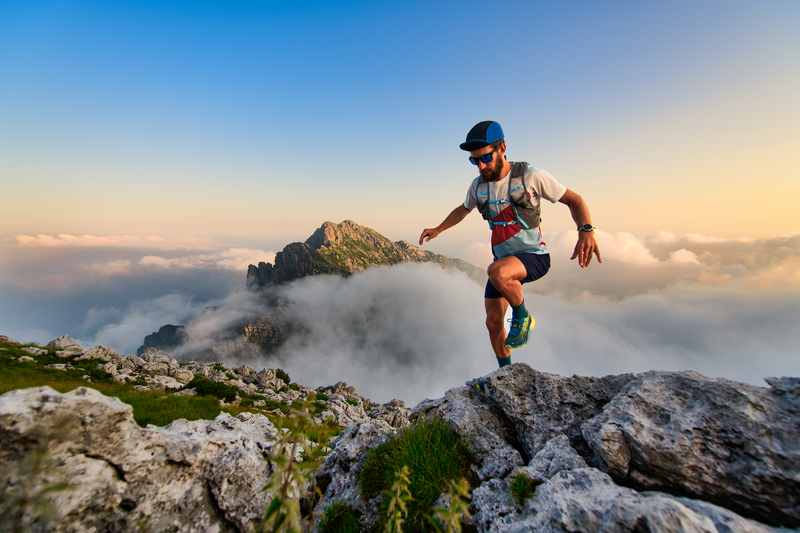
94% of researchers rate our articles as excellent or good
Learn more about the work of our research integrity team to safeguard the quality of each article we publish.
Find out more
ORIGINAL RESEARCH article
Front. Immunol. , 21 May 2021
Sec. Nutritional Immunology
Volume 12 - 2021 | https://doi.org/10.3389/fimmu.2021.664576
This article is part of the Research Topic Inflammation in Obesity: From Physiological to Pathological Aspects View all 7 articles
Type 2 diabetes (T2D) is a rising global health problem mainly caused by obesity and a sedentary lifestyle. In healthy individuals, white adipose tissue (WAT) has a relevant homeostatic role in glucose metabolism, energy storage, and endocrine signaling. Mast cells contribute to these functions promoting WAT angiogenesis and adipogenesis. In patients with T2D, inflammation dramatically impacts WAT functioning, which results in the recruitment of several leukocytes, including monocytes, that enhance this inflammation. Accordingly, the macrophages population rises as the WAT inflammation increases during the T2D status worsening. Since mast cell progenitors cannot arrive at WAT, the amount of WAT mast cells depends on how the new microenvironment affects progenitor and differentiated mast cells. Here, we employed a flow cytometry-based approach to analyze the number of mast cells from omental white adipose tissue (o-WAT) and subcutaneous white adipose tissue (s-WAT) in a cohort of 100 patients with obesity. Additionally, we measured the number of mast cell progenitors in a subcohort of 15 patients. The cohort was divided in three groups: non-T2D, pre-T2D, and T2D. Importantly, patients with T2D have a mild condition (HbA1c <7%). The number of mast cells and mast cell progenitors was lower in patients with T2D in both o-WAT and s-WAT in comparison to subjects from the pre-T2D and non-T2D groups. In the case of mast cells in o-WAT, there were statistically significant differences between non-T2D and T2D groups (p = 0.0031), together with pre-T2D and T2D groups (p=0.0097). However, in s-WAT, the differences are only between non-T2D and T2D groups (p=0.047). These differences have been obtained with patients with a mild T2D condition. Therefore, little changes in T2D status have a huge impact on the number of mast cells in WAT, especially in o-WAT. Due to the importance of mast cells in WAT physiology, their decrease can reduce the capacity of WAT, especially o-WAT, to store lipids and cause hypoxic cell deaths that will trigger inflammation.
The incidence of diabetes has increased four-fold in the last three decades, becoming the ninth death cause worldwide (1) and will affect 693 million people by 2045 (2). Of all the cases of patients with diabetes, type 2 diabetes (T2D) accounts for 90% (1). The development of T2D is strongly associated with obesity, which is driven by poor dietary habits and a sedentary lifestyle (1, 2).
When the caloric intake exceeds the energetic demands, adipocytes in white adipose tissue (WAT) store the excess energy as lipids, mainly triglycerides. Thus, when this positive energy imbalance continues for a long time, the WAT has to expand. So, adipocytes extend WAT via hypertrophy or hyperplasia. Adipocyte hyperplasia promotes insulin sensitivity and tissue homeostasis (3, 4). Conversely, adipocyte hypertrophy enhances insulin resistance and inflammation (3, 4). Interestingly, there are differences in adipose tissue depending on the depot location, including gene expression, metabolic features, and adipokine secretion (5, 6).
Noteworthy, subcutaneous white adipose tissue (s-WAT) has a higher adipogenic capacity than omental white adipose tissue (o-WAT). Therefore, when o-WAT expands, the amount of free fatty acids in the tissue increases. These free fatty acids trigger lipotoxicity and inflammation, producing tissue malfunctioning (7, 8). Consequently, the expansion of o-WAT, but not s-WAT, is a risk factor for developing T2D and cardiometabolic diseases (6, 9).
Mast cells are tissue-resident leukocytes located in all vascularized tissues. Depending on their location, they can develop two different phenotypes and become either connective tissue or mucosal mast cells (10, 11). Despite their widely known role in inflammation, mast cells also contribute to anti-inflammatory responses releasing TGF-beta and IL-10 (10, 12) and secreting proteases that can degrade pro-inflammatory cytokines (13). Additionally, they also participate in angiogenesis, lymphangiogenesis, tissue repair, and wound healing (12, 14). In these processes, it is crucial to remodel the extracellular matrix (ECM). Therefore, mast cells secrete proteases that directly digest the ECM or cause the proteolytic activation of ECM metalloproteases (13). In adipose tissue, mast cells have additional functions. They promote lipid uptake by macrophages and foam cell formation (15). In response to high glucose levels, mast cells release 15-deoxy-delta prostaglandin J2 (16, 17), which binds the peroxisome proliferator-activated receptor (PPAR) γ in pre-adipocytes promoting their differentiation to adipocytes (16, 17). Noteworthy, the absence of mast cells impairs the adipocyte differentiation (18).
When adipose tissue becomes insulin resistant, its physiology is altered. Firstly, it changes the adipokine profile (19). That affects mainly o-WAT since it secretes more pro-inflammatory adipokines than s-WAT (6). Secondly, some stress signals trigger the NLRP3 (NOD-, LRR- and pyrin domain-containing protein 3) inflammasome causing tissue inflammation (20, 21). These new conditions in the tissue microenvironment hamper adipogenesis (3, 4). This occurs by interfering with the PPAR-γ signaling pathway (22), which is critical for adipocyte hyperplasia (23, 24). The malfunctioning of the adipose tissue causes hyperglycemia and hyperlipidemia (24, 25). This leads to ectopic lipid accumulation in skeletal and heart muscle and several hepatic disorders, including hepatic steatosis (26–28).
Tissue-resident leukocytes, mainly macrophages and mast cells, play a key role in the homeostasis of the adipose tissue (8, 15, 16, 29). Nevertheless, the leukocytes recruited at WAT in patients with T2D mainly play a pro-inflammatory role (30, 31). Peripheral monocytes are recruited and differentiated to M1 macrophages that will produce more pro-inflammatory cytokines (7, 30). Such will recruit more monocytes forming a feed-forward loop (8, 29). Thus, as long as the glycemic control worsens, the number of macrophages sharply rises (7, 24, 29). However, the case of mast cells is not so straightforward. There is a tiny amount of mast cell progenitors circulating in peripheral blood (32). Noteworthy, these circulating mast cell progenitors arrive at some tissues, like the intestinal mucosa, but they do not enter in the WAT (33). Accordingly, several studies with murine models have demonstrated that the adipose tissue mast cell population depends on a local pool of embryonic mast cell progenitors instead of the circulating mast cell progenitors (11, 33, 34). Due to the scarce amount of circulating mast cell progenitors and their inability to reach adipose tissue, the number of mast cells will depend on how microenvironmental changes affect progenitor and differentiated mast cells.
Following the American Diabetes Association guidelines (35), T2D diagnosis requires two measures of fasting glucose. Accordingly, each patient gave 2 blood samples. The first one between 6 and 9 months before the surgery and it was used to measure the fasting plasma glucose. The second one was obtained on the day of the surgery before entering the operating room for a complete analysis. In both cases, the clinical analysis laboratory of San Cecilio University Hospital conducted the blood tests within 24h following approved protocols. Besides, fasting was defined as the absence of caloric intake for at least 8h.
For all patients, the Homeostatic Model Assessment for Insulin Resistance (HOMA-IR) was calculated with the data from the second blood test to evaluate insulin resistance.
This study includes 100 patients with morbid obesity who underwent laparoscopic bariatric surgery (gastric sleeve and gastric bypass). Patients with morbid obesity were further classified into non-T2D, pre-T2D, and T2D groups following the criteria of the American Diabetes Association (35). Patients with type I diabetes, gestational diabetes, genetic diabetes syndromes, diseases of the exocrine pancreas, drug-induced diabetes, and autoimmune diseases did not enter the cohort.
In addition to this, five patients without obesity, who underwent colonoscopy, provided samples of healthy mucosa. Such were employed as negative controls for the presence of a local pool of mast cell progenitors.
For each patient, two biopsies of adipose tissue were collected from laparoscopic bariatric surgery at San Cecilio University Hospital (Granada, Spain). The o-WAT (omental white adipose tissue) biopsies were taken from the greater omentum, close to the stomach. Alternatively, s-WAT (subcutaneous white adipose tissue) was sampled near the surgical incision. The biopsies were conserved in PBS and ice right after their extraction.
After that, visible blood vessels were removed from the samples. Then, approximately 2-2.5 g of each sample was weighed and cut into small pieces. Later, this was digested in RPMI 1640 medium supplemented with 2 mg/ml collagenase type I (Sigma) and 5 mM CaCl2 in a final volume of 10 ml, at 37°C during 2h. Subsequently, samples were washed with 35 ml of PBS, filtered through a 1 mm sieve, and centrifuged for 10 minutes at 900 x g. After, the pellet was resuspended in 10 ml of PBS, poured through a 100 μm filter, and centrifuged for 10 minutes at 900 x g. Finally, the pellet, that includes the stromal vascular fraction, was resuspended in 500 μl of antibody staining buffer (PBS, 2% fetal bovine serum, 0.09% albumin, and 0.05% sodium azide) and mixed with an internal standard (BD Truecount Absolute Counting Tubes) following manufacturer instructions.
The internal standard consists of a suspension of a known number of fluorescent microspheres, with size/complexity values far from any cellular population. The excitation and emission of these microspheres occur through a broad spectrum of wavelengths.
The stromal vascular fraction was labeled with 2 μl of controls or fluorophore-conjugated antibodies in Eppendorf tubes at room temperature for 20 minutes. After that, the cells were fixed, and the erythrocytes were lysed with 1 ml of BD FACS Lysing Solution for 30 minutes. Then, the samples were centrifuged 10 minutes at 3500 x g, and the pellets were resuspended in 500 μl of PBS. Subsequently, the samples were stored at 4°C until the next day. Flow cytometry was performed using a FACS ARIA III equipment, and data were acquired on a logarithmic scale. The internal standard was used to calculate the number of cells per mg of tissue.
The fluorescent-conjugated antibodies used to identify mast cells were: anti-CD45 PE-CF594 (clone HI30, BD), anti-CD117 APC (clone YB5.B8, BD), anti-FcϵRI PE-Cy7 (clone AER-37, BioLegend), and CD203c BV421 (clone NP4D6, BioLegend). Additionally, the antibodies CD34 BV785 (clone 561, BioLegend) and integrin β7 FITC (clone FIB504, BioLegend) were used in a subcohort of 15 patients (6 non-T2D, 6 pre-T2D, and 3 T2D). Compensation beads and isotype controls were purchased from BD Biosciences. MC were identified as CD45+ CD117+ CD203c+ FcϵRIα+ (Figure 1A).
Figure 1 Flow cytometry. (A–E) Flow cytometry gating employed to identify mast cells and mast cell progenitors in white adipose tissue. The red dots in the first scatter plot are the autofluorescent beads employed in the quantification. (F) Mast cell progenitor gate in colonic mucosa. MC (mast cells), MCp (Mast cell progenitors).
The data of mast cells per g was log-transformed to promote symmetry and normality of the analyzed data. The Kolmogorov-Smirnov test was used to test the normality of the distribution of the data, and the Levene test was employed to check the homoscedasticity of the groups. To evaluate the differences between non-T2D, pre-T2D, and T2D groups the one-way ANOVA test was used followed by a Tukey HSD. Student’s t-test was used to analyze the differences between o-WAT and s-WAT in non-T2D, pre-T2D, and T2D groups independently. P-values below 0.05 were considered significant. Additionally, we employed the principal component analysis (PCA), the Linear Discriminant Analysis (LDA), and Random Forest Analysis to study the internal structure of our data and to find patterns and the most important variables to discriminate the three groups of patients. All the tests were conducted with R software (36).
Table 1 shows the average data of age, sex, hypertension, BMI, waist-hip index, insulin, glucose, HbA1c, HOMA-IR, triglycerides, cholesterol, LDL, and HDL. In this study, females account for 66% of the cohort, which may be due to social constraints in our geographic zone. Of note, the T2D group has a low HbA1c mean. The American Diabetes Association considers that the goal for patients with T2D is to have their HbA1c below 7% since it considerably reduces the risk for cardiovascular disease. Thus, our subjects with T2D have a mild condition (37).
Mast cells were identified in the whole cohort as CD45+ CD117+ CD203c+ FcϵRIα+ by flow cytometry (Figures 1A–E). Moreover, in the subcohort of 15 patients, a CD34+ integrin b7+ subpopulation of mast cells was analyzed. This subpopulation was found in both types of WAT in these 15 patients (Figure 1E). Oppositely, this subpopulation was absent in the five samples of colonic mucosa (Figure 1F). Previous studies have shown that this subpopulation is made of mast cell progenitors in murine peripheral tissues (38, 39) and human blood (32). Besides, it has been reported that, in humans, the heterogeneous pool of progenitor cells in WAT contains cells that can give rise to mast cells (34). Therefore, the expression of CD34 and integrin b7 in a small fraction of the mast cell pool strongly suggests the presence of a stable pool of progenitor cells committed with mast cell lineage in human WAT.
These mast cell progenitors are more abundant in o-WAT than in s-WAT and seem to decrease in the T2D group in both types of WAT (Table 2). The proportion of mast cell progenitors in the whole population is displayed in Table 3.
Figures 2 and 3 show that the number of mast cells was smaller in the T2D group in both o-WAT and s-WAT. In the case of o-WAT (Figure 2 and Supplementary Figure 1), the differences are between non-T2D and T2D groups (p=0.0031), as well as pre-T2D and T2D groups (p=0.0097). However, in s-WAT (Figure 3 and Supplementary Figure 2), the differences are only between non-T2D and T2D groups (p=0.047). These results indicate that mast cells of both o-WAT and s-WAT are negatively affected by the dysregulation of glucose metabolism, particularly when T2D is reached.
Figure 2 Differences in mast cells per gram of tissue in omental white adipose tissue depending on the type 2 diabetes status. The data comes from the big cohort (n = 100). MC (mast cells), T2D (type 2 diabetes), “ns” (p-value > 0.05), “ ** ” (0,01 > p-value).
Figure 3 Differences in mast cells per gram of tissue in subcutaneous white adipose tissue depending on the type 2 diabetes status. The data comes from the big cohort (n = 100). MC (mast cells), T2D (type 2 diabetes), “ns” (p-value > 0.05), “ * ” (0.05 > p-value > 0.01).
Figures 4, 5, and 6 show that the difference in the number of mast cells between o-WAT and s-WAT becomes smaller in patients with T2D. Accordingly, these differences are significant only in non-T2D group (p=0.016) and pre-T2D group (p=0.021). Under normal conditions, the number of mast cells is higher in o-WAT than in s-WAT. Such occurs because o-WAT but not s-WAT is in contact with microbial products from the intestinal microbiota and plays a crucial role in peritoneal cavity immunology (40–42). Nonetheless, although in T2D group there is a sharp decrease in the number of mast cells in both locations, they are not equally affected. These results show that T2D condition has a higher impact on the number of mast cells in o-WAT than in s-WAT.
Figure 4 Differences in mast cells per gram of tissue between subcutaneous and omental white adipose tissue in patients without type 2 diabetes. The data comes from the big cohort (n = 100). MC (mast cells), s-WAT (subcutaneous white adipose tissue), o-WAT (omental white adipose tissue), T2D (type 2 diabetes), “ * ” (0.05 > p-value > 0.01).
Figure 5 Differences in mast cells per gram of tissue between subcutaneous and omental white adipose tissue in patients with pre-type 2 diabetes. The data comes from the big cohort (n = 100). MC (mast cells), s-WAT (subcutaneous white adipose tissue), o-WAT (omental white adipose tissue), T2D (type 2 diabetes), “ * ” (0.05 > p-value > 0.01).
Figure 6 Differences in mast cells per gram of tissue between subcutaneous and omental white adipose tissue in patients with type 2 diabetes. The data comes from the big cohort (n = 100). MC (mast cells), s-WAT (subcutaneous white adipose tissue), o-WAT (omental white adipose tissue), T2D (type 2 diabetes), “ns” (p-value > 0.05).
To see the patterns between our variables and the three groups of patients simplifying our high dimensional data, we performed a principal component analysis (Figure 7). This statistical analysis serves to summarize the variables dependence structure and to visualize the observed values. Interestingly, the non-T2D group tends to have higher numbers of mast cells in both o-WAT and s-WAT. The HbA1c was not included in this analysis and the following because it is used to define the groups.
Figure 7 Principal component analysis (PCA). This technique allows us to observe the patterns of our data reducing its dimension. The data comes from the big cohort (n = 100). NO (patients without type 2 diabetes), PRE (patients with pre-type 2 diabetes), YES (patients with type 2 diabetes), BMI (Body Mass Index), MC_V (mast cells from omental white adipose tissue), MC_S (mast cells from subcutaneous white adipose tissue), WH index (waist hip index).
After that, we performed a Linear Discriminant Analysis (LDA) to seek the linear combinations of the original variables that better serve to “separate” the observations in the transformed space when considering the levels of the “Type 2 Diabetes” variable (no, pre, yes) (Figure 8 and Supplementary Table 1). We can see that the first linear combination (LD1) separates well those observations. Negative values of LD1 are associated with “No” values, while positive ones are more related to “Pre” and “Yes”. Later, we studied the correlations of each variable with LD1 (Table 4). The stronger correlations are with age (0.50), waist-hip index (0.43), and the number of mast cells in o-WAT (-0.38). The negative sign of the correlation between mast cells and LD1 indicates that the number of mast cells in o-WAT is higher in patients with low LD1 values. Since the patients in the non-T2D group tend to have negative values of LD1, this agrees with our previous results.
Figure 8 Linear discriminant analysis (LDA). This technique finds the linear combinations of variables that better separate the observations. The data comes from the big cohort (n = 100). NO (patients without type 2 diabetes), PRE (patients with pre-type 2 diabetes), YES (patients with type 2 diabetes), LD1 (linear discriminant 1), LD2 (linear discriminant 2).
Finally, we performed the Random Forests Analysis. This technique also serves to measure the importance of the variables in discriminating the (three) levels of the categorical variable “Type 2 Diabetes”. That importance is determined through the “mean decrease in the Gini index”. Compellingly, the number of mast cells in o-WAT and s-WAT are respectively the third and fifth most important variables (Figure 9 and Supplementary Table 2). They are above some variables frequently studied in patients with T2D, including HDL, BMI, and waist-hip index.
Figure 9 Random Forests Analysis. This technique measures the importance of the variables to discriminate the three levels of the categorical variable “Type 2 diabetes”. The data comes from the big cohort (n = 100). MC_V (mast cells in omental white adipose tissue), MC_S (mast cells in subcutaneous white adipose tissue), WH index (waist-hip index).
These results together indicate that mast cells in adipose tissue (especially o-WAT) have a strong relationship with T2D status. Considering this occurs with patients with mild T2D, this phenomenon is expected to be more prominent in patents with T2D and poor glycemic control.
Mast cells are widely known for their role in inflammation and allergy. Thus, at first sight, it seems plausible that they play a deleterious role in obesity and T2D. This statement was supported by studies about mast cell numbers and mast cell stabilization in adipose tissue, and knockout models. Firstly, a previous study reported an increase in the number of mast cells in adipose tissue as the glycemic control worsens (43). However, this study worked with histological slides and normalized the number of mast cells with the surface of fibrosis in the tissue biopsy. This work provides valuable information about the tissue structure, but the cell counting method is not precise. Besides, mast cells are present not only in fibrotic tissue but also surrounding blood vessels (15). Here, we have digested the whole sample (2-2.5g), and we have employed a more accurate cell-counting method. Secondly, other studies in murine models reported that cromolyn, a mast cell stabilizer, reduces obesity and adipose tissue fibrosis, while it promotes insulin sensitivity (44, 45). Even though cromolyn is a widely used drug to treat asthma (46), its mechanism of action is still poorly understood (47). Curiously, mucosal mast cells are sensitive to cromolyn, but connective tissue mast cells are not (47, 48). Also, TNFα production by cell extracts from the peritoneum shows no significant difference between wild-type and mast cell-deficient mice (47). Nevertheless, in both wild-type and mast cell-deficient mice, cromolyn inhibited TNFα production in a dose-dependent manner (47). Therefore, the current knowledge suggests that cromolyn has a positive effect on patients with T2D but acting in other cell types. Accordingly, cromolyn can inhibit neutrophils (49–51), eosinophils (49, 52), monocytes (49, 52), and macrophages (52). Finally, the genetic ablation of c-kit, a critical receptor for mast cell development, protected mice from weight gain and insulin resistance (44). Nonetheless, c-kit is also crucial for the development of other leukocyte lineages. So, further experiments demonstrated that c-kit ablation but not mast cell depletion is what improves the metabolic profile in mice (53). Although more genetic models explored the effect of the absence of mast cells in WAT, the current evidence is that it does not protect from obesity and insulin resistance (54, 55). In a nutshell, mast cells are not critical players driving adipose tissue inflammation in patients with T2D.
The BMI is similar regardless of the T2D status. Therefore, it suggests that the problem is not the expansion of the adipose tissue itself but the mechanism to achieve it. When adipocytes expand the adipose tissue in response to an increase in the nutrients available, oxygen diffusion drops. This mild hypoxia produces stress signals that promote angiogenesis (56). Of note, angiogenesis is impaired in patients with T2D (57). Thus, hypoxic stress becomes greater, and some cells die because of it. These dead cells in the hypoxic areas of the tissue trigger inflammation and fibrosis (3, 4, 7, 21, 56).
Mast cells sense this hypoxic condition via reactive oxygen species (ROS) (15) and hypoxia-inducible factor 1α (HIF1α) production (58). Then, to promote angiogenesis, they release pro-angiogenic factors (VEGF, bFGF, TGF-beta), proteases to remodel the ECM, histamine to increase vascular permeability, and heparin (15). Moreover, mast cells downregulate the production of pro-inflammatory cytokines in response to hypoxic conditions (58). Apart from this, mast cells interact with endothelial cells to promote their proliferation (15) and the release of angiogenic factors (59). Consequently, in the absence of mast cells, the adipose tissue is less vascularized (60).
Furthermore, mast cells also contribute to foam cell formation (15) and pre-adipocyte to adipocyte differentiation (16–18). Therefore, the mast cell population reduction will affect the adipose tissue capacity to uptake and store lipids. Thus, the amount of free fatty acids will increase, causing hyperlipidemia. Such activates TLR2 and TLR4 on macrophages promoting inflammation (4, 21). Also, it boosts gluconeogenesis in the liver, prompting hyperglycemia (61) and causing hepatic steatosis (8). Finally, hyperlipidemia is an indicator of poor prognosis for the development of cardiovascular diseases (62).
Unfortunately, there are very few studies about mast cells in WAT, and most of them employ murine models. So, little is known about mast cells in human WAT. Moreover, many of the studies about mast cells in humans use histological slides instead of flow cytometry. Besides, the differences between humans and mice can also affect.
In mice, fibrosis in WAT causes tissue dysfunction. However, the situation in humans is more complicated. Fibrosis in human s-WAT was associated with a pathological metabolic profile, bigger adipocytes, and low weight loss after bariatric surgery (63). Inversely, fibrosis in o-WAT is associated with smaller adipocytes (64, 65). Since fibrosis in o-WAT limits adipocyte hypertrophy, it provides a better metabolic profile (64, 65). Mast cells locate in large numbers in fibrotic tissue. Where they can produce or degrade collagen depending on the molecular context (66). In adipose tissue fibrosis, it is unknown the role they play (66). Nonetheless, in the liver, mast cells play an antifibrotic role (67).
Previously, we reported three key aspects about the changes in adipose tissue physiology after weight loss induced by bariatric surgery (68). Firstly, WAT undergoes extensive tissue remodeling that increases insulin sensitivity. Secondly, pre-adipocytes increase their number. Thirdly, the size of adipocytes decreases. These three changes strongly suggest a remodeling process in WAT that leads to glucose metabolism normalization. Furthermore, bariatric surgery reduces the inflammatory status of both o-WAT and s-WAT, including a sharp decrease in the number of neutrophils (68). Importantly, after bariatric surgery, the number of mast cells increases 10-fold in o-WAT and 4-fold in s-WAT (68). Therefore, since mast cells increase in number (68) and promote adipogenesis (16–18), they may play a major role in the remodeling of the adipose tissue.
The study of Goldstein et al. (69) showed that in patients with obesity, a higher number of mast cells in o-WAT is associated with a lower cardiometabolic risk. Moreover, a higher amount of mast cells in o-WAT is linked with a higher weight loss after bariatric surgery. Finally, higher number of mast cells in o-WAT was associated with higher insulin sensitivity in hepatocytes (69).
Mast cells have a close relationship with WAT and have an essential function in its physiology (15–18, 58). Mast cell progenitors arrive at WAT during the embryonic stage (33). After that, the whole differentiation of mast cells takes place in WAT under the influence of the local microenvironment (70, 71). Nevertheless, currently, the differences between bone marrow progenitors and WAT progenitors are poorly understood.
Mast cells contribute to WAT homeostasis interacting with several adipokines including leptin (72) and lipoproteins like LDL (73) and stromal cells (74). Moreover, in cold conditions, the number of mast cells increases in WAT. These mast cells promote the browning of WAT in response to norepinephrine (55, 75, 76).
In conclusion, the number of mast cells decreases in patients with T2D. Noteworthy, the HbA1c mean difference between groups is small. Therefore, little changes in glycemia have a huge impact on the number of mast cells in WAT, especially in o-WAT. Since mast cells play a prominent homeostatic role in adipose tissue, their decrease can contribute to the deterioration of patients with T2D. One of the limitations of this study is that the patients with T2D have a mild condition (HbA1c <7%). It would be interesting to include a group with severe T2D. However, patients with severe T2D have an increased risk of complications during surgery and usually follow other therapeutic strategies.
The raw data supporting the conclusions of this article will be made available by the authors, without undue reservation.
The studies involving human participants were reviewed and approved by Andalucia’s Biomedical Research Ethics Committee of Granada (CEIM/CEI Granada). The patients/participants provided their written informed consent to participate in this study.
JG-R and FT performed the surgeries. DL-P, AR-R, JP-P, and SM-S performed experiments. DL-P, LG-E, AB, and CA analyzed and interpreted data. DL-P, CA, JS, JG, JL, and ÁC interpreted data, drafted the manuscript, and contributed with intellectual content. JG, JL, and ÁC designed and supervised the study. All authors contributed to the article and approved the submitted version.
Instituto de Salud Carlos III (grant PI18/01947). Instituto de Salud Carlos III is a research institution from the Spanish government that funds biomedical research. Instituto de Salud Carlos III funded all the experiments.
The authors declare that the research was conducted in the absence of any commercial or financial relationships that could be construed as a potential conflict of interest.
This work was supported by Instituto de Salud Carlos III (grant PI15/01361), Spain; and MINECO (grant DPI2017-84439-R), Madrid and FEDER. DL-P is a predoctoral fellow (“Programa de doctorado en Bioquímica y Biología Molecular”, B16.56.1) funded by the Spanish Ministry of Science and Innovation (“Formación de profesorado universitario” grant FPU18/04432). DL-P participated in this work thanks to a grant from University of Granada (“Becas de iniciación a la investigación del plan propio de la UGR”).
The Supplementary Material for this article can be found online at: https://www.frontiersin.org/articles/10.3389/fimmu.2021.664576/full#supplementary-material
1. Zheng Y, Ley SH, Hu FB. Global Aetiology and Epidemiology of Type 2 Diabetes Mellitus and its Complications. Nat Rev Endocrinol (2018) 14(2):88–98. doi: 10.1038/nrendo.2017.151
2. Cho NH, Shaw JE, Karuranga S, Huang Y, da Rocha Fernandes JD, Ohlrogge AW, et al. Idf Diabetes Atlas: Global Estimates of Diabetes Prevalence for 2017 and Projections for 2045. Diabetes Res Clin Pract (2018) 138:271–81. doi: 10.1016/j.diabres.2018.02.023
3. Choe SS, Huh JY, Hwang IJ, Kim JI, Kim JB. Adipose Tissue Remodeling: its Role in Energy Metabolism and Metabolic Disorders. Front Endocrinol (Lausanne) (2016) 7:1–16. doi: 10.3389/fendo.2016.00030
4. Kusminski CM, Bickel PE, Scherer PE. Targeting Adipose Tissue in the Treatment of Obesity-Associated Diabetes. Nat Rev Drug Discovery (2016) 15(9):639–60. doi: 10.1038/nrd.2016.75
5. Jeffery E, Wing A, Holtrup B, Sebo Z, Kaplan JL, Saavedra-Peña R, et al. The Adipose Tissue Microenvironment Regulates Depot-Specific Adipogenesis in Obesity. Cell Metab (2016) 24(1):142–50. doi: 10.1016/j.cmet.2016.05.012
6. Samaras K, Botelho NK, Chisholm DJ, Lord RV. Subcutaneous and Visceral Adipose Tissue Gene Expression of Serum Adipokines That Predict Type 2 Diabetes. Obes (2010) 18(5):884–9. doi: 10.1038/oby.2009.443
7. Donath MY, Shoelson SE. Type 2 Diabetes as an Inflammatory Disease. Nat Rev Immunol (2011) 11(2):98–107. doi: 10.1038/nri2925
8. Lackey DE, Olefsky JM. Regulation of Metabolism by the Innate Immune System. Nat Rev Endocrinol (2016) 12(1):15–28. doi: 10.1038/nrendo.2015.189
9. Lee JJ, Beretvas SN, Freeland-Graves JH. Abdominal Adiposity Distribution in Diabetic/Prediabetic and Nondiabetic Populations: A Meta-Analysis. J Obes (2014) 2014:1–20. doi: 10.1155/2014/697264
10. Mukai K, Tsai M, Saito H, Galli SJ. Mast Cells as Sources of Cytokines, Chemokines, and Growth Factors. Immunol Rev (2018) 282(1):121–50. doi: 10.1111/imr.12634
11. Varricchi, de Paulis, Marone, Galli. Future Needs in Mast Cell Biology. Int J Mol Sci (2019) 20(18):4397. doi: 10.3390/ijms20184397
12. da Silva EZM, Jamur MC, Oliver C. Mast Cell Function: A New Vision of an Old Cell. J Histochem Cytochem (2014) 62(10):698–738. doi: 10.1369/0022155414545334
13. Wernersson S, Pejler G. Mast Cell Secretory Granules: Armed for Battle. Nat Rev Immunol (2014) 14(7):478–94. doi: 10.1038/nri3690
14. Varricchi G, Galdiero MR, Loffredo S, Marone G, Iannone R, Marone G, et al. Are Mast Cells MASTers in Cancer? Front Immunol (2017) 8:1–13. doi: 10.3389/fimmu.2017.00424
15. Krystel-Whittemore M, Dileepan KN, Wood JG. Mast Cell: A Multi-Functional Master Cell. Front Immunol (2016) 6:1–12. doi: 10.3389/fimmu.2015.00620
16. Tanaka A, Nomura Y, Matsuda A, Ohmori K, Matsuda H. Mast Cells Function as an Alternative Modulator of Adipogenesis Through 15-deoxy-delta-12, 14-Prostaglandin J 2. Am J Physiol Physiol (2011) 301(6):C1360–7. doi: 10.1152/ajpcell.00514.2010
17. Shi MA, Shi G-P. Different Roles of Mast Cells in Obesity and Diabetes: Lessons From Experimental Animals and Humans. Front Immunol (2012) 3:1–12. doi: 10.3389/fimmu.2012.00007
18. Ishijima Y, Ohmori S, Ohneda K. Mast Cell Deficiency Results in the Accumulation of Preadipocytes in Adipose Tissue in Both Obese and non-Obese Mice. FEBS Open Bio (2014) 4(1):18–24. doi: 10.1016/j.fob.2013.11.004
19. Jung U, Choi M-S. Obesity and Its Metabolic Complications: The Role of Adipokines and the Relationship Between Obesity, Inflammation, Insulin Resistance, Dyslipidemia and Nonalcoholic Fatty Liver Disease. Int J Mol Sci (2014) 15(4):6184–223. doi: 10.3390/ijms15046184
20. Grant RW, Dixit VD. Mechanisms of Disease: Inflammasome Activation and the Development of Type 2 Diabetes. Front Immunol (2013) 4:1–10. doi: 10.3389/fimmu.2013.00050
21. Reilly SM, Saltiel AR. Adapting to Obesity With Adipose Tissue Inflammation. Nat Rev Endocrinol (2017) 13(11):633–43. doi: 10.1038/nrendo.2017.90
22. Larsen TM, Toubro S, Astrup A. Ppargamma Agonists in the Treatment of Type II Diabetes: Is Increased Fatness Commensurate With Long-Term Efficacy? Int J Obes (2003) 27(2):147–61. doi: 10.1038/sj.ijo.802223
23. Patel JJ, Butters OR, Arnett TR. PPAR Agonists Stimulate Adipogenesis at the Expense of Osteoblast Differentiation While Inhibiting Osteoclast Formation and Activity. Cell Biochem Funct (2014) 32(4):368–77. doi: 10.1002/cbf.3025
24. Petersen MC, Shulman GI. Mechanisms of Insulin Action and Insulin Resistance. Physiol Rev (2018) 98(4):2133–223. doi: 10.1152/physrev.00063.2017
25. Muoio DM, Newgard CB. Molecular and Metabolic Mechanisms of Insulin Resistance and β-Cell Failure in Type 2 Diabetes. Nat Rev Mol Cell Biol (2008) 9(3):193–205. doi: 10.1038/nrm2327
26. Smith U, Kahn BB. Adipose Tissue Regulates Insulin Sensitivity: Role of Adipogenesis, De Novo Lipogenesis and Novel Lipids. J Intern Med (2016) 280465–75(5):1–10. doi: 10.1111/joim.12540
27. Perry RJ, Camporez J-PG, Kursawe R, Titchenell PM, Zhang D, Perry CJ, et al. Hepatic Acetyl Coa Links Adipose Tissue Inflammation to Hepatic Insulin Resistance and Type 2 Diabetes. Cell (2015) 160(4):745–58. doi: 10.1016/j.cell.2015.01.012
28. Li Y, Wong K, Giles A, Jiang J, Lee JW, Adams AC, et al. Hepatic SIRT1 Attenuates Hepatic Steatosis and Controls Energy Balance in Mice by Inducing Fibroblast Growth Factor 21. Gastroenterol (2014) 146(2):539–49. doi: 10.1053/j.gastro.2013.10.059
29. McNelis JC, Olefsky JM. Macrophages, Immunity, and Metabolic Disease. Immun (2014) 41(1):36–48. doi: 10.1016/j.immuni.2014.05.010
30. Lee B-C, Lee J. Cellular and Molecular Players in Adipose Tissue Inflammation in the Development of Obesity-Induced Insulin Resistance. Biochim Biophys Acta - Mol Basis Dis (2014) 1842(3):446–62. doi: 10.1016/j.bbadis.2013.05.017
31. Zatterale F, Longo M, Naderi J, Raciti GA, Desiderio A, Miele C, et al. Chronic Adipose Tissue Inflammation Linking Obesity to Insulin Resistance and Type 2 Diabetes. Front Physiol (2020) 10:1–20. doi: 10.3389/fphys.2019.01607
32. Dahlin JS, Malinovschi A, Öhrvik H, Sandelin M, Janson C, Alving K, et al. Lin– CD34hi CD117int/Hi Fcϵri+ Cells in Human Blood Constitute a Rare Population of Mast Cell Progenitors. Blood (2016) 127(4):383–91. doi: 10.1182/blood-2015-06-650648
33. Li Z, Liu S, Xu J, Zhang X, Han D, Liu J, et al. Adult Connective Tissue-Resident Mast Cells Originate From Late Erythro-Myeloid Progenitors. Immun (2018) 49(4):640–53. doi: 10.1016/j.immuni.2018.09.023
34. Plotkin JD, Elias MG, Fereydouni M, Daniels-Wells TR, Dellinger AL, Penichet ML, et al. Human Mast Cells From Adipose Tissue Target and Induce Apoptosis of Breast Cancer Cells. Front Immunol (2019) 10:1–13. doi: 10.3389/fimmu.2019.00138
35. American Diabetes Association. 2. Classification and Diagnosis of Diabetes: Standards of Medical Care in Diabetes—2018. Diabetes Care (2018) 41(Supplement 1):S13–27. doi: 10.2337/dc19-S002
36. Core Team R. R: A Language and Environment for Statistical Computing. Vienna, Austria: R Foundation for Statistical Computing (2017). Available at: https://www.r-project.org/.
37. American Diabetes Association. Standards of Medical Care in Diabetes–2014. Diabetes Care (2014) 37(Supplement_1):S14–80. doi: 10.2337/dc14-S014
38. Bankova LG, Dwyer DF, Liu AY, Austen KF, Gurish MF. Maturation of Mast Cell Progenitors to Mucosal Mast Cells During Allergic Pulmonary Inflammation in Mice. Mucosal Immunol (2015) 8(3):596–606. doi: 10.1038/mi.2014.91
39. Dahlin JS, Hallgren J. Mast Cell Progenitors: Origin, Development and Migration to Tissues. Mol Immunol (2015) 63(1):9–17. doi: 10.1016/j.molimm.2014.01.018
40. Tremaroli V, Bäckhed F. Functional Interactions Between the Gut Microbiota and Host Metabolism. Nat (2012) 489(7415):242–9. doi: 10.1038/nature11552
41. Bénézech C, Luu N-T, Walker JA, Kruglov AA, Loo Y, Nakamura K, et al. Inflammation-Induced Formation of Fat-Associated Lymphoid Clusters. Nat Immunol (2015) 16(8):819–28. doi: 10.1038/ni.3215
42. Meza-Perez S, Randall TD. Immunological Functions of the Omentum. Trends Immunol (2017) 38(7):526–36. doi: 10.1016/j.it.2017.03.002
43. Divoux A, Moutel S, Poitou C, Lacasa D, Veyrie N, Aissat A, et al. Mast Cells in Human Adipose Tissue: Link With Morbid Obesity, Inflammatory Status, and Diabetes. J Clin Endocrinol Metab (2012) 97(9):E1677–85. doi: 10.1210/jc.2012-1532
44. Liu J, Divoux A, Sun J, Zhang J, Clément K, Glickman JN, et al. Genetic Deficiency and Pharmacological Stabilization of Mast Cells Reduce Diet-Induced Obesity and Diabetes in Mice. Nat Med (2009) 15(8):940–5. doi: 10.1038/nm.1994
45. Kumar D, Pandya SK, Varshney S, Shankar K, Rajan S, Srivastava A, et al. Temporal Immmunometabolic Profiling of Adipose Tissue in HFD-induced Obesity: Manifestations of Mast Cells in Fibrosis and Senescence. Int J Obes (2019) 43(6):1281–94. doi: 10.1038/s41366-018-0228-5
46. Morosco G, Kiley J. Expert Panel Report 3 (Epr-3): Guidelines for the Diagnosis and Management of Asthma-Summary Report 2007. J Allergy Clin Immunol (2007) 120(5):S93. doi: 10.1016/j.jaci.2007.09.029
47. Oka T, Kalesnikoff J, Starkl P, Tsai M, Galli SJ. Evidence Questioning Cromolyn’s Effectiveness and Selectivity as a ‘Mast Cell Stabilizer’ in Mice. Lab Investig (2012) 92(10):1472–82.
48. Forbes EE, Groschwitz K, Abonia JP, Brandt EB, Cohen E, Blanchard C, et al. Il-9– and Mast Cell–Mediated Intestinal Permeability Predisposes to Oral Antigen Hypersensitivity. J Exp Med (2008) 205(4):897–913. doi: 10.1084/jem.20071046
49. Kay A, Walsh G, Moqbel R, Macdonald A, Nagakura T, Carroll M, et al. Disodium Cromoglycate Inhibits Activation of Human Inflammatory Cells In Vitro. J Allergy Clin Immunol (1987) 80(1):1–8. doi: 10.1016/S0091-6749(87)80183-5
50. Kilpatrick LE, Jakabovics E, McCawley LJ, Kane LH, Korchak HM. Cromolyn Inhibits Assembly of the NADPH Oxidase and Superoxide Anion Generation by Human Neutrophils. J Immunol (1995) 154(7):3429–36.
51. Yazid S, Leoni G, Getting SJ, Cooper D, Solito E, Perretti M, et al. Antiallergic Cromones Inhibit Neutrophil Recruitment Onto Vascular Endothelium Via Annexin-A1 Mobilization. Arterioscler Thromb Vasc Biol (2010) 30(9):1718–24. doi: 10.1161/ATVBAHA.110.209536
52. Yang Y, Lu JY-L, Wu X, Summer S, Whoriskey J, Saris C, et al. G-Protein-Coupled Receptor 35 Is a Target of the Asthma Drugs Cromolyn Disodium and Nedocromil Sodium. Pharmacol (2010) 86(1):1–5. doi: 10.1159/000314164
53. Gutierrez DA, Muralidhar S, Feyerabend TB, Herzig S, Rodewald H-R. Hematopoietic Kit Deficiency, Rather Than Lack of Mast Cells, Protects Mice From Obesity and Insulin Resistance. Cell Metab (2015) 21(5):678–91. doi: 10.1016/j.cmet.2015.04.013
54. Chmelař J, Chatzigeorgiou A, Chung K-J, Prucnal M, Voehringer D, Roers A, et al. No Role for Mast Cells in Obesity-Related Metabolic Dysregulation. Front Immunol (2016) 7:1–8. doi: 10.3389/fimmu.2016.00524
55. Elieh Ali Komi D, Shafaghat F, Christian M. Crosstalk Between Mast Cells and Adipocytes in Physiologic and Pathologic Conditions. Clin Rev Allergy Immunol (2020) 58(3):388–400. doi: 10.1007/s12016-020-08785-7
56. Crewe C, An YA, Scherer PE. The Ominous Triad of Adipose Tissue Dysfunction: Inflammation, Fibrosis, and Impaired Angiogenesis. J Clin Invest (2017) 127(1):74–82. doi: 10.1172/JCI88883
57. Cheng R, Ma J. Angiogenesis in Diabetes and Obesity. Rev Endocr Metab Disord (2015) 16(1):67–75. doi: 10.1007/s11154-015-9310-7
58. Möllerherm H, Branitzki-Heinemann K, Brogden G, Elamin AA, Oehlmann W, Fuhrmann H, et al. Hypoxia Modulates the Response of Mast Cells to Staphylococcus Aureus Infection. Front Immunol (2017) 8:541. doi: 10.3389/fimmu.2017.00541
59. de Souza Junior DA, Mazucato VM, Santana AC, Oliver C, Jamur MC. Mast Cells Interact With Endothelial Cells to Accelerate in Vitro Angiogenesis. Int J Mol Sci (2017) 18(12):2674. doi: 10.3390/ijms18122674
60. Mraz M, Haluzik M. The Role of Adipose Tissue Immune Cells in Obesity and Low-Grade Inflammation. . J Endocrinol (2014) 222(3):R113–27. doi: 10.1530/JOE-14-0283
61. Samuel VT, Shulman GI. The Pathogenesis of Insulin Resistance: Integrating Signaling Pathways and Substrate Flux. J Clin Invest (2016) 126(1):12–22. doi: 10.1172/JCI77812
62. Ponikowski P, Voors AA, Anker SD, Bueno H, Cleland JGF, Coats AJS, et al. Esc Guidelines for the Diagnosis and Treatment of Acute and Chronic Heart Failure. Eur Heart J (2016) 37(27):2129–200. doi: 10.1093/eurheartj/ehw128
63. Bel Lassen P, Charlotte F, Liu Y, Bedossa P, Le Naour G, Tordjman J, et al. The FAT Score, a Fibrosis Score of Adipose Tissue: Predicting Weight-Loss Outcome After Gastric Bypass. J Clin Endocrinol Metab (2017) 102(7):2443–53. doi: 10.1210/jc.2017-00138
64. Divoux A, Tordjman J, Lacasa D, Veyrie N, Hugol D, Aissat A, et al. Fibrosis in Human Adipose Tissue: Composition, Distribution, and Link With Lipid Metabolism and Fat Mass Loss. Diabetes (2010) 59(11):2817–25. doi: 10.2337/db10-0585
65. Muir LA, Neeley CK, Meyer KA, Baker NA, Brosius AM, Washabaugh AR, et al. Adipose Tissue Fibrosis, Hypertrophy, and Hyperplasia: Correlations With Diabetes in Human Obesity. Obes (2016) 24(3):597–605. doi: 10.1002/oby.21377
66. Sun K, Tordjman J, Clément K, Scherer PE. Fibrosis and Adipose Tissue Dysfunction. Cell Metab (2013) 18(4):470–7. doi: 10.1016/j.cmet.2013.06.016
67. Amiot L, Vu N, Drenou B, Scrofani M, Chalin A, Devisme C, et al. The Anti-Fibrotic Role of Mast Cells in the Liver is Mediated by HLA-G and Interaction With Hepatic Stellate Cells. Cytokine (2019) 117:50–8. doi: 10.1016/j.cyto.2019.02.002
68. García-Rubio J, León J, Redruello-Romero A, Pavón E, Cozar A, Tamayo F, et al. Cytometric Analysis of Adipose Tissue Reveals Increments of Adipocyte Progenitor Cells After Weight Loss Induced by Bariatric Surgery. Sci Rep (2018) 8(1):15203. doi: 10.1038/s41598-018-33488-7
69. Goldstein N, Kezerle Y, Gepner Y, Haim Y, Pecht T, Gazit R, et al. Higher Mast Cell Accumulation in Human Adipose Tissues Defines Clinically Favorable Obesity Sub-Phenotypes. Cells (2020) 9(6):1508. doi: 10.3390/cells9061508
70. Poglio S, De Toni-Costes F, Arnaud E, Laharrague P, Espinosa E, Casteilla L, et al. Adipose Tissue as a Dedicated Reservoir of Functional Mast Cell Progenitors. Stem Cells (2010) 28(11):2065–72. doi: 10.1002/stem.523
71. Cildir G, Pant H, Lopez AF, Tergaonkar V. The Transcriptional Program, Functional Heterogeneity, and Clinical Targeting of Mast Cells. J Exp Med (2017) 214(9):2491–506. doi: 10.1084/jem.20170910
72. Żelechowska P, Wiktorska M, Różalska S, Stasikowska-Kanicka O, Wągrowska-Danilewicz M, Agier J, et al. Leptin Receptor is Expressed by Tissue Mast Cells. Immunol Res (2018) 66(5):557–66. doi: 10.1007/s12026-018-9029-0
73. Oxidized Low-Density Lipoprotein Contributes to Atherogenesis Via Co-activation of Macrophages and Mast Cells. PloS One (2015) 10(3):e0123088. doi: 10.1371/journal.pone.0123088
74. Komi DEA, Khomtchouk K, Santa Maria PL. A Review of the Contribution of Mast Cells in Wound Healing: Involved Molecular and Cellular Mechanisms. Clin Rev Allergy Immunol (2020) 58(3):298–312. doi: 10.1007/s12016-019-08729-w
75. Finlin BS, Zhu B, Confides AL, Westgate PM, Harfmann BD, Dupont-Versteegden EE, et al. Mast Cells Promote Seasonal White Adipose Beiging in Humans. Diabetes (2017) 66(5):1237–46. doi: 10.2337/db16-1057
Keywords: mast cell, T2D, adipose tissue, obesity, flow cytometry, angiogenesis, inflammation, adipogenesis
Citation: Lopez-Perez D, Redruello-Romero A, Garcia-Rubio J, Arana C, Garcia-Escudero LA, Tamayo F, Puentes-Pardo JD, Moreno-SanJuan S, Salmeron J, Blanco A, Galvez J, Leon J and Carazo Á (2021) In Patients With Obesity, the Number of Adipose Tissue Mast Cells Is Significantly Lower in Subjects With Type 2 Diabetes. Front. Immunol. 12:664576. doi: 10.3389/fimmu.2021.664576
Received: 05 February 2021; Accepted: 05 May 2021;
Published: 21 May 2021.
Edited by:
Marina Chaves De Oliveira, Federal University of Minas Gerais, BrazilReviewed by:
Laura W. Bowers, Purdue University, United StatesCopyright © 2021 Lopez-Perez, Redruello-Romero, Garcia-Rubio, Arana, Garcia-Escudero, Tamayo, Puentes-Pardo, Moreno-SanJuan, Salmeron, Blanco, Galvez, Leon and Carazo. This is an open-access article distributed under the terms of the Creative Commons Attribution License (CC BY). The use, distribution or reproduction in other forums is permitted, provided the original author(s) and the copyright owner(s) are credited and that the original publication in this journal is cited, in accordance with accepted academic practice. No use, distribution or reproduction is permitted which does not comply with these terms.
*Correspondence: Julio Galvez, amdhbHZlekB1Z3IuZXM=
Ángel Carazo, ZXh0LmFjYXJhem9AdWdyLmVz
†These authors have contributed equally to this work and share senior authorship
Disclaimer: All claims expressed in this article are solely those of the authors and do not necessarily represent those of their affiliated organizations, or those of the publisher, the editors and the reviewers. Any product that may be evaluated in this article or claim that may be made by its manufacturer is not guaranteed or endorsed by the publisher.
Research integrity at Frontiers
Learn more about the work of our research integrity team to safeguard the quality of each article we publish.