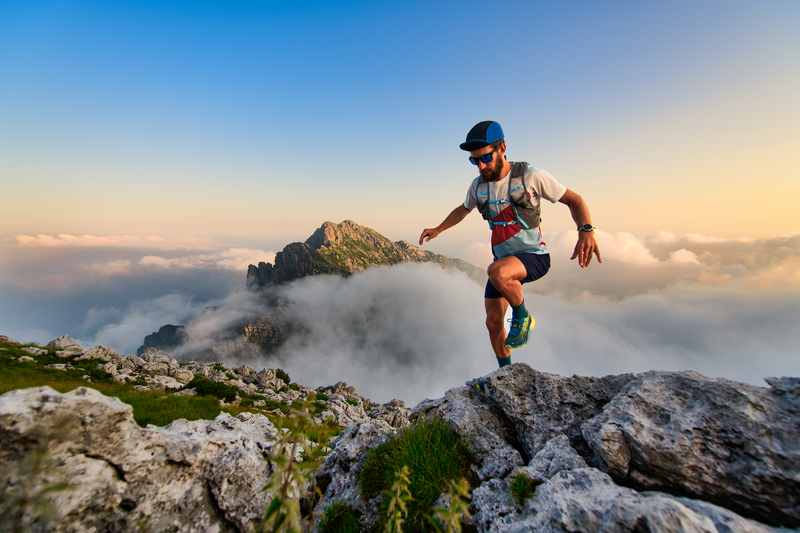
94% of researchers rate our articles as excellent or good
Learn more about the work of our research integrity team to safeguard the quality of each article we publish.
Find out more
REVIEW article
Front. Immunol. , 03 June 2021
Sec. Molecular Innate Immunity
Volume 12 - 2021 | https://doi.org/10.3389/fimmu.2021.684496
This article is part of the Research Topic Innate Immunity in Kidney Injury, Repair and Fibrosis View all 28 articles
Acute kidney injury (AKI) is a common and potential life-threatening disease in patients admitted to hospital, affecting 10%–15% of all hospitalizations and around 50% of patients in the intensive care unit. Severe, recurrent, and uncontrolled AKI may progress to chronic kidney disease or end-stage renal disease. AKI thus requires more efficient, specific therapies, rather than just supportive therapy. Mesenchymal stem cells (MSCs) are considered to be promising cells for cellular therapy because of their ease of harvesting, low immunogenicity, and ability to expand in vitro. Recent research indicated that the main therapeutic effects of MSCs were mediated by MSC-derived extracellular vesicles (MSC-EVs). Furthermore, compared with MSCs, MSC-EVs have lower immunogenicity, easier storage, no tumorigenesis, and the potential to be artificially modified. We reviewed the therapeutic mechanism of MSCs and MSC-EVs in AKI, and considered recent research on how to improve the efficacy of MSC-EVs in AKI. We also summarized and analyzed the potential and limitations of EVs for the treatment of AKI to provide ideas for future clinical trials and the clinical application of MSC-EVs in AKI.
Acute kidney injury (AKI) is a common and sometimes life-threatening disease in patients admitted to hospital, affecting 10%–15% of all hospitalizations and around 50% of patients in the intensive care unit (1). AKI is mainly caused by ischemia reperfusion injury (IRI), medication toxicity, and sepsis (1). Severe, recurrent, and uncontrolled AKI progresses to chronic kidney disease (CKD) or end-stage renal disease (2, 3). Treatments are currently limited to dialysis and kidney transplantation; however, these are restricted by the shortage of donor organs and high costs (4, 5), and there is thus a need to develop new and effective ways of treating AKI. The common pathological features of AKI include renal tubular epithelial cell (TEC) damage (6). TECs are injured as a result of an excessive inflammatory response, and undergo apoptosis via Bax/Bcl pathway activation (7, 8). In addition, the mechanistic target of rapamycin/mitogen-activated protein kinase (MAPK) signaling is consistently activated in TECs and the mitochondria are damaged, leading to maladaptive repair of the injured TECs, interstitial fibrosis, and the progression of AKI into CKD (9–12). Meanwhile, expression changes in various cytokines can be detected in AKI, including vascular endothelial growth factor (VEGF), hepatocyte growth factor (HGF), and insulin-like growth factor-1 (IGF-1) (13, 14). These mechanisms involving TECs are regarded as potential therapeutic targets in AKI.
Different types of stem cells have recently been transplanted to prevent kidney damage. Among these, mesenchymal stem cells (MSCs) are considered to be one of the most promising types of cells for cellular therapy because of their ease of harvesting, low immunogenicity, and ability to be stored and expanded in vitro (15, 16). Notably, numerous preclinical and clinical studies have confirmed the potential role of MSCs in kidney protection and repair (17–22). However, the mechanisms by which MSCs exert their therapeutic effects remain controversial. Researchers previously believed that MSCs replaced injured cells through differentiation after introduction into the body, but this view was challenged by the fact that MSCs disappeared from the injured kidney and other organs within 72 h after administration, suggesting that differentiation and replacement of damaged cells by stem cells is probably a rare and late event in AKI in vivo (22, 23).
Several studies have also demonstrated that MSCs depend on complex and powerful endocrine and paracrine functions, and secrete extracellular vesicles to promote the recovery of renal function in AKI (24, 25). Secretion of growth factors, regulation of the inflammatory response, promotion of mitosis and cell proliferation, anti-apoptosis and anti-inflammatory effects, the reduction of fibrosis, and the promotion of angiogenesis have been reported in multiple studies (17). However, the main therapeutic effects of MSCs appear to be mediated by MSC-EVs, rather than by the MSCs themselves (26). Compared with MSCs, MSC-EVs have lower immunogenicity, easier storage, no tumorigenesis, and the ability to be artificially modified (24, 27–29). Increasing numbers of researchers have accordingly recognized the curative potential of MSC-EVs for AKI, and extensive preclinical research has proven their effectiveness and safety in AKI (30).
In this paper, we review the possible therapeutic mechanisms of MSCs and MSC-EVs in AKI, and consider recent research aimed at improving the therapeutic efficacy of MSC-EVs in AKI. Finally, we summarize and analyze the potential and limitations of EVs for the treatment of AKI, to provide ideas for future clinical trials and clinical applications of MSC-EV-based therapy.
MSCs are the most widely used cells for AKI treatment and allograft protocols because they can be obtained from bone marrow and expanded in culture (17). MSCs originate from the mesoderm and have the ability to differentiate into mesenchymal and non-mesenchymal cell lines, including bone and cartilage (17).
Although MSCs are mainly obtained from bone marrow, they can also be isolated from other tissues such as liver, muscle, adipose tissue, and cord blood. Such cells are distinguished by their adherent growth in culture, expression of CD90, CD73, and CD105, and lack of expression of CD34, CD45, CD19, CD11a, and human leukocyte antigen-DR (18).
Previous studies indicated that the therapeutic effect of MSCs was largely dependent on their homing ability to injured organs (31). MSCs rely on their homing ability to localize in damaged tissues. In addition to their anti-inflammatory and vascular-support effects, the homing ability of MSCs supplements their paracrine function, and is involved in protecting microvessel density (32). Transplanted MSCs detect signals from injured kidney cells and are chemoattracted to the damaged site (33). During AKI, endothelial cells express high levels of tumor necrosis factor (TNF) and interleukins (ILs), which can up-regulate the β subunit of very late antigen-4 and vascular adhesion molecule 1 to mediate the effect of bone marrow MSCs on endothelial cell adhesion (33). CXC motif chemokine receptor 4 (CXCR4) is a specific receptor for chemokine stromal cell-derived factor-1 (CXCL12), and CXCR4 cells are responsible for the renal repair function in AKI (34). Location of the damaged tissue by MSCs can be mediated by stromal cell-derived factor-1, which is robustly up-regulated during AKI and mobilizes CXCR4 cell homing to the injured kidney tissue (35).
MSCs can exert significant therapeutic effects in terms of repairing the injured kidney and improving renal function after AKI, and are distributed to the spleen, lung, lymph nodes, and kidney following intravenous administration (36). However, intravenously administered MSCs disappear within 72 h, although some MSCs can remain around the glomerulus and perirenal capillaries following renal artery injection (37), suggesting that MSCs are unlikely to treat AKI through differentiation and the replacement of damaged cells. Meanwhile, MSCs located in the lung were also shown to affect AKI through a secretory function (38), and the hypothesis that the therapeutic effects of MSCs are mainly caused by a secretory mechanism appears to be more credible. Indeed, MSCs are involved in immune regulation, anti-inflammation, anti-apoptosis, and angiogenesis promotion in the local microenvironment in injured tissue, unrelated to their differentiation ability (39). Animal models of AKI are accompanied by significant changes in cytokines, including VEGF, HGF, epidermal growth factor (EGF), IGF-1, and transforming growth factor-β (TGF-β), which participate in endothelial cell apoptosis during AKI (40). These growth factors are also widely recognized as essential components in cell regeneration and kidney repair (41). MSCs secrete molecules directly or secrete EVs that carry molecules such as VEGF, HGF, IGF-1, IL-10, fibroblast growth factor (FGF), and TGF-α, and down-regulate the inducibility of related proinflammatory molecules (such as IL-1b and TNF-α), thus, having anti-inflammatory and anti-apoptosis effects and promoting kidney repair (42). Expression levels of related cytokines have been shown to be up-regulated during the treatment of AKI with MSCs, while kidney tissue inflammation was reduced, indicating the relationship between cytokines and kidney repair in MSC-based therapy; however, more studies are needed to identify the specific mechanism involved (43).
Further research into MSC therapy of AKI has shown the presence of MSC-EVs in AKI models treated with MSCs (44). MSCs can also release proteins (43), RNA (45), and mitochondria (46) into injured kidney tissue via EVs, in addition to their secretory function (30) (Figure 1). Various cytokines and their mRNAs are found in MSC-EVs, and there is gradual recognition that the therapeutic effects of MSCs are mainly mediated by MSC-EVs (43, 47). The effects exerted by MSC-EVs are similar to those reported following MSC administration in previous studies (48). EVs derived from cells such as MSCs act as messengers mediating cell-to-cell communication by carrying a train of biologically active molecules, which is regarded as a critical mechanism in AKI (24, 45). As intercellular messengers, EVs implement the therapeutic effects of MSCs, including regulating the damaged local microenvironment, regulating gene expression in injured kidney cells, improving the survival of damaged cells, resisting apoptosis, regulating inflammation, and reducing mitochondrial damage (49). In MSC-EV-based therapy of AKI, EVs can locate to the injured kidney tissue spontaneously after administration (50). Importantly, this effect is specific to MSC-EVs, involving the EV adhesion molecules CD44 and CD29, while EVs obtained from fibroblasts are ineffective (51). EVs, as endocytic vesicles, are bound to the membrane and are released by eukaryotic cells in an evolutionarily conserved manner, enabling cell-to-cell communication (52). MSC-EVs are generated by the paracrine and secretory functions of MSCs, carrying proteins, lipids, and nucleic acids into injured tissues (53). EVs can also deliver mRNAs and microRNAs (miRNAs) via endocytosis to regulate target cells at the transcriptional level (Figure 2) (45). As an intercellular messenger, EVs are responsible for regulating the damaged local microenvironment, regulating gene expression in AKI cells, improving the survival of damaged cells, resisting apoptosis and inflammation, and reducing mitochondrial damage (11, 12, 54).
Figure 1 MSC-EVs mediate transportation of biological modules to injured cells in AKI. Created with BioRender.com.
Figure 2 MSC-EVs carry various contents to exert therapeutic effects in AKI. Created with BioRender.com.
To identify the candidate therapeutic factors in EVs, Eirin et al. used proteomics to characterize the protein content of MSC-EVs derived from porcine MSCs from abdominal fat (42). The expression characteristics of MSC and EV markers detected by liquid chromatography-tandem mass spectrometry proteomics analysis showed that proteins enriched in MSC-EVs were related to a wide range of biological functions, including angiogenesis, blood coagulation, apoptosis, extracellular matrix remodeling, and inflammation regulation (43). Thus, EVs include selectively enriched protein cargo with specific biological characteristics, and these proteins are used for cell-to-cell communication to promote tissue repair. For example, MSC-derived EVs directly transferred IGF-1 receptors to renal tubular cells (13), accelerating kidney repair after AKI and reducing AKI by enhancing the activation of the Nrf2/ARE pathway to exert antioxidant effects (46, 55). Human adipose-derived MSCs (AD-MSCs) activated SOX9 in renal tubules and prevented the transformation of TGF-β1 into the pro-fibrotic phenotype induced by EV shuttling, resulting in anti-fibrotic effects (35, 56, 57).
Cytokines delivered by MSC-EVs and paracrine factors such as HGF, TGF, IGF-1, VEGF, and FGF-2 can regulate inflammation, resist apoptosis, promote damaged cell repair, and prevent fibrosis in AKI (36).
MSC-EV-based treatment of AKI involves the transport of various cytokines, including IGF-1. MSC-EVs secrete IGF-1 receptor mRNA directly to renal TECs, as well as directly secreting IGF-1 and carrying IGF-1 receptors to promote kidney repair in AKI (13). Several studies have indicated that this occurs via IGF-1-induced activation of the Akt survival pathway (38). AKI is associated with increased expression of the pro-apoptotic protein Bcl-2-associated X protein (Bax) and decreased expression of anti-apoptotic Bcl-2 (58). EVs can be integrated into injured renal TECs to promote the recovery of renal function and morphology. The beneficial effect of MSCs on renal tissue was related to the inhibition of renal tubular oxidative damage in a cisplatin-induced AKI model, manifested by the expression of nitrotyrosine and induction of Akt phosphorylation (59). IGF-1 activates Akt, as a kinase located on the phosphoinositide-3 kinase (PI3K) signaling pathway with an important regulatory role, through phosphorylation modification to inhibit Bax to resist apoptosis and up-regulate the Bcl-2 survival pathway, and to block mitochondrial caspase-9 to reduce inflammation and resist apoptosis (60). At the same time, IGF-1 activates the MAPK pathway to promote cell proliferation (13, 61). Morigi et al. confirmed that MSC treatment significantly increased phospho-Akt and activated downstream targets for cell proliferation and cell survival in a cisplatin-induced AKI model (56). In an in vitro model, proinflammatory factors such as TNF-α and IL-1b significantly reduced proximal tubular cell injury following treatment with MSCs and cisplatin (56). IGF-1 has demonstrated several biological effects in mouse kidney tissues treated with bone marrow MSCs, including promoting cell proliferation, changing the hemodynamics, and reducing apoptosis (13). In the same experimental model, Imberti et al. tested the effects of cord blood MSCs, which can improve kidney function and enhance the protective effect in AKI animal models. As a mitogenic and pro-survival factor, IGF-1 helps recruit self-derived progenitor cells or stem cells, thus, promoting the regeneration process in the kidney (62).
In addition to IGF-1, other growth factors transported by MSC-EVs also protect the microenvironment of the injured site and damaged cells. FGF-2 affects AKI via its anti-fibrotic effect, and knockout of FGF-2 blocked the repair process and induced a fibrotic response (63). TGF-β binds to the TGF receptor and activates downstream Smad and non-Smad pathways to perform a variety of biological effects in AKI (64). Acting via TGF-β-dependent macrophages, it can prevent ischemic kidney injury and tubular interstitial fibrosis (56, 60, 65). Up-regulation of VEGF receptor-2 can also be observed in kidney tissue during the ischemic AKI response, suggesting that VEGF may also be a potential therapeutic target (66–68). VEGF stabilized microvessel density to protect the renal microvascular structure and promote renal recovery through mitogenic and anti-apoptotic mechanisms (32). HGF can reduce kidney damage by promoting cell proliferation, anti-inflammation, and anti-apoptosis. HGF also promoted kidney repair and the proliferation of kidney cells via tyrosine phosphorylation of the c-met receptor in kidney injury induced by IRI and glycerol (13). Moreover, HGF gene therapy and HGF-modified MSCs had a significant therapeutic effect in AKI in an anti-apoptotic manner (13, 69, 70).
MSC-EVs can be internalized into renal TECs to treat AKI at the translational level. Recent studies showed that mRNAs for factors such as IL-10, IGF-1R, HGF, DNA-directed RNA polymerases I, II, and III subunit RPABC1, and VEGF could be loaded into EVs and transported to the target cells to exert translational effects, including anti-inflammation, anti-fibrosis, and anti-apoptosis effects, promoting proliferation, improving renal function, and reducing kidney injury (43, 71). Notably, the efficacy of MSC-EVs was partly mediated by miRNAs loaded in EVs, which directly regulated transcription and translation (50). EV-mediated miRNAs have also been shown to play a significant role in AKI (72). For example, miR-148b-3p and miR-548c-5p promoted cell viability (73, 74), miR-199a-3p reduced AKI via anti-apoptotic effects (75), miR-30 stabilized mitochondria, improved renal function, and exerted anti-apoptotic effects (76), and miRNA let-7a-5p reduced cell morbidity and improved cell survival (77, 78). Other miRNAs are regulated and actively participate in the regeneration process involving miR-21 in MSC-EV therapy of AKI, possibly related to renal tubular epithelium repair and internal cell reprogramming (79). EVs can also stabilize mitochondria through miRNAs, especially miRNA-200a-3p, which shuttles to TECs via MSC-EVs and targets the Keap1-Nrf2 signaling pathway to normalize mitochondrial membrane potential by reducing mitochondrial fragmentation, increasing the number of mitochondrial DNA copies, and protecting mitochondrial function in TECs during kidney repair (55, 71).
Mitochondria are considered to play an integral role in AKI development (80), suggesting the possibility that mitochondria might be transferred horizontally into kidney cells to reprogram cell metabolism and promote kidney recovery. Mitochondrial DNA or mitochondria can be transported directly into the damaged site by EVs, thus reducing kidney damage (81). Spees et al. first showed in 2006 that MSCs could serve as mitochondrial donors in cell survival (82). Actively transferring healthy mitochondria from MSCs can restore aerobic metabolism and protect cells from being eliminated (83). Plotnikov et al. subsequently confirmed the transport of mitochondria from MSCs to renal tubular cells in normal in vitro culture medium (84). In addition to renal tubular cells, vascular endothelial cells are also damaged during AKI. The delivery of mitochondria from MSC-EVs reduced apoptosis and increased cell viability, and restored the normal balance between aerobic respiration and glycolysis, indicating the re-establishment of aerobic respiration (12, 81). EVs derived from MSCs have previously demonstrated huge therapeutic potential in AKI via transporting proteins and RNAs with biological activity, and by conveying mitochondria and their DNA directly, suggesting that modifying the contents of MSC-EVs to affect specific signaling pathways may represent a promising therapeutic approach (28, 85).
MSCs-EVs have shown strong immune regulation in AKI treatment (Figure 3) and have demonstrated significant regulatory effects in a variety of immune cells, including inhibiting the transendothelial migration and chemotaxis of neutrophils, promoting macrophage M2 type polarization, inhibiting T cell activation, and inhibiting IFN-γ secretion (86–90). These processes were mainly mediated by TNF-α-stimulated gene 6 (TSG-6), which regulates inflammation with multiple functions (91–93). Apart from TSG-6, MSC-EVs also depend on IL-6, IL-10, prostaglandin E2, HGF, and indoleamine2,3-dioxygenase to regulate the immune microenvironment (94, 95), secreting miRNAs involving miR-155 regulate inflammation on the extracellular environment interact with dendritic cells to regulate endotoxin-induced inflammation (96–99). In addition, MSC-derived signals mediated by EVs can inhibit the proliferation of natural killer cells, reduce the activity of B lymphocytes, and secrete IL-17 to promote T cell transformation into Treg cells (86, 100).
Figure 3 MSC-EVs mediate immune regulation in AKI. Created with BioRender.com.
As noted above, AKI is usually associated with disorders and abnormal activation of the immune system, which can in turn be affected by treatment with MSCs and their EVs, suggesting that utilizing and modifying EVs to regulate the immunomicroenvironment might be an efficient and effective therapeutic approach for AKI (100–106). Although some researchers have reported that MSCs and MSC-EVs can affect the immune function and immune microenvironment in AKI both in vitro and in vivo, the findings have been inconsistent (107). Unfortunately, the specific mechanism by which MSC-EVs act as immune mediators remains elusive, and further research into the therapeutic mechanisms of EVs in AKI is warranted.
Although many preclinical studies have shown the effectiveness of MSCs and MSC-EVs in AKI, few clinical trials have utilized MSC-EVs for the clinical treatment of patients (49, 108, 109). Compared with MSCs, MSC-EVs generate a reduced inflammatory response with lower immunogenicity after administration, with beneficial effects in terms of administration dose and frequency, as well as reducing stem cell-associated risks such as cytokine release syndrome, ectopic tissue caused by poor differentiation, and tumorigenesis (31, 48, 110–113). In a comparative study, MSC-EVs were at least as effective as MSCs, indicating that many of the therapeutic effects of MSCs could be attributed to EVs (114, 115). In addition, MSCs may have more efficient homing ability than EVs (116, 117).
Preclinical studies in vitro and in vivo showed that EVs may have advantages over MSCs and may thus have great potential for future stem cell therapies (118, 119). First, MSC-EVs are highly stable and suitable for long-term storage without the need to add potentially toxic cryopreservatives (120, 121). Second, MSC-EVs can transfer functional proteins and miRNAs directly to recipient cells, inducing stronger signal transmission via cell-to-cell communication (45). Third, MSC-EVs have a lower risk of a rejection reaction, and an immune response after allogeneic application is rare (72). In addition, MSC-EVs avoid the potential tumorigenicity of MSCs, with no evidence of carcinogenic potential to date and no signs of unwanted differentiation (24, 28, 110). In contrast, MSCs have the possibility of tumorigenesis and poor differentiation (122). One study reported that MSC-EVs with anti-tumor activity inhibited tumor growth both in vivo and in vitro (123).
EVs have important unique characteristics. MSC-EVs may have a weaker homing ability than MSCs, which could partly reduce the accuracy of EV-based therapy; however, EVs are safe, with few adverse reactions (24, 28). EVs also show plasticity, and their contents can be modified artificially to improve and enhance not only their homing ability, but also their therapeutic effects (28, 29, 85). However, robust evidence is still lacking, and more research involving animal models and clinical studies is needed.
When administered via peripheral intravenous injection, most MSCs or MSC-EVs distribute to the lung, spleen, or celiac lymph nodes, thus, reducing their therapeutic efficacy (36). Moreover, the homing ability of EVs is lower than that of MSCs. However, a recent study showed that renal artery administration could transport more EVs and generate better therapeutic effects to injured kidney tissue with greater precision compared with other administration routes (30, 50, 124). Renal artery puncture in clinical patients may be performed under ultrasound image guidance (124). However, although EV injection via the renal artery provides a possible approach, this administration route is more difficult and is associated with ethical concerns in clinical practice (49, 114).
Bruno et al. utilized MSC-derived microvesicles, a kind of EVs, in lethal cisplatin-induced AKI and showed that increased administration times improved the therapeutic effects due to anti-apoptosis in AKI. The single administration of EVs ameliorated renal function and morphology and improved survival, but had no effect on chronic tubular injury and persistent increases in blood urea nitrogen (BUN) and creatinine. They also found that using multiple injections of EVs significantly reduced the mortality of mice, and mice surviving at day 21 showed normal histology and renal function (125).
Recent meta-analyses investigating the effects of administration and cell source on the therapeutic effects of EVs have indicated the importance of these factors in clinical research and applications. A meta-analysis using serum creatinine (Scr) as an indicator of efficacy compared the timing of administration in various studies (between 1 h and 3 days after the occurrence of AKI), and showed a better treatment effect following administration of MSC-EVs within 1 h after the occurrence of AKI, suggesting that they should be administered as early as possible (114). Current research mainly focuses on EVs secreted by MSCs derived from adipose tissue, bone marrow, and cord blood. However, the source tissue also has an impact on MSC-EVs (47, 126). For example, compared with cord blood-derived MSCs, signals mediated by EVs derived from bone marrow MSCs had greater effects on bone growth and differentiation (49, 114). In addition, adipose-derived MSCs had similar immune regulation effects to bone marrow-derived MSCs (17). The EV source should be selected flexibly according to the type of kidney injury and treatment needs. Meanwhile, because MSC from different sources have different characteristics, the EVs secreted by them will also differ. The therapeutic effects of EVs from other sources of MSCs in AKI are still unclear, and there is much need for further research. Bone marrow-derived MSCs may be more likely to express specific proteins via lentiviral expression vector transduction, such as angiogenin-1, IGF-1, and Akt, thus, influencing the restructuring and repair of injured tissue (13). This research could indicate the ability to utilize lentiviral vectors to modify MSCs to produce EVs with specific efficacies (127).
Several studies have focused on improving MSC-EVs in a variety of areas (128) (Table 1). These studies have mainly involved improving the technology for the isolation of EVs, investigating potential administration routes, the use of pulsed focused ultrasound (pFUS), preconditioning EVs, and inducing the overexpression of EVs by lentiviral vector transduction.
EVs are traditionally separated by ultracentrifugation, but this method has major limitations, including the co-precipitation of EVs with contaminants including protein aggregates, loss of EV function due to aggregation or distortion during the isolation process, and functional inhibition of EVs by the media used for density gradient ultracentrifugation (129). AKI is common in clinical practice, and improved large-scale production of EVs would be needed to satisfy the requirements if EVs were applied for the clinical treatment of these patients (1). Large-scale isolation of single-batch EVs by ultracentrifugation is also restricted by the limited instrument capacity (130). Lee et al. showed that it was possible to isolate adipose tissue-derived MSC-EVs stably and reproducibly on a large scale without loss of function using tangential flow filtration (131), with successful life-preserving effects in a cisplatin-induced lethal rat model of AKI. Other studies have indicated that the production of EVs by three-dimensional (3D) culture of MSCs could improve the efficacy and increase yield. Cao et al. produced EVs in 3D culture and showed that, compared with conventional 2D culture, the 3D culture system increased the total yield of EVs 19.4-fold, thus, indicating that the 3D culture system had a higher EV-collection efficiency. Surprisingly, EVs obtained by 3D culture were taken up more efficiently by renal TECs, showing better anti-inflammatory effects and increasing the survival rate of TECs in vitro (130).
pFUS was shown to alter the kidney microenvironment to enhance homing of subsequently infused MSCs. Mujib et al. investigated if the combined use of pFUS with MSC-EVs could improve the therapeutic effect by improving the homing ability of EVs in AKI (132). Surprisingly, although pFUS did not up-regulate local cytokine expression or improve bone marrow MSC homing, it did enhance the therapeutic efficacy of MSC-EVs in AKI. Further analysis showed that this effect was related to the up-regulation of heat shock protein (HSP) 20/HSP40 and subsequent PI3K/Akt signaling. This indicated that pFUS had independent as well as synergistic therapeutic effects in the context of AKI, and is thus, a promising affiliated method for MSC-EV therapy (132). Further studies of the combined use of MSC-EVs and pFUS showed that pFUS affected HSP70-mediated NLRP3 inflammasome suppression to improve the anti-inflammatory and regenerative effects (133). In addition, Mujib et al. also showed that pFUS upregulated the proliferative signaling (MAPK/extracellular signal-regulated kinase, PI3K/Akt) and regenerative pathways (endothelial nitric oxide synthase, sirtuin 3) to suppress inflammation in AKI (134).
The low stability and retention of MSC-EVs have partly limited their therapeutic efficacy, and methods involving novel biological materials such as arginine-glycine-aspartate (RGD) peptides and collagen matrix have been investigated with the aim of improving these features. Zhang et al. developed an RGD peptide scaffold to increase EV integrin-mediated loading, and found that RGD hydrogels facilitated MSC-EVs containing miRNA let-7a-5p, which improved their repair potential in AKI (78). Liu et al. isolated EVs from human placental mesenchymal stem cells and wrapped them in a collagen matrix, and Gaussia luciferase imaging confirmed that the collagen matrix effectively encapsulated the EVs and augmented their efficacy in AKI by improving the stability and promoting the sustained release of EVs (135). Biological modules can be used to cover EVs and interact with the microenvironment in AKI to improve the positive effects of MSC-EV-based therapy (78, 135, 136). However, few studies have investigated the mechanisms involved in the interaction between the injured tissue microenvironment and biological modules covering EVs. This warrants further investigation to guide clinical trials aimed at identifying the most efficient artificial biological modules to improve the therapeutic effects of MSC-EVs.
Scientists have also tried to improve the effects of MSC-EVs in AKI by preconditioning them with medications to prevent further damage deterioration and help renal recovery. Alzahrani et al. tested MSC-EVs preconditioned with melatonin in AKI induced by IRI with bilateral renal artery clipping, and showed that melatonin-preconditioned MSC-EVs performed better than untreated EVs in terms of alleviating kidney damage and inflammation, promoting regeneration, angiogenesis, and anti-oxidation, and inhibiting oxidative stress (136). EVs can also be artificially modified to overexpress specific modules. Zhang et al. transduced MSCs with Oct-4 via a lentiviral vector to produce Oct-4-overexpressing MSCs, which significantly reduced attenuated apoptosis, Scr and BUN levels, promoted renal TEC proliferation, and rescued renal fibrosis in IRI-induced AKI (137).
Recent studies concentrating on improving the limitations of MSC-EV-based therapy showed that it was possible to safely modify MSC-EVs and enhance their therapeutic effects. As noted above, their homing ability and tissue stability limit the therapeutic effects of EVs, but these issues can be overcome by the use of collagen matrix and RGD peptides (78, 135). In addition to enhancing the effects of EVs, 3D culture and tangential flow filtration may also allow the large-scale clinical application of EVs (130, 131). However, MSC-EVs still face numerous challenges and limitations before they can be clinically applied in patients.
Various challenges still need to be overcome before MSC-EVs can be utilized in clinical treatments. First, there is significant heterogeneity between MSC-EVs in terms of size, leading to variations in the secreted components and functional characteristics of the EVs (15). Further studies are therefore needed to choose the specific size of EVs according to their cargo (138). Second, the isolation and storage methods of EVs may affect their therapeutic efficacy (139). Ensuring the quality of EVs is an important problem, and producers are supposed to confirm that the EVs are derived from cellular matrix, rather than being components from cells damaged during cryopreservation and mechanical failure (130, 131, 135). It is advisable to optimize and confirm the preservation conditions to maintain the efficacy of the EVs after defrosting (128). Importantly, the follow-up time in previous studies was only between 1 day and 2 weeks, which is inadequate for evaluating the long-term outcome and prognosis (114), and more clinical trials with long-term follow-up are therefore needed to provide more robust evidence. Catering for the demands of large-scale clinical applications and producing enough high-quality MSCs then become critical issues (99). In addition, even though EVs have demonstrated similar effects to MSCs, their homing ability is much weaker than that of MSCs, representing a limitation of MSC-EV-based therapy that needs improving. Zhang et al. recently used monocyte mimics to enhance the homing ability of MSC-EVs to the injured site in a myocardial IRI model, suggesting a possible approach for improving the homing ability of MSC-EVs for the therapy of AKI (140). Regarding monitoring the distribution of EVs, their physicochemical properties may be affected by some lipophilic markers, which could affect the observations (13), and new tracing markers will be needed to detect the distribution and effects of EVs in clinical practice. Increasing research is currently focused on investigating ways of modifying the cargo of MSC-EVs to improve their therapeutic efficiency (28, 141). Clinical trials are currently required to verify and approve the use of customized EVs and to assess the safety and tolerance of modified MSC-EVs.
MSC-EVs are responsible for the main therapeutic effects of MSCs in AKI, and demonstrate specific therapeutic effects and improve the efficacy of regenerative stem cell therapies. However, the lack of clinical trials means that MSC-EVs still face many challenges before they can be used for clinical treatment. Nonetheless, we believe that MSC-EVs will become an effective approach to overcome the current limitations of AKI treatment.
J-KL and CY drafted the manuscript. ZL, G-WT and YS conceived the proposal, revised the manuscript and provided funding support. J-CL, M-HL and D-LH collected literatures and revised the manuscript. ZL and G-WT helped the language editing and provided funding support. All authors contributed to the article and approved the submitted version.
This article was supported by grants from the Research Funds of Shanghai Municipal Health Commission (2019ZB0105), Natural Science Foundation of Shanghai (20ZR1411100), Program of Shanghai Academic/Technology Research Leader (20XD1421000), National Natural Science Foundation of China (82070085), Clinical Research Funds of Zhongshan Hospital (2020ZSLC38 and 2020ZSLC27), and Smart Medical Care of Zhongshan Hospital (2020ZHZS01).
The authors declare that the research was conducted in the absence of any commercial or financial relationships that could be construed as a potential conflict of interest.
The handling editor has declared past co-authorships with one of the authors (CY) in the time of review.
1. Ronco C, Bellomo R, Kellum JA. Acute Kidney Injury. Lancet (2019) 394:1949–64. doi: 10.1016/s0140-6736(19)32563-2
2. Hsu CY. Yes, Aki Truly Leads to Ckd. J Am Soc Nephrol (2012) 23:967–9. doi: 10.1681/asn.2012030222
3. Zhao L, Han F, Wang J, Chen J. Current Understanding of the Administration of Mesenchymal Stem Cells in Acute Kidney Injury to Chronic Kidney Disease Transition: A Review With a Focus on Preclinical Models. Stem Cell Res Ther (2019) 10:3855. doi: 10.1186/s13287-019-1507-3
4. Pickkers P, Mehta RL, Murray PT, Joannidis M, Molitoris BA, Kellum JA, et al. Effect of Human Recombinant Alkaline Phosphatase on 7-Day Creatinine Clearance in Patients With Sepsis-Associated Acute Kidney Injury: A Randomized Clinical Trial. Jama (2018) 320:1998–2009. doi: 10.1001/jama.2018.14283
5. Forni LG, Darmon M, Ostermann M, Oudemans-van Straaten HM, Pettilä V, Prowle JR, et al. Renal Recovery After Acute Kidney Injury. Intensive Care Med (2017) 43:855–66. doi: 10.1007/s00134-017-4809-x
6. Hu L, Yang C, Zhao T, Xu M, Tang Q, Yang B, et al. Erythropoietin Ameliorates Renal Ischemia and Reperfusion Injury Via Inhibiting Tubulointerstitial Inflammation. J Surg Res (2012) 176:260–6. doi: 10.1016/j.jss.2011.06.035
7. Xu Y, Li D, Wu J, Zhang M, Shao X, Xu L, et al. Farnesoid X Receptor Promotes Renal Ischaemia-Reperfusion Injury by Inducing Tubular Epithelial Cell Apoptosis. Cell Proliferation (2021), 54(4):e13005. doi: 10.1111/cpr.13005
8. Wang J, Zhu P, Li R, Ren J, Zhang Y, Zhou H. Bax Inhibitor 1 Preserves Mitochondrial Homeostasis in Acute Kidney Injury Through Promoting Mitochondrial Retention of Phb2. Theranostics (2020) 10:384–97. doi: 10.7150/thno.40098
9. Liu G, Sabatini D. Mtor at the Nexus of Nutrition, Growth, Ageing and Disease. Nature Reviews. Mol Cell Biol (2020) 21:183–203. doi: 10.1038/s41580-019-0199-y
10. Rostamzadeh D, Yousefi M, Haghshenas M, Ahmadi M, Dolati S, Babaloo Z. Mtor Signaling Pathway as a Master Regulator of Memory Cd8 T-Cells, th17, and Nk Cells Development and Their Functional Properties. J Cell Physiol (2019) 234:12353–68. doi: 10.1002/jcp.28042
11. Tang C, Livingston M, Liu Z, Dong Z. Autophagy in Kidney Homeostasis and Disease. Nature Reviews. Nephrology (2020) 16:489–508. doi: 10.1038/s41581-020-0309-2
12. Emma F, Montini G, Parikh SM, Salviati L. Mitochondrial Dysfunction in Inherited Renal Disease and Acute Kidney Injury. Nat Rev Nephrol (2016) 12:267–80. doi: 10.1038/nrneph.2015.214
13. Gao L, Zhong X, Jin J, Li J, Meng X. Potential Targeted Therapy and Diagnosis Based on Novel Insight Into Growth Factors, Receptors, and Downstream Effectors in Acute Kidney Injury and Acute Kidney Injury-Chronic Kidney Disease Progression. Signal Transduct Targeted Ther (2020) 5:95. doi: 10.1038/s41392-020-0106-1
14. Yang C, Liu J, Li L, Hu M, Long Y, Liu X, et al. Proteome Analysis of Renoprotection Mediated by a Novel Cyclic Helix B Peptide in Acute Kidney Injury. Sci Rep (2015) 5:180455. doi: 10.1038/srep18045
15. Yun C, Lee S. Potential and Therapeutic Efficacy of Cell-Based Therapy Using Mesenchymal Stem Cells for Acute/Chronic Kidney Disease. Int J Mol Sci (2019) 20(7):1619. doi: 10.3390/ijms20071619
16. Rota C, Morigi M, Cerullo D, Introna M, Colpani O, Corna D, et al. Therapeutic Potential of Stromal Cells of Non-Renal or Renal Origin in Experimental Chronic Kidney Disease. Stem Cell Res Ther (2018) 9:220. doi: 10.1186/s13287-018-0960-8
17. Gwam C, Mohammed N, Ma X. Stem Cell Secretome, Regeneration, and Clinical Translation: A Narrative Review. Ann Transl Med (2021) 9:70. doi: 10.21037/atm-20-5030
18. Tögel FE, Westenfelder C. Kidney Protection and Regeneration Following Acute Injury: Progress Through Stem Cell Therapy. Am J Kidney Dis (2012) 60:1012–22. doi: 10.1053/j.ajkd.2012.08.034
19. Abumaree MH, Abomaray FM, Alshabibi MA, AlAskar AS, Kalionis B. Immunomodulatory Properties of Human Placental Mesenchymal Stem/Stromal Cells. Placenta (2017) 59:87–95. doi: 10.1016/j.placenta.2017.04.003
20. Bochon B, Kozubska M, Surygała G, Witkowska A, Kuźniewicz R, Grzeszczak W, et al. Mesenchymal Stem Cells-Potential Applications in Kidney Diseases. Int J Mol Sci (2019) 20(10):2462. doi: 10.3390/ijms20102462
21. De Miguel MP, Fuentes-Julián S, Blázquez-Martínez A, Pascual CY, Aller MA, Arias J, et al. Immunosuppressive Properties of Mesenchymal Stem Cells: Advances and Applications. Curr Mol Med (2012) 12:574–915. doi: 10.2174/156652412800619950
22. Wang X, Yang C, Hu L, Wei Z, Tang Q, Chen B, et al. Tolerance Induction With Donor Hematopoietic Stem Cell Infusion in Kidney Transplantation: A Single-Center Experience in China With a 10-Year Follow-Up. Ann Transl Med (2020) 8:13785. doi: 10.21037/atm-20-2502a
23. Bianco P, Robey PG, Simmons PJ. Mesenchymal Stem Cells: Revisiting History, Concepts, and Assays. Cell Stem Cell (2008) 2:313–9. doi: 10.1016/j.stem.2008.03.002
24. Aghajani Nargesi A, Lerman L, Eirin A. Mesenchymal Stem Cell-Derived Extracellular Vesicles for Kidney Repair: Current Status and Looming Challenges. Stem Cell Res Ther (2017) 8:2735. doi: 10.1186/s13287-017-0727-7
25. Keating A. Mesenchymal Stromal Cells: New Directions. Cell Stem Cell (2012) 10:709–16. doi: 10.1016/j.stem.2012.05.015
26. Zhao Y, Zhu X, Zhang L, Ferguson CM, Song T, Jiang K, et al. Mesenchymal Stem/Stromal Cells and Their Extracellular Vesicle Progeny Decrease Injury in Poststenotic Swine Kidney Through Different Mechanisms. Stem Cells Dev (2020) 29:1190–200. doi: 10.1089/scd.2020.0030
27. Bei Y, Das S, Rodosthenous RS, Holvoet P, Vanhaverbeke M, Monteiro MC, et al. Extracellular Vesicles in Cardiovascular Theranostics. Theranostics (2017) 7:4168–82. doi: 10.7150/thno.21274
28. Lai R, Yeo R, Tan K, Lim S. Exosomes for Drug Delivery - a Novel Application for the Mesenchymal Stem Cell. Biotechnol Adv (2013) 31:543–1. doi: 10.1016/j.biotechadv.2012.08.008
29. Du W, Zhang K, Zhang S, Wang R, Nie Y, Tao H, et al. Enhanced Proangiogenic Potential of Mesenchymal Stem Cell-Derived Exosomes Stimulated by a Nitric Oxide Releasing Polymer. Biomaterials (2017) 133:70–81. doi: 10.1016/j.biomaterials.2017.04.030
30. Grange C, Tapparo M, Bruno S, Chatterjee D, Quesenberry PJ, Tetta C, et al. Biodistribution of Mesenchymal Stem Cell-Derived Extracellular Vesicles in a Model of Acute Kidney Injury Monitored by Optical Imaging. Int J Mol Med (2014) 33:1055–63. doi: 10.3892/ijmm.2014.1663
31. Zheng X, He X, Zhang L, Qin H, Lin X, Liu X, et al. Bone Marrow-Derived Excr4-Overexpressing MSCs Display Increased Homing to Intestine and Ameliorate Colitis-Associated Tumorigenesis in Mice. Gastroenterol Rep (2019) 7:127–38. doi: 10.1093/gastro/goy017
32. Yuan L, Wu MJ, Sun HY, Xiong J, Zhang Y, Liu CY, et al. Vegf-Modified Human Embryonic Mesenchymal Stem Cell Implantation Enhances Protection Against Cisplatin-Induced Acute Kidney Injury. Am J Physiol Renal Physiol (2011) 300:F207–18. doi: 10.1152/ajprenal.00073.2010
33. Hervás-Salcedo R, Fernández-García M, Hernando-Rodríguez M, Quintana-Bustamante O, Segovia J, Alvarez-Silva M, et al. Enhanced Anti-Inflammatory Effects of Mesenchymal Stromal Cells Mediated by the Transient Ectopic Expression of Cxcr4 and Il10. Stem Cell Res Ther (2021) 12:124. doi: 10.1186/s13287-021-02193-0
34. Singh P, Mohammad K, Pelus L. Cxcr4 Expression in the Bone Marrow Microenvironment Is Required for Hematopoietic Stem and Progenitor Cell Maintenance and Early Hematopoietic Regeneration After Myeloablation. Stem Cells (Dayton Ohio) (2020) 38(7):849–59. doi: 10.1002/stem.3174
35. Togel F, Westenfelder C. Role of SDF-1 as a Regulatory Chemokine in Renal Regeneration After Acute Kidney Injury. Kidney Int Suppl (2011) 1:87–9. doi: 10.1038/kisup.2011.20
36. Luo C, Zhang F, Zhang L, Geng Y, Li Q, Hong Q, et al. Mesenchymal Stem Cells Ameliorate Sepsis-Associated Acute Kidney Injury in Mice. Shock (Augusta Ga) (2014) 41:123–9. doi: 10.1097/shk.0000000000000080
37. Westenfelder C, Togel F. Protective Actions of Administered Mesenchymal Stem Cells in Acute Kidney Injury: Relevance to Clinical Trials. Kidney Int Suppl (2011) 1:103–6. doi: 10.1038/kisup.2011.24
38. Ullah I, Subbarao RB, Rho GJ. Human Mesenchymal Stem Cells - Current Trends and Future Prospective. Biosci Rep (2015) 35(2):e00191. doi: 10.1042/BSR20150025.
39. Morigi M, Imberti B, Zoja C, Corna D, Tomasoni S, Abbate M, et al. Mesenchymal Stem Cells are Renotropic, Helping to Repair the Kidney and Improve Function in Acute Renal Failure. J Am Soc Nephrol (2004) 15:1794–804. doi: 10.1097/01.asn.0000128974.07460.34
40. Little MH, Rae FK. Review Article: Potential Cellular Therapies for Renal Disease: Can We Translate Results From Animal Studies to the Human Condition? Nephrol (Carlton) (2009) 14:544–53. doi: 10.1111/j.1440-1797.2009.01144.x
41. Yang C, Zhao T, Lin M, Zhao Z, Hu L, Jia Y, et al. Helix B Surface Peptide Administered After Insult of Ischemia Reperfusion Improved Renal Function, Structure and Apoptosis Through Beta Common Receptor/Erythropoietin Receptor and pi3k/akt Pathway in a Murine Model. Exp Biol Med (Maywood) (2013) 238:111–9. doi: 10.1258/ebm.2012.012185
42. Magnasco A, Corselli M, Bertelli R, Ibatici A, Peresi M, Gaggero G, et al. Mesenchymal Stem Cells Protective Effect in Adriamycin Model of Nephropathy. Cell Transplant (2008) 17:1157–67. doi: 10.3727/096368908787236567
43. Eirin A, Zhu X, Puranik A, Woollard J, Tang H, Dasari S, et al. Comparative Proteomic Analysis of Extracellular Vesicles Isolated From Porcine Adipose Tissue-Derived Mesenchymal Stem/Stromal Cells. Sci Rep (2016) 6:36120. doi: 10.1038/srep36120
44. Phan J, Kumar P, Hao D, Gao K, Farmer D, Wang A. Engineering Mesenchymal Stem Cells to Improve Their Exosome Efficacy and Yield for Cell-Free Therapy. J Extracell Vesicles (2018) 7:1522236. doi: 10.1080/20013078.2018.1522236
45. O’Brien K, Breyne K, Ughetto S, Laurent L, Breakefield X. RNA Delivery by Extracellular Vesicles in Mammalian Cells and Its Applications. Nature Reviews. Mol Cell Biol (2020) 21:585–606. doi: 10.1038/s41580-020-0251-y
46. Cao H, Cheng Y, Gao H, Zhuang J, Zhang W, Bian Q, et al. In Vivo Tracking of Mesenchymal Stem Cell-Derived Extracellular Vesicles Improving Mitochondrial Function in Renal Ischemia-Reperfusion Injury. ACS Nano (2020) 14:4014–26. doi: 10.1021/acsnano.9b08207
47. Heo JS, Choi Y, Kim HS, Kim HO. Comparison of Molecular Profiles of Human Mesenchymal Stem Cells Derived From Bone Marrow, Umbilical Cord Blood, Placenta and Adipose Tissue. Int J Mol Med (2016) 37:115–25. doi: 10.3892/ijmm.2015.2413
48. Anjos-Afonso F, Siapati EK, Bonnet D. In Vivo Contribution of Murine Mesenchymal Stem Cells Into Multiple Cell-Types Under Minimal Damage Conditions. J Cell Sci (2004) 117:5655–64. doi: 10.1242/jcs.01488
49. Liu C, Wang J, Hu J, Fu B, Mao Z, Zhang H, et al. Extracellular Vesicles for Acute Kidney Injury in Preclinical Rodent Models: A Meta-Analysis. Stem Cell Res Ther (2020) 11:115. doi: 10.1186/s13287-019-1530-4
50. Yin K, Wang S, Zhao RC. Exosomes From Mesenchymal Stem/Stromal Cells: A New Therapeutic Paradigm. Biomark Res (2019) 7:85. doi: 10.1186/s40364-019-0159-x
51. Munshi A, Mehic J, Creskey M, Gobin J, Gao J, Rigg E, et al. A Comprehensive Proteomics Profiling Identifies Nrp1 as a Novel Identity Marker of Human Bone Marrow Mesenchymal Stromal Cell-Derived Small Extracellular Vesicles. Stem Cell Res Ther (2019) 10:401. doi: 10.1186/s13287-019-1516-2
52. Baer PC, Geiger H. Mesenchymal Stem Cell Interactions With Growth Factors on Kidney Repair. Curr Opin Nephrol Hypertens (2010) 19:1–6. doi: 10.1097/MNH.0b013e328333062c
53. van Niel G, D’Angelo G, Raposo G. Shedding Light on the Cell Biology of Extracellular Vesicles. Nat Rev Mol Cell Biol (2018) 19:213–8. doi: 10.1038/nrm.2017.125
54. Bellomo R. Acute Kidney Injury—A Decade of Progress. Nat Rev Nephrol (2015) 11:636–37. doi: 10.1038/nrneph.2015.147
55. Nezu M, Souma T, Yu L, Suzuki T, Saigusa D, Ito S, et al. Transcription Factor Nrf2 Hyperactivation in Early-Phase Renal Ischemia-Reperfusion Injury Prevents Tubular Damage Progression. Kidney Int (2017) 91:387–401. doi: 10.1016/j.kint.2016.08.023
56. Morigi M, Rota C, Montemurro T, Montelatici E, Lo Cicero V, Imberti B, et al. Life-Sparing Effect of Human Cord Blood-Mesenchymal Stem Cells in Experimental Acute Kidney Injury. Stem Cells (2010) 28:513–22. doi: 10.1002/stem.293
57. Zhang K, Chen S, Sun H, Wang L, Li H, Zhao J, et al. In Vivo Two-Photon Microscopy Reveals the Contribution of sox9(+) Cell to Kidney Regeneration in a Mouse Model With Extracellular Vesicle Treatment. J Biol Chem (2020) 295:12203–13. doi: 10.1074/jbc.RA120.012732
58. Villanueva S, Céspedes C, Vio CP. Ischemic Acute Renal Failure Induces the Expression of a Wide Range of Nephrogenic Proteins. Am J Physiol Regul Integr Comp Physiol (2006) 290:R861–70. doi: 10.1152/ajpregu.00384.2005
59. Gnecchi M, He H, Liang OD, Melo LG, Morello F, Mu H, et al. Paracrine Action Accounts for Marked Protection of Ischemic Heart by Akt-Modified Mesenchymal Stem Cells. Nat Med (2005) 11:367–8. doi: 10.1038/nm0405-367
60. Gobé G, Zhang XJ, Willgoss DA, Schoch E, Hogg NA, Endre ZH. Relationship Between Expression of Bcl-2 Genes and Growth Factors in Ischemic Acute Renal Failure in the Rat. J Am Soc Nephrol (2000) 11:454–67. doi: 10.1681/ASN.V113454
61. Xinaris C, Morigi M, Benedetti V, Imberti B, Fabricio AS, Squarcina E, et al. A Novel Strategy to Enhance Mesenchymal Stem Cell Migration Capacity and Promote Tissue Repair in an Injury Specific Fashion. Cell Transplant (2013) 22:423–36. doi: 10.3727/096368912x653246
62. Imberti B, Morigi M, Benigni A. Potential of Mesenchymal Stem Cells in the Repair of Tubular Injury. Kidney Int Suppl (2011) 1:90–3. doi: 10.1038/kisup.2011.21
63. Iwasaki M, Adachi Y, Minamino K, Suzuki Y, Zhang Y, Okigaki M, et al. Mobilization of Bone Marrow Cells by G-Csf Rescues Mice From Cisplatin-Induced Renal Failure, and M-Csf Enhances the Effects of G-Csf. J Am Soc Nephrol JASN (2005) 16:658–65. doi: 10.1681/asn.2004010067
64. Chen H, Lin K, Wallace C, Chen Y, Yang C, Leu S, et al. Additional Benefit of Combined Therapy With Melatonin and Apoptotic Adipose-Derived Mesenchymal Stem Cell Against Sepsis-Induced Kidney Injury. J Pineal Res (2014) 57:16–32. doi: 10.1111/jpi.12140
65. Tögel F, Hu Z, Weiss K, Isaac J, Lange C, Westenfelder C. Administered Mesenchymal Stem Cells Protect Against Ischemic Acute Renal Failure Through Differentiation-Independent Mechanisms. Am J Physiol Renal Physiol (2005) 289:F31–42. doi: 10.1152/ajprenal.00007.2005
66. Mori da Cunha MG, Zia S, Beckmann DV, Carlon MS, Arcolino FO, Albersen M, et al. Vascular Endothelial Growth Factor Up-Regulation in Human Amniotic Fluid Stem Cell Enhances Nephroprotection After Ischemia-Reperfusion Injury in the Rat. Crit Care Med (2017) 45:e86–e96. doi: 10.1097/ccm.0000000000002020
67. Jiang M, Li G, Liu J, Liu L, Wu B, Huang W, et al. Nestin(+) Kidney Resident Mesenchymal Stem Cells for the Treatment of Acute Kidney Ischemia Injury. Biomaterials (2015) 50:56–66. doi: 10.1016/j.biomaterials.2015.01.029
68. Kinnaird T, Stabile E, Burnett MS, Lee CW, Barr S, Fuchs S, et al. Marrow-Derived Stromal Cells Express Genes Encoding a Broad Spectrum of Arteriogenic Cytokines and Promote In Vitro and In Vivo Arteriogenesis Through Paracrine Mechanisms. Circ Res (2004) 94:678–85. doi: 10.1161/01.Res.0000118601.37875.Ac
69. Du T, Zou X, Cheng J, Wu S, Zhong L, Ju G, et al. Human Wharton’s Jelly-Derived Mesenchymal Stromal Cells Reduce Renal Fibrosis Through Induction of Native and Foreign Hepatocyte Growth Factor Synthesis in Injured Tubular Epithelial Cells. Stem Cell Res Ther (2013) 4:59. doi: 10.1186/scrt215
70. Xing L, Cui R, Peng L, Ma J, Chen X, Xie R, et al. Mesenchymal Stem Cells, Not Conditioned Medium, Contribute to Kidney Repair After Ischemia-Reperfusion Injury. Stem Cell Res Ther (2014) 5:1015. doi: 10.1186/scrt489
71. Toh W, Lai R, Hui J, Lim S. MSC Exosome as a Cell-Free Msc Therapy for Cartilage Regeneration: Implications for Osteoarthritis Treatment. Semin Cell Dev Biol (2017) 67:56–64. doi: 10.1016/j.semcdb.2016.11.008
72. Sun X, Meng H, Wan W, Xie M, Wen C. Application Potential of Stem/Progenitor Cell-Derived Extracellular Vesicles in Renal Diseases. Stem Cell Res Ther (2019) 10:8. doi: 10.1186/s13287-018-1097-5
73. Zhang H, Ye Q, Du Z, Huang M, Zhang M, Tan H. Mir-148b-3p Inhibits Renal Carcinoma Cell Growth and Pro-Angiogenic Phenotype of Endothelial Cell Potentially by Modulating Fgf2. Biomed Pharmacother (2018) 107:359–67. doi: 10.1016/j.biopha.2018.07.054
74. Christofides A, Papagregoriou G, Dweep H, Makrides N, Gretz N, Felekkis K, et al. Evidence for mir-548c-5p Regulation of Foxc2 Transcription Through a Distal Genomic Target Site in Human Podocytes. Cell Mol Life Sci CMLS (2020) 77:2441–59. doi: 10.1007/s00018-019-03294-z
75. Zhu G, Pei L, Lin F, Yin H, Li X, He W, et al. Exosomes From Human-Bone-Marrow-Derived Mesenchymal Stem Cells Protect Against Renal Ischemia/Reperfusion Injury Via Transferring Mir-199a-3p. J Cell Physiol (2019) 234:23736–49. doi: 10.1002/jcp.28941
76. Cao G, Li S, Shi H, Yin P, Chen J, Li H, et al. Schisandrin B Attenuates Renal Fibrosis Via mir-30e-Mediated Inhibition of EMT. Toxicol Appl Pharmacol (2019) 385:114769. doi: 10.1016/j.taap.2019.114769
77. Ragni E, De Luca P, Perucca Orfei C, Colombini A, Viganò M, Lugano G, et al. Insights Into Inflammatory Priming of Adipose-Derived Mesenchymal Stem Cells: Validation of Extracellular Vesicles-Embedded MiRNA Reference Genes as a Crucial Step for Donor Selection. Cells (2019) 8(4):369. doi: 10.3390/cells8040369
78. Zhang C, Shang Y, Chen X, Midgley A, Wang Z, Zhu D, et al. Supramolecular Nanofibers Containing Arginine-Glycine-Aspartate (Rgd) Peptides Boost Therapeutic Efficacy of Extracellular Vesicles in Kidney Repair. ACS Nano (2020) 14:12133–47. doi: 10.1021/acsnano.0c05681
79. Baglio S, Rooijers K, Koppers-Lalic D, Verweij F, Pérez Lanzón M, Zini N, et al. Human Bone Marrow- and Adipose-Mesenchymal Stem Cells Secrete Exosomes Enriched in Distinctive Mirna and Trna Species. Stem Cell Res Ther (2015) 6:127. doi: 10.1186/s13287-015-0116-z
80. Li B, Liu J, Gu G, Han X, Zhang Q, Zhang W. Impact of Neural Stem Cell-Derived Extracellular Vesicles on Mitochondrial Dysfunction, Sirtuin 1 Level, and Synaptic Deficits in Alzheimer’s Disease. J Neurochem (2020) 154:502–18. doi: 10.1111/jnc.15001
81. Zhao M, Liu S, Wang C, Wang Y, Wan M, Liu F, et al. Mesenchymal Stem Cell-Derived Extracellular Vesicles Attenuate Mitochondrial Damage and Inflammation by Stabilizing Mitochondrial DNA. ACS Nano (2021) 15:1519–38. doi: 10.1021/acsnano.0c08947
82. Spees JL, Olson SD, Whitney MJ, Prockop DJ. Mitochondrial Transfer Between Cells Can Rescue Aerobic Respiration. Proc Natl Acad Sci USA (2006) 103:1283–8. doi: 10.1073/pnas.0510511103
83. Spees J, Lee R, Gregory C. Mechanisms of Mesenchymal Stem/Stromal Cell Function. Stem Cell Res Ther (2016) 7:1255. doi: 10.1186/s13287-016-0363-7
84. Plotnikov EY, Khryapenkova TG, Vasileva AK, Marey MV, Galkina SI, Isaev NK, et al. Cell-to-Cell Cross-Talk Between Mesenchymal Stem Cells and Cardiomyocytes in Co-Culture. J Cell Mol Med (2008) 12:1622–31. doi: 10.1111/j.1582-4934.2007.00205.x
85. Ingato D, Lee J, Sim S, Kwon Y. Good Things Come in Small Packages: Overcoming Challenges to Harness Extracellular Vesicles for Therapeutic Delivery. J Controlled Release Off J Controlled Release Soc (2016) 241:174–85. doi: 10.1016/j.jconrel.2016.09.016
86. Song T, Eirin A, Zhu X, Zhao Y, Krier J, Tang H, et al. Mesenchymal Stem Cell-Derived Extracellular Vesicles Induce Regulatory T Cells to Ameliorate Chronic Kidney Injury. Hypertension (Dallas Tex 1979) (2020) 75:1223–32. doi: 10.1161/hypertensionaha.119.14546
87. Gao F, Chiu SM, Motan DA, Zhang Z, Chen L, Ji HL, et al. Mesenchymal Stem Cells and Immunomodulation: Current Status and Future Prospects. Cell Death Dis (2016) 7:e20625. doi: 10.1038/cddis.2015.327
88. Robbins PD, Morelli AE. Regulation of Immune Responses by Extracellular Vesicles. Nat Rev Immunol (2014) 14:195–208. doi: 10.1038/nri3622
89. Martin-Rufino J, Espinosa-Lara N, Osugui L, Sanchez-Guijo F. Targeting the Immune System With Mesenchymal Stromal Cell-Derived Extracellular Vesicles: What is the Cargo’s Mechanism of Action? Front Bioeng Biotechnol (2019) 7:308. doi: 10.3389/fbioe.2019.00308
90. Wang S, Zhang C, Li J, Niyazi S, Zheng L, Xu M, et al. Erythropoietin Protects Against Rhabdomyolysis-Induced Acute Kidney Injury by Modulating Macrophage Polarization. Cell Death Dis (2017) 8:e27255. doi: 10.1038/cddis.2017.104
91. Roura S, Monguió-Tortajada M, Munizaga-Larroudé M, Clos-Sansalvador M, Franquesa M, Rosell A, et al. Potential of Extracellular Vesicle-Associated Tsg-6 From Adipose Mesenchymal Stromal Cells in Traumatic Brain Injury. Int J Mol Sci (2020) 21(18):6761. doi: 10.3390/ijms21186761.
92. Cheng A, Choi D, Lora M, Shum-Tim D, Rak J, Colmegna I. Human Multipotent Mesenchymal Stromal Cells Cytokine Priming Promotes rab27b-Regulated Secretion of Small Extracellular Vesicles With Immunomodulatory Cargo. Stem Cell Res Ther (2020) 11:539. doi: 10.1186/s13287-020-02050-6
93. Zhang R, Liu Y, Yan K, Chen L, Chen X, Li P, et al. Anti-Inflammatory and Immunomodulatory Mechanisms of Mesenchymal Stem Cell Transplantation in Experimental Traumatic Brain Injury. J Neuroinflamm (2013) 10:1065. doi: 10.1186/1742-2094-10-106
94. An J, Li Q, Ryu M, Nam A, Bhang D, Jung Y, et al. Tsg-6 in Extracellular Vesicles From Canine Mesenchymal Stem/Stromal Is a Major Factor in Relieving Dss-Induced Colitis. PloS One (2020) 15:e0220756. doi: 10.1371/journal.pone.0220756
95. Wang S, Cai S, Zhang W, Liu X, Li Y, Zhang C, et al. High-Mobility Group Box 1 Protein Antagonizes the Immunosuppressive Capacity and Therapeutic Effect of Mesenchymal Stem Cells in Acute Kidney Injury. J Trans Med (2020) 18:1755. doi: 10.1186/s12967-020-02334-8
96. Karachi A, Fazeli M, Karimi MH, Geramizadeh B, Moravej A, Ebrahimnezhad S, et al. Evaluation of Immunomodulatory Effects of Mesenchymal Stem Cells Soluble Factors on mir-155 and mir-23b Expression in Mice Dendritic Cells. Immunol Invest (2015) 44:427–37. doi: 10.3109/08820139.2015.1017046
97. Reis M, Mavin E, Nicholson L, Green K, Dickinson A, Wang X. Mesenchymal Stromal Cell-Derived Extracellular Vesicles Attenuate Dendritic Cell Maturation and Function. Front Immunol (2018) 9:2538. doi: 10.3389/fimmu.2018.02538
98. Favaro E, Carpanetto A, Caorsi C, Giovarelli M, Angelini C, Cavallo-Perin P, et al. Human Mesenchymal Stem Cells and Derived Extracellular Vesicles Induce Regulatory Dendritic Cells in Type 1 Diabetic Patients. Diabetologia (2016) 59:325–3. doi: 10.1007/s00125-015-3808-0
99. Mihajlovic M, Wever KE, van der Made TK, de Vries RBM, Hilbrands LB, Masereeuw R. Are Cell-Based Therapies for Kidney Disease Safe? A Systematic Review of Preclinical Evidence. Pharmacol Ther (2019) 197:191–211. doi: 10.1016/j.pharmthera.2019.01.004
100. Bai M, Zhang L, Fu B, Bai J, Zhang Y, Cai G, et al. Il-17a Improves the Efficacy of Mesenchymal Stem Cells in Ischemic-Reperfusion Renal Injury By Increasing Treg Percentages by The Cox-2/Pge2 Pathway. Kidney Int (2018) 93:814–25. doi: 10.1016/j.kint.2017.08.030
101. Zheng L, Gao W, Hu C, Yang C, Rong R. Immune Cells in Ischemic Acute Kidney Injury. Curr Protein Pept Sci (2019) 20:770–6. doi: 10.2174/1389203720666190507102529
102. Karbian N, Abutbul A, El-Amore R, Eliaz R, Beeri R, Reicher B, et al. Apoptotic Cell Therapy for Cytokine Storm Associated With Acute Severe Sepsis. Cell Death Dis (2020) 11:5355. doi: 10.1038/s41419-020-02748-8
103. Venkatachalam M, Weinberg J, Kriz W, Bidani A. Failed Tubule Recovery, Aki-Ckd Transition, and Kidney Disease Progression. J Am Soc Nephrol JASN (2015) 26:1765–76. doi: 10.1681/asn.2015010006
104. Messerer D, Halbgebauer R, Nilsson B, Pavenstädt H, Radermacher P, Huber-Lang M. Immunopathophysiology of Trauma-Related Acute Kidney Injury. Nat Rev Nephrol (2020) 17(2):91–111. doi: 10.1038/s41581-020-00344-9
105. Zilberman-Itskovich S, Efrati S. Mesenchymal Stromal Cell Uses for Acute Kidney Injury-Current Available Data and Future Perspectives: A Mini-Review. Front Immunol (2020) 11:1369. doi: 10.3389/fimmu.2020.01369
106. Grivei A, Giuliani K, Wang X, Ungerer J, Francis L, Hepburn K, et al. Oxidative Stress and Inflammasome Activation in Human Rhabdomyolysis-Induced Acute Kidney Injury. Free Radical Biol Med (2020) 160:690–95. doi: 10.1016/j.freeradbiomed.2020.09.011
107. Miura Y. [Current Basic Understanding and Clinical Application of Mesenchymal Stromal/Stem Cells]. Rinsho Ketsueki (2018) 59:1935–41. doi: 10.11406/rinketsu.59.1935
108. Witwer KW, Van Balkom BWM, Bruno S, Choo A, Dominici M, Gimona M, et al. Defining Mesenchymal Stromal Cell (MSC)-Derived Small Extracellular Vesicles for Therapeutic Applications. J Extracell Vesicles (2019) 8:16092065. doi: 10.1080/20013078.2019.1609206
109. Zhang K, Zhao X, Chen X, Wei Y, Du W, Wang Y, et al. Enhanced Therapeutic Effects of Mesenchymal Stem Cell-Derived Exosomes With an Injectable Hydrogel for Hindlimb Ischemia Treatment. ACS Appl Mater Interfaces (2018) 10:30081–91. doi: 10.1021/acsami.8b08449
110. Tolar J, Nauta AJ, Osborn MJ, Panoskaltsis Mortari A, McElmurry RT, Bell S, et al. Sarcoma Derived From Cultured Mesenchymal Stem Cells. Stem Cells (2007) 25:371–9. doi: 10.1634/stemcells.2005-0620
111. Zhang ZX, Guan LX, Zhang K, Wang S, Cao PC, Wang YH, et al. Cytogenetic Analysis of Human Bone Marrow-Derived Mesenchymal Stem Cells Passaged In Vitro. Cell Biol Int (2007) 31:645–8. doi: 10.1016/j.cellbi.2006.11.025
112. Xu S, De Becker A, De Raeve H, Van Camp B, Vanderkerken K, Van Riet I. In Vitro Expanded Bone Marrow-Derived Murine (c57bl/kalwrij) Mesenchymal Stem Cells can Acquire cd34 Expression and Induce Sarcoma Formation In Vivo. Biochem Biophys Res Commun (2012) 424:391–7. doi: 10.1016/j.bbrc.2012.06.118
113. Binato R, de Souza Fernandez T, Lazzarotto-Silva C, Du Rocher B, Mencalha A, Pizzatti L, et al. Stability of Human Mesenchymal Stem Cells During In Vitro Culture: Considerations for Cell Therapy. Cell Prolif (2013) 46:10–22. doi: 10.1111/cpr.12002
114. Zhang G, Wang D, Miao S, Zou X, Liu G, Zhu Y. Extracellular Vesicles Derived From Mesenchymal Stromal Cells may Possess Increased Therapeutic Potential for Acute Kidney Injury Compared With Conditioned Medium in Rodent Models: A Meta-Analysis. Exp Ther Med (2016) 11:1519–25. doi: 10.3892/etm.2016.3076
115. Kunter U, Rong S, Moeller M, Floege J. Mesenchymal Stem Cells as a Therapeutic Approach to Glomerular Diseases: Benefits and Risks. Kidney Int Suppl (2011) 1:68–73. doi: 10.1038/kisup.2011.16
116. Zhang M, Methot D, Poppa V, Fujio Y, Walsh K, Murry CE. Cardiomyocyte Grafting for Cardiac Repair: Graft Cell Death and Anti-Death Strategies. J Mol Cell Cardiol (2001) 33:907–21. doi: 10.1006/jmcc.2001.1367
117. Ashour R, Saad M, Sobh M, Al-Husseiny F, Abouelkheir M, Awad A, et al. Comparative Study of Allogenic and Xenogeneic Mesenchymal Stem Cells on Cisplatin-Induced Acute Kidney Injury in Sprague-Dawley Rats. Stem Cell Res Ther (2016) 7:1265. doi: 10.1186/s13287-016-0386-0
118. An X, Liao G, Chen Y, Luo A, Liu J, Yuan Y, et al. Intervention for Early Diabetic Nephropathy by Mesenchymal Stem Cells in a Preclinical Nonhuman Primate Model. Stem Cell Res Ther (2019) 10:3635. doi: 10.1186/s13287-019-1401-z
119. Cheng Y, Cao X, Qin L. Mesenchymal Stem Cell-Derived Extracellular Vesicles: A Novel Cell-Free Therapy for Sepsis. Front Immunol (2020) 11:647. doi: 10.3389/fimmu.2020.00647
120. Gholami L, Nooshabadi V, Shahabi S, Jazayeri M, Tarzemany R, Afsartala Z, et al. Extracellular Vesicles in Bone and Periodontal Regeneration: Current and Potential Therapeutic Applications. Cell Biosci (2021) 11:165. doi: 10.1186/s13578-020-00527-8
121. Bæk R, Søndergaard E, Varming K, Jørgensen M. The Impact of Various Preanalytical Treatments on the Phenotype of Small Extracellular Vesicles in Blood Analyzed by Protein Microarray. J Immunol Methods (2016) 438:11–20. doi: 10.1016/j.jim.2016.08.007
122. Caldas HC, Lojudice FH, Dias C, Fernandes-Charpiot IMM, Baptista M, Kawasaki-Oyama RS, et al. Induced Pluripotent Stem Cells Reduce Progression of Experimental Chronic Kidney Disease But Develop Wilms’ Tumors. Stem Cells Int (2017) 2017:7428316. doi: 10.1155/2017/7428316
123. Lopatina T, Grange C, Fonsato V, Tapparo M, Brossa A, Fallo S, et al. Extracellular Vesicles From Human Liver Stem Cells Inhibit Tumor Angiogenesis. Int J Cancer (2019) 144:322–3. doi: 10.1002/ijc.31796
124. Ullah M, Liu D, Rai S, Razavi M, Choi J, Wang J, et al. A Novel Approach to Deliver Therapeutic Extracellular Vesicles Directly Into the Mouse Kidney Via its Arterial Blood Supply. Cells (2020) 9(4):937. doi: 10.3390/cells9040937.
125. Bruno S, Grange C, Collino F, Deregibus M, Cantaluppi V, Biancone L, et al. Microvesicles Derived From Mesenchymal Stem Cells Enhance Survival in a Lethal Model of Acute Kidney Injury. PloS One (2012) 7:e331155. doi: 10.1371/journal.pone.0033115
126. Nancarrow-Lei R, Mafi P, Mafi R, Khan W. A Systemic Review of Adult Mesenchymal Stem Cell Sources and Their Multilineage Differentiation Potential Relevant to Musculoskeletal Tissue Repair and Regeneration. Curr Stem Cell Res Ther (2017) 12:601–10. doi: 10.2174/1574888x12666170608124303
127. Allan D, Tieu A, Lalu M, Burger D. Mesenchymal Stromal Cell-Derived Extracellular Vesicles for Regenerative Therapy and Immune Modulation: Progress and Challenges Toward Clinical Application. Stem Cells Transl Med (2020) 9:39–46. doi: 10.1002/sctm.19-0114
128. Cardoso R, Rodrigues S, Gomes C, Duarte F, Romao M, Leal E, et al. Development of an Optimized and Scalable Method for Isolation of Umbilical Cord Blood-Derived Small Extracellular Vesicles for Future Clinical Use. Stem Cells Trans Med (2021) 10(6):910–21. doi: 10.1002/sctm.20-0376
129. Tsiapalis D, O’Driscoll L. Mesenchymal Stem Cell Derived Extracellular Vesicles for Tissue Engineering and Regenerative Medicine Applications. Cells (2020) 9(4):991. doi: 10.3390/cells9040991
130. Cao J, Wang B, Tang T, Lv L, Ding Z, Li Z, et al. Three-Dimensional Culture of MSCs Produces Exosomes With Improved Yield and Enhanced Therapeutic Efficacy for Cisplatin-Induced Acute Kidney Injury. Stem Cell Res Ther (2020) 11:2065. doi: 10.1186/s13287-020-01719-2
131. Lee J, Ha D, Go H, Youn J, Kim H, Jin R, et al. Reproducible Large-Scale Isolation of Exosomes From Adipose Tissue-Derived Mesenchymal Stem/Stromal Cells and Their Application in Acute Kidney Injury. Int J Mol Sci (2020) 21(13):4774. doi: 10.3390/ijms21134774
132. Ullah M, Liu D, Rai S, Dadhania A, Jonnakuti S, Concepcion W, et al. Reversing Acute Kidney Injury Using Pulsed Focused Ultrasound and Msc Therapy: A Role for Hsp-Mediated pi3k/akt Signaling. Molecular Therapy. Methods Clin Dev (2020) 17:683–94. doi: 10.1016/j.omtm.2020.03.023
133. Ullah M, Liu D, Rai S, Concepcion W, Thakor A. Hsp70-mediated Nlrp3 Inflammasome Suppression Underlies Reversal of Acute Kidney Injury Following Extracellular Vesicle and Focused Ultrasound Combination Therapy. Int J Mol Sci (2020) 21(11):4085. doi: 10.3390/ijms21114085.
134. Ullah M, Liu D, Rai S, Razavi M, Concepcion W, Thakor A. Pulsed Focused Ultrasound Enhances the Therapeutic Effect of Mesenchymal Stromal Cell-Derived Extracellular Vesicles in Acute Kidney Injury. Stem Cell Res Ther (2020) 11:3985. doi: 10.1186/s13287-020-01922-1
135. Liu Y, Cui J, Wang H, Hezam K, Zhao X, Huang H, et al. Enhanced Therapeutic Effects of MSC-Derived Extracellular Vesicles With an Injectable Collagen Matrix for Experimental Acute Kidney Injury Treatment. Stem Cell Res Ther (2020) 11:1615. doi: 10.1186/s13287-020-01668-w
136. Alzahrani F. Melatonin Improves Therapeutic Potential of Mesenchymal Stem Cells-Derived Exosomes Against Renal Ischemia-Reperfusion Injury in Rats. Am J Trans Res (2019) 11(5):2887–907.
137. Zhang Z, Hou Y, Zou X, Xing X, Ju G, Zhong L, et al. Oct-4 Enhanced the Therapeutic Effects of Mesenchymal Stem Cell-Derived Extracellular Vesicles in Acute Kidney Injury. Kidney Blood Pressure Res (2020) 45:95–108. doi: 10.1159/000504368
138. Shekari F, Nazari A, Assar Kashani S, Hajizadeh-Saffar E, Lim R, Baharvand H. Pre-Clinical Investigation of Mesenchymal Stromal Cell-Derived Extracellular Vesicles: A Systematic Review. Cytotherapy (2021) 23(4):277–84. doi: 10.1016/j.jcyt.2020.12.009
139. Théry C, Witwer K, Aikawa E, Alcaraz M, Anderson J, Andriantsitohaina R, et al. Minimal Information for Studies of Extracellular Vesicles 2018 (Misev2018): A Position Statement of the International Society for Extracellular Vesicles and Update of the Misev2014 Guidelines. J Extracell Vesicles (2018) 7:1535750. doi: 10.1080/20013078.2018.1535750
140. Zhang N, Song Y, Huang Z, Chen J, Tan H, Yang H, et al. Monocyte Mimics Improve Mesenchymal Stem Cell-Derived Extracellular Vesicle Homing in a Mouse Mi/Ri Model. Biomaterials (2020) 255:1201685. doi: 10.1016/j.biomaterials.2020.120168
Keywords: acute kidney injury, mesenchymal stem cell, extracellular vesicle, cytokine, tubular epithelial cell
Citation: Li J-K, Yang C, Su Y, Luo J-C, Luo M-H, Huang D-L, Tu G-W and Luo Z (2021) Mesenchymal Stem Cell-Derived Extracellular Vesicles: A Potential Therapeutic Strategy for Acute Kidney Injury. Front. Immunol. 12:684496. doi: 10.3389/fimmu.2021.684496
Received: 23 March 2021; Accepted: 18 May 2021;
Published: 03 June 2021.
Edited by:
Bin Yang, University of Leicester, United KingdomReviewed by:
Min Shi, Sichuan University, ChinaCopyright © 2021 Li, Yang, Su, Luo, Luo, Huang, Tu and Luo. This is an open-access article distributed under the terms of the Creative Commons Attribution License (CC BY). The use, distribution or reproduction in other forums is permitted, provided the original author(s) and the copyright owner(s) are credited and that the original publication in this journal is cited, in accordance with accepted academic practice. No use, distribution or reproduction is permitted which does not comply with these terms.
*Correspondence: Zhe Luo, bHVvLnpoZUB6cy1ob3NwaXRhbC5zaC5jbg==; Guo-Wei Tu, dHUuZ3Vvd2VpQHpzLWhvc3BpdGFsLnNoLmNu
†These authors have contributed equally to this work
Disclaimer: All claims expressed in this article are solely those of the authors and do not necessarily represent those of their affiliated organizations, or those of the publisher, the editors and the reviewers. Any product that may be evaluated in this article or claim that may be made by its manufacturer is not guaranteed or endorsed by the publisher.
Research integrity at Frontiers
Learn more about the work of our research integrity team to safeguard the quality of each article we publish.