNH, FDSLNH, and DFLac in OOM. Butyrate was specifically positively correlated with highly abundant Micrococcaceae in OOM. Differentially abundant DFLNT was positively correlated with Micrococcaceae, Veillonellaceae, and Planococcaceae. Among these, only Micrococcaceae was found in Rochester communities but was not associated with HMOs and butyrate. Cluster OOM3: highly abundant Staphylococcaceae and LNFPII formed one community in both lifestyles but was also grouped with IgA1 specific to dust mite in OOM. Moreover, Staphylococcaceae was positively correlated with IgA1 to dust mite and mold in OOM. Cluster OOM4: IgA2 to mold was grouped with succinic acid in both lifestyles but was also clustered with Streptococcaceae and Lactobacillaceae in OOM. Cluster OOM5: only one cluster did not include any microbial taxa and was composed of fatty acids including glutaric acid and ethylmalonic acid among others, HMOs 2FL, LSTb, 3’FL, 3’SL, LNT, and IgA2 to birch and BLG and IgA1 to L. reuteri.
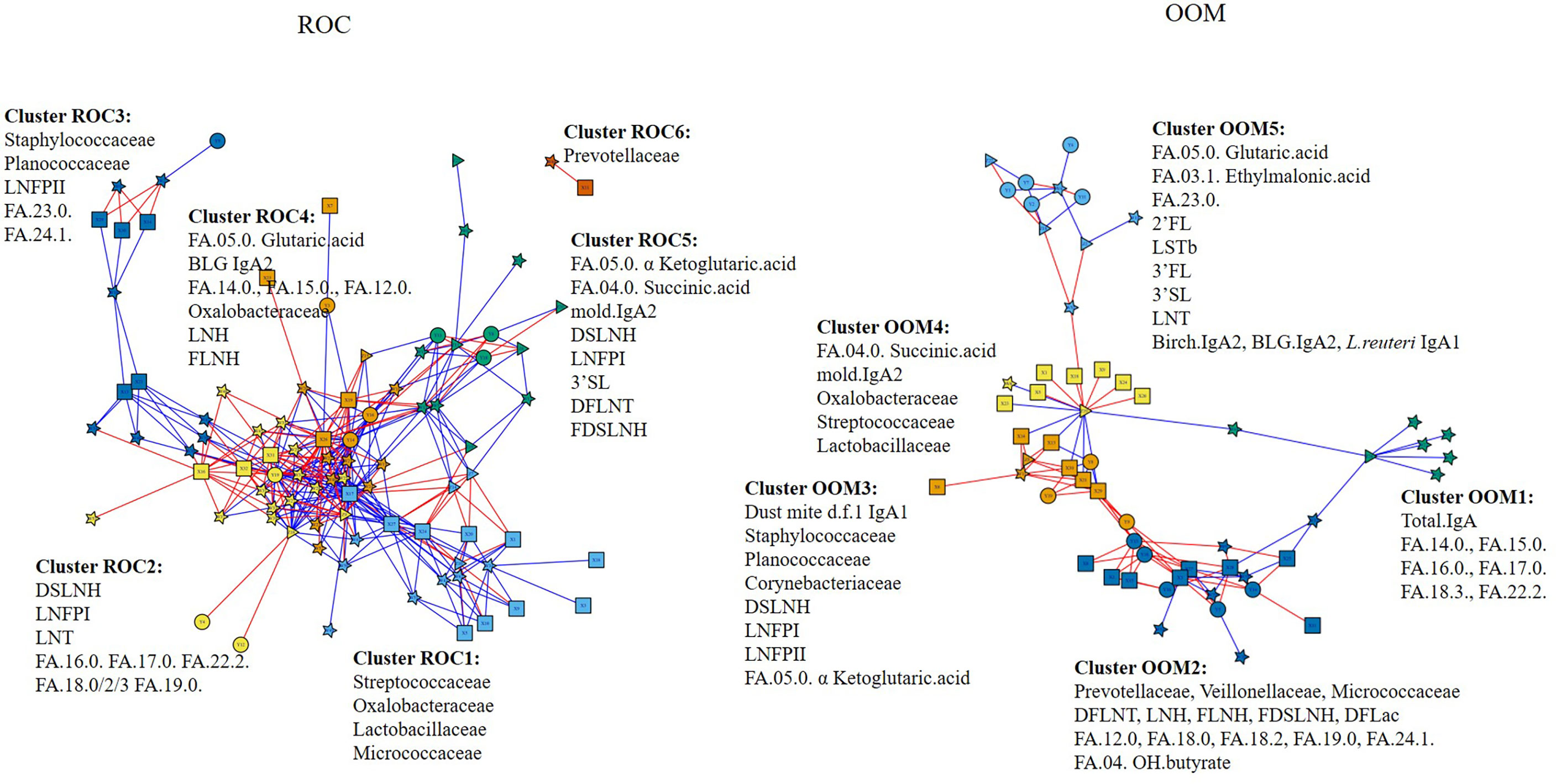
Figure 6 Integrated communities of human milk components in OOM and Rochester populations. Associations between HMOs, fatty acid metabolites, IgA, specific IgA, and milk microbiome were performed using partial least square regression to identify communities in eight Rochester mother (left) and 12 OOM mother (right) populations. Edges represent R2 > 0.7 for ROC and 0.6 for OOM. Red and blue edges represent positive and negative correlations, respectively. The shapes of the nodes represent the following components: squares, human milk OTUs; circles, HMOs; triangles, IgA; and stars, fatty acids.
Integration of HMOs With Infant Gut Microbiota
Although the number of samples were small, there were some interesting initial associations between human milk microbiota, HMOs, and infant gut microbiota. Especially, the association between Bifidobacteriaceae with LNT and LNFPII in Rochester, but between the same taxa and different HMOs, LNnT and LNFPIII in OOM infants, is of interest (Supplementary Figure 1).
Association of Human Milk Components With Development of Atopic Disease
Among the many cytokines, TSLP (p = 0.002) was found in lower concentrations in milk of mothers with an infant with atopic disease (Figure 7). Also, total higher levels of IgA (p = 0.000015) and IgA2 to dust mite (p = 0.0001) were associated with protection against atopic diseases (Figure 7).
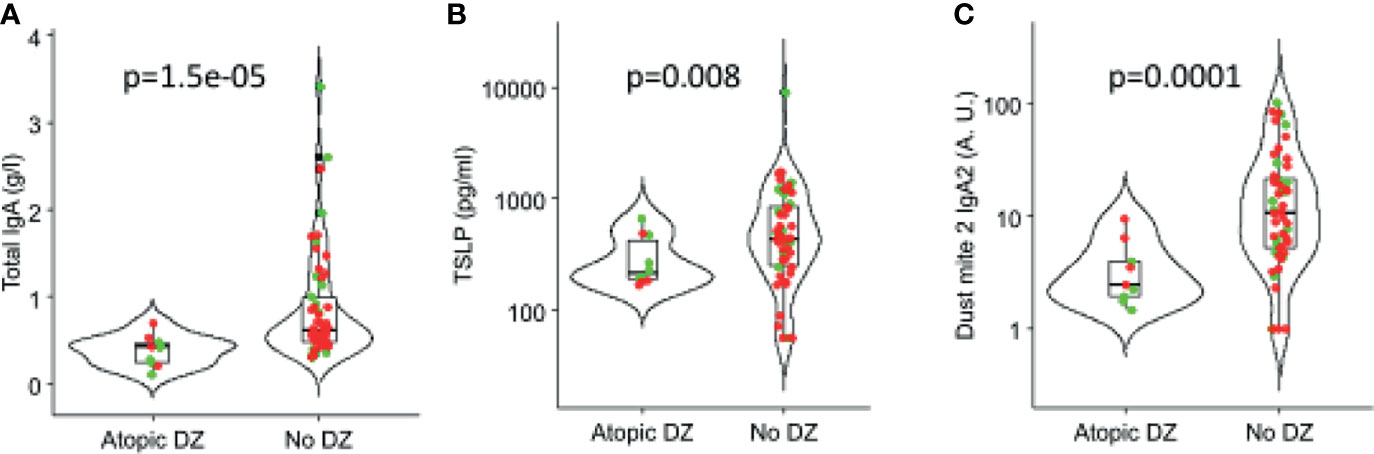
Figure 7 Association of IgA and cytokines in human milk with atopic outcomes in children in the first 3 years of life. (A) Total IgA, (B) TSLP (thymic stromal lymphopoietin), and (C) Dust mite 2 IgA2. Red: OOM. Green: Rochester children.
Discussion
To our knowledge, this is the first multicomponent characterization and network model of human milk composition spanning antibodies, cytokines, human milk oligosaccharides, metabolites, and gut microbiome. It is also the first of such characterization among mother-infant pairs with different lifestyles and risk for allergic diseases. We also performed the most comprehensive assessment of IgA1 and IgA2 antibodies to date. We show that OOM milk is more abundant in many immune components, especially those involved in IgA immunity, and LNnT which enhances Bifidobacterium enrichment. Maternal use of antibiotics was associated with differences in several HMO concentrations. These data support the concept that maternal lifestyle and antibiotic use are regulators of human milk composition, which may have downstream implications for postnatal developmental programming of infant’s gut microbiome and immune system. Considering that the OOM lifestyle dates back about a century, our data may also imply that there has been a change in human milk composition along with its protective properties over time. Although the function of all human milk components is not well known, many of them have downstream implications for postnatal developmental programming of infant’s gut microbiome and immune system suggesting potential benefit to infants born to the OOM community.
While the regulation of human milk composition of immune factors is poorly known, a few prior studies have documented differences in human milk IgA levels between mothers in different countries (14, 55, 56) and those avoiding or consuming particular foods (21), implying that maternal exposures impact milk composition. Consistent with an earlier farmlife study showing higher levels of IgA in farming lifestyle mothers’s milk, we showed upregulation of specific IgA antibodies in the OOM milk. Higher IgA response in the OOM milk was seen specifically to peanut, egg ovalbumin of hens, dust mite, and S. equi, a horse respiratory pathogen; whereas Rochester milk had higher IgA response only to a single antigen, namely E. cloacae, which is a gut commensal. These findings could be attributed to specific antigenic exposures between the OOM and Rochester mothers, for example, the higher levels of egg-specific IgA could reflect that eggs are a staple in their daily diet of the OOM who commonly keep chicken; however, elevated antibody levels against milk of cows or peanut are hardly due to more common exposures in the OOM. Furthermore, aeroallergen-specific IgA responses were elevated to a broad array of aeroallergens in the OOM (although only dust mite reached statistical significance), however, exposure to such inhalant antigens is unlikely to be more common in the OOM who as an example do not allow pets inside the home. These data may imply another mechanism, aside from higher exposures, boosting mucosal antibody production in the OOM. The ultimate function for these specific IgA antibodies is unknown, although we and others have previously reported that higher levels of IgA and specific IgA are associated with protection against milk allergy of cows in an infant (21, 57, 58). While mechanism of protection is unknown, IgA was shown by us not only to decrease the uptake of specific food antigens through the gut epithelium but also to enhance targeted antigen uptake to gut-associated lymphoid tissues in an antibody-dependent manner (21, 59). The presence or the role of aeroallergen- or commensal-specific IgA in human milk has not been previously assessed, although secretory IgA in breast milk plays a role in longterm gut homeostasis by regulating microbiota and host gene expression (16).
In this novel study, we report on the presence of both IgA1 and IgA2 to a broad selection of food, aeroantigens, and commensals. IgA1 antibodies appeared more commonly detectable than IgA2, and for example, commensal-specific IgA2 responses were undetectable. Our data are consistent with few previous studies suggesting that protein antigens such as BLG and tetanus toxoid predominantly induce IgA1; however, our data do not support a predominant IgA2 responses to polysaccharide antigens as reported with pneumococcal, meningococcal, and Hemophilus influenzae (60, 61). Clustering analyses showed that aeroallergens clustered together; however, they also clustered with peanut, which may be explained by the crossreactivity between peanut and pollen. Similarly, commensal IgA responses clustered together. Interestingly, milk and egg antigens were not clustered with either of the two larger clusters, neither were they clustered with each other. This may imply an exposure route that is different from that of the other antigens and from each other, such as the skin or a specific part of the GI tract based on the differential stability of these food antigens in the digestive tract.
The cytokines measured here are of interest because a few prior studies have documented differences in these human milk cytokine levels between geographic locations and countries. Higher levels of IL-10 were seen in Estonia mothers than in Swedish mother (55) and that of TGF-β1 in Finland compared with other European countries (14). Another study showed differences in cytokines and growth factor levels between developing and developed world countries (62, 63). Here, we found IFN-λ3 and TGF-β2 at higher levels in the OOM. Interestingly, a study performed in Sweden among mothers who had immigrated from a developing country early on had higher levels of IL-6, IL-8, and TGF-β1 in their milk suggesting that early life exposures have an impact on the immune system that sustains into adulthood and impacts human milk composition (64). In addition, we found several cytokines involved in IgA production clustered together, such as IL-6, TGF-β1, and TGF-β2, which clustered with TGF-β3 and APRIL and TSLP. This may indicate common pathways involved in cytokine production for the benefit of inducing upregulation of IgA levels in human milk. The cytokines measured here are of special interest, because we had previously identified those in higher concentrations associated with protection against food allergy (22). It may therefore be plausible that milk cytokines and IgA function in concert to support the infant against immune-mediated diseases.
Several large studies have confirmed the role of breastfeeding in modulating gut microbiome composition (24), showing that breast milk selects for intestinal microbiome dominated by Bifidobacterium for the first year of life (65). This is due to HMOs that are complex glycans and the third largest solid component in human milk. They are typically nondigestible by humans but provide the main substrate for infant’s gut microbiota, particularly, for Bifidobacterium and some Bacteroides (29, 30). Therefore, when breastfeeding ceases, signficant shifts in gut microbiome composition are seen with Clostridium and Bacteroides species replacing Lactobacilli-, Bifidobacteria-, and Enterobacteriaceae-dominated microbiota (66–68). We recently reported that HMO composition was related to maternal probiotic exposures (33). In the current report, we identified specific HMO levels associated with maternal antibiotics, suggesting a role for maternal microbiome in regulation of HMO composition. In this study, we found few differences in single HMOs between the OOM and Rochester mothers (LNnT, LFPIII, and DFLNT). However, because metabolic transitions between HMOs are not necessarily known, we sought to investigate whether certain conversions are different between OOM and Rochester groups. The path starting from LNH to FDSLNH were significantly higher in Rochester mothers; however, we found no specific HMO composition in relation to atopic disease development. This is in contrast with prior studies that have associated specific HMO profiles with atopic diseases (31–33), possibly explained by the small sample size.
Of particular interest in human milk is the presence of fatty acids, especially short chain fatty acids (SCFA), which are solely metabolized by gut bacteria from otherwise indigestible carbohydrates of fiber-rich diets. There is one known study so far indicating lower levels of acetate and butyrate in atopic mothers (42). SCFAs in human milk are likely produced by the maternal gut microbiota and distributed to the mammary gland via the circulation (42). We found an association between milk butyrate and specific taxa such as Prevotella, which is a known producer of butyrate (69), which may provide the first current evidence that SCFA in humans be produced by the human milk microbiota. Alternatively, maternal diet may contribute to SCFA levels in human milk. We also found higher levels of tricosanoic acid and palmitic acid in the OOM milk, but the implication of this unclear. FAs such as palmitic acid in human milk (70, 71) has been associated with changes in infant gut microbiota, but causality is unclear.
In this cohort, development of atopic disease in early childhood was more common in Rochester and associated with lower levels of IgA to dust mite as well as TSLP, but larger longitudinal studies are needed to conclusively assess whether OOM milk composition may provide benefit to their infants against development of allergic diseases. Assessing the relationships between human milk components and development of atopic outcomes in the children will only provide correlative data and causal relationship cannot be drawn. Human milk is only one of the many factors that can affect the development of atopic outcomes by 3 years of age. Not all the factors have been assessed or controlled for in this study. Further limitations include a small sample size of this pilot study and that all the assays were not performed on every sample due to limitation of volume. Allergic outcomes were limited to self-report of physician diagnosis or symptoms of atopic disease, although assessment was done by a pediatric allergist by telephone follow-up. Strenghts include access to a unique population living a traditional, agrarian lifestyle, with lifestyle representative of that commonly encountered a century ago, when allergic diseases were rare.
Boosting immunomodulatory properties of human milk might present an opportunity to modulate infant’s gut microbiome and risk for allergic diseases. Differences in human milk composition may have implications for developmental programming of the infant immune system postnatally by human milk but are also likely reflective of the maternal immune environment with implications to prenatal developmental programming. More studies are clearly needed to understand the role of maternally derived prenatal and postnatal factors on allergic diseases.
Data Availability Statement
The datasets presented in this study can be found in online repositories and in Supplementary Material. The names of the repository/repositories and accession number(s) can be found in the article.
Ethics Statement
The studies involving human participants were reviewed and approved by University of Rochester Institutional Review Board. Written informed consent to participate in this study was provided by the participants’ legal guardian/next of kin.
Author Contributions
AS, JL, CM, and KJ conceived the overall study and supervised the research. AS, RC, CP, RP, SF, PR, JS, CY, LB, KB, ST, EP, DW, MA, RL, JC, JT, and KJ contributed to the literature search, data collection, and data analysis. AS, JT, JC, and KJ provided the figures and drafted the manuscript, with additional input from all authors. All authors contributed to the article and approved the submitted version.
Funding
The project described was supported by Grant Numbers K08 AI091655 (KJ), U01 AI131344 from the National Institute of Infectious and Allergic Diseases (KJ), R21 TR002516 from the National Center for Advancing Translational Sciences, Founders’ Distinguished Professorship in Pediatric Allergy (KJ), and by Pilot Award (P30 ES001247) from Environmental Health Sciences Center (JL, CM), National Institute of Environmental Health Sciences, and Rochester CTSA award UL1 TR000042 from the National Center for Advancing Translational Sciences (JL, CM) of the National Institutes of Health. The content is solely the responsibility of the authors and does not necessarily represent the official views of the National Institutes of Health.
Conflict of Interest
The authors declare that the research was conducted in the absence of any commercial or financial relationships that could be construed as a potential conflict of interest.
Publisher’s Note
All claims expressed in this article are solely those of the authors and do not necessarily represent those of their affiliated organizations, or those of the publisher, the editors and the reviewers. Any product that may be evaluated in this article, or claim that may be made by its manufacturer, is not guaranteed or endorsed by the publisher.
Acknowledgments
We would like to thank Mary Ann Martin and the late Joyce Wade, CNM for their guidance and assistance in recruitment efforts within the Old Order Mennonite Community and the participating families without whom this research would not be possible.
Supplementary Material
The Supplementary Material for this article can be found online at: https://www.frontiersin.org/articles/10.3389/fimmu.2021.741513/full#supplementary-material
Supplementary Figure 1 | Association between HMOs, human milk microbiome, and infant gut microbiome. Associations were measured using partial least square regression to identify communities represented by networks with blue, green, yellow, and gold node colors; using six Rochester mother-infant pairs and six OOM mother-infant pairs. Edges represent R2 > 0.73 for ROC and 0.63 for OOM. Red and blue lines represent positive and negative correlations, respectively. The shapes of the nodes represent components: squares, human milk microbiota (underlined); circles, HMOs; triangles, infant stool microbiota.
Supplementary Table 1 | Milk HMOs, IgA, and cytokines.
Supplementary Table 2 | Human milk oligosaccharides measured.
Supplementary Table 3 | Milk metabolites.
Abbreviations
AUC, area under curve; BMI, body mass index; FA, fatty acid; qPCR, quantitative PCR; HMO, human milk oligosaccaharide; IQR, interquartile range; LefSe, linear discriminant analysis effect size; LNnT, lacto-N-neotetraose; OOM, Old Order Mennonite; OTU, operational taxonomic unit; PERMANOVA, permutational multivariate ANOVA; rRNA, ribosomal RNA; SCFA, short-chain fatty acid; Treg, regulatory T cell.
References
1. Beaglehole R, Bonita R, Alleyne G, Horton R, Li L, Lincoln P, et al. UN High-Level Meeting on Non-Communicable Diseases: Addressing Four Questions. Lancet (2011) 378(9789):449–55. doi: 10.1016/S0140-6736(11)60879-9
2. Riedler J, Braun-Fahrlander C, Eder W, Schreuer M, Waser M, Maisch S, et al. Exposure to Farming in Early Life and Development of Asthma and Allergy: A Cross-Sectional Survey. Lancet (2001) 358(9288):1129–33. doi: 10.1016/S0140-6736(01)06252-3
3. Ege MJ, Bieli C, Frei R, van Strien RT, Riedler J, Ublagger E, et al. Prenatal Farm Exposure Is Related to the Expression of Receptors of the Innate Immunity and to Atopic Sensitization in School-Age Children. J Allergy Clin Immunol (2006) 117(4):817–23. doi: 10.1016/j.jaci.2005.12.1307
4. Ege MJ, Herzum I, Buchele G, Krauss-Etschmann S, Lauener RP, Roponen M, et al. Prenatal Exposure to a Farm Environment Modifies Atopic Sensitization at Birth. J Allergy Clin Immunol (2008) 122(2):407–12, 12 e1-4. doi: 10.1016/j.jaci.2008.06.011
5. Holbreich M, Genuneit J, Weber J, Braun-Fahrlander C, Waser M, von Mutius E. Amish Children Living in Northern Indiana Have a Very Low Prevalence of Allergic Sensitization. J Allergy Clin Immunol (2012) 129(6):1671–3. doi: 10.1016/j.jaci.2012.03.016
6. Stein MM, Hrusch CL, Gozdz J, Igartua C, Pivniouk V, Murray SE, et al. Innate Immunity and Asthma Risk in Amish and Hutterite Farm Children. New Engl J Med (2016) 375(5):411–21. doi: 10.1056/NEJMoa1508749
7. Martina C, Looney RJ, Marcus C, Allen M, Stahlhut R. Prevalence of Allergic Disease in Old Order Mennonites in New York. Ann Allergy Asthma Immunol (2016) 177(5):562–3. doi: 10.1016/j.anai.2016.08.023
8. Phillips JT, Stahlhut RW, Looney RJ, Jarvinen KM. Food Allergy, Breastfeeding, and Introduction of Complementary Foods in the New York Old Order Mennonite Community. Ann Allergy Asthma Immunol (2020) 124(3):292–4 e2. doi: 10.1016/j.anai.2019.12.019
9. Tantoco JC, Elliott Bontrager J, Zhao Q, DeLine J, Seroogy CM. The Amish Have Decreased Asthma and Allergic Diseases Compared With Old Order Mennonites. Ann Allergy Asthma Immunol (2018) 121(2):252–3.e1. doi: 10.1016/j.anai.2018.05.016
10. Loss G, Bitter S, Wohlgensinger J, Frei R, Roduit C, Genuneit J, et al. Prenatal and Early-Life Exposures Alter Expression of Innate Immunity Genes: The PASTURE Cohort Study. J Allergy Clin Immunol (2012) 130(2):523–30.e9. doi: 10.1016/j.jaci.2012.05.049
11. Loss G, Apprich S, Waser M, Kneifel W, Genuneit J, Buchele G, et al. The Protective Effect of Farm Milk Consumption on Childhood Asthma and Atopy: The GABRIELA Study. J Allergy Clin Immunol (2011) 128(4):766–73 e4. doi: 10.1016/j.jaci.2011.07.048
12. Roduit C, Wohlgensinger J, Frei R, Bitter S, Bieli C, Loeliger S, et al. Prenatal Animal Contact and Gene Expression of Innate Immunity Receptors at Birth are Associated With Atopic Dermatitis. J Allergy Clin Immunol (2011) 127(1):179–85, 85.e1. doi: 10.1016/j.jaci.2010.10.010
13. Schaub B, Liu J, Hoppler S, Schleich I, Huehn J, Olek S, et al. Maternal Farm Exposure Modulates Neonatal Immune Mechanisms Through Regulatory T Cells. J Allergy Clin Immunol (2009) 123(4):774–82 e5. doi: 10.1016/j.jaci.2009.01.056
14. Orivuori L, Loss G, Roduit C, Dalphin JC, Depner M, Genuneit J, et al. Soluble Immunoglobulin A in Breast Milk is Inversely Associated With Atopic Dermatitis at Early Age: The PASTURE Cohort Study. Clin Exp Allergy: J Br Soc Allergy Clin Immunol (2014) 44(1):102–12. doi: 10.1111/cea.12199
15. Jarvinen KM, Martin H, Oyoshi MK. Immunomodulatory Effects of Breast Milk on Food Allergy. Ann Allergy Asthma Immunol (2019) 123(2):133–43. doi: 10.1016/j.anai.2019.04.022
16. Rogier EW, Frantz AL, Bruno ME, Wedlund L, Cohen DA, Stromberg AJ, et al. Secretory Antibodies in Breast Milk Promote Long-Term Intestinal Homeostasis by Regulating the Gut Microbiota and Host Gene Expression. Proc Natl Acad Sci United States America (2014) 111(8):3074–9. doi: 10.1073/pnas.1315792111
17. Ohsaki A, Venturelli N, Buccigrosso TM, Osganian SK, Lee J, Blumberg RS, et al. Maternal IgG Immune Complexes Induce Food Allergen-Specific Tolerance in Offspring. J Exp Med (2018) 215(1):91–113. doi: 10.1084/jem.20171163
18. Verhasselt V, Milcent V, Cazareth J, Kanda A, Fleury S, Dombrowicz D, et al. Breast Milk-Mediated Transfer of an Antigen Induces Tolerance and Protection From Allergic Asthma. Nat Med (2008) 14(2):170–5. doi: 10.1038/nm1718
19. Knoop A, Planitz P, Wust B, Thevis M. Analysis of Cobalt for Human Sports Drug Testing Purposes Using ICP- and LC-ICP-Ms. Drug Test Anal (2020) 12(11–12):133–43. doi: 10.1002/dta.2962
20. Knoop KA, McDonald KG, Coughlin PE, Kulkarni DH, Gustafsson JK, Rusconi B, et al. Synchronization of Mothers and Offspring Promotes Tolerance and Limits Allergy. JCI Insight (2020) 5(15):e137943. doi: 10.1172/jci.insight.137943
21. Jarvinen KM, Westfall JE, Seppo MS, James AK, Tsuang AJ, Feustel PJ, et al. Role of Maternal Elimination Diets and Human Milk IgA in the Development of Cow’s Milk Allergy in the Infants. Clin Exp Allergy (2014) 44(1):69–78. doi: 10.1111/cea.12228
22. Jarvinen KM, Suarez-Farinas M, Savilahti E, Sampson HA, Berin MC. Immune Factors in Breast Milk Related to Infant Milk Allergy are Independent of Maternal Atopy. J Allergy Clin Immunol (2015) 135(5):1390–3 e1-6. doi: 10.1016/j.jaci.2014.10.051
23. Azad MB, Konya T, Maughan H, Guttman DS, Field CJ, Chari RS, et al. Gut Microbiota of Healthy Canadian Infants: Profiles by Mode of Delivery and Infant Diet at 4 Months. CMAJ (2013) 185(5):385–94. doi: 10.1503/cmaj.121189
24. Levin AM, Sitarik AR, Havstad SL, Fujimura KE, Wegienka G, Cassidy-Bushrow AE, et al. Joint Effects of Pregnancy, Sociocultural, and Environmental Factors on Early Life Gut Microbiome Structure and Diversity. Sci Rep (2016) 6:31775. doi: 10.1038/srep31775
25. Fujimura KE, Sitarik AR, Havstad S, Lin DL, Levan S, Fadrosh D, et al. Neonatal Gut Microbiota Associates With Childhood Multisensitized Atopy and T Cell Differentiation. Nat Med (2016) 10:1187–91. doi: 10.1038/nm.4176
26. Azad MB, Konya T, Guttman DS, Field CJ, Sears MR, HayGlass KT, et al. Infant Gut Microbiota and Food Sensitization: Associations in the First Year of Life. Clin Exp Allergy (2015) 45(3):632–43. doi: 10.1111/cea.12487
27. Savage JH, Lee-Sarwar KA, Sordillo J, Bunyavanich S, Zhou Y, O’Connor G, et al. A Prospective Microbiome-Wide Association Study of Food Sensitization and Food Allergy in Early Childhood. Allergy (2018) 73(1):145–52. doi: 10.1111/all.13232
28. Arrieta MC, Stiemsma LT, Dimitriu PA, Thorson L, Russell S, Yurist-Doutsch S, et al. Early Infancy Microbial and Metabolic Alterations Affect Risk of Childhood Asthma. Sci Transl Med (2015) 7(307):307ra152. doi: 10.1126/scitranslmed.aab2271
29. Yu ZT, Chen C, Kling DE, Liu B, McCoy JM, Merighi M, et al. The Principal Fucosylated Oligosaccharides of Human Milk Exhibit Prebiotic Properties on Cultured Infant Microbiota. Glycobiology (2013) 23(2):169–77. doi: 10.1093/glycob/cws138
30. Sela DA, Mills DA. Nursing Our Microbiota: Molecular Linkages Between Bifidobacteria and Milk Oligosaccharides. Trends Microbiol (2010) 18(7):298–307. doi: 10.1016/j.tim.2010.03.008
31. Miliku K, Robertson B, Sharma AK, Subbarao P, Becker AB, Mandhane PJ, et al. Human Milk Oligosaccharide Profiles and Food Sensitization Among Infants in the CHILD Study. Allergy (2018) 73(10):2070–3. doi: 10.1111/all.13476
32. Lodge CJ, Lowe AJ, Milanzi E, Bowatte G, Abramson MJ, Tsimiklis H, et al. Human Milk Oligosaccharide Profiles and Allergic Disease Up to 18 Years. J Allergy Clin Immunol (2020) 147(3):1041–8. doi: 10.1016/j.jaci.2020.06.027
33. Seppo AE, Autran CA, Bode L, Jarvinen KM. Human Milk Oligosaccharides and Development of Cow’s Milk Allergy in Infants. J Allergy Clin Immunol (2017) 139(2):708–11 e5. doi: 10.1016/j.jaci.2016.08.031
34. Sprenger N, Odenwald H, Kukkonen AK, Kuitunen M, Savilahti E, Kunz C. FUT2-Dependent Breast Milk Oligosaccharides and Allergy at 2 and 5 Years of Age in Infants With High Hereditary Allergy Risk. Eur J Nutr (2016) 56(3):1293–1301. doi: 10.1007/s00394-016-1180-6
35. Pannaraj PS, Li F, Cerini C, Bender JM, Yang S, Rollie A, et al. Association Between Breast Milk Bacterial Communities and Establishment and Development of the Infant Gut Microbiome. JAMA Pediatr (2017) 171(7):647–54. doi: 10.1001/jamapediatrics.2017.0378
36. Asnicar F, Manara S, Zolfo M, Truong DT, Scholz M, Armanini F, et al. Studying Vertical Microbiome Transmission From Mothers to Infants by Strain-Level Metagenomic Profiling. mSystems (2017) 2(1):e00164–16. doi: 10.1128/mSystems.00164-16
37. Biagi E, Quercia S, Aceti A, Beghetti I, Rampelli S, Turroni S, et al. The Bacterial Ecosystem of Mother’s Milk and Infant’s Mouth and Gut. Front Microbiol (2017) 8:1214. doi: 10.3389/fmicb.2017.01214
38. Jost T, Lacroix C, Braegger CP, Rochat F, Chassard C. Vertical Mother-Neonate Transfer of Maternal Gut Bacteria via Breastfeeding. Environ Microbiol (2014) 16(9):2891–904. doi: 10.1111/1462-2920.12238
39. Murphy K, Curley D, O’Callaghan TF, O’Shea CA, Dempsey EM, O’Toole PW, et al. The Composition of Human Milk and Infant Faecal Microbiota Over the First Three Months of Life: A Pilot Study. Sci Rep (2017) 7:40597. doi: 10.1038/srep40597
40. Fehr K, Moossavi S, Sbihi H, Boutin RCT, Bode L, Robertson B, et al. Breastmilk Feeding Practices Are Associated With the Co-Occurrence of Bacteria in Mothers’ Milk and the Infant Gut: The CHILD Cohort Study. Cell Host Microbe (2020) 28(2):285–97 e4. doi: 10.1016/j.chom.2020.06.009
41. Seppo AE, Bu K, Jumabaeva M, Thakar J, Choudhury RA, Yonemitsu C, et al. Infant Gut Microbiome is Enriched With Bifidobacterium Longum Ssp. Infantis in Old Order Mennonites With Traditional Farming Lifestyle. Allergy (2021). doi: 10.1111/all.14877
42. Stinson LF, Gay MCL, Koleva PT, Eggesbo M, Johnson CC, Wegienka G, et al. Human Milk From Atopic Mothers Has Lower Levels of Short Chain Fatty Acids. Front Immunol (2020) 11:1427. doi: 10.3389/fimmu.2020.01427
43. Benckert J, Schmolka N, Kreschel C, Zoller MJ, Sturm A, Wiedenmann B, et al. The Majority of Intestinal IgA+ and IgG+ Plasmablasts in the Human Gut are Antigen-Specific. J Clin Invest (2011) 121(5):1946–55. doi: 10.1172/JCI44447
44. Planer JD, Peng Y, Kau AL, Blanton LV, Ndao IM, Tarr PI, et al. Development of the Gut Microbiota and Mucosal IgA Responses in Twins and Gnotobiotic Mice. Nature (2016) 534(7606):263–6. doi: 10.1038/nature17940
45. Caporaso JG, Lauber CL, Walters WA, Berg-Lyons D, Lozupone CA, Turnbaugh PJ, et al. Global Patterns of 16S rRNA Diversity at a Depth of Millions of Sequences Per Sample. Proc Natl Acad Sci USA (2011) 108 Suppl 1:4516–22. doi: 10.1038/ismej.2011.74
46. Clemente JC, Pehrsson EC, Blaser MJ, Sandhu K, Gao Z, Wang B, et al. The Microbiome of Uncontacted Amerindians. Sci Adv (2015) 1(3):e1500183. doi: 10.1126/sciadv.1500183
47. Han J, Lin K, Sequeira C, Borchers CH. An Isotope-Labeled Chemical Derivatization Method for the Quantitation of Short-Chain Fatty Acids in Human Feces by Liquid Chromatography-Tandem Mass Spectrometry. Anal Chim Acta (2015) 854:86–94. doi: 10.1016/j.aca.2014.11.015
48. Martini P, Sales G, Massa MS, Chiogna M, Romualdi C. Along Signal Paths: An Empirical Gene Set Approach Exploiting Pathway Topology. Nucleic Acids Res (2013) 41(1):e19. doi: 10.1093/nar/gks866
49. Uppal K, Ma C, Go YM, Jones DP, Wren J. xMWAS: A Data-Driven Integration and Differential Network Analysis Tool. Bioinformatics (2018) 34(4):701–2. doi: 10.1093/bioinformatics/btx656
50. Csardi G, Nepusz T. The Igraph Software Package for Complex Network Research. InterJournal Complex Systems 1695 (2006) (5):1–9.
51. Blondel VD, Guillaume JL, Lambiotte R, Lefebvre E. Fast Unfolding of Communities in Large Networks. J Stat Mechanics Theory Experiment (2008). doi: 10.1088/1742-5468/2008/10/P10008
52. Barabasi AL, Gulbahce N, Loscalzo J. Network Medicine: A Network-Based Approach to Human Disease. Nat Rev Genet (2011) 12(1):56–68. doi: 10.1038/nrg2918
53. Yang Z, Algesheimer R, Tessone CJ. Corrigendum: A Comparative Analysis of Community Detection Algorithms on Artificial Networks. Sci Rep (2017) 7:46845. doi: 10.1038/srep46845
54. Thakar J, Thatcher TH, Smith MR, Woeller CF, Walker DI, Utell MJ, et al. Integrative Network Analysis Linking Clinical Outcomes With Environmental Exposures and Molecular Variations in Service Personnel Deployed to Balad and Bagram. J Occup Environ Med (2019) 12(Suppl 12):S65–72. doi: 10.1097/JOM.0000000000001710
55. Tomicic S, Johansson G, Voor T, Bjorksten B, Bottcher MF, Jenmalm MC. Breast Milk Cytokine and IgA Composition Differ in Estonian and Swedish Mothers-Relationship to Microbial Pressure and Infant Allergy. Pediatr Res (2010) 68(4):330–4. doi: 10.1203/PDR.0b013e3181ee049d
56. Munblit D, Sheth S, Abrol P, Treneva M, Peroni DG, Chow LY, et al. Exposures Influencing Total IgA Level in Colostrum. J Dev Origins Health Dis (2016) 7(1):61–7. doi: 10.1017/S2040174415001476
57. Savilahti E, Tainio VM, Salmenpera L, Arjomaa P, Kallio M, Perheentupa J, et al. Low Colostral IgA Associated With Cow’s Milk Allergy. Acta Paediatr Scand (1991) 80(12):1207–13. doi: 10.1111/j.1651-2227.1991.tb11810.x
58. Jarvinen KM, Laine ST, Jarvenpaa AL, Suomalainen HK. Does Low IgA in Human Milk Predispose the Infant to Development of Cow’s Milk Allergy? Pediatr Res (2000) 48(4):457–62. doi: 10.1203/00006450-200010000-00007
59. Jarvinen KM, Westfall J, De Jesus M, Mantis NJ, Carroll JA, Metzger DW, et al. Role of Maternal Dietary Peanut Exposure in Development of Food Allergy and Oral Tolerance. PloS One (2015) 10(12):e0143855. doi: 10.1371/journal.pone.0143855
60. Ladjeva I, Peterman JH, Mestecky J. IgA Subclasses of Human Colostral Antibodies Specific for Microbial and Food Antigens. Clin Exp Immunol (1989) 78(1):85–90.
61. Sanchez-Salguero ES, Rodriguez-Chacon BC, Leyva-Daniel J, Zambrano-Carrasco J, Miguel-Rodriguez CE, Santos-Argumedo L. Antigenic Stimulation During Pregnancy Modifies Specific Iga1 and Iga2 Subclasses in Human Colostrum According to the Chemical Composition of the Antigen. Rev Invest Clin (2020) 72(2):80–7. doi: 10.24875/RIC.19003230
62. McGuire MK, Meehan CL, McGuire MA, Williams JE, Foster J, Sellen DW, et al. What’s Normal? Oligosaccharide Concentrations and Profiles in Milk Produced by Healthy Women Vary Geographically. Am J Clin Nutr (2017) 105(5):1086–100. doi: 10.3945/ajcn.116.139980
63. Ruiz L, Espinosa-Martos I, Garcia-Carral C, Manzano S, McGuire MK, Meehan CL, et al. What’s Normal? Immune Profiling of Human Milk From Healthy Women Living in Different Geographical and Socioeconomic Settings. Front Immunol (2017) 8:696. doi: 10.3389/fimmu.2017.00696
64. Amoudruz P, Holmlund U, Schollin J, Sverremark-Ekstrom E, Montgomery SM. Maternal Country of Birth and Previous Pregnancies are Associated With Breast Milk Characteristics. Pediatr Allergy Immunol (2009) 20(1):19–29. doi: 10.1111/j.1399-3038.2008.00754.x
65. Subramanian S, Huq S, Yatsunenko T, Haque R, Mahfuz M, Alam MA, et al. Persistent Gut Microbiota Immaturity in Malnourished Bangladeshi Children. Nature (2014) 510(7505):417–21. doi: 10.1038/nature13421
66. Bergstrom A, Skov TH, Bahl MI, Roager HM, Christensen LB, Ejlerskov KT, et al. Establishment of Intestinal Microbiota During Early Life: A Longitudinal, Explorative Study of a Large Cohort of Danish Infants. Appl Environ Microbiol (2014) 80(9):2889–900. doi: 10.1128/AEM.00342-14
67. Backhed F, Roswall J, Peng Y, Feng Q, Jia H, Kovatcheva-Datchary P, et al. Dynamics and Stabilization of the Human Gut Microbiome During the First Year of Life. Cell Host Microbe (2015) 17(5):690–703. doi: 10.1016/j.chom.2015.04.004
68. Voreades N, Kozil A, Weir TL. Diet and the Development of the Human Intestinal Microbiome. Front Microbiol (2014) 5:494. doi: 10.3389/fmicb.2014.00494
69. Esquivel-Elizondo S, Ilhan ZE, Garcia-Pena EI, Krajmalnik-Brown R. Insights Into Butyrate Production in a Controlled Fermentation System via Gene Predictions. mSystems (2017) 2(4):e00051–17. doi: 10.1128/mSystems.00051-17
70. Kumar H, du Toit E, Kulkarni A, Aakko J, Linderborg KM, Zhang Y, et al. Distinct Patterns in Human Milk Microbiota and Fatty Acid Profiles Across Specific Geographic Locations. Front Microbiol (2016) 7:1619. doi: 10.3389/fmicb.2016.01619
Keywords: human milk, infants, allergy, oligosaccharide, cytokines, IgA, lifestyle, farming
Citation: Seppo AE, Choudhury R, Pizzarello C, Palli R, Fridy S, Rajani PS, Stern J, Martina C, Yonemitsu C, Bode L, Bu K, Tamburini S, Piras E, Wallach DS, Allen M, Looney RJ, Clemente JC, Thakar J and Järvinen KM (2021) Traditional Farming Lifestyle in Old Older Mennonites Modulates Human Milk Composition. Front. Immunol. 12:741513. doi: 10.3389/fimmu.2021.741513
Received: 14 July 2021; Accepted: 01 September 2021;
Published: 11 October 2021.
Edited by:
Francisco José Pérez-Cano, University of Barcelona, SpainReviewed by:
Keith Stine, University of Missouri System, United StatesMarkus Xie, Genentech, United States
Copyright © 2021 Seppo, Choudhury, Pizzarello, Palli, Fridy, Rajani, Stern, Martina, Yonemitsu, Bode, Bu, Tamburini, Piras, Wallach, Allen, Looney, Clemente, Thakar and Järvinen. This is an open-access article distributed under the terms of the Creative Commons Attribution License (CC BY). The use, distribution or reproduction in other forums is permitted, provided the original author(s) and the copyright owner(s) are credited and that the original publication in this journal is cited, in accordance with accepted academic practice. No use, distribution or reproduction is permitted which does not comply with these terms.
*Correspondence: Kirsi M. Järvinen, a2lyc2lfamFydmluZW4tc2VwcG9AVVJNQy5Sb2NoZXN0ZXIuZWR1
†These authors share last authorship