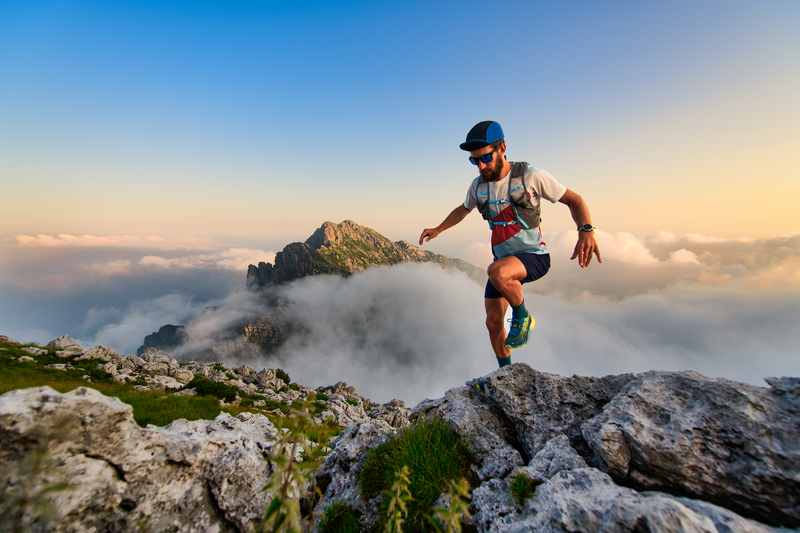
95% of researchers rate our articles as excellent or good
Learn more about the work of our research integrity team to safeguard the quality of each article we publish.
Find out more
ORIGINAL RESEARCH article
Front. Immunol. , 12 August 2021
Sec. Microbial Immunology
Volume 12 - 2021 | https://doi.org/10.3389/fimmu.2021.657449
The respiratory tract is considered the main port of entry of Mycobacterium leprae, the causative agent of leprosy. However, the great majority of individuals exposed to the leprosy bacillus will never manifest the disease due to their capacity to develop protective immunity. Besides acting as a physical barrier, airway epithelium cells are recognized as key players by initiating a local innate immune response that orchestrates subsequent adaptive immunity to control airborne infections. However, to date, studies exploring the interaction of M. leprae with the respiratory epithelium have been scarce. In this work, the capacity of M. leprae to immune activate human alveolar epithelial cells was investigated, demonstrating that M. leprae-infected A549 cells secrete significantly increased IL-8 that is dependent on NF-κB activation. M. leprae was also able to induce IL-8 production in human primary nasal epithelial cells. M. leprae-treated A549 cells also showed higher expression levels of human β-defensin-2 (hβD-2), MCP-1, MHC-II and the co-stimulatory molecule CD80. Furthermore, the TLR-9 antagonist inhibited both the secretion of IL-8 and NF-κB activation in response to M. leprae, indicating that bacterial DNA sensing by this Toll-like receptor constitutes an important innate immune pathway activated by the pathogen. Finally, evidence is presented suggesting that extracellular DNA molecules anchored to Hlp, a histone-like protein present on the M. leprae surface, constitute major TLR-9 ligands triggering this pathway. The ability of M. leprae to immune activate respiratory epithelial cells herein demonstrated may represent a very early event during infection that could possibly be essential to the generation of a protective response.
Leprosy is a chronic infectious disease caused by the obligate intracellular pathogen Mycobacterium leprae. The ability of this mycobacterium to infect Schwann cells may result in peripheral nerve damage with severe physical deformities, a hallmark of the disease (1). Despite the overall effectiveness of multidrug therapy (MDT), leprosy remains a public health problem with approximately 200,000 annual new registered cases worldwide (2). Even with the easy availability of MDT, it has largely failed to interrupt disease transmission. Furthermore, attempts to prevent the spread of M. leprae are severely constrained by the lack of an effective vaccine with potential prophylactic and therapeutic usages.
The disease is transmitted from person to person and the respiratory tract has been considered the most probable route of bacterial entry (3). It is well established, for example, that, in urban areas, the nasal discharge from leprosy patients with a high bacillary load (multibacillary [MB] patients) is the main source of infection (4). However, most M. leprae-infected individuals will generate an effective T helper 1 (Th1)-based immune response upon producing IFN-γ and will never develop any clinical sign of the disease (5, 6).
The epithelium of the respiratory tract serves as the first line of defense against invading airway microorganisms. Besides acting as a physical barrier, respiratory epithelium cells express a variety of pattern recognition receptors (PRRs), including Toll-like (TLRs), RIG-1-like, and NOD-like receptors that recognize the pathogen-associated molecular patterns (PAMPs), initiating a local innate immune response while orchestrating a subsequent adaptive immune response to control the infection. PAMPs recognition triggers signaling pathways mediated by Jak-Stat, NF-κB, and IRFs, leading to the secretion of cytokines, chemokines, reactive nitrogen and oxygen species, and anti-microbial peptides, all of which evoke immune cell recruitment along with a critical immune response for the early control of infection (7).
Over the years, many studies in the mycobacterial field have highlighted the prominent role played by the respiratory epithelium during Mycobacterium tuberculosis infection. After adhesion and internalization into epithelial cells, M. tuberculosis induces cytokine (such as TNF, IL-6 and IL-10) and chemokine (IL-8, IP-10, IL-27, MCP-1 and MIG) production that allows immune cells to migrate to the infection site and subsequent activation (8–10). However, very little is known about the interactions taking place between M. leprae and airway epithelial cells and even less about how these interactions may contribute to the infection outcome.
Interestingly, shortly after airborne or intranasal infection of mice with M. leprae, acid-fast bacilli are preferentially found in the lungs, and only rarely observed in the nasal mucosa. This demonstrates that the lungs are the main portal of both the entry and dissemination of the leprosy bacillus (11, 12). In a recent study, the capacity of M. leprae to infect nasal and alveolar epithelial cells was confirmed in in vitro cell culture assays (12). Moreover, the heparin-binding hemagglutinin (HBHA) protein and the histone-like protein (Hlp), previously implicated in the adhesion and internalization of M. tuberculosis in airway epithelial cells (13, 14), were also detected on the M. leprae surface and shown to promote mycobacterial interaction with these cells (12, 15).
Besides its role as an adhesin, Hlp, also referred to as a laminin-binding protein (LBP) (16), or mycobacterium DNA-binding protein 1 (MDP-1), was shown to elicit a protective immune response in mice against M. tuberculosis (17). This protective activity, occurring only when Hlp was administered together with mycobacterial DNA, and not when DNA or Hlp was injected alone, was dependent on TLR-9 recognition (17). Indeed, TLR-9, which recognizes unmethylated deoxycytidylate-phosphate-deoxyguanylate (CpG) motifs in viral and bacterial DNA (18), has been shown to be an important innate immune pathway for the generation of a protective immune response against M. tuberculosis (8, 19, 20). More recently, the M. leprae-derived DNA-Hlp complex was shown to activate the TLR-9 pathway (21).
In the present study, the capacity of M. leprae to immune activate alveolar epithelial cells by analyzing the secretion of cytokines and chemokines and the nuclear translocation of transcriptional factor NF-κB was tested. Moreover, due to the known involvement of TLR-9 in mycobacterial recognition and its expression in respiratory epithelial cells (8), the participation of this pathway in this activation was also examined. Finally, the potential role of bacterial surface-exposed DNA-Hlp complexes as TLR-9 ligands was explored.
It is hoped that the knowledge generated in this study may contribute to designing an effective leprosy vaccine.
M. leprae (Thai-5 strain), purified from the footpad of athymic mice (nu/nu), was kindly provided by Dr. Patrícia Sammarco Rosa from the Lauro de Souza Lima Institute (ILSL, Bauru, SP). Bacilli were γ-irradiated for 5 minutes using two linear electron accelerators, each equipped with 18 kW of power and 10 MeV of energy (Acelétron, Rio de Janeiro, RJ). The Mycobacterium smegmatis mc2 155 wild type (wt) and the knockout strain for the hlp gene (Δhlp) were donated by Dr. Thomas Dick of the University of Singapore. Mycobacterial strains were cultivated in Middlebrook 7H9 medium (Sigma-Aldrich), supplemented with 10% (v/v) ADC (albumin and dextrose) and 0.05% (v/v) Tween 80 at 37°C, under constant agitation, until the end of the exponential growth phase (OD600nm). M. smegmatis Δhlp was grown in the presence of 20 µg/mL of kanamycin.
The human alveolar epithelial cell line A549 and the murine macrophage RAW 264.7 were purchased from the American Type Culture Collection (ATCC). The cells were maintained, respectively, in DMEM/F12 and RPMI-1640 culture medium containing 2 mM L-Glutamine (LGC Biotechnology, SP, Brazil), and supplemented with 10% of fetal bovine serum (FBS) (Cultilab, SP, Brazil). Cultures were kept at 37°C within a humidified 5% CO2 atmosphere. The A549 cells were seeded for 24 hours at 37°C prior to stimulation in 6-well plates (5 x 105 cells for well) for the PCR assays and 24-well plates (7 x 104 cells for well) for the other assays. In the current study, we also used primary nasal epithelial cells obtained from the nasal polyps of patients submitted to polypectomy for nasal clearing (further details see [12]). The procedures described for the use of nasal polyps were approved by the Pedro Ernesto University Hospital, the State University of Rio de Janeiro, and the Oswaldo Cruz Foundation (FIOCRUZ) Ethical Committee located in Rio de Janeiro, RJ, Brazil. All participants provided their written consent.
A549 cells were incubated at different time periods at 37°C. For cell infected with live M. leprae cultures were incubated at 33°C. The stimuli used were: 1) live M. leprae; 2) irradiated-killed M. leprae; 3) CpG oligonucleotide (ODN 2395; 1 µM; Invivogen, San Diego, CA, USA); 4) M. leprae rHlp protein (0.5 µM); 5) CpG-rHlp complex; 6) LPS from Escherichia coli (1 µg/ml; Sigma-Aldrich, San Luis, MO, USA); and 7) M. smegmatis wt and Δhlp pre-incubated or not with 1 µM of CpG oligonucleotide. Human primary nasal epithelial cells were incubated only with irradiated-killed M. leprae for 24 hours at 37°C. For the experiment with RAW 264.7 cells, the stimuli used were: 1) CpG oligonucleotide (0.5 µM); 2) M. leprae rHlp protein (0.25 µM); 3) Hlp synthetic peptides (0.25 µM); 4) CpG-rHlp or -peptides complexes; and 5) LPS (100 ng/ml).
The rHlp protein was incubated with CpG oligo, as previously described (21), to obtain the CpG-Hlp complex. The same protocol was adopted to acquire the CpG-peptide complexes. The Hlp synthetic peptides p2, p3, and p10 (30 amino acids each) were donated by Dr. Tom Ottenhoff of the University Medical Center of Leiden (Netherlands). At the end of each incubation period, the cell culture supernatants were stored at -70°C for subsequent mediators quantification by commercial ELISA kits (R&D Systems, Minneapolis, MN, USA).
For the NF-κB translocation inhibitory experiments, pretreatment of the A549 cells was performed with the drugs wedelolactone (Sigma-Aldrich) at 80 µM or Bay11-7082 (Sigma-Aldrich) at 10 µM. Alternatively, the cells were also transfected with 2.5 µg of the expression plasmid pEGFP-C3 (Clontech, Kusatsu, Shiga, Japan) coding for a dominant-negative form of IκBα (DN-IκBα; kindly provided by Dr. Patrick Baeuerle of the Gene Center, Martinsried, Germany) (22) plus 1.25 ml of Lipofectamine-2000 reagent (Invitrogen, Carlsbad, CA, USA) according to the manufacturer’s instructions for 24 hours followed by washing with PBS and infection or stimulation as described above. For TLR-9 blocking assays, cells were pretreated with the synthetic antagonist E6446 (Eisai, Bunkyo-ku, Tokyo, Japan) at 0.2 µM.
Incubation times varied according to the type of experiment, as specified in the Results section. Each experiment was done in duplicate.
Total RNA of the treated A549 cells was extracted with the Trizol reagent (Life Technologies, Carlsbad, CA, EUA), according to the protocol provided by the manufacturer. The cDNA was obtained from the total RNA using the reverse transcriptase enzyme Superscript III® (Life Technologies) whereas qRT-PCR was performed using the SYBR Green I system (Applied Biosystems, Waltham, MA, USA), accompanied by specific primers for the coding sequences of the human β-defensin-2 (hβD-2) and 60S ribosomal protein L13 (RPL-13) genes in line with recommendations of the manufacturer, followed by incubating the reactions in the ViiA-7® qPCR system (Applied Biosystems). In each sample, the cDNA of the gene of interest (hβD-2) and the constitutive gene used as a normalizer (RPL-13) were amplified. Gene expression analysis was performed via the delta-delta CT (ΔΔCT) method. Once the ΔCT of the samples was determined, the cDNA in the experimental condition of the unstimulated cells was chosen as the normalizing sample. Relative gene expression values were obtained by applying the 2-ΔΔCT formula (23).
The A549 cells were resuspended in a hypotonic lysis buffer (10 mmol/L HEPES [pH 7.9], 1.5 mmol/L MgCl2, 10 nmol/L KCl, 0.05 mmol/L of PMSF, 0.5 mmol/L of dithiotheitirol, and a cocktail of protease inhibitors at 1x [Complete Mini® - Roche, Basel, Switzerland]) for 15 minutes after which 10% Igepal was added to complete the lysis. The homogenates were centrifuged (13,000 x g) for 30 seconds; and the pellet containing the nuclei was resuspended in a nuclear extraction hypertonic buffer (10 mmol/L HEPES [pH 7.9], 0.42 M NaCl, 1.5 mmol/L MgCl2, 10 nmol/L KCl, 0.5 mmol/L PMSF, and 1 mmol/L dithiotheitirol and protease inhibitors). After 40 minutes of continuous stirring, the extracts were centrifuged (13,000 x g) for 10 minutes at 4°C, and the proteins containing the nuclear extracts were quantified via the BCA technique (Pierce, Groton, CT, USA). The presence of NF-κB in the nuclear extract was determined by immunoblotting or ELISA.
For electrophoresis, in conjunction with the subsequent immunoblotting step, 10 µg of the nuclear protein extract were applied in gel with 15% polyacrylamide and 0.1% SDS. To perform the immunoblotting, the proteins placed in the gel were transferred to a nitrocellulose membrane (Hybond-C Extra - GE Healthcare, Chicago, IL, USA), incubated for 1 hour with anti-p65 monoclonal antibody (NF-κB subunit; Santa Cruz Biotechnology, Dallas, TX, USA) diluted 1:1000, followed by an additional 1 hour incubation period with anti-mouse IgG conjugated to peroxidase (Sigma-Aldrich) diluted 1:30000. Lamin A/C (1:1000; Santa Cruz Biotechnology) was used as a nuclear marker normalizer. The membrane was developed with the chemiluminescent substrate ECL (GE Healthcare) by exposing the membrane to a film that was later analyzed by densitometry through Image-J® software.
For the ELISA assays, 10 µg of nuclear extract protein were used, as stated in the manufacturer’s protocol (NF-κB p65 ELISA kit; e-Bioscience, Santa Clara, CA, USA), a methodology based on the detection of chemiluminescence caused by the binding of the NF-κB present in the nucleus (detection of the p65 subunit) in the consensus oligonucleotidal sequence attached to the plate. As a positive control, the kit includes proteins from purified nuclear extracts taken from treated cells.
The A549 cells were detached from the plate with 10 mM EDTA, washed with PBS containing 1% FSB, and incubated with monoclonal antibodies to MHC-II conjugated to allophycocyanine (APC) and CD80 conjugated to phycoerythrin (PE), in addition to their respective isotype controls (all from Biolegend, San Diego, CA, USA), at a concentration of 1:10 (v/v) at 4°C in the absence of light for 30 minutes. Finally, the cell suspension was fixed with 1% paraformaldehyde. The cells were analyzed by way of FACS Accuri flow cytometer (BD Bioscience, Franklin Lakes, NJ, USA), and the resulting data, via FlowJo V10 software (Tree Star).
Polystyrene microtiter plates (Corning Inc., Corning, NY, USA) were covered with 10 µg/mL of mycobacterial genomic DNA obtained from the culture mass of the Pasteur strain of M. bovis BCG (24), diluted in buffer carbonate/bicarbonate at 0.1 M (pH 9.6), and incubated overnight at 4°C. The wells were then washed with PBS and blocked for 2 hours at room temperature with PBS containing 3% bovine serum albumin (BSA; Sigma-Aldrich). After washing with PBS containing 0.05% Tween 20 (Sigma-Aldrich; PBS/T), different rHlp concentrations were added to the wells and incubated at 37°C for 2 hours. The wells were then incubated with the monoclonal antibody for Hlp (5G9; 1:500) (25) for 1 hour at 37°C, at which time the wells were once again washed with PBS/T, followed by incubation with peroxidase-conjugated anti-mouse IgG (1:1000; Sigma-Aldrich) for an additional period of 50 minutes at 37°C. Peroxidase activity was revealed by using hydrogen peroxide and tetramethylbenzidine (TMB; LGC Biotechnology). The colorimetric reaction was interrupted with 2.5 N sulfuric acid; and the optical density reading at 450nm was determined on a spectrophotometer via the SOFTmax®PRO 4.0 program (Life Sciences Edition, Molecular Devices Corporation).
Statistically significant differences among the values were determined using the GraphPad Prism 5 Project program (GraphPad Software Inc.), after applying the unpaired t test, the One-way analysis of the variance test (ANOVA) with Bonferroni, or the linear trend post-test, in which a p value of <0.05 was considered significant.
As a first step, an investigation was launched into whether M. leprae was able to induce the secretion of the chemokines MCP-1 (CCL2) and IL-8 (CXCL8) in alveolar type II pneumocytic epithelial A549 cells, known to play an important role in mediating immune cell migration to the infection site (26). For the quantification of MCP-1 and IL-8, the A549 cells were incubated or not with live or irradiated (killed) M. leprae for 24 and 48 hours and the chemokines were measured by ELISA in culture supernatants. The results showed that both live and killed M. leprae (bacterium:cell ratio of 10) induced the production of MCP-1 in A549 cells in view of the higher MCP-1 levels detected in the supernatants of the treated versus control cells after 24 hours of incubation (Figure 1A). However, at later time point of incubation (48 hours) only killed bacteria was able to induce MCP-1 (Supplementary Figure 1A). Both live and killed M. leprae were also able to induce IL-8 in A549 cells, but at higher bacterium:cell ratios (50 and 100), in a dose-dependent manner at 24 and 48 hours (p-values <0.05) (Figure 1B and Supplementary Figure 1B). To verify whether IL-8 induction also occurs in primary epithelial cells, we incubated human primary nasal epithelial cells isolated from four healthy volunteers with killed M. leprae for 24 hours at a bacterium:cell ratio of 10. Figure 1C shows that M. leprae is also able to induce IL-8 production in primary epithelial cells.
Figure 1 M. leprae induces secretion of chemokines and expression of hβD-2 gene in human respiratory epithelial cells. (A, B) ELISA quantification of MCP-1 (A) and IL-8 (B) levels in the culture supernatant of A549 cells stimulated with live or killed M. leprae at different bacterium:cell ratios for 24 hours. (C) ELISA quantification of IL-8 levels in the culture supernatants of primary human nasal epithelial cells obtained from 4 donors and stimulated with killed M. leprae at a bacterium:cell ratio of 10 for 24 hours. (D) Quantitative RT-PCR analysis of hβD-2 mRNA levels in A549 cells stimulated with live or killed M. leprae at different bacterium:cell ratios for 24 hours. (A, C, D) Unpaired t-test with differences considered statistically significant in relation to the untreated cells. (B) ANOVA test with differences considered statistically significant in relation to the untreated cells comparing the different doses by applying a linear-trend post-test. *p < 0.05; **p < 0.01; ****p < 0.0001. Values represent the mean ± standard deviation of at least 3 independent experiments performed in duplicate.
Alveolar epithelial cells are also able to produce such antimicrobial peptides as hβD-2 and LL-37 (8), known to be effective against mycobacteria (27–30). Therefore, as a next step, hβD-2 expression was evaluated by qRT-PCR in M. leprae-incubated A549 cells. Cells infected with live or treated with killed M. leprae at a bacterium:cell ratio of 100 for 24 hours showed significantly higher expression levels of hβD-2 over unstimulated cells (Figure 1D).
It has been shown that, in addition to MHC-II, type II pneumocytes can express several co-stimulatory molecules after different stimuli, indicating that these cells can take up, process, and then present antigens to T cells (31–36). Hence, we assessed by flow cytometry the expression of MHC-II and CD80 on the surface of A549 cells after incubation with live or killed mycobacteria (bacterium:cell ratio of 50) for 48 hours (Figure 2). Both the percentage of positive cells and median fluorescence intensity (M.F.I.) were significantly higher for MHC-II (Figure 2, left) and CD80 (Figure 2, right) when cells were treated with killed bacteria. Similar results were observed in cells infected with live bacteria, but statistical significance was reached only when the percentage of positive cells for MHC-II was evaluated (Figure 2, left). Altogether, these results indicate that independent of its viability M. leprae induces immune activation of epithelial cells of the respiratory tract.
Figure 2 Human alveolar epithelial cells express MHC-II and CD80 in response to M. leprae. Flow cytometry measurement of MHC-II (left) and CD80 (right) expression on the surface of A549 cells stimulated with live or killed M. leprae at a bacterium:cell ratio of 50 for 48 hours. Top panel shows the gating strategy to select the A549 cells population. The representative histogram plot together with the marker’s levels of positive cells and median fluorescence intensity (M.F.I.) are shown by immunofluorescent labeling using anti-MHC-II and anti-CD80 antibodies conjugated to APC and PE, respectively. Unpaired t-test with differences considered statistically significant in relation to the untreated cells. *p < 0.05. Results shown as representative of 3 independent experiments performed in duplicate.
NF-κB is the master transcriptional factor involved in the activation of the innate immune response (37). It was then investigated if M. leprae activates NF-κB in A549 cells by monitoring the translocation of the p65 subunit to the nucleus. The p65 levels in the nuclear extracts were determined by immunoblotting (Figure 3A) and ELISA (Figure 3B). Cells treated with killed M. leprae showed significantly higher p65 subunit levels in the nucleus at 30 minutes of stimulation than in untreated cells via both methods, indicating activation and translocation of NF-κB in epithelial cells. Comparable results were obtained with live M. leprae as shown in Figure 3B. To determine whether NF-κB activation was linked to the increased MCP-1 and IL-8 levels observed in M. leprae-stimulated cells, A549 cultures were infected with live or treated with killed bacteria for 24 hours in the presence of the pharmacological inhibitors wedelolactone (Figure 3C) or Bay11-7082 (Figure 3D). Both inhibitors impair the release of NF-κB from the cytosolic complex IκB/NF-κB, avoiding NF-κB translocation to the nucleus (38–40). Treatment with wedelolactone was able to reduce the amount of IL-8 to baseline levels in cells that were infected with live or incubated with killed bacteria (Figure 3C). Similar results were obtained with Bay11-7082 (Figure 3D). Interestingly, no effect of wedelolactone on MCP-1 levels was observed (Supplementary Figure 2).
Figure 3 M. leprae induces activation of the NF-κB transcription factor in human alveolar epithelial cells. The relative levels of p65 in the nuclear extract of A549 cells were measured by immunoblotting (A) and by ELISA (B). For immunoblotting assays, the cells were stimulated with killed M. leprae at a bacterium:cell ratio of 50 for 30, 60, and 120 minutes while for ELISA the cells were stimulated with live or killed M. leprae at bacterium:cell ratio of 50 for 30 minutes. Normalization was performed with lamin A/C and the densitometric units were arbitrary. Immunoblotting image shown as representative of 3 independent experiments performed in duplicate. (C, D) ELISA quantification of IL-8 levels in culture supernatants of A549 cells pretreated with wedelolactone (C) or Bay11-7082 (D) followed by stimulation with live or killed M. leprae at a bacterium:cell ratio of 50 for 24 hours. (E) ELISA quantification of IL-8 levels in culture supernatants of A549 cells transfected with DN-IκBα and stimulated with killed M. leprae at a bacterium:cell ratio of 50 for 24 hours. (A–E) Unpaired t-test with differences considered statistically significant between them (A, C–E) or in relation to the untreated cells (B). *p < 0.05; **p < 0.01; ****p < 0.0001. Values represent the mean ± standard deviation of at least 3 independent experiments performed in duplicate.
To confirm the involvement of NF-κB in IL-8 production in response to M. leprae, we also utilized DN-IκBα-transfected A549 cells, expressing a dominant-negative form of IκBα that lacks all N-terminal phosphorylation sites, thus being resistance to degradation but still with the ability of binding to NF-κB (22). Transfected cells incubated with killed M. leprae for 24 hours exhibited significant lower IL-8 levels in comparison with non-transfected cells or cells transfected with the empty vector used as negative control (Figure 3E). Identical results were obtained with transfected cells incubated for 48 hours (Supplementary Figure 3). Altogether, our results demonstrates that the production of IL-8 in A549 cells in response to M. leprae is dependent on NF-κB translocation to nucleus.
TLR-9 bacterial sensing has been shown to play an important role in the immune response against mycobacteria (19, 20). Since alveolar epithelial cells express TLR-9 (41), the potential involvement of this pathway in the immune activation induced by M. leprae in these cells was analyzed. Firstly, the capacity of the CpG-rHlp complex to stimulate A549 cells was evaluated by measuring IL-8 secretion. For these assays, the M. leprae recombinant Hlp was purified from E. coli and some tests were performed for quality control. When used to stimulate PBMC, the purified recombinant protein was unable to induce detectable levels of TNF production, pointing to low levels of LPS contamination (Supplementary Figure 4). As expected, M. leprae rHlp was also found to bind DNA (Supplementary Figure 5). In this assay, M. bovis BCG genomic DNA was used since Hlp, a highly conserved protein among mycobacterial species, was shown to bind in a non-specific way to nucleic acids molecules (42, 43). Moreover, as previously shown in the context of M. tuberculosis Hlp, M. leprae rHlp, when complexed to the CpG oligo, was able to significantly increase the capacity of CpG alone to induce TNF secretion in murine macrophages (Supplementary Figure 6B) (17). Furthermore, by using the 30 mer synthetic peptides p2, p3 and p10 with sequences derived from different regions of Hlp (44) (Supplementary Figure 6A), only the p3 peptide corresponding to the DNA-binding site of the protein (43) managed to increase CpG-induced TNF secretion in macrophages (Supplementary Figure 6B). As expected, rHlp or the peptides alone could not induce TNF (Supplementary Figure 6B). Altogether, these results confirm the capacity of recombinant Hlp to enhance DNA immune recognition by TLR-9, dependent on the DNA-binding site of the protein.
Figure 4A displays the results obtained after stimulating A549 cells with the CpG-rHlp complex. CpG and rHlp alone were also used to stimulate A549 cells; and LPS was included as a positive control. Supernatants of CpG-rHlp complex-stimulated cells for 24 hours showed significantly higher levels of IL-8 when compared to those collected from cells stimulated with CpG alone while the levels of IL-8 in cultures treated with rHlp alone ended up being similar to baseline levels (Figure 4A). We also evaluated the capacity of CpG-rHlp complex to activate NF-κB in A549 cells. LPS, a TLR-4 ligand, was also included as control. Through ELISA we detected a significant increase of p65 in the nuclear extract after 30 minutes of stimulation with CpG-rHlp or LPS (Figure 4B). Next, we showed that IL-8 production in response to the CpG-rHlp is dependent on NF-κB activation (Figures 4C, D). A549 cells pretreated with Bay11-7082 blocked the production of IL-8 after CpG-Hlp stimulation for 24 hours (Figure 4C). Moreover, DN-IκBα-transfected A549 incubated with CpG-Hlp for 24 (Figure 4D) and 48 hours (Supplementary Figure 7) also showed significantly lower levels of IL-8 production when compared to control cells. Hence, the stimulation of TLR-9 by CpG-Hlp in A549 cells triggers the activation of NF-κB, which subsequently leads to the production of IL-8.
Figure 4 Mycobacterial CpG-Hlp complex induces immune activation of human alveolar epithelial cells. Quantification of IL-8 levels in the culture supernatant (A, C, D) and assessment of the relative levels of p65 subunit in the nuclear extracts (B) of A549 cells through ELISA. (A) Cells were stimulated with CpG, rHlp, or CpG-Hlp complex for 48 hours. LPS was used as a positive control. (B) Cells were stimulated with CpG-Hlp complex or LPS for 30 minutes. (C, D) Cells were pretreated with Bay11-7082 (C) or transfected with DN-IκBα (D) and then stimulated with CpG-Hlp complex or LPS for 24 hours. (A) ANOVA test with differences considered statistically significant between them after applying Bonferroni post-test. (B–D) Unpaired t-test with differences considered statistically significant in relation to the untreated cells (B) or between them (C, D). *p < 0.05; **p < 0.01; ***p < 0.001. Values represent the mean ± standard deviation of at least 3 independent experiments performed in duplicate.
The question as to whether TLR-9 sensing plays a role in M. leprae immune recognition by A549 cells was then addressed by first stimulating them with increasing bacterium:cell ratios in the presence of E6446, a synthetic antagonist of TLR-9. LPS and the CpG-Hlp complex were included as negative and positive controls, respectively. After incubation, IL-8 levels were quantified in culture supernatants. E6446 was able to completely block the secretion of IL-8 by A549 cells in response to killed M. leprae after 24 (Figure 5A) and 48 hours (Supplementary Figure 8) of incubation at all bacterium:cell ratios tested. Comparable results were obtained with cells infected with live M. leprae, although statistical significance was reached only for the bacterium:cell ratio of 50 (Figure 5A and Supplementary Figure 8). As expected, the chemokine levels in the supernatant of LPS-stimulated cells were unaffected by the presence of E6446. In contrast, a complete inhibition of IL-8 secretion was observed in response to the CpG-Hlp complex. E6446 was also able to block the nuclear translocation of p65 induced by live/killed M. leprae in A549 cells as shown in Figure 5B. Altogether, these results demonstrate that production of IL-8 in response to M. leprae infection in A549 cells occurs via TLR-9/NF-κB.
Figure 5 M. leprae induces the secretion of IL-8 in human alveolar epithelial cells via TLR-9/NF-κB signaling. Quantification of IL-8 levels in the culture supernatant (A, C) and assessment of the relative levels of p65 subunit in the nuclear extracts (B) of A549 cells through ELISA. (A) Cells were pretreated with the TLR-9 antagonist E6446 and stimulated with live or killed M. leprae at different bacterium:cell ratios for 24 hours. CpG-Hlp complex and LPS were used as positive and negative controls, respectively. (B) Cells were pretreated with E6446 and stimulated with live or killed M. leprae at a bacterium:cell ratio of 50 for 30 minutes. (C) Cells were stimulated with M. smegmatis wt or Δhlp preincubated or not with CpG at a bacterium:cell ratio of 10, or with CpG alone for 48 hours. LPS was used as a positive control. (A, B) Unpaired t-test with differences considered statistically significant between them. (C) ANOVA test with differences considered statistically significant between them after applying Bonferroni post-test. *p < 0.05; **p < 0.01; ***p < 0.001. Values represent the mean ± standard deviation of at least 3 independent experiments performed in duplicate.
Since TLR-9 seems to play a prominent role in M. leprae immune recognition by alveolar epithelial cells, the hypothesis was raised that bacterial extracellular DNA anchored to Hlp molecules present on the bacterial surface could act as potent TLR-9 ligands by specifically triggering this immune activation pathway during infection. To test this hypothesis, experiments were conducted using M. smegmatis wild type (wt) and Δhlp mutant strains. This mycobacterium was chosen due to the complete inability to generate mutants in M. leprae. In addition, the Hlp protein is a highly conserved protein among the different species of mycobacteria (45). A549 cells were treated with M. smegmatis wt and Δhlp strains previously incubated or not with CpG oligo; and, after 48 hours, IL-8 levels were measured in culture supernatants. LPS-incubated A549 cells were included as positive controls. Both M. smegmatis strains were able to induce IL-8 production in A549 cells at similar levels. However, while pre-incubation of the wt strain with CpG resulted in approximately 2.3-fold more IL-8 when compared to cells stimulated with mycobacteria alone, this increment was not observed in the Δhlp strain (Figure 5C). These results strongly suggest that surface-exposed Hlp interacting with bacterial extracellular DNA forms DNA-Hlp complexes that may act as potent TLR-9 ligands during their interaction with host cells.
One of the most important gaps in knowledge concerning the natural course of M. leprae infection in humans revolves around the earliest interactions between the pathogen and the respiratory tract, considered the main infection route of the bacillus. Earlier studies have shown that, after airborne exposure or intranasal infection of mice, M. leprae can reach the lungs and is able to invade and survive inside pulmonary epithelial cells (11, 12). Given the proven weight of the respiratory pulmonary epithelium in generating an immune response against invading microorganisms (7), the present study investigated immune pathways activated in vitro in M. leprae-challenged alveolar epithelial cells.
In most experiments performed, the effects of live and killed M. leprae were compared, since in other cell types modulation of several host cell functions by M. leprae was shown to be induced only by live bacterium (46–48). To our surprise, in the case of A549 cells, all effects herein demonstrated were proven to be independent of bacterial viability. Initially, the capacity of M. leprae to induce the secretion of pro-inflammatory mediators in A549 cells was analyzed. Among the mediators evaluated, the chemokines MCP-1 and IL-8 showed higher levels in the supernatants of M. leprae-stimulated cells. M. leprae also augmented the secretion of IL-8 in human primary nasal epithelial cells, reinforcing that this response might occur in M. leprae-exposed individuals during bacterial entry through the respiratory airways.
MCP-1 is a chemoattracting molecule of CD4+ T cells and monocytes (49) whereas IL-8 predominantly attracts neutrophils, which are the first inflammatory cells to migrate to the infection site, restricting bacterial spread. The production of both chemokines by alveolar epithelial cells subsequent to M. tuberculosis infection has already been well demonstrated (50–55). The protective role of MCP-1 in mycobacterial infection has been documented in several studies as well. The presence of circulating MCP-1 serum levels was shown to be significantly greater in pulmonary as compared to extra-pulmonary tuberculosis patients and endemic individuals (56). Moreover, after being infected with BCG, mice overexpressing MCP-1 in type-II alveolar epithelial cells demonstrated increased pools of lung mononuclear phagocytes and significantly decreased mycobacterial loads in the bronchoalveolar space together with the rapid resolution of lung granuloma formation (57). Regarding IL-8, bronchoalveolar fluids from tuberculosis patients showed a dramatic uptick in the number of neutrophils in correlation with elevated concentrations of the chemokine (58, 59). Some studies also described the capacity of M. tuberculosis-induced IL-8 to act as a chemoattractant of CD4+ and CD8+ T cells in pulmonary granuloma, modulating the adaptive immune response (49, 60, 61).
Defensins are cationic peptides that participate in innate immunity due to their microbicidal activities, mainly through bacterial membrane permeabilization (62). Indeed, several studies indicate that A549 cells express hβD-2 after infection by mycobacteria, leading to a decrease in the intracellular bacillary load due to bacterial killing (27–30). In an earlier study, we showed that M. leprae was able to stay alive for at least 10 days within A549 cells (12). However, in that work cells were infected with 10x lower the bacterium:cell ratio needed to induce the expression of hβD-2, according to our current data (bacterium:cell ratio of 100). Thus, future studies are needed to confirm that hβD-2 produced by epithelial cells in response to M. leprae infection can promote bacterial killing.
In addition, significant hβD-2 levels have been detected in bronchoalveolar lavage fluid from patients with M. avium-intracellulare infection (63), corroborating the involvement of such peptides in the host defense and local remodeling of the respiratory tract in mycobacterial infection. Interestingly, one in vitro study showed low hβD-2 production in alveolar macrophages infected with M. tuberculosis, unlike the alveolar epithelial cells that produced large amounts of these peptides under the same conditions (27), inferring that epithelial cells, and not macrophages, are the main sources of hβD-2 during a primary mycobacterial lung infection. Defensins also exhibit chemotactic properties in initiating and regulating the immune response (64, 65). As to hβD-2 specifically, it is identified as capable of primarily attracting T cells but dendritic and mast cells as well (66–68). In a mouse model of pulmonary tuberculosis, β-defensin-2 immunostaining was detected in cells with dendritic morphology located nearby mediastinal lymph nodes, indicating its contribution to the establishment of a Th1 response, thus bridging the innate and adaptive immune responses (69).
In a next step, the effect of M. leprae stimulation of A549 cells on the expression of MHC-II and the co-stimulatory molecule CD80 was examined. Our data indicate that M. leprae was able to induce MHC-II and CD80 expression. These results suggest that, during post-M. leprae infection, alveolar epithelial cells not only recruit T cells as a consequence of producing pro-inflammatory mediators, but also assist in T cell activation by presenting antigens.
For the purpose of evaluating the mechanism of M. leprae-induced immune activation in alveolar epithelial cells, the involvement of NF-κB transcription factor was ascertained. The bacillus was found to be capable of inducing NF-κB activation in A549 cells by promoting the translocation of the p65 subunit to the nucleus. Moreover, cells treated with wedelolactone or Bay11-7082, two NF-κB inhibitors, were unable to produce IL-8 in response to M. leprae. Additionally, A549 cells overexpressing a dominat-negative IκBα mutant displayed lower levels of IL-8 in response to M. leprae. Interesting, in contrast to the clear activation of NF-κB in alveolar epithelial cells herein demonstrated, M. leprae has been shown to be both a weaker stimulator and even an inhibitor of this transcriptional factor in monocytes and Schwann cells (70, 71).
No effect on MCP-1 production was observed when supernatant MCP-1 was measured in A549 cells treated with wedelolactone, suggesting that the regulation of this chemokine is governed by a distinct mechanism. In fact, several studies have shown that different transcriptional pathways, i.e., other than NF-κB, are involved in MCP-1 production (72–74). In the context of lung epithelial cells, the IL-1β-induced MCP-1 expression in murine alveolar type II epithelial cells required multiple transcriptional factors in addition to NF-κB; namely, c-Jun N-terminal kinase (JNK), CCAAT/enhancer-binding proteins (C/EBPβ and C/EBPδ) and specificity protein 1 (Sp1) (75).
One of the pathways leading to NF-κB activation is the recognition of PAMPs by TLRs. Of interest, TLR-9 is one of the intracellular TLRs expressed by human alveolar epithelial cells (41) that has been considered in the context of mycobacterial infection due to its capacity to sense microbial DNA and mediate protective responses against infection (19, 21). Meeting expectations, A549 cells secreted IL-8 in response to the CpG-Hlp complex, a known mycobacterial TLR-9 ligand. Moreover, in agreement with previous reports (17, 21), the combination of rHlp with CpG almost doubled the levels of secreted IL-8 when compared to cells stimulated with CpG alone. One possible explanation for the synergistic effect of Hlp on CpG may be due to the ability of the protein to bind simultaneously to components of the extracellular matrix (14, 15, 45, 76) and to DNA (42, 43). The binding of Hlp to extracellular matrix components would promote the endocytosis of the CpG-Hlp complex, facilitating its subsequent recognition by the TLR-9 in the endosomal compartments. Moreover, histones are also able to induce DNA curvature, an effect that appears to significantly increase DNA binding by TLR-9 (77).
Based on this information, the next analysis centered on the potential involvement of TLR-9 activation in the M. leprae-induced immune activation in these cells. For this purpose, we employed E6446, a very selective antagonist for TLR-9 used in several previous reports (21, 78–82). The inclusion of TLR-4 and TLR-9 ligands as negative and positive controls, respectively, in our assays reinforced the specificity of E6446 towards TLR-9. A549 cells pre-treated with E6446 completely blocked the secretion of M. leprae-induced IL-8, elucidating that, perhaps, once internalized, bacterial DNA sensing by TLR-9 constitutes an important innate immune pathway becoming activated in infected respiratory epithelial cells. Moreover, by blocking TLR-9, the translocation of p65 to the nucleus and IL-8 production were inhibited, linking TLR-9 signaling with NF-κB activation and IL-8 induction in A549 cells stimulated with M. leprae.
Studies in a murine model of experimental malaria have reinforced the immunostimulatory properties of DNA-protein complexes (83, 84). The DNA-histone complex proved to be the main component in the plasmodium capable of activating dendritic cells and inducing the production of inflammatory mediators. The above-mentioned authors also demonstrated that histones facilitate the internalization of the DNA molecule and its subsequent recognition by TLR-9. These and several other studies have strengthened the idea that (naked) DNA per se might likely be an immunologically weak molecule, but its association with such DNA-binding proteins as histones, could transform it into a molecule with stronger immunostimulatory properties (17, 21, 85).
Previous studies have found the presence of DNA on the surface of M. tuberculosis (86, 87), possibly as a result of bacterial lysis or active secretion, as has already been seen in M. avium (88, 89). A prior study showed that M. avium cultivated in a “phagosome-mimicking” model secreted a high number of DNA-containing membrane vesicles (89). These same authors were also able to determine, by way of freeze-fracture transmission electron microscopy, the presence of these vesicles inside the macrophages as well as the presence of extracellular DNA in the matrix of M. avium biofilms (88). It is well established that the Hlp protein is present on the surface of M. leprae (12, 16, 25). So, it is reasonable to speculate that extracellular DNA anchored to Hlp constitute a major TLR-9 ligand triggering this immune activation pathway during mycobacterial infection. This hypothesis was reinforced when a mutant M. smegmatis strain for the hlp gene was employed. Pretreatment of the M. smegmatis wt strain with CpG increased the secretion of IL-8 by A549 cells, which did not occur with regard to the Δhlp strain. These assays made it possible to conclude that Hlp promotes the binding of DNA to the bacterial surface, leading to the subsequent activation of TLR-9 upon bacterial internalization.
Figure 6 summarizes our current model of M. leprae-airway epithelial cell interaction and the immune activation pathways triggered upon infection. DNA molecules, probably derived from the leakage of dead bacteria, bind to surface-exposed Hlp, allowing for the deposition and accumulation of DNA-Hlp complexes on the surface of both viable and dead bacilli. According to our Supplementary Data and previous findings (43), DNA binding to Hlp occurs via the prokaryotic DNA-binding motif located at the N-terminal half of the protein. Hlp mediates the adhesion and internalization of M. leprae to respiratory epithelial cells via its (Hlp) capacity to bind extracellular matrix proteoglycans such as heparan sulfate present on the cell surface. Once the bacilli reach the endosomal compartment, the DNA-Hlp complexes are recognized by the TLR-9 receptor, triggering a network of signals leading to NF-κB activation and the production of IL-8. M. leprae infection also induces MCP-1 and antimicrobial peptide production as well as the expression of MHC-II and co-stimulatory molecules like CD80. The signaling pathways triggered by M. leprae to induce these activation markers were not investigated in the present study, but bacterial recognition by other PRRs might play a role and should be explored in future studies. The M. leprae-induced immune activation of airway epithelial cells will mediate the recruitment of leukocytes to the infection site, contributing to the generation of a protective adaptive immune response.
Figure 6 Representative model of M. leprae-respiratory epithelial cell interaction with emphasis on the role of bacterial DNA-Hlp complex recognition in cellular immune activation. DNA molecules, probably derived from the leakage of dead bacteria, bind to surface-exposed Hlp, allowing the deposition and accumulation of DNA-Hlp complexes on the surface of viable and dead bacilli. Hlp mediates the adhesion and internalization of M. leprae to respiratory epithelial cells via its (Hlp) capacity to bind extracellular matrix proteoglycans. In the endosome, the DNA-Hlp complex interacts with the TLR-9, leading to activation and nuclear translocation of the NF-κB transcription factor with subsequent induction of IL-8. The hβD-2 is also induced, possibly via NF-κB (90–92) (dotted arrow). MCP-1 is also produced in response to M. leprae, however, in a NF-κB-independent manner. M. leprae also induces the expression of MHC-II and co-stimulatory molecules like CD80. This immune activation mediates the recruitment of leukocytes to the infection site, represented by dendritic cells (green), neutrophils (lilac), monocytes (blue) and T cells (red), contributing to the generation of a protective adaptive immune response.
Most individuals exposed to M. leprae are able to build a protective Th1-based immune response against the infection and, consequently, do not progress to active disease (6). Therefore, based on the data herein demonstrated it is reasonable to speculated that the ability of M. leprae to immune activate respiratory epithelial cells represents a very early event during infection that may be crucial to the development of this protective response. Indeed, mucosal vaccines are able to induce both local and systemic immunity against infectious agents (93–95). Of interest, the addition (or co-delivery) of potent adjuvants such as TLR-9 agonists has been described as efficient in potentiating a mucosal vaccination against mycobacterial infections (96).
In a scenario bereft of a leprosy vaccine, the findings described above may shed some much-needed light on creating potentially effective strategies toward achieving a viable vaccine in the near term. Moreover, since Hlp is a very conserved protein, we foresee its potential use when combined with DNA, both as a good immunogen as well as an adjuvant in immune interventions such as in intranasal vaccination against mycobacteria.
The original contributions presented in the study are included in the article/Supplementary Material. Further inquiries can be directed to the corresponding author.
The studies involving human participants were reviewed and approved by Pedro Ernesto University Hospital, the State University of Rio de Janeiro (CEP 2306/HUPE) and the Oswaldo Cruz Foundation (FIOCRUZ) Ethical Committee (CEP 483/08). The patients/participants provided their written informed consent to participate in this study.
AD, CMS, COS, and MP conceived and designed the experiments. AD, CMS, COS, NL, JS, AV, and PR performed the experiments. AD, CMS, COS, AC, MM, UL, MB-P, and MP analyzed the data. AD, CMS, and MP wrote the paper. All authors contributed to the article and approved the submitted version.
This work was supported by grants awarded to MCVP by the Conselho Nacional de Desenvolvimento Científico e Tecnológico (CNPq; 310155/2017-7), and the Fundação Carlos Chagas Filho de Amparo à Pesquisa do Estado do Rio de Janeiro (FAPERJ; 110.527/2014). AD was the recipient of a fellowship from Oswaldo Cruz Institute (IOC/FIOCRUZ/Brazil). The funders had no role in study design, data collection and analysis, decision to publish, or preparation of the manuscript.
The authors declare that the research was conducted in the absence of any commercial or financial relationships that could be construed as a potential conflict of interest.
All claims expressed in this article are solely those of the authors and do not necessarily represent those of their affiliated organizations, or those of the publisher, the editors and the reviewers. Any product that may be evaluated in this article, or claim that may be made by its manufacturer, is not guaranteed or endorsed by the publisher.
We thank the Eisai Co., Ltd. (Bunkyo-ku, Tokyo, Japan) and Dr. Ricardo T. Gazzinelli (from the Federal University of Minas Gerais and the Renê Rachou Institute FIOCRUZ) for providing the compound E6446-02. We are also grateful to the Rede de Plataformas Tecnológicas Fiocruz (Rpt08a-RJ) for support in the flow cytometry experiments and analyses, and Judy Grevan for editing the text.
The Supplementary Material for this article can be found online at: https://www.frontiersin.org/articles/10.3389/fimmu.2021.657449/full#supplementary-material
1. Scollard DM, Adams LB, Gillis TP, Krahenbuhl JL, Truman RW, Williams DL. The Continuing Challenges of Leprosy. Clin Microbiol Rev (2006) 19:338–81. doi: 10.1128/CMR.19.2.338-381.2006
2. WHO. Leprosy. WHO. Available at: http://www.who.int/lep/en/ (Accessed January 15, 2021).
3. Bratschi MW, Steinmann P, Wickenden A, Gillis TP. Current Knowledge on Mycobacterium Leprae Transmission: A Systematic Literature Review. Lepr Rev (2015) 86:142–55. doi: 10.47276/lr.86.2.142
4. Job CK, Jayakumar J, Kearney M, Gillis TP. Transmission of Leprosy: A Study of Skin and Nasal Secretions of Household Contacts of Leprosy Patients Using PCR. Am J Trop Med Hyg (2008) 78:518–21. doi: 10.4269/ajtmh.2008.78.518
5. Godal T, Negassi K. Subclinical Infection in Leprosy. Br Med J (1973) 3:557–9. doi: 10.1136/bmj.3.5880.557
6. Martins MVSB, Guimarães MM da S, Spencer JS, Hacker MAVB, Costa LS, Carvalho FM, et al. Pathogen-Specific Epitopes as Epidemiological Tools for Defining the Magnitude of Mycobacterium Leprae Transmission in Areas Endemic for Leprosy. PloS Negl Trop Dis (2012) 6:e1616. doi: 10.1371/journal.pntd.0001616
7. Whitsett JA, Alenghat T. Respiratory Epithelial Cells Orchestrate Pulmonary Innate Immunity. Nat Immunol (2015) 16:27–35. doi: 10.1038/ni.3045
8. Li Y, Wang Y, Liu X. The Role of Airway Epithelial Cells in Response to Mycobacteria Infection. Clin Dev Immunol (2012) 2012:791392. doi: 10.1155/2012/791392
9. Scordo JM, Knoell DL, Torrelles JB. Alveolar Epithelial Cells in Mycobacterium Tuberculosis Infection: Active Players or Innocent Bystanders? J Innate Immun (2016) 8:3–14. doi: 10.1159/000439275
10. Gupta N, Kumar R, Agrawal B. New Players in Immunity to Tuberculosis: The Host Microbiome, Lung Epithelium, and Innate Immune Cells. Front Immunol (2018) 9:709. doi: 10.3389/fimmu.2018.00709
11. Rees RJ, McDougall AC. Airborne Infection With Mycobacterium Leprae in Mice. J Med Microbiol (1977) 10:63–8. doi: 10.1099/00222615-10-1-63
12. Silva CAM, Danelishvili L, McNamara M, Berredo-Pinho M, Bildfell R, Biet F, et al. Interaction of Mycobacterium Leprae With Human Airway Epithelial Cells: Adherence, Entry, Survival, and Identification of Potential Adhesins by Surface Proteome Analysis. Infect Immun (2013) 81:2645–59. doi: 10.1128/IAI.00147-13
13. Pethe K, Alonso S, Biet F, Delogu G, Brennan MJ, Locht C, et al. The Heparin-Binding Haemagglutinin of M. Tuberculosis Is Required for Extrapulmonary Dissemination. Nature (2001) 412:190–4. doi: 10.1038/35084083
14. Aoki K, Matsumoto S, Hirayama Y, Wada T, Ozeki Y, Niki M, et al. Extracellular Mycobacterial DNA-Binding Protein 1 Participates in Mycobacterium-Lung Epithelial Cell Interaction Through Hyaluronic Acid. J Biol Chem (2004) 279:39798–806. doi: 10.1074/jbc.M402677200
15. Soares de Lima C, Zulianello L, Marques MA de M, Kim H, Portugal MI, Antunes SL, et al. Mapping the Laminin-Binding and Adhesive Domain of the Cell Surface-Associated Hlp/LBP Protein From Mycobacterium Leprae. Microbes Infect (2005) 7:1097–109. doi: 10.1016/j.micinf.2005.02.013
16. Shimoji Y, Ng V, Matsumura K, Fischetti VA, Rambukkana A. A 21-kDa Surface Protein of Mycobacterium Leprae Binds Peripheral Nerve Laminin-2 and Mediates Schwann Cell Invasion. Proc Natl Acad Sci USA (1999) 96:9857–62. doi: 10.1073/pnas.96.17.9857
17. Matsumoto S, Matsumoto M, Umemori K, Ozeki Y, Furugen M, Tatsuo T, et al. DNA Augments Antigenicity of Mycobacterial DNA-Binding Protein 1 and Confers Protection Against Mycobacterium Tuberculosis Infection in Mice. J Immunol (2005) 175:441–9. doi: 10.4049/jimmunol.175.1.441
18. Lamphier MS, Sirois CM, Verma A, Golenbock DT, Latz E. TLR9 and the Recognition of Self and Non-Self Nucleic Acids. Ann NY Acad Sci (2006) 1082:31–43. doi: 10.1196/annals.1348.005
19. Bafica A, Scanga CA, Feng CG, Leifer C, Cheever A, Sher A. TLR9 Regulates Th1 Responses and Cooperates With TLR2 in Mediating Optimal Resistance to Mycobacterium Tuberculosis. J Exp Med (2005) 202:1715–24. doi: 10.1084/jem.20051782
20. Pompei L, Jang S, Zamlynny B, Ravikumar S, McBride A, Hickman SP, et al. Disparity in IL-12 Release in Dendritic Cells and Macrophages in Response to Mycobacterium Tuberculosis Is Due to Use of Distinct TLRs. J Immunol (2007) 178:5192–9. doi: 10.4049/jimmunol.178.8.5192
21. Dias AA, Silva CO, Santos JPS, Batista-Silva LR, Acosta CCD, Fontes ANB, et al. DNA Sensing via TLR-9 Constitutes a Major Innate Immunity Pathway Activated During Erythema Nodosum Leprosum. J Immunol (2016) 197:1905–13. doi: 10.4049/jimmunol.1600042
22. Abbas S, Abu-Amer Y. Dominant-Negative IkappaB Facilitates Apoptosis of Osteoclasts by Tumor Necrosis Factor-Alpha. J Biol Chem (2003) 278:20077–82. doi: 10.1074/jbc.M208619200
23. Livak KJ, Schmittgen TD. Analysis of Relative Gene Expression Data Using Real-Time Quantitative PCR and the 2(-Delta Delta C(T)) Method. Methods (2001) 25:402–8. doi: 10.1006/meth.2001.1262
24. Santos AR, De Miranda AB, Sarno EN, Suffys PN, Degrave WM. Use of PCR-Mediated Amplification of Mycobacterium Leprae DNA in Different Types of Clinical Samples for the Diagnosis of Leprosy. J Med Microbiol (1993) 39:298–304. doi: 10.1099/00222615-39-4-298
25. de Melo Marques MA, Mahapatra S, Nandan D, Dick T, Sarno EN, Brennan PJ, et al. Bacterial and Host-Derived Cationic Proteins Bind Alpha2-Laminins and Enhance Mycobacterium Leprae Attachment to Human Schwann Cells. Microbes Infect (2000) 2:1407–17. doi: 10.1016/s1286-4579(00)01294-6
26. Hughes CE. Nibbs RJB. A Guide to Chemokines and Their Receptors. FEBS J (2018) 285:2944–71. doi: 10.1111/febs.14466
27. Rivas-Santiago B, Schwander SK, Sarabia C, Diamond G, Klein-Patel ME, Hernandez-Pando R, et al. Human {Beta}-Defensin 2 Is Expressed and Associated With Mycobacterium Tuberculosis During Infection of Human Alveolar Epithelial Cells. Infect Immun (2005) 73:4505–11. doi: 10.1128/IAI.73.8.4505-4511.2005
28. Méndez-Samperio P, Miranda E, Trejo A. Mycobacterium Bovis Bacillus Calmette-Guérin (BCG) Stimulates Human Beta-Defensin-2 Gene Transcription in Human Epithelial Cells. Cell Immunol (2006) 239:61–6. doi: 10.1016/j.cellimm.2006.04.001
29. Rodriguez-Carlos A, Valdez-Miramontes C, Marin-Luevano P, González-Curiel I, Enciso-Moreno JA, Rivas-Santiago B. Metformin Promotes Mycobacterium Tuberculosis Killing and Increases the Production of Human β-Defensins in Lung Epithelial Cells and Macrophages. Microbes Infect (2020) 22:111–8. doi: 10.1016/j.micinf.2019.10.002
30. Shiozawa A, Kajiwara C, Ishii Y, Tateda K. N-Acetyl-Cysteine Mediates Protection Against Mycobacterium Avium Through Induction of Human β-Defensin-2 in a Mouse Lung Infection Model. Microbes Infect (2020) 22(10):567–75. doi: 10.1016/j.micinf.2020.08.003
31. Cunningham AC, Kirby JA. Regulation and Function of Adhesion Molecule Expression by Human Alveolar Epithelial Cells. Immunology (1995) 86:279–86.
32. Salik E, Tyorkin M, Mohan S, George I, Becker K, Oei E, et al. Antigen Trafficking and Accessory Cell Function in Respiratory Epithelial Cells. Am J Respir Cell Mol Biol (1999) 21:365–79. doi: 10.1165/ajrcmb.21.3.3529
33. Lo B, Hansen S, Evans K, Heath JK, Wright JR. Alveolar Epithelial Type II Cells Induce T Cell Tolerance to Specific Antigen. J Immunol (2008) 180:881–8. doi: 10.4049/jimmunol.180.2.881
34. Corbière V, Dirix V, Norrenberg S, Cappello M, Remmelink M, Mascart F. Phenotypic Characteristics of Human Type II Alveolar Epithelial Cells Suitable for Antigen Presentation to T Lymphocytes. Respir Res (2011) 12:15. doi: 10.1186/1465-9921-12-15
35. Li R, Song Y, Chen W. Enhancing Radiosensitivity of Human Pulmonary Adenocarcinoma Cell Line A549 by CpG Odn1826. Cancer Biother Radiopharm (2011) 26:69–76. doi: 10.1089/cbr.2010.0849
36. Shen H, Liu C, Shao P, Yi L, Wang Y, Mills Ko E, et al. Enhanced Phenotypic Alterations of Alveolar Type II Cells in Response to Aflatoxin G1 -Induced Lung Inflammation. J Cell Physiol (2015) 230:1199–211. doi: 10.1002/jcp.24852
37. Napetschnig J, Wu H. Molecular Basis of NF-κb Signaling. Annu Rev Biophys (2013) 42:443–68. doi: 10.1146/annurev-biophys-083012-130338
38. Kobori M, Yang Z, Gong D, Heissmeyer V, Zhu H, Jung Y-K, et al. Wedelolactone Suppresses LPS-Induced Caspase-11 Expression by Directly Inhibiting the IKK Complex. Cell Death Differ (2004) 11:123–30. doi: 10.1038/sj.cdd.4401325
39. Solt LA, May MJ. The IkappaB Kinase Complex: Master Regulator of NF-kappaB Signaling. Immunol Res (2008) 42:3–18. doi: 10.1007/s12026-008-8025-1
40. Pierce JW, Schoenleber R, Jesmok G, Best J, Moore SA, Collins T, et al. Novel Inhibitors of Cytokine-Induced IkappaBalpha Phosphorylation and Endothelial Cell Adhesion Molecule Expression Show Anti-Inflammatory Effects In Vivo. J Biol Chem (1997) 272:21096–103. doi: 10.1074/jbc.272.34.21096
41. Schneberger D, Caldwell S, Kanthan R, Singh B. Expression of Toll-Like Receptor 9 in Mouse and Human Lungs. J Anat (2013) 222:495–503. doi: 10.1111/joa.12039
42. Matsumoto S, Yukitake H, Furugen M, Matsuo T, Mineta T, Yamada T. Identification of a Novel DNA-Binding Protein From Mycobacterium Bovis Bacillus Calmette-Guérin. Microbiol Immunol (1999) 43:1027–36. doi: 10.1111/j.1348-0421.1999.tb01232.x
43. Furugen M, Matsumoto S, Matsuo T, Matsumoto M, Yamada T. Identification of the Mycobacterial DNA-Binding Protein 1 Region Which Suppresses Transcription In Vitro. Microb Pathog (2001) 30:129–38. doi: 10.1006/mpat.2000.0416
44. Portugal MI, Todeschini AR, de Lima CS, Silva CAM, Mohana-Borges R, Ottenhoff THM, et al. Characterization of Two Heparan Sulphate-Binding Sites in the Mycobacterial Adhesin Hlp. BMC Microbiol (2008) 8:75. doi: 10.1186/1471-2180-8-75
45. Lefrançois LH, Pujol C, Bodier CC, Teixeira-Gomez AP, Drobecq H, Rosso M-L, et al. Characterization of the Mycobacterium Avium Subsp. Paratuberculosis Laminin-Binding/Histone-Like Protein (Lbp/Hlp) Which Reacts With Sera From Patients With Crohn’s Disease. Microbes Infect (2011) 13:585–94. doi: 10.1016/j.micinf.2011.02.002
46. Mattos KA, Oliveira VGC, D’Avila H, Rodrigues LS, Pinheiro RO, Sarno EN, et al. TLR6-Driven Lipid Droplets in Mycobacterium Leprae-Infected Schwann Cells: Immunoinflammatory Platforms Associated With Bacterial Persistence. J Immunol (2011) 187:2548–58. doi: 10.4049/jimmunol.1101344
47. Díaz Acosta CC, Dias AA, Rosa TLSA, Batista-Silva LR, Rosa PS, Toledo-Pinto TG, et al. PGL I Expression in Live Bacteria Allows Activation of a CD206/Pparγ Cross-Talk That may Contribute to Successful Mycobacterium Leprae Colonization of Peripheral Nerves. PloS Pathog (2018) 14:e1007151. doi: 10.1371/journal.ppat.1007151
48. Silva BJ de A, Barbosa MG de M, Andrade PR, Ferreira H, Nery JA da C, Côrte-Real S, et al. Autophagy Is an Innate Mechanism Associated With Leprosy Polarization. PloS Pathog (2017) 13:e1006103. doi: 10.1371/journal.ppat.1006103
49. Taub DD, Proost P, Murphy WJ, Anver M, Longo DL, van Damme J, et al. Monocyte Chemotactic Protein-1 (MCP-1), -2, and -3 Are Chemotactic for Human T Lymphocytes. J Clin Invest (1995) 95:1370–6. doi: 10.1172/JCI117788
50. Lin Y, Zhang M, Barnes PF. Chemokine Production by a Human Alveolar Epithelial Cell Line in Response to Mycobacterium Tuberculosis. Infect Immun (1998) 66:1121–6. doi: 10.1128/IAI.66.3.1121-1126.1998
51. Wickremasinghe MI, Thomas LH, O’Kane CM, Uddin J, Friedland JS. Transcriptional Mechanisms Regulating Alveolar Epithelial Cell-Specific CCL5 Secretion in Pulmonary Tuberculosis. J Biol Chem (2004) 279:27199–210. doi: 10.1074/jbc.M403107200
52. Boggaram V, Gottipati KR, Wang X, Samten B. Early Secreted Antigenic Target of 6 kDa (ESAT-6) Protein of Mycobacterium Tuberculosis Induces Interleukin-8 (IL-8) Expression in Lung Epithelial Cells via Protein Kinase Signaling and Reactive Oxygen Species. J Biol Chem (2013) 288:25500–11. doi: 10.1074/jbc.M112.448217
53. Sequeira PC, Senaratne RH, Riley LW. Inhibition of Toll-Like Receptor 2 (TLR-2)-Mediated Response in Human Alveolar Epithelial Cells by Mycolic Acids and Mycobacterium Tuberculosis Mce1 Operon Mutant. Pathog Dis (2014) 70:132–40. doi: 10.1111/2049-632X.12110
54. Mvubu NE, Pillay B, McKinnon LR, Pillay M. Mycobacterium Tuberculosis Strains Induce Strain-Specific Cytokine and Chemokine Response in Pulmonary Epithelial Cells. Cytokine (2018) 104:53–64. doi: 10.1016/j.cyto.2017.09.027
55. Hadifar S, Behrouzi A, Fateh A, Khatami S, Rahimi Jamnani F, Siadat SD, et al. Comparative Study of Interruption of Signaling Pathways in Lung Epithelial Cell by Two Different Mycobacterium Tuberculosis Lineages. J Cell Physiol (2019) 234:4739–53. doi: 10.1002/jcp.27271
56. Hasan Z, Zaidi I, Jamil B, Khan MA, Kanji A, Hussain R. Elevated Ex Vivo Monocyte Chemotactic Protein-1 (CCL2) in Pulmonary as Compared With Extra-Pulmonary Tuberculosis. BMC Immunol (2005) 6:14. doi: 10.1186/1471-2172-6-14
57. Schreiber O, Steinwede K, Ding N, Srivastava M, Maus R, Länger F, et al. Mice That Overexpress CC Chemokine Ligand 2 in Their Lungs Show Increased Protective Immunity to Infection With Mycobacterium Bovis Bacille Calmette-Guérin. J Infect Dis (2008) 198:1044–54. doi: 10.1086/591501
58. Kurashima K, Mukaida N, Fujimura M, Yasui M, Nakazumi Y, Matsuda T, et al. Elevated Chemokine Levels in Bronchoalveolar Lavage Fluid of Tuberculosis Patients. Am J Respir Crit Care Med (1997) 155:1474–7. doi: 10.1164/ajrccm.155.4.9105097
59. Sadek MI, Sada E, Toossi Z, Schwander SK, Rich EA. Chemokines Induced by Infection of Mononuclear Phagocytes With Mycobacteria and Present in Lung Alveoli During Active Pulmonary Tuberculosis. Am J Respir Cell Mol Biol (1998) 19:513–21. doi: 10.1165/ajrcmb.19.3.2815
60. Xu L, Kelvin DJ, Ye GQ, Taub DD, Ben-Baruch A, Oppenheim JJ, et al. Modulation of IL-8 Receptor Expression on Purified Human T Lymphocytes Is Associated With Changed Chemotactic Responses to IL-8. J Leukoc Biol (1995) 57:335–42. doi: 10.1002/jlb.57.2.335
61. Krupa A, Fol M, Dziadek BR, Kepka E, Wojciechowska D, Brzostek A, et al. Binding of CXCL8/IL-8 to Mycobacterium Tuberculosis Modulates the Innate Immune Response. Mediators Inflammation (2015) 2015:124762. doi: 10.1155/2015/124762
62. Bahar AA, Ren D. Antimicrobial Peptides. Pharmaceuticals (Basel) (2013) 6:1543–75. doi: 10.3390/ph6121543
63. Ashitani J, Mukae H, Hiratsuka T, Nakazato M, Kumamoto K, Matsukura S. Plasma and BAL Fluid Concentrations of Antimicrobial Peptides in Patients With Mycobacterium Avium-Intracellulare Infection. Chest (2001) 119:1131–7. doi: 10.1378/chest.119.4.1131
64. Dürr M, Peschel A. Chemokines Meet Defensins: The Merging Concepts of Chemoattractants and Antimicrobial Peptides in Host Defense. Infect Immun (2002) 70:6515–7. doi: 10.1128/iai.70.12.6515-6517.2002
65. Dong H, Lv Y, Zhao D, Barrow P, Zhou X. Defensins: The Case for Their Use Against Mycobacterial Infections. J Immunol Res (2016) 2016:7515687. doi: 10.1155/2016/7515687
66. Yang D, Chertov O, Bykovskaia SN, Chen Q, Buffo MJ, Shogan J, et al. Beta-Defensins: Linking Innate and Adaptive Immunity Through Dendritic and T Cell CCR6. Science (1999) 286:525–8. doi: 10.1126/science.286.5439.525
67. Yang D, Biragyn A, Kwak LW, Oppenheim JJ. Mammalian Defensins in Immunity: More Than Just Microbicidal. Trends Immunol (2002) 23:291–6. doi: 10.1016/s1471-4906(02)02246-9
68. Niyonsaba F, Iwabuchi K, Matsuda H, Ogawa H, Nagaoka I. Epithelial Cell-Derived Human Beta-Defensin-2 Acts as a Chemotaxin for Mast Cells Through a Pertussis Toxin-Sensitive and Phospholipase C-Dependent Pathway. Int Immunol (2002) 14:421–6. doi: 10.1093/intimm/14.4.421
69. Rivas-Santiago B, Cervantes-Villagrana A, Sada E, Hernández-Pando R. Expression of Beta Defensin 2 in Experimental Pulmonary Tuberculosis: Tentative Approach for Vaccine Development. Arch Med Res (2012) 43:324–8. doi: 10.1016/j.arcmed.2012.06.005
70. Pereira RMS, Calegari-Silva TC, Hernandez MO, Saliba AM, Redner P, Pessolani MCV, et al. Mycobacterium Leprae Induces NF-kappaB-Dependent Transcription Repression in Human Schwann Cells. Biochem Biophys Res Commun (2005) 335:20–6. doi: 10.1016/j.bbrc.2005.07.061
71. Sinsimer D, Fallows D, Peixoto B, Krahenbuhl J, Kaplan G, Manca C. Mycobacterium Leprae Actively Modulates the Cytokine Response in Naive Human Monocytes. Infect Immun (2010) 78:293–300. doi: 10.1128/IAI.00816-09
72. Caselli E, Benedetti S, Grigolato J, Caruso A, Di Luca D. Activating Transcription Factor 4 (ATF4) Is Upregulated by Human Herpesvirus 8 Infection, Increases Virus Replication and Promotes Proangiogenic Properties. Arch Virol (2012) 157:63–74. doi: 10.1007/s00705-011-1144-3
73. Cho SO, Lim JW, Kim H. Red Ginseng Extract Inhibits the Expression of MCP-1 and iNOS in Helicobacter Pylori-Infected Gastric Epithelial Cells by Suppressing the Activation of NADPH Oxidase and Jak2/Stat3. J Ethnopharmacol (2013) 150:761–4. doi: 10.1016/j.jep.2013.09.013
74. Kuroda M, Nishiguchi M, Ugawa N, Ishikawa E, Kawabata Y, Okamoto S, et al. Interferon Regulatory Factor 7 Mediates Obesity-Associated MCP-1 Transcription. PloS One (2020) 15:e0233390. doi: 10.1371/journal.pone.0233390
75. Yan C, Li B, Liu X, Deng C, Cai R, Shen Y, et al. Involvement of Multiple Transcription Factors in Regulation of IL-β-Induced MCP-1 Expression in Alveolar Type II Epithelial Cells. Mol Immunol (2019) 111:95–105. doi: 10.1016/j.molimm.2019.04.013
76. Dias AA, Raze D, de Lima CS, Marques MA de M, Drobecq H, Debrie A-S, et al. Mycobacterial Laminin-Binding Histone-Like Protein Mediates Collagen-Dependent Cytoadherence. Mem Inst Oswaldo Cruz (2012) 107(Suppl 1):174–82. doi: 10.1590/S0074-02762012000900025
77. Li Y, Berke IC, Modis Y. DNA Binding to Proteolytically Activated TLR9 Is Sequence-Independent and Enhanced by DNA Curvature. EMBO J (2012) 31:919–31. doi: 10.1038/emboj.2011.441
78. Franklin BS, Ishizaka ST, Lamphier M, Gusovsky F, Hansen H, Rose J, et al. Therapeutical Targeting of Nucleic Acid-Sensing Toll-Like Receptors Prevents Experimental Cerebral Malaria. Proc Natl Acad Sci USA (2011) 108:3689–94. doi: 10.1073/pnas.1015406108
79. Lamphier M, Zheng W, Latz E, Spyvee M, Hansen H, Rose J, et al. Novel Small Molecule Inhibitors of TLR7 and TLR9: Mechanism of Action and Efficacy In Vivo. Mol Pharmacol (2014) 85:429–40. doi: 10.1124/mol.113.089821
80. Ueda H, Yamaguchi O, Taneike M, Akazawa Y, Wada-Kobayashi H, Sugihara R, et al. Administration of a TLR9 Inhibitor Attenuates the Development and Progression of Heart Failure in Mice. JACC Basic Transl Sci (2019) 4:348–63. doi: 10.1016/j.jacbts.2019.01.002
81. Yoshida K, Abe K, Ishikawa M, Saku K, Shinoda-Sakamoto M, Ishikawa T, et al. Inhibition of TLR9-NF-κb-Mediated Sterile Inflammation Improves Pressure Overload-Induced Right Ventricular Dysfunction in Rats. Cardiovasc Res (2019) 115:658–68. doi: 10.1093/cvr/cvy209
82. Ishikawa T, Abe K, Takana-Ishikawa M, Yoshida K, Watanabe T, Imakiire S, et al. Chronic Inhibition of Toll-Like Receptor 9 Ameliorates Pulmonary Hypertension in Rats. J Am Heart Assoc (2021) 10:e019247. doi: 10.1161/JAHA.120.019247
83. Wu X, Gowda NM, Kumar S, Gowda DC. Protein-DNA Complex Is the Exclusive Malaria Parasite Component That Activates Dendritic Cells and Triggers Innate Immune Responses. J Immunol (2010) 184:4338–48. doi: 10.4049/jimmunol.0903824
84. Gowda NM, Wu X, Gowda DC. The Nucleosome (Histone-DNA Complex) is the TLR9-Specific Immunostimulatory Component of Plasmodium Falciparum That Activates DCs. PloS One (2011) 6:e20398. doi: 10.1371/journal.pone.0020398
85. Morizane S, Yamasaki K, Mühleisen B, Kotol PF, Murakami M, Aoyama Y, et al. Cathelicidin Antimicrobial Peptide LL-37 in Psoriasis Enables Keratinocyte Reactivity Against TLR9 Ligands. J Invest Dermatol (2012) 132:135–43. doi: 10.1038/jid.2011.259
86. Manzanillo PS, Shiloh MU, Portnoy DA, Cox JS. Mycobacterium Tuberculosis Activates the DNA-Dependent Cytosolic Surveillance Pathway Within Macrophages. Cell Host Microbe (2012) 11:469–80. doi: 10.1016/j.chom.2012.03.007
87. Watson RO, Manzanillo PS, Cox JS. Extracellular M. Tuberculosis DNA Targets Bacteria for Autophagy by Activating the Host DNA-Sensing Pathway. Cell (2012) 150:803–15. doi: 10.1016/j.cell.2012.06.040
88. Rose SJ, Babrak LM, Bermudez LE. Mycobacterium Avium Possesses Extracellular DNA That Contributes to Biofilm Formation, Structural Integrity, and Tolerance to Antibiotics. PloS One (2015) 10:e0128772. doi: 10.1371/journal.pone.0128772
89. Chiplunkar SS, Silva CA, Bermudez LE, Danelishvili L. Characterization of Membrane Vesicles Released by Mycobacterium Avium in Response to Environment Mimicking the Macrophage Phagosome. Future Microbiol (2019) 14:293–313. doi: 10.2217/fmb-2018-0249
90. Tsutsumi-Ishii Y, Nagaoka I. NF-Kappa B-Mediated Transcriptional Regulation of Human Beta-Defensin-2 Gene Following Lipopolysaccharide Stimulation. J Leukoc Biol (2002) 71:154–62. doi: 10.1189/jlb.71.1.154
91. Kao C-Y, Kim C, Huang F, Wu R. Requirements for Two Proximal NF-kappaB Binding Sites and IkappaB-Zeta in IL-17A-Induced Human Beta-Defensin 2 Expression by Conducting Airway Epithelium. J Biol Chem (2008) 283:15309–18. doi: 10.1074/jbc.M708289200
92. Steubesand N, Kiehne K, Brunke G, Pahl R, Reiss K, Herzig K-H, et al. The Expression of the Beta-Defensins hBD-2 and hBD-3 Is Differentially Regulated by NF-kappaB and MAPK/AP-1 Pathways in an In Vitro Model of Candida Esophagitis. BMC Immunol (2009) 10:36. doi: 10.1186/1471-2172-10-36
93. McGhee JR, Mestecky J, Dertzbaugh MT, Eldridge JH, Hirasawa M, Kiyono H. The Mucosal Immune System: From Fundamental Concepts to Vaccine Development. Vaccine (1992) 10:75–88. doi: 10.1016/0264-410x(92)90021-b
94. Hjelm BE, Kilbourne J, Herbst-Kralovetz MM. TLR7 and 9 Agonists Are Highly Effective Mucosal Adjuvants for Norovirus Virus-Like Particle Vaccines. Hum Vaccin Immunother (2014) 10:410–6. doi: 10.4161/hv.27147
95. Miquel-Clopés A, Bentley EG, Stewart JP, Carding SR. Mucosal Vaccines and Technology. Clin Exp Immunol (2019) 196:205–14. doi: 10.1111/cei.13285
96. Troy A, Esparza-Gonzalez SC, Bartek A, Creissen E, Izzo L, Izzo AA. Pulmonary Mucosal Immunity Mediated Through CpG Provides Adequate Protection Against Pulmonary Mycobacterium Tuberculosis Infection in the Mouse Model. A Role for Type I Interferon. Tuberculosis (Edinb) (2020) 123:101949. doi: 10.1016/j.tube.2020.101949
Keywords: Mycobacterium leprae, Toll-like receptor 9 (TLR-9), extracellular DNA, histone-like protein (Hlp), respiratory epithelial cells, innate immune response, chemokines
Citation: Dias AA, Silva CAM, Silva CO, Linhares NRC, Santos JPS, Vivarini AC, Marques MÂM, Rosa PS, Lopes UG, Berrêdo-Pinho M and Pessolani MCV (2021) TLR-9 Plays a Role in Mycobacterium leprae-Induced Innate Immune Activation of A549 Alveolar Epithelial Cells. Front. Immunol. 12:657449. doi: 10.3389/fimmu.2021.657449
Received: 22 January 2021; Accepted: 27 July 2021;
Published: 12 August 2021.
Edited by:
Xiao-Lian Zhang, Wuhan University, ChinaReviewed by:
Tomoyuki Fujisawa, Hamamatsu University School of Medicine, JapanCopyright © 2021 Dias, Silva, Silva, Linhares, Santos, Vivarini, Marques, Rosa, Lopes, Berrêdo-Pinho and Pessolani. This is an open-access article distributed under the terms of the Creative Commons Attribution License (CC BY). The use, distribution or reproduction in other forums is permitted, provided the original author(s) and the copyright owner(s) are credited and that the original publication in this journal is cited, in accordance with accepted academic practice. No use, distribution or reproduction is permitted which does not comply with these terms.
*Correspondence: Maria Cristina Vidal Pessolani, Y3Blc3NvbGFAaW9jLmZpb2NydXouYnI=
†These authors share first authorship
Disclaimer: All claims expressed in this article are solely those of the authors and do not necessarily represent those of their affiliated organizations, or those of the publisher, the editors and the reviewers. Any product that may be evaluated in this article or claim that may be made by its manufacturer is not guaranteed or endorsed by the publisher.
Research integrity at Frontiers
Learn more about the work of our research integrity team to safeguard the quality of each article we publish.