- Division of Hematology, Oncology and Stem Cell Transplantation and Regenerative Medicine, Department of Pediatrics, Stanford University School of Medicine, Stanford, CA, United States
Survival after solid organ transplantation (SOT) is limited by chronic rejection as well as the need for lifelong immunosuppression and its associated toxicities. Several preclinical and clinical studies have tested methods designed to induce transplantation tolerance without lifelong immune suppression. The limited success of these strategies has led to the development of clinical protocols that combine SOT with other approaches, such as allogeneic hematopoietic stem cell transplantation (HSCT). HSCT prior to SOT facilitates engraftment of donor cells that can drive immune tolerance. Recent innovations in graft manipulation strategies and post-HSCT immune therapy provide further advances in promoting tolerance and improving clinical outcomes. In this review, we discuss conventional and unconventional immunological mechanisms underlying the development of immune tolerance in SOT recipients and how they can inform clinical advances. Specifically, we review the most recent mechanistic studies elucidating which immune regulatory cells dampen cytotoxic immune reactivity while fostering a tolerogenic environment. We further discuss how this understanding of regulatory cells can shape graft engineering and other therapeutic strategies to improve long-term outcomes for patients receiving HSCT and SOT.
Introduction
Solid organ transplantation is a lifesaving therapeutic strategy for numerous end-stage organ failures. The past 25 years have witnessed undeniable progress in preventing graft rejection and graft-versus-host-disease (GvHD), but these gains rely on lifelong use of immune suppressive (IS) drugs (1). Long-term IS regimens contribute to poor clinical outcomes by leading to severe side effects including cardiovascular diseases, hypertension, diabetes, nephrotoxicity, and an increased risk of cancer and infections (2). Even with IS drugs, graft loss occurs in half of patients within 15 years for histocompatibility leukocyte antigen (HLA)-mismatch kidney transplant recipients and within 25 years for those who are fully HLA-matched (3). Currently, many children who receive a SOT at a young age will need at least one additional transplant during their lifetime because of inevitable loss of their graft caused by the combination of chronic rejection, infections, drug toxicity, and nonadherence (4).
Despite great advances in the induction of tolerogenesis in humanized mouse and classical preclinical models (5), there is still a large gap in translating this success to the bedside. Spontaneous operational tolerance remains rare, occurring in less than 5% of kidney and 20% of liver transplant recipients (6–9). Studies have found that some patients who have persistent graft acceptance with chronic IS drug use can become tolerant, allowing careful reduction and eventually full cessation of IS treatment. However, given current challenges in identifying biomarkers of graft rejection, removing IS—especially after kidney transplants—is risky and can lead to graft loss with consequent reductions in life expectancy (10–12). Enhancing long-term outcomes for patients of all ages requires new approaches to transplantation that can address these challenges.
In recent decades, investigators have focused on developing alternative approaches to induce immune tolerance toward the donor graft in transplant recipients. A standout example is the combination of allogeneic HLA-matched HSCT with SOT from the same donor (13). Despite promising results, over 70% of patients lack an HLA-identical sibling. For this reason, transplants from related full-haplotype mismatched (haploidentical) donors (14) and unrelated HLA-matched and mismatched donors have been performed to expand availability of this treatment protocol.
Successful allogeneic HSCT requires the development of immune tolerance towards both the donor and host allogeneic antigens. Induction of immune tolerance can prevent T-cell mediated graft-rejection and GvHD, which might lead to life threatening complications in HSCT recipients. Current approaches to prevent rejection and GvHD after HSCT primarily rely on pharmacological IS, either prior to or after HSCT. These approaches are limited by lack of antigen specificity, and the requirement for long-term therapy, which often leads to severe complications. Recent progress in understanding the mechanism of action of alloreactive and regulatory cell populations has led to the use of specific cell subsets to prevent/treat graft rejection and GvHD and induce immune tolerance. Peripheral tolerance after allogeneic HSCT may be achieved by several mechanisms, though blocking alloreactivity to the host human leukocyte antigens while preserving immune responses to pathogens and tumor antigens remains a challenge. Recently uncovered evidence regarding the mechanisms of post-HSCT immune reconstitution and tolerance in transplanted patients has allowed for the development of novel cell-based therapeutic approaches. These therapies are aimed at inducing long-term peripheral tolerance and reducing the risk GvHD, while sparing the graft-versus-leukemia (GvL) effect (15).
The use of sequential HSCT and SOT has resulted in meaningful improvements in kidney graft tolerance (16–24). With the addition of non-myeloablative conditioning, many HLA-matched recipients are able to taper and fully discontinue all IS drugs within two years after transplantation without GvHD or graft rejection (25). In HLA-mismatched recipients, though, achieving tolerance without IS has proven to be considerably more difficult and, when accomplished, has often come with heightened risks of GvHD and infections that can threaten graft survival (25–28). Although clinical studies have made strides in maintaining long-term organ engraftment with reduced IS regimens, there is an ongoing need to improve immune tolerance after sequential HSCT and SOT in order to completely eliminate the need for pharmacological IS and without potentially risky tradeoffs for patient outcomes.
A refined understanding of the mechanisms of immune tolerance creates opportunities for novel HSCT techniques- including graft engineering strategies- to optimize survival after SOT and enable a functional immune system that permanently accepts donor antigens without the need for IS. This review describes first key findings that influence our understanding of the cellular mechanisms involved in immune tolerance as well as the role of innate immunity in these regulatory processes. It then explores how development of new therapeutic strategies can harness this knowledge to more effectively induce tolerance, especially in the context of sequential HSCT and SOT.
Cellular Mechanisms of Conventional Immune Tolerance
Immune tolerance is multifaceted and involves the interaction of different cells, listed in Table 1, that serve critical regulatory roles. While investigators have long worked to identify processes of tolerogenesis, advanced methods, including single-cell technologies, have expanded the mechanistic understanding of cells that are actively involved in the development of immune tolerance.
‘Conventional’ Treg Cells
In 1970, a seminal study by Gershon and Kondo (60) described a subset of T cells distinct from T helper (Th) cells that decreased the immune response. Twenty-five years later, these cells were named regulatory T cells (Tregs) in a study that found athymic mice inoculated with purified CD4+CD25- T cells spontaneously developed autoimmune diseases (61) whereas the transfer of CD4+CD25+ cells inhibited CD4-mediated autoimmunity in lymphopenic mice.
The ontogeny of naturally emerged Tregs occurs in the thymus (tTregs) while other Tregs are converted or induced from CD4+CD25- in the periphery (iTregs or pTregs, respectively) (62). tTregs are crucial for control of immune self-tolerance, allergy, and allograft survival. In mice and humans, tTregs comprise 2-10% of peripheral CD4+ T cells (63, 64). Interleukin-2 (IL-2)-receptor α chain (CD25) is a cell surface marker that identifies Treg cells. Stimulation with TNF tumor necrosis factor (TNF) and IL-2 upregulates CD25 and activates Tregs (65); however, induction of CD25 expression in CD25- murine T cells is not sufficient to generate Treg suppressive function (66). Notably, activated memory and certain effector T cells (Teff) can also express CD25 (66). Thus, phenotype subsets of Tregs have been more precisely identified with other cell surface markers.
The identification of Forkhead Box P3 (FOXP3) as a master regulator of the Treg lineage commitment and differentiation has dramatically improved understanding of Treg biology (67–69). Loss-of-function mutations in human FOXP3 cause Immunodysregulation Polyendocrinopathy Enteropathy X-linked (IPEX) syndrome, a rare and life-threatening immune disease (70). FOXP3 mutation or deletion can also lead to loss of repression of oncogenes in some nonlymphoid cells, resulting in malignancies (71, 72). Early onset IPEX syndrome exclusively affects males and leads to fatal lymphoproliferative dysfunction in Tregs and subsequent severe autoimmunity (70, 73). The Treg specific demethylation of a highly conserved non-coding element within the FOXP3 gene (Treg-specific demethylated region, TSDR) is required for FOXP3 expression and can be used for Treg identification (74, 75). However, TSDR methylation status can vary; it is fully demethylated in tTregs, partially methylated in TGF-β polarized Tregs, and methylated in naïve cells (75–77). Accordingly, the methylation status of the FOXP3 TSDR is a marker for the stability of FOXP3 expression and Treg function during thymic differentiation, but it is not sufficient to isolate these cells. More recently, CD4+CD25+ CD127-/lo (IL-7R α chain) phenotype has been used for isolation and identification of Tregs (29–32).
During Treg thymic differentiation, FOXP3 expression depends on the coordination of several factors, including T cell receptor (TCR) signaling, CD28 co-stimulation (78), cytokines (IL-2, IL-15, and IL-7), transcription factors (NFAT and ICOS) (79, 80), and the PI3K-mTOR signaling network (81). Notably, FOXP3 can also be expressed in differentiating pTregs or in iTregs upon TCR stimulation with suboptimal co-stimulatory molecules. However, transient expression of FOXP3 in Teff cells did not correlate with regulatory functions previously reported in Tregs, indicating that FOXP3 may not be used as a marker solely for Tregs (82). TGF-β and IL-2 stimulation coupled with TCR signaling and co-stimulatory molecules skew the differentiation of naïve CD4+ T cells into Tregs. Mechanistically, IL-2 triggers the STAT5 signaling network and its downstream targets, including the expression of FOXP3, and polarizes CD4+ T cell differentiation to Tregs rather than IL-17-producing effector T cells (Th17) (83, 84). Although human TGF-β-induced Tregs have a suppressive function in vitro, the transcriptomic landscape does not recapitulate tTregs, and its suppressive capacity is compromised in vivo in humanized GvHD mouse models (85).
Tregs can suppress autoimmunity directly through the release of cytokines (e.g. IL-10, IL-35, and TGF-β) or mediate cytotoxicity toward Teff via the production of proteases that induce cell apoptosis, such as granzyme and perforin, or galectins (86–91). Indirect mechanisms of suppression include: 1. recruitment of other cells, such as modulating antigen presenting cell (APC) function through cytotoxic T-lymphocyte antigen 4 (CTLA4), 2. expression of CD39/CD73 ectonucleotidases that convert ATP to immunosuppressive metabolites such as AMP and adenosine, 3. shifting a proinflammatory environment to anti-inflammatory (92, 93), and 4. outcompeting Teffs in IL-2 uptake by overexpressing CD25 (94) (Figure 1).
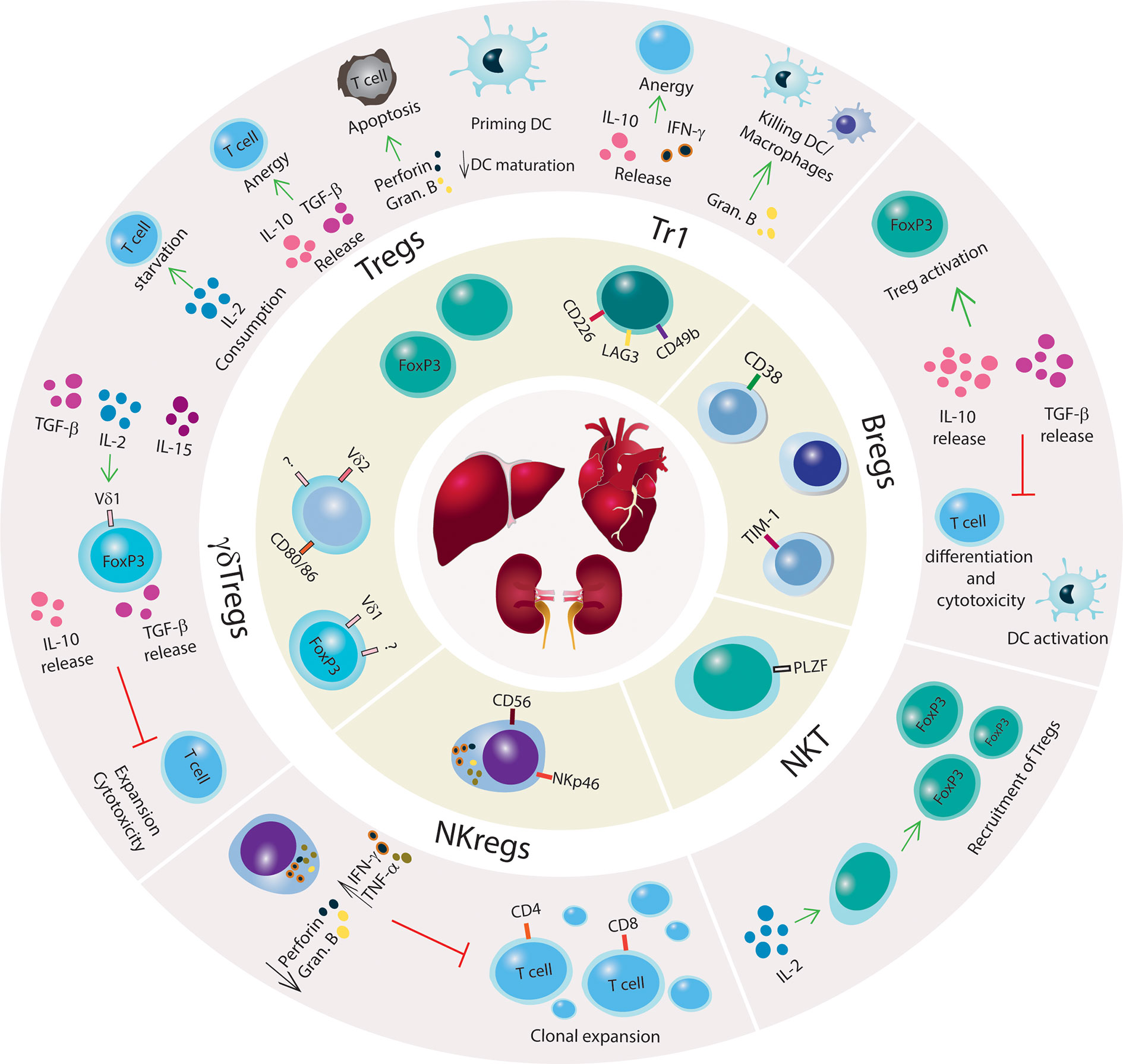
Figure 1 Mechanisms of immune tolerance to promote SOT engraftment and survival. Schematic illustration of regulatory innate and adaptive immune cells with a brief summary of mechanisms of immune suppression. Kidney, liver and heart are represented in the center of the figure, and regulatory immune cells (Tregs, Tr1, Bregs, NKT cells, NKregs, γδTregs) are shown surrounding the organs. The outer circle illustrates the main regulatory networks for each immune cell subset. Green arrows indicate promoting mechanisms, black arrows denote increase or decrease of cytokines production or biological processes, and red lines denote inhibitory networks.
In the allogeneic transplant context, Treg signaling mechanisms are crucial for allograft survival because of their dampening of the immune response from Teff cells. As Tregs do not produce IL-2, their activation depends on the release of IL-2 by Teff cells (95). In the absence of Tregs, the binding of Teff TCR to alloantigen-major histocompatibility complex (MHC) and CD28 to CD80/CD86 activates Teff cells, leading to the secretion of IL-2 (86, 96). By autocrine mechanisms, IL-2 signaling triggers other T cells, causing activation, proliferation, and differentiation that can all lead to allograft rejection. However, activated Tregs secrete IL-10 and TGF-β that convert Teff cells into anergic cells, creating a tolerogenic environment. The expression of the co-stimulatory molecule CTLA4 on Tregs interacts with CD80/86 on dendritic cells (DCs) to suppress the immune response and contribute to allograft tolerance (97, 98).
Given their involvement in a multitude of immune responses, Tregs are considered a heterogeneous population with diverse functions and markers. Most of these cells can be categorized as naïve, FOXP3+ effector non-Treg cells, or activated/effector Tregs with the latter being the most proliferative (Ki67+) and suppressive (CTLA4high) (33). Moreover, FOXP3+ effector non-Treg cells produce pro-inflammatory cytokines such as IFN-γ and IL-7 that have reduced immunosuppressive function and a high potential to become Teff. A further subset of human effector Tregs was identified using chemokine receptors and intracellular markers wherein T helper-like Tregs showed a memory-like phenotype (36). The migratory capacity (Table 1) and cytokine secretion of each subset offers crucial information in the graft tolerance context given that these cells have the ability to target specific tissue types, such as allografts or lymph nodes (99).
Tr1 Cells
In contrast to tTregs, regulatory type 1 T cells (Tr1) are a subpopulation of memory CD4+ T cells that can transiently express FOXP3 upon activation; however, FOXP3 expression in Tr1 cells is not constitutive or a requirement for Tr1 function and differentiation (100). Tr1 cells co-express integrin α2 subunit (CD49b) and lymphocyte activation gene 3 (LAG-3), which facilitate the identification of Tr1 in the peripheral blood of tolerant patients (39). LAG-3 is mostly expressed on activated Tr1 cells while CD49b expression is constitutive. Other cell surface markers have been identified including Tim-3, PD-1, TIGIT, and CD39, but they are not exclusive to Tr1 cells (101). Another crucial difference between Tr1 and tTregs is the metabolic profile on which these cells rely; Tr1 cells depend on aerobic glycolysis (102) while FOXP3+ Treg differentiation is associated with fatty acid oxidative phosphorylation (103).
Tr1 cell development, expansion, and function are independent of IL-2 and CD28 (104). The Tr1 cell mechanism of suppression is via secretion of TGF-β and IL-10 in which IL-10 constitutively triggers Tr1 cells to release additional IL-10, creating a feedback loop (Figure 1). In the absence of IL-10, Tr1 cells lose their capacity to produce IL-10 but retain secondary mechanisms of immune suppression that are driven by the expression of granzyme B and CTLA-4 (105). Tr1 can release IFN-γ but only low or absent levels of IL-2, IL-4, and IL-17 have been found in these cells. The activation of Tr1 cells is via cognate antigen binding by their TCR, which initiates the production of granzyme B and Tr1-mediated killing of DCs or macrophages (40, 106). Once activated, Tr1 cells perform bystander suppression. Tr1 cells also utilize suppressive mechanisms that are shared with FOXP3+ Tregs including interactions of co-stimulatory molecules CTLA-4 with CD80 and PD-1 with PD-L1 (107).
IL-10 is essential for Tr1 cell function in humans and mice, but the signaling mechanism has not been fully elucidated. The STAT pathway has been suggested as the downstream target of IL-10 signaling in Tr1 cells. Studies have shown that STAT3 interacts with proteins associated with a glycolytic metabolic environment that favors Tr1 cell differentiation (102). High activation of STAT3 in T cells induces Tr1 differentiation (108), and the induction of IL-10 is STAT1- and STAT3-mediated (109). IL-27 has been described in mice and humans as a critical cytokine that promotes IL-10 secretion and Tr1 differentiation (110, 111). Mechanistically, IL-27 triggers STAT3, which activates B lymphocyte-induced maturation protein-1 expression (112). Under specific conditions, IL-27 also triggers c-Maf and AhR transcription factors to activate IL-10 transcription such that AhR also contributes to granzyme B expression in Tr1 cells (111, 113, 114). Other transcription factors necessary for IL-27-mediated induction of Tr1 include BAFT, IRF1, and ITK (115, 116).
Single Cell Strategies to Identify Tregs and Tr1 Subpopulations
Previous studies have pioneered the investigation of cell surface markers to identify subsets of regulatory T cells. Given advances in single-cell strategies, the heterogeneity of regulatory T cells has been reflected in the stratification of these cells according to novel cell surface markers, intracellular markers, and transcriptomic signatures (34, 37, 38, 117, 118). For example, in a human T cell atlas study using single-cell RNA-seq, new evidence was reported for regulatory T cell ontogeny indicating that, in fact, there are two populations of Treg progenitors with specific transcriptional signatures in the human thymus (118). The cell population called Treg(diff) showed lower expression of FOXP3 and CTLA4 when compared to conventional Tregs. Another cell subset had features similar to Treg(diff) but not to Tregs. This population was referred to as Tagonist and presented low FOXP3 expression but high expression of a non-coding RNA (MIR155HG). The definition of these two populations opens new paths to investigate the functional roles of these recently identified progenitors and the mechanisms that skew Treg development to one progenitor or another. By understanding these features, it will be possible to improve the understanding of the post-transplantation scenario when Tregs from transplanted CD34+ are differentiated in the thymus.
Human Tregs and Teffs from peripheral blood as well as from mouse Foxp3GFP lymphoid organs were sorted and analyzed in a scRNA-seq screening (117). In both species, a similar transcriptomic profile (FOXP3, IL2Ra, IL2Rb, IKZF2, TNFRSF1B) was shown to distinguish Tregs from Teffs using an expression profile associated with cell ontogenesis, cell function, and metabolic processes. Notably, the intensity of TCR signaling strongly influenced the clusters of Treg cells, suggesting multiple differentiation states in the Treg pool (117). However, approximately 55% of the human Treg cell cluster overlapped with Teffs, indicating that FOXP3+Tregs with a CD4+CD25+CD127lo phenotype comprise a heterogeneous population with certain cells expressing an effector transcriptomic profile. In a scRNA-seq analysis of CD4+ T cells from pancreatic intragrafts of mice treated with CD47 monoclonal antibodies (mAb), two subpopulations of Tregs with low proliferative capacity and a distinct transcriptomic network were identified in rejected grafts (119). These results indicate that Treg heterogeneity is susceptible to changes in the microenvironment caused by, for instance, mAbs.
In a single-cell mass cytometry (CyTOF) study, human peripheral blood mononuclear cells (PBMCs) and isolated CD4+ T cells were analyzed for their cell surface and intracellular markers. Unsupervised high-dimension clustering analysis identified new subsets, their phenotypes, and the relationship among these cell subpopulations (37). In another single-cell CyTOF analysis of liver-transplanted children, a panel with 22 markers identified a remarkable enrichment for non-classic Tregs (CD4+ CD5+ CD25+ CD38-/lo CD45RA-) in tolerant recipients compared to patients under IS (34). Specifically, CD5 has been shown as a marker to promote extrathymic Treg development in response to self or tolerizing agents in the periphery (120–122), while lack of CD45RA indicates a memory phenotype in kidney transplanted patients (123). This shows that these induced Tregs in the periphery can have high plasticity to immune responses (120, 122) and be generated in a tolerogenic environment. The identification of new cell subtypes in tolerant patients can define novel diagnostic markers that will benefit other SOTs.
Similarly, CyTOF analysis of sorted Tregs from healthy donors showed a heterogeneous population of naïve Tregs that failed to express markers commonly reported for conventional Tregs such as CCR4, CD39, HLA-DR, ICOS, and CD147 (38). Interestingly, hierarchical analysis of naïve Tregs using CD31, CD103, and LAP markers showed subpopulations carrying a preprogrammed status, suggesting a transient state between naïve and fully differentiated Tregs. Thus, the heterogeneous population of Tregs identified in tolerogenic liver-transplanted recipients may have transient states that can contribute to prolonged graft survival. Current studies in lineage tracing and pseudotime analysis of single-cell data will provide valuable information about the biological trajectory for regulatory T cell specification.
Recently, Miragaia et al. (124) compared Tregs from murine and human non-lymphoid tissues to identify a conserved transcriptional signature for peripheral Tregs that have travelled across tissues. Two subpopulations of transient Tregs were found with tissue-specific gene signatures that had adapted toward either skin or colon tissues (124). Many factors have been previously reported to induce, maintain, and attract Tregs to the colon, including dietary antigens and the microbiota (125). Tr1 cells have also been reported in gut-related autoimmune disorders. Tr1 cells generated and expanded in vitro specific for ovalbumin (OVA-specific Tr1) have been previously tested in Crohn’s disease and colitis (106, 126). In a clinical trial with OVA-specific Tr1 clones, patients ingested OVA-enriched diets to stimulate OVA-specific Tr1 cell migration to the gut. This study reported a decrease in tissue inflammation up to five weeks post-treatment and OVA-specific Tr1 immunoregulatory function ex vivo. Moreover, Tr1 cells induced in vitro can express gut-homing markers GPR15 and CCR9 (41), and Tr1 cells induced in vivo have been found in tolerant mouse models (39), indicating the migratory capacity of Tr1 cells.
Previous reports have extensively discussed therapies using Tregs and Tr1 to control and prevent GvHD (127, 128). The understanding of key molecular features in Tregs and Tr1 will improve therapeutic approaches and clinical protocols to mitigate GvHD and promote allograft survival. Results from more recent cutting-edge technologies will provide new insights into T regulatory networks, cell function, cellular states and plasticity, cell migration markers, and cell expansion and survival. Altogether, these studies highlight the critical importance of taking precautions in expanding Tregs based on specific phenotypes because these cells can carry subpopulations with effector function that may negatively impact allograft survival and function.
Regulatory B Cells
Recent studies have identified potentially important contributions to tolerogenesis from humoral immunity, a section of the adaptive immune response. B cells are at the core of humoral immunity and are responsible for clonally producing antibodies, but immune regulatory function is less understood for B cells than for Tregs. In the bone marrow, various cytokines, chemokines, and transcription factors regulate B cell differentiation from hematopoietic stem cells. Premature B cells travel from the bone marrow to the spleen and secondary lymphoid tissues to mature and differentiate under antigen-dependent and independent phases of selection. Similar to T cells, B cell receptor (BCR) is expressed via V(D)J rearrangement during maturation and selection. The combination of the antigen recognition by BCR and a co-stimulatory signal (e.g., helper T cell binding) stimulates B cell proliferation into either plasma cells responsible for secreting antibodies or memory cells that have a high survival rate, high antigen affinity, and fast secondary response.
Studies have previously reported that the regulation of humoral immunity through either conventional mechanisms of immune suppression or B cell immunomodulatory functions can be crucial for the success of allograft transplant (Figure 1). Early evidence was found in 1970 when, upon B cell depletion, guinea pigs suffered severe and prolonged contact hypersensitivity responses, indicating a suppressive role of B cells toward T cell responses (129, 130). B regulatory cells (Bregs) have mostly been characterized by their capacity to secrete IL-10 and TGF-β; which curtail T cell differentiation and cytotoxic function. Briefly, the mechanism wherein Bregs also modulate T and Natural Killer (NK) cell apoptosis is via the production of granzyme B and FasL (131, 132). In humans, Bregs are phenotypically subdivided into multiple subsets including transitional TIM-1+ cells expressing IL-10 and memory/mature (Table 1) (44–46). In studies with human kidney allografts, the imbalance of IL-10/TNF-α expression in Breg cells was correlated with kidney injury (44). Additionally, tolerant recipients with complete eradication of the IS regimen showed elevated numbers of naïve, memory, and total B cells, upregulation in co-stimulatory and inhibitory molecules, and a genomic signature toward tolerogenesis (133, 134).
Role of Innate Immunity in Promoting Tolerance
Despite its fundamental role in immune defense, innate immunity can also involve regulatory functions. Specific subsets of cell types involved in innate immunity can contribute to graft tolerance or rejection after SOT.
γδ T Cells With Regulatory Properties
The classic identification of T lymphocytes involves the expression of either αβ TCR or γδ TCR (γδ T cells), although pro-inflammatory T cells bearing both receptors have been identified in mice and humans (135). Compared to αβ T cells, γδ T cells have less variability in the V and J gene segments, but γδ TCR have vast variation in the rearrangement of the D genes. Although other subsets have been identified within the γδ T population, Vδ1 and Vδ2 T cells remain the most studied subtypes. In humans, peripheral γδ T cells comprise up to 5% of the T cell population with Vδ2 as the major subset, but γδ T cells can rapidly expand in response to viral infections like human cytomegalovirus (CMV), inflammation, and tumors. Although Vδ1 T cells exist in the blood, they predominantly reside in the mucosal epithelia of solid tissues including the liver, skin, and intestines.
In comparison to αβ T cells, γδ T cells directly recognize antigens independent of MHC haplotype. The Vδ1 TCR binds to stress-induced proteins, such as MHC-I related chain A or B which are often found on tumorigenic cells and in post-SOT biopsies. The Vδ2 TCR recognizes small non-peptide phosphorylated antigens (pAg), which are intermediates of the mevalonate pathway in eukaryotes and in the non-mevalonate pathways in prokaryotes. For example, isopentenyl pyrophosphate (IPP) can accumulate in tumor cells carrying a defective mevalonate pathway. Mechanistically, members of the butyrophilin receptor family (e.g. BTN3A1) in either APC or tumor cells bind to IPP via intracellular domains and undergo conformational changes in the extracellular domains that are recognized by γδ TCR, leading to the activation of Vδ2 T cells (136). Recently, pAg-mediated coupling of BTN2A1 and BTN3A1 was suggested as the stimulatory trigger of Vδ2 T cells (137). Notably, γδ T cell subsets can recognize antigens via the expression of receptors commonly found on NK cells, such as NKG2D, DNAM-1, NKp30, and NKp44 (138).
Besides their anti-tumorigenic and anti-infectious role, γδ T cells can exert immune suppressive functions. In 1989, Patel et al. (139) reported regulatory properties of a specific subset of γδ T cells (γδ Tregs) involved in inhibiting mitomycin-activated CD4+ T cell to activate B cell maturation in vitro. The phenotypic identification of γδ Tregs has mostly been based on findings from functional assays in vitro and expression of markers previously reported for conventional Tregs. Peripheral γδ T cells from healthy donors have no detectable levels of FOXP3 but show low expression of CD25 and CTLA-4. However, under IL-2, IL-15, and/or TGF-β stimulation, γδ Tregs can express FOXP3, release IL-10 and TGF-β; and inhibit the effector function of previously activated CD4+ T cells (47–51) (Figure 1). Recently, γδ Tregs expressing CD73 that secrete IL-10 and TGF-β were identified in both the periphery and tumors of patients diagnosed with advanced metastatic breast cancer (140).
The expression of co-stimulatory molecules (e.g. CD80, CD86) and inhibitory molecules (PD-L1) on Vδ2 T cells and results from transwell assays have provided evidence of the cell-to-cell contact dependency for γδ Tregs immune suppressive function (48). However, no consensus has been achieved regarding the cell culture method to expand and activate regulatory mechanisms in γδ T cells. The variations include γδ T isolation strategies prior to or after cytokine stimulation, different types of cytokines stimulation, co-culture with either PBMCs (without the removal of conventional Tregs pool) or selectively activated CD4+ T cells, anti-TCR γδ for activation, and presence or absence of pAgs (52). Although the largest population of γδ T cells carrying regulatory features are Vδ1 T cells, few studies have compared the immune regulatory function of Vδ1 and Vδ2 T subsets, and a comprehensive analysis of the suppressive capacity of γδ T subsets has not been clarified.
γδ T cells reside in several tissues where they can exert immune suppressive functions. For example, patients with active celiac disease had reduced levels of TGF-β-expressing γδ T cells, but patients on a gluten-free diet benefited from γδ Treg expansion and abrogation of Teff response (141). Additionally, the expansion of peripheral Vδ1 T cells in pregnant women and the production of IL-10 and TGF-β by γδ T cells in the uterus can promote a suppressive environment that is likely necessary for fetal-maternal interface to avoid rejection early in pregnancy (142, 143).
NK and NKT Cells
Besides γδ T cells, NK and natural killer T (NKT) cells compose innate immunity. NK cells are known for exterminating tumor and virus-infected cells, and the term NKT cells derives from these cells’ similarities with both NK and T cells. Like NK cells, NKT cells express surface markers such as CD161. Like T cells, NKT cells differentiate and mature in the thymus and, phenotypically, can be CD4+, CD8+, or CD4-CD8-. Although CD4-CD8- is indicative of immature T cells, activated NKTs with this phenotype are fully competent to produce cytokines (IL-4 and IFN-γ). In mice and humans, another marker shared among NK, NKT, T, and γδ T cells is promyelocytic leukemia zinc finger (PLZF). In mice, PLZF, together with GATA-3, RORγT, and T-bet, can stratify subpopulations of thymic NKT cells (144). Notably, NKTs have limited diversity in αβ TCRs, especially in humans (Vα24Jα18); NKT cells are activated when NKT TCRs detect glycolipid Ags presented by CD1d molecules on APCs. In mice, subpopulations of NKT cells in different maturation stages have been identified by the expression of NK1.1 (145, 146).
Although some NKT permanently localize to the thymus (147), a subset migrates to other tissues. The largest accumulation is in the liver where these cells make up approximately 30% of the T lymphocyte population (148). NKT subpopulations sensitive to IL-15 and positive for the transcription factor T-bet express chemokine receptors (e.g. CXCR3 and CXCR6) that bind to ligands produced in the liver (e.g., CXCL9, CXCL16) (149). In an IL-2-dependent manner, NKT cells recruit and trigger Tregs to tissues (Figure 1), indicating a regulatory function for NKTs that is also crucial for tolerance in coupled stem cell and solid organ transplants (150). Recently, Zhou et al. (151) focused on single-cell analysis of human peripheric NKT cells to characterize the transcriptomic signatures in NKT subpopulations. By evaluating the gene expression of specific cytokines, one NKT subset showed an immune regulatory profile comprising IL-2+, IL-10+, ICOS+, IL-4–, IFN-γ–, and XCL–. In cancer studies, a CD4+ NKT population was reported with immune modulatory function (152, 153). In the context of allogeneic HSCT, low levels of CD4+ NKT cells were correlated with the development of chronic GvHD in patients that received grafts from BMT (154).
NK cells distinguish between autologous and allogeneic cells via inhibitory receptors present on the cell surface that identify self-antigens and prevent cell lysis. For example, at later stages of maturation, NK cells express killer immunoglobulin-like receptors (KIRs) that bind to classic MHC-I. A NK tolerogenic marker is the heterodimer CD94/NKG2A that specifically recognizes HLA-E and is expressed at the early stages of NK differentiation (155). KIRs and CD94/NKG2A can be co-expressed at intermediary stages of differentiation, but to avoid autoreactivity, mature NKs selectively express one or the other (156). In humans, the receptor NKG2D recognizes stress-related ligands MICA and MICB, triggering NK cell toxicity (157). However, NKG2D is not exclusive to NKs as it is also expressed by γδ T cells and NKT cells. Other cytotoxic-related receptors found in NK are Nkp30 and Nkp46 (158).
As in other immune subsets, human NKs are heterogeneous with subpopulations mostly distinguished by different expression levels of CD56 and CD16. Terminally mature NKs with a cytotoxic phenotype are CD56dimCD16+ and are the vast majority of circulating NKs in the periphery. These mature NKs also have higher expression of KIR or CD94/NKG2A. In two single-cell transcriptomic analyses of NK cells from peripheral blood and bone marrow of healthy donors (159, 160), CD56dimCD16+ were reported as heterogeneous with only one subset (also CD57+) showing a singular transcriptomic profile of terminally different NKs (high expression of CX3CR1, TIM-3, and ZEB-2). Conversely, CD56brightCD16-/low have an immature state and express NKG2A, but KIR is absent in these cells. In pseudotime trajectory analysis to determine lineage specification, CD56brightCD16-/low were found as precursors of CD56dimCD16+ based on their transcriptomic profile (160). A transitional state between immature and terminal NKs was also reported and indicated the following developmental trace: CD56brightCD16-/low cells to CD56dim CD57- and then CD56dim CD16+ CD57+.
The subset of CD56brightCD16-/low cells secrete IFN-γ and TNF-α but express low to no levels of perforin and granzyme B, indicating a regulatory profile (CD56bright NKreg) rather than a cytolytic role (Figure 1). However, prolonged stimulation with IL-2 and IL-5 can activate CD56bright cells to become cytolytic and differentiate into CD56dim in a mechanism mediated by the STAT3 signaling network (161). CD56bright NK cells have been identified in an immune suppressive environment, such as in the uterus and periphery of pregnant women, leading to high response against viral infections and tumorigenesis as well as positively affecting successful full-term pregnancies (162, 163).
Therapeutic Strategies to Improve Immune Cell Tolerance
While immunosuppression has contributed to substantial improvements in graft survival in SOT, investigators have recognized the need for other mechanisms to promote transplant tolerance in order to avoid the implications of long-term IS administration. The combination of HSCT and SOT is an important development, but numerous challenges remain in optimizing graft survival without GvHD, excess risk of infection, or lifelong need for IS drugs. Applying the growing understanding of cells involved in immune tolerance can improve HSCT and SOT as a therapeutic strategy and lead to enhanced long-term patient outcomes.
Tolerance in HSCT and SOT
Since the early 90s, Strober and collaborators have sought to develop the combination of haplo-HSCT with kidney transplant. Chimerism, the coexistence of both donor and recipient hematopoietic cells, is a critical mechanism for promoting tolerance in this approach. Chimerism that persists for at least six months after transplant is associated with improved kidney graft tolerance and effective immune response to infection (28, 164). Both HLA-matched and mismatched HSCT with SOT can achieve chimerism, but persistent chimerism that is believed to promote tolerance has been achieved more frequently in HLA-matched recipients (25).
Busque et al. (25) reported that 24 of 29 HLA-matched transplant recipients with stable mixed chimerism for at least 6 months were able to discontinue IS drugs within 6-14 months with no cases of GvHD and only one case of graft loss. Ten patients had mixed chimerism that persisted after cessation of IS drugs. The remaining patients lost mixed chimerism without IS, but only one experienced graft rejection (2, 25, 165, 166), suggesting that durable operational tolerance may be induced by prior mixed chimerism (28).
In contrast, HLA-mismatched HSCT and kidney transplant recipients have typically needed chronic IS drugs to avoid graft rejection and GvHD (26, 27, 165). HLA-mismatched patients with mixed chimerism 12 months post-transplant were able to taper to one IS drug (tacrolimus), but full cessation resulted in loss of chimerism and evidence of graft rejection that required reinstatement of single-agent tacrolimus. HLA-mismatched patients who do not develop mixed chimerism that lasts beyond three weeks after transplant were prone to engraftment syndrome and associated graft injury that occurred despite continued IS (25). Another approach in HLA-mismatched kidney transplants has been to induce tolerance with full rather than mixed chimerism. While this approach enabled 22 of 37 patients to discontinue IS therapy, there were two cases of GvHD, one of which was fatal and the other chronic (25, 28, 167). Side effects of an intensive conditioning regimen in this approach led to severe neutropenia and thrombocytopenia post-transplant, and two patients experienced graft loss due to infection (25, 28).
Multiple therapeutic strategies to avoid these difficult tradeoffs have been proposed and are being evaluated in preclinical and clinical studies. A promising approach involves HSCT graft engineering that capitalizes on a deepening understanding of regulatory cells to cultivate tolerance independent of IS drugs without GvHD or excess infection risk.
Graft Manipulation to Optimize Sequential HSCT and SOT
In the context of hematologic malignancies, HSCT graft manipulation techniques have shown clear benefits, and many of these approaches could be applied and enhanced to improve combined HSCT and SOT. A breakthrough approach in hematologic diseases reported that using G-CSF to mobilize HSCs and hematopoietic stem cell progenitors (CD34+) from the bone marrow of the donor allows infusion of more CD34+ cells. Subsequent studies selectively depleted T cells ex vivo for obtaining a CD34+-enriched graft (>10×106 cells/kg) and reported successful and prolonged engraftment in more than 90% of adult patients (168). However, slow immune reconstitution due to lymphocyte absence in the graft increased the susceptibility of these patients to lethal infection. With the discovery of Tregs and of their translational application, grafts enriched for CD34+ and co-infused with Tregs with a fraction of conventional T cells were infused in 43 conditioned patients with acute leukemia (169). These patients received no subsequent IS and had successful engraftment, but 15% developed acute GvHD, likely from the Teff cells in the graft.
In 2010, our group pioneered αβhaplo-HSCT (170), a new approach that eliminates the αβ T cells and CD19+ B cells from the graft. By removing the T cell subsets responsible for GvHD, this graft manipulation approach dramatically reduces the risk of severe acute and chronic GvHD (170, 171). Another benefit of this strategy is the presence of NK and γδ T cells in the graft that can immediately respond against infections, reducing patients’ morbidity and mortality. In fact, despite the removal of αβ T cells, the presence of mature donor-derived effector cells provides anti-infectious control while minimizing the risk of severe acute GvHD (172, 173). In both malignant and non-malignant disorders, αβhaplo-HSCT recipients have experienced excellent clinical outcomes including rapid immune reconstitution, low risk of infections, and low incidence of graft failure (170, 171, 174–176). As a result, αβhaplo-HSCT represents a potentially ideal approach for inducing a tolerogenic environment that enables successful SOT (177).
Regulatory T Cells
Encouraging preclinical and clinical studies of Treg and Tr1 cells in autoimmune and inflammatory diseases (178, 179) suggest that regulatory T cell infusion could improve outcomes of SOT. In 2016, Todo et al. (180) published data from the first clinical trial with Tregs and liver transplanted patients (Table 2). Seven patients showed signs of transplant tolerance and were weaned off IS drugs starting at 6 months after SOT with complete withdrawal within 18 months. However, the same strategy failed in kidney transplanted patients (186). Although the cells transferred to these patients also carried Teff cells, this clinical trial is considered the first pilot study in humans of a strategy to induce allograft tolerance using Treg infusion.
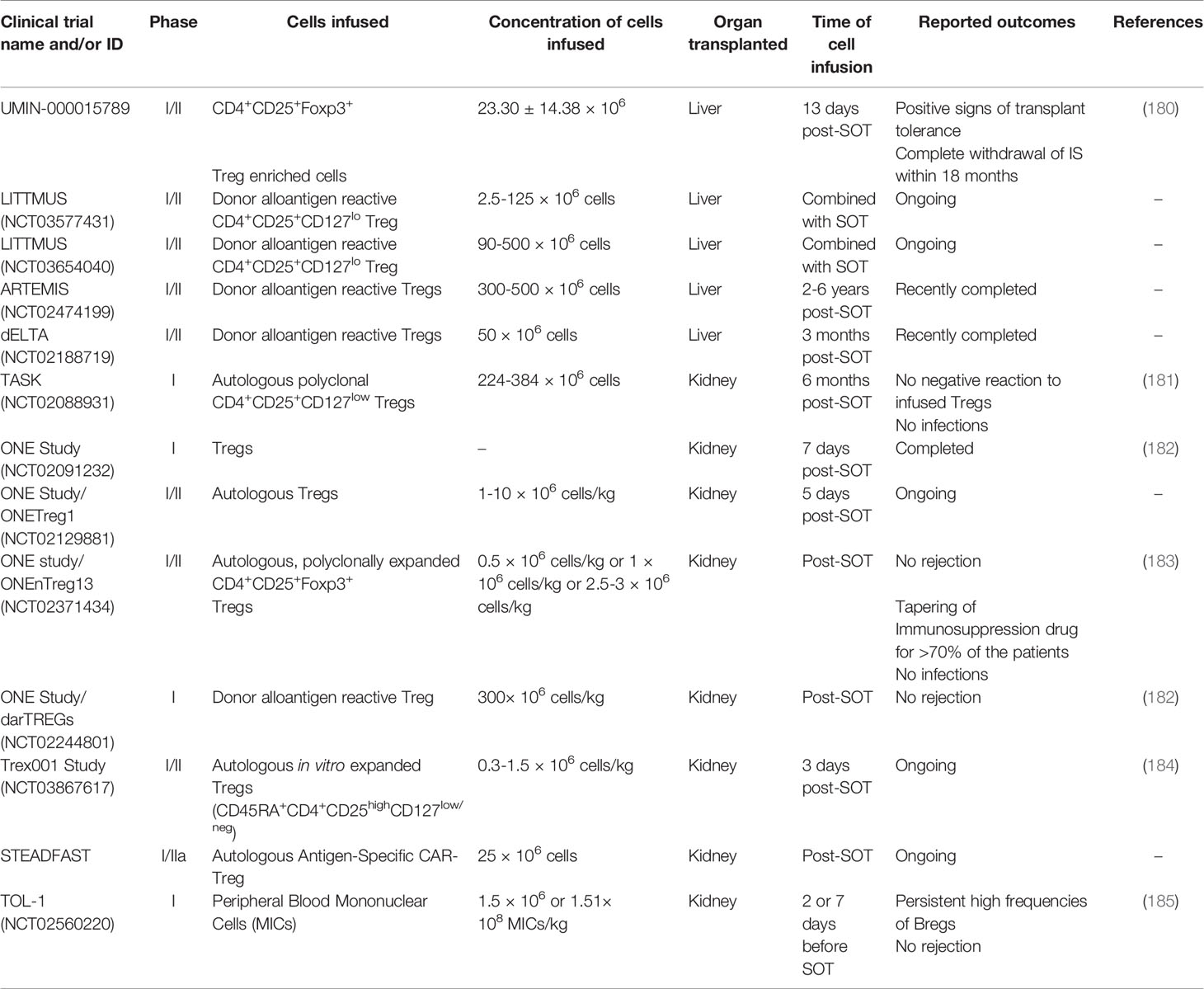
Table 2 Brief summary of ongoing or completed clinical trials combining immune cell infusion with solid organ transplantation.
Building on this pilot study, investigators have started turning to modified strategies for therapeutic Treg infusions. Expansion of human Tregs for clinical applications opened opportunities for the treatment of unwanted immune responses such as in autoimmunity and after transplantation. The identification of markers for subpopulations of Tregs (187) is allowing the isolation and removal of non-Tregs from the remaining Treg populations as part of cellular therapies for allograft tolerance. Additionally, manipulation of specific subsets of Treg effector cells may enable refining their immune suppressive functions (188, 189).
The advances in next generation sequencing-based strategies have been extended to evaluating TCR repertoire diversity and antigen specificity (190). T cell populations have multiple TCR clones resulting from previous and current exposure to antigens. The understanding of the TCR composition reflects prior infections, immunizations, and individual response to specific epitopes. For the transplantation field, clinical trials have evaluated polyclonal and donor antigen reactive Tregs (Table 2) to determine their therapeutic ability to promote a tolerogenic environment. Although patients were still under an immunosuppressive regimen, the analysis of donor-specific TCR repertoire from Tregs cultured with activated donor B cells separated nontolerant from tolerant kidney-transplanted patients (191). Moreover, tracking donor-specific Tregs repertoire may provide insights into stratifying patients according to the likelihood of successfully withdrawing immunosuppression. Growing evidence suggests that disease-relevant and antigen-specific Tregs offer advantages over polyclonal Tregs (192, 193). Donor Tregs have demonstrated better suppressive function towards alloreactive effector T-cells when compared to polyclonal Tregs, which can affect the number and purity of infused cells (194, 195). While expanded CD4+CD25+ Tregs have been used in clinical trials (196) with promising results in preventing GvHD, they are polyclonal, nonspecific and could induce universal immunosuppression. As a result, ongoing or recently completed clinical trials are focusing on purifying Tregs with or without alloantigen specificity (Table 2; LITTMUS, ARTEMIS, dELTA).
To improve tolerance in SOT recipients, other investigators explored the role of transient mixed chimerism (26). Previous observations showed that mild conditioning regimens can induce transient chimerism and tolerance, but myelosuppression was still required (197–200). The Trex001 Study (Table 2) will test an immunotherapy strategy to induce transient chimerism while reducing myelosuppression to promote a tolerogenic environment and prevent kidney rejection (184).
In kidney transplant recipients (Table 2; TASK), results from follow-up biopsies after two weeks and six months post-Treg infusion showed that no patient had a negative reaction to the Tregs, and no infections were observed (181). Interestingly, circulating Tregs peaked two weeks post-infusion and then declined until untraceable three months post-infusion. The ONE Study (Table 2) is a multi-center consortium testing the safety and feasibility of multiple Treg infusion protocols in kidney transplant recipients. Although the immunosuppression regimen is consolidated among the centers (tacrolimus, mycophenolate, and steroids for three months), differences include the clonality, donor origin, frozen or fresh cells, and expansion with or without co-stimulation. In the ONEnTreg13 trial, the infused nTregs became oligoclonal over time, favoring specific TCR repertoires selectively to alloantigens and potentially helping a tolerant environment post-SOT (183). As the numbers of Tregs in circulation decreased after a month of infusion, the study hypothesized that these cells homed to the graft. Although the protocol of the multicenter ONE Study was considered safe, the infusion of nTregs was insufficient to completely remove the three IS drug treatment post-SOT (182). These results indicate the need for strategies to improve Treg cellular therapy.
Collectively, these completed and ongoing trials will offer valuable information about safety, therapeutic strategy, and the most suitable time point in which to infuse Tregs after SOT.
Engineering Tregs
Gene therapy to engineer Tregs offers another intriguing approach for HSCT and SOT, and major advances in designing Treg cell therapies and various gene editing methods are comprehensively discussed by Ferreira et al. (201).
Antigen-specificity in regulatory T cells could be obtained through the TCR or gene transduction of a chimeric antigen receptor (CAR). HLA-A mismatching is one of the critical factors affecting graft outcome; therefore, targeting HLA-A via antigen-specific Tregs may be a promising method of inducing tolerance (202, 203). MacDonald et al. (195) generated CAR Tregs expressing an HLA‐A2‐specific CAR (A2-CAR), which maintained Treg phenotypes and stability and could suppress CD8 T cell proliferation in vitro. They demonstrated that CAR Tregs were more potent than Tregs expressing an irrelevant CAR in preventing GvHD in a xenogeneic mouse model receiving HLA-A2+ human PBMC (195). Their results imply that the “off-target” effects of CAR-expressing Tregs is not different from the polyclonal Tregs. Nevertheless, Treg suppressive response is more likely to be induced via CAR than via TCR because it requires fewer target antigens. Moreover, the CAR Treg strategy allows for a lower number of Tregs that in turn decrease the off-target toxicity (195).
Dawson et al. (204) showed that the insertion of the wild type CD28 co-stimulatory domain is essential to the effective function of CAR Tregs in vitro and in an HLA-A2-mismatched xenoGvHD mouse model. Notably, RNA-seq analysis of CAR Tregs highlighted that stable expression of Helios and ability to suppress CD80 expression on DCs were major predictors of an effective in vivo performance (204). By incorporating in silico analysis, this comprehensive study showed that humanization of scFvs decreased cross-reactivity to several HLA-A allelic variants but could alter affinity and antigen specificity of CAR. This highlights the importance of testing multiple CARs to identify the optimal constructs. Determining allo-antigen specificity of Tregs is critical in the transplantation context to ensure precise targeting of allogeneic cells, tissues, and organs. Tregs expressing the optimal humanized A2-CARs showed rapid trafficking and persistence in HLA-A2-expressing allografts, migrated to draining lymph nodes, prevented HLA-A2+ cell-mediated xenogeneic GvHD, and effectively suppressed rejection of human HLA-A2+ skin allografts (205).
Adoptive transfer of A2-CAR Tregs was utilized to prevent rejection of human skin allograft in mice (202). A2-CAR Tregs potently suppressed the allogeneic responses of delayed-type hypersensitivity and prevented rejection of HLA-A2-positive human skin grafts for over 40 days, an effect attributed to A2-CAR Tregs homing to skin grafts and long-term persistence (202). In a similar study, CAR Tregs exhibited a greater suppressive function than ΔCAR Tregs (lacking CD28-CD3ζ domain) or polyclonal Tregs in vitro and ameliorated the alloimmune‐mediated skin injury (203). These studies demonstrate that human CAR Tregs specific for HLA-A2 are more protective than polyclonal Tregs in humanized skin transplants. Altogether, these studies lay the foundation for developing HLA-specific CAR Tregs as adoptive cell therapy for autoimmune diseases and SOT.
To further extend CAR technology to Treg application in mice, Pierini et al. (206) showed that Tregs with transient expression of mAbCAR (engineered FITC-targeted-CARs activated with FITC-conjugated mAbs) promoted suppressive function once incubated with FITC-mAbs in vitro and in vivo and induced homing of mAbCAR Tregs to specific cells and organs (206) Adoptive transfer of mAbCAR Tregs reduced allograft responses such as GvHD, prolonged MHC-mismatched pancreatic islet allograft survival, and increased alloantigen-specific tolerance to secondary skin grafts (206). Although this strategy is promising, FITC could induce immunogenicity in humans, a limitation that can be resolved with the use of clinically safe antibody-tagged systems. Nevertheless, these findings highlight the flexibility of the mAbCAR Treg approach and suggest benefits in its application in transplantation to induce tolerance while controlling GvHD.
Although promising results were described with CAR Tregs in preclinical studies, there are several concerns surrounding the translation of these approaches to human HSCT and SOT. First, immune-deficient NSG mice lack the complexity of the human immune system, which may affect interpretation of data regarding tolerance and safety. Second, adoptively transferred CAR Tregs are only present at the initial phase after transplantation, which increases the chance of graft rejection (207). Third, obtaining clinically relevant numbers of CAR Tregs that can survive long-term in SOT patients is challenging. Indeed, IS drugs may reduce the number of CAR Tregs in liver and kidney transplants, which could impact CAR Treg efficacy. In 2019, Sangamo Therapeutics, Inc. (UK) started the STEADFAST clinical trial (Table 2) to evaluate CAR Treg therapy for the prevention of immune-mediated rejection following HLA-A2 mismatched kidney transplant in end-stage renal disease. This trial will soon provide information about the short-term safety and tolerability of CAR-Tregs as well as insights into the impact of CAR Tregs that can be incorporated into future SOT clinical trials.
B Cell Strategies
In the allograft context, antibody-mediated rejection (AMR) is a leading cause of graft loss (208, 209). The activation of long-lived plasma cells and B cells releases donor-specific antibodies (DSA) that bind to the endothelium of the allograft. This binding triggers the recruitment of NK cells, neutrophils, and macrophages, leading to a series of inflammatory events, cytotoxicity, and cellular necrosis (210, 211). The outcome is severe endothelial injury, platelet aggregation, thrombotic microangiopathy, and the eventual loss of allograft function. For this reason, influencing B cell activity to facilitate tolerance can directly influence the success of SOT.
Importantly, the choice of initial IS regimen can influence the overall differentiation profile of B cells (212). This, in turn, can impact the variety and quantity of specific Bregs after SOT. For example, sirolimus significantly expanded Bregs and FOXP3+ Tregs one month after liver transplant (213), but this effect was not observed for tacrolimus. Transcriptomic studies coupled with flow cytometry analysis have shown that Bregs express inhibitory/co-stimulatory molecules, such as PD-L1, CTLA-4/CD80, and CD86 (214–216), known to promote Treg function including dampening of Teff response. Although treatment with belatacept, a CTLA-4-immunoglobulin fusion protein, was first developed to target T cells, low levels of BAFF were detected in tolerant patients (217). The results from a 10-year follow-up trial showed that the numbers of Breg cells as well as FOXP3+ Tregs were constitutively elevated in patients treated with belatacept (218). This provides evidence that the combination of strategies targeting multiple levels of immunosuppression can benefit transplant recipients.
In a phase I clinical trial, modified immune cells (MICs) were stimulated with the alkylating agent mitomycin C, resulting in immature donor-derived DCs with high immune modulatory capacity (Table 2; TOL-1) (185). Although these patients were under steroid regimen, circulating Bregs were present in high numbers one month post-transplant with a persistent and significant increase in Breg frequencies two years later. The allograft function was normal, and, as the patients showed unmodified levels of Tregs compared to pretransplant and pretreatment levels, the effectiveness of the treatment could be associated with the tolerogenic capacity of Bregs. Other therapeutic strategies targeting molecular regulators of Breg function, such as TIM-1, histone deacetylase, and the STAT3 network pathway, can have meaningful impact in inducing humoral-mediated immune suppression in tolerant allograft recipients (219).
Therapies with mAbs in kidney, liver, and heart recipients have shown efficacy in mitigating graft loss and improving long-term outcomes. In renal-transplant patients, de novo and increased pre-formed DSA correlated with high levels of the B cell survival factor, BAFF, and elevated rates of AMR (220). Concordantly, in a phase II clinical trial, treatment with an anti-BAFF mAb (belimumab) after SOT reduced the formation of de novo DSA, dampened the number of active memory B cells, and expanded Breg cells (221). In another study, treatment with alemtuzumab, an anti-CD25 antibody, correlated with good clinical outcomes including expansion of transitional Bregs one year after kidney transplant (222). In heart allograft recipients, a single dose of rituximab pre-transplant was sufficient to support B cell differentiation, but a second dose at 15 days post-transplant accelerated graft rejection and led to poor outcomes (223). Taken together, these results indicate that clinical protocols using mAbs can modulate the humoral response with potential benefits in transplant recipients, and further studies will provide optimization of drug choice, dosage, and timing.
γδ T Cell Strategies
Preclinical and observational studies have reported γδ Treg function in SOT survival and homeostasis. In mouse models of kidney and liver transplant, the enrichment of peripheral CD8+ γδ T cells was positively correlated with graft tolerance as these cells secreted suppressive cytokines (e.g. IL-10 and IL-4) toward Th1 responses (53). Moreover, IL-4 dampened Vδ2 T cell function and increased the IL-10-secreting Vδ1 T cell population (224).
The ability of γδ T cells to control viral infections is important in the transplantation setting as HCMV infection is a major complication in transplant recipients. Interestingly, enrichment of cytotoxic Vδ1 T cells with an effector memory phenotype has been found in αβhaplo-HSCT (225) and kidney recipients (226) positive for CMV. In fact, HCMV reactivation was resolved one year after kidney transplant in patients with elevated Vδ2- T cells. To amplify the benefits of γδ T cells, Vδ2 T cells from αβhaplo-HSCT recipients can be expanded under zoledronic acid (Zol) treatment in vitro whereupon these cells show an effector memory phenotype and aggressive cytolytic capacity against leukemia cells (225). In 43 pediatric leukemia patients transplanted with αβhaplo-HSCT, multiple Zol infusions were safe and improved overall survival, potentially due to the promotion of strong cytotoxicity against leukemia cells from Vδ2 T cells (227). In previous studies, Zol-activated Vδ2 T cells infused in patients with solid tumors reestablished a γδ T cell reservoir and halted cancer progression (228). Notably, γδ Treg cells can be induced in vitro under Concanavalin A treatment (47), indicating its potential function for γδ Treg expansion ex vivo.
In pediatric liver transplant recipients, the increase in Vδ1/Vδ2 could indicate successful long-term tolerance (229). Reduced incidence of GvHD was associated with increased levels of CD27+ Vδ1 T cells in patients who received allogeneic HSCT (230). This study also reported that G-CSF can significantly increase donor γδ Tregs in vivo and in vitro, suggesting that the choice of mobilization agent can influence the immunosuppressive environment of the graft. However, a clear phenotype to identify and isolate γδ Tregs is still under investigation, which limits the understanding of the molecular mechanisms of suppression and the appreciation of findings in clinical tolerance. Despite an absence in the literature regarding approaches to engineer or expand γδ Tregs for clinical applications, other recently developed strategies for genetic modifications of γδ T cells may propel future studies in γδ Tregs (231).
NK and NKT Cell Strategies
In light of the importance of NK and NKT cells for both immune defense and tolerance, these cells have a potentially impactful role in successful transplant outcomes. NK and NKT cell infusions to prevent GvHD are under preclinical investigation (127). In previous studies of post-HSCT recipients, expansion of NKT cell subpopulations positively correlated with GvHD mitigation (232). CD56bright NKreg cells positive for NKp46 have been found to be related to a low incidence of GvHD and have been used as a marker in clinical studies (58). In fact, NKp46 receptors are the drivers of NK response to eliminate HCMV-infected DCs. In clinical trials of chronic HCMV-infected patients who received liver transplants, IS administration was removed for half of the recipients (233). The tolerogenic environment was associated with the expansion of CD8+ T cell expressing regulatory markers (CTLA-4, TIM-3, PD-1) and the upregulation of genes downstream of IFN-γ signaling (ISG15, IRF1/7/9), suggesting an immune response that includes NK cell mechanisms. Low levels of non-cytolytic NKregs were associated with chronic GvHD 100 days post-HSCT in HLA-matched recipients enrolled in the ABLE/PBMTC1202 study (58, 59). An ongoing clinical trial (NCT03605953) is testing the feasibility of expanding and injecting donor CD4- NKT cells post-allogeneic HSCT to promote graft versus leukemia (GvL) while reducing the risk of GvHD.
In the graft manipulation approach of αβhaplo-HSCT, donor NK cells (30-40 × 106 per kg) were included in cell infusion for children with acute myeloid and acute lymphocytic leukemia (170, 176). To improve the clinical outcome in terms of GvHD and GvL, the infused NK cells were selectively chosen according to their alloreactivity based on KIR/KIR-ligand model, KIR B haplotype, size of NK alloreactive subset, and high expression of NKp46 and NKG2C (170, 234). While the NK alloreactivity was not observed to be crucial for the overall GvL effect, NK cells were believed to reduce GvHD and short-term infection risk.
NK cell alloreactivity plays a major role in SOT as the graft can be recognized by NKs under a “missing self” mechanism which potentially leads to graft rejection (235). KIR-HLA mismatch has been shown to negatively impact short- and long-term survival of kidney grafts (236). However, to date, no clinical consensus has been reached regarding the use of KIR-ligand as a predictive model for transplantation outcome because other NK receptors can also mediate alloreactivity and tolerance. Solid organ transplant recipients may also benefit from infused or recently differentiated NKreg cells. In other clinical studies, immature NK cells (CD56brightNKG2AhighKIRlow) derived from hematopoietic stem cell differentiation were identified in the first weeks post-HSCT (237–239). These results have important implications in the context of SOT as immature NK cells may carry an NKreg subpopulation that will offer a tolerogenic environment to improve graft survival. Moreover, understanding the peak of the noncytolytic NKregs pool, as well as of other regulatory cells, can offer the best timing of SOT post-HSCT. Further studies identifying NKT cell phenotype and function will provide valuable information for understanding the cytotoxic and regulatory role of NKTs subsets, especially in the context of HSCT and SOT.
In recent years, there has been substantial investigation to develop off-the-shelf products for cell therapy. Although the synthesis of CAR-NKs is challenging, CAR-NK cells have the potential to become universal therapies. Preclinical and clinical studies have shown promising safety and efficacy for CAR-NK in cancer immunotherapies and in reducing GvHD (240). To date, the feasibility of CAR NKreg tolerogenic potential for SOT has not been tested. Understanding the mechanisms and phenotypes of NKreg cells will enable development of targeting strategies using CAR or CRISPR-Cas9 gain-of-function to create a tolerogenic environment and reduce graft loss.
Conclusion
The transplantation tolerance field has dramatically advanced over recent decades to improve organ engraftment and survival and abate the mortality and morbidity caused by IS. Despite major advances, widespread tolerance in SOT has not yet been achieved without dependence on IS regimens. Several preclinical studies have confirmed the feasibility for inducing transplantation tolerance; however, there remains a gap in translating these findings to the clinic.
One major challenge is represented by the variability in outcomes depending on the type of solid organ transplant. To prevent graft loss, immunosuppression regimens are proportional to the likelihood of graft rejection for specific organs. Allogeneic skin transplants are the most complex model of transplantation due to high immunogenicity and high numbers and varieties of APCs (241). Intestine transplants are also at high risk of rejection while heart, kidney, and liver transplants carry a lower risk. Given its function in metabolism and detoxification, the liver receives and processes large quantities of bacteria and dietary products and, accordingly, has a persistent, well-regulated immunoregulatory property. The benefit of low hepatic immunogenicity is to offer systemic immune tolerance and successful engraftment for a co-transplanted organ, such as liver and kidney co-transplants (242, 243). Mechanistically, liver-resident macrophages and hepatic myeloid and plasmacytoid DCs produce and secrete IL-10 and prostaglandins which reduce the expression of co-stimulatory receptors on APCs and compromise the activation of Teff cells (244–249). Myeloid populations provide additional regulatory mechanisms to prevent CD4+ T cell activation via IL-10, TGF-β; and IDO (250). In preclinical studies of kidney and heart transplants, host DCs rapidly replace donor DCs within days post-transplant and are associated with graft rejection (251–253). The depletion of graft DCs was reported to delay ongoing acute rejection. Thus, the diversity in immunogenicity across tissues poses a challenge in predicting the outcome of SOTs that apply similar transplantation strategies.
Despite these difficulties, pairing allogeneic HSCT with SOT is a promising approach. Besides dramatically increasing the chance of finding a suitable donor for the organ transplant, combining allogeneic HSCT with SOT can positively impact allograft survival and overall clinical outcomes. More recently, modifications in HSCT and SOT protocols have successfully decreased or eliminated IS administration for select patients. Further improvements are needed to consolidate and expand these results. In HSCT, graft manipulations, such as αβhaplo-HSCT, have successfully minimized IS administration while contributing to the prolonged survival of pediatric and adult patients (170, 174, 254). A deeper understanding of regulatory and suppressive immune mechanisms has vast applicability in inducing tolerance in transplant patients and bringing αβhaplo-HSCT and other techniques into SOT. In the era of single-cell data, novel regulatory subsets have been more comprehensively studied in their transcriptomic, epigenomic, and immunophenotypic profile, providing new avenues for amplifying immune tolerance. By recapitulating or increasing cellular regulatory networks, engineering strategies to manipulate immune cells in vitro for subsequent infusion can prolong tolerance post-HSCT which may offer a suitable window for SOT and allograft survival. Taken together, technological advances and ongoing clinical trials in these areas will appreciably change the field of transplantation tolerance.
Author Contributions
PFS and AB outlined and wrote the manuscript. PFS designed the figure and the tables. MY reviewed the literature and wrote about engineered CAR-Tregs. PFS, MY and AB edited and approved the final version of this manuscript. All authors contributed to the article and approved the submitted version.
Funding
This work was partially funded by the Kruzn For a Kure Foundation. PFS is funded by the Pediatric Nonmalignant Hematology and Stem Cell Biology NIH T32 training grant (DK098132).
Conflict of Interest
The authors declare that the research was conducted in the absence of any commercial or financial relationships that could be construed as a potential conflict of interest.
Acknowledgments
We would like to thank the members of the AB laboratory for fruitful suggestions to the development of this paper.
References
1. Dharnidharka VR, Fiorina P, Harmon WE. Kidney Transplantation in Children. N Engl J Med (2014) 371:549–58. doi: 10.1056/NEJMra1314376
2. Lowsky R, Strober S. Combined Kidney and Hematopoeitic Cell Transplantation to Induce Mixed Chimerism and Tolerance. Bone Marrow Transplant (2019) 54:793–7. doi: 10.1038/s41409-019-0603-4
3. Sordi V, Bianchi G, Buracchi C, Mercalli A, Marchesi F, D’Amico G, et al. Differential Effects of Immunosuppressive Drugs on Chemokine Receptor CCR7 in Human Monocyte-Derived Dendritic Cells: Selective Upregulation by Rapamycin. Transplantation (2006) 82:826–34. doi: 10.1097/01.tp.0000235433.03554.4f
4. LaRosa C, Jorge Baluarte H, Meyers KEC. Outcomes in Pediatric Solid-Organ Transplantation. Pediatr Transplant (2011) 15:128–41. doi: 10.1111/j.1399-3046.2010.01434.x
5. Mahr B, Wekerle T. Murine Models of Transplantation Tolerance Through Mixed Chimerism: Advances and Roadblocks. Clin Exp Immunol (2017) 189:181–9. doi: 10.1111/cei.12976
6. Dugast E, Chesneau M, Soulillou J-P, Brouard S. Biomarkers and Possible Mechanisms of Operational Tolerance in Kidney Transplant Patients. Immunol Rev (2014) 258:208–17. doi: 10.1111/imr.12156
7. Brouard S, Le Bars A, Dufay A, Gosselin M, Foucher Y, Guillet M, et al. Identification of a Gene Expression Profile Associated With Operational Tolerance Among a Selected Group of Stable Kidney Transplant Patients. Transpl Int (2011) 24:536–47. doi: 10.1111/j.1432-2277.2011.01251.x
8. Baron D, Ramstein G, Chesneau M, Echasseriau Y, Pallier A, Paul C, et al. A Common Gene Signature Across Multiple Studies Relate Biomarkers and Functional Regulation in Tolerance to Renal Allograft. Kidney Int (2015) 87:984–95. doi: 10.1038/ki.2014.395
9. Martínez-Llordella M, Lozano JJ, Puig-Pey I, Orlando G, Tisone G, Lerut J, et al. Using Transcriptional Profiling to Develop a Diagnostic Test of Operational Tolerance in Liver Transplant Recipients. J Clin Invest (2008) 118:2845–57. doi: 10.1172/JCI35342
10. Dugast E, Soulillou J-P, Foucher Y, Papuchon E, Guerif P, Paul C, et al. Failure of Calcineurin Inhibitor (Tacrolimus) Weaning Randomized Trial in Long-Term Stable Kidney Transplant Recipients. Am J Transplant (2016) 16:3255–61. doi: 10.1111/ajt.13946
11. Hricik DE, Formica RN, Nickerson P, Rush D, Fairchild RL, Poggio ED, et al. Adverse Outcomes of Tacrolimus Withdrawal in Immune–Quiescent Kidney Transplant Recipients. J Am Soc Nephrol (2015) 26:3114–22. doi: 10.1681/ASN.2014121234
12. Christakoudi S, Runglall M, Mobillo P, Rebollo-Mesa I, Tsui T-L, Nova-Lamperti E, et al. Development and Validation of the First Consensus Gene-Expression Signature of Operational Tolerance in Kidney Transplantation, Incorporating Adjustment for Immunosuppressive Drug Therapy. EBioMedicine (2020) 58:102899. doi: 10.1016/j.ebiom.2020.102899
13. Dey B, Sykes M, Spitzer TR. Outcomes of Recipients of Both Bone Marrow and Solid Organ Transplants: A Review. Med (Baltimore) (1998) 77:355–69. doi: 10.1097/00005792-199809000-00005
14. Fabricius WA, Ramanathan M. Review on Haploidentical Hematopoietic Cell Transplantation in Patients With Hematologic Malignancies. Adv Hematol (2016) 2016:1–8. doi: 10.1155/2016/5726132
15. Bertaina A, Roncarolo MG. Graft Engineering and Adoptive Immunotherapy: New Approaches to Promote Immune Tolerance After Hematopoietic Stem Cell Transplantation. Front Immunol (2019) 10:1342. doi: 10.3389/fimmu.2019.01342
16. Jacobsen N, Taaning E, Ladefoged J, Kvist Kristensen J, Pedersen F. Tolerance to An HLA-B,DR Disparate Kidney Allograft After Bone-Marrow Transplantation From Same Donor. Lancet (1994) 343:800. doi: 10.1016/S0140-6736(94)91881-3
17. Sellers MT, Deierhoi MH, Curtis JJ, Gaston RS, Julian BA, Lanier DC, et al. Tolerance in Renal Transplantation After Allogeneic Bone Marrow transplantation-6-year Follow-Up. Transplantation (2001) 71:1681–3. doi: 10.1097/00007890-200106150-00031
18. Helg C, Chapuis B, Bolle JF, Morel P, Salomon D, Roux E, et al. Renal Transplantation Without Immunosuppression in a Host With Tolerance Induced by Allogeneic Bone Marrow Transplantation. Transplantation (1994) 58:1420–2.
19. Fangmann J, Kathrin Al-Ali H, Sack U, Kamprad M, Tautenhahn HM, Faber S, et al. Kidney Transplant From the Same Donor Without Maintenance Immunosuppression After Previous Hematopoietic Stem Cell Transplant. Am J Transplant (2011) 11:156–62. doi: 10.1111/j.1600-6143.2010.03352.x
20. Vondran FWR, Eiermann T, Thaiss F, Schwinzer R, Nashan B, Koch M. In Vitro and In Vivo Proof of Tolerance After Two-Step Haploidentical Bone Marrow and Kidney Transplantation of the Same Donor. Transplantation (2012) 93:e23–5. doi: 10.1097/TP.0b013e3182492247
21. Schwarz C, Lawitschka A, Böhmig GA, Dauber EM, Greinix H, Kozakowski N, et al. Kidney Transplantation With Corticosteroids Alone After Haploidentical HSCT From The Same Donor. Transplantation (2016) 100:2219–21. doi: 10.1097/TP.0000000000001213
22. Beitinjaneh A, Burns LJ, Majhail NS. Solid Organ Transplantation in Survivors of Hematopoietic Cell Transplantation: A Single Institution Case Series and Literature Review. Clin Transplant (2010) 24:E94–E102. doi: 10.1111/j.1399-0012.2009.01155.x
23. Knüppel E, Medinger M, Stehle G, Infanti L, Halter J, Burkhalter F, et al. Haploidentical Hematopoietic Bone Marrow Transplantation Followed by Living Kidney Transplantation From the Same Donor in a Sickle Cell Disease Patient With End-Stage Renal Failure. Ann Hematol (2017) 96:703–5. doi: 10.1007/s00277-017-2936-7
24. Eder M, Schwarz C, Kammer M, Jacobsen N, Stavroula ML, Cowan MJ, et al. Allograft and Patient Survival After Sequential HSCT and Kidney Transplantation From the Same Donor-a Multicenter Analysis. Am J Transplant (2019) 19:475–87. doi: 10.1111/ajt.14970
25. Busque S, Scandling JD, Lowsky R, Shizuru J, Jensen K, Waters J, et al. Mixed Chimerism and Acceptance of Kidney Transplants After Immunosuppressive Drug Withdrawal. Sci Transl Med (2020) 12:eaax8863. doi: 10.1126/scitranslmed.aax8863
26. Kawai T, Cosimi AB, Spitzer TR, Tolkoff-Rubin N, Suthanthiran M, Saidman SL, et al. Hla-Mismatched Renal Transplantation Without Maintenance Immunosuppression. N Engl J Med (2008) 358:353–61. doi: 10.1056/NEJMoa071074
27. Kawai T, Sachs DH, Sprangers B, Spitzer TR, Saidman SL, Zorn E, et al. Long-Term Results in Recipients of Combined Hla-Mismatched Kidney and Bone Marrow Transplantation Without Maintenance Immunosuppression. Am J Transplant (2014) 14:1599–611. doi: 10.1111/ajt.12731
28. Kawai T, Leventhal J, Wood K, Strober S. Summary of the Third International Workshop on Clinical Tolerance. Am J Transplant (2019) 19:324–30. doi: 10.1111/ajt.15086
29. Trzonkowski P, Bieniaszewska M, Juścińska J, Dobyszuk A, Krzystyniak A, Marek N, et al. First-in-Man Clinical Results of the Treatment of Patients With Graft Versus Host Disease With Human Ex Vivo Expanded CD4+CD25+CD127– T Regulatory Cells. Clin Immunol (2009) 133:22–6. doi: 10.1016/j.clim.2009.06.001
30. Liu W, Putnam AL, Xu-yu Z, Szot GL, Lee MR, Zhu S, et al. CD127 Expression Inversely Correlates With FoxP3 and Suppressive Function of Human CD4+ T Reg Cells. J Exp Med (2006) 203:1701–11. doi: 10.1084/jem.20060772
31. Marek-Trzonkowska N, Myśliwiec M, Dobyszuk A, Grabowska M, Derkowska I, Juścińska J, et al. Therapy of Type 1 Diabetes With CD4+CD25highCD127-Regulatory T Cells Prolongs Survival of Pancreatic Islets — Results of One Year Follow-Up. Clin Immunol (2014) 153:23–30. doi: 10.1016/j.clim.2014.03.016
32. Yu N, Li X, Song W, Li D, Yu D, Zeng X, et al. Cd4+Cd25+Cd127low/– T Cells: A More Specific Treg Population in Human Peripheral Blood. Inflammation (2012) 35:1773–80. doi: 10.1007/s10753-012-9496-8
33. Miyara M, Yoshioka Y, Kitoh A, Shima T, Wing K, Niwa A, et al. Functional Delineation and Differentiation Dynamics of Human Cd4+ T Cells Expressing the FoxP3 Transcription Factor. Immunity (2009) 30:899–911. doi: 10.1016/j.immuni.2009.03.019
34. Lau AH, Vitalone MJ, Haas K, Shawler T, Esquivel CO, Berquist WE, et al. Mass Cytometry Reveals a Distinct Immunoprofile of Operational Tolerance in Pediatric Liver Transplantation. Pediatr Transplant (2016) 20:1072–80. doi: 10.1111/petr.12795
35. Qiu R, Zhou L, Ma Y, Zhou L, Liang T, Shi L, et al. Regulatory T Cell Plasticity and Stability and Autoimmune Diseases. Clin Rev Allergy Immunol (2020) 58:52–70. doi: 10.1007/s12016-018-8721-0
36. Halim L, Romano M, McGregor R, Correa I, Pavlidis P, Grageda N, et al. An Atlas of Human Regulatory T Helper-Like Cells Reveals Features of Th2-like Tregs That Support a Tumorigenic Environment. Cell Rep (2017) 20:757–70. doi: 10.1016/j.celrep.2017.06.079
37. Kunicki MA, Amaya Hernandez LC, Davis KL, Bacchetta R, Roncarolo M-G. Identity and Diversity of Human Peripheral Th and T Regulatory Cells Defined by Single-Cell Mass Cytometry. J Immunol (2018) 200:336–46. doi: 10.4049/jimmunol.1701025
38. Mason GM, Lowe K, Melchiotti R, Ellis R, de Rinaldis E, Peakman M, et al. Phenotypic Complexity of the Human Regulatory T Cell Compartment Revealed by Mass Cytometry. J Immunol (2015) 195:2030–7. doi: 10.4049/jimmunol.1500703
39. Gagliani N, Magnani CF, Huber S, Gianolini ME, Pala M, Licona-Limon P, et al. Coexpression of CD49b and LAG-3 Identifies Human and Mouse T Regulatory Type 1 Cells. Nat Med (2013) 19:739–46. doi: 10.1038/nm.3179
40. Magnani CF, Alberigo G, Bacchetta R, Serafini G, Andreani M, Roncarolo MG, et al. Killing of Myeloid APCs Via HLA Class I, CD2 and CD226 Defines a Novel Mechanism of Suppression by Human Tr1 Cells. Eur J Immunol (2011) 41:1652–62. doi: 10.1002/eji.201041120
41. Pellerin L, Jenks JA, Chinthrajah S, Dominguez T, Block W, Zhou X, et al. Peanut-Specific Type 1 Regulatory T Cells Induced In Vitro From Allergic Subjects are Functionally Impaired. J Allergy Clin Immunol (2018) 141:202–13. doi: 10.1016/j.jaci.2017.05.045
42. Gagliani N, Jofra T, Valle A, Stabilini A, Morsiani C, Gregori S, et al. Transplant Tolerance to Pancreatic Islets Is Initiated in the Graft and Sustained in the Spleen. Am J Transplant (2013) 13:1963–75. doi: 10.1111/ajt.12333
43. Roncarolo MG, Gregori S, Bacchetta R, Battaglia M, Gagliani N. The Biology of T Regulatory Type 1 Cells and Their Therapeutic Application in Immune-Mediated Diseases. Immunity (2018) 49:1004–19. doi: 10.1016/j.immuni.2018.12.001
44. Cherukuri A, Rothstein DM, Clark B, Carter CR, Davison A, Hernandez-Fuentes M, et al. Immunologic Human Renal Allograft Injury Associates With an Altered Il-10/TNF- α Expression Ratio in Regulatory B Cells. J Am Soc Nephrol (2014) 25:1575–85. doi: 10.1681/ASN.2013080837
45. Bigot J, Pilon C, Matignon M, Grondin C, Leibler C, Aissat A, et al. Transcriptomic Signature of the CD24 Hi CD38 Hi Transitional B Cells Associated With an Immunoregulatory Phenotype in Renal Transplant Recipients. Am J Transplant (2016) 16:3430–42. doi: 10.1111/ajt.13904
46. Aravena O, Ferrier A, Menon M, Mauri C, Aguillón JC, Soto L, et al. TIM-1 Defines a Human Regulatory B Cell Population That is Altered in Frequency and Function in Systemic Sclerosis Patients. Arthritis Res Ther (2017) 19:8. doi: 10.1186/s13075-016-1213-9
47. Kühl AA, Pawlowski NN, Grollich K, Blessenohl M, Westermann J, Zeitz M, et al. Human Peripheral γδ T Cells Possess Regulatory Potential. Immunology (2009) 128:580–8. doi: 10.1111/j.1365-2567.2009.03162.x
48. Peters C, Oberg H-H, Kabelitz D, Wesch D. Phenotype and Regulation of Immunosuppressive Vδ2-Expressing γδ T Cells. Cell Mol Life Sci (2014) 71:1943–60. doi: 10.1007/s00018-013-1467-1
49. Casetti R, Agrati C, Wallace M, Sacchi A, Martini F, Martino A, et al. Cutting Edge: Tgf-β1 and IL-15 Induce FOXP3 + γδ Regulatory T Cells in the Presence of Antigen Stimulation. J Immunol (2009) 183:3574–7. doi: 10.4049/jimmunol.0901334
50. Traxlmayr MW, Wesch D, Dohnal AM, Funovics P, Fischer MB, Kabelitz D, et al. Immune Suppression by γδ T-cells as a Potential Regulatory Mechanism After Cancer Vaccination With Il-12 Secreting Dendritic Cells. J Immunother (2010) 33:40–52. doi: 10.1097/CJI.0b013e3181b51447
51. Hua F, Kang N, Gao Y-A, Cui L-X, Ba D-N, He W. Potential Regulatory Role of In Vitro-Expanded Vδ1 T Cells From Human Peripheral Blood. Immunol Res (2013) 56:172–80. doi: 10.1007/s12026-013-8390-2
52. Wesch D, Peters C, Siegers GM. Human Gamma Delta T Regulatory Cells in Cancer: Fact or Fiction? Front Immunol (2014) 5:598. doi: 10.3389/fimmu.2014.00598
53. Zhou J, Appleton SE, Stadnyk A, Lee TDG, Nashan BAP. Cd8 + γδ T Regulatory Cells Mediate Kidney Allograft Prolongation After Oral Exposure to Alloantigen. Transpl Int (2008) 21:679–87. doi: 10.1111/j.1432-2277.2008.00669.x
54. Peters C, Kabelitz D, Wesch D. Regulatory Functions of γδ T Cells. Cell Mol Life Sci (2018) 75:2125–35. doi: 10.1007/s00018-018-2788-x
55. Takahashi T, Dejbakhsh-Jones S, Strober S. Expression of CD161 (Nkr-P1a) Defines Subsets of Human CD4 and CD8 T Cells With Different Functional Activities. J Immunol (2006) 176:211–6. doi: 10.4049/jimmunol.176.1.211
56. Savage AK, Constantinides MG, Han J, Picard D, Martin E, Li B, et al. The Transcription Factor PLZF Directs the Effector Program of the NKT Cell Lineage. Immunity (2008) 29:391–403. doi: 10.1016/j.immuni.2008.07.011
57. Pellicci DG, Koay H-F, Berzins SP. Thymic Development of Unconventional T Cells: How NKT Cells, MAIT Cells and γδ T Cells Emerge. Nat Rev Immunol (2020) 20:756–70. doi: 10.1038/s41577-020-0345-y
58. Schultz KR, Kariminia A, Ng B, Abdossamadi S, Lauener M, Nemecek ER, et al. Immune Profile Differences Between Chronic GVHD and Late Acute GVHD: Results of the ABLE/PBMTC 1202 Studies. Blood (2020) 135:1287–98. doi: 10.1182/blood.2019003186
59. Kariminia A, Ivison S, Ng B, Rozmus J, Sung S, Varshney A, et al. CD56 Bright Natural Killer Regulatory Cells in Filgrastim Primed Donor Blood or Marrow Products Regulate Chronic Graft- Versus -Host Disease: The Canadian Blood and Marrow Transplant Group Randomized 0601 Study Results. Haematologica (2017) 102:1936–46. doi: 10.3324/haematol.2017.170928
60. Gershon RK, Kondo K. Cell Interactions in the Induction of Tolerance: The Role of Thymic Lymphocytes. Immunology (1970) 18:723–37.
61. Sakaguchi S, Sakaguchi N, Asano M, Itoh M, Toda M. Immunologic Self-Tolerance Maintained by Activated T Cells Expressing IL-2 Receptor Alpha-Chains (CD25). Breakdown of a Single Mechanism of Self-Tolerance Causes Various Autoimmune Diseases. J Immunol (1995) 155:1151–64.
62. Caramalho Í, Nunes-Cabaço H, Foxall RB, Sousa AE. Regulatory T-Cell Development in the Human Thymus. Front Immunol (2015) 6:395. doi: 10.3389/fimmu.2015.00395
63. Fontenot JD, Gavin MA, Rudensky AY. Foxp3 Programs the Development and Function of CD4+CD25+ Regulatory T Cells. Nat Immunol (2003) 4:330–6. doi: 10.1038/ni904
64. Baecher-Allan C, Brown JA, Freeman GJ, Hafler DA. Cd4 + CD25 High Regulatory Cells in Human Peripheral Blood. J Immunol (2001) 167:1245–53. doi: 10.4049/jimmunol.167.3.1245
65. Chen X, Bäumel M, Männel DN, Howard OMZ, Oppenheim JJ. Interaction of TNF With TNF Receptor Type 2 Promotes Expansion and Function of Mouse Cd4 + CD25 + T Regulatory Cells. J Immunol (2007) 179:154–61. doi: 10.4049/jimmunol.179.1.154
66. Thornton AM, Shevach EM. Cd4+Cd25+ Immunoregulatory T Cells Suppress Polyclonal T Cell Activation in Vitro by Inhibiting Interleukin 2 Production. J Exp Med (1998) 188:287–96. doi: 10.1084/jem.188.2.287
67. Yagi H, Nomura T, Nakamura K, Yamazaki S, Kitawaki T, Hori S, et al. Crucial Role of FOXP3 in the Development and Function of Human CD25+CD4+ Regulatory T Cells. Int Immunol (2004) 16:1643–56. doi: 10.1093/intimm/dxh165
68. Hori S. Control of Regulatory T Cell Development by the Transcription Factor Foxp3. Sci (80- ) (2003) 299:1057–61. doi: 10.1126/science.1079490
69. Khattri R, Cox T, Yasayko S-A, Ramsdell F. An Essential Role for Scurfin in CD4+CD25+ T Regulatory Cells. Nat Immunol (2003) 4:337–42. doi: 10.1038/ni909
70. Bennett CL, Christie J, Ramsdell F, Brunkow ME, Ferguson PJ, Whitesell L, et al. The Immune Dysregulation, Polyendocrinopathy, Enteropathy, X-linked Syndrome (IPEX) is Caused by Mutations of FOXP3. Nat Genet (2001) 27:20–1. doi: 10.1038/83713
71. Chen X, Hamano R, Subleski JJ, Hurwitz AA, Howard OMZ, Oppenheim JJ. Expression of Costimulatory Tnfr2 Induces Resistance of CD4 + FoxP3 – Conventional T Cells to Suppression by CD4 + FoxP3 + Regulatory T Cells. J Immunol (2010) 185:174–82. doi: 10.4049/jimmunol.0903548
72. Martin F, Ladoire S, Mignot G, Apetoh L, Ghiringhelli F. Human FOXP3 and Cancer. Oncogene (2010) 29:4121–9. doi: 10.1038/onc.2010.174
73. Wildin RS, Ramsdell F, Peake J, Faravelli F, Casanova J-L, Buist N, et al. X-Linked Neonatal Diabetes Mellitus, Enteropathy and Endocrinopathy Syndrome is the Human Equivalent of Mouse Scurfy. Nat Genet (2001) 27:18–20. doi: 10.1038/83707
74. Toker A, Engelbert D, Garg G, Polansky JK, Floess S, Miyao T, et al. Active Demethylation of the Foxp3 Locus Leads to the Generation of Stable Regulatory T Cells Within the Thymus. J Immunol (2013) 190:3180–8. doi: 10.4049/jimmunol.1203473
75. Baron U, Floess S, Wieczorek G, Baumann K, Grützkau A, Dong J, et al. DNA Demethylation in the humanFOXP3 Locus Discriminates Regulatory T Cells From Activated FOXP3+ Conventional T Cells. Eur J Immunol (2007) 37:2378–89. doi: 10.1002/eji.200737594
76. Wieczorek G, Asemissen A, Model F, Turbachova I, Floess S, Liebenberg V, et al. Quantitative DNA Methylation Analysis of FOXP3 as a New Method for Counting Regulatory T Cells in Peripheral Blood and Solid Tissue. Cancer Res (2009) 69:599–608. doi: 10.1158/0008-5472.CAN-08-2361
77. Lal G, Zhang N, van der Touw W, Ding Y, Ju W, Bottinger EP, et al. Epigenetic Regulation of Foxp3 Expression in Regulatory T Cells by DNA Methylation. J Immunol (2009) 182:259–73. doi: 10.4049/jimmunol.182.1.259
78. Tai X, Cowan M, Feigenbaum L, Singer A. CD28 Costimulation of Developing Thymocytes Induces Foxp3 Expression and Regulatory T Cell Differentiation Independently of Interleukin 2. Nat Immunol (2005) 6:152–62. doi: 10.1038/ni1160
79. Mantel P-Y, Ouaked N, Rückert B, Karagiannidis C, Welz R, Blaser K, et al. Molecular Mechanisms Underlying FOXP3 Induction in Human T Cells. J Immunol (2006) 176:3593–602. doi: 10.4049/jimmunol.176.6.3593
80. Nazzal D, Gradolatto A, Truffault F, Bismuth J, Berrih-Aknin S. Human Thymus Medullary Epithelial Cells Promote Regulatory T-cell Generation by Stimulating Interleukin-2 Production Via ICOS Ligand. Cell Death Dis (2014) 5:e1420–0. doi: 10.1038/cddis.2014.377
81. Sauer S, Bruno L, Hertweck A, Finlay D, Leleu M, Spivakov M, et al. T Cell Receptor Signaling Controls Foxp3 Expression Via PI3K, Akt, and Mtor. Proc Natl Acad Sci (2008) 105:7797–802. doi: 10.1073/pnas.0800928105
82. Allan SE, Crome SQ, Crellin NK, Passerini L, Steiner TS, Bacchetta R, et al. Activation-Induced FOXP3 in Human T Effector Cells Does Not Suppress Proliferation or Cytokine Production. Int Immunol (2007) 19:345–54. doi: 10.1093/intimm/dxm014
83. Laurence A, Tato CM, Davidson TS, Kanno Y, Chen Z, Yao Z, et al. Interleukin-2 Signaling Via STAT5 Constrains T Helper 17 Cell Generation. Immunity (2007) 26:371–81. doi: 10.1016/j.immuni.2007.02.009
84. Horwitz DA, Zheng SG, Wang J, Gray JD. Critical Role of IL-2 and TGF-β in Generation, Function and Stabilization of Foxp3+CD4+ Treg. Eur J Immunol (2008) 38:912–5. doi: 10.1002/eji.200738109
85. Schmidt A, Eriksson M, Shang M-M, Weyd H, Tegnér J. Comparative Analysis of Protocols to Induce Human CD4+Foxp3+ Regulatory T Cells by Combinations of IL-2, TGF-Beta, Retinoic Acid, Rapamycin and Butyrate. PloS One (2016) 11:e0148474. doi: 10.1371/journal.pone.0148474
86. Vignali DAA, Collison LW, Workman CJ. How Regulatory T Cells Work. Nat Rev Immunol (2008) 8:523–32. doi: 10.1038/nri2343
87. Gondek DC, Lu L-F, Quezada SA, Sakaguchi S, Noelle RJ. Cutting Edge: Contact-Mediated Suppression by CD4 + CD25 + Regulatory Cells Involves a Granzyme B-Dependent, Perforin-Independent Mechanism. J Immunol (2005) 174:1783–6. doi: 10.4049/jimmunol.174.4.1783
88. Cao X, Cai SF, Fehniger TA, Song J, Collins LI, Piwnica-Worms DR, et al. Granzyme B and Perforin are Important for Regulatory T Cell-Mediated Suppression of Tumor Clearance. Immunity (2007) 27:635–46. doi: 10.1016/j.immuni.2007.08.014
89. Garín MI, Chu C-C, Golshayan D, Cernuda-Morollón E, Wait R, Lechler RI. Galectin-1: A Key Effector of Regulation Mediated by CD4+CD25+ T Cells. Blood (2007) 109:2058–65. doi: 10.1182/blood-2006-04-016451
90. Lu H, Dai X, Li X, Sun Y, Gao Y, Zhang C. Gal-1 Regulates Dendritic Cells-Induced Treg/Th17 Balance Though NF-κb/RelB-IL-27 Pathway. Ann Transl Med (2019) 7:628–8. doi: 10.21037/atm.2019.11.02
91. Madireddi S, Eun S-Y, Mehta AK, Birta A, Zajonc DM, Niki T, et al. Regulatory T Cell–Mediated Suppression of Inflammation Induced by DR3 Signaling Is Dependent on Galectin-9. J Immunol (2017) 199:2721–8. doi: 10.4049/jimmunol.1700575
92. Deaglio S, Dwyer KM, Gao W, Friedman D, Usheva A, Erat A, et al. Adenosine Generation Catalyzed by CD39 and CD73 Expressed on Regulatory T Cells Mediates Immune Suppression. J Exp Med (2007) 204:1257–65. doi: 10.1084/jem.20062512
93. Kobie JJ, Shah PR, Yang L, Rebhahn JA, Fowell DJ, Mosmann TR. T Regulatory and Primed Uncommitted CD4 T Cells Express CD73, Which Suppresses Effector CD4 T Cells by Converting 5′-Adenosine Monophosphate to Adenosine. J Immunol (2006) 177:6780–6. doi: 10.4049/jimmunol.177.10.6780
94. Pandiyan P, Zheng L, Ishihara S, Reed J, Lenardo MJ. Cd4+Cd25+Foxp3+ Regulatory T Cells Induce Cytokine Deprivation–Mediated Apoptosis of Effector CD4+ T Cells. Nat Immunol (2007) 8:1353–62. doi: 10.1038/ni1536
95. Chinen T, Kannan AK, Levine AG, Fan X, Klein U, Zheng Y, et al. An Essential Role for the IL-2 Receptor in Treg Cell Function. Nat Immunol (2016) 17:1322–33. doi: 10.1038/ni.3540
96. Schmidt A, Oberle N, Krammer PH. Molecular Mechanisms of Treg-Mediated T Cell Suppression. Front Immunol (2012) 3:51. doi: 10.3389/fimmu.2012.00051
97. Read S, Malmström V, Powrie F. Cytotoxic T Lymphocyte–Associated Antigen 4 Plays an Essential Role in the Function of Cd25+Cd4+ Regulatory Cells That Control Intestinal Inflammation. J Exp Med (2000) 192:295–302. doi: 10.1084/jem.192.2.295
98. Oderup C, Cederbom L, Makowska A, Cilio CM, Ivars F. Cytotoxic T Lymphocyte Antigen-4-Dependent Down-Modulation of Costimulatory Molecules on Dendritic Cells in CD4+ CD25+ Regulatory T-cell-mediated Suppression. Immunology (2006) 118:240–9. doi: 10.1111/j.1365-2567.2006.02362.x
99. Hoeppli RE, MacDonald KN, Leclair P, Fung VCW, Mojibian M, Gillies J, et al. Tailoring the Homing Capacity of Human Tregs for Directed Migration to Sites of Th1-Inflammation or Intestinal Regions. Am J Transplant (2019) 19:62–76. doi: 10.1111/ajt.14936
100. Passerini L, Di Nunzio S, Gregori S, Gambineri E, Cecconi M, Seidel MG, et al. Functional Type 1 Regulatory T Cells Develop Regardless of FOXP3 Mutations in Patients With IPEX Syndrome. Eur J Immunol (2011) 41:1120–31. doi: 10.1002/eji.201040909
101. White AM, Wraith DC. Tr1-Like T Cells – An Enigmatic Regulatory T Cell Lineage. Front Immunol (2016) 7:355. doi: 10.3389/fimmu.2016.00355
102. Mascanfroni ID, Takenaka MC, Yeste A, Patel B, Wu Y, Kenison JE, et al. Metabolic Control of Type 1 Regulatory T Cell Differentiation by AHR and HIF1-α. Nat Med (2015) 21:638–46. doi: 10.1038/nm.3868
103. Newton R, Priyadharshini B, Turka LA. Immunometabolism of Regulatory T Cells. Nat Immunol (2016) 17:618–25. doi: 10.1038/ni.3466
104. Bacchetta R, Sartirana C, Levings MK, Bordignon C, Narula S, Roncarolo M-G. Growth and Expansion of Human T Regulatory Type 1 Cells are Independent From TCR Activation But Require Exogenous Cytokines. Eur J Immunol (2002) 32:2237. doi: 10.1002/1521-4141(200208)32:8<2237::AID-IMMU2237>3.0.CO;2-2
105. Brockmann L, Gagliani N, Steglich B, Giannou AD, Kempski J, Pelczar P, et al. Il-10 Receptor Signaling Is Essential for T R 1 Cell Function In Vivo. J Immunol (2017) 198:1130–41. doi: 10.4049/jimmunol.1601045
106. Groux H, O’Garra A, Bigler M, Rouleau M, Antonenko S, de Vries JE, et al. A CD4+T-cell Subset Inhibits Antigen-Specific T-cell Responses and Prevents Colitis. Nature (1997) 389:737–42. doi: 10.1038/39614
107. Akdis M, Verhagen J, Taylor A, Karamloo F, Karagiannidis C, Crameri R, et al. Immune Responses in Healthy and Allergic Individuals Are Characterized by a Fine Balance Between Allergen-Specific T Regulatory 1 and T Helper 2 Cells. J Exp Med (2004) 199:1567–75. doi: 10.1084/jem.20032058
108. Schmetterer KG, Neunkirchner A, Wojta-Stremayr D, Leitner J, Steinberger P, Pickl WF. STAT3 Governs Hyporesponsiveness and Granzyme B-dependent Suppressive Capacity in Human CD4 + T Cells. FASEB J (2015) 29:759–71. doi: 10.1096/fj.14-257584
109. Stumhofer JS, Silver JS, Laurence A, Porrett PM, Harris TH, Turka LA, et al. Interleukins 27 and 6 Induce STAT3-Mediated T Cell Production of Interleukin 10. Nat Immunol (2007) 8:1363–71. doi: 10.1038/ni1537
110. Murugaiyan G, Mittal A, Lopez-Diego R, Maier LM, Anderson DE, Weiner HL. Il-27 Is a Key Regulator of IL-10 and IL-17 Production by Human Cd4 + T Cells. J Immunol (2009) 183:2435–43. doi: 10.4049/jimmunol.0900568
111. Pot C, Jin H, Awasthi A, Liu SM, Lai C-Y, Madan R, et al. Cutting Edge: Il-27 Induces the Transcription Factor C-Maf, Cytokine Il-21, and the Costimulatory Receptor ICOS That Coordinately Act Together to Promote Differentiation of IL-10-Producing Tr1 Cells. J Immunol (2009) 183:797–801. doi: 10.4049/jimmunol.0901233
112. Iwasaki Y, Fujio K, Okamura T, Yanai A, Sumitomo S, Shoda H, et al. Egr-2 Transcription Factor is Required for Blimp-1-mediated Il-10 Production in IL-27-stimulated Cd4 + T Cells. Eur J Immunol (2013) 43:1063–73. doi: 10.1002/eji.201242942
113. Gandhi R, Kumar D, Burns EJ, Nadeau M, Dake B, Laroni A, et al. Activation of the Aryl Hydrocarbon Receptor Induces Human Type 1 Regulatory T Cell–Like and Foxp3+ Regulatory T Cells. Nat Immunol (2010) 11:846–53. doi: 10.1038/ni.1915
114. Apetoh L, Quintana FJ, Pot C, Joller N, Xiao S, Kumar D, et al. The Aryl Hydrocarbon Receptor Interacts With c-Maf to Promote the Differentiation of Type 1 Regulatory T Cells Induced by IL-27. Nat Immunol (2010) 11:854–61. doi: 10.1038/ni.1912
115. Karwacz K, Miraldi ER, Pokrovskii M, Madi A, Yosef N, Wortman I, et al. Critical Role of IRF1 and BATF in Forming Chromatin Landscape During Type 1 Regulatory Cell Differentiation. Nat Immunol (2017) 18:412–21. doi: 10.1038/ni.3683
116. Huang W, Solouki S, Koylass N, Zheng S-G, August A. ITK Signalling Via the Ras/IRF4 Pathway Regulates the Development and Function of Tr1 Cells. Nat Commun (2017) 8:15871. doi: 10.1038/ncomms15871
117. Zemmour D, Zilionis R, Kiner E, Klein AM, Mathis D, Benoist C. Single-Cell Gene Expression Reveals a Landscape of Regulatory T Cell Phenotypes Shaped by the TCR. Nat Immunol (2018) 19:291–301. doi: 10.1038/s41590-018-0051-0
118. Park J-E, Botting RA, Domínguez Conde C, Popescu D-M, Lavaert M, Kunz DJ, et al. A Cell Atlas of Human Thymic Development Defines T Cell Repertoire Formation. Sci (80- ) (2020) 367:eaay3224. doi: 10.1126/science.aay3224
119. Pan Y, Lu F, Fei Q, Yu X, Xiong P, Yu X, et al. Single-Cell RNA Sequencing Reveals Compartmental Remodeling of Tumor-Infiltrating Immune Cells Induced by anti-CD47 Targeting in Pancreatic Cancer. J Hematol Oncol (2019) 12:124. doi: 10.1186/s13045-019-0822-6
120. Henderson JG, Opejin A, Jones A, Gross C, Hawiger D. Cd5 Instructs Extrathymic Regulatory T Cell Development in Response to Self and Tolerizing Antigens. Immunity (2015) 42:471–83. doi: 10.1016/j.immuni.2015.02.010
121. Blaize G, Daniels-Treffandier H, Aloulou M, Rouquié N, Yang C, Marcellin M, et al. CD5 Signalosome Coordinates Antagonist TCR Signals to Control the Generation of Treg Cells Induced by Foreign Antigens. Proc Natl Acad Sci (2020) 117:12969–79. doi: 10.1073/pnas.1917182117
122. Sprouse ML, Scavuzzo MA, Blum S, Shevchenko I, Lee T, Makedonas G, et al. High Self-Reactivity Drives T-bet and Potentiates Treg Function in Tissue-Specific Autoimmunity. JCI Insight (2018) 3:e97322. doi: 10.1172/jci.insight.97322
123. Braza F, Dugast E, Panov I, Paul C, Vogt K, Pallier A, et al. Central Role of CD45RA – Foxp3 Hi Memory Regulatory T Cells in Clinical Kidney Transplantation Tolerance. J Am Soc Nephrol (2015) 26:1795–805. doi: 10.1681/ASN.2014050480
124. Miragaia RJ, Gomes T, Chomka A, Jardine L, Riedel A, Hegazy AN, et al. Single-Cell Transcriptomics of Regulatory T Cells Reveals Trajectories of Tissue Adaptation. Immunity (2019) 50:493–504. doi: 10.1016/j.immuni.2019.01.001
125. Luu M, Steinhoff U, Visekruna A. Functional Heterogeneity of Gut-Resident Regulatory T Cells. Clin Transl Immunol (2017) 6:e156. doi: 10.1038/cti.2017.39
126. Desreumaux P, Foussat A, Allez M, Beaugerie L, Hébuterne X, Bouhnik Y, et al. Safety and Efficacy of Antigen-Specific Regulatory T-Cell Therapy for Patients With Refractory Crohn’s Disease. Gastroenterology (2012) 143:1207–17. doi: 10.1053/j.gastro.2012.07.116
127. Blazar BR, MacDonald KPA, Hill GR. Immune Regulatory Cell Infusion for Graft-Versus-Host Disease Prevention and Therapy. Blood (2018) 131:2651–60. doi: 10.1182/blood-2017-11-785865
128. Elias S, Rudensky AY. Therapeutic Use of Regulatory T Cells for Graft-Versus-Host Disease. Br J Haematol (2019) 187:25–38. doi: 10.1111/bjh.16157
129. Katz S, Parker D, Turk J. B-Cell Suppression of Delayed Hypersensitivity Reactions. Nature (1974) 251:550–1. doi: 10.1038/251550a0
130. Neta R, Salvin SB. Specific Suppression of Delayed Hypersensitivity: The Possible Presence of a Suppressor B Cell in the Regulation of Delayed Hypersensitivity. J Immunol (1974) 113:1716–25.
131. Durand J, Huchet V, Merieau E, Usal C, Chesneau M, Remy S, et al. Regulatory B Cells With a Partial Defect in CD40 Signaling and Overexpressing Granzyme B Transfer Allograft Tolerance in Rodents. J Immunol (2015) 195:5035–44. doi: 10.4049/jimmunol.1500429
132. Tian J, Zekzer D, Hanssen L, Lu Y, Olcott A, Kaufman DL. Lipopolysaccharide-Activated B Cells Down-Regulate Th1 Immunity and Prevent Autoimmune Diabetes in Nonobese Diabetic Mice. J Immunol (2001) 167:1081–9. doi: 10.4049/jimmunol.167.2.1081
133. Newell KA, Asare A, Kirk AD, Gisler TD, Bourcier K, Suthanthiran M, et al. Identification of a B Cell Signature Associated With Renal Transplant Tolerance in Humans. J Clin Invest (2010) 120:1836–47. doi: 10.1172/JCI39933
134. Pallier A, Hillion S, Danger R, Giral M, Racapé M, Degauque N, et al. Patients With Drug-Free Long-Term Graft Function Display Increased Numbers of Peripheral B Cells With a Memory and Inhibitory Phenotype. Kidney Int (2010) 78:503–13. doi: 10.1038/ki.2010.162
135. Edwards SC, Sutton CE, Ladell K, Grant EJ, McLaren JE, Roche F, et al. A Population of Proinflammatory T Cells Coexpresses αβ and γδ T Cell Receptors in Mice and Humans. J Exp Med (2020) 217:e20190834. doi: 10.1084/jem.20190834
136. Harly C, Guillaume Y, Nedellec S, Peigné C-M, Mönkkönen H, Mönkkönen J, et al. Key Implication of CD277/butyrophilin-3 (BTN3A) in Cellular Stress Sensing by a Major Human γδ T-cell Subset. Blood (2012) 120:2269–79. doi: 10.1182/blood-2012-05-430470
137. Rigau M, Ostrouska S, Fulford TS, Johnson DN, Woods K, Ruan Z, et al. Butyrophilin 2A1 is Essential for Phosphoantigen Reactivity by γδ T Cells. Sci (80- ) (2020) 367:eaay5516. doi: 10.1126/science.aay5516
138. Correia DV, Lopes AC, Silva-Santos B. Tumor Cell Recognition by γδ T Lymphocytes. Oncoimmunology (2013) 2:e22892. doi: 10.4161/onci.22892
139. Patel SS, Wacholtz MC, Duby AD, Thiele DL, Lipsky PE. Analysis of the Functional Capabilities of CD3+CD4-CD8- and CD3+CD4+CD8+ Human T Cell Clones. J Immunol (1989) 143:1108–17.
140. Chabab G, Barjon C, Abdellaoui N, Salvador-Prince L, Dejou C, Michaud H, et al. Identification of a Regulatory Vδ1 Gamma Delta T Cell Subpopulation Expressing CD73 in Human Breast Cancer. J Leukoc Biol (2020) 107:1057–67. doi: 10.1002/JLB.3MA0420-278RR
141. Bhagat G, Naiyer AJ, Shah JG, Harper J, Jabri B, Wang TC, et al. Small Intestinal CD8+Tcrγδ+NKG2A+ Intraepithelial Lymphocytes Have Attributes of Regulatory Cells in Patients With Celiac Disease. J Clin Invest (2008) 118:281–93. doi: 10.1172/JCI30989
142. Nagaeva O, Jonsson L, Mincheva-Nilsson L. Dominant IL-10 and TGF-β Mrna Expression in γδt Cells of Human Early Pregnancy Decidua Suggests Immunoregulatory Potential. Am J Reprod Immunol (2002) 48:9–17. doi: 10.1034/j.1600-0897.2002.01131.x
143. Fan D-X, Duan J, Li M-Q, Xu B, Li D-J, Jin L-P. The Decidual Gamma-Delta T Cells Up-Regulate the Biological Functions of Trophoblasts Via IL-10 Secretion in Early Human Pregnancy. Clin Immunol (2011) 141:284–92. doi: 10.1016/j.clim.2011.07.008
144. Lee YJ, Holzapfel KL, Zhu J, Jameson SC, Hogquist KA. Steady-State Production of IL-4 Modulates Immunity in Mouse Strains and is Determined by Lineage Diversity of iNKT Cells. Nat Immunol (2013) 14:1146–54. doi: 10.1038/ni.2731
145. Wilson MT, Johansson C, Olivares-Villagomez D, Singh AK, Stanic AK, Wang C-R, et al. The Response of Natural Killer T Cells to Glycolipid Antigens is Characterized by Surface Receptor Down-Modulation and Expansion. Proc Natl Acad Sci (2003) 100:10913–8. doi: 10.1073/pnas.1833166100
146. Gadue P, Stein PL. Nk T Cell Precursors Exhibit Differential Cytokine Regulation and Require Itk for Efficient Maturation. J Immunol (2002) 169:2397–406. doi: 10.4049/jimmunol.169.5.2397
147. Berzins SP, McNab FW, Jones CM, Smyth MJ, Godfrey DI. Long-Term Retention of Mature Nk1.1 + NKT Cells in the Thymus. J Immunol (2006) 176:4059–65. doi: 10.4049/jimmunol.176.7.4059
148. Gao B, Radaeva S, Park O. Liver Natural Killer and Natural Killer T Cells: Immunobiology and Emerging Roles in Liver Diseases. J Leukoc Biol (2009) 86:513–28. doi: 10.1189/JLB.0309135
149. Heymann F, Tacke F. Immunology in the Liver — From Homeostasis to Disease. Nat Rev Gastroenterol Hepatol (2016) 13:88–110. doi: 10.1038/nrgastro.2015.200
150. Hongo D, Tang X, Dutt S, Nador RG, Strober S. Interactions Between NKT Cells and Tregs are Required for Tolerance to Combined Bone Marrow and Organ Transplants. Blood (2012) 119:1581–9. doi: 10.1182/blood-2011-08-371948
151. Zhou L, Adrianto I, Wang J, Wu X, Datta I, Mi Q-S. Single-Cell RNA-Seq Analysis Uncovers Distinct Functional Human NKT Cell Sub-Populations in Peripheral Blood. Front Cell Dev Biol (2020) 8:384. doi: 10.3389/fcell.2020.00384
152. Terabe M, Matsui S, Noben-Trauth N, Chen H, Watson C, Donaldson DD, et al. NKT Cell–Mediated Repression of Tumor Immunosurveillance by IL-13 and the IL-4R–STAT6 Pathway. Nat Immunol (2000) 1:515–20. doi: 10.1038/82771
153. Bricard G, Cesson V, Devevre E, Bouzourene H, Barbey C, Rufer N, et al. Enrichment of Human CD4+ V(Alpha)24/Vbeta11 Invariant NKT Cells in Intrahepatic Malignant Tumors. J Immunol (2009) 182:5140–51. doi: 10.4049/jimmunol.0711086
154. Haraguchi K, Takahashi T, Hiruma K, Kanda Y, Tanaka Y, Ogawa S, et al. Recovery of Vα24+ NKT Cells After Hematopoietic Stem Cell Transplantation. Bone Marrow Transplant (2004) 34:595–602. doi: 10.1038/sj.bmt.1704582
155. Petrie EJ, Clements CS, Lin J, Sullivan LC, Johnson D, Huyton T, et al. Cd94-NKG2A Recognition of Human Leukocyte Antigen (HLA)-E Bound to an HLA Class I Leader Sequence. J Exp Med (2008) 205:725–35. doi: 10.1084/jem.20072525
156. Raulet DH, Vance RE, McMahon CW. Regulation of the Natural Killer Cell Receptor Repertoire. Annu Rev Immunol (2001) 19:291–330. doi: 10.1146/annurev.immunol.19.1.291
157. Bauer S. Activation of NK Cells and T Cells by NKG2D, A Receptor for Stress-Inducible Mica. Sci (80- ) (1999) 285:727–9. doi: 10.1126/science.285.5428.727
158. Sivori S, Vacca P, Del Zotto G, Munari E, Mingari MC, Moretta L. Human NK Cells: Surface Receptors, Inhibitory Checkpoints, and Translational Applications. Cell Mol Immunol (2019) 16:430–41. doi: 10.1038/s41423-019-0206-4
159. Yang C, Siebert JR, Burns R, Gerbec ZJ, Bonacci B, Rymaszewski A, et al. Heterogeneity of Human Bone Marrow and Blood Natural Killer Cells Defined by Single-Cell Transcriptome. Nat Commun (2019) 10:3931. doi: 10.1038/s41467-019-11947-7
160. Smith SL, Kennedy PR, Stacey KB, Worboys JD, Yarwood A, Seo S, et al. Diversity of Peripheral Blood Human NK Cells Identified by Single-Cell RNA Sequencing. Blood Adv (2020) 4:1388–406. doi: 10.1182/bloodadvances.2019000699
161. Cacalano NA. Regulation of Natural Killer Cell Function by STAT3. Front Immunol (2016) 7:128. doi: 10.3389/fimmu.2016.00128
162. Tao Y, Li Y-H, Piao H-L, Zhou W-J, Zhang D, Fu Q, et al. Cd56brightcd25+ NK Cells are Preferentially Recruited to the Maternal/Fetal Interface in Early Human Pregnancy. Cell Mol Immunol (2015) 12:77–86. doi: 10.1038/cmi.2014.26
163. Le Gars M, Seiler C, Kay AW, Bayless NL, Starosvetsky E, Moore L, et al. Pregnancy-Induced Alterations in NK Cell Phenotype and Function. Front Immunol (2019) 10:2469. doi: 10.3389/fimmu.2019.02469
164. Leventhal JR, Elliott MJ, Yolcu ES, Bozulic LD, Tollerud DJ, Mathew JM, et al. Immune Reconstitution/Immunocompetence in Recipients of Kidney Plus Hematopoietic Stem/Facilitating Cell Transplants. Transplantation (2015) 99:288–98. doi: 10.1097/TP.0000000000000605
165. Scandling JD, Busque S, Shizuru JA, Lowsky R, Hoppe R, Dejbakhsh-Jones S, et al. Chimerism, Graft Survival, and Withdrawal of Immunosuppressive Drugs in HLA Matched and Mismatched Patients After Living Donor Kidney and Hematopoietic Cell Transplantation. Am J Transplant (2015) 15:695–704. doi: 10.1111/ajt.13091
166. Messner F, Etra JW, Dodd-o JM, Brandacher G. Chimerism, Transplant Tolerance, and Beyond. Transplantation (2019) 103:1556–67. doi: 10.1097/TP.0000000000002711
167. Leventhal J, Abecassis M, Miller J, Gallon L, Tollerud D, Elliott MJ, et al. Tolerance Induction in HLA Disparate Living Donor Kidney Transplantation by Donor Stem Cell Infusion. Transplantation (2013) 95:169–76. doi: 10.1097/TP.0b013e3182782fc1
168. Aversa F, Tabilio A, Velardi A, Cunningham I, Terenzi A, Falzetti F, et al. Treatment of High-Risk Acute Leukemia With T-Cell–Depleted Stem Cells From Related Donors With One Fully Mismatched Hla Haplotype. N Engl J Med (1998) 339:1186–93. doi: 10.1056/NEJM199810223391702
169. Martelli MF, Di Ianni M, Ruggeri L, Falzetti F, Carotti A, Terenzi A, et al. HLA-Haploidentical Transplantation With Regulatory and Conventional T-cell Adoptive Immunotherapy Prevents Acute Leukemia Relapse. Blood (2014) 124:638–44. doi: 10.1182/blood-2014-03-564401
170. Bertaina A, Merli P, Rutella S, Pagliara D, Bernardo ME, Masetti R, et al. HLA-Haploidentical Stem Cell Transplantation After Removal of αβ+ T and B Cells in Children With Nonmalignant Disorders. Blood (2014) 124:822–6. doi: 10.1182/blood-2014-03-563817
171. Bertaina A, Zecca M, Buldini B, Sacchi N, Algeri M, Saglio F, et al. Unrelated Donor vs HLA-haploidentical α/β T-cell– and B-cell–depleted HSCT in Children With Acute Leukemia. Blood (2018) 132:2594–607. doi: 10.1182/blood-2018-07-861575
172. Locatelli F, Pende D, Mingari MC, Bertaina A, Falco M, Moretta A, et al. Cellular and Molecular Basis of Haploidentical Hematopoietic Stem Cell Transplantation in the Successful Treatment of High-Risk Leukemias: Role of Alloreactive NK Cells. Front Immunol (2013) 4:15. doi: 10.3389/fimmu.2013.00015
173. Merli P, Algeri M, Galaverna F, Milano GM, Bertaina V, Biagini S, et al. Immune Modulation Properties of Zoledronic Acid on Tcrγδ T-Lymphocytes After Tcrαβ/Cd19-Depleted Haploidentical Stem Cell Transplantation: An Analysis on 46 Pediatric Patients Affected by Acute Leukemia. Front Immunol (2020) 11:699. doi: 10.3389/fimmu.2020.00699
174. Locatelli F, Bauquet A, Palumbo G, Moretta F, Bertaina A. Negative Depletion of α/β+ T Cells and of CD19+ B Lymphocytes: A Novel Frontier to Optimize the Effect of Innate Immunity in HLA-Mismatched Hematopoietic Stem Cell Transplantation. Immunol Lett (2013) 155:21–3. doi: 10.1016/j.imlet.2013.09.027
175. Maschan M, Shelikhova L, Ilushina M, Kurnikova E, Boyakova E, Balashov D, et al. TCR-Alpha/Beta and CD19 Depletion and Treosulfan-Based Conditioning Regimen in Unrelated and Haploidentical Transplantation in Children With Acute Myeloid Leukemia. Bone Marrow Transplant (2016) 51:668–74. doi: 10.1038/bmt.2015.343
176. Locatelli F, Merli P, Pagliara D, Li Pira G, Falco M, Pende D, et al. Outcome of Children With Acute Leukemia Given HLA-haploidentical HSCT After αβ T-cell and B-cell Depletion. Blood (2017) 130:677–85. doi: 10.1182/blood-2017-04-779769
177. Bertaina A, Bacchetta R, Lewis DB, Grimm PC, Shah AJ, Agarwal R, et al. αβ T-Cell/CD19 B-Cell Depleted Haploidentical Stem Cell Transplantation: A New Platform for Curing Rare and Monogenic Disorders. Transplant Cell Ther Meet (2020) 26:S288. doi: 10.1016/j.bbmt.2019.12.560
178. Roncarolo MG, Gregori S, Bacchetta R, Battaglia M. Tr1 Cells and the Counter-Regulation of Immunity: Natural Mechanisms and Therapeutic Applications. In: Fillatreau S, O'Garra A. (eds) Current Topics in Microbiology and Immunology (2014) 380:39–68. doi: 10.1007/978-3-662-43492-5_3
179. Vaikunthanathan T, Safinia N, Boardman D, Lechler RI, Lombardi G. Regulatory T Cells: Tolerance Induction in Solid Organ Transplantation. Clin Exp Immunol (2017) 189:197–210. doi: 10.1111/cei.12978
180. Todo S, Yamashita K, Goto R, Zaitsu M, Nagatsu A, Oura T, et al. A Pilot Study of Operational Tolerance With a Regulatory T-cell-based Cell Therapy in Living Donor Liver Transplantation. Hepatology (2016) 64:632–43. doi: 10.1002/hep.28459
181. Chandran S, Tang Q, Sarwal M, Laszik ZG, Putnam AL, Lee K, et al. Polyclonal Regulatory T Cell Therapy for Control of Inflammation in Kidney Transplants. Am J Transplant (2017) 17:2945–54. doi: 10.1111/ajt.14415
182. Sawitzki B, Harden PN, Reinke P, Moreau A, Hutchinson JA, Game DS, et al. Regulatory Cell Therapy in Kidney Transplantation (the ONE Study): A Harmonised Design and Analysis of Seven non-Randomised, Single-Arm, Phase 1/2A Trials. Lancet (2020) 395:1627–39. doi: 10.1016/S0140-6736(20)30167-7
183. Roemhild A, Otto NM, Moll G, Abou-El-Enein M, Kaiser D, Bold G, et al. Regulatory T Cells for Minimising Immune Suppression in Kidney Transplantation: Phase I/IIa Clinical Trial. BMJ (2020) 371:m3734. doi: 10.1136/bmj.m3734
184. Oberbauer R, Edinger M, Berlakovich G, Kalhs P, Worel N, Heinze G, et al. A Prospective Controlled Trial to Evaluate Safety and Efficacy of In Vitro Expanded Recipient Regulatory T Cell Therapy and Tocilizumab Together With Donor Bone Marrow Infusion in HLA-Mismatched Living Donor Kidney Transplant Recipients (Trex001). Front Med (2021) 7:634260. doi: 10.3389/fmed.2020.634260
185. Morath C, Schmitt A, Kleist C, Daniel V, Opelz G, Süsal C, et al. Phase I Trial of Donor-Derived Modified Immune Cell Infusion in Kidney Transplantation. J Clin Invest (2020) 130:2364–76. doi: 10.1172/JCI133595
186. Koyama I, Bashuda H, Uchida K, Seino K, Habu S, Nakajima I, et al. A Clinical Trial With Adoptive Transfer of Ex Vivo-Induced, Donor-specific Immune-Regulatory Cells in Kidney Transplantation—a Second Report. Transplantation (2020) 104:2415–23. doi: 10.1097/TP.0000000000003149
187. Miyara M, Chader D, Sage E, Sugiyama D, Nishikawa H, Bouvry D, et al. Sialyl Lewis X (CD15s) Identifies Highly Differentiated and Most Suppressive FOXP3 High Regulatory T Cells in Humans. Proc Natl Acad Sci (2015) 112:7225–30. doi: 10.1073/pnas.1508224112
188. Eteghadi A, Pak F, Ahmadpoor P, Jamali S, Karimi M, Yekaninejad MS, et al. Th1, Th2, Th17 Cell Subsets in Two Different Immunosuppressive Protocols in Renal Allograft Recipients (Sirolimus vs Mycophenolate Mofetil): A Cohort Study. Int Immunopharmacol (2019) 67:319–25. doi: 10.1016/j.intimp.2018.12.033
189. Park CS, Moon HY, Han S, Chon JY, Chae MS, Hong SH, et al. Ischemic Time of Graft Liver Forces Th1-to-Th2 Activity Toward Th1 Activity in Patients Who Underwent Living Donor Liver Transplantation. Eur Cytokine Netw (2019) 30:23–8. doi: 10.1684/ecn.2019.0422
190. El-Ayachi I, Washburn WK, Schenk AD. Recent Progress in Treg Biology and Transplant Therapeutics. Curr Transplant Rep (2020) 7:131–9. doi: 10.1007/s40472-020-00278-y
191. Savage TM, Shonts BA, Obradovic A, Dewolf S, Lau S, Zuber J, et al. Early Expansion of Donor-Specific Tregs in Tolerant Kidney Transplant Recipients. JCI Insight (2018) 3:e124086. doi: 10.1172/jci.insight.124086
192. Picarda E, Bézie S, Boucault L, Autrusseau E, Kilens S, Meistermann D, et al. Transient Antibody Targeting of CD45RC Induces Transplant Tolerance and Potent Antigen-Specific Regulatory T Cells. JCI Insight (2017) 2:e90088. doi: 10.1172/jci.insight.90088
193. Mathew JM, Voss JH, McEwen ST, Konieczna I, Chakraborty A, Huang X, et al. Generation and Characterization of Alloantigen-Specific Regulatory T Cells For Clinical Transplant Tolerance. Sci Rep (2018) 8:1136. doi: 10.1038/s41598-018-19621-6
194. Lee K, Nguyen V, Lee K-M, Kang S-M, Tang Q. Attenuation of Donor-Reactive T Cells Allows Effective Control of Allograft Rejection Using Regulatory T Cell Therapy. Am J Transplant (2014) 14:27–38. doi: 10.1111/ajt.12509
195. MacDonald KG, Hoeppli RE, Huang Q, Gillies J, Luciani DS, Orban PC, et al. Alloantigen-Specific Regulatory T Cells Generated With a Chimeric Antigen Receptor. J Clin Invest (2016) 126:1413–24. doi: 10.1172/JCI82771
196. Ramlal R, Hildebrandt GC. Advances in the Use of Regulatory T-Cells for the Prevention and Therapy of Graft-vs.-Host Disease. Biomedicines (2017) 5:23. doi: 10.3390/biomedicines5020023
197. Granofszky N, Farkas AM, Muckenhuber M, Mahr B, Unger L, Maschke S, et al. Anti-Interleukin-6 Promotes Allogeneic Bone Marrow Engraftment and Prolonged Graft Survival in an Irradiation-Free Murine Transplant Model. Front Immunol (2017) 8:821. doi: 10.3389/fimmu.2017.00821
198. Blaha P, Bigenzahn S, Koporc Z, Schmid M, Langer F, Selzer E, et al. The Influence of Immunosuppressive Drugs on Tolerance Induction Through Bone Marrow Transplantation With Costimulation Blockade. Blood (2003) 101:2886–93. doi: 10.1182/blood-2002-10-3014
199. Yamada Y, Ochiai T, Boskovic S, Nadazdin O, Oura T, Schoenfeld D, et al. Use of CTLA4Ig for Induction of Mixed Chimerism and Renal Allograft Tolerance in Nonhuman Primates. Am J Transplant (2014) 14:2704–12. doi: 10.1111/ajt.12936
200. Pilat N, Baranyi U, Klaus C, Jaeckel E, Mpofu N, Wrba F, et al. Treg-Therapy Allows Mixed Chimerism and Transplantation Tolerance Without Cytoreductive Conditioning. Am J Transplant (2010) 10:751–62. doi: 10.1111/j.1600-6143.2010.03018.x
201. Ferreira LMR, Muller YD, Bluestone JA, Tang Q. Next-Generation Regulatory T Cell Therapy. Nat Rev Drug Discovery (2019) 18:749–69. doi: 10.1038/s41573-019-0041-4
202. Noyan F, Zimmermann K, Hardtke-Wolenski M, Knoefel A, Schulde E, Geffers R, et al. Prevention of Allograft Rejection by Use of Regulatory T Cells With an MHC-Specific Chimeric Antigen Receptor. Am J Transplant (2017) 17:917–30. doi: 10.1111/ajt.14175
203. Boardman DA, Philippeos C, Fruhwirth GO, Ibrahim MAA, Hannen RF, Cooper D, et al. Expression of a Chimeric Antigen Receptor Specific for Donor Hla Class I Enhances the Potency of Human Regulatory T Cells in Preventing Human Skin Transplant Rejection. Am J Transplant (2017) 17:931–43. doi: 10.1111/ajt.14185
204. Dawson NAJ, Rosado-Sánchez I, Novakovsky GE, Fung VCW, Huang Q, McIver E, et al. Functional Effects of Chimeric Antigen Receptor Co-Receptor Signaling Domains in Human Regulatory T Cells. Sci Transl Med (2020) 12:eaaz3866. doi: 10.1126/scitranslmed.aaz3866
205. Dawson NAJ, Lamarche C, Hoeppli RE, Bergqvist P, Fung V, McIver E, et al. Systematic Testing and Specificity Mapping of Alloantigen-Specific Chimeric Antigen Receptors in T Regulatory Cells. JCI Insight (2019) 4:e123672. doi: 10.1172/jci.insight.123672
206. Pierini A, Iliopoulou BP, Peiris H, Pérez-Cruz M, Baker J, Hsu K, et al. T Cells Expressing Chimeric Antigen Receptor Promote Immune Tolerance. JCI Insight (2017) 2:e92865. doi: 10.1172/jci.insight.92865
207. Edinger M. Driving Allotolerance: CAR-expressing Tregs for Tolerance Induction in Organ and Stem Cell Transplantation. J Clin Invest (2016) 126:1248–50. doi: 10.1172/JCI86827
208. Loupy A, Hill GS, Jordan SC. The Impact of Donor-Specific anti-HLA Antibodies on Late Kidney Allograft Failure. Nat Rev Nephrol (2012) 8:348–57. doi: 10.1038/nrneph.2012.81
209. Djamali A, Kaufman DB, Ellis TM, Zhong W, Matas A, Samaniego M. Diagnosis and Management of Antibody-Mediated Rejection: Current Status and Novel Approaches. Am J Transplant (2014) 14:255–71. doi: 10.1111/ajt.12589
210. Meehan SM, Kremer J, Ali FN, Curley J, Marino S, Chang A, et al. Thrombotic Microangiopathy and Peritubular Capillary C4d Expression in Renal Allograft Biopsies. Clin J Am Soc Nephrol (2011) 6:395–403. doi: 10.2215/CJN.05870710
211. Hidalgo LG, Sis B, Sellares J, Campbell PM, Mengel M, Einecke G, et al. Nk Cell Transcripts and NK Cells in Kidney Biopsies From Patients With Donor-Specific Antibodies: Evidence for NK Cell Involvement in Antibody-Mediated Rejection. Am J Transplant (2010) 10:1812–22. doi: 10.1111/j.1600-6143.2010.03201.x
212. Bottomley MJ, Chen M, Fuggle S, Harden PN, Wood KJ. Application of Operational Tolerance Signatures are Limited by Variability and Type of Immunosuppression in Renal Transplant Recipients: A Cross-Sectional Study. Transplant Direct (2017) 3:e125. doi: 10.1097/TXD.0000000000000638
213. Song J, Du G, Chen W, Bao P, Li B, Lu Q, et al. The Advantage of Sirolimus in Amplifying Regulatory B Cells and Regulatory T Cells in Liver Transplant Patients. Eur J Pharmacol (2020) 869:172872. doi: 10.1016/j.ejphar.2019.172872
214. Siewe B, Stapleton JT, Martinson J, Keshavarzian A, Kazmi N, Demarais PM, et al. Regulatory B Cell Frequency Correlates With Markers of HIV Disease Progression and Attenuates Anti-HIV CD8 + T Cell Function In Vitro. J Leukoc Biol (2013) 93:811–8. doi: 10.1189/jlb.0912436
215. Blair PA, Noreña LY, Flores-Borja F, Rawlings DJ, Isenberg DA, Ehrenstein MR, et al. CD19+CD24hiCD38hi B Cells Exhibit Regulatory Capacity in Healthy Individuals But Are Functionally Impaired in Systemic Lupus Erythematosus Patients. Immunity (2010) 32:129–40. doi: 10.1016/j.immuni.2009.11.009
216. Lemoine S, Morva A, Youinou P, Jamin C. Human T Cells Induce Their Own Regulation Through Activation of B Cells. J Autoimmun (2011) 36:228–38. doi: 10.1016/j.jaut.2011.01.005
217. Leibler C, Matignon M, Pilon C, Montespan F, Bigot J, Lang P, et al. Kidney Transplant Recipients Treated With Belatacept Exhibit Increased Naïve and Transitional B Cells. Am J Transplant (2014) 14:1173–82. doi: 10.1111/ajt.12721
218. Furuzawa-Carballeda J, Uribe-Uribe NO, Arreola-Guerra JM, Reyes-Acevedo R, Vilatobá M, López-Toledo A, et al. Tissue Talks: Immunophenotype of Cells Infiltrating the Graft Explains Histological Findings and the Benefits of Belatacept at 10 Years. Clin Exp Immunol (2019) 197:250–61. doi: 10.1111/cei.13296
219. Beckett J, Hester J, Issa F, Shankar S. Regulatory B Cells in Transplantation: Roadmaps to Clinic. Transpl Int (2020) 33:1353–68. doi: 10.1111/tri.13706
220. Snanoudj R, Candon S, Roelen DL, Jais J-P, Claas FH, Legendre C, et al. Peripheral B-Cell Phenotype and BAFF Levels are Associated With HLA Immunization in Patients Awaiting Kidney Transplantation. Transplantation (2014) 97:917–24. doi: 10.1097/01.TP.0000438211.34842.5e
221. Banham GD, Flint SM, Torpey N, Lyons PA, Shanahan DN, Gibson A, et al. Belimumab in Kidney Transplantation: An Experimental Medicine, Randomised, Placebo-Controlled Phase 2 Trial. Lancet (2018) 391:2619–30. doi: 10.1016/S0140-6736(18)30984-X
222. Heidt S, Hester J, Shankar S, Friend PJ, Wood KJ. B Cell Repopulation After Alemtuzumab Induction—Transient Increase in Transitional B Cells and Long-Term Dominance of Naïve B Cells. Am J Transplant (2012) 12:1784–92. doi: 10.1111/j.1600-6143.2012.04012.x
223. Starling RC, Armstrong B, Bridges ND, Eisen H, Givertz MM, Kfoury AG, et al. Accelerated Allograft Vasculopathy With Rituximab After Cardiac Transplantation. J Am Coll Cardiol (2019) 74:36–51. doi: 10.1016/j.jacc.2019.04.056
224. Mao Y, Yin S, Zhang J, Hu Y, Huang B, Cui L, et al. A New Effect of IL-4 on Human γδ T Cells: Promoting Regulatory Vδ1 T Cells Via IL-10 Production and Inhibiting Function of Vδ2 T Cells. Cell Mol Immunol (2016) 13:217–28. doi: 10.1038/cmi.2015.07
225. Airoldi I, Bertaina A, Prigione I, Zorzoli A, Pagliara D, Cocco C, et al. γδ T-cell Reconstitution After HLA-haploidentical Hematopoietic Transplantation Depleted of TCR-αβ+/CD19+ Lymphocytes. Blood (2015) 125:2349–58. doi: 10.1182/blood-2014-09-599423
226. Déchanet J, Merville P, Bergé F, Bone-Mane G, Taupin J, Michel P, et al. Major Expansion of γδ T Lymphocytes Following Cytomegalovirus Infection in Kidney Allograft Recipients. J Infect Dis (1999) 179:1–8. doi: 10.1086/314568
227. Bertaina A, Zorzoli A, Petretto A, Barbarito G, Inglese E, Merli P, et al. Zoledronic Acid Boosts γδ T-cell Activity in Children Receiving αβ + T and CD19 + Cell-Depleted Grafts From An HLA-haplo-identical Donor. Oncoimmunology (2017) 6:e1216291. doi: 10.1080/2162402X.2016.1216291
228. Noguchi A, Kaneko T, Kamigaki T, Fujimoto K, Ozawa M, Saito M, et al. Zoledronate-Activated Vγ9γδ T Cell-Based Immunotherapy is Feasible and Restores the Impairment of γδ T Cells in Patients With Solid Tumors. Cytotherapy (2011) 13:92–7. doi: 10.3109/14653249.2010.515581
229. Schulz-Juergensen S, Marischen L, Wesch D, Oberg HH, Fändrich F, Kabelitz D, et al. Markers of Operational Immune Tolerance After Pediatric Liver Transplantation in Patients Under Immunosuppression. Pediatr Transplant (2013) 17:348–54. doi: 10.1111/petr.12079
230. Xuan L, Wu X, Qiu D, Gao L, Liu H, Fan Z, et al. Regulatory γδ T Cells Induced by G-CSF Participate in Acute Graft-Versus-Host Disease Regulation in G-CSF-mobilized Allogeneic Peripheral Blood Stem Cell Transplantation. J Transl Med (2018) 16:144. doi: 10.1186/s12967-018-1519-2
231. Morandi F, Yazdanifar M, Cocco C, Bertaina A, Airoldi I. Engineering the Bridge Between Innate and Adaptive Immunity for Cancer Immunotherapy: Focus on γδ T and NK Cells. Cells (2020) 9:1757. doi: 10.3390/cells9081757
232. Mavers M, Maas-Bauer K, Negrin RS. Invariant Natural Killer T Cells As Suppressors of Graft-versus-Host Disease in Allogeneic Hematopoietic Stem Cell Transplantation. Front Immunol (2017) 8:900. doi: 10.3389/fimmu.2017.00900
233. Bohne F, Londono M-C, Benitez C, Miquel R, Martinez-Llordella M, Russo C, et al. Hcv-Induced Immune Responses Influence the Development of Operational Tolerance After Liver Transplantation in Humans. Sci Transl Med (2014) 6:242ra81–242ra81. doi: 10.1126/scitranslmed.3008793
234. Moretta L, Locatelli F, Pende D, Marcenaro E, Mingari MC, Moretta A. Killer Ig–like Receptor-Mediated Control of Natural Killer Cell Alloreactivity in Haploidentical Hematopoietic Stem Cell Transplantation. Blood (2011) 117:764–71. doi: 10.1182/blood-2010-08-264085
235. Koenig A, Chen C-C, Marçais A, Barba T, Mathias V, Sicard A, et al. Missing Self Triggers NK Cell-Mediated Chronic Vascular Rejection of Solid Organ Transplants. Nat Commun (2019) 10:5350. doi: 10.1038/s41467-019-13113-5
236. van Bergen J, Thompson A, Haasnoot GW, Roodnat JI, de Fijter JW, Claas FHJ, et al. Kir-Ligand Mismatches are Associated With Reduced Long-Term Graft Survival in HLA-Compatible Kidney Transplantation. Am J Transplant (2011) 11:1959–64. doi: 10.1111/j.1600-6143.2011.03621.x
237. Nguyen S, Dhedin N, Vernant J-P, Kuentz M, Jijakli AA, Rouas-Freiss N, et al. NK-Cell Reconstitution After Haploidentical Hematopoietic Stem-Cell Transplantations: Immaturity of NK Cells and Inhibitory Effect of NKG2A Override GvL Effect. Blood (2005) 105:4135–42. doi: 10.1182/blood-2004-10-4113
238. Vago L, Forno B, Sormani MP, Crocchiolo R, Zino E, Di Terlizzi S, et al. Temporal, Quantitative, and Functional Characteristics of Single-KIR–positive Alloreactive Natural Killer Cell Recovery Account for Impaired Graft-Versus-Leukemia Activity After Haploidentical Hematopoietic Stem Cell Transplantation. Blood (2008) 112:3488–99. doi: 10.1182/blood-2007-07-103325
239. Ruggeri L. Effectiveness of Donor Natural Killer Cell Alloreactivity in Mismatched Hematopoietic Transplants. Sci (80-) (2002) 295:2097–100. doi: 10.1126/science.1068440
240. Pfefferle A, Huntington ND. You Have Got a Fast Car: Chimeric Antigen Receptor Nk Cells in Cancer Therapy. Cancers (Basel) (2020) 12:706. doi: 10.3390/cancers12030706
241. Heath WR, Carbone FR. The Skin-Resident and Migratory Immune System in Steady State and Memory: Innate Lymphocytes, Dendritic Cells and T Cells. Nat Immunol (2013) 14:978–85. doi: 10.1038/ni.2680
242. Simpson N, Cho YW, Cicciarelli JC, Selby RR, Fong T-L. Comparison of Renal Allograft Outcomes in Combined Liver-Kidney Transplantation Versus Subsequent Kidney Transplantation in Liver Transplant Recipients: Analysis of UNOS Database. Transplantation (2006) 82:1298–303. doi: 10.1097/01.tp.0000241104.58576.e6
243. Hanish SI, Samaniego M, Mezrich JD, Foley DP, Leverson GE, Lorentzen DF, et al. Outcomes of Simultaneous Liver/Kidney Transplants Are Equivalent to Kidney Transplant Alone: A Preliminary Report. Transplantation (2010) 90:52–60. doi: 10.1097/TP.0b013e3181e17014
244. De Creus A, Abe M, Lau AH, Hackstein H, Raimondi G, Thomson AW. Low TLR4 Expression by Liver Dendritic Cells Correlates With Reduced Capacity to Activate Allogeneic T Cells in Response to Endotoxin. J Immunol (2005) 174:2037–45. doi: 10.4049/jimmunol.174.4.2037
245. Bamboat ZM, Stableford JA, Plitas G, Burt BM, Nguyen HM, Welles AP, et al. Human Liver Dendritic Cells Promote T Cell Hyporesponsiveness. J Immunol (2009) 182:1901–11. doi: 10.4049/jimmunol.0803404
246. Goddard S, Youster J, Morgan E, Adams DH. Interleukin-10 Secretion Differentiates Dendritic Cells From Human Liver and Skin. Am J Pathol (2004) 164:511–9. doi: 10.1016/S0002-9440(10)63141-0
247. Heymann F, Peusquens J, Ludwig-Portugall I, Kohlhepp M, Ergen C, Niemietz P, et al. Liver Inflammation Abrogates Immunological Tolerance Induced by Kupffer Cells. Hepatology (2015) 62:279–91. doi: 10.1002/hep.27793
248. Knoll P, Schlaak J, Uhrig A, Kempf P, zum Büschenfelde K-HM, Gerken G. Human Kupffer Cells Secrete IL-10 in Response to Lipopolysaccharide (LPS) Challenge. J Hepatol (1995) 22:226–9. doi: 10.1016/0168-8278(95)80433-1
249. Knolle, Uhrig, Hegenbarth, LÖser, Schmitt, Gerken, et al. Il-10 Down-Regulates T Cell Activation by Antigen-Presenting Liver Sinusoidal Endothelial Cells Through Decreased Antigen Uptake Via the Mannose Receptor and Lowered Surface Expression of Accessory Molecules. Clin Exp Immunol (1998) 114:427–33. doi: 10.1046/j.1365-2249.1998.00713.x
250. Gabrilovich DI, Nagaraj S. Myeloid-Derived Suppressor Cells as Regulators of the Immune System. Nat Rev Immunol (2009) 9:162–74. doi: 10.1038/nri2506
251. Grau V, Herbst B, Steiniger B. Dynamics of Monocytes/Macrophages and T Lymphocytes in Acutely Rejecting Rat Renal Allografts. Cell Tissue Res (1997) 291:117–26. doi: 10.1007/s004410050985
252. Saiki T, Ezaki T, Ogawa M, Matsuno K. Trafficking of Host- and Donor-Derived Dendritic Cells in Rat Cardiac Transplantation: Allosensitization in the Spleen and Hepatic Nodes. Transplantation (2001) 71:1806–15. doi: 10.1097/00007890-200106270-00017
253. Walch JM, Zeng Q, Li Q, Oberbarnscheidt MH, Hoffman RA, Williams AL, et al. Cognate Antigen Directs CD8+ T Cell Migration to Vascularized Transplants. J Clin Invest (2013) 123:2663–71. doi: 10.1172/JCI66722
Keywords: immune tolerance, hematopoietic stem cell transplantation, solid organ transplantation, innate immunity, adaptive immunity
Citation: Slepicka PF, Yazdanifar M and Bertaina A (2021) Harnessing Mechanisms of Immune Tolerance to Improve Outcomes in Solid Organ Transplantation: A Review. Front. Immunol. 12:688460. doi: 10.3389/fimmu.2021.688460
Received: 30 March 2021; Accepted: 24 May 2021;
Published: 10 June 2021.
Edited by:
Ying-Jun Chang, Peking University People’s Hospital, ChinaReviewed by:
Qing Ge, Peking University, ChinaHuidong Guo, Peking University People’s Hospital, China
Georg Böhmig, Medical University of Vienna, Austria
Nataliya Prokopenko Buxbaum, University at Buffalo, United States
Copyright © 2021 Slepicka, Yazdanifar and Bertaina. This is an open-access article distributed under the terms of the Creative Commons Attribution License (CC BY). The use, distribution or reproduction in other forums is permitted, provided the original author(s) and the copyright owner(s) are credited and that the original publication in this journal is cited, in accordance with accepted academic practice. No use, distribution or reproduction is permitted which does not comply with these terms.
*Correspondence: Alice Bertaina, aliceb1@stanford.edu