- 1Department of Pathology, Case Western Reserve University, Cleveland, OH, United States
- 2Division of Hematology/Oncology, Department of Medicine, Case Western Reserve University, Cleveland, OH, United States
- 3The Case Comprehensive Cancer Center, Case Western Reserve University School of Medicine, Cleveland, OH, United States
Natural Killer (NK) cells are cytotoxic lymphocytes critical to the innate immune system. We found that germline deficiency of NF-κB c-Rel results in a marked decrease in cytotoxic function of NK cells, both in vitro and in vivo, with no significant differences in the stages of NK cell development. We found that c-Rel binds to the promoters of perforin and granzyme B, two key proteins required for NK cytotoxicity, and controls their expression. We generated a NK cell specific c-Rel conditional knockout to study NK cell intrinsic role of c- Rel and found that both global and conditional c-Rel deficiency leads to decreased perforin and granzyme B expression and thereby cytotoxic function. We also confirmed the role of c-Rel in perforin and granzyme B expression in human NK cells. c-Rel reconstitution rescued perforin and granzyme B expressions in c-Rel deficient NK cells and restored their cytotoxic function. Our results show a previously unknown role of c-Rel in transcriptional regulation of perforin and granzyme B expressions and control of NK cell cytotoxic function.
Introduction
Natural Killer (NK) cells are innate immune cells with anti-tumor and anti-viral activity (1, 2). These cells express a wide range of activating and inhibitory receptors that upon engagement by cognate ligands on target tumor cells regulate their anti-tumor activity (3, 4). Upon activation, NK cells kill their target cells in a multistep-wise fashion which involves synapse formation between NK cells and target cells, expression of FAS ligand (Fas- L), and secretion of pro-inflammatory cytokines and cytotoxic granules that induce target cell death (5, 6). The granule exocytosis pathway is the primary mechanism used by NK cells to kill tumor and virus infected cells (7, 8). Cytotoxic granule-mediated killing by NK cells largely depends on two proteins, perforin (prf1) and granzyme B (gzmb) (9–12). Perforin is a protein produced by both cytotoxic T cells and NK cells that form holes in the target cell membrane where granzymes enter to destroy the target cell (13, 14). Granzymes are a family of serine proteases with gzmb being the most studied member of this family (15–17). Under pathological conditions such as in cancer, prf1 and gzmb are reported to be downregulated in NK cells compromising its cytotoxic function leading to NK cell exhaustion (18–21). Thus, it is imperative that we identify key factors that regulate cytotoxic function in NK cells. Previously, we reported that inhibition of GSK3 signaling led to increased NK cell cytotoxicity via NF-κB activation (22).
The Nuclear Factor-κB family of transcription factors consist of five subunits: p50, p52, RelB, p65, and c-Rel. Of these, c-Rel is predominantly expressed in the cells of myeloid and lymphoid lineages (23). c-Rel global knockout (c-Rel-/-) mice exhibit comparable morphology and behavior to that of wild type (WT) mice, however c-Rel-/- mice have profound immune system defects (24–26). One such defect is in the thymic development of Foxp3+ regulatory T-cells (27, 28). In addition, B cells from c-Rel-/- mice exhibit proliferation and immune-specific functional defects (29). Specifically, c-Rel-deficiency impairs B cells ability to produce antibodies and T-cells ability to produce the cytokines, IL-2, IL-3, GM-CSF, TNF-α, and IL-6 (26, 30). It was recently shown, that B and T lymphocytes from a patient with homozygous mutation that eradicate c-Rel expression exhibit impaired T and B cell activation (31). However, the role of c-Rel in NK cells remains poorly defined.
To address this, we used three different models including germline c-Rel knockout mice (c-Rel-/-), NK cell specific c-Rel conditional knockout mice (c-Relfl/flNcr1Cre) and c-Rel shRNA transduced primary human NK cells. We show that c-Rel deficiency impairs NK cell anti-tumor activity in vitro and in vivo suggesting c-Rel as a key regulator of NK cell anti-tumor function. Finally, we show that c-Rel regulates prf1 and gzmb expression by directly binding to their promoters and c-Rel reconstitution reverses functional defect in c-Rel-/- NK cells by restoring prf1 and gzmb expression. This study reveals a new role for c-Rel in NK cell-mediated anti-tumor immunity.
Methods
Mice
Mice were bred at the Case Western Reserve University in accordance with the guidelines of the Institutional Animal Care Use Committee. The following strains (C57BL/6 genetic background) were used in this study: c-Relfl/fl; (Purchased from The Jackson Laboratory), Ncr1iCre purchased from Dr. Sexl (32) (Institute of Pharmacology and Toxicology, Vienna, Austria), c-Rel germline knockout (c-Rel-/-) a generous gift from Dr. Ramakrishnan (Case Western Reserve University), and c-Relfl/flNcr1iCre/WT here called c-Relfl/flNcr1Cre were generated by backcrossing c-Relfl/fl to Ncr1cre at Case Western Reserve University. NOD SCID IL-2r Gamma (NSG) mice were purchased from Case Western Reserve University Athymic Animal Core. Experiments were conducted using age and gender-matched mice in accordance with approved institutional protocols.
Cell Isolation and Stimulation
Bone marrow, spleen, blood, lymph nodes, and liver were harvested, and single cell suspensions were generated by pressing through sterile 40 uM nylon filter. RBCs were lysed and cells were washed in PBS containing 5% FBS (Sigma). Cells were stained for Flow cytometry analysis. For cell sorting experiments, bone marrow and splenocytes were surface stained with anti-NKp46, anti-CD3 and NKp46+CD3- cells were sorted at the Case Western Reserve University Flow cytometry core using (BD Biosciences Aria) (purity>99%). For in vitro cytokine stimulations, MojoSort (BioLegends) purified NK cells (purity>80%) were plated at 1x106 per mL and added either media alone, rmIL-15 (100 ng/mL), or rmIL-2 (1000 U/mL). Cells were harvested by pipetting with cold PBS after the indicated time and used for western blot and cytotoxicity assays.
Flow Cytometry and Cell Sorting
Cell surface staining of single-cell suspensions was performed using the following fluorophore-conjugated antibodies: c-Rel (sc-71) and c-Rel (B-6) (purchased from Santa Cruz Biotechnology); CD3 (145-2C11), NK1.1 (PK136), IgG (MOCPC-21), IgG (HP6017), CD27 (3A10), CD11b (M1/70), CD43 (1B11), CD49b (DX5), NKG2D (CX5), Ly49D (4E5), DNAM-1 (10E5), Ly49 C-H (CF1H), NKG2A (16A11), CD244 (M2B4), CD16 (93), KLRG1 (2F1), Ly49A (A1), LIR/PIR (6C1), fasL (MFL3),
NKp46 (29A1.4), CD3 (H1T3A), CD56 (51H11), CD4 (A161A1), CD8 (SK1) (purchased from BioLegend).
Murine NK cells were defined as CD3-NK1.1+ cells. All data analysis was performed on viable cells by gating based on forward and side scatter. Flow cytometry was performed on Accuri 6C. Data were analyzed in Accuri 6C software analysis.
In Vitro Cytotoxicity Assays
Calcein AM Assay
NK cells were isolated from WT and c-Rel-/- mice, or from c-Relfl/fl and c-Relfl/flNcr1Cre using MojoSort Magnetic Cell Separation (purchased from BioLegend). For anti-tumor cytotoxicity, 2-3x106 NK cells were stimulated with the indicated cytokines or media alone for 24 hours prior to co-culture with tumor cells. The target cells were either the acute lymphoblastic leukemia cell line (8093) a kind gift from Nora Heisterkamp (City of Hope Comprehensive Cancer Center, Duarte, CA) or the melanoma cell line (B16F10) a kind gift from Dr. Bedogni (University of Miami, FL). Target cells were suspended in medium containing 5% FBS at a final concentration of 1x106 cells/mL and incubated with 5uM calcein-AM (Life Technologies, Grand Island, NY, USA) for 1 hour at 37°C with occasional shaking. After 3 washes with complete medium, 10,000 (B16F10) or 25,000 (8093) cells were plated in a 96 well plate. The number of NK cells was adjusted to achieve the different effector to target ratios. Cells were centrifuged and incubated at 37°C for four hours, after which the plate was centrifuged and 70-100 µl of each supernatant was harvested and transferred into 96-well plates.
Spontaneous (only target cells in serum free medium) and maximum release (target cells lysed using 0.1% Triton X-100) target cells were used as controls. Arbitrary Fluorescence Units (AFU) from each sample were measured using a Spectramax Gemini dual-scanning microplate spectrofluorimeter (Molecular Devices, Sunnyvale, CA, USA; excitation filter: 480 ± 9 nm; band-pass filter: 530 ± 10 nm). Percent lysis was calculated as described previously using the following formula: % specific lysis = 100 × (AFU mean experimental release−AFU mean spontaneous release)/(AFU means maximal release−AFU means spontaneous release) (22, 33). For the LDH-Glo cytotoxicity assay (Promega), 10,000 melanoma target cells were plated in a 96 well plate.
After adding 10,000 NK cells of each genotype, cells were centrifuged and incubated at 37°C for four hours. After the incubation period the plate was centrifuged for 5 minutes at 1200 RPM and 5 µl of each supernatant was harvested and transferred into a 96-well plate. Spontaneous (only target cells in serum free medium) and maximum release (target cells lysed using 0.1% Triton X-100) target cells were used as controls. Samples were measured as specified by manufacture’s protocol using GloMax Discover Microplate Reader (Promega).
Flow Cytometry-Based Cytotoxicity Assay
In vitro killing of human tumor cell lines by NK92 cells was evaluated using flow cytometry as previously described (32, 33). Briefly, tumor cells were resuspended in complete medium at a final concentration of 1x106 cells/mL and labeled with 5 uM eFluor 670 (Thermo Fischer Scientific) as per protocol instructions. After washing, labeled target cells (1 × 105) were added to 96-well plates along with indicated effector cells in complete RPMI media containing 100 U/mL of rhIL-2. Right before analysis 100ul of Propidium Iodide solution (Cell Signaling Technology) was added to the cells for 15 minutes at room temperature as indicated by manufacturer’s protocol. PI incorporation is used as a marker for late cell death as it intercalates with DNA in cells that have lost membrane integrity. Controls included target only wells (spontaneous death) and effector only wells to ensure that target cells only were in the eFluor 670+ gate. Results are shown as percent PI+ target cells ± SEM.
Cancer Cell Lines
The cancer cell lines mouse B16F10, human wm-164, and wm266-4 were a kind gift from Dr. B Bedogni (University of Miami, FL) and were cultured in DMEM supplemented with 10% FBS (Sigma) and 1% penicillin/streptomycin (HyClone). The murine acute lymphoblastic leukemia cell line (8093) was cultured in McCoy’s 5A media supplemented with: gentamycin (Gibco), 10% FBS (Sigma), Glutamax (Gibco), Sodium pyruvate (Gibco). Cells were washed every three days and supplemented with rmIL-3 (BioLegend), and BME (Gibco). Jeko-1 (purchased from ATCC) and RS411 cells (a kind gift from Dr. M. Dallas, Case Western Reserve University) were cultured in RPMI-1640 medium supplemented with 10% FBS (Sigma) and 1% penicillin/streptomycin (HyClone).
Tumor Mouse Models
Melanoma syngeneic tumors: B16F10 cells (1×106 cells in 0.1 ml complete RPMI media) were injected subcutaneously (s.c.) into 8-week-old C57BL/6 mice, and mice were surveyed every other day. Tumors were allowed to reach 50-100mm3 and treated intratumorally with either purified WT, or c-Rel NK cells (2.5x106/mouse) twice as indicated. For the melanoma (WM164) human in mouse xenograft: WM164 cells (5×106 cells in 0.1 ml complete RPMI media) were injected s.c. into 8-week-old NSG mice, and mice were surveyed every other day. We used pentoxifylline (PTXF) at 500 ug/mL to inhibit c-Rel in NK cells (Cayman Biochemicals). NK cells were pretreated with PTXF for 16-24 hours after which cells were washed to remove residual PTXF. PTXF or water control treated NK cells (10x106) were injected in 100ul volume intratumorally at the indicated times. Tumor volumes were calculated as follows: v=1/2(Length × Width2) (34) for the indicated times.
ELISAS
Supernatant were collected from the respective NK cells after treatment with either media only, IL-2, IL-15, or co-cultured with melanoma tumor cells (10: 1; NK: melanoma ratio) for 48 hours. NK cells were used at a final concentration of 1x106/mL. Samples were stored at −80°C and thawed prior to use. For mouse TNF- α, IL-10, and GM-CSF ELISAs, 100 ul of undiluted sample per condition was used as specified by the manufacturer’s instructions. For the mouse IFNγ ELISA, 50 ul of undiluted sample per condition was used as indicated by manufacturer’s protocol. All the ELISA kits used were purchased from BioLegend. Binding of TNF-α, IL-10, GM-CSF and IFNγ was detected using secondary antibody, streptavidin-HRP, and TMB substrate solution (provided with specified ELISA kit). Substrate conversion was stopped after 20 minutes with 100 µL stop solution (2N H2SO4) purchased from BioLegend. Plates were washed with PBS plus 0.05% Tween20 in-between incubations. Assay diluent provided by the manufacturer or RPMI medium (Sigma) was used as negative controls, and specific standard proteins were used as positive controls. Standard reconstitutions and curves were generated as per manufacturer’s instructions for each assay. Optical density values were obtained using a microplate reader set to 450 nm (Bio-Rad iMark Microplate reader).
Single-Cell Barcode Chip (SCBC) Assay
To evaluate the production of cytokine/chemokines by NK cells following c-Rel inhibition, SCBC assay was performed in collaboration with IsoPlexis (IsoPlexis, Brandord, CT) (35). Healthy donor PBMCs were treated with either water (negative control) or with PTXF (500 ug/mL) and stimulated with standard or high PMA-ionomycin (PI) and 10 ng/mL human IL-2 overnight. Cells were then placed in a IsoPlexis’s IsoLight micro-chamber pre-coated with antibodies specific for cytokines and NK cell phenotypic markers. Secreted cytokines are capture and fluorescence is analyzed to measure the number of cytokines producing cells and the number of cytokines produced by single cell (polyfunctionality).
Human NK92 and Primary NK Cells Culture
NK92 cells were a kind gift from Dr. Daniel Popkin (Innova Dermatology, TN, USA). Cells were cultured in alpha MEM media supplemented with: 2mM L-glutamine (Life Technologies), 1.5 g/L Sodium Bicarbonate (Fisher Chemical), 0.2 mM inositol (Acros Organics), 0.1 mM 2-mercaptoethanol (Life Technologies), 0.02 folic acid (Acros Organics), 12.5% horse serum (Sigma), 12.5% Fetal Bovine Serum (Sigma). Cells were washed and media was replaced every third day and supplemented with 200 U/mL IL-2 (PreproTech).
Human peripheral blood samples were collected from healthy donors in accordance with protocols approved by the Case Western Reserve University School of Medicine Human Studies Committee and Internal Review Board. Peripheral blood mononuclear cells (PBMCs) were obtained by using Ficoll-Paque and PBMCs were frozen in FBS containing 10% DMSO at a concentration of 10-20x106 cells per vial. NK cells expansion was performed as previously described (33), with minor modifications. Briefly, 10 x106 human PBMCs were cultured with 10 x106 irradiated K562 clone 9 cell line (a kind gift from Dr. Dean A. Lee (Nationwide Children’s Hospital, Columbus, OH) for 2-3 weeks. Cells were grown in RPMI-1640 medium supplemented with 10% FBS (Sigma) and 1% penicillin/streptomycin (HyClone). Fresh media was added every third day containing rhIL2 (100 U/mL; PeproTech). NK cells purity at the end of the 3-week coculture was (> 90%) as determined by flow cytometry (BD Accuri C6) using CD56 (5.11H11) and CD3 (HIT3a) antibodies from BioLegend.
Lentivirus Production and Infections
For shRNA mediated knockdown of c-Rel expression, NK92 cells were transduced with lentivirus vector pLKO- (Plasmid TRCN0000039984; Sigma), pLKO.1-puro eGFP shRNA control (Plasmid SHC005; Sigma) produced by packaging line HEK293FT. HEK293FT cells used for transfection were kindly provided by Dr. Ramakrishnan (Case Western Reserve University, Cleveland, OH).
Cells were grown in DMEM supplemented with 10% fetal bovine serum (Sigma) at 37°C in a 5% CO2 incubator. HEK cells were transfected with pMD2.G (Plasmid 12259; Addgene); psPAX2 (Plasmid 12260; Addgene) in conjunction with pLKO.1 lentiviral vector targeting GFP or human c-Rel at a ratio of (1.5:3:4) using X-tremeGENE HP DNA transfection reagent (Sigma). 48h and 72h later viral particle containing media was harvested, clarified, and concentrated using Lenti-X concentrator (Takara) as per manufacturer’s protocol. Viral particle containing supernatant was added to NK92 cells growing in rhIL-2 containing media (200 U/mL) in 15 mL round bottom tubes and spun down at room temperature, 3480 RPM for 90 minutes. After spinfection virus particles were removed and replaced with complete alpha MEM media supplemented as above. 24 hours post transduction 1 ug/mL puromycin (InvivoGen) was added to the cultures and cells were harvested after 48 hours post-puromycin selection. NK cells were assessed for expression of c-Rel by either western blot or PCR and for cytotoxicity against different tumor cells types via flow cytometry.
For rescue experiments, the complementary c-Rel cDNA was cloned into the pLM-CMV-Hapuro-PL3 lentiviral plasmid (36). Either bulk splenocytes plus bone marrow cells or isolated NK cells from WT or c-Rel-/- mice were transduced via spinfection. Briefly, 100x105 cells were plated in 96 well round bottom plates and 100 ul of viral particles was added. Cells and virus particles were spun at 22°C, 3480 RPM for 90 min. After centrifugation, virus particles were removed, and cells were cultured in complete RPMI media.
Quantitative Real-Time PCR
NK cells from WT, c-Rel-/-, cRelfl/fl, c-Relfl/flNcr1Cre mice were either pre-enriched using (Mojosort, BioLegend) or flow sorted (BD Biosciences Aria) and RNA was isolated using RNeasy Plus Mini Kit (Quiagen) according to manufacturer’s protocol. For bulk bone marrow plus splenocytes or human NK92 NK cells, RNA was isolated using High Pure RNA Isolation Kit (Roche). From purified RNA, complementary DNA was synthesized using the Bio-Rad iScript Reverse Transcription Supermix cDNA synthesis kit (Bio-Rad). qrtPCR was performed in duplicate or triplicates in a 96 well plate using Bio-Rad iQ SYBR Green Supermix according to the manufacturer’s instructions. Data are represented as fold induction over the mean of control samples, using the ΔΔCt method. Primer sequences are as follows:
Immunoblots
For western blot analysis, 4 x106 NK (Mojosort, purity >80%) or total combined splenocytes plus bone marrow from WT or c-Rel-/- mice were stimulated for 48 hours in complete RPMI containing 10% fetal bovine serum (FBS) and 1% pen strep with either rmIL-2 (1000 U/mL; BioLegend), rmIL-15 (100 ng/mL; BioLegend). Cells were washed with PBS and lysed with solubilization buffer containing 9 M urea. After sonication, lysates were spun down at 10,000 RPM for 5 minutes. Protein was quantified using (nanodrop nae, Case Comprehensive Cancer Center) and denatured with 4x laemmli buffer followed by boil for 10 min. 15-30 ug of total protein were loaded in 10% acrylamide gel. Proteins were transferred to nitrocellulose membranes (BioRad). Membranes were blocked with 5% dry milk in TBS containing 0.01% Tween and immunoblotted for mouse c-Rel (CD6), gzmb (sc-8022), p65 (sc-8008), GAPDH (sc-47724), prf1(sc-136994) purchased from Santa Cruz Biotechnology, mouse prf1 (3693s), human c-Rel (4727s) purchased from Cell Signaling, and alpha tubulin (cp06) purchased from Calbiochem. Mouse and Rabbit horseradish peroxidase (HRP) conjugated antibodies (Sigma) were used. Western blots were detected using chemiluminescence detection kit (Pierce) or chemiluminescence kit (BioRad).
Chromatin Immunoprecipitation (ChIP)
Analysis of c-Rel binding was performed as follows: 20 x106 expanded human NK cells were stimulated with IL-2 overnight. Cells were harvested, and proteins were cross- linked to DNA in two steps: (1) by adding DSG (Disuccinimidyl glutarate; ProteoChem) to a final concentration of 2mM for 45 minutes at room temperature followed by washing once with PBS and (2) by adding formaldehyde to a final concentration of 1.0% for 15 min at room temperature followed by the addition of 0.25 M glycine. Cells were washed twice with cold phosphate-buffered saline and resuspended in Farnham Lysis Buffer with the addition of protease inhibitor cocktail tablets (Sigma) followed by 15 minutes’ incubation with rotation at 4°C. Nuclear crude pellet was obtained by centrifugation at 4°C, 10,000 RPM for 10 minutes. Pellet was resuspended in RIPA (EMD Millipore) buffer and sonicated to shear chromatin by using the Sonicator 3000 Ultrasonic liquid processor optimized to 80 cycles of 8-s pulses with an output of 5 that gave a range of DNA fragments from 100 to 1,000 bp, as determined by 2% agarose gel electrophoresis (37). The soluble chromatin fraction was incubated with either anti-c-Rel antibody or anti-IgG control antibody (Biolegend) coupled to Protein G beads (Cell Signaling) overnight with rotation at 4°C. Immunocomplexes were washed with LiCl wash buffer and eluted with elution buffer. Protein-DNA cross-links were reversed at 65°C overnight with shaking. Samples were recovered by phenol/CHCl3/isoamyl alcohol extraction twice and purified as per kit instruction using Monarch PCR and DNA cleanup Kit (New England BioLabs). DNA was suspended in 200 ul pre-warm (55°C) elution buffer and stored at -80°C for real-time PCR amplification. The transcription factor binding site databases: Promo-ALGGEN and LASAGNA-Search 2.0 were used to find NF-κB or c-Rel specific binding sites in the promoter regions (10,000bp upstream and 1000 bp downstream) of perforin and granzyme B. Primers spanning the predicted NF-κB and c-Rel binding sites were designed using the Primer designing tool-NCBI-NIH. The following oligonucleotide sequences were used as primers to PCR amplify the region spanning the predicted site (atgggaaacta) NF-κB binding site in the granzyme b promoter: sense primer, 5’ CACCTTTGTCACCTGGAGAGT-3’ and antisense primer, 5’ AGAAACAGAAGCCAGGCCTAC-3’. For perforin, 5’TGGGCTAGGGTGGGATGTAG-3’ and antisense primer, 5’AGGGGTTGTTGTGAAGTGCT -3’ were used to amplify the region spanning the predicted site (agggaatggcca). Primers were taken from a predicted c-Rel non binding area of gzmb promoter to serve as a negative control for PCR. iQSYBR green PCR analysis was performed using CFx96 Real-Time System. Identical thermocycler conditions were used as per manufacturer’s instructions. Each PCR was performed in triplicates. Since PCR amplification was being monitored by SYBRgreen, the absence of nonspecific amplification was determined by analyzing the dissociation curve of the PCR amplification products. Data collected were analyzed using Microsoft Excel and plotted on PRISM software. The amount of precipitated target sequence was calculated as the fold increase in signal relative to the background signal (Fold enrichment method, Thermo Fisher Scientific).
Statistics
Data are shown as mean ± s.e.m. Two-tailed unpaired Student’s t test were used to determine significant differences (p< 0.05) using Prism software (Graph Pad, San Diego, CA). Sample size was not predetermined, but we used a number of mice that was consistent with prior experience with similar experiments.
Results
NK Cell Maturation Is Unaltered in c-Rel-/- Mice
c-Rel deficiency impairs the generation and maintenance of activated regulatory T cells and reduces activated B and T cell proliferation and survival (24, 27, 29). However, the role of c-Rel in NK cell has not been studied in detail. First, we analyzed c-Rel expression in NK cells compared to T and NKT cells. Flow cytometry analysis showed that murine NK cells express c-Rel comparable to T and NKT cells (Figure 1A). Murine NK cell maturation subsets are defined using CD27 and CD11b stainings on a gated population of CD3-NK1.1+ cells. We analyzed these NK cell subsets in spleen and bone marrow from wild type (WT) and c-Rel knockout mice (c-Rel-/-) (Supplementary Figure 1A). In mouse, four NK cell maturation subsets are defined based on CD27 and CD11b stainings (Figure 1B). We found that c-Rel expression is comparable in NK (NK1.1+ CD3-), NK-T (NK1.1+ CD3+) and T cell (NK1.1- CD3+) populations from spleen (Supplementary Figure 1B). NK cells develop from NK progenitor (NKp) cells, which originated from Common Lymphoid Progenitor (CLP) cells (Figure 1B). We confirmed c-Rel expression in NKp cells as well as differentiated CD27+ and CD11b+ NK cell subsets (Figure 1C and Supplementary Figure 1C). To determine whether c-Rel plays a role in NK cell development and maturation, we compared the total percentages and numbers of NK cells in germline c-Rel knockout mice (c-Rel-/-) compared to WT mice. Bone marrow (BM) and spleen (SPL) did not show any significant changes (Figures 1D, E), however, reduced percentages of NK cells were observed in the lymph nodes (LN) (.34% to.23%) and the liver (4.7 to 2.6%) of c-Rel-/- mice (Figure 1D). A pilot experiment indicated a potential reduction of both CD27+ and CD11b+ differentiated NK cells in the LN and liver of c-Rel-/- compared to WT mice (Supplementary Figure 1D). NK cell subsets from liver and lymph node are also shown (Supplementary Figure 1D). c-Rel-deficiency also led to reduced total NK cell numbers in the blood (0.47x106 to 0.2x106), LN (0.03x105 to 0.01x105), and liver (0.09x105 to 0.03x105) (Figure 1E). Analysis of these four NK cells subsets in bone marrow and spleen of c-Rel-/- mice did not show any major differences in percentages (Figure 1F) or absolute numbers (Figure 1G) compared to age/sex matched WT mice. In addition to CD11b, mature NK cells express markers such as DX5 and CD43. No measurable differences were found in the expression of these markers indicating that NK cell maturation process in c-Rel-/- mice, is similar to that of WT mice (Figure 1H). Genotyping followed by c-Rel flow analysis (Supplementary Figure 2A) were done prior to each experiment to confirm phenotype of mice.
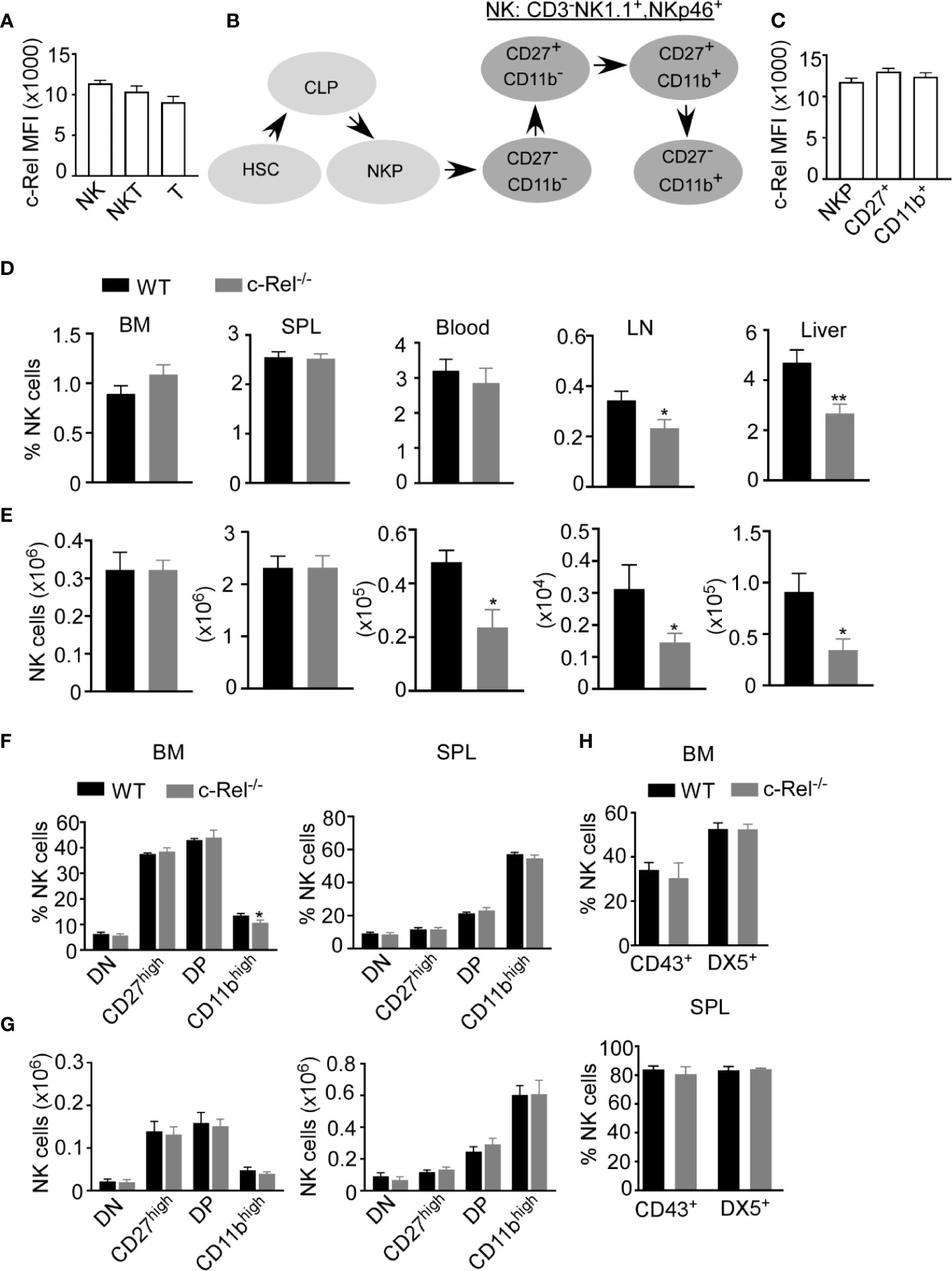
Figure 1 c-Rel is expressed in mouse NK cells. Total splenocytes from WT mice were stained intracellularly for c-Rel and analyzed via flow cytometry. (A) Summary data of c-Rel expression on gated CD3-NK1.1+ (NK), CD3+NK1.1+ (NKT), and CD3+NK1.1- (T) splenic cells. (B) Schematic of NK cell development: hematopoietic stem cell (HSC) commit to common lymphoid progenitor (CLP) cells, which give rise to NK progenitors (NKp). NK cell maturation stages are immature (CD27-, CD11b-) and (CD27+, CD11b-), mature (CD27+, CD11b+), and terminally differentiated NK cells (CD27-, CD11b+). (C) Summary data of c-Rel expression on gated CD3-NK1.1-CD122+ NK progenitors (NKp), immature and transient CD3-NK1.1+CD27+ NK cells, and mature CD3-NK1.1+CD11b+ NK cells. Each column (A, C) represents the mean ± SEM of data analyzed from 4 mice (n=4). Isotype IgG served as negative control for staining. (D) Percentages of gated CD3-NK1.1+ NK cells in WT and c-Rel-deficient (c-Rel-/-) mice in the indicated organs. (E) Total numbers of NK cells from WT and c-Rel-/- mice. CD3-NK1.1+ NK cells were analyzed for the expression of CD27 and CD11b maturation markers. (F) Percentages of double negative (DN), CD27high, double positive, and CD11bhigh cells gated on CD3-NK1.1+ cells from the indicated organs of WT and mice. (G) Total numbers of NK cell subsets from WT and c-Rel-/- mice. Each column represents the mean ± SEM of 13 mice. For all flow cytometry analysis live cells were gated using forward (FSC-A) and side scatter (SSC-A). Total numbers of NK cells per organ were derived by using the formula [(NK% of total cells/100) x total cell number per organ)] and NK cell subsets numbers were determined using the following formula [(NK cell subset % of total NK cells/100) x total NK cell number per organ)]. For bone marrow analysis femurs and tibia of mice were collected from 1-2 mice and the experiment was repeated 8 times. (H) Summary data of maturation markers CD43 and DX5 expression in gated NK1.1+CD3- cells from the indicated organs (n=3). All data represents mean ± SEM. Data were analyzed by unpaired Student’s t-test. *p < 0.05, **p < 0.01.
Cytotoxic Function Is Inhibited in NK Cells From c-Rel-/- Mice
As we did not observe any significant changes in NK cell maturation in c-Rel-/- mice, next we assessed if c-Rel is required for NK cell cytotoxic function. We performed calcein-AM release assay to test in vitro cytotoxicity of NK cells from WT and c-Rel-/- mice. Figure 2A shows that depending on the NK cell to target ratio, NK cells from WT mice killed 44-70% of murine 8093 acute lymphoblastic leukemia (ALL) cells compared to only 14–24% killed by c-Rel-/- NK cells. Interestingly, this is not specific to 8093 tumor cells. WT NK cells killed 39-85%, while c-Rel-/- NK cells killed only 17-40% of murine B16F10 melanoma cells (Figure 2A). Cytokines such as IL-2 and IL-15 are involved in the activation of NK cells (38, 39). Hence, we treated both WT and c-Rel-/- NK cells with IL-2 or IL-15 for 24 hours and analyzed cytotoxicity. As shown in Figure 2B and Supplementary Figure 2B, cytokine treatment did not restore cytotoxicity in c-Rel-/- NK cells. We then, tested if c-Rel-deficiency impairs NK cells killing in vivo. Melanoma tumor cells were implanted subcutaneously in WT recipient mice and tumor volume was monitored using calipers. Once tumors reached 50mm3, mice were randomized to be treated on day 5 and day 7 either with WT NK cells, c-Rel-/- NK cells, or PBS at the indicated time points (Figure 2C). Figure 2D shows that two injections of WT NK cells inhibited tumor growth, while c-Rel-/- NK cells treated tumors failed to do so. This signifies a key role of c-Rel in NK cell cytotoxic function.
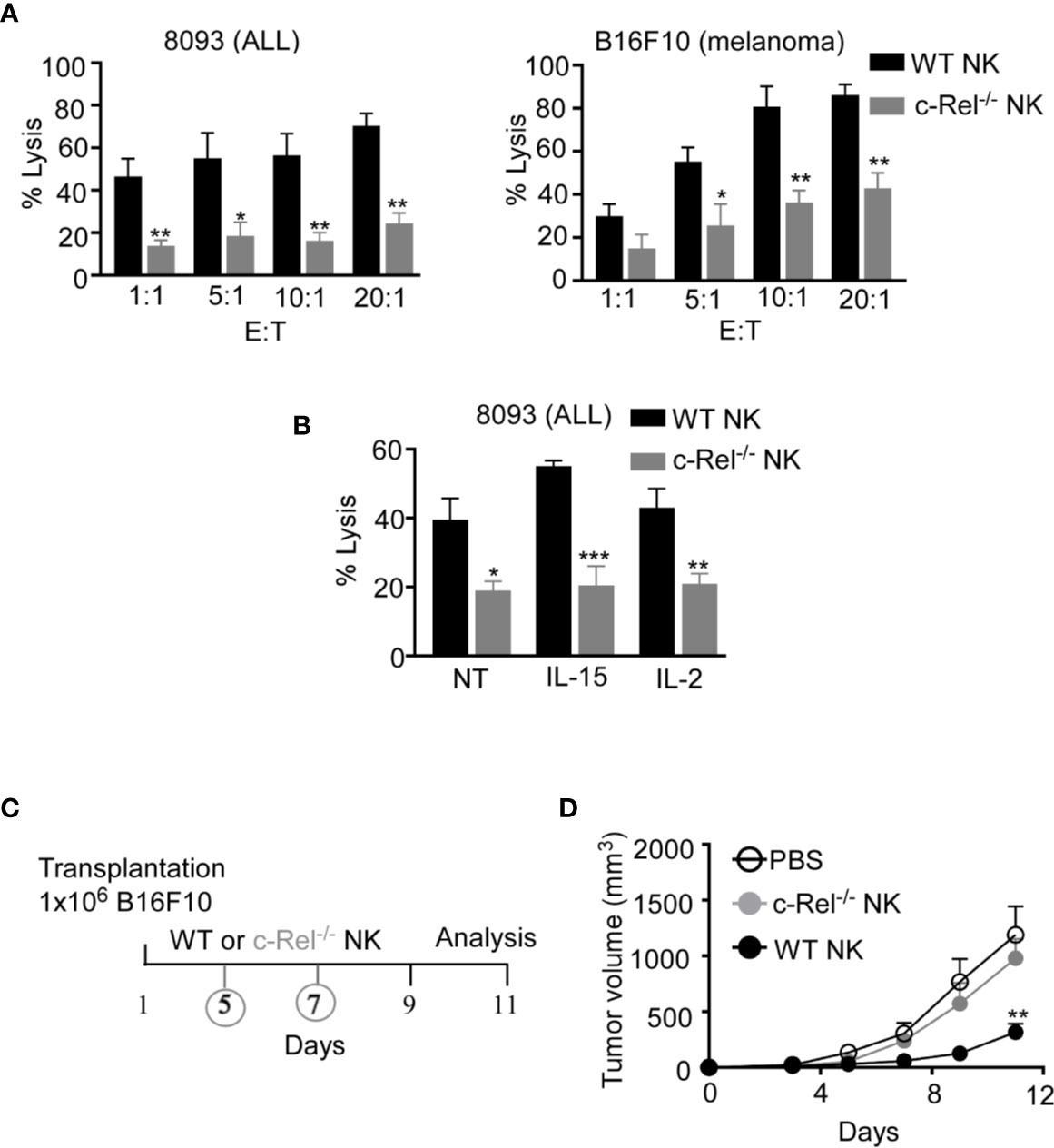
Figure 2 c-Rel deficiency impairs NK cell anti-tumor function. Cytotoxicity of NK cells from WT and c-Rel-/- mice was evaluated using calcein AM release assay. (A) Percent lysis of Acute Lymphoblastic Leukemia (ALL) and melanoma (B16F10) murine tumor cells co-cultured with either WT or c-Rel-/- NK cells for four hours at the indicated effector (E) target (T) ratio. Tumor cells alone was used as negative control and tumor cells lysed with detergent served as positive control. This was repeated 4 times. (B) Percent lysis of ALL cells after four-hour co-culture with either WT or c-Rel-/- NK cells pre-treated with media (NT), IL-15 (100 ng/mL), IL-2 (1000 U/mL) for 24 hours. The E:T ratio was 1:1 and cells were stimulated for 18 hours with the respective cytokines. Repeated 2 times. Data represents mean ± SEM of 2 or more independent experiments with NK cells pooled from the spleen and bone marrow of 2 mice of each genotype (n=4). (C) Schematic of in vivo melanoma subcutaneous tumor model in WT background, treated with either PBS (negative control), WT NK cells or c-Rel-/- NK cells on day 5 and day 7. (D) Tumor volume of WT mice treated with either PBS, WT NK cells or c-Rel-/- NK cells at the indicated time points. Data represents mean ± SEM of two independent experiments (n=5 per group). Data were analyzed using unpaired Student’s t-test. *p < 0.05; **p < 0.01; ***p < 0.001.
NK Cell Specific Conditional c-Rel Knockout Mice Exhibit NK Cell Dysfunction
Given that, c-Rel-deficiency has detrimental effects in B, T, and antigen presenting cells, it is crucial to dissect the cell intrinsic versus the extrinsic effects of c-Rel deficiency in NK cells. To understand how c-Rel-deficiency affect NK cell maturation and function in a cell intrinsic manner, we generated NK cell specific c-Rel-deficient mice (c-Relfl/flNcr1Cre) by crossing c-Rel-flox to Ncr1iCre mice. Western blot analysis (Figure 3A) and flow cytometry analysis (Supplementary Figure 3A) shows deletion of c-Rel in purified NK cells from c-Relfl/flNcr1Cre mice compared to controls c-Relfl/fl mice or WT mice. We then performed flow cytometry analysis of total NK cell population and found that compared to c-Relfl/fl control mice, c-Relfl/flNcr1Cre mice exhibit reduced percentages of NK cells in the spleen (2.8 to1.5%) and a slight reduction in the bone marrow (0.9 to 0.8%) (Figures 3B, C). Analysis of CD11b and CD27 expression on NK cells, reveals increased percentages of CD27 single positive NK cells (40 to 55%), but a reduction of double positive (41 to 33%), and a reduction of CD11b+ (15 to 11%) NK cells in the bone marrow of c-Relfl/flNcr1Cre mice compared to c-Relfl/fl mice (Figures 3D, E). Interestingly, NK cell subsets percentages were not altered in the spleen (Figure 3E). Similar to c-Rel-/- mice, c-Relfl/flNcr1Cre mice showed a reduction in the percentages of NK cell in the blood (3.3 to 1.8%), LN (0.38 to 0.26%), and liver (5.3 to 3.8%) compared to age/sex matched c-Relfl/fl mice (Figure 3F). Absolute cell numbers of total NK cells (Supplemental Figure 3B) and NK subsets are shown (Supplementary Figure 3C). Lastly, we tested whether c-Rel deficiency impairs NK cell ability to kill tumor cells in vitro and found that indeed, c-Relfl/flNcr1Cre NK cells kill only 17% of B16F10 tumor cells compared to 27% killing by NK cells from c-Relfl/fl control mice (Figure 3G).
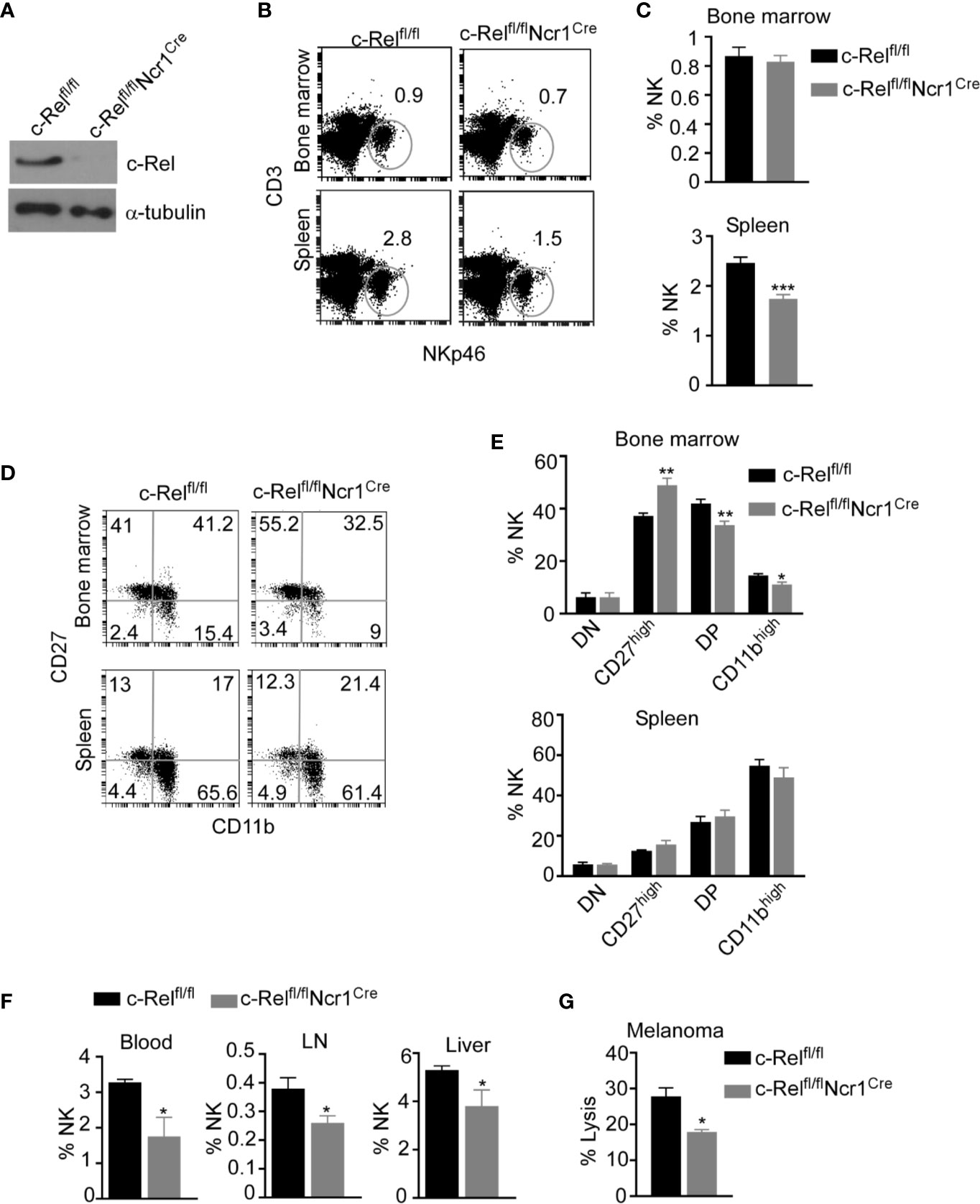
Figure 3 Loss of c-Rel alters cell-intrinsic NK cell maturation and anti-tumor function. NK cell specific c-Rel deficient (c-Relfl/flNcr1Cre) and c-Rel flox (c-Relfl/fl) mice were used to evaluate the cell-intrinsic effects of c-Rel deficiency in NK cells. (A) Western blot analysis of total lysate of uncultured NK cells pooled from the bone marrow and spleen of c-Relfl/fl and c-Relfl/flNcr1Cre mice (n=4 mice per group). Alpha tubulin was used as loading control. (B) Representative plots and (C) Summarized data of flow cytometry analysis CD3-NKp46+ NK cells from the bone marrow and spleen of the indicated mice. (D) Representative plots and (E) Summarized data of CD27 and CD11b expression in bone marrow and splenic CD3-NKp46+ NK cells. Each column represents the mean ± SEM of 5-8 mice (n=5-8). (F) Percentages of NK cells in the indicated organs of c-Relfl/fl and c-Relfl/flNcr1Cre mice (n=4). This experiment was repeated 6 times. (G) Percent lysis of melanoma tumor cells after co-culture with either c-Relfl/fl or c-Relfl/flNcr1Cre NK cells for 4 hours as measured by LDH release assay. Experiment repeated 2 times. Data represents mean ± SEM of technical replicates. Data were analyzed using unpaired Student’s t-test. *p < 0.05; **p < 0.01; ***p < 0.001.
c-Rel Deficiency Impairs Cytotoxic Function in Human NK Cells
Up to this point, we have shown that c-Rel-deficiency impairs murine NK cell’s anti-tumor function in a cell-extrinsic as well as cell-intrinsic manner. However, murine NK cells differ phenotypically from human NK cells and findings cannot simply be extrapolated from one system to the other. To determine if c-Rel play a role in human NK cell function, we first, evaluated c-Rel expression in primary NK cells from healthy donors. In accordance with our analysis of c-Rel in murine NK cells, human NK cells also express c-Rel at a comparable level to that of NKT and T cells (Figures 4A, B). Different human NK cell subsets also showed comparable c-Rel expression (Supplementary Figure 4A). We then used shRNA to ablate c-Rel expression in NK92 human NK cell line. Western blot analysis of NK92 cells transduced with shc-Rel shows around 50% reduction of c-Rel expression compared to shGFP control cells after 72 hours of viral transduction (Figure 4C), a repeat experiment western analysis also confirms this observation (Suppelementary Figure 4B). This reduction is also seen at the mRNA level (Figure 4D). We then analyzed cytotoxicity of these cells using four different human tumor cells as target cells. As shown in Figure 4E and Supplementary Figure 4C, c-Rel knockdown significantly impairs in vitro tumor killing by human NK cells at different effector/target ratios. We also tested the effect of reducing c-Rel expression in primary human NK cells. To do this, we used the c-Rel inhibitor pentoxifylline (40–42). Figure 4F and Supplementary Figures 4D, E show that PTXF treatment in primary NK cells leads to a reduction of c-Rel protein expression, without significantly affecting other NF-κB subunits including p65 expression (Supplementary Figures 4F, G) and cell viability (Suppelementary Figures 4H, I). Next, we tested in vivo cytotoxicity of NK cells pre-treated with or without PTXF using melanoma (WM164) xenograft tumor model (Figure 4G). Figure 4H, shows that four intratumor injections of control NK cells (pre-treated with water) significantly slows melanoma tumor growth from day 9-17 compared to PBS injected tumor bearing mice. NK cells pre-treatment with PTXF significantly reduced this tumor inhibitory property of NK cells (Figure 4H). Primary human NK cells transduced with c-Rel ShRNA showed reduced c-Rel expression (Suppelemementary Figure 5A) without altering viability (Supplementary Figure 5B) or receptor expression (Supplementary Figure 5C). Cytotoxic activity of c-Rel ShRNA cells was significantly reduced compared to ShGFP control cells, though modest effect has been observed compared to NK.92 cells (Supplementary Figure 5D).
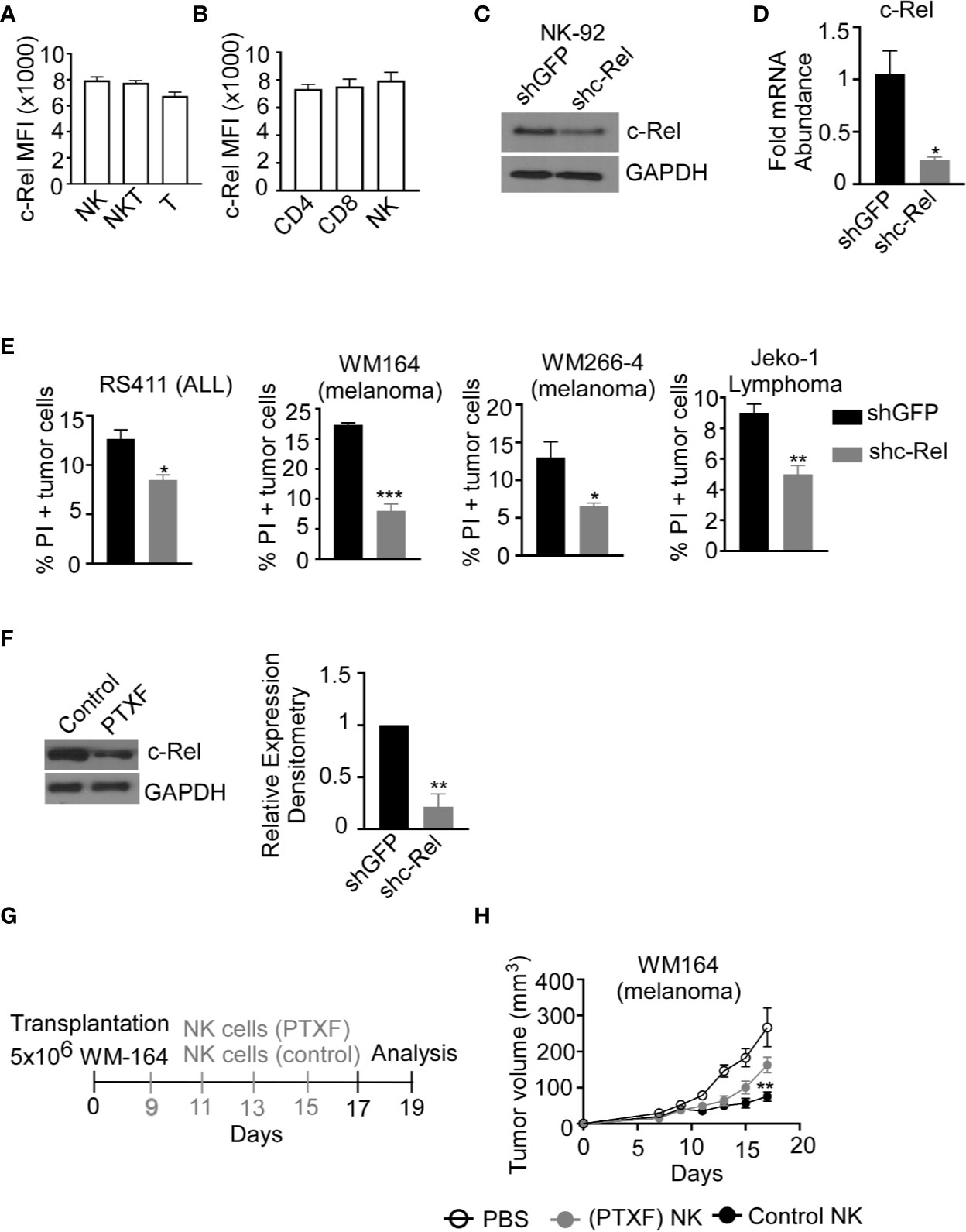
Figure 4 c-Rel regulates human NK cells anti-tumor function. c-Rel expression in primary human NK cells was evaluated by intracellular staining for c-Rel followed by flow cytometry analysis. (A) Summary data of Mean Fluorescence Intensity (MFI) of c-Rel expression on gated CD3-CD56+ (NK), CD3+CD56+ (NKT) and CD3+CD56- (T) human cells. (B) c-Rel expression in (CD3+ CD4+) or (CD3+CD8+) T cells compared to NK cells. Each column represents the mean ± SEM of data collected from peripheral mononuclear cells (PBMCs) from 3 healthy donors. The human NK cell line (NK92) was transduced with shRNA lentiviral vectors containing either GFP (shGFP), a negative control or shRNA targeting c-Rel (shc-Rel) and analyzed for western blot or PCR. (C) Western blot analysis on total cell lysates for c-Rel expression 48 hours post puromycin selection. GAPDH was used as loading control. Experiment done 3 times. (D) mRNA fold change expression of c-Rel in NK92 cell 48 hours post puromycin selection relative to ribosomal RNA 18s. (E) Percentage of Propidium iodide (PI) positive tumor cells after 4 hour co-culture with either shGFP or shc-Rel transduced NK92 cells, measured via flow cytometry. Effector:Target ratio is 1:1. Data represents mean ± SEM of three technical replicates. (F) Western blot analysis (left) and densitometry (right) of c-Rel expression in primary NK cells treated with water (vehicle control) or Pentoxiphyline (PTXF) inhibitor (500 ug/mL) for 16 hours. GAPDH was used as loading control. Data is representative of 3 different experiments using NK cells from 3 healthy donors. (G) Schematic of in vivo human melanoma (WM164) xenograft tumor model in NSG mice treated with either water pre-treated NK cells or PTXF pretreated NK cells for 16 hours prior to intratumor injections. (H) Tumor volume of NSG mice treated with either PBS (negative control), water pretreated NK cells (positive control) or PTXF pre-treated NK cells (16 hours). Four total treatments were administered every other day starting on day 9 post tumor inoculation (10x106 NK cells/injection). Data represents mean ± SEM of two independent experiments (n=5-6 per group). Data were analyzed using Student’s t-test. *p < 0.05; **p < 0.01; ***p < 0.001.
c-Rel-Deficiency Renders NK Cells Defective in Cytokine Production
NK cells recognize tumor cells via activating and inhibitory receptor repertoires on their cell surface. We evaluated the expression of activating and inhibitory receptors in c-Rel-/- NK cells compared to WT NK cells.
Supplementary Figures 6A, B shows comparable expression of the activating receptors (NKG2D, Ly49D, DNAM1, Ly49C-H, CD244, CD16) inhibitory receptors (NKG2A, KLRG1, Ly49A, and LIR/PIR), FAS ligand (FasL) and IL-2 receptors in c-Rel-/- NK cells and WT NK cells at steady state. Following recognition and target engagement, NK cells kill target cells mainly via the prf1 and gzmb lysis pathway, but also through ligands that bind to death receptors in tumor cells such as fasL (6–8). Flow cytometry analysis shows that c-Rel-/- NK cells express fasL at a level that is comparable to WT NK cells (Supplementary Figure 6B). c-Rel is known to binds to the promoters of IFNγ and GM-CSF (23, 43). We also measured cytokine production by WT NK cells compared to c-Rel-/- NK cells upon activation. Figure 5A shows that activation of NK cells with IL-15, IL-2, or melanoma tumor cells induces the release of pro-inflammatory cytokines, TNF-α, GM-CSF, and IFNγ in WT NK cells. Compared to WT NK cells, c-Rel-/- NK cells release less TNF-α, GM-CSF, and IFNγ upon activation with IL-15, IL-2, and B16F10 tumor cells (Figure 5A). The immune suppressive cytokine IL-10 is slightly increased in c-Rel-/- NK cells upon IL-15 and B16F10 tumor cell activation compared to WT NK cells (Figure 5A). This data shows that c-Rel-deficiency renders NK cells defective in their ability to produce cytokines after cytokine or tumor induced activation.
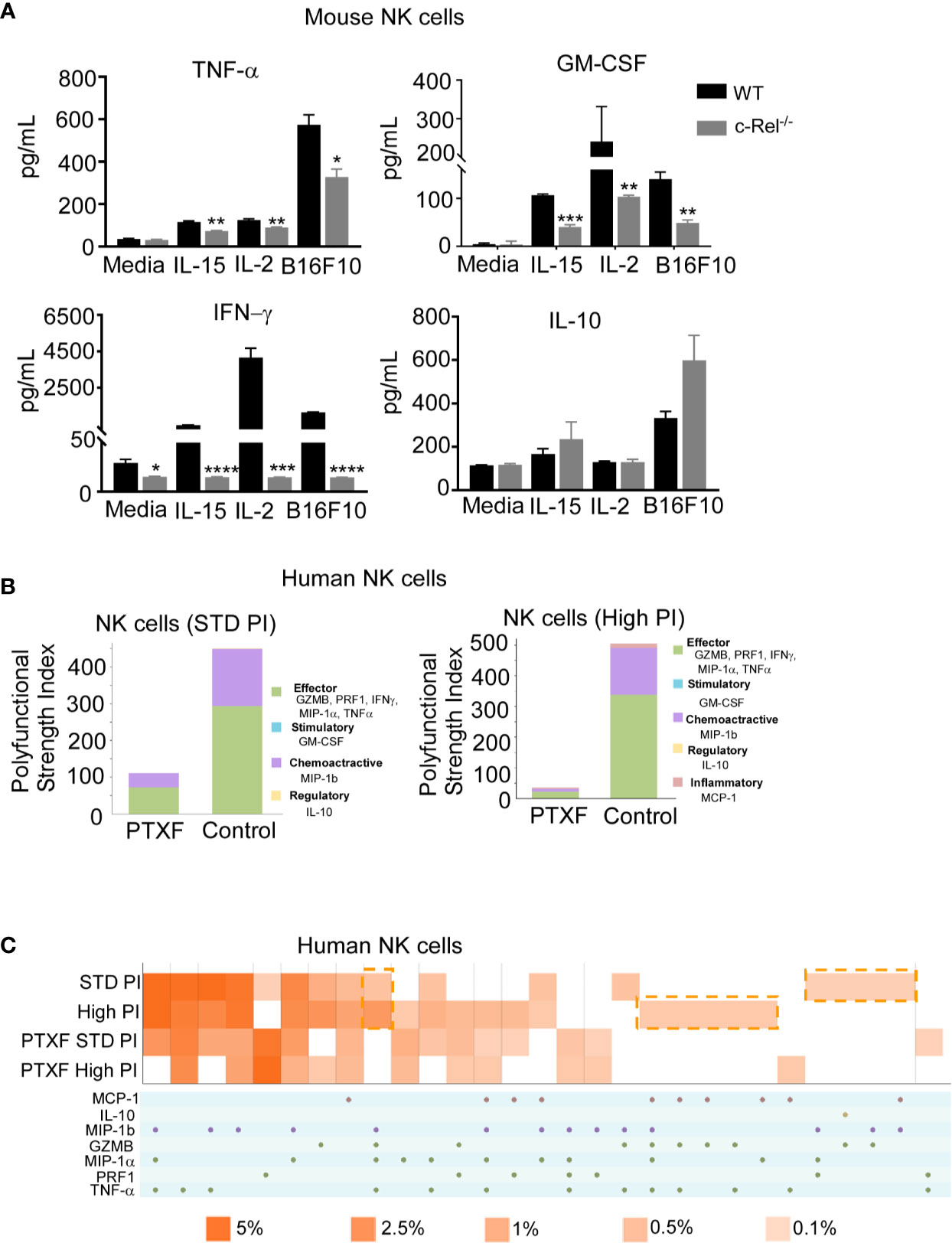
Figure 5 c-Rel deficiency impairs NK cell cytokine production. To evaluate the effect of c-Rel deficiency on the production of cytokines by NK cells, ELISA were performed using NK cells isolated from the bone marrow and spleen of c-Rel-/- mice and WT mice. (A) Summary data from ELISA used to measure TNF-α, GM-CSF, IFNγ, and IL-10 in the conditioned media of WT or c-Rel-/- NK cells cultured in media only (NT), IL-15 (100 ng/mL), IL-2 (1000 U/mL), or co-cultured with B16F10 melanoma murine tumor cells for 48 hours. NK cells were isolated from spleen and bone marrow of 3 WT and 3 c-Rel-/- mice (n=3/genotype). Data represents mean ± SEM of technical triplicates. (B) Polyfunctional Strength Index of the indicated cytokines/chemokines in healthy donor NK cells activated with IL-2 (10 ng/mL), and either standard (STD), or high PMA-neomycin (PI), following either water or PTXF (500 ug/mL) treatment for 12 hours. (C) Polyfunctional heat map illustrating single cell secretion of individual or combinations of cytokine/chemokines after the indicated treatments. Data were analyzed using unpaired Student’s t-test. *p < 0.05; **p < 0.01; ***p < 0.001; ****p < 0.0001.
To further confirm this, we performed a single cell cytokine analysis using control and PTXF treated human primary NK cells by Isoplexis. Polyfunctional Strength Index (PSI) is defined as the percentage of polyfunctional cells (secreting ≥2 cytokines) multiplied by the mean fluorescence intensity (MFI) of the secreted cytokines. Effector (GZMB, PRF1, IFNγ, MIP-1α, TNF-α), stimulatory (GM-CSF), chemoattractive (MIP-1b) and regulatory cytokines (IL-10) were measured at a single cell level. Here, the data show that activation with PMA/Ionomycin (PI) in control NK cells treated with water gives the expected high PSI, whereas treatment with the PTXF leads to lower PSI values (Figure 5B). This inhibitory effect is greater with higher dose of PI stimulation, as shown in Figure 5B. Figure 5C shows a heatmap visualization demonstrating that the positive PI stimulation of control NK cells gives the expected heterogenous heatmap visualization, with a higher frequency of polyfunctional cell subsets, while the PTXF treatment leads to a less heterogenous secretion profile, with a lower frequency of polyfunctional cell subsets. This effect was observed both in standard and high PI stimulation conditions.
Reduced Granzyme B and Perforin Expression Contributes to Cytotoxic Dysfunction in c-Rel-Deficient NK Cells
To determine the mechanism by which c-Rel regulates NK cell cytotoxicity, we evaluated the expression of prf1 and gzmb, two key cytotoxic mediators in NK cells (7, 9, 12, 14, 16, 44, 45). PCR analysis of c-Rel-/- NK cells shows reduced mRNA expression levels of gzmb (30%) and prf1 (50%) compared to WT NK cells (Figure 6A). Since it has been shown that cytokines such as IL-2 and IL-15 can induce the expression of gzmb and prf1 in cytotoxic lymphocytes, we treated c-Rel-/- and WT NK cells with either IL-2 or IL-15. Western blot analysis shows significant reduction of prf1 and gzmb protein levels in c-Rel-/- NK cells (Figure 6B) treated with IL-2, or IL-15. In this experiment we did not detected basal prf1 in NK cells, while gzmb showed reduced basal expression in c-Rel-/- NK cells (Figure 6B) compared to WT. We then asked whether this effect is unique to NK cells. PCR analysis of total pooled splenocytes and bone marrow cells shows a reduction in the mRNA levels of gzmb (51%) and prf1 (52%) in c-Rel-/- cells (Figure 6C). At the protein level, total cells show a reduction of both gzmb and prf1 in c-Rel-/- cells compared to WT cells (Supplementary Figures 7A, B). Degranulation was also analyzed in these cells detected by CD107+ cells (Supplementary Figure 7C). PCR analysis in isolated NK cells from c-Relfl/flNcr1Cre and c-Relfl/fl control mice also showed a reduction in gzmb (40%) and prf1 (50%) mRNA levels indicating that c-Rel regulates gzmb and prf1 both intrinsically and extrinsically (Figure 6C). Western blot analysis also shows reduced protein levels of gzmb and prf1 in NK cells from c-Relfl/flNcr1Cre mice compared to NK cells from c-Relfl/fl control mice (Figure 6D and Supplementary Figure 7D). We then evaluated gzmb and prf1 expression in NK92 cell line after c-Rel shRNA mediated knockdown. Figures 6E, F, shows a reduction in the protein levels of gzmb (30%) and prf1 (40%) in NK92 cells transduced with shc-Rel compared to shGFP. mRNA levels of gzmb and prf1 were reduced by 70 to 80% (Figure 6G). Finally, PTXF treated NK92 cells also showed reduced gzmb and prf1 expression correlating with reduced c-Rel in those cells, compared to control NK.92 cells treated with water (Figure 6H and Supplementary Figure 7E). We then used Chip analysis to determine if c-Rel regulates gzmb and prf1 by directly binding to their promoters. Figure 6I shows enrichment at the gzmb and prf1 promoter in the c-Rel pull-down compared to IgG control and primers targeted towards a predicted non c-Rel binding region (non-targeting) of granzyme B promoter was used as a negative control. This indicates that c-Rel binds the promoters of both gzmb and prf1. To determine whether c-Rel is sufficient to restore gzmb and prf1 expression in c-Rel-/- cells, we transduced c-Rel-/- pooled bone marrow and splenic cells (total cells) with either an empty or c-Rel containing lentivirus vector. Western blot analysis reveals that restoration of c-Rel expression in c-Rel-/- total cells increases the expression of gzmb and prf1 (Figure 6J). Finally, restoration of c-Rel restores c- Rel-/- NK cell’s cytotoxicity against ALL murine tumor cells to the level of WT NK cells (Figure 6K).
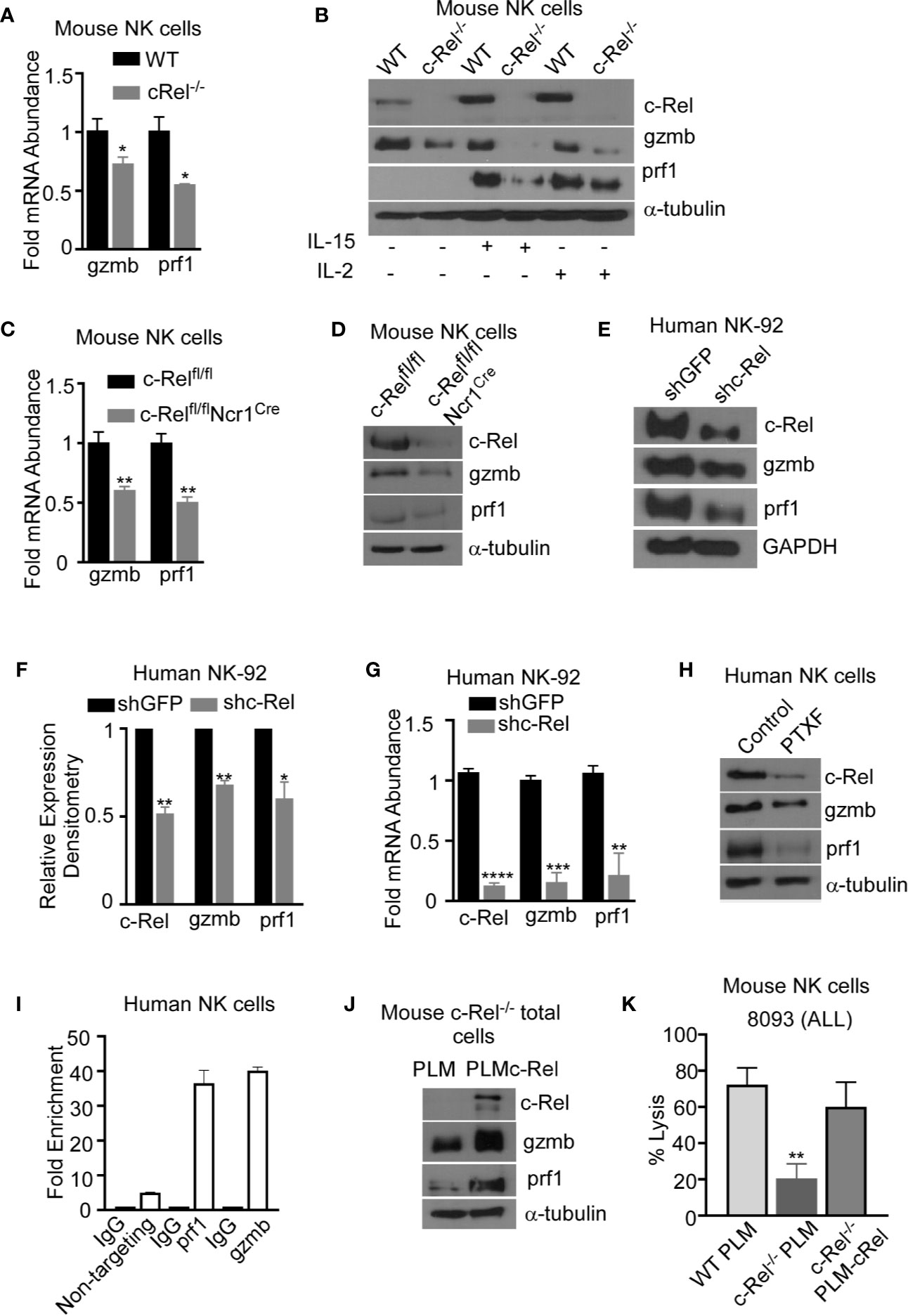
Figure 6 c-Rel regulates murine and human NK cell cytotoxicity via perforin and granzyme B. The expression of cytotoxic mediators granzyme B (gzmb) and perforin (prf1) was evaluated in NK cells pooled from the bone marrow and spleen of WT and c-Rel-/- mice. (A) PCR analysis of NK cells isolated from WT or c-Rel-/- mice. Data represents fold change relative to GAPDH from at least 3 independent experiments n=3 mice per group. (B) Western blot of WT and c-Rel-/- NK cells treated as indicated for 48 hours. Experiment done with NK cells pooled from 3 mice per group (n=6). (C) PCR of prf1 and gzmb in uncultured NK cells flow sorted from c-Relfl/flor c-Relfl/flNcr1Cre mice (n=2 mice per group). (D) Western blot analysis of prf1 and gzmb in c-Relfl/fl or c-Relfl/flNcr1Cre NK cells enriched with MojoSort. The loading control used for western blot analysis was α-tubulin. Repeated 3 times. NK cells were purified from 2-3 mice per genotype. (E) Western blot analysis and cumulative expression (F) of prf1 and gzmb in NK92 cells 72 hours after transduction with shGFP (empty vector) or shc-Rel (c-Rel, shc-Rel targeting vector). NK92 cells were selected with 1ug puromycin for 48 hours prior to analysis. Data repeated 2 times and GAPDH was used as loading control. (G) PCR analysis of c-Rel, prf1, and gzmb expression in NK92 cells 72 hours post lentiviral transduction. Data represents mean ± SEM of three independent experiments with similar results. (H) Western blot analysis of c-Rel, prf1 and gzmb in primary human NK cells 16 hours after treatment with PTXF. Water served as control. Experiment done 3 times with NK cells expanded from 2-3 different healthy donors. (I) Chip assay of c-Rel binding to the prf1 and gzmb promoters in human primary NK cells. The DNA precipitated by c-Rel was detected by PCR. As a negative control for PCR amplification, we designed primers from “nontargeting” area of Granzyme B, where there are no c-Rel binding predicted regions. (J) Western blot analysis of prf1 and gzmb of pooled bone marrow and splenic cells from c-Rel-/- mice 72 hours after transduction with either empty lentiviral vector (PLM) or lentiviral vector containing WT c-Rel (PLM-c-Rel). Experiment done 2 times (n=2). (K) Percent lysis of ALL cells by WT, or c-Rel-/- NK cells 72 hours after transduction with PLM lentivirus compared to c-Rel-/- NK cells transduced with PLM-WT-cRel lentivirus. Data represents 2 experiments done with NK cells isolated from 2-3 mice per genotype. Data represents mean ± SEM and were analyzed using Student’s t-test. *p < 0.05; **p < 0.01; ***p < 0.001; ****p < 0.0001.
Discussion
In this study, we show that loss of c-Rel in mice leads to reduced numbers of NK cells in the peripheral organs: blood, lymph nodes, and liver. This data is in concordance with a recent report of immunologic profiling of blood from a six-year-old patient with complete loss of c-Rel expression showing increased numbers of CD4+, CD8+ T cells, but reduced numbers of CD19+ B cells and reduced numbers of CD3-CD56+ NK cells (31). We did not observe any changes in total NK cell numbers or individual NK cell subsets in the bone marrow of c-Rel-/- mice indicating that c-Rel is not essential for the development or maturation of NK cells within the bone marrow niche. Indeed, the expression of maturation markers DX5 and CD43 on NK cells isolated from c-Rel-/- mice was also unaltered, compared to WT mice. This is not entirely unexpected as various studies in mice, report that c-Rel deficiency impairs T and B-cell proliferation and function without affecting the development and maturation of these cells (24–26). Although c-Rel-/- NK cells undergo normal maturation process, we provide evidence that loss of c-Rel impairs NK cell’s tumor killing function. We used ALL and melanoma cells as target cells representing liquid and solid tumors respectively. Tumor killing ability of c-Rel-/- NK cells was significantly reduced against both tumor cells regardless of the NK: Target ratio used. Given that IL-2 production is impaired in c-Rel-/- mice, one could argue that the lack of IL-2 activation in c-Rel-/- NK cells contributes to their cytotoxic dysfunction. However, this is unlikely as we show that exogenously added recombinant IL-2 or IL-15 fail to correct the NK cell dysfunction observed in c-Rel-/- NK cells, suggesting that c-Rel regulates NK cell function in a cell intrinsic way. This is also evident in the B16F10 melanoma in vivo data as shown in Figure 3D, where injecting WT NK cells into B16F10 melanoma tumors in a WT background led to tumor growth inhibition, while c-Rel-/- NK cells had minor effect on tumor growth.
The stromal cell compartment of the bone marrow, as well as interaction with other immune cells have been shown to play a role in NK cell development and function (4, 46). The functional defect of NK cells observed in c- Rel-/- mice might be influenced by these extrinsic factors and to exclude this possibility, we developed NK cell specific c-Rel-deficient mice (c-Relfl/flCre). Though total percentage of NK cells in bone marrow remain unaltered, liver and lymph node showed reduced NK cells in both c-Rel-/- and c-Relfl/flCre mice. In consistent with c-Rel-/- mice data, c-Relfl/flCre mice also showed a reduction in NK cell cytotoxicity confirming cell intrinsic role of c-Rel in these cells.
Deficiency of c-Rel either by knockout or PTXF treatment resulted in reduced tumor cell killing by NK cells both in syngeneic and xenograft melanoma models respectively. PTXF inhibits anti-CD3 induced c-Rel expression and TNF-α production in T cells. A more recent study reports that c-Rel inhibition in T regulatory cells with PTXF enhanced melanoma tumor killing in a CD8 T cell-dependent manner (42). Our data shows that PTXF treatment leads to reduced melanoma killing by NK cells. Reason behind these cell specific effect of c-Rel is not fully known. Recent reports show c-Rel playing an important role in regulatory T cells and myeloid cells checkpoint in cancer and inhibiting c-Rel as a method to enhance immunotherapy in cancer (42, 47). These observations suggest that the effects of PTXF or c-Rel inhibition differ among immune cell types and should be considered seriously while designing immunotherapies using c-Rel inhibitors.
NK cells recognize tumor cells through the direct interaction between its activating receptors with their ligands on tumor cells or through the lack of inhibitory receptor interaction with MHC-I on tumor cells (48). Of note, analysis of c-Rel-/- NK cells revealed no alterations in the expression of activating and inhibitory receptors indicating that the capacity to recognize tumor cells is not affected by c-Rel deficiency. c-Rel knockdown in primary human NK cells showed significant reduction in cytotoxicity, though effect was modest and we attribute that to the lower transduction efficacy of c-Rel shRNA resulting in modest c-Rel inhibition in these cells compared to NK.92 cells.
Polyfunctional T cells are T cells producing multiple cytokines and are known to provide a more effective immune response than cells producing only a single cytokine (49). We analyzed and confirmed polyfunctionality of NK cells producing effector, stimulatory, chemoattractive and regulatory cytokines as indicated by polyfunctionality strength index (PSI). PSI was inhibited more than 4 fold in c-Rel inhibited human NK cells as shown in Figure 4C, correlating to the reduced cytotoxicity observed in c-Rel deficient NK cells. NK cells kill tumor cells via release of cytotoxic mediators perforin and granzyme b or to a less degree via the interaction of NK cell FasL with the Fas receptor on tumor cells. We found comparable FasL expression in c-Rel-deficient NK cells compared to WT NK cells. However, a dramatic reduction in the mRNA and protein levels of perforin and granzyme B was noticed in c-Rel-deficient NK cells compared to WT. This was confirmed in both our c-Relfl/flCre mice, and shRNA mediated c-Rel knockdown in human NK cells. It is noteworthy that expression of perforin and granzyme was not completely blocked in c-Rel-/- NK cells, pointing to other transcription factors involved in the expression of these genes. As reported in previous studies, perforin basal level expression is minimal in NK cells, but can be activated with cytokines, IL-2, IL-15 (45). Interestingly, IL-2 or IL-15 treatment significantly increased perforin expression in WT NK cells, but not in c-Rel-/- NK cells.
Granzyme B is the most well characterized in the granzyme family. Other granzymes have also been implicated in mediating cytotoxicity, but Granzyme B is one of the most potent granzymes (50). Granzyme B levels were significantly reduced both at basal and cytokine induced conditions in the absence of c-Rel, pointing out to a differential transcriptional regulation of these genes by c-Rel. We show that c-Rel binds to both perforin and granzyme b promoters indicating a direct regulation of prf1 and gzmb by c-Rel. Rescuing c-Rel expression restores prf1 and gzmb expression and cytotoxicity in c-Rel-/- NK cells, confirming NK cell dysfunction caused by c-Rel deficiency is mostly resulting from decreased perforin and granzyme B expression.
Our data show that c-Rel plays a cell intrinsic role in regulating human and murine NK cell anti-tumor cytotoxicity by regulating the expression of cytotoxic molecules prf1 and gzmb. It would be of interest to check c-Rel expression in NK cells from cancer or viral infected patients and analyze its contribution to the NK cell dysfunction observed in these patients.
Data Availability Statement
The original contributions presented in the study are included in the article/Supplementary Material. Further inquiries can be directed to the corresponding author.
Ethics Statement
The studies involving human participants were reviewed and approved by University Hospitals IRB. Written informed consent for participation was not required for this study in accordance with the national legislation and the institutional requirements. The animal study was reviewed and approved by Institution’s Animal Care and Use Committee, Case Western Reserve University.
Author Contributions
YV performed the experiments, analyzed data, and wrote the manuscript. DPW, NKR, and ND designed and performed experiments for revision of this manuscript. KZ performed cloning and viral transductions. PR contributed to study design and discussions, provided key reagents including c-Rel knockout mice and protocols, and helped in troubleshooting and writing the manuscript. RP conceived the study, oversaw the study, designed experiments, guided research personnel, performed data analysis, and wrote the manuscript. All authors contributed to the article and approved the submitted version.
Funding
This work was supported by NIH Award F31CA232648 (YV), St. Baldrick’s scholar award (RP), The Andrew McDonough Be positive (B+) Foundation grant (RP), and 1R01AI116730-01A1 (PR). This work was also supported by the National Cancer Institute at National Institute of Health grant R21 CA246194 (P.R. and R.P.) National Institute of Allergy and Infectious Diseases at National Institute of Health grants R21 AI144264 (PR).
Conflict of Interest
RP is a consultant for Luminary therapeutics.
The remaining authors declare that the research was conducted in the absence of any commercial or financial relationships that could be construed as a potential conflict of interest.
Acknowledgments
This research was supported by the Athymic Animal and Hemapoietic Biorepository and Cellular therapy core facilities, Shared Resources of the Case Comprehensive Cancer Center (P30CA043703) for their support. We thank the flow cytometry core and the radiation core facilities at Case Western Reserve University. We thank the department of Pathology for providing the Accuri C6 and other valuable resources to conduct this study. We also thank Dr. A. Tomaka for providing assistance with in vitro expansion of NK92 cells, Dr. Nora Heisterkamp for providing 8093 mouse ALL cells and Dr. T. de Jesus for providing technical assistance with chip assays. Finally, we thank Drs. A. Huang and J. Letterio, for insightful discussions.
Supplementary Material
The Supplementary Material for this article can be found online at: https://www.frontiersin.org/articles/10.3389/fimmu.2021.652786/full#supplementary-material
Supplementary Figure 1 | Related to Figure 1: c-Rel expression in NK cells. Single suspension cells from bone marrow and spleen of WT and c-Rel-/- mice were analyzed via flow cytometry. (A) Dot plots of CD11b and CD27 expression on gated CD3-NK1.1+ NK cells from WT and c-Rel-/- mice (n=8-13). Total splenocytes from WT mice were stained intracellularly for c-Rel and analyzed via flow cytometry. (B) Histograms of c-Rel expression on gated CD3-NK1.1+ (NK), CD3+NK1.1+ (NKT), and CD3+NK1.1- (T) splenic cells (n=4). (C) Histograms of c-Rel expression on gated CD11b+ or CD27+ NK cells. Experiment was repeated four times with 4 mice of each genotype (n=4). Isotype IgG served as negative control for staining. (D) Dot plots representative of percentages of gated CD3-NK1.1+ NK cells (left) or histograms (right) showing the percentages of CD27+ and CD11b+ NK cells in WT (black) and c-Rel-/- (Blue) mice in the indicated organs.
Supplementary Figure 2 | Related to Figure 2: Flow cytometry analysis of c-Rel expression in WT and c-Rel-/- mice. Total splenocytes from WT and c-Rel-/- mice were stained intracellularly for c-Rel and analyzed via flow cytometry. (A) c-Rel expression in WT and c-Rel-/- mice (n=2). (B) Percent lysis of melanoma (B16F10) murine tumor cells co-cultured with either media or IL-2 pretreated (24 hours) WT or c-Rel-/- NK cells for four hours at the indicated effector (E) target (T) ratio. Tumor cells alone was used as negative control and tumor cells lysed with detergent served as positive control. Data represents mean ± SEM of two independent experiments (n=4). Data were analyzed using Student’s t-test. *p<0.05; **p<0.01.
Supplementary Figure 3 | Related to Figure 3: Cell-intrinsic effect of c-Rel deficiency in NK cell numbers. NK cell specific c-Rel deficient (c-Relfl/flNcr1Cre) and c-Rel flox (c-Relfl/fl) mice were used to evaluate the cell-intrinsic effects of c-Rel deficiency in NK cells. (A) Flow cytometry expression analysis of c-Rel in NK cells from WT and c-Relfl/flNcr1Cre mice (n=3). (B) Total numbers of NK cells in the indicated organ of c-Relfl/fl mice and c-Relfl/flNcr1Cre. CD3-NKp46+ NK cells were analyzed for the expression of CD27 and CD11b maturation markers. (C) Total numbers of NK cell subsets from c-Relfl/fl mice and c-Relfl/flNcr1Cre mice. Each column represents the mean ± SEM of 3-4 mice. For details on calculations of NK cell total numbers please see Figure 1 legend. Data were analyzed by unpaired Student’s t-test. *p<0.05.
Supplementary Figure 4 | Related to Figure 4: Effect of PTXF on NK cell viability and NF-κB subunits. c-Rel expression in primary human NK cells was evaluated by intracellular staining for c-Rel followed by flow cytometry analysis. (A) Dot plots of NK cell gating strategy (left) and histograms of c-Rel MFI in the indicated NK cell subset. Data from two individual human donors. The human NK cell line (NK92) was transduced with shRNA lentiviral vectors containing either GFP (shGFP), a negative control or shRNA targeting c-Rel (shc-Rel) and analyzed for western blot. (B) Western blot analysis (left) and densitometry (right) of c-Rel expression 48 hours post puromycin selection. GAPDH was used as loading control. (C) Percentage of Propidium iodide (PI) positive tumor cells after 4-hour co-culture with either shGFP or shc-Rel transduced NK92 cells, measured via flow cytometry. Data represents mean ± SEM of three technical replicates. (D) Dose response of PTXF on c-Rel expression in primary NK cells treated with water (vehicle control) or Pentoxiphyline (PTXF) inhibitor for 20 hours. (E) Densitometry analysis of c-Rel expression in primary NK cells treated with water (vehicle control) or Pentoxiphyline (PTXF) inhibitor with the indicated concentrations for 20 hours. GAPDH was used as loading control. (F) Western blot analysis and (G) Densitometry of NF-κB subunits expression in primary NK cells treated with water (vehicle control) or Pentoxiphyline (PTXF) inhibitor (500 ug/mL) for 16 hours. (H, I) Percent viable NK92 and primary NK cells after treatment with either water (vehicle control) or Pentoxiphyline (PTXF) inhibitor (500 ug/mL) for the indicated time. Experiment represents three technical replicates. Data were analyzed using Student’s t-test. *p<0.05; **p<0.01; ****p<0.0001.
Supplementary Figure 5 | Related to Figure 4: c-Rel knockdown in human NK cells. To evaluate the expression of receptors important for NK cell anti-tumor activity, human PBMCs were transuded with shRNA lentiviral vectors containing either GFP (shGFP), a negative control or shRNA targeting c-Rel (shc-Rel) and analyzed for western blot. (A) Western blot (left) and densitometry (right) analysis of c-Rel in primary NK cells transduced with shGFP or shc-Rel (72 hours). Experiment represents 3 independent analysis. (B) Viability of primary NK cells post-transduction as determined by Annexin V and Propidium iodide staining. (C) Histogram of flow cytometry analysis of activating and inhibitory receptors in shGFP or sh-cRel transduced primary NK cells. (D) Percent lysis of melanoma WM164 human tumor cells co-cultured with either shGFP or shc-Rel primary NK cells for four hours at an effector (E) target (T) ratio of 2.5:1 as measured by calcein-AM release assay. Tumor cells alone was used as negative control and tumor cells lysed with detergent served as positive control. Data represents technical triplicates. Data were analyzed using unpaired Student’s t-test. *p<0.05.
Supplementary Figure 6 | Related to Figure 5: Activating and inhibitory receptor expression in WT and c-Rel-/- NK cells. To evaluate the expression of receptors important for NK cell anti-tumor activity, single suspension cells were isolated from the bone marrow and spleen of c-Rel-/- mice and analyzed by flow cytometry. (A, B) Representative Mean Fluorescence Intensity (MFI) of gated NK cells expressing the indicated activating or inhibitory receptors from the bone marrow, the spleen or combine bone marrow and spleen. Data represents mean ± SEM of 2-3 independent experiments (n=2-3 per genotype) and were analyzed using unpaired Student’s t-test and all of these analysis in (A, B) were not significant with p>0.05.
Supplementary Figure 7 | Related to Figure 6: Effect of c-Rel deficiency in perforin and granzyme b expression. The expression of cytotoxic mediators granzyme B (gzmb) and perforin (prf1) was evaluated in NK and total cells pooled from the bone marrow and spleen of WT and c-Rel-/- mice. (A) Western blot analysis of total lysates from total cells pooled from the spleen and bone marrow of WT or c-Rel-/- mice treated for 48 hours with the indicated cytokines (n=3-4). (B) PCR analysis of gzmb and prf1 expression in isolated total cells from the spleen and bone marrow of WT or c-Rel-/- mice (n=3 per group). (C) Percentage CD107+ WT or c-Rel-/- NK cells cultured with media alone (no tumor cells), tumor cells 8093 (4 hours) at a 1:1 effector: target ratio, IL-15 (tumor+IL-15), or the combination of IL-15 with IL-2 (tumor+IL-2+IL-15) for 24 hours. (D) Western blot (left) and densitometry (right) analysis of c-Rel, perforin, and granzyme b expression in cells (spleen and bone marrow) from c-Relfl/flNcr1Cre and c-Relfl/fl mice. Densitometry data represents mean of three independent experiments. (E) Western blot (left) and densitometry (right) analysis of c-Rel, perforin, and granzyme b expression in primary human NK cells treated with water or PTXF for 16 hours. Densitometry data represents mean of three independent experiments. Data were analyzed using Student’s t-test. *p<0.05; **p<0.01; ***p<0.001.
References
1. Welsh RM, Brubaker JO, Vargas-Cortes ,M, O’Donnell CL. Natural Killer (NK) Cell Response to Virus Infections in Mice With Severe Combined Immunodeficiency. The Stimulation of NK Cells and the NK Cell-Dependent Control of Virus Infections Occur Independently of T and B Cell Function. J Exp Med (1991) 173:1053–63. doi: 10.1084/jem.173.5.1053
2. Crinier A, Narni-Mancinelli E, Ugolini S, Vivier E. Snapshot: Natural Killer Cells. Cell (2020) 180:1280–0. doi: 10.1016/j.cell.2020.02.029
3. Pegram HJ, Andrews DM, Smyth MJ, Darcy PK, Kershaw MH. Activating and Inhibitory Receptors of Natural Killer Cells. Immunol Cell Biol (2011) 89:216–24. doi: 10.1038/icb.2010.78
4. Wilford G, Huntington ND. Regulation of Murine Natural Killer Cell Development. Front Immunol (2017) 15:130. doi: 10.3389/fimmu.2017.00130
5. Smyth MJ, Cretney E, Kelly JM, Westwood JA, Street SE, Yagita H, et al. Activation of NK Cell Cytotoxicity. Mol Immunol (2005) 42:501–10. doi: 10.1016/j.molimm.2004.07.034
6. Paul S, Lal G. The Molecular Mechanism of Natural Killer Cells Function and its Importance in Cancer Immunotherapy. Front Immunol (2017) 8:1124. doi: 10.3389/fimmu.2017.01124
7. Prager I, Watzl C. Mechanisms of Natural Killer Cell-Mediated Cellular Cytotoxicity. J Leukoc Biol (2019) 105:1319– 1329. doi: 10.1002/JLB.MR0718-269R
8. Martínez-Lostao L, Alberto A, Pardo J. How do Cytotoxic Lymphocytes Kill Cancer Cells? Clin Cancer Res (2015) 21:5047–56. doi: 10.1158/1078-0432.CCR-15-0685
9. Voskoboinik I, Whisstock JC, Trapani JA. Perforin and Granzymes: Function, Dysfunction and Human Pathology. Nat Rev Immunol (2015) 15:388–400. doi: 10.1038/nri3839
10. Berthou C, Marolleau JP, Lafaurie C, Soulié A, Dal Cortivo L, Bourge JF, et al. Granzyme B and Perforin Lytic Proteins are Expressed in CD34+ Peripheral Blood Progenitor Cells Mobilized by Chemotherapy and Granulocyte Colony-Stimulating Factor. Blood (1995) 86:3500–6. doi: 10.1182/blood.V86.9.3500.bloodjournal8693500
11. Lowin B, Beermann F, Schmidt A, Tschopp J. A Null Mutation in the Perforin Gene Impairs Cytolytic T Lymphocyte- and Natural Killer Cell-Mediated Cytotoxicity. Proc Natl Acad Sci U S A (1994) 91:11571–5. doi: 10.1073/pnas.91.24.11571
12. Su B, Bochan MR, Hanna WL, Froelich CJ, Brahmi Z. Human Granzyme B is Essential for DNA Fragmentation of Susceptible Target Cells. Eur J Immunol (1994) 24:2073–80. doi: 10.1002/eji.1830240921
13. Lukoyanova N, Hoogenboom BW, Saibil HR. The Membrane Attack Complex, Perforin and Cholesterol- Dependent Cytolysin Superfamily of Pore-Forming Proteins. J Cell Sci (2016) 129:2125–33. doi: 10.1242/jcs.182741
14. Rudd-Schmidt JA, Trapani JA, Voskoboinik I. Distinguishing Perforin-Mediated Lysis and Granzyme- Dependent Apoptosis. Methods Enzymol (2019) 629:291–306. doi: 10.1016/bs.mie.2019.07.034
15. Trapani JA. Target Cell Apoptosis Induced by Cytotoxic T Cells and Natural Killer Cells Involves Synergy Between the Pore-Forming Protein, Perforin, and the Serine Protease, Granzyme B. Aust N Z J Med (1995) 25:793–9. doi: 10.1111/j.1445-5994.1995.tb02883.x
16. Bochan MR, Goebel WS, Brahmi Z. Stably Transfected Antisense Granzyme B and Perforin Constructs Inhibit Human Granule-Mediated Lytic Ability. Cell Immunol (1995) 164:234–9. doi: 10.1006/cimm.1995.1166
17. Tamang DL, Redelman D, Alves BN, Vollger L, Bethley C, Hudig D, et al. Induction of Granzyme B and T Cell Cytotoxic Capacity by IL-2 or IL-15 Without Antigens: Multiclonal Responses That are Extremely Lytic If Triggered and Short-Lived After Cytokine Withdrawal. Cytokine (2006) 36:148–59. doi: 10.1016/j.cyto.2006.11.008
18. Hodge G, Barnawi J, Jurisevic C, Moffat D, Holmes M, Reynolds PN, et al. Lung Cancer is Associated With Decreased Expression of Perforin, Granzyme B and Interferon (IFN)- Gamma by Infiltrating Lung Tissue T Cells, Natural Killer (NK) T-like and NK Cells. Clin Exp Immunol (2014) 178:79–85. doi: 10.1111/cei.12392
19. Bose A, Chakraborty T, Chakraborty K, Pal S, Baral R. Dysregulation in Immune Functions is Reflected in Tumor Cell Cytotoxicity by Peripheral Blood Mononuclear Cells From Head and Neck Squamous Cell Carcinoma Patients. Cancer Immun (2008) 8:10.
20. Kishi A, Takamori Y, Ogawa K, Takano S, Tomita S, Tanigawa M, et al. Differential Expression of Granulysin and Perforin by NK Cells in Cancer Patients and Correlation of Impaired Granulysin Expression With Progression of Cancer. Cancer Immunol Immunother (2002) 50:604–14. doi: 10.1007/s002620100228
21. He J, Li L, Wei W, Wei J, Long Z, Zhang Y, et al. Expression of Perforin and Granzyme-B in Peripheral Blood Lymphocyte in Patients With Prostate Cancer and the Clinical Significance. J Cent South Univ (2015) 40:387–91. doi: 10.11817/j.issn.1672-7347.2015.04.008
22. Parameswaran R, Ramakrishnan P, Moreton SA, Xia Z, Hou Y, Lee DA, et al. Repression of GSK3 Restores NK Cell Cytotoxicity in AML Patients. Nat Commun (2016) 7:11154. doi: 10.1038/ncomms11154
23. Gilmore TD, Gerondakis S. The c-Rel Transcription Factor in Development and Disease. Genes Cancer (2011) 2:695–711. doi: 10.1177/1947601911421925
24. Visekruna A, Volkov A, Steinhoff U. A Key Role for NF-κB Transcription Factor c-Rel in T-lymphocyte- Differentiation and Effector Functions. Clin Dev Immunol (2012) 2012:239368. doi: 10.1155/2012/239368
25. Milanovic M, Heise N, De Silva NS, Anderson MM, Silva K, Carette A, et al. Differential Requirements for the Canonical NF-κB Transcription Factors c-REL and RELA During the Generation and Activation of Mature B Cells. Immunol Cell Biol (2016) 95:261–71. doi: 10.1038/icb.2016.95
26. Kontgen F, Grumont RJ, Strasser A, Metcalf D, Li R, Tarlinton D, et al. Mice Lacking the C- Rel Proto-Oncogene Exhibit Defects in Lymphocyte Proliferation, Humoral Immunity, and Interleukin-2 Expression. Genes Dev (1995) 9:1965–77. doi: 10.1101/gad.9.16.1965
27. Isomura I, Palmer S, Grumont RJ, Bunting K, Hoyne G, Wilkinson N, et al. c-Rel is Required for the Development of Thymic Foxp3+ CD4 Regulatory T Cells. J Exp Med (2009) 206:3001–14. doi: 10.1084/jem.20091411
28. Ramakrishnan P, Yui MA, Tomalka JA, Majumdar D, Parameswaran R, Baltimore D. Deficiency of Nuclear Factor-κB c-Rel Accelerates the Development of Autoimmune Diabetes in NOD Mice. Diabetes (2016) 65:2367–79. doi: 10.2337/db15-1607
29. Basavarajappa SC, Ramakrishnan P. Regulation of B-Cell Function by NF-kappaB c-Rel in Health and Disease. Cell Mol Life Sci (2020) 17:3325–40. doi: 10.1007/s00018-020-03488-w
30. Almaden JV, Liu YC, Yang E, Otero DC, Birnbaum H, Davis-Turak J, et al. B-Cell Survival and Development Controlled by the Coordination of NF-κB Family Members RelB and cRel. Blood (2016) 127:1276–86. doi: 10.1182/blood-2014-10-606988
31. Beaussant-Cohen S, Jaber F, Massaad MJ, Weeks S, Jones J, Alosaimi MF, et al. Combined Immunodeficiency in a Patient With c-Rel Deficiency. J Allergy Clin Immunol (2019) 144:606–8. doi: 10.1016/j.jaci.2019.05.003
32. Eckelhart E, Warsch W, Zebedin E, Simma O, Stoiber D, Kolbe T, et al. A Novel Ncr1-Cre Mouse Reveals the Essential Role of STAT5 for NK-cell Survival and Development. Blood (2011) 117:1565–73. doi: 10.1182/blood-2010-06-291633
33. Vicioso Y, Gram H, Beck R, Asthana A, Zhang K, Wong DP, et al. Combination Therapy for Treating Advanced Drug-Resistant Acute Lymphoblastic Leukemia. Cancer Immunol Res (2019) 7:1106–19. doi: 10.1158/2326-6066.CIR-19-0058
34. Jensen MM, Jørgensen JT, Binderup T, Kjaer A. Tumor Volume in Subcutaneous Mouse Xenografts Measured by microCT is More Accurate and Reproducible Than Determined by 18F-FDG-microPET or External Caliper. BMC Med Imaging (2008) 8:16. doi: 10.1186/1471-2342-8-16
35. Rossi J, Paczkowski P, Shen YW, Morse K, Flynn B, Kaiser A, et al. Preinfusion Polyfunctional anti-CD19 Chimeric Antigen Receptor T Cells are Associated With Clinical Outcomes in NHL. Blood (2018) 132:804–14. doi: 10.1182/blood-2018-01-828343
36. Zhang K, Wong P, Salvaggio C, Salhi A, Osman I, Bedogni B. Synchronized Targeting of Notch and ERBB Signaling Suppresses Melanoma Tumor Growth Through Inhibition of Notch1 and ERBB3. J Invest Dermatol (2016) 136:464–72. doi: 10.1016/j.jid.2015.11.006
37. de Jesús TJ, Ramakrishnan P. Nf-κB c-Rel Dictates the Inflammatory Threshold by Acting as a Transcriptional Repressor. iScience (2020) 23:100876. doi: 10.1016/j.isci.2020.100876
38. Lehmann C, Zeis M, Uharek L. Activation of Natural Killer Cells With Interleukin 2 (IL-2) and IL-12 Increases Perforin Binding and Subsequent Lysis of Tumour Cells. Br J Haematol (2001) 114:660–5. doi: 10.1046/j.1365-2141.2001.02995.x
39. Wagner JA, Rosario M, Romee R, Berrien-Elliott MM, Schneider SE, Leong JW, et al. CD56bright NK Cells Exhibit Potent Antitumor Responses Following IL-15 Priming. J Clin Invest (2017) 127:4042–58. doi: 10.1172/JCI90387
40. Bemelmans MH, Abramowicz D, Gouma DJ, Goldman M, Buurman WA. In Vivo T Cell Activation by anti-CD3 Monoclonal Antibody Induces Soluble TNF Receptor Release in Mice. Effects of Pentoxifylline, Methylprednisolone, anti-TNF, and anti-IFN-gamma Antibodies. J Immunol (1994) 153:499–506.
41. Wang W, Tam WF, Hughes CC, Rath S, Sen R. c-Rel is a Target of Pentoxifylline-Mediated Inhibition of T Lymphocyte Activation. Immunity (1997) 6:165–74. doi: 10.1016/S1074-7613(00)80423-9
42. Grinberg-Bleyer Y, Oh H, Desrichard A, Bhatt DM, Caron R, Chan TA, et al. NF-κB c-Rel is Crucial for the Regulatory T Cell Immune Checkpoint in Cancer. Cell (2017) 170:1096–108. doi: 10.1016/j.cell.2017.08.004
43. Ghiorzo P, Musso M, Mantelli M, Garré C, Ravazzolo R, Bianchi-Scarrà G. c-Rel and p65 Subunits Bind to an Upstream NF-kappaB Site in Human Granulocyte Macrophage- Colony Stimulating Factor Promoter Involved in Phorbol Ester Response in 5637 Cells. FEBS Lett (1997) 418:215–8. doi: 10.1016/S0014-5793(97)01387-2
44. Pardo J, Balkow S, Anel A, Simon MM. Granzymes are Essential for Natural Killer Cell-Mediated and Perf- Facilitated Tumor Control. Eur J Immunol (2002) 32:2881–7. doi: 10.1002/1521-4141(2002010)32:10<2881::AID-IMMU2881>3.0.CO;2-K
45. Fehniger TA, Cai SF, Cao X, Bredemeyer AJ, Presti RM, French AR, et al. Acquisition of Murine NK Cell Cytotoxicity Requires the Translation of a Pre-Existing Pool of Granzyme B and Perforin mRNAs. Immunity (2007) 26:798–811. doi: 10.1016/j.immuni.2007.04.010
46. Stabile H, Fionda C, Santoni A, Gismondi A. Impact of Bone Marrow-Derived Signals on NK Cell Development and Functional Maturation. Cytokine Growth Factor Rev (2018) 42:13–9. doi: 10.1016/j.cytogfr.2018.03.008
47. Li T, Li X, Zamani A, Wang W, Lee CN, Li M, et al. c-Rel is a Myeloid Checkpoint for Cancer Immunotherapy. Nat Cancer (2020) 1:507–17. doi: 10.1038/s43018-020-0061-3
48. Martinet L, Smyth MJ. Balancing Natural Killer Cell Activation Through Paired Receptors. Nat Rev Immunol (2015) 15:243–54. doi: 10.1038/nri3799
49. Haining WN. Travels in Time: Assessing the Functional Complexity of T Cells. Proc Natl Acad Sci U S A (2012) 109(5):1359–60. doi: 10.1073/pnas.1119856109
Keywords: NF-κB c-Rel, granzyme, perforin (Prf1), NK cell, cytotoxicity
Citation: Vicioso Y, Wong DP, Roy NK, Das N, Zhang K, Ramakrishnan P and Parameswaran R (2021) NF-κB c-Rel Is Dispensable for the Development but Is Required for the Cytotoxic Function of NK Cells. Front. Immunol. 12:652786. doi: 10.3389/fimmu.2021.652786
Received: 13 January 2021; Accepted: 12 April 2021;
Published: 29 April 2021.
Edited by:
Aharon Freud, The Ohio State University, United StatesReviewed by:
Jennifer Ann Foltz, Washington University in St. Louis, United StatesKimberly Risma, Cincinnati Children’s Hospital Medical Center, United States
Copyright © 2021 Vicioso, Wong, Roy, Das, Zhang, Ramakrishnan and Parameswaran. This is an open-access article distributed under the terms of the Creative Commons Attribution License (CC BY). The use, distribution or reproduction in other forums is permitted, provided the original author(s) and the copyright owner(s) are credited and that the original publication in this journal is cited, in accordance with accepted academic practice. No use, distribution or reproduction is permitted which does not comply with these terms.
*Correspondence: Reshmi Parameswaran, cnhwMjc4QGNhc2UuZWR1