- 1Department of Immunology, University of Pittsburgh School of Medicine, Pittsburgh, PA, United States
- 2Tumor Microenvironment Center, University of Pittsburgh Medical Center (UPMC) Hillman Cancer Center, Pittsburgh, PA, United States
- 3Graduate Program of Microbiology and Immunology, University of Pittsburgh School of Medicine, Pittsburgh, PA, United States
- 4Cancer Immunology and Immunotherapy Program, UPMC Hillman Cancer Center, Pittsburgh, PA, United States
Regulatory T cells (Tregs) are key immunosuppressive cells that promote tumor growth by hindering the effector immune response. Tregs utilize multiple suppressive mechanisms to inhibit pro-inflammatory responses within the tumor microenvironment (TME) by inhibition of effector function and immune cell migration, secretion of inhibitory cytokines, metabolic disruption and promotion of metastasis. In turn, Tregs are being targeted in the clinic either alone or in combination with other immunotherapies, in efforts to overcome the immunosuppressive TME and increase anti-tumor effects. However, it is now appreciated that Tregs not only suppress cells intratumorally via direct engagement, but also serve as key interactors in the peritumor, stroma, vasculature and lymphatics to limit anti-tumor immune responses prior to tumor infiltration. We will review the suppressive mechanisms that Tregs utilize to alter immune and non-immune cells outside and within the TME and discuss how these mechanisms collectively allow Tregs to create and promote a physical and biological barrier, resulting in an immune-excluded or limited tumor microenvironment.
Introduction
Regulatory T cells (Tregs) are suppressive CD4+ T cells that are characterized, and largely regulated, by expression of the master transcription factor, forkhead box protein 3 (FoxP3) (1). Tregs are critical in the maintenance of peripheral tolerance to prevent autoimmune disease. During pathogenic insults, Tregs prevent overt immune activation in efforts to limit tissue damage. Tregs are also found in tumors with the ratio of Tregs to T cells positively correlating with poor prognosis and response to immunotherapy (2, 3). Strikingly, Treg depletion in murine tumor models results in complete tumor clearance, however these mice ultimately succumb to lethal systemic autoimmune disease (4–7). The drastic effect of Tregs on tumor growth has sparked interest in elucidating Treg function within the tumor microenvironment (TME) in efforts to selectively target tumor-infiltrating Tregs while sparing peripheral Tregs (8).
Immunotherapies designed to target intratumoral Tregs have focused on key surface markers that are highly expressed and contribute to their suppressive functions, such as CTLA-4, CD25, TIGIT, 4-1BB, OX-40, CCR4, and CCR8. Targeting these markers therapeutically has had some clinical success. The first FDA-approved immunotherapy utilized a blocking monoclonal antibody specific for cytotoxic T-lymphocyte-associated protein 4 (CTLA-4 or CD152) (ipilimumab), which preserves T cell activation via preventing CTLA-4 binding to CD28 thus allowing for CD28 engagement of CD80/86 (9). Currently, the complete mechanism for ipilimumab is not fully elucidated but may also involve depletion of Tregs via antibody-dependent cell-mediated cytotoxicity (ADCC) (10). Despite ipilimumab prolonging patient survival and increasing the five-year survival rate, 10-15% of patients experience Grade 3-4 immune-related adverse events, thus investigation of additional Treg-targeting strategies are warranted (11). Monoclonal antibodies against CD25, OX-40 and GITR have produced favorable anti-tumor effects, which were dependent on ADCC mediated Treg-depletion (12). Studies to uncover both novel molecules enriched on tumor infiltrating Tregs or mechanisms of suppression unique to the TME are warranted to improve targeted immunotherapy while limiting toxicity.
Tregs are found throughout the TME and can even exert suppressive function at a distance, forming physical, metabolic, and trafficking ‘barriers’ to exclude pro-inflammatory cells from the TME. These barriers can be both ‘physical’, by limiting the ability of effector T cells to enter into the tumor, and ‘functional’, by limiting the activity of effector cells already within the TME. Together, these barriers create an immune-excluded TME with studies showing that decreased CD8+ T cells, specifically, within the vicinity of tumor cells correlates with poor outcomes (13). The primary ‘barriers’ constructed by Tregs that prevent the infiltration of pro-inflammatory cells include poor activation of T cells in the periphery, disorganized vasculature, prevention of the formation of lymphatic structures in the TME and a stroma that hinders the migration of cells into and around the tumor bed (14, 15). These barriers of immune exclusion that Tregs erect will be discussed herein, starting with the tumor core and working outward through the peri-tumor to the stroma, ending with lymphatic structures and the periphery (Figure 1). Investigation of the pro-tumorigenic effects of Tregs in the whole tumor (non-micro) environment is necessary to elucidate novel therapeutic strategies to dismantle pro-tumor Tregs while maintaining peripheral tolerance.
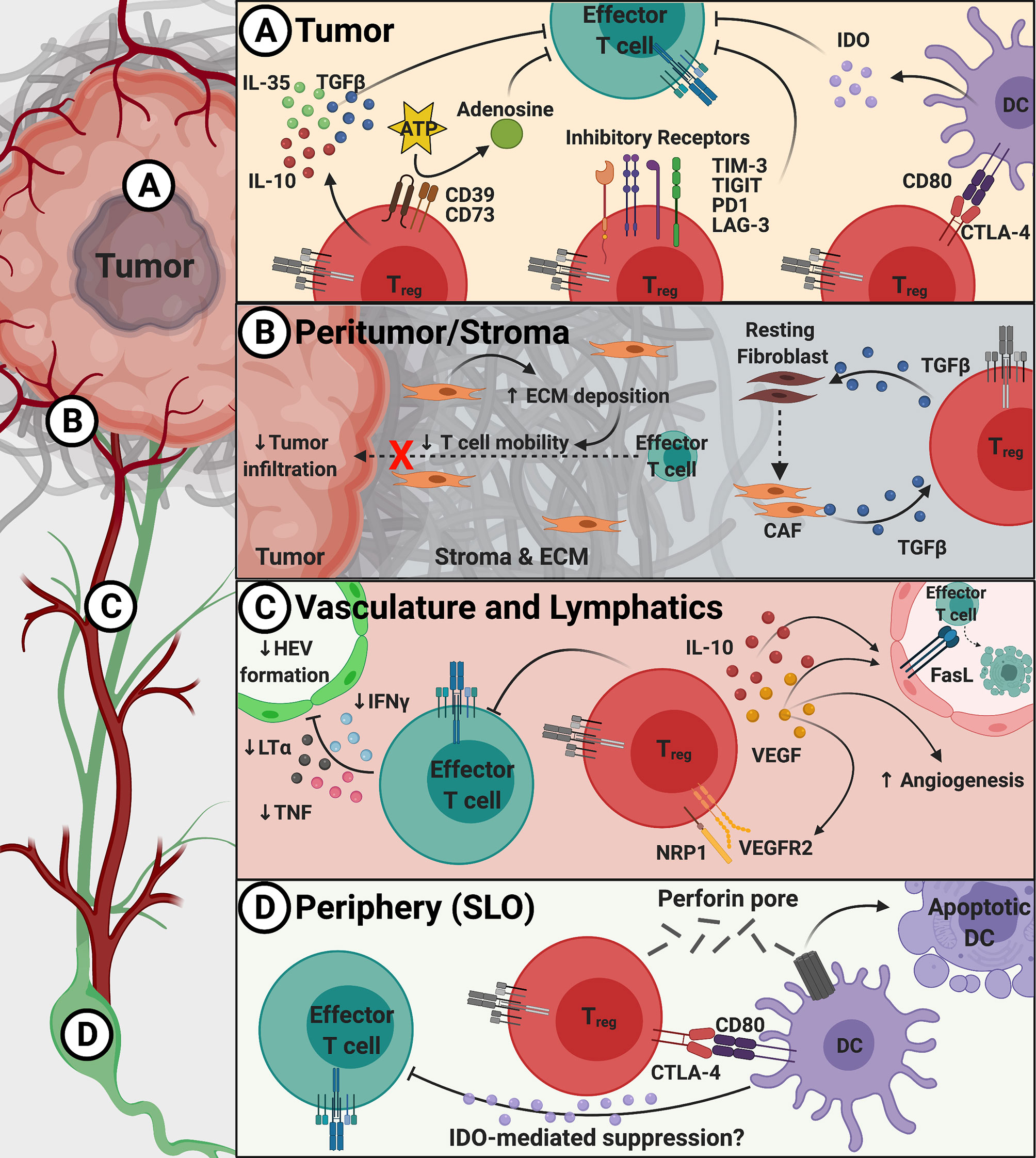
Figure 1 Overview of suppressive mechanisms used by Tregs to create barriers to immune infiltration into tumors. Panel (A) Within the TME, Tregs utilize inhibitory receptors (TIM-3, TIGIT, PD1, and LAG-3), inhibitory cytokines (TGFβ, IL-10, and IL-35), DC modulation (via CTLA-4 and LAG-3), and metabolic disruption (via CD39/CD73) to suppress the anti-tumor T cell response. (B) Treg-derived TGFβ induces cancer-associated fibroblast (CAF) development that increases extracellular matrix (ECM) production and deposition within the peritumoral space (stroma) to inhibit effector T cell migration. (C) Tregs block entry of effector T cells through preventing proper cytokine signals that promote high endothelial venule (HEV) formation as well as production of inhibitory IL-10 and VEGF to promote dysregulated angiogenesis. (D) In the periphery and secondary lymphoid organs (SLO), Tregs can modulate DC maturity and induce apoptosis to prevent proper effector T cell activation.
Tregs as Anti-Inflammatory Intratumoral Barriers
The frequency and organization of Tregs within the TME is diverse in cancer patients; however, high Treg infiltration often correlates with poor prognosis in many cancer types (16–18). The origin of these Tregs – either thymically (tTregs) or peripherally-derived (pTregs) – is still being debated (19). T cell receptor (TCR) sequencing studies in carcinogen-induced murine models and in human melanoma, gastrointestinal and ovarian cancers have shown distinct TCR sequences between intratumoral Tregs and FOXP3– conventional CD4+ T cells (20–22). A study using a genetically-driven prostate cancer murine model showed that intratumoral Tregs were thymically-derived, had less diverse TCRs, and had TCRs specific for the prostate tissue (23). Conversely, a recent study in breast cancer patients showed 65% TCR overlap of intratumoral Tregs with activated conventional CD4+ T cells (24). Overall, Treg conversion in the periphery and upon entry into the TME may be a rare event and may only be observed with the use of TCR transgenic mice or human tumors of specific tissue origins. However, having a TME that contains pTregs and/or tTregs may provide diverse functions (stability, effector and cytokine profile) that may provide a therapeutic opportunity to dedifferentiate Tregs to an unstable, non-immunosuppressive state (ex-Tregs) (25).
Tumors create an immunosuppressive environment that attracts Tregs and also support their anti-tumor function. Tumors secrete the CC chemokine ligand 22 (CCL22) and CCL17, which recruit Tregs to the tumor via Treg expression of the CC chemokine receptor 4 (CCR4) (26). Use of mogamulizumab (anti-CCR4) in patients with cutaneous T cell lymphoma or solid tumors, reduced the levels of circulating or intratumoral CCR4+ Tregs, respectively, but did not induce potent antitumor effects (27, 28). Combination of mogamulizumab with nivolumab (anti-PD1) in phase I clinical studies was tolerable and increased intratumoral CD8+ T cells and decreased Tregs in patients with solid tumors, making this therapeutic combination an effective option (29). Under hypoxic conditions, tumors secret CCL28 which recruits Tregs via CCR10 (30). Additionally, tumors secrete CCL5 which recruits Tregs via CCR5 and pre-clinical studies with CCR5 inhibitors have decreased Treg tumor infiltration and tumor growth (31, 32).
Conventionally, Tregs have higher affinity to self-antigen compared to other T cells which allows for suppression of autoreactive T cells and prevention of autoimmune disease. Tumors express self-antigens that are over expressed, inappropriately expressed, or mutated and preferentially promotes the activation and sequestration of Tregs as seen by the expansion of a few Treg clones specific for tumor antigens in cancer patients (33–35). A study using non-TCR transgenic mice showed that the TCRs of intratumoral Tregs are also found on Tregs from tumor draining lymph nodes (dLN), suggesting that Tregs are activated in the dLN, clonally expand, and migrate to the tumor where they accumulate (36). Although these data strongly suggest that Tregs recognize specific tumor antigens, albeit lower diversity compared to activated intratumoral conventional CD4+ cells, not all Tregs in the TME have tumor antigen-specific TCRs.
The high proliferation index of cancer cells creates a high energy demand, which forces the tumor to switch from oxidative phosphorylation to glycolysis (referred to as the Warburg effect), which generates a lactic acid-rich, glucose-poor, and hypoxic TME (37). Uptake of glucose by intratumoral Tregs promotes instability and loss of suppressive function. Instead, intratumoral Tregs upregulate pathways involved in lactic acid metabolism, and lactate uptake is required for maintenance of suppressive function of intratumoral, but not peripheral, Tregs (38). Mechanistically, Foxp3 promotes glycolysis via binding to the promoter of Myc and inducing expression (39). Deletion of hypoxia-inducible factor 2α (HIF-2α) from murine Tregs destabilized Tregs and prevented growth of MC38 colon adenocarcinoma (40). Collectively, consumption of glucose and oxygen by the proliferating tumor constructs a favorable metabolic landscape for Tregs to stably thrive in the TME.
Once in the tumor, Tregs suppress the anti-tumor response through contact-dependent and contact-independent mechanisms. Contact-dependent mechanisms utilizing CTLA-4, lymphocyte-activation gene 3 (LAG-3), and T cell immunoglobulin and ITIM domain (TIGIT) prevent activation and maturation of dendritic cells (DCs) thus preventing an effective anti-tumor T cell response (Figure 1A). CTLA-4 on Tregs binds CD80 molecules on DCs to induce transendocytosis and downregulation of CD80 expression and production of the inhibitory molecule indoleamine 2,3-dioxygenase (IDO) (41–44). While the intracellular domain of CTLA-4 is not thought to have a signaling function, it is important for the regulation of endocytosis and trafficking (45, 46). Specifically, a mouse model of Treg-specific CTLA-4 deletion resulted in fatal lymphoproliferative and autoimmune diseases while drastically limiting tumor progression, illustrating the importance of CTLA-4 in mediating Treg function through transendocytosis of CD80 and CD86 (44, 47, 48). LAG-3 binding to major histocompatibility complex class II on DCs reduces the expression levels of the costimulatory molecule CD86 and IL-12 cytokine production (49). TIGIT ligation of CD155 on DCs increased production of IL-10 and lowered IL-2, supporting an immunosuppressive environment (50, 51). While programmed cell death 1 (PD1) and T-cell immunoglobulin and mucin-domain containing-3 (TIM-3) are highly expressed on Tregs and important for suppressive function, the mechanisms are unknown (52, 53).
Contact-independent mechanisms of Tregs include the secretion of the inhibitory cytokines IL-10, IL-35, and transforming growth factor-β (TGFβ), which suppress the activity of effector cells (Figure 1A). IL-10 suppresses via inhibition of CD28 tyrosine phosphorylation and induction of CD8+ T cell exhaustion via upregulation of B lymphocyte-induced maturation protein-1 (BLIMP1) (54, 55). IL-35 limits the proliferation and memory formation, and promotes exhaustion in CD8+ T cells similarly to IL-10 by expression of BLIMP1 and downstream inhibitory receptors (54, 56). TGFβ decreases effector function via inhibiting the transcription of proinflammatory cytokines (interferon gamma [IFNγ]) and granzyme B, and T helper cell transcription factors (T-box transcription factor and GATA binding protein 3), although the precise mechanism of action remains unknown (57–60). While these activities represent the general role of TGFβ, it is important to understand that different isoforms may have differing functions based on the expression pattern in various cancers (61–63). Thus, secretion of these cytokines by Tregs acts as a functional ‘barrier’ that prevents the function and expansion of surrounding effector T cells.
Tregs in the TME also suppress anti-tumor immunity through metabolic disruption via CD25/IL-2, CD39/CD73, and IDO (Figure 1A). IL-2 is required for effector T cell differentiation and fate upon immune activation and is critical for the development, regulation, proliferation and maintenance of Tregs (64). Tregs express high levels of the IL-2 receptor, CD25, which also deprives surrounding effector T cells of IL-2 (65). Treg expression of the ectonucleotidases CD39 and CD73 convert ATP and ADP into adenosine, which suppresses effector T cells via the adenosine receptor 2A (66, 67). Interestingly, Treg ligation of CD80/CD86 on dendritic cells (DCs) via CTLA-4, increases the production of IDO (47) (Figure 1A). IDO metabolizes the essential amino acid tryptophan, limiting its availability, into different suppressive metabolites including kynurenine which inhibits T cell proliferation (43, 68). Despite promising findings in murine models and human in vitro studies, a Phase III clinical study with the IDO1 inhibitor epacadostat in combination with pembrolizumab (anti-PD1) in melanoma was disappointing (69). The lack of epacadostat efficacy in the clinic may be due to low initial levels of tryptophan and kynurenine in the TME, the presence of other enzymes able to catabolize tryptophan such as IDO2 and tryptophan 2,3-dioxygenase (TDO2), inefficient inhibition of IDO1, or adaptive resistance.
Through the expression of inhibitory receptors, inhibitory cytokines and metabolic disruptors, Tregs impose a terminal functional barrier within the TME to inhibit the infiltrated effector cells. However, Tregs also reside on the perimeter where the tumor meets the stroma (peritumor) and act as a functional and physical barrier to tumor immune infiltration.
Tregs as Peritumoral Anti-Inflammatory Barriers
The non-tumor cells within the TME make up the stromal compartment and include different lineages of fibroblasts that secrete various types and amounts of extracellular matrix (ECM) proteins that influence T cells migration. Among these proteins are fibronectin (FN) and collagen (COL), with COL being more abundant in the tumor stroma and having increased stiffness which impedes T cell motility (70). Tregs are found in the stroma of various tumors types and correlates with poor outcome (71–74) (Figure 1B). Using 3D in vitro culture of Tregs in a COL gel matrices, Treg markers were shown to be upregulated in high-density, compared to a low density, COL matrix, and also associated with decreased cytolytic activity (75). However, the interplay between Tregs and COL needs to be further defined. In a model of radiation-induced pulmonary fibrosis, Tregs promoted epithelium-to-mesenchymal transition (EMT) via β-catenin (76). In support of this, ectopic expression of Foxp3 by murine non-small cell lung cancer cells promoted EMT and tumor metastasis (77). Further studies to determine the direct role of Tregs in COL deposition and EMT are warranted.
IDO induces Treg differentiation through the generation of tryptophan metabolites and subsequent aryl hydrocarbon receptor signaling (78, 79). IDO inhibits effector T cell activity and it has been shown in gastric cancer cell lines to be associated with ECM, COL metabolic and catabolic processes. Specifically IDO1 and COL12A1 synergistically promoted cell migration in vitro (80). In a B16 melanoma model, the IDO1 inhibitor LW106 decreased tumor-associated stromal cells and COL deposition, and increased infiltration of effector cells. Additionally, LW106 decreased Tregs and delayed tumor growth, suggesting a potential role for Tregs in LW106 efficacy, however the direct impact of LW106 on Treg differentiation was undefined (81).
Fibroblasts isolated from tissue of invasive breast cancer patients had increased growth and invasion rate when treated with TGFβ, which was hypothesized to foster tumor invasion. Head and neck cancer patient-derived xenografts showed upregulation of TGFβ signaling in patients that progressed with cetuximab, an epidermal growth factor receptor inhibitor, compared to sensitive patients (82). This latter study showed elevated TGFβ1 signaling in cancer-associated fibroblasts (CAFs) in cetuximab progressors (83). In a model of pancreatic cancer, CAFs were found to express lower levels of Col and Fn1 mRNA when Tregs were deleted, which was accompanied by an increase in effector CD4+ and CD8+ T cell infiltration, and was proposed to result from the loss of Tgfb1 produced by Tregs (84). It is hypothesized that Treg production of TGFβ1 promotes fibroblast differentiation into CAFs (Figure 1B).
Collectively, these findings suggest a role for stromal Tregs in the promotion of COL and CAF formation, EMT and metastasis which creates a ‘rigid’ barrier to tumor immune infiltration. Ultimately, Tregs support an immunosuppressive stroma, and favor metastasis and disease progression. However, the mechanisms Tregs utilize to execute these pro-tumor effects and the therapeutic strategies to selectively inhibit these stromal Tregs, remain obscure.
Tregs as Barriers to Tumor Infiltration by Augmenting Tumor Angiogenesis
Blood supply into the TME is critical for the survival and growth of tumors, and angiogenesis positively correlates with disease progression (85). Metabolically active tumors utilize conserved angiogenic mechanisms found in wound healing to mediate growth of new blood vessels. Hallmarks of tumor vasculature includes disorganized and immature vessels that lack vessel hierarchy and have increased permeability (86). Additionally, lymphatic vessels in the TME are dilated and leaky, which results in the accumulation of fluid and waste products. However, functional lymphatics exist at the tumor margin and are sufficient to mediate metastasis (87). The consequences of these features include metastasis and poor delivery of cancer therapies, but of interest is the inability for tumor infiltration of anti-tumor immune cells.
Tumor angiogenesis is driven by high levels of pro-angiogenic molecules, such as members of the vascular endothelial growth factor (VEGF), platelet-derived growth factor (PDGF-B) and TGFβ families, as well as hypoxia (86) (Figure 1C). VEGF-A is produced upon binding of the hypoxia-inducible factor 1 (HIF-1) α and β heterodimer to the VEGF promoter (88). VEGF-A produced by intratumoral CCR10+ Tregs in a CCL28-expressing murine ovarian tumor model, increased angiogenesis and tumor growth (30). Similarly, Helios+ Tregs in a lymphoblastic leukemia model induced angiogenesis via the VEGF-VEGFR2 pathway (89). VEGF-C also utilizes VEGFR2 and VEGFR3 to induce lymphangiogenesis (90). Although Tregs do not produce VEGF-C, the lymphatic system represents another avenue in which Tregs prevent proper effector T cell tumor infiltration.
Another feature of tumor-associated vessels is the ability to communicate with the immune milieu. Endothelial cells induce Fas ligand (FasL) expression upon exposure to prostaglandin E2 (PGE2), hypoxia and Treg-produced VEGF and IL-10 to mediate T cell apoptosis (91, 92). Endothelial FasL preferentially kills CD8+ T cells, while sparing Tregs due to Treg expression of the anti-apoptotic gene, FADD-like IL-1β-converting enzyme (92) (Figure 1C). A feed-forward loop may exist in which VEGF-A and IL-10-producing Tregs in the TME promotes CD8+ T cell exclusion yet favors Treg infiltration, which further adds to the VEGF-A and IL-10 pools.
Targeting Tregs through inhibition of the VEGF pathway may be advantageous as Tregs not only produce, but also respond to, VEGF through expression of VEGFR2 and its co-receptor Neuropilin-1 (NRP1), the latter of which is highly expressed on murine and intratumoral human Tregs (93–95) (Figure 1C). Strikingly, a NRP1 antagonist increased CD8+ T cell infiltration and decreased tumor growth in a murine model (96, 97). The addition of a VEGF blocking antibody to a model of adoptive cell therapy led to increased tumor infiltration of transferred cells and a reduction in tumor growth (98). Use of the immunomodulatory drug thalidomide in chronic lymphocytic leukemia decreased NRP1 expression on Tregs, which may contribute to the reported antiangiogenic properties (99). However, efficacy of these therapies may vary depending on the organization and location of the blood vessels within and around the tumor bed. For example, location of the vasculature within the tumor, either throughout the tumor mass (tumor vessels) or within the stroma (stromal vessels), dictated the efficacy of VEGFR2-blocking antibodies, with only the former producing a significant anti-tumor response (100). In this study, stromal vessels mediated extravasation of immune cells directly to the stroma where they were trapped in the dense architecture surrounding the tumor mass, whereas tumor vessels mediated extravasation of immune cells directly to the tumor. The difference in therapeutic response may be attributed to the spatial distribution of vessels and Tregs and/or that this is simply reflective of a more immune-impacted tumor, which is known to be a positive prognostic indicator (101–103). Collectively, this may explain the seemingly paradoxical findings that Tregs may in certain circumstances appear to be a positive prognostic factor of survival
In summary, Tregs support pro-tumor angiogenesis in the TME via secretion of VEGF-A and IL-10, and expression of NRP1 (Figure 1C). Studies to further assess the impact of Tregs on the efficacy of VEGF/VEGFR inhibition/blockage and anti-NRP1, and the reorganization of the immune landscape of the TME post-therapy, will be critical to improving therapeutic response.
Tregs as Barriers to Immune Cell Egress in the Stroma and Periphery
Tregs are also found within tumor-associated tertiary lymphoid structures (TLS), in which case the positive prognostic value of mature TLS now predicts worse outcomes and relapse in many cancer types (104–106). Tregs in TLS of a lung adenocarcinoma model prevented an anti-tumor response, and Treg depletion resulted in increased proliferation and tumor infiltration of effector T cells (107). Similarly, CD8+ T cells and natural killer (NK) cells secrete IFNγ, tumor necrosis factor (TNF-α) and lymphotoxin α3, which induce neogenesis of high endothelial venules (HEV) that resemble lymph node (LN)-like vasculature and mediate T cell infiltration (108) (Figure 1C). A study showed that HEV formed when Tregs were depleted, and attributed HEV formation to increased TNF-α from T cells (109, 110) (Figure 1C). However, a study of colorectal cancer patients showed a positive correlation of TNF-α expression with positive LN stage and tumor recurrence (111). These studies illustrate the divergent role of lymphatics in the TME, thus more research is needed to understand the intricacies of TLS and HEV formation to therapeutically exploit their anti-tumoral role.
Tregs utilize unique mechanisms in the draining secondary lymphoid tissues to prevent recruitment to the TME. Tregs found in the peritumoral LN of a pancreatic ductal carcinoma model expressed CTLA-4, and CTLA-4/CD80 ligation with DCs inhibited conventional CD4+ T cell tumor infiltration (112). Although the mechanism is unclear, Treg : DC interaction decreases CD80/CD86 expression on DCs and induces production of IDO to suppress effector function (43, 47, 68) (Figure 1D). Similarly, Tregs utilize perforin to directly kill DCs in tumor-draining LN (113) (Figure 1D). Altogether, Treg suppression of DCs prevents effector T cell activation and lymphatic egress to the tumor site, thus promoting impaired anti-tumor immunity.
Collectively, Tregs in the stroma and periphery prevent tumor infiltration of immune cells by suppressing HEV formation, interfering with T cell activation by APCs and suppressing the production of proinflammatory cytokines by effector T cells. The anti-tumor effects seen with immunotherapies that block Treg-mediated suppression of the T cell/APC synapse and ultimately increase proinflammatory cytokines, may concurrently promote HEV formation and restructuring of the stroma, therefore the need for complimentary spatial and functional Treg studies is pertinent.
Conclusions
Tregs have diverse mechanisms to maintain tumor immune exclusion by affecting immune and non-immune cells, inside and outside of the tumor mass. Foundational studies interrogating intratumoral Tregs along with mechanisms of action for cancer immunotherapies have highlighted the impact intratumoral Tregs have on suppressing the anti-tumor response. However, mechanistic details of how to overcome these barriers are incomplete, leading to the following key questions:
(1) What is the extent of Treg and stromal cell interactions, and how do these interactions impact the composition of the stroma? Initial findings suggest that Tregs and stromal cells work together to prevent tumor immune infiltration via induction of CAFs by Treg-derived TGFβ. CAFs increase deposition of COL and FN and maintain Treg suppressive functions. However, it is unclear if CAFs and Tregs need to directly interact for this feedback loop to occur and if other signaling events are needed to establish this suppressive peritumoral barrier. If this is a contact-dependent mechanism, it may be advantageous to develop therapeutics (i.e. blocking antibodies or inhibitors) that prevent the interaction of these two cell types within the stroma.
(2) What are the mechanisms that retain Tregs in the stoma? CAFs support physical barriers that hinder effector T cells propagation in the stroma, where Tregs are abundant. Human TH2-like Tregs (GATA3+CCR4+) have the highest chemotaxis, viability and suppressive function, and are enriched in melanoma and colorectal cancer (114). GATA3 has been shown to bind to the promoter/enhancer of the IL-7 receptor and lL-7 signaling in Tregs is critical for development, expansion and peripheral homeostasis (115, 116). Additionally TGFβ promotes IL-7 receptor expression (117). One may then hypothesize that since CAFs produce IL-7, CAFs may support the proliferation of TH2-like Tregs in the stroma, thus maintaining an immunosuppressive stroma. Additionally, CAFs from hepatocellular carcinoma induce IDO in regulatory DCs, which promotes Treg proliferation (118). Collectively, these factors may provide a stromal environment favorable to Tregs, a notion strengthened by the observation that Treg-rich adenocarcinomas expressed higher TGFβ and VEGF which may reinforce Treg suppressive function and stability, respectively (119). These observations support the need for further investigation into the effects of anti-TGFβ and VEGF therapies on the stromal compartment and distribution of Tregs throughout. However, anti-VEGF therapy in this context may be detrimental if the stroma is heavily vascularized.
(3) Do Tregs utilize a common pathway to promote angiogenesis and lymphangiogenesis, and can this pathway be therapeutically inhibited to normalize tumor vascularization and increase immune infiltration? Peritumoral and intratumoral vasculature and lymphatics greatly dictates tumor infiltration of effector cells, however, specific mechanisms Tregs use to alter these structures is incomplete. Anti-angiogenic molecules in the clinic, such as sunitinib (receptor tyrosine kinase inhibitor) and bevacizumab (anti-VEGF), prevent the accumulation and function of Tregs by reducing their proliferative capacity and production of IL-10 and TGFβ, respectively (120, 121). As a VEGF co-receptor, NRP1 is a promising therapeutic as Treg-restricted deletion of NRP1 not only results in loss of suppressive function but also a gain of effector function via the expression of T-bet and production of IFNγ (95). The high expression of NRP1 by human tumoral Tregs in contrast to peripheral Tregs makes the VEGF/NRP1 axis a promising therapeutic target in order to normalize the vasculature and enhance effector T cell responses (122).
(4) Do Tregs utilize one suppressive mechanism preferentially to create multiple barriers to effective T cell infiltration, and if so, can this be targeted therapeutically to curtail multiple barriers of immune exclusion simultaneously? Tregs suppress the anti-tumor immune response through numerous mechanisms however, there are some recurring elements that when targeted could ameliorate multiple barriers (123). Of particular interest are IDO and NRP1. IDO inhibition may recruit peripheral effector T cells and reinvigorate intratumoral effector T cells, allowing for effective immune infiltration and anti-tumor activity, respectively. IDO inhibition in the peritumoral stroma may lower COL deposition which would increase the tumor infiltration of effector T cells. NRP1 blockade may lower the suppressive function of intratumoral Tregs and suppress angiogenesis. Targeting IDO and/or NRP1 may promote tumor infiltration and generate a less suppressive TME.
In summary, future studies must utilize mechanistic and spatial approaches to dissect the suppressive mechanisms employed by Tregs at various locations in the TME. These spatially-mapped functional studies will aid in the development of novel immunotherapies that aim to dismantle the Treg-induced physical, metabolic and trafficking barriers within the TME.
Author Contributions
ES, AG, CW, and DV wrote the article. All authors contributed to the article and approved the submitted version.
Funding
This work was supported by the National Institutes of Health [F32 CA247004 to AG, T32 CA082084 to AG and ES; P01 AI108545, R01 CA203689 and P30 CA047904 to DV].
Conflict of Interest
DV: cofounder and stockholder – Novasenta, Tizona and Trishula; stockholder – Oncorus, Werewolf and Apeximmune; patents licensed and royalties - Astellas, BMS; scientific advisory board member - Tizona, Werewolf, F-Star, Bicara, Apeximmune; consultant - Astellas, BMS, Incyte, Almirall, G1 Therapeutics; research funding – Novasenta, BMS and Astellas.
The remaining authors declare that the research was conducted in the absence of any commercial or financial relationships that could be construed as a potential conflict of interest.
Acknowledgments
We thank everyone in the DV Lab (Vignali-lab.com; @Vignali_Lab) for all their constructive comments and advice.
References
1. Hori S, Nomura T, Sakaguchi S. Control of Regulatory T Cell Development by the Transcription Factor Foxp3. Science (2003) 299:1057–61. doi: 10.1126/science.1079490
2. Xu T, Lu J, An H. The Relative Change in Regulatory T Cells / T Helper Lymphocytes Ratio as Parameter for Prediction of Therapy Efficacy in Metastatic Colorectal Cancer Patients. Oncotarget (2017) 8:109079–93. doi: 10.18632/oncotarget.22606
3. Solis-Castillo LA, Garcia-Romo GS, Diaz-Rodriguez A, Reyes-Hernandez D, Tellez-Rivera E, Rosales-Garcia VH, et al. Tumor-Infiltrating Regulatory T Cells, CD8/Treg Ratio, and Cancer Stem Cells Are Correlated With Lymph Node Metastasis in Patients With Early Breast Cancer. Breast Cancer (2020) 27:837–49. doi: 10.1007/s12282-020-01079-y
4. Bos PD, Plitas G, Rudra D, Lee SY, Rudensky AY. Transient Regulatory T Cell Ablation Deters Oncogene-Driven Breast Cancer and Enhances Radiotherapy. J Exp Med (2013) 210:2435–66. doi: 10.1084/jem.20130762
5. Shimizu J, Yamazaki S, Sakaguchi S. Induction of Tumor Immunity by Removing CD25+CD4+ T Cells: A Common Basis Between Tumor Immunity and Autoimmunity. J Immunol (1999) 163:5211–8.
6. Onizuka S, Tawara I, Shimizu J, Sakaguchi S, Fujita T, Nakayama E. Tumor Rejection by In Vivo Administration of Anti-CD25 (Interleukin-2 Receptor Alpha) Monoclonal Antibody. Cancer Res (1999) 59:3128–33.
7. Kim JM, Rasmussen JP, Rudensky AY. Regulatory T Cells Prevent Catastrophic Autoimmunity Throughout the Lifespan of Mice. Nat Immunol (2007) 8:191–7. doi: 10.1038/ni1428
8. Dadey RE, Workman CJ, Vignali DAA. Regulatory T Cells in the Tumor Microenvironment. Adv Exp Med Biol (2020) 1273:105–34. doi: 10.1007/978-3-030-49270-0_6
9. Krummel MF, Allison JP. CD28 and CTLA-4 Have Opposing Effects on the Response of T Cells to Stimulation. J Exp Med (1995) 182:459–65. doi: 10.1084/jem.182.2.459
10. Tang F, Du X, Liu M, Zheng P, Liu Y. Anti-CTLA-4 Antibodies in Cancer Immunotherapy: Selective Depletion of Intratumoral Regulatory T Cells or Checkpoint Blockade? Cell Biosci (2018) 8:30. doi: 10.1186/s13578-018-0229-z
11. Bowyer S, Prithviraj P, Lorigan P, Larkin J, McArthur G, Atkinson V, et al. Efficacy and Toxicity of Treatment With the Anti-CTLA-4 Antibody Ipilimumab in Patients With Metastatic Melanoma After Prior Anti-PD-1 Therapy. Br J Cancer (2016) 114:1084–9. doi: 10.1038/bjc.2016.107
12. Tanaka A, Sakaguchi S. Targeting Treg Cells in Cancer Immunotherapy. Eur J Immunol (2019) 49:1140–6. doi: 10.1002/eji.201847659
13. Joyce JA, Fearon DT. T Cell Exclusion, Immune Privilege, and the Tumor Microenvironment. Science (2015) 348:74–80. doi: 10.1126/science.aaa6204
14. Schaaf MB, Garg AD, Agostinis P. Defining the Role of the Tumor Vasculature in Antitumor Immunity and Immunotherapy. Cell Death Dis (2018) 9:115. doi: 10.1038/s41419-017-0061-0
15. Valkenburg KC, de Groot AE, Pienta KJ. Targeting the Tumour Stroma to Improve Cancer Therapy. Nat Rev Clin Oncol (2018) 15:366–81. doi: 10.1038/s41571-018-0007-1
16. Saito T, Nishikawa H, Wada H, Nagano Y, Sugiyama D, Atarashi K, et al. Two FOXP3(+)CD4(+) T Cell Subpopulations Distinctly Control the Prognosis of Colorectal Cancers. Nat Med (2016) 22:679–84. doi: 10.1038/nm.4086
17. deLeeuw RJ, Kost SE, Kakal JA, Nelson BH. The Prognostic Value of FoxP3+ Tumor-Infiltrating Lymphocytes in Cancer: A Critical Review of the Literature. Clin Cancer Res (2012) 18:3022–9. doi: 10.1158/1078-0432.CCR-11-3216
18. Sato E, Olson SH, Ahn J, Bundy B, Nishikawa H, Qian F, et al. Intraepithelial CD8+ Tumor-Infiltrating Lymphocytes and a High CD8+/Regulatory T Cell Ratio Are Associated With Favorable Prognosis in Ovarian Cancer. Proc Natl Acad Sci USA (2005) 102:18538–43. doi: 10.1073/pnas.0509182102
19. Abbas AK, Benoist C, Bluestone JA, Campbell DJ, Ghosh S, Hori S, et al. Regulatory T Cells: Recommendations to Simplify the Nomenclature. Nat Immunol (2013) 14:307–8. doi: 10.1038/ni.2554
20. Hindley JP, Ferreira C, Jones E, Lauder SN, Ladell K, Wynn KK, et al. Analysis of the T-Cell Receptor Repertoires of Tumor-Infiltrating Conventional and Regulatory T Cells Reveals No Evidence for Conversion in Carcinogen-Induced Tumors. Cancer Res (2011) 71:736–46. doi: 10.1158/0008-5472.CAN-10-1797
21. Ahmadzadeh M, Pasetto A, Jia L, Deniger DC, Stevanović S, Robbins PF, et al. Tumor-Infiltrating Human CD4+ Regulatory T Cells Display a Distinct TCR Repertoire and Exhibit Tumor and Neoantigen Reactivity. Sci Immunol (2019) 4:eaao4310. doi: 10.1126/sciimmunol.aao4310
22. Pacholczyk R, Kern J. The T-Cell Receptor Repertoire of Regulatory T Cells. Immunology (2008) 125:450–8. doi: 10.1111/j.1365-2567.2008.02992.x
23. Malchow S, Leventhal DS, Nishi S, Fischer BI, Shen L, Paner GP, et al. Aire-Dependent Thymic Development of Tumor-Associated Regulatory T Cells. Science (2013) 339:1219–24. doi: 10.1126/science.1233913
24. Xydia M, Rahbari R, Ruggiero E, Macaulay I, Tarabichi M, Lohmayer R, et al. Common Clonal Origin of Conventional T Cells and Induced Regulatory T Cells in Breast Cancer Patients. Nat Commun (2021) 12:1119. doi: 10.1038/s41467-021-21297-y
25. Burocchi A, Colombo MP, Piconese S. Convergences and Divergences of Thymus- and Peripherally Derived Regulatory T Cells in Cancer. Front Immunol (2013) 4:247. doi: 10.3389/fimmu.2013.00247
26. Lee GR. Phenotypic and Functional Properties of Tumor-Infiltrating Regulatory T Cells. Mediators Inflammation (2017) 2017:5458178. doi: 10.1155/2017/5458178
27. Ni X, Jorgensen JL, Goswami M, Challagundla P, Decker WK, Kim YH, et al. Reduction of Regulatory T Cells by Mogamulizumab, a Defucosylated anti-CC Chemokine Receptor 4 Antibody, in Patients With Aggressive/Refractory Mycosis Fungoides and Sézary Syndrome. Clin Cancer Res (2015) 21:274–85. doi: 10.1158/1078-0432.CCR-14-0830
28. Zamarin D, Hamid O, Nayak-Kapoor A, Sahebjam S, Sznol M, Collaku A, et al. Mogamulizumab in Combination With Durvalumab or Tremelimumab in Patients With Advanced Solid Tumors: A Phase I Study. Clin Cancer Res (2020) 26:4531–41. doi: 10.1158/1078-0432.CCR-20-0328
29. Doi T, Muro K, Ishii H, Kato T, Tsushima T, Takenoyama M, et al. A Phase I Study of the Anti-CC Chemokine Receptor 4 Antibody, Mogamulizumab, in Combination With Nivolumab in Patients With Advanced or Metastatic Solid Tumors. Clin Cancer Res (2019) 25:6614–22. doi: 10.1158/1078-0432.CCR-19-1090
30. Facciabene A, Peng X, Hagemann IS, Balint K, Barchetti A, Wang L-P, et al. Tumour Hypoxia Promotes Tolerance and Angiogenesis Via CCL28 and T(reg) Cells. Nature (2011) 475:226–30. doi: 10.1038/nature10169
31. Tan MCB, Goedegebuure PS, Belt BA, Flaherty B, Sankpal N, Gillanders WE, et al. Disruption of CCR5-Dependent Homing of Regulatory T Cells Inhibits Tumor Growth in a Murine Model of Pancreatic Cancer. J Immunol (2009) 182:1746–55. doi: 10.4049/jimmunol.182.3.1746
32. de Oliveira CE, Gasparoto TH, Pinheiro CR, Amôr NG, Nogueira MRS, Kaneno R, et al. Ccr5-Dependent Homing of T Regulatory Cells to the Tumor Microenvironment Contributes to Skin Squamous Cell Carcinoma Development. Mol Cancer Ther (2017) 16:2871–80. doi: 10.1158/1535-7163.MCT-17-0341
33. Wang HY, Lee DA, Peng G, Guo Z, Li Y, Kiniwa Y, et al. Tumor-Specific Human CD4+ Regulatory T Cells and Their Ligands: Implications for Immunotherapy. Immunity (2004) 20:107–18. doi: 10.1016/s1074-7613(03)00359-5
34. Vence L, Palucka AK, Fay JW, Ito T, Liu Y-J, Banchereau J, et al. Circulating Tumor Antigen-Specific Regulatory T Cells in Patients With Metastatic Melanoma. Proc Natl Acad Sci USA (2007) 104:20884–9. doi: 10.1073/pnas.0710557105
35. Onishi H, Morisaki T, Katano M. Immunotherapy Approaches Targeting Regulatory T-Cells. Anticancer Res (2012) 32:997–1003.
36. Sainz-Perez A, Lim A, Lemercier B, Leclerc C. The T-Cell Receptor Repertoire of Tumor-Infiltrating Regulatory T Lymphocytes Is Skewed Toward Public Sequences. Cancer Res (2012) 72:3557–69. doi: 10.1158/0008-5472.CAN-12-0277
37. Tekade RK, Sun X. The Warburg Effect and Glucose-Derived Cancer Theranostics. Drug Discov Today (2017) 22:1637–53. doi: 10.1016/j.drudis.2017.08.003
38. Watson MJ, Vignali PDA, Mullett SJ, Overacre-Delgoffe AE, Peralta RM, Grebinoski S, et al. Metabolic Support of Tumour-Infiltrating Regulatory T Cells by Lactic Acid. Nature (2021) 591:645–51. doi: 10.1038/s41586-020-03045-2
39. Angelin A, Gil-de-Gómez L, Dahiya S, Jiao J, Guo L, Levine MH, et al. Foxp3 Reprograms T Cell Metabolism to Function in Low-Glucose, High-Lactate Environments. Cell Metab (2017) 25:1282–93. doi: 10.1016/j.cmet.2016.12.018
40. Hsu T-S, Lin Y-L, Wang Y-A, Mo S-T, Chi P-Y, Lai AC-Y, et al. Hif-2α Is Indispensable for Regulatory T Cell Function. Nat Commun (2020) 11:5005. doi: 10.1038/s41467-020-18731-y
41. Mellor AL, Munn DH. IDO Expression by Dendritic Cells: Tolerance and Tryptophan Catabolism. Nat Rev Immunol (2004) 4:762–74. doi: 10.1038/nri1457
42. Cederbom L, Hall H, Ivars F. CD4+CD25+ Regulatory T Cells Down-Regulate Co-Stimulatory Molecules on Antigen-Presenting Cells. Eur J Immunol (2000) 30:1538–43. doi: 10.1002/1521-4141(200006)30:6<1538::AID-IMMU1538>3.0.CO;2-X
43. Fallarino F, Grohmann U, Hwang KW, Orabona C, Vacca C, Bianchi R, et al. Modulation of Tryptophan Catabolism by Regulatory T Cells. Nat Immunol (2003) 4:1206–12. doi: 10.1038/ni1003
44. Ovcinnikovs V, Ross EM, Petersone L, Edner NM, Heuts F, Ntavli E, et al. CTLA-4-Mediated Transendocytosis of Costimulatory Molecules Primarily Targets Migratory Dendritic Cells. Sci Immunol (2019) 4:eaaw0902. doi: 10.1126/sciimmunol.aaw0902
45. Paterson AM, Lovitch SB, Sage PT, Juneja VR, Lee Y, Trombley JD, et al. Deletion of CTLA-4 on Regulatory T Cells During Adulthood Leads to Resistance to Autoimmunity. J Exp Med (2015) 212:1603–21. doi: 10.1084/jem.20141030
46. Kumar P, Bhattacharya P, Prabhakar BS. A Comprehensive Review on the Role of Co-Signaling Receptors and Treg Homeostasis in Autoimmunity and Tumor Immunity. J Autoimmun (2018) 95:77–99. doi: 10.1016/j.jaut.2018.08.007
47. Wing K, Onishi Y, Prieto-Martin P, Yamaguchi T, Miyara M, Fehervari Z, et al. CTLA-4 Control Over Foxp3+ Regulatory T Cell Function. Science (2008) 322:271–5. doi: 10.1126/science.1160062
48. Qureshi OS, Zheng Y, Nakamura K, Attridge K, Manzotti C, Schmidt EM, et al. Trans-Endocytosis of CD80 and CD86: A Molecular Basis for the Cell-Extrinsic Function of CTLA-4. Science (2011) 332:600–3. doi: 10.1126/science.1202947
49. Liang B, Workman C, Lee J, Chew C, Dale BM, Colonna L, et al. Regulatory T Cells Inhibit Dendritic Cells by Lymphocyte Activation Gene-3 Engagement of MHC Class II. J Immunol (2008) 180:5916–26. doi: 10.4049/jimmunol.180.9.5916
50. Chauvin J-M, Zarour HM. TIGIT in Cancer Immunotherapy. J Immunother Cancer (2020) 8:e000957. doi: 10.1136/jitc-2020-000957
51. Yu X, Harden K, Gonzalez LC, Francesco M, Chiang E, Irving B, et al. The Surface Protein TIGIT Suppresses T Cell Activation by Promoting the Generation of Mature Immunoregulatory Dendritic Cells. Nat Immunol (2009) 10:48–57. doi: 10.1038/ni.1674
52. Du W, Yang M, Turner A, Xu C, Ferris RL, Huang J, et al. TIM-3 as a Target for Cancer Immunotherapy and Mechanisms of Action. Int J Mol Sci (2017) 18:645. doi: 10.3390/ijms18030645
53. Sharpe AH, Pauken KE. The Diverse Functions of the PD1 Inhibitory Pathway. Nat Rev Immunol (2018) 18:153–67. doi: 10.1038/nri.2017.108
54. Sawant DV, Yano H, Chikina M, Zhang Q, Liao M, Liu C, et al. Adaptive Plasticity of IL-10+ and IL-35+ Treg Cells Cooperatively Promotes Tumor T Cell Exhaustion. Nat Immunol (2019) 20:724–35. doi: 10.1038/s41590-019-0346-9
55. Akdis CA, Blaser K. Mechanisms of Interleukin-10-Mediated Immune Suppression. Immunology (2001) 103:131–6. doi: 10.1046/j.1365-2567.2001.01235.x
56. Turnis ME, Sawant DV, Szymczak-Workman AL, Andrews LP, Delgoffe GM, Yano H, et al. Interleukin-35 Limits Anti-Tumor Immunity. Immunity (2016) 44:316–29. doi: 10.1016/j.immuni.2016.01.013
57. Hilchey SP, De A, Rimsza LM, Bankert RB, Bernstein SH. Follicular Lymphoma Intratumoral CD4+CD25+GITR+ Regulatory T Cells Potently Suppress CD3/CD28-Costimulated Autologous and Allogeneic CD8+CD25- and CD4+CD25- T Cells. J Immunol (2007) 178:4051–61. doi: 10.4049/jimmunol.178.7.4051
58. Strauss L, Bergmann C, Szczepanski M, Gooding W, Johnson JT, Whiteside TL. A Unique Subset of CD4+CD25highFoxp3+ T Cells Secreting Interleukin-10 and Transforming Growth Factor-Beta1 Mediates Suppression in the Tumor Microenvironment. Clin Cancer Res (2007) 13:4345–54. doi: 10.1158/1078-0432.CCR-07-0472
59. Lin JT, Martin SL, Xia L, Gorham JD. TGF-Beta 1 Uses Distinct Mechanisms to Inhibit IFN-Gamma Expression in CD4+ T Cells at Priming and at Recall: Differential Involvement of Stat4 and T-Bet. J Immunol (2005) 174:5950–8. doi: 10.4049/jimmunol.174.10.5950
60. Gorelik L, Constant S, Flavell RA. Mechanism of Transforming Growth Factor Beta-Induced Inhibition of T Helper Type 1 Differentiation. J Exp Med (2002) 195:1499–505. doi: 10.1084/jem.20012076
61. Rodón L, Gonzà lez-Juncà A, Inda M del M, Sala-Hojman A, MartÃnez-Sáez E, Seoane J. Active CREB1 Promotes a Malignant Tgfβ2 Autocrine Loop in Glioblastoma. Cancer Discov (2014) 4:1230–41. doi: 10.1158/2159-8290.CD-14-0275
62. Flanders KC, Wakefield LM. Transforming Growth Factor-βs and Mammary Gland Involution; Functional Roles and Implications for Cancer Progression. J Mammary Gland Biol (2009) 14:131–44. doi: 10.1007/s10911-009-9122-z
63. Leivonen S-K, Kähäri V-M. Transforming Growth Factor-Beta Signaling in Cancer Invasion and Metastasis. Int J Cancer (2007) 121:2119–24. doi: 10.1002/ijc.23113
64. Ross SH, Cantrell DA. Signaling and Function of Interleukin-2 in T Lymphocytes. Annu Rev Immunol (2018) 36:411–33. doi: 10.1146/annurev-immunol-042617-053352
65. Pandiyan P, Zheng L, Ishihara S, Reed J, Lenardo MJ. Cd4+Cd25+Foxp3+ Regulatory T Cells Induce Cytokine Deprivation-Mediated Apoptosis of Effector CD4+ T Cells. Nat Immunol (2007) 8:1353–62. doi: 10.1038/ni1536
66. Deaglio S, Dwyer KM, Gao W, Friedman D, Usheva A, Erat A, et al. Adenosine Generation Catalyzed by CD39 and CD73 Expressed on Regulatory T Cells Mediates Immune Suppression. J Exp Med (2007) 204:1257–65. doi: 10.1084/jem.20062512
67. Vigano S, Alatzoglou D, Irving M, Ménétrier-Caux C, Caux C, Romero P, et al. Targeting Adenosine in Cancer Immunotherapy to Enhance T-Cell Function. Front Immunol (2019) 10:925. doi: 10.3389/fimmu.2019.00925
68. Fallarino F, Grohmann U, Vacca C, Bianchi R, Orabona C, Spreca A, et al. T Cell Apoptosis by Tryptophan Catabolism. Cell Death Differ (2002) 9:1069–77. doi: 10.1038/sj.cdd.4401073
69. Van den Eynde BJ, van Baren N, Baurain J-F. Is There a Clinical Future for IDO1 Inhibitors After the Failure of Epacadostat in Melanoma? Annu Rev Cancer Biol (2020) 4:241–56. doi: 10.1146/annurev-cancerbio-030419-033635
70. Turley SJ, Cremasco V, Astarita JL. Immunological Hallmarks of Stromal Cells in the Tumour Microenvironment. Nat Rev Immunol (2015) 15:669–82. doi: 10.1038/nri3902
71. Sell K, Barth PJ, Moll R, Thomas MA, Zimmer N, Oplesch E, et al. Localization of FOXP3-Positive Cells in Renal Cell Carcinoma. Tumour Biol (2012) 33:507–13. doi: 10.1007/s13277-011-0283-1
72. Ju M-J, Qiu S-J, Gao Q, Fan J, Cai M-Y, Li Y-W, et al. Combination of Peritumoral Mast Cells and T-Regulatory Cells Predicts Prognosis of Hepatocellular Carcinoma. Cancer Sci (2009) 100:1267–74. doi: 10.1111/j.1349-7006.2009.01182.x
73. Li JF, Chu YW, Wang GM, Zhu TY, Rong RM, Hou J, et al. The Prognostic Value of Peritumoral Regulatory T Cells and Its Correlation With Intratumoral Cyclooxygenase-2 Expression in Clear Cell Renal Cell Carcinoma. BJU Int (2009) 103:399–405. doi: 10.1111/j.1464-410X.2008.08151.x
74. Liu F, Lang R, Zhao J, Zhang X, Pringle GA, Fan Y, et al. CD8+ Cytotoxic T Cell and FOXP3+ Regulatory T Cell Infiltration in Relation to Breast Cancer Survival and Molecular Subtypes. Breast Cancer Res Treat (2011) 130:645–55. doi: 10.1007/s10549-011-1647-3
75. Kuczek DE, Larsen AMH, Thorseth M-L, Carretta M, Kalvisa A, Siersbæk MS, et al. Collagen Density Regulates the Activity of Tumor-Infiltrating T Cells. J Immunother Cancer (2019) 7:68. doi: 10.1186/s40425-019-0556-6
76. Xiong S, Pan X, Xu L, Yang Z, Guo R, Gu Y, et al. Regulatory T Cells Promote β-Catenin–Mediated Epithelium-to-Mesenchyme Transition During Radiation-Induced Pulmonary Fibrosis. Int J Radiat Oncol Biol Phys (2015) 93:425–35. doi: 10.1016/j.ijrobp.2015.05.043
77. Yang S, Liu Y, Li M-Y, Ng CSH, Yang S-L, Wang S, et al. FOXP3 Promotes Tumor Growth and Metastasis by Activating Wnt/β-Catenin Signaling Pathway and EMT in Non-Small Cell Lung Cancer. Mol Cancer (2017) 16:124. doi: 10.1186/s12943-017-0700-1
78. Munn DH, Mellor AL. Indoleamine 2,3-Dioxygenase and Tumor-Induced Tolerance. J Clin Invest (2007) 117:1147–54. doi: 10.1172/JCI31178
79. de Araújo EF, Feriotti C, Galdino NA de L, Preite NW, Calich VLG, Loures FV. The IDO-AhR Axis Controls Th17/Treg Immunity in a Pulmonary Model of Fungal Infection. Front Immunol (2017) 8:880. doi: 10.3389/fimmu.2017.00880
80. Xiang Z, Li J, Song S, Wang J, Cai W, Hu W, et al. A Positive Feedback Between IDO1 Metabolite and COL12A1 Via MAPK Pathway to Promote Gastric Cancer Metastasis. J Exp Clin Cancer Res (2019) 38:314. doi: 10.1186/s13046-019-1318-5
81. Fu R, Zhang Y-W, Li H-M, Lv W-C, Zhao L, Guo Q-L, et al. LW106, a Novel Indoleamine 2,3-Dioxygenase 1 Inhibitor, Suppresses Tumour Progression by Limiting Stroma-Immune Crosstalk and Cancer Stem Cell Enrichment in Tumour Micro-Environment. Br J Pharmacol (2018) 175:3034–49. doi: 10.1111/bph.14351
82. Yegodayev KM, Novoplansky O, Golden A, Prasad M, Levin L, Jagadeeshan S, et al. Tgf-Beta-Activated Cancer-Associated Fibroblasts Limit Cetuximab Efficacy in Preclinical Models of Head and Neck Cancer. Cancers (Basel) (2020) 12:339. doi: 10.3390/cancers12020339
83. Casey TM, Eneman J, Crocker A, White J, Tessitore J, Stanley M, et al. Cancer Associated Fibroblasts Stimulated by Transforming Growth Factor Beta1 (TGF-Beta 1) Increase Invasion Rate of Tumor Cells: A Population Study. Breast Cancer Res Treat (2008) 110:39–49. doi: 10.1007/s10549-007-9684-7
84. Zhang Y, Lazarus J, Steele NG, Yan W, Lee H-J, Nwosu ZC, et al. Regulatory T-Cell Depletion Alters the Tumor Microenvironment and Accelerates Pancreatic Carcinogenesis. Cancer Discov (2020) 10:422–39. doi: 10.1158/2159-8290.CD-19-0958
85. Lugano R, Ramachandran M, Dimberg A. Tumor Angiogenesis: Causes, Consequences, Challenges and Opportunities. Cell Mol Life Sci (2020) 77:1745–70. doi: 10.1007/s00018-019-03351-7
86. Viallard C, Larrivée B. Tumor Angiogenesis and Vascular Normalization: Alternative Therapeutic Targets. Angiogenesis (2017) 20:409–26. doi: 10.1007/s10456-017-9562-9
87. Stacker SA, Williams SP, Karnezis T, Shayan R, Fox SB, Achen MG. Lymphangiogenesis and Lymphatic Vessel Remodelling in Cancer. Nat Rev Cancer (2014) 14:159–72. doi: 10.1038/nrc3677
88. Forsythe JA, Jiang BH, Iyer NV, Agani F, Leung SW, Koos RD, et al. Activation of Vascular Endothelial Growth Factor Gene Transcription by Hypoxia-Inducible Factor 1. Mol Cell Biol (1996) 16:4604–13. doi: 10.1128/mcb.16.9.4604
89. Li X, Li D, Shi Q, Huang X, Ju X. Umbilical Cord Blood−Derived Helios−Positive Regulatory T Cells Promote Angiogenesis in Acute Lymphoblastic Leukemia in Mice Via CCL22 and the VEGFA−VEGFR2 Pathway. Mol Med Rep (2019) 19:4195–204. doi: 10.3892/mmr.2019.10074
90. Rauniyar K, Jha SK, Jeltsch M. Biology of Vascular Endothelial Growth Factor C in the Morphogenesis of Lymphatic Vessels. Front Bioeng Biotechnol (2018) 6:7. doi: 10.3389/fbioe.2018.00007
91. Yu JS, Lee PK, Ehtesham M, Samoto K, Black KL, Wheeler CJ. Intratumoral T Cell Subset Ratios and Fas Ligand Expression on Brain Tumor Endothelium. J Neurooncol (2003) 64:55–61. doi: 10.1007/BF02700020
92. Motz GT, Santoro SP, Wang L-P, Garrabrant T, Lastra RR, Hagemann IS, et al. Tumor Endothelium FasL Establishes a Selective Immune Barrier Promoting Tolerance in Tumors. Nat Med (2014) 20:607–15. doi: 10.1038/nm.3541
93. Kandalaft LE, Motz GT, Busch J, Coukos G. Angiogenesis and the Tumor Vasculature as Antitumor Immune Modulators: The Role of Vascular Endothelial Growth Factor and Endothelin. Curr Top Microbiol Immunol (2011) 344:129–48. doi: 10.1007/82_2010_95
94. Delgoffe GM, Woo S-R, Turnis ME, Gravano DM, Guy C, Overacre AE, et al. Stability and Function of Regulatory T Cells Is Maintained by a Neuropilin-1-Semaphorin-4a Axis. Nature (2013) 501:252–6. doi: 10.1038/nature12428
95. Overacre-Delgoffe AE, Chikina M, Dadey RE, Yano H, Brunazzi EA, Shayan G, et al. Interferon-γ Drives Treg Fragility to Promote Anti-Tumor Immunity. Cell (2017) 169:1130–41. doi: 10.1016/j.cell.2017.05.005
96. Hansen W, Hutzler M, Abel S, Alter C, Stockmann C, Kliche S, et al. Neuropilin 1 Deficiency on CD4+Foxp3+ Regulatory T Cells Impairs Mouse Melanoma Growth. J Exp Med (2012) 209:2001–16. doi: 10.1084/jem.20111497
97. Jung K, Kim J-A, Kim Y-J, Lee HW, Kim C-H, Haam S, et al. A Neuropilin-1 Antagonist Exerts Antitumor Immunity by Inhibiting the Suppressive Function of Intratumoral Regulatory T Cells. Cancer Immunol Res (2020) 8:46–56. doi: 10.1158/2326-6066.CIR-19-0143
98. Shrimali RK, Yu Z, Theoret MR, Chinnasamy D, Restifo NP, Rosenberg SA. Antiangiogenic Agents can Increase Lymphocyte Infiltration Into Tumor and Enhance the Effectiveness of Adoptive Immunotherapy of Cancer. Cancer Res (2010) 70:6171–80. doi: 10.1158/0008-5472.CAN-10-0153
99. Piechnik A, Dmoszynska A, Omiotek M, Mlak R, Kowal M, Stilgenbauer S, et al. The VEGF Receptor, Neuropilin-1, Represents a Promising Novel Target for Chronic Lymphocytic Leukemia Patients. Int J Cancer (2013) 133:1489–96. doi: 10.1002/ijc.28135
100. Smith NR, Baker D, Farren M, Pommier A, Swann R, Wang X, et al. Tumor Stromal Architecture Can Define the Intrinsic Tumor Response to VEGF-Targeted Therapy. Clin Cancer Res (2013) 19:6943–56. doi: 10.1158/1078-0432.CCR-13-1637
101. Farinha P, Al-Tourah A, Gill K, Klasa R, Connors JM, Gascoyne RD. The Architectural Pattern of FOXP3-Positive T Cells in Follicular Lymphoma Is an Independent Predictor of Survival and Histologic Transformation. Blood (2010) 115:289–95. doi: 10.1182/blood-2009-07-235598
102. Tzankov A, Meier C, Hirschmann P, Went P, Pileri SA, Dirnhofer S. Correlation of High Numbers of Intratumoral FOXP3+ Regulatory T Cells With Improved Survival in Germinal Center-Like Diffuse Large B-Cell Lymphoma, Follicular Lymphoma and Classical Hodgkin’s Lymphoma. Haematologica (2008) 93:193–200. doi: 10.3324/haematol.11702
103. Carreras J, Lopez-Guillermo A, Fox BC, Colomo L, Martinez A, Roncador G, et al. High Numbers of Tumor-Infiltrating FOXP3-Positive Regulatory T Cells Are Associated With Improved Overall Survival in Follicular Lymphoma. Blood (2006) 108:2957–64. doi: 10.1182/blood-2006-04-018218
104. Gobert M, Treilleux I, Bendriss-Vermare N, Bachelot T, Goddard-Leon S, Arfi V, et al. Regulatory T Cells Recruited Through CCL22/CCR4 Are Selectively Activated in Lymphoid Infiltrates Surrounding Primary Breast Tumors and Lead to an Adverse Clinical Outcome. Cancer Res (2009) 69:2000–9. doi: 10.1158/0008-5472.CAN-08-2360
105. Sautès-Fridman C, Petitprez F, Calderaro J, Fridman WH. Tertiary Lymphoid Structures in the Era of Cancer Immunotherapy. Nat Rev Cancer (2019) 19:307–25. doi: 10.1038/s41568-019-0144-6
106. Bruno TC. New Predictors for Immunotherapy Responses Sharpen Our View of the Tumour Microenvironment. Nature (2020) 577:474–6. doi: 10.1038/d41586-019-03943-0
107. Joshi NS, Akama-Garren EH, Lu Y, Lee D-Y, Chang GP, Li A, et al. Regulatory T Cells in Tumor-Associated Tertiary Lymphoid Structures Suppress Anti-Tumor T Cell Responses. Immunity (2015) 43:579–90. doi: 10.1016/j.immuni.2015.08.006
108. Peske JD, Thompson ED, Gemta L, Baylis RA, Fu Y-X, Engelhard VH. Effector Lymphocyte-Induced Lymph Node-Like Vasculature Enables Naive T-Cell Entry Into Tumours and Enhanced Anti-Tumour Immunity. Nat Commun (2015) 6:7114. doi: 10.1038/ncomms8114
109. Colbeck EJ, Jones E, Hindley JP, Smart K, Schulz R, Browne M, et al. Treg Depletion Licenses T Cell-Driven HEV Neogenesis and Promotes Tumor Destruction. Cancer Immunol Res (2017) 5:1005–15. doi: 10.1158/2326-6066.CIR-17-0131
110. Hindley JP, Jones E, Smart K, Bridgeman H, Lauder SN, Ondondo B, et al. T-Cell Trafficking Facilitated by High Endothelial Venules Is Required for Tumor Control After Regulatory T-Cell Depletion. Cancer Res (2012) 72:5473–82. doi: 10.1158/0008-5472.CAN-12-1912
111. Grimm M, Lazariotou M, Kircher S, Höfelmayr A, Germer CT, von Rahden BHA, et al. Tumor Necrosis Factor-α Is Associated With Positive Lymph Node Status in Patients With Recurrence of Colorectal Cancer-Indications for Anti-TNF-α Agents in Cancer Treatment. Cell Oncol (Dordr) (2011) 34:315–26. doi: 10.1007/s13402-011-0027-7
112. Bengsch F, Knoblock DM, Liu A, McAllister F, Beatty GL. Ctla-4/CD80 Pathway Regulates T Cell Infiltration Into Pancreatic Cancer. Cancer Immunol Immunother (2017) 66:1609–17. doi: 10.1007/s00262-017-2053-4
113. Boissonnas A, Scholer-Dahirel A, Simon-Blancal V, Pace L, Valet F, Kissenpfennig A, et al. Foxp3+ T Cells Induce Perforin-Dependent Dendritic Cell Death in Tumor-Draining Lymph Nodes. Immunity (2010) 32:266–78. doi: 10.1016/j.immuni.2009.11.015
114. Halim L, Romano M, McGregor R, Correa I, Pavlidis P, Grageda N, et al. An Atlas of Human Regulatory T Helper-Like Cells Reveals Features of Th2-Like Tregs That Support a Tumorigenic Environment. Cell Rep (2017) 20:757–70. doi: 10.1016/j.celrep.2017.06.079
115. Simonetta F, Gestermann N, Martinet KZ, Boniotto M, Tissières P, Seddon B, et al. Interleukin-7 Influences FOXP3+CD4+ Regulatory T Cells Peripheral Homeostasis. PloS One (2012) 7:e36596. doi: 10.1371/journal.pone.0036596
116. Wei G, Abraham BJ, Yagi R, Jothi R, Cui K, Sharma S, et al. Genome-Wide Analyses of Transcription Factor GATA3-Mediated Gene Regulation in Distinct T Cell Types. Immunity (2011) 35:299–311. doi: 10.1016/j.immuni.2011.08.007
117. Ouyang W, Oh SA, Ma Q, Bivona MR, Zhu J, Li MO. Tgf-β Cytokine Signaling Promotes CD8+ T Cell Development and Low-Affinity CD4+ T Cell Homeostasis by Regulation of Interleukin-7 Receptor α Expression. Immunity (2013) 39:335–46. doi: 10.1016/j.immuni.2013.07.016
118. Cheng JT, Deng YN, Yi HM, Wang GY, Fu BS, Chen WJ, et al. Hepatic Carcinoma-Associated Fibroblasts Induce IDO-Producing Regulatory Dendritic Cells Through IL-6-Mediated STAT3 Activation. Oncogenesis (2016) 5:e198. doi: 10.1038/oncsis.2016.7
119. Kinoshita T, Ishii G, Hiraoka N, Hirayama S, Yamauchi C, Aokage K, et al. Forkhead Box P3 Regulatory T Cells Coexisting With Cancer Associated Fibroblasts Are Correlated With a Poor Outcome in Lung Adenocarcinoma. Cancer Sci (2013) 104:409–15. doi: 10.1111/cas.12099
120. Terme M, Pernot S, Marcheteau E, Sandoval F, Benhamouda N, Colussi O, et al. Vegfa-VEGFR Pathway Blockade Inhibits Tumor-Induced Regulatory T-Cell Proliferation in Colorectal Cancer. Cancer Res (2013) 73:539–49. doi: 10.1158/0008-5472.CAN-12-2325
121. Liu D, Li G, Avella DM, Kimchi ET, Kaifi JT, Rubinstein MP, et al. Sunitinib Represses Regulatory T Cells to Overcome Immunotolerance in a Murine Model of Hepatocellular Cancer. Oncoimmunology (2017) 7:e1372079. doi: 10.1080/2162402X.2017.1372079
122. Chuckran CA, Liu C, Bruno TC, Workman CJ, Vignali DA. Neuropilin-1: A Checkpoint Target With Unique Implications for Cancer Immunology and Immunotherapy. J Immunother Cancer (2020) 8:e000967. doi: 10.1136/jitc-2020-000967
Keywords: regulatory T cells (Treg), immune infiltration, tumor microenvironment, cancer, vasculature, stroma
Citation: Scott EN, Gocher AM, Workman CJ and Vignali DAA (2021) Regulatory T Cells: Barriers of Immune Infiltration Into the Tumor Microenvironment. Front. Immunol. 12:702726. doi: 10.3389/fimmu.2021.702726
Received: 29 April 2021; Accepted: 27 May 2021;
Published: 10 June 2021.
Edited by:
Rieneke Van De Ven, VU University Medical Center, NetherlandsReviewed by:
Peter J. Siska, University Medical Center Regensburg, GermanyEyad Elkord, University of Salford, United Kingdom
Copyright © 2021 Scott, Gocher, Workman and Vignali. This is an open-access article distributed under the terms of the Creative Commons Attribution License (CC BY). The use, distribution or reproduction in other forums is permitted, provided the original author(s) and the copyright owner(s) are credited and that the original publication in this journal is cited, in accordance with accepted academic practice. No use, distribution or reproduction is permitted which does not comply with these terms.
*Correspondence: Dario A. A. Vignali, dvignali@pitt.edu
†These authors have contributed equally to this work and share first authorship