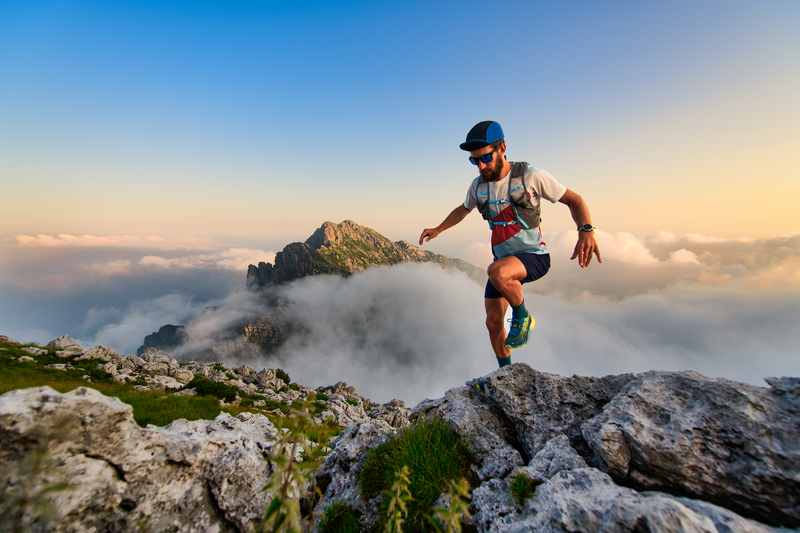
94% of researchers rate our articles as excellent or good
Learn more about the work of our research integrity team to safeguard the quality of each article we publish.
Find out more
ORIGINAL RESEARCH article
Front. Immunol. , 20 August 2021
Sec. Inflammation
Volume 12 - 2021 | https://doi.org/10.3389/fimmu.2021.716357
Lifestyle- and genetically induced disorders related to disturbances in cholesterol metabolism have shown the detrimental impact of excessive cholesterol levels on a plethora of pathological processes such as inflammation. In this context, two-hydroxypropyl-β-cyclodextrin (CD) is increasingly considered as a novel pharmacological compound to decrease cellular cholesterol levels due to its ability to increase cholesterol solubility. However, recent findings have reported contra-indicating events after the use of CD questioning the clinical applicability of this compound. Given its potential as a therapeutic compound in metabolic inflammatory diseases, in this study, we evaluated the inflammatory effects of CD administration in the context of cholesterol-induced metabolic inflammation in vivo and in vitro. The inflammatory and cholesterol-depleting effects of CD were first investigated in low-density lipoprotein receptor knockout (Ldlr-/) mice that were transplanted with Npc1nih or Npc1wt bone marrow and were fed either regular chow or a high-fat, high-cholesterol (HFC) diet for 12 weeks, thereby creating an extreme model of lysosomal cholesterol-induced metabolic inflammation. In the final three weeks, these mice received daily injections of either control (saline) or CD subcutaneously. Subsequently, the inflammatory properties of CD were investigated in vitro in two macrophage cell lines and in murine bone marrow-derived macrophages (BMDMs). While CD administration improved cholesterol mobilization outside lysosomes in BMDMs, an overall pro-inflammatory profile was observed after CD treatment, evidenced by increased hepatic inflammation in vivo and a strong increase in cytokine release and inflammatory gene expression in vitro in murine BMDMs and macrophages cell lines. Nevertheless, this CD-induced pro-inflammatory profile was time-dependent, as short term exposure to CD did not result in a pro-inflammatory response in BMDM. While CD exerts desired cholesterol-depleting effects, its inflammatory effect is dependent on the exposure time. As such, using CD in the clinic, especially in a metabolic inflammatory context, should be closely monitored as it may lead to undesired, pro-inflammatory side effects.
As one of the most important steroid alcohols of the human body, cholesterol carries a number of essential functions ranging from acting as a key structural component of cell membranes (1) to serving as precursor for bile acids, steroid hormones and vitamin D metabolites (2). Due to this key cellular function of cholesterol, a highly coordinated extra- and intracellular transport system maintains cholesterol homeostasis at a whole body as well as at a cellular level (3, 4). Evidently, deficiencies in this transport system leading to a disproportionate cellular supply of cholesterol result in severe pathogenic profiles. At one hand, these deficiencies can be induced via mutations in genes encoding for receptors and/or enzymes involved with cholesterol homeostasis. Examples of such disorders include Wolman disease (5) and the Niemann-Pick diseases (6) (both lysosomal storage disorders) as well as familial hypercholesterolemia (7). Though these diseases are rather rare, their pathogenesis is often severe and sometimes results in premature death. Besides genetic predisposition, another and more prevalent manner that influences cholesterol homeostasis is via excessive overnutrition, leading to metabolic syndrome and its associated diseases (8). In this context, excessive cholesterol accumulates at locations such as the vessel wall (atherosclerosis) and the liver (non-alcoholic fatty liver disease (NAFLD)) subsequently triggering resident macrophages to evolve into foam cells characterized by lysosomal cholesterol accumulation that induces an undesired inflammatory response, also referred to as metabolic inflammation (4, 9). This inflammatory response is an antecedent for more severe consequences both in the context of atherosclerosis (heart attack, stroke, peripheral vascular disease (10)) as well as NAFLD (advanced liver diseases such as cirrhosis and hepatocellular carcinoma (9)). Taken the prevalence and the severity of these disorders into consideration, efficient therapeutic options aimed at reducing cellular cholesterol levels are needed.
A straightforward and successfully applied approach to reduce cholesterol levels is via pharmacological approaches, which include the use of statins (11), fibrates (12) and more recently antibodies targeting proprotein convertase subtilisin-kexin type 9 (PCSK9) (13). However, substantial reduction of plasma cholesterol levels cannot be guaranteed for all patients (11), requiring alternative ways to improve cholesterol homeostasis. Relevantly, while the aforementioned interventions focus on reducing plasma cholesterol levels, a relative new approach aims at mobilizing cholesterol at the site of accumulation. This has led to the proposition to use 2-hydroxypropyl-β-cyclodextrin (CD), a substance initially used to solubilize lipophilic pharmaceutical agents, as a compound to treat cholesterol accumulation. Indeed, CD mobilized cholesterol from foam cells (14), promotes atherosclerosis regression (15), improved hepatic cholesterol metabolism (16), reduced lysosomal size of neural stem cells derived from Wolman disease patients (17) and is under evaluation in advanced human clinical trials for Niemann-Pick disease, type C1 (NPC1) (18). In contrast to these beneficial effects, CD causes massive damage to all cells of the developing (19) and some cells of the adult auditory system (20). Moreover, while dietary supplementation of β-cyclodextrin in hypercholesterolemic rats improved lipid metabolism, it concomitantly produced hepatotoxic effects characterized by increased plasma aminotransferase levels (21). Together, though the use of CD has clearly shown its benefits, the previous observations question whether this compound can be directly used in the clinic. Given its potential as therapeutic compound in metabolic inflammatory diseases, in this study, we therefore investigated the inflammatory aspects of CD administration in the context of cholesterol-induced metabolic inflammation in vivo and in vitro.
For this purpose, we investigated the inflammatory and cholesterol-depleting properties of CD in vivo and in a series of in vitro experiments for metabolic inflammation. First, we analyzed the effect of CD injection in low-density lipoprotein receptor knockout (Ldlr-/-) mice transplanted with Npc1nih or Npc1wt bone marrow on a high-fat, high-cholesterol (HFC) diet. Due to the absence of LDL receptors, and following an HFC diet, Ldlr-/- mice are characterized by high plasma LDL levels, mimicking diet-induced dyslipidemia and serving as an excellent animal model for metabolic diseases. Furthermore, Npc1nih bone marrow-transplanted mice feature a dysfunctional NPC1 protein in their immune cells, due to a deleterious frameshift mutation in the Npc1 allele, leading to lysosomal cholesterol buildup and a pro-inflammatory state. As such, chimeric Npc1nih transplanted (Npc1nih-tp) Ldlr-/- mice given an HFC diet constitute an extreme state of lysosomal cholesterol-induced metabolic inflammation (22). Next, as CD is able to mobilize lysosomal cholesterol, we assessed the ability of CD to influence lysosomal size of metabolically challenged bone marrow-derived macrophages (BMDM) by employing confocal microscopy. Finally, in a series of in vitro experiments we investigated the inflammatory properties of CD in more detail.
Throughout the study, mice were housed under standard conditions and had unlimited access to food and water, unless explicitly mentioned otherwise. For one week prior to and up to four weeks after bone marrow transplantation, Ldlr-/- mice were housed in filter-top cages and received antibiotics diluted in drinking water to prevent infections following immunosuppression (Neomycin, 100 mg/l, Gibco, Breda, the Netherlands; 6*104 U/L polymycin B sulfate). Six weeks old bone marrow donors Npc1nih and Npc1wt mice were derived from heterozygous founders of a C57BL/6 genetic background. Genotype of Npc1nih and Npc1wt mice was determined as previously described (23). On the day of the bone marrow transplant, Npc1nih and Npc1wt littermates were sacrificed via CO2 inhalation and their bone marrows were isolated. One day before and on the day of the bone marrow transplant, Ldlr-/- mice were subjected to six Gray of γ-radiation, thus having received 12 Gray of γ-radiation before receiving 1*107 bone marrow cells collected from Npc1wt or Npc1nih mice via intravenous injection. After ten weeks of recovery, transplanted mice were placed on a high-fat, high-cholesterol (HFC) diet (24) for twelve weeks. In the final three weeks (based on (16, 25)), mice received daily, subcutaneous injections of control (saline) or 2-hydroxypropyl-β-cyclodextrin (1800 mg/kg body weight; CD, Sigma-Aldrich), creating four experimental groups: (1) Npc1wt-tp NaCl (n = 6), (2) Npc1wt-tp CD (n = 9), (3) Npc1nih-tp NaCl (n = 6) and (4) Npc1nih-tp CD (n = 9). A schematic overview of the experimental set-up is provided in Supplementary Figure S1. All experiments were performed according to Dutch laws and approved by the Animal Experiment Committee of Maastricht University.
Upon sacrifice, all tissues were isolated and snap-frozen in liquid nitrogen and stored at -80°C or fixed in 4% formaldehyde/PBS. The collection of blood and tissue specimens, biochemical determination of lipids in plasma, RNA isolation, cDNA synthesis and qPCR were determined as described previously (26, 27). Primer sequences for genes are listed in Supplementary Table 1. Hepatic sterol content was determined by gas-liquid chromatography-mass spectroscopy, as described elsewhere (28).
Frozen liver sections (7 µm) were fixed in acetone and blocked for endogenous peroxidase by incubation with 0.25% of 0.03% H2O2 for 5 minutes. Primary antibodies used were against hepatic macrophages (1:100 rat anti-mouse CD68, clone FA11), infiltrated macrophages and neutrophils (1:500 rat anti-mouse Mac-1 (M1/70)) and neutrophils (1:100 rat anti-mouse NIMP (Ly6G)). 3-Amino-9-ethylcarbazole (Vector laboratories, CA, USA) was applied as color substrate and hematoxylin for nuclear counterstain. Sections were enclosed with Faramount aqueous mounting medium. Additional information concerning the immunostainings is also described elsewhere (29).
Pictures were taken with a Nikon digital camera DMX1200 and ACT-1 v2.63 software (Nikon Instruments Europe, Amstelveen, The Netherlands). Infiltrated macrophages and neutrophil cells (Mac-1+) and neutrophils (NIMP) were counted by two blinded researchers in six microscopical views (original magnification, 200x) and were indicated as number of cells per square millimeter (cells/mm2). Hepatic macrophages (CD68) were counted in six microscopical views (original magnification, 200x) and indicated as the percentage of CD68 positive area (Adobe Photoshop CS2 v.9.0.).
For assessing lysosomal size, we performed fluorescence confocal microscopy by staining for the lysosomal membrane marker lysosomal-associated membrane protein 1 (LAMP-1) followed by quantification of the number of lysosomes based on size. For this staining, fresh BMDM were fixed in paraformaldehyde (4%) and permeabilized in Triton-X (0.1%)/BSA (0.2%) solution. BMDM were incubated with the primary (1:100, rabbit polyclonal Lamp1, ab24170, Abcam, Cambridge, United Kingdom) and secondary (1:200, Alexa fluor 488 goat anti-rabbit IgG, A11008, Thermo Fisher Scientific, Waltham, Massachusetts, USA) antibodies and finally, sections were enclosed with glycerol mounting medium (DABCO-DAPI). Confocal pictures were taken with a LEICA DMI 4000 microscope (Leica microsystems, Wetzlar, Germany), providing 3D images of BMDM and their lysosomes.
For optimal quantification, one cell remained visible per purview. For this purpose, the original photo was cropped, and then configured into a TIFF file for further processing.
In this study we made use of two fluorescent stainings either for the nucleus (DAPI) or lysosome (LAMP-1). To determine the nucleus to lysosomal distance, the color channels were bifurcated for better differentiation, creating a nucleus channel and lysosomal channel.
To determine the lysosome to nucleus distance we first determined the location of the nucleus in the 3D picture. Therefore, we analyzed the nucleus channel with the 3D ObjectCounter.
To obtain the coordinates of the lysosomes, a mask was created by using the ObjectCounter. The mask was created by calculating the geometrical centers of the lysosomes (30). Threshold was determined before the picture was analyzed.
To discriminate between separated or combined lysosomes, the watershed method was used. The watershed method uses the mask to determine the centers of the masses (Parra et al.).
After the watershed, the Regions of interest (ROIs) were added to the 3D ROI Manager, where the volume and coordinates were calculated. To quantify the pictures in an efficient manner, a macro was created (Supplementary Methods).
After the coordinates and volumes were obtained, the distance for each lysosome to the nucleus was calculated. For this we used the Pythagoras equation.
Next, we created a plugin-Macro for ImageJ to identify the amount and volume of lysosomes. Finally, the number of lysosomes was quantified and categorized them by size (< 0.1 µm3; 0.1-1 µm3; > 1 µm3) and expressed the number of lysosomes per size relative to the total amount of lysosomes present inside the BMDM. Representative videos were created using Z-stack images played in series and recorded as.AVI files which were trimmed using quick time movie player. DAPI (blue) represents nuclei and LAMP-1 (green) represents lysosomes.
Data were statistically analyzed by performing the unpaired t-test or the two-way ANOVA and Tukey’s post hoc test using GraphPad Prism software (version 6 for Windows, GraphPad Software Inc, San Diego, CA, U.S; www.graphpad.com). Data were expressed as the group mean and standard error of the mean.
Npc1nih bone marrow-transplanted mice feature a dysfunctional NPC1 protein in their immune cells, due to a deleterious frameshift mutation in the Npc1 allele, leading to lysosomal cholesterol buildup and a pro-inflammatory state. As such, chimeric Npc1nih transplanted (Npc1nih-tp) Ldlr-/- mice given an HFC diet constitute an extreme state of lysosomal cholesterol-induced metabolic inflammation (22).
To confirm successful injection of 2-hydroxypropyl-β-cyclodextrin (CD) into the mice in the final three weeks of the experiments, plasma and hepatic lipid metabolism were profiled. CD-treated Npc1wt-tp Ldlr-/- mice showed reduced plasma triglycerides (Table 1), though this reduction was already apparent before the start of CD treatment (Table 1). While no effects were observed on plasma (Table 1) and hepatic (Figure 1) cholesterol levels, the ratios of the cholesterol oxidation products 7α-hydroxycholesterol to cholesterol and 27-hydroxycholesterol to cholesterol were both significantly increased in the livers of CD-treated Npc1nih- and Npc1wt-tp Ldlr-/- mice, suggesting increased mobilization of cholesterol (Figures 1B, C). Hepatic gene expression analysis of cytochrome P450 7A1 (Cyp7a1), the enzyme responsible for the conversion of cholesterol into 7α-hydroxycholesterol, was concomitantly increased (Figure 1D), while sterol 27-hydroxylase (Cyp27a1) hepatic gene expression levels remained unaffected upon CD-treatment (Figure 1E). Concerning other non-cholesterol sterols, CD-treatment reduced hepatic cholestanol and desmosterol levels in CD-treated Npc1nih-tp Ldlr-/- mice (Table 2). No effects were observed on liver and spleen weight (Supplementary Figures 2A, B). Body weight over time seemed to be lower in Npc1nih-tp Ldlr-/- mice compared to Npc1wt-tp Ldlr-/- mice, but this difference was not significant (Supplementary Figure 2C). Overall, these findings indicate that CD-treatment mobilizes hepatic cholesterol, confirming successful administration of CD.
Figure 1 Hepatic lipid parameters. (A) Hepatic cholesterol levels of Npc1wt-tp and Npc1nih-tp mice on a HFC diet that received CD or saline treatment. (B, C) Hepatic levels of the cholesterol degradation products 7α-hydroxycholesterol and 27-hydroxycholesterol. (D, E) Hepatic gene expression analysis of Cyp7a1 (D) and Cyp27a1 (E). n = 6-9 mice/group. Gene expression data are set relative to Npc1wt-tp mice treated with saline. *, ** and **** indicate p ≤ 0.05, 0.01 and 0.0001 resp.by means of two-way ANOVA followed by Tukey post-hoc analysis.
After we confirmed successful administration of CD, we further evaluated the impact of three-week CD treatment on hepatic inflammation by staining hepatic cryosections for the inflammatory markers Mac-1 (infiltrated macrophages and neutrophils; directed against Cd11b), NIMP (neutrophils) and CD68 (macrophages) (Figures 2A–C). While CD treatment only induced a minor non-significant increase for these inflammatory markers in Npc1wt-tp Ldlr-/- mice, this increase was more pronounced in Npc1nih-tp Ldlr-/- mice (Figures 2A, D). This pro-inflammatory effect of CD was confirmed by histological scoring of HE-staining of livers of the Npc1nih-tp Ldlr-/- mice (Supplementary Figure S3). To confirm these histological data, hepatic gene expression for the inflammatory markers tumor necrosis factor alpha (Tnfα), chemokine (C-C motif) ligand 2 (Ccl2), chemokine (C-C motif) ligand 5 (Ccl5) and serum amyloid A1 (Saa1) was analyzed. In line with the histological data, CD treatment increased the expression of these hepatic inflammatory markers in the Npc1wt-tp or in the Npc1nih-tp group (Figures 2E–H). Moreover, the hepatic fibrotic markers matrix metallopeptidase 9 (Mmp9) and plasminogen activator inhibitor-1 (Pai-1) were concomitantly increased in CD-treated Npc1nih-tp Ldlr-/- mice, while no significant changes were observed in transforming growth factor beta (Tgfβ) and tissue inhibitor of metalloproteinase (Timp1) (Supplementary Figure S4). Together, these findings indicate that three-week treatment with CD increased hepatic inflammation and mild elevations in fibrosis in our experimental model.
Figure 2 Parameters of hepatic inflammation. (A–C) Liver sections were stained for infiltrating macrophages and neutrophils (Mac-1), neutrophils (NIMP) and hepatic macrophages (CD68) and subsequently quantified by counting (Mac-1 and NIMP) or by assessing the positive area of the staining (CD68). (D) Representative images of liver sections stained for infiltrated macrophages and neutrophils (Mac-1). (E–H) Hepatic gene expression analysis of Tnfα, Ccl2, Ccl5 and Saa1. Gene expression data were set relative to control-treated Npc1wt-tp mice. * indicates p ≤ 0.05 by means of two-way ANOVA followed by Tukey post-hoc analysis.
As CD treatment mobilized cholesterol, but increased inflammation in our in vivo model for lysosomal cholesterol-induced inflammation, we opted to investigate the effect of CD on lysosomal size in metabolically challenged BMDM via confocal microscopy. For this purpose, we first compared lysosomal size between wildtype and Npc1nih BMDM. While Npc1nih BMDM showed a reduced number of small lysosomes (categorized as < 0.1 µm3), they showed an increase in the number of large lysosomes (categorized as > 1 µm3) as compared to wildtype BMDM, which is likely the result of lysosomal lipid accumulation that is characteristic for the mutation (Figure 3 Comparison A; Supplementary Table 2).
Figure 3 Lysosomal volume of metabolically-challenged bone marrow-derived macrophages with and without intervention. We quantified the number of lysosomes, categorized them by size (< 0.1 µm3; 0.1-1 µm3; > 1 µm3) and expressed the number of lysosomes per size relative to the total amount of lysosomes present inside the BMDM. Comparison A: Relative comparison of the distribution of lysosomal volumes between Wt and Npc1nih BMDM. Comparison B : Relative comparison of the distribution of lysosomal volumes between control and oxLDL (25µg/ml; 72hr) -stimulated Wt BMDM. Comparison C: Relative comparison of the distribution of lysosomal volumes between oxLDL-stimulated Wt BMDM treated with or without CD (1.95 mM; 4hr). Comparison D : Relative comparison of the distribution of lysosomal volumes between Npc1nih BMDM treated with or without CD. *, ** and *** indicate p < 0.05, 0.01 and 0.001 resp.by means of unpaired t-test. Data are the result of analysis of n = 5-7 BMDM per experimental condition.
As internalization of oxidized cholesterol-rich low-density lipoprotein (oxLDL) in BMDM also leads to lysosomal accumulation, we next compared lysosomal size of oxLDL (25µg/ml; 72hr)-incubated wildtype BMDM to control-incubated wildtype BMDM. Incubating wildtype BMDM with oxLDL similarly reduced the number of small lysosomes and increased the number of large lysosomes, confirming the effect of oxLDL on lysosomal size (Figure 3 Comparison B; Supplementary Table 3) as well as our method to quantify the number of lysosomes based on their size. Representative videos of all indicated conditions are shown in Supplementary Videos 1–5.
Next, to assess the impact of CD treatment, we compared lysosomal size of oxLDL-incubated wildtype BMDM that were treated with or without CD (1.95 mM; 4hr). As expected, CD treatment increased the number of small lysosomes and reduced the level of large lysosomes, thereby reversing the oxLDL-induced effects on lysosomal size (Figure 3 Comparison C; Supplementary Table 4). Furthermore, similar findings were observed when Npc1nih BMDM were treated with CD (Figure 3 Comparison D; Supplementary Table 5). Overall, these findings confirm the beneficial effect of CD treatment on lysosomes of metabolically challenged BMDM.
To increase our insight into the inflammatory effects of CD, we performed a series of in vitro experiments in BMDM, framed within the context of metabolic inflammation. First, we incubated wildtype and Npc1nih BMDM with CD for 4hr followed by 4hr lipopolysaccharide (LPS) stimulation. Four-hour CD treatment increased TNFα protein secretion in both wildtype and Npc1nih BMDM, suggesting an acute pro-inflammatory effect of CD also in wildtype and Npc1nih BMDM (Figure 4A; Supplementary Figure S5A). To provide stronger evidence for this inflammatory effect of CD, Npc1nih BMDM were challenged with oxLDL, followed by 4hr CD incubation and 4hr LPS stimulation, thereby creating a more severe model of metabolic inflammation. Similar to our previous findings, CD treatment increased TNFα protein secretion in both oxLDL- and control-challenged Npc1nih BMDM (Figure 4B; Supplementary Figure S5B). This was confirmed at gene expression level, showing increased levels of pro-inflammatory markers (Tnfα, iNos/Arg1 ratio) (Figure 4B; Supplementary Figure S5B) and decreased levels of anti-inflammatory markers (IL-10; Figure 4B; Supplementary Figure S5B) after CD treatment. These pro-inflammatory findings of CD were also confirmed in a separate BMDM experiment with similar set-up in wildtype BMDM (Supplementary Figure S6A) and without the final LPS stimulus (Supplementary Figure S6B).
Figure 4 Metabolically-challenged BMDM parameters of inflammation and cholesterol metabolism after four hour incubation with 2-hydroxypropyl-β-cyclodextrin. (A) TNFα protein levels of 2-hydroxypropyl-β-cyclodextrin (1.95 mM; 4hr)-treated Wt or Npc1nih BMDM that were terminally stimulated with LPS (100ng/ml; 4hr). (B) TNFα protein levels and Tnfα, IL10 and iNOS/Arg1 ratio gene expression data of oxLDL (25µg/ml; 24hr)-exposed Npc1nih BMDM treated with or without CD (4hr) that were terminally stimulated with LPS (100ng/ml; 4hr). Gene expression data were set relative to control-exposed Npc1nih BMDM treated with saline and stimulated with LPS. (C–E) Gene expression analysis of Tnfα, IL-1b, IL-18, Lxrα and Npc2 of oxLDL (25µg/ml; 24hr)-exposed Ldlr-/- and Wt BMDM treated with or without CD (1.95 mM; 4hr) and terminally stimulated with control (C), Pam3Cys (4hr) (D) or LPS (4hr) (E). Gene expression data were set relative to oxLDL-exposed Wt BMDM treated with saline. Colored (red or blue) boxes are compared to the box directly at their left via two-way ANOVA followed by Tukey post-hoc analysis, indicating the effect of CD. Data are the result of 2 or 3 independent experiments.
As another model for metabolic disturbance, we investigated the inflammatory effects of CD on Ldlr-/- BMDM, which is a mutation causing disturbances in cholesterol metabolism. Twenty-four-hour incubation with oxLDL followed by 4hr CD treatment resulted in increased gene expression levels of Tnfα, IL-1b, and IL-18, confirming the pro-inflammatory effects of CD also in Ldlr-/- BMDM (Figure 4C; Supplementary Figure S4C). Nevertheless, CD treatment increased Lxrα and Npc2 expression, confirming the cholesterol-mobilizing properties of CD. To further confirm these findings, a similar experiment was conducted with the addition of a final stimulation with Pam3Cys or LPS for 4hr. Stimulation with Pam3Cys (Figure 4D; Supplementary Figure S4D) and LPS (Figure 4E; Supplementary Figure S4E) showed similar results as previously described, showing pro-inflammatory and cholesterol-mobilizing effects of CD treatment in Ldlr-/- BMDM. Together, these findings show that while CD maintains its effect on cholesterol metabolism, pro-inflammatory effects become apparent after 4hr incubation in metabolically challenged BMDM.
To validate the inflammatory effect of CD in non-primary cells, we investigated the impact of CD treatment on the macrophage-derived mouse RAW 264.7 and human THP-1 cell lines. Firstly, RAW 264.7 cells were incubated with CD for 4 or 8hr, followed by 4hr LPS stimulation. While 4hr incubation did not show any inflammatory effect, CD treatment for 8hr increased TNFα protein secretion (Figures 5A, B; Supplementary Figures S7A, B). Furthermore, THP-1-derived macrophages were challenged with sphingomyelinase-aggregated LDL (smLDL), native LDL or control for 24hr and incubated with CD for 4hr. Secreted protein levels of TNFα increased in all three experimental groups after CD incubation (Figures 6A–C). Together, these results indicate that CD also shows pro-inflammatory properties in macrophage-derived cell lines.
Figure 5 RAW cell line-related parameters of inflammation after prolonged exposure to 2-hydroxypropyl-β-cyclodextrin. (A, B) TNFα protein levels of 2-hydroxypropyl-β-cyclodextrin (1.95 mM; 4 or 8hr)-treated RAW cells that were terminally stimulated with LPS (100ng/ml; 4hr). Data represent n = 3 for each experimental group. * indicates p < 0.05 by means of unpaired t-test.
Figure 6 THP1 cell line-related parameters of inflammation after prolonged exposure to 2-hydroxypropyl-β-cyclodextrin. (A–C) TNFα protein levels of control, nLDL or smLDL (24hr)-exposed THP1 cells treated with or without 2-hydroxypropyl-β-cyclodextrin (1.95 mM; 4hr). *, ** indicates p < 0.05 and 0.01 by means unpaired t-test. Data represent n = 3 for each experimental group.
As increased depletion of cholesterol from the plasma membrane was shown to result in pro-inflammatory responses (31), we first investigated whether incubation time influenced the pro-inflammatory effect of CD. For this purpose, wildtype BMDMs were incubated with CD for 5, 10 and 30 min as well as for 1 and 4hr. Protein secretion of TNFα showed a marked time-dependent response after CD treatment (Figure 7A). This time-dependent, pro-inflammatory effect of CD was confirmed at gene expression levels for the inflammatory markers Tnfα, IL-1b and Ccl2, only showing increased expression after 1 and 4hr incubation (Figures 7B–D). Lxrα showed a similar increase after 4 hours of CD incubation, Npc2 only showed a trend (Figures 7E, F). Expression levels of IL-18, Arg1, Abca1, Abcg1 and Npc1 remained similar (Supplementary Figure S8).
Figure 7 Time dependency of the inflammatory effect of 2-hydroxypropyl-β-cyclodextrin. (A) TNFα protein levels of Wt BMDM treated with 2-hydroxypropyl-β-cyclodextrin for different time durations. (B–D) Gene expression analysis of Tnfα, IL-1b, Ccl2. (E, F) Gene expression analysis of Lxrα and Npc2. Gene expression data were set relative to Wt BMDM treated with saline. Data are the result of 2 independent experiments. *, ** indicates p < 0.05 and 0.01 by means of unpaired t-test.
To further confirm our hypothesis, we also performed a dose-response curve with CD (4hr incubation) in wildtype BMDMs. As indicated in Figure 8, CD incubation showed a clear concentration-dependent effect on TNFα secretion, adding fuel to our argument that the pro-inflammatory effects of CD are related to cholesterol depletion from the plasma membrane.
Figure 8 Concentration dependency of the inflammatory effect of 2-hydroxypropyl-β-cyclodextrin. Dose response analysis of TNFα protein levels of Wt BMDM treated with 2-hydroxypropyl-β-cyclodextrin for 4hrs.
New perspectives to reduce cholesterol levels aim at improving cellular instead of plasma cholesterol levels, raising the argument to use CD as a pharmacological compound to improve cholesterol homeostasis. Here, we confirm the cholesterol-depleting effects of CD in a metabolic inflammatory context, but concomitantly show a detrimental time- and concentration- dependent inflammatory effect of CD treatment. Therefore, while CD is able to decrease cellular cholesterol levels, our findings demonstrate that its use in the clinic should be closely monitored especially in patients with a metabolic inflammatory background.
Our observation that CD treatment promotes cellular cholesterol mobilization, but induces a time- and concentration-dependent inflammatory effect implies the importance of the subcellular distribution of cholesterol and the subsequent impact of cholesterol depletion from these specific locations. Unesterified cholesterol serves a key structural function in the plasma membrane as it is critical for the formation of liquid-ordered rafts, which determine membrane fluidity. The membrane fluidity is therefore coupled to the free cholesterol/phospholipid ratio of the plasma membrane as this ratio determines the formation of the aforementioned rafts (32, 33). Under hyperlipidemic conditions, the plasma membrane serves as the first pool to deposit free cholesterol from external sources (34). However, accumulation of free cholesterol in the plasma membrane above an optimal free cholesterol/phospholipid ratio negatively impacts plasma membrane fluidity (33). Indeed, Yvan-Charvet et al. showed that increased accumulation of free cholesterol in the plasma membrane (induced by Abca1 or Abcg1 knockout) directly results in pro-inflammatory responses, supporting our current notion of the limited physiological capacity of the plasma membrane to harbor free cholesterol (31). Therefore, exceeding the plasma membrane’s capacity to harbor cholesterol directly results in intracellular cholesterol accumulation (mainly in lysosomes), which is considered a severe pathological phenomenon for mediating inflammatory responses (4). Evidently, under conditions that generate severe accumulation of intracellular cholesterol (exemplified by the NPC1 mutation and LDLR knockout), CD-mediated depletion of cholesterol is highly desirable. In line with this concept, the increased lysosomal size in metabolically challenged macrophages was reverted after incubation with CD treatment. This advantageous cholesterol-depleting effect of CD has been confirmed in multiple previous reports (15, 18, 31, 35). In contrast, in the current study, we consistently show a harmful, pro-inflammatory (and even mild pro-fibrotic) effect of CD under cholesterol-induced inflammatory conditions in vivo and in vitro. This pro-inflammatory effect can be rationalized by the prolonged cellular exposure to CD, which influences plasma membrane cholesterol combined with intracellular cholesterol levels. Indeed, given the key role of cholesterol in the formation of liquid-ordered rafts, excessive depletion of plasma membrane cholesterol (as is induced with CD treatment) is highly undesirable as it disrupts these rafts, affecting membrane fluidity. This rationale is further supported by findings showing that cholesterol extraction of the plasma membrane is a primary location where CD exerts its function (36, 37). Therefore, excessive depletion of cholesterol from the plasma membrane by CD might be an explanation for the observed pro-inflammatory findings in our study as well as the time-and concentration-dependent character of our findings. The time-dependent findings are also in line with experiments reported by Pilely et al. and Ding et al., that show that while incubation of different types of cyclodextrin for a short period of time (10 and 30 min) is anti-inflammatory (35), incubation for 24 and 48hr lead to ototoxicity (19). Together, these observations indicate that while CD has potential in advantageously depleting cellular cholesterol, it is essential to monitor the quantity of cellular cholesterol and adjust the therapeutic dose/time accordingly to prevent undesired pro-inflammatory side-effects.
Notably, while neutrophils generally constitute the major immune cell population under inflammatory conditions, in the current study we only focused on the effect of CD on macrophages. This choice is based on previous findings by our group that demonstrate macrophages to be the most important immune cell in the in vivo model here described (as evidenced by changes in hematopoiesis (38) and organ-specific inflammation (22)). Nevertheless, as neutrophil aberrations have also been described in NPC1 disease (39), future research should further explore the involvement of neutrophils in CD-induced inflammatory responses in a metabolic inflammatory context.
These dichotomic characteristics of CD raise the question whether structural manipulation of cyclodextrins can reduce the harmful pro-inflammatory effects, while maintaining the advantageous cholesterol-depleting effects of CD. Cyclodextrins are composed of cyclic oligosaccharides of 6, 7 or 8 glucose units (referred to as α-, β- and γ-cyclodextrins respectively), providing cyclodextrin a polar and hydrophilic surface combined with a non-polar cavity (40). This dual-property structure of CD grants itself for modification via polymerization, creating so-called polycyclodextrins. Indeed, various modifications have been performed on cyclodextrin, creating more efficient and effective cyclodextrins for a plethora of purposes (40). In line, Kulkarni et al. adapted the structure CD (the type we employed in our study) and showed that their linear degradable, high molecular weight polymer variation improved the pharmacokinetic profile and bioavailability in NPC mice (41). Moreover, using polyrotaxanes enabled specific release of CD inside lysosomes, thereby minimizing the effect on plasma membrane cholesterol (42). Based on these reports, it is anticipated that designing polycyclodextrins is a promising approach that can have a considerable clinical impact due to its ability to reduce the pro-inflammatory properties of CD described in our study.
In conclusion, though we confirm its cholesterol-depleting effects, we here demonstrate a time- and concentration-dependent harmful pro-inflammatory effect of CD under metabolic inflammatory conditions. As such, we suggest that clinical use of CD, in particular in a metabolic inflammatory context, should be closely monitored to prevent undesired side effects.
The raw data supporting the conclusions of this article will be made available by the authors, without undue reservation.
The animal study was reviewed and approved by Animal Experiment Committee of Maastricht University.
TH, TY, and RS-S conceived the study and designed the experiments. TH, TY, MG, JL, DK, MZ, MW, JP, DiL, and DaL contributed to sample collection, data collection, molecular experiments, and data analysis. TH, TY, and RS-S wrote the manuscript. All authors helped in editing and revising the manuscript. RS-S obtained funding. All authors contributed to the article and approved the submitted version.
This work was supported by the TKI-LSH (grant no. 40-41200-98-9306). MW was supported by VIDI grant 917.15.350 and an Aspasia grant from the Netherlands Organization of Scientific Research (NWO) and a Rosalind Franklin Fellowship from the University of Groningen.
The authors declare that the research was conducted in the absence of any commercial or financial relationships that could be construed as a potential conflict of interest.
All claims expressed in this article are solely those of the authors and do not necessarily represent those of their affiliated organizations, or those of the publisher, the editors and the reviewers. Any product that may be evaluated in this article, or claim that may be made by its manufacturer, is not guaranteed or endorsed by the publisher.
We thank the present and past members of Prof. Sverdlov’s group for their technical assistance in the experiments.
The Supplementary Material for this article can be found online at: https://www.frontiersin.org/articles/10.3389/fimmu.2021.716357/full#supplementary-material.
Supplementary Figure 1 | Overview of experimental set-up of in vivo experiment.
Supplementary Figure 2 | Relative organ weights.
Supplementary Figure 3 | Quantification of hepatic HE staining of Npc1nih-tp mice.
Supplementary Figure 4 | Hepatic gene expression of fibrotic markers.
Supplementary Figure 5 | Experimental set-ups related to Figure 4.
Supplementary Figure 6 | Heatmap of inflammatory mediators of CD-treated Wt BMDM. (A, B) TNFα protein levels and Tnfα, IL1b, IL-10, Ccl2, Lxrα and Npc2 gene expression of oxLDL (24hr)-exposed Wt BMDM treated with or without CD (4hr) that were terminally stimulated with LPS (A) or without (B) LPS for 4 hr. Gene expression data were set relative to control-exposed Wt BMDM treated with saline and stimulated with (A) or without (B) LPS. Colored (red or blue) boxes are compared to the box directly at their left via two-way ANOVA followed by Tukey post-hoc analysis, indicating the effect of CD. Data are the result of 2-4 independent experiments.
Supplementary Figure 7 | Experimental set-ups related to Figure 5.
Supplementary Figure 8 | Gene expression analysis of BMDM exposed to different time intervals of CD.
Supplementary Table 1 | Primer sequences of genes used for quantitative RT-PCR.
Supplementary Table 2 | Raw data related to confocal quantification of conditions Wt control vs. NPC1 control. Blue numbers are used for statistical analysis.
Supplementary Table 3 | Raw data related to confocal quantification of conditions Wt control vs. Wt oxLDL. Blue numbers are used for statistical analysis.
Supplementary Table 4 | Raw data related to confocal quantification of conditions Wt-oxLDL-control vs. Wt-oxLDL-CD. Blue numbers are used for statistical analysis.
Supplementary Table 5 | Raw data related to confocal quantification of conditions NPC1 control vs. NPC1 CD. Blue numbers are used for statistical analysis.
Supplementary Video 1 | wildtype control.
Supplementary Video 2 | Npc1nih control.
Supplementary Video 3 | wildtype oxLDL.
Supplementary Video 4 | wildtype oxLDL CD.
Supplementary Video 5 | Npc1nih CD.
1. Van Meer G, Voelker DR, Feigenson GW. Membrane Lipids: Where They Are and How They Behave. Nat Rev Mol Cell Biol (2008) 9:112–24. doi: 10.1038/nrm2330
2. Demer LL, Hsu JJ, Tintut Y. Steroid Hormone Vitamin D: Implications for Cardiovascular Disease. Circ Res (2018) 122:1576–85. doi: 10.1161/CIRCRESAHA.118.311585
3. Hegele RA. Plasma Lipoproteins: Genetic Influences and Clinical Implications. Nat Rev Genet (2009) 10:109–21. doi: 10.1038/nrg2481
4. Hendrikx T, Walenbergh SM, Hofker MH, Shiri-Sverdlov R. Lysosomal Cholesterol Accumulation: Driver on the Road to Inflammation During Atherosclerosis and Non-Alcoholic Steatohepatitis. Obes Rev (2014) 15:424–33. doi: 10.1111/obr.12159
5. Pericleous M, Kelly C, Wang T, Livingstone C, Ala A. Wolman’s Disease and Cholesteryl Ester Storage Disorder: The Phenotypic Spectrum of Lysosomal Acid Lipase Deficiency. Lancet Gastroenterol Hepatol (2017) 2:670–9. doi: 10.1016/S2468-1253(17)30052-3
6. Santos-Lozano A, Villamandos Garcia D, Sanchis-Gomar F, Fiuza-Luces C, Pareja-Galeano H, Garatachea N, et al. Niemann-Pick Disease Treatment: A Systematic Review of Clinical Trials. Ann Transl Med (2015) 3:360. doi: 10.3978/j.issn.2305-5839.2015.12.04
7. Defesche JC, Gidding SS, Harada-Shiba M, Hegele RA, Santos RD, Wierzbicki AS. Familial Hypercholesterolaemia. Nat Rev Dis Primers (2017) 3:17093. doi: 10.1038/nrdp.2017.93
8. O’neill S, O’driscoll L. Metabolic Syndrome: A Closer Look at the Growing Epidemic and Its Associated Pathologies. Obes Rev (2015) 16:1–12. doi: 10.1111/obr.12229
9. Gehrke N, Schattenberg JM. Metabolic Inflammation-A Role for Hepatic Inflammatory Pathways as Drivers of Comorbidities in Nonalcoholic Fatty Liver Disease? Gastroenterology (2020) 158:1929–47.e1926. doi: 10.1053/j.gastro.2020.02.020
10. Libby P, Buring JE, Badimon L, Hansson GK, Deanfield J, Bittencourt MS, et al. Atherosclerosis. Nat Rev Dis Primers (2019) 5:56. doi: 10.1038/s41572-019-0106-z
11. Hadjiphilippou S, Ray KK. Cholesterol-Lowering Agents. Circ Res (2019) 124:354–63. doi: 10.1161/CIRCRESAHA.118.313245
12. Staels B, Dallongeville J, Auwerx J, Schoonjans K, Leitersdorf E, Fruchart JC. Mechanism of Action of Fibrates on Lipid and Lipoprotein Metabolism. Circulation (1998) 98:2088–93. doi: 10.1161/01.CIR.98.19.2088
13. Schwartz GG, Steg PG, Szarek M, Bhatt DL, Bittner VA, Diaz R, et al. Alirocumab and Cardiovascular Outcomes After Acute Coronary Syndrome. N Engl J Med (2018) 379:2097–107. doi: 10.1056/NEJMoa1801174
14. Atger VM, de la Llera Moya M, Stoudt GW, Rodrigueza WV, Phillips MC, Rothblat GH. Cyclodextrins as Catalysts for the Removal of Cholesterol From Macrophage Foam Cells. J Clin Invest (1997) 99:773–80. doi: 10.1172/JCI119223
15. Zimmer S, Grebe A, Bakke SS, Bode N, Halvorsen B, Ulas T, et al. Cyclodextrin Promotes Atherosclerosis Regression via Macrophage Reprogramming. Sci Transl Med (2016) 8:333ra350. doi: 10.1126/scitranslmed.aad6100
16. Walenbergh SM, Houben T, Hendrikx T, Jeurissen ML, Van Gorp PJ, Vaes N, et al. Weekly Treatment of 2-Hydroxypropyl-Beta-Cyclodextrin Improves Intracellular Cholesterol Levels in LDL Receptor Knockout Mice. Int J Mol Sci (2015) 16:21056–69. doi: 10.3390/ijms160921056
17. Aguisanda F, Thorne N, Zheng W. Targeting Wolman Disease and Cholesteryl Ester Storage Disease: Disease Pathogenesis and Therapeutic Development. Curr Chem Genom Transl Med (2017) 11:1–18. doi: 10.2174/2213988501711010001
18. Ory DS, Ottinger EA, Farhat NY, King KA, Jiang X, Weissfeld L, et al. Intrathecal 2-Hydroxypropyl-Beta-Cyclodextrin Decreases Neurological Disease Progression in Niemann-Pick Disease, Type C1: A Non-Randomised, Open-Label, Phase 1-2 Trial. Lancet (2017) 390:1758–68. doi: 10.1016/S0140-6736(17)31465-4
19. Ding D, Manohar S, Jiang H, Salvi R. Hydroxypropyl-Beta-Cyclodextrin Causes Massive Damage to the Developing Auditory and Vestibular System. Hear Res (2020) 396:108073. doi: 10.1016/j.heares.2020.108073
20. Crumling MA, King KA, Duncan RK. Cyclodextrins and Iatrogenic Hearing Loss: New Drugs With Significant Risk. Front Cell Neurosci (2017) 11:355. doi: 10.3389/fncel.2017.00355
21. Garcia-Mediavilla V, Villares C, Culebras JM, Bayon JE, Gonzalez-Gallego J. Effects of Dietary Beta-Cyclodextrin in Hypercholesterolaemic Rats. Pharmacol Toxicol (2003) 92:94–9. doi: 10.1034/j.1600-0773.2003.920206.x
22. Houben T, Oligschlaeger Y, Bitorina AV, Hendrikx T, Walenbergh SMA, Lenders MH, et al. Blood-Derived Macrophages Prone to Accumulate Lysosomal Lipids Trigger oxLDL-Dependent Murine Hepatic Inflammation. Sci Rep (2017) 7:12550. doi: 10.1038/s41598-017-13058-z
23. Houben T, Magro Dos Reis I, Oligschlaeger Y, Steinbusch H, Gijbels MJJ, Hendrikx T, et al. Pneumococcal Immunization Reduces Neurological and Hepatic Symptoms in a Mouse Model for Niemann-Pick Type C1 Disease. Front Immunol (2018) 9:3089. doi: 10.3389/fimmu.2018.03089
24. Bieghs V, Van Gorp PJ, Wouters K, Hendrikx T, Gijbels MJ, Van Bilsen M, et al. LDL Receptor Knock-Out Mice Are a Physiological Model Particularly Vulnerable to Study the Onset of Inflammation in Non-Alcoholic Fatty Liver Disease. PloS One (2012b) 7:e30668. doi: 10.1371/journal.pone.0030668
25. Bieghs V, Hendrikx T, Van Gorp PJ, Verheyen F, Guichot YD, Walenbergh SM, et al. The Cholesterol Derivative 27-Hydroxycholesterol Reduces Steatohepatitis in Mice. Gastroenterology (2013) 144:167–8.e161. doi: 10.1053/j.gastro.2012.09.062
26. Bieghs V, Wouters K, Van Gorp PJ, Gijbels MJ, De Winther MP, Binder CJ, et al. Role of Scavenger Receptor A and CD36 in Diet-Induced Nonalcoholic Steatohepatitis in Hyperlipidemic Mice. Gastroenterol 138 (2010) 2477-2486:2486.e2471–73. doi: 10.1053/j.gastro.2010.02.051
27. Bieghs V, Van Gorp PJ, Walenbergh SM, Gijbels MJ, Verheyen F, Buurman WA, et al. Specific Immunization Strategies Against Oxidized Low-Density Lipoprotein: A Novel Way to Reduce Nonalcoholic Steatohepatitis in Mice. Hepatology (2012a) 56:894–903. doi: 10.1002/hep.25660
28. Sosic-Jurjevic B, Lutjohann D, Renko K, Filipovic B, Radulovic N, Ajdzanovic V, et al. The Isoflavones Genistein and Daidzein Increase Hepatic Concentration of Thyroid Hormones and Affect Cholesterol Metabolism in Middle-Aged Male Rats. J Steroid Biochem Mol Biol (2019) 190:1–10. doi: 10.1016/j.jsbmb.2019.03.009
29. Houben T, Bitorina AV, Oligschlaeger Y, Jeurissen ML, Rensen S, Kohler SE, et al. And Shiri-Sverdlov, RSex-Opposed Inflammatory Effects of 27-Hydroxycholesterol Are Mediated via Differences in Estrogen Signaling. J Pathol (2020) 251:429–39. doi: 10.1002/path.5477
30. Fabrice Cordelires JJ. 3d Object Counter. Available at: http://rsbweb.nih.gov/ij/plugins/track/objects.html.
31. Yvan-Charvet L, Welch C, Pagler TA, Ranalletta M, Lamkanfi M, Han S, et al. Increased Inflammatory Gene Expression in ABC Transporter-Deficient Macrophages: Free Cholesterol Accumulation, Increased Signaling via Toll-Like Receptors, and Neutrophil Infiltration of Atherosclerotic Lesions. Circulation (2008) 118:1837–47. doi: 10.1161/CIRCULATIONAHA.108.793869
32. Simons K, Ikonen E. How Cells Handle Cholesterol. Science (2000) 290:1721–6. doi: 10.1126/science.290.5497.1721
33. Tabas I. Consequences of Cellular Cholesterol Accumulation: Basic Concepts and Physiological Implications. J Clin Invest (2002) 110:905–11. doi: 10.1172/JCI0216452
34. Ikonen E. Cellular Cholesterol Trafficking and Compartmentalization. Nat Rev Mol Cell Biol (2008) 9:125–38. doi: 10.1038/nrm2336
35. Pilely K, Bakke SS, Palarasah Y, Skjoedt MO, Bartels ED, Espevik T, et al. Alpha-Cyclodextrin Inhibits Cholesterol Crystal-Induced Complement-Mediated Inflammation: A Potential New Compound for Treatment of Atherosclerosis. Atherosclerosis (2019) 283:35–42. doi: 10.1016/j.atherosclerosis.2019.01.034
36. Christian AE, Haynes MP, Phillips MC, Rothblat GH. Use of Cyclodextrins for Manipulating Cellular Cholesterol Content. J Lipid Res (1997) 38:2264–72. doi: 10.1016/S0022-2275(20)34940-3
37. Lopez CA, De Vries AH, Marrink SJ. Molecular Mechanism of Cyclodextrin Mediated Cholesterol Extraction. PloS Comput Biol (2011) 7:e1002020. doi: 10.1371/journal.pcbi.1002020
38. Magro Dos Reis I, Houben T, Gijbels MJJ, Lutjohann D, Plat J, Shiri-Sverdlov R. Anti-Inflammatory Effects of Dietary Plant Stanol Supplementation Are Largely Dependent on the Intake of Cholesterol in a Mouse Model of Metabolic Inflammation. Biomedicines (2021) 9(5):518. doi: 10.3390/biomedicines9050518.
39. Parra J, Klein AD, Castro J, Morales MG, Mosqueira M, Valencia I, et al. Npc1 Deficiency in the C57BL/6J Genetic Background Enhances Niemann-Pick Disease Type C Spleen Pathology. Biochem Biophys Res Commun (2011) 413:400–6. doi: 10.1016/j.bbrc.2011.08.096
40. Seidi F, Jin Y, Xiao H. Polycyclodextrins: Synthesis, Functionalization, and Applications. Carbohydr Polym (2020) 242:116277. doi: 10.1016/j.carbpol.2020.116277
41. Kulkarni A, Caporali P, Dolas A, Johny S, Goyal S, Dragotto J, et al. Linear Cyclodextrin Polymer Prodrugs as Novel Therapeutics for Niemann-Pick Type C1 Disorder. Sci Rep (2018) 8:9547. doi: 10.1038/s41598-018-27926-9
Keywords: 2-hydroxypropyl-β-cyclodextrin, metabolic inflammation, cholesterol, hepatic inflammation, macrophage
Citation: Houben T, Yadati T, de Kruijf R, Gijbels MJJ, Luiken JJFP, van Zandvoort M, Kapsokalyvas D, Lütjohann D, Westerterp M, Plat J, Leake D and Shiri-Sverdlov (2021) Pro-Inflammatory Implications of 2-Hydroxypropyl-β-cyclodextrin Treatment. Front. Immunol. 12:716357. doi: 10.3389/fimmu.2021.716357
Received: 28 May 2021; Accepted: 23 July 2021;
Published: 20 August 2021.
Edited by:
Rudolf Lucas, Augusta University, United StatesReviewed by:
Éva Fenyvesi, CycloLab (Hungary), HungaryCopyright © 2021 Houben, Yadati, de Kruijf, Gijbels, Luiken, van Zandvoort, Kapsokalyvas, Lütjohann, Westerterp, Plat, Leake and Shiri-Sverdlov. This is an open-access article distributed under the terms of the Creative Commons Attribution License (CC BY). The use, distribution or reproduction in other forums is permitted, provided the original author(s) and the copyright owner(s) are credited and that the original publication in this journal is cited, in accordance with accepted academic practice. No use, distribution or reproduction is permitted which does not comply with these terms.
*Correspondence: Ronit Shiri-Sverdlov, ci5zdmVyZGxvdkBtYWFzdHJpY2h0dW5pdmVyc2l0eS5ubA==; orcid.org/0000-0002-6736-7814
†These authors have contributed equally to this work
Disclaimer: All claims expressed in this article are solely those of the authors and do not necessarily represent those of their affiliated organizations, or those of the publisher, the editors and the reviewers. Any product that may be evaluated in this article or claim that may be made by its manufacturer is not guaranteed or endorsed by the publisher.
Research integrity at Frontiers
Learn more about the work of our research integrity team to safeguard the quality of each article we publish.