- 1State Key Laboratory of Agricultural Microbiology, College of Veterinary Medicine, Huazhong Agricultural University, Wuhan, China
- 2James L. Winkle College of Pharmacy University of Cincinnati, Cincinnati, OH, United States
Endocannabinoids are endogenous ligands of cannabinoid receptors and activation of these receptors has strong physiological and pathological significance. Structurally, endocannabinoids are esters (e.g., 2-arachidonoylglycerol, 2-AG) or amides (e.g., N-arachidonoylethanolamine, AEA). Hydrolysis of these compounds yields arachidonic acid (AA), a major precursor of proinflammatory mediators such as prostaglandin E2. Carboxylesterases are known to hydrolyze esters and amides with high efficiency. CES1, a human carboxylesterase, has been shown to hydrolyze 2-AG, and shares a high sequence identity with pig carboxylesterases: PLE1 and PLE6 (pig liver esterase). The present study was designed to test the hypothesis that PLE1 and PLE6 hydrolyze endocannabinoids and promote inflammatory response. Consistent with the hypothesis, purified PLE1 and PLE6 efficaciously hydrolyzed 2-AG and AEA. PLE6 was 40-fold and 3-fold as active as PLE1 towards 2-AG and AEA, respectively. In addition, both PLE1 and PLE6 were highly sensitive to bis(4-nitrophenyl) phosphate (BNPP), an aryl phosphodiester known to predominately inhibit carboxylesterases. Based on the study with BNPP, PLEs contributed to the hydrolysis of 2-AG by 53.4 to 88.4% among various organs and cells. Critically, exogenous addition or transfection of PLE6 increased the expression and secretion of proinflammatory cytokines in response to the immunostimulant lipopolysaccharide (LPS). This increase was recapitulated in cocultured alveolar macrophages and PLE6 transfected cells in transwells. Finally, BNPP reduced inflammation trigged by LPS accompanied by reduced formation of AA and proinflammatory mediators. These findings define an innovative connection: PLE-endocannabinoid-inflammation. This mechanistic connection signifies critical roles of carboxylesterases in pathophysiological processes related to the metabolism of endocannabinoids.
Introduction
Carboxylesterases (E.C.3.1.1.1) constitute a class of enzymes critical in drug metabolism, detoxification and lipid mobilization (1–4). These enzymes rapidly hydrolyze carboxylic acid esters, amides and thioesters (2). Carboxylesterases split a drug into two parts and cause large changes in structure, lipophilicity or both (2). As a result, hydrolysis determines the efficacy and toxicity of drugs metabolized by these enzymes. For example, the antiplatelet agent clopidogrel undergoes hydrolysis and its hydrolytic metabolite no longer has antiplatelet activity (5, 6). In contrast, the anti-influenza viral agent oseltamivir requires hydrolysis to exert therapeutic activity (7). Furthermore, hydrolysis of clopidogrel represents detoxification and the opposite is true with oseltamivir (6, 7). In addition to hydrolysis-based detoxification, carboxylesterases interact covalently with organophosphates stoichiometrically (2). Such interactions reduce the amount of organophosphates, which otherwise interact with acetylcholineesterase and cause toxicity.
Carboxylesterases belong to the superfamily of α/β fold hydrolases (8, 9). This superfamily includes structurally similar enzymes such as lipases. For many years, carboxylesterases have been recognized to have lipase activity (10–18). Indeed, several carboxylesterases have been shown to hydrolyze triglycerides and cholesterol esters (11, 12). While hydrolysis of lipids is considered to favor lipid elimination, we and others have shown that overexpression of carboxylesterases leads to lipid accumulation instead (19–22). It has been assumed that the action of carboxylesterases participates in remodeling lipid droplets, favoring lipid retentions (20). In addition, hydrolysis of lipid compounds has been implicated in signal transduction, notably endocannabinoids (23–25): 2-arachidonoylglycerol (2-AG) and N-arachidonoylethanolamine (AEA). Those are two major endogenous ligands of cannabinoid receptors and activation of these receptors exerts a variety of biological activities in a wide range of organs such as the brain, liver, spleen, lungs, and small intestine (26).
AEA and 2-AG are traditionally established to be hydrolyzed by monoacylglycerol lipase (MAGL), fatty acid amide hydrolase and α/β fold hydrolase 6/12 (ABHD6/12) (27, 28). However, several studies have demonstrated a strong involvement of carboxylesterases in their hydrolysis. In human THP1 cells (a monocytic cell line), MAGL and carboxylesterases contribute 32-40% and 40-50% to the hydrolysis of 2-AG (29, 30), respectively. The involvement of carboxylesterases is implicated in mice (30). Hydrolysis of these endocannabinoids leads to the formation of arachidonic acid (AA). This metabolite undergoes oxidation by cyclooxygenase 2 and is converted into prostaglandins (PGs), potent proinflammatory mediators (30–32). These findings suggest that carboxylesterases play critical roles in inflammatory responses.
All mammalian species studied, without exceptions, express multiple carboxylesterases. However, the number of carboxylesterase genes varies greatly from one species to another. In rodents such as mouse, as many as 20 genes are described (1). In contrast, the human genome has only seven including a pseudogene (1). Nevertheless, two human carboxylesterases, CES1 and CES2, are recognized to play major roles in drug metabolism and nutrient processing (1, 2). CES1 is encoded by two highly identical genes (1A1 and 1A2) (1, 33, 34) whereas CES2, on the other hand, is encoded by a single gene with several alternative splicing variants (35). Based on cDNA cloning-sequencing analysis, pigs express a few carboxylesterases (pig liver esterase, PLE) (36). However, the genomic basis for the multiplicity remains to be established. PLE1 and PLE6 share a high sequence identity with CES1, a human carboxylesterase that efficaciously hydrolyzes 2-AG (36, 37).
Pigs are increasingly important animals for modeling human pathophysiological conditions (38). Critically for this study, the pig immune system resembles man for over 80% in contrast to rodents with only about 10% in terms of anatomy and functions (38). For example, pigs and humans but not rodents have tonsils (38). The aim of the present study was to test the hypothesis that PLE1 and PLE6 efficaciously hydrolyze major endocannabinoids with immunostimulating activity. As expected, both PLE1 and PLE6 hydrolyzed 2-AG and AEA with high efficiency. In addition, both carboxylesterases were highly sensitive to bis(4-nitrophenyl) phosphate (BNPP), an established serine enzyme inhibitor predominately toward carboxylesterases (39–41). Based on the inhibitory study with BNPP, PLEs contributed to the hydrolysis of 2-AG by 33.1 to 88.4% among various organs and cells. To ascertain the functional significance of the hydrolysis, exogenous addition or transfection of PLE6 increased the expression and secretion of proinflammatory cytokines when cells were treated with the immunostimulant lipopolysaccharide (LPS). This increase was recapitulated in both in vitro and in vivo experiments. These findings define an innovative connection: PLE-endocannabinoid-inflammation. This mechanistic connection signifies critical roles of carboxylesterases in important pathophysiological processes related to the metabolism of endocannabinoids.
Results
Hydrolysis of Major Endocannabinoids by PLE1 and PLE6
PLE1 and PLE6 are abundant pig carboxylesterases and have been shown to hydrolyze foreign compounds such as amoxicillin (42). This study was performed to test whether these enzymes hydrolyze endogenous signaling compounds such as endocannabinoids (Figure 1A), essential molecular species in the endocannabinoid system (26–28). To test this possibility, PLE1 and PLE6 were expressed in a heterologous system and purified to homogeneity as described in our previous publications (36, 42). The homogeneity was confirmed by SDS-PAGE (sodium dodecyl sulfate-polyacrylamide gel electrophoresis) followed by Coomassie blue staining (Figures S1A, 1B). Western blotting, with purified PLE1 and PLE6 as the standards, showed that PLEs were highly abundant in the liver S9 fractions (Figure S1C).
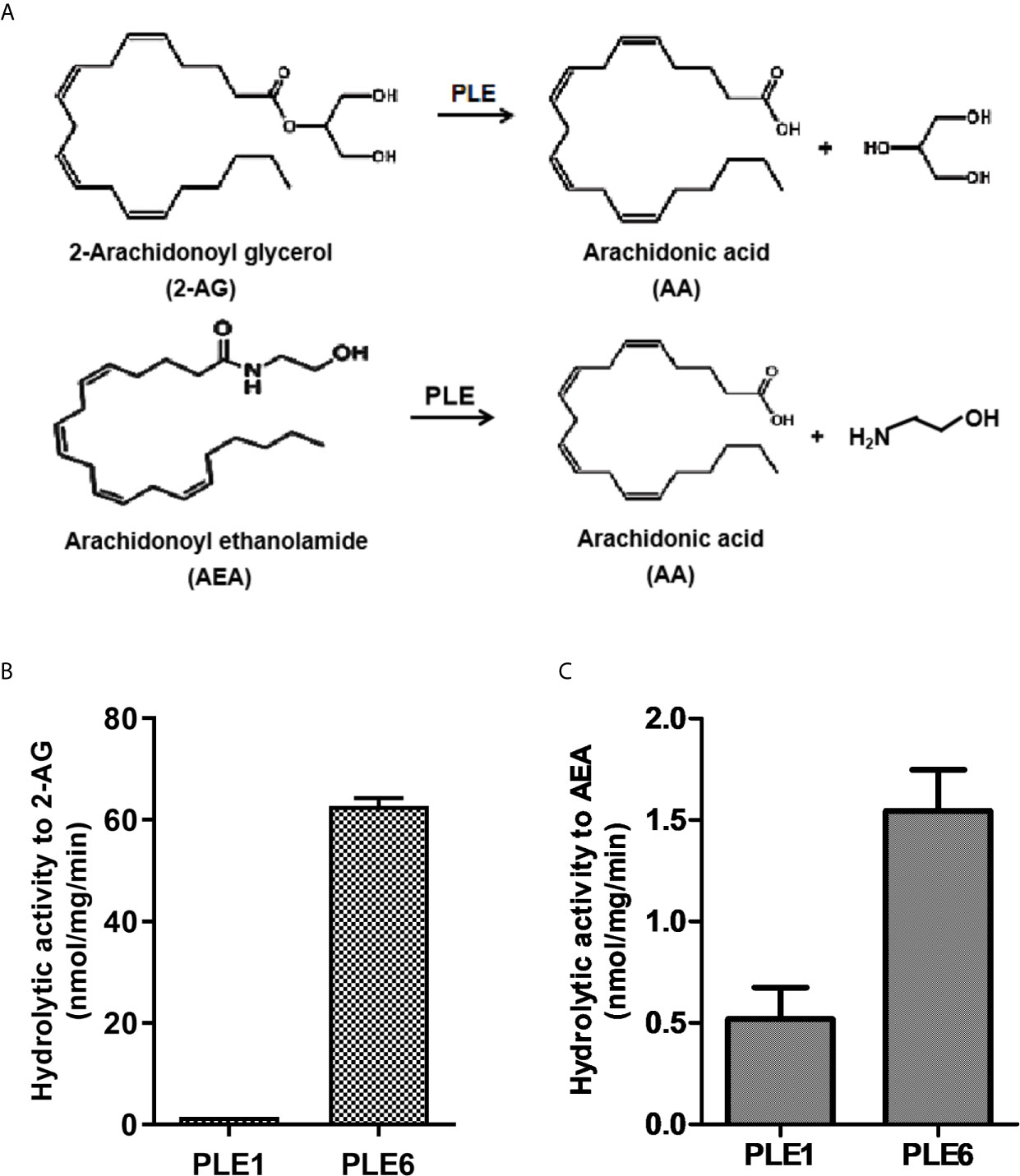
Figure 1 Hydrolysis of 2-AG and AEA by purified PLE1 and PLE6. (A) Reaction diagram for 2-AG and AEA hydrolysis. (B) Hydrolysis of 2-AG. (C) Hydrolysis of AEA. Substrates (2-AG or AEA) at 200 µM was prepared in Tris-HCl buffer (50 mM, pH 7.4) and then mixed with purified PLE1 or PLE6 (10 µg). After incubation for 10 min at 37°C, the reactions were terminated, and the formation of AA was monitored with LC-MS/MS. The data in Figures 1B and C are presented as the mean ± standard error of the mean (SEM) of 3 independent experiments.
To gain catalytic insight, purified PLE1 and PLE6 were tested for the hydrolysis of the standard substrate p-nitrophenylacetate (p-NPA) and two major endocannabinoids: 2-AG and AEA (Figures S1 and 1). As shown in Figures S1D, E, both PLE1 and PLE6 hydrolyzed p-NPA with a comparable specific activity: 1.70 and 2.13 µmol/mg/min, respectively. Liver S9 fractions had a specific rate of 5.98 µmol/mg/min (Figure S1E), suggesting that PLE1 and PLE6 together represented the major hydrolytic activity in the liver towards p-NPA. Likewise, both PLE1 and PLE6 hydrolyzed 2-AG and AEA (Figures 1B, C). However, the relative specific rates varied greatly depending on an enzyme and a substrate. PLE1 had a specific rate of 1.56 nmol/mg/min, while PLE6 had a rate of 62.70 nmol/mg/min (Figure 1B), representing a 40-fold difference. Similarly, both PLE1 and PLE6 hydrolyzed AEA. PLE1 had a specific rate of 0.52 nmol/mg/min whereas PLE6 had 1.54 nmol/mg/min (Figure 1C). Overall, 2-AG was a better substrate for both enzymes (Figures 1B, C).
Next we tested whether PLE1 and PLE6 differ in the sensitivity towards BNPP, an aryl phosphodiester known to inhibit carboxylesterases with high efficacy (39–41). As shown in Figure S2, BNPP caused a concentration-dependent inhibition of both PLE1 and PLE6. However, the relative sensitivity varied considerably. PLE6 had an estimated IC50 value of 10 µM, whereas PLE1 had 0.1 µM (Figure S2A), representing a 100-fold difference in the relative sensitivity. However, comparable inhibitions of both enzymes were detected with higher concentrations of BNPP. For example, BNPP at 100 µM inhibited PLE6 by 66.0% and PLE1 by 75.4%, respectively (Figure S2A). As expected, liver S9 fractions hydrolyzed both 2-AG (2.94 nmol/mg/min) and AEA (0.20 nmol/mg/min). The hydrolysis of 2-AG and AEA by liver S9 fractions was inhibited significantly by BNPP (Figure S2B) with 2-AG being inhibited by 53.4% whereas AEA by 75.0%. These results suggest that PLE1 and PLE6 are major hepatic enzymes in hydrolyzing these endocannabinoids. Finally, BNPP (0.1-100 µM) caused little cytotoxicity (Figure S2C).
Inverse Regulation of Inflammation by 2-AG and PLE
AEA and 2-AG, 2-AG in particular, are two major molecular species in the cannabinoid signaling and exert a wide range of pathophysiological functions including inflammatory responses (23–26). We next tested potential roles of PLEs in the inflammation. The focus was on PLE6 and 2-AG based on the high relative activity of this enzyme toward this cannabinoid (Figure 1B). Two types of primary cells were used: pig alveolar macrophages (PAMs) and pig hepatocytes (PHCs). PAMs have strong immune significance, whereas PHCs have strong metabolizing functions, although both types of cells share these functions (43–46). As shown in Figures S3A, B, primary cells with high purity were isolated and cultured. Western blotting detected abundant expression of PLEs in both PAMs and PHCs with the latter expressing to a much greater extent (Figure S3C). Critically, homogenates of PAMs and PHCs effectively hydrolyzed 2-AG at a specific rate of 4.47 nmol/min/mg and 3.25 nmol/min/mg, respectively. BNPP (100 µM) profoundly inhibited 2-AG hydrolysis in both PAM (77.3%) and PHC (88.4%) homogenates (Figures S3D, E).
Next we tested whether the immunostimulant LPS triggers immune response in PAMs and whether the action of PLE6 promotes the response. As expected, LPS greatly upregulated the mRNA expression of multiple proinflammatory cytokines such as IL-1β (interleukin-1β), IL-6 (interleukin-6) and TNF-α (tumor necrosis factor-α) (Figure 2). Importantly, the upregulation was attenuated by 2-AG in a concentration-dependent manner (Figure 2A). To specify whether hydrolysis is involved in the attenuation, 2-AG was pre-incubated with PLE6 for 30 min and the pre-incubation mixtures were tested for the attenuation activity. As shown in Figure 2B, the attenuation was significantly decreased with the pre-incubation mixture (Figure 2B). Actually, the pre-incubation mixtures with lower concentrations of 2-AG (e.g., 1 µM) increased the expression of proinflammatory cytokines (Figure 2B). On the other hand, the pre-incubation mixtures with increased concentrations of 2-AG (e.g., 10 µM for IL-1β) was less effective (Figure 2B). It was likely that the amount of PLE6 and the pre-incubation time used here were not sufficient to completely hydrolyze the amount of 2-AG at higher concentrations. Consistent with this notion, BNPP (100 µM) significantly suppressed LPS-induced expression of proinflammatory cytokines in PAMs (Figure 2C). These results conclude that 2-AG inhibits inflammatory responses in PAMs and hydrolysis of 2-AG by PLE6 reverses the inhibition.
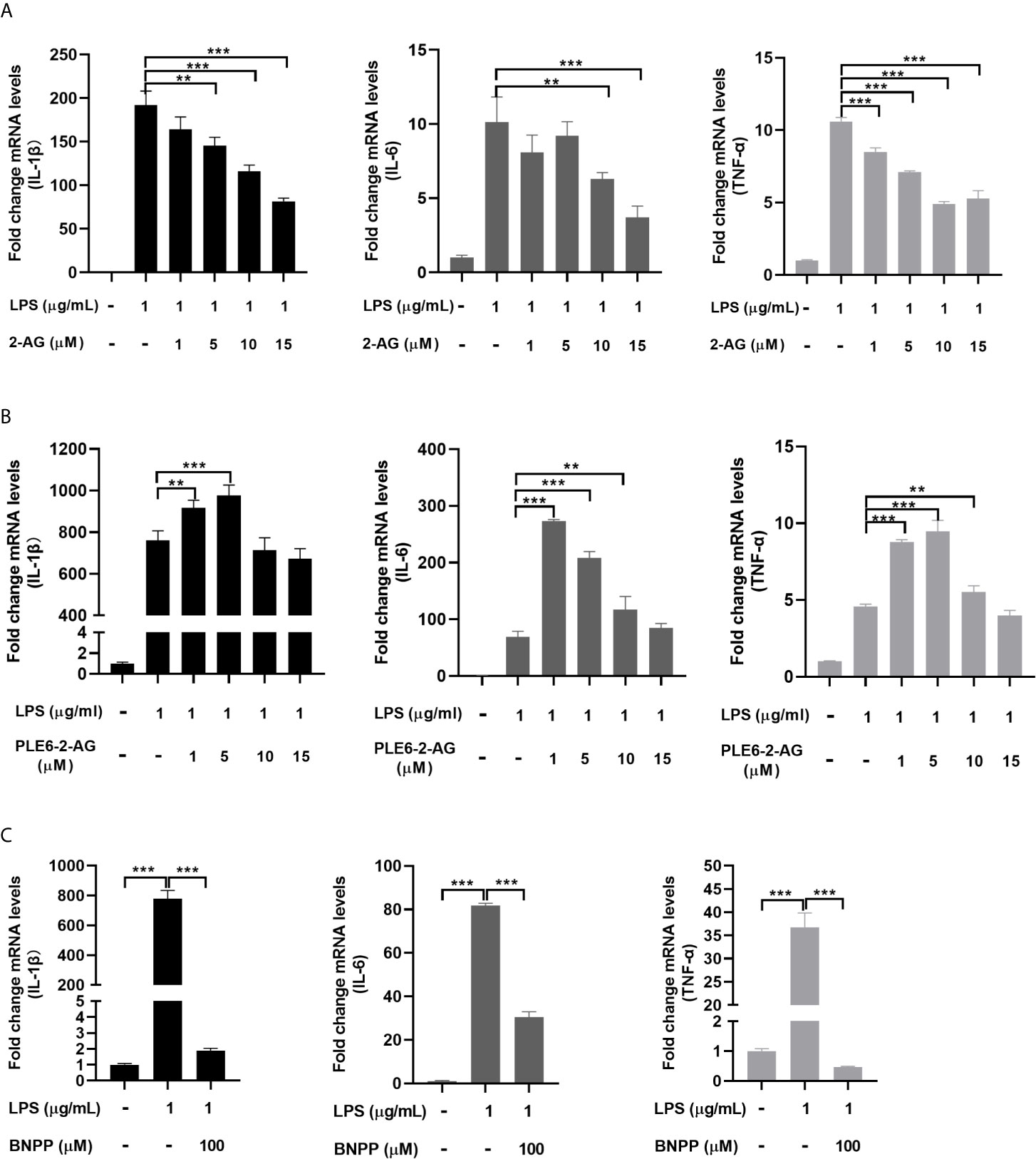
Figure 2 Effect of 2-AG, PLE6-hydrolyzed 2-AG and BNPP on the LPS-stimulated mRNA expression of proinflammatory cytokines in PAMs. (A) Effect of 2-AG on the LPS-stimulated mRNA expression of proinflammatory cytokines. PAMs were cultured and incubated with LPS (1 µg/mL) and 2-AG at various concentration (1 µM, 5 µM, 10 µM, 15 µM) for 24 h. Total RNA was isolated and analyzed for the mRNA level of IL-1β, IL-6 and TNF-α by RT-qPCR. (B) Effect of PLE6 hydrolyzed 2-AG on the LPS-stimulated mRNA expression of proinflammatory cytokines. PAMs were cultured and incubated with LPS (1 µg/mL) and a 0.5-h preincubation mixture of PLE6 with 2-AG at various concentration (1 µM, 5 µM, 10 µM, 15 µM) for 24 h. Total RNA was isolated and analyzed for the mRNA level of IL-1β, IL-6 and TNF-α by RT-qPCR. (C) Effect of BNPP on the LPS-stimulated mRNA expression of proinflammatory cytokines. PAMs were cultured and preincubated with BNPP (100 µM) for 3 h, and then LPS (1 µg/mL) was treated for another 24 h. The levels of inflammatory factors in the cell lysates were detected. Statistical significance was indicated by asterisks (**P < 0.01; ***P < 0.001).
Enhanced Inflammatory Response by PLE1 or PLE6 With Endogenous 2-AG
LPS has been shown by many investigators to induce release of 2-AG (31, 47, 48). Next we tested whether cultured PAMs incubated with purified PLE1 or PLE6 without addition of 2-AG increases the expression of proinflammatory cytokines stimulated by LPS. As shown in Figures 3A, B, both RT-qPCR and protein chip demonstrated that PLE1 and PLE6 further enhanced the levels of IL-1β, IL-6, TNF-α and IL-12 in PAMs. These results suggest that LPS induced the release of 2-AG some of which was secreted into media and hydrolyzed by PLEs, eventually leading to increased expression of inflammatory cytokines.
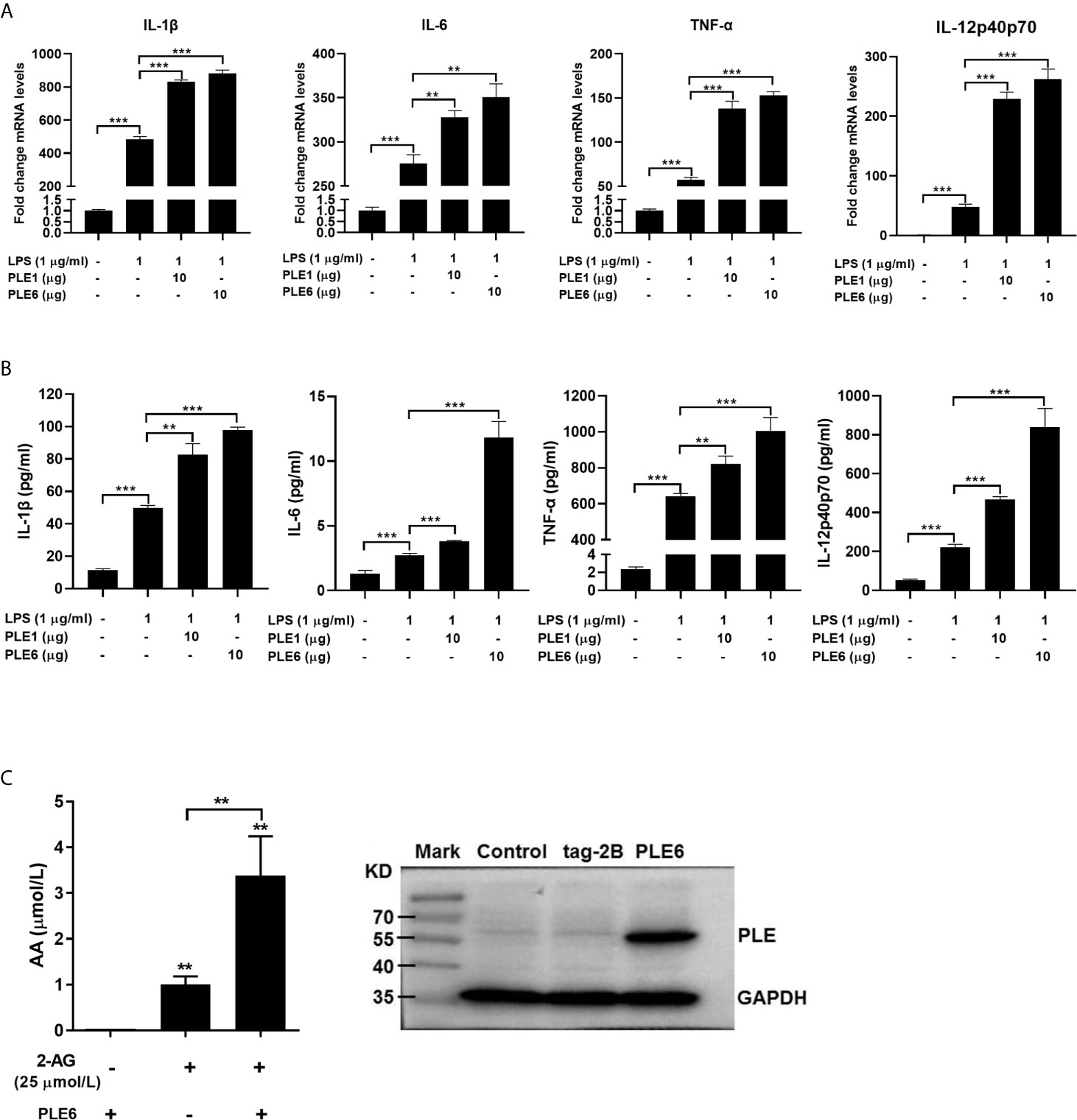
Figure 3 Enhanced LPS-stimulation on the expression of proinflammatory cytokines in PAMs by addition of PLE1/6 or increased AA secretion in transfected PLE6. (A) Enhanced LPS-stimulation on the mRNA expression of proinflammatory cytokines in PAMs by addition of purified PLE1 or PLE6. PAMs were cultured for 24 h, and then treated with LPS (1 µg/ml) and PLE1 or PLE6 (10 µg). After incubation for additional 6 h, total RNA was isolated and analyzed for the mRNA level of proinflammatory cytokines by RT-qPCR. (B) Enhanced LPS-stimulation on the protein expression of proinflammatory cytokines in PAMs. Cells were treated as Experiment A (above). However, culture supernatant was collected and analyzed by protein chip. (C) Increased AA secretion by PLE6 transfected cells. 293T cells were transfected with pCMV-tag-2B-PLE6 or the corresponding vector. The transfected cells were treated with 2-AG (25 µM). After treatment for 1 h, the supernatant was extracted and analyzed for the level of AA by LC-MS/MS (Left). The expression of transfected PLE6 was confirmed by Western blotting (Right). Data are presented as the mean ± SEM of 3 independent experiments. Statistical significance was indicated by asterisks (** P < 0.01; *** P < 0.001).
To complement the experiment with added exogenous PLEs (extracellular), we next tested whether overexpression of PLE6 through transfection increases the hydrolysis of 2-AG. Cells (293T) were transfected to overexpress PLE6, treated with 2-AG and monitored for its hydrolysis. The selection of 293T cells was based on two important considerations: (a) 293T cells support high-levels of transfection efficiency and (b) these cells express little carboxylesterases (49). As shown in Figure 3C (Left), transfection of PLE6 increased the hydrolysis of 2-AG (increased formation of AA) by as many as 3-fold. Transfection indeed resulted in increased expression of PLE6 (Right of Figure 3C). These results conclude that exogenous and endogenous PLE6 both increases 2-AG hydrolysis, signifying enhanced inflammatory response.
Enhanced Proinflammatory Response by PLEs in Coculture Models
The increases of LPS-induced proinflammatory cytokines were further studied with double-layered cocultures. This was of significance as it specifies whether the hydrolytic metabolites by PLEs travels from one compartment to another, signifying distance effect in vivo. As structured in Figure 4A, PAM cells were cultured in the insert whereas the PLE6 transfected cells were seeded at the bottom of the external compartment. As anticipated, transfection of PLE6 enhanced LPS-induced expression of proinflammatory cytokines, and reduced the 2-AG-decreased expression of proinflammatory cytokines (Figures 4B, C). The reductions were also confirmed by BNPP, an PLE6 inhibitor (Figures 4D, S2A). It should be noted that similar patterns of changes were detected on the protein levels of the pro-inflammatory cytokines (Figure 4E).
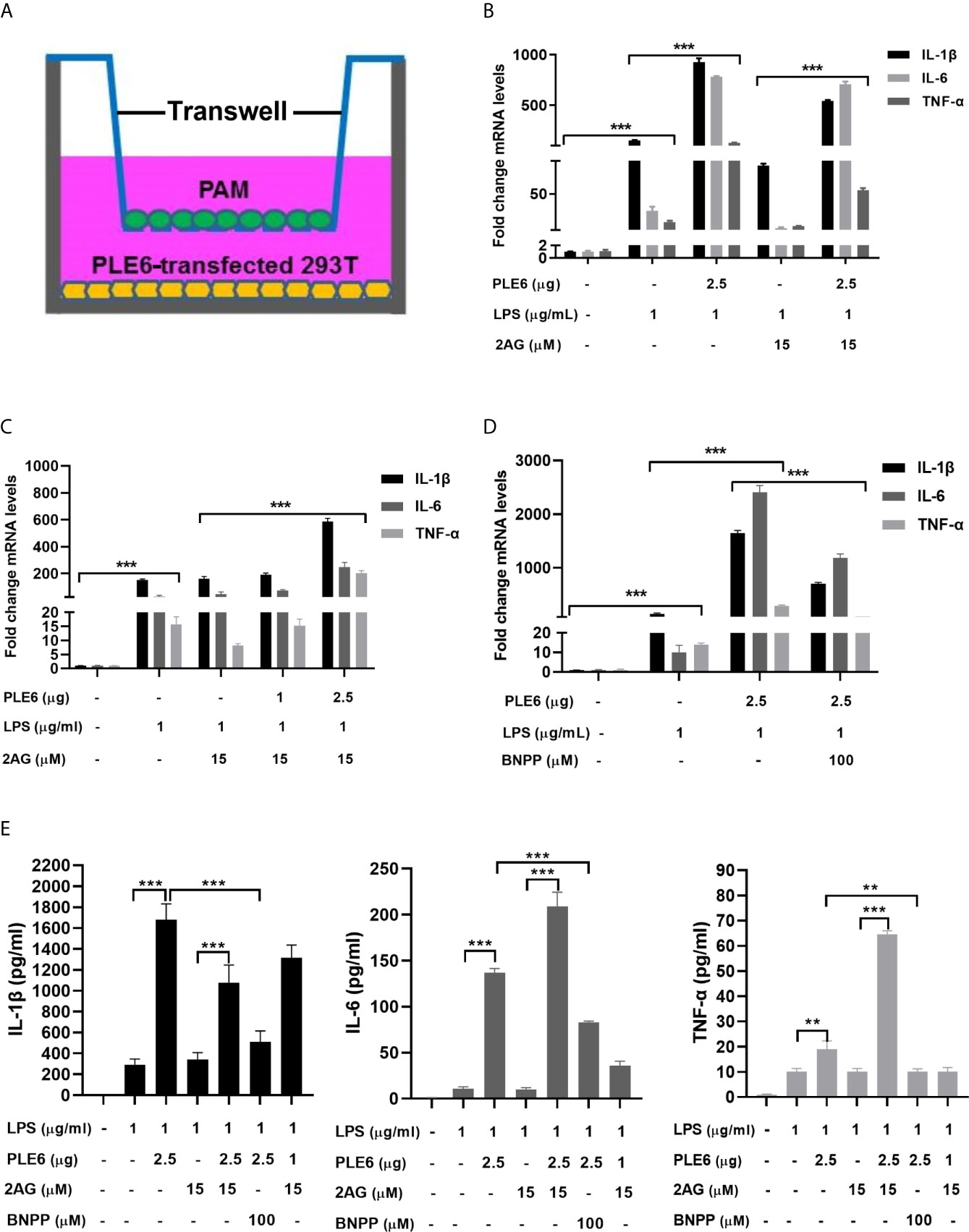
Figure 4 Enhanced LPS-stimulation on the expression of proinflammatory cytokines in coculture model of PAMs and 293T cells. (A) Diagrammatic presentation of the coculture model. (B) Enhanced LPS-stimulation on the expression of proinflammatory cytokines in coculture model. PAM and PLE6-transfected 293T cells (non-transfected cells as controls) were cocultured for 24 h along with LPS (1 µg/mL), 2-AG (15 µM) or both. total RNA was isolated and analyzed for the mRNA level of proinflammatory cytokines by RT-qPCR. (C) Enhanced LPS-stimulation on the expression of proinflammatory cytokines in coculture model as a function of the amount of PLE6. Coculture was performed as described for Experiment B (above). However, the transfection of PLE6 plasmid was performed at 1 and 2.5 µg. (D) Reduced PLE6 increases on the expression of proinflammatory cytokines by BNPP. Coculture was performed similarly as described. However, cells were cocultured in the presence of BNPP at 100 µM. Likewise, the mRNA expression of proinflammatory cytokines was determined. (E) Effect of PLE6, 2-AG, BNPP or in combination on protein expression of proinflammatory cytokines in the coculture model. Cells were cultured and treated similarly. The levels of proinflammatory cytokines was determined with a protein chip. The data in Figure 4 are presented as the mean ± SEM of 3 independent experiments. Statistical significance was indicated by asterisks (** P < 0.01; *** P < 0.001).
In addition, the role of endogenous PLEs in LPS-induced proinflammatory cytokines was assessed in PAMs transfected with siRNA negative control (siNC) or siRNAs targeting the PLEs. Notably, the results on the levels of mRNA and the corresponding proteins of the proinflammatory cytokines induced by LPS were attenuated by siRNA and the PLEs levels were down-regulated by siRNA, respectively (Figure 5), indicating that siRNA are effective in the cells. Consistently, cotransfection of PLE1 or PLE6 with the corresponding siRNA significantly abolished the effect of PLEs overexpression on the levels of proinflammatory cytokines, pointing to the specificity of this experiment (Figure S4).
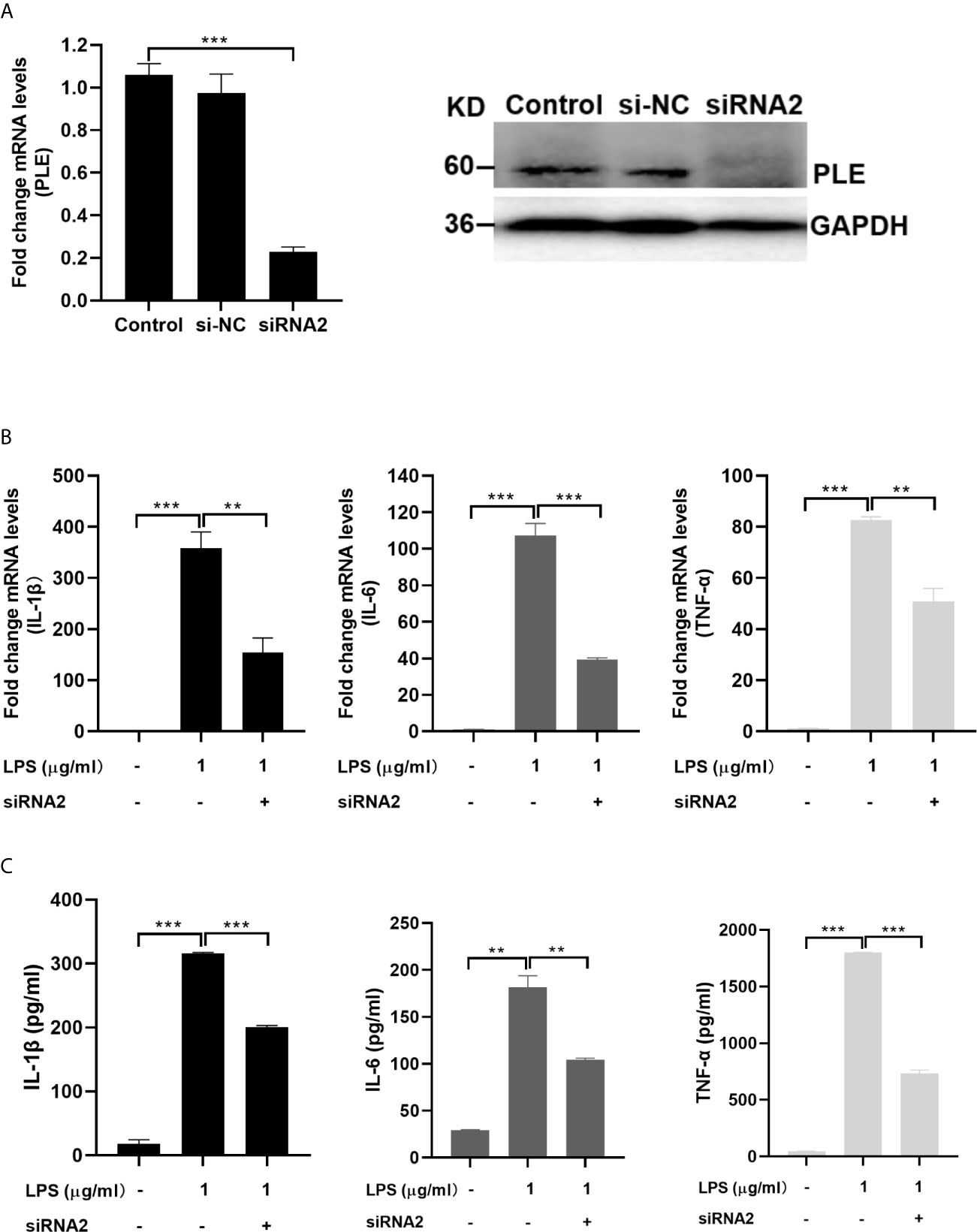
Figure 5 Decreased LPS-stimulation on the expression of proinflammatory cytokines in PAMs by transfection of siRNA2 targeting PLEs. (A) The expression of PLEs was confirmed by RT-qPCR (left) and western blotting (Right). si-NC: si negative control. (B) Decreased LPS-stimulation on the mRNA expression of proinflammatory cytokines in PAMs by transfection of siRNA2 targeting PLEs. PAMs were cultured for 24 h, and then transfected with siRNA. After incubation for 24 h, LPS (1 µg/mL) was treated for additional 6 h Then, total RNA was isolated and analyzed for the mRNA level of proinflammatory cytokines by RT-qPCR. (C) Decreased LPS-stimulation on the protein expression of proinflammatory cytokines in PAMs by transfection of siRNA2 targeting PLEs. Cells were treated as Experiment B (above). However, culture supernatant was collected and analyzed by ELISA. Data are presented as the mean ± SEM of 3 independent experiments. Statistical significance was indicated by asterisks (** P < 0.01; *** P < 0.001).
Association of PLEs With Tissue Injury and Inflammation In Vivo
To gain insight into in vivo consequences of PLEs-enhanced inflammation, pigs (1 month old, male) were treated with LPS to induce inflammatory responses with or without BNPP, and cell injury and levels of inflammatory cytokines as well as the overall whole body response were monitored. Indeed, LPS-treated pigs initially (1-6 h) exhibited labored breathing, lethargy and reduced feed intake. Subsequently, pigs became lateral decubitus, hypersalivation, shivering and severe dyspnea. These symptoms gradually disappeared after 9 h. The pigs pretreated with BNPP, nevertheless, exhibited lesser severity, notably during the first 4 h right after LPS-treatment, when the symptoms were most severe in pigs treated with LPS alone. It should be noted that the selection of male pigs at an age of 1 month was based on several considerations. Nevertheless, our previous study has shown that there is minimal difference in terms of sex in carboxylesterases (36), and the cost-effective consideration is another factor for the study design with male animals only.
To gain pathological insight into the observed overall changes induced by LPS alone or LPS-BNPP-cotreatment, pathological examinations were performed. As shown in Figure 6, LPS caused necrosis in the liver, disintegration of hepatocytes, thickening of alveolar septa, and shedding and necrosis of intestinal villi were also observed. In contrast, pretreatment with BNPP, an efficacious inhibitor of PLEs provided substantial protection against LPS-induced tissue injury (Figure 6A). Neutrophil infiltration in the liver and duodenum, a cellular marker for inflammation, was examined by myeloperoxidase (MPO) staining and integral optical density (IOD). Pretreatment with BNPP offered effective counteractive activity against LPS-induced neutrophil infiltration (Figure 6B).
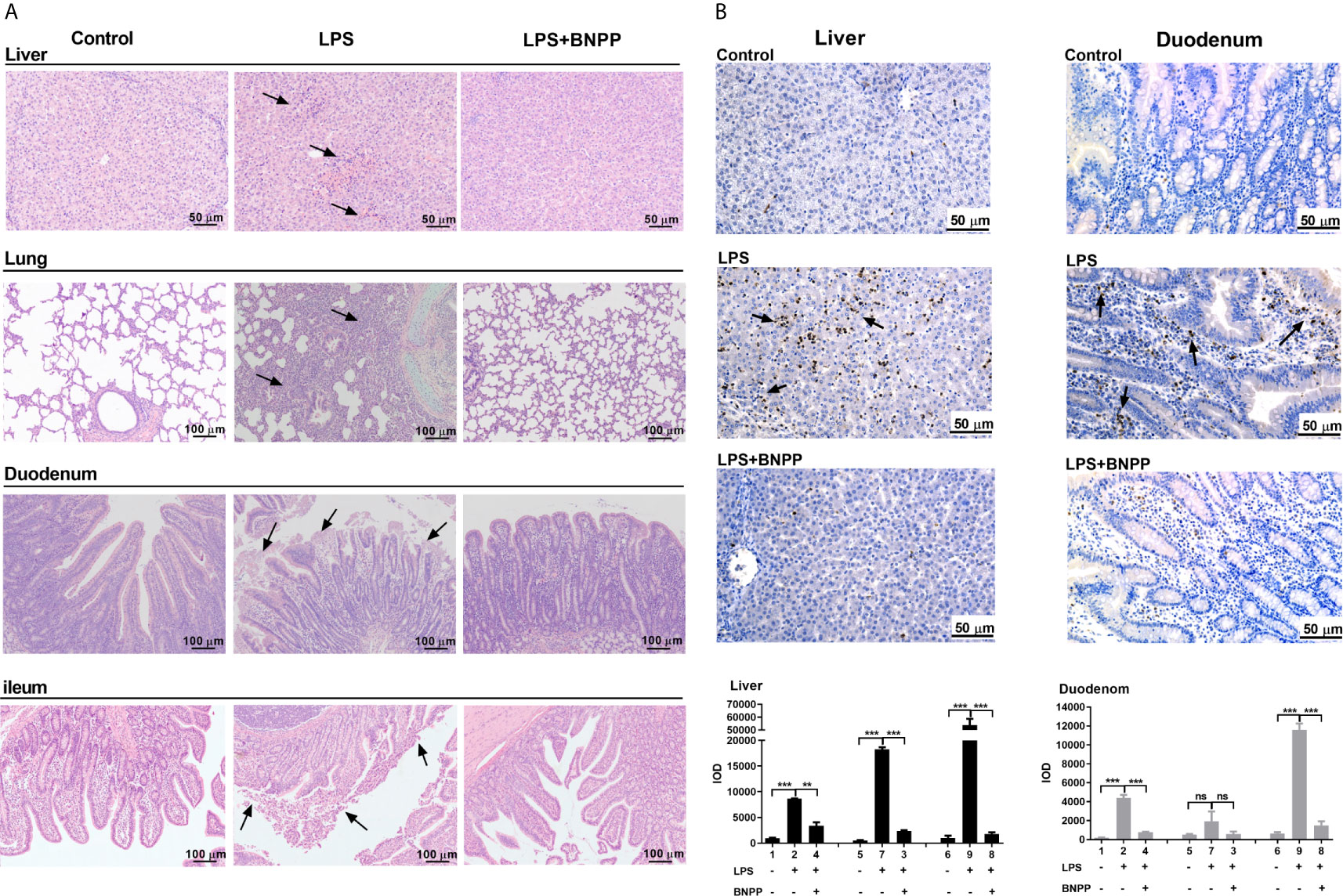
Figure 6 PLE inactivation attenuates tissue injury and the inflammatory response in vivo. (A) Pathological examination (H&E staining) of pig tissues. Pigs (n=3) were treated with sterile saline or LPS (25 µg/kg) or the combination of LPS and BNPP (25 mg/kg, i.p., given prior to 1 h of LPS induction). Then, tissue injuries were detected after 24 h. (B) Neutrophil infiltration was assessed by MPO staining (brown staining), and quantitative analysis was performed by CaseViewer software. Representative images are shown, and the black arrow shows the damage in the tissues. Integral optical density (IOD) is the value of the light absorbed or transmitted by the result of dye staining in different tissue cells under the same illumination condition. The numbers in the figures represent the corresponding pigs. Statistical significance was indicated by asterisks (**P < 0.01; ***P < 0.001).
Decreased Formation of AA and PGs In Vivo by Inhibition of PLEs
Hydrolysis of endocannabinoids produces AA, promoting inflammatory response by participating in the differentiation and proliferation of Th1 and Th17 cells (50). Therefore, we next tested whether inhibition of PLEs in vivo reduces the formation of AA and its inflammatory effectors: prostaglandin D2 (PGD2) and prostaglandin E2 (PGE2) (30, 32). The results are summarized in Figure 7. Among the examined tissues, the spleen, an immune organ, had the highest levels of AA (31.99 nmol/g), PGD2 (12.98 nmol/g) and PGE2 (2.20 nmol/g). Treatment with LPS robustly increased the level of these molecular species. The increases, however, were blunted by BNPP (25 mg/kg) in almost all the examined tissues. These data confirm that PLEs contribute to the AA precursor pool for PGs in vivo. It should be noted that replacement of PLE6 transfected cells with primary hepatocytes produced similar results (Figure S5), suggesting that the observed distant hydrolysis by carboxylesterases is applicable to in vivo situation.
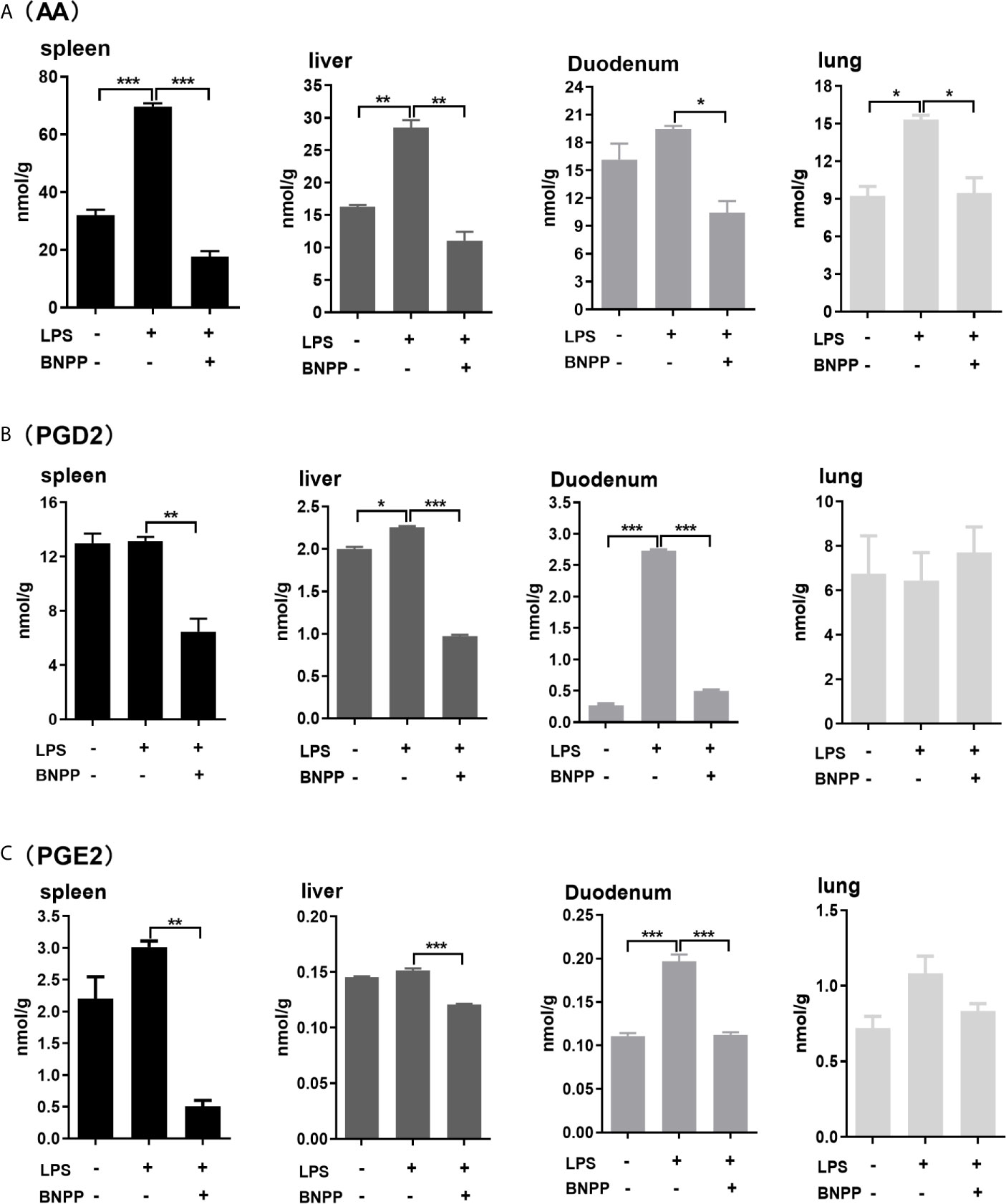
Figure 7 Levels of AA (A), PGD2 (B) and PGE2 (C) in tissues from pigs treated with BNPP and LPS. Pigs (n = 3) were treated with BNPP (25 mg/kg, i.p.) initially and 1 h later with LPS (25 µg/kg, i.p.) in pigs. Animals were euthanized 24 h after LPS administration. Tissues were harvested, extracted and analyzed for the level of AA, PGD2 and PGE2 with LC-MS/MS. The data in Figure 7 are presented as the mean ± SEM of 3 independent experiments. Statistical significnce was considered at values of P < 0.05 and indicated by an asterisk (*P < 0.05; **P < 0.01; ***P < 0.001).
Discussion
Carboxylesterases have been established to play critical roles in drug metabolism and detoxifications (1, 2). Emerging evidence, nonetheless, has linked the action of these hydrolases to lipid metabolism/processing (3, 4, 10–18). Many lipid species and their precursors are signaling molecules, pointing to regulatory involvement of these hydrolases. In this study, we have shown that PLE6 hydrolyzed with high specific activity the endocannabinoid 2-AG (Figure 1B), a major molecular species implicated in an array of physiological functions including behaviors, energy balance, pain and inflammation (23). In addition, PLE6 was sensitive to the predominant carboxylesterase inhibitor BNPP with an IC50 value of 10 µM (Figure S2A). Critically, addition (extracellular) or transfection (intracellular) of PLE6 increased the expression/secretion of proinflammatory cytokines (Figures 2, 3), and the increase was recapitulated in cocultured alveolar macrophages and PLE6 transfected cells in transwells (Figure 4). Finally, inactivation of PLEs by BNPP or knockdown of PLEs by siRNA reduced the magnitude of inflammatory responses trigged by the immunostimulant LPS accompanied by decreased formation of AA, PGD2 and PGE2 (Figures 5 and 7). These findings define a new mechanistic connection related to the metabolism of endocannabinoids: PLE-endocannabinoid-inflammation.
The carboxylesterases-based mechanistic connection likely operates in species and/or disease dependent manners. It is established that external inflammatory stimuli (e.g., LPS) activates G-protein-coupled receptors (GPCRs) and produces endocannabinoids such as 2-AG through an enzymatic cascade (Figure 8). This cascade uses membrane-bound lipids as the resource for endocannabinoids (51). In this study, we have shown that both transfection (intracellular) and addition (extracellular) of PLE6 increased the expression and secretion of proinflammatory cytokines (Figures 2–4). The increase via exogenous addition used extracellular 2-AG, presumably serum 2-AG under in vivo situation. Indeed, it has been reported that elevated serum 2-AG reflects certain disease conditions such as hypertensive individuals with depression and LPS has been shown by many investigators to induce release of 2-AG (31, 47, 48, 52). Such disease conditions are associated with inflammation, and at least partially through carboxylesterases-based hydrolysis of endocannabinoids.
On the other hand, the abundance of serum carboxylesterases varies depending on a species. Both mouse and rat have abundant presence of carboxylesterases in the blood (1, 53). In contrast, humans have little serum carboxylesterases unless organ injury takes place (54). As described in our previous publication (49), carboxylesterases with an endoplasmic retention signal (HXEL) stay in the endoplasmic reticulum (ER), whereas those without it are secreted into the serum (1, 53). We have reported that pigs had low levels of carboxylesterases detected by a highly cross-recognizing antibody (specific towards multiple carboxylesterases) (36). Given the fact that both PLE1 and PLE6 have the ER-retention signal (Table S1), it is likely that the detected carboxylesterases in the serum are neither of the enzymes, or otherwise, the samples were collected under disease conditions.
It is interesting to notice that PLE1 and PLE6, with a sequence identity of 96% at amino acid (Table S1), exhibited profound differences in hydrolyzing one substrate but not the other. In this study, we have shown that PLE1 and PLE6 hydrolyzed p-NPA at a comparable rate of specific activity: 1.70 versus 2.13 µmol/mg/min, respectively (Figure S1). However, they exhibited large differences towards 2-AG and AEA (Figure 1). PLE1 hydrolyzed 2-AG at a specific rate of 1.56 nmol/mg/min, while PLE6 at a rate of 62.70 nmol/mg/min (Figure 1B), representing a 40-fold difference. Likewise, PLE1 hydrolyzed AEA at a specific rate of 0.52 nmol/mg/min, whereas PLE6 at 1.54 nmol/mg/min (Figure 1C), a 3-fold difference. The precise mechanism remains to be determined. Sequence alignment identifies only a total of 18 amino acid substitutions between PLE1 and PLE6 (Figure S6), and half of these substitutions are identical between PLE6 and CES1 (human). CES1 (human) has been shown to effectively hydrolyze endocannabinoids (25, 30). The amino acids shared by PLE6 and CES1 differ markedly from those in PLE1 in terms of charge, hydrophilicity and aromatic side-chain (Figure S6). It is assumed that those amino acids shared with CES1 but differed from PLE1 likely contribute significantly to recognizing lipophilic substrates such as endocannabinoids (2-AG) but less so towards relatively hydrophilic substrates such as p-NPA.
The lipophilicity-based rationale for the substrate specificity, on the other hand, is not quite applicable to the inhibitor BNPP. Actually, BNPP and p-NPA have a similar LogP value (1.7 versus 1.5), an indicator for lipophilicity. However, PLE1 and PLE6 had a 100-fold difference, based on their IC50 values, toward BNPP (Figure S2A). BNPP is an irreversible carboxylesterase inhibitor (55). It was likely that the contact initiated with PLE1 induced conformational changes favoring the covalent complex in compare with the contact with PLE6. Nevertheless, both pig carboxylesterases, even with PLE6 at an IC50 value of 10 µM, were sensitive to BNPP compared with those (e.g., MAGL) known to hydrolyze endocannabinoids (e.g., 2-AG) (55, 56). It was therefore convincible that the concentrations and dose used in vitro and in vivo in this study had overwhelmingly inhibited PLE6-based hydrolysis (Figures 2–7). Particularly, BNPP reduced the formation of AA and its derivatives by as much as 33.1% to 56.3% among various organs (Figure 7) consistent with the relative potency on the inhibited hydrolysis by S9 and culture primary cells (Figures S2 and 3). The finding on the formation of AA was largely confirmed on the formation of PGD2 and PGE2 (Figures 7B, C). It should be noted that carboxylesterases, compared with other endocannabinoid hydrolases (e.g., MAGL) are abundantly expressed in peripheral organs, particularly the intestine, kidney, liver and lung. As a result, the innovative connection specified in this study, PLE-endocannabinoid-inflammation, signifies reduced inflammatory responses in these organs by inhibiting carboxylesterases. Consistently, previous studies demonstrated that MAGL inhibited cancer growth through hydrolyzing endocannabinoid (57).
In summary, our work points to several important conclusions. First, carboxylesterases are efficacious hydrolases towards endocannabinoids such as 2-AG, constituting alternative mechanisms for the degradation of these biologically active molecules. Second, PLE1 and PLE6, although sharing a 96% sequence identity at amino acid level, differ profoundly in the hydrolysis of endocannabinoids (e.g., 2-AG) (Table S1). The parent endocannabinoids and their metabolites have distinct and probably opposite biological functions, notably related to inflammatory response. The slight differences in the sequences of carboxylesterases (e.g., polymorphisms) may have profound clinical implications. Third, we have shown that transfection of PLE6 (intracellular) and addition of purified PLE6 (extracellular) both enhance the expression and secretion of proinflammatory cytokines. This is of significance as serum presence of carboxylesterases varies depending a species and disease condition. And finally, we have shown that BNPP, an inhibitor with high potency towards carboxylesterases, minimizes cell injury in vivo induced by the classic immunostimulant LPS. The cannabinoid system exerts a variety of biological activities in a wide range of organs. The results from this study concludes that hydrolysis of these ligands by carboxylesterases has profound implications in terms of their engagement in inflammatory responses.
Experimental Procedures
Materials
Provided in the section of Supplementary Materials in “Supporting Information”.
Hydrolysis of p-NPA
Described in the section of Supplementary Materials in “Supporting Information”.
Hydrolysis of 2-AG and AEA
Hydrolysis of 2-AG and AEA was performed at 37°C in Tris-HCl (50 mM, pH 7.4) buffer containing substrate concentrations of 200 μM in a total volume of 100 μL. Hydrolysis was initiated by adding purified enzyme (10 μg PLE1 or PLE6), tissue S9 fractions (100 μg) or cell lysates of PAMs/PHCs (20 μg). After 15 min, 30 min or 45 min, reactions were quenched with 200 μL acetonitrile (ACN) containing arachidonic acid-d8 (AA-d8) (100 ng/mL). Quenched reactions were placed in ice bath for 10 min and then centrifuged at 12,000 rpm (4°C, 10 min). The supernatants (200 μL) were diluted with ACN (800 μL). The hydrolytic metabolite AA was monitored by LC-MS/MS. Control samples were enzymatic reactions stopped at 0 min.
The contribution of PLEs to the hydrolysis of 2-AG and AEA in tissue S9 fractions or in cell lysates was assessed with BNPP (100 μM). Tissue S9 fractions (100 μg) or cell lysates (20 μg) were added to Tris-HCl (50 mM, pH 7.4) buffer along with BNPP (100 μM) in a total volume of 100 μL. After a 10 min-preincubation at 37°C, the reactions were initiated by the addition of 2-AG or AEA (200 μM). After 15 or 30 min, reactions. were quenched with ACN (200 μL) containing AA-d8 (100 ng/mL), placed in ice for 10 min, and centrifuged at 12,000 rpm (4°C, 10 min). Supernatants (200 μL) were transferred, diluted with ACN (800 μL) and analyzed by LC-MS/MS.
LC-MS/MS Analysis
LC-MS/MS assays were performed using a Waters Xevo G2 QTOF mass spectrometer and Waters Acquity Ultra Performance Liquid Chromatography system (Waters, USA). Samples (1 μL) were injected onto a reverse-phase C18 column (2.1 mm×100 mm, 1.7 μm, Acquity UPLC BEH), and the analytes were eluted with the gradient mobile phase (A: 0.1% formic acid, B: acetonitrile): 0-0.5 min (A-99%, B-1%); 6.5-7.5 min (A-1%, B-99%); 10 min (A-99%, B-1%) with additional 10 min running-time before next injection. The ESI source was set to negative ion mode. The quantifications were based on the standard curves for AA, PGD2 and PGE2 (Cayman). The autosampler was set at 10°C, and the column temperature was at 45°C. The capillary voltage was set to 1 kV, the sampling cone was 30 v and the source temperature was 120°C. N2 was used as the desolvation gas with 800 L/h at 450°C, and argon was used as the collision gas with 50 L/h. The collision energy was set to 6.0 eV, and the scanning range was m/z=190-600. Data analysis was performed with Mass Lynx Software Version 4.1. The MS spectrograms of analyte ions were detected as follows: 2-AG, [M + COOH]- m/z=423.275; AEA, [M + COOH]- m/z=392.280; AA, [M - H]- m/z=303.232; AA-d8, [M - H]- m/z=311.283; PGD2, [M - H]- m/z=351.217; PGE2, [M - H]- m/z=351.217. The polarity of PGE2 was larger and the signal peak appeared earlier than PGD2. The hydrolytic activities of PLEs were quantified with the amount of product AA.
Transient Transfection
All expression constructs encoding PLE were prepared by inserting cDNA into the pCMV-tag-2B vector. The primers used for the cloning were shown in Table 1. Cells (293T) were cultured in Dulbecco’s modified Eagle’s medium containing 1% penicillin-streptomycin and 1% fetal bovine serum. Transfection was performed with Lipofectamine 2000 Reagent (Invitrogen). The expression of transfected PLEs was detected by western blot. To specify the metabolism of 2-AG, 24 h after transfection, 2-AG (25 μM) was added to the medium and incubated for another 1 h. The culture medium was spiked with AA-d8 (100 ng/ml) and extracted with 3 volumes of ethyl acetate containing 0.1% acetic acid. The mixture was vortexed and centrifuged (4°C, 12000 rpm, 10 min). The ethyl acetate layer was recovered and dried under N2. The residues were dissolved in 200 μL of acetonitrile and transferred to LC vials for the analysis by LC-MS/MS (liquid chromatography with tandem mass spectrometry).
PAMs were cultured in RPIM medium containing 10% fetal bovine serum. siRNA was transfected into PAM with X-treme GENE HP DNA Transfection Reagent (Roche) and the expression of PLEs was detected by western blot after 24h. To specify the effect on the expression of proinflammatory cytokines, the transfected cells (24 h after transfection) were treated with LPS (1 μg/ml) for another 6 h. The culture medium and total RNA were collected and analyzed for the expression (both mRNA and protein) of PLEs and proinflammatory cytokines.
Quantitative Real-Time PCR (qPCR)
Total RNA was isolated from cells with TRIzol reagent (Invitrogen) and reverse-transcribed (RT) with Superscript Reverse Transcriptase (TaKaRa). RT-qPCR used iQ™ SYBR Green PCR Supermix (TaKaRa) and detected with the Bio-Rad CXF real-time PCR system (Bio-Rad, USA). Primer sequences are shown in Table 2. These quences of siRNAs for PLEs are shown in Table 3.
Western Blotting Analysis
Equal amounts of proteins were separated by 12.5% sodium dodecyl sulfate-polyacrylamide gel electrophoresis and transferred electrophoretically to polyvinylidene fluoride membranes. The primary antibody against PLEs, recognizing the common motif, was described in our previous publication (42). The antibody against glyceraldehyde-3-phosphate dehydrogenase was purchased from Proteintech. The secondary Ab was goat anti-rabbit IgG conjugated to horseradish peroxidase, and the proteins recognized by the primary antibody were visualized by the ECL chemiluminescence system (Bio-Rad). The relative intensities were quantified by ImageJ software.
Isolation and Culture of PHCs and Alveolar Macrophages PAMs
PHCs were isolated from 25-day-old Large White pigs (6 kg, male) according to a modified protocol of Puviani et al. (58). PAMs were collected by bronchoalveolar lavage with phosphate-buffered saline (PBS, pH 7.4) and frozen in liquid nitrogen as the modified protocol previously described (59). Briefly, 30-day-old piglets were euthanized, and lungs were collected. The lungs were washed through a suitable funnel with PBS (pH 7.4) containing penicillin (100 units/mL) and streptomycin (100 µg/mL). Three washings were collected. Cells were centrifuged at 1000 rpm for 10 min and resuspended in RPMI containing 10% (v/v) fetal bovine serum (FBS, Gibco) and 1% penicillin-streptomycin (Gibco). Then, PHCs and PAMs were cultured at 37°C in a humidified incubator with 5% CO2. PHCs treated with LPS or 2-AG were cultured with DMEM/high glucose containing 1% FBS, while PAMs treated with LPS or 2-AG were cultured with RPMI containing 1% FBS.
Preparation of Double-Layered Coculture System
Transwell inserts (polycarbonate membrane, 0.4 µm) were used to construct the double-layered coculture system. PLE6-transfected 293T cells were seeded into 12-well plates at a density of approximately 1×106 cells/well, and PAMs were seeded into transwell polyester membrane inserts at a density of 5×105 cells/well Confluent monolayer cells were formed after a 24 h-culture,. The double-layered coculture system was then prepared in 12-well plates by placing inserts with a confluent PAM layer over the confluent 293T cell layer. The volume of the applied culture medium was 0.5 mL in the apical compartment and 1 ml in the basolateral compartment. After coculture for 24 h, the medium was placed with RPMI containing 1% FBS. BNPP (100 µM) was added to the double-layered coculture system and preincubated for 3 h. Then, LPS (1 µg/mL) or 2-AG (5 µM, 10 µM, 15 µM) was added to the double-layered coculture system and further incubated for 24 h at 37°C in a humidified incubator with 5% CO2. PAM and PLE6-transfected 293T cells were harvested for detection of proinflammatory cytokines by RT-qPCR, and medium supernatants were harvested and detected by protein chip.
In Vivo Experiment
Animal experiments were performed in accordance with the Guide for the Care and Use of Laboratory Animals Monitoring Committee of Hubei Province, China, and the protocol was approved by the Committee on the Ethics of Animal Experiments at the College of Veterinary Medicine, Huazhong Agricultural University.
Crossbred healthy pigs (Landrace × Large White, 1 month old, male, 5~7 kg) were purchased from Wuhan Zhaohui Xingda Animal Husbandry Co., Ltd., Hubei Province, China. All pigs were maintained at an ambient temperature of 20-25°C in an environmentally controlled room by air conditioning and illumination (12 h light and dark cycles). Each cage was equipped with a feeder and water nipple to allow free access to food and drinking water. Nine pigs were divided into 3 groups (N=3/group) with each group having the similar average weight. The pigs were fed in their home cages 10 days before beginning of the experiment. BNPP was administered at 25 mg/kg (0.5 ml/kg) by intraperitoneal (i.p.) injection (1 h before LPS induction). An equal volume of saline or proinflammatory agent LPS (25 μg/kg, 0.5 ml/kg, BW) was administered by i.p. injection. Pigs were euthanized 24 h after LPS injection, and organs were collected and frozen in liquid nitrogen.
Histological and Immunohistochemical Analyses in LPS-Treated Pigs
Histological analysis of tissue damage was assessed by standard hematoxylin and eosin (H&E) staining of tissue sections (5 μm thickness). For immunohistochemical staining of neutrophils in the liver and duodenum, a primary antibody against pig myeloperoxidase was used (Abcam, ab9535).
Extraction of AA, PGD2 and PGE2
Tissues were weighed and homogenized (45 Hz, 4°C, 10 min) in a mixture of PBS (50 mM, pH 7.4, 2 ml) and hexane:ethyl acetate (2 ml, 1:1 v/v). Hexane:ethyl acetate (1:1 v/v, 6 mL) containing the internal standards (AA-d8, 100 ng/ml) was added. The mixtures were vortexed and then centrifuged (4°C, 8000 rpm for 10 min). The organic layer was removed, evaporated under a stream of nitrogen and resolubilized in 200 μL of chloroform. Metabolites were quantified by LC-MS/MS.
Statistical Analysis
Statistical analyses were performed using Microsoft Excel and GraphPad Prism 8 (GraphPad Software, Inc., San Diego, CA, USA). All assays were performed in triplicate, and the data are expressed as the mean ± SEM (standard error of the mean). Statistical significance was evaluated using the two-tailed Student’s t-test, and statistically significant differences are indicated by asterisks as follows: *P < 0.05, **P < 0.01, ***P < 0.001.
Data Availability Statement
The raw data supporting the conclusions of this article will be made available by the authors, without undue reservation.
Ethics Statement
The animal study was reviewed and approved by Committee on the Ethics of Animal Experiments at the College of Veterinary Medicine, Huazhong Agricultural University.
Author Contributions
DS, QZ, and BY designed and coordinated the experiments. WS, QC, QX, YX, and XW performed experiments and analyzed results. DS, QZ, and BY wrote the manuscript. All authors contributed to the article and approved the submitted version.
Conflict of Interest
The authors declare that the research was conducted in the absence of any commercial or financial relationships that could be construed as a potential conflict of interest.
Acknowledgments
This work was supported by the Natural Science Foundation of China (No. 31772706 and No. 31372484) and National Institutes of Health Grants R01EB018748 and R21AI153031.
Supplementary Material
The Supplementary Material for this article can be found online at: https://www.frontiersin.org/articles/10.3389/fimmu.2021.670427/full#supplementary-material
Abbreviations
AA, arachidonic acid; ACN, acetonitrile; AEA, N-arachidonoylethanolamine; 2-AG, 2-arachidonoylglycerol; BNPP, Bis(4-nitrophenyl) phosphate; FBS, fetal bovine serum; IL-1β, interleukin-1β; IL-6, interleukin-6; IOD, integral optical density; LC-MS/MS, liquid chromatography with tandem mass spectrometry; MAGL, monoacylglycerol lipase; PAMs, pig alveolar macrophages; PBS, phosphate-buffered saline; PGD2, prostaglandin D2; PGE2, prostaglandin E2; PHCs, pig hepatocytes; PLE, pig liver esterase; LPS, lipopolysaccharide; MPO, myeloperoxidase; p-NPA, p-nitrophenylacetate; RT-qPCR, reverse transcription-quantitative polymerase chain reaction; TNF-α, tumor necrosis factor-α.
References
1. Holmes R, Wright M, Laulederkind S, Cox L, Hosokawa M, Imai T, et al. Recommended Nomenclature for Five Mammalian Carboxylesterase Gene Families: Human, Mouse and Rat Genes and Proteins. Mamm Genome (2010) 21:427–41. doi: 10.1007/s00335-010-9284-4
2. Yan B. Hydrolytic Enzymes in Metabolism of Drugs and Other Xenobiotics. In: P Anzenbacher, UM Zanger, editors. Weinheim: WILEY-VCH Verlag GmbH & Co. KGaA (2013). p. 165–98.
3. Aguilera CM, Gomez-Llorente C, Tofe I, Gil-Campos M, Cañete R, Gil Á. Genome-Wide Expression in Visceral Adipose Tissue From Obese Prepubertal Children. Int J Mol Sci (2015) 16:7723–37. doi: 10.3390/ijms16047723
4. Qiao Q, Bouwman FG, van Baak MA, Roumans NJT, Vink RG, Coort SLM, et al. Adipocyte Abundances of CES1, Cryab, ENO1 and GANAB are Modified in-Vitro by Glucose Restriction and are Associated With Cellular Remodelling During Weight Regain. Adipocyte (2019) 8:190–200. doi: 10.1080/21623945.2019.1608757
5. Qian Y, Markowitz JS. Natural Products as Modulators of CES1 Activity. Drug Metab Dispos (2020) 48:993–1007. doi: 10.1124/dmd.120.000065
6. Tang M, Mukundan M, Yang J, Charpentier N, LeCluyse EL, Black C, et al. Antiplatelet Agents Aspirin and Clopidogrel are Hydrolyzed by Distinct Carboxylesterases, and Clopidogrel is Transesterificated in the Presence of Ethyl Alcohol. J Pharmacol Exp Ther (2006) 319:1467–76. doi: 10.1124/jpet.106.110577
7. Shi D, Yang J, Yang D, LeCluyse EL, Black C, You L, et al. Anti-Influenza Prodrug Oseltamivir is Activated by Carboxylesterase HCE1 and the Activation is Inhibited by Anti-Platelet Agent Clopidogrel. J Pharmacol Exp Ther (2006) 319:1477–84. doi: 10.1124/jpet.106.111807
8. Lenfant N, Bourne Y, Marchot P, Chatonnet A. Relationships of Human Alpha/Beta Hydrolase Fold Proteins and Other Organophosphate-Interacting Proteins. Chem Biol Interact (2016) 259(Pt B):343–51. doi: 10.1016/j.cbi.2016.04.027
9. Comoletti D, Trobiani L, Chatonnet A, Bourne Y, Marchot P. Review - Comparative Mapping of Selected Structural Determinants on the Extracellular Domains of Cholinesterase-Like Cell-Adhesion Molecules. Neuropharmacology (2020) 6:108381. doi: 10.1016/j.neuropharm.2020.108381
10. Xu Y, Zhu Y, Bawa FC, Hu S, Pan X, Yin L, et al. Hepatocyte-Specific Expression of Human Carboxylesterase 1 Attenuates Diet-Induced Steatohepatitis and Hyperlipidemia in Mice. Hepatol Commun (2020) 4:527–39. doi: 10.1002/hep4.1487
11. Xu J, Xu Y, Xu Y, Yin L, Zhang Y. Global Inactivation of Carboxylesterase 1 (Ces1/Ces1g) Protects Against Atherosclerosis in Ldlr (-/-) Mice. Sci Rep (2017) 7:17845. doi: 10.1038/s41598-017-18232-x
12. Steinberg GR, Kemp BE, Watt MJ. Adipocyte Triglyceride Lipase Expression in Human Obesity. Am J Physiol Endocrinol Metab (2007) 293:E958–64. doi: 10.1152/ajpendo.00235.2007
13. Ross MK, Borazjani A, Mangum LC, Wang R, Crow JA. Effects of Toxicologically Relevant Xenobiotics and the Lipid-Derived Electrophile 4-Hydroxynonenal on Macrophage Cholesterol Efflux: Silencing Carboxylesterase 1 has Paradoxical Effects on Cholesterol Uptake and Efflux. Chem Res Toxicol (2014) 27:1743–56. doi: 10.1021/tx500221a
14. Bie J, Wang J, Marqueen KE, Osborne R, Kakiyama G, Korzun W, et al. Liver-Specific Cholesteryl Ester Hydrolase Deficiency Attenuates Sterol Elimination in the Feces and Increases Atherosclerosis in Ldlr-/- Mice. Arterioscler Thromb Vasc Biol (2013) 33:1795–802. doi: 10.1161/ATVBAHA.113.301634
15. Diczfalusy MA, Björkhem I, Einarsson C, Hillebrant CG, Alexson SE. Characterization of Enzymes Involved in Formation of Ethyl Esters of Long-Chain Fatty Acids in Humans. J Lipid Res (2001) 42:1025–32. doi: 10.1016/S0022-2275(20)31590-X
16. Zhao B, Song J, Ghosh S. Hepatic Overexpression of Cholesteryl Ester Hydrolase Enhances Cholesterol Elimination and In Vivo Reverse Cholesterol Transport. J Lipid Res (2008) 49:2212–7. doi: 10.1194/jlr.M800277-JLR200
17. Becker A, Böttcher A, Lackner KJ, Fehringer P, Notka F, Aslanidis C, et al. Purification, Cloning, and Expression of a Human Enzyme With Acyl Coenzyme A: Cholesterol Acyltransferase Activity, Which is Identical to Liver Carboxylesterase. Arterioscler Thromb (1994) 14:1346–55. doi: 10.1161/01.ATV.14.8.1346
18. Igarashi M, Osuga J, Uozaki H, Sekiya M, Nagashima S, Takahashi M, et al. The Critical Role of Neutral Cholesterol Ester Hydrolase 1 in Cholesterol Removal From Human Macrophages. Circ Res (2010) 107:1387–95. doi: 10.1161/CIRCRESAHA.110.226613
19. Xiao D, Chen YZ, Yang D, Yan B. Age-Related Inducibility of Carboxylesterases by the Antiepileptic Agent Phenobarbital and Implications in Drug Metabolism and Lipid Accumulation. Biochem Pharmacol (2012) 84:232–39. doi: 10.1016/j.bcp.2012.04.002
20. Quiroga AD, Lehner R. Role of Endoplasmic Reticulum Neutral Lipid Hydrolases. Trends Endocrinol Metab (2011) 22:218–25. doi: 10.1016/j.tem.2011.03.003
21. Wei E, Ben Ali Y, Lyon J, Wang H, Nelson R, Dolinsky VW, et al. Loss of TGH/Ces3 in Mice Decreases Blood Lipids, Improves Glucose Tolerance, and Increases Energy Expenditure. Cell Metab (2010) 11:183–93. doi: 10.1016/j.cmet.2010.02.005
22. Lian J, Wei E, Wang SP, Quiroga AD, Li L, Pardo AD, et al. Liver Specific Inactivation of Carboxylesterase 3/Triacylglycerol Hydrolase Decreases Blood Lipids Without Causing Severe Steatosis. Hepatology (2012) 56:2154–62. doi: 10.1002/hep.25881
23. Ghonghadze M, Pachkoria K, Okujava M, Antelava N, Gongadze N. Endocannabinoids Receptors Mediated Central and Peripheral Effects (Review). Georgian Med News (2020) 298:137–43.
24. Szafran BN, Lee JH, Borazjani A, Morrison P, Zimmerman G, Andrzejewski KL, et al. Characterization of Endocannabinoid-Metabolizing Enzymes in Human Peripheral Blood Mononuclear Cells Under Inflammatory Conditions. Molecules (2018) 23:3167. doi: 10.3390/molecules23123167
25. Scheaffer HL, Borazjani A, Szafran BN, Ross MK. Inactivation of CES1 Blocks Prostaglandin D(2) Glyceryl Ester Catabolism in Monocytes/Macrophages and Enhances its Anti-Inflammatory Effects, Whereas the Pro-Inflammatory Effects of Prostaglandin E(2) Glyceryl Ester are Attenuated. ACS Omega (2020) 5:29177–88. doi: 10.1021/acsomega.0c03961
26. Pasquarelli N, Porazik C, Hanselmann J, Weydt P, Ferger B, Witting A. Comparative Biochemical Characterization of the Monoacylglycerol Lipase Inhibitor KML29 in Brain, Spinal Cord, Liver, Spleen, Fat and Muscle Tissue. Neuropharmacology (2015) 91:148–56. doi: 10.1016/j.neuropharm.2014.12.001
27. Sándor B, Douglas OH, Hao P, Osama EA, Mohanraj R, Partha M, et al. Cannabinoid-2 Receptor Mediates Protection Against Hepatic Ischemia/Reperfusion Injury. FASEB J (2007) 21:1788–800. doi: 10.1096/fj.06-7451com
28. Caraceni P, Pertosa AM, Giannone F, Domenicali M, Grattagliano I, Principe A, et al. Antagonism of the Cannabinoid CB-1 Receptor Protects Rat Liver Against Ischaemia-Reperfusion Injury. Gut (2009) 58:1135–43. doi: 10.1136/gut.2007.147652
29. Ran W, Abdolsamad B, Matthews AT, Mangum LC, Edelmann MJ, Ross MK. Identification of Palmitoyl Protein Thioesterase 1 in Human THP1 Monocytes and Macrophages and Characterization of Unique Biochemical Activities for This Enzyme. Biochemistry (2013) 52:7559–74. doi: 10.1021/bi401138s
30. Szafran B, Borazjani A, Lee JH, Ross MK, Kaplan BLF. Lipopolysaccharide Suppresses Carboxylesterase 2g Activity and 2-Arachidonylglycerol Hydrolysis: A Possible Mechanism to Regulate Inflammation. Prostaglandins Other Lipid Mediat (2015) 121:199–206. doi: 10.1016/j.prostaglandins.2015.09.005
31. Turcotte C, Chouinard F, Lefebvre JS, Flamand N. Regulation of Inflammation by Cannabinoids, the Endocannabinoids 2-Arachidonoyl-Glycerol and Arachidonoyl-Ethanolamide, and Their Metabolites. J Leukoc Biol (2015) 97:1049–70. doi: 10.1189/jlb.3RU0115-021R
32. Johnston MG, Hay JB, Movat HZ. The Role of Prostaglandins in Inflammation. Curr Topics Pathol Ergebnisse Der Pathol (1979) 68:259. doi: 10.1007/978-3-642-67311-5_10
33. Fukami T, Nakajima M, Maruichi T, Takahashi S, Takamiya M, Aoki Y, et al. Structure and Characterization of Human Carboxylesterase 1A1, 1A2, and 1A3 Genes. Pharmacogenet Genomics (2008) 18:911–20. doi: 10.1097/FPC.0b013e32830b0c5e
34. Hosokawa M, Furihata T, Yaginuma Y, Yamamoto N, Watanabe N, Tsukada E, et al. Structural Organization and Characterization of the Regulatory Element of the Human Carboxylesterase (CES1A1 and CES1A2) Genes. Drug Metab Pharmacokinet (2008) 23:73–84. doi: 10.2133/dmpk.23.73
35. Sanghani SP, Sanghani PC, Schiel MA, Bosron WF. Human Carboxylesterases: An Update on CES1, CES2 and CES3. Protein Pept Lett (2009) 16:1207–14. doi: 10.2174/092986609789071324
36. Xiao Q, Zhou Q, Yang L, Tian Z, Wang X, Xiao Y, et al. Breed Differences in Pig Liver Esterase (PLE) Between Tongcheng (Chinese Local Breed) and Large White Pigs. Sci Rep (2018) 8:16364. doi: 10.1038/s41598-018-34695-y
37. Brusehaber E, Bottcher D, Bornscheuer UT. Insights Into the Physiological Role of Pig Liver Esterase: Isoenzymes Show Differences in the Demethylation of Prenylated Proteins. Bioorgan Med Chem (2009) 17:7878–88. doi: 10.1016/j.bmc.2009.10.033
38. Pabst R. The Pig as a Model for Immunology Research. Cell Tissue Res (2020) 380:287–304. doi: 10.1007/s00441-020-03206-9
39. Long JZ, Nomura DK, Cravatt BF. Characterization of Monoacylglycerol Lipase Inhibition Reveals Differences in Central and Peripheral Endocannabinoid Metabolism. Chem Biol (2009) 16:744–53. doi: 10.1016/j.chembiol.2009.05.009
40. Heymann E, Krisch K. [Phosphoric Acid-Bis-(P-Nitro-Phenylester), a New Inhibitor of Microsomal Carboxylesterases]. cHoppe Seylers Z. Physiol Chem (1967) 348:609–19. doi: 10.1515/bchm2.1967.348.1.609
41. Lamego J, Coroadinha AS, Simplício AL. Detection and Quantification of Carboxylesterase 2 Activity by Capillary Electrophoresis. Anal Chem (2011) 83:881–7. doi: 10.1021/ac102547c
42. Zhou Q, Xiao Q, Zhang Y, Wang X, Xiao Y, Shi D. Pig Liver Esterases PLE1 and PLE6: Heterologous Expression, Hydrolysis of Common Antibiotics and Pharmacological Consequences. Sci Rep (2019) 9:15564. doi: 10.1038/s41598-019-51580-4
43. Shi T, Denney L, An H, Ho LP, Zheng YJ. Alveolar and Lung Interstitial Macrophages: Definitions, Functions, and Roles in Lung Fibrosis. Leukoc Biol (2020) 1–8. doi: 10.1002/JLB.3RU0720-418R
44. Oesch F, Fabian E, Landsiedel R. Xenobiotica-Metabolizing Enzymes in the Lung of Experimental Animals, Man and in Human Lung Models. Arch Toxicol (2019) 93:3419–89. doi: 10.1007/s00204-019-02602-7
45. Rasmussen MK. Induction of Cytochrome P450 mRNA in Porcine Primary Hepatocytes Cultured Under Serum Free Conditions: Comparison of Freshly Isolated Cells and Cryopreserved. Exp Cell Res (2017) 360:218–25. doi: 10.1016/j.yexcr.2017.09.011
46. Zhou Z, Xu MJ, Gao B. Hepatocytes: A Key Cell Type for Innate Immunity. Cell Mol Immunol (2016) 13:301–15. doi: 10.1038/cmi.2015.97
47. Piomelli D, Giuffrida A, Calignano A, Rodríguez de Fonseca F. The Endocannabinoid System as a Target for Therapeutic Drugs. Trends Pharmacol Sci (2000) 21:218–24. doi: 10.1016/S0165-6147(00)01482-6
48. Ninomiya N, Nemoto K, Kaji M, Shiga N, Shimada Y, Yamamoto Y. Regulation of the Endocannabinoid System in Endotoxicosis of Conscious Guinea Pigs. JJAAM (2005) 16(5):218–26. doi: 10.3893/jjaam.16.218
49. Xie M, Yang D, Liu L, Xue B, Yan B. Human and Rodent Carboxylesterases: Immunorelatedness, Overlapping Substrate Specificity, Differential Sensitivity to Serine Enzyme Inhibitors, and Tumor-Related Expression. Drug Metab Dispos (2002) 30:541–7. doi: 10.1124/dmd.30.5.541
50. Isabel M, Pierre P, Pierangelo O, Michel S, Joel P, Vincenzo DM. Presence and Regulation of the Endocannabinoid System in Human Dendritic Cells. FEBS J (2010) 269:3771–8. doi: 10.1046/j.1432-1033.2002.03078.x
51. Moussa-Tooks AB, Larson ER, Gimeno AF, Leishman E, Bartolomeo LA, Bradshaw HB, et al. Long-Term Aberrations to Cerebellar Endocannabinoids Induced by Early-Life Stress. Sci Rep (2020) Apr 2910(1):7236. doi: 10.1038/s41598-020-64075-4
52. Hill MN, Miller GE, Ho WS, Gorzalka BB, Hillard CJ. Serum Endocannabinoid Content is Altered in Females With Depressive Disorders: A Preliminary Report. Pharmacopsychiatry (2008) 41:48–53. doi: 10.1055/s-2007-993211
53. Yan B, Yang D, Bullock P, Parkinson A. Rat Serum Carboxylesterase. Cloning, Expression, Regulation, and Evidence of Secretion From Liver. J Biol Chem (1995) 270:19128–34. doi: 10.1074/jbc.270.32.19128
54. Feng J, Xu Y, Huang W, Kong H, Li Y, Cheng H, et al. A Magnetic SERS Immunosensor for Highly Sensitive and Selective Detection of Human Carboxylesterase 1 in Human Serum Samples. Anal Chim Acta (2020) 1097:176–85. doi: 10.1016/j.aca.2019.11.004
55. Crow JA, Bittles V, Borazjani A, Potter PM, Ross MK. Covalent Inhibition of Recombinant Human Carboxylesterase 1 and 2 and Monoacylglycerol Lipase by the Carbamates JZL184 and URB597. Biochem Pharmacol (2012) 84:1215–22. doi: 10.1016/j.bcp.2012.08.017
56. Mentlein R, Berge RK, Heymann E. Identity of Purified Monoacylglycerol Lipase, palmitoyl-CoA Hydrolase and Aspirin-Metabolizing Carboxylesterase From Rat Liver Microsomal Fractions. A Comparative Study With Enzymes Purified in Different Laboratories. Biochem J (1985) 232:479–83. doi: 10.1042/bj2320479
57. Leishman E, Mackie K, Luquet S, Bradshaw HB. Lipidomics Profile of a NAPE-PLD KO Mouse Provides Evidence of a Broader Role of This Enzyme in Lipid Metabolism in the Brain. Biochim Biophys Acta (2016) Jun1861(6):491–500. doi: 10.1016/j.bbalip.2016.03.003
58. Puviani AC, Ottolenghi C, Tassinari B, Pazzi P, Morsiani E. An Update on High-Yield Hepatocyte Isolation Methods and on the Potential Clinical Use of Isolated Liver Cells. Comp Biochem Physiol A Mol Integr Physiol (1998) 121:99–109. doi: 10.1016/S1095-6433(98)10109-5
Keywords: Carboxylesterases, pig liver esterase, endocannabinoid, arachidonic acid, prostaglandins, inflammation
Citation: Zhou Q, Yan B, Sun W, Chen Q, Xiao Q, Xiao Y, Wang X and Shi D (2021) Pig Liver Esterases Hydrolyze Endocannabinoids and Promote Inflammatory Response. Front. Immunol. 12:670427. doi: 10.3389/fimmu.2021.670427
Received: 21 February 2021; Accepted: 26 April 2021;
Published: 17 May 2021.
Edited by:
Guochang Hu, University of Illinois at Chicago, United StatesReviewed by:
Philip Kingsley, Vanderbilt University, United StatesEmma Leishman, Indiana University Bloomington, United States
Copyright © 2021 Zhou, Yan, Sun, Chen, Xiao, Xiao, Wang and Shi. This is an open-access article distributed under the terms of the Creative Commons Attribution License (CC BY). The use, distribution or reproduction in other forums is permitted, provided the original author(s) and the copyright owner(s) are credited and that the original publication in this journal is cited, in accordance with accepted academic practice. No use, distribution or reproduction is permitted which does not comply with these terms.
*Correspondence: Deshi Shi, cm9ja0BtYWlsLmh6YXUuZWR1LmNu