- 1Medical Center of Hematology, Xinqiao Hospital, Third Military Medical University (Army Medical University), Chongqing, China
- 2State Key Laboratory of Trauma, Burns and Combined Injury, Third Military Medical University (Army Medical University), Chongqing, China
- 3Department of Cell Biology, College of Basic Medicine, Third Military Medical University (Army Medical University), Chongqing, China
Allogeneic hematopoietic stem cell transplantation (allo-HSCT) is a potentially curative therapy for many hematological disorders and autoimmune diseases, but acute graft-versus-host disease (aGVHD) has remained a major obstacle that limits allo-HSCT and exhibits a daunting mortality rate. The gastrointestinal system is among the most common sites affected by aGVHD. Experimental advances in the field of intestinal microbiota research enhanced our understanding - not only of the quantity and diversity of intestinal microbiota - but also their association with homeostasis of the immune system and disease pathogenesis, including that of aGVHD. Meanwhile, ever-growing clinical evidence suggest that the intestinal microbiota is dysregulated in patients who develop aGVHD and that the imbalance may affect clinical outcomes, indicating a potential predictive role for microbiota dysregulation in aGVHD severity and prognosis. The current animal and human studies investigating the intestinal microbiota in aGVHD and the understanding of the influence and management of the microbiota in the clinic are reviewed herein. Taken together, monitoring and remodeling the intestinal microecology following allo-HSCT may provide us with promising avenues for diagnosing, preventing or treating aGVHD in the clinic.
Introduction
Malignancies of the hematopoietic system and therapy-refractory autoimmune diseases are frequently associated with high mortality and hence represent the most common indications to perform allogeneic hematopoietic stem cell transplantation (allo-HSCT) (1, 2). However, graft-versus-host disease (GVHD), in which donor-derived T cells recognize host tissues as foreign, causing inappropriate and aberrant immune attacks, remains one of the major limitations to HSCT. Approximately 40-60% of patients receiving allo-HSCT may suffer from GVHD (3–5) with a mortality rate of 15% to 20% (3, 4, 6–9). Clinically, prophylaxis of acute GVHD (aGVHD) involves immunosuppression of donor cells, but there is no standard approach, and it often varies by institution (3). Treatment protocols can also be challenging because therapeutic options are limited, response rates for corticosteroids are only approximately 50%, and response durations are typically brief (6, 7). In addition, several drugs are reported to be effective in patients not responding to corticosteroids, but most data are unconvincing, and combination therapies tried to date have yielded modest or no benefit over corticosteroids alone (10–12). Because of the small number of results from well-designed, large-scale, clinical studies, there is considerable variability in dealing with aGVHD worldwide, which leads to updated consensus recommendations that still have problems (13).
Much work has been done to research the biological mechanisms participating in the pathogenesis of aGVHD, but the specific nature of these interactions has not been fully elucidated, especially the relationship between aGVHD and the intestinal microbiota. The intestinal microbiota has been proven to be critical for maintaining healthy tissues and stimulating immunity (14), and increasing evidence has revealed that dysbiosis in intestinal microbial populations is linked to human disease and defects in immunity (15–18). Recent studies have notably widened our understanding of the interactions between the loss of intestinal bacterial diversity and aGVHD following allo-HSCT. This review provides an update of current knowledge on the cross-talk between them, with the purpose of determining improved prophylactics and therapies for aGVHD based on the role of the intestinal microbiota.
The Intestinal Tract and Intestinal Microbiota
The gastrointestinal tract consists of the mucous layer, submucous layer, muscular layer and serosa from the inner to outer layers. The intestinal mucosa, the innermost layer of the gastrointestinal tract, can be further divided into the epithelium, lamina propria and muscularis mucosae (19). The epithelium is a single-cell layer that contains unique secretory cells and stem cells, and many immune cells in the lamina propria help to monitor pathogens and maintain immune tolerance to food and commensal antigens (20). The mucosal surface maintains an intact biological barrier that prevents substantial bacterial and other detrimental invasion into the host tissue and blood circulation under steady-state homeostasis; this function is implemented by epithelial tissues, gut-associated lymphoid tissue (GALT) and important secretory components (21). The commensal intestinal microbiota also contributes to the maintenance of intestinal ecological balance. An overview of homeostasis between the microbiota and the host intestinal mucosa is shown in Figure 1A.
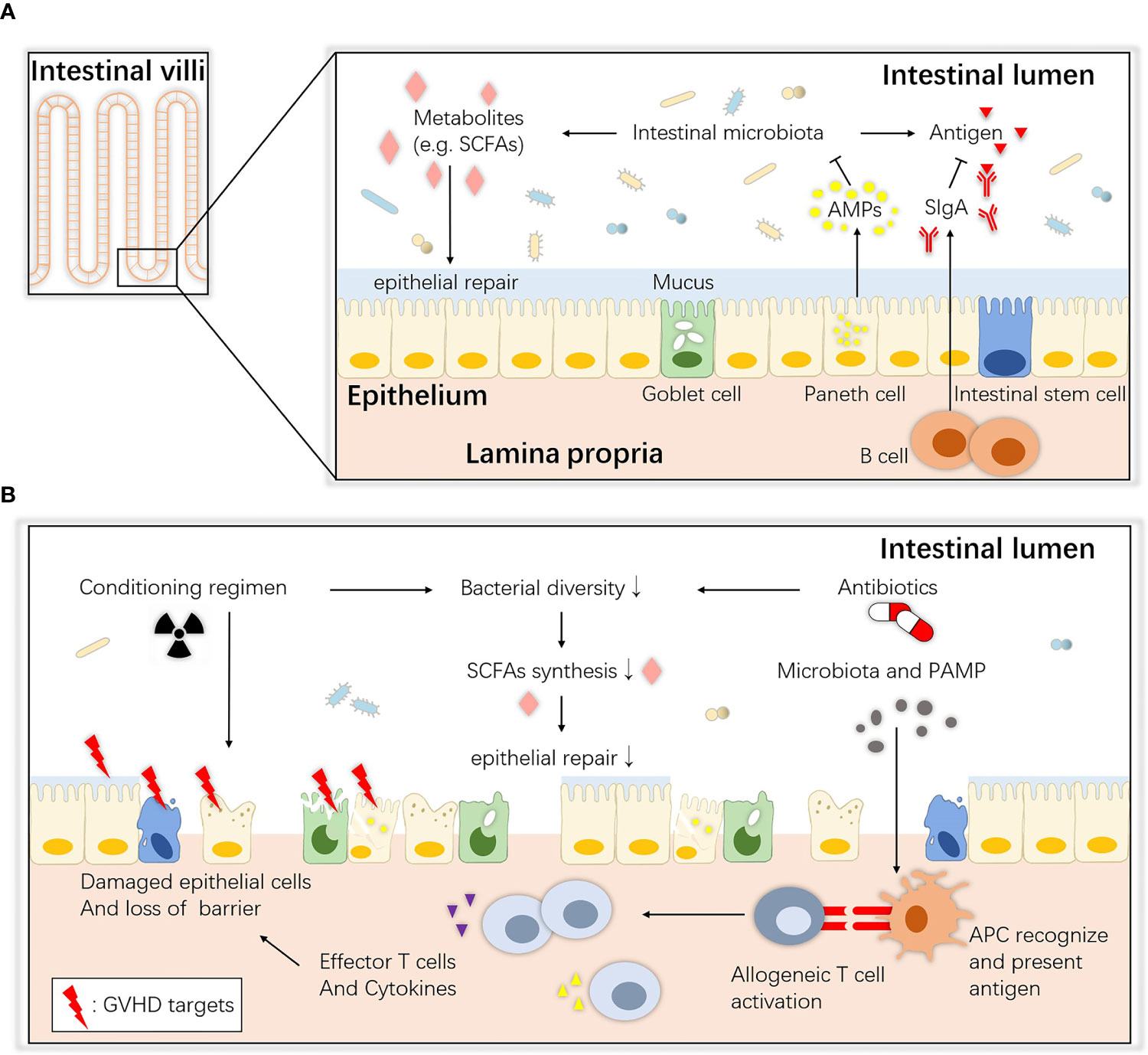
Figure 1 Overview of intestinal ecology and gastrointestinal aGVHD. (A) Homeostasis between the commensals and host intestinal epithelium. At steady state, host intestinal epithelial cells live with commensals, and their interaction maintains immune and biological homeostasis. ISCs maintain the regeneration of the epithelium, Paneth cells secrete AMPs that create a sterility gradient, goblet cells produce mucus to separate the microbiota from host epithelial tissue, and immune cells such as B lymphocytes secrete SIgA to neutralize biologically active microbial antigens. Together, they maintain an intact barrier on the mucosa surface. SCFAs (e.g., butyrate) are bacterial fermentation products that can be used as an energy source and regulate the differentiation, recruitment and activation of immune cells. (B) Pathogenesis of gastrointestinal aGVHD. During allo-HSCT, the antibiotics and altered diet and cell damage caused by the conditioning regimen all lead to dysbiosis and metabolic disorders. Then, the depletion of SCFAs may also contribute to epithelial defects, allowing translocation of pathogenic bacteria and PAMPs. APCs (e.g., DCs) recognize them and elicit Th1 and Th17 responses and the release of proinflammatory factors that enhance tissue damage. ISCs, intestinal stem cells; AMPs, antimicrobial peptides; SCFAs, short-chain fatty acids; SIgA, secretory immunoglobulin A; APC, antigen-presenting cell; PAMP, pathogen-associated molecular pattern.
The human intestinal tract hosts 1013 to 1014 microbial organisms of approximately 1000 species (Table 1) (22, 23). Although viruses and fungi are also present in considerable amounts and diversity, the vast majority of these organisms are bacteria collectively termed the gut microbiota (24–26), which play an important role in the synthesis of a variety of vitamins and amino acids, participating in the metabolism of carbohydrates and proteins and promoting the absorption of various mineral elements (27). The balance and diversity of the gut microbiota is of great importance for the human body, as researchers have found close connections between changes in the gut microbiota and human diseases, such as obesity (28), diabetes (29, 30), functional bowel syndrome (31), autism (32), and autoimmune diseases (e.g., rheumatoid arthritis (33)). For decades, the analysis of the intestinal microbiota has been largely dependent on ex vivo cultivation of bacteria, which yields only 10–30% of the population, limiting knowledge of the bacterial composition (34). In recent years, the development of next-generation sequencing technologies, such as 16S rDNA sequencing and metagenomics, has allowed for further identification of microorganisms, which clarifies the detailed and specific role of the intestinal microbiota in aGVHD, leading to a new era of research (35, 36).
Intestinal Microbiota in the Mechanism of aGVHD
Pathophysiology
Development of aGVHD is considered a three-step process. The microbiota-linked pathogenesis of gastrointestinal aGVHD is summarized herein.
In the first step, when the conditioning regimen of allo-HSCT damages the intestinal epithelium, homeostasis between the host and intestinal commensals is disturbed (Figure 1B). Total-body irradiation (TBI) induces dose-dependent damage to the gut lining including killing intestinal stem cells (ISCs), depleting or inhibiting non-epithelial cells, injuring intestinal crypts and causing gastrointestinal tract syndrome (37–39), by mechanisms such as increasing p53-mediated epithelial apoptosis (40) and plasminogen activator inhibitor-type 1 (PAI-1)-mediated enteritis (41). The intestinal mucosa is the major target tissue, and histological evidence has shown villous shortening, increased lymphocytic cell infiltration, crypt destruction and epithelial apoptosis. Crypt cell degeneration has been suggested to be the initial lesion of gastrointestinal aGVHD (42–44), and loss of goblet cells and Paneth cells has been shown to lead to translocation of dominant luminal pathogens and pathogen-associated molecular patterns (PAMPs) as well as intestinal dysbiosis, which further accelerates gastrointestinal aGVHD and infections (45). Moreover, some studies have provided evidence that these toxic effects are partially mediated by the intestinal microbiota. Lai et al. found that mice treated with antibiotics or deficient in myeloid differentiation primary response gene 88 (MyD88), a crucial adaptor for recognition of microbial molecules, showed less crypt loss and less damage to progenitor and stem cells after radiation (46). Seth et al. showed that the microbiota protects against dextran sodium sulfate–induced intestinal damage after radiation (47). It is possible that the microbiota affects the initiation of damage.
In terms of the alloreactive cells at the second step, it is well known that potential pathogens and their antigen molecules can activate T cells and affect differentiation. A study showed that cohousing laboratory mice with feral mice produced mice with immune systems closer to those of adult humans, with a preference toward effector and memory T cell populations, suggesting that ‘dirtier’ mice have more intrinsic activation leading to more GVHD (48). After observing their existence, studies have shown that activated T cells access the epithelium via gut-specific homing molecules on the T cells and adhesion ligands on the vasculature, such as MAdCAM-1; the intestinal crypts are the primary location invaded by T cells, wherein they directly interact with ISCs (49–54). For T cell differentiation, the induction of both T helper (Th) and regulatory T (Treg) cells is influenced by the microbiota. Breaching the intestinal barrier or penetration by the microbiota in aGVHD activates the IL-23 pathway by JAK-STAT, stimulating epithelial cells to produce serum amyloid A proteins, which leads to Th17 differentiation, an important subset in aggravating aGVHD (55–57). Interestingly, some species, such as Bacteroides fragilis, prevent IL-17 production by releasing polysaccharide A (PSA) and promoting Foxp3+ Tregs, and Tregs maintain gastrointestinal homeostasis via the release of IL-10 (58), which could alleviate aGVHD. Other species, i.e., Clostridiales, produce SCFAs (e.g., butyrate and propionate), which block histone deacetylases (HDACs) through the G-protein receptor (GPR) to promote acetylation of histone H3 in Tregs at the Foxp3 locus, which also induces Treg differentiation (59). In addition, innate lymphoid cells 3 (ILC3s), an activated population with expression of the natural cytotoxicity receptor (NCR, such as NKp44) and nuclear hormone receptor RORγt were found mainly in mucosal tissues and emerged as modulators of conditioning-induced tissue damage in the context of aGVHD, secreting IL-22 and IL-17, which are necessary in defense against bacterial pathogens (60, 61). NCR+ ILC3-derived IL-22 has already been found to be crucial in epithelial recovery and protect ISCs from damage by activating STAT3 and downstream regulators of cellular proliferation and survival which finally attenuates aGVHD (62, 63). But there is no direct evidence that NCR- ILC3s-derived IL-17 is involved in the pathology of aGVHD (64). Granulocyte macrophage colony-stimulating factor (GM-CSF) produced by ILC3s is also essential for the normal development of intestinal dendritic cells (DCs) involved in Treg induction (65). These interactions are summarized in Figure 2.
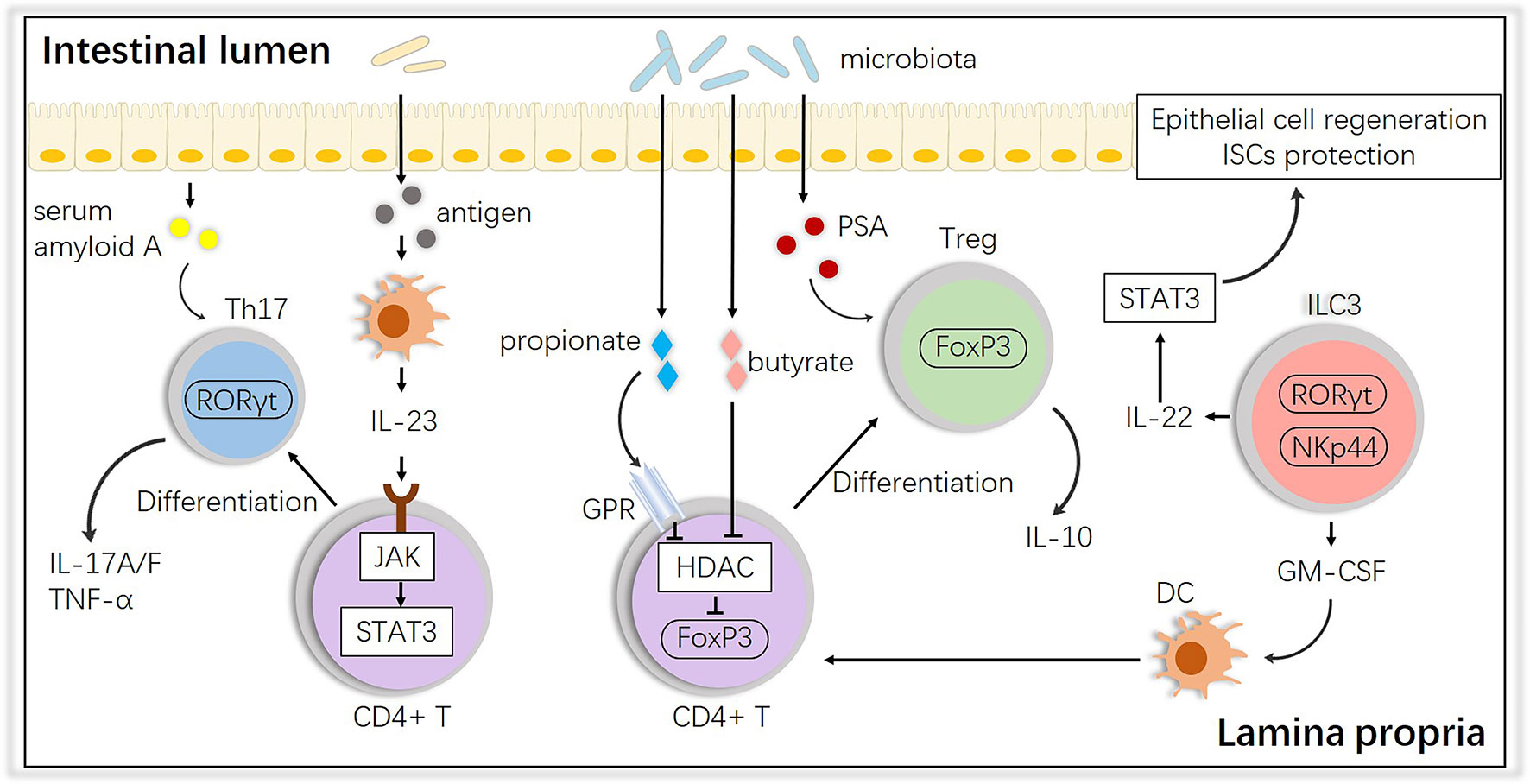
Figure 2 Impact of the intestinal microbiota on T cell subsets and interactions with ILC3s. The intestinal microbiota influences the differentiation of T cells into anti-inflammatory Tregs or proinflammatory Th17 cells, and ILCs play an important role in this process. PSA, polysaccharide A; GM-CSF, granulocyte macrophage colony-stimulating factor; HDAC, histone deacetylase; GPR, G protein receptor.
The third step links cytokine storms and inflammatory amplification, which induce direct damage and establish typical aGVHD injury. Damage to the intestine plays a central role in amplifying systemic GVHD by propagating a proinflammatory cytokine milieu (66). Moreover, the many combinations of cytokines (e.g., TNF, INF-γ, IL-1, IL-2, and IL-17) and costimulatory networks at the T cell surface are definitely complex, in addition to numerous products produced by the intestinal microbiota. The role of the intestinal microbiota in regulating cytokines has been elucidated in some previous studies. Atarashi et al. showed that on the basis of high potency in enhancing Treg abundance and inducing anti-inflammatory molecules, 17 rationally selected strains of Clostridia result the increase of IL-10 in the gut (67). Another study reported that increased abundance of Enterobacteriaceae is positively correlated with IL-17A aggravating aGVHD (68).
In addition to the abovementioned mechanisms, the pathogenesis of aGVHD involves many other specific mechanisms that require deeper investigation. However, it is increasingly clear that the intestinal microbiota indeed participates in the initiation and development of aGVHD.
Metabolites
The intestinal microbiota generates a wide range of bioactive metabolites serving as mediators and has pervasive consequences in aGVHD; modifications in bacteria-derived metabolites may be a new perspective regarding this disease (69).
Short-chain fatty acids (SCFAs) are one of the major microbial-derived metabolites found exclusively in the intestinal tract, which function in maintaining the epithelial barrier and colonocyte survival as well as play a diverse array of immune regulatory roles (70, 71). It has been reported that butyrate, one of the three main SCFAs, has a protective effect against aGVHD in murine models; butyrate restoration improved histone acetylation and IEC junctional integrity and decreased IEC apoptosis, ultimately mitigating aGVHD (72–74).
3-Indoxyl sulfate (3-IS), another promising metabolite analyzed in aGVHD, is a tryptophan metabolite of commensal colonic bacteria that has been identified as an indirect marker of a balanced microbiota and predicts the outcome of allo-HSCT (75, 76). Moreover, studies have also shown that gut tryptophan-produced indole metabolites reduce GVHD severity via type I interferon (IFN I) (77).
Respiratory metabolites may hold potential as surrogate markers for aGVHD (78–80). Various microbiota constituents are known to produce volatile metabolites, and volatile organic compounds (VOCs) generated during pathologic processes have been reported monitored in diseases such as obesity (81), hepatitis (82) and IBD (83). More recently, Hamilton et al. analyzed the VOCs of patients with and without aGVHD and correctly classified 89% (17 of 19) and 90% (9 of 10) of them, respectively, showing that breath analysis is a feasible and promising noninvasive method to detect and potentially monitor aGVHD (84).
Choline, phosphatidylcholine and carnitine-containing dietary ingredients can be metabolized into trimethylamine (TMA) and subsequently converted into trimethylamine N-oxide (TMAO), which could induce vascular inflammation and endothelial dysfunction (85). Dietary habit such as high-choline diet producing high level of TMAO alters distinct quality and quantity of gut microbiota which might affect microbial metabolites and GVHD severity. The latest research further explored TMAO in aGVHD and found that TMAO enhanced the allogenic GVH reaction. In an animal model, the group stimulated with TMAO showed a worse survival rate, higher GVHD scores and more damage to target tissues, which resulted from Th1 and Th17 differentiation (86).
These studies innovatively provide the link between microbiota-derived metabolites and aGVHD, which sheds light on alleviating aGVHD by controlling metabolism.
Loss of Microbiota Diversity in aGVHD
Views about the role of the microbiota in aGVHD are separated into two roles: a direct role and an indirect role. The former considers that the intestinal microbiota directly promotes tissue injury or inflammation after allo-HSCT, and animals possess normal immunocompetence but abnormal microbiota community compositions, so eliminating this proinflammatory factor by antimicrobial treatment should protect the recipient from aGVHD (87–89). The latter considers the intestinal microbiota to play an indirect role, as animals raised in germ-free conditions have abnormal development with highly aberrant immunity, and this kind of animal does not possess a prerequisite immune ability to develop aGVHD (90–92). Some researchers even support that both direct and indirect effects may occur simultaneously (93). Furthermore, we can identify some common alterations or differences from animal experiments and clinical studies.
Animal Studies
Earlier transplantation studies on animals in the 1970s focused on manifestations such as better survival and lower mortality with the management of the intestinal microbiota (94, 95). Subsequently, protection from GVHD in germ-free animals was confirmed in the 1980s (87), including xenogeneic transplantation that typically abrogates severe GVHD (88, 89). Antibiotic-mediated gut decontamination in mice (96) and dogs (97) showed that both microbes and aberrant immune development most proximately affect the development of GVHD. However, few animal studies have measured the impact of antibiotic treatment on microbiota composition or linked specific bacterial species to GVHD at this time.
In the early 21st century, advances in technology advanced research on the alterations (Table 2). Shono et al. found that piperacillin-tazobactam and imipenem-cilastatin therapy led to distinct patterns of gut microbiota composition in GVHD mice, with an increasing abundance of an Akkermansia strain, a bacterium with mucus-degrading capabilities, which raises the possibility that mucus degradation may contribute to murine GVHD (102). At the phylum level, the proportions of Firmicutes and Bacteroidetes, two of the major enteric commensals, were found to be decreased in GVHD mice, while the abundance of E. coli at the genus level was higher, which could be due to the development of systemic infection (100, 101). Furthermore, for Clostridiales and Lactobacillales, at the order level of Firmicutes, diversity analysis showed loss of the former but expansion of the latter, while eliminating Lactobacillales from mice before transplantation aggravated GVHD, and reintroducing the predominant Lactobacillus species showed a significant protective effect (99). Similar to these results, a report showed that the acute phase of GVHD was characterized by a shift toward the Enterobacteriaceae family. Bacteroides and Enterococcus abundances increased during GVHD, whereas Clostridia, Bifidobacteria and Bacillus were less abundant, but in this report, Lactobacillus abundance was decreased in GVHD (98), Moreover, subsequent studies also presented contrary results that the abundances of Lactobacillus and another uncultured bacterium from Firmicutes increased while the abundances of E. coli and another uncultured bacterium from Bacteroidetes decreased in allogeneic immunoglobulin yolk (IgY)-treated GVHD animals (103). However, the exact mechanisms of these alterations remain unclear, although several mechanisms have been proposed in earlier publications, including agglutination, opsonization, and toxin neutralization (106, 107).
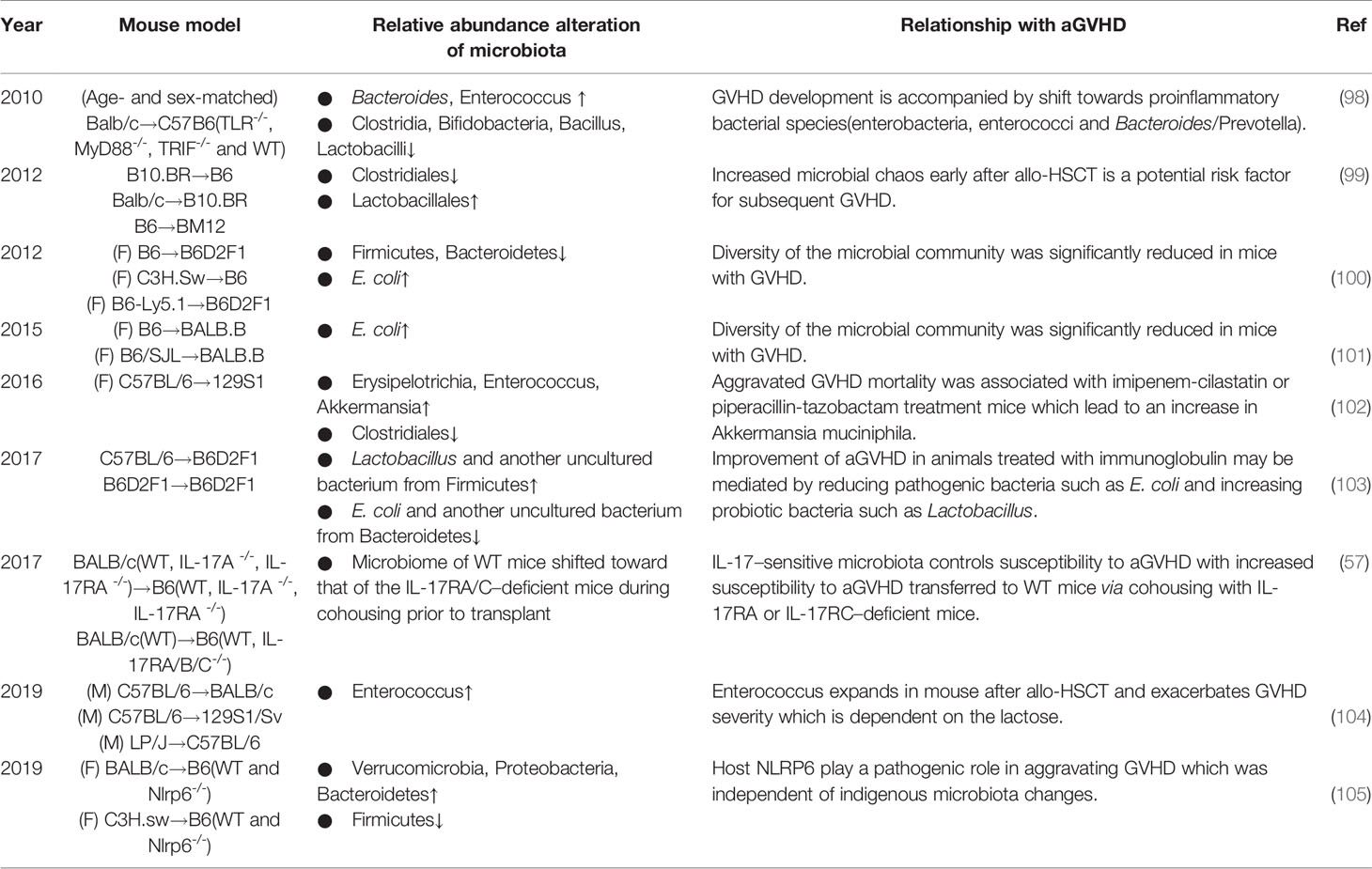
Table 2 Summary of nearly 10 years of experimental studies investigating the association between alterations in the intestinal microbiota and aGVHD.
Collectively, it is evident that not all bacteria have the same effect on GVHD, the composition of bacterial species and their function matter. The perturbations in the diversity and function of bacterial species may be caused by animal strains or research environment. However, one special bacterial species seems to be adverse in GVHD. Enterococci, gram-positive, facultative, anaerobic bacteria, occur in low abundance in the healthy host gut, with reports showing that Enterococcus expansion is associated with increased bloodstream infection and mortality in HSCT (108, 109). The data mentioned above (98) also revealed an increase in enterococci in the development of GVHD. In a recent study (104), in line with previous suspicion, enterococci similarly expanded and dominated the microbiota after allo-HSCT in GVHD mice; lactose drove this growth, and a lactose-free diet attenuated enterococcal expansion and T cell–driven inflammation in GVHD. Indeed, fecal domination by specific translocation of pathogenic bacteria, such as Enterococcus, is a significant risk factor for the development of aGVHD and increased overall GVHD-related mortality. Some easily overlooked factors, such as dietary elements, may play important roles in the progression, which should be closely managed.
The development of biogenetics has led to a focus on specific gene sites. For example, host NOD-like receptor family pyrin domain-containing 6 (NLRP6) regulates microbiota-dependent protection in intestinal colitis and tumorigenesis (110–113), but Tomomi et al. found an inverse effect in GVHD, in which host NLRP6 played a pathogenic role in aggravating intestinal damage (105). Interestingly, this influence was independent of indigenous microbiota changes. Toll-like receptors (TLRs), which sense bacterial lipoproteins and LPS and DNA, are suspected to modulate innate immune responses, and a major role of TLR9-mediated sensing of bacterial DNA in the aggravation of GVHD has been reported (114–116). With gene knockout, Markus et al. similarly proved that bacterial innate immune receptor TLR-/- mice showed significantly reduced GVHD mortality, which was further confirmed by less pronounced GVHD scores over time (98).
Furthermore, some groups have demonstrated substantial differences between the gut microbiota of mice purchased from different commercial vendors or repositories (117). There are clear differences in microbiota composition and diversity between sexes in previous studies (118, 119). In addition, Floris et al. showed that BALB/c mice had higher abundance and diversity of immunoglobulin A (IgAs) than C57BL/6 mice which is correlated with increased microbiota diversity (120). And aging alters the gut microbiota in mice, in which aged microbiome leads to an exaggerated systemic inflammatory response and reduced levels of SCFAs in young mice (121). In conclusion, gut microbiota composition can be influenced by housing, gender, host genetic, age, et al, and further studies are needed to determine the impact of different murine models and strains on aGVHD.
Human Studies
Early in the 1980s, in a clinical report of 130 patients with aplastic anemia undergoing allo-HSCT, gut decontamination and laminar airflow isolation were shown to lower the incidence of aGVHD (122). However, subsequent clinical research of human aGVHD has demonstrated that loss of intestinal microbiota diversity is associated with aGVHD, as microbiota disruption characterized by expansions of potentially pathogenic bacteria and reduction in alpha diversity (a variable that reflects the number of unique bacterial taxa present and their relative frequencies) have been reported. Some advanced clinical studies performed in recent years may further provide us with a better understanding of these alterations (Table 3).
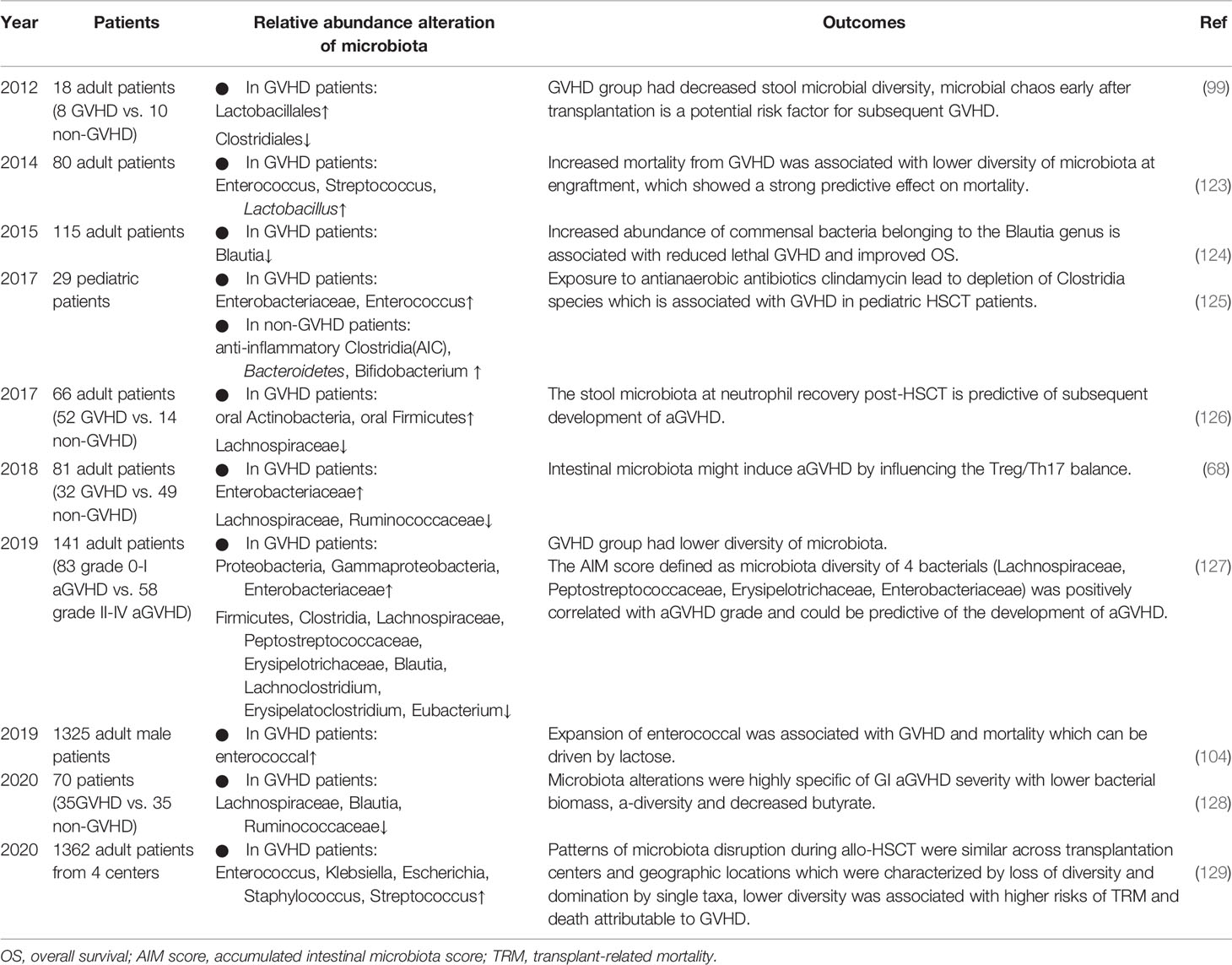
Table 3 Summary of human studies investigating the association between the microbiota and GVHD in the past 10 years.
In 2012, Jenq et al. studied changes in the microbiota of patients undergoing allo-HSCT and found that only the GVHD group had decreased microbial diversity with increased Lactobacillales abundance and loss of Clostridiales, suggesting that shifts in diversity were a result of GVHD rather than allo-HSCT or antibiotic exposure (99). In 2014, Taur Y et al. found that loss of bacterial diversity in stool specimens was associated with increased mortality from GVHD, and survival at 3 years after allo-HSCT was 36%, 60%, and 67% for patients with low, intermediate, and high microbiota diversity, respectively (123). However, patients in this study received T cell-depleted grafts, which could also reduce GVHD. Jenq et al. further investigated 64 allo-HSCT recipients of T cell–replete grafts and found that a higher abundance of Blautia was associated with a reduced risk of GVHD-related mortality and increased overall survival (124). Blautia is a genus that belongs to the class Clostridia. As reported in previous studies, Clostridiales rescue intestinal epithelial cell damage by upregulating Treg cells through the production of the SCFA butyrate (59, 67, 130), and alteration of the indigenous microbiota with 17 rationally selected strains of high butyrate-producing Clostridia led to decreased GVHD (74). Consistent with these findings, in 2017, Simms et al. reported a significant decline in anti-inflammatory Clostridia in pediatric patients with aGVHD (125). Therefore, it can be speculated that some microbial taxa, such as Blautia, are beneficial for the outcomes of HSCT and mitigation of aGVHD, as they behave as drivers in this process, which should be protected and used in a probiotic approach. By contrast, the Enterococcus genus from Lactobacillales contributes to inflammation, whose role in human aGVHD is the same as that in animals, as expansion of Enterococcus association with increased GVHD in humans has been reported (104, 131). Lactobacillus, another genus of Lactobacillales, showed a possible protective effect in human GVHD (99). In 2018, Lijie Han et al. found that GVHD patients showed a higher abundance of Proteobacteria and a lower abundance of Clostridia, which was correlated with the Treg/Th17 ratio and H3 acetylation, indicating an interaction among alterations in the microbiota, allogenic T cell activation and histone acetylation (68). One year later, this team (127) proved again that in aGVHD, the diversity of the microbiota was significantly lower, with decreases in Clostridia, Lachnospiraceae, Blautia, Eubacterium, and Erysipelatoclostridium abundance and increases in Enterobacteriaceae abundance. This finding was consistent with a study in 2017 showing a persistent lack of Lachnospiraceae and Bacteroidaceae species in GVHD patients, whereas Lachnospiraceae was negatively correlated with neutrophil recovery (126). In addition, the specific actors in the intestinal ecosystem involved in the pathologic process of aGVHD have been explored more recently (128). Shown in stool samples, microbiota alterations were highly specific to gastrointestinal aGVHD severity, and a negative correlation was observed with the Lachnospiraceae, especially the Blautia genus, and Ruminococcaceae families. On the other hand, geographic variations matter, while a recent study analyzing 8767 fecal samples from 1362 patients with allo-HSCT at 4 different centers likewise showed a similar association between lower intestinal diversity and higher risks of transplantation-related death and death attributable to GVHD (129).
From these data, we conclude that restoring intestinal microbiota diversity after allo-HSCT is beneficial in the clinic, protective indigenous probiotics should be preserved to balance the alteration of intestinal microbiota community for patients.
Clinical Interventions and Value of the Intestinal Microbiota in aGVHD
Diet
Certain diets may contribute to the development of GVHD given that food is one of the most important factors affecting the composition of the intestinal microbiota (132). One example is a choline diet, and a murine model has shown that a high-choline diet enhances the allogenic GVH reaction, which leads to more aGVHD (86). The effect of parenteral or enteral nutrition on the intestinal ecosystem during HSCT has also been evaluated, with preference toward the latter choice, as enteral feeding has been shown to protect against GVHD in several studies (133–135), while parenteral nutrition was associated with poor outcomes and other complications (136, 137). The type of oral nutrition may be another important factor. Recently, elaborate foods known as a neutropenic diet for allo-HSCT patients have been reexamined, and advanced evidence has shown limited benefit and even potentially harm of supplying aGVHD patients with neutropenic diets (138, 139).
Prebiotics and Probiotics
Intervention in the intestinal microbiota with a nutritional approach including prebiotics and probiotics may be another promising treatment option for aGVHD.
Prebiotics are indigestible compounds, usually indigestible carbohydrates, that bacteria have an advantage in metabolizing, resulting in the production of SCFAs and metabolites with a potential immunomodulatory role (140, 141). Strategies have been studied in the setting of aGVHD to modulate the intestinal microbiota by supplementation with inulin, oligosaccharides, galacto-oligosaccharides, and potato starch, showing beneficial results. In a recent study, Yoshifuji et al. found that intake of resistant starch and GFO (glutamine, fiber, and oligosaccharide) shortened the duration of oral mucositis and diarrhea and reduced the incidence and severity of aGVHD (142). Other clinical trials focused on fructooligosaccharide, potato-based starch, and gluten-free diets are currently being studied for potential benefit (143).
Probiotics are ingestible formulations of live bacteria that can modulate intestinal homeostasis. A probiotic strategy achieved by FMT consists of introducing one strain or selected strains of microorganisms that confer a benefit. An early report showed that a probiotic-rich diet prior to HSCT is associated with earlier neutrophil engraftment and a shorter duration of febrile neutropenia (144). Some probiotics were found to be safe to administer during aGVHD, such as Lactobacillus plantarum reported in pediatric patients (145) and Lactobacillus rhamnosus GG in murine models (146). However, controversies exist. Recently, there have been some concerns regarding the safety of administering living microorganisms to immunocompromised patients with altered gut permeability, as some clinical cases have demonstrated sepsis (147), bacteremia (148) and meningitis (149) after treatment of pediatric patients.
Antibiotics
Given that the intestinal microbiota critically affects transplant outcomes, correctly managing the influence of the microbiota in GVHD—antibiotics has been encouraged. The advent of techniques to generate and maintain germ-free rodents since the 1940s made it possible to examine the microbiota in animals (123), and subsequent studies demonstrated the benefits of using antibiotics. Bekkum and Jones et al. found that germ-free mice housed in sterile conditions or mice treated with antibiotics developed less severe aGVHD (94, 95). Vossen et al. showed that in a cohort of 112 pediatric patients, recipients treated with total gastrointestinal decontamination (GID) using high doses of nonabsorbable antibiotics prevented moderate-to-severe aGVHD, suggesting that the translocation of luminal bacteria and their cell wall-derived compounds might be inhibited during total GID (150). Weber et al. analyzed 394 patients receiving allo-HSCT and found that the treatment of rifaximin correlated with lower enterococcal positivity and higher urinary 3-indoxyl sulfate concentrations. Patients on rifaximin showed lower 1-year transplant-related mortality and higher overall survival. Infectious complications with systemic antibiotic use did not abrogate the beneficial effects of rifaximin on intestinal microbiota composition in the early course of allo-HSCT or outcome (151).
However, the use of gut-decontamination prophylactic antibiotics seems to be a doubled-edged sword. A retrospective analysis in 2018 mentioned above (68) proved that β-lactam antibiotic administration was an independent risk factor for aGVHD according to the Cox regression model for multivariate analysis of aGVHD. Although showing no significant adverse effect, in an allo-BMT murine model, treatment of both donor mice with broad-spectrum antibiotics and control SPF donor mice induced GVHD mortality at a similar rate, and reducing and altering the microbial flora in the donors had no effect on their T cell alloreactivity and induction of GVHD after allogeneic BMT (152). In human studies, the concept that gut decontamination prevents aGVHD is controversial given that some subsequent clinical trials have failed to demonstrate consistent benefits (68, 153–155). Prophylactic use of antibiotics is reported to be associated with more severe aGVHD that involves the intestinal tract and liver, impacting 1-y and 2-y overall survival (OS) in patients receiving myeloablative regimens (155). Earlier antibiotic treatment in patients prior to allo-HSCT was further associated with higher transplant-related mortality than no antibiotics (76). A potential role for anaerobic bacteria, in particular Clostridiales, was supported that Blautia abundance was inversely correlated with the risk of developing gastrointestinal GVHD (124), while clindamycin, an anti-anaerobic bacterial agent, increased the risk of GVHD by depleting anti-inflammatory clostridia (125). Reported previously, piperacillin-tazobactam and imipenem-cilastatin increased the incidence, severity, and mortality of GVHD, and piperacillin-tazobactam reduced Bacteroidetes and Lactobacillus abundance (102). Subsequent evidence further supports the adverse role of anti-anaerobic bacterial penicillin derivatives and carbapenems, as both are associated with a higher incidence of GVHD (156). Although seemingly negative, these findings may enlighten us that antibiotics preserving anaerobic bacteria may potentially reduce the risk of developing gut GVHD.
In conclusion, it is obvious that there remain many controversies for GVHD patients to undergo antibiotic therapy, which needs further exploration. Thus, it is of great necessity to identify new strategies to maintain the diversity and richness of the intestinal microbiota. Different attempts have been made in clinical practice involving narrow-spectrum antibiotics and modulating the timing and duration of treatment.
FMT
Loss of microbiota diversity creates an opportunity to intervene in aGVHD by reestablishing diversity using microbes that modulate inflammation. FMT has been investigated as a potential therapeutic strategy for gut GVHD in both preventive and therapeutic strategies in recent years. The recent insight of FMT considers it the ‘ultimate probiotic’ for GVHD in allo-HSCT because it directly modifies the host’s intestinal microbiome composition to restore eubiosis and gut homeostasis (157). Indeed, according to some previous reports, FMT significantly improves the outcome of GVHD. Kakihana et al. reported that 4 patients received FMT 92 days after HSCT, all patients responded to FMT within several days, with 3 complete responses and 1 partial response, and an increase in peripheral effector regulatory T cells during the response to FMT was also observed (158). Similarly, another study reported that two of three patients achieved a complete response with multiple FMTs, while the other obtained a partial response; the FMT response was correlated with increased microbial diversity and richness (159). More recent reports further illustrate the role of FMT in GVHD, with promising results (160–162). Currently, a number of ongoing clinical trials to investigate the role of FMT in preventing intestinal GVHD following allo-HSCT, summarized in Table 4, are being carried out to try to find the best treatment protocol.
Although many reports have shown that FMT is a safe and effective strategy used in different situations to modulate the microbiota and cure aGVHD, it should be noted that patients are usually immunocompromised with altered intestinal permeability, as infectious complications after FMT have been reported in other settings at the same time (163, 164). Moreover, the evidence mentioned above suggests a beneficial role of FMT in GVHD and needs to be confirmed in larger studies. The optimization of all practical aspects of FMT still needs to be addressed in the future.
Predictive Marker
As reported in the current literature, a reduction in microbiota diversity and alteration of metabolites can predict transplantation outcomes and aGVHD, shedding light on the value of the microbiota. In 2015, Weber et al. detected 3-indoxyl sulfate (3-IS) in urine specimens within the first 28 days after allo-HSCT in 131 patients and found that a low level of 3-IS within the first 10 days was associated with significantly higher transplant-related mortality and worse overall survival 1 year after allo-HSCT. Furthermore, the class Bacilli was proven to be associated with low 3-IS levels (75). In 2017, Golob et al. showed that a gradient of 20 types of bacterial species could predict severe aGVHD by calculating a gradient of the sum of the relative abundance of positively correlated bacteria minus the sum of the relative abundance of negative correlates (126). Similarly, a study in 2019 by Lijie Han et al. showed that microbiota diversity combined with the gradients of 4 bacteria (Peptostreptococcaceae, Erysipelotrichaceae, Enterobacteriaceae, and Lachnospiraceae) can predict the development and severity of aGVHD (127). Geographic variations in the composition of human microbial communities and differences in clinical practices across institutions raise the question of whether relationships between microbiota composition and clinical outcomes after allo-HSCT are generalizable. In 2020, Peled et al. conducted a study with data from four clinical research institutions by comparing risk scores from regularized Cox regression with cross-validation; they showed that not only a diversity metric but also a signature of specific bacterial abundances was informative about the risk of death after allo-HSCT across institutions (129). Similarly, another study analyzing data from stool and blood samples of 150 patients from two centers who underwent allo-HSCT also showed that gut microbiota score (GMS) from a LASSO (least absolute shrinkage and selection operator) model at neutrophil engraftment could predict aGVHD (165).
Thus, studies on the microbiota as a predictive marker for aGVHD are worth further exploration to provide assistance with the currently available tools for predicting the development of aGVHD.
Conclusion and Prospects
Alteration of the intestinal microbiota is a corollary given that the gut is an aGVHD target organ. Although we could further explore the intestinal microbiota by better dissecting compositional and functional microbiota structures, most of the research has concentrated on characterization, with data analyzed only via correlation. In this regard, determining which specific bacterial taxa are the main taxa affecting aGVHD is of great urgency and importance. In addition to descriptive investigations, mechanistic investigations are needed, which will help translate these associations into therapies for aGVHD if the microbiota is causal.
New approaches to prevent and cure aGVHD remain an unmet need that can be best addressed by understanding the complex pathophysiology, with increasing evidence indicating that the intestinal microbiota indeed participates in this process. Future studies are essential to explore further the role of the intestinal microbiota in aGVHD to elucidate the real impact of microbiota ecology. A promising approach may involve altering certain microbiota species by more precise and safer methods, which may consist of diet, prebiotics, probiotics, advanced antibiotic therapies, FMT, and microbiota metabolism analyses (Figure 3). Fully understanding the mechanism by which loss of microbiota diversity influences specific molecules and pathways and regulates the pathogenesis and development of aGVHD remains a top concern. Only by attempting to find better prophylactic and therapeutic schemes for allo-HSCT complications can we focus on what truly matters, which is also the appeal and value of the Human Microbiome Project and precision medicine.
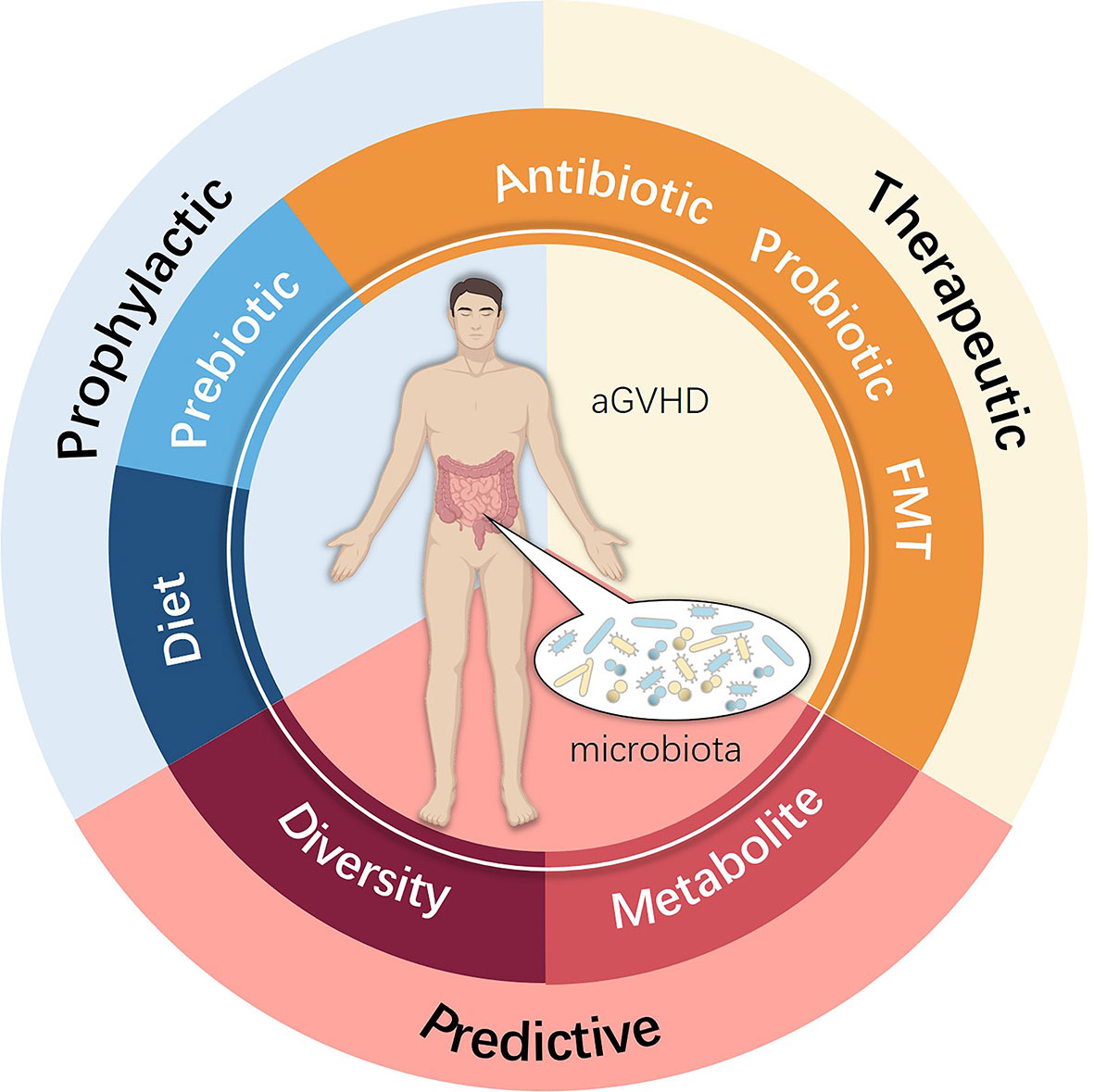
Figure 3 Potential clinical intervention associated with the intestinal microbiota used for preventing, treating and predicting aGVHD.
Author Contributions
The manuscript was conceptualized by XZ and QG. TH wrote the majority of the manuscript. RW and XW co-wrote the manuscript. The figures were designed by TH and drawn by XW and SY. WW, RW, and TH summarized the tables. All authors contributed to the article and approved the submitted version.
Funding
This work was supported by The 2020 Open Project (Key Project) of the National Center for Clinical Medicine Research on Hematological System Diseases (Grant No. 2020ZKZC02), National Key Research and Development Plan “Stem Cell and Transformation Research” Key Special Project (grant no. 2017YFA0105502), and Science and Health Joint Project of Chongqing (grant no. 2018QNXM015).
Conflict of Interest
The authors declare that the research was conducted in the absence of any commercial or financial relationships that could be construed as a potential conflict of interest.
References
1. Ferrara JLM, Levine JE, Reddy P, Holler E. Graft-versus-host disease. Lancet (2009) 373(9674):1550–61. doi: 10.1016/S0140-6736(09)60237-3
2. Blazar BR, Murphy WJ, Abedi M. Advances in graft-versus-host disease biology and therapy. Nat Rev Immunol (2012) 12(6):443–58. doi: 10.1038/nri3212
3. Ramachandran V, Kolli SS, Strowd LC. Review of Graft-Versus-Host Disease. Dermatol Clin (2019) 37(4):569–82. doi: 10.1016/j.det.2019.05.014
4. Jagasia M, Arora M, Flowers ME, Chao NJ, McCarthy PL, Cutler CS, et al. Risk factors for acute GVHD and survival after hematopoietic cell transplantation. Blood (2012) 119(1):296–307. doi: 10.1182/blood-2011-06-364265
5. Atkinson K, Horowitz MM, Gale RP, van Bekkum DW, Gluckman E, Good RA, et al. Risk factors for chronic graft-versus-host disease after HLA-identical sibling bone marrow transplantation. Blood (1990) 75(12):2459–64. doi: 10.1182/blood.V75.12.2459.bloodjournal75122459
6. Zahid MF, Lazarus HM, Ringden O, Barrett JA, Gale RP, Hashmi SK. Can we prevent or treat graft-versus-host disease with cellular-therapy? Blood Rev (2020) 43:100669. doi: 10.1016/j.blre.2020.100669
7. Sung AD, Chao NJ. Concise review: acute graft-versus-host disease: immunobiology, prevention, and treatment. Stem Cells Transl Med (2013) 2(1):25–32. doi: 10.5966/sctm.2012-0115
8. Barton-Burke M, Dwinell DM, Kafkas L, Lavalley C, Sands H, Proctor C, et al. Graft-versus-host disease: a complex long-term side effect of hematopoietic stem cell transplant. Oncol (Williston Park) (2008) 22(11 Suppl Nurse Ed):31–45.
9. Hashmi S, Ahmed M, Murad MH, Litzow MR, Adams RH, Ball LM, et al. Survival after mesenchymal stromal cell therapy in steroid-refractory acute graft-versus-host disease: systematic review and meta-analysis. Lancet Haematol (2016) 3(1):e45–52. doi: 10.1016/S2352-3026(15)00224-0
10. Martin PJ, Inamoto Y, Flowers ME, Carpenter PA. Secondary treatment of acute graft-versus-host disease: a critical review. Biol Blood Marrow Transpl (2012) 18(7):982–8. doi: 10.1016/j.bbmt.2012.04.006
11. Schroeder MA, Khoury HJ, Jagasia M, Ali H, Schiller GJ, Staser K, et al. A phase 1 trial of itacitinib, a selective JAK1 inhibitor, in patients with acute graft-versus-host disease. Blood Adv (2020) 4(8):1656–69. doi: 10.1182/bloodadvances.2019001043
12. Malard F, Huang XJ, Sim JPY. Treatment and unmet needs in steroid-refractory acute graft-versus-host disease. Leukemia (2020) 34(5):1229–40. doi: 10.1038/s41375-020-0804-2
13. Penack O, Marchetti M, Ruutu T, Aljurf M, Bacigalupo A, Bonifazi F, et al. Prophylaxis and management of graft versus host disease after stem-cell transplantation for haematological malignancies: updated consensus recommendations of the European Society for Blood and Marrow Transplantation. Lancet Haematol (2020) 7(2):e157–e67. doi: 10.1016/S2352-3026(19)30256-X
14. Maynard CL, Elson CO, Hatton RD, Weaver CT. Reciprocal interactions of the intestinal microbiota and immune system. Nature (2012) 489(7415):231–41. doi: 10.1038/nature11551
15. Turnbaugh PJ, Ley RE, Mahowald MA, Magrini V, Mardis ER, Gordon JI. An obesity-associated gut microbiome with increased capacity for energy harvest. Nature (2006) 444(7122):1027–31. doi: 10.1038/nature05414
16. Suez J, Korem T, Zeevi D, Zilberman-Schapira G, Thaiss CA, Maza O, et al. Artificial sweeteners induce glucose intolerance by altering the gut microbiota. Nature (2014) 514(7521):181–6. doi: 10.1038/nature13793
17. Collins SM. A role for the gut microbiota in IBS. Nat Rev Gastroenterol Hepatol (2014) 11(8):497–505. doi: 10.1038/nrgastro.2014.40
18. Hsiao EY, McBride SW, Hsien S, Sharon G, Hyde ER, McCue T, et al. Microbiota modulate behavioral and physiological abnormalities associated with neurodevelopmental disorders. Cell (2013) 155(7):1451–63. doi: 10.1016/j.cell.2013.11.024
19. Luissint AC, Parkos CA, Nusrat A. Inflammation and the Intestinal Barrier: Leukocyte-Epithelial Cell Interactions, Cell Junction Remodeling, and Mucosal Repair. Gastroenterology (2016) 151(4):616–32. doi: 10.1053/j.gastro.2016.07.008
20. Peled JU, Hanash AM, Jenq RR. Role of the intestinal mucosa in acute gastrointestinal GVHD. Blood (2016) 128(20):2395–402. doi: 10.1182/blood-2016-06-716738
21. Shono Y, van den Brink MRM. Gut microbiota injury in allogeneic haematopoietic stem cell transplantation. Nat Rev Cancer (2018) 18(5):283–95. doi: 10.1038/nrc.2018.10
22. Turnbaugh PJ, Ley RE, Hamady M, Fraser-Liggett CM, Knight R, Gordon JI. The human microbiome project. Nature (2007) 449(7164):804–10. doi: 10.1038/nature06244
23. Murphy S, Nguyen VH. Role of gut microbiota in graft-versus-host disease. Leuk Lymphoma (2011) 52(10):1844–56. doi: 10.3109/10428194.2011.580476
24. Ott SJ, Kuhbacher T, Musfeldt M, Rosenstiel P, Hellmig S, Rehman A, et al. Fungi and inflammatory bowel diseases: Alterations of composition and diversity. Scand J Gastroenterol (2008) 43(7):831–41. doi: 10.1080/00365520801935434
25. Qin J, Li R, Raes J, Arumugam M, Burgdorf KS, Manichanh C, et al. A human gut microbial gene catalogue established by metagenomic sequencing. Nature (2010) 464(7285):59–65. doi: 10.1038/nature08821
26. Reyes A, Haynes M, Hanson N, Angly FE, Heath AC, Rohwer F, et al. Viruses in the faecal microbiota of monozygotic twins and their mothers. Nature (2010) 466(7304):334–8. doi: 10.1038/nature09199
27. Shono Y, Docampo MD, Peled JU, Perobelli SM, Jenq RR. Intestinal microbiota-related effects on graft-versus-host disease. Int J Hematol (2015) 101(5):428–37. doi: 10.1007/s12185-015-1781-5
28. Rodriguez J, Hiel S, Neyrinck AM, Le Roy T, Potgens SA, Leyrolle Q, et al. Discovery of the gut microbial signature driving the efficacy of prebiotic intervention in obese patients. Gut (2020) 69(11):1975–87. doi: 10.1136/gutjnl-2019-319726
29. Thingholm LB, Ruhlemann MC, Koch M, Fuqua B, Laucke G, Boehm R, et al. Obese Individuals with and without Type 2 Diabetes Show Different Gut Microbial Functional Capacity and Composition. Cell Host Microbe (2019) 26(2):252–64 e10. doi: 10.1016/j.chom.2019.07.004
30. Leiva-Gea I, Sanchez-Alcoholado L, Martin-Tejedor B, Castellano-Castillo D, Moreno-Indias I, Urda-Cardona A, et al. Gut Microbiota Differs in Composition and Functionality Between Children With Type 1 Diabetes and MODY2 and Healthy Control Subjects: A Case-Control Study. Diabetes Care (2018) 41(11):2385–95. doi: 10.2337/dc18-0253
31. Hod K, Ringel Y. Probiotics in functional bowel disorders. Best Pract Res Clin Gastroenterol (2016) 30(1):89–97. doi: 10.1016/j.bpg.2016.01.003
32. Strati F, Cavalieri D, Albanese D, De Felice C, Donati C, Hayek J, et al. New evidences on the altered gut microbiota in autism spectrum disorders. Microbiome (2017) 5(1):24. doi: 10.1186/s40168-017-0242-1
33. Pan H, Guo R, Ju Y, Wang Q, Zhu J, Xie Y, et al. A single bacterium restores the microbiome dysbiosis to protect bones from destruction in a rat model of rheumatoid arthritis. Microbiome (2019) 7(1):107. doi: 10.1186/s40168-019-0719-1
34. Kumari R, Palaniyandi S, Hildebrandt GC. Microbiome: An Emerging New Frontier in Graft-Versus-Host Disease. Dig Dis Sci (2019) 64(3):669–77. doi: 10.1007/s10620-018-5369-9
35. Wang WL, Xu SY, Ren ZG, Tao L, Jiang JW, Zheng SS. Application of metagenomics in the human gut microbiome. World J Gastroenterol (2015) 21(3):803–14. doi: 10.3748/wjg.v21.i3.803
36. Morgan XC, Huttenhower C. Meta’omic analytic techniques for studying the intestinal microbiome. Gastroenterology (2014) 146(6):1437–48.e1. doi: 10.1053/j.gastro.2014.01.049
37. Leibowitz BJ, Wei L, Zhang L, Ping X, Epperly M, Greenberger J, et al. Ionizing irradiation induces acute haematopoietic syndrome and gastrointestinal syndrome independently in mice. Nat Commun (2014) 5:3494. doi: 10.1038/ncomms4494
38. Ch’ang HJ, Maj JG, Paris F, Xing HR, Zhang J, Truman JP, et al. ATM regulates target switching to escalating doses of radiation in the intestines. Nat Med (2005) 11(5):484–90. doi: 10.1038/nm1237
39. Powell DW, Pinchuk IV, Saada JI, Chen X, Mifflin RC. Mesenchymal cells of the intestinal lamina propria. Annu Rev Physiol (2011) 73:213–37. doi: 10.1146/annurev.physiol.70.113006.100646
40. Wang X, Wei L, Cramer JM, Leibowitz BJ, Judge C, Epperly M, et al. Pharmacologically blocking p53-dependent apoptosis protects intestinal stem cells and mice from radiation. Sci Rep (2015) 5:8566. doi: 10.1038/srep08566
41. Rannou E, Francois A, Toullec A, Guipaud O, Buard V, Tarlet G, et al. In vivo evidence for an endothelium-dependent mechanism in radiation-induced normal tissue injury. Sci Rep (2015) 5:15738. doi: 10.1038/srep15738
42. Epstein RJ, McDonald GB, Sale GE, Shulman HM, Thomas ED. The diagnostic accuracy of the rectal biopsy in acute graft-versus-host disease: a prospective study of thirteen patients. Gastroenterology (1980) 78(4):764–71. doi: 10.1016/0016-5085(80)90681-2
43. Wall AJ, Rosenberg JL, Reilly RW. Small intestinal injury in the immunologically runted mouse. Morphologic and autoradiographic studies. J Lab Clin Med (1971) 78(5):833–4.
44. Sale GE, Shulman HM, McDonald GB, Thomas ED. Gastrointestinal graft-versus-host disease in man. A clinicopathologic study of the rectal biopsy. Am J Surg Pathol (1979) 3(4):291–9. doi: 10.1097/00000478-197908000-00001
45. Penack O, Henke E, Suh D, King CG, Smith OM, Na IK, et al. Inhibition of neovascularization to simultaneously ameliorate graft-vs-host disease and decrease tumor growth. J Natl Cancer Inst (2010) 102(12):894–908. doi: 10.1093/jnci/djq172
46. Lai XY, Egan LJ. Suppression of radiation-induced DNA double-strand break repair by MyD88 is accompanied by apoptosis and crypt loss in mouse colon. Oncogenesis (2013) 2:e62. doi: 10.1038/oncsis.2013.22
47. Rakoff-Nahoum S, Paglino J, Eslami-Varzaneh F, Edberg S, Medzhitov R. Recognition of commensal microflora by toll-like receptors is required for intestinal homeostasis. Cell (2004) 118(2):229–41. doi: 10.1016/j.cell.2004.07.002
48. Beura LK, Hamilton SE, Bi K, Schenkel JM, Odumade OA, Casey KA, et al. Normalizing the environment recapitulates adult human immune traits in laboratory mice. Nature (2016) 532(7600):512–6. doi: 10.1038/nature17655
49. Berlin C, Bargatze RF, Campbell JJ, von Andrian UH, Szabo MC, Hasslen SR, et al. alpha 4 integrins mediate lymphocyte attachment and rolling under physiologic flow. Cell (1995) 80(3):413–22. doi: 10.1016/0092-8674(95)90491-3
50. Butcher EC, Picker LJ. Lymphocyte homing and homeostasis. Science (1996) 272(5258):60–6. doi: 10.1126/science.272.5258.60
51. Johansson-Lindbom B, Agace WW. Generation of gut-homing T cells and their localization to the small intestinal mucosa. Immunol Rev (2007) 215:226–42. doi: 10.1111/j.1600-065X.2006.00482.x
52. Masopust D, Schenkel JM. The integration of T cell migration, differentiation and function. Nat Rev Immunol (2013) 13(5):309–20. doi: 10.1038/nri3442
53. Mora JR, Bono MR, Manjunath N, Weninger W, Cavanagh LL, Rosemblatt M, et al. Selective imprinting of gut-homing T cells by Peyer’s patch dendritic cells. Nature (2003) 424(6944):88–93. doi: 10.1038/nature01726
54. Fu YY, Egorova A, Sobieski C, Kuttiyara J, Calafiore M, Takashima S, et al. T Cell Recruitment to the Intestinal Stem Cell Compartment Drives Immune-Mediated Intestinal Damage after Allogeneic Transplantation. Immunity (2019) 51(1):90–103 e3. doi: 10.1016/j.immuni.2019.06.003
55. Shih VF, Cox J, Kljavin NM, Dengler HS, Reichelt M, Kumar P, et al. Homeostatic IL-23 receptor signaling limits Th17 response through IL-22-mediated containment of commensal microbiota. Proc Natl Acad Sci U S A (2014) 111(38):13942–7. doi: 10.1073/pnas.1323852111
56. Sano T, Huang W, Hall JA, Yang Y, Chen A, Gavzy SJ, et al. An IL-23R/IL-22 Circuit Regulates Epithelial Serum Amyloid A to Promote Local Effector Th17 Responses. Cell (2015) 163(2):381–93. doi: 10.1016/j.cell.2015.08.061
57. Varelias A, Ormerod KL, Bunting MD, Koyama M, Gartlan KH, Kuns RD, et al. Acute graft-versus-host disease is regulated by an IL-17-sensitive microbiome. Blood (2017) 129(15):2172–85. doi: 10.1182/blood-2016-08-732628
58. Round JL, Mazmanian SK. Inducible Foxp3+ regulatory T-cell development by a commensal bacterium of the intestinal microbiota. Proc Natl Acad Sci U S A (2010) 107(27):12204–9. doi: 10.1073/pnas.0909122107
59. Furusawa Y, Obata Y, Fukuda S, Endo TA, Nakato G, Takahashi D, et al. Commensal microbe-derived butyrate induces the differentiation of colonic regulatory T cells. Nature (2013) 504(7480):446–50. doi: 10.1038/nature12721
60. Artis D, Spits H. The biology of innate lymphoid cells. Nature (2015) 517(7534):293–301. doi: 10.1038/nature14189
61. Cella M, Fuchs A, Vermi W, Facchetti F, Otero K, Lennerz JK, et al. A human natural killer cell subset provides an innate source of IL-22 for mucosal immunity. Nature (2009) 457(7230):722–5. doi: 10.1038/nature07537
62. Pickert G, Neufert C, Leppkes M, Zheng Y, Wittkopf N, Warntjen M, et al. STAT3 links IL-22 signaling in intestinal epithelial cells to mucosal wound healing. J Exp Med (2009) 206(7):1465–72. doi: 10.1084/jem.20082683
63. Hanash AM, Dudakov JA, Hua G, O’Connor MH, Young LF, Singer NV, et al. Interleukin-22 protects intestinal stem cells from immune-mediated tissue damage and regulates sensitivity to graft versus host disease. Immunity (2012) 37(2):339–50. doi: 10.1016/j.immuni.2012.05.028
64. Konya V, Mjosberg J. Innate lymphoid cells in graft-versus-host disease. Am J Transpl (2015) 15(11):2795–801. doi: 10.1111/ajt.13394
65. Mortha A, Chudnovskiy A, Hashimoto D, Bogunovic M, Spencer SP, Belkaid Y, et al. Microbiota-dependent crosstalk between macrophages and ILC3 promotes intestinal homeostasis. Science (2014) 343(6178):1249288. doi: 10.1126/science.1249288
66. Teshima T, Reddy P, Zeiser R. Acute Graft-versus-Host Disease: Novel Biological Insights. Biol Blood Marrow Transpl (2016) 22(1):11–6. doi: 10.1016/j.bbmt.2015.10.001
67. Atarashi K, Tanoue T, Oshima K, Suda W, Nagano Y, Nishikawa H, et al. Treg induction by a rationally selected mixture of Clostridia strains from the human microbiota. Nature (2013) 500(7461):232–6. doi: 10.1038/nature12331
68. Han L, Jin H, Zhou L, Zhang X, Fan Z, Dai M, et al. Intestinal Microbiota at Engraftment Influence Acute Graft-Versus-Host Disease via the Treg/Th17 Balance in Allo-HSCT Recipients. Front Immunol (2018) 9:669. doi: 10.3389/fimmu.2018.00669
69. Michonneau D, Latis E, Curis E, Dubouchet L, Ramamoorthy S, Ingram B, et al. Metabolomics analysis of human acute graft-versus-host disease reveals changes in host and microbiota-derived metabolites. Nat Commun (2019) 10(1):5695. doi: 10.1038/s41467-019-13498-3
70. Rios-Covian D, Ruas-Madiedo P, Margolles A, Gueimonde M, de Los Reyes-Gavilan CG, Salazar N. Intestinal Short Chain Fatty Acids and their Link with Diet and Human Health. Front Microbiol (2016) 7:185. doi: 10.3389/fmicb.2016.00185
71. Koh A, De Vadder F, Kovatcheva-Datchary P, Backhed F. From Dietary Fiber to Host Physiology: Short-Chain Fatty Acids as Key Bacterial Metabolites. Cell (2016) 165(6):1332–45. doi: 10.1016/j.cell.2016.05.041
72. Fujiwara H, Docampo MD, Riwes M, Peltier D, Toubai T, Henig I, et al. Microbial metabolite sensor GPR43 controls severity of experimental GVHD. Nat Commun (2018) 9(1):3674. doi: 10.1038/s41467-018-06048-w
73. Scott NA, Andrusaite A, Andersen P, Lawson M, Alcon-Giner C, Leclaire C, et al. Antibiotics induce sustained dysregulation of intestinal T cell immunity by perturbing macrophage homeostasis. Sci Transl Med (2018) 10(464). doi: 10.1126/scitranslmed.aao4755
74. Mathewson ND, Jenq R, Mathew AV, Koenigsknecht M, Hanash A, Toubai T, et al. Gut microbiome-derived metabolites modulate intestinal epithelial cell damage and mitigate graft-versus-host disease. Nat Immunol (2016) 17(5):505–13. doi: 10.1038/ni.3400
75. Weber D, Oefner PJ, Hiergeist A, Koestler J, Gessner A, Weber M, et al. Low urinary indoxyl sulfate levels early after transplantation reflect a disrupted microbiome and are associated with poor outcome. Blood (2015) 126(14):1723–8. doi: 10.1182/blood-2015-04-638858
76. Weber D, Jenq RR, Peled JU, Taur Y, Hiergeist A, Koestler J, et al. Microbiota Disruption Induced by Early Use of Broad-Spectrum Antibiotics Is an Independent Risk Factor of Outcome after Allogeneic Stem Cell Transplantation. Biol Blood Marrow Transpl (2017) 23(5):845–52. doi: 10.1016/j.bbmt.2017.02.006
77. Swimm A, Giver CR, DeFilipp Z, Rangaraju S, Sharma A, Ulezko Antonova A, et al. Indoles derived from intestinal microbiota act via type I interferon signaling to limit graft-versus-host disease. Blood (2018) 132(23):2506–19. doi: 10.1182/blood-2018-03-838193
78. De Vadder F, Kovatcheva-Datchary P, Goncalves D, Vinera J, Zitoun C, Duchampt A, et al. Microbiota-generated metabolites promote metabolic benefits via gut-brain neural circuits. Cell (2014) 156(1-2):84–96. doi: 10.1016/j.cell.2013.12.016
79. Koeth RA, Wang Z, Levison BS, Buffa JA, Org E, Sheehy BT, et al. Intestinal microbiota metabolism of L-carnitine, a nutrient in red meat, promotes atherosclerosis. Nat Med (2013) 19(5):576–85. doi: 10.1038/nm.3145
80. Rothhammer V, Quintana FJ. The aryl hydrocarbon receptor: an environmental sensor integrating immune responses in health and disease. Nat Rev Immunol (2019) 19(3):184–97. doi: 10.1038/s41577-019-0125-8
81. Alkhouri N, Eng K, Cikach F, Patel N, Yan C, Brindle A, et al. Breathprints of childhood obesity: changes in volatile organic compounds in obese children compared with lean controls. Pediatr Obes (2015) 10(1):23–9. doi: 10.1111/j.2047-6310.2014.221.x
82. Hanouneh IA, Zein NN, Cikach F, Dababneh L, Grove D, Alkhouri N, et al. The breathprints in patients with liver disease identify novel breath biomarkers in alcoholic hepatitis. Clin Gastroenterol Hepatol (2014) 12(3):516–23. doi: 10.1016/j.cgh.2013.08.048
83. Rieder F, Kurada S, Grove D, Cikach F, Lopez R, Patel N, et al. A Distinct Colon-Derived Breath Metabolome is Associated with Inflammatory Bowel Disease, but not its Complications. Clin Transl Gastroenterol (2016) 7(11):e201. doi: 10.1038/ctg.2016.57
84. Hamilton BK, Rybicki LA, Grove D, Ferraro C, Starn J, Hodgeman B, et al. Breath analysis in gastrointestinal graft-versus-host disease after allogeneic hematopoietic cell transplantation. Blood Adv (2019) 3(18):2732–7. doi: 10.1182/bloodadvances.2019000345
85. Chen ML, Zhu XH, Ran L, Lang HD, Yi L, Mi MT. Trimethylamine-N-Oxide Induces Vascular Inflammation by Activating the NLRP3 Inflammasome Through the SIRT3-SOD2-mtROS Signaling Pathway. J Am Heart Assoc (2017) 6(9). doi: 10.1161/JAHA.117.006347
86. Wu K, Yuan Y, Yu H, Dai X, Wang S, Sun Z, et al. Gut microbial metabolite trimethylamine N-oxide aggravates GVHD by inducing M1 macrophage polarization in mice. Blood (2020) 36(4):501–15. doi: 10.1182/blood.2019003990
87. Bealmear PM, Mirand EA, Holtermann OA. Modification of graft-vs-host disease following bone marrow transplantation in germfree mice. Prog Clin Biol Res (1983) 132C:409–21.
88. Wade AC, Luckert PH, Tazume S, Niedbalski JL, Pollard M. Characterization of xenogeneic mouse-to-rat bone marrow chimeras. I. Examination of hematologic and immunologic function. Transplantation (1987) 44(1):88–92. doi: 10.1097/00007890-198707000-00019
89. Heidt PJ, Vossen JM. Experimental and clinical gnotobiotics: influence of the microflora on graft-versus-host disease after allogeneic bone marrow transplantation. J Med (1992) 23(3-4):161–73.
90. Bauer H, Horowitz RE, Levenson SM, Popper H. The response of the lymphatic tissue to the microbial flora. Studies on germfree mice. Am J Pathol (1963) 42:471–83.
91. Hooper LV, Littman DR, Macpherson AJ. Interactions between the microbiota and the immune system. Science (2012) 336(6086):1268–73. doi: 10.1126/science.1223490
92. Round JL, Mazmanian SK. The gut microbiota shapes intestinal immune responses during health and disease. Nat Rev Immunol (2009) 9(5):313–23. doi: 10.1038/nri2515
93. Fredricks DN. The gut microbiota and graft-versus-host disease. J Clin Invest (2019) 129(5):1808–17. doi: 10.1172/JCI125797
94. van Bekkum DW, Roodenburg J, Heidt PJ, van der Waaij D. Mitigation of secondary disease of allogeneic mouse radiation chimeras by modification of the intestinal microflora. J Natl Cancer Inst (1974) 52(2):401–4. doi: 10.1093/jnci/52.2.401
95. Jones JM, Wilson R, Bealmear PM. Mortality and gross pathology of secondary disease in germfree mouse radiation chimeras. Radiat Res (1971) 45(3):577–88. doi: 10.2307/3573066
96. Lampert IA, Moore RH, Huby R, Cohen J. Observations on the role of endotoxin in graft-versus-host disease. Prog Clin Biol Res (1988) 272:351–9.
97. Vriesendorp HM, Heidt PJ, Zurcher C. Gastrointestinal decontamination of dogs treated with total body irradiation and bone marrow transplantation. Exp Hematol (1981) 9(9):904–16.
98. Heimesaat MM, Nogai A, Bereswill S, Plickert R, Fischer A, Loddenkemper C, et al. MyD88/TLR9 mediated immunopathology and gut microbiota dynamics in a novel murine model of intestinal graft-versus-host disease. Gut (2010) 59(8):1079–87. doi: 10.1136/gut.2009.197434
99. Jenq RR, Ubeda C, Taur Y, Menezes CC, Khanin R, Dudakov JA, et al. Regulation of intestinal inflammation by microbiota following allogeneic bone marrow transplantation. J Exp Med (2012) 209(5):903–11. doi: 10.1084/jem.20112408
100. Eriguchi Y, Takashima S, Oka H, Shimoji S, Nakamura K, Uryu H, et al. Graft-versus-host disease disrupts intestinal microbial ecology by inhibiting Paneth cell production of alpha-defensins. Blood (2012) 120(1):223–31. doi: 10.1182/blood-2011-12-401166
101. Eriguchi Y, Nakamura K, Hashimoto D, Shimoda S, Shimono N, Akashi K, et al. Decreased secretion of Paneth cell alpha-defensins in graft-versus-host disease. Transpl Infect Dis (2015) 17(5):702–6. doi: 10.1111/tid.12423
102. Shono Y, Docampo MD, Peled JU, Perobelli SM, Velardi E, Tsai JJ, et al. Increased GVHD-related mortality with broad-spectrum antibiotic use after allogeneic hematopoietic stem cell transplantation in human patients and mice. Sci Transl Med (2016) 8(339):339ra71. doi: 10.1126/scitranslmed.aaf2311
103. Bouazzaoui A, Huber E, Dan A, Al-Allaf FA, Pfirstinger J, Sprotte G, et al. Reduction of aGVHD using chicken antibodies directed against intestinal pathogens in a murine model. Blood (2017) 129(8):1052–5. doi: 10.1182/blood-2016-06-722538
104. Stein-Thoeringer CK, Nichols KB, Lazrak A, Docampo MD, Slingerland AE, Slingerland JB, et al. Lactose drives Enterococcus expansion to promote graft-versus-host disease. Science (2019) 366(6469):1143–9. doi: 10.1126/science.aax3760
105. Toubai T, Fujiwara H, Rossi C, Riwes M, Tamaki H, Zajac C, et al. Host NLRP6 exacerbates graft-versus-host disease independent of gut microbial composition. Nat Microbiol (2019) 4(5):800–12. doi: 10.1038/s41564-019-0373-1
106. Xu Y, Li X, Jin L, Zhen Y, Lu Y, Li S, et al. Application of chicken egg yolk immunoglobulins in the control of terrestrial and aquatic animal diseases: a review. Biotechnol Adv (2011) 29(6):860–8. doi: 10.1016/j.biotechadv.2011.07.003
107. Li X, Jing K, Wang X, Li Y, Zhang M, Li Z, et al. Protective effects of chicken egg yolk antibody (IgY) against experimental Vibrio splendidus infection in the sea cucumber (Apostichopus japonicus). Fish Shellfish Immunol (2016) 48:105–11. doi: 10.1016/j.fsi.2015.11.024
108. Taur Y, Xavier JB, Lipuma L, Ubeda C, Goldberg J, Gobourne A, et al. Intestinal domination and the risk of bacteremia in patients undergoing allogeneic hematopoietic stem cell transplantation. Clin Infect Dis (2012) 55(7):905–14. doi: 10.1093/cid/cis580
109. Ford CD, Gazdik MA, Lopansri BK, Webb B, Mitchell B, Coombs J, et al. Vancomycin-Resistant Enterococcus Colonization and Bacteremia and Hematopoietic Stem Cell Transplantation Outcomes. Biol Blood Marrow Transpl (2017) 23(2):340–6. doi: 10.1016/j.bbmt.2016.11.017
110. Elinav E, Strowig T, Kau AL, Henao-Mejia J, Thaiss CA, Booth CJ, et al. NLRP6 inflammasome regulates colonic microbial ecology and risk for colitis. Cell (2011) 145(5):745–57. doi: 10.1016/j.cell.2011.04.022
111. Levy M, Shapiro H, Thaiss CA, Elinav E. NLRP6: A Multifaceted Innate Immune Sensor. Trends Immunol (2017) 38(4):248–60. doi: 10.1016/j.it.2017.01.001
112. Hu B, Elinav E, Huber S, Strowig T, Hao L, Hafemann A, et al. Microbiota-induced activation of epithelial IL-6 signaling links inflammasome-driven inflammation with transmissible cancer. Proc Natl Acad Sci U S A (2013) 110(24):9862–7. doi: 10.1073/pnas.1307575110
113. Anand PK, Malireddi RK, Lukens JR, Vogel P, Bertin J, Lamkanfi M, et al. NLRP6 negatively regulates innate immunity and host defence against bacterial pathogens. Nature (2012) 488(7411):389–93. doi: 10.1038/nature11250
114. Durakovic N, Radojcic V, Skarica M, Bezak KB, Powell JD, Fuchs EJ, et al. Factors governing the activation of adoptively transferred donor T cells infused after allogeneic bone marrow transplantation in the mouse. Blood (2007) 109(10):4564–74. doi: 10.1182/blood-2006-09-048124
115. Taylor PA, Ehrhardt MJ, Lees CJ, Panoskaltsis-Mortari A, Krieg AM, Sharpe AH, et al. TLR agonists regulate alloresponses and uncover a critical role for donor APCs in allogeneic bone marrow rejection. Blood (2008) 112(8):3508–16. doi: 10.1182/blood-2007-09-113670
116. Calcaterra C, Sfondrini L, Rossini A, Sommariva M, Rumio C, Menard S, et al. Critical role of TLR9 in acute graft-versus-host disease. J Immunol (2008) 181(9):6132–9. doi: 10.4049/jimmunol.181.9.6132
117. Denning TL, Norris BA, Medina-Contreras O, Manicassamy S, Geem D, Madan R, et al. Functional specializations of intestinal dendritic cell and macrophage subsets that control Th17 and regulatory T cell responses are dependent on the T cell/APC ratio, source of mouse strain, and regional localization. J Immunol (2011) 187(2):733–47. doi: 10.4049/jimmunol.1002701
118. Org E, Mehrabian M, Parks BW, Shipkova P, Liu X, Drake TA, et al. Sex differences and hormonal effects on gut microbiota composition in mice. Gut Microbes (2016) 7(4):313–22. doi: 10.1080/19490976.2016.1203502
119. Peng C, Xu X, Li Y, Li X, Yang X, Chen H, et al. Sex-specific association between the gut microbiome and high-fat diet-induced metabolic disorders in mice. Biol Sex Differ (2020) 11(1):5. doi: 10.1186/s13293-020-0281-3
120. Fransen F, Zagato E, Mazzini E, Fosso B, Manzari C, El Aidy S, et al. BALB/c and C57BL/6 Mice Differ in Polyreactive IgA Abundance, which Impacts the Generation of Antigen-Specific IgA and Microbiota Diversity. Immunity (2015) 43(3):527–40. doi: 10.1016/j.immuni.2015.08.011
121. Spychala MS, Venna VR, Jandzinski M, Doran SJ, Durgan DJ, Ganesh BP, et al. Age-related changes in the gut microbiota influence systemic inflammation and stroke outcome. Ann Neurol (2018) 84(1):23–36. doi: 10.1002/ana.25250
122. Storb R, Prentice RL, Buckner CD, Clift RA, Appelbaum F, Deeg J, et al. Graft-versus-host disease and survival in patients with aplastic anemia treated by marrow grafts from HLA-identical siblings. Beneficial effect of a protective environment. N Engl J Med (1983) 308(6):302–7. doi: 10.1056/NEJM198302103080602
123. Taur Y, Jenq RR, Perales MA, Littmann ER, Morjaria S, Ling L, et al. The effects of intestinal tract bacterial diversity on mortality following allogeneic hematopoietic stem cell transplantation. Blood (2014) 124(7):1174–82. doi: 10.1182/blood-2014-02-554725
124. Jenq RR, Taur Y, Devlin SM, Ponce DM, Goldberg JD, Ahr KF, et al. Intestinal Blautia Is Associated with Reduced Death from Graft-versus-Host Disease. Biol Blood Marrow Transpl (2015) 21(8):1373–83. doi: 10.1016/j.bbmt.2015.04.016
125. Simms-Waldrip TR, Sunkersett G, Coughlin LA, Savani MR, Arana C, Kim J, et al. Antibiotic-Induced Depletion of Anti-inflammatory Clostridia Is Associated with the Development of Graft-versus-Host Disease in Pediatric Stem Cell Transplantation Patients. Biol Blood Marrow Transpl (2017) 23(5):820–9. doi: 10.1016/j.bbmt.2017.02.004
126. Golob JL, Pergam SA, Srinivasan S, Fiedler TL, Liu C, Garcia K, et al. Stool Microbiota at Neutrophil Recovery Is Predictive for Severe Acute Graft vs Host Disease After Hematopoietic Cell Transplantation. Clin Infect Dis (2017) 65(12):1984–91. doi: 10.1093/cid/cix699
127. Han L, Zhang H, Chen S, Zhou L, Li Y, Zhao K, et al. Intestinal Microbiota Can Predict Acute Graft-versus-Host Disease Following Allogeneic Hematopoietic Stem Cell Transplantation. Biol Blood Marrow Transpl (2019) 25(10):1944–55. doi: 10.1016/j.bbmt.2019.07.006
128. Payen M, Nicolis I, Robin M, Michonneau D, Delannoye J, Mayeur C, et al. Functional and phylogenetic alterations in gut microbiome are linked to graft-versus-host disease severity. Blood Adv (2020) 4(9):1824–32. doi: 10.1182/bloodadvances.2020001531
129. Peled JU, Gomes ALC, Devlin SM, Littmann ER, Taur Y, Sung AD, et al. Microbiota as Predictor of Mortality in Allogeneic Hematopoietic-Cell Transplantation. N Engl J Med (2020) 382(9):822–34. doi: 10.1056/NEJMoa1900623
130. Arpaia N, Campbell C, Fan X, Dikiy S, van der Veeken J, deRoos P, et al. Metabolites produced by commensal bacteria promote peripheral regulatory T-cell generation. Nature (2013) 504(7480):451–5. doi: 10.1038/nature12726
131. Holler E, Butzhammer P, Schmid K, Hundsrucker C, Koestler J, Peter K, et al. Metagenomic analysis of the stool microbiome in patients receiving allogeneic stem cell transplantation: loss of diversity is associated with use of systemic antibiotics and more pronounced in gastrointestinal graft-versus-host disease. Biol Blood Marrow Transpl (2014) 20(5):640–5. doi: 10.1016/j.bbmt.2014.01.030
132. David LA, Maurice CF, Carmody RN, Gootenberg DB, Button JE, Wolfe BE, et al. Diet rapidly and reproducibly alters the human gut microbiome. Nature (2014) 505(7484):559–63. doi: 10.1038/nature12820
133. Mattsson J, Westin S, Edlund S, Remberger M. Poor oral nutrition after allogeneic stem cell transplantation correlates significantly with severe graft-versus-host disease. Bone Marrow Transpl (2006) 38(9):629–33. doi: 10.1038/sj.bmt.1705493
134. Gonzales F, Bruno B, Alarcon Fuentes M, De Berranger E, Guimber D, Behal H, et al. Better early outcome with enteral rather than parenteral nutrition in children undergoing MAC allo-SCT. Clin Nutr (2018) 37(6 Pt A):2113–21. doi: 10.1016/j.clnu.2017.10.005
135. Guieze R, Lemal R, Cabrespine A, Hermet E, Tournilhac O, Combal C, et al. Enteral versus parenteral nutritional support in allogeneic haematopoietic stem-cell transplantation. Clin Nutr (2014) 33(3):533–8. doi: 10.1016/j.clnu.2013.07.012
136. Pierre JF. Gastrointestinal immune and microbiome changes during parenteral nutrition. Am J Physiol Gastrointest Liver Physiol (2017) 312(3):G246–G56. doi: 10.1152/ajpgi.00321.2016
137. Seguy D, Berthon C, Micol JB, Darre S, Dalle JH, Neuville S, et al. Enteral feeding and early outcomes of patients undergoing allogeneic stem cell transplantation following myeloablative conditioning. Transplantation (2006) 82(6):835–9. doi: 10.1097/01.tp.0000229419.73428.ff
138. Moody KM, Baker RA, Santizo RO, Olmez I, Spies JM, Buthmann A, et al. A randomized trial of the effectiveness of the neutropenic diet versus food safety guidelines on infection rate in pediatric oncology patients. Pediatr Blood Cancer (2018) 65(1). doi: 10.1002/pbc.26711
139. Trifilio S, Helenowski I, Giel M, Gobel B, Pi J, Greenberg D, et al. Questioning the role of a neutropenic diet following hematopoetic stem cell transplantation. Biol Blood Marrow Transpl (2012) 18(9):1385–90. doi: 10.1016/j.bbmt.2012.02.015
140. Smith PM, Howitt MR, Panikov N, Michaud M, Gallini CA, Bohlooly YM, et al. The microbial metabolites, short-chain fatty acids, regulate colonic Treg cell homeostasis. Science (2013) 341(6145):569–73. doi: 10.1126/science.1241165
141. Zama D, Bossu G, Leardini D, Muratore E, Biagi E, Prete A, et al. Insights into the role of intestinal microbiota in hematopoietic stem-cell transplantation. Ther Adv Hematol (2020) 11. doi: 10.1177/2040620719896961
142. Yoshifuji K, Inamoto K, Kiridoshi Y, Takeshita K, Sasajima S, Shiraishi Y, et al. Prebiotics protect against acute graft-versus-host disease and preserve the gut microbiota in stem cell transplantation. Blood Adv (2020) 4(19):4607–17. doi: 10.1182/bloodadvances.2020002604
143. Schwabkey ZI, Jenq RR. Microbiome Anomalies in Allogeneic Hematopoietic Cell Transplantation. Annu Rev Med (2020) 71:137–48. doi: 10.1146/annurev-med-052918-122440
144. Tavil B, Koksal E, Yalcin SS, Uckan D. Pretransplant nutritional habits and clinical outcome in children undergoing hematopoietic stem cell transplant. Exp Clin Transpl (2012) 10(1):55–61. doi: 10.6002/ect.2011.0082
145. Ladas EJ, Bhatia M, Chen L, Sandler E, Petrovic A, Berman DM, et al. The safety and feasibility of probiotics in children and adolescents undergoing hematopoietic cell transplantation. Bone Marrow Transpl (2016) 51(2):262–6. doi: 10.1038/bmt.2015.275
146. Gerbitz A, Schultz M, Wilke A, Linde HJ, Scholmerich J, Andreesen R, et al. Probiotic effects on experimental graft-versus-host disease: let them eat yogurt. Blood (2004) 103(11):4365–7. doi: 10.1182/blood-2003-11-3769
147. Mehta A, Rangarajan S, Borate U. A cautionary tale for probiotic use in hematopoietic SCT patients-Lactobacillus acidophilus sepsis in a patient with mantle cell lymphoma undergoing hematopoietic SCT. Bone Marrow Transpl (2013) 48(3):461–2. doi: 10.1038/bmt.2012.153
148. Vahabnezhad E, Mochon AB, Wozniak LJ, Ziring DA. Lactobacillus bacteremia associated with probiotic use in a pediatric patient with ulcerative colitis. J Clin Gastroenterol (2013) 47(5):437–9. doi: 10.1097/MCG.0b013e318279abf0
149. Robin F, Paillard C, Marchandin H, Demeocq F, Bonnet R, Hennequin C. Lactobacillus rhamnosus meningitis following recurrent episodes of bacteremia in a child undergoing allogeneic hematopoietic stem cell transplantation. J Clin Microbiol (2010) 48(11):4317–9. doi: 10.1128/JCM.00250-10
150. Vossen JM, Guiot HF, Lankester AC, Vossen AC, Bredius RG, Wolterbeek R, et al. Complete suppression of the gut microbiome prevents acute graft-versus-host disease following allogeneic bone marrow transplantation. PloS One (2014) 9(9):e105706. doi: 10.1371/journal.pone.0105706
151. Weber D, Oefner PJ, Dettmer K, Hiergeist A, Koestler J, Gessner A, et al. Rifaximin preserves intestinal microbiota balance in patients undergoing allogeneic stem cell transplantation. Bone Marrow Transpl (2016) 51(8):1087–92. doi: 10.1038/bmt.2016.66
152. Tawara I, Liu C, Tamaki H, Toubai T, Sun Y, Evers R, et al. Influence of donor microbiota on the severity of experimental graft-versus-host-disease. Biol Blood Marrow Transpl (2013) 19(1):164–8. doi: 10.1016/j.bbmt.2012.09.001
153. Whangbo J, Ritz J, Bhatt A. Antibiotic-mediated modification of the intestinal microbiome in allogeneic hematopoietic stem cell transplantation. Bone Marrow Transpl (2017) 52(2):183–90. doi: 10.1038/bmt.2016.206
154. Kimura S, Akahoshi Y, Nakano H, Ugai T, Wada H, Yamasaki R, et al. Antibiotic prophylaxis in hematopoietic stem cell transplantation. A meta-analysis of randomized controlled trials. J Infect (2014) 69(1):13–25. doi: 10.1016/j.jinf.2014.02.013
155. Routy B, Letendre C, Enot D, Chenard-Poirier M, Mehraj V, Seguin NC, et al. The influence of gut-decontamination prophylactic antibiotics on acute graft-versus-host disease and survival following allogeneic hematopoietic stem cell transplantation. Oncoimmunology (2017) 6(1):e1258506. doi: 10.1080/2162402X.2016.1258506
156. Farowski F, Bucker V, Vehreschild JJ, Biehl L, Cruz-Aguilar R, Scheid C, et al. Impact of choice, timing, sequence and combination of broad-spectrum antibiotics on the outcome of allogeneic haematopoietic stem cell transplantation. Bone Marrow Transpl (2018) 53(1):52–7. doi: 10.1038/bmt.2017.203
157. DeFilipp Z, Hohmann E, Jenq RR, Chen YB. Fecal Microbiota Transplantation: Restoring the Injured Microbiome after Allogeneic Hematopoietic Cell Transplantation. Biol Blood Marrow Transpl (2019) 25(1):e17–22. doi: 10.1016/j.bbmt.2018.10.022
158. Kakihana K, Fujioka Y, Suda W, Najima Y, Kuwata G, Sasajima S, et al. Fecal microbiota transplantation for patients with steroid-resistant acute graft-versus-host disease of the gut. Blood (2016) 128(16):2083–8. doi: 10.1182/blood-2016-05-717652
159. Spindelboeck W, Schulz E, Uhl B, Kashofer K, Aigelsreiter A, Zinke-Cerwenka W, et al. Repeated fecal microbiota transplantations attenuate diarrhea and lead to sustained changes in the fecal microbiota in acute, refractory gastrointestinal graft-versus-host-disease. Haematologica (2017) 102(5):e210–e3. doi: 10.3324/haematol.2016.154351
160. Qi X, Li X, Zhao Y, Wu X, Chen F, Ma X, et al. Treating Steroid Refractory Intestinal Acute Graft-vs.-Host Disease With Fecal Microbiota Transplantation: A Pilot Study. Front Immunol (2018) 9:2195. doi: 10.3389/fimmu.2018.02195
161. Kaito S, Toya T, Yoshifuji K, Kurosawa S, Inamoto K, Takeshita K, et al. Fecal microbiota transplantation with frozen capsules for a patient with refractory acute gut graft-versus-host disease. Blood Adv (2018) 2(22):3097–101. doi: 10.1182/bloodadvances.2018024968
162. Zhang J, Ren G, Li M, Lu P, Yi S. The Effects of Fecal Donors with Different Feeding Patterns on Diarrhea in a Patient Undergoing Hematopoietic Stem Cell Transplantation. Case Rep Hematol (2019) 2019:4505238. doi: 10.1155/2019/4505238
163. Quera R, Espinoza R, Estay C, Rivera D. Bacteremia as an adverse event of fecal microbiota transplantation in a patient with Crohn’s disease and recurrent Clostridium difficile infection. J Crohns Colitis (2014) 8(3):252–3. doi: 10.1016/j.crohns.2013.10.002
164. Kuijper EJ, Allegretii J, Hawkey P, Sokol H, Goldenberg S, Ianiro G, et al. A necessary discussion after transmission of multidrug-resistant organisms through faecal microbiota transplantations. Lancet Infect Dis (2019) 19(11):1161–2. doi: 10.1016/S1473-3099(19)30545-6
Keywords: hematopoietic stem cell transplantation, acute graft-versus-host disease, intestinal microbiota, diversity, strategies
Citation: Hong T, Wang R, Wang X, Yang S, Wang W, Gao Q and Zhang X (2021) Interplay Between the Intestinal Microbiota and Acute Graft-Versus-Host Disease: Experimental Evidence and Clinical Significance. Front. Immunol. 12:644982. doi: 10.3389/fimmu.2021.644982
Received: 22 December 2020; Accepted: 26 February 2021;
Published: 16 March 2021.
Edited by:
Nicolaus Martin Kröger, Universität Hamburg, GermanyReviewed by:
Marit Inngjerdingen, University of Oslo, NorwayOlle Thor, Hans Ringden, Karolinska Institutet (KI), Sweden
Yuho Najima, Tokyo Metropolitan Komagome Hospital, Japan
Copyright © 2021 Hong, Wang, Wang, Yang, Wang, Gao and Zhang. This is an open-access article distributed under the terms of the Creative Commons Attribution License (CC BY). The use, distribution or reproduction in other forums is permitted, provided the original author(s) and the copyright owner(s) are credited and that the original publication in this journal is cited, in accordance with accepted academic practice. No use, distribution or reproduction is permitted which does not comply with these terms.
*Correspondence: Xi Zhang, emhhbmd4eGlAc2luYS5jb20=
†These authors have contributed equally to this work