- 1Department of Immunology, Harvard Medical School, Boston, MA, United States
- 2Department of Biology, Eastern Washington University, Cheney, WA, United States
- 3Department of Microbiology and Immunology, Geisel School of Medicine at Dartmouth College, Hanover, NH, United States
The symbiotic relationship between animals and their resident microorganisms has profound effects on host immunity. The human microbiota comprises bacteria that reside in the gastrointestinal tract and are involved in a range of inflammatory and autoimmune diseases. The gut microbiota’s immunomodulatory effects extend to extraintestinal tissues, including the central nervous system (CNS). Specific symbiotic antigens responsible for inducing immunoregulation have been isolated from different bacterial species. Polysaccharide A (PSA) of Bacteroides fragilis is an archetypical molecule for host-microbiota interactions. Studies have shown that PSA has beneficial effects in experimental disease models, including experimental autoimmune encephalomyelitis (EAE), the most widely used animal model for multiple sclerosis (MS). Furthermore, in vitro stimulation with PSA promotes an immunomodulatory phenotype in human T cells isolated from healthy and MS donors. In this review, we discuss the current understanding of the interactions between gut microbiota and the host in the context of CNS inflammatory demyelination, the immunomodulatory roles of gut symbionts. More specifically, we also discuss the immunomodulatory effects of B. fragilis PSA in the gut-brain axis and its therapeutic potential in MS. Elucidation of the molecular mechanisms responsible for the microbiota’s impact on host physiology offers tremendous promise for discovering new therapies.
Introduction
Mammals have co-evolved with eons of resident microorganisms that play an integral role in regulating the host immunity (1). These microorganisms live in a complex community called microbiota, which is dominated by bacteria and includes archaea, fungi, and viruses (2). Analysis of the composition and the human microbiota’s diversity has significantly improved by culture-independent methods and next-generation sequencing (3, 4). An updated catalog based on a metagenomic assembly of microbiomes across world populations shows that over 150,000 bacterial genomes can be found in the human body (5). The gastrointestinal tract harbors most of these microbes, some producing immunomodulatory molecules to educate the host immune system (6). The composition and gut microbiota function can affect the susceptibility to and progression of a wide range of diseases in the intestine and the extraintestinal tissues such as the central nervous system (CNS) (7, 8). While several microbial species have been identified concerning specific pathologies in humans, the mechanistic understanding of how the symbiotic molecules interact with the host is still limited. Determining the molecular mechanisms of the microbial molecules is crucial for the development of prophylactic and therapeutic interventions. B. fragilis polysaccharide A (PSA) is a prototypical symbiotic antigen that has been invaluable for understanding the mechanisms directing microbiota–host interactions (9). PSA mediates gut homeostasis by directing cellular and physical development of the immune system (10), stimulating Tregs (11) via plasmacytoid dendritic cells (PDCs) (12), and protecting animals from experimental diseases like colitis (11, 12), asthma (13), or pulmonary inflammation (14), and experimental autoimmune encephalomyelitis (EAE) (15–17). EAE is an animal model of multiple sclerosis (MS), an inflammatory demyelinating CNS disease (18). MS is characterized by inflammation and axonal damage resulting in progressive disability due to neurodegeneration. Although the disease’s etiology is not entirely understood, multiple genetic and environmental factors have been implicated in MS’s onset and progression. Mounting evidence suggests that microbiota plays an essential role in the development of the disease (19).
In this review, we cover some of the most recent literature on the gut-brain axis in the context of CNS inflammatory demyelination. Because of the impact of gut microbes regulating the immune system and the immune-mediated responses that characterize EAE/MS, we hypothesize that the large pool of microbes and microbial products present in the gut is an excellent source of novel therapeutics. Furthermore, we propose that PSA produced by B. fragilis is an identified symbiont factor model for immunomodulation. PSA could be one of the possibly numerous bacterial cellular components capable of promoting protective responses against neuroinflammation. Accordingly, our review will summarize how PSA regulates immune responses in MS/EAE and discusses PSA’s therapeutic potential.
Gut Microbiota
Although utero colonization is still debated (20), it is known that microbial colonization starts mainly after birth (21). It is suggested that establishing a diverse and balanced microbiota in early life is essential for developing a healthy immune system (22, 23). An imbalance in the microbiota’s composition and function (dysbiosis) during this window of opportunity can have long-lasting consequences later in life, causing a wide range of immune diseases (24). The microbial composition of the infant’s gut depends on many factors (25), including the type of delivery (26), gestational age (27), antibiotic use (28), and the mode of feeding (29). Human milk oligosaccharides (HMOs) in breast milk promote beneficial microbes like Bifidobacterium species in breast-fed infants (30). Cessation of breast-feeding and introducing solid foods drives the infant’s gut microbiome’s maturation (31), gradually reaching an adult-like composition after three years of life (32). Gut microbiota in adulthood is dominated by Bacteroidetes and Firmicutes and includes Actinobacteria, Proteobacteria, Verrucomicrobia, archaea, viruses, fungi, and protozoa (33, 34). While the gut microbiota in adults is more stable than in infants, the specific microbial species can vary interpersonally, creating a unique composition for every individual.
In homeostatic conditions, the host and the microbiota benefit each other and coexist in a mutualistic symbiosis (35). The host offers a nutrient-rich environment for the microbiota. In return, the microbiota provides metabolites (36), vitamins (37), and other micronutrients to the host by fermenting undigested dietary components in the large intestine (38). The microbiota’s ability to produce energy by digesting complex carbohydrates in the gut has been an evolutionary driving force for establishing the host-microbiota symbiotic relationship (39, 40). In addition, the gut microbiota participates in the development of the host immune system and balances defense and tolerance to maintain homeostasis (41).
While the use of fecal microbiota transplantation (FMT) as a treatment for gastrointestinal problems in Chinese medicine dates back to the 4th century (42), the interconnectedness of gut microbiota, CNS, and neuropsychiatric health is a concept from the early 19th century (43). It is now known that the fundamental impact of the microbiota on host physiology reaches far outside the gastrointestinal tract and extends to CNS. Recently it is proposed that there is a bidirectional relationship between the gut microbiota and the CNS (44, 45). This reciprocal interaction occurs through different routes involving endocrine, immune, and neural mechanisms. The function of microbiota in the gut-brain axis is believed to affect the etiology of a wide range of neuropsychiatric disorders, including Parkinson’s disease (46, 47), Alzheimer’s disease (48, 49), depression (50, 51), anxiety (52, 53), autism (54, 55), amyotrophic lateral sclerosis (56) and multiple sclerosis (57–66). Increasing evidence points to alterations in the gut microbiota composition in patients with neuropsychiatric disorders. However, the molecular mechanisms by which the microbiota modulates these diseases are not fully understood. A mechanistic understanding of microbiota’s role in the gut–brain axis will help develop prophylactic and therapeutic interventions for CNS diseases that are increasingly affecting large populations.
Gut Microbiota and CNS Inflammatory Demyelination
Multiple Sclerosis (MS) is an immune-mediated debilitating disease initiated by the immune system attacking the neuron protecting myelin sheet, which results in inflammation, chronic demyelination, axonal degeneration, and loss of brain volume (67, 68). MS affects around 400,000 people in the United States (69) and 2.5 million worldwide, mainly living in higher latitudes (70). MS is divided into four clinical types: Relapsing-Remitting (RR-MS), Secondary Progressive (SP-MS), Progressive Relapsing (PR-MS), and Primary Progressive (PP-MS), with the majority of patients suffering from RR-MS type. There are various environmental risk factors associated with disease onset and progression (vitamin D, latitude, viral infections, smoking, diet) and genetic disposition (71). The immunopathology of MS is mainly driven by inflammatory CD4+ T cell responses characterized by an increase in Th1 and Th17 cells (72) and decreased or impairment in Treg cells (73). Increasing importance is now appreciated for B cells’ role in both the progression and modulation of this condition (74). CD20+ B cells are targeted with monoclonal antibodies as approved MS therapies (75). Cerebrospinal fluid (CSF) of MS patients contains oligoclonal bands produced by plasmablasts and plasma cells (76), some of them auto-reactive against myelin self-peptides (77). B cells are present in CSF, CNS parenchyma, and meninges of MS patients, and germinal centers were identified in MS CNS (78). A recent study of single-cell RNA sequencing in CSF and blood suggest that in MS patients, B cells are clonally expanded and show an active inflammatory signature with memory plasma cell or plasmablast phenotype (79). More significant to this review’s context, IgA produced by B cells that cross-react with gut microbes have been identified in the CNS of MS patients with active lesions (80) and regulate neuroinflammation through IL-10 production (81), highlighting the importance of B cells on the gut-microbiota-brain axis. In addition to T and B cells, dendritic cells and CD8+ T cells also play a role in modifying MS’s pathology (82).
Gut microbiota is considered an environmental factor that can promote protective roles in the development of multiple sclerosis. Other environmental risk factors for MS, such as diet, vitamin D, or geography, can directly affect the microbiota’s composition. Gut microbiota can activate immune cells in the intestine or secrete immunomodulatory molecules and metabolites that orchestrate immune responses in the gut-brain axis. Previous studies suggest a link between changes in the composition of gut microbiota and MS pathogenesis. Limited but accumulating evidence points to an altered microbiota in MS patients compared to healthy individuals (57–66). The MS patients’ microbiota shows a decreased abundance of Bacteroides, Parabacteroides, Prevotella, and Lactobacillus genera and increased Akkermansia Blautia, Ruminococcus, and Bifidobacterium (83). The relative abundances of members of the domain Archaea, such as Methanobrevibacter, are also increased in MS patients’ gut and the bacteria such as Akkermansia, while Butyricimonas is decreased when compared to healthy controls (63). Recent findings link immunoglobulin (Ig) A (IgA)-coated gut microbiota with MS. IgA is the major neutralizing Ig in the human mucosa, including the gut, but is also found in circulation and periphery and a recent paper reports elevated IgA levels in cerebrospinal fluid of MS patients suffering active neuroinflammation (80). IgA+ B cells capable of recognizing gut microbiota are present within active CNS lesions, where elevated IL-10 transcripts are observed. Previous findings from the same group indicate that IL-10 was a principal component of gut-derived plasma cells’ immunoregulatory role against neuroinflammation (81). There is now increasing evidence for the gut microbiota’s role in the associated neuroinflammatory condition, neuromyelitis optica syndrome (84).
Despite the limitations of any experimental model of disease, EAE mice offer a practical approach to elucidate the clinical relevance of gut microbes in neuroinflammatory disorders, such as MS. The oral administration of broad-spectrum antibiotics reduces the severity of EAE (85–88). The treatment of C57BL/6 EAE mice with kanamycin, colistin, and vancomycin induced protection mediated by invariant natural killer cells T (iNKT) cells associated with reduced production of proinflammatory cytokines (IFN-γ, TNF-α, IL-6, and IL-17) in draining lymph nodes (LN) and a reduction in the percentages of mesenteric LN Th17 cells. In the mesenteric LNs of antibiotics-treated EAE mice, the levels of proinflammatory cytokines were reduced while IL-10 was increased (86). The treatment of SJL/J EAE mice with vancomycin, metronidazole, ampicillin, and neomycin induced protection against the disease that was associated with a reduced production of proinflammatory cytokines (IFN-γ, IL-17) in draining lymph nodes and increased frequencies of Foxp3 expressing CD25+CD4+T cells and increased levels of IL-10 and IL-13 (85). The ablation of CD25-expressing cells resulted in the lack of protection with antibiotics, while the adoptive transfer of CD25+CD4+ T cells with enhanced expression of Foxp3 isolated from antibiotics-treated SJL/J mice reduced the severity of EAE in recipient mice (85). The observation that antibiotics given orally but not intraperitoneally can protect the animals from disease emphasizes gut microbiota’s importance in EAE pathophysiology. The results obtained after oral versus intraperitoneal administration of ampicillin supported these findings (87). A significant reduction in inflammatory antigen-presenting cells (peripheral macrophages and resident microglia) was also observed and associated with the protective effects promoted by antibiotics against EAE (88). Mechanistically, the protective effects induced by the oral treatment with antibiotics could be associated with alterations in microbial populations capable of triggering molecular mimicry pathways towards autoimmunity (87). Studies in GF mice confirmed the impact of the presence of gut microbiota in the severity of EAE. In GF conditions, mice show reduced EAE severity and reduced peripheral proinflammatory signals (89, 90). The causative association between the gut microbiota and EAE remains to be elucidated. EAE induction promotes alterations in the composition of the gut microbiota at early phases of disease (44), and disease results in alterations in the intestinal permeability and intestinal proinflammatory responses (91).
The use of gnotobiotic mice in EAE studies helped identify a few essential bacteria for positively or negatively modulating the disease outcome. When GF mice are monocolonized with Segmented Filamentous Bacteria (SFB), an increase in Th17 cell-mediated responses correlate with exacerbated EAE severity (89). When the MS patients’ microbiota was transferred to germ-free mice, it caused more severe symptoms in the EAE (57) and spontaneous brain autoimmunity (58). Furthermore, fecal microbiota transplantation (FMT) is reported to alleviate disease symptoms in EAE mice (92) and MS patients (93–95). Although it is still elusive if alterations in microbiota’s composition and function are the cause or the result of the disease, microbiome-based therapeutics offer promise for MS treatment.
It has been shown that Prevotella histicola suppresses EAE through Tregs (96), while Lactobacillus reuteri exacerbates the disease through pathogenic CD4+ and CD8+ T cell responses (97). The prophylactic administration of individual lactobacilli strains reduces EAE severity through diminished myelin oligodendrocyte glycoprotein (MOG)- T cell reactivity (98). In contrast, the treatment with a mixture of three strains (Lactobacillus paracasei DSM 13434, Lactobacillus plantarum DSM 15312, and Lactobacillus plantarum DSM 15313) was able to reduce the progression of established severe EAE in a mechanism mediated by IL-10-producing Tregs (98). Single species of Enterococci, Escherichia coli, and others have also shown promising EAE study results (Table 1). The EAE model has successfully addressed the protective role of probiotic formulations with multiple species (105), some of which have already been assessed in MS patients (Table 1). A systematic review of the use of probiotic formulations in EAE and MS studies has been recently published (111).
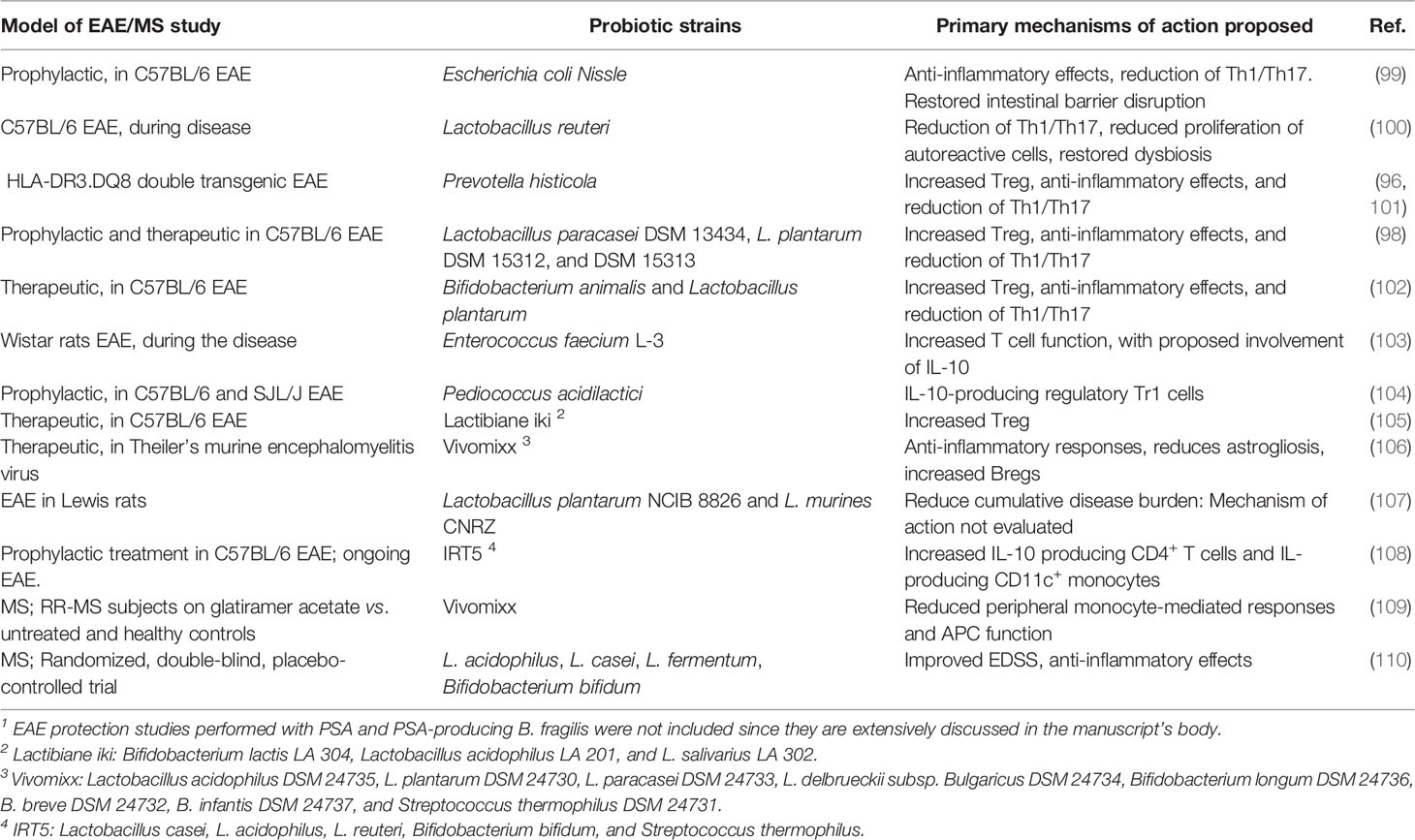
Table 1 Single probiotic species and probiotic multi-species mixes evaluated for protection in murine EAE 1 and MS clinical studies.
As discussed above, gut microbiota modulates CNS inflammatory demyelination in murine models. In the following sections, we discuss Bacteroides fragilis, and its capsular polysaccharide A (PSA), identified as a member of the gut microbiota and microbial product with immunomodulatory effects that we hypothesize can regulate the extent of neuroinflammatory mechanisms associated with CNS demyelinating diseases.
The Gut Microbiota as a Source for Immunomodulatory Factors: Polysaccharide A (PSA) Produced by Bacteroides Fragilis
Bacteroides fragilis (B. fragilis), a prominent species of the genus Bacteroides within the Gram-negative Bacteroidetes phylum is part of the normal microbiota of the human colon. Bacteroides species are among the most abundant and adept colonizers of the human gut due to their ability to efficiently utilize complex host and dietary glycans (112) and express different surface structures by phase variation (113). B. fragilis, an obligate anaerobic Gram-negative bacillus, colonizes the majority of healthy individuals (114) and has profound effects on host physiology (115). B. fragilis was initially identified as the most common anaerobe in clinical isolates from abscesses caused by abdominal trauma and bacteremia (116). When contained in the gut, B. fragilis plays an intricate role in the colon and develops a beneficial relationship with the host (9). Monocolonization of germ-free mice with B. fragilis leads to the immune system’s cellular and physical development (10, 117, 118). B. fragilis can also alleviate intestinal inflammation in animal models of colitis (12, 117, 119) and confer protection against infections (120–124). Recent studies show that B. fragilis exerts its beneficiary effects not only locally in the intestine but also systemically in extraintestinal tissues (13, 14, 125, 126) including CNS (15, 54, 127).
The capsular polysaccharide structure of B. fragilis plays an essential role in establishing a symbiotic relationship with its host (128). A large part of the B. fragilis genome is allocated to enzymes that degrade dietary polysaccharides and produce capsular polysaccharides of the organism (129–132). B. fragilis produces eight different distinct capsular polysaccharides, regulated by phase variation at the promoter region (133, 134). Variable expression of polysaccharides A through H creates a remarkable surface diversity which is vital for symbiosis (135, 136) and immunomodulation (112, 137). PSA is most abundantly expressed among those polysaccharides, and its immunomodulatory properties are most extensively studied (138).
PSA is a zwitterionic immunomodulatory polysaccharide consisting of a tetrasaccharide repeating unit (139, 140). The zwitterionic structure with a negative and a positive charge in each repeating unit is essential for the immunological potency of PSA (141, 142) and is required to activate T cells through the major histocompatibility complex II (MHCII) pathway (143, 144). High-resolution LC-MS/MS analysis shows that the terminal-reducing end of PSA contains a covalently attached lipid moiety required to activate antigen-presenting cells and protect against EAE (17). PSA is processed by antigen-presenting cells (APCs) through depolymerization in endocytic compartments in a nitric oxide-dependent manner (145, 146) and presented through the MHCII pathway to activate T cells (143, 147). The beneficial effects of PSA on the host immune system are manifested through multiple mechanisms. Microbial colonization in the gut is essential for host health. Host-specific microbiota is required for the full maturation of a functional immune system (148). Germ-free mice grown in sterile conditions develop physical and functional defects in their immune system, making them predisposed to infectious and inflammatory diseases (149). Recolonizing germ-free (GF) mice with PSA expressing B. fragilis mediates immune system development and can correct germ-free animals’ deficiencies (10). PSA-dependent colonization of B. fragilis in a unique mucosal niche in the gut results in the induction of regulatory T cells and suppression of Th17 cells (150). WT B. fragilis but not the ΔPSA mutant induces anti-inflammatory CD4+ CD45Rblow T cell population (119) and protects animals from the T cell transfer model of experimental colitis. In addition, animals orally treated with pure PSA (119) or PSA containing outer-membrane vesicles (OMVs) from WT B. fragilis (151) can protect animals from intestinal inflammation. PSA-mediated immunomodulation requires tolerogenic plasmacytoid dendritic cells (pDCs) (12), which activate a specific set of T cells defined as IL-10–producing CD4+CD25+Foxp3+ Treg cells with an inducible phenotype (11). Innate and adaptive immune responses initiated by PSA require toll-like receptor 2 (TLR2). TLR2 is necessary for inducing the genes (e.g., iNOS, MHCII, and CD86) required for processing and presenting PSA by APCs (152). As a result, PSA exposure of APCs increases the antigen presentation capacity of the cells by increasing the expression of MHCII and costimulatory signals, including ICOSL (12). The enhanced production of IL-10 triggered by pDCs after PSA recognition is ablated in the absence of ICOSL/ICOS signal (12). In addition, TLR2 expression on APCs is necessary to induce IL-10 producing CD4+ T cells (12) and protection against colitis (11, 12) and EAE (16, 17). PSA is recognized by the TLR2/TLR1 heterodimer in collaboration with Dectin-1 initiating a signaling cascade that involves the phosphoinositide 3-kinase (PI3K) pathway (17). Activation of PI3K pathway leads to phosphorylation and inactivation of glycogen synthase kinase 3β (GSK3β), promoting cAMP response element-binding protein (CREB)-dependent transcription of anti-inflammatory genes. Furthermore, TLR2 directs the expansion of CD39+CD4+ T cells in response to PSA, required for PSA-mediated protection against EAE. PSA’s EAE protection is ablated in TLR2 (16, 17), TLR1, and Dectin-1 deficient mice (17).
The interactions between PSA and the host’s intestinal dendritic cells are multifactorial. PSA recognition by colonic dendritic cells through TLR4-TRIF (TIR domain-containing adapter-inducing interferon-β) domain pathway, through the activation of interferon regulatory factors (IRFs), induces the production of IFN-β with anti-viral activity (153). The activation of the TLR4/TRIF pathway depends on the presence of a lipooligosaccharide (LOS) fraction linked covalently to the polysaccharide, anchoring the macromolecule to the outer membrane of B. fragilis (17). PSA produced by B. fragilis was identified as a symbiont factor promoting IFN-β-dependent protection against vesicular stomatitis virus infection. PSA’s anti-viral effects were lost in the absence of both TLR4 and IFN-β (153). Thus, the production of IFN-β by colonic dendritic cells with CD103+CD11b- and CD103-CD11b+ phenotypes is regulated by PSA and B. fragilis and likely by other commensal microbiota (153). Figure 1 summarizes the recognition and cellular signaling pathways triggered by PSA in dendritic cells that result in the activation of immunomodulation dominated by IL-10-producing CD4+ T cells and anti-viral responses.
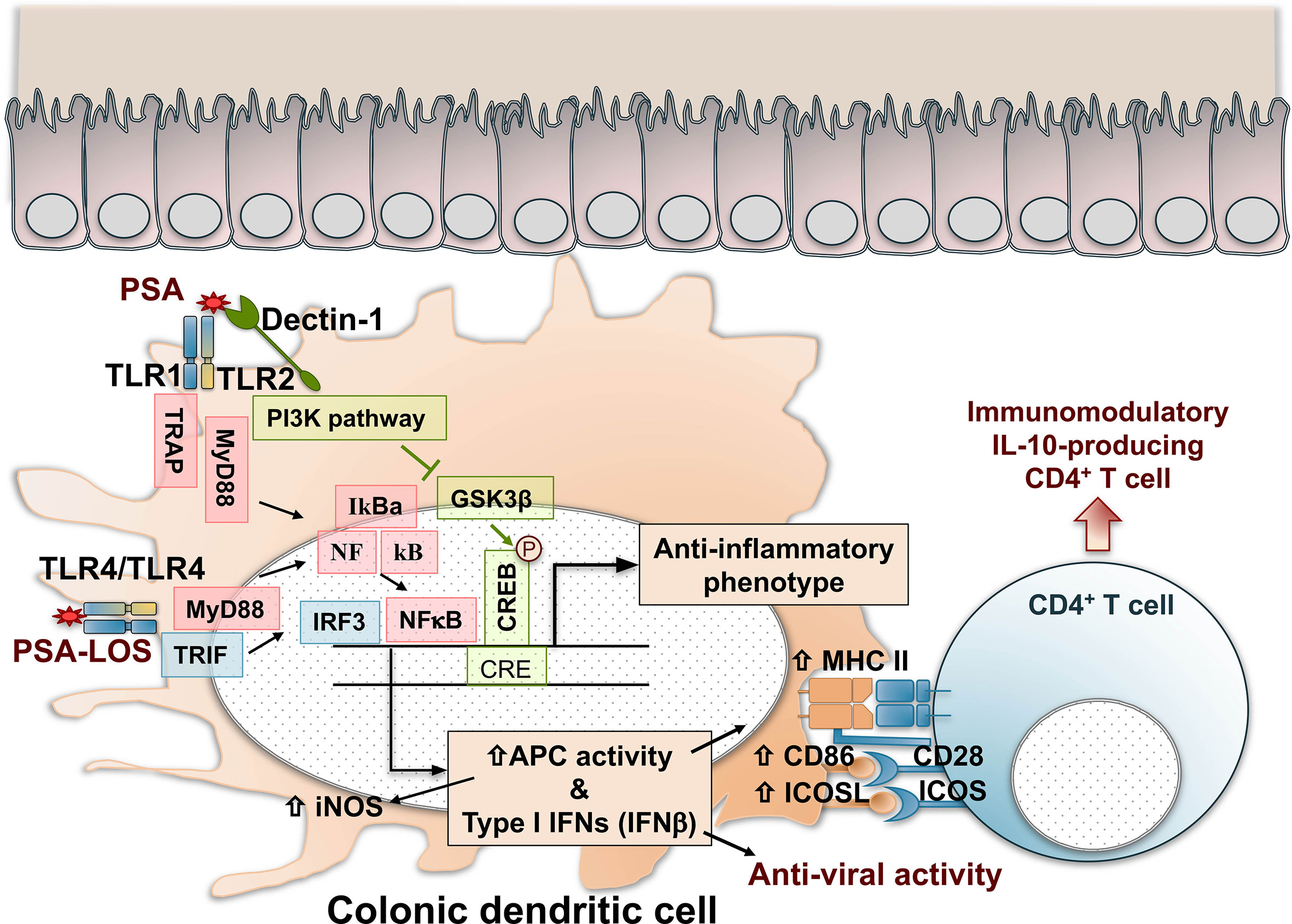
Figure 1 Recognition, cell signaling, and immunomodulatory pathways triggered by PSA in colonic dendritic cells. PSA is recognized by TLR1/TLR2 dimers that result in NF-kB nuclear translocation and IRF-mediated activation of Type I IFN gene expression and enhanced antigen processing and presentation by increased expression of iNOS, MHC class II molecules, and costimulatory signals mediated by CD86 and ICOSL. In addition, Dectin-1, a C-Type Lectin pattern recognition receptor, contributes with TLR2 in the cell signal activation through the PI3K pathway, resulting in the nuclear phosphorylation and activation of CREB, triggering the expression of anti-inflammatory genes. As a result, naïve CD4+ T cells are activated and differentiated in IL-10-producing immunomodulatory cells with Foxp3, CD39, Tr1 phenotypes that might depend on the inflammatory condition (IBD, asthma, EAE, or other). PSA recognition by TLR4 dimers induces the production of IFN-β with anti-viral activity through a MyD88 and TRIF-dependent pathway. The activation of TLR4/TRIF is dependent on the lipooligosaccharide (LOS) portion of the polysaccharide. CREB, cAMP response element–binding protein; GSK3β, glycogen synthase kinase 3β; IFN, interferon; IL-10, interleukin 10; iNOS, inducible nitric oxide synthase; IRF, interferon regulatory factors; MHC II, major histocompatibility complex class II; MyD88, myeloid differentiation primary response 88; NFkB, nuclear factor-kB; PSA, polysaccharide A; PI3K, phosphoinositide 3-kinase; TLR, toll-like receptor; TRAP, tumor necrosis factor receptor-associated protein. TRIF, TIR domain-containing adapter-inducing interferon-beta.
PSA Against Neuroinflammation
Studies demonstrating the impact of Bacteroides fragilis and PSA on EAE pathology (15, 127) were the first mechanistic examples of immunomodulation by gut microbiota in multiple sclerosis. B. fragilis and PSA’s beneficial effects beyond the gastrointestinal tract are observed most strikingly in the gut-brain axis using EAE. When antibiotic-treated mice were colonized with WT B. fragilis but not the ΔPSA mutant, they were protected from EAE (127). Immunoprotection by PSA in EAE requires TLR2, TLR1, and Dectin-1 (17) and a specific tolerogenic DC subset called plasmacytoid dendritic cells (pDC), as shown in Figure 1 (12). In the EAE model, WT B. fragilis protects by inducing Foxp3+ Tregs and IL-10, whereas the ΔPSA mutant induces proinflammatory cytokines IL-17 IL-6 and causes pathology. In addition, CD103+CD11c+ DCs isolated from cervical lymph nodes of ΔPSA-colonized animals are unable to convert Foxp3-CD4+ T cells into Foxp3+Treg cells (127). Furthermore, prophylactic or therapeutic treatment with pure PSA is sufficient to protect animals from EAE (15). PSA can induce accumulation of CD11c+CD103+ DCs in cervical lymph nodes, which convert naive CD4+ T cells into Foxp3+ Treg cells (15). IL-10–deficient mice are not protected from EAE, showing that PSA’s immunoprotection in the EAE model requires IL-10–producing Treg cells induced by tolerogenic DCs (15). In addition, PSA-induced regulatory T cells express CD39 independent of their Foxp3 expression and require TLR2 for activation (16). CD39 [nucleoside triphosphate diphosphohydrolase-1 (NTPDase 1)] is an ectoenzyme that degrades ATP released from damaged cells to AMP and adenosine. Previous studies have shown that CD39 is expressed by regulatory T cells (145) and has an essential role in suppressing Th17 cells (154, 155). CD39+ Tregs are reduced in MS patients (154), contributing to the Th17-driven pathology in this disease. CD39 deficiency increases IL-17 and decreases IL-10 production in mice and abrogates PSA-induced immunoprotection in EAE (16).
Furthermore, CD39 enhances the migratory capacity of CD4+ T cells, which results in PSA-dependent accumulation of CD39+ CD4+ Foxp3+ regulatory T cells in the CNS (156). In the DC-T cell coculture system, using cells from healthy human peripheral blood mononuclear cells (PBMCs), PSA induces IL-10 producing CD39+Foxp3+ cells in vitro (157). Furthermore, PSA increases the expression of CD39 and IL-10 and enhances the suppressive function of Foxp3+CD4+ cells (157). Similarly, PSA drives differentiation of regulatory T cells and IL-10 production using naïve T cells from MS patients (158). Notably, induced expression of Foxp3 in response to PSA was higher in MS patients than in healthy controls (158).
The protective effects of PSA against neuroinflammation were also addressed in a murine model of viral encephalitis. The induction of neuroinflammation with Herpes Virus was controlled by PSA’s administration that promoted a protective mechanism by IL-10 (126). The phenotypes of IL-10-producing cells induced by the PSA treatment were heterogeneous, with inductions of ICOS+CD39+CD37+CD4+ T cells, CD37+CD8+ T cells, and IL-10-producing B cells. IL-10-producing Tregs were increased in draining LNs of PSA-treated mice compared to PBS-treated mice. The protection against neuroinflammation triggered by the virus was also observed when mice were treated with PSA-producing B. fragilis (126). Thus, it appears that PSA is a potent modulator of neuroinflammation, in addition to the protective effects observed against infections (120–124), autoimmunity at the intestinal level (12, 117, 119), asthma (13), or pulmonary inflammation (14). More work is necessary to elucidate whether PSA’s phenotypes depend on the inflammatory pathways triggered in specific target tissues of the different disorders. A recent paper showed that PSA promotes the activation of an interferon responsive gene (IRG) signature responsible for producing inflammatory cytokines and cellular signals resulting in PD1, Lag3, and Tim3 expression (159). PSA regulates Type I interferons’ production by colonic dendritic cells, specifically IFN-β by TLR4-TRIF domain signaling mechanisms (153). The production of IFN-β directs the anti-viral protective responses induced by PSA (153).
Conclusions
The microbiome research field had expanded continuously since the early 2000s, which helped us understand the human microbiome’s role in health and disease. In recent years, the field’s scope shifted from the characterization of the microbiota composition and its association to diseases to mechanistic and causative understanding of microbes on human health.
The most prominent and most studied effect of gut microbiota is on the immune system, which can influence a wide range of infectious, inflammatory, metabolic, and autoimmune diseases (160). The immune system plays an essential role in the bidirectional communication within the gut-brain axis and modulates diseases in the CNS, including multiple sclerosis. Several different microbial species have been associated with susceptibility to and progression of multiple sclerosis. However, this is still an emerging field, and researchers must address numerous challenges in their research.
Separating correlation from causation in microbiome-disease association studies is one of the biggest challenges in the field. The literature has been dominated by associative studies comparing differences in microbiomes of MS patients with healthy controls. To translate these association studies into the clinic, researchers need to discover which of these differences are causing the disease. In addition, it is challenging to define “healthy microbiota” since there is tremendous intrapersonal and interpersonal variability in the composition of the human microbiota. Dysbiosis referring to disturbances in the microbiota structure is widely used without specific definitions of balanced and imbalanced microbial communities’ compositions. Furthermore, while the GF and gnotobiotic animals are invaluable for microbiome research, animal studies’ applicability to humans needs to be verified.
An accumulating body of research has proven the potential use of gut symbionts as microbial therapeutics. Following the success of FMT in treating C. difficile infections, several companies are testing groups of microbes or individual bacteria in clinical trials. However, understanding molecular interactions that shape host-bacterial interactions is crucial for the effective design of microbial therapeutics. This review highlights the microbiota’s role in mediating immune responses to multiple sclerosis, focusing on an archetypical microbial molecule PSA. PSA is a hallmark of symbiotic molecules with immunoregulatory functions. Although further protection and toxicity studies are needed to address PSA’s applicability as a therapeutic, we hypothesize that PSA, and likely other unidentified gut symbiont factors, is a safe and effective alternative for treating multiple sclerosis and other CNS diseases.
Author Contributions
DE-H and JO-R contributed equally to the design and preparation of the manuscript, tables, and figures. DK and LK contributed equally to the idea, the design, and the review of the manuscript. All authors contributed to the article and approved the submitted version.
Conflict of Interest
The authors declare that the research was conducted in the absence of any commercial or financial relationships that could be construed as a potential conflict of interest.
References
1. Ferreiro A, Crook N, Gasparrini AJ, Dantas G. Multiscale Evolutionary Dynamics of Host-Associated Microbiomes. Cell (2018) 172:1216–27. doi: 10.1016/j.cell.2018.02.015
2. Erturk-Hasdemir D, Kasper DL. Resident Commensals Shaping Immunity. Curr Opin Immunol (2013) 25:450–5. doi: 10.1016/j.coi.2013.06.001
3. HMP. The Integrative Human Microbiome Project: Dynamic Analysis of Microbiome-Host Omics Profiles During Periods of Human Health and Disease. Cell Host Microbe (2014) 16:276–89. doi: 10.1016/j.chom.2014.08.014
4. Proctor LM, Creasy HH, Fettweis JM, Lloyd-Price J, Mahurkar A, Zhou W, et al. The Integrative Human Microbiome Project. Nature (2019) 569:641–8. doi: 10.1038/s41586-019-1238-8
5. Pasolli E, Asnicar F, Manara S, Quince C, Huttenhower C, Correspondence NS. Extensive Unexplored Human Microbiome Diversity Revealed by Over 150,000 Genomes From Metagenomes Spanning Age, Geography, and Lifestyle. Cell (2019) 176:649–62. doi: 10.1016/j.cell.2019.01.001
6. Zhao Q, Elson CO. Adaptive Immune Education by Gut Microbiota Antigens. Immunology (2018) 154:28–37. doi: 10.1111/imm.12896
7. Zheng D, Liwinski T, Elinav E. Interaction Between Microbiota and Immunity in Health and Disease. Cell Res (2020) 30:492–506. doi: 10.1038/s41422-020-0332-7
8. Riccio P, Rossano R. The Human Gut Microbiota is Neither an Organ Nor a Commensal. FEBS Lett (2020) 594:3262–71. doi: 10.1002/1873-3468.13946
9. Erturk-Hasdemir D, Kasper DL. Finding a Needle in a Haystack: Bacteroides Fragilis Polysaccharide A as the Archetypical Symbiosis Factor. Ann N Y Acad Sci (2018) 1417:116–29. doi: 10.1111/nyas.13660
10. Mazmanian SK, Liu CH, Tzianabos AO, Kasper DL. An Immunomodulatory Molecule of Symbiotic Bacteria Directs Maturation of the Host Immune System. Cell (2005) 122:107–18. doi: 10.1016/j.cell.2005.05.007
11. Round JL, Mazmanian SK. Inducible Foxp3+ Regulatory T-cell Development by a Commensal Bacterium of the Intestinal Microbiota. Proc Natl Acad Sci U S A (2010) 107:12204–9. doi: 10.1073/pnas.0909122107
12. Dasgupta S, Erturk-Hasdemir D, Ochoa-Reparaz J, Reinecker H-C, Kasper DL. Plasmacytoid Dendritic Cells Mediate Anti-Inflammatory Responses to a Gut Commensal Molecule Via Both Innate and Adaptive Mechanisms. Cell Host Microbe (2014) 15:413–23. doi: 10.1016/j.chom.2014.03.006
13. Johnson JL, Jones MB, Cobb BA. Bacterial Capsular Polysaccharide Prevents the Onset of Asthma Through T-cell Activation. Glycobiology (2015) 25:368–75. doi: 10.1093/glycob/cwu117
14. Johnson JL, Jones MB, Cobb BA. Polysaccharide-Experienced Effector T Cells Induce IL-10 in FoxP3+ Regulatory T Cells to Prevent Pulmonary Inflammation. Glycobiology (2018) 28:50–8. doi: 10.1093/glycob/cwx093
15. Ochoa-Repáraz J, Mielcarz DW, Wang Y, Begum-Haque S, Dasgupta S, Kasper DL, et al. A Polysaccharide From the Human Commensal Bacteroides Fragilis Protects Against CNS Demyelinating Disease. Mucosal Immunol (2010) 3:487–95. doi: 10.1038/mi.2010.29
16. Wang Y, Telesford KM, Ochoa-Reparaz J, Haque-Begum S, Christy M, Kasper EJ, et al. An Intestinal Commensal Symbiosis Factor Controls Neuroinflammation Via TLR2-mediated CD39 Signalling. Nat Commun (2014) 5:4432. doi: 10.1038/ncomms5432
17. Erturk-Hasdemir D, Oh SF, Okan NA, Stefanetti G, Gazzaniga FS, Seeberger PH, et al. Symbionts Exploit Complex Signaling to Educate the Immune System. Proc Natl Acad Sci U S A (2019) 116:26157–66. doi: 10.1073/PNAS.1915978116
18. Procaccini C, De Rosa V, Pucino V, Formisano L, Matarese G. Animal Models of Multiple Sclerosis. Eur J Pharmacol (2015) 759:182–91. doi: 10.1016/j.ejphar.2015.03.042
19. Ochoa-Repáraz J, Kirby TO, Kasper LH. The Gut Microbiome and Multiple Sclerosis. Cold Spring Harbor Perspect Med (2018) 1:a029017. doi: 10.1101/cshperspect.a029017
20. Rackaityte E, Halkias J, Fukui EM, Mendoza VF, Hayzelden C, Crawford ED, et al. Viable Bacterial Colonization is Highly Limited in the Human Intestine In Utero. Nat Med (2020) 26:599–607. doi: 10.1038/s41591-020-0761-3
21. Milani C, Duranti S, Bottacini F, Casey E, Turroni F, Mahony J, et al. The First Microbial Colonizers of the Human Gut: Composition, Activities, and Health Implications of the Infant Gut Microbiota. Microbiol Mol Biol Rev (2017) 81:e00036–17. doi: 10.1128/mmbr.00036-17
22. Olszak T, An D, Zeissig S, Vera MP, Richter J, Franke A, et al. Microbial Exposure During Early Life has Persistent Effects on Natural Killer T Cell Function. Science (2012) 336:489–93. doi: 10.1126/science.1219328
23. Ganal-Vonarburg SC, Hornef MW, Macpherson AJ. Microbial–Host Molecular Exchange and its Functional Consequences in Early Mammalian Life. Science (2020) 368:604–7. doi: 10.1126/science.aba0478
24. Gensollen T, Iyer SS, Kasper DL, Blumberg RS. How Colonization by Microbiota in Early Life Shapes the Immune System. Science (2016) 352:539–44. doi: 10.1126/science.aad9378
25. Chong CYL, Bloomfield FH, O’Sullivan JM. Factors Affecting Gastrointestinal Microbiome Development in Neonates. Nutrients (2018) 10:274. doi: 10.3390/nu10030274
26. Rutayisire E, Huang K, Liu Y, Tao F. The Mode of Delivery Affects the Diversity and Colonization Pattern of the Gut Microbiota During the First Year of Infants’ Life: A Systematic Review. BMC Gastroenterol (2016) 16:86. doi: 10.1186/s12876-016-0498-0
27. Tirone C, Pezza L, Paladini A, Tana M, Aurilia C, Lio A, et al. Gut and Lung Microbiota in Preterm Infants: Immunological Modulation and Implication in Neonatal Outcomes. Front Immunol (2019) 10:2910. doi: 10.3389/fimmu.2019.02910
28. Bokulich NA, Chung J, Battaglia T, Henderson N, Jay M, Li H, et al. Antibiotics, Birth Mode, and Diet Shape Microbiome Maturation During Early Life. Sci Translat Med (2016) 8:343ra82. doi: 10.1126/scitranslmed.aad7121
29. Li Y, Faden HS, Zhu L. The Response of the Gut Microbiota to Dietary Changes in the First Two Years of Life. Front Pharmacol (2020) 11:334. doi: 10.3389/fphar.2020.00334
30. Zivkovic AM, German JB, Lebrilla CB, Mills DA. Human Milk Glycobiome and its Impact on the Infant Gastrointestinal Microbiota. Proc Natl Acad Sci U S A (2011) 108:4653–8. doi: 10.1073/pnas.1000083107
31. Bäckhed F, Roswall J, Peng Y, Feng Q, Jia H, Kovatcheva-Datchary P, et al. Dynamics and Stabilization of the Human Gut Microbiome During the First Year of Life. Cell Host Microbe (2015) 17:690–703. doi: 10.1016/j.chom.2015.04.004
32. Yatsunenko T, Rey FE, Manary MJ, Trehan I, Dominguez-Bello MG, Contreras M, et al. Human Gut Microbiome Viewed Across Age and Geography. Nature (2012) 486:222–7. doi: 10.1038/nature11053
33. Eckburg PB, Bik EM, Bernstein CN, Purdom E, Dethlefsen L, Sargent M, et al. Diversity of the Human Intestinal Microbial Flora. Science (2005) 308:1635–8. doi: 10.1126/science.1110591
34. Matijašić M, Meštrović T, Paljetak HČ, Perić M, Barešić A, Verbanac D. Gut Microbiota Beyond Bacteria-Mycobiome, Virome, Archaeome, and Eukaryotic Parasites in IBD. Int J Mol Sci (2020) 21:2668. doi: 10.3390/ijms21082668
35. Dethlefsen L, McFall-Ngai M, Relman DA. An Ecological and Evolutionary Perspective on Human-Microbe Mutualism and Disease. Nature (2007) 449:811–8. doi: 10.1038/nature06245
36. Postler TS, Ghosh S. Understanding the Holobiont: How Microbial Metabolites Affect Human Health and Shape the Immune System. Cell Metab (2017) 26:110–30. doi: 10.1016/j.cmet.2017.05.008
37. LeBlanc JG, Milani C, de Giori GS, Sesma F, van Sinderen D, Ventura M. Bacteria as Vitamin Suppliers to Their Host: A Gut Microbiota Perspective. Curr Opin Biotechnol (2013) 24:160–8. doi: 10.1016/j.copbio.2012.08.005
38. Flint HJ, Scott KP, Duncan SH, Louis P, Forano E. Microbial Degradation of Complex Carbohydrates in the Gut. Gut Microbes (2012) 3:289–306. doi: 10.4161/gmic.19897
39. Sonnenburg ED, Smits SA, Tikhonov M, Higginbottom SK, Wingreen NS, Sonnenburg JL. Diet-Induced Extinctions in the Gut Microbiota Compound Over Generations. Nature (2016) 529:212–5. doi: 10.1038/nature16504
40. Rook G, Bäckhed F, Levin BR, McFall-Ngai MJ, McLean AR. Evolution, Human-Microbe Interactions, and Life History Plasticity. Lancet (2017) 390:521–30. doi: 10.1016/S0140-6736(17)30566-4
41. Guzman-Bautista ER, Suzuki K, Asami S, Fagarasan S. Bacteria-Immune Cells Dialog and the Homeostasis of the Systems. Curr Opin Immunol (2020) 66:82–9. doi: 10.1016/j.coi.2020.05.010
42. Young VB. Therapeutic Manipulation of the Microbiota: Past, Present, and Considerations for the Future. Clin Microb Infect (2016) 22:905–9. doi: 10.1016/j.cmi.2016.09.001
43. Miller I. The Gut–Brain Axis: Historical Reflections. Microb Ecol Health Dis (2018) 29:1542921. doi: 10.1080/16512235.2018.1542921
44. Colpitts SL, Kasper EJ, Keever A, Liljenberg C, Kirby T, Magori K, et al. A Bidirectional Association Between the Gut Microbiota and CNS Disease in a Biphasic Murine Model of Multiple Sclerosis. Gut Microbes (2017) 8:561–73. doi: 10.1080/19490976.2017.1353843
45. Cox LM, Weiner HL. Microbiota Signaling Pathways That Influence Neurologic Disease. Neurotherapeutics (2018) 15:135–45. doi: 10.1007/s13311-017-0598-8
46. Sampson TR, Debelius JW, Thron T, Janssen S, Shastri GG, Ilhan ZE, et al. Gut Microbiota Regulate Motor Deficits and Neuroinflammation in a Model of Parkinson’s Disease. Cell (2016) 167:1469–80. doi: 10.1016/j.cell.2016.11.018
47. Chao YX, Gulam MY, Chia NSJ, Feng L, Rotzschke O, Tan EK. Gut–Brain Axis: Potential Factors Involved in the Pathogenesis of Parkinson’s Disease. Front Neurol (2020) 11:849. doi: 10.3389/fneur.2020.00849
48. Kim MS, Kim Y, Choi H, Kim W, Park S, Lee D, et al. Transfer of a Healthy Microbiota Reduces Amyloid and Tau Pathology in an Alzheimer’s Disease Animal Model. Gut (2020) 69:283–94. doi: 10.1136/gutjnl-2018-317431
49. He Y, Li B, Sun D, Chen S. Gut Microbiota: Implications in Alzheimer’s Disease. J Clin Med (2020) 9:2042. doi: 10.3390/jcm9072042
50. Kelly JR, Borre Y, O’ Brien C, Patterson E, El Aidy S, Deane J, et al. Transferring the Blues: Depression-associated Gut Microbiota Induces Neurobehavioural Changes in the Rat. J Psychiatr Res (2016) 82:109–18. doi: 10.1016/j.jpsychires.2016.07.019
51. Capuco A, Urits I, Hasoon J, Chun R, Gerald B, Wang JK, et al. Current Perspectives on Gut Microbiome Dysbiosis and Depression. Adv Ther (2020) 37:1328–46. doi: 10.1007/s12325-020-01272-7
52. Jiang Hy, Zhang X, Yu Z, Zhang Z, Deng M, Zhao Jh, et al. Altered Gut Microbiota Profile in Patients With Generalized Anxiety Disorder. J Psychiatr Res (2018) 104:130–6. doi: 10.1016/j.jpsychires.2018.07.007
53. Peirce JM, Alviña K. The Role of Inflammation and the Gut Microbiome in Depression and Anxiety. J Neurosci Res (2019) 97:1223–41. doi: 10.1002/jnr.24476
54. Hsiao EY, McBride SW, Hsien S, Sharon G, Hyde ER, McCue T, et al. Microbiota Modulate Behavioral and Physiological Abnormalities Associated With Neurodevelopmental Disorders. Cell (2013) 155:1451–63. doi: 10.1016/j.cell.2013.11.024
55. Srikantha P, Hasan Mohajeri M. The Possible Role of the Microbiota-Gut-Brain-Axis in Autism Spectrum Disorder. Int J Mol Sci (2019) 20:2115. doi: 10.3390/ijms20092115
56. Burberry A, Wells MF, Limone F, Couto A, Smith KS, Keaney J, et al. C9orf72 Suppresses Systemic and Neural Inflammation Induced by Gut Bacteria. Nature (2020) 582:89–94. doi: 10.1038/s41586-020-2288-7
57. Berer K, Gerdes LA, Cekanaviciute E, Jia X, Xiao L, Xia Z, et al. Gut Microbiota From Multiple Sclerosis Patients Enables Spontaneous Autoimmune Encephalomyelitis in Mice. Proc Natl Acad Sci U S A (2017) 114:10719–24. doi: 10.1073/pnas.1711233114
58. Cekanaviciute E, Yoo BB, Runia TF, Debelius JW, Singh S, Nelson CA, et al. Gut Bacteria From Multiple Sclerosis Patients Modulate Human T Cells and Exacerbate Symptoms in Mouse Models. Proc Natl Acad Sci U S A (2017) 114:10713–8. doi: 10.1073/pnas.1711235114
59. Cignarella F, Cantoni C, Ghezzi L, Salter A, Dorsett Y, Chen L, et al. Intermittent Fasting Confers Protection in CNS Autoimmunity by Altering the Gut Microbiota. Cell Metab (2018) 27:1222–35.e6. doi: 10.1016/j.cmet.2018.05.006
60. Kohl HM, Castillo AR, Ochoa-Repáraz J. The Microbiome as a Therapeutic Target for Multiple Sclerosis: Can Genetically Engineered Probiotics Treat the Disease? Diseases (2020) 8:33. doi: 10.3390/diseases8030033
61. Miyake S, Kim S, Suda W, Oshima K, Nakamura M, Matsuoka T, et al. Dysbiosis in the Gut Microbiota of Patients With Multiple Sclerosis, With a Striking Depletion of Species Belonging to Clostridia Xiva and IV Clusters. PLoS One (2015) 10:e0137429. doi: 10.1371/journal.pone.0137429
62. Chen J, Chia N, Kalari KR, Yao JZ, Novotna M, Soldan MMP, et al. Multiple Sclerosis Patients Have a Distinct Gut Microbiota Compared to Healthy Controls. Sci Rep (2016) 6:28484. doi: 10.1038/srep28484
63. Jangi S, Gandhi R, Cox LM, Li N, von Glehn F, Yan R, et al. Alterations of the Human Gut Microbiome in Multiple Sclerosis. Nat Commun (2016) 7:12015. doi: 10.1038/ncomms12015
64. Tremlett H, Fadrosh DW, Faruqi AA, Hart J, Roalstad S, Graves J, et al. Associations Between the Gut Microbiota and Host Immune Markers in Pediatric Multiple Sclerosis and Controls. BMC Neurol (2016) 16:182. doi: 10.1186/s12883-016-0703-3
65. Tremlett H, Fadrosh DW, Faruqi AA, Zhu F, Hart J, Roalstad S, et al. Us Network of Pediatric Ms Centers. Gut Microbiota in Early Pediatric Multiple Sclerosis: A Case-Control Study. Eur J Neurol (2016) 23:1308–21. doi: 10.1111/ene.13026
66. Takewaki D, Suda W, Sato W, Takayasu L, Kumar N, Kimura K, et al. Alterations of the Gut Ecological and Functional Microenvironment in Different Stages of Multiple Sclerosis. Proc Natl Acad Sci U S A (2020) 117:22402–12. doi: 10.1073/pnas.2011703117
67. Dargahi N, Katsara M, Tselios T, Androutsou ME, De Courten M, Matsoukas J, et al. Multiple Sclerosis: Immunopathology and Treatment Update. Brain Sci (2017) 7:78. doi: 10.3390/brainsci7070078
68. Lemus HN, Warrington AE, Rodriguez M. Multiple Sclerosis: Mechanisms of Disease and Strategies for Myelin and Axonal Repair. Neurol Clin (2018) 36:1–11. doi: 10.1016/j.ncl.2017.08.002
69. Dilokthornsakul P, Valuck RJ, Nair KV, Corboy JR, Allen RR, Campbell JD. Multiple Sclerosis Prevalence in the United States Commercially Insured Population. Neurology (2016) 86:1014–21. doi: 10.1212/WNL.0000000000002469
70. Ghareghani M, Reiter RJ, Zibara K, Farhadi N. Latitude, Vitamin D, Melatonin, and Gut Microbiota Act in Concert to Initiate Multiple Sclerosis: A New Mechanistic Pathway. Front Immunol (2018) 9:2484. doi: 10.3389/fimmu.2018.02484
71. Baecher-Allan C, Kaskow BJ, Weiner HL. Multiple Sclerosis: Mechanisms and Immunotherapy. Neuron (2018) 97:742–68. doi: 10.1016/j.neuron.2018.01.021
72. Stromnes IM, Cerretti LM, Liggitt D, Harris RA, Goverman JM. Differential Regulation of Central Nervous System Autoimmunity by T H1 and TH17 Cells. Nat Med (2008) 14:337–42. doi: 10.1038/nm1715
73. Viglietta V, Baecher-Allan C, Weiner HL, Hafler DA. Loss of Functional Suppression by CD4+CD25+ Regulatory T Cells in Patients With Multiple Sclerosis. J Exp Med (2004) 199:971–9. doi: 10.1084/jem.20031579
74. Sabatino JJ, Zamvil SS, Hauser SL. B-Cell Therapies in Multiple Sclerosis. Cold Spring Harb Perspect Med (2019) 9:a032037. doi: 10.1101/cshperspect.a032037
75. Roach CA, Cross AH. Anti-CD20 B Cell Treatment for Relapsing Multiple Sclerosis. Front Neurol (2021) 11:595547. doi: 10.3389/fneur.2020.595547
76. Obermeier B, Mentele R, Malotka J, Kellermann J, Kümpfel T, Wekerle H, et al. Matching of Oligoclonal Immunoglobulin Transcriptomes and Proteomes of Cerebrospinal Fluid in Multiple Sclerosis. Nat Med (2008) 14:688–93. doi: 10.1038/nm1714
77. Warren KG, Catz I, Johnson E, Mielke B. Anti-Myelin Basic Protein and Anti-Proteolipid Protein Specific Forms of Multiple Sclerosis. Ann Neurol (1994) 35:280–9. doi: 10.1002/ana.410350307
78. Serafini B, Rosicarelli B, Magliozzi R, Stigliano E, Aloisi F. Detection of Ectopic B-Cell Follicles With Germinal Centers in the Meninges of Patients With Secondary Progressive Multiple Sclerosis. Brain Pathol (2004) 14:164–74. doi: 10.1111/j.1750-3639.2004.tb00049.x
79. Ramesh A, Schubert RD, Greenfield AL, Dandekar R, Loudermilk R, Sabatino JJ, et al. A Pathogenic and Clonally Expanded B Cell Transcriptome in Active Multiple Sclerosis. Proc Natl Acad Sci U S A (2020) 117:22932–43. doi: 10.1073/pnas.2008523117
80. Pröbstel A-K, Zhou X, Baumann R, Wischnewski S, Kutza M, Rojas OL, et al. Gut Microbiota-Specific Iga+ B Cells Traffic to the CNS in Active Multiple Sclerosis. Sci Immunol (2020) 5:eabc7191. doi: 10.1126/sciimmunol.abc7191
81. Rojas OL, Pröbstel A-K, Porfilio EA, Wang AA, Charabati M, Sun T, et al. Recirculating Intestinal Iga-Producing Cells Regulate Neuroinflammation Via IL-10. Cell (2019) 177:492–3. doi: 10.1016/j.cell.2019.03.037
82. Rangachari M, Kerfoot SM, Arbour N, Alvarez JI. Editorial: Lymphocytes in MS and EAE: More Than Just a CD4+ World. Front Immunol (2017) 8:133. doi: 10.3389/fimmu.2017.00133
83. Freedman SN, Shahi SK, Mangalam AK. The “Gut Feeling”: Breaking Down the Role of Gut Microbiome in Multiple Sclerosis. Neurotherapeutics (2018) 15:109–25. doi: 10.1007/s13311-017-0588-x
84. Zamvil SS, Spencer CM, Baranzini SE, Cree BAC. The Gut Microbiome in Neuromyelitis Optica. Neurotherapeutics (2018) 15:92–101. doi: 10.1007/s13311-017-0594-z
85. Yokote H, Miyake S, Croxford JL, Oki S, Mizusawa H, Yamamura T. NKT Cell-Dependent Amelioration of a Mouse Model of Multiple Sclerosis by Altering Gut Flora. Am J Pathol (2008) 173:1714–23. doi: 10.2353/ajpath.2008.080622
86. Ochoa-Repáraz J, Mielcarz DW, Ditrio LE, Burroughs AR, Foureau DM, Haque-Begum S, et al. Role of Gut Commensal Microflora in the Development of Experimental Autoimmune Encephalomyelitis. J Immunol (2009) 183:6041–50. doi: 10.4049/jimmunol.0900747
87. Miyauchi E, Kim S-W, Suda W, Kawasumi M, Onawa S, Taguchi-Atarashi N, et al. Gut Microorganisms Act Together to Exacerbate Inflammation in Spinal Cords. Nature (2020) 585:102–6. doi: 10.1038/s41586-020-2634-9
88. Seifert HA, Benedek G, Nguyen H, Gerstner G, Zhang Y, Kent G, et al. Antibiotics Protect Against EAE by Increasing Regulatory and Anti-Inflammatory Cells. Metab Brain Dis (2018) 33:1599–607. doi: 10.1007/s11011-018-0266-7
89. Lee YK, Menezes JS, Umesaki Y, Mazmanian SK. Proinflammatory T-cell Responses to Gut Microbiota Promote Experimental Autoimmune Encephalomyelitis. Proc Natl Acad Sci U S A (2011) 108(Suppl 1):4615–22. doi: 10.1073/pnas.1000082107
90. Berer K, Mues M, Koutrolos M, Rasbi ZA, Boziki M, Johner C, et al. Commensal Microbiota and Myelin Autoantigen Cooperate to Trigger Autoimmune Demyelination. Nature (2011) 479:538–41. doi: 10.1038/nature10554
91. Nouri M, Bredberg A, Weström B, Lavasani S. Intestinal Barrier Dysfunction Develops At the Onset of Experimental Autoimmune Encephalomyelitis, and can be Induced by Adoptive Transfer of Auto-Reactive T Cells. PLoS One (2014) 9:e106335. doi: 10.1371/journal.pone.0106335
92. Li K, Wei S, Hu L, Yin X, Mai Y, Jiang C, et al. Protection of Fecal Microbiota Transplantation in a Mouse Model of Multiple Sclerosis. Mediators Inflamm (2020) 2020:2058272. doi: 10.1155/2020/2058272
93. Borody T, Leis S, Campbell J, Torres M, Nowak A. Fecal Microbiota Transplantation (FMT) in Multiple Sclerosis (Ms). Am J Gastroenterol (2011) 106:S352. doi: 10.14309/00000434-201110002-00942
94. Makkawi S, Camara-Lemarroy C, Metz L. Fecal Microbiota Transplantation Associated With 10 Years of Stability in a Patient With SPMS. Neurol Neuroimmunol NeuroInflamm (2018) 5:e459. doi: 10.1212/NXI.0000000000000459
95. Engen PA, Zaferiou A, Rasmussen H, Naqib A, Green SJ, Fogg LF, et al. Single-Arm, Non-randomized, Time Series, Single-Subject Study of Fecal Microbiota Transplantation in Multiple Sclerosis. Front Neurol (2020) 11:978. doi: 10.3389/fneur.2020.00978
96. Mangalam A, Shahi SK, Luckey D, Karau M, Marietta E, Luo N, et al. Human Gut-Derived Commensal Bacteria Suppress CNS Inflammatory and Demyelinating Disease. Cell Rep (2017) 20:1269–77. doi: 10.1016/j.celrep.2017.07.031
97. Montgomery TL, Künstner A, Kennedy JJ, Fang Q, Asarian L, Culp-Hill R, et al. Interactions Between Host Genetics and Gut Microbiota Determine Susceptibility to CNS Autoimmunity. Proc Natl Acad Sci U S A (2020) 117:27516–27. doi: 10.1073/pnas.2002817117
98. Lavasani S, Dzhambazov B, Nouri M, Fåk F, Buske S, Molin G, et al. A Novel Probiotic Mixture Exerts a Therapeutic Effect on Experimental Autoimmune Encephalomyelitis Mediated by IL-10 Producing Regulatory T Cells. PLoS One (2010) 5:e9009. doi: 10.1371/journal.pone.0009009
99. Secher T, Kassem S, Benamar M, Bernard I, Boury M, Barreau F, et al. Oral Administration of the Probiotic Strain Escherichia Coli Nissle 1917 Reduces Susceptibility to Neuroinflammation and Repairs Experimental Autoimmune Encephalomyelitis-Induced Intestinal Barrier Dysfunction. Front Immunol (2017) 8:1096. doi: 10.3389/fimmu.2017.01096
100. He B, Hoang TK, Tian X, Taylor CM, Blanchard E, Luo M, et al. Lactobacillus Reuteri Reduces the Severity of Experimental Autoimmune Encephalomyelitis in Mice by Modulating Gut Microbiota. Front Immunol (2019) 10:385. doi: 10.3389/fimmu.2019.00385
101. Shahi SK, Freedman SN, Murra AC, Zarei K, Sompallae R, Gibson-Corley KN, et al. Prevotella Histicola, A Human Gut Commensal, Is as Potent as COPAXONE® in an Animal Model of Multiple Sclerosis. Front Immunol (2019) 10:462. doi: 10.3389/fimmu.2019.00462
102. Salehipour Z, Haghmorad D, Sankian M, Rastin M, Nosratabadi R, Soltan Dallal MM, et al. Bifidobacterium Animalis in Combination With Human Origin of Lactobacillus Plantarum Ameliorate Neuroinflammation in Experimental Model of Multiple Sclerosis by Altering CD4+ T Cell Subset Balance. Biomed Pharmacother (2017) 95:1535–48. doi: 10.1016/j.biopha.2017.08.117
103. Abdurasulova IN, Ermolenko EI, Matsulevich AV, Abdurasulova KO, Tarasova EA, Kudryavtsev IV, et al. Effects of Probiotic Enterococci and Glatiramer Acetate on the Severity of Experimental Allergic Encephalomyelitis in Rats. Neurosci Behav Phys (2017) 47:866–76. doi: 10.1007/s11055-017-0484-1
104. Takata K, Kinoshita M, Okuno T, Moriya M, Kohda T, Honorat JA, et al. The Lactic Acid Bacterium Pediococcus Acidilactici Suppresses Autoimmune Encephalomyelitis by Inducing IL-10-producing Regulatory T Cells. PLoS One (2011) 6:e27644. doi: 10.1371/journal.pone.0027644
105. Calvo-Barreiro L, Eixarch H, Ponce-Alonso M, Castillo M, Lebrón-Galán R, Mestre L, et al. A Commercial Probiotic Induces Tolerogenic and Reduces Pathogenic Responses in Experimental Autoimmune Encephalomyelitis. Cells (2020) 9:906. doi: 10.3390/cells9040906
106. Mestre L, Carrillo-Salinas FJ, Feliú A, Mecha M, Alonso G, Espejo C, et al. How Oral Probiotics Affect the Severity of an Experimental Model of Progressive Multiple Sclerosis? Bringing Commensal Bacteria Into the Neurodegenerative Process. Gut Microbes (2020) 12:1813532. doi: 10.1080/19490976.2020.1813532
107. Maassen CBM, Claassen E. Strain-Dependent Effects of Probiotic Lactobacilli on EAE Autoimmunity. Vaccine (2008) 26:2056–7. doi: 10.1016/j.vaccine.2008.02.035
108. Kwon H-K, Kim G-C, Kim Y, Hwang W, Jash A, Sahoo A, et al. Amelioration of Experimental Autoimmune Encephalomyelitis by Probiotic Mixture is Mediated by a Shift in T Helper Cell Immune Response. Clin Immunol (2013) 146:217–27. doi: 10.1016/j.clim.2013.01.001
109. Tankou SK, Regev K, Healy BC, Cox LM, Tjon E, Kivisakk P, et al. Investigation of Probiotics in Multiple Sclerosis. Mult Scler (2018) 24:58–63. doi: 10.1177/1352458517737390
110. Kouchaki E, Tamtaji OR, Salami M, Bahmani F, Daneshvar Kakhaki R, Akbari E, et al. Clinical and Metabolic Response to Probiotic Supplementation in Patients With Multiple Sclerosis: A Randomized, Double-Blind, Placebo-Controlled Trial. Clin Nutr (2017) 36:1245–9. doi: 10.1016/j.clnu.2016.08.015
111. Morshedi M, Hashemi R, Moazzen S, Sahebkar A, Hosseinifard E-S. Immunomodulatory and Anti-Inflammatory Effects of Probiotics in Multiple Sclerosis: A Systematic Review. J Neuroinflamm (2019) 16:231. doi: 10.1186/s12974-019-1611-4
112. Comstock LE. Importance of Glycans to the Host-Bacteroides Mutualism in the Mammalian Intestine. Cell Host Microbe (2009) 5:522–6. doi: 10.1016/j.chom.2009.05.010
113. Troy EB, Carey VJ, Kasper DL, Comstock LE. Orientations of the Bacteroides Fragilis Capsular Polysaccharide Biosynthesis Locus Promoters During Symbiosis and Infection. J Bacteriol (2010) 192:5832–6. doi: 10.1128/JB.00555-10
114. Zitomersky NL, Coyne MJ, Comstock LE. Longitudinal Analysis of the Prevalence, Maintenance, and IgA Response to Species of the Order Bacteroidales in the Human Gut. Infect Immun (2011) 79:2012–20. doi: 10.1128/IAI.01348-10
115. Wexler AG, Goodman AL. An Insider’s Perspective: Bacteroides as a Window Into the Microbiome. Nat Microbiol (2017) 2:17026. doi: 10.1038/nmicrobiol.2017.26
116. Polk F, Kasper D. Bacteroides Fragilis Subspecies in Clinical Isolates. Ann Intern Med (1977) 86:569–671. doi: 10.7326/0003-4819-86-5-569
117. An D, Oh SF, Olszak T, Neves JF, Avci FY, Erturk-Hasdemir D, et al. Sphingolipids From a Symbiotic Microbe Regulate Homeostasis of Host Intestinal Natural Killer T Cells. Cell (2014) 156:123–33. doi: 10.1016/j.cell.2013.11.042
118. Ennamorati M, Vasudevan C, Clerkin K, Halvorsen S, Verma S, Ibrahim S, et al. Intestinal Microbes Influence Development of Thymic Lymphocytes in Early Life. Proc Natl Acad Sci U S A (2020) 117:2570–8. doi: 10.1073/pnas.1915047117
119. Mazmanian SK, Round JL, Kasper DL. A Microbial Symbiosis Factor Prevents Intestinal Inflammatory Disease. Nature (2008) 453:620–5. doi: 10.1038/nature07008
120. Macfarlane GT, Hopkins MJ. Changes in Predominant Bacterial Populations in Human Faeces With Age and With Clostridium Difficile Infection. J Med Microbiol (2002) 51:448–54. doi: 10.1099/0022-1317-51-5-448
121. Pagliuca C, Cicatiello AG, Colicchio R, Greco A, Cerciello R, Auletta L, et al. Novel Approach for Evaluation of Bacteroides Fragilis Protective Role Against Bartonella Henselae Liver Damage in Immunocompromised Murine Model. Front Microbiol (2016) 7:1750. doi: 10.3389/fmicb.2016.01750
122. Li Z, Deng H, Zhou Y, Tan Y, Wang X, Han Y, et al. Bioluminescence Imaging to Track Bacteroides Fragilis Inhibition of Vibrio Parahaemolyticus Infection in Mice. Front Cell Infect Microbiol (2017) 7:170. doi: 10.3389/fcimb.2017.00170
123. Nagpal R, Tsuji H, Takahashi T, Nomoto K, Kawashima K, Nagata S, et al. Gut Dysbiosis Following C-section Instigates Higher Colonisation of Toxigenic Clostridium Perfringens in Infants. Benef Microbes (2017) 8:353–65. doi: 10.3920/BM2016.0216
124. Deng H, Yang S, Zhang Y, Qian K, Zhang Z, Liu Y, et al. Bacteroides Fragilis Prevents Clostridium Difficile Infection in a Mouse Model by Restoring Gut Barrier and Microbiome Regulation. Front Microbiol (2018) 9:2976. doi: 10.3389/fmicb.2018.02976
125. Li D, Pan Y, Xia X, Liang J, Liu F, Dou H, et al. Bacteroides Fragilis Alleviates the Symptoms of Lupus Nephritis Via Regulating CD1d and CD86 Expressions in B Cells. Eur J Pharmacol (2020) 884:173421. doi: 10.1016/j.ejphar.2020.173421
126. Ramakrishna C, Kujawski M, Chu H, Li L, Mazmanian SK, Cantin EM. Bacteroides Fragilis Polysaccharide A Induces IL-10 Secreting B and T Cells That Prevent Viral Encephalitis. Nat Commun (2019) 10:2153. doi: 10.1038/s41467-019-09884-6
127. Ochoa-Repáraz J, Mielcarz DW, Ditrio LE, Burroughs AR, Begum-Haque S, Dasgupta S, et al. Central Nervous System Demyelinating Disease Protection by the Human Commensal Bacteroides Fragilis Depends on Polysaccharide A Expression. J Immunol (2010) 185:4101–8. doi: 10.4049/jimmunol.1001443
128. Comstock LE, Kasper DL. Bacterial Glycans: Key Mediators of Diverse Host Immune Responses. Cell (2006) 126:847–50. doi: 10.1016/j.cell.2006.08.021
129. Cerdeno-Tarraga AM, Patrick S, Crossman LC, Blakely G, Abratt V, Lennard N, et al. Extensive DNA Inversions in the B. Fragilis Genome Control Variable Gene Expression. Science (2005) 307:1463–5. doi: 10.1126/science.1107008
130. Cao Y, Rocha ER, Smith CJ. Efficient Utilization of Complex N-linked Glycans is a Selective Advantage for Bacteroides Fragilis in Extraintestinal Infections. Proc Natl Acad Sci U S A (2014) 111:12901–6. doi: 10.1073/pnas.1407344111
131. Cuskin F, Lowe EC, Temple MJ, Zhu Y, Cameron EA, Pudlo NA, et al. Human Gut Bacteroidetes can Utilize Yeast Mannan Through a Selfish Mechanism. Nature (2015) 517:165–9. doi: 10.1038/nature13995
132. Pudlo NA, Urs K, Kumar SS, German JB, Mills DA, Martens EC. Symbiotic Human Gut Bacteria With Variable Metabolic Priorities for Host Mucosal Glycans. mBio (2015) 6:e01282–15. doi: 10.1128/mBio.01282-15
133. Pantosti A, Tzianabos A, Reinap BG, Onderdonk AB, Kasper DL. Bacteroides Fragilis Strains Express Multiple Capsular Polysaccharides. J Clin Microbiol (1993) 31:1850–5. doi: 10.1128/JCM.31.7.1850-1855.1993
134. Krinos CM, Coyne MJ, Weinacht KG, Tzianabos AO, Kasper DL, Comstock LE. Extensive Surface Diversity of a Commensal Microorganism by Multiple DNA Inversions. Nature (2001) 414:555–8. doi: 10.1038/35107092
135. Liu CH, Lee SM, Vanlare JM, Kasper DL, Mazmanian SK. Regulation of Surface Architecture by Symbiotic Bacteria Mediates Host Colonization. Proc Natl Acad Sci U S A (2008) 105:3951–6. doi: 10.1073/pnas.0709266105
136. Coyne MJ, Chatzidaki-Livanis M, Paoletti LC, Comstock LE. Role of Glycan Synthesis in Colonization of the Mammalian Gut by the Bacterial Symbiont Bacteroides Fragilis. Proc Natl Acad Sci U S A (2008) 105:13099–104. doi: 10.1073/pnas.0804220105
137. Blandford LE, Johnston EL, Sanderson JD, Wade WG, Lax AJ. Promoter Orientation of the Immunomodulatory Bacteroides Fragilis Capsular Polysaccharide A (PSA) is Off in Individuals With Inflammatory Bowel Disease (IBD). Gut Microbes (2019) 10:569–77. doi: 10.1080/19490976.2018.1560755
138. Surana NK, Kasper DL. The Yin Yang of Bacterial Polysaccharides: Lessons Learned From B. Fragilis PSA. Immunol Rev (2012) 245:13–26. doi: 10.1111/j.1600-065X.2011.01075.x
139. Baumann H, Tzianabos a O, Brisson JR, Kasper DL, Jennings HJ. Structural Elucidation of Two Capsular Polysaccharides From One Strain of Bacteroides Fragilis Using High-Resolution NMR Spectroscopy. Biochemistry (1992) 31:4081–9. doi: 10.1021/bi00131a026
140. Sharma S, Erickson KM, Troutman JM. Complete Tetrasaccharide Repeat Unit Biosynthesis of the Immunomodulatory Bacteroides Fragilis Capsular Polysaccharide a. ACS Chem Biol (2017) 12:92–101. doi: 10.1021/acschembio.6b00931
141. Tzianabos AO, Onderdonk AB, Rosner B, Cisneros RL, Kasper DL. Structural Features of Polysaccharides That Induce Intra-Abdominal Abscesses. Science (1993) 262:416–9. doi: 10.1126/science.8211161
142. Tzianabos AO, Kasper DL, Cisneros RL, Smith RS, Onderdonk AB. Polysaccharide-Mediated Protection Against Abscess Formation in Experimental Intra-Abdominal Sepsis. J Clin Invest (1995) 96:2727–31. doi: 10.1172/JCI118340
143. Cobb BA, Wang Q, Tzianabos AO, Kasper DL. Polysaccharide Processing and Presentation by the MHCII Pathway. Cell (2004) 117:677–87. doi: 10.016/j.cell.2004.05.001
144. Cobb BA, Kasper DL. Zwitterionic Capsular Polysaccharides: The New MHCII-dependent Antigens. Cell Microbiol (2005) 7:1398–403. doi: 10.1111/j.1462-5822.2005.00591.x
145. Duan J, Avci FY, Kasper DL. Microbial Carbohydrate Depolymerization by Antigen-Presenting Cells: Deamination Prior to Presentation by the MHCII Pathway. Proc Natl Acad Sci U S A (2008) 105:5183–8. doi: 10.1073/pnas.0800974105
146. Duan J, Kasper DL. Oxidative Depolymerization of Polysaccharides by Reactive Oxygen/Nitrogen Species. Glycobiology (2011) 21:401–9. doi: 10.1093/glycob/cwq171
147. Kalka-Moll WM, Tzianabos AO, Bryant PW, Niemeyer M, Ploegh HL, Kasper DL. Zwitterionic Polysaccharides Stimulate T Cells by MHC Class II-dependent Interactions. J Immunol (2002) 169:6149–53. doi: 10.4049/jimmunol.169.11.6149
148. Chung H, Pamp SJ, Hill JA, Surana NK, Edelman SM, Troy EB, et al. Gut Immune Maturation Depends on Colonization With a Host-Specific Microbiota. Cell (2012) 149:1578–93. doi: 10.1016/j.cell.2012.04.037
149. Fiebiger U, Bereswill S, Heimesaat MM. Dissecting the Interplay Between Intestinal Microbiota and Host Immunity in Health and Disease: Lessons Learned From Germfree and Gnotobiotic Animal Models. Eur J Microbiol Immunol (2016) 6:253–71. doi: 10.1556/1886.2016.00036
150. Round JL, Lee SM, Li J, Tran G, Jabri B, Chatila T a. Mazmanian SK. The Toll-like Receptor 2 Pathway Establishes Colonization by a Commensal of the Human Microbiota. Science (2011) 332:974–7. doi: 10.1126/science.1206095
151. Shen Y, Torchia MLG, Lawson GW, Karp CL, Ashwell JD, Mazmanian SK. Outer Membrane Vesicles of a Human Commensal Mediate Immune Regulation and Disease Protection. Cell Host Microbe (2012) 12:509–20. doi: 10.1016/j.chom.2012.08.004
152. Wang Q, McLoughlin RM, Cobb BA, Charrel-Dennis M, Zaleski KJ, Golenbock D, et al. A Bacterial Carbohydrate Links Innate and Adaptive Responses Through Toll-like Receptor 2. J Exp Med (2006) 203:2853–63. doi: 10.1084/jem.20062008
153. Stefan KL, Kim MV, Iwasaki A, Kasper DL. Commensal Microbiota Modulation of Natural Resistance to Virus Infection. Cell (2020) 183:1312–24.e10. doi: 10.1016/j.cell.2020.10.047
154. Fletcher JM, Lonergan R, Costelloe L, Kinsella K, Moran B, O’Farrelly C, et al. Cd39 + Foxp3 + Regulatory T Cells Suppress Pathogenic Th17 Cells and Are Impaired in Multiple Sclerosis. J Immunol (2009) 183:7602–10. doi: 10.4049/jimmunol.0901881
155. Borsellino G, Kleinewietfeld M, Di Mitri D, Sternjak A, Diamantini A, Giometto R, et al. Expression of Ectonucleotidase CD39 by Foxp3+ Treg Cells: Hydrolysis of Extracellular ATP and Immune Suppression. Blood (2007) 110:1225–32. doi: 10.1182/blood-2006-12-064527
156. Wang Y, Begum-Haque S, Telesford KM, Ochoa-Repáraz J, Christy M, Kasper EJ, et al. A Commensal Bacterial Product Elicits and Modulates Migratory Capacity of CD39+ Cd4 T Regulatory Subsets in the Suppression of Neuroinflammation. Gut Microbes (2014) 5:552–61. doi: 10.4161/gmic.29797
157. Telesford KM, Yan W, Ochoa-Reparaz J, Pant A, Kircher C, Christy MA, et al. A Commensal Symbiotic Factor Derived From Bacteroides Fragilis Promotes Human CD39+Foxp3+ T Cells and Treg Function. Gut Microbes (2015) 6:234–42. doi: 10.1080/19490976.2015.1056973
158. Burgess JN, Pant AB, Kasper LH, Colpitts Brass S. Cd4+ T Cells From Multiple Sclerosis Patients Respond to a Commensal-Derived Antigen. Ann Clin Transl Neurol (2017) 4:825–9. doi: 10.1002/acn3.465
159. Alvarez CA, Jones MB, Hambor J, Cobb BA. Characterization of Polysaccharide A Response Reveals Interferon Responsive Gene Signature and Immunomodulatory Marker Expression. Front Immunol (2020) 11:556813. doi: 10.3389/fimmu.2020.556813
Keywords: immunomodulation, microbiota, EAE (experimental autoimmune encephalomyelitis), multiple sclerosis, symbiotic molecules, Bacteroides fragilis, polysaccharide A (PSA)
Citation: Erturk-Hasdemir D, Ochoa-Repáraz J, Kasper DL and Kasper LH (2021) Exploring the Gut-Brain Axis for the Control of CNS Inflammatory Demyelination: Immunomodulation by Bacteroides fragilis’ Polysaccharide A. Front. Immunol. 12:662807. doi: 10.3389/fimmu.2021.662807
Received: 01 February 2021; Accepted: 16 April 2021;
Published: 05 May 2021.
Edited by:
Paulus Stefan Rommer, Medical University of Vienna, AustriaReviewed by:
Carlos Rodrigo Camara-Lemarroy, University of Calgary, CanadaAnne-Katrin Pröbstel, University Hospital of Basel, Switzerland
Copyright © 2021 Erturk-Hasdemir, Ochoa-Repáraz, Kasper and Kasper. This is an open-access article distributed under the terms of the Creative Commons Attribution License (CC BY). The use, distribution or reproduction in other forums is permitted, provided the original author(s) and the copyright owner(s) are credited and that the original publication in this journal is cited, in accordance with accepted academic practice. No use, distribution or reproduction is permitted which does not comply with these terms.
*Correspondence: Javier Ochoa-Repáraz, jochoareparaz@ewu.edu
†These authors have contributed equally to this work and share first authorship