- 1Immunomodulation and Vaccines Programme, Medical Research Council/Uganda Virus Research Institute and London School of Hygiene & Tropical Medicine Uganda Research Unit, Entebbe, Uganda
- 2Department of Parasitology, Leiden University Medical Center, Leiden, Netherlands
- 3Center for Tropical Medicine and Infectious Diseases, Texas Tech University School of Medicine, Lubbock, TX, United States
- 4Department of Internal Medicine, Center for Tropical Medicine and Infectious Diseases, Texas Tech University Health Sciences Center, Lubbock, TX, United States
Despite mass drug administration programmes with praziquantel, the prevalence of schistosomiasis remains high. A vaccine is urgently needed to control transmission of this debilitating disease. As some promising schistosomiasis vaccine candidates are moving through pre-clinical and clinical testing, we review the immunological challenges that these vaccine candidates may encounter in transitioning through the clinical trial phases in endemic settings. Prior exposure of the target population to schistosomes and other infections may impact vaccine response and efficacy and therefore requires considerable attention. Schistosomes are known for their potential to induce T-reg/IL-10 mediated immune suppression in populations which are chronically infected. Moreover, endemicity of schistosomiasis is focal whereby target and trial populations may exhibit several degrees of prior exposure as well as in utero exposure which may increase heterogeneity of vaccine responses. The age dependent distribution of exposure and development of acquired immunity, and general differences in the baseline immunological profile, adds to the complexity of selecting suitable trial populations. Similarly, prior or concurrent infections with other parasitic helminths, viral and bacterial infections, may alter immunological responses. Consequently, treatment of co-infections may benefit the immunogenicity of vaccines and may be considered despite logistical challenges. On the other hand, viral infections leave a life-long immunological imprint on the human host. Screening for serostatus may be needed to facilitate interpretation of vaccine responses. Co-delivery of schistosome vaccines with PZQ is attractive from a perspective of implementation but may complicate the immunogenicity of schistosomiasis vaccines. Several studies have reported PZQ treatment to induce both transient and long-term immuno-modulatory effects as a result of tegument destruction, worm killing and subsequent exposure of worm antigens to the host immune system. These in turn may augment or antagonize vaccine immunogenicity. Understanding the complex immunological interactions between vaccine, co-infections or prior exposure is essential in early stages of clinical development to facilitate phase 3 clinical trial design and implementation policies. Besides well-designed studies in different target populations using schistosome candidate vaccines or other vaccines as models, controlled human infections could also help identify markers of immune protection in populations with different disease and immunological backgrounds.
Introduction
Schistosomiasis is a poverty associated chronic disease caused by parasitic trematodes of the genus Schistosoma (1). Over 190 million people globally are actively infected, of which 90% live in Africa (2) and over 200,000 deaths result from schistosomiasis disease annually in Sub-Saharan Africa (SSA) (1). The two main forms of African schistosomiasis, caused by Schistosoma haematobium and S. mansoni parasites, affect the urinary and gastro-intestinal tract, respectively. The intermediate host, fresh water snails of the genus Bulinus and Biomphalaria shed infective cercariae in water where they penetrate the skin of the human host. Water-related livelihood activities, such as fishing, thus drive transmission in resource limited settings with poor hygiene and sanitary facilities (3). Following exposure, schistosomula migrate through the blood stream and lungs to the mesenteric and/or peri-vesical vessels intestinal venules where they mature into female and male adult worms. Adult worms mate and the female produces eggs that are released from the intestinal or urinary tract to complete the cycle. Pathology results mainly from accumulation of deposited eggs that induce inflammatory responses, granuloma formation and fibrosis. This results in strictures and calcification in the urinary tract due to S. haematobium egg deposition, and liver fibrosis from S. mansoni egg deposition in the Liver. Long term consequences include hydronephrosis and kidney failure in the case of S. haematobium infection, and hepatosplenomegaly and portal hypertension in the case of S. mansoni infection, accounting for the morbidity and mortality in schistosomiasis disease (4, 5).
In the last decade, the World Health Organization (WHO) set an ambitious goal of controlling schistosomiasis by the year 2020 and eliminating it as a public health burden by 2025. Initially, increasing coverage of mass drug administration (MDA) of praziquantel (PZQ), the only drug currently used for schistosomiasis treatment (6), was thought to be sufficient to achieve this. Unfortunately, the increased coverage of MDA decreases the intensity of infection and thus suffices as a tool to alleviate schistosomiasis morbidity but does not prevent re-infection. Consequently, prevalence can be restored in 6–8 months after PZQ administration (7). As a result, control programs rely on the repeated administration of PZQ, whilst facing significant challenges with drug uptake, adherence and sustainability. Hence, schistosomiasis remains a paramount public health concern and economic burden in the resource limited countries (6). Also, the full dependency on a single drug poses a threat of drug resistance (7). This leaves vaccination a key approach for the control and possible elimination of schistosomiasis. An effective vaccine could contribute to prevention and protection against re-infection. Consequently, schistosomiasis has been ranked among the top ten diseases for which a vaccine is urgently needed (8). The current overall consensus on preferred product characteristics (PPC) for an effective prophylactic vaccine is induction of 75% reduction in worm burden in immunized individuals and egg excretion in infected patients (9).
However, development of a novel schistosome vaccine faces several scientific challenges due to the immune evasive nature of adult schistosomes and schistosome-induced host immune-modulation. Illustrated by the fact that no human anti-helminth vaccines exists, the development of a schistosomiasis vaccine is likely to be more complex as compared to the recent rapid development of SARS-CoV-2 vaccines. Most importantly, the multi-stage nature of schistosomes, transforming from cercariae to Schistosomula, adult worms and ultimately eggs, involves complex antigenic switches which are life-stage specific, but can also be present across life stages. In addition, the induction of IgE responses, associated risk of allergic reactions and the potential of aggravating granulomas and fibrosis by egg-mediated responses (10) makes schistosomiasis vaccine development complex.
Moreover, poly-parasitism and co-infections, a predominant phenomenon in schistosomiasis endemic regions, mediate cross-immune-regulation whereby one infection influences susceptibility, intensity and immune responses to the other (11). In addition, there is inadequate understanding of how past and existing schistosome infections, repeated exposure, poly-parasitism and prior treatment explicitly structure the immune system in individuals, within and between the different populations. These factors are likely to increase heterogeneity in vaccine responses. Further, the need to implement vaccines in the context of the current MDA control programmes necessitates an integrated approach to praziquantel and vaccine administration (9). However, co-administration of vaccines and praziquantel presents divergent immunological dynamics that have to be considered. Here, we review the immunological challenges for schistosome vaccine development and testing in endemic settings and provide perspectives of how this scientific priority area can be accelerated.
The Progress Toward a Schistosome Vaccine
The initial approach in the quest for a schistosomiasis vaccine involved vaccinating mice with live attenuated cercariae and schistosomula followed by a challenge infection to assess protectivity. These studies demonstrated that 60–70% protection was achievable with a single immunization and could be boosted up to over 90% with subsequent immunisations (12, 13). This approach was similarly efficacious in baboons eliciting protection of over 50% to schistosome larvae challenge and 89% reduction in worm burden, upon immunization with gamma-irradiated cercariae (14). However, despite induction of close to 100% protection (13), this approach is difficult to translate to humans (15).
Nevertheless, these studies provided a strong indication for schistosome vaccine discovery, and knowledge on induction of protective immunity. Acquired immunity was found to be mainly associated with a Th1 type IFN-γ and TNFα pulmonary inflammatory and antibody response, IL-4, IL-5, and eosinophils are of negligible importance meanwhile, IL-10 and IgG4 negates protection and are associated with re-infection (9, 13, 14). Conclusively, these studies denoted that excessive induction of either Th2 or Th1 could lead to damaging pathology and thus, induction a balanced Th1 and Th2 response is fundamental for achieving optimal protection (15).
Utilizing advancements in technology, vaccine development has, in the recent past, shifted focus from irradiated parasite vaccines to specific antigen molecules. Nonetheless, vaccination with irradiated S. mansoni cercarie has contributed to the identification of five such molecules including the Sh28GST and the Sm14 which have proceeded to human clinical trials (12, 15) as discussed below and further reviewed by Molehin (9) and McManus et al. (15)
S. haematobium 28-kD Glutathione S-Transferase (rSh28GST)
This glutathione S-transferase (GST) antigen is a worm detoxification enzyme which is important in preventing parasite oxidative stress (16). Ultrastructural localization of the 28GST show a dense cytosolic, as well as genital, tegumental surface and parenchyma distribution in the schistosome parasite (17). Thus, the 28GST is closely associated with the parasites' muscular organs and anti-28GST specific antibodies mediate Antibody-Dependent-Cellular-Cytoxicity (ADCC) and also inhibit transferase activity of the enzyme interfering with muscle function and ultimately in reduced worm fecundity (18, 19).
Rodent studies demonstrate that 28-kDa fraction of GST (Sh28GSGT) elicits an antibody response which is capable of inhibiting the native GST enzymes, conferring between 40 and 70% protection in rats, mice, hamsters and baboons (20). Experimental infection studies in non-human primate models revealed an anti-fecundity effect with significant reduction in tissue egg load and fecal egg excretion despite a lack of effect on adult worm burden (21).
The phase I trial reported safety and tolerability of the r28GST in Alum formulation in human subjects. The immunological read-abouts demonstrated that 28GST vaccine candidate is highly immunogenic, inducing high levels of specific IgG1, IgG2, IgG3 antibodies. A strong Th2 cytokine response was also observed, typically IL-5 and IL-13 cytokines (22). Though findings from the phase II trial are yet to be published, the phase III trial, a randomized parallel-group double-blinded trial in school children in endemic Senegal, reported a good tolerance of the vaccine with induction of long lasting vaccine specific response. High titres of IgG1, IgG2, and IgG were induced in the vaccine group. However, this vaccine candidate did not show sufficient protection and this was attributed to failure to induce the desired specific-IgG3. The investigators of this trial also attribute this to interreference by the praziquantel treatment that was administered after the first vaccine dose and before the booster dose (18). Post vaccination treatment with PZQ, which coincides with elaboration of immune response to a vaccine, has been shown to interfere with cytokine response (18, 23, 24).
S. mansoni 14-kDA Fatty Acid Binding Protein (Sm14)
Sm14 is one of the fatty acid binding (FABP) proteins that play a crucial role in the uptake, transport and compartmentalization of host-derived sterols by schistosomes (25). Sm-14 protein enzymes are localized in the cytosol and in tissues adjoining the interfaces of parasite/host contact such as the basal lamella of the worm tegument and muscles layers to enable acquisition of lipids from the host (26). They are also found in the gut epithelium for lipid transportation and utilization throughout the parasite. Sm14-specific antibodies bind to tubercles on the parasite's dorsal surface and interfere with uptake of lipids essential for parasite survival as well as mediate ADCC (27).
In rodent studies, Vaccination with the rSm14 induced up to 66 and 89% protection, of Swiss mice and New Zealand white rabbits with, respectively (28). Sera from immunized mice showed generally high reactivity and significant level of rSm14-specific IgM, although IgG and IgA titres were low (29).
In the phase I trial, the Sm14/GLA-SE formulation was safe, highly tolerable and immunogenic in adult male human subjects. It induced a strong CD4+ T cell response producing single Th1 cytokines, particularly the TNF and IL-2. Also, high titres of Sm14-specific IgG, IgG1, and IgG3 antibodies were elicited in vaccinated individuals (27, 30). The Sm14 vaccine has now advanced to a phase IIb trial to be conducted in school children in the endemic Senegal river basin region (25) (NCT03799510).
S. mansoni Tetraspanin-2 (Sm-TSP-2)
Sm-tetraspanin is a member of a four-domain-structured tetraspanin surface membrane protein linked by two extracellular loops and made of two types, TSP-1 and TSP2 (31). The main vaccine antigen in the Sm-TSP candidate vaccine is comprised of the extracellular loop and the TSP-2 type. The TSP-2 antigen was found to be more strongly recognized by IgG1 and IgG3 antibodies in the sera of naturally immune populations, unlike TSP-1 (32). The TSP-2 is a readily immune-accessible antigen on the surface of newly transformed schistosomula and a critical tegument protein for nutrient acquisition, waste excretion and immune evasion. Anti-TSP-2 antibodies interfere with these parasite survival mechanisms and elicit a protective immunity against infection in a vaccinated host (32).
Immunization with Sm-TSP-2 result in 57% and 62% reduction in worm and liver egg burden, respectively, and 69% reduction in fecal egg count, in rodent models (32). An increased production of Sm-TSP-2 specific antibodies and IL-4, IL-10 cytokines by spleen cells is also observed in immunized animals (33).
Phase Ia and phase Ib trials of the Sm-TSP-2/Alhydrogel (Sm-TSP-2/AI) have both been initiated to investigate safety and immunogenicity of this vaccine candidate in human subject (NCT03910972). Phase I trial of the Sm-TSP/Alhydrogel with or without glucopyranosyl lipid A (GLA-AF) formulation in non-endemic setting show the vaccine is safe and tolerable among Sm-naïve individuals (34). The “Sm-TSP/Al with GLA-AF” formulation elicited higher sero-response than the “Sm-TSP/Al without GLA-AF” and placebo, with a dose-response relationship exhibited by Sm-TSP/Al with GLA-AF (34).
S. mansoni Sm-p80/GLA-SE
Sm-p80 is the large subunit of calpain, a calcium activated neutral tegument protease, which is located in and on the surface epithelial syncytium and mediates tegument biogenesis for host immune evasion. Sm-p80 is a highly immuno-dominant membrane antigen with no cross-reactivity with vertebrate calpains (35).
A multitude of studies performed in vitro, in rodents and non-human primates over the past 23 years have demonstrated that Sm-p80 is a very promising vaccine candidate with prophylactic, therapeutic, anti-pathology and transmission blocking efficacies (36). Significant reduction in adult worm burden, tissue egg load and fecal egg excretion following Sm-p80 vaccination has been demonstrated, whereas potentially allergic IgE responses have not been registered (37). Sm-p80 immunization elicits significant complement-dependent killing of schistosomula (36). Administration of PZQ preceding Sm-p80 vaccination is proven to profoundly reduce tissue egg retention and hatching in non-human primates (38, 39). Following the desirable responses and results from rodent and non-human primate studies, Sm-p80/GLA-SE has now been approved for phase I clinical trial (9).
As schistosomiasis candidate vaccines are progressing from phase I studies to testing in the target populations in several endemic settings, the distinctive disease exposure, co-infections and transmission settings that uniquely shape the immunological profiles may result in heterogeneity in vaccine responses. Hence, in addition to the existing logistical, accessibility and resource-limitation challenges, the immunological complexity presents exceptional challenges to vaccine development and testing in schistosomiasis endemic regions.
Prior/Current Infection With Schistosomes and Antigen Sensitization
Generally, prophylactic vaccines are given to naïve individuals but for potential Schistosoma vaccines, this may be challenging as sensitization to Schistosoma antigens is likely to occur early in life or even in utero in endemic settings (40). Prior exposure to antigens included in a vaccine may enhance or suppress vaccine responses.
At birth, the offspring of Schistosoma infected mothers already show signs of B and T-cell sensitization to parasite antigens (41), with detectable schistosome-specific antibodies (including IgE), T cell responses in cord blood, and schistosome antigens in the urine of new-borns (40, 42). Even with zero egg counts, antibodies in infant serum recognize some worm antigens (43, 44). Following childhood exposure, infection peaks in 6–15 years old children (19) and can be as high as 90% in <12 years olds (45, 46). The peak and dispersion in worm burden gradually declines with age (46), which could be a reflection of reduced exposure or, in high exposure settings, the induction of naturally acquired immunity. Naturally acquired immunity is characterized by a broadening of antibody repertoire and a switch from predominantly egg-specific IgG1, IgG2, and IgM antibodies in infancy to a protective larval and adult worm-specific IgE antibodies toward adulthood and increasing duration of exposure (43, 47–49). IgE response, elicited after repeated exposure and or PZQ treatment is associated with protection (though not exclusively) in endemic populations (10). Natural or PZQ-driven death of adult worms releases parasite antigens from migrating larvae and this induces IgE response (50) which mediates antibody-dependent-cellular-cytoxicity (ADCC) killing of more parasites (31). Although not useful in vaccine development due to the potential to induce hypersensitivity (10), an IgE mediated protective immunity is subsequently developed in individuals, over many cycles of infection and or treatment (51).
This raises the question of how pre-exposure and pre-existing immunity influences vaccine responses. Depending on the vaccine antigen and the pathogen, the effect of pre-exposure may vary. For instance, reduced cytokine responses to Bacillus Calmette-Guérin (BCG) vaccination have been linked to previous exposure to other mycobacteria, but studies with hepatitis B vaccines show conflicting results with increased antibody responses after pre-exposure in one study, and lower in another study (52). For schistosome antigens, there is no evidence so far that pre-existing exposure to vaccine antigens negatively impacts schistosomiasis vaccine responses. In the rSh28GST Phase 3 study, pre-existing immune responses to the 28GST antigen were observed in children aged 6–7, but there was no evidence of pre-existing anti-28GST responses negatively impacting vaccine immune responses (18). In addition, in studies with recombinant Sm14, high levels of the Th1 cytokines IFN-γ, and TNF-α could be observed despite a history of chronic exposure (53). This is in agreement with animal experiments which showed that successful immunization could be achieved in previously infected and cured baboons (39). As such, current data suggest that schistosome vaccines may retain immunogenicity despite prior exposure. However, the impact of prior exposure on vaccine responses may differ for each antigen and should therefore be carefully assessed in early-stage trials to avoid reduced efficacy results in phase three trials or implementation settings.
Population Differences in Immune Profile Affecting Vaccine Responses
Differences in the baseline immune profiles between populations are known to affect both quantitative and qualitative response to vaccines (54). Since novel vaccine candidates are typically assessed for phase I safety trials in a non-endemic European population, it should be borne in mind that generally lower vaccine responses are found in African populations (55–58).
The immune profile of African cohorts, in contrast to Caucasian populations, typically contains more exhausted and activated NK cells, differentiated T and B cells and pro-inflammatory monocytes (56, 59). This immune profile was associated with a low vaccine-specific neutralizing antibody response and a poor efficacy to the yellow fever vaccine 17D (YF-17D) as compared to a Swiss cohort (56). BCG vaccination in African adults and children elicited low IFN-γ response and a mixed cytokine profile in a setting where BCG efficacy is low, but a predominant Th1 cytokine profile in the United Kingdom where BCG efficacy is 50–80% (55, 57, 60). Also, African infants did not show increase in the magnitude of T cell response to the MVA85A TB candidate vaccine unlike their UK counterparts (61). Furthermore, the HIV-adenovirus-vectored (Ad26.EnvA.01 and Ad35-EnvA) candidate vaccines induced better efficacy and greater T cell responses in an American cohort compared to South and East African subjects (58). These findings suggest that the baseline immune profile of African populations may interfere with adequate vaccine responses and this effect is especially important for the viral vectored and live vaccines such as the MVA85A and Ad26/35-EnvA, and BCG and YF-17D, respectively (55, 57, 61).
Genetic difference between populations could contribute to heterogeneity in vaccine responses. Vaccine immune responses are regulated by multiple gene complexes and networks which may be subject to genetic variations such as inherent cytokine and HLA gene polymorphisms (62, 63) and thus, difference in immune phenotype and functional response. For instance, Indonesians were shown to express the unique CD11c+ IL-10 producing B cell subset as compared to Europeans (64), depicting a possible genetic disparity. In a malaria endemic region in Mali, both adults and children exhibited an atypical FcRL4+ expressing memory B cell population unlike their American counterparts (65). Furthermore, volunteers of Congolese origin expressed a distinctly high number of STAT6 and IL10RA regulatory gene polymorphisms, a genetic evidence that explains the predominantly asymptomatic-uncomplicated malaria infection manifestation in this population (66). Differences in response to vaccines such as the measles vaccine have also been attributed to genetic polymorphisms in the CD46 and SLAM gene receptor (67).
However, there is increasing evidence suggesting that inter-population differences in immunological profiles are driven by non-inheritable environmental factors such as prior and current infection status which may typically be vastly different between rural vs. urban living (59, 68, 69). For example, rural African populations express a superior immune activated state, larger memory pool and Th2 polarization as compared to urban Africans (64) and Indonesians from a non-endemic urban setting showed a similar immune profile to European subjects yet markedly differed from Indonesians from an endemic rural area (59). More so, a type 2 immune profile comprising of Th2 cells, IL-4, IL-5, and IL-13 cytokines is typical of schistosomiasis high-exposure endemic populations (70). Subjects from the endemic setting exhibited a high frequency of CD161+Th2 cells, CCR6-KLGR1+ ILC2, CTLA+ T regs and IL-10+ CD11c+ B cells (64). Subjects from a rural setting in Uganda showed a decreased specific cytokine and antibody response to tetanus toxoid and Mtb purified derived protein (PPD) compared to their urban counterparts which persisted after adjusting for helminth infection (71).
Therefore, early phase clinical trials should preferably be conducted in target populations to ensure no gaps in translation at a later stage. In addition, booster regimens, potent adjuvants and higher antigen dose can be considered particularly for endemic populations such as in SSA where low vaccine response and efficacy is predominant.
Co-infections
Besides prior and current schistosome exposure, schistosomiasis endemic populations are also highly diverse with regards to exposure to other infections. Chronic viral infections and poly-parasitism in schistosome endemic populations constantly expose the host immune system to a complex array of antigens and epitopes causing antigenic competition and immune sensitization (72). In addition, parasitic infections such as lymphatic filariasis, onchocerciasis and leishmaniasis often occur in schistosome endemic areas. This trains and pre-sets the immune phenotype and functionality, potentially distorting the host response to, vaccine candidates.
Noteworthy, in this review we focus on the concomitant parasitic (malaria and soil-transmitted helminths) and viral (Cytomegalovirus) infections which are highly prevalent among schistosomiasis endemic populations in the low and middle income countries (LMIC) (73, 74). Parasitic co-infections are of particular importance due to the significant negative impact on immunization especially among parasite endemic populations which are the target for schistosomiasis vaccine trials (73).
Malaria Co-infection
Malaria and schistosomiasis are both parasitic diseases that share co-endemicity, and cause significant morbidity and damaging socio-economic effects (75). With schistosomiasis only ranking second to malaria, these two parasitic diseases exhibit an overlapping geographical distribution pattern and a high prevalence of co-infection across the tropics and sub tropics (75). The prevalence of malaria is highest in children (76) and is mainly caused by Plasmodium falciparum. In fishing communities, which are a target population for schistosomiasis vaccine trials due to the high schistosomiasis transmission and intense exposure, the risk and prevalence of malaria co-infection is very high (77). The presence of breeding sites in the lake environment such as stagnant pools of water on the shores and unused old boats, fish bait mines and finger-ponds traps which sustain a heavy vector population in these communities (78). Also, fishing activities such as nocturnal fishing promote outdoor transmission as well as the temporary makeshift housing that is porous to the malaria vector (79).
Primary malaria infection induces CD4+ T cell differentiation into CXCR+ T follicular (Tfh) cells to provide B cell help and the highly proliferative Vγ9+Vδ2+ T cells produce high levels of IFN-γ (80). Production of pro-inflammatory cytokines and chemokines such as IL-1β, IL-6, IL-8, IL-12, IFN-γ, and TNF induce fever and other signs and symptoms in previously unexposed individuals resulting in severe and fatal malaria (81). Increased TNF levels are associated with severe and cerebral malaria infection although sustained high levels result in reduction in parasitemia and improvement of fever (82, 83). However, this Plasmodium-induced inflammatory response also controls parasite replication and resolves infection through IFN-γ and TNF mediated killing of parasite (84). Meanwhile, acute uncomplicated infection activates the less functional CXCR3+PD1+ Tfh cells which enhances production of IL-21 and pro-inflammatory cytokines (85). P. falciparum malaria infection additionally prompts dysregulation of B- and CD4+T cell function subsequently, causing significant and rapid loss of Pf-antigen-specific antibodies (85, 86). Asymptomatic malaria is an important consideration for clinical trials since it is the most common form among adults, the ideal early-phase trial subjects in endemic settings. Asymptomatic malaria is characterized by increased Treg numbers, IL-10 and TGF-β production, and dampened TNF, IFN-γ pro-inflammatory response characterizes (87). P. falciparum-induced upregulation of IL-10 production by CD4+Foxp3- Th1 cells rather than T regs, is usually sustained in persistent asymptomatic infection (88). Malaria infection additionally affects B cell phenotype and function. In repeated malaria infections and persistent asymptomatic parasitemia, accumulation of atypical memory B cells, a hyporesponsive anergic B cell subset expressing several inhibitory receptors, is seen (89, 90). Expansion of atypical memory B cells associated with clinical immunity following acute malaria has also been reported (91). This immunological memory may have implications on vaccine response given that it may distort the balance between antibody affinity maturation and B cell clonal selection following vaccination (90, 92).
Acute malaria has been reported to lower response to tetanus and diphtheria toxoids, meningococcal polysaccharide, Salmonella and Haemophilus influenza conjugate and whole vaccines (93–95). Fever associated with malaria infection contributes to a diminishing response to Haemophilus influenza vaccine (94). More so, asymptomatic parasitemia in malaria infection is associated with a decreased response to the newer acellular pertussis and meningococcal vaccine (85). Similarly, malaria infection during pregnancy and in infancy decreases antibody response to infant measles vaccination (96). These findings suggest and support treatment of malaria before vaccination is necessary to alleviate the antagonizing immune effects. Indeed, there is evidence that the response to some vaccines (such as the tetanus vaccine) improve following malaria chemoprophylaxis (93). However, pre-treatment does not improve response to or even impair immunogenicity of some vaccines such as the measles vaccine (93).
In co-endemic populations, it seems reasonable to test and treat malaria infections prior to vaccine administration. However, the added complexity of malaria pre-treatment in implementation settings makes it imperative to investigate the effect of asymptomatic malaria on vaccine response before phase 3 trials are performed. In addition, the optimal timing of malaria chemotherapy with regards to vaccine administration will need to be considered in order to optimize effects in different malaria transmission settings.
Soil-Transmitted Helminths (STHs) Co-infection
Soil-transmitted helminths (STHs) and schistosomiasis are predominantly co-endemic (97). It is estimated that one-third of the population in SSA is infected with one or more STH (98). This prevalence is driven by the overlapping poverty-related conditions of poor environmental hygiene, improper waste disposal, in adequate water supply and pollution of water bodies (99). The common STHs are the round worm (Ascaris lumbricoides), hookworms (Necator americanus and Anyclostoma duodenale), whipworm (Trichuris tichuria), and Strongloides stercoralis (97). However, hookworm and A. lumbroicoides infections seem the most prevalent of helminth co-infections in schistosomiasis infected individuals in many endemic areas (100, 101). Young children and males are more prone to the infections due to poor hand hygiene practices and active behavior that exposes them to contaminated soils and water (99). This high prevalence among adult males is an indication of a greater likelihood of existing or past infection, a caution for possible distortion of host immune response to other infections and vaccines. And also a challenge to the selection of trial subjects in co-endemic settings.
STH infection impairs development of protective immunity possibly as a result of the potent chronic suppression of the Th1 response required for protection against pathogens (72). STH infections typically induce a type-2 biased immune response (102). Therefore, elevated levels of IL-4, IL-5, IL-13, IgE in addition to general modulation of both innate and adaptive immune systems can be expected in STH co-infection (102). Chronic STH infection not only induces potent local but also systemic down regulation of the immune system. For instance, human subjects challenged with N. americanus exhibit a strong local and systemic Th2 and T reg response with high levels of IL-10 and TGF-β production (103). In chronic STH infection, it is the increased functional activity of the FOXP3+ Tregs which mediates immune suppression, rather than an increased frequency in chronically infected human hosts (104).
This potentially affects not only susceptibility and outcome of concurrent infections but response and efficacy of vaccine candidates (105). Studies in both human and mouse models report poor immunogenicity of the BCG vaccine in STH infected subjects (106). Decreased T cell proliferative response to BCG in helminth infected children was found to result from an enhanced T reg functionality and subsequent immune suppression during chronic infection (104). Besides, the immune profile to recombinant cholera toxin B subunit following live oral cholera CVD 103-HgR vaccination in helminth infected subjects is characterized by low IFN-γ, IL-2, and IL-12 (107), indicating a suppressed immune response. Particularly, existing Onchocerca infection significantly decreases immune response to tetanus vaccination compared to subjects without helminth infection (108). On the other hand, STH infections do not alter IgG antibody responses to previously administered measles and tetanus vaccines (109). Hence, with the changing lifestyle, better hygiene practices and anti-helminth treatment, there is a gradual reduction in helminth infections in many LMIC and one would expect better vaccine responses. This has indeed been shown in animal studies and in humans, findings from number of studies do support that treatment of STHs alleviate immune suppression and improve vaccine responses as reviewed elsewhere (110).
Helminth treatment, usually by oral administration of benzimidazoles i.e., albendazole (400 mg/kg) and mebendazole (500 mg), before vaccination may be beneficial for boosting vaccine responses. Deworming of subjects with Albendazole before BCG vaccination was reported to enhance BCG vaccine-specific response (111). A similar boosting effect on oral cholera vaccine among helminth infected subjects is exhibited (112). This is possibly due to alleviation of the helminth-induced immune suppression by anti-helminth treatment. Albendazole deworming is associated with enhanced pro-inflammatory responses and down regulation of inhibitory receptor expression CTLA, by the immune suppressive Tregs (113). However, one study did not find any significant effect of albendazole treatment on influenza vaccine response, despite the increased total IgA titres in the anti-helminth treated group (114).
Nevertheless, testing and treating of helminth infections may be beneficial in phase I schistosomiasis vaccine trials. Treatment before vaccination would alleviate the immune suppression induced by helminth infection prior to vaccine administration and thus, enable better response to vaccines. Meanwhile, treatment around time of vaccine administration possibly drives antigenic unmasking to expose more antigens for host immune recognition. However, antigenic unmasking, following PZQ treatment of intense pre-existing infection which results in release of worm antigens in excess of vaccine antigen, poses a risk of antigenic competition. This may result in poor and or non-specific immune response following PZQ treatment and vaccination. In this case, intensity of pre-existing infection should be taken into consideration. It should be noted that the duration and intensity of anti-helminth treatment itself does have a differential effect on B and T cell response (115). Therefore, not only the effect of STH infections but also their treatment on vaccine response and efficacy should be investigated in late-phase trials in endemic settings.
Human Cytomegalovirus (CMV) Co-infection
This double stranded DNA and enveloped virus belonging to the Beta-herpesvirinae infects between 40 and 100% of adults, varying in prevalence between populations (116). Approximately 90% of the infection incidence occurs in the LMIC of Africa, Asia and South America where up to 60% of the young adults and 90% of elderly adults are sero-positive (117–119).
Besides the high prevalence, the induced immune modulation and establishment of a periodically re-activated life-long latent infection marks CMV a very important viral infection (120, 121). CMV infection typically distorts the T cell repertoire and overall phenotype by inducing a rapid differentiation and clonal expansion of CMV-specific CD8+ T cells. This T cell subset can constitute up to 20% of the T cell repertoire, especially in older adults, but is not functional in controlling the infection (122). Meanwhile, the CMV-specific CD4+T cells increasingly produce IFN-γ, and MIP-1β that mediate re-activation of other latent infections in the host such as Tuberculosis (123). Chronic CMV infection thus causes a continuous accumulation of highly differentiated effector memory T cells. Besides, expression of co-stimulatory receptors in these T cells is inhibited, together resulting in a phenomenon known as “memory inflation” (124, 125), whilst at the same time depleting the naïve T cell pool (126).
Despite the universal host immune phenotype, the negative effect of CMV infection on vaccine responses is most pronounced in the elderly (120). CMV sero-positive elderly adults have been reported to exhibit a decreased response to Influenza vaccine (127, 128). A defective CD8+pSTAT1 and pSTAT3 pathway, expanding senescent CD57+KLG1+ T cell repertoire and the increasing TNF-α and IL-6 levels with infection duration, reduces cytokine responsiveness of vaccine-induced T-cells (120, 128, 129). Findings in younger CMV infected adults are conflicting. An enhanced response, characterized by high levels of circulating Th1 and Th2 cytokines to Influenza vaccine is seen (127), possibly due to the cross-reactive CMV-specific CD8+ T-cell epitopes (130). Meanwhile, response to Ebola candidate vaccines, ChAd3 and MVA, is impaired (120).
Given that CMV establishes a life-long infection that cannot be cleared or treated, the attention for vaccine trial design and implementation should rather be focused around different age of target populations. Phase I trials may consider testing and exclusion of CMV sero-positive subjects, especially in CMV low and moderate prevalence areas, for any vaccine targeting young children or infants. However, this may not be possible in populations where CMV infection is prevalent and almost universal, even among children. For late-phase trials, inclusion of CMV sero-negative vs. sero-positive subjects, could help decipher possible interaction between CMV and vaccine responses. Alternatively, general understanding of the possible effect of CMV could first be investigated through fundamental studies and then regimens such as booster doses for the sero-positive subjects in late phase trials can be considered and tailored accordingly.
Other Co-infections
Prevalent co-infections with obvious immune-modulation and even immune incompetence induction are HIV and TB.
HIV/AIDS, a life-long viral infection with no cure presently and the cause of profound immune-incompetence, is a prevalent viral infection in SSA and other developing regions of the world (131). Over 20 million people are infected in South and Eastern Africa alone (132). HIV establishes a preferential infection and depletion of CD4+ T cells, increases T cell activation, exhaustion and death, impairs antigen presentation, CD4+ and CD8+ T cell functionality (131, 133). This results in very low CD4+ T cell count (below the 500 cells/mm3 lower limit), severe immune impairment, poor vaccine response as well as rapid vaccine induced decline (133, 134).
Combination anti-retroviral therapy facilitates immune reconstitution and viral load suppression, despite persistence of the virus in latent reservoirs (133), suggesting a near-optimal immune response can be expected. Indeed improved vaccine response and reduced risk of infection following Influenza, PPV23 and PCV pneumococcal vaccinations has been reported in patients on cART (135–137). Nevertheless, immune responses in HIV infected patients may be lower, or wane off quicker, despite cART therapy as has been demonstrated in trials with hepatitis B, Influenza, experimental TB and BCG vaccination both in adults and children (138–143).
Despite the potential public health importance of vaccination in HIV infected patients given their increased susceptibility to vaccine-preventable diseases (144), they are often excluded from vaccine trials because of safety concerns and unfavorable benefit/risk profiles (145, 146). For example, the unfavorable benefit/risk profile of BCG vaccination in HIV infected has to led its constant contraindication (146–148). Therefore, testing for HIV, exclusion of sero-positives from trials and referral for cART treatment, is paramount for safety. However, given the high percentage of HIV positive individuals in some areas and the increased use of cART and non-viable vaccines, these populations should be included at a later stage clinical testing of the vaccine to expedite roll-out in these populations after potential registration.
Tuberculosis is another prevalent co-infection among schistosomiasis endemic populations in LMICs affecting over 10 million people and causing significant immune-modulatory impact on infected hosts (132). In active TB infection, levels of IFN-γ, IL-1β, IL-18, are severely decreased and Treg numbers rise thus immune suppression ensues in the infected host. Consequently, a high FOXP3, TGF-β, and IL-4 and low IFN-γ, expression in active TB patients is associated with poor response to BCG vaccination (149, 150). However, an increased antibody response to several unrelated antigens such as measles and tetanus toxoid in active TB patients has also been reported (151).
Similar to HIV infection, active TB is a factor for vaccination deferral due to safety reasons. Nevertheless, further studies within and or outside vaccine trial studies may be important to ensure no interaction occurs between latent TB infection and schistosomiasis candidate vaccine response and efficacy.
The current COVID-19 pandemic has led to over 1.9 million deaths globally (152) inducing serious, potentially long-term immunological perturbations in the infected host that may influence vaccine responses in the same individual. The severity of the COVID-19 disease is associated with the hyper-inflammatory response, “cytokine storm,” especially in the presence of co-morbidities such as diabetes and obesity which are characterized by elevated levels of pro-inflammatory IFN-y,IL-1B,IL-12,IL-6,IL-27, and TNF cytokines (153). It has been suggested that the Th2 biased, T-reg, IL-10 mediated immune hyporesponsive and controlled inflammatory state in schistosomiasis infection (154) could counteract the damaging effects of COVID-19 disease and limit morbi-mortality (155). This theory could explain the relatively low severity and fatality rates of the COVID-19 in the helminth-endemic Africa (156, 157). However, there is currently no evidence to prove this COVID-19 and helminths interaction.
PZQ Co-administration
PZQ, an acylated isoquionoline-pyrazine derivative, is the main drug used in the treatment of schistosomiasis and the basis of community-based mass drug administration (MDA) programs for the last 30 years (51, 158). PZQ, administered orally at dosages of 40–60 mg.kg−1, is efficacious against adult worms with low toxicity and safe, even in pre-school age children and in third trimester pregnancy (159, 160). Although the mechanism of action of PZQ is not entirely understood, it is widely accepted that it disrupts the worm calcium homeostasis leading to worm muscle contraction and paralysis (158, 161, 162). The ensuing PZQ-induced tegument vacuolation and blebbing exposes the worm surface antigens, previously concealed in the intact live parasite to host's immune system (Figure 1) (162). In vivo studies show extensive structural changes in the tegument, sub-tegumental and gastro-dermal musculature, causing leakage of tegumental cytoplasm from live worm in the first 15 min of PZQ treatment (163). Rodent studies have shown that PZQ-induced tegument disruption leads to exposure of a previously concealed native GST enzyme 90 minutes post PZQ treatment (164). Also in humans, drastic increases in cytokine release after PZQ treatment suggests that chemotherapy results into a pulse release of antigens (165) (Figure 1). This suggests that PZQ not only exposes tegument antigens but also cytosolic antigens and enzymes essential for parasite survival to the host immune system, enhancing further tegument damage and parasite killing.
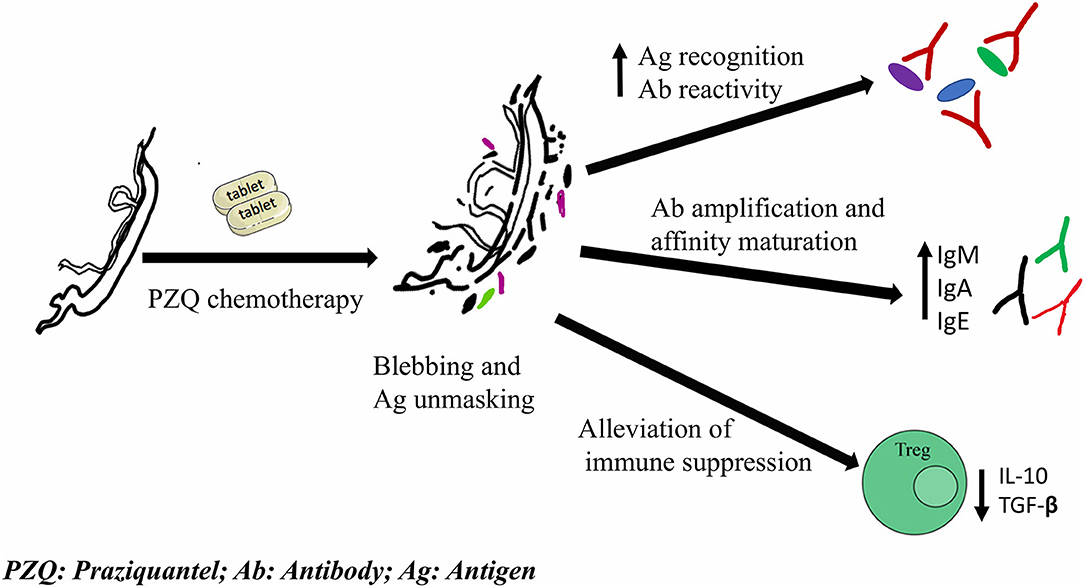
Figure 1. Schematic summary of PZQ-induced immune-modulatory responses that potentially impact vaccine response upon co-administration/delivery. PZQ acts on parasite tegument causing vacuolation and blebbing. This unmasks worm antigens and elicits an increased antigen recognition, antibody reactivity, amplification and affinity maturation, and alleviates the immune suppression induced by live parasites.
As a consequence of antigenic unmasking, PZQ treatment may enhance antigen recognition of antigen-protein-isoforms that would otherwise be hidden (166). Post-treatment serum samples from S. haematobium patients have been shown to recognize a greater number of proteins compared to pre-treatment sera. More so, some proteins and their isoforms are recognized only in post-treatment serum samples (166). Noteworthy, the proteins that show enhanced recognition post treatment are generally those that are associated with parasite musculature and glycolytic metabolism (167), consistent with the disruptive mechanism of action of PZQ. This enhanced antigen recognition permits better neutralization by antibodies raised by vaccination (164, 168). Increased antigen recognition consequently enhances affinity maturation of immunoglobulin responses such as worm specific IgE. For instance, high IgE titres following PZQ chemotherapy ensues from directed IgG affinity maturation induced by PZQ (167), an effect which may also be conferred to co-administered vaccines and moreover lead to a more sustained antibody response following vaccination (164). Besides, antibody titres IgG1, IgG2, IgG4, IgM against worm antigens and IgG2, IgE to egg antigens are boosted following PZQ administration (169).
On the other hand, antigenic competition as a result of PZQ-induced parasite killing could suppress response to the single vaccine antigens. This is because PZQ-induced parasite killing avails large amounts and or a variety of crude worm antigens into the blood stream, in excess of vaccine antigen load. This can potentially overshadow vaccine response or elicit non-specific or undesirable immune response (170). In the phase 3 human trials of the rSh28GST vaccine candidate, PZQ was administered before and after the initial vaccination as well as just before and after the booster vaccination. No significant delay in schistosomiasis recurrence was seen in the vaccine group compared to the control group despite vaccine induced immunity (18). Although this may be attributed mainly to the failure to induce the desired IgG3 and IgA protective antibody response but rather IgG4 responses, interference by the repeated PZQ treatment was also suggested to have, partly, contributed to the observed results (18). Previous studies have demonstrated PZQ-induced modulation of cellular cytokine response to GST (23, 24). Likewise, more subjects exhibit an increased Sh28GST specific cytokine with a shift toward a more pro-inflammatory phenotype following PZQ treatment than before treatment (23, 24). However, a definite conclusion on the role of the PZQ treatment in the outcome of this trial cannot be made. Preferably, next studies should include a control arm whereby the timing of PZQ treatment is shifted several weeks before or after the vaccine administration.
Indeed, PZQ treatment markedly alters both the polarization and magnitude of schistosome specific cytokine responses with longer-term immunological impact than just transient clearance of infection. In the human host, Th2 biased response in form of increased parasite-specific IgE, eosinophil numbers, soluble-high and low affinity IgE receptors on eosinophils and CD23+B cells following PZQ administration is observed (23, 171, 172), which has been related to resistance to reinfection (172–174). In addition, PZQ chemotherapy alleviates the immune suppression induced by schistosomes by downregulating the immune dampening T-reg, Th17 cell numbers and IL-10 cytokine levels (23, 24, 175, 176). Clearance of infection following PZQ administration results in increased effector T cell frequency, increased Schistosome-specific cytokine response and decreased levels of T reg. This contrasts with the immune suppressive profile during infection such as antigen specific hypo-responsiveness, T cell memory pool distortion and increased CD4+CD25+FOXP3+T cell numbers (177). Consequently, in a randomized trial in Entebbe, Uganda, PZQ treatment significantly, although transiently, improved vaccine response to measles immunization in S. mansoni infected pre-school children (169). Noteworthy, this effect seems greatest when PZQ is administered a few weeks before immunization (169).
In conclusion, although PZQ has been deemed to be non-immunogenic in itself and thus have no impact on vaccine efficacy, PZQ-induced antigenic release elicits both transient and long term immune modulatory effects (23, 165, 175, 178) (Figure 1) which in turn, potentially augment or mask vaccine responses. This is particularly important given that future schistosome vaccine trials in endemic settings target adult subjects, whose immunological and exposure profile has been shaped by repeated exposures and or PZQ treatment. As such PZQ-vaccine co-delivery could either be a very effective, more wholistic strategy to enhance vaccine response and efficacy or be detrimental to detecting vaccine efficacy in late-stage clinical trials. Therefore, different vaccine schedules with PZQ chemotherapy needs to be assessed in order to select the most appropriate vaccine-PZQ combination and immunization-chemotherapy treatment schedule before embarking on such strategies in phase 3 studies.
Outlook
Given the potentially complex interaction of the above mentioned factors within the target population (43), the appropriate selection of trial subjects in which confounders have been taken into account appropriately, has become a true challenge to vaccine development and testing. Recognizing the fact that large phase 3 efficacy trials will be performed in a randomized fashion, most likely correcting for potential unknown or unidentified confounders within the trial, understanding or recognizing the potential of these factors will ultimately be important for extrapolation of trial results and implementation of any schistosome vaccine across sub-Sahara Africa. Moreover, the phase 3 design will have to take into account the treatment of co-infections in the context of already existing MDA programmes.
Therefore, we argue that considerable investment should be made during early phase clinical testing in an attempt to understand and disentangle these confounders. As a start, thorough screening of subjects for co-infections, chronic diseases and physiological disorders should be done in order to identify confounders in early-stage clinical trials, and enable appropriate selection of trial populations with sufficient heterogeneity at a later stage. The inclusion of several trial arms with different population subsets (e.g., high vs. low pre-exposure) is helpful to unravel the interaction between co-infections and vaccine responses. For treatable co-infections, the effects of pre-treatment such as PZQ, artemisinin and or albendazole administration at the same time or prior to vaccination should be carefully incorporated into trial designs especially from phase II trial stage. Additionally, timing of treatment and vaccine administration maybe a crucial determining factor for PZQ masking of or synergy with vaccine response and efficacy. Understanding the interplay between drug administration and vaccine responses will prove to be essential for implementation of novel vaccines in MDA settings. In the case of co-infections such as HIV/AIDS (which can be treated but not eliminated), testing and exclusion of sero-positive volunteers is the current practice in phase 1 trials. However, including seropositive individuals in phase II and III trials should be promoted to ensure population-wide implementation of the vaccine after registration.
In larger, late-stage stage clinical trials, the predominantly age dependent distribution of infection intensity and development of protective immunity among endemic populations (46) supports the need for careful selection of analysis methods and tools for handling trial data. Implementing approaches such as pre-defined sub-group analysis, hierarchical clustering analysis and stratified randomization with regards to age, and minimal and intense prior exposure in trials is likely to be useful. This would help to explicitly identify the immunologically distinct heterogenous groups and give useful insights into differential vaccine efficacy.
Controlled Human infection (CHI) models present an opportunity to not only disentangle the different parameters at play in endemic settings, but also accelerate the vaccine development pipeline in general. In the implementation of the CHI model, healthy volunteers are intentionally infected with a pathogen with the aim of generating knowledge on natural history of a disease, testing vaccines or therapeutics, and developing reliable and defined models of infection for future studies (179). These studies are controlled in terms of dose and route, production and selection of the pathogen strain administered. Additionally, a “controlled” condition/environment is key to prevent natural infection during the study period (179). Therefore, signs and symptoms of disease, and evolution of responses following a defined timing of the exposure can be observed in a well described and managed manner. This makes it possible to study the natural history of infection, host-pathogen interactions, evolution of immune responses and provide a preliminary assessment of vaccine efficacy (180, 181). The CHI models are also proof of concept studies and furthermore enable identification of correlates of protection. This approach allows for testing and early selection of promising vaccine candidates in a smaller group of healthy volunteers (<100), within a shorter period of time under controlled conditions compared to the classical vaccine testing methods (182). Hence, CHI studies reduce the burdening cost and time requirements, and the high downstream vaccine efficacy failure, associated with classical clinical trials approach. Noteworthy, for safety and ethical concerns, the CHI approach is applicable only for infections/diseases that are self-resolving and or are treatable such as schistosomiasis (179, 180).
The recent development of a controlled Human Infection model for Schistosomiasis (CHI-S), utilized single-sex cercariae for infection of healthy Dutch volunteers (181). This approach prevents the risk of egg-related pathologies as seen in the “natural” mixed-sex schistosome infections, while preserving the ability to cause infection and mature into adult schistosomes in human subjects (183). The highly sensitive diagnostic test, Circulating Anodic Antigen (CAA) test is used to detect infection that usually occurs between 6 and 12 weeks after the CHI procedure (181). Subjects are then treated with PZQ at 8 or 12 weeks post exposure to ensure clearance of infection (181). The first CHI-S trial demonstrates that the male-only CHI-S is safe and tolerable among naïve Dutch volunteers with about 82% infection rate, and absence of schistosome egg production (181). Despite obvious limitations of the single-sex nature of the CHI-S model, it can nevertheless be used as a screening tool for future schistosomiasis vaccine candidates and new drugs. More importantly, the implementation of the CHI-S model in endemic settings provides a unique opportunity to disentangle the aforementioned distinctive immune responses and potential natural resistance, to Schistosoma infection and vaccines among endemic populations (184). This would not only accelerate vaccine development and testing but also help unravel the heterogeneity in vaccine immunogenicity, safety, and efficacy.
Conclusion
The urgent need for a vaccine to eliminate the burden of the schistosomiasis in LMICs calls for acceleration in the testing and subsequent approval of the current vaccine candidates. However, endemic populations exhibit a multiplicity of distinctive disease transmission, exposure, pathogenesis, genetic and environmental factors that result in immunological challenges as summarized in Table 1. These potentially impact vaccine responses and efficacy and amplify the chances of vaccine failures. Therefore, significant consideration has to be conceded to these immunological challenges in order to accelerate vaccine development and testing. Studies to investigate and accurately establish the impact and extent to which these immunological challenges affect host immune and vaccine response should become a fundamental facet of baseline and or preparatory studies as well the actual trial protocols for vaccine testing in endemic settings.
Author Contributions
MR, AME, JPRK, and ED: conceptualization. ED and JPRK: writing (original draft preparation). MR, AME, AAS, MY, and SC: writing (review and editing). MR and AME: supervision. All authors contributed to the article and approved the submitted version.
Funding
This work was conducted at the MRC/UVRI and LSHTM Uganda Research Unit which is jointly funded by the UK Medical Research Council (MRC) and the UK Department for International Development (DFID) under the MRC/DFID Concordat agreement and is also part of the EDCTP2 programme supported by the European Union and was supported by funding from the European Union's Horizon 2020 research and innovation programme under grant agreement No. 815643.
Conflict of Interest
The authors declare that the research was conducted in the absence of any commercial or financial relationships that could be construed as a potential conflict of interest.
References
1. WHO. Schistosomiasis Fact Sheet No 115. (2010). Available online at: https://wwwwhoint/news-room/fact-sheets/detail/schistosomiasis (accessed November 27, 2020).
2. Li EY, Gurarie D, Lo NC, Zhu X, King CH. Improving public health control of schistosomiasis with a modified WHO strategy: a model-based comparison study. Lancet Global Health. (2019) 10:e1414–e22. doi: 10.1016/S2214-109X(19)30346-8
3. Friedman JF, Mital P, Kanzaria HK, Olds GR, Kurtis JD. Schistosomiasis and pregnancy. Trends Parasitol. (2007) 23:159–64. doi: 10.1016/j.pt.2007.02.006
4. Barsoum RS. Urinary schistosomiasis review. J Adv Res. (2013) 4:453–9. doi: 10.1016/j.jare.2012.08.004
5. Colley DG, Secor WE, King CH. Human schistosomiasis. Lancet. (2014) 9936:2253–64. doi: 10.1016/S0140-6736(13)61949-2
6. Deol AK, Fleming FM, Calvo-Urbano B, Walker M, Bucumi V, Gnandou I, et al. Schistosomiasis - Assessing Progress toward the 2020 and (2025). Global Goals. N Engl J Med. (2019) 381:2519–28. doi: 10.1056/NEJMoa1812165
7. Crellen T, Walker M, Lamberton PH, Kabatereine NB, Tukahebwa EM, Cotton JA, et al. Reduced efficacy of praziquantel against schistosoma mansoni is associated with multiple rounds of mass drug administration. Clin Infect Dis. (2016) 63:1151–9. doi: 10.1093/cid/ciw506
9. Molehin AJ. A Schistosomiasis vaccine development: update on human clinical trials. J Biomed Sci. (2020) 27:1–7. doi: 10.1186/s12929-020-0621-y
10. Molehin AJ. Current understanding of immunity against schistosomiasis: impact on vaccine and drug development. Res Rep Trop Med. (2020) 11:119–28. doi: 10.2147/RRTM.S274518
11. Pullan R, Booker S. The health impact of polyparasitism in humans: are we under-estimating the burden of parasitic diseases? Parasitol. (2008) 135:783–94. doi: 10.1017/S0031182008000346
12. Ricciardi A, Ndao M. Still hope for schistosomiasis vaccine. Hum Vacc Immunother. (2015) 11:2504–8. doi: 10.1080/21645515.2015.1059981
13. Wilson RA, Li XH, Castro-Borges W. Do schistosome vaccine trials in mice have an intrinsic flaw that generates spurious protection data? Parasites Vect. (2016) 9:89. doi: 10.1186/s13071-016-1369-9
14. El Ridi R, Tallima H. Why the radiation-attenuated cercarial immunization studies failed to guide the road for an effective schistosomiasis vaccine: A review. J Adv Res. (2015) 6:255–67. doi: 10.1016/j.jare.2014.10.002
15. McManus DP, Bergquist R, Cai P, Ranasignhe S, Tebeje BM, You H. Schistosomiasis—from immunopathology to vaccines. Semin Immunopathol. (2020) 42:355–71. doi: 10.1007/s00281-020-00789-x
16. Torres-Rivera A, Landa A. Glutathione transferases from parasites: a biochemical view. Acta Trop. (2008) 105:99–112. doi: 10.1016/j.actatropica.2007.08.005
17. Liu JL, Fontaine J, Capron A, Grzych J-M. Ultrastructural localization of Sm28 GST protective antigen in Schistosoma mansoni adult worms. Parasitology. (1996) 113:377–91. doi: 10.1017/S003118200006652X
18. Riveau G, Schacht AM, Dompnier J, Deplanque D, Seck M, Waucquier N, et al. Safety and efficacy of the rSh28GST urinary schistosomiasis vaccine: a phase 3 randomized, controlled trial in Senegalese children. PLoS Negl Trop Dis. (2018) 12:e0006968. doi: 10.1371/journal.pntd.0006968
19. Remoué F, Mani JC, Pugnière M, Schacht AM, Capron A, Riveau G. Functional specific binding of testosterone to Schistosoma haematobium 28-kilodalton glutathione S-transferase. Infect Immun. (2002) 70:601–5. doi: 10.1128/IAI.70.2.601-605.2002
20. Riveau G, Odile PG, Dupré L, Remoué F, Mielcarek N, Locht C, et al. Glutathione S-Transferases of 28kDa as Major Vaccine Candidates against Schistosomiasis. Mem Inst Oswaldo Cruz, Rio de Janeiro. (1998) 93:87–94. doi: 10.1590/S0074-02761998000700012
21. Boulanger D, Warter A, Sellin B, Lindner V, Pierce RJ, Chippaux JP, et al. Vaccine potential of a recombinant glutathione S-transferase clonedfrom Schistosoma haematobium in primates experimentally infected with an homologous challenge. Vaccine. (1999) 17:319–26. doi: 10.1016/S0264-410X(98)00202-3
22. Riveau G, Deplanque D, Remoué F, Schacht AM, Vodougnon H, Capron M, et al. Safety and immunogenicity of rSh28GST antigen in humans: phase 1 randomized clinical study of a vaccine candidate against urinary schistosomiasis. PLoS Negl Trop Dis. (2012) 6:e1704. doi: 10.1371/journal.pntd.0001704
23. Bourke CD, Nausch N, Rejuni N, Appleby LJ, Mitchell KM, Midzi N, et al. Integrated analysis of innate, th1, th2, th17 and regulatory cytokines identifies changes and regulatory cytokines identifies changes in immune polarisation following treatment of human schistosomiasis. J Infect Dis. (2013) 208:159–69. doi: 10.1093/infdis/jis524
24. Bourke CD, Nausch N, Rujeni N, Appleby LJ, Trottein F, et al. Cytokine responses to the anti-schistosome vaccine candidate antigen glutathione-s-transferase vary with host age and are boosted by praziquantel treatment. PLoS Negl Trop Dis. (2014). 8:e2846. doi: 10.1371/journal.pntd.0002846
25. Tendler M, Simpspn AJ. The biotechnology-value chain: development of Sm14 as a schistosomiasis vaccine. Acta Trop. (2008) 108:263–6. doi: 10.1016/j.actatropica.2008.09.002
26. Brito CF, Oliveira GC, Oliveira SC, Street M, Riengrojpitak S, Wilson RA, et al. Sm14 gene expression in different stages of the Schistosoma mansoni life cycle and immunolocalization of the Sm14 protein within the adult worm. Braz J Med Biol Res. (2002) 35:377–81. doi: 10.1590/S0100-879X2002000300014
27. Tendler M, Almeida M, Simpson A. Development of the brazilian anti schistosomiasis vaccine based on the recombinant fatty acid binding protein Sm14 Plus GLA-SE adjuvant. Front Immunol. (2015) 6:218. doi: 10.3389/fimmu.2015.00218
28. Tendler M, Brito CA, Vilar MM, Serra-Freire N, Diogo CM, Almeida MS, et al. A Schistosoma mansoni fatty acid-binding protein, Sm14, is the potential basis of a dual-purpose anti-helminth vaccine. Proc Natl Acad Sci USA. (1996) 93:269–73. doi: 10.1073/pnas.93.1.269
29. Ribeiro F, Vieira SC, Fernandes A, Araujo N, Katz N. The effects of immunization with recombinant Sm14 (rSm14) in reducing worm burden and mortality of mice infected with Schistosoma mansoni. Rev Soc Bras Med Trop. (2002) 35:11–7. doi: 10.1590/S0037-86822002000100003
30. Santini-Oliveira M, Coler RN, Parra J, Veloso V, Jayashankar L, Pinto PM, et al. Schistosomiasis vaccine candidate Sm14/GLA-SE: Phase 1 safety and immunogenicity clinical trial in healthy, male adults. Vaccine. (2015) 34:586–94. doi: 10.1016/j.vaccine.2015.10.027
31. Fonseca CT, Oliveira SC, Alves CC. Eliminating schistosomes through vaccination: what are the best immune weapons? Front Immunol. (2015) 6:95. doi: 10.3389/fimmu.2015.00095
32. Tran MH, Pearson MS, Bethony JM, Smyth DJ, Jones MK, Duke M, et al. Tetraspanins on the surface of Schistosoma mansoni are protective antigens against schistosomiasis. Nat Med. (2006) 12:835–40. doi: 10.1038/nm1430
33. Pearson MS, Pickering DA, McSorley HJ, Bethony JM, Tribolet L, Dougall AM, et al. Enhanced protective efficacy of a chimeric form of the schistosomiasis vaccine antigen Sm-TSP-2. PLoS Negl Trop Dis. (2012) 6:e1564. doi: 10.1371/journal.pntd.0001564
34. Keitel WA, Potter GE, Diemert D, Bethony J, El Sahly HM, Kennedy JK, et al. A phase 1 study of the safety, reactogenicity, and immunogenicity of a Schistosoma mansoni vaccine with or without glucopyranosyl lipid A aqueous formulation (GLA-AF) in healthy adults from a non-endemic area. Vaccine. (2019) 37:6500–9. doi: 10.1016/j.vaccine.2019.08.075
35. Siddiqui AA, Zhou Y, Podesta RB, Karcz SR, Tognon CE, Strejan GH, et al. Characterization of Ca(2+)-dependent neutral protease (calpain) from human blood flukes, Schistosoma mansoni. Biochim Biophys Acta. (1993) 1181:37–44. doi: 10.1016/0925-4439(93)90087-H
36. Zhang W, Le L, Ahmad G, Molehin AJ, Siddiqui AJ, Torben W, et al. Fifteen years of sm-p80-based vaccine trials in nonhuman primates: antibodies from vaccinated baboons confer protection in vivo and in vitro from schistosoma mansoni and identification of putative correlative markers of protection. Front Immunol. (2020) 11:1246. doi: 10.3389/fimmu.2020.01246
37. Ahmad G, Zhang W, Torben W, Ahrorov A, Damian RT, Wolf RF, et al. Preclinical prophylactic efficacy testing of Sm-p80-based vaccine in a nonhuman primate model of Schistosoma mansoni infection and immunoglobulin G and E responses to Sm-p80 in human serum samples from an area where schistosomiasis is endemic. J Infect Dis. (2011) 204:1437–49. doi: 10.1093/infdis/jir545
38. Gaze S, Driguez P, Pearson MS, Mendes T, Doolan DL, Trieu A, et al. An immunomics approach to schistosome antigen discovery: antibody signatures of naturally resistant and chronically infected individuals from endemic areas. PLoS Pathog. (2014) 10:e1004033. doi: 10.1371/journal.ppat.1004033
39. Siddiqui AJ, Molehin AJ, Zhang W, Ganapathy PK, Kim E, Rojo JU, et al. Sm- p80-based vaccine trial in baboons: efficacy when mimicking natural conditions of chronic disease, praziquantel therapy, immunization, and Schistosoma mansoni re-encounter. Ann N Y Acad Sci. (2018) 1425:19–37. doi: 10.1111/nyas.13866
40. Malhotra I, Ouma JH, Wamachi A, Kioko J, Mungai P, Omollo A, et al. In Utero exposure to helminth and mycobacterial antigens generates cytokine responses similar to that observed in adults. J Clin Invest. (1997) 99:1759–66. doi: 10.1172/JCI119340
41. Dauby N, Goetghebuer T, Kollmann TR, Levy J, Marchant A. Uninfected but not unaffected: chronic maternal infections during pregnancy, fetal immunity, and susceptibility to postnatal infections. Lancet Infect Dis. (2012) 12:330–40. doi: 10.1016/S1473-3099(11)70341-3
42. Attallah AM, Gahanem GE, Ismail H, El Waseef AM. Placental and oral delivery of Schistosoma mansoni antigen from infected mothers to their newborns and children. Am J Trop Med Hyg. (2003) 68:647–51. doi: 10.4269/ajtmh.2003.68.647
43. Mutapi F, Burchmore R, Mduluza T, Midzi N, Turner CMR, Maizels RM. Age-related and infection intensity-related shifts in antibody recognition of defined protein antigens in a schistosome-exposed population. J Infect Dis. (2008) 198:167–75. doi: 10.1086/589511
44. Woolhouse MEJ, Mutapi F, Ndhlovu PD, Chandiwana SK, Hagan P. Exposure, infection and immune responses to Schistosoma haematobium in young children. Parasitology. (2000) 120:37–44. doi: 10.1017/S0031182099005156
45. Mnkugwe RH, Minzi OS, Kinung'hi SM, Kamuhabwa AA, Akilillu E. Prevalence and correlates of intestinal schistosomiasis infection among school-aged children in North-Western Tanzania. PLoS ONE. (2020) 15:e0228770. doi: 10.1371/journal.pone.0228770
46. Fulford AJ, Butterworth AE, Sturrock RF, Ouma JH. On the use of age–intensity data to detect immunity to parasitic infections, with special reference to Schistosoma mansoni in Kenya. Parasitology. (1992) 105:219–28. doi: 10.1017/S003118200007414X
47. Lacorcia M, da-Costa PCU. Maternal Schistosomiasis: immunomodulatory effects with lasting impact on allergy and vaccine responses. Front Immunol. (2018) 9:2960. doi: 10.3389/fimmu.2018.02960
48. Coulibaly JT, N'Gbesso YK, N'Guessan NA, Winkler MS, Utzinger J, N'Goran EK. Epidemiology of schistosomiasis in two high-risk communities of south Cote d'Ivoire with particular emphasis on pre-school-aged children. Am J Trop Med Hyg. (2013) 89:32–41. doi: 10.4269/ajtmh.12-0346
49. Raso G, Vounatsou P, McManus DP, N'Goran EK, Utzinger J. A Bayesian approach to estimate the age-specific prevalence of Schistosoma mansoni and implications for schistosomiasis control. Int J Parasitol. (2007) 37:1491–500. doi: 10.1016/j.ijpara.2007.05.004
50. Fitzsimmons CM, Jones F, Pinot de Moira A, Protasio AV, Khalife J, Dickinson HA, et al. Progressive cross-reactivity in IgE responses: an explanation for the slow development of human immunity to schistosomiasis?. Infect Immun. (2012) 80:4264–70. doi: 10.1128/IAI.00641-12
51. McManus DP, Dunne DW, Sacko M, Utizinger J, Vennervald B, Zhou X-N, et al. Schistosomiasis. Nat Rev Dis Primers. (2018) 4:13. doi: 10.1038/s41572-018-0013-8
52. Zimmermann P, Curtis N. Factors that influence the immune response to vaccination. Clin Microbiol Rev. (2019) 32:e00084–18. doi: 10.1128/CMR.00084-18
53. Brito CF, Caldas IR, Coura FP, Correa-Oliveira R, Oliveira SC. CD4+ T cells of schistosomiasis naturally resistant individuals living in an endemic area produce interferon-gamma and tumour necrosis factor-alpha in response to the recombinant 14KDA Schistosoma mansoni fatty acid-binding protein. Scand J Immunol. (2000) 51:595–601. doi: 10.1046/j.1365-3083.2000.00710.x
54. Fine PE. Variation in protection by BCG: implications of and for heterologous immunity. Lancet. (1995) 347:1339–45. doi: 10.1016/S0140-6736(95)92348-9
55. Black GF, Weir RE, Floyd S, Bliss L, Warndorff DK, Crampin AC, et al. BCG-induced increase in interfer- on-gamma response to mycobacterial antigens and efficacy of BCG vaccination in Malawi and the UK: two randomised controlled studies. Lancet. (2002) 9315:1393–401. doi: 10.1016/S0140-6736(02)08353-8
56. Muyanja E, Ssemaganda A, Ngauv P, Cubas R, Perrin H, Srinivasan D, et al. Immune activation alters cellular and humoral responses to yellow fever 17D vaccine. J Clin Invest. (2014) 124:4669. doi: 10.1172/JCI77956
57. Lalor MK, Floyd S, Gorak-Stolinska P, Ben-Smith A, Weir RE, Smith SG, et al. BCG vaccination induces different cytokine profiles following infant BCG vaccination in the UK and Malawi. J Infect Dis. (2011) 204:1075–85. doi: 10.1093/infdis/jir515
58. Gilmour J, Baden LR, Frahm N, Laufer D, Hayes P, Peter L, et al. Immunogenicity of homologous and heterologous regimens of Ad26-Enva.01 and Ad35-Enva HIV vaccines in HIV-uninfected volunteers in the U.S. and Africa. AIDS Res Hum Retroviruses. (2013) 29:A69. Available online at: http://hdl.handle.net/11295/73714
59. Mbow M, de-Jong SE, Meurs L, Mboup S, Dieye TN, Polman K, et al. Changes in immunological profile as a function of urbanization and lifestyle. Immunology. (2014) 143:569–77. doi: 10.1111/imm.12335
60. Rook GA, Dheda K, Zumla A. Immune systems in developed and developing countries; implications for the design of vaccines that will work where BCG does not. Tuberculosis (Edinb). (2006) 86:152–62. doi: 10.1016/j.tube.2006.01.018
61. Pathan AA, Minassian AM, Sander CR, Rowland R, Porter DW, Poulton ID, et al. Effect of vaccine dose on the safety and immunogenicity of a candidate TB vaccine, MVA85A, in BCG vaccinated UK adults. Vaccine. (2012) 30 (38):5616–24. doi: 10.1016/j.vaccine.2012.06.084
62. Ovsyannikova IG, Dhiman N, Jacobson RM, Poland GA. Human leukocyte antigen polymorphisms: variable humoral immune responses to viral vaccines. Expert Rev Vaccines. (2006) 5:33–43. doi: 10.1586/14760584.5.1.33
63. Yucesoy B, Yalzhanov Y, Johnson VJ, Wilson NW, Biagini RE, Wang Wea. Genetic variants within the MHC region are associated with immune responsive- ness to childhood vaccinations. Vaccine. (2013) 31:5381–91. doi: 10.1016/j.vaccine.2013.09.026
64. de Ruiter K, Jochems SP, Tahapary DL, Stam KA, König M, van Unen V, et al. Helminth infections drive heterogeneity in human type 2 and regulatory cells. Sci Transl Med. (2020) 12:eaaw3703. doi: 10.1126/scitranslmed.aaw3703
65. Weiss GE, Crompton PD, Li S, Walsh LA, Moir S, Traore B, et al. Atypical memory B-cells are greatly expanded in individuals living in a malaria- endemic area. J Immunol. (2009) 183:2176–82. doi: 10.4049/jimmunol.0901297
66. Koukouikila-Koussounda F, Ntoumi F, Ndounga M, Tong HV, Abena A-A, Velavan TP. Genetic evidence of regulatory gene var- iants of the STAT6, IL10R and FOXP3 locus as a susceptibility factor in uncomplicated malaria and parasitaemia in Congolese children. Malar J. (2013) 12:1475–2875. doi: 10.1186/1475-2875-12-9
67. Haralambieva IH, Ovsyannikova IG, Pankratz VS, Kennedy RB, Jacobson RM, Poland GA. The genetic basis for interindividual immune response variation to measles vaccine: new understanding and new vaccine approaches. Expert Rev Vacc. (2013) 12:57–70. doi: 10.1586/erv.12.134
68. Brodin P, Jojic V, Gao T, Bhattacharya S, Angel CJ, Furman D, et al. Variation in the human immune system is largely driven by non-heritable influences. Cell. (2015) 160:37–47. doi: 10.1016/j.cell.2014.12.020
69. Hotez PJ, Brindley PJ, Bethony JM, King CH, Pearce EJ, Jacobson J. Helminth infections: the great neglected tropical diseases. J Clin Invest. (2008) 118:1311–21. doi: 10.1172/JCI34261
70. Maizels RM, Yazdanbakhsh M. Immune regulation by helminth parasites: cellular and molecular mechanisms. Nat Rev Immunol. (2003) 9:733–44. doi: 10.1038/nri1183
71. Kabagenyi J, Natukunda A, Nassuuna J, Sanya RE, Nampijja M, Webb EL, et al. Urban-rural differences in immune responses to mycobacterial and tetanus vaccine antigens in a tropical setting: A role for helminths. Parasitol Int. (2020) 78:102132. doi: 10.1016/j.parint.2020.102132
72. Babu SN, Nutman TB. Helminth-Tuberculosis co-infection: an immunologic perspective. Trends Immunol. (2016) 37 597– 607. doi: 10.1016/j.it.2016.07.005
73. Wait LF, Dobson AP, Graham AL. Do parasite infections interfere with immunisation? A review and meta-analysis. Vaccine. (2020) 38 (35):5582–90 doi: 10.1016/j.vaccine.2020.06.064
74. Lim CT, Kumar R. Hepatitis B and concomitant hepatic steatosis. Ann Transl Med. (2016) 5:38. doi: 10.21037/atm.2016.12.04
75. Ojo OE, Adebayo AS, Awobode HO, Nguewa PCI. Schistosoma haematobium and Plasmodium falciparum co-infection in Nigeria 2001–2018: a systematic review and meta-analysis. Scientific African. (2019) 6:e00186. doi: 10.1016/j.sciaf.2019.e00186
76. WHO. World malaria report (2019). Geneva: Licence: CC BY-NC-SA 3.0 IGO (2019). Available online at: https://wwwwhoint/malaria (accessed November 27, 2020).
77. Omwega RN, Abila R, Lwenya C. Fishing and poverty levels around Lake Victoria. In: 11th World Lakes Conference. 2006:193-9 (Kenya).
78. Mukabana W. Artisanal fishing supports breeding of malaria mosquitoes in Western Kenya. Malar J. (2019) 18:77. doi: 10.1186/s12936-019-2708-z
79. Kitakule JS, Reynolds JE. Fishing communities of lake Victoria - Uganda: A first assessment. Food Agricult Organ United Nations. (1991) 2:UGA/87/007.
80. Moormann AM, Nixon CE, Forconi CS. Immune effector mechanisms in malaria: An update focusing on human immunity. Parasite Immunol. (2019) 41:e12628. doi: 10.1111/pim.12628
81. Collins WE, Jeffery GM. A retrospective examination of secondary sporozoite- and trophozoite- induced infections with Plasmodium falciparum: development of parasitologic and clinical immunity fol- lowing secondary infection. Am J Trop Med Hyg. (1999) 61:20–35. doi: 10.4269/tropmed.1999.61-020
82. Karunaweera ND, Grau GE, Gamage P, Carter R, Mendis KN. Dynamics of fever and serum levels of tumor necrosis factor are closely associated during clinical paroxysms in Plasmodium vivax malaria. Proc Natl Acad Sci USA. (1992) 89:3200–3. doi: 10.1073/pnas.89.8.3200
83. Depinay N, Franetich JF, Grüner AC, Mauduit M, Chavatte J-M, Luty AJF, et al. Inhibitory effect of TNF-α on malaria pre-erythrocytic stage development: influence of host hepatocyte/parasite combinations. PLoS ONE. (2011) 6:e17464. doi: 10.1371/journal.pone.0017464
84. Schofield L, Mueller I. Clinical immunity to malaria. Curr Mol Med. (2006) 6:205–21. doi: 10.2174/156652406776055221
85. Banga S, Coursen JD, Portugal S, Tran TM, Hancox L, Ongoiba Aea. Impact of acute malaria on pre-existing antibodies to viral and vaccine antigens in mice and humans. PLoS ONE. (2015). 10:e0125090. doi: 10.1371/journal.pone.0125090
86. Walther M, Jeffries D, Finney OC, Njie M, Ebonyi A, Deininger S, et al. Distinct roles for FOXP3 and FOXP3 CD4 T cells in regulating cellular immunity to un- complicated and severe Plasmodium falciparum malaria. PLoS Pathog. (2009) 5:e1000364. doi: 10.1371/journal.ppat.1000364
87. Jagannathan P, Eccles-James I, Bowen K, Nankya F, Auma A, Wamala S, et al. IFNγ/IL-10 co-producing cells dominate the CD4 response to malaria in highly exposed children. PLoS Pathog. (2014) 10:e1003864. doi: 10.1371/journal.ppat.1003864
88. Tran TM, Samal B, Kirkness E, Crompton PD. Systems immunology of human malaria. Trends Parasitol. (2012) 28:248–57. doi: 10.1016/j.pt.2012.03.006
89. Pérez-Mazliah D, Ndungu FM, Aye R, Langhorne J. B-cell memory in malaria: Myths and realities. Immunol Rev. (2020) 293:57–69. doi: 10.1111/imr.12822
90. Mendonça VR, Barral-Netto M. Immunoregulation in human malaria: the challenge of understanding asymptomatic infection. Memorias do Instituto Oswaldo Cruz. (2015) 110:945–55. doi: 10.1590/0074-02760150241
91. Sullivan RT, Ssewanyana I, Wamala S, Nankya F, Jagannathan P, Tappero JW, et al. Erratum to: B cell sub-types following acute malaria and associations with clinical immunity. Malar J. (2016) 188:139. doi: 10.1186/s12936-016-1236-3
92. Levashina EA, Kremsner PG, Mordmüller B, Höfer T, Wardemann H. Clonal selection drives protective memory B cell responses in controlled human malaria infection. Sci Immunol. (2018) 20:eaap8029. doi: 10.1126/sciimmunol.aap8029
93. Rosen JB, Breman JG, Manclark CR, Meade BD, Collins WE, Lobel HO, et al. Malaria Chemoprophylaxis and the serologic response to measles and diphtheria-tetanus-whole-cell pertussis vaccines. Malaria J. (2005) 4:53. doi: 10.1186/1475-2875-4-53
94. Usen S, Milligan P, Ethevenaux C, Greenwood B, Mulholland K. Effect of fever on the serum antibody response of Gambian chil- dren to Haemophilus influenzae type b conjugate vaccine. Pediatr Infect Dis J. (2000) 19:444–9. doi: 10.1097/00006454-200005000-00010
95. Williamson WA, Greenwood BM. Impairment of the immune response to vaccination after acute malaria. Lancet. (1978) 1:1328–9. doi: 10.1016/S0140-6736(78)92403-0
96. Wajja A, Kizito D, Nassanga BR, Nalwoga A, Kabagenyi J, Kimuda S, et al. The effect of current Schistosoma mansoni infection on the immunogenicity of a candidate TB vaccine, MVA85A, in BCG-vaccinated adolescents: An open-label trial. PLoS Negl Trop Dis. (2017) 11:e0005440. doi: 10.1371/journal.pntd.0005440
97. Uneke CJ. Soil transmitted helminth infections and schistosomiasis in school age children in Sub-Saharan Africa: Efficacy of chemotherapeutic intervention since World Health Assembly Resolution (2001). Tanzania J Health Res. (2010) 12:86–99. doi: 10.4314/thrb.v12i1.56366
98. Sakari S, Mbugua AK, Mkoji GM. Prevalence of soil-transmitted helminthiases and schistosomiasis in preschool age children in mwea division, kirinyaga south district, kirinyaga county, and their potential effect on physical growth. J Trop Med. (2017) 2017:1013802. doi: 10.1155/2017/1013802
99. Aribodor DN, Bassey SA, Yoonuan T, Sam-Wobo SO, Aribodor OB, Ugwuanyia IK. Analysis of Schistosomiasis and soil-transmitted helminths mixed infections among pupils in Enugu State, Nigeria: Implications for control. Infect Dis Health. (2019) 24:98–106. doi: 10.1016/j.idh.2018.12.003
100. Agbolade OM, Agu NC, Adesanya OO, Odejayi AO, Adigun AA, Adesanlu EB, et al. Intestinal helminthiases and schistosomi- asis among school children in an urban center and some rural communities in southwest Nigeria, Intestinal helminthiases and schistosomiasis among school children in an urban center and some rural communities in southwest Nigeria. Kor J Parasitol. (2007) 45:233e8. doi: 10.3347/kjp.2007.45.3.233
101. Rutagwera DG, Tylleskär T. Coinfection with Malaria, hookworm and schistosomiasis among school children in zambezi: a school-based rapid survey. Med J Zambia. (2012) 39:18–23.
102. Salgame P, Yap GS, Gause WC. Effect of helminth-induced immunity on infections with microbial pathogens. Nat Immunol. (2013) 14:1118–126. doi: 10.1038/ni.2736
103. Gaze S, McSorley HJ, Daveson J, Jones D, Bethony JM, Oliveira LM, et al. Characterising the mucosal and systemic immune responses to experimental human hookworm infection. PLoS Pathog. (2012) 8:e1002520. doi: 10.1371/journal.ppat.1002520
104. Wammes LJ, Hamid F, Wiria AE, Gier B, Sartono E, Maizels R, et al. Regulatory T cells in human geohelminth infection suppress immune responses to BCG and Plasmodium falciparum. Europ J Immunol. (2010) 40:437–42. doi: 10.1002/eji.200939699
105. Colombo SAP, Grencis RK. Immunity to soil-transmitted helminths: evidence from the field and laboratory models. Front Immunol. (2020) 11:1286. doi: 10.3389/fimmu.2020.01286
106. Elias D, Britton S, Aseffa A, Engers H, Akuffo H. Poor immunogenicity of BCG in helminth infected population is associated with increased in vitro TGF-β production. Vaccine. (2008) 26:3897–902. doi: 10.1016/j.vaccine.2008.04.083
107. Cooper PJ, Chico M, Sandoval C, Espinel I, Guevara A, Levine MM, et al. Human infection with ascaris lumbricoides is associated with suppression of the interleukin-2 response to recombinant cholera toxin B subunit following vaccination with the live oral cholera vaccine CVD 103-HgR. Infect Immun. (2001) 69:1574–80. doi: 10.1128/IAI.69.3.1574-1580.2001
108. Cooper PJ, Espinel I, Wieseman M, Paredes W, Espinel M, Guderian RH, et al. Human onchocerciasis and tetanus vaccination: impact on the postvaccination antitetanus antibody response. Infect Immun. (1999) 67:5951–7. doi: 10.1128/IAI.67.11.5951-5957.1999
109. Storey HL, Singa B, Naulikh J, Horton H, Richardson BA, John-Stewart G, et al. Soil transmitted helminth infections are not associated with compromised antibody responses to previously administered measles and tetanus vaccines among HIV-1 infected, ART naïve Kenyan adults. Parasite Epidemiol Control 2. (2017) 2:13–20. doi: 10.1016/j.parepi.2016.12.003
110. Sanya RE, Nkurunungi G, Andia BI, Mpairwe H, Elliott AM. A life without worms. Trans R Soc Trop Med Hyg. (2017) 111:3–11. doi: 10.1093/trstmh/trx010
111. Tawfik AF, Colley DG. Effects of anti-schistosomal chemotherapy on immune responses, protection and immunity. II. Concomitant immunity and immunization with irradiated cercariae. Am J Trop Med Hyg. (1986) 35:110–7. doi: 10.4269/ajtmh.1986.35.110
112. Cooper PJ, Chico ME, Losonsky G, Sandoval C, Espinel I, Sridhara RM, et al. Albendazole treatment of children with ascariasis enhances the vibriocidal antibody response to the live attenuated oral cholera vaccine CVD 103-HgR. J Infect Dis. (2000) 182:1199–206. doi: 10.1086/315837
113. Wammes LJ, Hamid F, Wiria AE, May L, Kaisar MM, Prasetyani-Gieseler MA, et al. Community deworming alleviates geohelminth-induced immune hyporesponsiveness. Proc Natl Acad Sci USA. (2016) 113:12526–31. doi: 10.1073/pnas.1604570113
114. Brückner S, Agnandji ST, Berberich S, Bache E, Fernandes JF, Schweiger B, et al. Effect of antihelminthic treatment on vaccine immunogenicity to a seasonal influenza vaccine in primary school children in gabon: a randomized placebo-controlled trial. PLoS Negl Trop Dis. (2015) 9:e0003768. doi: 10.1371/journal.pntd.0003768
115. de Ruiter K, Tahapary DL, Wammes LJ, Wiria AE, Hamid F, van Lieshout L, et al. The effect of three-monthly albendazole treatment on Th2 responses: Differential effects on IgE and IL-5. Parasite Immunol. (2017) 39:12428. doi: 10.1111/pim.12428
116. Brizić I, Hiršl L, Britt WJ, Krmpotić A, Jonjić S. Immune responses to congenital cytomegalovirus infection. Microbes Infect. (2018) 20:543–51. doi: 10.1016/j.micinf.2017.12.010
117. Picarda G, Benedict CA. Cytomegalovirus: shape-shifting the immune system. J Immunol. (2018) 200:3881–9. doi: 10.4049/jimmunol.1800171
118. Zuhair M, Smit GSA, Wallis G, Jabbar F, Smith C, Devleesschauwer B, et al. Estimation of the worldwide seroprevalence of cytomegalovirus: a systematic review and meta-analysis. Rev Med Virol. (2019) 29:e2034. doi: 10.1002/rmv.2034
119. Staras SA, Dollard SC, Radford KW, Flanders WD, Pass RF, Cannon MJ. Seroprevalence of cytomegalovirus infection in the United States. Clin Infect Dis. (2006) 43:1143–51. doi: 10.1086/508173
120. Bowyer G, Sharpe H, Venkatraman N, Ndiaye PB, Wade D, Brenner N, et al. Reduced Ebola vaccine responses in CMV+ young adults is associated with expansion of CD57+KLRG1+ T cells. J Exp Med. (2020) 217:e20200004. doi: 10.1084/jem.20200004
121. McGeoch DJ, Rixon FJ, Davison AJ. Topics in herpesvirus genomics and evolution. Virus Research. (2006) 117:90–104. doi: 10.1016/j.virusres.2006.01.002
122. Miles DJ, van-der-Sande M, Jeffries D, Kaye S, Ojuola O, Sanneh M, et al. Maintenance of large subpopulations of differentiated CD8 T-cells two years after cytomegalovirus infection in Gambian infants. PLoS ONE. (2008) 8:e2905. doi: 10.1371/journal.pone.0002905
123. Portevin D, Moukambi F, Mpina M, Bauer A, Haraka F, Chachage M, et al. Maturation and mip-1beta production of cytomegalovirus-specific t cell responses in tanzanian children, adolescents and adults: impact by HIV and Mycobacterium tuberculosis co-infections. PLoS ONE. (2015) 10:e0126716. doi: 10.1371/journal.pone.0126716
124. O'Connor D, Trück J, Rajeka L, Clutterbuck EA, Voysey M, Jeffery K, et al. The effect of chronic cytomegalovirus infection on pneumococcal vaccine responses. J Infect Dis. (2014) 209:1635–41. doi: 10.1093/infdis/jit673
125. Karrer U, Sierro S, Wagner M, Oxenius A, Hengel H, Koszinowski UH, et al. Memory inflation: continuous accumulation of antiviral CD8+ T cells over time. J Immunol. (2003) 170:2022–9. doi: 10.4049/jimmunol.170.4.2022
126. Henson SM, Riddell NE, Akbar AN. Properties of end-stage human T cells defined by CD45RA re-expression. Curr Opin Immunol. (2012) 24:476–81. doi: 10.1016/j.coi.2012.04.001
127. Furman D, Jojic V, Sharma S, Shen-Orr SS. Cytomegalovirus infection enhances the immune response to influenza. Sci Transl Med. (2015) 7:281ra43. doi: 10.1126/scitranslmed.aaa2293
128. Trzonkowski P, My sliwska J, Szmit E, Wieckiewicz J, Lukaszuk K, Brydak LB, et al. Association between cytomegalovirus infection, enhanced proinflammatory response and low level of anti-hemagglutinins during the anti-influenza vaccina- tion–an impact of immunosenescence. Vaccine. (2003) 21:3826–36. doi: 10.1016/S0264-410X(03)00309-8
129. Botto S, Streblow DN, DeFilippis V, White L, Kreklywich CN, Smith PP, et al. IL-6 in human cytomegalovirus secretome promotes angiogenesis and survival of endothelial cells through the stimulation of survivin. Blood. (2011) 117:352–61. doi: 10.1182/blood-2010-06-291245
130. Welsh RM, Che JW, Brehm MA, Selin LK. Heterologous immunity between viruses. Immunol Rev. (2010) 235:244– 66. doi: 10.1111/j.0105-2896.2010.00897.x
131. Boasso A, Shearer GM, Chougnet C. Immune dysregulation in human immunodeficiency virus infection: know it, fix it, prevent it? J Inter Med. (2009) 265:78–96. doi: 10.1111/j.1365-2796.2008.02043.x
132. UNAIDS. Global HIV and AIDS statistics. (2020). Fact sheet. (2020). Available online at: https://www.unaids.org/en/resources/fact-sheet (accessed November 27, 2020).
133. Elfaki MG. Immunosuppression induced by HIV infection. Biol Med. (2014) 6:1000e111. doi: 10.4172/0974-8369.1000e111
134. Kernéis S, Launay O, Turbelin C, Batteux F, Hanslik T, Boëlle PY. Long-term immune responses to vaccination in HIV-infected patients: a systematic review and meta-analysis. Clin Infect Dis. (2014) 58:1130–9. doi: 10.1093/cid/cit937
135. Evison J, Farese S, Seitz M, Uehlinger DE, Furrer H, Mühlemann K. Randomized, double-blind comparative trial of subunit and virosomal influenza vaccines for immunocompromised patients. Clin Infect Dis. (2009) 48:1402–12. doi: 10.1086/598193
136. Teshale EH, Hanson D, Flannery B, Phares C, Wolfe M, Schuchat A, et al. Effectiveness of 23-valent poly-saccharide pneumococcal vaccine on pneumonia in HIV-infected adults in the United States, 1998–2003. Vaccine. (2008) 46:5830–4. doi: 10.1016/j.vaccine.2008.08.032
137. Rodriguez-Barradas MC, Goulet J, Brown S, Goetz MB, Rimland D, Simberkoff MS, et al. Impact of pneumococcal vaccination on the incidence of pneumonia by HIV infection status among patients enrolled in the Veterans Aging Cohort 5-Site Study. Clin Infect Dis. (2008) 46:1093–100. doi: 10.1086/529201
138. Ndiaye BP, Thienemann F, Ota M, Landry BS, Camara M, Dièye S, et al. Safety, immunogenicity, and efficacy of the candidate tuberculosis vaccine MVA85A in healthy adults infected with HIV-1: a randomised, placebo-controlled, phase 2 trial. Lancet Respir Med. (2015) 3:190–200. doi: 10.1016/S2213-2600(15)00037-5
139. Viganò A, Zuccotti GV, Pacei M, Erba P, Castelletti E, Giacomet V, et al. Humoral and cellular response to influenza vaccine in HIV-infected children with full viroimmunologic response to antiretroviral therapy. J AIDS. (2008) 48:289–96. doi: 10.1097/QAI.0b013e3181632cda
140. Nair N, Moss WJ, Scott S, Mugala N, Ndhlovu ZM, Lilo K, et al. HIV-1 infection in Zambian children impairs the development and avidity maturation of measles virus-specific immuno- globulin G after vaccination and infection. J Infect Dis. (2009) 200:1031– 8. doi: 10.1086/605648
141. Kim HN, Harrington RD, Crane HM, Dhanireddy S, Dellit TH, Spach DH. Hepatitis B vaccination in HIV- infected adults: current evidence, recommendations and practical considera- tions. Int J Std AIDS. (2009) 20:595–600. doi: 10.1258/ijsa.2009.009126
142. Haban H, Benchekroun S, Sadeq M, Benjouad A, Amzazi S, Oumzil H, et al. Assessment of the HBV vaccine response in a group of HIV-infected children in Morocco. BMC Public Health. (2017) 17:752. doi: 10.1186/s12889-017-4776-8
143. Mansoor N, Scriba TJ, de Kock M, Tameris M, Abel B, Keyser A, et al. HIV-1 infection in infants severely impairs the immune response induced by Bacille Calmette-Guérin vaccine. J Infect Dis. (2009) 199:982–90. doi: 10.1086/597304
144. British GAM. HIV Association guidelines for immunization of HIV- infected adults. HIV Med. (2008) 9:795–848. doi: 10.1111/j.1468-1293.2008.00637.x
145. WHO. Meeting of the immunization Strategic Advisory Group of Experts, April (2007). — conclusions and recommendations. Weekly Epidemiological Record = Relevé épidémiologique hebdomadaire. (2007) 21:181–96. Available online at: https://apps.who.int/iris/handle/10665/240948
146. Hesseling AC, Cotton MF, Fordham VRC, Graham SM, Gie RP, Hussey GD. Consensus statement on the revised World Health Organization recommendations for BCG vaccination in HIV-infected infants. Int J Tuberc Lung Dis. (2008) 12:1376–9.
147. Hesseling AC, Marais BJ, Gie RP, Schaaf HS, Fine PE, Godfrey-Faussett P, et al. The risk of disseminated Bacille Calmette-Guerin (BCG) disease in HIV infected children. Vaccine. (2007) 25:14–8. doi: 10.1016/j.vaccine.2006.07.020
148. Fallo A, Torrado L, Sánchez A. Delayed complications of Bacille Calmette-Guérin (BCG) vaccination in HIV-infected children. In: Presented at the International AIDS Society Meeting (Rio de Janeiro). (2005)
149. Roberts TBN, Aguirre A, Walzl G. Immunosuppression during active tuberculosis is characterized by decreased interferon-γ production and CD25 expression with elevated Forkhead Box P3, transforming growth factor-β, and interleukin-4 mRNA levels. J Infect Dis. (2007) 195:870–8. doi: 10.1086/511277
150. de Martino MLL, Galli L, Chiappini E. Immune response to mycobacterium tuberculosis: a narrative review. Front Pediatrics. (2019) 7:350. doi: 10.3389/fped.2019.00350
151. Kimuda SG, Andia BI, Sebina I, Egesa M, Nalwoga A, Smith SG, et al. Mycobacterium tuberculosis infection is associated with increased B cell responses to unrelated pathogens. Scientific Reports. (2020) 10:14324. doi: 10.1038/s41598-020-71044-4
152. WHO. Coronavirus Disease (COVID-10) Dashboard. Available online at: https://covid19whoint/table (accessed January 09, 2021). (2021). doi: 10.46945/bpj.10.1.03.01
153. Azkur AK, Akdis M, Azkur D, Sokolowska M, Van de Veen W, Bruggen MC, et al. Immune response to SARSCoV-2 and mechanisms of immunopathological changes in COVID-19. Allergy. (2020) 75:1564–81. doi: 10.1111/all.14364
154. Smits HH, Hartgers FC, Yazdanbakhsh M. Helminth infections: Protection from atopic disorders. Curr Allergy Asthma Rep. (2005) 5:42–50 doi: 10.1007/s11882-005-0053-5
155. Rajamanickam A, Munisankar S, Dolla C, Menon PA, Thiruvengadam K, Nutman TB, et al. Helminth infection modulates systemic pro-inflammatory cytokines and chemokines implicated in type 2 diabetes mellitus pathogenesis. PLoS Negl Trop Dis. (2020) 14:e0008101. doi: 10.1371/journal.pntd.0008101
156. Bradbury RS, Piedrafita D, Greenhill A, Mahanty S. Will helminth co-infection modulate COVID-19 severity in endemic regions? Nat Rev Immunol. (2020) 20:342. doi: 10.1038/s41577-020-0330-5
157. Siles-Lucus M, Javier G, Ron G, Rafael S, José P-A, Álvaro M-M. Potential influence of helminth molecules on COVID-19 pathology. Trends in Parasitology. (2021) 37:11–4. doi: 10.1016/j.pt.2020.10.002
158. da Silva VBR, Campos BR, de Oliveira JF, Decout J, de Lima MCA. Medicinal chemistry of antischistosomal drugs: Praziquantel and oxamniquine. Bioorg Med Chem. (2017) 25:3259–77. doi: 10.1016/j.bmc.2017.04.031
159. Bustinduy AL, Waterhouse D, de Sousa-Figueiredo JC, Roberts SA, Atuhaire A, Van Dam GJ, et al. Population pharmacokinetics and pharmacodynamics of praziquantel in Ugandan children with intestinal schistosomiasis: higher dosages are required for maximal efficacy. mBio. (2016) 4:e00227–16. doi: 10.1128/mBio.00227-16
160. Colley DG, Secor WE. Immunology of Human schistosomiasis. Parasite Immunol. (2014) 36:347–57. doi: 10.1111/pim.12087
161. Cupit MP, Cunningham C. What is the mechanism of action of praziquantel and how might resistance strike? Fut Med Chem. (2015) 7:701–5. doi: 10.4155/fmc.15.11
162. Chan JD, Zarowiecki M, Marchant JS. Ca2 + channels and Praziquantel: A view from the free world. Parasitol Int. (2013) 62:619–28. doi: 10.1016/j.parint.2012.12.001
163. Winkelmann F, Frank M, Rabes A, Koslowski N, Schulz C, Bischofsberger M, et al. Human serum activates the tegument of female schistosomes and supports recovery from Praziquantel. Parasitol Res. (2020) 120:209–21. doi: 10.1007/s00436-020-06968-x
164. Dupre L, Herve M, Schacht A, Capron A, Riveau G. Control of schistosomiasis pathology by combination of Sm28GST DNA immunization and praziquantel treatment. JID. (1999) 180:454–63. doi: 10.1086/314875
165. Fitzsimmons CM, Joseph S, Jones FM, Reimert CM, Hoffmann KF, Kazibwe F, et al. Chemotherapy for schistosomiasis in uganda fishermen: treatment can cause a rapid increase in il-5 levels in plasma but decreased levels of eosinophilia and worm-specific immunoglobulin E. Infect Immun. (2004) 72:4023–30. doi: 10.1128/IAI.72.7.4023-4030.2004
166. Mutapi F, Burchmore R, Mduluza T, Foucher A, Yvonne Harcus Y, Nicoll Gea. Praziquantel treatment of individuals exposed to schistosoma heamatobium enhances serological recognition of defined parasite antiegens. J Infect Dis. (2005) 192:1108–18. doi: 10.1086/432553
167. Rujeni N, Nausch N, Midzi N, Cowan GJ, Burchmore R, Cavanagh DR, et al. Immunological consequences of antihelminthic treatment in preschool children exposed to urogenital schistosome infection. J Trop Med. (2013) 2013:283619. doi: 10.1155/2013/283619
168. Reda ES, Ouhtit A, Abdeen SH, El-Shabasy EA. Structural changes of Schistosoma mansoni adult worms recovered from C57BL/6 mice treated with radiation-attenuated vaccine and/or praziquantel against infection. Parasitol Res. (2012) 110:979–92. doi: 10.1007/s00436-011-2583-1
169. Tweyongyere R, Nassanga BR, Muhwezi A, Odongo M, Lule SA, Nsubuga RN, et al. Effect of Schistosoma mansoni infection and its treatment on antibody responses to measles catch- up immunisation in pre-school children: A randomised trial. PLoS Negl Trop Dis. (2019) 13:e0007157. doi: 10.1371/journal.pntd.0007157
170. Mutapi F. Heterogeneities in anti-schistosome humoral responses fol- lowing chemotherapy. Trends Parasitol. (2001) 17:518–24. doi: 10.1016/S1471-4922(01)02118-3
171. Oliviera RR, Figueiredo JP, Cardoso LS, Jabar RL, Souza RP, Wells MT, et al. Factors associated with resistance to Schistosoma mansoni infection in an endemic area of Bahia, Brazil. Am J Trop Med Hyg. (2012) 86:296–305. doi: 10.4269/ajtmh.2012.11-0204
172. Joseph S, Jones FM, Walter K, Fulford AJ, Kimani G, Mwatha JK, et al. Increases in human T helper 2 cytokine responses to Schistosoma mansoni worm and worm-tegument antigens are induced by treatment with praziquantel. J Infect Dis. (2004) 190 835–42. doi: 10.1086/422604
173. Leenstra T, Acosta LP, Wu HW, Langdon GC, Solomon JS, Manala DL, et al. T-helper-2 cytokine responses to Sj97 predict resistance to reinfection with Schistosoma japonicum. Infect Immun. (2006) 74:370–81. doi: 10.1128/IAI.74.1.370-381.2006
174. Scott JT, Turner CMR, Mutapi F, Woolhouse MEJ, Chandiwana SK, Mduluza T, et al. Dissociation of interleukin-4 and interleu- kin-5 production following treatment for Schistosoma haematobium infection in humans. Parasite Immunol. (2000) 22:341–8. doi: 10.1046/j.1365-3024.2000.00311.x
175. Naglaa F, Abd E, Faten AM, Magdy M. Immunoprotection of soluble egg antigens and praziquantel in challenged murine schistosoma mansoni. J Egypt Soc Parasitol. (2017) 47:1–12.
176. Schmiedel Y, Mombo-Ngoma G, Labuda LA, Janse JJ, de Gier B, Adegnika AA, et al. CD4+CD25hiFOXP3+ regulatory T cells and cytokine responses in human schistosomiasis before and after treatment with praziquantel. PLoS Negl Trop Dis. (2015) 9:e0003995. doi: 10.1371/journal.pntd.0003995
177. Labuda LA, adegnika AA, Rosa BA, Martin J, Ateba-Ngoa U, Amoah AS, et al. A praziquantel treatment study of immune and transcriptome profiles in schistosoma haematobium-infected gabonese schoolchildren. J Infect Dis. (2020) 222:2103–13. doi: 10.1093/infdis/jiz641
178. Rabia I, Nagy F, Aly E, Mohamed A, EL-Assal F, El-Amir A. Effect of treatment with antifibrotic drugs in combination with PZQ in immunized schsitosoma mansoni infected murine model. J Am Sci. (2010) 6:208–16. doi: 10.1016/S1201-9712(10)60047-1
179. Jamrozik E, Selgelid MJ. Human infection challenge studies in endemic settings and/or low-income and middle-income countries: key points of ethical consesus and controversy. J Med Ethics. (2020) 46:601–9. doi: 10.1136/medethics-2019-106001
180. Darton TC, Blohmke CJ, Moorthy VS, Altmann DM, Hayden FG, Clutterbuck EA, et al. Design, recruitment, and microbiological considerations in human challenge studies. Lancet Infect Dis. (2015) 15:840–51. doi: 10.1016/S1473-3099(15)00068-7
181. Langenberg MCC, Hoogerwerf M, Koopman JPR, Janse JJ, Osterhoud JK, Feijt C, et al. A controlled human Schistosoma mansoni infection model to advance novel drugs, vaccines and diagnostics. Nat Med. (2020) 26:326–32. doi: 10.1038/s41591-020-0759-x
182. Roestenberg M, Hoogerwerf MA, Ferreira DM, Mordmüller B, Yazdanbakhsh M. Experimental infection of human volunteers. Lancet Infect Dis. (2018) 18:e312–e22. doi: 10.1016/S1473-3099(18)30177-4
183. Janse JJ, Langenberg MCC, Kos-Van OJ, Ozir-Fazalalikhan A, Brienen EAT, Winkel BMF, et al. Establishing the production of male schistosoma mansoni cercariae for a controlled human infection model. J Infect Dis. (2018) 218:1142–6. doi: 10.1093/infdis/jiy275
184. Elliott AM, Roestenberg M, Wajja A, Opio C, Angumya F, Adriko M, et al. Ethical and scientific considerations on the establishment of a controlled human infection model for schistosomiasis in Uganda: report of a stakeholders' meeting held in Entebbe, Uganda. AAS Open Res. (2018) 1:2. doi: 10.12688/aasopenres.12841.1
185. Gryseels B, Polman K, Clerinx J, Kestens L, Human schistosomiasis. Lancet. (2006) 368:1106–18: doi: 10.1016/S0140-6736(06)69440-3
186. Sissoko MS, Healy SA, Katile A, Omaswa F, Zaidi I, Gabriel EE, et al. Safety and efficacy of PfSPZ Vaccine against Plasmodium falciparum via direct venous inoculation in healthy malaria-exposed adults in Mali: a randomised, double-blind phase 1 trial. Lancet Infect Dis. (2017) 17:498–509. doi: 10.1016/S1473-3099(17)30104-4
187. Jongo SA, Shekalaghe SA, Church LWP, Ruben AJ, Schindler T, Zenklusen I, et al. Safety, immunogenicity, and protective efficacy against controlled human malaria infection of Plasmodium falciparum sporozoite vaccine in Tanzanian adults. Am J Trop Med Hyg. (2018) 99:338–49. doi: 10.4269/ajtmh.17-1014
Keywords: schistosomiasis, vaccine-candidate, praziquantel, co-infections, endemic, vaccine response, co-administration, co-delivery
Citation: Driciru E, Koopman JPR, Cose S, Siddiqui AA, Yazdanbakhsh M, Elliott AM and Roestenberg M (2021) Immunological Considerations for Schistosoma Vaccine Development: Transitioning to Endemic Settings. Front. Immunol. 12:635985. doi: 10.3389/fimmu.2021.635985
Received: 30 November 2020; Accepted: 11 February 2021;
Published: 04 March 2021.
Edited by:
Rashika El Ridi, Cairo University, EgyptReviewed by:
Donald McManus, The University of Queensland, AustraliaAlan Wilson, University of York, United Kingdom
Kenji Hirayama, Nagasaki University, Japan
Birgitte Vennervald, University of Copenhagen, Denmark
Copyright © 2021 Driciru, Koopman, Cose, Siddiqui, Yazdanbakhsh, Elliott and Roestenberg. This is an open-access article distributed under the terms of the Creative Commons Attribution License (CC BY). The use, distribution or reproduction in other forums is permitted, provided the original author(s) and the copyright owner(s) are credited and that the original publication in this journal is cited, in accordance with accepted academic practice. No use, distribution or reproduction is permitted which does not comply with these terms.
*Correspondence: Meta Roestenberg, bS5yb2VzdGVuYmVyZ0BsdW1jLm5s