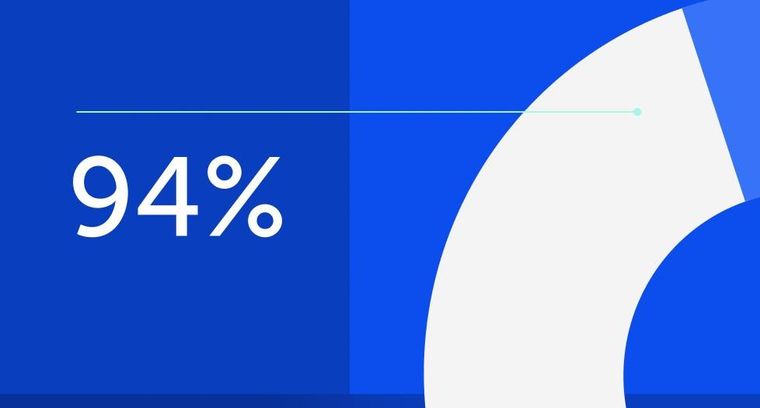
94% of researchers rate our articles as excellent or good
Learn more about the work of our research integrity team to safeguard the quality of each article we publish.
Find out more
REVIEW article
Front. Immunol., 17 September 2021
Sec. Immunological Memory
Volume 12 - 2021 | https://doi.org/10.3389/fimmu.2021.738955
This article is part of the Research TopicQuantification of Immunological MemoryView all 14 articles
There is increasing evidence that lung-resident memory T and B cells play a critical role in protecting against respiratory reinfection. With a unique transcriptional and phenotypic profile, resident memory lymphocytes are maintained in a quiescent state, constantly surveying the lung for microbial intruders. Upon reactivation with cognate antigen, these cells provide rapid effector function to enhance immunity and prevent infection. Immunization strategies designed to induce their formation, alongside novel techniques enabling their detection, have the potential to accelerate and transform vaccine development. Despite most data originating from murine studies, this review will discuss recent insights into the generation, maintenance and characterisation of pulmonary resident memory lymphocytes in the context of respiratory infection and vaccination using recent findings from human and non-human primate studies.
Respiratory tract infections remain the leading overall cause of death in developing countries, contributing to 5.4 million deaths annually (1) despite advances in vaccination uptake and technology. Recent evidence has revealed resident memory lymphocyte populations play a key role in the response to reinfection and the development of immune “memory”. Two populations of circulating memory T cells with distinct effector and migratory properties were initially described: central memory T cells (TCM) and effector memory T cells (TEM). Mechanistic studies in mice demonstrated that TEM were more prevalent in tissues, while TCM were more prevalent in lymph nodes (LN) and persisted following infection (2). TCM access and survey the LN for pathogens using the LN homing receptors C-C chemokine receptor type 7 (CCR7) and CD62-L and have a high proliferative capacity but exhibit low cytotoxicity (2–4). TEM lack or express low levels of CCR7 and CD62-L but express receptors enabling access to peripheral tissues, where upon reencounter with cognate antigen they rapidly exhibit high cytotoxicity (2–4). This concept has since been refined after it was found that TEM are largely excluded from tissue and are restricted to the spleen and intravascular compartment (4). A novel subset of memory T cells that share similarities to both TCM and TEM, termed peripheral memory T cells (TPM), have been identified as the predominant subset that re-circulate between blood and peripheral tissues (4). It is now recognised that additional subset designations exist, and memory T cells fall on a continuum, rather than rigid subsets, based on their localisation, trafficking, metabolism, longevity, and phenotypic characteristics (5).
During the last decade, a memory T cell subgroup found to reside long-term in tissues without recirculating in blood has been identified. Lacking CD62-L and CCR7, resident memory T cells (TRM) function as a first line of adaptive immune defence against subsequent re-infection and constitute the majority of T cells within the lung (5, 6). Lung-resident memory B cells (BRM) have also been recently recognised for their critical role in immunity to respiratory infection (7). Maintained in a quiescent state, BRM await secondary challenge where they accelerate secondary B cell responses.
Humans frequently develop respiratory infections throughout life and the current global coronavirus disease 2019 (COVID-19) pandemic has highlighted the need to develop and distribute effective vaccines to prevent/reduce key infectious respiratory diseases. Therefore, the development of new vaccines (e.g. COVID-19, respiratory syncytial virus, Middle East Respiratory Syndrome coronavirus) and the improvement of existing vaccines (e.g. tuberculosis, pertussis, pneumococcal and influenza) able to induce long-lasting immunity and prevent such diseases is urgently needed. The role of TRM and BRM in the control of respiratory infections has been highlighted recently in human and animal models (7, 8). Vaccination strategies that enhance either pre-existing memory T and B cells or promote the establishment of new antigen-specific TRM/BRM populations and their maintenance, alongside novel techniques for their in situ detection and functional characterisation, will be important tools for developing vaccines that provide long-lasting immunity against heterosubtypic infection. Here, we discuss the current knowledge of pulmonary TRM and BRM in human and animal models in the context of infection, highlighting knowledge gaps and opportunities in vaccine development.
Professional antigen presenting cells (APCs) including dendritic cells (DCs) are key regulators of innate and adaptive immune responses. During primary viral/bacterial respiratory infection, lung-resident DCs process and present the pathogen’s antigens and migrate to the mediastinal lymph node (MLN) to prime naïve T cells and stimulate their proliferation (Figure 1). Migratory lung DCs within the MLN imprint T cell lung homing through site-specific surface molecular signatures (15, 16) and help influence pulmonary TRM generation. In human and humanized mice, pulmonary CD1c+ and CD141+ DCs have both been shown to present viral antigens, however only CD1c+ DCs drive the expression of CD103 (a key marker of TRM – see “Phenotypic Characterisation”) on both naïve and memory CD8+ T cells (17). Multiple chemokine receptors involved in lung trafficking are expressed by TRM including C-X-C Motif Chemokine Receptor 3 (CXCR3), CXCR6 and CCR5 (11, 18–20). Although no specific combination of homing markers have been identified for pulmonary TRM, CD4+ are likely recruited to the airway during Respiratory Syncytial Virus (RSV) infection in human via C-X-C motif chemokine 10 (CXCL10 - the ligand for CXCR3), as chemokine levels correlated with activated CD4+ T cell recruitment in bronchoalveolar lavage (BAL) (18).
Figure 1 Pulmonary resident memory lymphocyte formation. 1) Inhaled respiratory pathogen (viral/bacterial) antigens are processed and presented by dendritic cells (DCs) that migrate to the mediastinal lymph node (MLN). 2) DCs prime naïve CD4+ and CD8+ T cells in MLN with cognate antigen expressed on MHC II and MHC I, respectively, resulting in T cell proliferation. B cells interact with cognate CD4+ T cells at the border between the B and T cell zones within Germinal Centres (GCs), becoming short-lived, antibody-secreting plasma cells or early memory B cells (IgM+) or enter the GC and undergo somatic hypermutation and isotype switching, with low affinity B cells differentiating into memory cells to ensure a degree of poly-reactivity. High affinity B cells differentiate into long-lived plasma cells and migrate to the bone marrow where they secrete antibodies for decades (9). 3) Stimulation within the MLN leads to the expression of chemokine receptors CXCR3, CXCR6 and CCR5 that enable T cell trafficking to the lung and airways following CXCL9/CXCL10/CXCL11/CXCL16 chemokine gradients. Pulmonary epithelial cells, DCs and macrophages secrete CCR5 and CXCR3 binding chemokines following respiratory infection (10). The CXCR6 ligand, CXCL16, is also expressed by lung bronchial epithelial cells and may also play a role in T cell homing (11). Memory B cells also migrate to the infected lung, following interferon-inducible chemokines CXCL9, CXCL10 and CXCL11 via CXCR3 (12, 13) where they are strategically located for subsequent reinfection. 4) Once entered the lung, effector T cells and short-lived plasma cells help clear infection and undergo apoptosis. A minority of effector T cells differentiate into pulmonary-resident memory T cells (TRM). IgM+ pulmonary-resident memory B cells (BRM) seed the lung early after infection, followed by isotype-switched BRM (7). 5) CD8+ TRM accumulate and self-renew in areas undergoing tissue regeneration following infection known as repair-associated memory depots (RAMD) where they seed airway CD8+ TRM, which are ideally located for pathogen clearance in the case of reinfection. 6) CD4+ TRM and BRM reside within GCs of inducible bronchus-associated lymphoid tissue (iBALT). Associated with prolonged persistence of antigens, iBALT GCs in infected lungs serve as sites for exaggerated B cell proliferation and cross-reactive clonal selection of plasma cells/memory progenitors following B cell/CD4+ TRM interactions (14).
Shortly after activation in the MLN, effector T cells migrate to the lungs and contribute towards pathogen clearance. The majority of pathogen-specific T cells then undergo apoptosis, however a minority differentiate into TRM in response to environmental cues (21), with the number of T cells persisting in the lung following infection correlating with the efficiency of TRM differentiation (22).
Effector T cells entering the lung express sphingosine-1-phosphate receptor (S1PR1), sensing increasing sphingosine-1-phosphate (S1P) gradients in blood and lymph, leading to tissue egress (10). S1PR1 expression is regulated by local cytokine-induced transcriptional downregulation and early activation marker CD69-mediated post-transcriptional antagonism (10). CD69 is a cell-surface receptor that is rapidly and transiently expressed on all recently activated T cells. Induction of the membrane‐bound type II C‐lectin receptor CD69 by antigen stimulation and inflammatory cytokine exposure leads to downregulation of S1PR1, which when combined with inflammation-induced chemotactic signalling, supports effector T cell retention and TRM generation (10, 23). Transition of recruited effector T cells to TRM in murine lung requires simultaneous tissue damage and T cell receptor (TCR) activation by pulmonary cognate antigen encounter (24–27). Overlapping TCR genes from human TRM and non-TRM indicate that environment, rather than epitope specificity, drives TRM formation (19). Antigen-dependent cross-competition however does promote TRM formation, with effector T cells recognising antigen presented by infected tissue cells preferentially entering the local TRM pool (28). Although demonstrated in murine skin, it is possible the same rules also apply to the lung. Naïve T cells in LNs may also be epigenetically preconditioned during steady state conditions by migratory DCs to differentiate into TRM upon exposure to cognate antigen (29). Dependent on DC-driven, transforming growth factor β (TGF-β), altering local or systemic TGF-β activity prior to vaccination may help promote TRM formation (29).
Once established, TRM remain lung-resident and contribute towards immunosurveillance and homeostasis (6). Maintained in a quiescent state, human transplant studies have demonstrated donor CD4+ and CD8+ TRM to persist in the lungs for over 15 months, with single cell transcriptome analysis confirming de novo TRM generation via the identification of a “mature TRM” and an immature “TRM-like” population that gradually acquire TRM markers (CD69, CD103 and CD49a) over time (30, 31). Pulmonary CD8+ TRM are however more short-lived than those found in other tissues such as the skin and intestine (32, 33). As microbes are constantly being inhaled, the limited longevity of pulmonary CD8+ TRM may provide a mechanism for avoiding unnecessary inflammation and pathogenesis in this tissue (34).
In human, Notch signalling alongside low levels of T-bet and Eomesodermin (EOMES) are required for the development and maintenance of CD4+/CD8+ TRM, with Notch regulating TRM metabolic programs (11, 20). Human pulmonary CD8+ TRM display elevated levels of the transcription factors Hobit (encoded by the gene ZNF683) and Runx3, that may be involved in TRM generation and/or maintenance (30). Interestingly, despite showing elevated mRNA levels, Hobit protein expression was reported absent in human CD4+ TRM, suggesting differences between CD4+/CD8+ TRM formation/maintenance (11).
Heterogeneity in effector function and phenotype is evident within TRM populations, particularly within CD4+ TRM (19). Transcriptome profiling of human lung CD69+ TRM has revealed the differential expression of 31 core genes associated with migration, adhesion and regulatory molecules when compared to CD69- subsets (19). This transcriptional profile is conserved across CD4+/CD8+ CD69+ lineages as well as tissues (19). Pulmonary TRM exhibit high transcript levels for genes encoding for several chemokine receptors, pro-inflammatory cytokines and cytotoxic mediators, enabling them to be recruited and retained within the lung and undergo rapid, polyfunctional responses (11, 20). TRM respond rapidly with effector functions, however, expression of regulatory genes (e.g. cytotoxic T-lymphocyte-associated protein 4 [CTLA4] and B-and T-lymphocyte attenuator 4 [BTLA4]) in CD8+ TRM may present a safety mechanism to minimise aberrant activation and associated inflammation/tissue damage (20).
Human antigen-experienced lungs are enriched with B cells containing a resident memory phenotype (35). As human and non-human primate (NHP) BRM data are limited, most findings are derived from mouse studies. During primary respiratory infection, naïve B cells, primed by either free antigen or antigen delivered by subcapsular sinus (SCS) macrophages (36), interact with cognate CD4+ T cells at the T-B border within the MLN (9, 37). Following initial proliferation at the outer follicles, B cells may differentiate into extrafollicular short-lived plasma cells, early (germinal centre (GC)-independent) memory cells or proliferate to form the GC (Figure 1). Following somatic hypermutation, B cells can exit as long-lived plasma cells, migrating to the bone marrow where they secrete antibodies for decades, or memory B cells (9, 37). Having migrated to the lungs to participate in pathogen clearance, most of the responding B cells undergo apoptosis, leaving a few resting memory cells in the respiratory tract and lymphoid organs where they wait for the same antigen.
Murine parabiosis studies have demonstrated BRM generation requires local antigen encounter and is dependent on early CD40-interactions with T cells (7). Once established, BRM remain lung resident due to expression of CD69 (7). Here they undergo metabolic reprogramming, switching from anabolic to catabolic pathways to reduce their requirement for high levels of cytokines for their maintenance (37). In mice, BRM are quiescent and long-lived, maintained from precursors within persisting GCs in areas known as inducible bronchus-associated lymphoid tissue (iBALT) (14), however BRM have also been detected in the absence of iBALT (39). Established one week after influenza infection, murine pulmonary BRM have been demonstrated to be phenotypically and functionally distinct from their systemic counterparts (7).
Few studies have investigated gene regulation in pulmonary BRM, particularly in humans. Although the possibility of a “master transcription factor” for BRM generation has been suggested, no unique transcription factor has been identified so far (9). Increased expression of the transcription factors Bach2, KLF2, ZBTB32, ABF1 and STAT5 are associated with BRM formation in mice, however their exact roles are yet to be understood (9, 40). The transcriptional regulation of pulmonary BRM differentiation is likely to be unique – understanding these transcription factors may help identify methods for modulating their formation (41).
Due to their similarities to human, NHPs provide an invaluable tool for investigating host response to respiratory infection and vaccination. Although heterogenous within the lung, human and NHP TRM are phenotypically distinct from TCM and TEM and are primarily identified by the high expression of the C-type lectin receptor CD69, and integrins CD103 and CD49a (30). The transmembrane CD69 is a key marker of pulmonary TRM, distinguishing memory T cells in tissue from those in circulation (19), however murine evidence suggests its expression is not essential for the establishment and maintenance of TRM in the lung (25, 42, 43). Although considered as an early activation marker for TCR signalling, TRM CD69 expression is not associated with markers of recent activation and appears to be a function of previous antigen exposure (19).
Preferentially expressed on CD8+ TRM compared to CD4+, CD103 promotes adherence to E-cadherin, an adhesion molecule expressed by epithelial cells (22, 30, 44). CD103 expression is driven by membrane-bound TGF-β (mediated by IL-10) on APCs (CD1c+ DCs and monocytes) (17, 45) and is thought to contribute towards initial recruitment and persistence of CD8+ TRM to aide surveillance rather than long-term maintenance (42). CD49a, expressed by both CD4+ and CD8+ TRM, is an integrin specific to collagen IV that facilitates locomotion for surveillance and is essential for TRM survival by limiting apoptosis following ligand engagement (42). Other recognised surface markers of pulmonary TRM are outlined in Table 1 - understanding the full function of these markers, whether they represent different subsets/maturation states and whether they are pathogen-dependent remains to be determined.
Although no specific marker of BRM residency has been described, pulmonary BRM are phenotypically distinct from their systemic memory and non-memory counterparts (7) – see Table 2. As well as lacking CD62-L, murine pulmonary BRM express markers associated with TRM, such as CD69, CXCR3 and CD44, which retain BRM within the lung (7, 12, 35, 39). CD69 has also been found on human pulmonary BRM (35). Whether other markers found in mice are also expressed on human and NHP pulmonary BRM requires further investigation.
Functional studies have revealed BRM established early after murine influenza infection are positive for immunoglobulin M (IgM+) which are later followed by isotype-switched BRM (7). Following murine pneumococcal infection, the majority of isotype-switched BRM are IgG+, with a small fraction IgA+ (35). The majority of BRM found in healthy human lung are also isotype-switched (35).
TRM/BRM persist at sites of previous antigen encounter (13). CD8+CD103+ TRM are found at higher frequencies in the airway than in parenchyma due to adhesion to epithelial E-cadherin, making them ideally located to respond to reinfection (30). Murine CD8+ TRM reside and self-renew in peribronchiolar foci in areas undergoing tissue remodelling, known as repair-associated memory depots (RAMDs) (25, 38) (Figure 2). Tissue damage is a requirement for RAMDs (25) and may have implications for vaccine design and delivery. The existence of human RAMDs containing CD8+ TRM remains to be confirmed.
Figure 2 Compartmentalisation of Pulmonary TRM and BRM. 1) CD8+ TRM are maintained in repair-associated memory depots (RAMDs) located in peribronchiolar foci in areas previously damaged from primary infection. RAMDs can be identified via the presence of cytokeratin-expressing cell aggregates which contain distal airway stem cells that help reconstruct damaged lung tissue (10). Murine evidence suggests interstitial CD8+ TRM are primarily maintained by a process of homeostatic proliferation and seed airway TRM, driven by CXCR6 in response to airway CXCL16 (38). 2) CD4+ TRM surround BRM cell follicles in iBALT located within the pulmonary parenchyma, where prolonged antigen persistence enhances CD4+ TRM/BRM formation. Just like RAMDs, iBALT requires tissue damage/inflammation for their establishment. CD4+ TRM are then recruited to the alveolar space via CXCL10/CXCR3.
CD4+ TRM and BRM are located and maintained around the small airways within iBALT located within the parenchyma (13, 14, 25, 30) (Figure 2). iBALT contain GCs that serve as sites for B cell selection and maturation following murine influenza infection (50), generating cross-reactive memory B cells to ensure heterosubtypic humoral protection (14). Formation is associated with inflammation (44) and prolonged antigen persistence (14). BRM have also been observed in non-lymphoid areas below the airway epithelium and airways (13) as well as lungs of pneumococcal-recovered mice lacking iBALT, indicating that they are components of histologically unremarkable lungs that may not require iBALT for their maintenance (35, 39).
Growing evidence indicates that virus-specific T cells resident along the respiratory tract are highly effective at providing potent and rapid protection against inhaled pathogens. In human influenza infection, CD8+ TRM have been shown to recognise the internal, conserved proteins of the virus whereas CD4+ TRM recognise both internal and external proteins, with both cell types contributing towards heterosubtypic protection (33). CD8+ TRM have been shown to be cross-reactive against three influenza strains (51), with single cell sequencing revealing diverse TCR profiles “capable of recognising newly emerging viral escape variants” (22).
Influenza-specific CD8+ TRM have a low activation requirement, requiring only cognate antigen in the absence of helper cell-derived signals (52). Once stimulated, they are highly proliferative, producing polyfunctional progeny (producing ≥2 cytokines – IFN-γ, TNF, Granzyme B and IL-2) with effector function superior even to their parent population (22, 44). Polyfunctional TRM offer enhanced protection by producing higher levels of cytokines whilst simultaneously driving effector responses (53) - activated CD8+ TRM exert their cytotoxic function to kill infected cells (10) whilst CD4+ TRM interact with B cells in iBALT to generate new neutralising antibodies (14, 54). A newly identified, long-lived CD4+ T resident helper (TRH) population with functional and phenotypical similarities to lymphoid T follicular helper cells (TFH) has also been described following murine influenza infection. Residing within iBALT, TRH are tightly localised with BRM to support local antibody production following reinfection (55).
In an experimental human RSV infection model, the abundance of RSV-specific, pulmonary CD8+ TRM before infection was associated with reduced symptoms and viral load, implying that CD8+ TRM can confer protection against severe respiratory viral disease when humoral immunity is overcome (8). RSV-specific CD8+ TRM displayed phenotypic changes representative of advanced differentiation, with downregulation of both co-stimulatory and cytotoxicity markers, suggesting cells can respond rapidly to reinfection, but function is restricted to minimise excessive tissue damage (8).
RSV infection in African Green Monkeys (AGM) also induced virus-specific airway CD8+ TRM capable of reducing viral titres, however failed to induce robust CD4+ TRM and humoral responses (21). Previously protective RSV-candidate vaccines in AGM induced a strong T cell response, whilst those eliciting a strong neutralisation antibody response without detectable T cell response were not as effective (56). Similar to influenza, CD8+ TRM recognise internal proteins of RSV, whilst CD4+ TRM recognise external proteins (18). RSV-induced immunopathology relates to a dysregulated T cell response – RSV-specific memory CD8+ T cells in blood display little evidence of multiple cytokine production unlike those seen against influenza (8, 18). CD8+ TRM however appear to be more polyfunctional, generating IFN-γ, IL-2 and TNF (21), however fail to undergo proliferation when activated and express reduced cytotoxicity markers compared to peripheral memory cells (8).
Activated CD4+ and CD8+ TRM have been identified in the lungs of patients infected with Mycobacterium tuberculosis (Mtb), where they help limit intracellular macrophage Mtb replication (46). TRM were polyfunctional, expressing IFN-γ, TNF ± IL-2, and exhibited a highly cytotoxic profile, with CD4+ TRM appearing more polyfunctional than CD8+ TRM (46). CD49d is upregulated on airway CD4+ TRM and optimises the localisation of human Mtb-specific recall responses (47). Mtb infection in macaques drives a cellular T helper 1 (TH1) and humoral response, without protective efficacy, however repeated pulmonary Bacillus Calmette-Guérin (BCG) delivery was shown to induce polyfunctional, TH17 CD4+ TRM, leading to airway IgA secretions in BAL (48), presumably through the generation of BRM. Interstitial CD4+ depletion with simian immunodeficiency virus (SIV) following Mtb infection identified CD4+ TRM (57), proliferating CD8+ memory T cells (TCM, TEM and likely TRM) and B cells within iBALT (58) as critical for suppressing latent Mtb reactivation.
TH17 CD4+ TRM are also critical in protecting against murine nasal Bordetella pertussis (Bp) colonization (59). Although both capable of protecting against Bp lung infection, whole cell Bp vaccine, unlike the acellular vaccine, induced nasal IL-17-producing CD103+ CD4+ TRM (similar to natural Bp infection) that recruited neutrophils to enhance bacterial clearance.
Lung TRM also display “innate-like” behaviour, amplifying inflammation following noncognate bacterial infection. APC-derived IL-12/IL-18 activated virus-specific CD8+ TRM within the lung parenchyma, leading to the rapid synthesis of IFN-γ. This “bystander activation” boosted neutrophil recruitment to improve bacterial clearance. Despite being performed in mice, the authors demonstrated in vitro that human CD8+ TRM similarly synthesise IFN-γ in response to IL-12/IL-18 (60).
Alongside long-lived antibody-secreting plasma cells, BRM contribute towards the protective humoral immune response to pulmonary reinfection (12). The presence of BRM is a common feature of antigen‐experienced lungs and is important for acquired immunity (7). B cells in the airways secrete antibodies that act both locally and at mucosal surfaces. These antibodies, predominantly IgM and IgA, bind to glandular epithelial and mucosal surfaces to promote pathogen clearance (61). B cells activated in respiratory lymphoid tissue also differentiate into IgA‐secreting plasma cells that predominantly act in the airway. Current knowledge of B cell homing and class switching in the airway remains limited.
Murine parabiosis/adoptive transfer/depletion studies have demonstrated the protective role played by BRM in response to both viral (7, 12, 54) and bacterial lung infection (35). BRM provide rapid antibody-secreting cells (ASC), producing a range of class switched neutralising antibodies (7, 12). Lung BRM produce greater numbers of ASC than splenic memory cells following exposure to drifted virus, indicative of heterosubtypic protection (14). Cross-neutralising antibodies to conserved, internal influenza proteins provide heterosubtypic protection (14, 54). Although IgA is more effective than IgG at preventing upper respiratory infection, in combination they achieve maximal neutralising activity against influenza in mice (12). Following murine pneumococcal infection, BRM contribute towards bacterial clearance by rapidly secreting cross-reactive antibodies, even when reactivated by a serotype-mismatched strain (35). In macaques, iBALT persistence is associated with reduced Mtb reactivation due to enhanced B-cell and humoral immunity (58). BRM are also potent APC, binding and endocytosing antigen via their BCR to increase peptide/MHC II presentation and further enhance CD4+/B cell responses (9, 13).
Pulmonary immunity to respiratory pathogens wanes over time, meaning individuals are susceptible to recurrent infections throughout their lifetime. Although antigen drift may contribute to loss of protection, the gradual loss of pulmonary CD8+ TRM is a major contributor (32). Murine lung CD8+ TRM are less durable than those found in skin due to an increased susceptibility to apoptosis (32), and have been shown to undergo “retrograde migration” to the MLN where they provide longer-lived regional memory (62). Loss of RAMDs due to tissue repair correlated with a decline in CD8+ TRM number in mice (10, 25), whilst in humans iBALT diminishes with age (6) which may explain why older age groups are more susceptible to respiratory infection due to a reduced ability to mount CD4+ TRM/BRM responses.
Although TRM-driven immunopathology has been described in other tissues (63), less is known regarding pulmonary TRM. Moderate-severe asthma patients display increased numbers of CD4+CD103+ TRM in their airways (64) and vaccine-enhanced disease in children with formalin-inactivated RSV is driven by TH2 CD4+ memory cells that induce excessive inflammation (65). Exacerbations of pulmonary pathology following RSV infection have also been linked to iBALT which stimulate increased, yet detrimental, immune responses (66). CD8+ TRM may impact gas exchange via the presence of RAMD or through inflammation induced by bystander activation (60). In vitro, CD8+ T cells damage non-infected epithelial cells during influenza infection through TNF and IFN-γ release (67). Although the detrimental effect due to TRM/BRM has not been demonstrated in vivo, TRM/BRM formation may not always be beneficial if accompanied by another immune cell influx such as that found following acute infections.
The presence of pathogen-specific TRM cells in the lungs has been shown to correlate with protection in human and animal models. It has therefore been proposed that TRM represent one of several immune mechanisms that should be harnessed together for optimal vaccine-mediated protection. A better understanding of how lung TRM are generated and maintained is required for optimal vaccine development. Vaccination strategies to promote TRM have been successfully demonstrated in mouse models, including engineered biomaterials that modulate antigen delivery and retention time, adjuvant combinations, viral vectors and virus-like particles, as well as direct APC targeting (68), however studies in human and NHP are limited.
In mice and human, inactivated influenza vaccines induce systemic humoral responses but fail to induce T cell immunity in the lungs (33, 69, 70). Intranasal live-attenuated influenza virus vaccines however generate mucosal IgA, lung CD4+ TRM and virus-specific CD8+ TRM similar in phenotype to those generated by influenza virus infection, providing long term, heterosubtypic protection, independent of circulating T cells and neutralising antibodies (70, 71). Tissue-resident alveolar macrophages have been found to limit CD8+ TRM formation following murine influenza infection and may offer an attractive target for manipulation (72).
Intravascular, but not subcutaneous, administration of an agonistic anti-CD40 antibody alongside poly-IC : LC (a Toll-like receptor 3 activator) with HIV envelope peptide antigen directly stimulated APCs in the blood, MLN and lung to enhance pulmonary CD8+CD103+ TRM formation in macaques (45). Intravenous BCG in macaques induces more antigen responsive pulmonary CD4+ and CD8+ TRM than intradermal administration, with protection lasting 6 months later (73). Intratracheal boosting with BCG however following intradermal BCG vaccination enhances protection (74). Although this study only analysed peripheral blood to correlate increased CD4+ TEM populations with improved protection, it is anticipated that local delivery of antigen to the lungs would also increase TRM/BRM populations. Pulmonary mucosal BCG vaccination therefore offers superior protection against Mtb compared to standard intradermal vaccination (48, 75, 76).
VPM1002, a live BCG vaccine genetically modified to improve immunogenicity, outperforms live-attenuated BCG in preclinical testing and is undergoing clinical trials (NCT03152903) (77). Aerosol immunization with a mutated Mtb strain MtbΔsigH reduced bacterial burden and lung pathology when compared to aerosolised BCG following Mtb challenge in macaques (78). MtbΔsigH persisted for longer in the lungs than BCG and generated increased iBALT and CD69+ T cells in BAL, which likely include TRM. Since antigen is required for TRM/BRM establishment, increasing its persistence enhances generation. Increasing antigen persistence using a cytomegalovirus vector encoding Mtb antigen inserts prevented disease in macaques through the establishment and maintenance of lung TRM (79).
It is also possible that skin-resident TRM generated through intradermal vaccination may enhance both local and systemic host responses to Staphylococcus aureus, a common commensal of the skin and nasal mucosa, to help minimise Staphylococcal pneumonia (80).
Strategies to induce pulmonary BRM require delivery of antigen to the lung (7). In mice, intranasal vaccination extended antibody specificity to confer heterosubtypic protection by inducing GCs that generated cross-reactive antibody responses (14). In human, the squalene emulsion adjuvants AS03 or MF59 augmented neutralising antibody production when co-administered intramuscularly with influenza vaccine (81). Both adjuvants enhanced antigen uptake and presentation in local tissue leading to increased CD4+ and B cell responses, with AS03 also shown to increase naïve B cell activation and the adaptability of pre-existing memory B cells (82). Despite increasing the breadth of B cell repertoire following seasonal Flu vaccine (83), the impact of adjuvants on pulmonary TRM/BRM remains to be demonstrated for such intramuscular vaccine. We could not exclude that a boost from an adjuvanted vaccine in humans previously exposed to a similar antigen encountered in the lung could re-activate and maintain pulmonary TRM/BRM.
In contrast, certain respiratory viruses such as RSV are known to trigger a TH2-like, dysregulated antiviral response (84). Acute RSV infection limits pulmonary BRM formation (85) and encodes a number of immunomodulatory proteins that impair antigen presentation and type 1 interferon release (18), which may explain why infection is associated with a low level antibody response (21). Similarly, COVID-19 also suppresses MHC I/MHC II antigen presentation and interferon response (86). These issues of dysregulated T cell responses should be avoided or overcome through vaccination, leading to long-term humoral protection.
Most vaccine studies in humans rely on peripheral blood sampling to evaluate protection. Serum haemagglutination inhibition (HAI), ELISA or ELISpot may indicate the humoral response generated against a given pathogen/vaccine, however does not always reflect immunity, as protection against influenza has been seen despite the absence of HAI titres (87). Nasal IgA is also a better reflector of protection to RSV than serum IgG (85). Circulating memory T and B cells do not always correlate with protection (8, 85) and immune responses can differ from those in lung (44).
Limited peripherally accessible biomarkers have been identified following immunization relating to resident memory lymphocyte generation. Early rises in plasma IL-10 correlated with pulmonary CD8+CD103+ TRM generation following immunization in macaques (45). The CXCL10/CXCR3 axis has also been postulated as a potential biomarker for CD4+ migration to the lung (18). Further immunization studies correlating peripheral biomarkers with TRM/BRM formation are required.
Airway TRM can be isolated via BAL (30). Virus-specific CD8+ frequencies have been found to be 10 times higher in BAL than in peripheral blood in AGM, highlighting the quantitative differences between local and systemic T cell responses (21). BAL can be collected multiple times, providing temporal information on airway populations, but not interstitial. Post-mortem analysis is often the only method for assessing TEM/TRM. Tissue sections can be collected for histology or enzymatic tissue digestion, however accessing human/NHP tissue is difficult. Lung tissue is easily contaminated with alveolar/intravascular cells unless the organ is perfused and BAL collected (however this is not 100% effective). Intravenous antibody staining can distinguish tissue resident from circulatory cells, however, is not performed in humans/NHP. TRM/BRM are identified through surface marker expression or gene signature, with pathogen-specificity evaluated through intracellular cytokine staining following exposure to antigen/MHC tetramers (TRM) or binding of labelled-antigen (BRM). Given the limited information gained on pulmonary TRM/BRM populations using current sampling methods, new detection techniques are required.
Optical endomicroscopy imaging (88), recently used for the detection of human alveolar neutrophils in situ (89), may provide a valuable tool for assessing pulmonary-resident memory lymphocytes and quantifying immunological memory following vaccination. Fluorescently tagged ligands or antibodies, capable of binding to specific TRM/BRM surface markers, can be delivered to the airways via a bronchoscope to enable visualisation (Figure 3). The information gained can be combined with systemic data to evaluate vaccine efficacy and expected degree of protection against respiratory pathogens. In situ optical imaging may also be used to screen lungs for transplantation, as the presence of TRM in donor tissue is associated with reduced adverse clinical events in the recipient (30).
Figure 3 In Situ Optical Imaging of Resident Memory Lymphocytes. Optical endomicroscopy imaging within the lungs may allow for the in situ detection and quantification of resident memory lymphocyte populations. Monitoring numbers following immunization may help reflect vaccine efficacy and immunological memory. Fluorescently tagged ligands or antibodies, capable of binding to specific TRM/BRM surface markers, can be delivered to the airways via a bronchoscope to enable visualisation. Using a combination of fluorescent ligands/antibodies could help differentiate resident memory lymphocyte populations.
The COVID-19 pandemic has highlighted how immune responses in the airways differ from those in the circulation and that it is tissues, not blood, where immune cells function (90). Assessing tissue-based immunity following infection and vaccination is therefore essential. Ex vivo lung perfusion (EVLP), using human lungs deemed non-suitable for transplantation, provides an ideal model for assessing tissue immunity and optimising in situ optical imaging. As well as studying populations in situ, EVLP offers the ability to isolate large numbers of human TRM/BRM, far higher than those obtained from a typical BAL, for in-depth analysis (including phenotype, function, and antigen-specificity). Intraperfusate delivery of a fluorescently tagged CD45 antibody can also differentiate circulating (labelled) from tissue-resident (non-labelled) cells. This technique has recently revealed how human lung TRM colocalise with lung-resident macrophages, preferentially around the airways, where they receive costimulatory signals to augment effector cytokine production and degranulation (91).
Resident memory lymphocytes in the lung enhance immunity against respiratory pathogens. Understanding the mechanisms that drive TRM and BRM formation will improve vaccine design, with the hope of generating long lived, polyfunctional TRM and broadly reactive, neutralising-antibody-secreting BRM in the lung. Targeting respiratory APCs with antigen followed by subsequent “boosts” may establish and maintain these populations. Assessing the local and systemic responses using a combination of in situ imaging and peripheral blood sampling may reveal the efficacy of novel vaccines designed specifically to induce resident memory lymphocyte populations in the lung. Human ex vivo lung perfusion provides an ideal model for researching TRM/BRM populations and optimising novel methods for their in situ detection to help quantify immunological memory.
DH, KD, MC-R, and VP contributed to conception and design of the manuscript. DH wrote the first draft of the manuscript. DH, RO’C, DL, MC-R, and VP wrote sections of the manuscript. All authors contributed to the article and approved the submitted version.
This work was funded by Sanofi Pasteur.
KD is a founder and shareholder of Edinburgh Molecular Imaging. DH, DL, MC-R and VP were employed by Sanofi Pasteur.
The authors declare that this study received funding from Sanofi Pasteur. The funder had the following involvement in the study: study design, preparation of the manuscript and decision to publish.
The remaining author declares that the research was conducted in the absence of any commercial or financial relationships that could be construed as a potential conflict of interest.
All claims expressed in this article are solely those of the authors and do not necessarily represent those of their affiliated organizations, or those of the publisher, the editors and the reviewers. Any product that may be evaluated in this article, or claim that may be made by its manufacturer, is not guaranteed or endorsed by the publisher.
All figures created with BioRender.com.
1. Ferkol T, Schraufnagel D. The Global Burden of Respiratory Disease. Ann Am Thorac Soc (2014) 11:404–6. doi: 10.1513/AnnalsATS.201311-405PS
2. Wherry EJ, Teichgräber V, Becker TC, Masopust D, Kaech SM, Antia R, et al. Lineage Relationship and Protective Immunity of Memory CD8 T Cell Subsets. Nat Immunol (2003) 4:225–34. doi: 10.1038/ni889
3. Masopust D, Soerens AG. Tissue-Resident T Cells and Other Resident Leukocytes. Annu Rev Immunol (2019) 37:521–46. doi: 10.1146/annurev-immunol-042617-053214
4. Gerlach C, Moseman EA, Loughhead SM, Alvarez D, Zwijnenburg AJ, Waanders L, et al. The Chemokine Receptor CX3CR1 Defines Three Antigen-Experienced CD8 T Cell Subsets With Distinct Roles in Immune Surveillance and Homeostasis. Immunity (2016) 45:1270–84. doi: 10.1016/j.immuni.2016.10.018
5. Jameson SC, Masopust D. Understanding Subset Diversity in T Cell Memory. Immunity (2018) 48:214–26. doi: 10.1016/j.immuni.2018.02.010
6. Snyder ME, Farber DL. Human Lung Tissue Resident Memory T Cells in Health and Disease. Curr Opin Immunol (2019) 59:101–8. doi: 10.1016/j.coi.2019.05.011
7. Allie SR, Bradley JE, Mudunuru U, Schultz MD, Graf BA, Lund FE, et al. The Establishment of Resident Memory B Cells in the Lung Requires Local Antigen Encounter. Nat Immunol (2019) 20:97–108. doi: 10.1038/s41590-018-0260-6
8. Jozwik A, Habibi MS, Paras A, Zhu J, Guvenel A, Dhariwal J, et al. RSV-Specific Airway Resident Memory CD8+ T Cells and Differential Disease Severity After Experimental Human Infection. Nat Commun (2015) 6:1–15. doi: 10.1038/ncomms10224
9. Palm AKE, Henry C. Remembrance of Things Past: Long-Term B Cell Memory After Infection and Vaccination. Front Immunol (2019) 10:1787. doi: 10.3389/fimmu.2019.01787
10. Takamura S. Persistence in Temporary Lung Niches: A Survival Strategy of Lung-Resident Memory CD8+ T Cells. Viral Immunol (2017) 30:438–50. doi: 10.1089/vim.2017.0016
11. Oja AE, Piet B, Helbig C, Stark R, van der Zwan D, Blaauwgeers H, et al. Trigger-Happy Resident Memory CD4 + T Cells Inhabit the Human Lungs. Mucosal Immunol (2018) 11:654–67. doi: 10.1038/mi.2017.94
12. Onodera T, Takahashi Y, Yokoi Y, Ato M, Kodama Y, Hachimura S, et al. Memory B Cells in the Lung Participate in Protective Humoral Immune Responses to Pulmonary Influenza Virus Reinfection. Proc Natl Acad Sci USA (2012) 109:2485–90. doi: 10.1073/pnas.1115369109
13. Allie SR, Randall TD. Resident Memory B Cells. Viral Immunol (2020) 44:282–93. doi: 10.1089/vim.2019.0141
14. Adachi Y, Onodera T, Yamada Y, Daio R, Tsuiji M, Inoue T, et al. Distinct Germinal Center Selection at Local Sites Shapes Memory B Cell Response to Viral Escape. J Exp Med (2015) 212:1709–23. doi: 10.1084/jem.20142284
15. Mikhak Z, Strassner JP, Luster AD. Lung Dendritic Cells Imprint T Cell Lung Homing and Promote Lung Immunity Through the Chemokine Receptor CCR4. J Exp Med (2013) 210:1855–69. doi: 10.1084/jem.20130091
16. Pejoski D, Ballester M, Auderset F, Vono M, Christensen D, Andersen P, et al. Site-Specific DC Surface Signatures Influence CD4+ T Cell Co-Stimulation and Lung-Homing. Front Immunol (2019) 10:1650. doi: 10.3389/fimmu.2019.01650
17. Yu CI, Becker C, Wang Y, Marches F, Helft J, Leboeuf M, et al. Human CD1c+ Dendritic Cells Drive the Differentiation of CD103+ CD8+ Mucosal Effector T Cells. Via Cytokine TGF-β Immun (2013) 38:818–30. doi: 10.1016/j.immuni.2013.03.004
18. Guvenel A, Jozwik A, Ascough S, Ung SK, Paterson S, Kalyan M, et al. Epitope-Specific Airway-Resident CD4+ T Cell Dynamics During Experimental Human RSV Infection. J Clin Invest (2020) 130:523–38. doi: 10.1172/JCI131696
19. Kumar BV, Ma W, Miron M, Granot T, Guyer RS, Carpenter DJ, et al. Human Tissue-Resident Memory T Cells Are Defined by Core Transcriptional and Functional Signatures in Lymphoid and Mucosal Sites. Cell Rep (2017) 20:2921–34. doi: 10.1016/j.celrep.2017.08.078
20. Hombrink P, Helbig C, Backer RA, Piet B, Oja AE, Stark R, et al. Programs for the Persistence, Vigilance and Control of Human CD8 + Lung-Resident Memory T Cells. Nat Immunol (2016) 17:1467–78. doi: 10.1038/ni.3589
21. Li H, Callahan C, Citron M, Wen Z, Touch S, Monslow MA, et al. Respiratory Syncytial Virus Elicits Enriched CD8+ T Lymphocyte Responses in Lung Compared With Blood in African Green Monkeys. PloS One (2017) 12:1–19. doi: 10.1371/journal.pone.0187642
22. Pizzolla A, Nguyen THO, Sant S, Jaffar J, Loudovaris T, Mannering SI, et al. Influenza-Specific Lung-Resident Memory T Cells are Proliferative and Polyfunctional and Maintain Diverse TCR Profiles. J Clin Invest (2018) 128:721–33. doi: 10.1172/JCI96957
23. Mackay LK, Braun A, Macleod BL, Collins N, Tebartz C, Bedoui S, et al. Cutting Edge: CD69 Interference With Sphingosine-1-Phosphate Receptor Function Regulates Peripheral T Cell Retention. J Immunol (2015) 194:2059–63. doi: 10.4049/jimmunol.1402256
24. Ogongo P, Zachary Porterfield J, Leslie A. Lung Tissue Resident Memory T-Cells in the Immune Response to Mycobacterium Tuberculosis. Front Immunol (2019) 10:992. doi: 10.3389/fimmu.2019.00992
25. Takamura S, Yagi H, Hakata Y, Motozono C, McMaster SR, Masumoto T, et al. Specific Niches for Lung-Resident Memory CD8+ T Cells at the Site of Tissue Regeneration Enable CD69-Independent Maintenance. J Exp Med (2016) 213:3057–73. doi: 10.1084/jem.20160938
26. McMaster SR, Wein AN, Dunbar PR, Hayward SL, Cartwright EK, Denning TL, et al. Pulmonary Antigen Encounter Regulates the Establishment of Tissue-Resident CD8 Memory T Cells in the Lung Airways and Parenchyma Article. Mucosal Immunol (2018) 11:1071–8. doi: 10.1038/s41385-018-0003-x
27. McKinstry KK, Strutt TM, Bautista B, Zhang W, Kuang Y, Cooper AM, et al. Effector CD4 T-Cell Transition to Memory Requires Late Cognate Interactions That Induce Autocrine IL-2. Nat Commun (2014) 5:5377. doi: 10.1038/ncomms6377
28. Muschaweckh A, Buchholz VR, Fellenzer A, Hessel C, König P-A, Tao S, et al. Antigen-Dependent Competition Shapes the Local Repertoire of Tissue-Resident Memory CD8+ T Cells. J Exp Med (2016) 213:3075–86. doi: 10.1084/jem.20160888
29. Mani V, Bromley SK, Äijö T, Mora-Buch R, Carrizosa E, Warner RD, et al. Migratory DCs Activate TGF-β to Precondition Naïve CD8(+) T Cells for Tissue-Resident Memory Fate. Science (80-) (2019) 366(6462):eaav5728. doi: 10.1126/science.aav5728
30. Snyder ME, Finlayson MO, Connors TJ, Dogra P, Senda T, Bush E, et al. Generation and Persistence of Human Tissue-Resident Memory T Cells in Lung Transplantation. Sci Immunol (2019) 4:eaav5581. doi: 10.1126/sciimmunol.aav5581
31. Mami-Chouaib F, Tartour E. Editorial: Tissue Resident Memory T Cells. Front Immunol (2019) 10:1018. doi: 10.3389/fimmu.2019.01018
32. Slutter B, Van Braeckel-Budimir N, Abboud G, Varga SM, Salek-Ardakani S, Harty JT. Dynamic Equilibrium of Lung Trm Dictates Waning Immunity After Influenza A Infection. Sci Immunol (2017) 2:3374–82. doi: 10.1126/sciimmunol.aag2031.Dynamic
33. Pizzolla A, Wakim LM. Memory T Cell Dynamics in the Lung During Influenza Virus Infection. J Immunol (2019) 202:374–81. doi: 10.4049/jimmunol.1800979
34. Reagin KL, Klonowski KD. Incomplete Memories: The Natural Suppression of Tissue-Resident Memory CD8 T Cells in the Lung. Front Immunol (2018) 9:17. doi: 10.3389/fimmu.2018.00017
35. Barker KA, Etesami NS, Shenoy AT, Arafa EI, Lyon de Ana C, Smith NM, et al. Lung-Resident Memory B Cells Protect Against Bacterial Pneumonia. J Clin Invest (2021) 131(11):e141810. doi: 10.1172/JCI141810
36. Carrasco YR. Batista FD. B Cells Acquire Particulate Antigen in a Macrophage-Rich Area at the Boundary Between the Follicle and the Subcapsular Sinus of the Lymph Node. Immunity (2007) 27:160–71. doi: 10.1016/j.immuni.2007.06.007
37. Kurosaki T, Kometani K, Ise W. Memory B Cells. Nat Rev Immunol (2015) 15:149–59. doi: 10.1038/nri3802
38. Takamura S, Kato S, Motozono C, Shimaoka T, Ueha S, Matsuo K, et al. Interstitial-Resident Memory CD8+ T Cells Sustain Frontline Epithelial Memory in the Lung. J Exp Med (2019) 216:2736–47. doi: 10.1084/jem.20190557
39. Barker KA, Smith NM, Shenoy AT, Martin IMC, Jones MR, Quinton LJ, et al. Repeated Respiratory Bacterial Exposures Elicit Lung Resident Memory B Cells in the Absence of Organized Tertiary Lymphoid Tissue. J Immunol (2019) 202:66.22.
40. Song S, Matthias PD. The Transcriptional Regulation of Germinal Center Formation. Front Immunol (2018) 9:2026. doi: 10.3389/fimmu.2018.02026
41. Laidlaw BJ, Cyster JG. Transcriptional Regulation of Memory B Cell Differentiation. Nat Rev Immunol (2021) 21:209–20. doi: 10.1038/s41577-020-00446-2
42. Reilly EC, Emo KL, Buckley PM, Reilly NS, Smith I, Chaves FA, et al. TRM Integrins CD103 and CD49a Differentially Support Adherence and Motility After Resolution of Influenza Virus Infection. Proc Natl Acad Sci USA (2020) 117:12306–14. doi: 10.1073/pnas.1915681117
43. Walsh DA, Borges da Silva H, Beura LK, Peng C, Hamilton SE, Masopust D, et al. The Functional Requirement for CD69 in Establishment of Resident Memory CD8 + T Cells Varies With Tissue Location. J Immunol (2019) 203:946–55. doi: 10.4049/jimmunol.1900052
44. Pichyangkul S, Yongvanitchit K, Limsalakpetch A, Kum-Arb U, Im-Erbsin R, Boonnak K, et al. Tissue Distribution of Memory T and B Cells in Rhesus Monkeys Following Influenza A Infection. J Immunol (2015) 195:4378–86. doi: 10.4049/jimmunol.1501702
45. Thompson EA, Darrah PA, Foulds KE, Hoffer E, Caffrey-Carr A, Norenstedt S, et al. Monocytes Acquire the Ability to Prime Tissue-Resident T Cells via IL-10-Mediated TGF-β Release. Cell Rep (2019) 28:1127–35. doi: 10.1016/j.celrep.2019.06.087
46. Yang Q, Zhang M, Chen Q, Chen W, Wei C, Qiao K, et al. Cutting Edge: Characterization of Human Tissue-Resident Memory T Cells at Different Infection Sites in Patients With Tuberculosis. J Immunol (2020) 204:2331–6. doi: 10.4049/jimmunol.1901326
47. Walrath JR, Silver RF. The α4β1 Integrin in Localization of Mycobacterium Tuberculosis-Specific T Helper Type 1 Cells to the Human Lung. Am J Respir Cell Mol Biol (2011) 45:24–30. doi: 10.1165/rcmb.2010-0241OC
48. Dijkman K, Sombroek CC, Vervenne RAW, Hofman SO, Boot C, Remarque EJ, et al. Prevention of Tuberculosis Infection and Disease by Local BCG in Repeatedly Exposed Rhesus Macaques. Nat Med (2019) 252:255–62. doi: 10.1038/s41591-018-0319-9
49. Tian Y, Sun Y, Gao F, Koenig MR, Sunderland A, Fujiwara Y, et al. CD28H Expression Identifies Resident Memory CD8 + T Cells With Less Cytotoxicity in Human Peripheral Tissues and Cancers. Oncoimmunology (2019) 8:e1538440. doi: 10.1080/2162402X.2018.1538440
50. Tan H-X, Esterbauer R, Vanderven HA, Juno JA, Kent SJ, Wheatley AK. Inducible Bronchus-Associated Lymphoid Tissues (iBALT) Serve as Sites of B Cell Selection and Maturation Following Influenza Infection in Mice. Front Immunol (2019) 10:611. doi: 10.3389/fimmu.2019.00611
51. Koutsakos M, Illing PT, Nguyen THO, Mifsud NA, Crawford JC, Rizzetto S, et al. Human CD8 + T Cell Cross-Reactivity Across Influenza A, B and C Viruses. Nat Immunol (2019) 20:613–25. doi: 10.1038/s41590-019-0320-6
52. De Bree GJ, Van Leeuwen EMM, Out TA, Jansen HM, Jonkers RE, Van Lier RAW. Selective Accumulation of Differentiated CD8+ T Cells Specific for Respiratory Viruses in the Human Lung. J Exp Med (2005) 202:1433–42. doi: 10.1084/jem.20051365
53. Gray JI, Westerhof LM, MacLeod MKL. The Roles of Resident, Central and Effector Memory CD4 T-Cells in Protective Immunity Following Infection or Vaccination. Immunology (2018) 154:574–81. doi: 10.1111/imm.12929
54. Rangel-Moreno J, Carragher DM, Misra RS, Kusser K, Hartson L, Moquin A, et al. Randall TD. B Cells Promote Resistance to Heterosubtypic Strains of Influenza. Via Multiple Mechanisms J Immunol (2008) 180:454–63. doi: 10.4049/jimmunol.180.1.454
55. Swarnalekha N, Schreiner D, Litzler LC, Iftikhar S, Kirchmeier D, Künzli M, et al. T Resident Helper Cells Promote Humoral Responses in the Lung. Sci Immunol (2021) 6(55):eabb6808. doi: 10.1126/sciimmunol.abb6808
56. Eyles JE, Johnson JE, Megati S, Roopchand V, Cockle PJ, Weeratna R, et al. Nonreplicating Vaccines can Protect African Green Monkeys From the Memphis 37 Strain of Respiratory Syncytial Virus. J Infect Dis (2013) 208:319–29. doi: 10.1093/infdis/jit169
57. Corleis B, Bucsan AN, Deruaz M, Vrbanac VD, Lisanti-Park AC, Gates SJ, et al. HIV-1 and SIV Infection Are Associated With Early Loss of Lung Interstitial CD4+ T Cells and Dissemination of Pulmonary Tuberculosis. Cell Rep (2019) 26:1409–18. doi: 10.1016/j.celrep.2019.01.021
58. Foreman TW, Mehra S, LoBato DN, Malek A, Alvarez X, Golden NA, et al. CD4+ T-Cell-Independent Mechanisms Suppress Reactivation of Latent Tuberculosis in a Macaque Model of HIV Coinfection. Proc Natl Acad Sci USA (2016) 113:E5636–44. doi: 10.1073/pnas.1611987113
59. Dubois V, Chatagnon J, Thiriard A, Bauderlique-Le Roy H, Debrie AS, Coutte L, et al. Suppression of Mucosal Th17 Memory Responses by Acellular Pertussis Vaccines Enhances Nasal Bordetella Pertussis Carriage. NPJ Vaccines (2021) 6(1):6. doi: 10.1038/s41541-020-00270-8
60. Ge C, Monk IR, Pizzolla A, Wang N, Bedford JG, Stinear TP, et al. Bystander Activation of Pulmonary Trm Cells Attenuates the Severity of Bacterial Pneumonia by Enhancing Neutrophil Recruitment. Cell Rep (2019) 29:4236–44. doi: 10.1016/j.celrep.2019.11.103
61. Kiyono H, Fukuyama S. NALT-Versus Peyer’s-Patch-Mediated Mucosal Immunity. Nat Rev Immunol (2004) 4(9):699–710. doi: 10.1038/nri1439
62. Stolley JM, Johnston TS, Soerens AG, Beura LK, Rosato PC, Joag V, et al. Retrograde Migration Supplies Resident Memory T Cells to Lung-Draining LN After Influenza Infection. J Exp Med (2020) 217:e20192197. doi: 10.1084/jem.20192197
63. Sasson SC, Gordon CL, Christo SN, Klenerman P, Mackay LK. Local Heroes or Villains: Tissue-Resident Memory T Cells in Human Health and Disease. Cell Mol Immunol (2020) 17:113–22. doi: 10.1038/s41423-019-0359-1
64. Smyth LJC, Eustace A, Kolsum U, Blaikely J, Singh D. Increased Airway T Regulatory Cells in Asthmatic Subjects. Chest (2010) 138:905–12. doi: 10.1378/chest.09-3079
65. Christiaansen AF, Knudson CJ, Weiss KA, Varga SM. The CD4 T Cell Response to Respiratory Syncytial Virus Infection. Immunol Res (2014) 59:109–17. doi: 10.1007/s12026-014-8540-1
66. Silva-Sanchez A, Randall TD. (2019) Role of iBALT in Respiratory Immunity. In: Kabashima K., Egawa G. (eds) Inducible Lymphoid Organs. Current Topics in Microbiology and Immunology, vol 426. Cham: Springer. doi: 10.1007/82_2019_191
67. Van De Sandt CE, Barcena M, Koster AJ, Kasper J, Kirkpatrick CJ, Scott DP, et al. Human CD8+ T Cells Damage Noninfected Epithelial Cells During Influenza Virus Infection In Vitro. Am J Respir Cell Mol Biol (2017) 57:536–46. doi: 10.1165/rcmb.2016-0377OC
68. Knight FC, Wilson JT. Engineering Vaccines for Tissue-Resident Memory T Cells. Adv Ther (2021) 4(4):2000230. doi: 10.1002/adtp.202000230
69. Koutsakos M, Wheatley AK, Loh L, Clemens EB, Sant S, Nüssing S, et al. Circulating TFH Cells, Serological Memory, and Tissue Compartmentalization Shape Human Influenza-Specific B Cell Immunity. Sci Transl Med (2018) 10(428):eaan8405. doi: 10.1126/scitranslmed.aan8405
70. Mohn KGI, Smith I, Sjursen H, Cox RJ. Immune Responses After Live Attenuated Influenza Vaccination. Hum Vaccines Immunother (2018) 14:571–8. doi: 10.1080/21645515.2017.1377376
71. Zens KD, Chen JK, Farber DL. Vaccine-Generated Lung Tissue–Resident Memory T Cells Provide Heterosubtypic Protection to Influenza Infection. JCI Insight (2019) 1:1–12. doi: 10.1172/jci.insight.85832
72. Goplen NP, Huang S, Zhu B, Cheon IS, Son YM, Wang Z, et al. Tissue-Resident Macrophages Limit Pulmonary CD8 Resident Memory T Cell Establishment. Front Immunol (2019) 10:2332. doi: 10.3389/fimmu.2019.02332
73. Darrah PA, Zeppa JJ, Maiello P, Hackney JA, Wadsworth MH, Hughes TK, et al. Prevention of Tuberculosis in Macaques After Intravenous BCG Immunization. Nature (2020) 577:95–102. doi: 10.1038/s41586-019-1817-8
74. Sharpe S, White A, Sarfas C, Sibley L, Gleeson F, McIntyre A, et al. Alternative BCG Delivery Strategies Improve Protection Against Mycobacterium Tuberculosis in non-Human Primates: Protection Associated With Mycobacterial Antigen-Specific CD4 Effector Memory T-Cell Populations. Tuberculosis (2016) 101:174–90. doi: 10.1016/j.tube.2016.09.004
75. Verreck FAW, Tchilian EZ, Vervenne RAW, Sombroek CC, Kondova I, Eissen OA, et al. Variable BCG Efficacy in Rhesus Populations: Pulmonary BCG Provides Protection Where Standard Intra-Dermal Vaccination Fails. Tuberculosis (2017) 104:46–57. doi: 10.1016/j.tube.2017.02.003
76. White AD, Sarfas C, West K, Sibley LS, Wareham AS, Clark S, et al. Evaluation of the Immunogenicity of Mycobacterium Bovis BCG Delivered by Aerosol to the Lungs of Macaques. Clin Vaccine Immunol (2015) 22:992–1003. doi: 10.1128/CVI.00289-15
77. Nieuwenhuizen NE, Kulkarni PS, Shaligram U, Cotton MF, Rentsch CA, Eisele B, et al. The Recombinant Bacille Calmette-Guérin Vaccine VPM1002: Ready for Clinical Efficacy Testing. Front Immunol (2017) 8:1147. doi: 10.3389/fimmu.2017.01147
78. Kaushal D, Foreman TW, Gautam US, Alvarez X, Adekambi T, Rangel-Moreno J, et al. Mucosal Vaccination With Attenuated Mycobacterium Tuberculosis Induces Strong Central Memory Responses and Protects Against Tuberculosis. Nat Commun (2015) 26:8533. doi: 10.1038/ncomms9533
79. Hansen SG, Zak DE, Xu G, Ford JC, Marshall EE, Malouli D, et al. Prevention of Tuberculosis in Rhesus Macaques by a Cytomegalovirus-Based Vaccine. Nat Med (2018) 24:130–43. doi: 10.1038/nm.4473
80. Clegg J, Soldaini E, Bagnoli F, McLoughlin RM. Targeting Skin-Resident Memory T Cells via Vaccination to Combat Staphylococcus Aureus Infections. Trends Immunol (2021) 42(1):6–17. doi: 10.1016/j.it.2020.11.005
81. Jackson LA, Campbell JD, Frey SE, Edwards KM, Keitel WA, Kotloff KL, et al. Effect of Varying Doses of a Monovalent H7N9 Influenza Vaccine With and Without AS03 and MF59 Adjuvants on Immune Response a Randomized Clinical Trial. JAMA J Am Med Assoc (2015) 314:237–46. doi: 10.1001/jama.2015.7916
82. Galson JD, Trück J, Kelly DF, van der Most R. Investigating the Effect of AS03 Adjuvant on the Plasma Cell Repertoire Following Ph1n1 Influenza Vaccination. Sci Rep (2016) 6:37229. doi: 10.1038/srep37229
83. Lofano G, Mancini F, Salvatore G, Cantisani R, Monaci E, Carrisi C, et al. Oil-In-Water Emulsion MF59 Increases Germinal Center B Cell Differentiation and Persistence in Response to Vaccination. J Immunol (2015) 195:1617–27. doi: 10.4049/jimmunol.1402604
84. Openshaw PJ, Chiu C. Protective and Dysregulated T Cell Immunity in RSV Infection. Curr Opin Virol (2013) 3:468–74. doi: 10.1016/j.coviro.2013.05.005
85. Habibi MS, Jozwik A, Makris S, Dunning J, Paras A, DeVincenzo JP, et al. Impaired Antibody-Mediated Protection and Defective IgA B-Cell Memory in Experimental Infection of Adults With Respiratory Syncytial Virus. Am J Respir Crit Care Med (2015) 191:1040–9. doi: 10.1164/rccm.201412-2256OC
86. Taefehshokr N, Taefehshokr S, Hemmat N, Heit B. Covid-19: Perspectives on Innate Immune Evasion. Front Immunol (2020) 11:580641. doi: 10.3389/fimmu.2020.580641
87. Hobson D, Curry RL, Beare AS, Ward-Gardner A. The Role of Serum Haemagglutination-Inhibiting Antibody in Protection Against Challenge Infection With Influenza A2 and B Viruses. J Hyg (Lond) (1972) 70:767–77. doi: 10.1017/S0022172400022610
88. Krstajin N, Mills B, Murray I, Marshall A, Norberg D, Craven TH, et al. Low-Cost High Sensitivity Pulsed Endomicrocopy to Visualize Tricolor Optical Signatures. J Biomed Optics (2018) 23:1–12. doi: 10.1117-1.JBO.23.7.076005
89. Craven TH, Walton T, Akram AR, Scholefield E, McDonald N, Marshall ADL, et al. Activated Neutrophil Fluorescent Imaging Technique for Human Lungs. Sci Rep (2021) 11:976. doi: 10.1038/s41598-020-80083-w
90. Farber DL. Tissues, Not Blood, are Where Immune Cells Function. Nature (2021) 593:506–9. doi: 10.1038/d41586-021-01396-y
Keywords: lung, resident memory T cells, resident memory B cells, infection, vaccination, in situ optical imaging, EVLP
Citation: Humphries DC, O’Connor RA, Larocque D, Chabaud-Riou M, Dhaliwal K and Pavot V (2021) Pulmonary-Resident Memory Lymphocytes: Pivotal Orchestrators of Local Immunity Against Respiratory Infections. Front. Immunol. 12:738955. doi: 10.3389/fimmu.2021.738955
Received: 09 July 2021; Accepted: 01 September 2021;
Published: 17 September 2021.
Edited by:
Francesca Di Rosa, Italian National Research Council, ItalyReviewed by:
Wolfgang Kastenmüller, Julius Maximilian University of Würzburg, GermanyCopyright © 2021 Humphries, O’Connor, Larocque, Chabaud-Riou, Dhaliwal and Pavot. This is an open-access article distributed under the terms of the Creative Commons Attribution License (CC BY). The use, distribution or reproduction in other forums is permitted, provided the original author(s) and the copyright owner(s) are credited and that the original publication in this journal is cited, in accordance with accepted academic practice. No use, distribution or reproduction is permitted which does not comply with these terms.
*Correspondence: Duncan C. Humphries, RHVuY2FuLkh1bXBocmllc0BlZC5hYy51aw==; Kevin Dhaliwal, S2V2LkRoYWxpd2FsQGVkLmFjLnVr
Disclaimer: All claims expressed in this article are solely those of the authors and do not necessarily represent those of their affiliated organizations, or those of the publisher, the editors and the reviewers. Any product that may be evaluated in this article or claim that may be made by its manufacturer is not guaranteed or endorsed by the publisher.
Research integrity at Frontiers
Learn more about the work of our research integrity team to safeguard the quality of each article we publish.