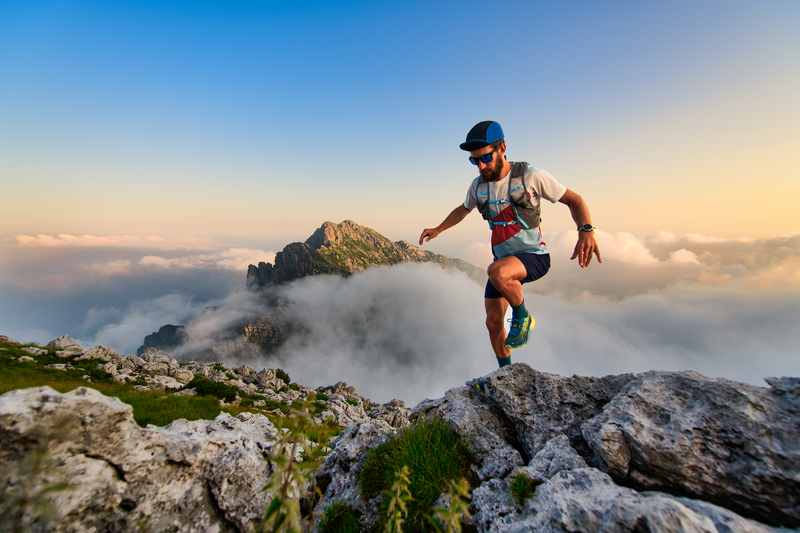
95% of researchers rate our articles as excellent or good
Learn more about the work of our research integrity team to safeguard the quality of each article we publish.
Find out more
REVIEW article
Front. Immunol. , 08 April 2021
Sec. Microbial Immunology
Volume 12 - 2021 | https://doi.org/10.3389/fimmu.2021.616713
This article is part of the Research Topic Interactions of the gut microbiota and the innate immune system View all 18 articles
In order to inhibit pathogenic complications and to enhance animal and poultry growth, antibiotics have been extensively used for many years. Antibiotics applications not only affect target pathogens but also intestinal beneficially microbes, inducing long-lasting changes in intestinal microbiota associated with diseases. The application of antibiotics also has many other side effects like, intestinal barrier dysfunction, antibiotics residues in foodstuffs, nephropathy, allergy, bone marrow toxicity, mutagenicity, reproductive disorders, hepatotoxicity carcinogenicity, and antibiotic-resistant bacteria, which greatly compromise the efficacy of antibiotics. Thus, the development of new antibiotics is necessary, while the search for antibiotic alternatives continues. Probiotics are considered the ideal antibiotic substitute; in recent years, probiotic research concerning their application during pathogenic infections in humans, aquaculture, poultry, and livestock industry, with emphasis on modulating the immune system of the host, has been attracting considerable interest. Hence, the adverse effects of antibiotics and remedial effects of probiotics during infectious diseases have become central points of focus among researchers. Probiotics are live microorganisms, and when given in adequate quantities, confer good health effects to the host through different mechanisms. Among them, the regulation of host immune response during pathogenic infections is one of the most important mechanisms. A number of studies have investigated different aspects of probiotics. In this review, we mainly summarize recent discoveries and discuss two important aspects: (1) the application of probiotics during pathogenic infections; and (2) their modulatory effects on the immune response of the host during infectious and non-infectious diseases.
The term probiotic is derived from the Greek word (πρoβιoτικó: πρó and óς) meaning “for life” (1, 2). Probiotics have a very old history since their first description; the first clinical trial investigating the remedial effects of probiotics in constipation was started in 1930 (3). Probiotics have a wide range of applications in poultry, livestock, aquaculture, and also in humans for the prevention and treatment of disorders, ailments, and infectious and non-infectious diseases (e.g., bacterial, viral, parasitic, or fungal diseases, nervous system disorders, obesity, cancer, and allergic problems), as well as preoperative and postoperative processes. Nowadays, probiotics are an inevitable part of human nutrition with elevated consumption levels through naturally and microbially fermented products with enormous amounts of viable beneficial microbes, such as fermented animal products, fermented fruits and their juices, and various other food products (4). Different probiotics like Lactobacillus, Lactococcus, Leuconostoc, Pediococcus, Enterococcus, Vagococcus, Bacillus, Clostridium butyricum, Micrococcus, Rhodococcus, Brochothrix, Kocuria, Pseudomonas, Aeromonas, Shewanella, Enterobacter, Roseobacter, Vibrio, Zooshikella, Flavobacterium, and some yeasts are commonly used probiotics to control infectious diseases as well as to improve health and quality of aquaculture production (5, 6). The application of specific probiotics culture in the poultry and livestock industry has become very common in recent days. Many economically important poultry diseases like Salmonellosis, Clostridial diseases, Coccidiosis etc., respond positively during probiotics treatment (7). Genus Bacillus, Pediococcus, Lactobacillus, Enterococcus, Streptococcus, Aspergillus, and Saccharomyces are usually used in poultry (1).
To increase meat production and inhibit pathogenic growth, antibiotics are usually supplemented in the feed of poultry and livestock leading to the emergence of antibiotic-resistant bacteria. Antibiotic-resistant bacteria are becoming very common, presenting difficulties to the treatment of clinical infections with current chemotherapeutics, thus effective and novel strategies which will enable the host immune system to combat the infections are urgently needed (8). Probiotics exert beneficial effects to their hosts by diverse mechanisms, e.g., antimicrobial peptide (AMP) production, fatty acids production, stabilization of disturbed intestinal microflora, competitive pathogen exclusion, and modulation of host innate and adaptive immune responses (9). Nowadays, strategies using probiotics as an immunomodulator to control infectious diseases have become popular. Antimicrobial effects of probiotics by modulating the innate and adaptive immune responses of hosts have been extensively reported in numerous in vitro and in vivo studies.
Immune cells or epithelial cells can express a series of pattern recognition receptors (PRRs). The typical PRRs consist of Toll-like receptors (TLRs), retinoic acid-inducible gene-I-like receptors (RLRs), nucleotide oligomerization domain (NOD)-like receptors (NLRs), and C-type lectin receptors (10). Pathogen-associated molecular patterns (PAMPs) of probiotics interact with PRRs to initiate appropriate signaling pathways for the expression of different genes and subsequent production of immune mediators, which help the hosts to counteract the pathogenic infections (11). Besides these immune remedial effects, probiotics also provide other health-promoting effects on hosts. Indigenous microbiota possess different biological activities extending from anabolism to catabolism of large molecules, resulting in beneficial effects on host health as well as microbiota themselves. Intestinal microflora can ferment endogenous mucus and indigestible diet residues and produce vitamins, such as vitamin K and B (12). The following sections of this review provide a brief introduction to probiotics and discuss the mechanism of probiotic functions and their application during pathogenic infections.
In the early 1900s, Louis Pasteur asserted that microorganisms were responsible for food fermentation, while Élie Metchnikoff stated that the increased longevity of individuals living in the rural areas of Bulgaria was closely associated with the daily consumption of fermented dairy products, such as yogurt. He claimed that lactobacilli could mitigate the putrefactive effects of gastrointestinal metabolism, which contributed to diseases and aging. Approximately 2,000 years earlier, Hippocrates claimed that “death sits in the bowl” (13). Fermented foods have a long history; fermented milk can be traced back to the Neolithic age. The fermentation of milk was first reported around 10,000 BC in the Middle East and India, and around 7,000–5,000 BC in Egypt, Rome, Greece, and the rest of Europe. The first appearance of soy sauce is estimated around 4,000 BC and 3,000 BC in China, Japan, and Korea; fermented rice first appeared around 2,000 BC in Asia. Fish sauce originated from northern Africa and South East Asia around 1,000 BC. The use of wine possibly started in North Africa around 3,000 BC, and subsequently expanded in the Middle East, Greece, Egypt, and Rome. The use of beer may have started around 7,000 BC in China and probably around 5,000 BC in Mesopotamia (2, 14) (Table 1).
A number of microbes have been used as probiotics. The number of microbial organisms with probiotic characteristics is remarkable. Among them, lactic acid bacteria (LAB) are a group of non-spore forming, Gram-positive rods or cocci with tolerability to markedly low pH; they are fermenters of carbohydrates and use carbon as final electron acceptors. LAB have a wide range of applications and are the most commonly used probiotics (15, 16). They are classified on the basis of their cellular morphology and glucose fermentation mode, into Phylum-Firmicutes, Class-Bacilli, and Order-Lactobacillales. Currently, the LAB genera include Lactobacillus, Streptococcus, Leuconostoc, Carnobacterium, Lactococcus, Aerococcus, Enterococcus, Pediococcus, Oenococcus, Weissella, Alloiococcus, Tetragenococcus, Dolosigranulum, and Vagococcus (17, 18). The most frequently utilized genera of bacteria used in probiotic formulations are Lactobacillus, Enterococcus, Streptococcus, Bacillus, and Bifidobacterium, as well as some fungal strains of the genus Saccharomyces, such as Saccharomyces boulardii (S. boulardii). Most of these are regarded as the intestinal commensal microbiota (2).
The process for the identification of newly-isolated probiotic candidates is the first problem that needs to be addressed. From isolation to market launching, knowledge needs to be collected on host health, adhesion properties, and resistance to host biochemical environments. Probiotics must be safe, adhere to the lining of intestinal cells with high survival potential, have an immunostimulatory function, have the ability to colonize the tract lumen, withstand exposure to low pH and bile salt, and should have antipathogenic characteristics (19, 20). Other probiotic properties may be considered for selecting probiotic strains with cognitive effects, such as their ability to lower cholesterol, antioxidant function, or cytotoxic impact on cancer cells. Of note, a prospective probiotic does not need to follow or meet all aforementioned selection criteria (21). Figure 1 shows some properties of good probiotics.
The microbiota inhabiting the animal body provide crucial services to the ecosystem, such as the production of important resources and bioconversion of different nutrients, which are beneficial for both the host and microbes. Microbiota can execute different crucial biological activities, ranging from anabolism to catabolism of large molecules. These biological activities can be beneficial for host health and the microbes. The metabolic functions of intestinal microflora reduce the energy costs of their host, as they ferment endogenous mucus and indigestible food residues, and also produce vitamins such as vitamin K and B (12). Therefore, due to their biological activities, probiotics have positive health effects on hosts, including reduction of the energy required during digestion and provision of beneficial nutrients. Different kinds of commercially available probiotics products are available to boost the health of adults and children (www.probioticchart.ca, www.usprobioticguide.come) (22).
Because of the substantial decrease in their viability in the harsh gastrointestinal environment of the host (gastric pH, protease, lipase, and peristalsis) and during different food processing and storage conditions (high temperature, pH changes, oxygen, and hydrogen), the possible beneficial health effects of probiotics may not be recognized. A number of systems have been designed to improve orally administered probiotics viable number in gastrointestinal tract (GIT), including coating and embedding systems (23). Microencapsulation is an efficient technique that is used to increase the viability and resistance of probiotics against the harsh environmental conditions of GIT and during storage conditions. Microencapsulation is a physicochemical or mechanical process in which probiotics are usually inserted or coated with food-grade materials like lipids, biopolymers, or other hydrocolloidal materials, providing protection against adverse conditions such as heat shock, low pH, bile salts, cold shock, etc. (24). Several studies have been reported that microencapsulation increases the viability of probiotics Encapsulation of Bifidobacterium longum with milk increases its viability during storage time (25). Lactococcus lactis subsp. cremoris LM0230 encapsulation in alginate increases its stability and viability (26). Similarly, Lactobacillus rhamnosus GG encapsulation with pectin increases its viability in simulated GIT conditions. Muhammad et al. (27) reported Lactobacillus plantarum KLDS 1.0344 ability to alleviate chronic lead toxicity in mice increases when encapsulated with starch originated from tomatoes (27). The study of Riaz et al. (28) shows that the survival rate under simulated GIT conditions of zein-coated alginate Bifidobacterium bifidum significantly increases.
The mechanisms of probiotic function are complex, heterogeneous, and specific to probiotic strains. They include competitive exclusion of pathogens (29), ability to colonize the intestine (30), intestinal barrier function improvement by increasing the expression of tight junction proteins and mucin expression along with the interaction of PAMP to PRRs, AMP production (31), and immune system regulation. Some important mechanisms are briefly discussed below.
This refers to a condition in which one bacterial species has a greater potential to attach the epithelia, through a receptor, than other species (11). The known mechanisms of competitive exclusion mainly include lowering the pH in the lumen, contesting for nutrient utilization, and AMP production against competitors (32). Interaction between molecules distributed in the gut epithelia and the surface of bacterial cells mediates the adhesion and colonization of bacteria. Commensal or probiotic bacteria produce adhesive surface molecules (e.g., enolases, glyceraldehyde-3-phosphate, and pyruvate dehydrogenase) and adhere to the extracellular matrix of the host (33, 34). These adhesive surface molecules assist commensal bacteria and probiotics in contesting and preventing pathogenic bacterial attachment and colonization (35, 36). Lactobacillus fermentum (L. fermentum) competitively binds to collagen I of host epithelial cells by expressing its collagen-binding protein genes and inhibits the binding of Campylobacter jejuni. Similarly, Lactobacillus gasseri expresses aggregation-promoting factors on their cell surface, which helps in self-aggregation and its binding with the host extracellular matrix fibronectin component. This facilitates the colonization of probiotics and the exclusion of pathogens from the GIT (37). L. gasseri also inhibits the adhesion of Helicobacter pylori (H. pylori) to AGS gastric epithelial cell lines by expressing its Sortase A (srtA) gene, which produces surface molecules that facilitate L. gasseri aggregation, as well as binding and adhesion to AGS cell lines (38). Pretreatment with some probiotics impedes pathogenic bacterial attachment to host cell receptor sites by steric hindrance pose, and reduces the colonization of unwanted microbes by producing negative growth factors for pathogens (39). Seaweed Bacillus probiotics have good adhesion properties to shrimp intestinal mucosa with competitive exclusion ability and eliminate Vibrio parahaemolyticus strain 3HP (40).
Competitive exclusion of probiotics exerts the beneficial effects on the GIT and other organs of the host, increases the adhesion of probiotics, and performs protective actions against pathogens by competing for binding sites of the host. Furthermore, this adhesion of probiotics increases the opportunity for interaction with the host, which favors the immunostimulatory effects of probiotic surface molecules (ligands for receptors of the host) and their metabolites (41, 42). Therefore, the competitive exclusion properties of probiotics offer several benefits to host health, including the reduction of pathogenic attachment, colonization (many diseases arise because of pathogen colonization), further spread of the pathogen, and pathogenic load in hosts. Furthermore, this property of probiotics enables them to colonize the host GIT, which is necessary for the further beneficial action of probiotics to their hosts.
The potential of probiotics to colonize the intestine is one of the most important properties recommended by WHO/The Food and Agriculture Organization of the United Nations (FAO). The positive characteristics of probiotics, such as antagonisms to harmful microbes or the modulation of the immune system, are linked to their intestinal colonization, which is investigated in vitro using simulated intestinal cells, as in vivo investigation is difficult (43). The adhesion of LAB with intestinal cells has been extensively reported. Interaction between molecules distributed on gut epithelia and the surface of bacterial cells mediates the adhesion and colonization of bacteria and is highly variable between different bacterial strains. García-Ruiz et al. (44) reported 0.37–12.2% adhesion of wine-isolated LAB (44) and Pisano et al. (45) reported 3–20% adhesion of LAB (45).
As the intestinal epithelial barrier acts as a physical and biochemical barrier and is important for preventing systemic entry of toxins, bacteria, and other foreign unwanted compounds, so its integrity and full function are quite important. It has been reported in many studies that LAB can improve intestinal epithelial barrier damage induced by pathogenic infection (46–51). Probiotics possess a diverse mechanism of action to improve the intestinal barrier function and maintain homeostasis. “Lactobacillus contains a HSP27-inducible polyphosphate (poly P) fraction. Probiotic-derived polyphosphates, strengthen the epithelial barrier function and keep intestinal homeostasis through the integrin-p38 MAPK pathway” (52). Lactobacillus casei DN-114 001 and Lactobacillus acidophilus strain LB have the potential to improve intestinal epithelial barrier during Escherichia coli infection (53, 54). Strains of Lactobacillus, Bifidobacterium, and Streptococcus stimulate tight junction proteins (occludin, claudin-1) results in enhanced barrier stability (55). L. plantarum WCFS1 significantly increases occludin and ZO-1 in tight junction vicinity by TLR2 dependent pathway and protect tight junction disruption by toxins, pathogens, and cytokines (49). Qin et al., also showed that L. plantarum has protective effects on intestinal barrier by rearranging tight junction proteins (occludin, claudin-1, JAM-1 ZO-1) disturbed by E. coli and ameliorates barrier function (50). Another strain of L. plantarum, MB452 increases occludin expression and improves intestinal barrier integrity (46). E. coli Nissle 1917 (EcN) ameliorates E. coli induced intestinal epithelial barrier dysfunction by regulating the expression of occludin and claudin (56). L. rhamnosus (LR: MTCC-5897) and L. fermentum (LF: MTCC-5898) significantly improve the E. coli disturbed tight junction proteins (Occludin, ZO-1, cingulin-1, claudin-1) in Caco-2 cells (57).
Several other reports of Lactobacilli study have also been shown that Lactobacilli ameliorate the intestinal barrier damage and pro-inflammatory cytokines production induced by Salmonella (47, 58). Probiotics are also effective to improve malnutritional induced intestinal barrier damaged as indicated by the study of Garg et al. on a malnutritional mice model, in which they reported that Lactobacillus reuteri LR6 feeding significantly improves the intestinal morphology damaged during malnutrition (59).
Different criteria are applied to AMP classification according to their source (animals, fungi, plants, and bacteria), mechanisms of action (AMP acting on cell surface molecules or intracellular components), structure (patterns of covalent bonding), and biosynthetic pathway (non-ribosomally synthesized or ribosomally synthesized) (60). Bacteriocins (AMP from prokaryotes) of LAB are classified into three classes: Class I, post-translationally modified (e.g., lantibiotics); Class II, non-modified, heat stable with size <10 kDa (e.g., pediocin PA1, leucocin A, plantaricin A, and enterocin X); and Class III, heat labile, large peptides with size >30 kDa (e.g., helveticin J) (16). Bacteriocins have low molecular weight and form pores in target cell membranes, leading to the death (61) of pathogenic bacteria, and also act as anti-cancerous agents. Furthermore, bacteriocins also possess immunomodulatory properties with pronounced anti-inflammatory effects during pathogenic infections. As bacteriocins are non-toxic, particularly those derived from LAB, they are used in food preservation. A number of studies showed that certain kinds of probiotics inhibit many types of pathogenic bacteria (proteus spp., E. coli, Acinetobacter baumannii, Pseudomonas aeruginosa, Klebsiella pneumoniae, Listeria monocytogenes, Citrobacter freundii, H. pylori, Enterobacter aerogenes, Compylobacter jejuni, Micrococcus luteus, Salmonella spp., Shigella spp., and some fungi) by the action of their bacteriocins (62). Bacteriocins from Lactobacillus salivarius inhibit foodborne and other medically important bacteria, such as Listeria monocytogenes, many genera of staphylococcus, Neisseria gonorrhoeae, Bacillus, and Enterococcus; the bacteriocins kill these bacteria by creating membrane pores and subsequent leakage of cellular material. Further, these bacteriocins also assist L. salivarius colonization in the intestine without showing any prominent adverse effects on other lactic acid bacteria (63) L. plantarum also exerts antimicrobial activities by producing many types of bacteriocins with antimicrobial effects against food spoilage bacteria, such as Alicyclobacillus acidoterrestris (64), Salmonella spp., Listeria monocytogenes, Staphylococcus aureus, and E. coli; thus, they may be used as preservatives for pork meat (65). Apart from bacteria, some bacteriocins from L. plantarum are also effective against yeast and molds, such as Fusarium, Candida, Aspergillus, and Mucor (66). Bacteriocins from other probiotic species markedly induce apoptosis and inhibit tumor formation, cancer cell proliferation, and membrane depolarization of cancer cells during treatment (61). There are different classification systems for AMP and, owing to their diverse mechanism of actions, they have a wide range of applications in humans and animals, as well as aquaculture fields (67). They inhibit growth and even kill diverse pathogens by creating pores in their cell membranes, as well as initiating appropriate immune responses.
It is well-established that probiotic bacteria exert an immunomodulatory effect and have the potential to communicate and interact with a series of immune cells (e.g., DCs, lymphocytes, epithelial cells, monocytes, and macrophages). The immune response generally comprises the innate immune response and adaptive immune response. Innate immune response responds to PAMPs distributed on the majority of bacteria (11). The principle immune response to any pathogen is activated following the interaction of PRRs (i.e., TLRs, NLRs, and C-type lectin receptors) with PAMPs and initiates cell signaling. Intestinal epithelial cells are the host cells that most extensively come into contact with probiotics. However, probiotics may also interact with DCs, which play a significant role in the innate immune response and bridge the innate and adaptive immune responses. Through their PRRs, both intestinal epithelial cells and DCs can communicate and react to gut microorganisms (68, 69). Under the effects of probiotics/commensal microbiota, the activated DCs induce the appropriate immune response (e.g., naïve CD4 T cells to Treg cell differentiation), which generally inhibits Th1-, Th2-, and Th17-mediated inflammatory response. Furthermore, probiotics blunt intestinal inflammation (70) by downregulating the expression of TLRs via secretion of TNF-α inhibitory metabolites and inhibition of nuclear factor-κB (NF-κB) signaling in enterocytes (68). Probiotics also modulate the expression of various kinds of cytokine production.
Probiotic benefits related to immunoregulation for the treatment of various diseases have been extensively studied. Immunomodulatory effects of probiotics are mainly due to the induction of the release of cytokines including interleukins, transforming growth factor (TGF), tumor necrosis factors (TNFs), interferons (INFs), and immune cells released chemokines, which further regulate the immune system (71, 72). Immunostimulatory and immunoregulatory actions of probiotics have been reported in various studies. Immunostimulatory probiotics are capable of acting against infection and cancer cells, inducing the release of IL-12, which stimulates the NK cells and produces the Th1 cells. By maintaining the balance between Th1 and Th2, these probiotics also work against allergies. Contrary to this finding, immunoregulatory probiotics are attributed to Treg cells and IL-10 production to blunt excessive inflammatory responses, inflammatory bowel disease, and autoimmune disorders (73, 74). So, probiotics immunomodulatory effects via cytokines are strain-specific as indicated by the in vitro study of Haller et al. (75) using Caco-2 cells in which they reported that Lactobacillus sakei is capable of inducing pro-inflammatory cytokines (IL-1β, TNFα, and IL-8) whereas Lactobacillus johnsonii induced anti-inflammatory cytokines (TGF-β) (75). A mixture of Lactobacillus paracasei and L. reuteri to Helicobacter hepaticus IL-10-defcient mice leads to reduced colitis and pro-inflammatory cytokines production (76). Kourelis et al. (77) study on Fisher-344 inbred rats and BALB/c, demonstrated that L. acidophilus NCFB 1748 and L. paracasei subsp. Paracasei DC412 induce specific immune markers and innate immune responses via recruiting polymorphonuclear cell and production of TNFα (77). Probiotics-induced cytokines expression for immune system modulation of the host has been briefly discussed in the relevant section.
Toll-like receptors and single-pass membrane-spanning receptors are very important protein receptors expressed on several non-immune (epithelial, fibroblasts) and immune [macrophages, B cells, natural killer (NK) cells, DCs] cells. Activation of the TLR signaling pathway, except TLR3 (78), generally leads to the recruitment of MyD88, which results in activation of the NF-κB and mitogen-activated protein kinase (MAPK) pathway. TLR-induced signaling is also responsible for the maturation of DCs characterized by increased expression levels of DC markers (CD80, CD83, and CD86) and chemokines receptor C-C motif chemokine receptor 7 (CCR7). TLR9 is crucial for the mediation of the anti-inflammatory effects of probiotics, though many other receptors are also involved.
Lactobacilli ligands initiate cell signaling after binding to TLR2 in combination with TLR6, endorsing dimerization and NF-κB activation via recruitment of MyD88 (79). Engagement of a bacterial ligand with TLR2 results in cytokine production and increases the transepthelial resistance for conquering microbes (79, 80). Several Lactobacillus strains induce their immunomodulatory effects by binding to TLR2, which recognizes peptidogycan (a component of the cell wall of Gram-positive bacteria). An in vitro study showed that L. plantarum and L. rhamnosus increased TLR2 expression in human cells (Caco-2). L. casei showed similar effects in Salmonella-infected and healthy mice, and induced TLR expression, as well as interleukin-10 (IL-10), interferon-gamma (IFN-γ), and TNF-α production (81, 82).
Numerous other probiotics interact with TLR4 to induce an appropriate immune response. For example, during pre-and post-Salmonella challenges in mice, L. casei increased the production of IL10, IFN-γ, and IL6, and reduced the levels of TNF-α by interacting with TLR4 (82, 83). Likewise, L. rhamnosus GG (heat-inactivated) and Lactobacillus delbrueckii subsp. Bulgaricus (L. delbrueckii) reduce TLR4 expression in DCs (human monocyte-derived) (84). TLR9, another important TLR, identifies bacterial CpG DNA and CpG-ODN (engineered unmethylated oligonucleotide mimics). Unmethylated pieces of DNA comprising CpG patterns produced from probiotics also have the propensity to mediate anti-inflammatory activities via TLR9.
In the case of the differentiated epithelium, apical, and basolateral stimulation results in the activation of different signaling pathways. Basolateral TLR9 activation causes activation of the NF-κB cascade by the degradation of IκBα. Of note, apical activation of TLR9 results in the suppression of NF-κB by the aggregation of ubiquitinated IκB in the cytoplasm (85). Apical or basolateral stimulation of these receptors is important and involves different signaling cascades leading to various immune responses. The results from the study conducted by Ghadimi et al. show that polarized T84 and HT-29 cells increase TLR9 expression in a specific manner in response to apically applied natural commensal origin DNA. They reported that when LGG DNA is applied to these cells, it attenuates TNF-α enhanced NF-κB activity by reducing IκBα degradation and p38 phosphorylation (86).
Lactobacillus plantarum-purified DNA also modulates the immune response of host cells by interacting with TLRs, as reported by Kim, whose studies show that L. plantarum-purified DNA inhibits LPS induced TNF-α production in THP-1 cells. Furthermore, L. plantarum-purified DNA blunt the expression of TLR4, TLR2, and TLR9, which induce NF-κB activation through the LPS signaling pathway, leading to pro-inflammatory cytokines upregulation (87, 88). TLRs are important membrane receptors; most intracellular signaling pathways involve the activation of membrane receptors. Furthermore, TLRs play a key role in the induction of immune response by probiotics through the recruitment of specific intracellular signaling molecules. Depending on their interaction with specific TLRs, probiotics may decrease or increase TLR expression.
In tissues with blunt TLR expression, NLRs are important and present in the cytoplasm. Thus far, more than 20 NLRs have been recognized. Among them, NOD1 and NOD2 are the most studied and important NLRs (89). NOD1 is expressed in many cells and recognizes peptidoglycan moieties (comprising Gram-negative meso diaminopimelic acid). NOD2 is mainly expressed on DCs, lungs cells, macrophages, intestinal cells, buccal epithelium, and Paneth cells. It senses muramyl dipeptide motifs which are present in a wide range of bacteria (90). NOD1 and NOD2 undergo self-oligomerization following recognition by their agonist. This results in the recruitment and activation of receptor interacting serine/threonine kinase 2 (RICK; an adaptor protein, kinase responsible for the regulation of apoptosis via CD95), which is necessary for MAPKs and NF-κB activation and the subsequent production of inflammatory mediators such as cytokines and chemoattractants. Another important signaling factor that NLRs trigger is, apoptosis-associated speck-like protein with caspase induction to trigger caspase 1 (CASP1; an adaptor protein required for the functionally effective and mature forms of pro IL18 and pro IL1). NLRs are involved in the formation of the inflammasome that results in CASP1 activation. There are three major inflammasomes named according to the NLRs involved: NOD-like receptor family pyrin domain containing protein 1 (NLRP1), NLRP3, and NLRC4. Murymyl dipeptide, bacterial and viral RNA, and lipopolysaccharides are sensed by NLRP3 (91). Many Lactobacillus species exert their immune regulatory effects via NLRs. In galactose-1-phosphate uridylyltransferase (GALT) of swine, L. gasseri and L. delbrueckii increase the expression of NLRP3 via TLR and the NOD signaling cascade, leading to appropriate activation of NLRP3. Furthermore, NOD1, NOD2, TLR2, and TLR9 agonists also enhance NLRP3 expression. L. salivarius exerts its anti-inflammatory effect by producing IL10 via regulation of NOD2 (92, 93). Probiotics modulate systemic and local immune responses of the host in a strain-specific manner by the expression of PAMPS, such as flagellin, lipopolysaccharides, CpG-DNA, and other surface proteins. PAMPs are recognized by PRRs expressed on numerous immune and epithelial cells. TLRs, C-lectin type receptors, and NLRs are the best studied PRRs. PRRs have broad specificity and their limited number can recognize a wide range of PAMPs. Interaction between PAMPs and PRRs results in the activation of multiple molecular signaling cascades that generate a specific cellular response against the encountered microbes.
The NF-κB pathway is involved in many pathological conditions and controls the expression of many (~150) pro-inflammatory and anti-inflammatory cytokines genes. These genes are extensively involved in both adaptive and innate immune responses. NF-κB is found in nearly all types of cells (94, 95). Many probiotics regulate the activation of the NF-κB pathway. L. casei inhibits Shigella fexneri-induced activation of the NF-κB pathway (96). L. rhamnosus and Lactobacillus helveticus downregulate the Th1 pro-inflammatory response and improve Th2 response during Citrobacter rodentium infection (97). Bifdobacterium lactis inhibits IκBα degradation during colitis (98). Some researchers have claimed that dietary yeast downregulates TLR2, NF-κβ p65, MyD88, IL8, and IL1β (99). L. reuteri, L. casei, and L. paracasei show anti-inflammatory characteristics via NF-κB pathway regulation; for example, L. reuteri decreases the expression of inflammatory mRNA cytokines production and increases anti-inflammatory cytokines production, and also improves the production of apoptosis-inhibiting proteins to improve cell survival and its immune response. L. reuteri do this by interfering the ubiquitination of IκB and nuclear translocation of p65 (NF-κB subunit), respectively (100–102). L. casei and L. paracasei hinder the production of pro-inflammatory cytokines by inhibiting the phosphorylation of IκBα and nuclear translocation of p65, and also reverse the degradation of IκBα (103, 104). Similar inhibitory effects on the NF- κB pathway have been shown by L. plantarum and L. brevis. L. plantarum inhibits NF-κB-activating factors by decreasing the binding activity of NF-κB (105), while L. brevis prevents interleukin 1 receptor associated kinase 1 (IRAK1) and AKT phosphorylation (106). Bifdobacterium infantis and Streptococcus salivarius also reduce NF-κB activation (101).
Besides these probiotics have several other mechanisms of action related to antifungal, antibacterial, antiviral, antiparasitic, antiallergic, anti-cancerous, antidiabetic, amelioration of the cardiovascular system, the reproductive system, and the central nervous system which has been briefly discussed in the relevant section.
Probiotics have a wide range of applications covering numerous non-infectious and infectious diseases, including bacterial, viral, parasitic, fungal, and many other non-infectious diseases. They exert anti-pathogenic effects by modulating both the innate and adaptive immune responses of the host.
Due to the several disadvantages associated with the preventive use of antibiotics, strict controls have been introduced to prohibit or reduce their use during the treatment of bacterial diseases. In the last three decades, the dietary application of feed additives has been attracting attention as a replacement for antibiotics. Probiotics have been among the most effective feed additives for the control or treatment of bacterial diseases (5). Immune modulatory therapies with probiotics for some selected pathogens are briefly discussed below (Table 2).
Probiotics may be used as alternatives to the prophylactic use of drugs for the control and prevention of salmonellosis (137). Salmonella causes a foodborne disease in both animals and humans with high morbidity (93.8 million human infections) and mortality (155,000 deaths) worldwide annually (138–142). After attachment and internalization into the lamina propria, Salmonella induces an inflammatory response, e.g., release of pro-inflammatory cytokines, followed by inflammation, ulceration, diarrhea, and destruction of the mucosa (143). Persistent infection is established due to the ability of Salmonella to evade the host immune system (144). The persistence of infection is further aided by virulent factors of Salmonella that are responsible for the clonal deletion of CD+ T cells (145).
When administered in adequate amounts, probiotics have the ability to modulate the expression of immune-related cytokines, including interleukins IL4, IL6, IL12, IFN-γ, and IL1β in lymphoid cells during Salmonella infection (47, 107, 108, 142, 146). L. rhamnosus S1K3 maintains IL-4 and IL-12 protein levels and reduces TGFβ during the late stage of Salmonella enterica serovar Typhimurium (S. Typhimurium) NCDC infection in mice and also increases the level of IgA secreting cells in lamina propria, IgA in serum, and secretory IgA level in intestinal fluids during S. Typhimurium NCDC infection in mice. This probiotic also reduces the S. Typhimurium NCDC count in feces, prevents its further spread in the liver, spleen, and intestine of mice, and improves overall health. Furthermore, in an in vitro study on Caco-2 cells, L. rhamnosus S1K3 improves the tight junction proteins (occludin and claudin-1) (107). The production of IFN-γ, a pro-inflammatory cytokine, is induced by Salmonella. IFN-γ delays recovery from intestinal inflammation, boosts inflammatory mediators [TNF, ILβ, inducible nitric oxide synthase (iNOS)], and hampers IL22- and lectin REGIIIβ-mediated antimicrobial defense (147). Probiotics beneficially regulate the immune response of the host and suppress the expression of pro-inflammatory cytokines and subsequent inflammation. IFN-γ is suppressed by the anti-inflammatory action of probiotics, greatly reducing the severity of Salmonella infection. During salmonellosis, immune players, macrophages, and monocytes secrete IL6, which serves as a pro-inflammatory cytokine and its expression levels are reduced by Lactobacillus spp. for the effective and rapid prevention of Salmonella infection in broiler chickens (47). A study conducted by Chen et al. showed that L. plantarum (LPZ01) reduces S. Typhimurium load, IFN-γ expression, TNF-α level, and associated inflammation in broiler chickens by regulating the expression of certain miRNAs involved in immune regulation and inflammatory responses (108). Supplementations with some probiotics increase the activation of B cells and antibody production by increasing IL10 expression. The latter is an important immunoregulatory and anti-inflammatory cytokine involved in antibody production during Salmonella infection. L. casei (DBN023) improves, regulates, and enhances intestinal immune functions, while cytokines balance and reverse the detrimental effects of Salmonella pullorum, characterized by higher levels of anti-inflammatory cytokines (IL10) and lower levels of pro-inflammatory cytokines (TNF-α, IFN-γ, and IL17). During prophylactic feeding of probiotics in chicken infected by Salmonella pullorum, L. casei (DBN023) increases villi height and muscle thickness and reduces Salmonella pullorum-associated mortality and pathological changes in intestinal epithelial tissues (58). L. casei CRL 431 also increases the expression of IL10 to reduce the severity of S. Typhimurium infection in BALB/c mice (82). In this manner, probiotics improve the host immune response by hampering the overexpression of inflammatory cytokines, as well as increasing the expression of anti-inflammatory cytokines and production of anti-Salmonella antibodies to blunt the severity of Salmonella infection.
Some yeasts are also used as immunobiotics and are effective in reducing Salmonella infection. The study by Martins et al. shows that Saccharomyces cerevisiae strain 905 (S. cerevisiae 905) protects and reduces the mortality of mice, orally challenged by Salmonella Typhimurium (109), and also significantly reduces the translocation of S. Typhimurium to the liver of gnotobiotic mice, and to other organs (Peyer's patches, the spleen, the mesenteric lymph nodes, and the liver) of the conventional mice. The same author in another study shows that this strain increases the number of Kupffer cells in the liver and induces a higher level of secretory IgA in the intestinal contents and IgA and IgM in the serum of mice (110). Furthermore, this strain reduces pro-inflammatory cytokines (IL-6, TNF-α, and IFN-γ) levels and modulates activation of MAPK (p38 and JNK, but not ERK1/2), NF-κB and activator protein-1, signaling pathways which are involved in transcriptional activation of pro-inflammatory mediator during Salmonella infection (111). Another yeast strain S. boulardii reduces S. Typhimurium induced IL-8 production in T84 cells by exerting its inhibitory effects on S. Typhimurium induced activation of the MAPKs ERK1/2, p38, and JNK as well as on activation of NF-kB (112). S. boulardii possesses the capability to bind with S. Typhimurium leading to reduced organ translocation of this pathogen, which results in decreased activation of MAPK (p38, JNK, and ERK1/2), phospho-IkB, p65-RelA, phospho-jun, and c-fos in the colon and signal pathways, involved in the activation of inflammation, induced by S. Typhimurium kB (148). Therefore, yeast can survive in host GIT, colonize there, reduce the pathogenic load from the host, and can modulate the immune response of their hosts toward a beneficial pattern.
A series of studies show that short-chain fatty acids (SCFAs) exert diverse beneficial effects on the health of the host gut and body (e.g., anti-inflammatory effects, prevention of histone deacetylases, and suppression of NF-κB resulting in IL1β downregulation), and play a vital role in maintaining intestinal homeostasis. Many probiotics possess regulatory properties for SCFA and can directly or indirectly increase their production. L. acidophilus reduces S. Typhimurium-induced inflammation directly by increasing the production of SCFA and indirectly by increasing that of other SCFA-producing gut bacteria (149). Moreover, L. acidophilus balances Salmonella-induced dysbiosis in infected mice (150).
Other probiotics have also shown beneficial effects on the prevention of Salmonella infection and inhibit the pathogenesis of Salmonella at initial steps. L. plantarum (MTCC5690) improves the intestinal defense through modulation of TLR2 and TLR4, and prevents the colonization and further spread of Salmonella in mice (151). Similarly, E. faecium (PXN33) in combination with L. salivarius (59) also inhibits Salmonella Enteritidis colonization in the GIT of poultry (152). Supplementation of probiotics greatly reduced the severity of Salmonella infection by their immunomodulatory mechanisms of action. As probiotics decrease the expression of inflammatory cytokines and increase the antibody production and anti-inflammatory cytokine expression during salmonellosis, supplementation can improve the overall health of the host.
Helicobacter pylori, a Gram-negative and spiral-shaped pathogenic bacterium, resides in >50% of the population worldwide and causes different diseases characterized by prominent gastric inflammation which is associated with gastric ulcers. The mechanism of H. pylori-induced inflammation includes chemokine (IL8)-mediated infiltration of neutrophils, increased RANTES level, and H pylori urease-induced degradation of NF-κB inhibitor (IκBα) (115, 120, 153–155). H. pylori can survive inside macrophages, arrest phagocytosis, and induce their apoptosis by preventing nitric oxide (NO) production. Furthermore, H. pylori stimulates macrophages to secret TNF-α and IL6, which are associated with gastric inflammation, by expressing the TNF-α-converting enzyme17 (ADAM17). ADAM17 is a crucial enzyme for the maturation and functioning of TNF-α and IL6. L. gasseri Kx110A1 inhibits these pro-inflammatory cytokines from H. pylori-infected THP-1 cells by inhibiting the expression of the H. pylori ADAM17 enzyme (113). L. fermentum UCO-979C regulates the immune response of host macrophages (HumanTHP-1 cell line) and human gastric epithelial cells (AGS cell line) by stimulating them to secrete specific cytokines and chemokines. Moreover, it significantly increases the secretion of inflammatory cytokines (IL6, TNF-α, and IL1β) in both AGS and macrophages, and the secretion of IL10, IFN-γ, and IL12p70 only in macrophages prior to H. pylori challenge. In contrast, it decreases the levels of H. pylori-induced inflammatory cytokines [IL8, IL1β, monocyte chemoattractant protein-1 (MCP-1), and IL6] in AGS, and those of TNF-α in both AGS and macrophages. Thus, prior to infection, treatment with L. fermentum UCO-979C increases inflammatory cytokines to counter future infections. In contrast, during infection, L. fermentum UCO-979C treatment lessens the over-activated immune response of host cells, as also shown by Garcia-Castillo et al. (114). The study reported that L. fermentum has the ability to decrease H. pylori-associated inflammation by improving TGF-β production in the AGS cell line. TGF-β inhibits NF-κB activation by upregulating the levels of IκBα. Notably, H. pylori infection impedes this TGF-β-associated signaling pathway by inducing SMAD7 expression to promote inflammation.
Similar to L. fermentum, L. acidophilus, and L. rhamnosus also regulate the immune response of host cells and decrease their pro-inflammatory immune response against H. pylori. As shown by their anti-inflammatory effects in AGS cells, in which both probiotics greatly reduced the CagA-induced expression of IL8 by inhibiting its translocation into host cells. CagA is an H. pylori virulent factor responsible for inflammation by the degradation of cytoplasmic IκBα and increasing translocation of NF-κB into the nucleus (116, 156, 157). Moreover, L. acidophilus activates Th1 response to counter H. pylori infection, suppresses H. pylori-induced SMAD7 expression as well as the activation of the NF-κB and MAPK signaling pathways, and decreases subsequent inflammatory response (production of IL8, IL6, MAP-2, IL1β, TNF-α, and granulocyte-colony stimulating factor) during H. pylori infection (115, 117). L. bulgaricus NQ2508 also shows similar anti-inflammatory effects by reducing H. pylori-induced IκBα degradation and subsequent IL8 production in the human gastric epithelial cell line-1 (GES-1). It may also secrete some soluble proteins which exert inhibitory effects on TLR4 and inhibit its activation by H. pylori. Moreover, it blocks subsequent signaling pathways toward NF-κB activation and its delivery to the nucleus for the transcription of pro-inflammatory cytokines (118). As mentioned above, gastric ulcers and cancer are prominent complications of H. pylori infection. They mainly arise due to the over-immune response of host cells and the subsequent production of inflammatory cytokines, which are involved in gastric ulceration. Many probiotics reduce these complications by regulating the H. pylori-disrupted immune response. L. rhamnosus GG reduces gastric ulceration and cancer induced by H. pylori via the IL8/TNF-α/Gastrin-17 pathway. H. pylori upregulates Gastrin-17 by increasing the levels of IL8 and TNF-α, which in turn upregulate Gastrin-17. Gastrin-17 typically causes gastric cancer, whereas IL8 and TNF-α cause inflammation and apoptosis leading to ulceration of the stomach. L. rhamnosus GG shows significant immunobiotic properties with anti-inflammatory effects and attenuates Gastrin-17 levels by suppressing the expression of IL8 and TNF-α (119, 158–161). Similarly, L. paracasei may ameliorate H. pylori-induced gastric inflammation by regulating the immune response of host cells. L. paracasei 06TCa19 inhibits H. pylori CagA-induced p38 and IκBα phosphorylation and increases the levels of these NF-κB inhibitors in MKN45 cells. This results in inhibition of the transcription of the inflammatory chemokine genes (120). Numerous other probiotics are extensively used to ameliorate H. pylori-induced complications with the aim to regulate the immune system of the host (162, 163).
Escherichia coli causes different problems for humans and animals. Enterotoxigenic E. coli (ETEC) causes diarrhea in piglets and other species by secreting heat-labile and heat-stable toxins. Through a complex mechanism, these toxins activate the chloride channel (cystic fibrosis transmembrane channel) resulting in diarrhea. The E. coli causing postweaning diarrhea mostly carries F4 (K88) fimbriae (164). F4+ ETEC increases the expression of membrane and cytoplasmic-associated receptors (TLRs and NLRs), which are involved in the NF-κB signaling pathway and subsequent production of pro-inflammatory cytokines (IL8 and TNF-α) leading to inflammation (130, 164, 165).
Probiotics greatly reduce the expression of these pro-inflammatory cytokines by reducing the interaction of E. coli with membrane receptors. L. rhamnosus ACTT 7469 weakens the E. coli-induced expression of TLR4, TNF-α, and IL8 at the protein and mRNA levels in piglets. Furthermore, L. rhamnosus increases the expression of TLR2, TLR9, and NLR in the case of E. coli infection in piglets, which results in decreased intestinal inflammation (130). As mentioned above, TLR2 and TLR9 are involved in the anti-inflammatory effects of many probiotics.
Similar anti-inflammatory effects have also been shown by supplementation of L. plantarum B1, which reduces E. coli-induced inflammation in broiler chickens by decreasing the expression of TLR4 and the levels of cytokines (IL2, IL4, and IFN-γ) involved in inflammation. L. plantarum also increases the levels of mucosal antibodies (IgA) (131, 132). Hence, probiotics (mainly, the Lactobacillus species), regulate the immune response in a beneficial manner by decreasing the expression of membrane receptors (TLR4) involved in inflammation associated with pathogens. On the other hand, probiotics increase the expression of membrane receptors (TLR2, TLR9) involved in the reduction of pathogen-induced inflammation. Like, Lactobacillus jensenii TL2937 in porcine intestinal epithelial cells decreases the expression of TLRs by increasing the negative regulators [IRAK-M, BCL3, toll interacting protein (TOLLIP), and A20] of these receptors and reduces the E. coli induced inflammation (133). Another study also reported similar anti-inflammatory effects of other probiotics (Lactobacillus amylovorus DSM 1669 and L. delbrueckii TUA 4408), including inhibition of ETEC-induced activation of the NF-κB and MAPK pathways via negative regulation of TLRs, which results in a decrease of pro-inflammatory cytokines (IL1, IL6, IL-1β, and IL8) and an increase of anti-inflammatory cytokine (IL10) in pig explant, caco-2, and porcine intestinal epithelial cells (134, 135). Amdekar et al. also demonstrated that Lactobacillus species play a key protective role against E. coli-induced urinary tract infection, and clearance of pathogens by regulating the expression of TLRs (TLR2 and TLR4) and subsequent production of anti-inflammatory cytokines (166). Probiotics induce the expression of different kinds of cytokines involved in host immune response during pathogenic infection by regulating the expression of TLR and their intracellular signaling pathways. They increase the expression of anti-inflammatory cytokines and reduce the inflammatory response of host cells during infection. L. amylovorus shows protective and anti-inflammatory effects in pig explants and caco-2 cells against E. coli infection and decreases E. coli-mediated inflammation by increasing the levels of TLR4 negative regulators (IRAK-M and TOLLIP) and decreasing those of extracellular heat shock proteins (HSP90 and HSP72), which are crucial for TLR4 functioning. This effect leads to inhibition of the E. coli-induced increase in the levels of TLR4 and MyD88, phosphorylation of IκBα, IκB kinase α (IKKα), IKKβ, and NF-κB subunit p65, as well as the overproduction of inflammatory cytokines (IL8 and IL1β) (134). Treatment with L. rhamnosus ATCC 7469 decreases TLR4 and NOD2 mRNA expression during ETEC infection in IPEC-J2 cell model and reduces the associated inflammatory response of the host. Notably, ETEC induced higher mRNA expression of these membrane and cytoplasmic receptors that lead to the transcription of inflammatory genes via the NF-κB pathway (136).
Some probiotics improve the immune status of aging mice to increase their resistance against infection. The study of Sharma et al. on mice reported that L. rhamnosus MTCC 5897 feeding alleviates the imbalance of Th1/Th2 immune response and also increases the activity of antioxidant enzymes (catalase, glutathione peroxidase, and superoxide dismutase) and reduces E. coli load in the liver, spleen, and intestines by increasing the level of E. coli specific antibodies (IgA and IgG) (167). Similarly, L. fermentum MTCC 5898 feeding in aged mice increases their protection against E. coli infection by increasing the IgA and IgG1 levels and inflammatory proteins and reduces the E. coli load in the intestines, liver, spleen, and peritoneal fluids (168). Other lactobacilli improve the E. coli disturbed intestinal barrier function as, E. coli significantly decreases the intestinal permeability by decreasing the level of tight junction proteins (Occludin, ZO-1, cingulin-1, claudin-1, etc.) as observed by Bhat et al. in Caco-2 cells (169). L. rhamnosus (LR: MTCC-5897) improves these tight junction proteins and significantly reduces the E. coli induced hyperpermeability in Caco-2 cells (170). Similar effects were also observed by L. fermentum (LF: MTCC-5898) treatment during E. coli infection in Caco-2 cells in which L. fermentum (LF: MTCC-5898) improves the barrier integrity by reducing E. coli induced lower mRNA expression of Occludin, ZO-1, cingulin-1, and claudin-1 (57).
Thus, probiotics positively regulate the immune response of host cells at various steps through different mechanisms of action and protect the host from ETEC-induced deleterious effects.
Clostridial species are rod-shaped, Gram-positive toxins and spore-producing bacteria. Clostridium difficile is linked to a wide range of clinical problems (171) and produces many toxins (e.g., cytotoxins and enterotoxins), which cause diarrhea (172). It mainly produces the exotoxins TcdA and TcdB with a size of ~300 kDa. When it binds apically with epithelial gut cells, TcdA causes tight junction interruption and also facilitates the binding of TcdB toxins to the basal lamina. TcdB causes an increase in vascular permeability, release of neurotensin, induction of pro-inflammatory cytokines, fluid secretion, and eventually diarrhea (173).
Probiotics may subside the detrimental effects of clostridial infection by modulating the innate (mucus, lysozymes, and alpha defensin production, and modulation of membrane receptors such as TLRs and NLRs) and adaptive (production of immunoglobulins, anti-inflammatory cytokines, antigen uptake, and modulation of antigen-presenting cells) immune responses and cell signaling pathways (NF-κB and MAPK) of the host (173, 174). S. boulardii is a type of yeast that may be used as a probiotic against clostridial toxins. It increases the production of antibodies (IgA, IgG, and IgM) acting as adjuvant in BALB/c mice (121) and has numerous other mechanisms of action associated with immune regulation. It inhibits the activation of the NF-κB and MAPK signaling pathways, and pro-inflammatory cytokine (IL8) production induced by C. difficile toxin A in human colonic epithelial cells (NCM460). This toxin activates the extracellular signal-regulated kinase 1/2 (ERK1/2) and stress-activated protein kinases (SAPK)/Jun amino-terminal kinases (JNK) (JNK/SAPK) pathways, resulting in the transcription of pro-inflammatory cytokine (IL8) genes and leading to inflammation. S. boulardii inhibits the Clostridium toxins A-induced ERK1/2 and JNK/SAPK signaling pathways in mice (122). Furthermore, it degrades C. difficile toxins by its protease action and decreases the binding of toxins to host cell (rat ileum) receptors (123).
Staphylococcus is a major cause of bovine contagious mastitis and persistent infection in bovine mammary epithelial cells in animals. Via upregulation of TLR2 and TLR4, Staphylococcus aureus (S. aureus) increases the secretion of basic fibroblast growth factor and TGF-β1 through activation of the NF-κB pathway by inhibiting NF-κB inhibitors in bovine mammary epithelial cells (175). Many probiotics are used to treat and control Staphylococcus infection. Probiotic L. casei (BL23) significantly reduces inflammation of the mammary glands during S. aureus infection by suppressing the expression of S. aureus-induced pro-inflammatory cytokines (IL8, IL6, TNF-α, IL1β, and IL1α). This results in potent anti-inflammatory effects against S. aureus infection in bovine mammary epithelial cells (124). Bacillus subtilis has shown protective effects against S. aureus infection in mice, by activating macrophages, limiting systemic inflammation induced by S. aureus, and decreasing the pathogen load. Bacillus subtilis-secreted exopolysaccharides (EPS) have an immunomodulatory function, producing hybrid macrophages (having the functions of both M1 and M2) with anti-inflammatory and bactericidal phagocytic characteristics. These hybrid macrophages limit S. aureus—induced T-cell activation and kill S. aureus by increasing the levels of reactive oxygen species and decreasing the levels of pro-inflammatory cytokines and chemokines [chemokine (C-C motif) ligand 2 (CCL2), CCL3, CCL4, TNF] (125). Paynich et al. (176) study on mice showed that Bacillus subtilis-exopolysaccharides induces anti-inflammatory macrophages (M2), which inhibit T-cell (CD4+ and CD8+) activation by secreting TGF-β and PD-L1 molecules. These molecules have inhibitory effects on CD4+ and CD8+ cells, showing a significant anti-inflammatory property in T cell-dependent immune reaction (176). In this way, probiotics beneficially regulate the immune response of host cells; they activate immune cells to kill S. aureus and decrease pathogen-associated inflammation by limiting the overexpression of inflammatory cytokines from pathogen-activated immune cells.
Listeria monocytogenes causes several infections, including maternal-fetal infection, septicemic pneumonia, pleural infection (177), foodborne diseases with a 20–30% mortality rate (178), and neurolisteriosis leading to meningitis and encephalitis (179). Several probiotics (mostly Lactobacilli species) are used to protect the host against L. monocytogenes infection. L. salivarius (BGHO1) therapies against L. monocytogenes exert protective effects by modulating the adaptive and innate immune responses during L. monocytogenes infection in rats. BGHO1 increases the mRNA expression of CD14, TNF-α, and IL1β and decreases listeriolysin (Listeria toxins) in the intestinal tissues. In mesenteric lymph nodes, BGHO1 co-administered with L. monocytogenes enhances CD69 and OX-62 mRNA expression (126). L. delbrueckii induces the production of TNF-α and IFN-γ, which stimulates the macrophages to kill L. monocytogenes. Mice infected with L. monocytogenes which received L. delbrueckii UFV-H2b20 have a longer lifespan, less liver immunopathology, and less bacterial load in the spleen and liver (127). These probiotics stimulate macrophages by inducing the expression of specific cytokines to increase their bactericidal activities and decrease the level of toxins, as well as assist the host in eliminating pathogens from their body and accelerate recovery.
Heat-killed E. faecium BGPAS1-3 cell wall protein, which is resistant to high temperature, has shown protective and strong anti-listeria activity. It stimulates Caco-2 cells to increase TGF-β production. TGF-β exerts protective effects on epithelial tight junctions by upregulating the expression of claudin (128). These innate immunomodulatory effects are achieved by modulation of the MyD88-dependent TLR2 and TLR4 pathways in intestinal cells against Listeria infection. L. monocytogenes induces TLR2 and suppresses the expression of TLR4 mRNA in Caco-2 cells. Heat-killed BGPAS1-3 decreases the expression of TLR2 mRNA in Caco-2 cells. In contrast, the expression of TLR4 mRNA in Caco-2 cells is increased by both heat-killed and live BGPAS1-3 before and after L. monocytogenes infection, respectively. Furthermore, heat-killed or live BGPAS1-3 has inhibitory effects on the expression of IL8 in uninfected and infected L. monocytogenes Caco-2 cells (180). Heat-killed and live probiotics, as well as their cellular components, can regulate the immune response of the host through interaction with TLRs, increase the protective innate immune response, and decrease the inflammatory response of host cells. Cho et al. showed the protective and immunomodulatory effects of heat-killed and live E. faecium JWS 833 using a L. monocytogenes mice model and peritoneal mouse macrophages, respectively. Both heat-killed and live JWS833 show immunomodulatory properties. When administered orally, live JWS833 increases the levels of serum cytokines (TNF-α and IL1β) and NO against L. monocytogenes in mice. Heat-killed JWS833 stimulates the macrophages to produce TNF-α, NO, and IL1β (129). Probiotics have diverse immunomodulatory functions, assisting the host to counter pathogenic infections.
The threat of viral illness has recently increased significantly due to the changes in the environment (e.g., anthropogenic climate change and increased global movement of passengers and cargo). Viral infections cause variable morbidity and mortality with a detrimental effect on community well-being and cause widespread economic losses. Respiratory Syndrome Coronavirus 2 (SARS-CoV-2), which infected millions of people worldwide during the 2019–2020 pandemic is a good example of this global economic loss (181). Thus, finding alternative and effective strategies to prevent viral infections and reducing the morbidity and mortality of viral infections is critical (Table 3). Nevertheless, many vaccines and antiviral drugs aiming to be effective in infections are available, but a major challenge is the new viral strains that appeared after mutations, particularly in RNA viruses. It is wise to have some alternative strategies that could be used as supplemental or preventive remedies. To reduce the severity of viral infections and their numbers, a balanced diet including nutrients or food additives that boost and potentiate immune system response, is a beneficial alternative measure. The use of probiotics is one of the dietary approaches used in recent years to increase immunity and decrease the risk of infections (213). Many probiotics (mainly Lactobacilli species) are used for the prevention or treatment of viral illnesses. In addition, to alter the crosstalk between gut bacteria and the mucosal immune system, probiotics have many other immune modulatory and non-immune functions to combat viral incursion. The application of probiotics for the control and prevention of clinically important viral diseases is briefly discussed below.
Bifidobacterium infantis (MCC12) and Bifidobacterium breve (MCC1274) modulate immune response during human rotavirus infection in the porcine intestinal epithelial cell line. Both species are able to blunt IL8 production and increase IFN production by increasing the activation of interferon regulatory factor 3 (IRF3) through the suppression of A20 (a zinc-finger protein with negative effects on IRF3 activation) (182). These probiotics activate various interferon-stimulated genes (ISGs), including RNase L (2′-5′ oligoadenuylate dependent endoribonulecase) and myxovirus resistance protein A (MxA) (183). MxA decreases virus replication by binding with virus nucleoproteins (219). RNase L has antiviral activity and lessens viral replication through the elimination of infected cells by inducing apoptosis and IFN amplification by activating RLRs (220, 221). RLRs are intracellular PRRs involved in virus recognition. L. rhamnosus GG (strain ATCC 53103) and B. lactis Bb12 enhance the efficacy of human attenuated rotavirus vaccine (AttHRV) during rotavirus infection in gnotobiotic human rotavirus pig model, by increasing T-cells subset (CD3+, CD4+) in intestinal tissues and T-cells subset (CD3+, CD8+) in the blood and spleen. Further, the severity of diarrhea and virus load was also less in vaccinated pigs receiving ATCC 53103 and Bb12 as compared to only vaccinated pigs (184). Similarly, S. boulardii and several Bifidobacterium and Lactobacillus species have anti-rotaviral effects, mitigate the severity and duration of diarrhea, viral shedding, and incidence of infections associated with rotavirus, and modulate the immune response of the host (222–226) Lactobacillus species and Bifidobacterium in combination with some prebiotics (human milk oligosaccharide, short-chain galactooligosaccharides, and long-chain fructooligosaccharides) show antiviral response. L. casei (Lafti L26-DSL) and Bifidobacterium adolescentis (DSM 20083) reduced the infectivity of virus in MA104 cells (embryonic Rhesus monkey kidney cells) by interacting with virus protein (NSP4). NSP4 has been characterized as virus toxin and is associated with diarrhea in host (185, 186). L. rhamnosus (strain GG) and Gram-negative E. coli Nissle (EcN) decrease human rotaviral complications by modulating the immune response and interacting with rotavirus. In the pig rotavirus model, EcN and L. rhamnosus GG induced higher total IgA levels in the intestine and serum post- and pre-human rotavirus challenge, respectively, and reduced viral shedding. EcN can regulate the expression of cytokines (IL6 and IL10) and bind with rotavirus protein 4 to reduce rotavirus attachment to the host cells (227, 228). In the rotavirus gnotobiotic pig model, Lactobacilli species (L. acidophilus and L. reuteri) significantly increased total intestinal IgM and IgG and serum IgM titers and total intestinal IgA secreting cell responses (187). Furthermore, Azevedo et al. (229) demonstrated that these probiotics (L. acidophilus and L. reuteri) significantly increased Th1 and Th2 cytokines in human rotavirus infected pigs, and also help in maintaining immunological homeostasis during human rotavirus infection by regulating the production of TGF-β. Different probiotics have anti-rotavirus activities involving various immunomodulatory mechanisms. Bifidobacterium stimulates ISGs and lowers various pro-inflammatory cytokines, while Lactobacillus increases anti-rotavirus antibodies and reduces rotavirus-associated complications.
A randomized controlled trial on 96 elderly people showed that a yogurt fermented with L. delbrueckii ssp. bulgaricus OLL1073R-1 (1073R-1-yogurt) affected the level of influenza A H3N2 bound IgA levels in saliva (188). L. acidophilus NCFM and Bifidobacterium animalis subsp. lactis Bi-07 reduce the incidence of coughing (41.4%), rhinorrhea (28.2%), and fever (53%) in a double blind placebo controlled study on 326 children during the winter season (198). Different clinical trial studies on children, elderly people, adults, and animals compiled by Lehtoranta et al. (230) shows that probiotic administration reduced the risk respiratory viruses including influenza viruses. In mice, L. paracasei showed anti-influenza effects and beneficially modulated the immune response against influenza infection, while reducing the viral load, morbidity, and mortality. L. paracasei increases the levels of pro-inflammatory cytokines (IL1α and IL1β) and recruitment of immune cells before infection. This accelerates viral clearance and reduces the levels of inflammatory cytokines [macrophage inflammatory protein-1α (MIP1α), IFN-γ, MCP-1, and MIP1β] after influenza infection. Moreover, L. paracasei has shown anti-inflammatory characteristics at the late stage of infection by increasing the levels of IL10 (189). Heat-killed L. casei DK128 shows similar anti-inflammatory effects against influenza infection by decreasing influenza virus-induced pro-inflammatory cytokines (IL6 and TNF-α), monocytes, and activated NK cells in the lungs of mice, thereby preventing pulmonary inflammation. Furthermore, DK128 increases the levels of antibodies (IgG1 and IgG2a) against the influenza virus at an earlier time point and provides cross-immunity against secondary heterosubtypic influenza infection with improved health and survival rate in mice (190). L. plantarum (O6CC2) beneficially modulates the host immune response during influenza infection in mice by increasing the production of IFN-α and Th1 cytokines (IL12 and IFN-γ) as well as the expression of Th1 cytokine receptors which potentiate NK cell activity at the early stage of influenza infection in mice. Of note, NK cells are an important line of defense during this early phase (191, 192). At the late stage of infection, L. plantarum (O6CC2) decreases IL6 and TNF-α production to control influenza-mediated inflammation. Furthermore, O6CC2 decreases neutrophil and macrophage infiltration to overcome the inflammatory response to influenza infection (231). L. plantarum (AYA) has shown protective immunological effects against influenza virus infection by increasing production of mouse mucosal IgA (193). L. GG and L. johnsonii (NCC 533) are also associated with increased IgA production (232, 233). B. longum (MM-2) has shown anti-influenza activity by enhancing the innate immunity through increases in the expression of NK cell activator genes (IFN-g, IL2, IL12, IL18) activities. This probiotic reduces mortality, morbidity, virus titer, cell death, virus-induced inflammation, and the expression of mRNA for pro-inflammatory cytokines (IL6, TNF-α, IL1β, MIP2, and MCP-1) in mice infected with influenza virus (194). Similar immune regulatory and anti-influenza effects of Bifidobacterium have been observed by other researchers. B. longum BB536 enhances the activities of neutrophils and NK cells, reduces fever in human beings (195), reduces IL6 and IFN-γ at the late stage of infection, and prevents body weight loss and virus replication in the lungs of mice infected with the influenza virus (196). L. plantarum (137) induces higher type-1 interferon (IFN-β) levels in the serum of mice at the early stage of influenza infection (197). Notably, innate immunity of type-1 interferon is involved in countering viral infection at the early stage (234). In the case of the influenza virus infection, gut microbiota have preventive effects and modulate type I IFNs (235). These IFNs are involved in innate immunity during viral infection with antiviral activities, as well as the degradation and inhibition of viral nucleic acids and viral gene expression, respectively (236, 237). These studies showed that various probiotics show anti-influenza activities along with immunoregulatory effects during infection.
Coronavirus disease 2019 (COVID-19) was officially declared as a pandemic by WHO on March 11, 2020 (238). SARS-CoV-2 was first identified in Wuhan city (China) in December 2019 (239) in patients with pneumonia and rapidly spread to 216 countries (240, 241). Coronaviruses (CoVs) belong to the family Coronaviridae and genus coronavirus order Nidovirales and subfamilies: Alphacoronavirus, Betacoronavirus, Gammacoronavirus, and Deltacoronavirus (242). Subfamilies alphacoronavirus and Betacoronavirus originate from mammals mainly bats, and Gammacoronavirus and Deltacoronavirus subfamilies originate from pigs and birds (243). In SARS-CoVs virion envelop, there are three main structural proteins—protein S (Spike), protein M (membrane), and protein E (envelop). Protein S (Spike) facilitates the SARS-CoVs adherence and fusion (52). All CoVs are positive sense, single stranded RNA, and pleomorphic viruses with typical crown shape peplomers of 27–32 kb and 80–160 nM size (239, 244). Genomic structure analysis showed that the viruses belong to β-coronavirus including MERS-CoV and SARS-CoV with high mutation rates because of RNA dependent DNA polymerase transcription error (242), which is also the main target of drug discovery (245). Pathogenesis of SARS-CoV-2 includes binding of its spikes proteins (S) to Angiotensin-Converting-Enzyme-2, which are highly expressed in lungs as well as in esophagus and enterocytes in the colon and ileum, to get entry into the cells for infection (246). TMPRSS2 is a protein, which helps the “S” proteins of SARS-CoV-2 to get entry into cells, is also highly expressed in absorbent enterocytes (247). Clinical signs of COVID-19 disease are different ranging from asymptomatic to non-specific flu and severe pneumonia, Middle Eastern respiratory syndrome (MERS) (248), and life-threatening consequences like acute respiratory distress syndrome and different organ failure. It can also affect neurological, gastrointestinal, and hepatic systems (249). According to data from Wuhan city in China, 14% of the infected cases were severe, 4% died, and 5% needed intensive care (250).
In spite of the different measurements including hygienic improvement, screening, and social distancing, COVID-19 is rapidly spreading and progressing worldwide (22, 240), while the search for effective drugs and vaccine therapies is underway. Scientists are battling against the time needed to develop a vaccine, but it is hard to make an efficient and safe product as rapidly as the virus is spreading (251). Thus far, there are no effective drugs available for SARS-CoV-2. However, according to genomic structure analysis and its similarity with SARS and MERS, certain drugs (e.g., lopinavir, ritonavir, and nitazoxanide) may be applicable (252). At the same time, several studies have compiled alternative data related to general viruses management and treatment (253–258) including nutritional supplements like vitamins and some other immune boosts medicine (259). Some in silico data are in favor of probiotics use for the treatment of COVID 19 as data indicate that probiotics derived molecules like lactococcin Gb (L. lactis), subtilisin (Bacillus amyloliquefaciens), sakacin P (L. sakei) may inactivate “S” glycoprotein and its receptors molecules i.e., Angiotensin-Converting-Enzyme-2 (260). Similarly, several other studies have published their data regarding the use of probiotics for the general management of viral diseases as it is indicated by some clinical evidence that some kinds of probiotics are helpful in preventing bacterial and viral infections like respiratory tract infections, sepsis, and gastroenteritis. Viruses account for over 90% of upper RTIs as etiological agents. Many studies have recorded the positive effect of probiotics on the protection of upper respiratory tract infections. Reduced risk of getting upper respiratory tract infections in probiotic supplementations was recorded in a meta-analysis study of 12 randomized control trials involving 3,720 children and adults. It was observed in 479 adults of a randomized, double-blind, placebo-controlled intervention study that B. bifidum MF 20/5, L. gasseri PA 16/8, and B. longum SP 07/3 along with mineral and vitamins reduced the duration of fever and common cold (22). Streptococcus salivarius strain K12 may possibly reduce the severity of COVID-19 complications by its ability to maintain stable upper respiratory tract microbiota. As advanced studies have shown that lung microbiota have an important role in the homeostasis of immune responses (261), and its dysbiosis makes the patient more vulnerable to viral infections. In the case of COVID-19, a significant difference in lung microbiota has been observed in patients with COVID-19 and normal persons (262). Probiotic consumption triggers pro-and anti-inflammatory cytokines production to clear the viral infection, reduce the cell damage in the lungs, and improve the levels of T cells, B cells, NK cells, and type 1 interferons in the immune system of the lungs, and it may help to prevent COVID-19 complications (261).
Probiotics and recombinant probiotics with antiviral effects are effectively used to combat and minimize the detrimental effects of other coronaviruses, such as alphacoronaviruses—particularly transmissible gastroenteritis virus (TGEV) and porcine epidemic diarrhea virus (PEDV)—which cause substantial economic losses in the pork meat industry. Recombinant L. plantarum inhibits TGEV and PEDV infections in the IPEC-J2 cell line by enhancing ISGs (OASL, ISG15, and Mx1) which have strong antiviral effects (199). Recombinant L. plantarum (containing the surface S antigen of TGEV) elicits an immune response characterized by higher numbers of activated DC cells, B+IgA+ cells, secretory IgA (sIgA), serum IgG, IFN-γ, and IL4 which help the host to combat TGEV (200). Similar effects were observed by Jiang and colleague who reported that a recombinant L. casei ATCC39392 vaccine modulates the immune response against TGEV infection, induces IL4, mucosal (IgA), and systemic (Ghosh and Higgins) antibodies, and polarized Th2 immune response with enhanced the expression of IL17 against TGEV in a pig model (201). Similarly, immune protective effects with the elicitation of sIgA and IgG production against PEDV have also been shown by a L. casei-based vaccine, consisting of a DC-targeting peptide attached to the PEDV core antigen (263). Antibiotics and porcine bile-resistant L. plantarum Probio-38 and L. salivarius Probio-37 have shown antiviral effects in vitro ST cell line and inhibit TGE coronavirus without cytotoxic effects (202). Another study shows that cell-free supernatants of different LAB (L. plantarum 22F, 25F, and 31F) and live L. plantarum (22F, 25F) have anti PEDV activity with any cytotoxic effects on Vero cells (203). E. faecium has protective effects against enteropathogenic coronavirus TGEV and hinders the virus entry into cells by interacting with cell surface molecules, reducing viral structural proteins, and inducing antiviral NO (264, 265). Furthermore, E. faecium stimulates an antiviral response by increasing the expression of IL8 and IL6 mRNA (266), which contribute to the immune regulation against many other enteric pathogens (267). Studies show that E. faecium (probio-63) and E. faecalis (KCTC 10700BP) suppress coronavirus growth, responsible for porcine epidemic diarrhea (268, 269). These findings indicate that probiotics have antiviral effects, and stimulate the immune response of the host against viruses. Many probiotics enhance vaccine efficacy; some probiotics inhibit virus entry into cells and also stimulate the production of different cytokines during viral infection.
Probiotics are widely applicable to the treatment and prevention of parasitic infections (Table 4). Oral administration of L. rhamnosus MTCC 1423 during Giardia infection in mice modulates both cellular and humoral immune responses, enhances sIgA, IgA+ cells, CD4+ T lymphocytes, and anti-inflammatory cytokine IL10, and decreases pro-inflammatory cytokine IFN-γ (277). E. faecium SF 68 stimulates an anti-giardia immune response, increases CD4+ T cells and the production of anti-giardia antibodies (intestinal IgA and serum IgG), and reduces the parasitic load (278). Lactobacillus and S. boulardii also have positive effects in the treatment of giardiasis, minimizing interaction between the host and pathogen, reducing parasite load, and modulating the immune response of the host. L. johnsonii La1 (NCC533) reduces active trophozoite of Giardia intestinalis strain WB and infection duration in Meriones unguiculatus (286). Recombinant L. plantarum NC8 (containing Eimeria tenella protein) induced a higher percentage of a T-cell subset (CD3+, CD4+, and CD8+) and antibody levels, provided protection against E. tenella infection in chickens, and reduced lesion, cecum damage, and oocyst shedding (270). L. salivarius, L. johnsonii, and S. cerevisiae provided protection against Eimeria infection in chickens; reduced oocyst count, improved weight gain and FCR, and stimulated the immune response with higher antibodies (IgM and IgG) titer and lymphoproliferative response (271). Pender et al. revealed that chickens receiving supplementation of commercially available probiotics; Primalac W/S (L. acidophilus, L. casei, E. faecium, and B. bifidium) showed lower mortality, higher body weight, and fewer Eimeria maxima-, Eimeria tenella-, and Eimeria acervulina-induced lesions; however, there was no effect on the immune response (272). Lactic acid from L. acidophillus stimulates the host immune response during Cryptosporidium infection, increasing the number of lymphocytes, levels of complement proteins (C3, C4), and antibodies (IgM, IgG), as well as reducing oocyst shedding from infected rabbits (273). L. casei, Bifidium bacteria, and E. faecalis exert protective effects during Cryptosporidium parvum infection and greatly reduce parasite load and oocyst shedding from the intestine of infected mice (287–289). In contrast, Oliveira and Widmer demonstrated that some commercially available probiotics enhanced the severity of cryptosporidia infection by altering the intestinal environment in favor of C. parvum proliferation (290). Bifidobacterium animalis subspecies lactis strain Bb12 stimulates local immune response during Ascaris suum infection in juvenile pigs and production of anti-parasite antibodies (IgA in serum and IgG1 and IgG2 in ileal fluid) and glucose uptake (274). Similarly, L. rhamnosus modulates the expression of TNF-α, TLR9, IFN-γ, and IL10 gene, which results in decrease in eosinophil action and allergic skin reaction induced by Ascaris suum in the pig model (275, 276). Many probiotics are effective against schistosomiasis; Zymomonas mobilis stimulates immune response and provides 61% protection during schistosomiasis (291). L. plantarum, L. reuteri, L. casei, and L. acidophilus stimulate IgM antibodies against Schistosoma mansoni infection in mice (279). L. sporogenes reduces schistosomiasis cytokine-induced chromosomal aberration in mice (280). During trichinellosis (Trichinella spiralis infection in mice), L. plantarum increases the levels of IFN-γ and reduces larval count (281). L. fermentum, E. faecium, and Enterococcus durans enhance the activity of phagocytes during Trichinella spiralis infection in mice (282). L. casei induces IgA and IgG during T. spiralis infection in mice (283, 292). In trichuriasis mice model, L. rhamnosus (JB-1) increases IL10 and mucus-secreting goblet cells, resulting in the faster removal of larvae (284). E. faecalis CECT7121 (Ef 7121) and S. boulardii are associated with larvicidal activity and high production of IL12 and IFN-γ, respectively, during Toxocara canis infection in mice (285, 293). Different probiotics have different mechanisms of action during parasitic infections. They reduce complications, regulate cytokine production, and facilitate the production of anti-parasitic antibodies. However, it has been shown that some probiotics enhance the parasitic infection as indicated in the study by Dea-Ayuela and colleague on mice in which they reported that L. casei decreases cytokines (IFN-γ, TNF-α, IL-4, and Il-13) and antibodies (fecal IgA) against Trichuris muris, increasing the susceptibility of T. muris infection. This L. casei associated increased susceptibility to infection may be related to deactivation of TNF-α dependent Th2 effector responses against T. muris due to the strong inhibitory effect of L. casei on this cytokine (294).
Probiotics improve the central nervous system and mental function with beneficial effects reported in anxiety, Alzheimer's disease, depression, schizophrenia, and autism (295). In an autism spectrum disorder mice model, an L. reuteri diet led to a behavioral improvement in an oxytocin-dependent manner (296). Probiotics can alter the composition of gut microbiota (297), which in turn acts on the gut–brain axis by secreting neuroactive substances (298) and significantly influences and regulates cerebrovascular diseases, neurodegeneration, and mental dysfunction (299). B. infantis reduces stress by increasing the levels of tryptophan in plasma, decreasing the levels of serotonin in the frontal cortex, and regulating the hypothalamic–pituitary–adrenal axis. L. rhamnosus JB-1 decreases the expression of gamma aminobutyric acid receptor and corticosterone levels in mice, which are induced during stress (300–302). Moreover, B. longum, L. helveticus, and L. plantarum reduce anxiety (303). L. fermentum NCIMB can produce ferulic acid, which is a strong antioxidant that can stimulate the proliferation of the nervous system stem cells and be used to treat neurodegenerative disorder, diabetes, and obesity. In mice, feeding ferulic acid ameliorates Alzheimer's disease symptoms, oxidative stress, and neuroinflammation (65). Thus, probiotics have positive effects on brain function, by affecting the functions of the nervous system as well as some related hormones and their receptors (Table 5). However, a detailed study of the effects of probiotics on the nervous system is needed to support the currently available evidence.
Different probiotics regulate obesity (323), which predisposes individuals to different diseases, such as non-alcoholic fatty liver diseases, cardiovascular diseases, diabetes, cancers, and some disorders related to the immune system (324). L. plantarum CBT LP3 and B. breve CBT BR3 reduce obesity related marker, and L. rhamnosus, E. faecium, L. acidophilus, B. bifidum, and B. longum decrease low-density lipoprotein cholesterol, total cholesterol and oxidative stress level in an in vivo human trial (320). B. bifidum W23, B. lactis W51&W52, L. lactis W19&W58, L. brevis W63, L. casei W56, L. acidophilus W37, and L. salivarius W24 regulate the obesity by decreasing triglyceride, total cholesterol, homocysteine, and TNF-α level in a randomized double-blind placebo-controlled trial on 50 women who were obese (321). Indigenous microbiota play a key role in obesity by harvesting energy for the host through different metabolic pathways. Probiotics change the composition of gut microbiota, thereby influencing obesity (12). Gut microbiota contribute to obesity via several potential mechanisms, such as lipogenesis, carbohydrate fermentation, and energy storage, and through numerous pathways (e.g., different hormones, metabolites, and neurotranmitters), which regulate energy balance and food intake (Table 5).
Probiotics also reduce the risk of cancer by different mechanisms of action, which include the exclusion of oncogenic bacteria, improvement of epithelial barrier function, increase of tumor cell death by apoptosis, production of immune-modulating metabolites (acetate, butyrate, propionate, conjugated linoleic acids, etc.), increase of cytokine production with an antitumor response, and TLR modulation. Butyrate regulates cell proliferation, differentiation, and apoptosis (325), it can stimulate anti-inflammatory cytokines and IL10 production and decrease the production of inflammatory cytokines via inhibition of NF-κB. Furthermore, butyrate regulates apoptosis-regulating proteins [CASP7, CASP3, BCL2 antagonist/killer (BAK), and BCL2], suppresses COX2 activity, stimulates the production of AMPs, and increases glutathione-S-transferase. These effects lead to downregulation or upregulation of genes related to the apoptosis, proliferation, and differentiation of cells (326, 327). Propionic acids and acetic acid have also shown anti-inflammatory activities by suppressing NF-κB activation and modulating the expression of pro-inflammatory genes (328). Some probiotics (Lactobacilli, bifidobacteria, and streptococcus) can produce conjugated linoleic acid, which has pro-apoptotic and anti-proliferative activities. This is achieved by increasing the expression of peroxisome proliferator-activated gamma receptor (PPARγ), which is involved in immune function and apoptosis. Some probiotics show their anti-cancerous activities via cation exchange between their peptidoglycan and the carcinogenic compound. Furthermore, probiotics decrease the COX2 enzyme-mediated production of prostaglandins, which increases the risk of colorectal cancer (329, 330). Probiotics can increase the production of immunoglobulins, such as IgA, generating an anti-inflammatory environment. IgA does not provoke activation of the complement system and acts as a barrier to reduce contact between the carcinogenic compound in the lumen and colonocytes, thereby reducing the risk of cancer (331). A prospective study involving 82,220 individuals showed that individuals who consume yogurt and sour milk are less susceptible to bladder cancer. An Italian cohort study on 45,000 volunteers of a 12-year follow up without comparative group, reports that yogurt consumption decrease in colorectal cancer (332). L. casei administration in humans for 4 years showed less recurrence of adenoma atypia, and probiotics with oligofructose-enriched inulin preparation reduce DNA damage in colonic epithelial cells and HT29 cells (322). Animal studies supported the beneficial effects of yogurt against genotoxic amines and cancer of the bladder and colon. In a breast cancer mice model, L. acidophilus isolated from yogurt promoted the proliferation of lymphocytes and decreases tumor growth (323) (Table 5). Hence, probiotics reduce the risk of cancer by different mechanisms. Some probiotics assist in excluding the oncogenic bacteria, while others inhibit inflammatory pathways and increase apoptosis of tumor cells. Furthermore, probiotics stimulate the production of immune-modulating metabolites involved in cell growth, proliferation, and apoptosis.
Many probiotics have beneficial effects on allergies (Table 5). L. rhamnosus (MTCC5897) fermented milk (PFM) feeding in newborn mice alleviates allergic symptoms by shifting Th2 to Th1 pathway by decreasing albumin specific antibodies (IgE, IgG, and IgG1), ratio of IgE/IgG2a and IgG1/IgG2a and IL-4, and by increasing IFN-γ, IgA+ cells, and goblet cells (304, 333). Bifidobacteriales, Bacteroidales, and Lactobacillales in the gut affect the activities of inhaled allergens. Bifidobacteriales and Lactobacillales suppress allergen sensitization and are effective against allergic rhinitis (334). B. infantis CGMCC313-2 represses allergen-mediated inflammatory cells, IL4, IL13, IgE, IgG1, and blunt inflammation during allergy in mice model (306). E. faecalis FK-23 inhibits the development of Th17 cells in the intestine, spleen, and lungs of infected mice by inhibiting the expression of TGF-β and IL6 mRNA, thereby facilitating to reduce ovalbumin-induced allergic complication (307). Staphylococcus succinus 14BME20 has also shown antiallergic potential; it significantly decreases the influx of inflammatory cells into the lungs, suppresses airway hyperresponsiveness, and reduces the serum IgE and Th2 cells cytokines production in an ovalbumin mice model (308). Clostridium butyricum CGMCC0313 increases forkhead box P3 (FOXP3) Treg cells, TGF-β, and IL10, inverts the imbalance between Th1/Th2 and Th17/Treg cells, and reduces β-lactoglobulin-mediated intestinal anaphylaxis, thereby contributing to the reduction of the risk of allergy in mice (309). Orally administered L. acidophilus KLDS 1.0738 ameliorates allergic symptoms by increasing Treg cells, CD25, FOXP3, and TGF-β mRNA expression, and inhibiting inflammatory cells, IgE production, IL6 levels, and Th17 response in mice (310).
Many probiotics are used for the prevention and treatment of diabetes (Table 5). L. rhamnosus MTCC: 5957, L. rhamnosus MTCC: 5897 and L. fermentum MTCC: 5898 feeding Improves glucose metabolism (fasting blood glucose, serum insulin, and glycated hemoglobin), oxidative stress (glutathione peroxidase, superoxide dismutase, catalase activity, and thiobarbituric acid reactive substances,) serum inflammation status (TNF-α and IL-6) and serum lipid profile in diabetic rats, and also significantly reduces mRNA expression of gluconeogenesis related genes (pepck and g6pase) (318). L. acidophilus KLDS 1.0901 shows antidiabetic characteristics by reducing glycosylated hemoglobin, fasting blood glucose level, and increasing the level of glucagon-like peptide 1 in the serum of mice. Further, L. acidophilus KLDS 1.0901 increases glutathione peroxidase and superoxide dismutase activities and also increases the level of glutathione with the reduction of malondialdehyde level in mice serum (335). Similarly, L. paracasei 1F-20, L. fermentum F40-4, Bifidobacterium animalis subsp. lactis F1-7 also exhibit the potential to manage the diabetic problem as shown by the in vitro study of Zhang et al. (241) using CACO-2, STC-, RAW246.7, and HepG2 cells in which these probiotics increase glucagon-like peptide 1 and peptide YY hormones and decrease IL-6 and TNF-α levels (336).
Different species of other Lactobacilli and yeast strains act also as antidiabetic, preventing pancreatic cell apoptosis via upregulation of the PI3K/AKT pathway and increased GATA-like protein 1 (GLP1) production. GLP1-induced insulin secretion by upregulating the G protein-coupled receptor 43/41 (GPR43/41), proconvertase 1/3, and proglucagon activity in mice (319). GLP1 is an antidiabetic hormone involved in glucose homeostasis, and reduction of glucagon secretion and appetite (337–339). Many probiotics improve glucose metabolism (340) and inhibit NF-κB pathway overactivation. NF-κB is associated with diabetes and its inhibition leads to improvement in insulin sensitivity (94, 309). Probiotics reduce the risk of diabetes by regulating different cellular signaling pathways and the expression of sugar metabolism hormones.
Some probiotics improve sperm maturation; L. casei and B. lactis enhanced the maturation of sperm in diabetic rats and decreased their glucose levels (341). L. rhamnosus increased the mRNA expression of androgen receptors α and β, activin and progesterone receptor 1, serum follicle-stimulating hormone, luteinizing hormone, and testosterone. These effects were associated with improvement in spermatogenesis, sperm motility, and sperm production, along with a decrease in the percentage of immotile sperm (342, 343). Bacillus amyloliquefaciens has shown similar beneficial effects on semen density, live sperm, and overall quality in breeder chicken (344).
Probiotics are also widely applied to cardiovascular diseases; they significantly decrease hypertension, oxidative stress, blood pressure, inflammatory mediators, and cholesterol levels (311, 345–348). It is observed that cholesterol-enriched fed mice show significantly higher levels of serum triacylglycerols, total cholesterol, low-density lipoprotein cholesterol, atherogenic index, lipid peroxidation, coronary artery risk index, and IL-6 and TNF-α in the liver whereas significantly lower levels of catalase, anti-oxidative enzymes activities, glutathione peroxidase, and superoxide dismutase in the kidney and liver. Whereas, L. fermentum MTCC: 5898-fermented milk improves these adverse physiological conditions (311). Similarly, feeding of L. rhamnosus MTCC: 5957 and L. rhamnosus MTCC: 5897 maintains healthy liver and kidney conditions of Wistar rats by increasing antioxidant activities and by decreasing lipid peroxidation, diet-induced hypercholesterolemia in the feces, kidney, liver, and blood of the rats. These probiotics also reduce the expression of mRNA of the TNF α and IL-6 inflammatory markers (312).
Lactobacillus plantarum has shown beneficial effects during the meta-analysis of a randomized controlled trial of 653 participants having cardiovascular diseases, lower diastolic and systolic blood pressure (313), total serum cholesterol, low-density lipoprotein cholesterol levels, atherosclerosis index, and hepatocyte steatosis risk. Furthermore, L. plantarum decreases liver triglyceride and cholesterol, whereas it increases cholesterol in feces and excretion of bile acid (314). In vivo study of Robles-Vera et al. (315) showed that L. fermentum CECT5716 and Bifidobacterium breve CECT7263 feeding prevent the development of hypertension, endothelial dysfunctioning, and increase in blood pressure in rats (315). L. rhamnosus GR-1 and L. plantarum 299v reduce the risk of myocardial infarction, improve ventricular function, and reduce the infarct size by decreasing the levels of leptin in rats (316, 317). Probiotics also decreased the levels of toxic circulating metabolites (indoxyl-sulfate and p-cresyl sulfate) associated with cardiovascular diseases and reduced mortality in patients undergoing dialysis (349). Probiotics exert beneficial effects on cardiovascular diseases through different mechanisms of action (i.e., improving the ratio of low-density and high-density lipids, lowering cholesterol levels, improving endothelial function, and regulating the immune cells) (Table 5).
Due to increasing antibiotic-resistant bacteria and antibiotic side effects, the use of antibiotics as a feed supplementation is prohibited in many countries. China also bans the supplementation of growth-promoting antibiotics in animal feed since January 1, 2021. Probiotics are considered as a good alternative for antibiotics, providing an alternative treatment option. Probiotics are widely used in human aquaculture, livestock, and poultry to promote health and counteract enteric pathogens. Probiotics are widely used for the management and treatment of bacterial, viral, parasitic infections as well as non-infectious disorders like mental disorders, cancer, allergies, and metabolic disorders. Concerning their mechanisms of action, probiotics have immunomodulatory and many other mechanisms of action, and work in diverse ways to exert beneficial effects on their hosts, if applied properly. However, concerning the safety and efficacy of probiotics, recent screening techniques rely on the capacity of microbes to elicit cytokine production mostly through cell lines or ex vivo isolated residual immune cells, even though they do not reflect the phenotype of intestinal cells. Awareness of the capability and usage of probiotics to improve the microbiota equilibrium in the host gut, to serve as immunomodulators, growth promoters, and to inhibit pathogenic infections is crucial from a practical point of view. It will help to make more progress by investigating more expertise, knowledge, and research on the understanding of probiotics, their specific mechanism of action, and their complete applicability for the safety of the host. More importantly, the safety of probiotics during application should also be carefully considered and strictly evaluated in the future in case of the emergence and spread of antibiotic-resistant bacteria between hosts. Thus, high-throughput validation approaches, as well as comprehensive and credible clinical, in vivo, and in vitro research on probiotic administration are warranted to clearly illustrate the advantages and adverse effects of probiotics.
SC and LL designed, modified, and reviewed the manuscript. AR and GZ wrote the manuscript. All authors contributed to the article and approved the submitted version.
This work was supported by Beijing Innovation Consortium of Agriculture Research System (BAIC04-2021), the Agricultural Science and Technology Innovation Program (Grant Number: ASTIP-IAS15), and Central Public-interest Scientific Institution Basal Research Fund (Grant Number: 2021-YWF-ZYSQ-10).
The authors declare that the research was conducted in the absence of any commercial or financial relationships that could be construed as a potential conflict of interest.
1. Dhama K, Verma V, Sawant P, Tiwari R, Vaid R, Chauhan R. Applications of probiotics in poultry: enhancing immunity and beneficial effects on production performances and health: a review. J Immunol Immunopathol. (2011) 13:1–19.
2. Santacroce L, Charitos IA, Bottalico L. A successful history: probiotics and their potential as antimicrobials. Expert Rev Anti Infect Ther. (2019) 17:635–45. doi: 10.1080/14787210.2019.1645597
3. Kopp-Hoolihan L. Prophylactic and therapeutic uses of probiotics: a review. J Am Diet Assoc. (2001) 101:229–41. doi: 10.1016/S0002-8223(01)00060-8
4. Zommiti M, Feuilloley MG, Connil N. Update of probiotics in human world: a nonstop source of benefactions till the end of time. Microorganisms. (2020) 8:1907. doi: 10.3390/microorganisms8121907
5. Hoseinifar SH, Sun Y-Z, Wang A, Zhou Z. Probiotics as means of diseases control in aquaculture, a review of current knowledge and future perspectives. Front Microbiol. (2018) 9:2429. doi: 10.3389/fmicb.2018.02429
6. Zorriehzahra MJ, Delshad ST, Adel M, Tiwari R, Karthik K, Dhama K, et al. Probiotics as beneficial microbes in aquaculture: an update on their multiple modes of action: a review. Vet Q. (2016) 36:228–41. doi: 10.1080/01652176.2016.1172132
7. Dhama K, Mahendran M, Tomar S, Chauhan R. Beneficial effects of probiotics and prebiotics in livestock and poultry: the current perspectives. Intas Polivet. (2008) 9:1–12.
8. Cutino-Moguel MT, Eades C, Rezvani K, Armstrong-James D. Immunotherapy for infectious diseases in haematological immunocompromise. Br J Haematol. (2017) 177:348–56. doi: 10.1111/bjh.14595
9. Plaza-Diaz J, Ruiz-Ojeda FJ, Gil-Campos M, Gil A. Mechanisms of action of probiotics. Adv Nutr. (2019) 10:S49–66. doi: 10.1093/advances/nmy063
10. Takeuchi O, Akira S. Pattern recognition receptors and inflammation. Cell. (2010) 140:805–20. doi: 10.1016/j.cell.2010.01.022
11. Bermudez-Brito M, Plaza-Díaz J, Muñoz-Quezada S, Gómez-Llorente C, Gil A. Probiotic mechanisms of action. Ann Nutr Metab. (2012) 61:160–74. doi: 10.1159/000342079
12. Mazloom K, Siddiqi I, Covasa M. Probiotics: how effective are they in the fight against obesity? Nutrients. (2019) 11:258. doi: 10.3390/nu11020258
13. Gasbarrini G, Bonvicini F, Gramenzi A. Probiotics history. J Clin Gastroenterol. (2016) 50:S116–9. doi: 10.1097/MCG.0000000000000697
14. Gogineni VK, Morrow LE, Gregory PJ, Malesker MA. Probiotics: history and evolution. J Anc Dis Prev Rem. (2013) 1:1–7. doi: 10.4172/2329-8731.1000107
15. König H, Fröhlich J. Lactic acid bacteria. In: König H, Unden G, Fröhlich J, editors. Biology of Microorganisms on Grapes Must and in Wine. Cham: Springer (2017). p. 3–41. doi: 10.1007/978-3-319-60021-5_1
16. Mokoena MP. Lactic acid bacteria and their bacteriocins: classification, biosynthesis and applications against uropathogens: a mini-review. Molecules. (2017) 22:1255. doi: 10.3390/molecules22081255
17. Quinto EJ, Jiménez P, Caro I, Tejero J, Mateo J, Girbés T. Probiotic lactic acid bacteria: a review. Food Nutr Sci. (2014) 5:1765. doi: 10.4236/fns.2014.518190
18. Vinderola G, Ouwehand A, Salminen S, von Wright A (editors). Lactic Acid Bacteria: Microbiological and Functional Aspects. CRC Press (2019).
19. Sagheddu V, Guidesi E, Galletti S, Elli M. Original paper selection and characterization criteria of probiotics intended for human use from the past to the future. Food Sci Nutr. (2019) 3. doi: 10.22158/fsns.v3n2p73
20. Thakur N, Rokana N, Panwar H. Probiotics: selection criteria, safety and role in health and disease. J Innov Biol. (2016) 3:259–70.
21. Shokryazdan P, Faseleh Jahromi M, Liang JB, Ho YW. Probiotics: from isolation to application. J Am Coll Nutr. (2017) 36:666–76. doi: 10.1080/07315724.2017.1337529
22. Baud D, Dimopoulou Agri V, Gibson GR, Reid G, Giannoni E. Using probiotics to flatten the curve of coronavirus disease COVID-2019 pandemic. Front Public Health. (2020) 8:186. doi: 10.3389/fpubh.2020.00186
23. Yao M, Xie J, Du H, McClements DJ, Xiao H, Li L. Progress in microencapsulation of probiotics: a review. Comprehens Rev Food Sci Food Safety. (2020) 19:857–74. doi: 10.1111/1541-4337.12532
24. Iravani S, Korbekandi H, Mirmohammadi SV. Technology and potential applications of probiotic encapsulation in fermented milk products. J Food Sci Technol. (2015) 52:4679–96. doi: 10.1007/s13197-014-1516-2
25. Hansen LT, Allan-Wojtas P, Jin Y-L, Paulson A. Survival of Ca-alginate microencapsulated Bifidobacterium spp. in milk and simulated gastrointestinal conditions. Food Microbiol. (2002) 19:35–45. doi: 10.1006/fmic.2001.0452
26. Yeung TW, Arroyo-Maya IJ, McClements DJ, Sela DA. Microencapsulation of probiotics in hydrogel particles: enhancing Lactococcus lactis subsp. cremoris LM0230 viability using calcium alginate beads. Food Funct. (2016) 7:1797–804. doi: 10.1039/C5FO00801H
27. Muhammad Z, Ramzan R, Zhang S, Hu H, Hameed A, Bakry AM, et al. Comparative assessment of the bioremedial potentials of potato resistant starch-based microencapsulated and non-encapsulated Lactobacillus plantarum to alleviate the effects of chronic lead toxicity. Front Microbiol. (2018) 9:1306. doi: 10.3389/fmicb.2018.01306
28. Riaz T, Iqbal MW, Saeed M, Yasmin I, Hassanin HA, Mahmood S, et al. In vitro survival of Bifidobacterium bifidum microencapsulated in zein-coated alginate hydrogel microbeads. J Microencapsul. (2019) 36:192–203. doi: 10.1080/02652048.2019.1618403
29. Yan F, Polk DB. Probiotics and probiotic-derived functional factors-mechanistic insights into applications for intestinal homeostasis. Front Immunol. (2020) 11:1428. doi: 10.3389/fimmu.2020.01428
30. Wang J, Zeng Y, Wang S, Liu H, Zhang D, Zhang W, et al. Swine-derived probiotic Lactobacillus plantarum inhibits growth and adhesion of enterotoxigenic Escherichia coli and mediates host defense. Front Microbiol. (2018) 9:1364. doi: 10.3389/fmicb.2018.01364
31. Gaspar C, Donders G, Palmeira-de-Oliveira R, Queiroz J, Tomaz C, Martinez-de-Oliveira J, et al. Bacteriocin production of the probiotic Lactobacillus acidophilus KS400. AMB Express. (2018) 8:153. doi: 10.1186/s13568-018-0679-z
32. Collado MC, Gueimonde M, Salminen S. Chapter 23 - probiotics in adhesion of pathogens: mechanisms of action. In: Watson RR, Preedy VR, editors. Bioactive Foods in Promoting Health. Elsevier (2010). p. 353–70. doi: 10.1016/B978-0-12-374938-3.00023-2
33. Fukuda K. Is it feasible to control pathogen infection by competitive binding of probiotics to the host? Virulence. (2017) 8:1502–5. doi: 10.1080/21505594.2017.1382798
34. Kline KA, Fälker S, Dahlberg S, Normark S, Henriques-Normark B. Bacterial adhesins in host-microbe interactions. Cell Host Microbe. (2009) 5:580–92. doi: 10.1016/j.chom.2009.05.011
35. Glenting J, Beck HC, Vrang A, Riemann H, Ravn P, Hansen AM, et al. Anchorless surface associated glycolytic enzymes from Lactobacillus plantarum 299v bind to epithelial cells and extracellular matrix proteins. Microbiol Res. (2013) 168:245–53. doi: 10.1016/j.micres.2013.01.003
36. Vastano V, Salzillo M, Siciliano RA, Muscariello L, Sacco M, Marasco R. The E1 beta-subunit of pyruvate dehydrogenase is surface-expressed in Lactobacillus plantarum and binds fibronectin. Microbiol Res. (2014) 169:121–7. doi: 10.1016/j.micres.2013.07.013
37. Nishiyama K, Nakazato A, Ueno S, Seto Y, Kakuda T, Takai S, et al. Cell surface-associated aggregation-promoting factor from Lactobacillus gasseri SBT 2055 facilitates host colonization and competitive exclusion of Campylobacter jejuni. Mol Microbiol1. (2015) 98:712–26. doi: 10.1111/mmi.13153
38. Zuo F, Appaswamy A, Gebreegziabher H, Jonsson A-B. Role of sortase A in Lactobacillus gasseri Kx110A1 adhesion to gastric epithelial cells and competitive exclusion of Helicobacter pylori. Front Microbiol. (2019) 10:2770. doi: 10.3389/fmicb.2019.02770
39. Sharma K, Pooranachithra M, Balamurugan K, Goel G. Probiotic mediated colonization resistance against E. coli infection in experimentally challenged Caenorhabditis elegans. Microb Pathog. (2019) 127:39–47. doi: 10.1016/j.micpath.2018.11.041
40. Lim S-Y, Loo KW, Wong W-L. Synergistic antimicrobial effect of a seaweed-probiotic blend against acute hepatopancreatic necrosis disease (AHPND)-causing Vibrio parahaemolyticus. Probiotics Antimicrob Proteins. (2019) 12:906–17. doi: 10.1007/s12602-019-09616-8
41. Celebioglu HU, Olesen SV, Prehn K, Lahtinen SJ, Brix S, Hachem MA, et al. Mucin-and carbohydrate-stimulated adhesion and subproteome changes of the probiotic bacterium Lactobacillus acidophilus NCFM. J Proteomics. (2017) 163:102–10. doi: 10.1016/j.jprot.2017.05.015
42. Monteagudo-Mera A, Rastall RA, Gibson GR, Charalampopoulos D, Chatzifragkou A. Adhesion mechanisms mediated by probiotics and prebiotics and their potential impact on human health. Appl Microbiol Biotechnol. (2019) 103:6463–72. doi: 10.1007/s00253-019-09978-7
43. Falah F, Vasiee A, Behbahani BA, Yazdi FT, Moradi S, Mortazavi SA, et al. Evaluation of adherence and anti-infective properties of probiotic Lactobacillus fermentum strain 4-17 against Escherichia coli causing urinary tract infection in humans. Microb Pathog. (2019) 131:246–53. doi: 10.1016/j.micpath.2019.04.006
44. García-Ruiz A, de Llano DG, Esteban-Fernández A, Requena T, Bartolomé B, Moreno-Arribas MV. Assessment of probiotic properties in lactic acid bacteria isolated from wine. Food Microbiol. (2014) 44:220–5. doi: 10.1016/j.fm.2014.06.015
45. Pisano MB, Viale S, Conti S, Fadda ME, Deplano M, Melis MP, et al. Preliminary evaluation of probiotic properties of Lactobacillus strains isolated from Sardinian dairy products. Biomed Res Int. (2014) 2014:286390. doi: 10.1155/2014/286390
46. Anderson RC, Cookson AL, McNabb WC, Park Z, McCann MJ, Kelly WJ, et al. Lactobacillus plantarum MB452 enhances the function of the intestinal barrier by increasing the expression levels of genes involved in tight junction formation. BMC Microbiol. (2010) 10:316. doi: 10.1186/1471-2180-10-316
47. Chen C-Y, Tsen H-Y, Lin C-L, Yu B, Chen C-S. Oral administration of a combination of select lactic acid bacteria strains to reduce the Salmonella invasion and inflammation of broiler chicks. Poult Sci. (2012) 91:2139–47. doi: 10.3382/ps.2012-02237
48. Heeney DD, Zhai Z, Bendiks Z, Barouei J, Martinic A, Slupsky C, et al. Lactobacillus plantarum bacteriocin is associated with intestinal and systemic improvements in diet-induced obese mice and maintains epithelial barrier integrity in vitro. Gut Microbes. (2019) 10:382–97. doi: 10.1080/19490976.2018.1534513
49. Karczewski J, Troost FJ, Konings I, Dekker J, Kleerebezem M, Brummer R-JM, et al. Regulation of human epithelial tight junction proteins by Lactobacillus plantarum in vivo and protective effects on the epithelial barrier. Am J Physiol Gastrointestinal Liver Physiol. (2010) 298:G851–9. doi: 10.1152/ajpgi.00327.2009
50. Qin H, Zhang Z, Hang X, Jiang Y. L. plantarum prevents enteroinvasive Escherichia coli-induced tight junction proteins changes in intestinal epithelial cells. BMC Microbiol. (2009) 9:63. doi: 10.1186/1471-2180-9-63
51. Zhang Y-g, Wu S, Xia Y, Sun J. Salmonella infection upregulates the leaky protein claudin-2 in intestinal epithelial cells. PLoS ONE. (2013) 8:e58606. doi: 10.1371/journal.pone.0058606
52. Stavropoulou E, Bezirtzoglou E. Probiotics as a weapon in the fight against COVID-19. Front Nutr. (2020) 7:614986. doi: 10.3389/fnut.2020.614986
53. Lievin-Le Moal V, Amsellem R, Servin A, Coconnier M. Lactobacillus acidophilus (strain LB) from the resident adult human gastrointestinal microflora exerts activity against brush border damage promoted by a diarrhoeagenic Escherichia coli in human enterocyte-like cells. Gut. (2002) 50:803–11. doi: 10.1136/gut.50.6.803
54. Parassol N, Freitas M, Thoreux K, Dalmasso G, Bourdet-Sicard R, Rampal P. Lactobacillus casei DN-114 001 inhibits the increase in paracellular permeability of enteropathogenic Escherichia coli-infected T84 cells. Res Microbiol. (2005) 156:256–62. doi: 10.1016/j.resmic.2004.09.013
55. Wang Y, Kasper LH. The role of microbiome in central nervous system disorders. Brain Behav Immun. (2014) 38:1–12. doi: 10.1016/j.bbi.2013.12.015
56. Alvarez C-S, Giménez R, Cañas M-A, Vera R, Díaz-Garrido N, Badia J, et al. Extracellular vesicles and soluble factors secreted by Escherichia coli Nissle 1917 and ECOR63 protect against enteropathogenic E. coli-induced intestinal epithelial barrier dysfunction. BMC Microbiol. (2019) 19:166. doi: 10.1186/s12866-019-1534-3
57. Bhat MI, Kapila S, Kapila R. Lactobacillus fermentum (MTCC-5898) supplementation renders prophylactic action against Escherichia coli impaired intestinal barrier function through tight junction modulation. LWT. (2020) 123:109118. doi: 10.1016/j.lwt.2020.109118
58. Wang Y, Yan X, Han D, Liu Y, Song W, Tong T, et al. Lactobacillus casei DBN023 protects against jejunal mucosal injury in chicks infected with Salmonella pullorum CMCC-533. Res Vet Sci. (2019) 127:33–41. doi: 10.1016/j.rvsc.2019.09.010
59. Garg S, Singh T, Reddi S, Malik R, Kapila S. Intervention of probiotic L. reuteri fermented milk as an adjuvant to combat protein energy malnourishment induced gut disturbances in albino mice. J Funct Foods. (2017) 36:467–79. doi: 10.1016/j.jff.2017.07.017
60. Yang X, Yousef AE. Antimicrobial peptides produced by Brevibacillus spp.: structure, classification and bioactivity: a mini review. World J Microbiol Biotechnol. (2018) 34:57. doi: 10.1007/s11274-018-2437-4
61. Baindara P, Korpole S, Grover V. Bacteriocins: perspective for the development of novel anticancer drugs. Appl Microbiol Biotechnol. (2018) 102:10393–408. doi: 10.1007/s00253-018-9420-8
62. Mandal SM, Pati BR, Chakraborty R, Franco OL. New insights into the bioactivity of peptides from probiotics. Front Biosci. (2016) 8:450–9. doi: 10.2741/e779
63. Messaoudi S, Manai M, Kergourlay G, Prévost H, Connil N, Chobert J-M, et al. Lactobacillus salivarius: bacteriocin and probiotic activity. Food Microbiol. (2013) 36:296–304. doi: 10.1016/j.fm.2013.05.010
64. Borrero J, Kelly E, O'Connor PM, Kelleher P, Scully C, Cotter PD, et al. Plantaricyclin A, a novel circular bacteriocin produced by Lactobacillus plantarum NI326: purification, characterization, and heterologous production. Appl Environ Microbiol. (2018) 84:e01801–17. doi: 10.1128/AEM.01801-17
65. Oleskin AV, Shenderov BA. Probiotics and psychobiotics: the role of microbial neurochemicals. Probiotics Antimicrob Proteins. (2019) 11:1071–85. doi: 10.1007/s12602-019-09583-0
66. Dinev T, Beev G, Tzanova M, Denev S, Dermendzhieva D, Stoyanova A. Antimicrobial activity of Lactobacillus plantarum against pathogenic and food spoilage microorganisms: a review. Bulgarian J Vet Med. (2018) 21:253–68. doi: 10.15547/bjvm.1084
67. Sahoo TK, Jena PK, Patel AK, Seshadri S. Bacteriocins and their applications for the treatment of bacterial diseases in aquaculture: a review. Aquac Res. (2016) 47:1013–27. doi: 10.1111/are.12556
68. Gómez-Llorente C, Munoz S, Gil A. Role of Toll-like receptors in the development of immunotolerance mediated by probiotics. Proc Nutr Soc. (2010) 69:381–9. doi: 10.1017/S0029665110001527
69. Lebeer S, Vanderleyden J, De Keersmaecker SC. Host interactions of probiotic bacterial surface molecules: comparison with commensals and pathogens. Nat Rev Microbiol. (2010) 8:171–84. doi: 10.1038/nrmicro2297
70. Rhayat L, Maresca M, Nicoletti C, Perrier J, Brinch KS, Christian S, et al. Effect of Bacillus subtilis strains on intestinal barrier function and inflammatory response. Front Immunol. (2019) 10:564. doi: 10.3389/fimmu.2019.00564
71. Foligné B, Dewulf J, Breton J, Claisse O, Lonvaud-Funel A, Pot B. Probiotic properties of non-conventional lactic acid bacteria: immunomodulation by Oenococcus oeni. Int J Food Microbiol. (2010) 140:136–45. doi: 10.1016/j.ijfoodmicro.2010.04.007
72. Savan R, Sakai M. Genomics of fish cytokines. Compar Biochem Physiol Part D Genomics Proteomics. (2006) 1:89–101. doi: 10.1016/j.cbd.2005.08.005
73. Azad M, Kalam A, Sarker M, Wan D. Immunomodulatory effects of probiotics on cytokine profiles. Biomed Res Int. (2018) 2018:8063647. doi: 10.1155/2018/8063647
74. Chiba Y, Shida K, Nagata S, Wada M, Bian L, Wang C, et al. Well-controlled proinflammatory cytokine responses of Peyer's patch cells to probiotic Lactobacillus casei. Immunology. (2010) 130:352–62. doi: 10.1111/j.1365-2567.2009.03204.x
75. Haller D, Bode C, Hammes W, Pfeifer A, Schiffrin E, Blum S. Non-pathogenic bacteria elicit a differential cytokine response by intestinal epithelial cell/leucocyte co-cultures. Gut. (2000) 47:79–87. doi: 10.1136/gut.47.1.79
76. Peña JA, Rogers AB, Ge Z, Ng V, Li SY, Fox JG, et al. Probiotic Lactobacillus spp. diminish Helicobacter hepaticus-induced inflammatory bowel disease in interleukin-10-deficient mice. Infect Immun. (2005) 73:912–20. doi: 10.1128/IAI.73.2.912-920.2005
77. Kourelis A, Zinonos I, Kakagianni M, Christidou A, Christoglou N, Yiannaki E, et al. Validation of the dorsal air pouch model to predict and examine immunostimulatory responses in the gut. J Appl Microbiol. (2010) 108:274–84. doi: 10.1111/j.1365-2672.2009.04421.x
78. Wells JM. Immunomodulatory mechanisms of lactobacilli. Paper presented at the Microbial Cell Factories. Wageningen: University of Wageningen, Animal Sciences (2011). doi: 10.1186/1475-2859-10-S1-S17
79. Kwon H-K, Lee C-G, So J-S, Chae C-S, Hwang J-S, Sahoo A, et al. Generation of regulatory dendritic cells and CD4+ Foxp3+ T cells by probiotics administration suppresses immune disorders. Proc Natl Acad Sci USA. (2010) 107:2159–64. doi: 10.1073/pnas.0904055107
80. Pinto MGV, Gómez MR, Seifert S, Watzl B, Holzapfel WH, Franz CM. Lactobacilli stimulate the innate immune response and modulate the TLR expression of HT29 intestinal epithelial cells in vitro. Int J Food Microbiol. (2009) 133:86–93. doi: 10.1016/j.ijfoodmicro.2009.05.013
81. Abreu MT, Fukata M, Arditi M. TLR signaling in the gut in health and disease. J Immunol. (2005) 174:4453–60. doi: 10.4049/jimmunol.174.8.4453
82. Castillo NA, Perdigón G, de LeBlanc AdM. Oral administration of a probiotic Lactobacillus modulates cytokine production and TLR expression improving the immune response against Salmonella enterica serovar Typhimurium infection in mice. BMC Microbiol. (2011) 11:177. doi: 10.1186/1471-2180-11-177
83. Weiss DS, Raupach B, Takeda K, Akira S, Zychlinsky A. Toll-like receptors are temporally involved in host defense. J Immunol. (2004) 172:4463–9. doi: 10.4049/jimmunol.172.7.4463
84. Giahi L, Aumueller E, Elmadfa I, Haslberger A. Regulation of TLR4, p38 MAPkinase, IκB and miRNAs by inactivated strains of lactobacilli in human dendritic cells. Benef Microbes. (2012) 3:91–8. doi: 10.3920/BM2011.0052
85. Lee J, Mo J-H, Katakura K, Alkalay I, Rucker AN, Liu Y-T, et al. Maintenance of colonic homeostasis by distinctive apical TLR9 signalling in intestinal epithelial cells. Nat Cell Biol. (2006) 8:1327–36. doi: 10.1038/ncb1500
86. Ghadimi D, Vrese Md, Heller KJ, Schrezenmeir J. Effect of natural commensal-origin DNA on toll-like receptor 9 (TLR9) signaling cascade, chemokine IL-8 expression, and barrier integritiy of polarized intestinal epithelial cells. Inflamm Bowel Dis. (2010) 16:410–27. doi: 10.1002/ibd.21057
87. Verstrepen L, Bekaert T, Chau T-L, Tavernier J, Chariot A, Beyaert R. TLR-4, IL-1R and TNF-R signaling to NF-κB: variations on a common theme. Cell Mol Life Sci. (2008) 65:2964–78. doi: 10.1007/s00018-008-8064-8
88. Hee Kim C, Geun Kim H, Yun Kim J, Ra Kim N, Jun Jung B, Hye Jeong J, et al. Probiotic genomic DNA reduces the production of pro-inflammatory cytokine tumor necrosis factor-alpha. FEMS Microbiol Lett. (2012) 328:13–9. doi: 10.1111/j.1574-6968.2011.02470.x
89. Hakansson A, Molin G. Gut microbiota and inflammation. Nutrients. (2011) 3:637–82. doi: 10.3390/nu3060637
90. Biswas A, Petnicki-Ocwieja T, Kobayashi KS. Nod2: a key regulator linking microbiota to intestinal mucosal immunity. J Mol Med. (2012) 90:15–24. doi: 10.1007/s00109-011-0802-y
91. Chen G, Shaw MH, Kim Y-G, Nuñez G. NOD-like receptors: role in innate immunity and inflammatory disease. Annu Rev Pathol Mech Dis. (2009) 4:365–98. doi: 10.1146/annurev.pathol.4.110807.092239
92. Fernandez EM, Valenti V, Rockel C, Hermann C, Pot B, Boneca IG, et al. Anti-inflammatory capacity of selected lactobacilli in experimental colitis is driven by NOD2-mediated recognition of a specific peptidoglycan-derived muropeptide. Gut. (2011) 60:1050–9. doi: 10.1136/gut.2010.232918
93. Tohno M, Shimosato T, Aso H, Kitazawa H. Immunobiotic Lactobacillus strains augment NLRP3 expression in newborn and adult porcine gut-associated lymphoid tissues. Vet Immunol Immunopathol. (2011) 144:410–6. doi: 10.1016/j.vetimm.2011.09.010
94. Bhardwaj R, Singh BP, Sandhu N, Singh N, Kaur R, Rokana N, et al. Probiotic mediated NF-κB regulation for prospective management of type 2 diabetes. Mol Biol Rep. (2020) 47:2301–13. doi: 10.1007/s11033-020-05254-4
95. Tang C, Zhu G. Classic and novel signaling pathways involved in cancer: targeting the NF-κB and Syk signaling pathways. Curr Stem Cell Res Ther. (2019) 14:219–25. doi: 10.2174/1574888X13666180723104340
96. Tien M-T, Girardin SE, Regnault B, Le Bourhis L, Dillies M-A, Coppée J-Y, et al. Anti-inflammatory effect of Lactobacillus casei on Shigella-infected human intestinal epithelial cells. J Immunol. (2006) 176:1228–37. doi: 10.4049/jimmunol.176.2.1228
97. Johnson-Henry KC, Nadjafi M, Avitzur Y, Mitchell DJ, Ngan B-Y, Galindo-Mata E, et al. Amelioration of the effects of Citrobacter rodentium infection in mice by pretreatment with probiotics. J Infect Dis. (2005) 191:2106–17. doi: 10.1086/430318
98. Kim SW, Kim HM, Yang KM, Kim S-A, Kim S-K, An MJ, et al. Bifidobacterium lactis inhibits NF-κB in intestinal epithelial cells and prevents acute colitis and colitis-associated colon cancer in mice. Inflamm Bowel Dis. (2010) 16:1514–25. doi: 10.1002/ibd.21262
99. Bu X, Lian X, Wang Y, Luo C, Tao S, Liao Y, et al. Dietary yeast culture modulates immune response related to TLR2-MyD88-NF-kβ signaling pathway, antioxidant capability and disease resistance against Aeromonas hydrophila for Ussuri catfish (Pseudobagrus ussuriensis). Fish Shellfish Immunol. (2019) 84:711–8. doi: 10.1016/j.fsi.2018.10.049
100. Iyer C, Kosters A, Sethi G, Kunnumakkara AB, Aggarwal BB, Versalovic J. Probiotic Lactobacillus reuteri promotes TNF-induced apoptosis in human myeloid leukemia-derived cells by modulation of NF-κB and MAPK signalling. Cell Microbiol. (2008) 10:1442–52. doi: 10.1111/j.1462-5822.2008.01137.x
101. Kaci G, Lakhdari O, Doré J, Ehrlich SD, Renault P, Blottière HM, et al. Inhibition of the NF-κB pathway in human intestinal epithelial cells by commensal Streptococcus salivarius. Appl Environ Microbiol. (2011) 77:4681–4. doi: 10.1128/AEM.03021-10
102. Liu Y, Fatheree NY, Mangalat N, Rhoads JM. Lactobacillus reuteri strains reduce incidence and severity of experimental necrotizing enterocolitis via modulation of TLR4 and NF-κB signaling in the intestine. Am J Physiol Gastrointestinal Liver Physiol. (2012) 302:G608–17. doi: 10.1152/ajpgi.00266.2011
103. Lee J-M, Hwang K-T, Jun W-J, Park C-S, Lee M-Y. Antiinflammatory effect of lactic acid bacteria: inhibition of cyclooxygenase-2 by suppressing nuclear factor-kappaB in Raw264. 7 macrophage cells. J Microbiol Biotechnol. (2008) 18:1683–8.
104. Sun K-Y, Xu D-H, Xie C, Plummer S, Tang J, Yang XF, et al. Lactobacillus paracasei modulates LPS-induced inflammatory cytokine release by monocyte-macrophages via the up-regulation of negative regulators of NF-kappaB signaling in a TLR2-dependent manner. Cytokine. (2017) 92:1–11. doi: 10.1016/j.cyto.2017.01.003
105. Petrof EO, Claud EC, Sun J, Abramova T, Guo Y, Waypa TS, et al. Bacteria-free solution derived from Lactobacillus plantarum inhibits multiple NF-kappaB pathways and inhibits proteasome function. Inflamm Bowel Dis. (2009) 15:1537–47. doi: 10.1002/ibd.20930
106. Jang SE, Hyam S, Han M, Kim SY, Lee BG, Kim DH. Lactobacillus brevis G-101 ameliorates colitis in mice by inhibiting NF-κB, MAPK and AKT pathways and by polarizing M1 macrophages to M2-like macrophages. J Appl Microbiol. (2013) 115:888–96. doi: 10.1111/jam.12273
107. Kemgang TS, Kapila S, Shanmugam VP, Reddi S, Kapila R. Fermented milk with probiotic Lactobacillus rhamnosus S1K3 (MTCC5957) protects mice from salmonella by enhancing immune and nonimmune protection mechanisms at intestinal mucosal level. J Nutr Biochem. (2016) 30:62–73. doi: 10.1016/j.jnutbio.2015.11.018
108. Chen Q, Tong C, Ma S, Zhou L, Zhao L, Zhao X. Involvement of microRNAs in probiotics-induced reduction of the cecal inflammation by Salmonella Typhimurium. Front Immunol. (2017) 8:704. doi: 10.3389/fimmu.2017.00704
109. Martins FS, Nardi RM, Arantes RM, Rosa CA, Neves MJ, Nicoli JR. Screening of yeasts as probiotic based on capacities to colonize the gastrointestinal tract and to protect against enteropathogen challenge in mice. J Gen Appl Microbiol. (2005) 51:83–92. doi: 10.2323/jgam.51.83
110. Martins FS, Rodrigues ACP, Tiago FC, Penna FJ, Rosa CA, Arantes RM, et al. Saccharomyces cerevisiae strain 905 reduces the translocation of Salmonella enterica serotype Typhimurium and stimulates the immune system in gnotobiotic and conventional mice. J Med Microbiol. (2007) 56:352–9. doi: 10.1099/jmm.0.46525-0
111. Martins FS, Elian SD, Vieira AT, Tiago FC, Martins AK, Silva FC, et al. Oral treatment with Saccharomyces cerevisiae strain UFMG 905 modulates immune responses and interferes with signal pathways involved in the activation of inflammation in a murine model of typhoid fever. Int J Med Microbiol. (2011) 301:359–64. doi: 10.1016/j.ijmm.2010.11.002
112. Martins FS, Dalmasso G, Arantes RM, Doye A, Lemichez E, Lagadec P, et al. Interaction of Saccharomyces boulardii with Salmonella enterica serovar Typhimurium protects mice and modifies T84 cell response to the infection. PLoS ONE. (2010) 5:e8925. doi: 10.1371/journal.pone.0008925
113. Gebremariam HG, Qazi RK, Somiah T, Psthak SK, Sjölinder H, Sverremark-Ekström E, et al. Lactobacillus gasseri suppresses the production of the proinflammatory cytokines in Helicobacter pylori-infected macrophages by inhibiting the expression of ADAM17. Front Immunol. (2019) 10:2326. doi: 10.3389/fimmu.2019.02326
114. Garcia-Castillo V, Zelaya H, Ilabaca A, Espinoza-Monje M, Komatsu R, Albarracín L, et al. Lactobacillus fermentum UCO-979C beneficially modulates the innate immune response triggered by Helicobacter pylori infection in vitro. Benef Microbes. (2018) 9:829–41. doi: 10.3920/bm2018.0019
115. Yu H-J, Liu W, Chang Z, Shen H, He L-J, Wang S-S, et al. Probiotic BIFICO cocktail ameliorates Helicobacter pylori induced gastritis. World J Gastroenterol. (2015) 21:6561. doi: 10.3748/wjg.v21.i21.6561
116. Chen Y-H, Tsai W-H, Wu H-Y, Chen C-Y, Yeh W-L, Chen Y-H, et al. Probiotic Lactobacillus spp. act against Helicobacter pylori-induced inflammation. J Clin Med. (2019) 8:90. doi: 10.3390/jcm8010090
117. Boltin D. Probiotics in Helicobacter pylori-induced peptic ulcer disease. Best Prac Res Clin Gastroenterol. (2016) 30:99–109. doi: 10.1016/j.bpg.2015.12.003
118. Song H, Zhou L, Liu D, Ge L, Li Y. Probiotic effect on Helicobacterápylori attachment and inhibition of inflammation in human gastric epithelial cells. Exp Ther Med. (2019) 18:1551–62. doi: 10.3892/etm.2019.7742
119. Westerik N, Reid G, Sybesma W, Kort R. The probiotic Lactobacillus rhamnosus for alleviation of Helicobacter pylori-associated gastric pathology in East Africa. Front Microbiol. (2018) 9:1873. doi: 10.3389/fmicb.2018.01873
120. Takeda S, Igoshi K, Tsend-Ayush C, Oyunsuren T, Sakata R, Koga Y, et al. Lactobacillus paracasei strain 06TCa19 suppresses inflammatory chemokine induced by Helicobacter pylori in human gastric epithelial cells. Hum Cell. (2017) 30:258–66. doi: 10.1007/s13577-017-0172-z
121. Qamar A, Aboudola S, Warny M, Michetti P, Pothoulakis C, LaMont JT, et al. Microbial immunity and vaccines- Saccharomyces boulardii stimulates intestinal immunoglobulin A immune response to Clostridium difficile toxin A in mice. Infect Immun. (2001) 69:2762–5. doi: 10.1128/IAI.69.4.2762-2765.2001
122. Chen X, Kokkotou EG, Mustafa N, Bhaskar KR, Sougioultzis S, O'Brien M, et al. Saccharomyces boulardii inhibits ERK1/2 mitogen-activated protein kinase activation both in vitro and in vivo and protects against Clostridium difficile toxin A-induced enteritis. J Biol Chem. (2006) 281:24449–54. doi: 10.1074/jbc.M605200200
123. Na X, Kelly C. Probiotics in Clostridium difficile infection. J Clin Gastroenterol. (2011) 45:S154. doi: 10.1097/MCG.0b013e31822ec787
124. Souza RFS, Rault L, Seyffert N, Azevedo V, Le Loir Y, Even S. Lactobacillus casei BL23 modulates the innate immune response in Staphylococcus aureus-stimulated bovine mammary epithelial cells. Benef Microbes. (2018) 9:985–95. doi: 10.3920/BM2018.0010
125. Paik W, Alonzo F, Knight KL. Probiotic exopolysaccharide protects against systemic Staphylococcus aureus infection, inducing dual-functioning macrophages that restrict bacterial growth and limit inflammation. Infect Immun. (2019) 87:e00791–18. doi: 10.1128/IAI.00791-18
126. Lukic J, Jancic I, Mirkovic N, Bufan B, Djokic J, Milenkovic M, et al. Lactococcus lactis and Lactobacillus salivarius differently modulate early immunological response of Wistar rats co-administered with Listeria monocytogenes. Benef Microbes. (2017) 8:809–22. doi: 10.3920/BM2017.0007
127. Dos Santos LM, Santos MM, de Souza Silva HP, Arantes RME, Nicoli JR, Vieira LQ. Monoassociation with probiotic Lactobacillus delbrueckii UFV-H2b20 stimulates the immune system and protects germfree mice against Listeria monocytogenes infection. Med Microbiol Immunol. (2011) 200:29–38. doi: 10.1007/s00430-010-0170-1
128. Rachakonda G, Vu T, Jin L, Samanta D, Datta PK. Role of TGF-β-induced Claudin-4 expression through c-Jun signaling in non-small cell lung cancer. Cell Signal. (2016) 28:1537–44. doi: 10.1016/j.cellsig.2016.07.006
129. Choi HJ, Shin MS, Lee SM, Lee WK. Immunomodulatory properties of Enterococcus faecium JWS 833 isolated from duck intestinal tract and suppression of Listeria monocytogenes infection. Microbiol Immunol. (2012) 56:613–20. doi: 10.1111/j.1348-0421.2012.00486.x
130. Li X-Q, Zhu Y-H, Zhang H-F, Yue Y, Cai Z-X, Lu Q-P, et al. Risks associated with high-dose Lactobacillus rhamnosus in an Escherichia coli model of piglet diarrhoea: intestinal microbiota and immune imbalances. PLoS ONE. (2012) 7:e40666. doi: 10.1371/journal.pone.0040666
131. Wang S, Peng Q, Jia H, Zeng X, Zhu J, Hou C, et al. Prevention of Escherichia coli infection in broiler chickens with Lactobacillus plantarum B1. Poult Sci. (2017) 96:2576–86. doi: 10.3382/ps/pex061
132. Zhang F, Zeng X, Yang F, Huang Z, Liu H, Ma X, et al. Dietary N-carbamylglutamate supplementation boosts intestinal mucosal immunity in Escherichia coli challenged piglets. PLoS ONE. (2013) 8:e66280. doi: 10.1371/journal.pone.0066280
133. Shimazu T, Villena J, Tohno M, Fujie H, Hosoya S, Shimosato T, et al. Immunobiotic Lactobacillus jensenii elicits anti-inflammatory activity in porcine intestinal epithelial cells by modulating negative regulators of the Toll-like receptor signaling pathway. Infect Immun. (2012) 80:276–88. doi: 10.1128/IAI.05729-11
134. Finamore A, Roselli M, Imbinto A, Seeboth J, Oswald IP, Mengheri E. Lactobacillus amylovorus inhibits the TLR4 inflammatory signaling triggered by enterotoxigenic Escherichia coli via modulation of the negative regulators and involvement of TLR2 in intestinal Caco-2 cells and pig explants. PLoS ONE. (2014) 9:e94891. doi: 10.1371/journal.pone.0094891
135. Wachi S, Kanmani P, Tomosada Y, Kobayashi H, Yuri T, Egusa S, et al. Lactobacillus delbrueckii TUA 4408 L and its extracellular polysaccharides attenuate enterotoxigenic Escherichia coli-induced inflammatory response in porcine intestinal epitheliocytes via T oll-like receptor-2 and 4. Mol Nutr Food Res. (2014) 58:2080–93. doi: 10.1002/mnfr.201400218
136. Zhang W, Zhu Y-H, Yang J-C, Yang G-Y, Zhou D, Wang J-F. A selected Lactobacillus rhamnosus strain promotes EGFR-independent Akt activation in an enterotoxigenic Escherichia coli K88-infected IPEC-J2 cell model. PLoS ONE. (2015) 10:e125717. doi: 10.1371/journal.pone.0125717
137. Zhen W, Shao Y, Gong X, Wu Y, Geng Y, Wang Z, et al. Effect of dietary Bacillus coagulans supplementation on growth performance and immune responses of broiler chickens challenged by Salmonella enteritidis. Poult Sci. (2018) 97:2654–66. doi: 10.3382/ps/pey119
138. Gallati C, Stephan R, Hächler H, Malorny B, Schroeter A, Nüesch-Inderbinen M. Characterization of Salmonella enterica subsp. enterica serovar 4,[5], 12: i:-clones isolated from human and other sources in Switzerland between 2007 and 2011. Foodborne Pathog Dis. (2013) 10:549–54. doi: 10.1089/fpd.2012.1407
139. Gilchrist JJ, MacLennan CA, Hill AV. Genetic susceptibility to invasive Salmonella disease. Nat Rev Immunol. (2015) 15:452–63. doi: 10.1038/nri3858
140. Majowicz S, Musto J, Scallan E, Angulo F, Kir KO, Brien SJ, et al. The global burden of collibacillosis. Clin Infect Dis. (2010) 50:882–9. doi: 10.1086/650733
141. Mascaro V, Pileggi C, Crinò M, Proroga YTR, Carullo MR, Graziani C, et al. Non-typhoidal Salmonella in Calabria, Italy: a laboratory and patient-based survey. BMJ Open. (2017) 7:e017037. doi: 10.1136/bmjopen-2017-017037
142. Zhang W, Wu Q, Zhu Y-H, Yang G, Yu J, Wang J, et al. Probiotic Lactobacillus rhamnosus GG induces alterations in ileal microbiota with associated CD3– CD19– T-bet+ IFNγ+/– cell subset homeostasis in pigs challenged with Salmonella enterica serovar 4,[5], 12: i. Front Microbiol. (2019) 10:977. doi: 10.3389/fmicb.2019.00977
143. Gut AM, Vasiljevic T, Yeager T, Donkor O. Salmonella infection-prevention and treatment by antibiotics and probiotic yeasts: a review. Microbiology. (2018) 164:1327–44. doi: 10.1099/mic.0.000709
144. Ruby T, McLaughlin L, Gopinath S, Monack D. Salmonella's long-term relationship with its host. FEMS Microbiol Rev. (2012) 36:600–15. doi: 10.1111/j.1574-6976.2012.00332.x
145. Ertelt JM, Johanns TM, Mysz MA, Nanton MR, Rowe JH, Aguilera MN, et al. Selective culling of high avidity antigen-specific CD4+ T cells after virulent Salmonella infection. Immunology. (2011) 134:487–97. doi: 10.1111/j.1365-2567.2011.03510.x
146. Pontier-Bres R, Munro P, Boyer L, Anty R, Imbert V, Terciolo C, et al. Saccharomyces boulardii modifies Salmonella typhimurium traffic and host immune responses along the intestinal tract. PLoS ONE. (2014) 9:e103069. doi: 10.1371/journal.pone.0103069
147. Dolowschiak T, Mueller AA, Pisan LJ, Feigelman R, Felmy B, Sellin ME, et al. IFN-γ hinders recovery from mucosal inflammation during antibiotic therapy for Salmonella gut infection. Cell Host Microbe. (2016) 20:238–49. doi: 10.1016/j.chom.2016.06.008
148. Martins FS, Vieira AT, Elian SD, Arantes RM, Tiago FC, Sousa LP, et al. Inhibition of tissue inflammation and bacterial translocation as one of the protective mechanisms of Saccharomyces boulardii against Salmonella infection in mice. Microbes Infect. (2013) 15:270–9. doi: 10.1016/j.micinf.2012.12.007
149. Rooks MG, Garrett WS. Gut microbiota, metabolites and host immunity. Nat Rev Immunol. (2016) 16:341–52. doi: 10.1038/nri.2016.42
150. Pradhan B, Guha D, Naik AK, Banerjee A, Tambat S, Chawla S, et al. Probiotics L. acidophilus and B. clausii modulate gut microbiota in Th1-and Th2-biased mice to ameliorate salmonella typhimurium-induced diarrhea. Probiotics Antimicrob Proteins. (2019) 11:887–904. doi: 10.1007/s12602-018-9436-5
151. Rokana N, Singh R, Mallappa RH, Batish VK, Grover S. Modulation of intestinal barrier function to ameliorate Salmonella infection in mice by oral administration of fermented milks produced with Lactobacillus plantarum MTCC 5690–a probiotic strain of Indian gut origin. J Med Microbiol. (2016) 65:1482–93. doi: 10.1099/jmm.0.000366
152. Carter A, Adams M, La Ragione RM, Woodward MJ. Colonisation of poultry by Salmonella Enteritidis S1400 is reduced by combined administration of Lactobacillus salivarius 59 and Enterococcus faecium PXN-33. Vet Microbiol. (2017) 199:100–7. doi: 10.1016/j.vetmic.2016.12.029
153. de Jesus Souza M, de Moraes JA, Da Silva VN, Helal-Neto E, Uberti AF, Scopel-Guerra A, et al. Helicobacter pylori urease induces pro-inflammatory effects and differentiation of human endothelial cells: cellular and molecular mechanism. Helicobacter. (2019) 24:e12573. doi: 10.1111/hel.12573
154. De Vries AC, Van Driel HF, Richardus JH, Ouwendijk M, Van Vuuren AJ, De Man RA, et al. Migrant communities constitute a possible target population for primary prevention of Helicobacter pylori-related complications in low incidence countries. Scand J Gastroenterol. (2008) 43:403–9. doi: 10.1080/00365520701814077
155. Mori N, Wada A, Hirayama T, Parks TP, Stratowa C, Yamamoto N. Activation of intercellular adhesion molecule 1 expression by Helicobacter pylori is regulated by NF-κB in gastric epithelial cancer cells. Infect Immun. (2000) 68:1806–14. doi: 10.1128/IAI.68.4.1806-1814.2000
156. Kim S-H, Woo H, Park M, Rhee K-J, Moon C, Lee D, et al. Cyanidin 3-O-glucoside reduces Helicobacter pylori VacA-induced cell death of gastric KATO III cells through inhibition of the SecA pathway. Int J Med Sci. (2014) 11:742. doi: 10.7150/ijms.7167
157. Tegtmeyer N, Neddermann M, Asche CI, Backert S. Subversion of host kinases: a key network in cellular signaling hijacked by Helicobacter pylori CagA. Mol Microbiol. (2017) 105:358–72. doi: 10.1111/mmi.13707
158. Kim SO, Sheikh HI, Ha SD, Martins A, Reid G. G-CSF-mediated inhibition of JNK is a key mechanism for Lactobacillus rhamnosus-induced suppression of TNF production in macrophages. Cell Microbiol. (2006) 8:1958–71. doi: 10.1111/j.1462-5822.2006.00763.x
159. Myllyluoma E, Ahonen A-M, Korpela R, Vapaatalo H, Kankuri E. Effects of multispecies probiotic combination on Helicobacter pylori infection in vitro. Clin Vaccine Immunol. (2008) 15:1472–82. doi: 10.1128/CVI.00080-08
160. Myllyluoma E, Kajander K, Mikkola H, Kyrönpalo S, Rasmussen M, Kankuri E, et al. Probiotic intervention decreases serum gastrin-17 in Helicobacter pylori infection. Digestive Liver Dis. (2007) 39:516–23. doi: 10.1016/j.dld.2007.02.015
161. Rokka S, Myllykangas S, Joutsjoki V. Effect of specific colostral antibodies and selected lactobacilli on the adhesion of Helicobacter pylori on AGS cells and the Helicobacter-induced IL-8 production. Scand J Immunol. (2008) 68:280–6. doi: 10.1111/j.1365-3083.2008.02138.x
162. Kim J-E, Kim M-S, Yoon Y-S, Chung M-J, Yum D-Y. Use of selected lactic acid bacteria in the eradication of Helicobacter pylori infection. J Microbiol. (2014) 52:955–62. doi: 10.1007/s12275-014-4355-y
163. Tamura A, Kumai H, Nakamichi N, Sugiyama T, Deguchi R, Takagi A, et al. Suppression of Helicobacter pylori-induced interleukin-8 production in vitro and within the gastric mucosa by a live Lactobacillus strain. J Gastroenterol Hepatol. (2006) 21:1399–406. doi: 10.1111/j.1440-1746.2006.04318.x
164. Fleckenstein JM, Hardwidge PR, Munson GP, Rasko DA, Sommerfelt H, Steinsland H. Molecular mechanisms of enterotoxigenic Escherichia coli infection. Microbes Infect. (2010) 12:89–98. doi: 10.1016/j.micinf.2009.10.002
165. Fairbrother JM, Nadeau É, Gyles CL. Escherichia coli in postweaning diarrhea in pigs: an update on bacterial types, pathogenesis, and prevention strategies. Anim Health Res Rev. (2005) 6:17. doi: 10.1079/AHR2005105
166. Amdekar S, Singh V, Singh DD. Probiotic therapy: immunomodulating approach toward urinary tract infection. Curr Microbiol. (2011) 63:484. doi: 10.1007/s00284-011-0006-2
167. Sharma R, Kapila R, Dass G, Kapila S. Improvement in Th1/Th2 immune homeostasis, antioxidative status and resistance to pathogenic E. coli on consumption of probiotic Lactobacillus rhamnosus fermented milk in aging mice. Age. (2014) 36:1–17. doi: 10.1007/s11357-014-9686-4
168. Sharma R, Kapila R, Kapasiya M, Saliganti V, Dass G, Kapila S. Dietary supplementation of milk fermented with probiotic Lactobacillus fermentum enhances systemic immune response and antioxidant capacity in aging mice. Nutr Res. (2014) 34:968–81. doi: 10.1016/j.nutres.2014.09.006
169. Bhat MI, Sowmya K, Kapila S, Kapila R. Escherichia coli K12: an evolving opportunistic commensal gut microbe distorts barrier integrity in human intestinal cells. Microb Pathog. (2019) 133:103545. doi: 10.1016/j.micpath.2019.103545
170. Bhat MI, Sowmya K, Kapila S, Kapila R. Potential probiotic Lactobacillus rhamnosus (MTCC-5897) inhibits Escherichia coli impaired intestinal barrier function by modulating the host tight junction gene response. Probiotics Antimicrob Proteins. (2019) 12:1149–60. doi: 10.1007/s12602-019-09608-8
171. Ma GK, Brensinger CM, Wu Q, Lewis JD. Increasing incidence of multiply recurrent Clostridium difficile infection in the United States: a cohort study. Ann Intern Med. (2017) 167:152–8. doi: 10.7326/M16-2733
172. Sharma SK, Nakajima K, Shukla PJ. Clostridium difficile infection. N Engl J Med. (2015) 373:287. doi: 10.1056/NEJMc1506004
173. Hell M, Bernhofer C, Stalzer P, Kern J, Claassen E. Probiotics in Clostridium difficile infection: reviewing the need for a multistrain probiotic. Benef Microbes. (2013) 4:39–51. doi: 10.3920/BM2012.0049
174. Ollech JE, Shen NT, Crawford CV, Ringel Y. Use of probiotics in prevention and treatment of patients with Clostridium difficile infection. Best Prac Res Clin Gastroenterol. (2016) 30:111–8. doi: 10.1016/j.bpg.2016.01.002
175. Wu J, Ding Y, Wang J, Wang F. Staphylococcus aureus induces TGF-β1 and bFGF expression through the activation of AP-1 and NF-κB transcription factors in bovine mammary epithelial cells. Microb Pathog. (2018) 117:276–84. doi: 10.1016/j.micpath.2018.02.024
176. Paynich ML, Jones-Burrage SE, Knight KL. Exopolysaccharide from Bacillus subtilis induces anti-inflammatory M2 macrophages that prevent T cell–mediated disease. J Immunol. (2017) 198:2689–98. doi: 10.4049/jimmunol.1601641
177. Morgand M, Leclercq A, Maury M, Bracq-Dieye H, Thouvenot P, Vales G, et al. Listeria monocytogenes-associated respiratory infections: a study of 38 consecutive cases. Clin Microbiol Infect. (2018) 24:1339.e1–5. doi: 10.1016/j.cmi.2018.03.003
178. Jordan K, McAuliffe O. Listeria monocytogenes in foods. Adv Food Nutr Res. (2018) 86:181–213. doi: 10.1016/bs.afnr.2018.02.006
179. Ghosh P, Higgins DE. Listeria monocytogenes infection of the brain. J Visual Exp. (2018) 140:e58723. doi: 10.3791/58723
180. Djokic J, Popovic N, Brdaric E, Dinic M, Terzić-Vidojević A, Golic N, et al. The influence of heat-killed Enterococcus faecium BGPAS1-3 on the tight junction protein expression and immune function in differentiated Caco-2 cells infected with Listeria monocytogenes ATCC 19111. Front Microbiol. (2019) 10:412. doi: 10.3389/fmicb.2019.00412
181. Villena J, Shimosato T, Vizoso-Pinto MG, Kitazawa H. Nutrition, immunity and viral infections. Front Nutr. (2020) 7:125. doi: 10.3389/fnut.2020.00125
182. Saitoh T, Yamamoto M, Miyagishi M, Taira K, Nakanishi M, Fujita T, et al. A20 is a negative regulator of IFN regulatory factor 3 signaling. J Immunol. (2005) 174:1507–12. doi: 10.4049/jimmunol.174.3.1507
183. Ishizuka T, Kanmani P, Kobayashi H, Miyazaki A, Soma J, Suda Y, et al. Immunobiotic bifidobacteria strains modulate rotavirus immune response in porcine intestinal epitheliocytes via pattern recognition receptor signaling. PLoS ONE. (2016) 11:e152416. doi: 10.1371/journal.pone.0152416
184. Chattha KS, Vlasova AN, Kandasamy S, Rajashekara G, Saif LJ. Divergent immunomodulating effects of probiotics on T cell responses to oral attenuated human rotavirus vaccine and virulent human rotavirus infection in a neonatal gnotobiotic piglet disease model. J Immunol. (2013) 191:2446–56. doi: 10.4049/jimmunol.1300678
185. Gonzalez-Ochoa G, Flores-Mendoza LK, Icedo-Garcia R, Gomez-Flores R, Tamez-Guerra P. Modulation of rotavirus severe gastroenteritis by the combination of probiotics and prebiotics. Arch Microbiol. (2017) 199:953–61. doi: 10.1007/s00203-017-1400-3
186. Olaya Galán N, Ulloa Rubiano J, Velez Reyes F, Fernandez Duarte K, Salas Cardenas S, Gutierrez Fernandez M. In vitro antiviral activity of Lactobacillus casei and Bifidobacterium adolescentis against rotavirus infection monitored by NSP 4 protein production. J Appl Microbiol. (2016) 120:1041–51. doi: 10.1111/jam.13069
187. Zhang W, Azevedo MS, Gonzalez AM, Saif LJ, Van Nguyen T, Wen K, et al. Influence of probiotic Lactobacilli colonization on neonatal B cell responses in a gnotobiotic pig model of human rotavirus infection and disease. Vet Immunol Immunopathol. (2008) 122:175–81. doi: 10.1016/j.vetimm.2007.10.003
188. Yamamoto Y, Saruta J, Takahashi T, To M, Shimizu T, Hayashi T, et al. Effect of ingesting yogurt fermented with Lactobacillus delbrueckii ssp. bulgaricus OLL1073R-1 on influenza virus-bound salivary IgA in elderly residents of nursing homes: a randomized controlled trial. Acta Odontol Scand. (2019) 77:517–24. doi: 10.1080/00016357.2019.1609697
189. Belkacem N, Serafini N, Wheeler R, Derrien M, Boucinha L, Couesnon A, et al. Lactobacillus paracasei feeding improves immune control of influenza infection in mice. PLoS ONE. (2017) 12:e0184976. doi: 10.1371/journal.pone.0184976
190. Jung Y-J, Lee Y-T, Le Ngo V, Cho Y-H, Ko E-J, Hong S-M, et al. Heat-killed Lactobacillus casei confers broad protection against influenza A virus primary infection and develops heterosubtypic immunity against future secondary infection. Sci Rep. (2017) 7:1–12. doi: 10.1038/s41598-017-17487-8
191. Culley FJ. Natural killer cells in infection and inflammation of the lung. Immunology. (2009) 128:151–63. doi: 10.1111/j.1365-2567.2009.03167.x
192. Ritz BW, Nogusa S, Ackerman EA, Gardner EM. Supplementation with active hexose correlated compound increases the innate immune response of young mice to primary influenza infection. J Nutr. (2006) 136:2868–73. doi: 10.1093/jn/136.11.2868
193. Kikuchi Y, Kunitoh-Asari A, Hayakawa K, Imai S, Kasuya K, Abe K, et al. Oral administration of Lactobacillus plantarum strain AYA enhances IgA secretion and provides survival protection against influenza virus infection in mice. PLoS ONE. (2014) 9:e86416. doi: 10.1371/journal.pone.0086416
194. Kawahara T, Takahashi T, Oishi K, Tanaka H, Masuda M, Takahashi S, et al. Consecutive oral administration of Bifidobacterium longum MM-2 improves the defense system against influenza virus infection by enhancing natural killer cell activity in a murine model. Microbiol Immunol. (2015) 59:1–12. doi: 10.1111/1348-0421.12210
195. Namba K, Hatano M, Yaeshima T, Takase M, Suzuki K. Effects of Bifidobacterium longum BB536 administration on influenza infection, influenza vaccine antibody titer, and cell-mediated immunity in the elderly. Biosci Biotechnol Biochem. (2010) 74:939–45. doi: 10.1271/bbb.90749
196. Iwabuchi N, Xiao J-Z, Yaeshima T, Iwatsuki K. Oral administration of Bifidobacterium longum ameliorates influenza virus infection in mice. Biol Pharm Bull. (2011) 34:1352–5. doi: 10.1248/bpb.34.1352
197. Maeda N, Nakamura R, Hirose Y, Murosaki S, Yamamoto Y, Kase T, et al. Oral administration of heat-killed Lactobacillus plantarum L-137 enhances protection against influenza virus infection by stimulation of type I interferon production in mice. Int Immunopharmacol. (2009) 9:1122–5. doi: 10.1016/j.intimp.2009.04.015
198. Leyer GJ, Li S, Mubasher ME, Reifer C, Ouwehand AC. Probiotic effects on cold and influenza-like symptom incidence and duration in children. Pediatrics. (2009) 124:e172–9. doi: 10.1542/peds.2008-2666
199. Liu Y, Liu Q, Jiang Y, Yang W, Huang H, Shi C, et al. Surface-displayed porcine IFN-λ3 in Lactobacillus plantarum inhibits porcine enteric coronavirus infection of porcine intestinal epithelial cells. J Microbiol Biotechnol. (2019) 30:515–25. doi: 10.4014/jmb.1909.09041
200. Yang W-T, Li Q-Y, Ata EB, Jiang Y-L, Huang H-B, Shi C-W, et al. Immune response characterization of mice immunized with Lactobacillus plantarum expressing spike antigen of transmissible gastroenteritis virus. Appl Microbiol Biotechnol. (2018) 102:8307–18. doi: 10.1007/s00253-018-9238-4
201. Jiang X, Hou X, Tang L, Jiang Y, Ma G, Li Y. A phase trial of the oral Lactobacillus casei vaccine polarizes Th2 cell immunity against transmissible gastroenteritis coronavirus infection. Appl Microbiol Biotechnol. (2016) 100:7457–69. doi: 10.1007/s00253-016-7424-9
202. Seo BJ, Mun MR, Kim C-J, Lee I, Kim H, Park Y-H. Putative probiotic Lactobacillus spp. from porcine gastrointestinal tract inhibit transmissible gastroenteritis coronavirus and enteric bacterial pathogens. Trop Anim Health Prod. (2010) 42:1855–60. doi: 10.1007/s11250-010-9648-5
203. Sirichokchatchawan W, Temeeyasen G, Nilubol D, Prapasarakul N. Protective effects of cell-free supernatant and live lactic acid bacteria isolated from Thai pigs against a pandemic strain of porcine epidemic diarrhea virus. Probiotics Antimicrob Proteins. (2018) 10:383–90. doi: 10.1007/s12602-017-9281-y
204. Corano Scheri G, Fard SN, Schietroma I, Mastrangelo A, Pinacchio C, Giustini N, et al. Modulation of tryptophan/serotonin pathway by probiotic supplementation in human immunodeficiency virus–positive patients: preliminary results of a new study approach. Int J Tryptophan Res. (2017) 10:1178646917710668. doi: 10.1177/1178646917710668
205. Hummelen R, Changalucha J, Butamanya NL, Cook A, Habbema JDF, Reid G. Lactobacillus rhamnosus GR-1 and L. reuteri RC-14 to prevent or cure bacterial vaginosis among women with HIV. Int J Gynecol Obstetrics. (2010) 111:245–8. doi: 10.1016/j.ijgo.2010.07.008
206. Cunningham-Rundles S, Ahrné S, Johann-Liang R, Abuav R, Dunn-Navarra A-M, Grassey C, et al. Effect of probiotic bacteria on microbial host defense, growth, and immune function in human immunodeficiency virus type-1 infection. Nutrients. (2011) 3:1042–70. doi: 10.3390/nu3121042
207. Brun P, Scarpa M, Marchiori C, Sarasin G, Caputi V, Porzionato A, et al. Saccharomyces boulardii CNCM I-745 supplementation reduces gastrointestinal dysfunction in an animal model of IBS. PLoS ONE. (2017) 12:e0181863. doi: 10.1371/journal.pone.0181863
208. Palma E, Recine N, Domenici L, Giorgini M, Pierangeli A, Panici PB. Long-term Lactobacillus rhamnosus BMX 54 application to restore a balanced vaginal ecosystem: a promising solution against HPV-infection. BMC Infect Dis. (2018) 18:1–7. doi: 10.1186/s12879-017-2938-z
209. Abdolalipour E, Mahooti M, Salehzadeh A, Torabi A, Mohebbi SR, Gorji A, et al. Evaluation of the antitumor immune responses of probiotic Bifidobacterium bifidum in human papillomavirus-induced tumor model. Microb Pathog. (2020) 145:104207. doi: 10.1016/j.micpath.2020.104207
210. Ou Y-C, Fu H-C, Tseng C-W, Wu C-H, Tsai C-C, Lin H. The influence of probiotics on genital high-risk human papilloma virus clearance and quality of cervical smear: a randomized placebo-controlled trial. BMC Women's Health. (2019) 19:1–7. doi: 10.1186/s12905-019-0798-y
211. Khani S, Motamedifar M, Golmoghaddam H, Hosseini HM, Hashemizadeh Z. In vitro study of the effect of a probiotic bacterium Lactobacillus rhamnosus against herpes simplex virus type 1. Brazil J Infect Dis. (2012) 16:129–35. doi: 10.1016/S1413-8670(12)70293-3
212. Oo KM, AYELWIN A, Kyaw YY, Tun WM, Fukada K, Goshima A, et al. Safety and long-term effect of the probiotic FK-23 in patients with hepatitis C virus infection. Biosci Microbiota Food Health. (2016) 2015–24. doi: 10.12938/bmfh.2015-024
213. Lopez-Santamarina A, Lamas A, del Carmen Mondragón A, Cardelle-Cobas A, Regal P, Rodriguez-Avila JA, et al. Probiotic effects against virus infections: new weapons for an old war. Foods. (2021) 10:130. doi: 10.3390/foods10010130
214. Zhang H, Yeh C, Jin Z, Ding L, Liu BY, Zhang L, et al. Prospective study of probiotic supplementation results in immune stimulation and improvement of upper respiratory infection rate. Synth Syst Biotechnol. (2018) 3:113–20. doi: 10.1016/j.synbio.2018.03.001
215. Chong H-X, Yusoff NAA, Hor Y-Y, Lew L-C, Jaafar MH, Choi S-B, et al. Lactobacillus plantarum DR7 improved upper respiratory tract infections via enhancing immune and inflammatory parameters: a randomized, double-blind, placebo-controlled study. J Dairy Sci. (2019) 102:4783–97. doi: 10.3168/jds.2018-16103
216. Preidis GA, Saulnier DM, Blutt SE, Mistretta T-A, Riehle KP, Major AM, et al. Host response to probiotics determined by nutritional status of rotavirus-infected neonatal mice. J Pediatr Gastroenterol Nutr. (2012) 55:299. doi: 10.1097/MPG.0b013e31824d2548
217. Freedman SB, Xie J, Nettel-Aguirre A, Pang X-L, Chui L, Williamson-Urquhart S, et al. A randomized trial evaluating virus-specific effects of a combination probiotic in children with acute gastroenteritis. Nat Commun. (2020) 11:1–9. doi: 10.1038/s41467-020-16308-3
218. Pu F, Guo Y, Li M, Zhu H, Wang S, Shen X, et al. Yogurt supplemented with probiotics can protect the healthy elderly from respiratory infections: a randomized controlled open-label trial. Clin Interv Aging. (2017) 12:1223. doi: 10.2147/CIA.S141518
219. Mitchell PS, Patzina C, Emerman M, Haller O, Malik HS, Kochs G. Evolution-guided identification of antiviral specificity determinants in the broadly acting interferon-induced innate immunity factor MxA. Cell Host Microbe. (2012) 12:598–604. doi: 10.1016/j.chom.2012.09.005
220. Liang SL, Quirk D, Zhou A. RNase L: its biological roles and regulation. IUBMB Life. (2006) 58:508–14. doi: 10.1080/15216540600838232
221. Morelli M, Ogden KM, Patton JT. Silencing the alarms: innate immune antagonism by rotavirus NSP1 and VP3. Virology. (2015) 479:75–84. doi: 10.1016/j.virol.2015.01.006
222. Lee DK, Park JE, Kim MJ, Seo JG, Lee JH, Ha NJ. Probiotic bacteria, B. longum and Lacidophilus inhibit infection by rotavirus in vitro and decrease the duration of diarrhea in pediatric patients. Clin Res Hepatol Gastroenterol. (2015) 39:237–44. doi: 10.1016/j.clinre.2014.09.006
223. Maragkoudakis PA, Chingwaru W, Gradisnik L, Tsakalidou E, Cencic A. Lactic acid bacteria efficiently protect human and animal intestinal epithelial and immune cells from enteric virus infection. Int J Food Microbiol. (2010) 141:S91–7. doi: 10.1016/j.ijfoodmicro.2009.12.024
224. Riaz M, Alam S, Malik A, Ali SM. Efficacy and safety of Saccharomyces boulardii in acute childhood diarrhea: a double blind randomised controlled trial. Indian J Pediatr. (2012) 79:478–82. doi: 10.1007/s12098-011-0573-z
225. Rigo-Adrover M, Saldaña-Ruíz S, Van Limpt K, Knipping K, Garssen J, Knol J, et al. A combination of scGOS/lcFOS with Bifidobacterium breve M-16V protects suckling rats from rotavirus gastroenteritis. Eur J Nutr. (2017) 56:1657–70. doi: 10.1007/s00394-016-1213-1
226. Varyukhina S, Freitas M, Bardin S, Robillard E, Tavan E, Sapin C, et al. Glycan-modifying bacteria-derived soluble factors from Bacteroides thetaiotaomicron and Lactobacillus casei inhibit rotavirus infection in human intestinal cells. Microbes Infect. (2012) 14:273–8. doi: 10.1016/j.micinf.2011.10.007
227. Kandasamy S, Vlasova AN, Fischer D, Kumar A, Chattha KS, Rauf A, et al. Differential effects of Escherichia coli Nissle and Lactobacillus rhamnosus strain GG on human rotavirus binding, infection, and B cell immunity. J Immunol. (2016) 196:1780–9. doi: 10.4049/jimmunol.1501705
228. Ludert JE, Feng N, Yu JH, Broome RL, Hoshino Y, Greenberg HB. Genetic mapping indicates that VP4 is the rotavirus cell attachment protein in vitro and in vivo. J Virol. (1996) 70:487–93. doi: 10.1128/JVI.70.1.487-493.1996
229. Azevedo M, Zhang W, Wen K, Gonzalez A, Saif L, Yousef A, et al. Lactobacillus acidophilus and L. reuteri modulate cytokine responses in gnotobiotic pigs infected with human rotavirus. Benef Microbes. (2012) 3:33. doi: 10.3920/BM2011.0041
230. Lehtoranta L, Pitkäranta A, Korpela R. Probiotics in respiratory virus infections. Eur J Clin Microbiol Infect Dis. (2014) 33:1289–302. doi: 10.1007/s10096-014-2086-y
231. Takeda S, Takeshita M, Kikuchi Y, Dashnyam B, Kawahara S, Yoshida H, et al. Efficacy of oral administration of heat-killed probiotics from Mongolian dairy products against influenza infection in mice: alleviation of influenza infection by its immunomodulatory activity through intestinal immunity. Int Immunopharmacol. (2011) 11:1976–83. doi: 10.1016/j.intimp.2011.08.007
232. He F, Morita H, Kubota A, Ouwehand AC, Hosoda M, Hiramatsu M, et al. Effect of orally administered non-viable Lactobacillus cells on murine humoral immune responses. Microbiol Immunol. (2005) 49:993–7. doi: 10.1111/j.1348-0421.2005.tb03695.x
233. Inoue R, Nishio A, Fukushima Y, Ushida K. Oral treatment with probiotic Lactobacillus johnsonii NCC533 (La1) for a specific part of the weaning period prevents the development of atopic dermatitis induced after maturation in model mice, NC/Nga. Br J Dermatol. (2007) 156:499–509. doi: 10.1111/j.1365-2133.2006.07695.x
234. La Gruta NL, Kedzierska K, Stambas J, Doherty PC. A question of self-preservation: immunopathology in influenza virus infection. Immunol Cell Biol. (2007) 85:85–92. doi: 10.1038/sj.icb.7100026
235. Yitbarek A, Alkie T, Taha-Abdelaziz K, Astill J, Rodriguez-Lecompte J, Parkinson J, et al. Gut microbiota modulates type I interferon and antibody-mediated immune responses in chickens infected with influenza virus subtype H9N2. Benef Microbes. (2018) 9:417–27. doi: 10.3920/BM2017.0088
236. Barjesteh N, Shojadoost B, Brisbin JT, Emam M, Hodgins DC, Nagy É, et al. Reduction of avian influenza virus shedding by administration of Toll-like receptor ligands to chickens. Vaccine. (2015) 33:4843–9. doi: 10.1016/j.vaccine.2015.07.070
238. Morais AH, Passos TS, Maciel BL, da Silva-Maia JK. Can probiotics and diet promote beneficial immune modulation and purine control in coronavirus infection? Nutrients. (2020) 12:1737. doi: 10.3390/nu12061737
239. Olaimat AN, Aolymat I, Al-Holy M, Ayyash M, Ghoush MA, Al-Nabulsi AA, et al. The potential application of probiotics and prebiotics for the prevention and treatment of COVID-19. NPJ Sci Food. (2020) 4:1–7. doi: 10.1038/s41538-020-00078-9
240. Sohrabi C, Alsafi Z, O'Neill N, Khan M, Kerwan A, Al-Jabir A, et al. World Health Organization declares global emergency: a review of the 2019 novel coronavirus (COVID-19). Int J Surg. (2020) 76:71–6. doi: 10.1016/j.ijsu.2020.02.034
241. Zhang G, Li B, Yoo D, Qin T, Zhang X, Jia Y, et al. Animal coronaviruses and SARS-CoV-2. Transbound Emerg Dis. (2020) 1–14. doi: 10.1111/tbed.13791
242. Chen Y, Liu Q, Guo D. Emerging coronaviruses: genome structure, replication, and pathogenesis. J Med Virol. (2020) 92:418–23. doi: 10.1002/jmv.25681
243. Gohil K, Samson R, Dastager S, Dharne M. Probiotics in the prophylaxis of COVID-19: something is better than nothing. 3 Biotech. (2021) 11:1–10. doi: 10.1007/s13205-020-02554-1
244. Banerjee A, Kulcsar K, Misra V, Frieman M, Mossman K. Bats and coronaviruses. Viruses. (2019) 11:41. doi: 10.3390/v11010041
245. Maxmen A. Slew of trials launch to test coronavirus treatments in China. Nature. (2020) 347–8. doi: 10.1038/d41586-020-00444-3
246. Hoffmann M, Kleine-Weber H, Schroeder S, Krüger N, Herrler T, Erichsen S, et al. SARS-CoV-2 cell entry depends on ACE2 and TMPRSS2 and is blocked by a clinically proven protease inhibitor. Cell. (2020) 181:271–80. e278. doi: 10.1016/j.cell.2020.02.052
247. Zhang H, Kang Z, Gong H, Xu D, Wang J, Li Z, et al. The digestive system is a potential route of 2019-nCov infection: a bioinformatics analysis based on single-cell transcriptomes. bioRxiv. (2020). doi: 10.1101/2020.01.30.927806
248. Cascella M, Rajnik M, Cuomo A, Dulebohn SC, Di Napoli R. Features, Evaluation and Treatment Coronavirus (COVID-19). Statpearls (2020).
249. Pan L, Mu M, Yang P, Sun Y, Wang R, Yan J, et al. Clinical characteristics of COVID-19 patients with digestive symptoms in Hubei, China: a descriptive, cross-sectional, multicenter study. Am J Gastroenterol. (2020) 115:766–73. doi: 10.14309/ajg.0000000000000620
250. Grech V. Unknown unknowns–COVID-19 and potential global mortality. Early Hum Dev. (2020) 144:105026. doi: 10.1016/j.earlhumdev.2020.105026
251. Infusino F, Marazzato M, Mancone M, Fedele F, Mastroianni CM, Severino P, et al. Diet supplementation, probiotics, and nutraceuticals in SARS-CoV-2 infection: a scoping review. Nutrients. (2020) 12:1718. doi: 10.3390/nu12061718
252. Li G, De Clercq E. Therapeutic Options for the 2019 Novel Coronavirus (2019-nCoV). Nature Publishing Group (2020).
253. Chien M, Anderson TK, Jockusch S, Tao C, Li X, Kumar S, et al. Nucleotide analogues as inhibitors of SARS-CoV-2 polymerase, a key drug target for COVID-19. J Proteome Res. (2020) 19:4690–7. doi: 10.1021/acs.jproteome.0c00392
254. Ju J, Li X, Kumar S, Jockusch S, Chien M, Tao C, et al. Nucleotide analogues as inhibitors of SARS-CoV polymerase. Pharmacol Res Perspect. (2020) 8:e00674. doi: 10.1002/prp2.674
255. Kearney JE. Chloroquine as a potential treatment and prevention measure for the 2019 novel coronavirus: a review. Preprints. (2020) 2020030275. doi: 10.20944/preprints202003.0275.v1
256. Liu C, Zhou Q, Li Y, Garner LV, Watkins SP, Carter LJ, et al. Research and Development on Therapeutic Agents and Vaccines for COVID-19 and Related Human Coronavirus Diseases. ACS Publications (2020).
257. Monteil V, Kwon H, Prado P, Hagelkrüys A, Wimmer RA, Stahl M, et al. Inhibition of SARS-CoV-2 infections in engineered human tissues using clinical-grade soluble human ACE2. Cell. (2020) 181:905–13. e907. doi: 10.1016/j.cell.2020.04.004
258. Popov D. Treatment of Covid-19 infection. a rationale for current and future pharmacological approach. EC Pulmonol Respir Med. (2020) 9.4:38–58.
259. Zhang L, Liu Y. Potential interventions for novel coronavirus in China: a systematic review. J Med Virol. (2020) 92:479–90. doi: 10.1002/jmv.25707
260. Manna S, Chowdhury T, Chakraborty R, Mandal SM. Probiotics-derived peptides and their immunomodulatory molecules can play a preventive role against viral diseases including COVID-19. Probiotics Antimicrob Proteins. (2020) 1–13. doi: 10.1007/s12602-020-09727-7
261. Sundararaman A, Ray M, Ravindra P, Halami PM. Role of probiotics to combat viral infections with emphasis on COVID-19. Appl Microbiol Biotechnol. (2020) 104:8089–104. doi: 10.1007/s00253-020-10832-4
262. Di Pierro F. A possible probiotic (S. salivarius K12) approach to improve oral and lung microbiotas and raise defenses against SAR S-CoV-2. Minerva Med. (2020) 111:281–3. doi: 10.23736/S0026-4806.20.06570-2
263. Wang X, Wang L, Huang X, Ma S, Yu M, Shi W, et al. Oral delivery of probiotics expressing dendritic cell-targeting peptide fused with porcine epidemic diarrhea virus COE antigen: a promising vaccine strategy against PEDV. Viruses. (2017) 9:312. doi: 10.3390/v9110312
264. Åkerström S, Mousavi-Jazi M, Klingström J, Leijon M, Lundkvist Å, Mirazimi A. Nitric oxide inhibits the replication cycle of severe acute respiratory syndrome coronavirus. J Virol. (2005) 79:1966–9. doi: 10.1128/JVI.79.3.1966-1969.2005
265. Jung K, Gurnani A, Renukaradhya GJ, Saif LJ. Nitric oxide is elicited and inhibits viral replication in pigs infected with porcine respiratory coronavirus but not porcine reproductive and respiratory syndrome virus. Vet Immunol Immunopathol. (2010) 136:335–9. doi: 10.1016/j.vetimm.2010.03.022
266. Chai W, Burwinkel M, Wang Z, Palissa C, Esch B, Twardziok S, et al. Antiviral effects of a probiotic Enterococcus faecium strain against transmissible gastroenteritis coronavirus. Arch Virol. (2013) 158:799–807. doi: 10.1007/s00705-012-1543-0
267. Liu F, Li G, Wen K, Bui T, Cao D, Zhang Y, et al. Porcine small intestinal epithelial cell line (IPEC-J2) of rotavirus infection as a new model for the study of innate immune responses to rotaviruses and probiotics. Viral Immunol. (2010) 23:135–49. doi: 10.1089/vim.2009.0088
268. Park Yong HA, Seon LI, Kim Hong IK. Acid- and Bile-Tolerant Probiotic Enterococcus Faecium Probio-63 Which Can Suppress Growth Of Corona Virus and Porcine Circovirus Type 2 and Pharmaceutical or Food Composition Containing the Same. Republic of Korea KR100468522B (2004).
269. Park Yong HA, Seon LI, Kim Hong IK. Acid Tolerant Probiotic Enterococcus Faecalis Probio-056 That Can Suppresses the Growth of Pathogenic Microorganisms and Ped Coronavirus to Stabilize the Intestinal Microflora, and to Treat or Prevent Symptoms Resulted From Abnormal Fermentation of Pathogenic Microorganisms. Republic of Korea KR20050023475 (2003).
270. Liu Q, Jiang Y, Yang W, Liu Y, Shi C, Liu J, et al. Protective effects of a food-grade recombinant Lactobacillus plantarum with surface displayed AMA1 and EtMIC2 proteins of Eimeria tenella in broiler chickens. Microb Cell Fact. (2020) 19:28. doi: 10.1186/s12934-020-1297-4
271. Awais MM, Jamal MA, Akhtar M, Hameed MR, Anwar MI, Ullah MI. Immunomodulatory and ameliorative effects of Lactobacillus and Saccharomyces based probiotics on pathological effects of eimeriasis in broilers. Microb Pathog. (2019) 126:101–8. doi: 10.1016/j.micpath.2018.10.038
272. Pender C, Kim S, Potter T, Ritzi M, Young M, Dalloul R. Effects of in ovo supplementation of probiotics on performance and immunocompetence of broiler chicks to an Eimeria challenge. Benef Microbes. (2016) 7:699–705. doi: 10.3920/BM2016.0080
273. Al-alousi TI, Abdullah AH, Jasim MM. Prophylactic role of lactic acid prepared from Lactobacillus acidophilus on cryptosporidiosis. Tikrit J Pure Sci. (2018) 23:38–42. Available online at: http://dx.doi.oc5130/tjps.23.2018.127
274. Solano-Aguilar G, Shea-Donohue T, Madden KB, Quinoñes A, Beshah E, Lakshman S, et al. Bifidobacterium animalis subspecies lactis modulates the local immune response and glucose uptake in the small intestine of juvenile pigs infected with the parasitic nematode Ascaris suum. Gut Microbes. (2018) 9:422–36. doi: 10.1080/19490976.2018.1460014
275. Jang S, Lakshman S, Molokin A, Urban JF Jr, Davis CD, Solano-Aguilar G. Lactobacillus rhamnosus and flavanol-enriched cocoa powder altered the immune response to infection with the parasitic nematode Ascaris suum in a pig model. FASEB J. (2016) 30(1_Suppl):1176.1114.
276. Thomas DJ, Husmann RJ, Villamar M, Winship TR, Buck RH, Zuckermann FA. Lactobacillus rhamnosus HN001 attenuates allergy development in a pig model. PLoS ONE. (2011) 6:e16577. doi: 10.1371/journal.pone
277. Goyal N, Shukla G. Probiotic Lactobacillus rhamnosus GG modulates the mucosal immune response in Giardia intestinalis-infected BALB/c mice. Dig Dis Sci. (2013) 58:1218–25. doi: 10.1007/s10620-012-2503-y
278. Benyacoub J, Perez P, Rochat F, Saudan K, Reuteler G, Antille N, et al. Enterococcus faecium SF68 enhances the immune response to Giardia intestinalis in mice. J Nutr. (2005) 135:1171–6. doi: 10.1093/jn/135.5.1171
279. Ghanem KZ, Abdel-Salam AM, Magharby AS. Immunoprophylactic effect of probiotic yoghurt feeding on Schistosoma mansoni-infected mice. Pol J Food Nutr Sci. (2005) 14–55:123–6.
280. Zowail ME, Osman GY, Mohamed AH, El-Esawy HMI. Protective role of Lactobacillus sporogenes (probiotic) on chromosomal aberrations and DNA fragmentation in Schistosoma mansoni infected mice. Egypt J Exp Biol. (2012) 8:121–30.
281. El Temsahy MM, Ibrahim IR, Mossallam SF, Mahrous H, Bary AA, Salam SAA. Evaluation of newly isolated probiotics in the protection against experimental intestinal trichinellosis. Vet Parasitol. (2015) 214:303–14. doi: 10.1016/j.vetpar.2015.08.029
282. DvoroŽnáková E, Bucková B, Hurníková Z, Revajová V, Lauková A. Effect of probiotic bacteria on phagocytosis and respiratory burst activity of blood polymorphonuclear leukocytes (PMNL) in mice infected with Trichinella spiralis. Vet Parasitol. (2016) 231:69–76. doi: 10.1016/j.vetpar.2016.07.004
283. Martínez-Gómez F, Fuentes-Castro BE, Bautista-Garfias CR. The intraperitoneal inoculation of Lactobacillus casei in mice induces total protection against Trichinella spiralis infection at low challenge doses. Parasitol Res. (2011) 109:1609–17. doi: 10.1007/s00436-011-2432-2
284. McClemens J, Kim JJ, Wang H, Mao Y-K, Collins M, Kunze W, et al. Lactobacillus rhamnosus ingestion promotes innate host defense in an enteric parasitic infection. Clin Vaccine Immunol. (2013) 20:818–26. doi: 10.1128/CVI.00047-13
285. de Avila LFDC, De Leon PMM, De Moura MQ, Berne MEA, Scaini CJ, Leivas Leite FP, et al. Modulation of IL-12 and IFN γ by probiotic supplementation promotes protection against Toxocara canis infection in mice. Parasite Immunol. (2016) 38:326–30. doi: 10.1111/pim.12314
286. Humen MA, De Antoni GL, Benyacoub J, Costas ME, Cardozo MI, Kozubsky L, et al. Lactobacillus johnsonii La1 antagonizes Giardia intestinalis in vivo. Infect Immun. (2005) 73:1265–9. doi: 10.1128/IAI.73.2.1265-1269.2005
287. Del Coco VF, Sparo MD, Sidoti A, Santín M, Basualdo JA, Córdoba MA. Effects of Enterococcus faecalis CECT 7121 on Cryptosporidium parvum infection in mice. Parasitol Res. (2016) 115:3239–44. doi: 10.1007/s00436-016-5087-1
288. Khalifa EA. Probiotics as a promising treatment of experimental cryptosporidiosis in an immuno suppressed mouse model. Int J Curr Microbiol App Sci. (2016) 5:97–106. doi: 10.20546/ijcmas.2016.503.014
289. Mohammed S, Jabur K, Aja H. Effect of Yogurt and bifidobacterium on Cryptosporidium parvum infection in experiential infected mice. Ibn AL Haitham J Pure Appl Sci. (2017) 24:40–8.
290. Oliveira BC, Widmer G. Probiotic product enhances susceptibility of mice to cryptosporidiosis. Appl Environ Microbiol. (2018) 84:e01408–18. doi: 10.1128/AEM.01408-18
291. Santos JdFM, Vasconcelos J, Souza JRd, Coutinho EdM, Montenegro SML, Azevedo-Ximenes E. The effect of Zymomonas mobilis culture on experimental Schistosoma mansoni infection. Rev Soc Bras Med Trop. (2004) 37:502–4. doi: 10.1590/S0037-86822004000600015
292. Martínez-Gómez F, Santiago-Rosales R, Bautista-Garfias CR. Effect of Lactobacillus casei Shirota strain intraperitoneal administration in CD1 mice on the establishment of Trichinella spiralis adult worms and on IgA anti-T. spiralis production. Vet Parasitol. (2009) 162:171–5. doi: 10.1016/j.vetpar.2009.02.010
293. Chiodo PG, Sparo MD, Pezzani BC, Minvielle MC, Basualdo JA. In vitro and in vivo effects of Enterococcus faecalis CECT7121 on Toxocara canis. Memórias Inst Oswaldo Cruz. (2010) 105:615–20. doi: 10.1590/S0074-02762010000500003
294. Dea-Ayuela MA, Rama-Iñiguez S, Bolás-Fernandez F. Enhanced susceptibility to Trichuris muris infection of B10Br mice treated with the probiotic Lactobacillus casei. Int Immunopharmacol. (2008) 8:28–35. doi: 10.1016/j.intimp.2007.10.003
295. Ansari F, Pourjafar H, Tabrizi A, Homayouni A. The effects of probiotics and prebiotics on mental disorders: a review on depression, anxiety, Alzheimer, and autism spectrum disorders. Curr Pharm Biotechnol. (2020) 21:555–65. doi: 10.2174/1389201021666200107113812
296. Kong X-J, Liu J, Li J, Kwong K, Koh M, Sukijthamapan P, et al. Probiotics and oxytocin nasal spray as neuro-social-behavioral interventions for patients with autism spectrum disorders: a pilot randomized controlled trial protocol. Pilot Feasibil Stud. (2020) 6:20. doi: 10.1186/s40814-020-0557-8
297. Wieërs G, Belkhir L, Enaud R, Leclercq S, Philippart de Foy J-M, Dequenne I, et al. How probiotics affect the microbiota. Front Cell Infect Microbiol. (2020) 9:454. doi: 10.3389/fcimb.2019.00454
298. Liu QF, Kim H-M, Lim S, Chung M-J, Lim C-Y, Koo B-S, et al. Effect of probiotic administration on gut microbiota and depressive behaviors in mice. DARU J Pharm Sci. (2020) 28:181–9. doi: 10.1007/s40199-020-00329-w
299. Zhu S, Jiang Y, Xu K, Cui M, Ye W, Zhao G, et al. The progress of gut microbiome research related to brain disorders. J Neuroinflamm. (2020) 17:25. doi: 10.1186/s12974-020-1705-z
300. Bravo JA, Forsythe P, Chew MV, Escaravage E, Savignac HM, Dinan TG, et al. Ingestion of Lactobacillus strain regulates emotional behavior and central GABA receptor expression in a mouse via the vagus nerve. Proc Natl Acad Sci USA. (2011) 108:16050–5. doi: 10.1073/pnas.1102999108
301. Desbonnet L, Garrett L, Clarke G, Bienenstock J, Dinan TG. The probiotic Bifidobacteria infantis: an assessment of potential antidepressant properties in the rat. J Psychiatr Res. (2008) 43:164–74. doi: 10.1016/j.jpsychires.2008.03.009
302. Desbonnet L, Garrett L, Clarke G, Kiely B, Cryan JF, Dinan TG. Effects of the probiotic Bifidobacterium infantis in the maternal separation model of depression. Neuroscience. (2010) 170:1179–88. doi: 10.1016/j.neuroscience.2010.08.005
303. Li N, Wang Q, Wang Y, Sun A, Lin Y, Jin Y, et al. Oral probiotics ameliorate the behavioral deficits induced by chronic mild stress in mice via the gut microbiota-inflammation axis. Front Behav Neurosci. (2018) 12:266. doi: 10.3389/fnbeh.2018.00266
304. Saliganti V, Kapila R, Sharma R, Kapila S. Feeding probiotic Lactobacillus rhamnosus (MTCC 5897) fermented milk to suckling mothers alleviates ovalbumin-induced allergic sensitisation in mice offspring. Br J Nutr. (2015) 114:1168–79. doi: 10.1017/S000711451500286X
305. George Kerry R, Patra JK, Gouda S, Park Y, Shin HS, Das G. Benefaction of probiotics for human health: a review. J Food Drug Anal. (2018) 26:927–39. doi: 10.1016/j.jfda.2018.01.002
306. Liu M-Y, Yang Z-Y, Dai W-K, Huang J-Q, Li Y-H, Zhang J, et al. Protective effect of Bifidobacterium infantis CGMCC313-2 on ovalbumin-induced airway asthma and β-lactoglobulin-induced intestinal food allergy mouse models. World J Gastroenterol. (2017) 23:2149. doi: 10.3748/wjg.v23.i12.2149
307. Zhang B, An J, Shimada T, Liu S, Maeyama K. Oral administration of Enterococcus faecalis FK-23 suppresses Th17 cell development and attenuates allergic airway responses in mice. Int J Mol Med. (2012) 30:248–54. doi: 10.3892/ijmm.2012.1010
308. Kim TS, Song J, Lim HX, Lee A, Lee J-H. Staphylococcus succinus 14BME20 prevents allergic airway inflammation by induction of regulatory T cells via interleukin-10. Front Immunol. (2019) 10:1269. doi: 10.3389/fimmu.2019.01269
309. Zhang J, Su H, Li Q, Wu H, Liu M, Huang J, et al. Oral administration of Clostridium butyricum CGMCC0313-1 inhibits β-lactoglobulin-induced intestinal anaphylaxis in a mouse model of food allergy. Gut Pathog. (2017) 9:11. doi: 10.1186/s13099-017-0160-6
310. Li A-l, Meng X-c, Duan C-c, Huo G-c, Zheng Q-l, Li D. Suppressive effects of oral administration of heat-killed Lactobacillus acidophilus on Th17 immune responses in a bovine β-lactoglobulin-sensitized mice model. Biol Pharm Bull. (2012) 36:202–7. doi: 10.1248/bpb.b12-00437
311. Yadav R, Khan SH, Mada SB, Meena S, Kapila R, Kapila S. Consumption of probiotic Lactobacillus fermentum MTCC: 5898-fermented milk attenuates dyslipidemia, oxidative stress, and inflammation in male rats fed on cholesterol-enriched diet. Probiotics Antimicrob Proteins. (2019) 11:509–18. doi: 10.1007/s12602-018-9429-4
312. Yadav R, Vij R, Kapila S, Khan SH, Kumar N, Meena S, et al. Milk fermented with probiotic strains Lactobacillus rhamnosus MTCC: 5957 and Lactobacillus rhamnosus MTCC: 5897 ameliorates the diet-induced hypercholesterolemia in rats. Ann Microbiol. (2019) 69:483–94. doi: 10.1007/s13213-018-1433-0
313. Lewis-Mikhael A-M, Davoodvandi A, Jafarnejad S. Effect of Lactobacillus plantarum containing probiotics on blood pressure: a systematic review and meta-analysis. Pharmacol Res. (2020) 153:104663. doi: 10.1016/j.phrs.2020.104663
314. Liu DM, Guo J, Zeng XA, Sun DW, Brennan CS, Zhou QX, et al. The probiotic role of Lactobacillus plantarum in reducing risks associated with cardiovascular disease. Int J Food Sci Technol. (2017) 52:127–36. doi: 10.1111/ijfs.13234
315. Robles-Vera I, Toral M, de la Visitación N, Sánchez M, Gómez-Guzmán M, Romero M, et al. Probiotics prevent dysbiosis and the raise in blood pressure in genetic hypertension: role of short-chain fatty acids. Mol Nutr Food Res. (2020) 64:e1900616. doi: 10.1002/mnfr.201900616
316. Gan XT, Ettinger G, Huang CX, Burton JP, Haist JV, Rajapurohitam V, et al. Probiotic administration attenuates myocardial hypertrophy and heart failure after myocardial infarction in the rat. Circulation Heart Failure. (2014) 7:491–9. doi: 10.1161/CIRCHEARTFAILURE.113.000978
317. Lam V, Su J, Koprowski S, Hsu A, Tweddell JS, Rafiee P, et al. Intestinal microbiota determine severity of myocardial infarction in rats. FASEB J. (2012) 26:1727–35. doi: 10.1096/fj.11-197921
318. Yadav R, Dey DK, Vij R, Meena S, Kapila R, Kapila S. Evaluation of anti-diabetic attributes of Lactobacillus rhamnosus MTCC: 5957, Lactobacillus rhamnosus MTCC: 5897 and Lactobacillus fermentum MTCC: 5898 in streptozotocin induced diabetic rats. Microb Pathog. (2018) 125:454–62. doi: 10.1016/j.micpath.2018.10.015
319. Wang Y, Dilidaxi D, Wu Y, Sailike J, Sun X, Nabi X-h. Composite probiotics alleviate type 2 diabetes by regulating intestinal microbiota and inducing GLP-1 secretion in db/db mice. Biomed Pharmacother. (2020) 125:109914. doi: 10.1016/j.biopha.2020.109914
320. Aponte M, Murru N, Shoukat M. Therapeutic, prophylactic, and functional use of probiotics: a current perspective. Front Microbiol. (2020) 11:2120. doi: 10.3389/fmicb.2020.562048
321. Majewska K, Kregielska-Narozna M, Jakubowski H, Szulińska M, Bogdański P. The multispecies probiotic effectively reduces homocysteine concentration in obese women: a randomized double-blind placebo-controlled study. J Clin Med. (2020) 9:998. doi: 10.3390/jcm9040998
322. Sanders ME, Guarner F, Guerrant R, Holt PR, Quigley EM, Sartor RB, et al. An update on the use and investigation of probiotics in health and disease. Gut. (2013) 62:787–96. doi: 10.1136/gutjnl-2012-302504
323. Pei R, Martin DA, DiMarco DM, Bolling BW. Evidence for the effects of yogurt on gut health and obesity. Crit Rev Food Sci Nutr. (2017) 57:1569–83. doi: 10.1080/10408398.2014.883356
324. Gérard P. Gut microbiota and obesity. Cell Mol Life Sci. (2016) 73:147–62. doi: 10.1007/s00018-015-2061-5
325. Serban DE. Gastrointestinal cancers: influence of gut microbiota, probiotics and prebiotics. Cancer Lett. (2014) 345:258–70. doi: 10.1016/j.canlet.2013.08.013
326. Kumar M, Nagpal R, Verma V, Kumar A, Kaur N, Hemalatha R, et al. Probiotic metabolites as epigenetic targets in the prevention of colon cancer. Nutr Rev. (2013) 71:23–34. doi: 10.1111/j.1753-4887.2012.00542.x
327. Vipperla K, O'Keefe SJ. The microbiota and its metabolites in colonic mucosal health and cancer risk. Nutr Clin Prac. (2012) 27:624–35. doi: 10.1177/0884533612452012
328. Tedelind S, Westberg F, Kjerrulf M, Vidal A. Anti-inflammatory properties of the short-chain fatty acids acetate and propionate: a study with relevance to inflammatory bowel disease. World J Gastroenterol. (2007) 13:2826. doi: 10.3748/wjg.v13.i20.2826
329. Urbanska AM, Paul A, Bhahena J, Prakash S. Suppression of tumorigenesis: modulation of inflammatory cytokines by oral administration of microencapsulated probiotic yogurt formulation. Int J Inflam. (2010) 2011:891015. doi: 10.4061/2010/894972
330. Zhu Q, Gao R, Wu W, Qin H. The role of gut microbiota in the pathogenesis of colorectal cancer. Tumor Biol. (2013) 34:1285–300. doi: 10.1007/s13277-013-0684-4
331. dos Reis SA, da Conceição LL, Siqueira NP, Rosa DD, da Silva LL, Maria do Carmo GP. Review of the mechanisms of probiotic actions in the prevention of colorectal cancer. Nutr Res. (2017) 37:1–19. doi: 10.1016/j.nutres.2016.11.009
332. Pala V, Sieri S, Berrino F, Vineis P, Sacerdote C, Palli D, et al. Yogurt consumption and risk of colorectal cancer in the Italian European prospective investigation into cancer and nutrition cohort. Int J Cancer. (2011) 129:2712–9. doi: 10.1002/ijc.26193
333. Saliganti V, Kapila R, Kapila S. Consumption of probiotic Lactobacillus rhamnosus (MTCC: 5897) containing fermented milk plays a key role in development of the immune system in newborn mice during the suckling–weaning transition. Microbiol Immunol. (2016) 60:261–7. doi: 10.1111/1348-0421.12342
334. Nomura A, Matsubara A, Goto S, Takahata J, Sawada K, Ihara K, et al. Relationship between gut microbiota composition and sensitization to inhaled allergens. Allergol Int. (2020) 69:437–42. doi: 10.1016/j.alit.2019.12.010
335. Yan F, Li N, Yue Y, Wang C, Zhao L, Evivie SE, et al. Screening for potential novel probiotics with dipeptidyl peptidase IV-inhibiting activity for type 2 diabetes attenuation in vitro and in vivo. Front Microbiol. (2020) 10:2855. doi: 10.3389/fmicb.2019.02855
336. Zhang Z, Liang X, Lv Y, Yi H, Chen Y, Bai L, et al. Evaluation of probiotics for improving and regulation metabolism relevant to type 2 diabetes in vitro. J Funct Foods. (2020) 64:103664. doi: 10.1016/j.jff.2019.103664
337. Lee Y-S, Jun H-S. Anti-diabetic actions of glucagon-like peptide-1 on pancreatic beta-cells. Metab Clin Exp. (2014) 63:9–19. doi: 10.1016/j.metabol.2013.09.010
338. Timper K, Dalmas E, Dror E, Rütti S, Thienel C, Sauter NS, et al. Glucose-dependent insulinotropic peptide stimulates glucagon-like peptide 1 production by pancreatic islets via interleukin 6, produced by α cells. Gastroenterology. (2016) 151:165–79. doi: 10.1053/j.gastro.2016.03.003
339. Wang L, Li P, Tang Z, Yan X, Feng B. Structural modulation of the gut microbiota and the relationship with body weight: compared evaluation of liraglutide and saxagliptin treatment. Sci Rep. (2016) 6:33251. doi: 10.1038/srep33251
340. Tiderencel KA, Hutcheon DA, Ziegler J. Probiotics for the treatment of type 2 diabetes: a review of randomized controlled trials. Diabetes Metab Res Rev. (2020) 36:e3213. doi: 10.1002/dmrr.3213
341. Abasi S, Keshtmand Z. The effect of probiotic bifidobacterium lactis and Lactobacillus casei on sperm maturation in streptozotocin-diabetic rats. ISMJ. (2020) 22:392–401. doi: 10.29252/ismj.22.6.392
342. Dardmeh F, Alipour H, Gazerani P, van der Horst G, Brandsborg E, Nielsen HI. Lactobacillus rhamnosus PB01 (DSM 14870) supplementation affects markers of sperm kinematic parameters in a diet-induced obesity mice model. PLoS ONE. (2017) 12:e185964. doi: 10.1371/journal.pone.0185964
343. Vílchez MC, Santangeli S, Maradonna F, Gioacchini G, Verdenelli C, Gallego V, et al. Effect of the probiotic Lactobacillus rhamnosus on the expression of genes involved in European eel spermatogenesis. Theriogenology. (2015) 84:1321–31. doi: 10.1016/j.theriogenology.2015.07.011
344. Inatomi T, Otomaru K. Effect of dietary probiotics on the semen traits and antioxidative activity of male broiler breeders. Sci Rep. (2018) 8:1–6. doi: 10.1038/s41598-018-24345-8
345. Jennifer B. Probiotics and cardiovascular disease (dissertation/master's thesis), Nevada, ND: University of North Dakota (2017).
346. Thushara RM, Gangadaran S, Solati Z, Moghadasian MH. Cardiovascular benefits of probiotics: a review of experimental and clinical studies. Food Funct. (2016) 7:632–42. doi: 10.1039/C5FO01190F
347. Tomaro-Duchesneau C, Jones ML, Shah D, Jain P, Saha S, Prakash S. Cholesterol assimilation by Lactobacillus probiotic bacteria: an in vitro investigation. Biomed Res Int. (2014) 2014:380316. doi: 10.1155/2014/380316
348. Vasquez EC, Pereira T, Peotta VA, Baldo MP, Campos-Toimil M. Probiotics as beneficial dietary supplements to prevent and treat cardiovascular diseases: uncovering their impact on oxidative stress. Oxid Med Cell Longev. (2019) 2019:3086270. doi: 10.1155/2019/3086270
349. March DS, Jones AW, Bishop NC, Burton JO. The efficacy of prebiotic, probiotic, and synbiotic supplementation in modulating gut-derived circulatory particles associated with cardiovascular disease in individuals receiving dialysis: a systematic review and meta-analysis of randomized controlled trials. J Renal Nutr. (2019) 30:347–59. doi: 10.1053/j.jrn.2019.07.006
Keywords: antibiotic resistant bacteria, antibiotics alternative, probiotics, pathogenic infections, immunomodulating
Citation: Raheem A, Liang L, Zhang G and Cui S (2021) Modulatory Effects of Probiotics During Pathogenic Infections With Emphasis on Immune Regulation. Front. Immunol. 12:616713. doi: 10.3389/fimmu.2021.616713
Received: 13 October 2020; Accepted: 12 February 2021;
Published: 08 April 2021.
Edited by:
Takahiro Masuda, Kyushu University, JapanReviewed by:
Kuldeep Dhama, Indian Veterinary Research Institute (IVRI), IndiaCopyright © 2021 Raheem, Liang, Zhang and Cui. This is an open-access article distributed under the terms of the Creative Commons Attribution License (CC BY). The use, distribution or reproduction in other forums is permitted, provided the original author(s) and the copyright owner(s) are credited and that the original publication in this journal is cited, in accordance with accepted academic practice. No use, distribution or reproduction is permitted which does not comply with these terms.
*Correspondence: Shangjin Cui, Y3Vpc2hhbmdqaW5AY2Fhcy5jbg==; Guangzhi Zhang, WmhhbmdndWFuZ3poaUBjYWFzLmNu
Disclaimer: All claims expressed in this article are solely those of the authors and do not necessarily represent those of their affiliated organizations, or those of the publisher, the editors and the reviewers. Any product that may be evaluated in this article or claim that may be made by its manufacturer is not guaranteed or endorsed by the publisher.
Research integrity at Frontiers
Learn more about the work of our research integrity team to safeguard the quality of each article we publish.