- 1Department of Radiation Oncology, Shanghai Pulmonary Hospital, Tongji University School of Medicine, Shanghai, China
- 2Department of Oncology, Shanghai Pulmonary Hospital, Tongji University School of Medicine, Shanghai, China
Radiotherapy is an effective local treatment modality of NSCLC. Its capabilities of eliminating tumor cells by inducing double strand DNA (dsDNA) damage and modulating anti-tumor immune response in irradiated and nonirradiated sites have been elucidated. The novel ICIs therapy has brought hope to patients resistant to traditional treatment methods, including radiotherapy. The integration of radiotherapy with immunotherapy has shown improved efficacy to control tumor progression and prolong survival in NSCLC. In this context, biomarkers that help choose the most effective treatment modality for individuals and avoid unnecessary toxicities caused by ineffective treatment are urgently needed. This article summarized the effects of radiation in the tumor immune microenvironment and the mechanisms involved. Outcomes of multiple clinical trials investigating immuno-radiotherapy were also discussed here. Furthermore, we outlined the emerging biomarkers for the efficacy of PD-1/PD-L1 blockades and radiation therapy and discussed their predictive value in NSCLC.
1 Introduction
Non-small cell lung cancer (NSCLC) is a prevalent disease with high morbidity and mortality, accounting for 85% of lung cancer cases. Most NSCLC patients are at late stages when diagnosed and lose the chance for tumor resection, with a low 5-year survival rate of approximately 24% (1). Therefore, it is imperative to explore new regimens and combination strategies to improve the survival prospects of NSCLC. With a remarkable development in recent years, radiotherapy has become an indispensable locally therapeutic strategy, applied to over 50% patients in different periods for treatment. Advanced technologies such as intensity-modulated radiation therapy (IMRT) and image-guided radiotherapy (IGRT) can deliver radiation rays to the delineated tumor target precisely with minor damage to the surrounding healthy tissues. However, resistance to radiotherapy often occurs and may lead to local, nodal, and distant recurrence. Moreover, increasing researchers has shed light on both of the influence of radiation therapy on immune tumor microenvironment. It is known irradiated tumor cells can serve as in situ vaccine that stimulates systemic adaptive immune response and promotes distant tumor regression. This phenomenon was first depicted by Mole RH et al. and is called the “abscopal effect”. Although the activated CD8+ T cells and other immune stimulative cells can migrate to and infiltrate metastasis sites, anti-tumor effects could be stifled by the upregulation of immune-suppressive cells, cytokines, and other molecules (2). The problems above indicate that radiotherapy alone is insufficient to eliminate both primary and metastatic tumor lesions, and exploring novel partners for treatment is imperative.
Immune checkpoints are surface proteins on T cells and other immune cells that can negatively regulate immune response activation to maintain self-tolerance by various antigens, including tumor antigens. PD-L1, expressed on tumor cells as well as tumor-infiltrating lymphocytes, can inhibit the proliferation of T cells and secretion of cytokine through binding to PD-1 and B7 receptors on activated immune cells (3). Cytotoxic T lymphocyte-associated protein 4 (CTLA-4) is another well characterized immune checkpoint that is primarily expressed on activated T cells and FoxP3+ regulatory T cells (Tregs) (4). It prevents the second costimulatory signal in T cell activation through binding to CD80/86 on APC competitively with a higher affinity than CD28 (5). CTLA-4 pathway also contributes to the immunosuppressive function of Tregs (6). Immune checkpoint inhibitors (ICIs) can enhance the intrinsic immune response against tumor antigens by removing the brake on T-cell activation and function. Immunotherapeutic agents that target immune checkpoint pathways have shown to be promising in clinical trials. Since the approval of ipilimumab (CTLA-4 monoclonal antibody) for metastatic melanoma in 2011, a variety of ICIs have been emerging, especially antibodies that target PD-1 and PD-L1. Some of them have been rapidly incorporated into the first or multiple line treatments, dramatically altering the treatment landscape of many solid tumors including malignant melanoma, NSCLC, renal cell carcinoma, bladder cancer and so on (7). Compared with CTLA-4 blockades, PD-1/PD-L1 blockades have shown efficacy in a wider range of cancer types and lower toxicity (8). As for metastatic NSCLC, nivolumab, pembrolizumab, and atezolizumab have been recommended in clinical practice for treatment. Notably, Pembrolizumab is recommended for monotherapy as first-line treatment for NSCLC patients without oncogenic driving mutations and with a high PD-L1 expression (tumor proportion score (TPS) ≥ 50%). However, the response rate to immune checkpoint blockade monotherapy has been disappointing in multiple clinical trials. Moreover, a range of immune-related toxicities such as colitis, hypophysitis, pneumonitis, thyroiditis, inflammatory arthritis, and more have been reported (9). Under these conditions, it has been recognized that combining anti-PD-1/PD-L1 treatment and radiation therapy could help overcome treatment resistance and improve survival benefits of patients synergistically. Studies on melanoma and NSCLC have accounted for a big part of studies relative to radioimmunotherapy. They carry a large mutation load and are characterized as high immunogenicity. Therefore, administration of radiation and ICIs to increase the tumor immunogenicity and immunoreactivity is critical in tumor regression.
Currently, delivery of radiotherapy and PD-1/PD-L1 blockades in the clinical practice is still imprecise, with a limited ability to identify patients who will get survival benefit from immune blockades and radiation treatments or patients who are likely to suffer from adverse effects caused by these modalities. For this reason, predictive biomarkers are in urgent need in order to tailor the treatment strategy for individuals. At present, only PD-L1 expression levels on tumor cells have been widely used as a standard predictor to drive anti-PD-1/PD-L1 treatment in the clinic, while multiple other markers detected by genomic, transcriptomic, proteomic, and metabolomic analysis are still under investigation and validation. This article will discuss the immune effects of radiotherapy in tumor microenvironment and the advances in applying radiotherapy plus immunotherapy. We will also outline the emerging biomarkers which show potential to predict response to PD-1/PD-L1 inhibitors as well as radiotherapy in NSCLC.
2 Radiotherapy Effects on Immune Tumor Microenvironment
Radiotherapy brings damage to target tumor cells through not only DNA strand break but also the killing effects of activated immune cells. Irradiation has been known to modulate the landscape of immune tumor micro-environment (TME) and systemic response both positively and negatively in a variety of ways (Figure 1).
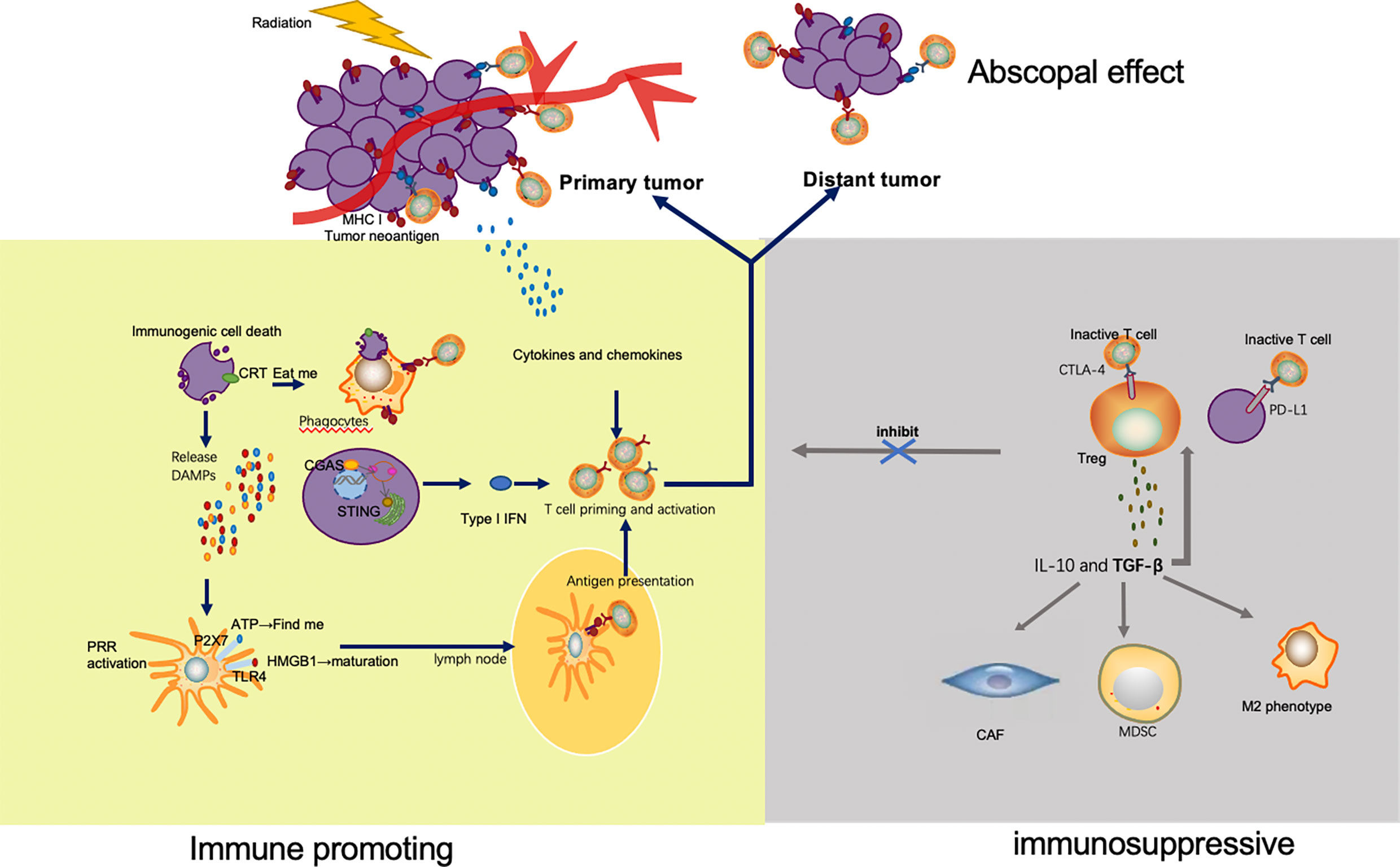
Figure 1 Mechanisms by which radiation enhances and inhibits immune response. Irradiation of tumor leads to immunogenic cell death and release of tumor neoantigens. Multiple DAMPs secreted from apoptotic tumor cells facilitate the uptake and presentation of neoantigens. Released ATP and exposure of CRT send the ‘find me’ and ‘eat me’ signal to APC and phagocyte respectively. HMGB1 promotes maturation of APC through binding with TLR4. As a result, APC migrates to lymph nodes and presents neoantigens to T cells mediated by MHC pathway. In addition to antigen presentation, type I IFN via cGAS-STING pathway and a variety of other cytokines and chemokines also take part in T cell priming and activation. The activated T cells, especially the CD8+ T cells proliferate, home to tumor, and exert their killing effect. Apart from the irradiated tumor site, effector T cells also migrate to distant tumors and drive abscopal effect. However, immune escape appears after radiation, which limits the efficacy of radiation. Upregulation of immune checkpoints, such as CTLA-4 on Tregs and PD-L1 on tumor cells, inhibits the activation and function of T cells. TGF β secreted by Tregs is capable to significantly increase the number of immunosuppressive cells in the TME, including Treg, CAF, MDSC and M2 phenotype macrophage.
2.1 Radiation Reprograms Immune TME
2.1.1 Radiation Induces Immunogenic Cell Death
Immunogenic cell death (ICD) can be induced by radiation therapy in a dose-dependent way and is composed of the induction of organellar and cellular stress, accompanied by the release of neoantigen and endogenous danger signals-damage associated molecular patterns (DAMPs) from dying tumor cells, such as high-mobility group box 1 (HMGB1), adenosine triphosphate (ATP), as well as exposure of calreticulin (10, 11). Extracellular ATP was determined to be a critical ‘find me’signal in phagocytes recruitment through binding to P2Y2 receptor on apoptotic cells (12). It also promotes secretion of IL-1β and IL-18 (13). The translocation of calreticulin on cell membrane sends the important ‘eat me’ danger signal and induces clearance of tumor cells by antigen presenting cells(APC), such as dendritic cells(DCs) and phagocytic cells. HMGB1 enhances antigen processing and presentation and maturation of APC especially dendritic cells (DCs) after binding to specific pattern recognition receptors (PRRs), such as Toll like receptor-2 and Toll like receptor-4 (14, 15). Increased type I IFN via cytosolic DNA sensing pathway——cyclic guanosine monophosphate-adenosine monophosphate (cGAMP) synthase (cGAS) and its downstream effector Stimulator of Interferon Genes (STING) also plays a critical role in T cell activation and the drive of immune response after radiotherapy (16, 17). Radiotherapy effectuates the killing of tumor cells by DNA double strand break (DSB) induction, which leads to the mechanism by which self-DNA becomes exposed to the cytoplasm (18). Studies conducted by Deng et al. demonstrates that cytosolic DNA sensed by cGAS triggers IFN-I stimulated genes (ISG) induction and type I IFN secretion mainly by CD11c+DCs. They also determine that STING is critical to tumor control through radiotherapy in vivo (17).
2.1.2 T Cell Infiltration and Activation
It is known that cytotoxic CD8+ T lymphocyte immunity is critical in the control of the tumor. Although radiotherapy has destructive effects on immune cells, recent studies have shown that a large proportion of T cells in irradiated tumor sites survived with increased motility and higher production of IFN γ compared with T cells in unirradiated tumors (19). T cell activation and priming could be elicited by radiotherapy through sufficient antigen and co-stimulation provided by local antigen presenting cells (APC) as well as chemokines secretion such as CXCL16 (20, 21). Numerous genes involved in antigen processing and presentation pathways were found to be upregulated in many cell lines after irradiation through transcriptome analysis (22). Reits et al. reported that radiotherapy could dose-dependently upregulate the expression of MHC-I molecules on neoplastic cells.
The increase of intracellular existing protein degradation leads to increased peptide pool, upregulated protein translation and peptide production through the kinase mammalian target of rapamycin (mTOR), and new antigen production by immunogenicity mutation, which are induced by irradiation (23, 24). In addition, fractionated radiotherapy leads to T cell repertoire broaden as well as expansion of preexisting polyclonal T-cell in the locally irradiated tumor (25–27). Consequently, ICIs may help further potentiate the systemic immune, enabling T cells to recognize more tumor antigen peptides.
2.1.3 Immune-Suppressive Effects After Radiation
However, radiotherapy also elicits immunosuppressive signals such as the PD-1/PD-L1 and CTLA-4 pathway, restricting local and systemic anti-tumor immune effects. Mechanistic analysis suggested that IFN γ secreted by CD8+ T cells mediated upregulation of PD-L1 on tumor cells, thus inducing T cell exhaustion and weakening the tumor-antigen specific immune response (28, 29). This challenge could be overcome through combination with anti-PD-1/PD-L1 regimen to rescue T cell activity. In addition to PD-1 and CTLA-4 axis, regulatory CD4 + FOXP3 + T cells (Tregs) also increase after radiotherapy. Irradiated Tregs show elevated immunosuppressive capability than nonirradiated ones. Treg cells generate immunosuppressive transforming growth factor beta (TGFβ) and IL-10 through the CTLA4 signal pathway (30).
TGFβ in the TME is a potent suppressor of anti-tumor immune response. It not only promotes the recruitment and proliferation of immunosuppressive cells, including Tregs, cancer associated fibroblasts (CAF), myeloid suppressor cells (MDSCs), M2 macrophage phenotype, but also inhibit effects of CD8+ T lymphocytes and natural killer (NK) cells (31). Upregulation of these immune inhibitory cells contributes to the restricted efficacy of radiotherapy and immunotherapy (32, 33). Moreover, TGFβ plays a role in normal tissue toxicity especially chronic fibrosis after radiotherapy from a variety of pathways, leading to remodeling of lung architecture through promoting accumulation of collagen and myofibroblasts conversion (31). It is interesting to note that TGFβ contributes to radio-resistance of intra-tumoral T cells (19). Rube et al. found that higher dose of radiation (12Gy vs. 6Gy) was related to more significant release of TGFβ, reaching the summit after 12 hours and returning to basal level subsequently within one week (34). Similarly, delivery of low-dose radiation has been reported to repolarize macrophages from M2- to the pro-immunogenic M1-phenotype and enhancing infiltration of NK cells (35, 36). Therefore, radiation dose is a critical factor that influences tumor control as well as tissue toxicity post radiotherapy.
2.2 The Abscopal Effect
Abscopal effects have been known as the observation of both local and distant tumor control after applying irradiation to the regional tumor target. Accumulated evidence supports the hypothesis that irradiated tumor cells may act as an “in situ vaccine” through the production of tumor-associated antigens, induction of DAMPs, and modulation of the tumor microenvironment. Both preclinical and clinical studies showed that IFN β induced by cGAS-STING pathway after radiation played a key role in distant tumor regression (37, 38). The exposure of immunogenic mutation on tumor cells mediated by radiotherapy is another mechanism that contributes to a robust systemic T cell response and occurrence of abscopal effects (38). However, the occurrence of abscopal effect is rare and limited to case report. Therefore, radiotherapy alone is not enough to drive abscopal effect.
Demaria et al. reported 2 patients responding to the combination therapy of radiation and ICI when they failed with chemotherapy or anti-CTLA-4 therapy. It indicates that radiotherapy has a potential to drive the regression of distant tumors together with immunotherapy (38). The combination of radiotherapy and ICIs has shown to improve the opportunity to boost abscopal response rates, ranging from 23% to 38%, compared with immunotherapy or radiotherapy alone in multiple clinical trials (38–43). In addition, a volume of preclinical studies added immune-stimulative drug to radiotherapy, such as immunoadjuvant FMS-like tyrosine kinase receptor 3 ligand (sFLT3L) (44), DCs (45), granulocyte-macrophage colony-stimulating factor (GM-CSF) (46), CTLA-4 blockades (47, 48), PD-1/PD-L1 blockades (2, 25). Despite the delivered irradiation dose and fraction as well as types of immune enhancers varied, most combination therapy groups in mice models with established tumors derived from different cell lines demonstrated improved survival and distal tumor regression compared with mono-radiotherapy groups. Therefore, we hypothesize that immuno-radiotherapy has the potential to transfer rare abscopal events to a therapeutic effect.
3 Combination of Radiotherapy and Immunotherapy in NSCLC
3.1 Clinical Trials Combing RT and ICIs
Radiotherapy has emerged as a promising partner of immunotherapy due to its ability to modulate the tumor microenvironment and elicit immune responses. Increasing prospective and retrospective studies have investigated the impacts of radiation plus anti-PD-1/PD-L1 therapies on patient survival, tumor control, abscopal effects, and toxicities in different clinical scenarios (Table 1). Stereotactic ablative radiotherapy (SABR) is now considered the standard treatment in early-stage (stage I and stage IIA) NSCLC patients who are unfit or unwilling to accept surgery (54). SABR is characterized by accurate delivery of high-dose radiation to the target area with hypofractionation and short treatment time (55). Despite the high local control and less toxicity with SABR, the risk of distant recurrence (20%-25%) prompts ongoing trials to assess the efficacy of adjuvant PD-1/PD-L1 blockades after SABR (56). As for locally advanced NSCLC, many novel approaches were investigated as consolidation therapy to improve the efficacy of platinum-based chemoradiotherapy (CRT), but most of them failed. The PACIFIC trial showed a striking survival benefit for the addition of durvalumab to definitive CRT in stage III unresectable NSCLC patients. This randomized phase 3 trial observed an improved 24 month-OS rate (66.3% vs. 55.6%) and prolonged PFS duration (17.2 months vs. 5.6 months) in the durvalumab group compared with the placebo group. The time for distant metastasis was also delayed with adjuvant durvalumab treatment (28.3 months vs. 16.2 months). Interestingly, the improved outcomes were independent of PD-L1 status (49, 50). These results greatly encouraged the promise of administrating ICI as consolidation treatment to enhance the efficacy of local CRT in unresectable stage III patients.
Apart from the validated benefits from adjuvant ICI therapy after CRT, the safety profile of immuno-radiotherapy as part of definitive therapy or neoadjuvant therapy in locally advanced NSCLC has also been confirmed and its influence on patient survival will be conducted in larger cohorts (52, 53). Similarly, clinical benefits from the combination of ICI and radiotherapy in metastatic NSCLC have been validated in preclinical and clinical evidence. Combination of immunotherapy and SABR (I-SABR) can reactivate the immune system of patients undergone progression to ICI monotherapy and thus enhancing patients’ distant tumor control and clinical benefits (43). A randomized multicenter phase 2 study (PEMBRO-RT) enrolled 92 patients with advanced NSCLC and divided them into experiment arm (Pembrolizumab after SBRT of 8 Gy×3) and control arm (Pembrolizumab alone). Although the increase in response rate and survival benefits were insignificant in the experiment arm compared with the control arm, the value of PFS and OS multiplied, especially in patients with negative PD-L1 expression (42).
3.2 Exploration of the Optimal Combination Scheme
The positive results of immuno-radiotherapy have prompted researchers to explore the optimal combination schedule, including the radiation dose and fractionation, the sequence for radiotherapy and ICI administration, as well as the number of irradiated targets. Currently, a wide range of doses and fractions are used in clinical trials, and the consensus about the best schedule has not been determined yet. Generally higher dose in SABR seems to activate a more robust systemic immune response and associate with a higher response rate of unirradiated lesions compared with conventionally fractionated radiotherapy (40, 41). However, the single dose of radiation higher than 12 to 18 Gy was demonstrated to hinder anti-tumor T-cell response stimulated by IFNβ because of the dose-dependent upregulation of nuclease TREX1. Overexpressed TREX1 would degrade cytosolic dsDNA remarkedly, which became an obstacle of activating the cGAS-STING pathway as well as the abscopal effect (37). Many other studies also showed better control of distant tumors in hypofractionation rather than single-dose ablative radiotherapy (48, 57). However, preclinical and clinical studies completed by James Welsh et al. indicate that it is promising to combine the low-dose radiation of 1 Gy fraction to metastatic tumor lesions, with the high-dose radiation to the primary tumor lesions in order to further drive abscopal effects. This novel radiation strategy that is called ‘RadScopal’ technique could be a preferred partner of immunotherapy, compared with high-dose or low-dose radiation alone (36, 58, 59). The mechanisms underlying the positive results involve modulation of the immune-inhibitory tumor stroma such as TGF-β down-regulation and M1 macrophage polarization, and promote infiltration and activation of anti-tumor effective cells such as NK and CD4+ T cells. Relative prospective clinical trials are ongoing to confirm the effects of low-dose radiation.
The most appropriate time window for the combination therapy has not been determined yet. Administration of ICIs before or concurrent with radiotherapy is preferred in multiple preclinical and clinical trials (28, 60). A subgroup analysis of the PACIFIC trial suggested that delivery of ICI within 14 days after irradiation could obtain improved survival benefits in NSCLC. It is confusing that the delayed immunotherapy post radiotherapy was also effective in other studies (51, 61, 62). In a case report by Postow et al., the patient who received ipilimumab 2 months after palliative radiotherapy showed both primary and distant tumor regression significantly (62). Preliminary data from DETERRED showed that combing ICI with radiotherapy concurrently rather than sequentially might better enhance the synergistic effect of radiotherapy and immunotherapy (52), but the strong killing effect of radiation on lymphocytes must be taken into consideration. Whether this will affect subsequent immunotherapy or whether concurrent chemoradiotherapy combined with triple therapy of immunity will bring more serious side effects, especially the occurrence of radioactive pneumonia, remains to be further explored.
Other factors such as the volume and number of targets also need to be further explored. Irradiation on the tumor-draining lymph node was found to reduce the CD8+ and stem-like CD8+ T-cell and therefore hinder the distant tumor elimination (63). Furthermore, multisite or all sites irradiation can increase the release and presentation of tumor-associated antigens and improve patients’ clinical outcomes compared with single-site irradiation, but radiation-related toxicities need to be controlled in a tolerable extend (64).
To date, most of the studies in terms of combination therapy are retrospective or with small-sized cohorts. Robust evidence for the optimal scheme of immuno-radiotherapy is required in larger randomized clinical trials with minimized heterogeneity among patients.
4 Predictive Biomarkers of Immuno-Radiotherapy Efficacy
Considering the improved survival benefits for responders and the unnecessary adverse effects and costs for non-responders, developing predictive biomarkers to help physicians choose the most suitable therapeutic scheme to individuals is imperative. We have summarized the emerging biomarkers that have a potential to stratify patients into groups with different responses and risk levels in clinical practice (Table 2).
4.1 PD-L1
Currently, tumor PD-L1 expression level is the only approved biomarker to drive ICIs therapy in clinical practice (108). A good correlation between higher tumor PD-L1 expression and more significant survival benefits from anti-PD-1/PD-L1 therapy has been validated in many clinical trials. KEYNOTE-024 and KEYNOTE-042 trials, which compared pembrolizumab with platinum-based chemotherapy in previously untreated, PD-L1-expressing advanced NSCLC, showed that the best survival benefit appeared in patients who had a PD-L1 TPS ≥ 50% rather than those with PD-L1 TPS ≥ 20% and ≥ 1% (65, 66). Outcomes from CheckMate-057 also confirmed the enhanced survival benefit with higher PD-L1 expression in advanced NSCLC patients treated with Nivolumab after the failure of chemotherapy (67). However, PD-L1 is not a perfect biomarker to predict response to PD-1/PD-L1 inhibitors. Some recent randomized trials showed no significant association of PD-L1 with clinical response to anti-PD-1/PD-L1 monotherapy and anti-PD-1/PD-L1 plus chemotherapy or chemoradiotherapy in NSCLC (67, 109, 110).
The role of tumor cell PD-L1 expression level in predicting the efficacy of radiotherapy or chemoradiotherapy in various cancer types has been investigated either. Higher baseline PD-L1 level was associated with poor response and prognosis in NSCLC patients treated with chemoradiotherapy (111, 112). Moreover, the significant benefit from the addition of SBRT before immunotherapy was only seen in the PD-L1 negative subgroup in the PEMBRO-RT study (42). However, it is disappointing that patients with PD-L1 expression levels lower than 25% obtain similar survival benefits to patients with higher PD-L1 levels in the PACIFIC study (50). Therefore, it is hard to confirm the predictive role of PD-L1 in NSCLC undergoing chemoradiotherapy alone or plus immunotherapy, considering the limited number of studies and conflicting results.
The heterogeneous expression of PD-L1 across time and space (113, 114), difference in sensibility among antibodies for PD-L1 IHC testing (115), and debatable threshold for PD-L1 expression assessment may account for the conflicting results above. Therefore, researches in larger cohorts are needed to standardize the sampling method, cut point, and companion antibodies for PD-L1 assessment.
4.2 Tumor Infiltrating Lymphocytes
Immune checkpoint inhibitors require adequate lymphocyte infiltration in tumor tissue in order to exert anti-tumor effects. Radiation can stimulate local innate and adaptive immune responses by increasing TIL. Therefore, the characteristics of TIL, including composition, density, functional state, and organization, could be used as predictors of response to radiotherapy and immunotherapy (116). Cytotoxic T cell is critical to tumor elimination and metastasis, which partly account for the positive association of CD8+T cell infiltrating with improved prognosis. An immuno-score determined by both CD3+ and cytotoxic CD8+ T cells densities in two locations—tumor section and invasive margin based on IHC was developed with a higher score representing the low risk of recurrence and improved survival benefits (117). In addition, TIL density over ≥10% could predict better survival benefits from ICIs (70). Higher level of PD-1+CD8+T cells in TME has been proved to predict better outcomes in NSCLC treated with PD-1/PD-L1 inhibitors (71). However, Results from a retrospective study conducted by Mazzaschi et al. showed that low density of PD-1+CD8+T cells predicted prolonged OS and DFS and response to nivolumab in NSCLC (72). Therefore, the association between PD-1+CD8+T cells and clinical outcome after ICIs treatment has not reached a consensus. A CD8xPD-L1 signature based on image analysis showed addictive power to the densities of CD8+ or PD-L1 alone as well as tumor cell PD-L1 expression level to predict the response to durvalumab therapy (73). Concomitantly, the enrichment of myeloid-derived suppressor cell (MDSCs), regulatory T cells (Tregs), tumor-associated macrophages, and neutrophils negatively impacts prognosis because of their inhibitory function on the immune response (118, 119). For example, a high frequency of PD-L1+ CD4+ CD25+Tregs in TME can predict improved response to ICI and PFS in NSCLC (71). In addition, German Corredor et al. established a computationally derived signature–Spatial TIL. The spatial patterns and arrangement of TILs were correlated with the risk of recurrence in NSCLC of early stages (120). In terms of radiotherapy, CD8+ TIL was significantly correlated with favorable clinical outcomes (68, 69).
Of note, the prognostic value of TILs differs according to histologic types, smoking habits, and mutation landscape (121). Although the presence of TILs is essential, the direct evaluation of TILs remains challenging due to the small histologic material derived from the biopsy and varied microenvironment in different metastases. Therefore, ongoing studies are trying to create and validate guidelines for TIL assessment.
4.3 Gene Expression Profiling
4.3.1 Tumor Inflammation Signatures
Recently, multiple studies found that gene expression analysis related to tumor microenvironment through RNA isolated from formalin-fixed paraffin-embedded (FFPE) samples from patients before treatment with PD-1/PD-L1 blockades was predictive of treatment outcome in various cancer types (74, 75, 122, 123). Mark Ayers et al. developed T cell inflamed Gene Expression Profiles (GEPs) of 18 genes, representing a T cell–inflamed microenvironment and characterized by active IFN-γ signaling, T cell cytolytic activity, antigen presentation, chemokine production, and adaptive resistance. This profile was identified based on the Nano String nCounter gene expression system (NanoString Technologies, Inc., Seattle, WA) and conducted rigorous stepwise validation. Meanwhile, it demonstrated strong predictive value over clinical outcomes in a wide variety of solid tumors treated with pembrolizumab (124). Although TIS scores were higher in immunogenic tumor types, they were more variable within than between tumors types. Thus, a subset of patients who possess higher TIS scores is more likely to respond to anti-PD-1 blockade within any tumor type (123). Notably, this 18 gene profile performed favorably compared with PD-L1 immunohistochemistry (IHC) in a group of PD-L1-unselected patients, and one possible explanation for its better performance is that T-cell inflamed GEP measures multiple microenvironmental features which may influence induction and function of IFN-γ rather than a single analyte (124).
As expected, the correlations of T cell inflamed GEP with PD-L1 expression with TMB were low when analyzed in the Cancer Genome Atlas (TCGA). Meanwhile, each of the three biomarkers showed predictive value individually. Furthermore, tumors with high levels of both inflammatory and mutational biomarkers were most likely to derive clinical efficacy (74, 122, 123). Moreover, in tumor types with limited variability of TMB, TIS may provide potent predictive power on patient stratification (123). The outcomes of analyzing GEP in different aspects suggested that T cell inflamed GEP may function as a complementary predictor of response to anti-PD-1 treatment. Therefore, combining the two biomarkers may increase the accuracy of identifying populations who can potentially benefit from ICIs.
In addition to the 18 gene-T cell inflamed profile, researchers also identified other signatures of immune-related genes for NSCLC (75, 125). Brandon W et al. defined a four-gene IFNγ positive (IFNγ+) signature comprising IFNγ, CD274, LAG3, and CXCL9 through RNA sequencing of biopsies from advanced-stage NSCLC treated with durvalumab. The high signature patients showed improved survival benefits irrespectively of PD-L1 expression level (75). A profile of 24 chemokines and immunosuppressive molecules have been identified and validated, using the dataset of NSCLC patients treated with pembrolizumab from Rizvi et al. study (76). Addition of 23 chemokine and immune-suppressive molecule expression profiles to a PD-L1 profile raised the predictive correlation to 85.0% among NSCLC responders (76). Furthermore, some gene profiles that target specific immune mechanisms such as antigen processing and presentation and epithelial-to-mesenchymal transition (EMT) have been proved to be valuable predictors of ICB treatment in lung cancer (77, 78).
These patient-specific profiles of tumors defined above are used in investigations only and still under clinical validation at present. These multigene signatures can characterize TME and immune-related mechanisms comprehensively, which indicates the tumor’s immune status and thus holds a promise for identifying responding patients of ICI treatments.
4.3.2 Radiosensitivity Gene Signatures
Exploration of radiosensitivity at the genome level has attracted much attention. An early radiosensitivity gene signature, including three known genes and one unknown gene, which was RbAp48, was developed by Torres-Roca JF et al. This radiation sensitivity classifier correctly predicted survival fraction at 2 Gy (SF2) of a high proportion (22 of 35) of tumor cell lines from the National Cancer Institute panel of 60 (NCI 60). Further analysis showed that overexpression of RbAp48 in HS-578T cell line increased the proportion of cells in the G2-M phase of cell cycle and was correlated with dephosphorylation of Akt, which suggests that RbAp48 may sensitize tumor cells through antagonizing Ras (126). A similar gene signature has been developed by Kim et al., including 31 genes. The radiation-related functions of these genes are involved in the cell cycle and DNA replication, cell junction, and cell adhesion. Through gene set analysis, these genes were found to be over-presented in the integrin, VEGF, phosphatidylinositol, MAPK, JAK-STAT, Wnt, and p53 signaling pathways (79). It was found that patients with high PD-L1 were more common in the radioresistant (RR) group than radiosensitive (RS) group, and the clinical outcome of the PD-L1-high-RR group was worse than other groups (80, 81). Therefore, integrating the 31-gene signature and PD-L1 expression status can help classify patient populations who may benefit most from the combination of radiotherapy and PD-1/PD-L1 blockade in clinic (80, 81, 127). In addition to focusing on the intrinsic tumor radiosensitivity only, integrating radiosensitivity signature and immune signature could significantly interact with radiotherapy. For example, the independent predictors RSS (radiosensitivity gene signature) and IMS (antigen processing and presentation-based immune signature) were developed by Cui et al. in radiation-treated breast cancer patients. When integrating both signatures, individuals in the radiation-sensitive and immune-effective group showed prolonged survival. In contrast, in the radiation-resistant + immune-defective group and either radiation-resistant or immune-defective group, a reverse trend and no significant differences were observed (82).
Stratifying patients for radiotherapy by performance on these gene signatures is still in its infancy and needs to be confirmed in future retrospective and prospective studies. Furthermore, radiation dose adjustment based on radiosensitivity gene signatures has been under investigation, predicting the most effective dose for delivery of personalized treatment plans, thus helping spare unnecessary radiotoxicity (128). Of note, tissue of origin could influence the expression of genes involved in these signatures. For example, in a10 hub genes model, radio-resistance induced by knockdown of c-Jun is mainly found in lung cell lines (129). Therefore, signatures validated as efficient predictors in other certain tumor types may not be valuable in NSCLC.
4.4 Tumor Mutational Burden
Tumor Mutational Burden is defined as the total number of nonsynonymous mutations, including base substitutions and short insertions/deletions, per coding area of a tumor genome and calculated as mutations per DNA Megabase (Mb). A high TMB can enhance tumor immunogenicity by inducing the production of tumor-associated neoantigen, which can be recognized by T cells and promote the activation of immune attacks (83). The relation between higher TMB and better response to anti-PD-1 or PD-L1 therapy has been demonstrated in multiple clinical trials (83–85). In addition, TMB can act as an independent predictive biomarker and does not correlate with tumor PD-L1 expression level in many trials which compared the efficacy of immunotherapy with chemotherapy (84, 85). However, CheckMate026 found that the overall survival (OS) of patients treated with nivolumab in the subgroup of high TMB was comparable with chemotherapy, which questioned the predictive role of TMB (84). Although the high rate of subsequent nivolumab use (68% of patients) in the chemotherapy group may contribute to that controversial result, further investigations need to confirm the predictive role of TMB in ICI therapy and explore the additive value of combining TMB with other markers.
The role of TMB in predicting radiotherapy efficacy remains uncertain. In many clinical studies of immuno-radiotherapy in NSCLC, the level of TMB in the population was not obtained. High TMB was demonstrated to correlate with poor outcome in head and neck squamous cell carcinoma (HNSCC) patients (130), however, a group of irradiated NSCLC patients with higher TMB showed durable survival, and this benefit was not observed in the nonirradiated patient group in another study (86). In terms of immune-radiotherapy, TMB could not serve as a well-qualified predictor as well. In a case report, both regional and abscopal response were obtained after combining PD-1 inhibitor and SBRT treatments in three advanced or recurrent NSCLC patients with low TMB, microsatellite stable (MSS), proficient mismatch repair (MMR), and negative PD-L1 expression (131).
The following problems could restrict the TMB evaluation in patient selection. Firstly, TMB varies in NSCLCs with different smoking histories, ages, and mutations in genes essential for DNA repair and replication (132). Secondly, the optimal methodology for TMB measurement has not been made a consensus. Secondly, a high TMB has been defined at various thresholds across different platforms, which may influence the accuracy and predictive power of TMB (132, 133). Therefore, the good cut point of TMB and the optimal gene panel size in clinical use are urgent to be defined in further studies.
4.5 Liquid Biopsies for Circulating Biomarkers
It is difficult to obtain enough lung biopsy in some cases, therefore, invasive biomarkers derived from peripheral blood are attractive options compared with tissue derived biomarkers. In addition, the circulating predictors could make it convenient for dynamic evaluation of patient response during treatment.
4.5.1 ct-DNA
Circulating tumor DNA (ctDNA) is derived from tumor cells and includes the genomic information of tumors. It could be detected through different methods, such as NGS, qPCR and droplet digital PCR (ddPCR) (134).
Apart from monitoring tumor progression and recurrence, qualification of ctDNA level is also an effective way to monitor tumor cell death in real time. The drop in ctDNA after administration of ICI has been validated to predict responders and the prolonged survival in NSCLC, and vice versa. Notably, this biomarker changed more rapidly and profoundly than tumor size measured by imaging ways, such as CT (97–99). Another advantage of ctDNA reported by Jenny H et al. is that it could accurately discriminate tumor pseudo-progression from true progression in melanoma cohort within 12 weeks of PD-1 antibodies treatment. More surprisingly, outcomes of a prospective study in advanced conducted by Laura et al. showed that augmentation of ctDNA predicted progression after immunotherapy with 100% specificity (135).
The predictive value of ctDNA in radiotherapy is still unclear yet. It has been confirmed to increase shortly after radiation and then decrease at one week in advanced NSCLC (136, 137). They hypothesize that the ctDNA may originate from apoptotic tumor cells since higher ctDNA level was detected at 24h post radiation, which is similar to the kinetics of tumor cell apoptosis (136). However, data from relative studies is too limited to reach a consensus.
4.5.2 Peripheral Blood Cells and Lymphocyte Ratios
The circulating lymphocytes are critical to the activation of systemic anti-tumor immune response and thus the efficacy of various treatment modalities. Although there are differences between the composition of lymphocytes in peripheral blood and TME, scientists hypothesize that circulating lymphocytes can reflect the immune responses in TME indirectly. In this context, multiple inflammation-related blood parameters such as white blood cells, neutrophil cells, NK cells, monocytes, platelet, MDSC and lactate dehydrogenase (LDH) have been explored for their predictive or prognostic power of ICI treatment across different cancer types, including NSCLC (88). Higher baseline median absolute lymphocyte count (ALC) significantly associates with appearance of abscopal effects post radiotherapy and improved survival benefits in patients with ICI treatment (87, 88). A larger number of proliferative PD-1+ CD8 T+ cells and active NK cells have been detected in the peripheral blood of responders that obtained better survival benefits after anti-PD-1/PD-L1 treatments (89, 90).
Inflammatory indexes such as high level of baseline neutrophil to lymphocyte ratio (NLR), platelet-to-lymphocyte ratio (PLR), and low level of lymphocyte to monocyte ratio (LMR) are reliable indicators of poor survival in advanced NSCLC in multiple retrospective pool analysis (138–140). A similar association of NLR with patient survival was also observed in radiotherapy. Both high baseline NLR and the significantly increased NLR after treatment were associated with higher mortality in SBRT treated early-stage NSCLC patients (91). Post-Stereotactic radiosurgery (SRS) NLR> 6 was a significant marker for worse OS of NSCLC with brain metastasis (92). Integrating NLR and other known biomarkers has demonstrated better predictive value for immunotherapy. Laura Mezquita et al. generated a Lung Immune Prognostic Index (LIPI) based on the derived NLR and lactate dehydrogenase (LDH) as a predictor for anti-PD-1/PD-L1 treatment through stratifying patients into three risk groups (good, 0 factors; intermediate, 1 factor; poor, 2 factors) with the poor LIPI patients having the worst survival outcome and highest risk of progressive disease (93). Shortly afterward, several studies confirmed the prognostic value of LIPI in terms of specific PD-L1 blockades, including Atezolizumab and nivolumab (94, 95). Apart from LIPI, NLR level added independent prognostic value to TMB in the context of 1714 patients across a wide range of tumor types. The chance of benefiting from ICI treatment was three times higher in NLR-low/TMB-high group than in the NLR-high/TMB-low group, which showed that this combination had a promise to improve patient selection in clinical practice (96).
4.5.3 MicroRNAs
miRNAs represent single-stranded, ~22 nucleotide long non-coding RNAs. They bind to miRNA response elements (MREs), the short core sequence mainly in the 3′untranslated region (UTR) of their target messenger RNAs (mRNAs), and lead to inhibition of translation or mRNA degradation. Many miRNAs have been associated with radiosensitivity and radiotherapy efficacy by targeting different molecules and influencing various mechanisms, such as DNA damage repair, cell cycle regulation, apoptosis, glycolysis, autophagy (141–143). Great efforts have been focused on characterizing miRNA expression signatures for radiotherapy selection and patient stratification (144, 145).
Recent studies showed that multiple miRNAs could regulate PD-L1 expression. These miRNAs can affect tumor immune microenvironment and T cell activities which are pivotal to PD-1/PD-L1 blockades responsiveness. Researchers have identified several miRNA signatures that demonstrate the ability to predict clinical outcomes of NSCLC patients treated with nivolumab (146, 147). Of note, the combination of a plasma microRNA-signature classifier (MSC) and PD-L1 showed the more potent predictive value and could identify NSCLC patients with the worst clinical outcome (0% one-year PFS and OS subgroup with no favorable markers) in immunotherapy (100).
Overall, reports from accumulative studies have recognized the role of miRNA signatures as potential biomarkers to radiotherapy and immunotherapy. Compared with tumor tissue-based markers, detection of circulating miRNAs is a less invasive and more cost-effective way to monitor diseases. Circulating miRNA has advantages of stability and real-time supervision because they can be packaged in exosomes or other micro-vesicles and bind to protein complexes such as Argonaute2, which help them avoid degradation in plasma (148, 149). However, the predictive value of these miRNA signatures needs to be validated in larger NSCLC cohorts before they could be applied to clinical use.
4.6 Imaging Biomarkers
Tumor tissue is characterized by the high metabolism status and glycolysis rate because of the rapid proliferation of both tumor cells and lymphocytes and activation of immune responses. Chang. et al. suggested that PD-L1 enhanced glucose metabolism in tumor cells, leaving immune cells with insufficient glycolysis to maintain anti-tumor activity and proliferation, thus promoting tumor progression. While Immune checkpoint blockade reversed the tumor-induced glucose depletion in TILs and hence improving their effector function (150). The increase of metabolic parameters, such as standardized uptake value (SUV), metabolic tumor volume (MTV), and tumor lesion glycolysis (TLG) based on [18F]-fluoro-2-deoxy-d-glucose (18F-FDG) positron emission tomography (PET), was found to associate with a high PD-L1 level and TILs that express PD-1, CD8, CD163 (tumor-associated macrophages) and Foxp3 (Tregs) by IHC (101, 151, 152). Therefore, increasing studies have tried to validate the predictive role of tumor metabolic variables in immunotherapy (101, 102).
18F-FDG PET/CT is recognized as an essential tool in radiation treatment planning, such as the tumoral target delineation. It is valuable in predicting the outcome in less malignant lung cancer patients treated with SABR or SBRT. There seems to be a consensus that patients with high baseline SUV, MTV, and TLG are correlated with poorer clinical outcomes after radiotherapy (103–105). In addition to metabolic predictors, radiomics— a more advanced high-throughput image analysis method has been recently under investigation. It can reflect intra-tumoral histopathologic properties quantitatively and showed great power in predicting patients’ survival benefits, local recurrence, and distant metastasis after radiotherapy (106).
Generally, evaluation based on imaging tools, including PET and CT, is a good way to predict responsiveness to radiotherapy and immunotherapy, especially for patients who cannot get biopsy samples because of infection or multiple tumor lesions. Signatures integrating imaging predictors and other biomarkers, such as miRNAs signatures and dNLR, have demonstrated better choices (107, 145). However, the highly variable repeatability among those radiomic features could restrict their wide application in the future clinic (106). Many details of these imaging biomarkers, such as the optimal time for evaluation and cutoff values of these parameters, should be further defined in more prospective multicenter studies.
5 Discussion
Radiotherapy is one of the traditional anti-tumor treatment modalities for NSCLC. Its technological advances have allowed precise delivery of radiation to the regional tumor with an improved toxicity profile. Radiation on tumor cells can induce double strand DNA break and secretion of type I IFN through cytosolic DNA sensing pathway activation. In addition, radiotherapy stimulates anti-tumor immune responses in multiple ways, including antigen presentation enhancement, T cell priming, and T cell repertoire expansion. However, the efficacy of radiotherapy is not satisfying. Abscopal effects induced by irradiation are rare due to immunosuppressive effects caused by upregulated immune checkpoints and recruitment of immune inhibitory cells. Therefore, the combination of radiotherapy with ICIs is promising to generate synergistic immune effects. Multiple preclinical and clinical trials have evaluated patients’ responses to and clinical outcomes for immuno-radiotherapy. Generally, this combination strategy demonstrated improved response rate and survival benefits in NSCLC of different stages. However, details for this combination strategy need to be further investigated regarding the optimal dose and fraction of radiation, the sequence for radiotherapy and ICIs treatment.
Furthermore, biomarkers for predicting immune response to anti-PD-1/PD-L1 blockades and tumor radiosensitivity are essential in the era of precision medicine. It will allow clinicians to choose the most effective treatment modality for every individual and discontinue the inefficient regimens as early as possible. PD-L1 is the only biomarker widely used for patient stratification for ICI treatment in clinical practice, but its predictive role is debatable. Many other markers based on tumor tissue, peripheral blood, and radiological images have been investigated, including TMB, infiltrated lymphocytes, tumor gene signatures, ctDNA, peripheral lymphocytes, microRNAs, and imaging biomarkers. Some of these predictors can help personalize therapeutic management, such as adjusting the radiation dose prescription according to risk assessment for better efficacy and fewer injuries. In addition to biomarkers mentioned in this review, other molecules such as T cell clonality and gut microbiome have also been reported to correlate with response to immunotherapy or radiotherapy. However, all of them showed the limited capability of discriminating non-responders from responders regarding radiotherapy and immunotherapy. Moreover, the standardized cutoff points, methodologies, optimal timepoint for detection in these biomarkers have not been determined yet. In this context, the combination of different biomarkers has been proved to add accuracy to a single marker. Real-time supervision of these markers may improve their predictive values as well. Therefore, more investigations are needed to develop novel cycling markers and explore the predictive power of previously tumor tissue-based markers from periphery blood.
Author Contributions
LM, JFX and YY have contributed to the writing of this manuscript. All authors contributed to the article and approved the submitted version.
Funding
This research was supported by Scientific and Innovative Action Plan of Shanghai (20Y11913600), Natural Science Foundation of Shanghai (21ZR1453300), Shanghai Pulmonary Hospital (fkgg1808) and National Natural Science Foundation of China (81602657).
Conflict of Interest
The authors declare that the research was conducted in the absence of any commercial or financial relationships that could be construed as a potential conflict of interest.
Publisher’s Note
All claims expressed in this article are solely those of the authors and do not necessarily represent those of their affiliated organizations, or those of the publisher, the editors and the reviewers. Any product that may be evaluated in this article, or claim that may be made by its manufacturer, is not guaranteed or endorsed by the publisher.
References
1. Garon EB, Hellmann MD, Rizvi NA, Carcereny E, Leighl NB, Ahn MJ, et al. Five-Year Overall Survival for Patients With Advanced Non‐Small-Cell Lung Cancer Treated With Pembrolizumab: Results From the Phase I KEYNOTE-001 Study. J Clin Oncol (2019) 37(28):2518–27. doi: 10.1200/jco.19.00934
2. Park SS, Dong H, Liu X, Harrington SM, Krco CJ, Grams MP, et al. PD-1 Restrains Radiotherapy-Induced Abscopal Effect. Cancer Immunol Res (2015) 3(6):610–9. doi: 10.1158/2326-6066.Cir-14-0138
3. Patsoukis N, Wang Q, Strauss L, Boussiotis VA. Revisiting the PD-1 Pathway. Sci Adv (2020) 6(38):eabd2712. doi: 10.1126/sciadv.abd2712
4. Takahashi T, Tagami T, Yamazaki S, Uede T, Shimizu J, Sakaguchi N, et al. Immunologic Self-Tolerance Maintained by CD25(+)CD4(+) Regulatory T Cells Constitutively Expressing Cytotoxic T Lymphocyte-Associated Antigen 4. J Exp Med (2000) 192(2):303–10. doi: 10.1084/jem.192.2.303
5. Walunas TL, Lenschow DJ, Bakker CY, Linsley PS, Freeman GJ, Green JM, et al. CTLA-4 can Function as a Negative Regulator of T Cell Activation. Immunity (1994) 1(5):405–13. doi: 10.1016/1074-7613(94)90071-x
6. Peggs KS, Quezada SA, Chambers CA, Korman AJ, Allison JP. Blockade of CTLA-4 on Both Effector and Regulatory T Cell Compartments Contributes to the Antitumor Activity of Anti-CTLA-4 Antibodies. J Exp Med (2009) 206(8):1717–25. doi: 10.1084/jem.20082492
7. Guibert N, Mazières J. Nivolumab for Treating non-Small Cell Lung Cancer. Expert Opin Biol Ther (2015) 15(12):1789–97. doi: 10.1517/14712598.2015.1114097
8. Das S, Johnson DB. Immune-Related Adverse Events and Anti-Tumor Efficacy of Immune Checkpoint Inhibitors. J Immunother Cancer (2019) 7(1):306. doi: 10.1186/s40425-019-0805-8
9. Wang PF, Chen Y, Song SY, Wang TJ, Ji WJ, Li SW, et al. Immune-Related Adverse Events Associated With Anti-PD-1/PD-L1 Treatment for Malignancies: A Meta-Analysis. Front Pharmacol (2017) 8:730. doi: 10.3389/fphar.2017.00730
10. Golden EB, Frances D, Pellicciotta I, Demaria S, Helen Barcellos-Hoff M, Formenti SC. Radiation Fosters Dose-Dependent and Chemotherapy-Induced Immunogenic Cell Death. Oncoimmunology (2014) 3:e28518. doi: 10.4161/onci.28518
11. Garg AD, Krysko DV, Verfaillie T, Kaczmarek A, Ferreira GB, Marysael T, et al. A Novel Pathway Combining Calreticulin Exposure and ATP Secretion in Immunogenic Cancer Cell Death. EMBO J (2012) 31(5):1062–79. doi: 10.1038/emboj.2011.497
12. Elliott MR, Chekeni FB, Trampont PC, Lazarowski ER, Kadl A, Walk SF, et al. Nucleotides Released by Apoptotic Cells Act as a Find-Me Signal to Promote Phagocytic Clearance. Nature (2009) 461(7261):282–6. doi: 10.1038/nature08296
13. Perregaux DG, McNiff P, Laliberte R, Conklyn M, Gabel CA. ATP Acts as an Agonist to Promote Stimulus-Induced Secretion of IL-1 Beta and IL-18 in Human Blood. J Immunol (2000) 165(8):4615–23. doi: 10.4049/jimmunol.165.8.4615
14. Paludan SR, Reinert LS, Hornung V. DNA-Stimulated Cell Death: Implications for Host Defence, Inflammatory Diseases and Cancer. Nat Rev Immunol (2019) 19(3):141–53. doi: 10.1038/s41577-018-0117-0
15. Gong T, Liu L, Jiang W, Zhou R. DAMP-Sensing Receptors in Sterile Inflammation and Inflammatory Diseases. Nat Rev Immunol (2020) 20(2):95–112. doi: 10.1038/s41577-019-0215-7
16. Wang H, Hu S, Chen X, Shi H, Chen C, Sun L, et al. cGAS Is Essential for the Antitumor Effect of Immune Checkpoint Blockade. Proc Natl Acad Sci U.S.A. (2017) 114(7):1637–42. doi: 10.1073/pnas.1621363114
17. Deng L, Liang H, Xu M, Yang X, Burnette B, Arina A, et al. STING-Dependent Cytosolic DNA Sensing Promotes Radiation-Induced Type I Interferon-Dependent Antitumor Immunity in Immunogenic Tumors. Immunity (2014) 41(5):843–52. doi: 10.1016/j.immuni.2014.10.019
18. Mackenzie KJ, Carroll P, Martin CA, Murina O, Fluteau A, Simpson DJ, et al. cGAS Surveillance of Micronuclei Links Genome Instability to Innate Immunity. Nature (2017) 548(7668):461–5. doi: 10.1038/nature23449
19. Arina A, Beckett M, Fernandez C, Zheng W, Pitroda S, Chmura SJ, et al. Tumor-Reprogrammed Resident T Cells Resist Radiation to Control Tumors. Nat Commun (2019) 10(1):3959. doi: 10.1038/s41467-019-11906-2
20. Matsumura S, Wang B, Kawashima N, Braunstein S, Badura M, Cameron TO, et al. Radiation-Induced CXCL16 Release by Breast Cancer Cells Attracts Effector T Cells. J Immunol (2008) 181(5):3099–107. doi: 10.4049/jimmunol.181.5.3099
21. Yoon MS, Pham CT, Phan MT, Shin DJ, Jang YY, Park MH, et al. Irradiation of Breast Cancer Cells Enhances CXCL16 Ligand Expression and Induces the Migration of Natural Killer Cells Expressing the CXCR6 Receptor. Cytotherapy (2016) 18(12):1532–42. doi: 10.1016/j.jcyt.2016.08.006
22. Punnanitinont A, Kannisto ED, Matsuzaki J, Odunsi K, Yendamuri S, Singh AK, et al. Sublethal Radiation Affects Antigen Processing and Presentation Genes to Enhance Immunogenicity of Cancer Cells. Int J Mol Sci (2020) 21(7):2573. doi: 10.3390/ijms21072573
23. Reits EA, Hodge JW, Herberts CA, Groothuis TA, Chakraborty M, Wansley EK, et al. Radiation Modulates the Peptide Repertoire, Enhances MHC Class I Expression, and Induces Successful Antitumor Immunotherapy. J Exp Med (2006) 203(5):1259–71. doi: 10.1084/jem.20052494
24. Chang MC, Chen YL, Lin HW, Chiang YC, Chang CF, Hsieh SF, et al. Irradiation Enhances Abscopal Anti-Tumor Effects of Antigen-Specific Immunotherapy Through Regulating Tumor Microenvironment. Mol Ther (2018) 26(2):404–19. doi: 10.1016/j.ymthe.2017.11.011
25. Dovedi SJ, Cheadle EJ, Popple AL, Poon E, Morrow M, Stewart R, et al. Fractionated Radiation Therapy Stimulates Antitumor Immunity Mediated by Both Resident and Infiltrating Polyclonal T-Cell Populations When Combined With PD-1 Blockade. Clin Cancer Res (2017) 23(18):5514–26. doi: 10.1158/1078-0432.Ccr-16-1673
26. Twyman-Saint Victor C, Rech AJ, Maity A, Rengan R, Pauken KE, Stelekati E, et al. Radiation and Dual Checkpoint Blockade Activate non-Redundant Immune Mechanisms in Cancer. Nature (2015) 520(7547):373–7. doi: 10.1038/nature14292
27. Rudqvist NP, Pilones KA, Lhuillier C, Wennerberg E, Sidhom JW, Emerson RO, et al. Radiotherapy and CTLA-4 Blockade Shape the TCR Repertoire of Tumor-Infiltrating T Cells. Cancer Immunol Res (2018) 6(2):139–50. doi: 10.1158/2326-6066.Cir-17-0134
28. Dovedi SJ, Adlard AL, Lipowska-Bhalla G, McKenna C, Jones S, Cheadle EJ, et al. Acquired Resistance to Fractionated Radiotherapy can be Overcome by Concurrent PD-L1 Blockade. Cancer Res (2014) 74(19):5458–68. doi: 10.1158/0008-5472.Can-14-1258
29. Gong X, Li X, Jiang T, Xie H, Zhu Z, Zhou F, et al. Combined Radiotherapy and Anti-PD-L1 Antibody Synergistically Enhances Antitumor Effect in Non-Small Cell Lung Cancer. J Thorac Oncol (2017) 12(7):1085–97. doi: 10.1016/j.jtho.2017.04.014
30. Muroyama Y, Nirschl TR, Kochel CM, Lopez-Bujanda Z, Theodros D, Mao W, et al. Stereotactic Radiotherapy Increases Functionally Suppressive Regulatory T Cells in the Tumor Microenvironment. Cancer Immunol Res (2017) 5(11):992–1004. doi: 10.1158/2326-6066.Cir-17-0040
31. Farhood B, Khodamoradi E, Hoseini-Ghahfarokhi M, Motevaseli E, Mirtavoos-Mahyari H, Eleojo Musa A, et al. TGF-β in Radiotherapy: Mechanisms of Tumor Resistance and Normal Tissues Injury. Pharmacol Res (2020) 155:104745. doi: 10.1016/j.phrs.2020.104745
32. Zhu J, Powis de Tenbossche CG, Cané S, Colau D, van Baren N, Lurquin C, et al. Resistance to Cancer Immunotherapy Mediated by Apoptosis of Tumor-Infiltrating Lymphocytes. Nat Commun (2017) 8(1):1404. doi: 10.1038/s41467-017-00784-1
33. Jones KI, Tiersma J, Yuzhalin AE, Gordon-Weeks AN, Buzzelli J, Im JH, et al. Radiation Combined With Macrophage Depletion Promotes Adaptive Immunity and Potentiates Checkpoint Blockade. EMBO Mol Med (2018) 10(12):e9342. doi: 10.15252/emmm.201809342
34. Rube CE, Uthe D, Schmid KW, Richter KD, Wessel J, Schuck A, et al. Dose-Dependent Induction of Transforming Growth Factor Beta (TGF-Beta) in the Lung Tissue of Fibrosis-Prone Mice After Thoracic Irradiation. Int J Radiat Oncol Biol Phys (2000) 47(4):1033–42. doi: 10.1016/s0360-3016(00)00482-x
35. Martinez-Zubiaurre I, Chalmers AJ, Hellevik T. Radiation-Induced Transformation of Immunoregulatory Networks in the Tumor Stroma. Front Immunol (2018) 9:1679. doi: 10.3389/fimmu.2018.01679
36. Barsoumian HB, Ramapriyan R, Younes AI, Caetano MS, Menon H, Comeaux NI, et al. Low-Dose Radiation Treatment Enhances Systemic Antitumor Immune Responses by Overcoming the Inhibitory Stroma. J Immunother Cancer (2020) 8(2):e000537. doi: 10.1136/jitc-2020-000537
37. Vanpouille-Box C, Alard A, Aryankalayil MJ, Sarfraz Y, Diamond JM, Schneider RJ, et al. DNA Exonuclease Trex1 Regulates Radiotherapy-Induced Tumour Immunogenicity. Nat Commun (2017) 8:15618. doi: 10.1038/ncomms15618
38. Formenti SC, Rudqvist NP, Golden E, Cooper B, Wennerberg E, Lhuillier C, et al. Radiotherapy Induces Responses of Lung Cancer to CTLA-4 Blockade. Nat Med (2018) 24(12):1845–51. doi: 10.1038/s41591-018-0232-2
39. Tang C, Welsh JW, de Groot P, Massarelli E, Chang JY, Hess KR, et al. Ipilimumab With Stereotactic Ablative Radiation Therapy: Phase I Results and Immunologic Correlates From Peripheral T Cells. Clin Cancer Res (2017) 23(6):1388–96. doi: 10.1158/1078-0432.Ccr-16-1432
40. Trommer M, Yeo SY, Persigehl T, Bunck A, Grüll H, Schlaak M, et al. Abscopal Effects in Radio-Immunotherapy-Response Analysis of Metastatic Cancer Patients With Progressive Disease Under Anti-PD-1 Immune Checkpoint Inhibition. Front Pharmacol (2019) 10:511. doi: 10.3389/fphar.2019.00511
41. Welsh J, Menon H, Chen D, Verma V, Tang C, Altan M, et al. Pembrolizumab With or Without Radiation Therapy for Metastatic non-Small Cell Lung Cancer: A Randomized Phase I/II Trial. J Immunother Cancer (2020) 8(2):e001001. doi: 10.1136/jitc-2020-001001
42. Theelen W, Peulen HMU, Lalezari F, van der Noort V, de Vries JF, Aerts J, et al. Effect of Pembrolizumab After Stereotactic Body Radiotherapy vs Pembrolizumab Alone on Tumor Response in Patients With Advanced Non-Small Cell Lung Cancer: Results of the PEMBRO-RT Phase 2 Randomized Clinical Trial. JAMA Oncol (2019) 5(9):1276–82. doi: 10.1001/jamaoncol.2019.1478
43. Sett RC, Morales-Orue I, Castilla-Martinez JF, Blanco J, Kannemann A, Zafra J, et al. I-SABR Induces Local and Abscopal Responses in Metastatic Patients After Failure to ICI Treatment. Radiother Oncol (2019) 133:S24–S. doi: 10.1016/s0167-8140(19)30480-3
44. Habets TH, Oth T, Houben AW, Huijskens MJ, Senden-Gijsbers BL, Schnijderberg MC, et al. Fractionated Radiotherapy With 3 X 8 Gy Induces Systemic Anti-Tumour Responses and Abscopal Tumour Inhibition Without Modulating the Humoral Anti-Tumour Response. PloS One (2016) 11(7):e0159515. doi: 10.1371/journal.pone.0159515
45. Akutsu Y, Matsubara H, Urashima T, Komatsu A, Sakata H, Nishimori T, et al. Combination of Direct Intratumoral Administration of Dendritic Cells and Irradiation Induces Strong Systemic Antitumor Effect Mediated by GRP94/gp96 Against Squamous Cell Carcinoma in Mice. Int J Oncol (2007) 31(3):509–15. doi: 10.3892/ijo.31.3.509
46. Golden EB, Chhabra A, Chachoua A, Adams S, Donach M, Fenton-Kerimian M, et al. Local Radiotherapy and Granulocyte-Macrophage Colony-Stimulating Factor to Generate Abscopal Responses in Patients With Metastatic Solid Tumours: A Proof-of-Principle Trial. Lancet Oncol (2015) 16(7):795–803. doi: 10.1016/s1470-2045(15)00054-6
47. Demaria S, Kawashima N, Yang AM, Devitt ML, Babb JS, Allison JP, et al. Immune-Mediated Inhibition of Metastases After Treatment With Local Radiation and CTLA-4 Blockade in a Mouse Model of Breast Cancer. Clin Cancer Res (2005) 11(2 Pt 1):728–34.
48. Dewan MZ, Galloway AE, Kawashima N, Dewyngaert JK, Babb JS, Formenti SC, et al. Fractionated But Not Single-Dose Radiotherapy Induces an Immune-Mediated Abscopal Effect When Combined With Anti-CTLA-4 Antibody. Clin Cancer Res (2009) 15(17):5379–88. doi: 10.1158/1078-0432.Ccr-09-0265
49. Antonia SJ, Villegas A, Daniel D, Vicente D, Murakami S, Hui R, et al. Overall Survival With Durvalumab After Chemoradiotherapy in Stage III NSCLC. N Engl J Med (2018) 379(24):2342–50. doi: 10.1056/NEJMoa1809697
50. Antonia SJ, Villegas A, Daniel D, Vicente D, Murakami S, Hui R, et al. Durvalumab After Chemoradiotherapy in Stage III Non-Small-Cell Lung Cancer. N Engl J Med (2017) 377(20):1919–29. doi: 10.1056/NEJMoa1709937
51. Durm GA, Jabbour SK, Althouse SK, Liu Z, Sadiq AA, Zon RT, et al. A Phase 2 Trial of Consolidation Pembrolizumab Following Concurrent Chemoradiation for Patients With Unresectable Stage III non-Small Cell Lung Cancer: Hoosier Cancer Research Network LUN 14-179. Cancer (2020) 126(19):4353–61. doi: 10.1002/cncr.33083
52. Lin S, Lin X, Clay D, Yao L, Mok I, Gomez D, et al. DETERRED: Phase II Trial Combining Atezolizumab Concurrently With Chemoradiation Therapy in Locally Advanced Non-Small Cell Lung Cancer. J Thorac Oncol (2018) 13(10):S320–S1. doi: 10.1016/j.jtho.2018.08.237
53. Peters S, Felip E, Dafni U, Belka C, Guckenberger M, Irigoyen A, et al. Safety Evaluation of Nivolumab Added Concurrently to Radiotherapy in a Standard First Line Chemo-Radiotherapy Regimen in Stage III non-Small Cell Lung Cancer-The ETOP NICOLAS Trial. Lung Cancer (2019) 133:83–7. doi: 10.1016/j.lungcan.2019.05.001
54. Chalkidou A, Macmillan T, Grzeda MT, Peacock J, Summers J, Eddy S, et al. Stereotactic Ablative Body Radiotherapy in Patients With Oligometastatic Cancers: A Prospective, Registry-Based, Single-Arm, Observational, Evaluation Study. Lancet Oncol (2021) 22(1):98–106. doi: 10.1016/s1470-2045(20)30537-4
55. Ball D, Mai GT, Vinod S, Babington S, Ruben J, Kron T, et al. Stereotactic Ablative Radiotherapy Versus Standard Radiotherapy in Stage 1 non-Small-Cell Lung Cancer (TROG 09.02 CHISEL): A Phase 3, Open-Label, Randomised Controlled Trial. Lancet Oncol (2019) 20(4):494–503. doi: 10.1016/s1470-2045(18)30896-9
56. Frick MA, Feigenberg SJ, Jean-Baptiste SR, Aguarin LA, Mendes A, Chinniah C, et al. Circulating Tumor Cells Are Associated With Recurrent Disease in Patients With Early-Stage Non-Small Cell Lung Cancer Treated With Stereotactic Body Radiotherapy. Clin Cancer Res (2020) 26(10):2372–80. doi: 10.1158/1078-0432.Ccr-19-2158
57. Abuodeh Y, Venkat P, Kim S. Systematic Review of Case Reports on the Abscopal Effect. Curr Probl Cancer (2016) 40(1):25–37. doi: 10.1016/j.currproblcancer.2015.10.001
58. Menon H, Chen D, Ramapriyan R, Verma V, Barsoumian HB, Cushman TR, et al. Influence of Low-Dose Radiation on Abscopal Responses in Patients Receiving High-Dose Radiation and Immunotherapy. J Immunother Cancer (2019) 7(1):237. doi: 10.1186/s40425-019-0718-6
59. Welsh JW, Tang C, de Groot P, Naing A, Hess KR, Heymach JV, et al. Phase II Trial of Ipilimumab With Stereotactic Radiation Therapy for Metastatic Disease: Outcomes, Toxicities, and Low-Dose Radiation-Related Abscopal Responses. Cancer Immunol Res (2019) 7(12):1903–9. doi: 10.1158/2326-6066.Cir-18-0793
60. Golden EB, Demaria S, Schiff PB, Chachoua A, Formenti SC. An Abscopal Response to Radiation and Ipilimumab in a Patient With Metastatic non-Small Cell Lung Cancer. Cancer Immunol Res (2013) 1(6):365–72. doi: 10.1158/2326-6066.Cir-13-0115
61. Durm G, Althouse S, Sadiq A, Jalal S, Jabbour S, Zon R, et al. Updated Results of a Phase II Trial of Concurrent Chemoradiation With Consolidation Pembrolizumab in Patients With Unresectable Stage III NSCLC. J Thorac Oncol (2018) 13(10):S321–S. doi: 10.1016/j.jtho.2018.08.238
62. Postow MA, Callahan MK, Barker CA, Yamada Y, Yuan J, Kitano S, et al. Immunologic Correlates of the Abscopal Effect in a Patient With Melanoma. N Engl J Med (2012) 366(10):925–31. doi: 10.1056/NEJMoa1112824
63. Buchwald ZS, Nasti TH, Lee J, Eberhardt CS, Wieland A, Im SJ, et al. Tumor-Draining Lymph Node Is Important for a Robust Abscopal Effect Stimulated by Radiotherapy. J Immunother Cancer (2020) 8(2):e000867. doi: 10.1136/jitc-2020-000867
64. Brooks ED, Chang JY. Time to Abandon Single-Site Irradiation for Inducing Abscopal Effects. Nat Rev Clin Oncol (2019) 16(2):123–35. doi: 10.1038/s41571-018-0119-7
65. Reck M, Rodríguez-Abreu D, Robinson AG, Hui R, Csőszi T, Fülöp A, et al. Pembrolizumab Versus Chemotherapy for PD-L1-Positive Non-Small-Cell Lung Cancer. N Engl J Med (2016) 375(19):1823–33. doi: 10.1056/NEJMoa1606774
66. Mok TSK, Wu YL, Kudaba I, Kowalski DM, Cho BC, Turna HZ, et al. Pembrolizumab Versus Chemotherapy for Previously Untreated, PD-L1-Expressing, Locally Advanced or Metastatic non-Small-Cell Lung Cancer (KEYNOTE-042): A Randomised, Open-Label, Controlled, Phase 3 Trial. Lancet (2019) 393(10183):1819–30. doi: 10.1016/s0140-6736(18)32409-7
67. Borghaei H, Paz-Ares L, Horn L, Spigel DR, Steins M, Ready NE, et al. Nivolumab Versus Docetaxel in Advanced Nonsquamous Non-Small-Cell Lung Cancer. N Engl J Med (2015) 373(17):1627–39. doi: 10.1056/NEJMoa1507643
68. Tokito T, Azuma K, Kawahara A, Ishii H, Yamada K, Matsuo N, et al. Predictive Relevance of PD-L1 Expression Combined With CD8+ TIL Density in Stage III non-Small Cell Lung Cancer Patients Receiving Concurrent Chemoradiotherapy. Eur J Cancer (2016) 55:7–14. doi: 10.1016/j.ejca.2015.11.020
69. Yoneda K, Kuwata T, Kanayama M, Mori M, Kawanami T, Yatera K, et al. Alteration in Tumoural PD-L1 Expression and Stromal CD8-Positive Tumour-Infiltrating Lymphocytes After Concurrent Chemo-Radiotherapy for non-Small Cell Lung Cancer. Br J Cancer (2019) 121(6):490–6. doi: 10.1038/s41416-019-0541-3
70. Gataa I, Mezquita L, Rossoni C, Auclin E, Kossai M, Aboubakar F, et al. Tumour-Infiltrating Lymphocyte Density Is Associated With Favourable Outcome in Patients With Advanced non-Small Cell Lung Cancer Treated With Immunotherapy. Eur J Cancer (2021) 145:221–9. doi: 10.1016/j.ejca.2020.10.017
71. Wu SP, Liao RQ, Tu HY, Wang WJ, Dong ZY, Huang SM, et al. Stromal PD-L1-Positive Regulatory T Cells and PD-1-Positive CD8-Positive T Cells Define the Response of Different Subsets of Non-Small Cell Lung Cancer to PD-1/PD-L1 Blockade Immunotherapy. J Thorac Oncol (2018) 13(4):521–32. doi: 10.1016/j.jtho.2017.11.132
72. Mazzaschi G, Madeddu D, Falco A, Bocchialini G, Goldoni M, Sogni F, et al. Low PD-1 Expression in Cytotoxic CD8(+) Tumor-Infiltrating Lymphocytes Confers an Immune-Privileged Tissue Microenvironment in NSCLC With a Prognostic and Predictive Value. Clin Cancer Res (2018) 24(2):407–19. doi: 10.1158/1078-0432.Ccr-17-2156
73. Althammer S, Tan TH, Spitzmüller A, Rognoni L, Wiestler T, Herz T, et al. Automated Image Analysis of NSCLC Biopsies to Predict Response to Anti-PD-L1 Therapy. J Immunother Cancer (2019) 7(1):121. doi: 10.1186/s40425-019-0589-x
74. Ott PA, Bang YJ, Piha-Paul SA, Razak ARA, Bennouna J, Soria JC, et al. T-Cell-Inflamed Gene-Expression Profile, Programmed Death Ligand 1 Expression, and Tumor Mutational Burden Predict Efficacy in Patients Treated With Pembrolizumab Across 20 Cancers: KEYNOTE-028. J Clin Oncol (2019) 37(4):318–27. doi: 10.1200/jco.2018.78.2276
75. Higgs BW, Morehouse CA, Streicher K, Brohawn PZ, Pilataxi F, Gupta A, et al. Interferon Gamma Messenger RNA Signature in Tumor Biopsies Predicts Outcomes in Patients With Non-Small Cell Lung Carcinoma or Urothelial Cancer Treated With Durvalumab. Clin Cancer Res (2018) 24(16):3857–66. doi: 10.1158/1078-0432.Ccr-17-3451
76. Brogden KA, Parashar D, Hallier AR, Braun T, Qian F, Rizvi NA, et al. Genomics of NSCLC Patients Both Affirm PD-L1 Expression and Predict Their Clinical Responses to Anti-PD-1 Immunotherapy. BMC Cancer (2018) 18(1):225. doi: 10.1186/s12885-018-4134-y
77. Thompson JC, Davis C, Deshpande C, Hwang WT, Jeffries S, Huang A, et al. Gene Signature of Antigen Processing and Presentation Machinery Predicts Response to Checkpoint Blockade in non-Small Cell Lung Cancer (NSCLC) and Melanoma. J Immunother Cancer (2020) 8(2):e000974. doi: 10.1136/jitc-2020-000974
78. Thompson JC, Hwang WT, Davis C, Deshpande C, Jeffries S, Rajpurohit Y, et al. Gene Signatures of Tumor Inflammation and Epithelial-to-Mesenchymal Transition (EMT) Predict Responses to Immune Checkpoint Blockade in Lung Cancer With High Accuracy. Lung Cancer (2020) 139:1–8. doi: 10.1016/j.lungcan.2019.10.012
79. Kim HS, Kim SC, Kim SJ, Park CH, Jeung HC, Kim YB, et al. Identification of a Radiosensitivity Signature Using Integrative Metaanalysis of Published Microarray Data for NCI-60 Cancer Cells. BMC Genomics (2012) 13:348. doi: 10.1186/1471-2164-13-348
80. Jang BS, Kim IA. A Radiosensitivity Gene Signature and PD-L1 Predict the Clinical Outcomes of Patients With Lower Grade Glioma in TCGA. Radiother Oncol (2018) 128(2):245–53. doi: 10.1016/j.radonc.2018.05.003
81. Jang BS. Kim IA. A Radiosensitivity Gene Signature and PD-L1 Status Predict Clinical Outcome of Patients With Glioblastoma Multiforme in The Cancer Genome Atlas Dataset. Cancer Res Treat (2020) 52(2):530–42. doi: 10.4143/crt.2019.440
82. Cui Y, Li B, Pollom EL, Horst KC, Li R. Integrating Radiosensitivity and Immune Gene Signatures for Predicting Benefit of Radiotherapy in Breast Cancer. Clin Cancer Res (2018) 24(19):4754–62. doi: 10.1158/1078-0432.Ccr-18-0825
83. Rizvi NA, Hellmann MD, Snyder A, Kvistborg P, Makarov V, Havel JJ, et al. Cancer Immunology. Mutational Landscape Determines Sensitivity to PD-1 Blockade in non-Small Cell Lung Cancer. Science (2015) 348(6230):124–8. doi: 10.1126/science.aaa1348
84. Carbone DP, Reck M, Paz-Ares L, Creelan B, Horn L, Steins M, et al. First-Line Nivolumab in Stage IV or Recurrent Non-Small-Cell Lung Cancer. N Engl J Med (2017) 376(25):2415–26. doi: 10.1056/NEJMoa1613493
85. Kowanetz M, Zou W, Shames D, Cummings C, Rizvi N, Spira A, et al. Tumor Mutation Burden (TMB) Is Associated With Improved Efficacy of Atezolizumab in 1L and 2L+NSCLC Patients. J Thorac Oncol (2017) 12(1):S321–S2. doi: 10.1016/j.jtho.2016.11.343
86. Jia Q, Chu Q, Zhang A, Yu J, Liu F, Qian K, et al. Mutational Burden and Chromosomal Aneuploidy Synergistically Predict Survival From Radiotherapy in non-Small Cell Lung Cancer. Commun Biol (2021) 4(1):131. doi: 10.1038/s42003-021-01657-6
87. Grimaldi AM, Simeone E, Giannarelli D, Muto P, Falivene S, Borzillo V, et al. Abscopal Effects of Radiotherapy on Advanced Melanoma Patients Who Progressed After Ipilimumab Immunotherapy. Oncoimmunology (2014) 3:e28780. doi: 10.4161/onci.28780
88. Tanizaki J, Haratani K, Hayashi H, Chiba Y, Nakamura Y, Yonesaka K, et al. Peripheral Blood Biomarkers Associated With Clinical Outcome in Non-Small Cell Lung Cancer Patients Treated With Nivolumab. J Thorac Oncol (2018) 13(1):97–105. doi: 10.1016/j.jtho.2017.10.030
89. Kamphorst AO, Pillai RN, Yang S, Nasti TH, Akondy RS, Wieland A, et al. Proliferation of PD-1+ CD8 T Cells in Peripheral Blood After PD-1-Targeted Therapy in Lung Cancer Patients. Proc Natl Acad Sci USA (2017) 114(19):4993–8. doi: 10.1073/pnas.1705327114
90. Cho YH, Choi MG, Kim DH, Choi YJ, Kim SY, Sung KJ, et al. Natural Killer Cells as a Potential Biomarker for Predicting Immunotherapy Efficacy in Patients With Non-Small Cell Lung Cancer. Target Oncol (2020) 15(2):241–7. doi: 10.1007/s11523-020-00712-2
91. Sebastian N, Wu T, Bazan J, Driscoll E, Willers H, Yegya-Raman N, et al. Pre-Treatment Neutrophil-Lymphocyte Ratio Is Associated With Overall Mortality in Localized non-Small Cell Lung Cancer Treated With Stereotactic Body Radiotherapy. Radiother Oncol (2019) 134:151–7. doi: 10.1016/j.radonc.2019.01.032
92. Chowdhary M, Switchenko JM, Press RH, Jhaveri J, Buchwald ZS, Blumenfeld PA, et al. Post-Treatment Neutrophil-to-Lymphocyte Ratio Predicts for Overall Survival in Brain Metastases Treated With Stereotactic Radiosurgery. J Neurooncol (2018) 139(3):689–97. doi: 10.1007/s11060-018-2914-5
93. Mezquita L, Auclin E, Ferrara R, Charrier M, Remon J, Planchard D, et al. Association of the Lung Immune Prognostic Index With Immune Checkpoint Inhibitor Outcomes in Patients With Advanced Non-Small Cell Lung Cancer. JAMA Oncol (2018) 4(3):351–7. doi: 10.1001/jamaoncol.2017.4771
94. Ruiz-Bañobre J, Areses-Manrique MC, Mosquera-Martínez J, Cortegoso A, Afonso-Afonso FJ, de Dios-Álvarez N, et al. Evaluation of the Lung Immune Prognostic Index in Advanced non-Small Cell Lung Cancer Patients Under Nivolumab Monotherapy. Transl Lung Cancer Res (2019) 8(6):1078–85. doi: 10.21037/tlcr.2019.11.07
95. Kazandjian D, Gong Y, Keegan P, Pazdur R, Blumenthal GM. Prognostic Value of the Lung Immune Prognostic Index for Patients Treated for Metastatic Non-Small Cell Lung Cancer. JAMA Oncol (2019) 5(10):1481–5. doi: 10.1001/jamaoncol.2019.1747
96. Valero C, Lee M, Hoen D, Weiss K, Kelly DW, Adusumilli PS, et al. Pretreatment Neutrophil-to-Lymphocyte Ratio and Mutational Burden as Biomarkers of Tumor Response to Immune Checkpoint Inhibitors. Nat Commun (2021) 12(1):729. doi: 10.1038/s41467-021-20935-9
97. Anagnostou V, Forde PM, White JR, Niknafs N, Hruban C, Naidoo J, et al. Dynamics of Tumor and Immune Responses During Immune Checkpoint Blockade in Non-Small Cell Lung Cancer. Cancer Res (2019) 79(6):1214–25. doi: 10.1158/0008-5472.Can-18-1127
98. Goldberg SB, Narayan A, Kole AJ, Decker RH, Teysir J, Carriero NJ, et al. Early Assessment of Lung Cancer Immunotherapy Response via Circulating Tumor DNA. Clin Cancer Res (2018) 24(8):1872–80. doi: 10.1158/1078-0432.Ccr-17-1341
99. Passiglia F, Galvano A, Castiglia M, Incorvaia L, Calò V, Listì A, et al. Monitoring Blood Biomarkers to Predict Nivolumab Effectiveness in NSCLC Patients. Ther Adv Med Oncol (2019) 11:1758835919839928. doi: 10.1177/1758835919839928
100. Boeri M, Milione M, Proto C, Signorelli D, Lo Russo G, Galeone C, et al. Circulating miRNAs and PD-L1 Tumor Expression Are Associated With Survival in Advanced NSCLC Patients Treated With Immunotherapy: A Prospective Study. Clin Cancer Res (2019) 25(7):2166–73. doi: 10.1158/1078-0432.Ccr-18-1981
101. Seban RD, Mezquita L, Berenbaum A, Dercle L, Botticella A, Le Pechoux C, et al. Baseline Metabolic Tumor Burden on FDG PET/CT Scans Predicts Outcome in Advanced NSCLC Patients Treated With Immune Checkpoint Inhibitors. Eur J Nucl Med Mol Imaging (2020) 47(5):1147–57. doi: 10.1007/s00259-019-04615-x
102. Castello A, Toschi L, Rossi S, Mazziotti E, Lopci E. The Immune-Metabolic-Prognostic Index and Clinical Outcomes in Patients With non-Small Cell Lung Carcinoma Under Checkpoint Inhibitors. J Cancer Res Clin Oncol (2020) 146(5):1235–43. doi: 10.1007/s00432-020-03150-9
103. Mazzola R, Fiorentino A, Di Paola G, Giaj Levra N, Ricchetti F, Fersino S, et al. Stereotactic Ablative Radiation Therapy for Lung Oligometastases: Predictive Parameters of Early Response by (18)FDG-PET/CT. J Thorac Oncol (2017) 12(3):547–55. doi: 10.1016/j.jtho.2016.11.2234
104. Chin AL, Kumar KA, Guo HH, Maxim PG, Wakelee H, Neal JW, et al. Prognostic Value of Pretreatment FDG-PET Parameters in High-Dose Image-Guided Radiotherapy for Oligometastatic Non-Small-Cell Lung Cancer. Clin Lung Cancer (2018) 19(5):e581–e8. doi: 10.1016/j.cllc.2018.04.003
105. Okazaki E, Seura H, Hasegawa Y, Okamura T, Fukuda H. Prognostic Value of the Volumetric Parameters of Dual-Time-Point (18)F-FDG PET/CT in Non-Small Cell Lung Cancer Treated With Definitive Radiation Therapy. AJR Am J Roentgenol (2019) 213(6):1366–73. doi: 10.2214/ajr.19.21376
106. Lovinfosse P, Janvary ZL, Coucke P, Jodogne S, Bernard C, Hatt M, et al. FDG PET/CT Texture Analysis for Predicting the Outcome of Lung Cancer Treated by Stereotactic Body Radiation Therapy. Eur J Nucl Med Mol Imaging (2016) 43(8):1453–60. doi: 10.1007/s00259-016-3314-8
107. Dissaux G, Visvikis D, Da-Ano R, Pradier O, Chajon E, Barillot I, et al. Pretreatment (18)F-FDG PET/CT Radiomics Predict Local Recurrence in Patients Treated With Stereotactic Body Radiotherapy for Early-Stage Non-Small Cell Lung Cancer: A Multicentric Study. J Nucl Med (2020) 61(6):814–20. doi: 10.2967/jnumed.119.228106
108. Prelaj A, Tay R, Ferrara R, Chaput N, Besse B, Califano R. Predictive Biomarkers of Response for Immune Checkpoint Inhibitors in non-Small-Cell Lung Cancer. Eur J Cancer (2019) 106:144–59. doi: 10.1016/j.ejca.2018.11.002
109. Rittmeyer A, Barlesi F, Waterkamp D, Park K, Ciardiello F, von Pawel J, et al. Atezolizumab Versus Docetaxel in Patients With Previously Treated non-Small-Cell Lung Cancer (OAK): A Phase 3, Open-Label, Multicentre Randomised Controlled Trial. Lancet (2017) 389(10066):255–65. doi: 10.1016/s0140-6736(16)32517-x
110. Spigel DR, Chaft JE, Gettinger S, Chao BH, Dirix L, Schmid P, et al. FIR: Efficacy, Safety, and Biomarker Analysis of a Phase II Open-Label Study of Atezolizumab in PD-L1-Selected Patients With NSCLC. J Thorac Oncol (2018) 13(11):1733–42. doi: 10.1016/j.jtho.2018.05.004
111. Wang Y, Kim TH, Fouladdel S, Zhang Z, Soni P, Qin A, et al. PD-L1 Expression in Circulating Tumor Cells Increases During Radio(chemo)therapy and Indicates Poor Prognosis in Non-Small Cell Lung Cancer. Sci Rep (2019) 9(1):566. doi: 10.1038/s41598-018-36096-7
112. Sui X, Jiang L, Teng H, Mi L, Li B, Shi A, et al. Prediction of Clinical Outcome in Locally Advanced Non-Small Cell Lung Cancer Patients Treated With Chemoradiotherapy by Plasma Markers. Front Oncol (2020) 10:625911. doi: 10.3389/fonc.2020.625911
113. Mansfield AS, Aubry MC, Moser JC, Harrington SM, Dronca RS, Park SS, et al. Temporal and Spatial Discordance of Programmed Cell Death-Ligand 1 Expression and Lymphocyte Tumor Infiltration Between Paired Primary Lesions and Brain Metastases in Lung Cancer. Ann Oncol (2016) 27(10):1953–8. doi: 10.1093/annonc/mdw289
114. Zhou J, Gong Z, Jia Q, Wu Y, Yang ZZ, Zhu B. Programmed Death Ligand 1 Expression and CD8(+) Tumor-Infiltrating Lymphocyte Density Differences Between Paired Primary and Brain Metastatic Lesions in non-Small Cell Lung Cancer. Biochem Biophys Res Commun (2018) 498(4):751–7. doi: 10.1016/j.bbrc.2018.03.053
115. Tsao MS, Kerr KM, Kockx M, Beasley MB, Borczuk AC, Botling J, et al. PD-L1 Immunohistochemistry Comparability Study in Real-Life Clinical Samples: Results of Blueprint Phase 2 Project. J Thorac Oncol (2018) 13(9):1302–11. doi: 10.1016/j.jtho.2018.05.013
116. Miller BC, Sen DR, Al Abosy R, Bi K, Virkud YV, LaFleur MW, et al. Subsets of Exhausted CD8(+) T Cells Differentially Mediate Tumor Control and Respond to Checkpoint Blockade. Nat Immunol (2019) 20(3):326–36. doi: 10.1038/s41590-019-0312-6
117. Pagès F, Mlecnik B, Marliot F, Bindea G, Ou FS, Bifulco C, et al. International Validation of the Consensus Immunoscore for the Classification of Colon Cancer: A Prognostic and Accuracy Study. Lancet (2018) 391(10135):2128–39. doi: 10.1016/s0140-6736(18)30789-x
118. Kalathil SG, Thanavala Y. Importance of Myeloid Derived Suppressor Cells in Cancer From a Biomarker Perspective. Cell Immunol (2021) 361:104280. doi: 10.1016/j.cellimm.2020.104280
119. Shang B, Liu Y, Jiang SJ, Liu Y. Prognostic Value of Tumor-Infiltrating FoxP3+ Regulatory T Cells in Cancers: A Systematic Review and Meta-Analysis. Sci Rep (2015) 5:15179. doi: 10.1038/srep15179
120. Corredor G, Wang X, Zhou Y, Lu C, Fu P, Syrigos K, et al. Spatial Architecture and Arrangement of Tumor-Infiltrating Lymphocytes for Predicting Likelihood of Recurrence in Early-Stage Non-Small Cell Lung Cancer. Clin Cancer Res (2019) 25(5):1526–34. doi: 10.1158/1078-0432.Ccr-18-2013
121. Kinoshita T, Muramatsu R, Fujita T, Nagumo H, Sakurai T, Noji S, et al. Prognostic Value of Tumor-Infiltrating Lymphocytes Differs Depending on Histological Type and Smoking Habit in Completely Resected non-Small-Cell Lung Cancer. Ann Oncol (2016) 27(11):2117–23. doi: 10.1093/annonc/mdw319
122. Cristescu R, Mogg R, Ayers M, Albright A, Murphy E, Yearley J, et al. Pan-Tumor Genomic Biomarkers for PD-1 Checkpoint Blockade-Based Immunotherapy. Science (2018) 362(6411):eaar3592. doi: 10.1126/science.aar3593
123. Danaher P, Warren S, Lu R, Samayoa J, Sullivan A, Pekker I, et al. Pan-Cancer Adaptive Immune Resistance as Defined by the Tumor Inflammation Signature (TIS): Results From The Cancer Genome Atlas (TCGA). J Immunother Cancer (2018) 6(1):63. doi: 10.1186/s40425-018-0367-1
124. Ayers M, Lunceford J, Nebozhyn M, Murphy E, Loboda A, Kaufman DR, et al. IFN-γ-Related mRNA Profile Predicts Clinical Response to PD-1 Blockade. J Clin Invest (2017) 127(8):2930–40. doi: 10.1172/jci91190
125. Prat A, Navarro A, Paré L, Reguart N, Galván P, Pascual T, et al. Immune-Related Gene Expression Profiling After PD-1 Blockade in Non-Small Cell Lung Carcinoma, Head and Neck Squamous Cell Carcinoma, and Melanoma. Cancer Res (2017) 77(13):3540–50. doi: 10.1158/0008-5472.Can-16-3556
126. Torres-Roca JF, Eschrich S, Zhao H, Bloom G, Sung J, McCarthy S, et al. Prediction of Radiation Sensitivity Using a Gene Expression Classifier. Cancer Res (2005) 65(16):7169–76. doi: 10.1158/0008-5472.Can-05-0656
127. Jang BS, Kim IA. A Radiosensitivity Gene Signature and PD-L1 Status Predict Clinical Outcome of Patients With Invasive Breast Carcinoma in The Cancer Genome Atlas (TCGA) Dataset. Radiother Oncol (2017) 124(3):403–10. doi: 10.1016/j.radonc.2017.05.009
128. Scott JG, Berglund A, Schell MJ, Mihaylov I, Fulp WJ, Yue B, et al. A Genome-Based Model for Adjusting Radiotherapy Dose (GARD): A Retrospective, Cohort-Based Study. Lancet Oncol (2017) 18(2):202–11. doi: 10.1016/s1470-2045(16)30648-9
129. Eschrich S, Zhang H, Zhao H, Boulware D, Lee JH, Bloom G, et al. Systems Biology Modeling of the Radiation Sensitivity Network: A Biomarker Discovery Platform. Int J Radiat Oncol Biol Phys (2009) 75(2):497–505. doi: 10.1016/j.ijrobp.2009.05.056
130. Eder T, Hess AK, Konschak R, Stromberger C, Jöhrens K, Fleischer V, et al. Interference of Tumour Mutational Burden With Outcome of Patients With Head and Neck Cancer Treated With Definitive Chemoradiation: A Multicentre Retrospective Study of the German Cancer Consortium Radiation Oncology Group. Eur J Cancer (2019) 116:67–76. doi: 10.1016/j.ejca.2019.04.015
131. Liu X, Yao J, Song L, Zhang S, Huang T, Li Y. Local and Abscopal Responses in Advanced Intrahepatic Cholangiocarcinoma With Low TMB, MSS, pMMR and Negative PD-L1 Expression Following Combined Therapy of SBRT With PD-1 Blockade. J Immunother Cancer (2019) 7(1):204. doi: 10.1186/s40425-019-0692-z
132. Chalmers ZR, Connelly CF, Fabrizio D, Gay L, Ali SM, Ennis R, et al. Analysis of 100,000 Human Cancer Genomes Reveals the Landscape of Tumor Mutational Burden. Genome Med (2017) 9(1):34. doi: 10.1186/s13073-017-0424-2
133. Rizvi H, Sanchez-Vega F, La K, Chatila W, Jonsson P, Halpenny D, et al. Molecular Determinants of Response to Anti-Programmed Cell Death (PD)-1 and Anti-Programmed Death-Ligand 1 (PD-L1) Blockade in Patients With Non-Small-Cell Lung Cancer Profiled With Targeted Next-Generation Sequencing. J Clin Oncol (2018) 36(7):633–41. doi: 10.1200/jco.2017.75.3384
134. Thurin M, Cesano A, Marincola FM eds. Biomarkers for Immunotherapy of Cancer: Methods and Protocols. In: Biomarkers for Immunotherapy of Cancer: Methods and Protocols. 2055. Totowa: Humana Press Inc. p. 1–733. Methods in Molecular Biology.
135. Keller L, Guibert N, Casanova A, Brayer S, Farella M, Delaunay M, et al. Early Circulating Tumour DNA Variations Predict Tumour Response in Melanoma Patients Treated With Immunotherapy. Acta Derm Venereol (2019) 99(2):206–10. doi: 10.2340/00015555-3080
136. Kageyama SI, Nihei K, Karasawa K, Sawada T, Koizumi F, Yamaguchi S, et al. Radiotherapy Increases Plasma Levels of Tumoral Cell-Free DNA in non-Small Cell Lung Cancer Patients. Oncotarget (2018) 9(27):19368–78. doi: 10.18632/oncotarget.25053
137. Walls GM, McConnell L, McAleese J, Murray P, Lynch TB, Savage K, et al. Early Circulating Tumour DNA Kinetics Measured by Ultra-Deep Next-Generation Sequencing During Radical Radiotherapy for non-Small Cell Lung Cancer: A Feasibility Study. Radiat Oncol (2020) 15(1):132. doi: 10.1186/s13014-020-01583-7
138. Li Y, Zhang Z, Hu Y, Yan X, Song Q, Wang G, et al. Pretreatment Neutrophil-To-Lymphocyte Ratio (NLR) May Predict the Outcomes of Advanced Non-Small-Cell Lung Cancer (NSCLC) Patients Treated With Immune Checkpoint Inhibitors (ICIs). Front Oncol (2020) 10:654. doi: 10.3389/fonc.2020.00654
139. Russo A, Franchina T, Ricciardi GRR, Battaglia A, Scimone A, Berenato R, et al. Baseline Neutrophilia, Derived Neutrophil-to-Lymphocyte Ratio (dNLR), Platelet-to-Lymphocyte Ratio (PLR), and Outcome in non Small Cell Lung Cancer (NSCLC) Treated With Nivolumab or Docetaxel. J Cell Physiol (2018) 233(10):6337–43. doi: 10.1002/jcp.26609
140. Soyano AE, Dholaria B, Marin-Acevedo JA, Diehl N, Hodge D, Luo Y, et al. Peripheral Blood Biomarkers Correlate With Outcomes in Advanced non-Small Cell Lung Cancer Patients Treated With Anti-PD-1 Antibodies. J Immunother Cancer (2018) 6(1):129. doi: 10.1186/s40425-018-0447-2
141. He J, Feng X, Hua J, Wei L, Lu Z, Wei W, et al. miR-300 Regulates Cellular Radiosensitivity Through Targeting P53 and Apaf1 in Human Lung Cancer Cells. Cell Cycle (2017) 16(20):1943–53. doi: 10.1080/15384101.2017.1367070
142. Li L, Liu H, Du L, Xi P, Wang Q, Li Y, et al. miR-449a Suppresses LDHA-Mediated Glycolysis to Enhance the Sensitivity of Non-Small Cell Lung Cancer Cells to Ionizing Radiation. Oncol Res (2018) 26(4):547–56. doi: 10.3727/096504017x15016337254605
143. Li H, Jin X, Chen B, Li P, Li Q. Autophagy-Regulating microRNAs: Potential Targets for Improving Radiotherapy. J Cancer Res Clin Oncol (2018) 144(9):1623–34. doi: 10.1007/s00432-018-2675-8
144. Li AL, Chung TS, Chan YN, Chen CL, Lin SC, Chiang YR, et al. microRNA Expression Pattern as an Ancillary Prognostic Signature for Radiotherapy. J Transl Med (2018) 16(1):341. doi: 10.1186/s12967-018-1711-4
145. Fan L, Cao Q, Ding X, Gao D, Yang Q, Li B. Radiotranscriptomics Signature-Based Predictive Nomograms for Radiotherapy Response in Patients With Nonsmall Cell Lung Cancer: Combination and Association of CT Features and Serum miRNAs Levels. Cancer Med (2020) 9(14):5065–74. doi: 10.1002/cam4.3115
146. Halvorsen AR, Sandhu V, Sprauten M, Flote VG, Kure EH, Brustugun OT, et al. Circulating microRNAs Associated With Prolonged Overall Survival in Lung Cancer Patients Treated With Nivolumab. Acta Oncol (2018) 57(9):1225–31. doi: 10.1080/0284186x.2018.1465585
147. Fan J, Yin Z, Xu J, Wu F, Huang Q, Yang L, et al. Circulating microRNAs Predict the Response to Anti-PD-1 Therapy in non-Small Cell Lung Cancer. Genomics (2020) 112(2):2063–71. doi: 10.1016/j.ygeno.2019.11.019
148. Wang K, Zhang S, Weber J, Baxter D, Galas DJ. Export of microRNAs and microRNA-Protective Protein by Mammalian Cells. Nucleic Acids Res (2010) 38(20):7248–59. doi: 10.1093/nar/gkq601
149. Arroyo JD, Chevillet JR, Kroh EM, Ruf IK, Pritchard CC, Gibson DF, et al. Argonaute2 Complexes Carry a Population of Circulating microRNAs Independent of Vesicles in Human Plasma. Proc Natl Acad Sci USA (2011) 108(12):5003–8. doi: 10.1073/pnas.1019055108
150. Chang CH, Qiu J, O’Sullivan D, Buck MD, Noguchi T, Curtis JD, et al. Metabolic Competition in the Tumor Microenvironment Is a Driver of Cancer Progression. Cell (2015) 162(6):1229–41. doi: 10.1016/j.cell.2015.08.016
151. Takada K, Toyokawa G, Okamoto T, Baba S, Kozuma Y, Matsubara T, et al. Metabolic Characteristics of Programmed Cell Death-Ligand 1-Expressing Lung Cancer on (18) F-Fluorodeoxyglucose Positron Emission Tomography/Computed Tomography. Cancer Med (2017) 6(11):2552–61. doi: 10.1002/cam4.1215
Keywords: non-small cell lung cancer (NSCLC), radiotherapy, immunotherapy, immune checkpoint inhibitor (ICI), biomarker
Citation: Meng L, Xu J, Ye Y, Wang Y, Luo S and Gong X (2021) The Combination of Radiotherapy With Immunotherapy and Potential Predictive Biomarkers for Treatment of Non-Small Cell Lung Cancer Patients. Front. Immunol. 12:723609. doi: 10.3389/fimmu.2021.723609
Received: 11 June 2021; Accepted: 03 September 2021;
Published: 21 September 2021.
Edited by:
Douglas Clayton Palmer, AstraZeneca, United StatesReviewed by:
Simon Heeke, University of Texas MD Anderson Cancer Center, United StatesRobert J. Canter, University of California, Davis, United States
Rachel Evans, King’s College London, United Kingdom
Copyright © 2021 Meng, Xu, Ye, Wang, Luo and Gong. This is an open-access article distributed under the terms of the Creative Commons Attribution License (CC BY). The use, distribution or reproduction in other forums is permitted, provided the original author(s) and the copyright owner(s) are credited and that the original publication in this journal is cited, in accordance with accepted academic practice. No use, distribution or reproduction is permitted which does not comply with these terms.
*Correspondence: Xiaomei Gong, Z29uZ3hpYW9tZWkxOTgxQDE2My5jb20=
†These authors have contributed equally to this work