- 1Department of Cardiovascular Surgery, Nanfang Hospital, Southern Medical University, Guangzhou, China
- 2Department of Burn Surgery and Skin Regeneration, The First People’s Hospital of Foshan, Foshan, China
- 3Department of Cardiothoracic Surgery, Nanjing Drum Tower Hospital, Nanjing University Medical School, Nanjing, China
- 4Laboratory Animal Research Center, Nanfang Hospital, Southern Medical University, Guangzhou, China
- 5Department of Cardiac Surgery, Heidelberg University Hospital, Heidelberg, Germany
- 6Department of Cardiac Surgery, University Hospital Halle (Saale), Halle, Germany
Although solid organ transplantation remains the definitive management for patients with end-stage organ failure, this ultimate treatment has been limited by the number of acceptable donor organs. Therefore, efforts have been made to expand the donor pool by utilizing marginal organs from donation after circulatory death or extended criteria donors. However, marginal organs are susceptible to ischemia-reperfusion injury (IRI) and entail higher requirements for organ preservation. Recently, machine perfusion has emerged as a novel preservation strategy for marginal grafts. This technique continually perfuses the organs to mimic the physiologic condition, allows the evaluation of pretransplant graft function, and more excitingly facilitates organ reconditioning during perfusion with pharmacological, gene, and stem cell therapy. As mesenchymal stem cells (MSCs) have anti-oxidative, immunomodulatory, and regenerative properties, mounting studies have demonstrated the therapeutic effects of MSCs on organ IRI and solid organ transplantation. Therefore, MSCs are promising candidates for organ reconditioning during machine perfusion. This review provides an overview of the application of MSCs combined with machine perfusion for lung, kidney, liver, and heart preservation and reconditioning. Promising preclinical results highlight the potential clinical translation of this innovative strategy to improve the quality of marginal grafts.
Background
Organ transplantation provides a life-saving opportunity for patients with end-stage organ failure. However, the existing donor pool is far from meeting the ever-growing demand for transplantable organs. One approach to alleviate the shortage of suitable organs has been the expansion of the deceased donor pool by utilizing marginal organs from donation after circulatory death (DCD) (1) and extended criteria donors (ECD) (2). However, marginal grafts are vulnerable to ischemia and have not enough physiological reserves to tolerate ischemia-reperfusion injury (IRI) during transplantation (3), leading to an increased incidence of primary graft dysfunction (PGD) and delayed graft function (DGF) (4, 5). Therefore, improving the quality of marginal organs will be a significant strategy to increase the source of donors.
In the process of organ transplantation, the donor grafts will be deprived of blood supply during procurement and suffer from a long duration of ischemia when the grafts are preserved and transported. The deprivation of blood supply leads to consumption of energy storage, anaerobic metabolism, as well as disorders in cellular activities. Toxic metabolites accumulate during ischemia and will exacerbate the oxidative injury and inflammation after reperfusion (6). The restoration of blood flow following engraftment aggravates cell death via complex cellular cascades and further worsens the quality of grafts (7). Therefore, efforts are needed to improve the quality of marginal organs by alleviating the IRI during transplantation.
Static cold storage (SCS) is the most widely used method for organ preservation, but in recent years, machine perfusion has emerged as a promising alternative to SCS (8). Machine perfusion can continually perfuse the organs to mimic the physiologic condition. Grafts can restore metabolism and even resume their function during perfusion, especially in the normothermic environment with the supply of substrates and oxygen (9). Machine perfusion is proposed to reduce the IRI with an improvement of graft quality and prolong organ preservation time, as well as allow objective evaluation for the viability of grafts during preservation (10). Another highlight of machine perfusion is offering a pivotal opportunity to recondition the high-risk grafts during preservation (11).
Mesenchymal stem cells (MSCs) have attracted tremendous attention due to their immunomodulatory property and regenerative effects. Current literature has suggested the beneficial effects of MSCs or their secretory factors in cases of organ IRI and solid organ transplantation (12). However, the majority of administered MSCs in animals or humans would be entrapped in lungs and could not survive for a long time (13, 14). Also, the high dosage of MSCs would lead to microvascular obstruction (15).
MSCs therapy and machine perfusion may supplement each other if combined properly, as machine perfusion can provide a separate platform and time window for MSCs to recondition the isolated grafts via the immunomodulatory and regenerative effect (9). In this review, we presented an overview of the current literature regarding the application of MSCs during machine perfusion on solid organ transplantation.
A Brief Introduction of IRI in Organ Transplantation
IRI is inevitable during organ transplantation. It occurs when the blood supply of grafts is stopped and restored. Hypoxia during ischemia leads to adenosine triphosphate (ATP) depletion and anaerobic metabolism, as well as subsequent disorders in membrane transport, calcium excretion, mitochondrial activity, and reactive oxygen species (ROS) turnover (16). Toxic metabolites accumulate during ischemia and induce further injury at the phase of reperfusion. For example, lactate, the production of anaerobic metabolism, leads to a drop in intracellular pH (17), while the accumulation of hypoxanthine can increase ROS production during reperfusion (18). The suddenly increased oxygen concentration during reperfusion results in a burst of ROS production, subsequently exacerbating the oxidative damage (17). The burst of ROS was validated to damage mitochondrial respiratory chain and metabolism enzymes further leading to more ROS production and impaired ATP production (19, 20). The excessive free radical also causes oxidative damage to the mitochondrial membrane and consequently increases mitochondrial permeability resulting in the release of pro-apoptotic factors to the cytoplasm (19). Inflammation is an important aspect of IRI. The danger-associated molecular patterns released by the injured cells could activate the innate immune responses via the Toll-like receptors (TLRs) and recruit immune cells (6). The activated immune responses initiate the production and release of inflammatory cytokines, and upregulation of endothelial adhesion molecules, which facilitates leukocyte adhesion and migration into grafts during reperfusion and further augments the inflammatory response (6, 21). The processive oxidative damage and inflammatory response ultimately lead to the activation of different cell death programs (apoptosis, necrosis, necroptosis, pyroptosis, and autophagy-associated cell death) (22). The pathophysiological process of IRI plays an important role in the deterioration of graft quality at the time of transplantation. Therefore, continuous efforts are necessary to alleviate the IRI of grafts during transplantation.
Machine Perfusion: A Promising Technique for Organ Preservation
SCS is introduced as a standard approach for organ preservation—grafts are cooled down and transported in the “ice box” (23). Hypothermia can slow down metabolic activity and then alleviate the impact of ischemia on grafts during preservation. Consequently, the grafts can tolerate a short time of ischemia with maintained cell viability (24). However, the remaining metabolism still leads to progressive damage with the decreased energy stores, acidosis, and ROS production (25). As marginal organs are vulnerable to IRI, SCS seems unable to meet the high preservation requirements of marginal grafts due to the anoxic environment (26).
In the past decades, the expanding donor pool has fueled the interest in machine perfusion. The machine perfusion system consists of a pump that maintains continuous perfusion to the grafts through the vasculature until engraftment (27). Machine perfusion can be simply divided into hypothermic machine perfusion (HMP) at 0-8°C, subnormothermic machine perfusion (SNMP) at 20-34°C, and normothermic machine perfusion (NMP) at 35-38°C (24).
HMP can keep the organs in a low metabolic state. The dynamic perfusion in the HMP system allows the supply of substrates and removal of toxic metabolites produced during preservation. Besides, if perfusate were actively oxygenated, HMP can further fulfill the remaining metabolic demand, restore tissue energy reserves and reduce oxidative stress (28, 29). Therefore, it is expected to minimize the IRI and allow a longer preservation time for grafts. In 2009, a landmark study conducted by Moers et al. demonstrated the superior potential of HMP to protect the deceased-donor kidneys from IRI compared with SCS (30) and prompted more and more researchers to focus on the area of machine perfusion and organ preservation (31–33).
Compared with HMP, NMP can provide an approximately physiological condition where the organs are kept at body temperature. With the continual supply of nutrients and oxygen, the organs can maintain an active metabolism so that blood-based perfusate is always necessary for the effective delivery of oxygen (34). The closer physiological microenvironment is thought to provide extra benefits. Urbanellis et al. showed that NMP significantly improved early renal function and alleviated renal IRI during preservation compared to HMP and SCS (35). A randomized trial also demonstrated that NMP was associated with less graft injury and discarded organs, and longer preservation time than SCS (36). In addition, NMP enables the grafts to resume their function. For example, livers can produce bile and lungs allow gas exchange while ventilated. Consequently, assessment of the function and viability of grafts during preservation comes to reality. It may assist the clinicians to evaluate whether the grafts are suitable for transplantation, which is especially necessary for the marginal organs (37). Glucose, pH, and biliary bicarbonate have been suggested as the indicators of bile duct injury during NMP of livers (38), while left ventricular end-systolic elastance is a prognostic factor for heart transplantation (39). As a midway approach between HMP and NMP, SNMP is expected to take advantage of reduced oxygen demand under subnormothermic conditions and ensure sufficient metabolism of the grafts for viability assessment (24, 40).
More importantly, machine perfusion provides an organ-repairing platform where pharmacological, gene and stem cell therapy can be administered to recondition and repair the grafts (9). Numerous agents combined with machine perfusion have been tested in preclinical studies, such as urokinase (41), prostaglandin E1 (PGE1) (42), steroids (43), and siRNAs (44).
The Features and Properties of MSCs
MSCs represent a heterogeneous population of multipotent stem cells, which are plastic adherent cells, express specific surface antigens and have the potential to differentiate into adipocytes, osteoblasts, and chondrocyte progenitors (45). MSCs can be isolated from many tissues, including adipose tissue, umbilical cords, and bone marrow. With few expressions of human leukocyte antigen, MSCs present with limited immunogenicity and are able to evade allogeneic immune response (6, 46). MSCs are well documented to exert antioxidant, immunomodulatory, and regenerative properties mainly by the direct interaction with adjacent cells and paracrine effects (12). The secretome of MSCs consists of cytokines, adhesion molecules, growth factors, and extracellular vesicles (EVs). EVs are membrane-packed vesicles including apoptotic bodies, exosomes, and microvesicles (MVs) (47). Containing a cargo of proteins and genetic materials, EVs participate in cell-to-cell communication via transferring the contents (48).
Both the immunoregulatory and regenerative roles make MSCs of great interest in ameliorating organ IRI (6). MSCs-derived exosomes can convert macrophages into anti-inflammatory phenotype, which releases immunosuppressive cytokines and regulates the T-regulatory phenotype (49, 50). Tryptophan is an essential amino acid necessary for T-cell proliferation. The indoleamine 2,3-dioxygenase secreted by MSCs could eliminate the tryptophan and subsequently affect the proliferation and apoptosis of T-cells (51, 52). Also, MSCs suppress TLR4-dependent activation of dendritic cells, leading to an inhibition of cytokine production and antigen presentation to T-cells (53). Furthermore, MSCs activate tissue repairing by releasing various growth factors such as vascular endothelial growth factor (VEGF), hepatocyte growth factor (HGF), fibroblast growth factor (FGF), keratinocyte growth factor (KGF), insulin-like growth factor-1, and stromal cell-derived factor-1α (6, 54).
The Potential of MSCs in Organ Reconditioning
Many studies have demonstrated the potential and underlying mechanism of MSCs to alleviate the organ IRI (Figure 1). Inflammation is an important aspect of IRI. MSCs can suppress the inflammatory response of renal IRI by inducing CD4+ Foxp3+ T-regulatory proliferation (55). MSCs can also improve hepatic IRI probably due to the suppressed transcription of inflammation-related genes within liver tissue, including high mobility group box chromosomal protein-1 (HMGB-1), interleukin-1β (IL-1β), and intercellular cell adhesion molecule-1 (ICAM-1) (56). As mentioned above, immune cell recruitment augments the inflammatory injury to the grafts at the reperfusion phase. Li et al. proved that MSCs ameliorated hepatic IRI predominantly via the inhibition of neutrophil migration and infiltration. They suggested MSCs not only reduced the neutrophil chemoattractant CXCL2 (CXC chemokine ligand-2) production in macrophages by suppressing nuclear factor κB (NF-κB) p65 phosphorylation but also promoted the p38 mitogen-activated protein kinase (MAPK) phosphorylation to decrease the CXCR2 (CXC chemokine receptor-2.) expression on the surface of neutrophils (57).
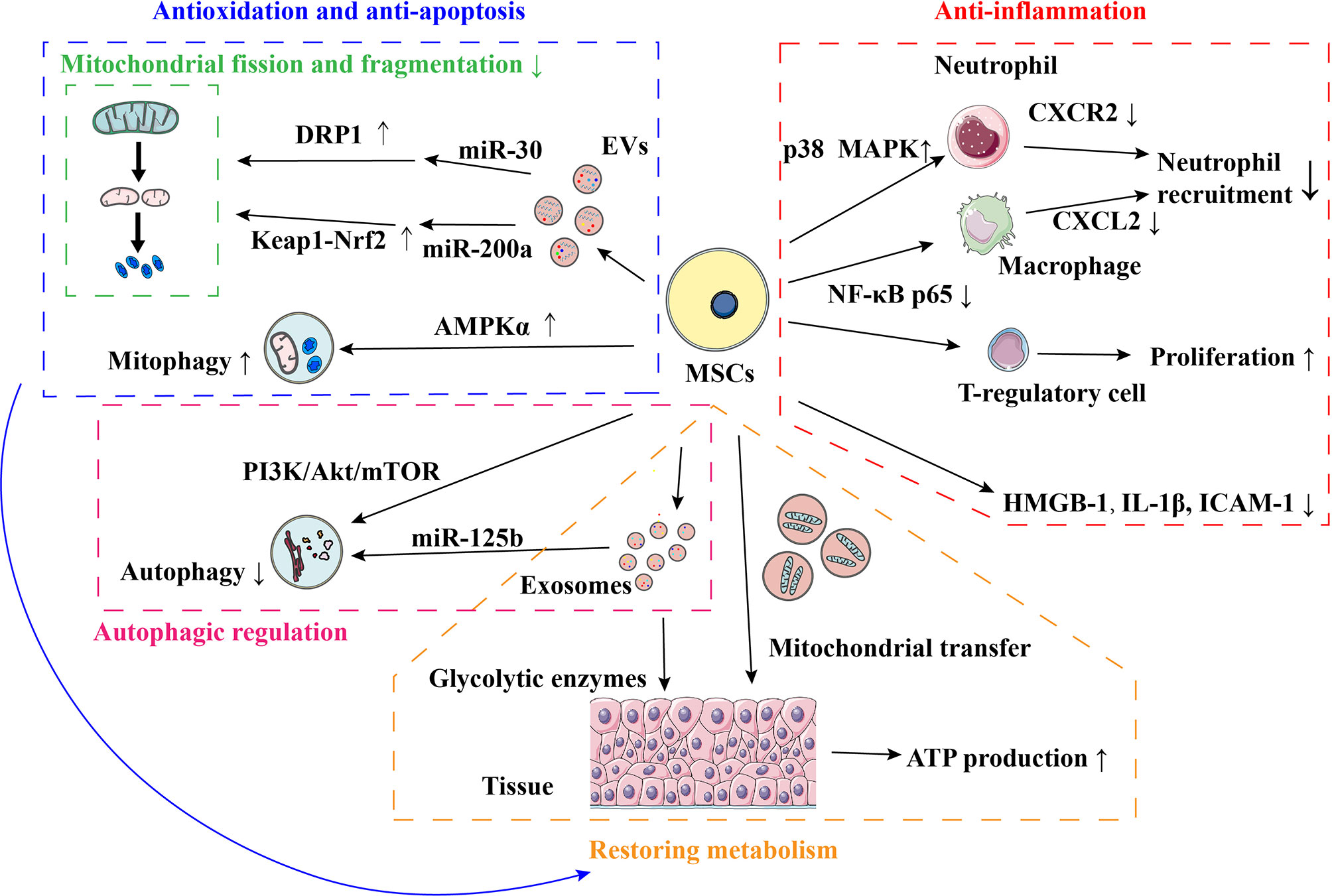
Figure 1 Mechanisms of MSCs in ameliorating organ IRI. AMPK, Adenosine monophosphate-activated protein kinase; ATP, Adenosine triphosphate; CXCL, CXC chemokine ligand; CXCR, CXC chemokine receptor; DRP1, Dynamin-related protein 1; EVs, Extracellular vesicles; HMGB-1, High mobility group box chromosomal protein-1; ICAM-1, Intercellular adhesion molecule-1; IRI, Ischemia-reperfusion injury; Keap1, Kelch-like ECH-associated protein 1; MAPK, Mitogen-activated protein kinase; NF-κB, Nuclear factor-Kb; Nrf2, NF-E2-related factor 2; IL, Interleukin; MSCs, Mesenchymal stem cells; PI3K, Phosphatidylinositol 3-kinase; mTOR, Mechanistic target of rapamycin.
MSCs were found to alleviate the organ IRI by improving metabolic activity. Lai et al. suggested that glycolytic enzymes were present in MSCs-derived exosomes and could be transferred to the reperfused myocardium to prompt the glycolytic flux and ATP production. They believed that the rapid ATP production could help the initiation of cellular processes in cardiac tissue immediately following reperfusion (58). Mitochondrial dysfunction plays an important role in the pathophysiological process of IRI, leading to excessive ROS generation, impaired ATP production, and apoptosis. Tseng et al. observed that mitochondria were transferred from MSCs to neurons in vitro after oxidative insult through cell-to-cell contact. The transfer of mitochondria recovered the metabolic activity in neurons with the improvement of mitochondrial respiration, basal metabolic rate, spare respiratory capacity, proton leak, and ATP production (59). Mitochondria constantly undergo fission and fusion and would shift to the fission state in response to IRI, leading to mitochondrial fragmentation and cell apoptosis (60, 61). Gu et al. uncovered that the administration of MSCs-derived EVs (MSC-EVs) immediately after reperfusion suppressed mitochondrial fission and subsequently mitochondrial apoptotic pathways in rat model of renal IRI. The inhibition of mitochondrial fission was probably mediated by miR-30 contained in MSC-EVs (61). Cao et al. suggested that MSC-EVs prompted renal repair after IRI by targeting and restoring mitochondrial function. They found that MSC-EVs protected kidneys from oxidative insult by reducing mitochondrial fragmentation and normalizing membrane potential. The transfer of miR-200a from MSCs was likely to increase mitochondrial antioxidant defense and ATP generation by activating the Keap1 (Kelch-like ECH-associated protein 1)/Nrf2 (NF-E2-related factor 2) pathway (62).
Autophagy participates in the process in IRI. It can be activated by ischemia, hypoxia, and nutrient deprivation to degrade and recycle the cytosolic proteins and damaged organelles for ATP production and protein synthesis (63). Xiao et al. observed a significantly reduced autophagic flux and apoptosis in infarcted mouse hearts treated with MSCs. They suggested the transplantation of MSCs after myocardial infarction could suppress the autophagic flux and cell death partially by exosomal transfer of miR-125b. In rat model of acute lung IRI, Lin et al. also found that the treatment of MSCs strongly downregulated the autophagic signaling pathway (64). As a special type of autophagy, mitophagy sequesters and eliminates unhealthy mitochondria (20). Anzell et al. deemed that clearance of unhealthy mitochondria is vital to avoid oxidative injury and apoptosis. In mouse liver with IRI, MSCs were administered immediately after reperfusion. Zheng et al. observed that the hepatoprotective effect of MSCs was accompanied by reduced mitochondrial ROS production, suppressed mitochondrial fragmentation, decreased apoptosis, and restored ATP generation. They suggested that MSCs upregulated the PTEN (phosphatase and tensin homolog)-induced putative kinase 1-dependent mitophagy to control the mitochondrial quality by adenosine monophosphate-activated protein kinase α (AMPKα) activation.
In recent years, MSCs have been suggested to recondition the donor grafts during transplantation. Montanari et al. found the intravenous infusion of MSCs to rats after heterotopic heart transplantation led to an early improvement in cardiac function and subsequent reduction in ventricular remodeling, cardiac fibrosis, and apoptosis in transplanted hearts (65). On the other hand, brain-dead donor hearts preserved by solution supplemented with MSCs or their secretome resulted in an improvement of posttransplant cardiac contractility and caspase-independent apoptosis (66, 67), while the hypoxic precondition could further enhance the beneficial effects of MSCs-derived secretome on apoptosis, histopathology, inflammation and functional performance to donor hearts after transplantation (68). Heme oxygenase-1 (HO-1) has shown the potential to inhibit oxidative stress (69), suppress inflammation (70), and enhance the quality of MSCs (71). Yang et al. delivered HO-1-transduced MSCs and MSCs respectively to rats immediately after liver transplantation. They found the HO-1 transduction could augment the positive impact of MSCs on the recovery of microcirculation and energy metabolism in transplanted livers (72).
MSCs are commonly infused through a peripheral vein to both animals and patients (73). But intravenous administration of MSCs or their secretome is facing the problem of entrapment in the lungs or absorption by other tissue with only a small proportion remaining in the target organs (13). Although a higher dosage may address this concern, the risk of microvascular embolism and side effects on other organs increase (74). Also, the infused MSCs have a lower long-term survival rate in the recipients (14). Machine perfusion provides an ideal platform for MSCs to directly recondition the target organs irrespective of the physiological barriers and adverse effects on other organs. Additionally, the amount of MSCs could be downregulated to avoid microvascular obstruction and MSCs could be protected from the whole immune system during perfusion, which guarantees their therapeutic effects on donor grafts. The combination of machine perfusion and MSCs is likely to have extra benefits to the grafts and is possible to convert the marginal organs to transplantable ones (Figure 2).
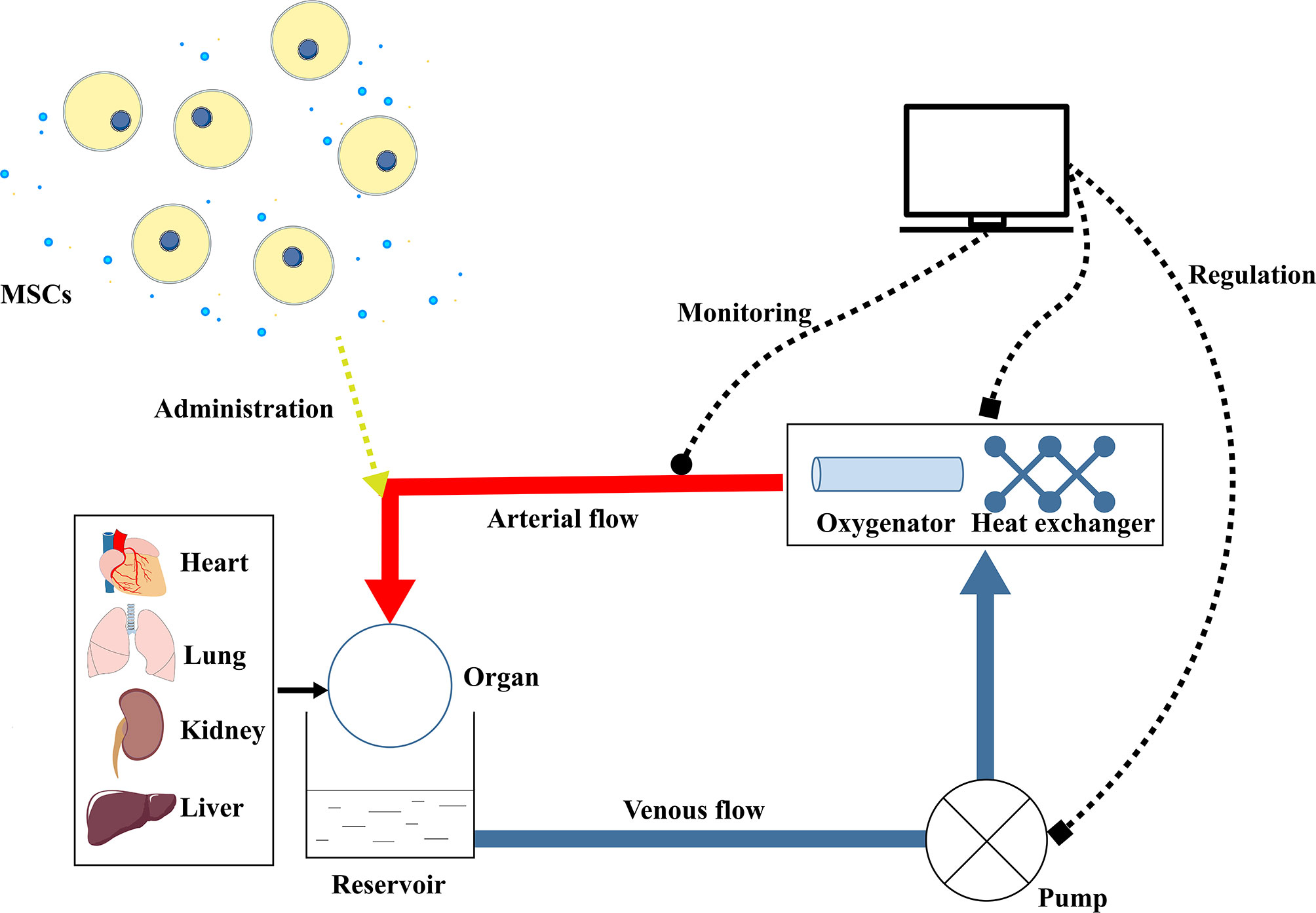
Figure 2 Schematic picture of MSCs combined with machine perfusion on organ preservation and reconditioning. The machine perfusion system consists of a pump, reservoir, oxygenator, and heat exchanger. The pump can maintain a continuous flow to the organ (heart, lung, kidney, or liver) through the vasculature. Recycled in the circuit, the perfusate is oxygenated by the oxygenator and kept at a certain temperature by the heat exchanger. The perfusate temperature, perfusion pressure, and perfusion flow rate are monitored and the pump and heat exchanger are accordingly regulated. During perfusion, MSCs or their secreted factors are administered in the perfusate to the isolated organ, which is expected to ameliorate the IRI and quality of the graft. IRI, Ischemia-reperfusion injury; MSCs, Mesenchymal stem cells.
Application of MSCs Combined With Machine Perfusion in Organ Transplantation
Recently, multiple efforts have been made to investigate the therapeutic effects of MSCs combined with machine perfusion on donated organs (Tables 1 and 2).
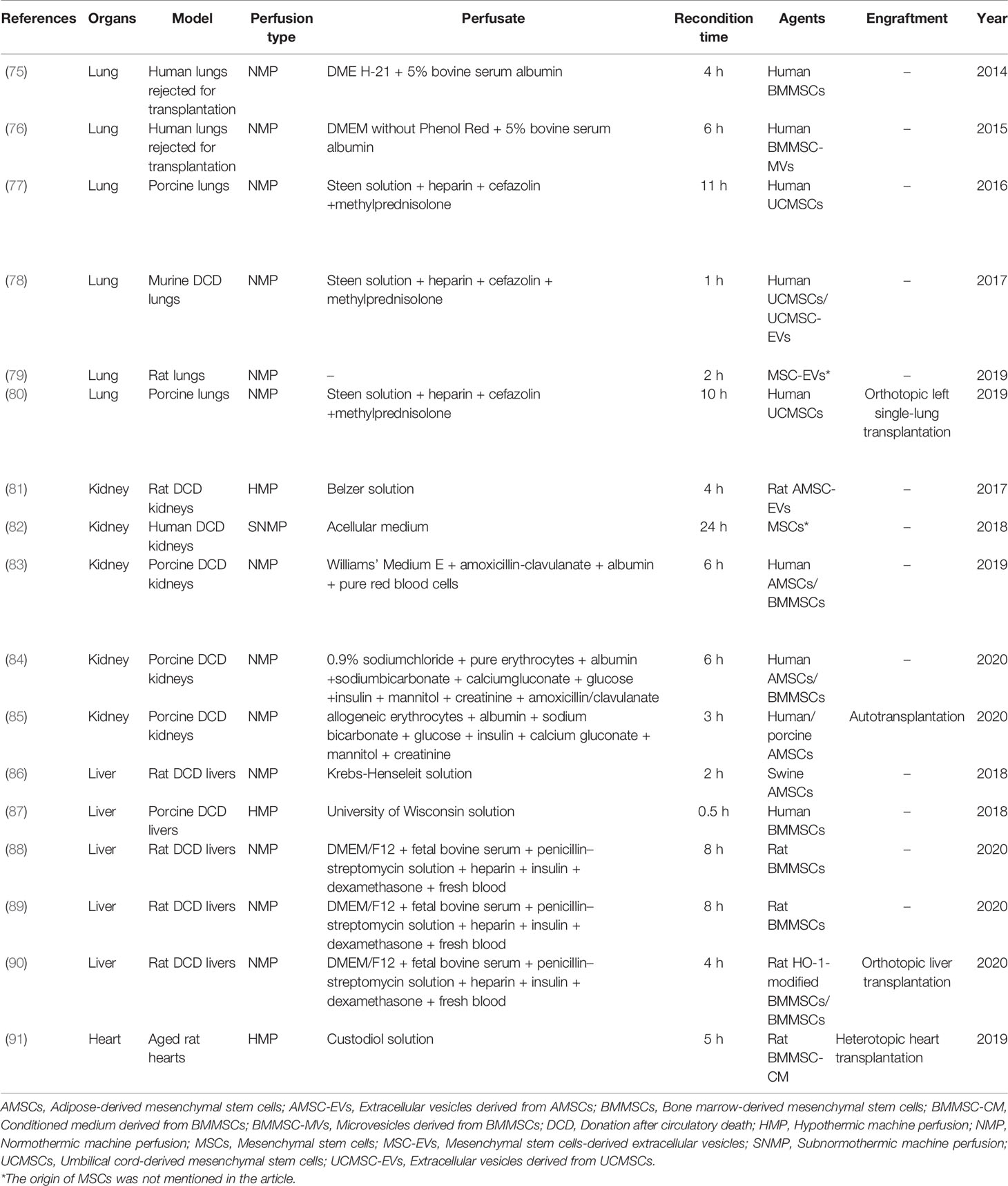
Table 1 Recent studies regarding the applications of MSCs during machine perfusion on organ transplantation.
MSCs and Machine Perfusion for Lung Transplantation
Since both pulmonary vascular and bronchial trees are direct access to the entire parenchyma (9), MSCs can be administered via the bronchus or the vasculature. In NMP of porcine lungs, Mordant et al. reported that 50×106 MSCs delivered through the pulmonary artery showed higher retention in the parenchyma and similar tolerance to the intrabronchial administration of the same dose (77). They also compared the impact of the bronchial fluid and perfusate (Steen fluid) harvested during NMP on the viability of MSCs in vitro. Equivalent to unadulterated Steen fluid, MSCs after 18-hour exposure to bronchial fluid presented lower viability than those exposed to the perfusate, suggesting bronchial fluid was not the ideal environment for MSCs and protective factors for the MSCs survival were released into the perfusate during perfusion (77). However, they didn’t compare the outcome of lungs exposed to MSCs under different administration routes. Lee et al. previously found that there was no difference between the intravascular and intrabronchial routes in the efficacy of MSCs on the isolated lungs injured by E. coli bacteria, which might be related to the paracrine effect of MSCs (92).
Additionally, concern about the microvascular embolism will be raised if MSCs are intravascularly administered during machine perfusion (15). The majority of MSCs administered in the perfusate retained in lungs within a few minutes and could be observed in both capillaries and the alveolar interstitium at the end of NMP (77, 80). Mordant et al. found that higher doses of MSCs were associated with higher retention of cells in the pig lungs. Also, 150×106 MSCs delivered during NMP were well tolerated without change in pulmonary vascular resistance and showed significant improvement in PaO2/FIO2 and static lung compliance. However, the double dose of MSCs was associated with increased pulmonary vascular resistance without benefits to pulmonary physiology. Accordingly, the optimal dose was suggested to be 5×106 MSCs per kilogram of animal weight (77).
Noncardiogenic pulmonary edema occurs in the early phase of PGD after lung transplantation (93). Alveolar fluid clearance (AFC) is defined as the ability of the lung to reabsorb the fluid in alveoli, which is dependent on an intact epithelial barrier (94). Accordingly, IRI may impair the integrity of epithelium and subsequent accumulation of fluid in alveoli may lead to pulmonary edema and reduced oxygenation (95, 96). In the human lungs rejected for transplantation, McAuley et al. demonstrated that the AFC could be normalized by MSCs in combination with NMP, partially mediated by KGF secretion (75). It was reported that exogenous KGF could increase the fluid transport capacity of the alveolar epithelium (97). In a model of acute lung injury, MSCs were proved to restore lung fluid balance by transferring KGF mRNA to the injured alveolar epithelium through the MVs (98). In line with previous studies, Gennai et al. found that the administration of MSCs-derived MVs (MSC-MVs) during NMP restored the AFC in a dose-dependent manner and decreased lung weight gain following perfusion. Additionally, the reduced perfusate level of syndecan-1 and the elevated level of angiotensin-I in the injured alveolus suggested a partial restoration of lung endothelium (76). Mordant et al. reported that the administration of MSCs during NMP could significantly decrease the perfusate level of IL-8 and increase the parenchymal concentration of VEGF (77), which was inversely correlated with alveolar epithelial damage (99). HGF has been shown to protect the integrity of endothelial junction and improve endothelial permeability (100, 101). Nakajima et al. suggested that HGF probably mediated the amelioration of pulmonary edema and lung injury after transplantation in the group treated with MSCs and NMP, since its concentration was high in both perfusate and lung tissue. Also, Nakajima et al. reported that MSCs administered during NMP could reduce the level of apoptosis, T-cell infiltration, and proinflammatory cytokines in lung tissue after transplantation. They inferred that the anti-inflammatory and anti-apoptotic effects of MSCs were pratially mediated by HGF (80), which could reduce the production of IL-12, IL-18, and tumor necrosis factor-α (TNF-α) (102), and inhibit apoptosis by regulating PI3K (phosphatidylinositol 3-kinase)/Akt and MAPK pathways (103).
Stone et al. found that NMP could improve pulmonary function and edema in murine models. Interestingly, MSCs and MSC-EVs could comparably and effectively enhance the protective and rehabilitative effects of NMP with significant improvement in pulmonary compliance and pulmonary artery pressure. The MSCs/MSC-EVs delivered to the lungs during NMP also attenuated neutrophil infiltration, pulmonary edema, and lung injury compared to NMP alone. They suggested that MSCs/MSC-EVs might present immunomodulatory and endothelial barrier-protective properties during NMP, via upregulating the anti-inflammatory molecules (IL-10, KGF, PGE2) expression, suppressing the activation of alveolar macrophages and invariant natural killer T cells, and mitigating neutrophil transendothelial migration (78). Besides, Lonati et al. found that MSC-EVs could induce the expression of various genes involved in anti-inflammatory response and resolution of oxidative stress in rat lungs during NMP. They showed that MSC-EVs could transfer hyaluronan into lung tissue and induce pulmonary production of hyaluronan during NMP (79). The transferred hyaluronan was primarily medium-high-molecular-weight, which was involved in the immunomodulatory and regenerative effects of MSCs (104). Similar to the study conducted by Gennai et al. (76), Lonati et al. found that the airway and hemodynamic parameters were significantly ameliorated in lungs treated with MSC-EVs during NMP, which might be attributed to the increased perfusate level of nitric oxide. Besides, the improvement of perfusate lactate and tissue ATP content in the group treated with MSC-EVs suggested the recovery of aerobic metabolism (79).
MSCs and Machine Perfusion for Kidney Transplantation
The high risk of primary nonfunction and DGF hinders the adoption of marginal grafts in kidney transplantation. Fortunately, previous studies have demonstrated the beneficial effects of HMP on the quality of high-risk renal grafts (105). Thereafter, Gregorini et al. proved the protective effect of MSCs/MSC-EVs combined with HMP on rat DCD kidneys. 3×106 MSCs were administered during HMP and detected in vessels, tubules, and interstitium of the kidneys at the end of 4-hour perfusion. The histologic evaluation showed that MSCs could significantly ameliorate the severe lesions (bleb formation, tubular necrosis, and tubular lumen obstruction) in DCD kidneys compared to those perfused only. Besides, genes involved in molecular transport, respiratory electron transport, and citric acid cycle were significantly up-regulated in the MSCs-treated group. They suggested that MSCs ameliorated the cellular metabolism and ischemic injury of DCD kidneys during HMP, as indicated by the increased pyruvate level and reduced perfusate level of lactate dehydrogenase, malonaldehyde (MDA), lactate, and glucose. More importantly, the MSC-EVs were found to ameliorate renal ischemic injury during HMP more effectively and more rapidly, which might be attributed to the prompt availability of MSCs mediators contained in EVs (81).
A does-effect study was conducted by Brasile et al. (82) to determine the optimal number of MSCs delivered to the discarded human kidneys during 24-hour SNMP. 25, 50, 75, 100, and 200×106 MSCs were respectively administered during SNMP (32℃) and 100×106 was suggested as the optimal dose which would not adversely affect the perfusion pressure, vascular flow, and oxygen consumption. The histologic examination demonstrated that the infused MSCs retained in the vasculature without migration to the renal parenchyma, which was quite different from the findings by Gregorini et al. (81). In their subsequent experiments, they showed that the addition of MSCs resulted in a more evident ATP storage, reduced perfusate level of proinflammatory cytokines, and increased synthesis of epidermal growth factor (EGF), FGF-2, and transforming growth factor-α in comparison to perfusion only. Furthermore, upregulation of cell proliferation in kidneys treated with MSCs during SNMP was observed. The increased synthesis of growth factors probably mediated the regenerative effect of MSCs on injured kidneys (82), as EGF and FGF-2 were proved to promote tubular regeneration (106), downregulate proinflammatory signaling (107), and attenuate renal IRI (108).
Moreover, a recent study has shown the feasibility of delivering MSCs during NMP to a porcine kidney. Kidneys were perfused with warm oxygenated blood-based perfusate for 7 hours and treated with different dosages of MSCs. Pool et al. showed that when the dosage was as high as 10×106, a proportion of the MSCs could be detected in lumen of glomerular capillaries with intact structure after NMP, which indicated that the infused MSCs might remain viable and functional. Intriguingly, MSCs did not retain in the most neighboring glomeruli. The magnetic resonance imaging also showed an inhomogeneous distribution of MSCs in the perfused kidneys, which might be resorted to the anatomical difference of microvasculature leading MSCs to the path with less resistance during perfusion (83). In their subsequent study, Pool et al. investigated the alteration of renal function and factors secreted into the perfusate after the addition of MSCs during NMP. They found that the delivery of 10×106 MSCs was not associated with very early renal function during NMP, but with increased levels of IL-6 and IL-8 in the perfusate. Besides, with fewer damage markers and more secretion of HGF in the perfusate, the perfused kidneys suffered less injury due to the addition of MSCs during NMP (84). Recently, the effect of MSCs combined with NMP on porcine kidneys after autotransplantation was investigated. Lohmann et al. found that the number of viable MSCs retaining in the transplanted kidneys dramatically dropped on postoperative day 14, and they failed to find the evidence of MSCs-induced recovery in transplanted kidneys, in line with the lack of improvement of renal function, early fibrosis markers and histology in the posttransplant phase (85).
MSCs and Machine Perfusion for Liver Transplantation
Recently, Verstegen et al. reported the bioluminescent imaging of infused MSCs in porcine livers during HMP. A wide range and patchy distribution of infused MSCs in livers were observed throughout the 30-minute HMP, regardless of the arterial or venous infusion (87). However, lacking is strong evidence to illustrate the therapeutic effect of MSCs combined with HMP on DCD livers. As for the application of MSCs during NMP on DCD livers, Sasajima et al. found that the addition of MSCs during NMP could improve the bile production and ameliorate the sinusoidal space narrower and hepatocellular vacuolation in rat DCD livers (86).
Based on the promising results, a series of studies regarding the application of MSCs combined with NMP on rat DCD livers have been conducted by a research group. In the experimental group, Yang et al. delivered MSCs through the portal vein to livers at the initiation of NMP. During 6-hour NMP, MSCs were found to continually colonize in the hepatic sinusoid. Compared to livers solely submitted to NMP, livers treated with MSCs released less alanine aminotransferase and aspartate aminotransferase and produced bile more effectively. Histopathology evaluation also showed a significant improvement in MSCs-treated livers, regarding apoptosis, liver swelling, hepatic sinusoid congestion, cell vacuolar degeneration, and inflammatory cell infiltration. The level of MDA and myeloperoxidase was significantly decreased in livers treated with MSCs, with an increase of glutathione level. The MSCs-treated livers also presented less mitochondrial damage. Yang et al. confirmed in subsequent experiments that the inhibition of c-Jun N-terminal kinase/NF-κB pathway and the AMPK activation might mediate the positive effects of MSCs on DCD livers during NMP, as these two pathways were involved in oxidative stress. Also, MSCs were found to improve the microcirculation of DCD livers during NMP, via suppressing macrophage activation, reducing ICAM expression, and ameliorating epithelial cell damage (88, 89). Furthermore, in a subsequent study, the preserved DCD livers were used for orthotopic liver transplantation. In line with previous studies, Cao et al. found MSCs could improve the functional and histopathological performance of DCD livers after transplantation compared to those only exposed to NMP. Also, the recipient survival time was dramatically prolonged in the MSCs-treated group. Interestingly, MSCs significantly attenuated the inflammatory response in DCD livers after transplantation, as indicated by the reduced level of IL-1β, IL-6, and TNF-α, and decreased expression of HMGB1 and TLR4/NF-κB pathway-related molecules. Therefore, they suggested that MSCs could promote the protective effect of NMP on DCD livers (90). Cao et al. subsequently reported that HO-1 transduction into MSCs could further improve the beneficial effects of MSCs combined with NMP on DCD livers, regarding liver function, histopathology, inflammation, and recipient survival time after transplantation (90).
MSCs and Machine Perfusion for Heart Transplantation
Hearts from the elderly have been regarded as a promising source of donor grafts (26). However, aging was associated with cardiac structural and functional deteriorations (109) and aged hearts were vulnerable to IRI (110). Recently, Korkmaz-Icöz et al. demonstrated HMP combined with MSCs-derived secretome could protect the donor hearts harvested from 15-month-old rats after prolonged storage. They found that the grafts harvested from the aged rats presented a significantly impaired left ventricular contractile function and relaxation compared with those from younger rats. Furthermore, HMP with perfusate supplemented with MSCs-derived secretome improved the posttransplant cardiac function of the aged grafts, via regulating the gene expression involved in apoptosis, inflammation, oxidative stress, and PI3K/Akt pathway (91). However, only one research has investigated the cardioprotective effect of machine perfusion with MSCs in donor heart preservation so far.
Concerns and Future Perspectives
The fate of MSCs after delivery to grafts during machine perfusion is worth considering. The administered MSCs are under the influence of temperature, perfusion pressure, flow rate, perfusate, and graft. Sierra Parraga et al. previously investigated the impact of perfusate on the attached and suspended MSCs in vitro. They found that both the suspension condition and blood-based perfusate affected the survival rate of MSCs and their adhesion ability to endothelial cells. Cultured by the perfusate, the attached MSCs showed higher viability compared to MSCs in suspension. Besides, although the perfusate induced an increase in the secretion of inflammatory cytokines from adherent MSCs, the secretory profile of MSCs was unaffected (111). In an NMP system without an organ in the circuit, the number of MSCs in suspension decreased over time and only approximately 10% of the cells remained detectable after 6 hours of perfusion (83). Notably, the suspension status is expected to be transient as MSCs can retain in the organs during machine perfusion. However, the retention of MSCs varies among organs. Nakajima et al. and Mordant et al. showed the majority of MSCs administered in the perfusate retained in lungs within a few minutes (77, 80), while Brasile et al. found MSCs remained predominately in the perfusate instead of the perfused kidney (82). Pool et al. showed MSCs could travel through the kidney and stop circulating over time, but only a small proportion of MSCs could be detected in the kidney (83). Further comprehensive research may be encouraged to figure out the impacts of machine perfusion on the administered MSCs.
In addition, the higher dosage of MSCs is associated with higher retention of cells in lungs (77), which can also apply to other organs. The optimal dosage of MSCs administered during machine perfusion was suggested under the basis of acellular perfusate (77, 82). The blood-based perfusate is always necessary for NMP in the clinic with a higher viscosity than acellular perfusion solution. Therefore, the optimal dosage of MSCs in the NMP would be probably limited by the viscosity of blood-based perfusate to avoid alteration of hemodynamic parameters. Since the application of MSCs has a risk of malignant development and microvascular embolism (15, 112) and MSCs rely more on the paracrine effect, further research may be encouraged to focus on the application of cell-free therapy combined with machine perfusion on organ preservation.
Another concern for the application of MSCs during machine perfusion is the heterogeneity of MSCs, a result of different donors, tissue sources, culture methods, and individual cells within a colonial population. The heterogeneity leads to disparities in surface markers, proliferation, differentiation potential, and secretory profile of MSCs from different sources (113). Sierra Parraga et al. demonstrated that the response of human MSCs to the NMP conditions was different from that of porcine MSCs. Human MSCs had higher resistance to suspension condition and better adhesion to endothelial cells in perfusion fluid than porcine MSCs. Additionally, human MSCs incubated in perfusate showed higher metabolic activity of mitochondria but more ROS production compared to porcine MSCs. What’s more, the impact of cryoprotection on MSCs was different between the human and porcine sources regarding survival, proliferation, adherent capacity, ROS production, and metabolic activity (111). Wilson et al. suggested that the cell populations were insufficiently defined in many studies and the MSCs heterogeneity was likely to compromise the reproducibility as well as the clinical translation of those researches (113). In the present review, contradictory results were found in studies focusing on the application of MSCs and machine perfusion on organ transplantation, especially on kidney transplantation. Therefore, the heterogeneity of MSCs may in part account for the contradictory findings. Considering MSCs heterogeneity is unavoidable, future studies are encouraged to present more detail about the origin and identification of MSCs to ensure the comparability of studies in this field. Besides, a better understanding of the mechanisms of MSCs in improving the graft quality can help determine the acceptable degree of MSCs heterogeneity by quantifying the active ingredients in future studies or even clinical practice (113).
Most of the aforementioned studies shared a common limitation that the long-term effect of MSCs combined with machine perfusion on grafts after transplantation was not evaluated. The impact of such an innovative strategy on the postoperative outcome should be investigated in further studies.
Conclusion
In conclusion, because of the ever-increasing demand for transplantable organs, enormous efforts have been made to expand the deceased donor pool. Mounting evidence has demonstrated the superiority of machine perfusion plus MSCs to improve the graft quality, especially the marginal organs. It is expectable for the further development of such an innovative strategy in organ transplantation and final application in the clinic.
Author Contributions
JL, GS, SZ and PYZ contributed to the conception and design of the study. JL, QP, RY and KL searched the literature, wrote the manuscript, and created the figure and table. PZ and YZ participated in drafting the manuscript. PYZ, GS and SZ revised the manuscript. All authors read and approved the final manuscript.
Funding
This research was supported by grants from Guangzhou Science and Technology Planning Project (No. 201804010067), Guangdong Science and Technology Planning Project (No. 2017ZC0064 and 2017A030303022), President Foundation of Nanfang Hospital, Southern Medical University (No. 2019c030), the Fellowship of China Postdoctoral Science Foundation (No. 2020M681559), and the National Natural Science Foundation of China (No. 82170274 and 82100410).
Conflict of Interest
The authors declare that the research was conducted in the absence of any commercial or financial relationships that could be construed as a potential conflict of interest.
The reviewer QL has declared a shared affiliation, with no collaboration, with several of the authors JL, QP, PZ, YZ, and SZ, to the handling Editor at the time of review.
Publisher’s Note
All claims expressed in this article are solely those of the authors and do not necessarily represent those of their affiliated organizations, or those of the publisher, the editors and the reviewers. Any product that may be evaluated in this article, or claim that may be made by its manufacturer, is not guaranteed or endorsed by the publisher.
Acknowledgments
Figures 1 and 2 were modified from Servier Medical Art (http://smart.servier.com/), licensed under a Creative Common Attribution 3.0 Generic License (https://creativecommons.org/licenses/by/3.0/).
Glossary
AFC: Alveolar fluid clearance
ALP: Alkaline phosphatase
ALT: Alanine aminotransferase
AMSCs: Adipose-derived mesenchymal stem cells
AMSC-EVs: Extracellular vesicles derived from AMSCs
AMPK: Adenosine monophosphate-activated protein kinase
AST: Aspartate aminotransferase
ATP: Adenosine triphosphate
BMMSCs: Bone marrow-derived mesenchymal stem cells
BMMSC-CM: Conditioned medium derived from BMMSCs
BMMSC-MVs: Microvesicles derived from BMMSCs
CXCL: CXC chemokine ligand
CXCR: CXC chemokine receptor
DCD: Donation after circulatory death
DGF: Delayed graft function
DRP1: Dynamin-related protein 1
ECD: Extended criteria donors
EGF: Epidermal growth factor
ET-1: Endothelin-1
EVs: Extracellular vesicles
FGF-2: Fibroblast growth factor-2
GGT: Glutamyl transpeptidase
GSH: Glutathione
HGF: Hepatocyte growth factor
HMGB1: High mobility group box chromosomal protein-1
HMP: Hypothermic machine perfusion
HO-1: Heme oxygenase-1
Keap1: Kelch-like ECH-associated protein 1
ICAM-1: Intercellular adhesion molecule-1
IFN-γ: Interferon- γ
IL: Interleukin
IRI: Ischemia-reperfusion injury
JNK: c-Jun N-terminal kinase
KGF: Keratinocyte growth factor
LDH: Lactate dehydrogenase
MAPK: Mitogen-activated protein kinase
MDA: Malonaldehyde
MPO: Myeloperoxidase
MSCs: Mesenchymal stem cells
MSC-EVs: Mesenchymal stem cells-derived extracellular vesicles
MSC-MVs: Mesenchymal stem cells-derived microvesicles
mTOR: Mechanistic target of rapamycin
MVs: Microvesicles
NF-κB: Nuclear factor-κB
NMP: Normothermic machine perfusion
NO: Nitric oxide
Nrf2: NF-E2-related factor 2
PAP: Pulmonary artery pressure
PAR: Pulmonary artery resistance
PCNA: Proliferating cell nuclear antigen
PGE: Prostaglandin E
PGD: Primary graft dysfunction
PI3K: Phosphatidylinositol 3-kinase
PTEN: Phosphatase and tensin homolog
ROS: Reactive oxygen species
SCS: Static cold storage
SNMP: Subnormothermic machine perfusion
TGF-α: Transforming growth factor-α
TLRs: Toll-like receptors
TNF-α: Tumor necrosis factor-α
UCMSCs: Umbilical cord-derived mesenchymal stem cells
UCMSC-EVs: Extracellular vesicles derived from UCMSCs
VCAM-1: Vascular cell adhesion molecule-1
VEGF: Vascular endothelial growth factor
vWF: Von willebrand factor
References
1. Smith M, Dominguez-Gil B, Greer DM, Manara AR, Souter MJ. Organ Donation After Circulatory Death: Current Status and Future Potential. Intensive Care Med (2019) 45:310–21. doi: 10.1007/s00134-019-05533-0
2. Vodkin I, Kuo A. Extended Criteria Donors in Liver Transplantation. Clinics Liver Dis (2017) 21:289–301. doi: 10.1016/j.cld.2016.12.004
3. Bonaccorsi-Riani E, Brüggenwirth IMA, Buchwald JE, Iesari S, Martins PN. Machine Perfusion: Cold Versus Warm, Versus Neither. Update on Clinical Trials. Semin Liver Dis (2020) 40(3):264–81. doi: 10.1055/s-0040-1713118
4. Tennankore KK, Kim SJ, Alwayn IPJ, Kiberd BA. Prolonged Warm Ischemia Time Is Associated With Graft Failure and Mortality After Kidney Transplantation. Kidney Int (2016) 89:648–58. doi: 10.1016/j.kint.2015.09.002
5. Botha P, Trivedi D, Weir CJ, Searl CP, Corris PA, Dark JH, et al. Extended Donor Criteria in Lung Transplantation: Impact on Organ Allocation. J Thorac Cardiovasc Surg (2006) 131:1154–60. doi: 10.1016/j.jtcvs.2005.12.037
6. Rowart P, Erpicum P, Detry O, Weekers L, Grégoire C, Lechanteur C, et al. Mesenchymal Stromal Cell Therapy in Ischemia/Reperfusion Injury. J Immunol Res (2015) 2015:602597. doi: 10.1155/2015/602597
7. Pickell Z, Williams AM, Alam HB, Hsu CH. Histone Deacetylase Inhibitors: A Novel Strategy for Neuroprotection and Cardioprotection Following Ischemia/Reperfusion Injury. J Am Heart Assoc (2020) 9:e016349. doi: 10.1161/JAHA.120.016349
8. O’Neill S, Srinivasa S, Callaghan CJ, Watson CJE, Dark JH, Fisher AJ, et al. Novel Organ Perfusion and Preservation Strategies in Transplantation - Where Are We Going in the United Kingdom? Transplantation (2020) 104:1813–24. doi: 10.1097/TP.0000000000003106
9. Van Raemdonck D, Neyrinck A, Rega F, Devos T, Pirenne J. Machine Perfusion in Organ Transplantation: A Tool for Ex-Vivo Graft Conditioning With Mesenchymal Stem Cells? Curr Opin Organ Transplant (2013) 18:24–33. doi: 10.1097/MOT.0b013e32835c494f
10. Xu J, Buchwald JE, Martins PN. Review of Current Machine Perfusion Therapeutics for Organ Preservation. Transplantation (2020) 104:1792–803. doi: 10.1097/TP.0000000000003295
11. Weissenbacher A, Vrakas G, Nasralla D, Ceresa CDL. The Future of Organ Perfusion and Re-Conditioning. Transpl Int (2019) 32:586–97. doi: 10.1111/tri.13441
12. Vandermeulen M, Erpicum P, Weekers L, Briquet A, Lechanteur C, Detry O, et al. Mesenchymal Stromal Cells in Solid Organ Transplantation. Transplantation (2020) 104:923–36. doi: 10.1097/TP.0000000000003077
13. Fischer UM, Harting MT, Jimenez F, Monzon-Posadas WO, Xue H, Savitz SI, et al. Pulmonary Passage Is a Major Obstacle for Intravenous Stem Cell Delivery: The Pulmonary First-Pass Effect. Stem Cells Dev (2009) 18:683–92. doi: 10.1089/scd.2008.0253
14. Eggenhofer E, Benseler V, Kroemer A, Popp FC, Geissler EK, Schlitt HJ, et al. Mesenchymal Stem Cells Are Short-Lived and do Not Migrate Beyond the Lungs After Intravenous Infusion. Front Immun (2012) 3:297. doi: 10.3389/fimmu.2012.00297
15. Gleeson BM, Martin K, Ali MT, Kumar AHS, Pillai MG-K, Kumar SPG, et al. Bone Marrow-Derived Mesenchymal Stem Cells Have Innate Procoagulant Activity and Cause Microvascular Obstruction Following Intracoronary Delivery: Amelioration by Antithrombin Therapy. Stem Cells (2015) 33:2726–37. doi: 10.1002/stem.2050
16. Fernández AR, Sánchez-Tarjuelo R, Cravedi P, Ochando J, López-Hoyos M. Review: Ischemia Reperfusion Injury-A Translational Perspective in Organ Transplantation. Int J Mol Sci (2020) 21(22):8549. doi: 10.3390/ijms21228549
17. Nieuwenhuijs-Moeke GJ, Pischke SE, Berger SP, Sanders JSF, Pol RA, Struys MMRF, et al. Ischemia and Reperfusion Injury in Kidney Transplantation: Relevant Mechanisms in Injury and Repair. J Clin Med (2020) 9(1):253. doi: 10.3390/jcm9010253
18. Battelli MG, Polito L, Bortolotti M, Bolognesi A. Xanthine Oxidoreductase-Derived Reactive Species: Physiological and Pathological Effects. Oxid Med Cell Longev (2016) 2016:3527579. doi: 10.1155/2016/3527579
19. Ma Z, Xin Z, Di W, Yan X, Li X, Reiter RJ, et al. Melatonin and Mitochondrial Function During Ischemia/Reperfusion Injury. Cell Mol Life Sci (2017) 74:3989–98. doi: 10.1007/s00018-017-2618-6
20. Lesnefsky EJ, Chen Q, Tandler B, Hoppel CL. Mitochondrial Dysfunction and Myocardial Ischemia-Reperfusion: Implications for Novel Therapies. Annu Rev Pharmacol Toxicol (2017) 57:535–65. doi: 10.1146/annurev-pharmtox-010715-103335
21. Duni A, Liakopoulos V, Koutlas V, Pappas C, Mitsis M, Dounousi E. The Endothelial Glycocalyx as a Target of Ischemia and Reperfusion Injury in Kidney Transplantation-Where Have We Gone So Far? Int J Mol Sci (2021) 22(4):2157. doi: 10.3390/ijms22042157
22. Quaglia M, Dellepiane S, Guglielmetti G, Merlotti G, Castellano G, Cantaluppi V. Extracellular Vesicles as Mediators of Cellular Crosstalk Between Immune System and Kidney Graft. Front Immunol (2020) 11:74. doi: 10.3389/fimmu.2020.00074
23. Pirenne J. Time to Think Out of the (Ice) Box. Curr Opin Organ Transplant (2010) 15:147–9. doi: 10.1097/MOT.0b013e328336959f
24. Jing L, Yao L, Zhao M, Peng L-P, Liu M. Organ Preservation: From the Past to the Future. Acta Pharmacol Sin (2018) 39:845–57. doi: 10.1038/aps.2017.182
25. Maathuis M-HJ, Leuvenink HGD, Ploeg RJ. Perspectives in Organ Preservation. Transplantation (2007) 83:1289–98. doi: 10.1097/01.tp.0000265586.66475.cc
26. Resch T, Cardini B, Oberhuber R, Weissenbacher A, Dumfarth J, Krapf C, et al. Transplanting Marginal Organs in the Era of Modern Machine Perfusion and Advanced Organ Monitoring. Front Immunol (2020) 11:631. doi: 10.3389/fimmu.2020.00631
27. Sierra-Parraga JM, Eijken M, Hunter J, Moers C, Leuvenink H, Møller B, et al. Mesenchymal Stromal Cells as Anti-Inflammatory and Regenerative Mediators for Donor Kidneys During Normothermic Machine Perfusion. Stem Cells Dev (2017) 26:1162–70. doi: 10.1089/scd.2017.0030
28. Venema LH, Brat A, Moers C, ‘t Hart NA, Ploeg RJ, Hannaert P, et al. Effects of Oxygen During Long-Term Hypothermic Machine Perfusion in a Porcine Model of Kidney Donation After Circulatory Death. Transplantation (2019) 103:2057–64. doi: 10.1097/TP.0000000000002728
29. Czigany Z, Lurje I, Schmelzle M, Schöning W, Öllinger R, Raschzok N, et al. Ischemia-Reperfusion Injury in Marginal Liver Grafts and the Role of Hypothermic Machine Perfusion: Molecular Mechanisms and Clinical Implications. J Clin Med (2020) 9(3):846. doi: 10.3390/jcm9030846
30. Moers C, Smits JM, Maathuis M-HJ, Treckmann J, van Gelder F, Napieralski BP, et al. Machine Perfusion or Cold Storage in Deceased-Donor Kidney Transplantation. N Engl J Med (2009) 360:7–19. doi: 10.1056/NEJMoa0802289
31. Tullius SG, García-Cardeña G. Organ Procurement and Perfusion Before Transplantation. N Engl J Med (2009) 360:78–80. doi: 10.1056/NEJMe0809215
32. Kox J, Moers C, Monbaliu D, Strelniece A, Treckmann J, Jochmans I, et al. The Benefits of Hypothermic Machine Preservation and Short Cold Ischemia Times in Deceased Donor Kidneys. Transplantation (2018) 102:1344–50. doi: 10.1097/TP.0000000000002188
33. Henry SD, Nachber E, Tulipan J, Stone J, Bae C, Reznik L, et al. Hypothermic Machine Preservation Reduces Molecular Markers of Ischemia/Reperfusion Injury in Human Liver Transplantation. Am J Transplant (2012) 12:2477–86. doi: 10.1111/j.1600-6143.2012.04086.x
34. Kaths JM, Paul A, Robinson LA, Selzner M. Ex Vivo Machine Perfusion for Renal Graft Preservation. Transplant Rev (Orlando) (2018) 32:1–9. doi: 10.1016/j.trre.2017.04.002
35. Urbanellis P, Hamar M, Kaths JM, Kollmann D, Linares I, Mazilescu L, et al. Normothermic Ex Vivo Kidney Perfusion Improves Early DCD Graft Function Compared With Hypothermic Machine Perfusion and Static Cold Storage. Transplantation (2020) 104:947–55. doi: 10.1097/TP.0000000000003066
36. Nasralla D, Coussios CC, Mergental H, Akhtar MZ, Butler AJ, Ceresa CDL, et al. A Randomized Trial of Normothermic Preservation in Liver Transplantation. Nature (2018) 557:50–6. doi: 10.1038/s41586-018-0047-9
37. Beuth J, Falter F, Pinto Ribeiro RV, Badiwala M, Meineri M. New Strategies to Expand and Optimize Heart Donor Pool: Ex Vivo Heart Perfusion and Donation After Circulatory Death: A Review of Current Research and Future Trends. Anesth Analg (2019) 128:406–13. doi: 10.1213/ANE.0000000000003919
38. Matton APM, de Vries Y, Burlage LC, van Rijn R, Fujiyoshi M, de Meijer VE, et al. Biliary Bicarbonate, Ph, and Glucose Are Suitable Biomarkers of Biliary Viability During Ex Situ Normothermic Machine Perfusion of Human Donor Livers. Transplantation (2019) 103:1405–13. doi: 10.1097/TP.0000000000002500
39. Xiao W, Xin L, Gao S, Peng Y, Luo J, Yao W, et al. Single-Beat Measurement of Left Ventricular Contractility in Normothermic Ex Situ Perfused Porcine Hearts. IEEE Trans BioMed Eng (2020) 67(12):3288–95. doi: 10.1109/TBME.2020.2982655
40. Bejaoui M, Pantazi E, Folch-Puy E, Baptista PM, García-Gil A, Adam R, et al. Emerging Concepts in Liver Graft Preservation. World J Gastroenterol (2015) 21:396–407. doi: 10.3748/wjg.v21.i2.396
41. Inci I, Zhai W, Arni S, Inci D, Hillinger S, Lardinois D, et al. Fibrinolytic Treatment Improves the Quality of Lungs Retrieved From Non-Heart-Beating Donors. J Heart Lung Transplant (2007) 26:1054–60. doi: 10.1016/j.healun.2007.07.033
42. Maida K, Akamatsu Y, Hara Y, Tokodai K, Miyagi S, Kashiwadate T, et al. Short Oxygenated Warm Perfusion With Prostaglandin E1 Administration Before Cold Preservation as a Novel Resuscitation Method for Liver Grafts From Donors After Cardiac Death in a Rat In Vivo Model. Transplantation (2016) 100:1052–8. doi: 10.1097/TP.0000000000001127
43. Martens A, Boada M, Vanaudenaerde BM, Verleden SE, Vos R, Verleden GM, et al. Steroids can Reduce Warm Ischemic Reperfusion Injury in a Porcine Donation After Circulatory Death Model With Ex Vivo Lung Perfusion Evaluation. Transpl Int (2016) 29:1237–46. doi: 10.1111/tri.12823
44. Thijssen MF, Brüggenwirth IMA, Gillooly A, Khvorova A, Kowalik TF, Martins PN. Gene Silencing With siRNA (RNA Interference): A New Therapeutic Option During Ex Vivo Machine Liver Perfusion Preservation. Liver Transpl (2019) 25:140–51. doi: 10.1002/lt.25383
45. Dominici M, Le Blanc K, Mueller I, Slaper-Cortenbach I, Marini F, Krause D, et al. Minimal Criteria for Defining Multipotent Mesenchymal Stromal Cells. The International Society for Cellular Therapy Position Statement. Cytotherapy (2006) 8:315–7. doi: 10.1080/14653240600855905
46. Amable P, Teixeira MV, Carias RB, Granjeiro J, Borojevic R. Protein Synthesis and Secretion in Human Mesenchymal Cells Derived From Bone Marrow, Adipose Tissue and Wharton’s Jelly. Stem Cell Res Ther (2014) 5:53. doi: 10.1186/scrt442
47. Keshtkar S, Azarpira N, Ghahremani MH. Mesenchymal Stem Cell-Derived Extracellular Vesicles: Novel Frontiers in Regenerative Medicine. Stem Cell Res Ther (2018) 9:63. doi: 10.1186/s13287-018-0791-7
48. Rani S, Ryan AE, Griffin MD, Ritter T. Mesenchymal Stem Cell-Derived Extracellular Vesicles: Toward Cell-Free Therapeutic Applications. Mol Ther (2015) 23:812–23. doi: 10.1038/mt.2015.44
49. Heo JS, Choi Y, Kim HO. Adipose-Derived Mesenchymal Stem Cells Promote M2 Macrophage Phenotype Through Exosomes. Stem Cells Int (2019) 2019:7921760. doi: 10.1155/2019/7921760
50. Deng S, Zhou X, Ge Z, Song Y, Wang H, Liu X, et al. Exosomes From Adipose-Derived Mesenchymal Stem Cells Ameliorate Cardiac Damage After Myocardial Infarction by Activating S1P/SK1/S1PR1 Signaling and Promoting Macrophage M2 Polarization. Int J Biochem Cell Biol (2019) 114:105564. doi: 10.1016/j.biocel.2019.105564
51. Gazdic M, Simovic Markovic B, Vucicevic L, Nikolic T, Djonov V, Arsenijevic N, et al. Mesenchymal Stem Cells Protect From Acute Liver Injury by Attenuating Hepatotoxicity of Liver Natural Killer T Cells in an Inducible Nitric Oxide Synthase- and Indoleamine 2,3-Dioxygenase-Dependent Manner. J Tissue Eng Regener Med (2018) 12:e1173–85. doi: 10.1002/term.2452
52. Li X, Xu Z, Bai J, Yang S, Zhao S, Zhang Y, et al. Umbilical Cord Tissue-Derived Mesenchymal Stem Cells Induce T Lymphocyte Apoptosis and Cell Cycle Arrest by Expression of Indoleamine 2, 3-Dioxygenase. Stem Cells Int (2016) 2016:7495135. doi: 10.1155/2016/7495135
53. Chiesa S, Morbelli S, Morando S, Massollo M, Marini C, Bertoni A, et al. Mesenchymal Stem Cells Impair In Vivo T-Cell Priming by Dendritic Cells. Proc Natl Acad Sci USA (2011) 108:17384–9. doi: 10.1073/pnas.1103650108
54. Sid-Otmane C, Perrault LP, Ly HQ. Mesenchymal Stem Cell Mediates Cardiac Repair Through Autocrine, Paracrine and Endocrine Axes. J Transl Med (2020) 18:336. doi: 10.1186/s12967-020-02504-8
55. Bai M, Zhang L, Fu B, Bai J, Zhang Y, Cai G, et al. IL-17A Improves the Efficacy of Mesenchymal Stem Cells in Ischemic-Reperfusion Renal Injury by Increasing Treg Percentages by the COX-2/PGE2 Pathway. Kidney Int (2018) 93:814–25. doi: 10.1016/j.kint.2017.08.030
56. Anger F, Camara M, Ellinger E, Germer C-T, Schlegel N, Otto C, et al. Human Mesenchymal Stromal Cell-Derived Extracellular Vesicles Improve Liver Regeneration After Ischemia Reperfusion Injury in Mice. Stem Cells Dev (2019) 28:1451–62. doi: 10.1089/scd.2019.0085
57. Li S, Zheng X, Li H, Zheng J, Chen X, Liu W, et al. Mesenchymal Stem Cells Ameliorate Hepatic Ischemia/Reperfusion Injury via Inhibition of Neutrophil Recruitment. J Immunol Res (2018) 2018:7283703. doi: 10.1155/2018/7283703
58. Lai RC, Yeo RWY, Tan KH, Lim SK. Mesenchymal Stem Cell Exosome Ameliorates Reperfusion Injury Through Proteomic Complementation. Regener Med (2013) 8:197–209. doi: 10.2217/rme.13.4
59. Tseng N, Lambie SC, Huynh CQ, Sanford B, Patel M, Herson PS, et al. Mitochondrial Transfer From Mesenchymal Stem Cells Improves Neuronal Metabolism After Oxidant Injury In Vitro: The Role of Miro1. J Cereb Blood Flow Metab (2021) 41:761–70. doi: 10.1177/0271678X20928147
60. Anzell AR, Maizy R, Przyklenk K, Sanderson TH. Mitochondrial Quality Control and Disease: Insights Into Ischemia-Reperfusion Injury. Mol Neurobiol (2018) 55:2547–64. doi: 10.1007/s12035-017-0503-9
61. Gu D, Zou X, Ju G, Zhang G, Bao E, Zhu Y. Mesenchymal Stromal Cells Derived Extracellular Vesicles Ameliorate Acute Renal Ischemia Reperfusion Injury by Inhibition of Mitochondrial Fission Through miR-30. Stem Cells Int (2016) 2016:2093940. doi: 10.1155/2016/2093940
62. Cao H, Cheng Y, Gao H, Zhuang J, Zhang W, Bian Q, et al. In Vivo Tracking of Mesenchymal Stem Cell-Derived Extracellular Vesicles Improving Mitochondrial Function in Renal Ischemia-Reperfusion Injury. ACS Nano (2020) 14:4014–26. doi: 10.1021/acsnano.9b08207
63. Zheng Y, Shi B, Ma M, Wu X, Lin X. The Novel Relationship Between Sirt3 and Autophagy in Myocardial Ischemia-Reperfusion. J Cell Physiol (2019) 234:5488–95. doi: 10.1002/jcp.27329
64. Lin K-C, Yeh J-N, Chen Y-L, Chiang JY, Sung P-H, Lee F-Y, et al. Xenogeneic and Allogeneic Mesenchymal Stem Cells Effectively Protect the Lung Against Ischemia-Reperfusion Injury Through Downregulating the Inflammatory, Oxidative Stress, and Autophagic Signaling Pathways in Rat. Cell Transplant (2020) 29:963689720954140. doi: 10.1177/0963689720954140
65. Montanari S, Dayan V, Yannarelli G, Billia F, Viswanathan S, Connelly KA, et al. Mesenchymal Stromal Cells Improve Cardiac Function and Left Ventricular Remodeling in a Heart Transplantation Model. J Heart Lung Transplant (2015) 34:1481–8. doi: 10.1016/j.healun.2015.05.008
66. Korkmaz-Icöz S, Li K, Loganathan S, Ding Q, Ruppert M, Radovits T, et al. Brain-Dead Donor Heart Conservation With a Preservation Solution Supplemented by a Conditioned Medium From Mesenchymal Stem Cells Improves Graft Contractility After Transplantation. Am J Transplant (2020) 20(10):2847–56. doi: 10.1111/ajt.15843
67. Wang M, Yan L, Li Q, Yang Y, Turrentine M, March K, et al. Mesenchymal Stem Cell Secretions Improve Donor Heart Function Following Ex Vivo Cold Storage. J Thorac Cardiovasc Surg (2020) S0022-5223(20)32487–9. doi: 10.1016/j.jtcvs.2020.08.095
68. Zhou P, Liu H, Liu X, Ling X, Xiao Z, Zhu P, et al. Donor Heart Preservation With Hypoxic-Conditioned Medium-Derived From Bone Marrow Mesenchymal Stem Cells Improves Cardiac Function in a Heart Transplantation Model. Stem Cell Res Ther (2021) 12:56. doi: 10.1186/s13287-020-02114-7
69. Wang Y, Yang C, Elsheikh NAH, Li C, Yang F, Wang G, et al. HO-1 Reduces Heat Stress-Induced Apoptosis in Bovine Granulosa Cells by Suppressing Oxidative Stress. Aging (Albany NY) (2019) 11:5535–47. doi: 10.18632/aging.102136
70. Ryter SW. Heme Oxygenase-1/Carbon Monoxide as Modulators of Autophagy and Inflammation. Arch Biochem Biophys (2019) 678:108186. doi: 10.1016/j.abb.2019.108186
71. Yu ZY, Ma D, He ZC, Liu P, Huang J, Fang Q, et al. Heme Oxygenase-1 Protects Bone Marrow Mesenchymal Stem Cells From Iron Overload Through Decreasing Reactive Oxygen Species and Promoting IL-10 Generation. Exp Cell Res (2018) 362:28–42. doi: 10.1016/j.yexcr.2017.10.029
72. Yang L, Shen Z-Y, Wang R-R, Yin M-L, Zheng W-P, Wu B, et al. Effects of Heme Oxygenase-1-Modified Bone Marrow Mesenchymal Stem Cells on Microcirculation and Energy Metabolism Following Liver Transplantation. World J Gastroenterol (2017) 23:3449–67. doi: 10.3748/wjg.v23.i19.3449
73. Alagesan S, Griffin MD. Autologous and Allogeneic Mesenchymal Stem Cells in Organ Transplantation: What do We Know About Their Safety and Efficacy? Curr Opin Organ Transplant (2014) 19:65–72. doi: 10.1097/MOT.0000000000000043
74. Cui L, Kerkelä E, Bakreen A, Nitzsche F, Andrzejewska A, Nowakowski A, et al. The Cerebral Embolism Evoked by Intra-Arterial Delivery of Allogeneic Bone Marrow Mesenchymal Stem Cells in Rats Is Related to Cell Dose and Infusion Velocity. Stem Cell Res Ther (2015) 6:11. doi: 10.1186/scrt544
75. McAuley DF, Curley GF, Hamid UI, Laffey JG, Abbott J, McKenna DH, et al. Clinical Grade Allogeneic Human Mesenchymal Stem Cells Restore Alveolar Fluid Clearance in Human Lungs Rejected for Transplantation. Am J Physiology-Lung Cell Mol Physiol (2014) 306:L809–15. doi: 10.1152/ajplung.00358.2013
76. Gennai S, Monsel A, Hao Q, Park J, Matthay MA, Lee JW. Microvesicles Derived From Human Mesenchymal Stem Cells Restore Alveolar Fluid Clearance in Human Lungs Rejected for Transplantation. Am J Transplant (2015) 15:2404–12. doi: 10.1111/ajt.13271
77. Mordant P, Nakajima D, Kalaf R, Iskender I, Maahs L, Behrens P, et al. Mesenchymal Stem Cell Treatment Is Associated With Decreased Perfusate Concentration of Interleukin-8 During Ex Vivo Perfusion of Donor Lungs After 18-Hour Preservation. J Heart Lung Transplant (2016) 35:1245–54. doi: 10.1016/j.healun.2016.04.017
78. Stone ML, Zhao Y, Robert Smith J, Weiss ML, Kron IL, Laubach VE, et al. Mesenchymal Stromal Cell-Derived Extracellular Vesicles Attenuate Lung Ischemia-Reperfusion Injury and Enhance Reconditioning of Donor Lungs After Circulatory Death. Respir Res (2017) 18:212. doi: 10.1186/s12931-017-0704-9
79. Lonati C, Bassani GA, Brambilla D, Leonardi P, Carlin A, Maggioni M, et al. Mesenchymal Stem Cell–Derived Extracellular Vesicles Improve the Molecular Phenotype of Isolated Rat Lungs During Ischemia/Reperfusion Injury. J Heart Lung Transplant (2019) 38:1306–16. doi: 10.1016/j.healun.2019.08.016
80. Nakajima D, Watanabe Y, Ohsumi A, Pipkin M, Chen M, Mordant P, et al. Mesenchymal Stromal Cell Therapy During Ex Vivo Lung Perfusion Ameliorates Ischemia-Reperfusion Injury in Lung Transplantation. J Heart Lung Transplant (2019) 38:1214–23. doi: 10.1016/j.healun.2019.07.006
81. Gregorini M, Corradetti V, Pattonieri EF, Rocca C, Milanesi S, Peloso A, et al. Perfusion of Isolated Rat Kidney With Mesenchymal Stromal Cells/Extracellular Vesicles Prevents Ischaemic Injury. J Cell Mol Med (2017) 21:3381–93. doi: 10.1111/jcmm.13249
82. Brasile L, Henry N, Orlando G, Stubenitsky B. Potentiating Renal Regeneration Using Mesenchymal Stem Cells. Transplantation (2019) 103:307–13. doi: 10.1097/TP.0000000000002455
83. Pool M, Eertman T, Sierra Parraga J, t Hart N, Roemeling-van Rhijn M, Eijken M, et al. Infusing Mesenchymal Stromal Cells Into Porcine Kidneys During Normothermic Machine Perfusion: Intact MSCs Can Be Traced and Localised to Glomeruli. IJMS (2019) 20:3607. doi: 10.3390/ijms20143607
84. Pool MBF, Vos J, Eijken M, van Pel M, Reinders MEJ, Ploeg RJ, et al. Treating Ischemically Damaged Porcine Kidneys With Human Bone Marrow- and Adipose Tissue-Derived Mesenchymal Stromal Cells During Ex Vivo Normothermic Machine Perfusion. Stem Cells Dev (2020) 29:1320–30. doi: 10.1089/scd.2020.0024
85. Lohmann S, Pool MBF, Rozenberg KM, Keller AK, Moers C, Møldrup U, et al. Mesenchymal Stromal Cell Treatment of Donor Kidneys During Ex Vivo Normothermic Machine Perfusion: A Porcine Renal Autotransplantation Study. Am J Transplant (2020) 21(7):2348–59. doi: 10.1111/ajt.16473
86. Sasajima H, Miyagi S, Kakizaki Y, Kamei T, Unno M, Satomi S, et al. Cytoprotective Effects of Mesenchymal Stem Cells During Liver Transplantation From Donors After Cardiac Death in Rats. Transplant Proc (2018) 50:2815–20. doi: 10.1016/j.transproceed.2018.02.180
87. Verstegen MMA, Mezzanotte L, Ridwan RY, Wang K, de Haan J, Schurink IJ, et al. First Report on Ex Vivo Delivery of Paracrine Active Human Mesenchymal Stromal Cells to Liver Grafts During Machine Perfusion. Transplantation (2020) 104:e5–7. doi: 10.1097/TP.0000000000002986
88. Yang L, Cao H, Sun D, Lin L, Zheng W-P, Shen Z-Y, et al. Normothermic Machine Perfusion Combined With Bone Marrow Mesenchymal Stem Cells Improves the Oxidative Stress Response and Mitochondrial Function in Rat Donation After Circulatory Death Livers. Stem Cells Dev (2020) 29(13):835–52. doi: 10.1089/scd.2019.0301
89. Yang L, Cao H, Sun D, Hou B, Lin L, Shen Z-Y, et al. Bone Marrow Mesenchymal Stem Cells Combine With Normothermic Machine Perfusion to Improve Rat Donor Liver Quality-the Important Role of Hepatic Microcirculation in Donation After Circulatory Death. Cell Tissue Res (2020) 381:239–54. doi: 10.1007/s00441-020-03202-z
90. Cao H, Yang L, Hou B, Sun D, Lin L, Song H-L, et al. Heme Oxygenase-1-Modified Bone Marrow Mesenchymal Stem Cells Combined With Normothermic Machine Perfusion to Protect Donation After Circulatory Death Liver Grafts. Stem Cell Res Ther (2020) 11:218. doi: 10.1186/s13287-020-01736-1
91. Korkmaz-Icöz S, Li S, Hüttner R, Ruppert M, Radovits T, Loganathan S, et al. Hypothermic Perfusion of Donor Heart With a Preservation Solution Supplemented by Mesenchymal Stem Cells. J Heart Lung Transplant (2019) 38:315–26. doi: 10.1016/j.healun.2018.12.003
92. Lee JW, Krasnodembskaya A, McKenna DH, Song Y, Abbott J, Matthay MA. Therapeutic Effects of Human Mesenchymal Stem Cells in Ex Vivo Human Lungs Injured With Live Bacteria. Am J Respir Crit Care Med (2013) 187:751–60. doi: 10.1164/rccm.201206-0990OC
93. Christie JD, Bavaria JE, Palevsky HI, Litzky L, Blumenthal NP, Kaiser LR, et al. Primary Graft Failure Following Lung Transplantation. Chest (1998) 114:51–60. doi: 10.1378/chest.114.1.51
94. Huppert LA, Matthay MA. Alveolar Fluid Clearance in Pathologically Relevant Conditions: In Vitro and In Vivo Models of Acute Respiratory Distress Syndrome. Front Immunol (2017) 8:371. doi: 10.3389/fimmu.2017.00371
95. den Hengst WA, Gielis JF, Lin JY, Van Schil PE, De Windt LJ, Moens AL. Lung Ischemia-Reperfusion Injury: A Molecular and Clinical View on a Complex Pathophysiological Process. Am J Physiol Heart Circ Physiol (2010) 299:H1283–1299. doi: 10.1152/ajpheart.00251.2010
96. Hidalgo MA, Shah KA, Fuller BJ, Green CJ. Cold Ischemia–Induced Damage to Vascular Endothelium Results in Permeability Alterations in Transplanted Lungs. J Thorac Cardiovasc Surg (1996) 112:1027–35. doi: 10.1016/S0022-5223(96)70104-6
97. Wang Y, Folkesson HG, Jayr C, Ware LB, Matthay MA. Alveolar Epithelial Fluid Transport can be Simultaneously Upregulated by Both KGF and Beta-Agonist Therapy. J Appl Physiol (1985) (1999) 87:1852–60. doi: 10.1152/jappl.1999.87.5.1852
98. Zhu Y-G, Feng X-M, Abbott J, Fang X-H, Hao Q, Monsel A, et al. Human Mesenchymal Stem Cell Microvesicles for Treatment of Escherichia Coli Endotoxin-Induced Acute Lung Injury in Mice. Stem Cells (2014) 32:116–25. doi: 10.1002/stem.1504
99. Fehrenbach A, Pufe T, Wittwer T, Nagib R, Dreyer N, Pech T, et al. Reduced Vascular Endothelial Growth Factor Correlates With Alveolar Epithelial Damage After Experimental Ischemia and Reperfusion. J Heart Lung Transplant (2003) 22:967–78. doi: 10.1016/s1053-2498(02)01157-9
100. Chen Q-H, Liu A-R, Qiu H-B, Yang Y. Interaction Between Mesenchymal Stem Cells and Endothelial Cells Restores Endothelial Permeability via Paracrine Hepatocyte Growth Factor In Vitro. Stem Cell Res Ther (2015) 6:44. doi: 10.1186/s13287-015-0025-1
101. Meng F, Meliton A, Moldobaeva N, Mutlu G, Kawasaki Y, Akiyama T, et al. Asef Mediates HGF Protective Effects Against LPS-Induced Lung Injury and Endothelial Barrier Dysfunction. Am J Physiol Lung Cell Mol Physiol (2015) 308:L452–463. doi: 10.1152/ajplung.00170.2014
102. Cao X-P, Han D-M, Zhao L, Guo Z-K, Xiao F-J, Zhang Y-K, et al. Hepatocyte Growth Factor Enhances the Inflammation-Alleviating Effect of Umbilical Cord-Derived Mesenchymal Stromal Cells in a Bronchiolitis Obliterans Model. Cytotherapy (2016) 18:402–12. doi: 10.1016/j.jcyt.2015.12.006
103. Xiao GH, Jeffers M, Bellacosa A, Mitsuuchi Y, Vande Woude GF, Testa JR. Anti-Apoptotic Signaling by Hepatocyte Growth Factor/Met via the Phosphatidylinositol 3-Kinase/Akt and Mitogen-Activated Protein Kinase Pathways. Proc Natl Acad Sci USA (2001) 98:247–52. doi: 10.1073/pnas.011532898
104. Kota DJ, Prabhakara KS, Cox CS, Olson SD. MSCs and Hyaluronan: Sticking Together for New Therapeutic Potential? Int J Biochem Cell Biol (2014) 55:1–10. doi: 10.1016/j.biocel.2014.07.022
105. Henry SD, Guarrera JV. Protective Effects of Hypothermic Ex Vivo Perfusion on Ischemia/Reperfusion Injury and Transplant Outcomes. Transplant Rev (Orlando) (2012) 26:163–75. doi: 10.1016/j.trre.2011.09.001
106. Tsurkan MV, Hauser PV, Zieris A, Carvalhosa R, Bussolati B, Freudenberg U, et al. Growth Factor Delivery From Hydrogel Particle Aggregates to Promote Tubular Regeneration After Acute Kidney Injury. J Control Release (2013) 167:248–55. doi: 10.1016/j.jconrel.2013.01.030
107. Zhang GH, Ichimura T, Wallin A, Kan M, Stevens JL. Regulation of Rat Proximal Tubule Epithelial Cell Growth by Fibroblast Growth Factors, Insulin-Like Growth Factor-1 and Transforming Growth Factor-Beta, and Analysis of Fibroblast Growth Factors in Rat Kidney. J Cell Physiol (1991) 148:295–305. doi: 10.1002/jcp.1041480216
108. Tan X, Tao Q, Li G, Xiang L, Zheng X, Zhang T, et al. Fibroblast Growth Factor 2 Attenuates Renal Ischemia-Reperfusion Injury via Inhibition of Endoplasmic Reticulum Stress. Front Cell Dev Biol (2020) 8:147. doi: 10.3389/fcell.2020.00147
109. Chiao YA, Rabinovitch PS. The Aging Heart. Cold Spring Harb Perspect Med (2015) 5:a025148. doi: 10.1101/cshperspect.a025148
110. Korkmaz S, Bährle-Szabó S, Loganathan S, Li S, Karck M, Szabó G. Marginal Donors: Can Older Donor Hearts Tolerate Prolonged Cold Ischemic Storage? Aging Clin Exp Res (2013) 25:597–600. doi: 10.1007/s40520-013-0131-9
111. Sierra Parraga JM, Rozenberg K, Eijken M, Leuvenink HG, Hunter J, Merino A, et al. Effects of Normothermic Machine Perfusion Conditions on Mesenchymal Stromal Cells. Front Immunol (2019) 10:765. doi: 10.3389/fimmu.2019.00765
112. Brasile L, Stubenitsky B. Will Cell Therapies Provide the Solution for the Shortage of Transplantable Organs? Curr Opin Organ Transplant (2019) 24:568–73. doi: 10.1097/MOT.0000000000000686
Keywords: mesenchymal stem cells, machine perfusion, ischemia-reperfusion injury, organ preservation, transplantation
Citation: Li J, Peng Q, Yang R, Li K, Zhu P, Zhu Y, Zhou P, Szabó G and Zheng S (2021) Application of Mesenchymal Stem Cells During Machine Perfusion: An Emerging Novel Strategy for Organ Preservation. Front. Immunol. 12:713920. doi: 10.3389/fimmu.2021.713920
Received: 24 May 2021; Accepted: 03 December 2021;
Published: 22 December 2021.
Edited by:
Brian Duncan Tait, The University of Melbourne, AustraliaReviewed by:
Sarah Hosgood, University of Cambridge, United KingdomQifa Liu, Southern Medical University, China
James Hunter, University of Oxford, United Kingdom
Copyright © 2021 Li, Peng, Yang, Li, Zhu, Zhu, Zhou, Szabó and Zheng. This is an open-access article distributed under the terms of the Creative Commons Attribution License (CC BY). The use, distribution or reproduction in other forums is permitted, provided the original author(s) and the copyright owner(s) are credited and that the original publication in this journal is cited, in accordance with accepted academic practice. No use, distribution or reproduction is permitted which does not comply with these terms.
*Correspondence: Shaoyi Zheng, emhzeUBzbXUuZWR1LmNu; Gábor Szabó, Z2Fib3Iuc3phYm9AdWstaGFsbGUuZGU=; Pengyu Zhou, cHl6aG91QHNtdS5lZHUuY24=
†These authors have contributed equally to this work and share first authorship