- 1Department of Clinical Research and Innovation, Foch Hospital, Suresnes, France
- 2Centre de Recherche Clinique, Grand Hôpital de l’Est Francilien (GHEF), Meaux, France
- 3University Hospital Center (CHU) Amiens Picardie, University of Picardie Jules Verne (UPJV), Amiens, France
- 4Laboratory of Mathematics and Applications (LMA), Unité Mixte de Recherche (UMR) Centre National de la Recherche Scientifique (CNRS) 7348, University of Poitiers, Poitiers, France
The Coronavirus disease 2019 (COVID-19), caused by the novel coronavirus SARS-CoV-2 (severe acute respiratory syndrome coronavirus 2), has quickly reached pandemic proportions. Cytokine profiles observed in COVID-19 patients have revealed increased levels of IL-1β, IL-2, IL-6, and TNF-α and increased NF-κB pathway activity. Recent evidence has shown that the upregulation of the WNT/β-catenin pathway is associated with inflammation, resulting in a cytokine storm in ARDS (acute respire distress syndrome) and especially in COVID-19 patients. Several studies have shown that the WNT/β-catenin pathway interacts with PPARγ in an opposing interplay in numerous diseases. Furthermore, recent studies have highlighted the interesting role of PPARγ agonists as modulators of inflammatory and immunomodulatory drugs through the targeting of the cytokine storm in COVID-19 patients. SARS-CoV2 infection presents a decrease in the angiotensin-converting enzyme 2 (ACE2) associated with the upregulation of the WNT/β-catenin pathway. SARS-Cov2 may invade human organs besides the lungs through the expression of ACE2. Evidence has highlighted the fact that PPARγ agonists can increase ACE2 expression, suggesting a possible role for PPARγ agonists in the treatment of COVID-19. This review therefore focuses on the opposing interplay between the canonical WNT/β-catenin pathway and PPARγ in SARS-CoV2 infection and the potential beneficial role of PPARγ agonists in this context.
Introduction
The Coronavirus disease 2019 (COVID-19), caused by the novel coronavirus SARS-CoV-2 (severe acute respiratory syndrome coronavirus 2), has quickly reached pandemic proportions. Like SARS-CoV, SARS-CoV-2 is a member of the Beta-coronavirus family. Although the majority of COVID-19 patients present mild to moderate clinical features (1, 2), some may develop severe pneumonia or suffer from the acute respiratory distress syndrome (ARDS) and multi-organ failure, leading to high death rates. Nevertheless, the pathophysiology of Corona Virus Disease-19 (COVID-19) remains unclear. Currently, in patients with life-threatening ARDS, there is growing evidence that virally-induced pro-inflammatory cytokines (such as Interleukin (IL)-6 and tumor necrosis factor-α (TNF-α)) enhance inflammation in the latter stages of this disease (3–5). Such findings are further corroborated by recent studies indicating that high levels of IL-6 are predictors of mortality (6). Cytokine profiles in COVID-19 patients have revealed increased levels of interleukin-1β (IL-1β), IL-2, IL-6 and tumor necrosis factor-alpha (TNFα) (7). TNF-α is one of the main activators of IL-6 expression and an increase in baseline plasma levels of IL-6 may predict that survival chances are poor (7). Moreover, an increase in the proportion of Th17 cells has been observed in COVID-19 patients, leading to the stimulation of IL-6 (8). Recent evidence has shown that the upregulation of the canonical WNT/β-catenin pathway is associated with inflammation and a cytokine storm in ARDS (9) and especially COVID-19 patients (10). Several studies have shown that, in numerous diseases (11–14), the WNT/β-catenin pathway interacts with PPARγ (peroxisome proliferator-activated receptor gamma) in an opposing interplay, with the effects of one opposing those of the other. Recent studies have also highlighted the possible role of PPARγ agonists as modulators of inflammatory and immunomodulatory drugs by targeting the cytokine storm in COVID-19 patients (15, 16). This review focuses on the opposing interplay between WNT/β-catenin and PPARγ in SARS-CoV-2 infection and the potential role of PPARγ agonists in this context.
Inflammation and SARS-CoV-2 Infection
The severity of symptoms in SARS-CoV-2 infection depends on the viral infection and the host immune system. The COVID-19 cytokine profile of patients is closely associated with cytokine release, indicating macrophage activation, and an increase in the level of cytokines such as the TNFα, IL-6 and interferon-gamma (IFN-γ) (4).
The increased levels of these cytokines is a characteristic of ARDS, with a low level of oxygen in the blood and shortness of breath (17). The SARS-CoV-2 infection mainly damages the endothelial cells of the airway, alveoli, vascular system, and macrophages in the lungs. SARS-CoV-2 recruits the receptor of angiotensin-converting enzyme 2 (ACE2) for infection (18). The expression of the ACE2 receptor is decreased in the lungs in the SARS-CoV-2 infection, dysregulating the renin-angiotensin system, which damages the fluid and electrolyte balance, blood pressure levels, and increases the vascular permeability and inflammatory processes in the airway (19–21).
SARS-CoV recruits several immune-suppressive proteins thereby increasing the immune response (22). SARS-CoV enhances several structural and non-structural proteins acting as antagonists of interferon signaling. Stopping interferon signaling could be a response to: a) prevent the recognition of viral RNA via the pattern recognition receptor (PRR), b) decrease the synthesis of type I interferon protein by interrupting the toll-like receptor-1 (TLR-1) and the retinoic acid-inducible gene I (RIG-I), and c) increase the STAT pathway activity (23).
The SARS-CoV-2 virus causes massive damage to the infected epithelial and endothelial cells, with an excessive release of cytokines and chemokines (18). In SARS-CoV-2, stimulation of the caspase-1 enhances the production of pro-inflammatory cytokines such as IL-1β and IL-6 (24). These cytokines bind with other immune cells, including T-lymphocytes and monocytes (8, 25). Severe COVID-19 patients show increased levels of the granulocyte colony-stimulating factor (G-CSF), IL-2, IL-6, IL-10, monocyte chemo-attractant peptide (MCP)-1), macrophage inflammatory protein 1α (MIP1α) and TNF-α (26).
The nuclear factor-κB (NF-κB) pathway is one of the main inflammation processes. NF-κB is a hetero-dimeric transcription factor belonging to the Rel protein family. Under physiological conditions, RelA/p50, the heterodimer’s predominant form of the NF-κB pathway, is inactivated in the cytoplasm by the IkB protein (27). SARS-CoV infection leads to a release of pro-inflammatory cytokines and growth factors to activate the IkB Kinase (IKK), which phosphorylates and degrades the IkB protein through an ubiquitination mechanism (28).
The NF-κB pathway can modulate the expression of pro-inflammatory genes responsible for the cytokine storm. SARS-CoV-2 can induce the nuclear translocation of the NF-κB pathway to stimulate IL-6 expression (28). Numerous studies have shown that SARS-CoV (29–31), including SARS-Cov-2 (32), can activate the NF-κB pathway.
The Canonical WNT/β-Catenin Pathway
The name WNT is derived from Wingless drosophila melanogaster and its mouse homolog Int. The canonical WNT/β-catenin pathway is involved in several mechanisms, controlling signaling, including embryogenesis, cell proliferation, migration and polarity, apoptosis, and organogenesis (33). Nevertheless, the WNT/β-catenin pathway can be altered in several pathological diseases, such as inflammation, metabolic, neurological and psychiatric disorders, fibrosis and cancer processes (34–42).
The WNT ligands belongs to the family of secreted lipid-modified glycoproteins (43). WNT ligands are produced by neurons and immune cells localized in the central nervous system (CNS) (44). WNT pathway dysfunction can affect numerous neurodegenerative pathologies (11, 45–48). The canonical WNT pathway comprises the β-catenin, T-cell factor and lymphoid enhancer factor (TCF/LEF). Cytoplasmic accumulation of β-catenin is modulated by the destruction complex AXIN, tumor suppressor adenomatous polyposis coli (APC), and glycogen synthase kinase-3 (GSK-3β). In the absence of WNT ligands, the destruction complex has a role in the phosphorylation of the cytoplasmic β-catenin and leads to its proteasomal destruction. However, when they are present, WNT ligands bind with Frizzled (FZL) and LDL receptor-related protein 5/6 (LRP 5/6) to interrupt the destruction complex and prevent β-catenin degradation into the proteasome. β-catenin translocates to the nucleus to interact with the TCF/LEF. This stimulates the WNT target genes (49–51).
Glycogen synthase kinase-3β (GSK-3β) is one of the major inhibitors of the WNT/β-catenin pathway (35, 36, 52–55). As an intracellular serine-threonine kinase, GSK-3β is a major negative controller of WNT signaling (56). GSK-3β is involved in the control of numerous kinds of pathophysiological pathways, including cell membrane signaling, cell polarity, and inflammation (57–59). GSK-3β interacts by downregulating the cytoplasmic β-catenin and stabilizing it to enhance its nuclear migration (60).
A positive interplay has been recently observed between the WNT/β-catenin pathway and inflammation, expressed by an activated NF-ϰB pathway (37). Over-stimulation of WNT/β-catenin leads to the enhancement of IϰB-α degradation and then NF-ϰB pathway transactivation (61). The WNT/β-catenin pathway increases COX expression, which then influences the inflammatory response (62). In addition, the NF-ϰB pathway downregulates GSK-3β and positively enhances β-catenin signaling (63, 64).
WNT/β-Catenin Pathway and SARS-CoV-2 Infection
Several studies have shown that WNT ligands, secreted by immune cells, can control inflammatory response and immune cell modulation (65–68). Moreover, WNT ligands play major roles in tissue damage and repair (65). The WNT/β-catenin pathway is upregulated in severe sepsis-induced acute lung injury and sepsis mouse models (67, 69). The WNT pathway is damaged in sepsis or ARDS, and therefore plays a major role in fibrosis and inflammation (66, 70). In COVID-19 patients, the transforming growth factor (TGF-β) stimulates the WNT/β-catenin pathway, leading to an increased risk of pulmonary fibrosis (70) and pulmonary infection (10, 71) (Table 1). Several studies have shown that the TGF-β and WNT/β-catenin pathways upregulate each other in a positive feedback (54, 88).
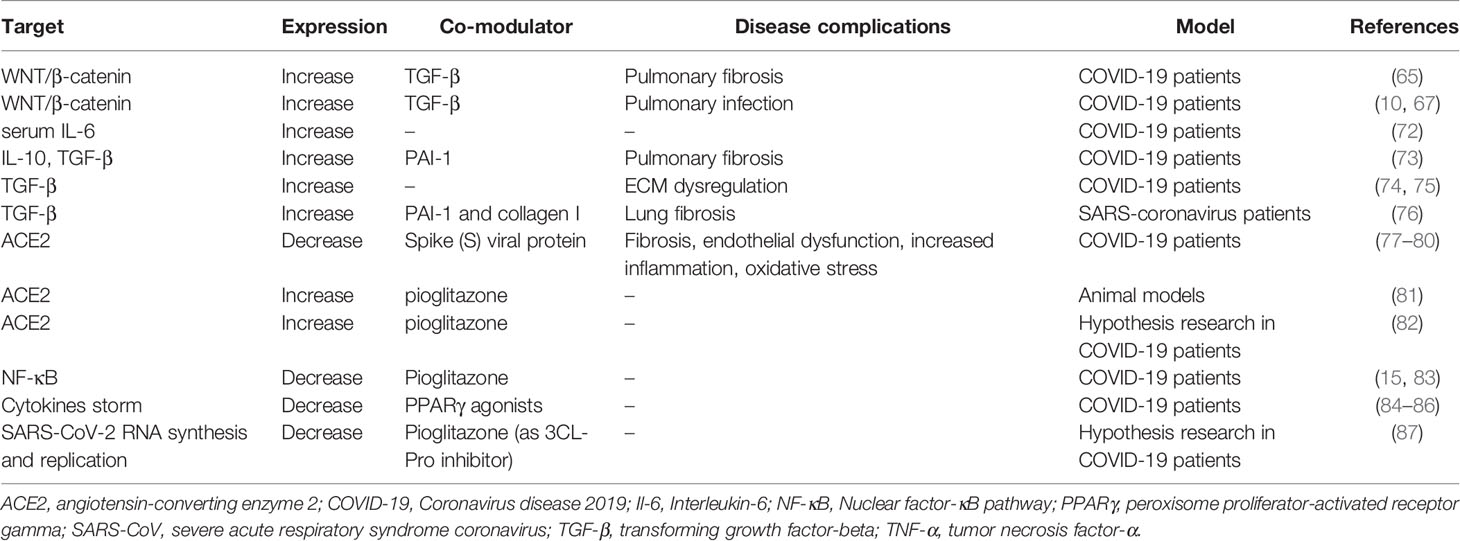
Table 1 Mechanisms by which the WNT/β-catenin pathway is modulated and the possible roles of PPARγ agonists in treating SARS-CoV-2 infection.
The TGF-β pathway is one of the main signaling pathways involved in fibrosis through the enhancement of EMT and fibroblast differentiation (89). Several inflammatory cytokines have a positive relationship with the TGF-β pathway (89). Interactions between the TGF-β pathway and Smad pathway are involved in pulmonary fibrosis (90). The TGF-β/Smad pathway has been shown to be a promotor of PAI-1 synthesis in SARS-CoV (91). Moreover, cytokines can activate the JAK/STAT pathway (92) to dysregulate cellular homeostasis, proliferation and apoptosis (93). IL-6 can activate the JAK/STAT pathway in T helper cells (4, 94) to induce several biological functions, such as immune regulation, lymphocyte differentiation and oxidative stress (72, 95). The increase in IL-6 observed in severe COVID-19 patients is associated with significantly lower survival rates (6, 96). COVID-19 patients present both dysregulated JAK/STAT pathway (97) and important role of TGF-β/Smad pathway (98).
In severe COVID-19 patients, serum IL-6 is significantly greater than in non COVID-19 subjects (99). The excessive production of inflammatory cytokines in the lungs of COVID-19 patients is associated with the increase in macrophage activation (100). In a mouse model of systemic inflammation, PAI-1 is involved in the regulation of host inflammatory responses through Toll- like Receptor-4 (TLR4)-mediated macrophage activation (101). Activation of the NF-κB pathway results in stimulating the TGF-β pathway in a vicious loop (73) and in concordance with PAI-1 (74). Thus, PAI-1 seems to be partly responsible for the excessive production of cytokines by macrophages in severe COVID-19 patients (75). PAI-1 expression is associated with increased IL-10 and an activated TGF-β pathway (102). Thus, the activated TGF-β pathway observed in COVID-19 patients may drive the pulmonary fibrosis process (102). In COVID-19 patients, ECM dysregulation could be one of the sources of stimulation of the TGF-β pathway (76, 103) (Table 1). This stimulation is responsible for the activation of integrin αvβ6 and thrombospondin induced by the STAT pathway (76, 104). In COVID-19 patients, a vicious loop is involved between the TGF-β pathway, the STAT pathway and PAI-1 (75). Furthermore, the targets involved in fibrosis, such as collagens, proteoglycans, integrins, the connective tissue growth factor, and matrix metalloproteinases (MMPs) are activated by the TGF-β pathway (105). SARS-CoV proteins may enhance the TGF-β-induced expression of PAI-1 and collagen I to induce lung fibrosis (106).
PPARγ
PPARs (peroxisome proliferator-activated receptors) are ligand-activated transcription factors that bind PPREs (PPAR-response elements). In the nucleus, PPARs form a heterodimer with the retinoid X receptor (RXR) (107). They are composed of a ligand-binding domain that interacts with a DNA-binding domain to modulate it (108). PPARs are involved in numerous pathophysiological processes, such as cell differentiation, protein metabolism, lipid metabolism, carcinogenesis (109, 110), adipocyte differentiation, insulin sensitivity and inflammation (111, 112). PPARs are subdivided into three isoforms: PPARα, PPARγ and PPARβ (113). PPARγ is highly expressed in adipose tissue and macrophages (114). PPARγ ligands can be synthetic or natural. PPARγ ligands have hypoglycemic and hypocholesterolemic roles. PPARγ agonists such as thiazolidinediones (TZDs) have been used to treat type 2 diabetes patients (115) and to decrease inflammatory activity (115). Natural ligands include prostaglandins and unsaturated fatty acids (116). Natural ligands include prostaglandins and unsaturated fatty acids. Moreover, PPARγ ligands, such as thiazolidinediones, can directly decrease inflammatory activity (12), fibrosis processes (117) and lung inflammation (118). In adipocyte mitochondria, pioglitazone (30–45 mg/day for three months) can reduce the expression of cytokines, including IL-6 and TNFα in humans (119). In patients with impaired glucose tolerance, four months (45 mg/day) of treatment with pioglitazone is associated with a reduction of monocyte IL-1, IL-6, IL-8 and lymphocyte IL-2, IL-6 and IL-8 (120). Pioglitazone has also shown a potential for decreasing ferritin in a rat model of angiotensin II-induced hypertension (121). Moreover, pioglitazone can decrease the secretion of pro-inflammatory cytokines (IL-1b, IL-6, and IL-8) and increase the anti-inflammatory ones (e.g. IL-4 and IL-10) in astrocytes stimulated by lipopolysaccharide (122). Pioglitazone (treatment for 7 days) could decrease TNFα and IL-6 mRNA expression in the peritoneal lavage fluid (15, 123). Furthermore, pioglitazone is a well-known inhibitor of lung inflammation and fibrosis (118), through normalization of the expression of TNF-α (124). Pioglitazone and rosiglitazone use can reduce both the increase in inflammatory markers and the decrease in the glutamate transporter (GLT-1) expression, in a primary mixed culture of astrocytes and microglia caused by exposure to in vitro viral proteins (HIVADA gp120) and in vivo models (125). Pioglitazone can decrease the neuro-inflammation and maintain mitochondrial respiration (126). The use of pioglitazone has also produced encouraging results in the form of decreasing CRP and IL-6 levels (127). In animal studies, pioglitazone has been shown to decrease mortality from sepsis and lung injury by reducing inflammatory cytokine production in omental tissue (123).
Opposing Interplay Between the WNT/β-Catenin Pathway and PPARγ
Several studies have shown that the canonical WNT/β-catenin pathway and PPARγ act in an opposing manner in numerous disorders, including cancers, neurodegenerative diseases, psychiatric disorders and fibrosis processes (47, 117, 128). In many diseases, PPARγ expression is downregulated by β-catenin signaling over-activation (12, 13, 48, 129–131). PPARγ agonists are considered to offer promising treatment through this crosstalk process (13, 132, 133). Indeed, PPARγ is considered to be a negative β-catenin target gene (134, 135). The WNT/β-catenin pathway and PPARγ interact through a TCF/LEF domain of β-catenin and a catenin-binding domain within PPARγ (77, 136). Through this link, a decrease in WNT/β-catenin pathway activity enhances the activation of PPARγ (78), while PPARγ over-expression inhibits β-catenin signaling (79).
Opposing Interplay Between the WNT/β-Catenin Pathway and PPARγ in SARS-CoV-2 Infection: The ACE2 Hypothesis
SARS-CoV-2 uses the angiotensin-converting enzyme 2 (ACE2) as a main cell receptor to infect humans (80, 137–139) (Table 1). ACE2 plays a leading role in the regulation of cardiovascular and renal functions (140) and has also been shown to have a major role in SARS-CoV-2 infection (80, 138). SARS-CoV-2 may invade human organs besides the lungs through the expression of ACE2 (141). Recent findings have revealed that ACE2 is downregulated in SARS-CoV-2-infected lung tissue (142). Evidence from studies has shown that SARS-CoV-2 gains direct access to cells through ACE2 receptors (80, 143), as happens with SARS-CoV (144). SARS-CoV-2 infection leads to the downregulation of the expression of ACE2 by binding with the spike (S) viral protein – 1273 amino acid long protein (145). The pivotal role of ACE2 is its degradation of angiotensin II into angiotensin1-7 (146). This degradation mechanism is blocked by a selective ACE2 inhibitor, such as MLN-4760 (147). A recent study focusing on SARS-CoV-2 has shown that angiotensin II accumulation leads to fibrosis, endothelial dysfunction, increased inflammation and oxidative stress (81). Moreover, angiotensin II is associated with macrophage activation and both IL-6 and TNF-α overexpression (148). Furthermore, the deficiency in ACE2 could exacerbate outcomes in COVID-19 patients (148). In COVID-19 patients, ACE2 expression is inversely associated with the WNT/β-catenin and TGF-β pathways (141). ACE2 presents a positive association with PD-L1, a predictive marker for active response to immune inhibitors (142). The stimulation of ACE2 allows it to play a major protective role in the treatment of hypertension, heart disease, cancer and COVID-19 (141), which are all disorders that show an upregulation of the WNT/β-catenin pathway. Rats with renal ischemia/reperfusion-induced injury treated by pioglitazone have shown a downregulated WNT/β-catenin pathway and increased ACE2 expression (82). Even though very few studies have so far highlighted the possible role of PPARγ agonists in treating COVID-19 patients, rosiglitazone has been shown to increase ACE2 expression in animal models (81) and it could also potentially be used in diabetic patients with COVID-19 (85).
PPARγ Agonists in SARS-CoV-2 Infection
To date, few studies have focused on the potentially interesting link between PPARγ agonists and the development of COVID-19. The role of these agonists in SARS-CoV-2 infection therefore remains hypothetical (15, 16) (Table 1). Currently, no clinical and randomized trials have been investigated. However, in a recent research review article it was hypothesized that pioglitazone could play a role in attenuating lung injury in COVID-19 patients (15). Pioglitazone is another available thiazolidinedione that may inhibit the activation of NF-kB and MAPK pathways by reducing the expression of CARD9 in COVID-19 patients (15, 86). Several reports have indicated that PPARγ agonists could be candidates for modulating the cytokine storm in the COVID-19 disease (87, 149, 150). A possible therapeutic role for pioglitazone has been identified with respect to the SARS-CoV-2 infection (16). Pioglitazone can act as a 3CL-Pro inhibitor to downregulate SARS-CoV-2 RNA synthesis and replication (151). More specifically, PPARγ agonists can decrease the secretion of several pro-inflammatory cytokines, including TNF-α, IL-1, and IL-6, in both the monocytes and macrophages (152).
Recent studies have shown that numerous COVID-19 patients present hypertension and diabetes, whereas few patients present chronic obstructive-pulmonary diseases (153, 154). Moreover, a recent meta-analysis showed that hypertension and diabetes were highly associated with comorbidities in COVID-19 patients (155). One of the major roles of PPARγ agonists is to decrease TNF-α expression, the proportion of Th17 cells and NF-κB activity in order to repress inflammation (12). Numerous inflammatory cytokines, chemokines, or intracellular pathways, such as TNF-α and IL-6, can downregulate PPARγ expression, whereas in adipocytes, adiponectin increases PPARγ expression and then downregulates the LPS-induced NF-ϰB expression and IL-6 production (156). Pioglitazone suppresses inflammation by reducing TNF-α and MCP-1 expression, two important mediators of inflammation (157). However, the use of PPARγ agonists may have some side effects, even though newer molecules now have fewer disadvantages. The use of PPARγ agonists may therefore increase cardiovascular events, despite numerous studies showing no significant increase in side effects (14).
Conclusion
In the rapidly evolving situation surrounding the COVID-19 pandemic, it is essential to better understand the different pathways involved in the disease. In the SARS-CoV-2 infection, the canonical WNT/β-catenin pathway seems to be upregulated in association with the TGF-β and STAT pathways, whereas both ACE2 and PPARγ expression is downregulated, coupled with an increased number of pro-inflammatory markers. Since increased WNT/β-catenin pathway activity is associated with the increase of immune signaling and fibrosis processes, the inhibition of this pathway could result in the negative modulation of the SARS-CoV-2 infection. PPARγ agonists provide inexpensive treatments that are commonly used around the globe. By directly targeting inflammation, ACE2 and the WNT/β-catenin pathway, PPARγ agonists may well be prospective candidates for delivering SARS-CoV-2 therapy in clinical settings.
Author Contributions
All authors listed have made substantial, direct, and intellectual contributions to the work and approved it for publication.
Conflict of Interest
The authors declare that the research was conducted in the absence of any commercial or financial relationships that could be construed as a potential conflict of interest.
Acknowledgments
The authors thank Mr. Brian Keogh, PhD, for the proofreading of the manuscript.
References
1. Cascella M, Rajnik M, Cuomo A, Dulebohn SC, Di Napoli R. Features, Evaluation, and Treatment of Coronavirus. In: StatPearls. Treasure Island (FL: StatPearls Publishing. Available at: http://www.ncbi.nlm.nih.gov/books/NBK554776/ (Accessed February 8, 2021).
2. Guan W, Ni Z, Hu Y, Liang W, Ou C, He J, et al. Clinical Characteristics of Coronavirus Disease 2019 in China. N Engl J Med (2020) 382:1708–20. doi: 10.1056/NEJMoa2002032
3. Chen N, Zhou M, Dong X, Qu J, Gong F, Han Y, et al. Epidemiological and clinical characteristics of 99 cases of 2019 novel coronavirus pneumonia in Wuhan, China: a descriptive study. Lancet Lond Engl (2020) 395:507–13. doi: 10.1016/S0140-6736(20)30211-7
4. Mehta P, McAuley DF, Brown M, Sanchez E, Tattersall RS, Manson JJ. HLH Across Speciality Collaboration, UK. COVID-19: consider cytokine storm syndromes and immunosuppression. Lancet Lond Engl (2020) 395:1033–4. doi: 10.1016/S0140-6736(20)30628-0
5. Roumier M, Paule R, Vallée A, Rohmer J, Ballester M, Brun A-L, et al. Tocilizumab for Severe Worsening COVID-19 Pneumonia: a Propensity Score Analysis. J Clin Immunol (2020) 41(2):303–41. doi: 10.1007/s10875-020-00911-6
6. Zhou F, Yu T, Du R, Fan G, Liu Y, Liu Z, et al. Clinical course and risk factors for mortality of adult inpatients with COVID-19 in Wuhan, China: a retrospective cohort study. Lancet Lond Engl (2020) 395:1054–62. doi: 10.1016/S0140-6736(20)30566-3
7. McGonagle D, O’Regan A, Sharif K, Bridgewood C. The Role of Cytokines including Interleukin-6 in COVID-19 induced Pneumonia and Macrophage Activation Syndrome-Like Disease. Autoimmun Rev (2020) 19(6):102537. doi: 10.1016/j.autrev.2020.102537
8. Xu Z, Shi L, Wang Y, Zhang J, Huang L, Zhang C, et al. Pathological findings of COVID-19 associated with acute respiratory distress syndrome. Lancet Respir Med (2020) 8:420–2. doi: 10.1016/S2213-2600(20)30076-X
9. Villar J, Zhang H, Slutsky AS. Lung Repair and Regeneration in ARDS: Role of PECAM1 and Wnt Signaling. Chest (2019) 155:587–94. doi: 10.1016/j.chest.2018.10.022
10. Choi EY, Park HH, Kim H, Kim HN, Kim I, Jeon S, et al. Wnt5a and Wnt11 as acute respiratory distress syndrome biomarkers for severe acute respiratory syndrome coronavirus 2 patients. Eur Respir J (2020) 56:2001531. doi: 10.1183/13993003.01531-2020
11. Vallée A, Lecarpentier Y. Alzheimer Disease: Crosstalk between the Canonical Wnt/Beta-Catenin Pathway and PPARs Alpha and Gamma. Front Neurosci (2016) 10:459. doi: 10.3389/fnins.2016.00459
12. Vallée A, Lecarpentier Y. Crosstalk Between Peroxisome Proliferator-Activated Receptor Gamma and the Canonical WNT/β-Catenin Pathway in Chronic Inflammation and Oxidative Stress During Carcinogenesis. Front Immunol (2018) 9:745. doi: 10.3389/fimmu.2018.00745
13. Vallée A, Vallée J-N, Lecarpentier Y. PPARγ agonists: potential treatment for autism spectrum disorder by inhibiting the canonical WNT/β-catenin pathway. Mol Psychiatry (2018) 24(5):643–52. doi: 10.1038/s41380-018-0131-4
14. Vallée A, Lévy BL, Blacher J. Interplay between the renin-angiotensin system, the canonical WNT/β-catenin pathway and PPARγ in hypertension. Curr Hypertens Rep (2018) 20:62. doi: 10.1007/s11906-018-0860-4
15. Carboni E, Carta AR, Carboni E. Can pioglitazone be potentially useful therapeutically in treating patients with COVID-19? Med Hypotheses (2020) 140:109776. doi: 10.1016/j.mehy.2020.109776
16. Jagat JM, Kalyan KG, Subir R. Use of pioglitazone in people with type 2 diabetes mellitus with coronavirus disease 2019 (COVID-19): Boon or bane? Diabetes Metab Syndr (2020) 14:829–31. doi: 10.1016/j.dsx.2020.06.015
17. Zhang B, Zhou X, Qiu Y, Song Y, Feng F, Feng J, et al. Clinical characteristics of 82 cases of death from COVID-19. PloS One (2020) 15:e0235458. doi: 10.1371/journal.pone.0235458
18. Fu Y, Cheng Y, Wu Y. Understanding SARS-CoV-2-Mediated Inflammatory Responses: From Mechanisms to Potential Therapeutic Tools. Virol Sin (2020) 35:266–71. doi: 10.1007/s12250-020-00207-4
19. Abassi Z, Knaney Y, Karram T, Heyman SN. The Lung Macrophage in SARS-CoV-2 Infection: A Friend or a Foe? Front Immunol (2020) 11:1312. doi: 10.3389/fimmu.2020.01312
20. Barbieri A, Robinson N, Palma G, Maurea N, Desiderio V, Botti G. Can Beta-2-Adrenergic Pathway Be a New Target to Combat SARS-CoV-2 Hyperinflammatory Syndrome?-Lessons Learned From Cancer. Front Immunol (2020) 11:588724. doi: 10.3389/fimmu.2020.588724
21. Perrotta F, Matera MG, Cazzola M, Bianco A. Severe respiratory SARS-CoV2 infection: Does ACE2 receptor matter? Respir Med (2020) 168:105996. doi: 10.1016/j.rmed.2020.105996
22. Siu K-L, Chan C-P, Kok K-H, Chiu-Yat Woo P, Jin D-Y. Suppression of innate antiviral response by severe acute respiratory syndrome coronavirus M protein is mediated through the first transmembrane domain. Cell Mol Immunol (2014) 11:141–9. doi: 10.1038/cmi.2013.61
23. Frieman M, Ratia K, Johnston RE, Mesecar AD, Baric RS. Severe acute respiratory syndrome coronavirus papain-like protease ubiquitin-like domain and catalytic domain regulate antagonism of IRF3 and NF-kappaB signaling. J Virol (2009) 83:6689–705. doi: 10.1128/JVI.02220-08
24. Rios CI, Cassatt DR, Hollingsworth BA, Satyamitra MM, Tadesse YS, Taliaferro LP, et al. Commonalities Between COVID-19 and Radiation Injury. Radiat Res (2021) 195:1–24. doi: 10.1667/RADE-20-00188.1
25. Tian S, Hu W, Niu L, Liu H, Xu H, Xiao S-Y. Pulmonary Pathology of Early-Phase 2019 Novel Coronavirus (COVID-19) Pneumonia in Two Patients With Lung Cancer. J Thorac Oncol Off Publ Int Assoc Study Lung Cancer (2020) 15:700–4. doi: 10.1016/j.jtho.2020.02.010
26. Huang C, Wang Y, Li X, Ren L, Zhao J, Hu Y, et al. Clinical features of patients infected with 2019 novel coronavirus in Wuhan, China. Lancet Lond Engl (2020) 395:497–506. doi: 10.1016/S0140-6736(20)30183-5
27. Liang Y, Zhou Y, Shen P. NF-kappaB and its regulation on the immune system. Cell Mol Immunol (2004) 1:343–50.
28. Zhang X, Wu K, Wang D, Yue X, Song D, Zhu Y, et al. Nucleocapsid protein of SARS-CoV activates interleukin-6 expression through cellular transcription factor NF-kappaB. Virology (2007) 365:324–35. doi: 10.1016/j.virol.2007.04.009
29. Liao Q-J, Ye L-B, Timani KA, Zeng Y-C, She Y-L, Ye L, et al. Activation of NF-kappaB by the full-length nucleocapsid protein of the SARS coronavirus. Acta Biochim Biophys Sin (2005) 37:607–12. doi: 10.1111/j.1745-7270.2005.00082.x
30. DeDiego ML, Nieto-Torres JL, Regla-Nava JA, Jimenez-Guardeño JM, Fernandez-Delgado R, Fett C, et al. Inhibition of NF-κB-mediated inflammation in severe acute respiratory syndrome coronavirus-infected mice increases survival. J Virol (2014) 88:913–24. doi: 10.1128/JVI.02576-13
31. Siu K-L, Yuen K-S, Castaño-Rodriguez C, Ye Z-W, Yeung M-L, Fung S-Y, et al. Severe acute respiratory syndrome coronavirus ORF3a protein activates the NLRP3 inflammasome by promoting TRAF3-dependent ubiquitination of ASC. FASEB J Off Publ Fed Am Soc Exp Biol (2019) 33:8865–77. doi: 10.1096/fj.201802418R
32. Celik O, Celik N, Aydin S, Baysal B, Aydin S, Saglam A, et al. Combating sars-cov-2 through lipoxins, proteasome, caveolin and nuclear factor-κb pathways in non-pregnant and pregnant populations. Cell Mol Biol Noisy–Gd Fr (2020) 66:221–9. doi: 10.14715/cmb/2020.66.3.36
33. Loh KM, van Amerongen R, Nusse R. Generating Cellular Diversity and Spatial Form: Wnt Signaling and the Evolution of Multicellular Animals. Dev Cell (2016) 38:643–55. doi: 10.1016/j.devcel.2016.08.011
34. Oren O, Smith BD. Eliminating Cancer Stem Cells by Targeting Embryonic Signaling Pathways. Stem Cell Rev (2017) 13:17–23. doi: 10.1007/s12015-016-9691-3
35. Clevers H, Nusse R. Wnt/β-catenin signaling and disease. Cell (2012) 149:1192–205. doi: 10.1016/j.cell.2012.05.012
36. Inestrosa NC, Montecinos-Oliva C, Fuenzalida M. Wnt signaling: role in Alzheimer disease and schizophrenia. J Neuroimmune Pharmacol Off J Soc NeuroImmune Pharmacol (2012) 7:788–807. doi: 10.1007/s11481-012-9417-5
37. Ma B, Hottiger MO. Crosstalk between Wnt/β-Catenin and NF-κB Signaling Pathway during Inflammation. Front Immunol (2016) 7:378. doi: 10.3389/fimmu.2016.00378
38. Vallée A, Lecarpentier Y, Vallée J-N. Targeting the Canonical WNT/β-Catenin Pathway in Cancer Treatment Using Non-Steroidal Anti-Inflammatory Drugs. Cells (2019) 8:726. doi: 10.3390/cells8070726
39. Sorcini D, Bruscoli S, Frammartino T, Cimino M, Mazzon E, Galuppo M, et al. Wnt/β-Catenin Signaling Induces Integrin α4β1 in T Cells and Promotes a Progressive Neuroinflammatory Disease in Mice. J Immunol (2017) 199:3031–41. doi: 10.4049/jimmunol.1700247
40. De Ferrari GV, Avila ME, Medina MA, Perez-Palma E, Bustos BI, Alarcon MA. Wnt/β-catenin signaling in Alzheimer’s disease. CNS Neurol Disord Drug Targets (2014) 13:745–54. doi: 10.2174/1871527312666131223113900
41. Fancy SPJ, Baranzini SE, Zhao C, Yuk D-I, Irvine K-A, Kaing S, et al. Dysregulation of the Wnt pathway inhibits timely myelination and remyelination in the mammalian CNS. Genes Dev (2009) 23:1571–85. doi: 10.1101/gad.1806309
42. Giacoppo S, Bereshchenko O, Bruscoli S, Riccardi C, Bramanti P, Mazzon E. Aberrant expression of β-catenin in CD4+ T cells isolated from primary progressive multiple sclerosis patients. Neurosci Lett (2017) 653:159–62. doi: 10.1016/j.neulet.2017.05.057
43. Al-Harthi L. Wnt/β-catenin and its Diverse Physiological Cell Signaling Pathways in Neurodegenerative and Neuropsychiatric Disorders. J Neuroimmune Pharmacol (2012) 7:725–30. doi: 10.1007/s11481-012-9412-x
44. Marchetti B, Pluchino S. Wnt your brain be inflamed? Yes, it Wnt! Trends Mol Med (2013) 19:144–56. doi: 10.1016/j.molmed.2012.12.001
45. Lecarpentier Y, Claes V, Duthoit G, Hébert J-L. Circadian rhythms, Wnt/beta-catenin pathway and PPAR alpha/gamma profiles in diseases with primary or secondary cardiac dysfunction. Front Physiol (2014) 5:429. doi: 10.3389/fphys.2014.00429
46. Lecarpentier Y, Vallée A. Opposite Interplay between PPAR Gamma and Canonical Wnt/Beta-Catenin Pathway in Amyotrophic Lateral Sclerosis. Front Neurol (2016) 7:100. doi: 10.3389/fneur.2016.00100
47. Vallée A, Lecarpentier Y, Guillevin R, Vallée J-N. Thermodynamics in Neurodegenerative Diseases: Interplay Between Canonical WNT/Beta-Catenin Pathway-PPAR Gamma, Energy Metabolism and Circadian Rhythms. Neuromolecular Med (2018) 20:174–204. doi: 10.1007/s12017-018-8486-x
48. Vallée A, Vallée J-N, Lecarpentier Y. Parkinson’s Disease: Potential Actions of Lithium by Targeting the WNT/β-Catenin Pathway, Oxidative Stress, Inflammation and Glutamatergic Pathway. Cells (2021) 10:230. doi: 10.3390/cells10020230
49. He TC, Sparks AB, Rago C, Hermeking H, Zawel L, da Costa LT, et al. Identification of c-MYC as a target of the APC pathway. Science (1998) 281:1509–12. doi: 10.1126/science.281.5382.1509
50. Shtutman M, Zhurinsky J, Simcha I, Albanese C, D’Amico M, Pestell R, et al. The cyclin D1 gene is a target of the beta-catenin/LEF-1 pathway. Proc Natl Acad Sci USA (1999) 96:5522–7. doi: 10.1073/pnas.96.10.5522
51. Angers S, Moon RT. Proximal events in Wnt signal transduction. Nat Rev Mol Cell Biol (2009) 10(7):468–77. doi: 10.1038/nrm2717
52. Sharma C, Pradeep A, Wong L, Rana A, Rana B. Peroxisome proliferator-activated receptor gamma activation can regulate beta-catenin levels via a proteasome-mediated and adenomatous polyposis coli-independent pathway. J Biol Chem (2004) 279:35583–94. doi: 10.1074/jbc.M403143200
53. Rosi MC, Luccarini I, Grossi C, Fiorentini A, Spillantini MG, Prisco A, et al. Increased Dickkopf-1 expression in transgenic mouse models of neurodegenerative disease. J Neurochem (2010) 112:1539–51. doi: 10.1111/j.1471-4159.2009.06566.x
54. Vallée A, Lecarpentier Y, Guillevin R, Vallée J-N. Interactions between TGF-β1, canonical WNT/β-catenin pathway and PPAR γ in radiation-induced fibrosis. Oncotarget (2017) 8:90579–604. doi: 10.18632/oncotarget.21234
55. Vallée A, Lecarpentier Y, Vallée J-N. Hypothesis of Opposite Interplay Between the Canonical WNT/beta-catenin Pathway and PPAR Gamma in Primary Central Nervous System Lymphomas. Curr Issues Mol Biol (2019) 31:1–20. doi: 10.21775/cimb.031.001
56. Aberle H, Bauer A, Stappert J, Kispert A, Kemler R. β-catenin is a target for the ubiquitin–proteasome pathway. EMBO J (1997) 16:3797–804. doi: 10.1093/emboj/16.13.3797
57. Wu D, Pan W. GSK3: a multifaceted kinase in Wnt signaling. Trends Biochem Sci (2010) 35:161–8. doi: 10.1016/j.tibs.2009.10.002
58. Hur E-M, Zhou F-Q. GSK3 signalling in neural development. Nat Rev Neurosci (2010) 11:539–51. doi: 10.1038/nrn2870
59. Ambacher KK, Pitzul KB, Karajgikar M, Hamilton A, Ferguson SS, Cregan SP. The JNK- and AKT/GSK3β- Signaling Pathways Converge to Regulate Puma Induction and Neuronal Apoptosis Induced by Trophic Factor Deprivation. PloS One (2012) 7:e46885. doi: 10.1371/journal.pone.0046885
60. Orellana AMM, Vasconcelos AR, Leite JA, de Sá Lima L, Andreotti DZ, Munhoz CD, et al. Age-related neuroinflammation and changes in AKT-GSK-3β and WNT/ β-CATENIN signaling in rat hippocampus. Aging (2015) 7:1094–111. doi: 10.18632/aging.100853
61. Spiegelman VS, Slaga TJ, Pagano M, Minamoto T, Ronai Z, Fuchs SY. Wnt/beta-catenin signaling induces the expression and activity of betaTrCP ubiquitin ligase receptor. Mol Cell (2000) 5:877–82. doi: 10.1016/S1097-2765(00)80327-5
62. Phelps RA, Broadbent TJ, Stafforini DM, Jones DA. New perspectives on APC control of cell fate and proliferation in colorectal cancer. Cell Cycle Georget Tex (2009) 8:2549–56. doi: 10.4161/cc.8.16.9278
63. Saegusa M, Hashimura M, Kuwata T, Hamano M, Okayasu I. Crosstalk between NF-kappaB/p65 and beta-catenin/TCF4/p300 signalling pathways through alterations in GSK-3beta expression during trans-differentiation of endometrial carcinoma cells. J Pathol (2007) 213:35–45. doi: 10.1002/path.2198
64. Buss H, Dörrie A, Schmitz ML, Frank R, Livingstone M, Resch K, et al. Phosphorylation of serine 468 by GSK-3beta negatively regulates basal p65 NF-kappaB activity. J Biol Chem (2004) 279:49571–4. doi: 10.1074/jbc.C400442200
65. Staal FJT, Luis TC, Tiemessen MM. WNT signalling in the immune system: WNT is spreading its wings. Nat Rev Immunol (2008) 8:581–93. doi: 10.1038/nri2360
66. Villar J, Cabrera-Benítez NE, Ramos-Nuez A, Flores C, García-Hernández S, Valladares F, et al. Early activation of pro-fibrotic WNT5A in sepsis-induced acute lung injury. Crit Care Lond Engl (2014) 18:568. doi: 10.1186/s13054-014-0568-z
67. Gatica-Andrades M, Vagenas D, Kling J, Nguyen TTK, Benham H, Thomas R, et al. WNT ligands contribute to the immune response during septic shock and amplify endotoxemia-driven inflammation in mice. Blood Adv (2017) 1:1274–86. doi: 10.1182/bloodadvances.2017006163
68. Chae W-J, Bothwell ALM. Canonical and Non-Canonical Wnt Signaling in Immune Cells. Trends Immunol (2018) 39:830–47. doi: 10.1016/j.it.2018.08.006
69. Pereira C, Schaer DJ, Bachli EB, Kurrer MO, Schoedon G. Wnt5A/CaMKII signaling contributes to the inflammatory response of macrophages and is a target for the antiinflammatory action of activated protein C and interleukin-10. Arterioscler Thromb Vasc Biol (2008) 28:504–10. doi: 10.1161/ATVBAHA.107.157438
70. Newman DR, Sills WS, Hanrahan K, Ziegler A, Tidd KM, Cook E, et al. Expression of WNT5A in Idiopathic Pulmonary Fibrosis and Its Control by TGF-β and WNT7B in Human Lung Fibroblasts. J Histochem Cytochem Off J Histochem Soc (2016) 64:99–111. doi: 10.1369/0022155415617988
71. Shen B, Yi X, Sun Y, Bi X, Du J, Zhang C, et al. Proteomic and Metabolomic Characterization of COVID-19 Patient Sera. Cell (2020) 182:59–72.e15. doi: 10.1016/j.cell.2020.05.032
72. Kang S, Tanaka T, Narazaki M, Kishimoto T. Targeting Interleukin-6 Signaling in Clinic. Immunity (2019) 50:1007–23. doi: 10.1016/j.immuni.2019.03.026
73. Zhu C, Shen H, Zhu L, Zhao F, Shu Y. Plasminogen Activator Inhibitor 1 Promotes Immunosuppression in Human Non-Small Cell Lung Cancers by Enhancing TGF-B1 Expression in Macrophage. Cell Physiol Biochem Int J Exp Cell Physiol Biochem Pharmacol (2017) 44:2201–11. doi: 10.1159/000486025
74. Kutz SM, Hordines J, McKeown-Longo PJ, Higgins PJ. TGF-beta1-induced PAI-1 gene expression requires MEK activity and cell-to-substrate adhesion. J Cell Sci (2001) 114:3905–14.
75. Matsuyama T, Kubli SP, Yoshinaga SK, Pfeffer K, Mak TW. An aberrant STAT pathway is central to COVID-19. Cell Death Differ (2020) 27:3209–25. doi: 10.1038/s41418-020-00633-7
76. Lodyga M, Hinz B. TGF-β1 - A truly transforming growth factor in fibrosis and immunity. Semin Cell Dev Biol (2020) 101:123–39. doi: 10.1016/j.semcdb.2019.12.010
77. Lu D, Carson DA. Repression of beta-catenin signaling by PPAR gamma ligands. Eur J Pharmacol (2010) 636:198–202. doi: 10.1016/j.ejphar.2010.03.010
78. Garcia-Gras E, Lombardi R, Giocondo MJ, Willerson JT, Schneider MD, Khoury DS, et al. Suppression of canonical Wnt/beta-catenin signaling by nuclear plakoglobin recapitulates phenotype of arrhythmogenic right ventricular cardiomyopathy. J Clin Invest (2006) 116:2012–21. doi: 10.1172/JCI27751
79. Moldes M, Zuo Y, Morrison RF, Silva D, Park B-H, Liu J, et al. Peroxisome-proliferator-activated receptor gamma suppresses Wnt/beta-catenin signalling during adipogenesis. Biochem J (2003) 376:607–13. doi: 10.1042/BJ20030426
80. Hoffmann M, Kleine-Weber H, Schroeder S, Krüger N, Herrler T, Erichsen S, et al. SARS-CoV-2 Cell Entry Depends on ACE2 and TMPRSS2 and Is Blocked by a Clinically Proven Protease Inhibitor. Cell (2020) 181:271–280.e8. doi: 10.1016/j.cell.2020.02.052
81. Zhang H, Penninger JM, Li Y, Zhong N, Slutsky AS. Angiotensin-converting enzyme 2 (ACE2) as a SARS-CoV-2 receptor: molecular mechanisms and potential therapeutic target. Intensive Care Med (2020) 46:586–90. doi: 10.1007/s00134-020-05985-9
82. Ali RM, Al-Shorbagy MY, Helmy MW, El-Abhar HS. Role of Wnt4/β-catenin, Ang II/TGFβ, ACE2, NF-κB, and IL-18 in attenuating renal ischemia/reperfusion-induced injury in rats treated with Vit D and pioglitazone. Eur J Pharmacol (2018) 831:68–76. doi: 10.1016/j.ejphar.2018.04.032
83. Sánchez-Aguilar M, Ibarra-Lara L, Del Valle-Mondragón L, Rubio-Ruiz ME, Aguilar-Navarro AG, Zamorano-Carrillo A, et al. Rosiglitazone, a Ligand to PPARγ, Improves Blood Pressure and Vascular Function through Renin-Angiotensin System Regulation. PPAR Res (2019) 2019:1371758. doi: 10.1155/2019/1371758
84. Filardi T, Morano S. COVID-19: is there a link between the course of infection and pharmacological agents in diabetes? J Endocrinol Invest (2020) 43:1053–60. doi: 10.1007/s40618-020-01318-1
85. Ceriello A, Standl E, Catrinoiu D, Itzhak B, Lalic NM, Rahelic D, et al. Diabetes and Cardiovascular Disease (D&CVD) EASD Study Group. Issues of Cardiovascular Risk Management in People With Diabetes in the COVID-19 Era. Diabetes Care (2020) 43:1427–32. doi: 10.2337/dc20-0941
86. Erol A. Role of oxidized LDL-induced “trained macrophages” in the pathogenesis of COVID-19 and benefits of pioglitazone: A hypothesis. Diabetes Metab Syndr (2020) 14:713–4. doi: 10.1016/j.dsx.2020.05.007
87. Ciavarella C, Motta I, Valente S, Pasquinelli G. Pharmacological (or Synthetic) and Nutritional Agonists of PPAR-γ as Candidates for Cytokine Storm Modulation in COVID-19 Disease. Molecules (2020) 25:2076. doi: 10.3390/molecules25092076
88. Vallée A, Lecarpentier Y. TGF-β in fibrosis by acting as a conductor for contractile properties of myofibroblasts. Cell Biosci (2019) 9:98. doi: 10.1186/s13578-019-0362-3
89. Khalil N, Parekh TV, O’Connor R, Antman N, Kepron W, Yehaulaeshet T, et al. Regulation of the effects of TGF-beta 1 by activation of latent TGF-beta 1 and differential expression of TGF-beta receptors (T beta R-I and T beta R-II) in idiopathic pulmonary fibrosis. Thorax (2001) 56:907–15. doi: 10.1136/thorax.56.12.907
90. Watanabe-Takano H, Takano K, Hatano M, Tokuhisa T, Endo T. DA-Raf-Mediated Suppression of the Ras–ERK Pathway Is Essential for TGF-β1-Induced Epithelial-Mesenchymal Transition in Alveolar Epithelial Type 2 Cells. PloS One (2015) 10:e0127888. doi: 10.1371/journal.pone.0127888
91. Zhao X, Nicholls JM, Chen Y-G. Severe acute respiratory syndrome-associated coronavirus nucleocapsid protein interacts with Smad3 and modulates transforming growth factor-beta signaling. J Biol Chem (2008) 283:3272–80. doi: 10.1074/jbc.M708033200
92. Villarino AV, Kanno Y, Ferdinand JR, O’Shea JJ. Mechanisms of Jak/STAT signaling in immunity and disease. J Immunol Baltim Md 1950 (2015) 194:21–7. doi: 10.4049/jimmunol.1401867
93. Lee M, Rhee I. Cytokine Signaling in Tumor Progression. Immune Netw (2017) 17:214–27. doi: 10.4110/in.2017.17.4.214
94. Seif F, Khoshmirsafa M, Aazami H, Mohsenzadegan M, Sedighi G, Bahar M. The role of JAK-STAT signaling pathway and its regulators in the fate of T helper cells. Cell Commun Signal CCS (2017) 15:23. doi: 10.1186/s12964-017-0177-y
95. Garbers C, Heink S, Korn T, Rose-John S. Interleukin-6: designing specific therapeutics for a complex cytokine. Nat Rev Drug Discovery (2018) 17:395–412. doi: 10.1038/nrd.2018.45
96. Ruan Q, Yang K, Wang W, Jiang L, Song J. Clinical predictors of mortality due to COVID-19 based on an analysis of data of 150 patients from Wuhan, China. Intensive Care Med (2020) 46(5):846–8. doi: 10.1007/s00134-020-05991-x
97. Luo W, Li YX, Jiang Lj, Chen Q, Wang T, Ye DW. Targeting JAK-STAT Signaling to Control Cytokine Release Syndrome in COVID-19. Trends Pharmacol Sci (2020) 41:531–43. doi: 10.1016/j.tips.2020.06.007
98. Aydemir MN, Aydemir HB, Korkmaz EM, Budak M, N Cekin E. Pinarbasi. Computationally predicted SARS-COV-2 encoded microRNAs target NFKB, JAK/STAT and TGFB signaling pathways. Gene Rep (2021) 22:101012. doi: 10.1016/j.genrep.2020.101012
99. Han H, Ma Q, Li C, Liu R, Zhao L, Wang W, et al. Profiling serum cytokines in COVID-19 patients reveals IL-6 and IL-10 are disease severity predictors. Emerg Microbes Infect (2020) 9:1123–30. doi: 10.1080/22221751.2020.1770129
100. McQuattie-Pimentel AC, Budinger GRS, Ballinger MN. Monocyte-derived Alveolar Macrophages: The Dark Side of Lung Repair? Am J Respir Cell Mol Biol (2018) 58:5–6. doi: 10.1165/rcmb.2017-0328ED
101. Gupta KK, Xu Z, Castellino FJ, Ploplis VA. Plasminogen activator inhibitor-1 stimulates macrophage activation through Toll-like Receptor-4. Biochem Biophys Res Commun (2016) 477:503–8. doi: 10.1016/j.bbrc.2016.06.065
102. Xiong Y, Liu Y, Cao L, Wang D, Guo M, Jiang A, et al. Transcriptomic characteristics of bronchoalveolar lavage fluid and peripheral blood mononuclear cells in COVID-19 patients. Emerg Microbes Infect (2020) 9:761–70. doi: 10.1080/22221751.2020.1747363
103. Chen W. A potential treatment of COVID-19 with TGF-β blockade. Int J Biol Sci (2020) 16:1954–5. doi: 10.7150/ijbs.46891
104. Bao Q, Zhang B, Suo Y, Liu C, Yang Q, Zhang K, et al. Intermittent hypoxia mediated by TSP1 dependent on STAT3 induces cardiac fibroblast activation and cardiac fibrosis. eLife (2020) 9:9e49923. doi: 10.7554/eLife.49923
105. Walton KL, Johnson KE, Harrison CA. Targeting TGF-β Mediated SMAD Signaling for the Prevention of Fibrosis. Front Pharmacol (2017) 8:461. doi: 10.3389/fphar.2017.00461
106. Zuo W, Zhao X, Chen Y-G. SARS Coronavirus and Lung Fibrosis. Mol Biol SARS-Coronavirus (2009) 22:247–58. doi: 10.1007/978-3-642-03683-5_15
107. Smirnov AN. Nuclear receptors: nomenclature, ligands, mechanisms of their effects on gene expression. Biochem Biokhimiia (2002) 67:957–77. doi: 10.1023/A:1020545200302
108. Kota BP, Huang TH-W, Roufogalis BD. An overview on biological mechanisms of PPARs. Pharmacol Res (2005) 51:85–94. doi: 10.1016/j.phrs.2004.07.012
109. Lee C-H, Olson P, Evans RM. Minireview: lipid metabolism, metabolic diseases, and peroxisome proliferator-activated receptors. Endocrinology (2003) 144:2201–7. doi: 10.1210/en.2003-0288
110. Marx N, Duez H, Fruchart J-C, Staels B. Peroxisome proliferator-activated receptors and atherogenesis: regulators of gene expression in vascular cells. Circ Res (2004) 94:1168–78. doi: 10.1161/01.RES.0000127122.22685.0A
111. Cunard R, Ricote M, DiCampli D, Archer DC, Kahn DA, Glass CK, et al. Regulation of cytokine expression by ligands of peroxisome proliferator activated receptors. J Immunol Baltim Md 1950 (2002) 168:2795–802. doi: 10.4049/jimmunol.168.6.2795
112. Ricote M, Li AC, Willson TM, Kelly CJ, Glass CK. The peroxisome proliferator-activated receptor-gamma is a negative regulator of macrophage activation. Nature (1998) 391:79–82. doi: 10.1038/34178
113. Brown JD, Plutzky J. Peroxisome proliferator-activated receptors as transcriptional nodal points and therapeutic targets. Circulation (2007) 115:518–33. doi: 10.1161/CIRCULATIONAHA.104.475673
114. Ivanova EA, Parolari A, Myasoedova V, Melnichenko AA, Bobryshev YV, Orekhov AN. Peroxisome proliferator-activated receptor (PPAR) gamma in cardiovascular disorders and cardiovascular surgery. J Cardiol (2015) 66:271–8. doi: 10.1016/j.jjcc.2015.05.004
115. Giannini S, Serio M, Galli A. Pleiotropic effects of thiazolidinediones: taking a look beyond antidiabetic activity. J Endocrinol Invest (2004) 27:982–91. doi: 10.1007/BF03347546
116. Rogue A, Spire C, Brun M, Claude N, Guillouzo A. Gene Expression Changes Induced by PPAR Gamma Agonists in Animal and Human Liver. PPAR Res (2010) 2010:325183. doi: 10.1155/2010/325183
117. Vallée A, Lecarpentier Y, Vallée J-N. Thermodynamic Aspects and Reprogramming Cellular Energy Metabolism during the Fibrosis Process. Int J Mol Sci (2017) 18:2537. doi: 10.3390/ijms18122537
118. Aoki Y, Maeno T, Aoyagi K, Ueno M, Aoki F, Aoki N, et al. Pioglitazone, a peroxisome proliferator-activated receptor gamma ligand, suppresses bleomycin-induced acute lung injury and fibrosis. Respir Int Rev Thorac Dis (2009) 77:311–9. doi: 10.1159/000168676
119. Xie X, Sinha S, Yi Z, Langlais PR, Madan M, Bowen BP, et al. Role of adipocyte mitochondria in inflammation, lipemia and insulin sensitivity in humans: effects of pioglitazone treatment. Int J Obes 2005 (2017) 14:10. doi: 10.1038/ijo.2017.192
120. Zhang W-Y, Schwartz EA, Permana PA, Reaven PD. Pioglitazone inhibits the expression of inflammatory cytokines from both monocytes and lymphocytes in patients with impaired glucose tolerance. Arterioscler Thromb Vasc Biol (2008) 28:2312–8. doi: 10.1161/ATVBAHA.108.175687
121. Sakamoto A, Hongo M, Saito K, Nagai R, Ishizaka N. Reduction of renal lipid content and proteinuria by a PPAR-γ agonist in a rat model of angiotensin II-induced hypertension. Eur J Pharmacol (2012) 682:131–6. doi: 10.1016/j.ejphar.2012.02.027
122. Qiu D, Li X-N. Pioglitazone inhibits the secretion of proinflammatory cytokines and chemokines in astrocytes stimulated with lipopolysaccharide. Int J Clin Pharmacol Ther (2015) 53:746–52. doi: 10.5414/CP202339
123. Kutsukake M, Matsutani T, Tamura K, Matsuda A, Kobayashi M, Tachikawa E, et al. Pioglitazone attenuates lung injury by modulating adipose inflammation. J Surg Res (2014) 189:295–303. doi: 10.1016/j.jss.2014.03.007
124. Barbarin V, Nihoul A, Misson P, Arras M, Delos M, Leclercq I, et al. The role of pro- and anti-inflammatory responses in silica-induced lung fibrosis. Respir Res (2005) 6:112. doi: 10.1186/1465-9921-6-112
125. Omeragic A, Hoque MT, Choi U-Y, Bendayan R. Peroxisome proliferator-activated receptor-gamma: potential molecular therapeutic target for HIV-1-associated brain inflammation. J Neuroinflamm (2017) 14:183. doi: 10.1186/s12974-017-0957-8
126. Patel SP, Cox DH, Gollihue JL, Bailey WM, Geldenhuys WJ, Gensel JC, et al. Pioglitazone treatment following spinal cord injury maintains acute mitochondrial integrity and increases chronic tissue sparing and functional recovery. Exp Neurol (2017) 293:74–82. doi: 10.1016/j.expneurol.2017.03.021
127. Agarwal R. Anti-inflammatory effects of short-term pioglitazone therapy in men with advanced diabetic nephropathy. Am J Physiol Renal Physiol (2006) 290:F600–605. doi: 10.1152/ajprenal.00289.2005
128. Vallée A, Lecarpentier Y, Guillevin R, Vallée J-N. Thermodynamics in Gliomas: Interactions between the Canonical WNT/Beta-Catenin Pathway and PPAR Gamma. Front Physiol (2017) 8:352. doi: 10.3389/fphys.2017.00352
129. Jeon K-I, Kulkarni A, Woeller CF, Phipps RP, Sime PJ, Hindman HB, et al. Inhibitory effects of PPARγ ligands on TGF-β1-induced corneal myofibroblast transformation. Am J Pathol (2014) 184:1429–45. doi: 10.1016/j.ajpath.2014.01.026
130. Lee Y, Kim SH, Lee YJ, Kang ES, Lee B-W, Cha BS, et al. Transcription factor Snail is a novel regulator of adipocyte differentiation via inhibiting the expression of peroxisome proliferator-activated receptor γ. Cell Mol Life Sci CMLS (2013) 70:3959–71. doi: 10.1007/s00018-013-1363-8
131. Qian J, Niu M, Zhai X, Zhou Q, Zhou Y. β-Catenin pathway is required for TGF-β1 inhibition of PPARγ expression in cultured hepatic stellate cells. Pharmacol Res (2012) 66:219–25. doi: 10.1016/j.phrs.2012.06.003
132. Sabatino L, Pancione M, Votino C, Colangelo T, Lupo A, Novellino E, et al. Emerging role of the β-catenin-PPARγ axis in the pathogenesis of colorectal cancer. World J Gastroenterol (2014) 20:7137–51. doi: 10.3748/wjg.v20.i23.7137
133. Vallée A, Lecarpentier Y, Guillevin R, Vallée J-N. Opposite Interplay Between the Canonical WNT/β-Catenin Pathway and PPAR Gamma: A Potential Therapeutic Target in Gliomas. Neurosci Bull (2018) 34(3):573–88. doi: 10.1007/s12264-018-0219-5
134. Ajmone-Cat MA, D’Urso MC, di Blasio G, Brignone MS, De Simone R, Minghetti L. Glycogen synthase kinase 3 is part of the molecular machinery regulating the adaptive response to LPS stimulation in microglial cells. Brain Behav Immun (2016) 55:225–35. doi: 10.1016/j.bbi.2015.11.012
135. Jansson EA, Are A, Greicius G, Kuo I-C, Kelly D, Arulampalam V, et al. The Wnt/beta-catenin signaling pathway targets PPARgamma activity in colon cancer cells. Proc Natl Acad Sci USA (2005) 102:1460–5. doi: 10.1073/pnas.0405928102
136. Liu J, Wang H, Zuo Y, Farmer SR. Functional interaction between peroxisome proliferator-activated receptor gamma and beta-catenin. Mol Cell Biol (2006) 26:5827–37. doi: 10.1128/MCB.00441-06
137. Lan J, Ge J, Yu J, Shan S, Zhou H, Fan S, et al. Structure of the SARS-CoV-2 spike receptor-binding domain bound to the ACE2 receptor. Nature (2020) 581:215–20. doi: 10.1038/s41586-020-2180-5
138. Wang Q, Zhang Y, Wu L, Niu S, Song C, Zhang Z, et al. Structural and Functional Basis of SARS-CoV-2 Entry by Using Human ACE2. Cell (2020) 181:894–904.e9. doi: 10.1016/j.cell.2020.03.045
139. Badawi S, Ali BR. ACE2 Nascence, trafficking, and SARS-CoV-2 pathogenesis: the saga continues. Hum Genomics (2021) 15:8. doi: 10.1186/s40246-021-00304-9
140. Danilczyk U, Penninger JM. Angiotensin-converting enzyme II in the heart and the kidney. Circ Res (2006) 98:463–71. doi: 10.1161/01.RES.0000205761.22353.5f
141. Zhang Z, Li L, Li M, Wang X. The SARS-CoV-2 host cell receptor ACE2 correlates positively with immunotherapy response and is a potential protective factor for cancer progression. Comput Struct Biotechnol J (2020) 18:2438–44. doi: 10.1016/j.csbj.2020.08.024
142. Yang J, Li H, Hu S, Zhou Y. ACE2 correlated with immune infiltration serves as a prognostic biomarker in endometrial carcinoma and renal papillary cell carcinoma: implication for COVID-19. Aging (2020) 12:6518–35. doi: 10.18632/aging.103100
143. Duan L, Zheng Q, Zhang H, Niu Y, Lou Y, Wang H. The SARS-CoV-2 Spike Glycoprotein Biosynthesis, Structure, Function, and Antigenicity: Implications for the Design of Spike-Based Vaccine Immunogens. Front Immunol (2020) 11:576622. doi: 10.3389/fimmu.2020.576622
144. Li W, Moore MJ, Vasilieva N, Sui J, Wong SK, Berne MA, et al. Angiotensin-converting enzyme 2 is a functional receptor for the SARS coronavirus. Nature (2003) 426:450–4. doi: 10.1038/nature02145
145. Smith JC, Sausville EL, Girish V, Yuan ML, Vasudevan A, John KM, et al. Cigarette Smoke Exposure and Inflammatory Signaling Increase the Expression of the SARS-CoV-2 Receptor ACE2 in the Respiratory Tract. Dev Cell (2020) 53:514–29.e3. doi: 10.1016/j.devcel.2020.05.012
146. Turner AJ, Hiscox JA, Hooper NM. ACE2: from vasopeptidase to SARS virus receptor. Trends Pharmacol Sci (2004) 25:291–4. doi: 10.1016/j.tips.2004.04.001
147. Trask AJ, Averill DB, Ganten D, Chappell MC, Ferrario CM. Primary role of angiotensin-converting enzyme-2 in cardiac production of angiotensin-(1-7) in transgenic Ren-2 hypertensive rats. Am J Physiol Heart Circ Physiol (2007) 292:H3019–24. doi: 10.1152/ajpheart.01198.2006
148. Verdecchia P, Cavallini C, Spanevello A, Angeli F. The pivotal link between ACE2 deficiency and SARS-CoV-2 infection. Eur J Intern Med (2020) 76:14–20. doi: 10.1016/j.ejim.2020.04.037
149. Costa FF, Rosário WR, Ribeiro Farias AC, de Souza RG, Duarte Gondim RS, Barroso WA. Metabolic syndrome and COVID-19: An update on the associated comorbidities and proposed therapies. Diabetes Metab Syndr (2020) 14:809–14. doi: 10.1016/j.dsx.2020.06.016
150. Francisqueti-Ferron FV, Garcia JL, Ferron AJT, Nakandakare-Maia ET, Gregolin CS, Silva JP das C, et al. Gamma-oryzanol as a potential modulator of oxidative stress and inflammation via PPAR-y in adipose tissue: a hypothetical therapeutic for cytokine storm in COVID-19? Mol Cell Endocrinol (2021) 520:111095. doi: 10.1016/j.mce.2020.111095
151. Wu C, Liu Y, Yang Y, Zhang P, Zhong W, Wang Y, et al. Analysis of therapeutic targets for SARS-CoV-2 and discovery of potential drugs by computational methods. Acta Pharm Sin B (2020) 10:766–88. doi: 10.1016/j.apsb.2020.02.008
152. Bassaganya-Riera J, Song R, Roberts PC, Hontecillas R. PPAR-gamma activation as an anti-inflammatory therapy for respiratory virus infections. Viral Immunol (2010) 23:343–52. doi: 10.1089/vim.2010.0016
153. Li B, Yang J, Zhao F, Zhi L, Wang X, Liu L, et al. Prevalence and impact of cardiovascular metabolic diseases on COVID-19 in China. Clin Res Cardiol Off J Ger Card Soc (2020) 109:531–8. doi: 10.1007/s00392-020-01626-9
154. Zhang J-J, Dong X, Cao Y-Y, Yuan Y-D, Yang Y-B, Yan Y-Q, et al. Clinical characteristics of 140 patients infected with SARS-CoV-2 in Wuhan, China. Allergy (2020) 75(7):1730–41. doi: 10.1111/all.14238
155. Yang J, Zheng Y, Gou X, Pu K, Chen Z, Guo Q, et al. Prevalence of comorbidities and its effects in coronavirus disease 2019 patients: A systematic review and meta-analysis. Int J Infect Dis IJID Off Publ Int Soc Infect Dis (2020) 94:91–5. doi: 10.1016/j.ijid.2020.03.017
156. Ajuwon KM, Spurlock ME. Adiponectin inhibits LPS-induced NF-kappaB activation and IL-6 production and increases PPARgamma2 expression in adipocytes. Am J Physiol Regul Integr Comp Physiol (2005) 288:R1220–5. doi: 10.1152/ajpregu.00397.2004
Keywords: COVID-19, WNT/β-catenin pathway, PPARγ, ACE2, cytokine storm
Citation: Vallée A, Lecarpentier Y and Vallée J-N (2021) Interplay of Opposing Effects of the WNT/β-Catenin Pathway and PPARγ and Implications for SARS-CoV2 Treatment. Front. Immunol. 12:666693. doi: 10.3389/fimmu.2021.666693
Received: 10 February 2021; Accepted: 26 March 2021;
Published: 13 April 2021.
Edited by:
Matthijs Kox, Radboud University Nijmegen Medical Centre, NetherlandsReviewed by:
Oxana Bereshchenko, University of Perugia, ItalyCassiano Felippe Gonçalves-de-Albuquerque, Rio de Janeiro State Federal University, Brazil
Copyright © 2021 Vallée, Lecarpentier and Vallée. This is an open-access article distributed under the terms of the Creative Commons Attribution License (CC BY). The use, distribution or reproduction in other forums is permitted, provided the original author(s) and the copyright owner(s) are credited and that the original publication in this journal is cited, in accordance with accepted academic practice. No use, distribution or reproduction is permitted which does not comply with these terms.
*Correspondence: Alexandre Vallée, YWxleGFuZHJlLmcudmFsbGVlQGdtYWlsLmNvbQ==