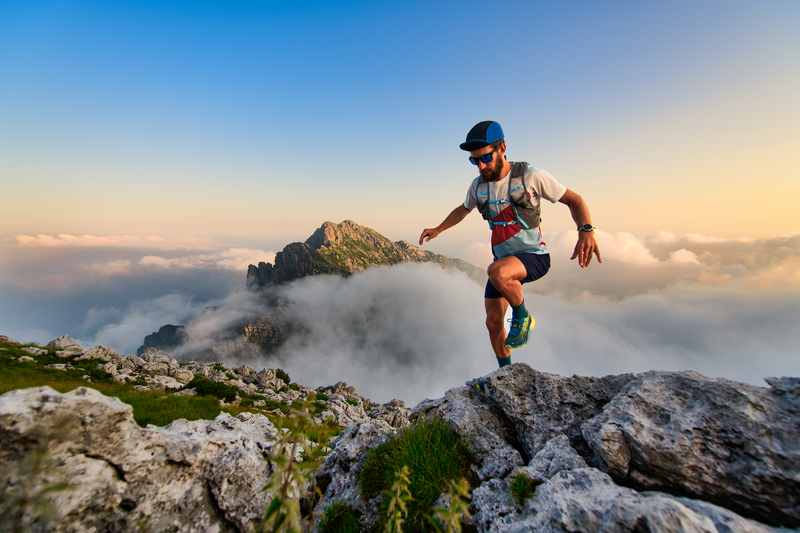
94% of researchers rate our articles as excellent or good
Learn more about the work of our research integrity team to safeguard the quality of each article we publish.
Find out more
ORIGINAL RESEARCH article
Front. Immunol. , 10 September 2021
Sec. Nutritional Immunology
Volume 12 - 2021 | https://doi.org/10.3389/fimmu.2021.682724
Bacterial infection presents severe challenge to tilapia farming, which is largely influenced by water temperature. However, how water temperature determines tilapias’ survival to infection is not well understood. Here, we address this issue from the perspective of metabolic state. Tilapias were more susceptible to Aeromonas sobria infection at 33°C than at 18°C, which is associated with differential metabolism of the fish. Compared to the metabolome of tilapia at 18°C, the metabolome at 33°C was characterized with increased an tricarboxylic acid cycle and a reduced level of myo-inositol which represent the most impactful pathway and crucial biomarker, respectively. These alterations were accompanied with the elevated transcriptional level of 10 innate immune genes with infection time, where il-1b, il-6, il-8, and il-10 exhibited a higher expression at 33°C than at 18°C and was attenuated by exogenous myo-inositol in both groups. Interestingly, exogenous myo-inositol inactivated the elevated TCA cycle via inhibiting the enzymatic activity of succinate dehydrogenase and malate dehydrogenase. Thus, tilapias showed a higher survival ability at 33°C. Our study reveals a previously unknown relationship among water temperature, metabolic state, and innate immunity and establishes a novel approach to eliminate bacterial pathogens in tilapia at higher water temperature.
GIFT (genetically improved farmed tilapia, Oreochromis niloticus) is one of the most extensively farmed economic fish species in the world due to its high protein content, large size, rapid growth and great adaptability to a wide range of rearing conditions (1, 2). The animal is cultured in more than 100 tropical and subtropical countries, and thereby fishing productivity is improved and the development of aquaculture is facilitated in the world (3, 4). Although tilapia accounts for 7.4% of global production in 2015, the demand for tilapia is increasing in recent years. Therefore, many countries in Asia, Africa, and Latin America expand the tilapia farming for driving the global growth. China is the biggest producer and exporter of the fish, accounting for around 26% of total supply in 2019. In 2020, an increase of 3%–4% compared to 2018 is obtained, which increases income approximately 252 million USD (http://www.fao.org/in-action/globefish/market-reports/tilapia/). Therefore, culture of tilapia is valuable in aquaculture in the world.
However, as tilapia aquaculture has intensified and become more global, disease outbreaks are the main cause of reduced production that results in huge economic loss (5), where bacteriosis continues to be one of the major issues compromising sustainability (6). Among environmental factors that are related to bacterial infection, water temperature plays a crucial role especially due to fish as a poikilothermal animal. The water temperature fluctuates violently as the seasons change (7) and is a major factor in the outbreak of bacterial infection (8–13). Reports have indicated that tilapia is more sensitive to pathogens such as Streptococcus agalactiae, S. iniae, and Francisella noatunensis at higher water temperature than at lower water temperature (8–12). Temperature also impacts vaccine efficacy and innate immune response in cultured tilapia (14–17). Therefore, control of bacterial infection especially at higher water temperature is particularly important for sustainability of tilapia farming. However, how to control the higher water temperature-related bacterial infection is largely unknown.
Like S. agalactiae and Edwardsiella tarda, Aeromonas sobria is one of the most important pathogens in tilapia farms worldwide (18). Higher water temperature (above 30°C) has been designated as a predisposing factor for the disease in tilapias (11). Unfortunately, there are still few effective measures to prevent and control temperature-related infectious diseases in tilapia instead of antibiotics. Thus, development of effective approaches is especially important for tilapia culture sustainability.
Recently, we have developed a functional metabolomics approach to identify crucial biomarkers (19–22). Then, the crucial biomarkers are used to reprogram an antibiotic-resistant metabolome and an infective metabolome to an anti-sensitive metabolome and an anti-infective metabolome, respectively, thereby decreasing the viability of antibiotic-resistant bacteria and elevating the survival ability of hosts infected by pathogens (23–28). We have also demonstrated that the approach is effective in increasing the ability of fish against bacterial infection at higher temperature (11, 13). In the present study, we extended the approach to tilapia infected with A. sobria at higher temperature and showed a higher temperature-mediated differential metabolome. Of the selected biomarkers of the differential metabolome, myo-inositol was identified as the most crucial biomarker. Myo-inositol is a bioactive compound that lies in dual functions as signals and as key metabolites under stress (29). It modulates innate immunity response and thereby elevates survival of tilapia infected with A. sobria at higher temperature.
Juvenile GIFT (1 month old, body length, 3–4 cm; body weight, 2 ± 0.2 g) were commercially obtained from a tilapia-breeding corporation in Guangzhou, P.R. China. Microbiological detection confirmed that these animals were free of specific pathogens. Tilapias were cultured as previously described (13). In brief, they were maintained in 25-l open-circuit water tanks with aeration and fed on a balanced commercial diet (Jinfeng®, Jiangmen, P.R. China). Water temperature was then slowly changed until these fish were adapted to constant water temperatures at 18°C or 33°C. The water temperatures were monitored regularly. Tilapias were kept at one of the two temperatures for 7 days before all experiments including bacterial challenge, measurement of enzyme activity, and innate immunity genes. The culture and treatment of the experiments were approved by the Institutional Animal Care and Use Committee of Sun Yat-sen University (Approval NO. SYSU-IACUC-2020-B126716).
Bacterial strain A. sobria is from the collection of our laboratory, which was isolated from a diseased fish. A single colony was picked out from an agar plate and grown in LB medium (028324 and 028334, Huankai Microbial Co., Ltd., Guangzhou, P.R. China) overnight (16 h) up to OD600 value 2.0. The overnight culture was diluted into fresh LB medium at 1:100 and grown with shaking at 200 rpm at 30°C until the OD600 of the culture reached 1.0. Followed by centrifugation at 10,000 rpm for 10 min, bacterial cells were pelleted. After being washed with 0.85% saline solution three times, these bacterial cells were suspended in saline solution and used for bacterial challenge. Each tilapia was intraperitoneally injected with 10 µl of 1 × 105 CFU A. sobria (LD50) using a microsyringe (F519160, Sangon Biotech Co., Ltd., Shanghai, China) as an experiment group and was intraperitoneally injected with the same volume of saline solution as a control (n = 30 for each treatment). The infective dose of A. sobria used was previously determined at 33°C. These tilapias were observed for symptoms twice daily for 15 days for accumulative death. The symptom of infected fish includes slight bleeding around the eyes, spotty bleeding around the gill cover, inflated intestinal tract, and congestive necrosis spleen, suggesting that these tilapias died of multiple-organ hemorrhagic septicemia (30, 31). Germs were isolated for identification of 16S rDNA to quarantine that the infection was caused by A. sobria.
To study the metabolic response of tilapia under different temperatures, metabolite abundance was measured by a GC-MS platform. Sample preparation was carried out as previously described (27). In brief, these challenged tilapias and control were euthanized in ice slush for at least 10 min following cessation of gill movement. The fish were rinsed with distilled water and then wiped thoroughly. Spleens were removed ascetically, where 25 mg of spleen was cut. Three spleens were mixed for one sample and then immediately immersed in 1 ml cold methanol. The samples were sonicated for 5 min at a 10-W-power setting using the Ultrasonic Processor (JY92-IIDN, Scientz, China), followed by centrifugation at 12,000 rpm in 4°C for 10 min. Supernatant was collected, and 10 µl ribitol (0.1 mg per mL, Sigma-Aldrich, USA) was added into each sample tube as an internal quantitative standard. The supernatant was concentrated for metabolite derivatization of GC-MS analysis. Every experiment was repeated by five biological replicates. GC-MS detection and spectral processing for GC-MS were carried out using the Agilent 7890A GC equipped with an Agilent 5975C VL MSD detector (Agilent Technologies, USA) as previously described (27).
To investigate the effect of exogenous myo-inositol on resistance to infection, tilapias (n = 120) were randomly divided into four groups, including three treatment groups (n = 30 per group) and one control group (n = 30), and acclimatized for seven days at 33°C. For the three treatment groups, tilapias were intraperitoneally injected with 200, 400, or 800 µg myo-inositol per fish, where 400 µg myo-inositol was calculated by the increased myo-inositol/g spleen at 33°C × fish weight; n = 30 for each dose (Sigma-Aldrich, USA). For the control group, tilapias were injected with saline only (n = 30). The injection was conducted once daily for 3 days. Finally, tilapias were challenged by intraperitoneal injection of A. sobria with 10 µl of 1 × 105 CFU and fish mortality was observed for 15 days for accumulative death. The bacterial challenge was performed twice.
To investigate the effect of exogenous myo-inositol on metabolic flux, enzyme activity was measured as previously described (27). In brief, spleens were removed from three tilapias, rinsed with precooled 1× PBS (50 mM, pH 7.4; Invitrogen, USA), resuspended in lysate buffer, and disrupted by sonication for 5 min at a 10-W-power setting. Supernatant with 100 and 250 µg was applied for pyruvate dehydrogenase (PDH) and α-ketoglutarate dehydrogenase (KGDH) assay and succinate dehydrogenase (SDH) and malate dehydrogenase (MDH) assay, respectively. The enzymatic assay was carried out in a 96-well plate with a final volume of 200 µl by mixing an equal volume of the protein sample and reaction buffer.
To investigate the expression of innate immunity genes, the same procedures of the exogenous administration of myo-inositol and bacterial challenge as earlier described were performed to collect spleens, an important immune organ, for RNA isolation at 0, 3, 6, and 9 h postinfection of 1 × 103 CFU A. sobria. One spleen was used as a biological sample. Each experimental group contained six biological samples, which were subjected to two technical replicates, respectively. qRT-PCR was performed as previously described (32). In brief, primers for each gene are listed in Table 1 and each primer pair was specific. Actin, tubulin, and GAPDH genes were chosen as the internal control. The relative expression of each gene was determined by the comparative threshold cycle method (2-ΔΔCT method).
Data transformations and manipulations were done using Excel. The Mann–Whitney U test (α = 0.05) with SPSS 23.0 (IBM, USA) was used to compare the difference in abundance of metabolites between two groups. The MetaboAnalyst online website (www.metaboanalyst.ca) was adopted to perform a multivariate statistical analysis of the metabolomic data (33, 34). Z-score analysis was carried out using the following formula: xij represented metabolites’ peak area, AVGi represented the average of the control group, and SDi represented the standard deviation of the control group. Enriched metabolic pathways were identified using the MetaboAnalyst online website (www.metaboanalyst.ca) (5, 34). Prism v5.01 (GraphPad, La Jolla, CA, USA) was used to draw the histogram and the scatter plot. Comparative metabolic pathway analysis between the two groups was performed using iPath 2.0 (https://pathways.embl.de/) (35).
For the data processing of qRT-PCR and enzyme activity, non-parametric Kruskal–Wallis one-way analysis with Dunn’s multiple-comparison post hoc test was used using SPSS 23.0; p < 0.05 and p < 0.01 were considered significant.
To investigate the effect of water temperature on tilapias’ ability against bacterial infection, tilapia were cultured at 18°C or 33°C and challenged with 1 × 105 CFU of A. sobria, which is an LD50 dose obtained in pretest. Survival rates were different between the two temperatures as assessed by cumulative survival rates until 15 days postinfection. The survival rate of the fish was 86.67% and 50% at 18°C and 33°C, respectively (Figure 1). These results indicate that tilapias are more susceptible to A. sobria infection at 33°C than at 18°C.
Figure 1 Survival of GIFT (genetically improved farmed tilapia, Oreochromis niloticus) to A. sobria infection cultured at 18°C and 33°C. Tilapias (n = 30 per group) were acclimated at 18°C and 33°C for 7 days before bacterial challenge. For bacterial infection, tilapias were injected with 10 µl 1 × 105 CFU/fish A. sobria or 10 µl saline solution as negative control. Accumulative death was monitored for a total of 15 days. **p < 0.01.
Then, we investigated the effect of water temperature on tilapia metabolome. Spleens were surgically removed from tilapia that was maintained at 18°C and 33°C for a week. The spleens were homogenated and prepared for GC-MS-based metabolomic analysis. Ten individuals with two technical repeats were carried out in each group, yielding a total of 40 data sets. Representative total ion current chromatograms from the 18°C and 33°C groups are listed in Figure 2A. A total of 280 aligned individual peaks were obtained from each sample. The correlation coefficients between technical replicates varied between 0.9307 and 0.9996, assuring the reproducibility of the data (Figure 2B). A total of 115 metabolites were identified, after the removal of the internal standard and any known artificial peaks. According to the annotation of KEGG (http://www.kegg.jp/) and NCBI PubChem (https://pubchem.ncbi.nlm.nih.gov/), the metabolites were classified into five categories, carbohydrate (26.96%), amino acid (20.06%), nucleotide (15.65%), fatty acid (16.52%), and others (14.78%) (Figure 2C). Metabolomic profiles of the two groups were displayed as a heat map (Figure 2D). These results indicate that the tilapia have differential metabolism when cultured at different temperatures.
Figure 2 Tilapia cultured at 18°C or 33°C had a different metabolome. (A) Representative total ion current chromatogram from 18°C or 33°C of samples. (B) Reproducibility of the metabolomic profiling platform used in the discovery phase. Abundance of metabolites quantified in samples over two technical replicates is shown. The Pearson correlation coefficient between technical replicates varies between 0.9307 and 0.9996. (C) Categories of the differential metabolites. One hundred fifteen metabolites with differential abundance are searched against in KEGG for their categories, and the pie chart is generated in Excel 2010 (Microsoft, USA). (D) Heat map of unsupervised hierarchical clustering of different metabolites (row). Blue and red indicate decrease and increase of the metabolites scaled to mean and standard deviation of row metabolite level, respectively (see color scale).
We speculated that the difference in the mortality was related to the characteristic metabolome constructed by differential metabolites. Thus, the differential metabolome that distinguished 33°C groups from 18°C was explored. A two-sided Mann–Whitney U test coupled with permutation test was used to identify the differential abundance of metabolites between the 18°C group and the 33°C group. A total of 97 differential abundance of metabolites (p < 0.01) was identified at the 33°C group, which corresponded to a false discovery rate (FDR) less than 0.048737. The identified metabolites are shown in Figure 3A as a heat map, where the two groups were clearly separated. The Z-score plot spans from 52.52 to -214.06 in these groups. In comparison to the 18°C control group, 75 metabolites were increased and 22 metabolites were decreased in the 33°C group (Figure 3B). Among them, myo-inositol was the most depressed metabolite. The differential metabolites were classified into five categories, carbohydrate (23.71%), amino acid (29.9%), nucleotide (16.43%), fatty acid (18.56%), and others (11.34%) (Figure 3C). Increased and decreased metabolites in these categories are shown in Figure 3D, showing that the number of increased metabolites is higher than that of decreased metabolites. These results indicate that the metabolome at 33°C is different from the metabolome at 18°C.
Figure 3 Differential metabolic profiles of tilapias cultured at different temperatures. (A) Heat map showing 97 differential metabolites. Red and blue indicate increase and decrease of metabolites relative to the median metabolite level, respectively (see color scale). (B) Z-score plot of differential metabolites based on the 18°C group. Each point represents one metabolite in one technical repeat and colored by sample types. (C) The different metabolites were classified into five categories, carbohydrate (23.71%), amino acid (29.9%), nucleotide (16.43%), fatty acid (18.56%), and others (11.34%). (D) Number of increased and decreased metabolites in these categories (C), 17 and 6 (carbohydrate), 24 and 5 (amino acid), 14 and 2 (nucleotide), 12 and 6 (fatty acid), and 8 and 3 (others), respectively.
To explore the most crucial metabolites differentiating 33°C groups from 18°C groups, principal component analysis (PCA) and orthogonal partial least square discriminant analysis (OPLS-DA) were conducted to recognize the sample pattern. PC1 (96.16%) and PC2 (1.5%) of PCA (Figure 4A) and Component 1 (T score [1] = 91.29%) and Component 2 (orthogonal T score [1] = 2.4%) of OPLS-DA (Figure 4B) separated the samples into two quarters. Using unsupervised pattern recognition and supervised pattern recognition, PC 1 and Component 1 clearly separate the 33°C group from the 18°C group. Discriminating variables were shown with the S-plot (Figure 4C) (R2X = 0.926, R2Y = 0.979, Q2 = 0.977) when cutoff values were set as greater or equal to 0.05 and 0.5 for the absolute values of covariance p and correlation p(corr), respectively. Eleven biomarkers screened by component p[1] and p(corr)[1] are shown in Figure 4C in red oval. Among these biomarkers, the abundance of five biomarkers was reduced including myo-inositol and its derivatized forms, myo-inositol-1-phosphate and myo-inositol-2-phosphate. The abundance of these metabolites is shown in Figure 4D. These results with the above analysis on the most depressed myo-inositol indicate that myo-inositol can be identified as a crucial biomarker.
Figure 4 Identification of crucial metabolites. (A) PCA analysis of 18°C and 33°C groups according to the treatment set. Each dot represents the technological replicate analysis of samples in the plot. PC 1 and PC 2 used in this plot explain 97.66% of the total variance which allows confident interpretation of the variation. (B) OPLS-DA analysis of 18°C and 33°C groups. Dots represent technological replicates. Component 1 (T score [1] = 91.29%) and Component 2 (orthogonal T score [1] = 2.4%) of OPLS-DA explain 93.69% of the total variance. (C) S-plot generates from OPLS-DA (R2X = 0.926, R2Y = 0.979, Q2 = 0.977). Predictive component p[1] and correlation p(corr)[1] differentiate 18°C from 33°C. Dot represents metabolites, and candidate biomarkers are highlighted in red. (D) Scatter plot of myo-inositol, myo-inositol-1-phosphate, and myo-inositol-2-phosphate. **p < 0.01.
We further investigated the pathways that were impacted in these two temperatures, which are important indicators for the alterations of the metabolome. Using MetaboAnalyst 4.0, 9 metabolic pathways were enriched for the 33°C group (Figure 5A). According to impact value, the nine most impacted pathways included alanine, aspartate, and glutamate metabolism; taurine and hypotaurine metabolism; D-glutamine and D-glutamate metabolism; citrate cycle (TCA cycle); purine metabolism; arginine biosynthesis; aminoacyl-tRNA biosynthesis; pantothenate and CoA biosynthesis; and valine, leucine, and isoleucine biosynthesis. Integrative analysis of metabolites in the enriched pathways is carried out, where red and blue indicate increased and decreased metabolites, respectively. Of particular interest is that all metabolites of the TCA cycle were increased in the 33°C group (Figure 5B). These results indicate that the increased TCA cycle forms a characteristic feature in tilapias that survived at 33°C.
Figure 5 Pathway enrichment and analysis. (A) Pathway enrichment of differential metabolites in the 33°C group compared with the 18°C group. In terms of impact value from the largest to the smallest, 1 to 9, respectively, represent alanine, aspartate and glutamate metabolism; taurine and hypotaurine metabolism; D-glutamine and D-glutamate metabolism; citrate cycle (TCA cycle); purine metabolism; arginine biosynthesis; aminoacyl-tRNA biosynthesis; pantothenate and CoA biosynthesis; and valine, leucine, and isoleucine biosynthesis. Significantly enriched pathways are selected to plot. (B) Integrative analysis of metabolites in significantly enriched pathways. Red and blue indicate increased and decreased metabolites, respectively.
Furthermore, comparative metabolic pathway analysis between the 18°C and 33°C groups was performed in iPath 2.0. The iPath analysis generates a global view and provides a better insight into the effects of culture temperature on the metabolic profile of the tilapias, where red line represents increased pathways in the 33°C group and blue line represents decreased pathways in the 33°C group. We found activation of the TCA cycle, amino acid metabolism, energy metabolism, and nucleotide metabolism as the elevated metabolic pathways and inactivation of carbohydrate metabolism and weak inositol metabolism as the reduced production of key metabolites (Figure 6). The integrated analysis of the metabolites with differential abundance and pathway enrichment suggests that the reduction of myo-inositol abundance and the activation of the TCA cycle play a role in fish death at higher water temperature.
Figure 6 Comparative metabolic pathway analysis between the 18°C and 33°C groups. Analysis of the metabolic profiles resulting from tilapias cultured at 18°C and 33°C provides a better insight into the effect of 97 differential abundances of metabolites (p < 0.01). Based on the KEGG compound (http://www.kegg.jp/kegg/compound/), metabolic network pathways in tilapias are further analyzed with iPath2.0 (http://pathways.embl.de/iPath2.cgi). Red line represents increase in the 33°C group; blue line represents decrease in the 33°C group.
We hypothesized that elevation of myo-inositol in tilapia may promote fish survival to bacterial challenge at 33°C. To explore this idea, tilapias were intraperitoneally injected with 200, 400, or 800 µg myo-inositol per fish, followed by the challenge with A. sobria at 33°C. The different doses of exogenous myo-inositol showed a protective impact on the bacterial infection in a dose-dependent manner (Figure 7A). The occurrence may be related to the possibility that exogenous myo-inositol impacts the TCA cycle since the elevated TCA cycle is associated with the susceptibility at 33°C. Activity of PDH, SDH, KGDH, and MDH in the TCA cycle was quantified in tilapias cultured at 18°C and 33°C. Higher activity of the four enzymes was detected at 33°C than at 18°C. In detail, PDH activity was increased from 6.55 ± 0.48 U/mg at 18°C to 8.67 ± 0.75 U/mg at 33°C; KGDH activity was increased from 8.83 ± 0.28 U/mg at 18°C to 12.39 ± 0.44 U/mg at 33°C; SDH activity was increased from 30.28 ± 1.59 U/mg at 18°C to 41.04 ± 2.39 U/mg at 33°C; MDH activity was increased from 16.03 ± 0.59 U/mg at 18°C to 20.73 ± 0.75 U/mg at 33°C) (Figure 7B). Then, the activity was further detected at 33°C in the absence or presence of 800 µg exogenous myo-inositol. The exogenous myo-inositol inhibited the activity of SDH and MDH but did not affect the activity of PDH and KGDH. Specifically, SDH activity was reduced from 42.35 ± 0.66 to 36.89 ± 2.00 U/mg and MDH activity was reduced from 20.27 ± 1.56 to 14.79 ± 0.34 U/mg at 33°C compared with that at 18°C (Figure 7C). These results indicate that myo-inositol reprograms the TCA cycle, leading to the elevated survival in A. sobria infection at 33°C.
Figure 7 Myo-inositol impacts the TCA cycle and promote tilapia’s ability against bacterial infection. (A) Survival of tilapias post A sobria infection in the presence of myo-inositol. Tilapias were treated with saline control (0 µg per fish) or different doses of myo-inositol (200, 400, and 800 µg per fish) at 33°C for 3 days, followed by bacterial challenge through intraperitoneal injection (1 × 105 CFU A. sobria). The accumulative fish death was monitored for a total of 15 days postinfection (n =30 per group). (B) Activity of PDH, KGDH, SDH, and MDH of spleens at 18°C and 33°C. (C) Activity of PDH, KGDH, SDH, and MDH of spleens in the presence or absence of 800 µg myo-inositol per fish. Values are means ± SEM (n = 6 per group), and statistic difference is analyzed with non-parametric Kruskal–Wallis one-way analysis with Dunn’s multiple-comparison post hoc test. *p < 0.05; **p < 0.01 (B, C).
Elimination of bacterial pathogens is dependent on immunity. It is reasonable that myo-inositol is related to immune response in the temperature-mediated survival. To demonstrate this, we quantified the dynamic transcriptional level of 10 innate immune genes, il-1b, il-6, il-8, il-10, il-21, tnf-α, inf-γ, tlr-1, cox 2, and lysozyme C, at 0, 3, 6, and 9 h postinfection after a 3-day injection of myo-inositol. The expression of the genes represents four patterns. The expression of il-1b, il-6, il-8, and il-10 was increased along with the infection time, where the expression of the four genes was higher at 33°C than at 18°C. However, myo-inositol attenuated the expression at both groups. The expression of il-21 and inf-γ represents the second pattern, where the expression of both genes was increased upon infection but was unaffected by inositol. In the third pattern, the expression of tnf-α and cox 2 was increased and reduced, respectively, during infection, and interestingly, inositol dramatically increased the gene expression at 33°C but only slightly increased at the 18°C group. In the fourth pattern, the expression of tlr-1 and lysozyme C was elevated with time postinfection, where a higher expression was detected at 33°C than at 18°C but not regulated by myo-inositol (tlr-1) or regulated by myo-inositol (lysozyme C) (Figure 8A). These results indicate that myo-inositol modulates innate immune response that is related to the survival ability against A. sobria infection at 33°C. To further explore whether the myo-inositol regulation for bacterial infection is effective at 18°C for understanding the role of the TCA cycle in tilapias against the bacterial infection, the same experiment on bacterial challenge was carried out at 18°C. Fish survival was elevated in a myo-inositol dose-dependent manner at 18°C (Figure 8B) in comparison with the event that the elevation of inflammatory cytokines il-1b, il-6, il-8, and il-10 was attenuated by exogenous myo-inositol, as described above (Figure 8A). The finding supports the conclusion that the TCA cycle is related to survival of tilapias against A. sobria infection. These results indicate that myo-inositol regulates innate immune response which contributes to the survival ability against A. sobria infection.
Figure 8 Innate immune responses of tilapias. (A) qRT-PCR for innate immune genes of tilapia treated with saline solution (control) or 800 µg myo-inositol for 3 days following A. sobria challenge through intraperitoneal injection (1 × 103 CFU A. sobria). Spleens were collected at 0, 3, 6, and 9 h postinjection for RNA extraction and qRT-PCR. Values are means ± SEM from six biological replicates. **p < 0.01. (B) Survival of tilapias post A. sobria infection in the presence of myo-inositol. Tilapias were treated with saline control (0 µg per fish) or different doses of myo-inositol (200, 400, and 800 µg per fish) at 18°C for 3 days, followed by bacterial challenge through intraperitoneal injection (1 × 105 CFU A. sobria). The accumulative fish death was monitored for a total of 15 days postinfection (n =30 per group).
Water temperature affecting tilapia’s ability against bacterial infection is documented (8, 10, 11). However, the underlying mechanisms are largely unknown. The present study explores the metabolic mechanisms accounting for such capability. Tilapias can grow between 16°C and 38°C, with an optimum range between 25°C and 28°C (36). For identifying big contrast biomarkers between higher and lower water temperatures, 33°C and 18°C were selected. It is clear that tilapias have differential metabolomes between 18°C and 33°C, which are related to higher and lower survival after A. sobria infection, respectively. The lower water temperature metabolome is more efficient against bacterial infection than the higher water temperature metabolome. This is because the metabolome is associated with host anti-infective innate immunity (11, 13, 37). Thus, metabolome reflects the metabolic state that demonstrates host ability against bacterial pathogens. Notably, low temperature inhibits bacterial proliferation, which affects bacterial virulence and thereby leads to fish higher survival. However, exogenous myo-inositol promoted the survival of fish challenged by A. sobria at 33°C than at 18°C, indicating metabolic modulation plays a role in the temperature-related survival.
In the present study, the increased TCA cycle and the decreased level of myo-inositol were identified as the metabolic signature to differentiate the higher water temperature metabolome from the lower water temperature metabolome. More importantly, there is a close link between the TCA and myo-inositol that myo-inositol inhibits the activity of SDH and MDH and thereby weakens the TCA cycle. This is a previously unrecognized correlation of the events that can explain that the reduced level of myo-inositol is accompanied with the increased TCA cycle. Since the elevated TCA cycle is related to the low survival of tilapia at 33°C, the reduced TCA cycle mediated by myo-inositol contributes to the survival of the fish in the same water temperature. Meanwhile, myo-inositol also potentiates fish against the infection at 18°C. Thus, myo-inositol can be a potential drug to elevate the survival of tilapia at the higher water temperature. These findings indicate that the elevation of the TCA cycle is a stress response at higher water temperature. However, the response affects the survival of tilapia. Recently, the relationship between the TCA cycle and bacterial infection has been explored. In response to zebrafish infection caused by Vibrio alginolyticus, the activation and inactivation of the TCA cycle are responsible for the survival and death, respectively. Exogenous malate boosts the TCA cycle to regulate the expression of innate immunity genes via taurine and thereby enhances the survival of zebrafish to the V. alginolyticus infection (20, 27). However, exogenous glucose enhances tilapia against Edwardsiella tarda infection in association with the attenuation of the TCA cycle (24). Therefore, the role of the TCA in bacterial infection can be an ideal breakthrough point to understand the metabolic modulation strategy to the infection.
The present study further showed that differential innate immune responses were detected between 18°C and 33°C, which were consistent with the differential metabolomes between the two temperatures. These findings motivated us to hypothesize that the innate immune response is regulated by a crucial metabolite identified from the differential metabolomes. Therefore, the effect of myo-inositol on the expression of innate immune genes was explored. Exogenous myo-inositol modulated the expression of the most measured genes. These results indicate that the metabolic state is closely related to the innate immune state and a crucial metabolite can restore the host’s anti-infective innate immune response (30, 38–40).
A line of studies has indicated that myo-inositol is crucial in several metabolic and regulatory processes, such as second messenger, lipid signaling, osmolarity, immunomodulation, glucose, and insulin metabolism (32, 41–44). Exogenous myo-inositol appears to be a valuable alternative for treatment of several diseases as well as for improvements in metabolic performance (29, 45, 46). However, information regarding action of myo-inositol against bacterial infection at the higher water temperature is not defined. The present study reveals the previously unknown function of myo-inositol in elevating anti-infective ability at the higher temperature. Recently, our group has shown that myo-inositol improves the host’s ability to eliminate balofloxacin-resistant Escherichia coli, which is related to promotion of the phosphorylation of Akt1 (47). Other authors indicate that myo-inositol is involved in signaling the immune response through phosphorylation (41, 42). The present study identified that not only myo-inositol but also myo-inositol-1-phosphate and myo-inositol-2-phosphate were also significantly reduced at higher water temperature. Therefore, the phosphorylation of myo-inositol is a clue to further understand the mechanisms by which myo-inositol restores the ability against bacterial infection at the higher temperature.
The present study investigated 10 innate immune genes, which are categorized to β-trefoil cytokines (il-1b), type I α helical cytokines (il-6, il-10), chemokine (il-8), TLR family (tlr 1), cyclooxygenase 2 (cox 2), and β-jellyroll cytokines (tnf-α) (48). Among the 10 innate immune genes detected, the expression was elevated with time postinfection at 18°C and 33°C. However, higher il-1b, il-6, il-8, il-10, and and lysozyme C and lower cox 2 were measured at 33°C than at 18°C, where il-1b, il-6, il-8, and il-10 levels were attenuated and cox 2 and lysozyme C levels were increased by exogenous myo-inositol. In addition, the elevated tlr-1 was reverted by exogenous myo-inositol. These results are acccompanied with the increased survival of tilapias infected by A. sobria and treated by myo-inositol at 33°C and 18°C, suggesting that myo-inositol regulation to innate immune response is related to the tilapia survival that is associated with water temperature. In addition, juvenile tilapias were used in the present study. This is because juvenile tilapias with an immature immune system are extremely vulnerable to infection, which causes huge economic losses and thereby requires to be investigated for solution (49–51). Our finding on exogenous myo-inositol modulation to elevate survival provides an effective approach to cope with bacterial infection in juvenile tilapias.
In summary, the present study explores the relationship among water temperature, metabolic state, and innate immune response against bacterial infection. Our results showed that lower survival of tilapia infected with A. sobria at 33°C than 18°C is related to a respective metabolome and innate immune response. Furthermore, there is a close link between the metabolome and the innate immune response, i.e., higher-temperature metabolome and lower-temperature metabolome decide strong and weak innate immune responses, respectively. Interestingly, exogenous myo-inositol restores the reasonable innate immune response against bacterial infection at the higher and lower water temperature. These findings highlight the way in eliminating bacterial pathogens by metabolic modulation.
The raw data supporting the conclusions of this article will be made available by the authors, without undue reservation.
The animal study was reviewed and approved by the Institutional Animal Care and Use Committee of Sun Yat-sen University. Written informed consent was obtained from the owners for the participation of their animals in this study.
HL conceptualized and designed the project. M-JY and MJ performed the experiments and data analysis. HL and X-XP interpreted the data. HL wrote the manuscript. All the authors reviewed the manuscript. All authors contributed to the article and approved the submitted version.
This work was sponsored by grants from the Guangzhou Science and Technology Project (201904020042), the Fellowship of China Postdoctoral Science Foundation (2020M683023, 2020TQ0368), the International Exchanges Scheme (NSFC-RS) (319115301830), and the Innovation Group Project of Southern Marine Science and Engineering Guangdong Laboratory (Zhuhai) (311021006).
The authors declare that the research was conducted in the absence of any commercial or financial relationships that could be construed as a potential conflict of interest.
All claims expressed in this article are solely those of the authors and do not necessarily represent those of their affiliated organizations, or those of the publisher, the editors and the reviewers. Any product that may be evaluated in this article, or claim that may be made by its manufacturer, is not guaranteed or endorsed by the publisher.
1. Tacon AG, Metian M. Global Overview on the Use of Fish Meal and Fish Oil in Industrially Compounded Aquafeeds: Trends and Future Prospects. Aquaculture (2008) 285:146–58. doi: 10.1016/j.aquaculture.2008.08.015
2. Hai NV. Research Findings From the Use of Probiotics in Tilapia Aquaculture: A Review. Fish Shellfish Immunol (2015) 45:592–7. doi: 10.1016/j.fsi.2015.05.026
3. Kevin C, Bromage NR. Reproductive Physiology of Female Tilapia Broodstock. Rev Fish Biol Fisher (2000) 10:1–25. doi: 10.1023/A:1008942318272
4. Lèveque C. Out of Africa: The Success Story of Tilapias. Environ Biol Fish (2002) 64:461–4. doi: 10.1023/A:1016190529697
5. Wang YQ, Wang XY, Ali F, Li ZQ, Fu YY, Yang XJ, et al. Comparative Extracellular Proteomics of Aeromonas Hydrophila Reveals Iron-Regulated Secreted Proteins as Potential Vaccine Candidates. Front Immunol (2019) 10:256. doi: 10.3389/fimmu.2019.00256
6. Pridgeon JW, Klesius PH. Major Bacterial Diseases in Aquaculture and Their Vaccine Development. CAB Rev (2012) 7:48. doi: 10.1079/PAVSNNR20127048
7. Shapiro RS, Cowen LE. Thermo Control of Microbial Development and Virulence: Molecular Mechanism of Microbial Temperature Sensing. mBio (2012) 3:e00238–12. doi: 10.1128/mBio.00238-12
8. Ndong D, Chen YY, Lin YH, Vaseeharan B, Chen JC. The Immune Response of Tilapia Oreochromis Mossambicus and its Susceptibility to Streptococcus Iniae Under Stress in Low and High Temperatures. Fish Shellfish Immunol (2007) 22:686–94. doi: 10.1016/j.fsi.2006.08.015
9. Soto E, Abrams SB, Revan F. Effects of Temperature and Salt Concentration on Francisella Noatunensis Subsp. Orientalis Infections in Nile Tilapia Oreochromis Niloticus. Dis Aquat Organ (2012) 101:217–23. doi: 10.3354/dao02533
10. Kayansamruaj P, Pirarat N, Hirono I, Rodkhum C. Increasing of Temperature Induces Pathogenicity of Streptococcus Agalactiae and the Up-Regulation of Inflammatory Related Genes in Infected Nile Tilapia (Oreochromis Niloticus). Vet Microbiol (2014) 172:265–71. doi: 10.1016/j.vetmic.2014.04.013
11. Zhao XL, Han Y, Ren ST, Ma YM, Li H. Peng XX. L-Proline Increases Survival of Tilapias Infected by Streptococcus Agalactiae in Higher Water Temperature. Fish Shellfish Immunol (2015) 44:33–42. doi: 10.1016/j.fsi.2015.01.025
12. Tavares GC, Carvalho AF, Pereira FL, Rezende CP, Azevedo VAC, Leal CAG, et al. Transcriptome and Proteome of Fish-Pathogenic Streptococcus Agalactiae are Modulated by Temperature. Front Microbiol (2018) 9:2639. doi: 10.3389/fmicb.2018.02639
13. Jiang M, Chen ZG, Zheng J, Peng B. Metabolites-Enabled Survival of Crucian Carps Infected by Edwardsiella Tarda in High Water Temperature. Front Immunol (2019) 10:1991. doi: 10.3389/fimmu.2019.01991
14. Martins ML, Xu DH, Shoemaker CA, Klesius PH. Temperature Effects on Immune Response and Hematological Parameters of Channel Catfish Ictalurus Punctatus Vaccinated With Live Theronts of Ichthyophthirius Multifiliis. Fish Shellfish Immunol (2011) 31:774–80. doi: 10.1016/j.fsi.2011.07.015
15. Soto E, Brown N, Gardenfors ZO, Yount S, Revan F, Francis S, et al. Effect of Size and Temperature at Vaccination on Immunization and Protection Conferred by a Live Attenuated Francisella Noatunensis Immersion Vaccine in Red Hybrid Tilapia. Fish Shellfish Immunol (2014) 41:593–9. doi: 10.1016/j.fsi.2014.10.009
16. Erkinharju T, Dalmo RA, Vågsnes Ø, Hordvik I, Seternes T. Vaccination of Atlantic Lumpfish (Cyclopterus Lumpus L.) at a Low Temperature Leads to a Low Antibody Response Against Aeromonas Salmonicida. J Fish Dis (2018) 41:613–23. doi: 10.1111/jfd.12760
17. Boltana S, Aguilar A, Sanhueza N, Donoso A, Mercado L, Imarai M, et al. Behavioral Fever Drives Epigenetic Modulation of the Immune Response in Fish. Front Immunol (2018) 9:1241. doi: 10.3389/fimmu.2018.01241
18. Li Y, Cai SH. Identification and Pathogenicity of Aeromonas Sobria on Tail-Rot Disease in Juvenile Tilapia Oreochromis Niloticus. Curr Microbiol (2011) 62:623–7. doi: 10.1007/s00284-010-9753-8
19. Peng B, Su YB, Li H, Han Y, Guo C, Tian YM, et al. Exogenous Alanine or/and Glucose Plus Kanamycin Kills Antibiotic-Resistant Bacteria. Cell Metab (2015) 21:249–61. doi: 10.1016/j.cmet.2015.01.008
20. Yang MJ, Cheng ZX, Jiang M, Zeng ZH, Peng B, Peng XX, et al. Boosted TCA Cycle Enhances Survival of Zebrafish to Vibrio Alginolyticus Infection. Virulence (2018) 9:634–44. doi: 10.1080/21505594.2017.1423188
21. Cheng ZX, Guo C, Chen ZG, Yang TC, Zhang JY, Wang J, et al. Glycine, Serine and Threonine Metabolism Confounds Efficacy of Complement-Mediated Killing. Nat Commun (2019) 10:3325. doi: 10.1038/s41467-019-11129-5
22. Li L, Su YB, Peng B, Peng XX, Li H. Metabolic Mechanism of Colistin Resistance and its Reverting in Vibrio Alginolyticus. Environ Microbiol (2020) 22(10):4295–313. doi: 10.1111/1462-2920.15021
23. Chen XH, Liu SR, Peng B, Li D, Cheng ZX, Zhu JX, et al. Exogenous L-Valine Promotes Phagocytosis to Kill Multidrug-Resistant Bacterial Pathogens. Front Immunol (2017) 8:207. doi: 10.3389/fimmu.2017.00207
24. Zeng ZH, Du CC, Liu SR, Li H, Peng XX, Peng B. Glucose Enhances Tilapia Against Edwardsiella Tarda Infection Through Metabolome Reprogramming. Fish Shellfish Immunol (2017) 61:34–43. doi: 10.1016/j.fsi.2016.12.010
25. Su YB, Peng B, Li H, Cheng ZX, Zhang TT, Zhu JX, et al. The Pyruvate Cycle Increases Aminoglycosides Efficacy and Provides Respiratory Energy in Bacteria. Proc Natl Acad Sci USA (2018) 115:E1578–87. doi: 10.1073/pnas.1714645115
26. Zhang S, Wang J, Jiang M, Xu D, Peng B, Peng XX, et al. Reduced Redox-Dependent Mechanism and Glucose-Mediated Reversal in Gentamicin-Resistant Vibrio Alginolyticus. Environ Microbiol (2019) 21:4724–39. doi: 10.1111/1462-2920.14811
27. Yang MJ, Xu D, Yang DX, Yang DX, Li L, Peng XX, et al. Malate Enhances Survival of Zebrafish Against Vibrio Alginolyticus Infection in the Same Manner as Taurine. Virulence (2020) 11:349–64. doi: 10.1080/21505594.2020.1750123
28. Su YB, Kuang SF, Ye JZ, Tao JJ, Li H, Peng XX, et al. Enhanced Biosynthesis of Fatty Acids is Associated With the Acquisition of Ciprofloxacin Resistance in Edwardsiella Tarda. mSystems (2021) 6:e00694–21. doi: 10.1128/mSystems.00694-21
29. Gonzalez-Uarquin F, Kenéz Á, Rodehutscord M, Huber K. Dietary Phytase and Myo-Inositol Supplementation Are Associated With Distinct Plasma Metabolome Profile in Broiler Chickens. Animal (2020) 14:549–59. doi: 10.1017/S1751731119002337
30. Dar GH, Dar SA, Kamili AN, Chishti MZ, Ahmad F. Detection and Characterization of Potentially Pathogenic Aeromonas Sobria Isolated From Fish Hypophthalmichthys Molitrix (Cypriniformes: Cyprinidae). Microb Pathog (2016) 91:136–40. doi: 10.1016/j.micpath.2015.10.017
31. Majtán J, Cerny J, Ofúkaná A, Takáč P, Kozánek M. Mortality of Therapeutic Fish Garra Rufa Caused by Aeromonas Sobria. Asian Pac J Trop Biomed (2012) 2:85–7. doi: 10.1016/S2221-1691(11)60197-4
32. Jiang M, Kuang SF, Lai SS, Zhang S, Yang J, Peng B, et al. Na+-NQR Confers Aminoglycoside Resistance via the Regulation of L-Alanine Metabolism. mBio (2020) 11:e02086–20. doi: 10.1128/mBio.02086-20
33. Tyan ML. Modulation of the Antibody Response to Sheep Red Blood Cells in Normal and Immunodeficient XID Mice by Myo-Inositol. Proc Soc Exp Biol Med (1997) 215:258–63. doi: 10.3181/00379727-215-44136
34. Chong J, Soufan O, Li C, Caraus I, Li S, Bourque G, et al. MetaboAnalyst 4.0: Towards More Transparent and Integrative Metabolomics Analysis. Nucleic Acids Res (2018) 46:W486–94. doi: 10.1093/nar/gky310
35. Yamada T, Letunic I, Okuda S, Kanehisa M, Bork P. Ipath2.0: Interactive Pathway Explorer. Nucleic Acids Res (2011) 39:W412–5. doi: 10.1093/nar/gkr313
36. Wohlfarth GW, Hulata GI. Applied Genetics of Tilapias. 2nd edition. Manila (Philippines): ICLARM Studies and Reviews 6, International Center for Living Aquatic Resources Management (1983).
37. Jiang M, Yang LF, Zeng J, Chen ZG, Peng B. Maltose Promotes Crucian Carp Survival Against Aeromonas Sobrial Infection at High Temperature. Virulence (2020) 11:877–88. doi: 10.1080/21505594.2020.1787604
38. Gong QY, Yang DX, Jiang M, Zheng JG, Peng B. L-Aspartic Acid Promotes Fish Survival Against Vibrio Alginolyticus Infection Through Nitric Oxide-Induced Phagocytosis. Fish Shellfish Immunol (2019) 97:359–66. doi: 10.1016/j.fsi.2019.12.061
39. Gong QY, Yang MJ, Yang LF, Chen ZG, Jiang M, Peng B. Metabolic Modulation of Redox State Confounds Fish Survival Against Vibrio Alginolyticus Infection. Microb Biotechnol (2020) 13:796–812. doi: 10.1111/1751-7915.13553
40. Yang DX, Yang MJ, Yin Y, Kou TS, Peng LT, Chen ZG, et al. Serine Metabolism Tunes Immune Responses to Promote Oreochromis Niloticus Survival Upon Edwardsiella Tarda Infection. mSystems (2021) 6:e00426–21. doi: 10.1128/mSystems.00426-21
41. Zhu L, Jones C, Zhang G. The Role of Phospholipase C Signaling in Macrophage-Mediated Inflammatory Response. J Immunol Res (2018) 2018:5201759. doi: 10.1155/2018/5201759
42. Dong H, Adams NM, Xu Y, Cao J, Allan DSJ, Carlyle JR, et al. The IRE1 Endoplasmic Reticulum Stress Sensor Activates Natural Killer Cell Immunity in Part by Regulating C-Myc. Nat Immunol (2019) 20:865–78. doi: 10.1038/s41590-019-0388-z
43. Kim W, Kim E, Min H, Kim MG, Eisenbeis VB, Dutta AK, et al. Inositol Polyphosphates Promote T Cell-Independent Humoral Immunity via the Regulation of Bruton’s Tyrosine Kinase. Proc Natl Acad Sci USA (2019) 116:12952–7. doi: 10.1073/pnas.1821552116
44. de Paepe B, Merckx C, Jarošová J, Cannizzaro M, de Bleecker JL. Myo-Inositol Transporter SLC5A3 Associates With Degenerative Changes and Inflammation in Sporadic Inclusion Body Myositis. Biomolecules (2020) 10:521. doi: 10.3390/biom10040521
45. Troisi J, Cinque C, Giugliano L, Symes S, Richards S, Adair D, et al. Metabolomic Change Due to Combined Treatment With Myo-Inositol, D-Chiro-Inositol and Glucomannan in Polycystic Ovarian Syndrome Patients: A Pilot Study. J Ovarian Res (2019) 12:25. doi: 10.1186/s13048-019-0500-x
46. Wojciechowska A, Osowski A, Jóźwik M, Górecki R, Rynkiewicz A, Wojtkiewicz J. Inositols’ Importance in the Improvement of the Endocrine-Metabolic Profile in PCOS. Int J Mol Sci (2019) 20:5787. doi: 10.3390/ijms20225787
47. Chen XH, Zhang BW, Li H, Peng XX. Myo-Inositol Improves the Host’s Ability to Eliminate Balofloxacin-Resistant Escherichia Coli. Sci Rep (2015) 5:10720. doi: 10.1038/srep10720
48. Zou J, Secombes CJ. The Function of Fish Cytokines. Biol (2016) 5:E23. doi: 10.3390/biology5020023
49. Liu W, Ran C, Liu Z, Gao Q, Xu S, Ringø E, et al. Effects of Dietary Lactobacillus Plantarum and AHL Lactonase on the Control of Aeromonas Hydrophila Infection in Tilapia. MicrobiologyOpen (2016) 5:687–99. doi: 10.1002/mbo3.362
50. Liu W, Ren P, He S, Xu L, Yang Y, Gu Z, et al. Comparison of Adhesive Gut Bacteria Composition, Immunity, and Disease Resistance in Juvenile Hybrid Tilapia Fed Two Different Lactobacillus Strains. Fish Shellfish Immunol (2013) 35:54–62. doi: 10.1016/j.fsi.2013.04.010
Keywords: water temperature, bacterial infection, Aeromonas sobria, myo-inositol, metabolome, innate immunity
Citation: Yang M-j, Jiang M, Peng X-x and Li H (2021) Myo-Inositol Restores Tilapia’s Ability Against Infection by Aeromonas sobria in Higher Water Temperature. Front. Immunol. 12:682724. doi: 10.3389/fimmu.2021.682724
Received: 19 March 2021; Accepted: 23 August 2021;
Published: 10 September 2021.
Edited by:
Arturo Bevilacqua, Sapienza University of Rome, ItalyReviewed by:
Xianyong Bu, East China Normal University, ChinaCopyright © 2021 Yang, Jiang, Peng and Li. This is an open-access article distributed under the terms of the Creative Commons Attribution License (CC BY). The use, distribution or reproduction in other forums is permitted, provided the original author(s) and the copyright owner(s) are credited and that the original publication in this journal is cited, in accordance with accepted academic practice. No use, distribution or reproduction is permitted which does not comply with these terms.
*Correspondence: Hui Li, bGlodWkzMkBzeXN1LmVkdS5jbg==
†These authors have contributed equally to this work
Disclaimer: All claims expressed in this article are solely those of the authors and do not necessarily represent those of their affiliated organizations, or those of the publisher, the editors and the reviewers. Any product that may be evaluated in this article or claim that may be made by its manufacturer is not guaranteed or endorsed by the publisher.
Research integrity at Frontiers
Learn more about the work of our research integrity team to safeguard the quality of each article we publish.