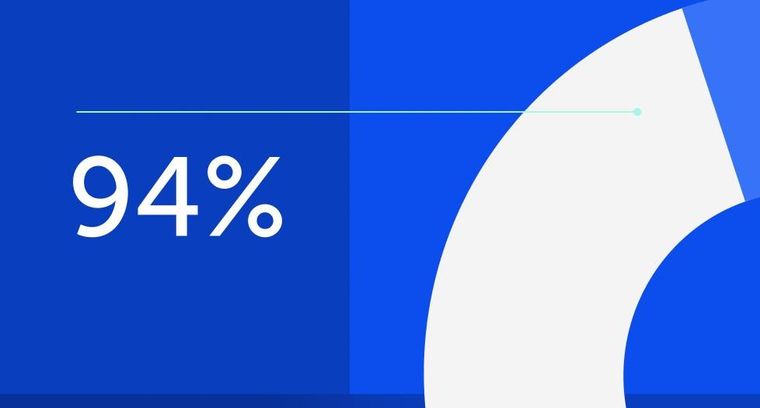
94% of researchers rate our articles as excellent or good
Learn more about the work of our research integrity team to safeguard the quality of each article we publish.
Find out more
MINI REVIEW article
Front. Immunol., 23 February 2021
Sec. Comparative Immunology
Volume 12 - 2021 | https://doi.org/10.3389/fimmu.2021.613729
This article is part of the Research TopicNovel Insights into Insect Antiviral ImmunityView all 16 articles
Viral infection triggers insect immune response, including RNA interference, apoptosis and autophagy, and profoundly changes the gene expression profiles in infected cells. Although intracellular degradation is crucial for restricting viral infection, intercellular communication is required to mount a robust systemic immune response. This review focuses on recent advances in understanding the intercellular communications in insect antiviral immunity, including protein-based and virus-derived RNA based cell-cell communications, with emphasis on the signaling pathway that induces the production of the potential cytokines. The prospects and challenges of future work are also discussed.
Viral infection has posed a significant threat to human and animal health, agricultural production and environmental safety. The frequent outbreaks of pandemics caused by viral infection taught us bitter lessons that the long-standing battles between the hosts and viruses are much rougher than expected. As obligate intracellular pathogens, viruses heavily rely on the host cell machinery and resources to replicate and propagate. Accordingly, host cells develop multiple strategies, including intrinsic antiviral response that directly restricts viral replication and assembly, and induced antiviral response that potentiates the antiviral activity of viral-restricting factors or cells to suppress and eliminate the invading pathogens (1–4).
Insects are the most abundant and diverse group of animals in the world. Some of them are regarded as model organisms, disease vectors, agriculture and household pests or industrial animals. A lot of studies have been carried out to investigate molecules, pathways and mechanisms that are involved in the immune response of different insects upon viral challenges. Among them, a few attentions are given to how extracellular signaling networks cooperate with intracellular pathways to mount a robust systemic immune response. Pieces of evidence have proposed that intricate intercellular communications occur in response to viral infection in insects, and helped us better understand the insect antiviral immunity in a systematic way.
The best characterized antiviral immune response in insects is RNA interference (RNAi) (3, 5). Three RNAi pathways have been identified in insects, including the small interfering RNA (siRNA) pathway, the microRNA (miRNA) pathway and the (PIWI-interacting RNA) piRNA pathway. Among them, siRNA has been most intensively studied as a potent antiviral defense strategy. siRNA is initiated by recognition and cleavage of double-stranded RNA (dsRNA) produced either as viral replication intermediate or as base-pairing viral transcript by Dicer-2 in host cells. Dicer-2, an RNase III family endonuclease, processes dsRNA into 19-23-nucleotide (nt) long siRNA duplex, which is subsequently loaded onto Argonaute-2 (Ago-2) endonuclease and integrated into a multiple protein complex, RNA-induced silencing complex (RISC). siRNA duplex is then unwound to generate the guide strand, which targets viral mRNA or genomic RNA containing complementary sequence for degradation through the RNase activity of Ago-2, thereby restricting viral infection. miRNA pathway was previously charactered in post-transcriptional regulation of gene expression during development, in which a 22-nt duplex miRNA processed by RNase III enzyme Drosha and Dicer-1 sequentially forms miRNA programmed RNA induced silencing complex (miRISC) with Ago-1 protein. Recently, both virus derived miRNAs that regulate insect gene expression and insect-encoded miRNAs that target virus mRNA were reported, highlighting its role in host-virus interaction (6, 7). The antiviral role of piRNA which commonly involves in genomic control of transposable elements is controversial in Drosophila (8, 9), while in mosquito piRNAs that are derived from acquired viral cDNA with the characteristic size range of 24–30 nt and features of ping-pong amplification cycle were discovered to specifically inhibit viral replication (10, 11).
Besides RNAi, viral-induced apoptosis and autophagy also play important roles in restricting viral infection (12, 13). The expression level of several pro-apoptotic genes, such as reaper, hid, and p53, increased in response to virus-induced stress, while anti-apoptotic genes, such as diap1 decreased, resulting in onset of apoptosis and subsequent phagocytosis of viral-infected cells by haemocytes (14–17). Interestingly, sometimes this antiviral apoptosis is suppressed by host protein, as evidence found in silkworm that peptidoglycan recognition protein (PGRP) 2-2, inhibited baculovirus-induced apoptosis via Akt activation, reflecting arms race between insect and virus (18, 19). Recently, a few studies found autophagy occurs after Drosophila infected with vesicular stomatitis virus (VSV), Rift Valley fever virus (RVFV) or Zika virus as evidenced by the elevation of lipidated Atg8 (Atg8-II) level and accumulation of Atg8 in autophagic punctae (20–24). Silencing core autophagy genes, such as atg5 or atg8, led to significant increase of viral load. Plasma membrane receptor Toll-7 has also been demonstrated to activate autophagy upon sensing VSV glycoproteins or RVFV (24, 25), which is independent of transcription factor NF-κB, whereas eliminating Zika virus by autophagy in Drosophila appears to be NF-κB-dependent (23).
In addition, genome-wide RNAi screening and transcriptional profiling has revealed a plethora of genes involved in antiviral immune response. Some of them have broad antiviral activity. For instance, negative elongation factor (NELF) and positive elongation factor b (P-TEFb) collaboratively mediate transcriptional pausing to potentiate the rapid activation of some inducible genes and are required to restrict viral replication in adult flies and mosquito cells (26). Some have been reported to be involved in anti-microbial immunity with uncharacterized antiviral activity. For instance, two anti-microbial peptide (AMP) coding genes, diptericinB and attacinC were up-regulated in transgenic flies expressing a Sindbis virus (SINV) replicon (27). Knocking-down their expression led to a modest but significant increase in SINV load, confirming their antiviral functions. In mosquito cells, Dengue virus (DENV) infection up-regulated the expression of a cecropin-like peptide which does not only have anti-bacterial activity, but also have anti-DENV and anti-Chikungunya virus activity (28). The enhanced expression of gloverin, lebocin, attacin was also observed in silkworm larvae infected with Bombyx mori nucleopolyhedrovirus (BmNPV) (29). Based on these facts, the Toll and IMD pathways, which are the two canonical NF-κB pathways responsible for immune response against bacterial and fungi infection, are considered to be implicated in anti-viral immunity (30, 31). But most of viral-induced genes remain enigmas in terms of the molecular mechanism underling their antiviral activity. For instance, virus-induced RNA 1 (vir-1), a marker of the induction of anti-viral response, is mainly regulated by JAK/STAT pathway (32). Loss of function of JAK (named Hopscotch in Drosophila) caused decreased expression of vir-1, increased viral load and decreased survival after Drosophila C virus (DCV) infection. However, the molecular mechanism of antiviral activity of Vir-1 is unknown.
Although intracellular degradation is crucial to virus elimination, intercellular communication is believed to orchestrate and coordinate the cellular events. In the following, we will review the recent studies on extracellular signaling networks during antiviral immune response (Figure 1) and discuss the prospects and challenges of future work.
Figure 1 Intercellular communications in insect antiviral immune response. (A) Cytokines produced and released from viral infected cells bind to receptors and activate antiviral immune response in target cells. (B) Double-stranded RNA (dsRNA) and Ago-2 is transferred through tunneling nanotubes bridging infected cells and neighboring cells. (C) Viral-derived dsRNAs (vsRNAs) produced in viral-infected cells engulfed by haemocytes are reverse-transcribed into vDNAs by endogenous transposon reverse transcriptase. vDNAs then serve as template for transcription of secondary vsRNAs which are secreted in exosome-like vesicles and processed into siRNA by cells taking up these vesicles.
As a comparison, the potent antiviral immune response in mammalian cells is largely dependent on a group of secretory protein collectively named cytokines, which are produced and secreted by viral-infected cells, and bind specific receptors on its own, neighboring or distant cells to initiate intracellular signaling mainly via JAK/STAT pathway (33, 34). Cytokines are divided into several subgroups, including interferon, chemokine, interleukin and tumor necrosis factor, among which interferon is particularly important for the immune response to virus. Cells activated by interferon synthesize various molecules that inhibit virus entry, replication and assembly, or produce inflammatory reactions to initiate apoptosis, autophagy and necrosis (35). Although comparative genomic analysis and evolutionary study revealed that insects do not possess the homologous molecules to vertebrate cytokines, the core components of JAK/STAT pathway including Hopscotch (JAK), STAT92E (STAT), negative regulators SOCS and PIAS have been identified in insects, and parallels between gain-of-function studies with mammalian homologs suggests the functional similarity of insect JAK/STAT pathway to vertebrates. Thanks to the genetic analysis of mutants defective in embryonic development, ligands and receptor of JAK/STAT pathway were first discovered in Drosophila (36). All three ligands, named unpaired (Upd), Upd2, and Upd3 bind the same receptor named Domelss (Dome) which shares sequence similarity with mammalian IL-6 receptor (37), but only Udp2 and Upd3 are induced by viral infection and provide protection from a viral infection (38). Notably, JAK/STAT pathway has been considered to be triggered in bystander cells rather than in infected cells, since vir-1 was not induced in DCV-infected fat body and periovarian sheath, but was substantially induced in epithelial cells of the ventral epidermis or in the oviduct, in which no viral load was detected, suggesting that vir-1 was induced after a signal generated by the DCV-infected cells (32).
The signaling pathways responsible for induction of mammalian cytokines may also give some clues to whether there exists any “cytokine” that transmits antiviral signals between insect cells. Viral nucleic acids in mammalian cells are recognized by diverse cytosolic RNA or DNA sensors, including toll-like receptors (TLRs), retinoic acid-inducible gene I (RIG-I), absent in melanoma 2 (AIM2), DNA-dependent activator of IFN-regulatory factors (DAI) and cyclic GMP-AMP synthase (cGAS) (39). The signal is eventually relayed to transcriptional factors, including interferon regulatory factor 3 (IRF3) and NF-κB via signaling adaptors, such as antiviral-signaling protein (MAVS) and stimulator of interferon genes (STING) to activate interferon expression (40, 41). Despite the fact that far less nucleic acids sensors have been identified in insects, STING-mediated antiviral immunity has been discovered in Drosophila and Bombyx mori recently (23, 42, 43). Epistatic analysis showed that dSTING acts upstream of IKKβ and NF-κB transcriptional factor Relish to regulate the expression of a set of antiviral molecules, including a putative transmembrane protein named Nazo. Flies bearing dSTING or Relish mutant displayed higher susceptibility to infection of DCV, VSV or Cricket paralysis virus (CrPV). Activation of Relish by BmSTING was also detected in silkworm cell as evidenced by the cleavage of Relish carboxy-terminal Ankyrin repetitive sequence, which releases Relish from sequestration in cytoplasm, when BmSTING was over-expressed. The evolutionary conservation in STING- and NF-κB-dependent antiviral signaling pathway between insects and mammals suggests functional similarity in their downstream effectors. Indeed, a few years ago an antiviral factor Vago which bears no sequence homology to mammalian cytokines was first reported to be induced in the fat body of flies upon DCV infection, later its mosquito homologs that may act like interferon have been identified in Culex, Aedes and Anopheles (44, 45). CxVago was produced and secreted by West Nile virus (WNV)-infected cells. Incubating naïve cells with supernatant collected from Vago-expressing cells activated the JAK/STAT signaling pathway and induced the expression of vir-1 in naïve cells independent of Dome. A NF-κB binding site was identified in CxVago promoter region afterwards, and Culex Rel2 which is a Drosophila Relish homolog has been demonstrated to be required for induction of CxVago subsequently. In addition, activation of Rel2 upon SINV infection was observed in mosquito cells (46). Intriguingly, after incubating with supernatants harvested from cells expressing Relish activated form, naïve silkworm cells displayed substantial resistance to BmNPV infection. Certain polypeptides purified from the supernatants of DNV-infected mosquito cells also acted like cytokines, conferring antiviral activity to naïve cells (47, 48).
Interestingly, in both flies and mosquito cells, induction of Vago has been characterized to be Dicer-2 dependent, since Dicer-2-mutant flies or Dicer-2-silenced mosquito cells had significantly lower levels of Vago induced by viral infection compared to Dicer-2-intact controls. But mutation of other RNAi key players, such as Ago-2 and R2D2 had no impact on Vago expression, indicating that induction of Vago is independent of RNAi pathway. Phylogenetic analysis revealed Dicer-2, which is a key player in RNAi, is closely related to mammalian RIG-I in terms of their DExD/H-box helicase domain (49, 50). Both of them belong to RIG-I-like receptor (RLR) family along with some other cytoplasmic RNA sensors, including MDA5 and Laboratory of Genetics and Physiology 2 (LGP2). More recently, Dicer-2 has been reported to modulate viral DNA production via acting as a pattern recognition receptor similar to RLR that senses defective viral genomes (DVGs) (51). The absence of RIG-I proteins in insects but presence of the activity of RNA sensing and induction of antiviral factors which is carried out in a Dicer-2 dependent manner suggests Dicer-2 may be the archetypal RLR that activates the antiviral signaling pathway in insects. It is worth exploring whether Dicer-2, STING and Relish constitute a signaling axis that leads to the production of antiviral effectors and contributes to cell-cell communication.
Apparently, not all viral-induced molecules potentiate antiviral immunity, some may promote host survival by preventing immune signaling from over-activation. Diedel has been characterized as an immunomodulatory cytokine in Drosophila that was strongly induced following infection with slowly replicating viruses, such as SINV and VSV (52). diedel mutant flies developed persistent inflammation as a few immune-related genes, most of which are considered to be controlled by the IMD pathway, were up-regulated in the absence of viral infection. They also showed reduced survival after immune challenges without an increase in viral load, suggesting the IMD pathway which may contribute to viral-induced pathogenesis is required to be down-regulated. Interestingly, Diedel homologs have also been identified in the genome of three different and unrelated families of DNA viruses that infect Lepidoptera, including Entomopoxvirinae, Baculoviridae, and Ascoviridae (53). Transcriptome analysis found elevated expression of ascovirus diedel in infected Spodoptera frugiperda larvae (54), and expression of the ascovirus diedel partially rescued the reduced viability of diedel mutant flies (52). The possible horizontal transfer of immunomodulatory genes from host to virus represents a strategy that virus exploits to manipulate host immune response in favor of its own replication and dissemination.
Intercellular transferring of virus- or host-derived RNA, DNA and proteins from infected cells to neighboring cells are increasingly recognized as an important mean to mount a self-sustaining and even amplified innate immune response. Gap junctions, exosomes, microvesicles and plant plasmodesmata have been reported to deliver the substances originated from viral infected cells to immunize the other cells before arrival of the virus (55–58). Although the open circulatory system in insects is always believed to allow fast spread of virus in the hemolymph and migration beyond the primary site of replication, the possible cell-cell communication is supported by evidence of intercellular transferring of virus-derived RNA. Flies defective in dsRNA endocytosis or intracellular transport were hypersensitive to viral infection, and the high mortality was accompanied by hundredfold increase in viral titer, suggesting a systemic spread of dsRNA is required for antiviral immunity (59). Nanotube-like structures made of actin and tubulin were first reported in a study of the intercellular communication between Drosophila cells (60). Those membrane projections generated by viral-infected cells bridge neighboring cells for transferring of components of RNAi machinery, including Ago-2 and dsRNA between cells. A more recent study discovered that haemocytes acquire virus-derived dsRNA (vsRNA) by phagocytosing virus-infected cells and reverse-transcribe the viral RNA through endogenous transposon reverse transcriptases into DNA which serves as a template for transcription of secondary vsRNA in an Ago-2 dependent manner (61). The secondary vsRNA is secreted by haemocytes in exosome-like vesicles (ELVs) and spreads through the haemolymph. It is then processed into siRNA by cells taking up these ELVs and confers virus-specific immunity. Of note, this systemic antiviral potential of haemocyte-derived ELVs persists weeks after the onset of viral infection, thus it was proposed as an RNAi-based “adaptive immunity” in Drosophila.
Extracellular signaling network coordinates the systemic immune response through alarming or even arming the non-infected cells with messages from viral infected cells. Although it is one of the most important parts of immune response, much less have we learnt about the molecules or vesicles secreted by viral infected cells, ways to deliver them or the pathways they influence. Integrated omics approaches might be required to characterize the soluble substances in the fractionated extracellular fluid of viral infected cells in the future research, screening of target genes under regulation of signaling pathways that are activated by viral sensors would also help to narrow down the candidates. Furthermore, the absence of viral loads in tissues expressing antiviral marker genes (32) or passive protection of naïve flies against viral challenges conferred by injection of purified ELVs from viral infected flies (61) suggests a tissue-targeted delivery or diffusion throughout the entire body, therefore identification of molecules that act as receptors or carriers of those extracellular substances will decipher how the antiviral signal is transmitted between cells, which tissues or organs are targeted and which intracellular pathways are activated.
Although the lack of sequence similarity between insect and vertebrate cytokines impedes a sequence-function relationship analysis, the structural features they share suggest they are functionally related. For example, one subdomain of Diedel, consisting of an antiparallel β-sheet covered by an α-helix, resembles certain CC or CXC chemokine family members (62), which modulate immune response by maintaining proliferative homeostasis and attenuating apoptosis. Interestingly, recombinant human IL-8 was reported to promote the phagocytic activity of Drosophila S2 cells and enhance the expression of Upd-3 as well as some AMP genes, including defensin, cecropin A1, and diptericin (63), implying that certain membrane bound molecule may function as receptor to ligand that resembles the structure of IL-8.
Some danger signals, such as metabolites produced by viral-infected cells or damage-associated molecular pattern (DAMP) released by dead or damaged cells, may also serve as mediator for systemic inflammatory response. For instance, in mammalian models nitric oxide (NO) generated through NO synthase (NOS) which is upregulated upon viral infection can diffuse freely across cell membranes and activate antiviral mechanisms in various ways, including direct and indirect damage to viral genomes (64, 65). In insects, it is well documented that NO regulates immune response to bacteria, nematode and parasites characterized by AMP expression and melanin production (66–68), and a cell-based assay showed that NO inhibits DENV replication partly through suppressing RNA-dependent RNA polymerase (69), although its role in insect antiviral immunity has not been characterized. Actin, an evolutionarily-conserved DAMP was reported to selectivity induce JAK/STAT target genes through cytokine Upd3 in Drosophila, whether it confers antiviral activity needs further investigation (70).
Antiviral immune response induced by different viruses varies, which might be another factor that complicates the understanding of insect antiviral immunity. For instance, Vago/Vago-like expression was down-regulated upon the infection of virulent virus but not with avirulent virus in bumblebee (71). Fast replicating viruses, such as DCV, CrPV and Flock House virus (FHV), unlike slowly replicating viruses, did not induce Diedel expression (52). In the mosquito midgut, transcriptional level of Rel2 and its canonical target genes, such as diptericin and attancin, was not induced by DENV (72), but the activation of Rel2 was detected on protein level and knockdown of Rel2 significantly increased WNV viral load (44). The seemingly disagreement on the involvement of certain molecules in antiviral immunity suggests a careful assessment of their general or specific functions is required.
In addition to their potential roles in the antiviral immune response, some molecules also participate in the defense against other microbial challenges. For example, knock-down of dSTING resulted in more susceptibility to Listeria infection (73) and mutation of BmSTING led to defective autophagy of microsporidia in silkworm larvae (74), suggesting insect STING mediates immune signaling pathways in response to various pathogens. However, some cytokines that have been identified in insect immune defenses against bacteria or parasites, such as growth blocking peptide (GBP) which has been characterized as a cytokine switching humoral and cellular immune response (75, 76), and TNF ortholog Eiger which promotes apoptotic cell death via JNK pathway and aids clearance of extracellular pathogens (77, 78), are not reported in the antiviral response. Therefore, it will be interesting to investigate whether there exist multifaceted mediators in insect innate immunity.
While studies on the viral-induced intercellular communication are still preliminary in insects, they provide valuable insights into artificial manipulation of host immune response. Insulin/insulin-like peptide has been recently reported to potentiate JAK/STAT pathway via ERK to broadly inhibit flavivirus replication in fly and mosquito cells, and insulin-supplemented meal effectively reduced WNV titers in infected Culex mosquitos (79, 80). Although the change in insulin level induced by viral infection was not yet reported in insects or even linked to antiviral immunity prior to this report, the decrease in insulin secretion was found to be common in mammals after viral infection. Research efforts aimed at characterizing the intercellular communication will not only provide a greater depth of knowledge regarding extracellular signaling networks, but also potential targets for pest or disease control based on interfering intercellular communication or priming insects with molecules transmitting antiviral messengers.
The author confirms being the sole contributor of this work and has approved it for publication.
This work is supported by grant of “National Natural Science Foundation of China” (No. 31672495) and Natural Science Foundation of Chongqing, China (cstc2020jcyj-msxmX0193).
The author declares that the research was conducted in the absence of any commercial or financial relationships that could be construed as a potential conflict of interest.
The author thanks Prof. Qingyou Xia and Yang Cao for inspiring discussion.
2. Schneider WM, Chevillotte MD, Rice CM. Interferon-stimulated genes: A complex web of host defenses. Annu Rev Immunol (2014) 32:513–45. doi: 10.1146/annurev-immunol-032713-120231
3. Mussabekova A, Daeffler L, Imler J-L. Innate and intrinsic antiviral immunity in Drosophila. Cell Mol Life Sci (2017) 74:2039–54. doi: 10.1007/s00018-017-2453-9
4. Lamiable O, Imler J-L. Induced antiviral innate immunity in Drosophila. Curr Opin Microbiol (2014) 20:62–8. doi: 10.1016/j.mib.2014.05.006
5. Kemp C, Imler J-L. Antiviral immunity in Drosophila. Curr Opin Immunol (2009) 21:3–9. doi: 10.1016/j.coi.2009.01.007
6. Hussain M, Asgari S. MicroRNAs as mediators of insect host-pathogen interactions and immunity. J Insect Physiol (2014) 70:151–8. doi: 10.1016/j.jinsphys.2014.08.003
7. Asgari S. Role of microRNAs in insect host-microorganism interactions. Front Physiol (2011) 2:48:48. doi: 10.3389/fphys.2011.00048
8. Wu Q, Luo Y, Lu R, Lau N, Lai EC, Li W-X, et al. Virus discovery by deep sequencing and assembly of virus-derived small silencing RNAs. Proc Natl Acad Sci USA (2010) 107:1606–11. doi: 10.1073/pnas.0911353107
9. Petit M, Mongelli V, Frangeul L, Blanc H, Jiggins F, Saleh MC. piRNA pathway is not required for antiviral defense in Drosophila melanogaster. Proc Natl Acad Sci USA (2016) 113:E4218–E27. doi: 10.1073/pnas.1607952113
10. Tassetto M, Kunitomi M, Whitfield ZJ, Dolan PT, Sanchez-Vargas I, Garcia-Knight M, et al. Control of RNA viruses in mosquito cells through the acquisition of vDNA and endogenous viral elements. Elife (2019) 8:e41244. doi: 10.7554/eLife.41244
11. Varjak M, Leggewie M, Schnettler E. The antiviral piRNA response in mosquitoes? J Gen Virol (2018) 99:1551–62. doi: 10.1099/jgv.0.001157
12. Lamiable O, Arnold J, de Faria I, Olmo RP, Bergami F, Meignin C, et al. Analysis of the contribution of hemocytes and autophagy to Drosophila antiviral immunity. J Virol (2016) 90:5415–26. doi: 10.1128/jvi.00238-16
13. Nainu F, Shiratsuchi A, Nakanishi Y. Induction of apoptosis and subsequent phagocytosis of virus-infected cells as an antiviral mechanism. Front Immunol (2017) 8:1220. doi: 10.3389/fimmu.2017.01220
14. Makino S, Hamajima R, Saito A, Tomizaki M, Iwamoto A, Kobayashi M, et al. Bombyx mori homolog of tumor suppressor p53 is involved in apoptosis-mediated antiviral immunity of B. mori cells infected with nucleopolyhedrovirus. Dev Comp Immunol (2018) 84:133–41. doi: 10.1016/j.dci.2018.02.009
15. Mocarski E, Liu B, Behura SK, Clem RJ, Schneemann A, Becnel J, et al. P53-mediated rapid induction of apoptosis conveys resistance to viral infection in Drosophila melanogaster. PloS Pathog (2013) 9:e1003137. doi: 10.1371/journal.ppat.1003137
16. Settles EW, Friesen PD. Flock house virus induces apoptosis by depletion of Drosophila inhibitor-of-apoptosis protein DIAP1. J Virol (2008) 82:1378–88. doi: 10.1128/jvi.01941-07
17. Nainu F, Tanaka Y, Shiratsuchi A, Nakanishi Y. Protection of insects against viral infection by apoptosis-dependent phagocytosis. J Immunol (2015) 195:5696–706. doi: 10.4049/jimmunol.1500613
18. Jiang L, Liu W, Guo H, Dang Y, Cheng T, Yang W, et al. Distinct functions of Bombyx mori peptidoglycan recognition protein 2 in immune responses to bacteria and viruses. Front Immunol (2019) 10:776. doi: 10.3389/fimmu.2019.00776
19. Jiang L, Goldsmith MR, Xia Q. Advances in the arms race between silkworm and baculovirus. Front Immunol (2021). doi: 10.3389/fimmu.2021.628151
20. Delorme-Axford E, Klionsky DJ. Inflammatory-dependent sting activation induces antiviral autophagy to limit Zika virus in the Drosophila brain. Autophagy (2018) 15:1–3. doi: 10.1080/15548627.2018.1539585
21. Liu Y, Cherry S. Zika virus infection activates STING-dependent antiviral autophagy in the Drosophila brain. Autophagy (2018) 15:174–5. doi: 10.1080/15548627.2018.1528813
22. Shelly S, Lukinova N, Bambina S, Berman A, Cherry S. Autophagy is an essential component of Drosophila immunity against vesicular stomatitis virus. Immunity (2009) 30:588–98. doi: 10.1016/j.immuni.2009.02.009
23. Liu Y, Gordesky-Gold B, Leney-Greene M, Weinbren NL, Tudor M, Cherry S. Inflammation-induced, STING-dependent autophagy restricts Zika virus infection in the Drosophila brain. Cell Host Microbe (2018) 24:57–68. doi: 10.1016/j.chom.2018.05.022
24. Moy Ryan H, Gold B, Molleston Jerome M, Schad V, Yanger K, Salzano M-V, et al. Antiviral autophagy restricts Rift Valley fever virus infection and is conserved from flies to mammals. Immunity (2014) 40:51–65. doi: 10.1016/j.immuni.2013.10.020
25. Nakamoto M, Moy Ryan H, Xu J, Bambina S, Yasunaga A, Shelly Spencer S, et al. Virus recognition by Toll-7 activates antiviral autophagy in Drosophila. Immunity (2012) 36:658–67. doi: 10.1016/j.immuni.2012.03.003
26. Xu J, Grant G, Sabin Leah R, Gordesky-Gold B, Yasunaga A, Tudor M, et al. Transcriptional pausing controls a rapid antiviral innate immune response in Drosophila. Cell Host Microbe (2012) 12:531–43. doi: 10.1016/j.chom.2012.08.011
27. Huang Z, Kingsolver MB, Avadhanula V, Hardy RW. An antiviral role for antimicrobial peptides during the arthropod response to alphavirus replication. J Virol (2013) 87:4272–80. doi: 10.1128/JVI.03360-12
28. Diamond MS, Luplertlop N, Surasombatpattana P, Patramool S, Dumas E, Wasinpiyamongkol L, et al. Induction of a peptide with activity against a broad spectrum of pathogens in the Aedes aegypti salivary gland, following infection with Dengue virus. PloS Pathog (2011) 7:e1001252. doi: 10.1371/journal.ppat.1001252
29. Bao Y-Y, Tang X-D, Lv Z-Y, Wang X-Y, Tian C-H, Xu YP, et al. Gene expression profiling of resistant and susceptible Bombyx mori strains reveals nucleopolyhedrovirus-associated variations in host gene transcript levels. Genomics (2009) 94:138–45. doi: 10.1016/j.ygeno.2009.04.003
30. Merkling SH, van Rij RP. Beyond RNAi: Antiviral defense strategies in Drosophila and mosquito. J Insect Physiol (2013) 59:159–70. doi: 10.1016/j.jinsphys.2012.07.004
31. Xu J, Cherry S. Viruses and antiviral immunity in Drosophila. Dev Comp Immunol (2014) 42:67–84. doi: 10.1016/j.dci.2013.05.002
32. Dostert C, Jouanguy E, Irving P, Troxler L, Galiana-Arnoux D, Hetru C, et al. The Jak-STAT signaling pathway is required but not sufficient for the antiviral response of drosophila. Nat Immunol (2005) 6:946–53. doi: 10.1038/ni1237
33. Majoros A, Platanitis E, Kernbauer-Hölzl E, Rosebrock F, Müller M, Decker T. Canonical and non-canonical aspects of JAK–STAT signaling: lessons from interferons for cytokine responses. Front Immunol (2017) 8:29:29. doi: 10.3389/fimmu.2017.00029
34. Morris R, Kershaw NJ, Babon JJ. The molecular details of cytokine signaling via the JAK/STAT pathway. Protein Sci (2018) 27:1984–2009. doi: 10.1002/pro.3519
35. Schoggins JW, Rice CM. Interferon-stimulated genes and their antiviral effector functions. Curr Opin Virol (2011) 1:519–25. doi: 10.1016/j.coviro.2011.10.008
36. Zeidler MP, Bausek N. The Drosophila JAK-STAT pathway. JAKSTAT (2014) 2:e25353. doi: 10.4161/jkst.25353
37. Chen HW, Chen X, Oh SW, Marinissen MJ, Gutkins JS, Hou SX. Mom identifies a receptor for the Drosophila JAK/STAT signal transduction pathway and encodes a protein distantly related to the mammalian cytokine receptor family. Gene Dev (2002) 16:388–98. doi: 10.1101/gad.955202
38. Kemp C, Mueller S, Goto A, Barbier V, Paro S, Bonnay F, et al. Broad RNA interference–mediated antiviral immunity and virus-specific inducible responses in Drosophila. J Immunol (2013) 190:650–8. doi: 10.4049/jimmunol.1102486
39. Wu J, Chen ZJ. Innate immune sensing and signaling of cytosolic nucleic acids. Annu Rev Immunol (2014) 32:461–88. doi: 10.1146/annurev-immunol-032713-120156
40. Liu S, Cai X, Wu J, Cong Q, Chen X, Li T, et al. Phosphorylation of innate immune adaptor proteins MAVS, STING, and TRIF induces IRF3 activation. Science (2015) 347:aaa2630. doi: 10.1126/science.aaa2630
41. Ran Y, Shu H-B, Wang Y-Y. MITA/STING: a central and multifaceted mediator in innate immune response. Cytokine Growth Factor Rev (2014) 25:631–9. doi: 10.1016/j.cytogfr.2014.05.003
42. Hua X, Li B, Song L, Hu C, Li X, Wang D, et al. Stimulator of interferon genes (STING) provides insect antiviral immunity by promoting Dredd caspase–mediated NF-κB activation. J Biol Chem (2018) 293:11878–90. doi: 10.1074/jbc.RA117.000194
43. Goto A, Okado K, Martins N, Cai H, Barbier V, Lamiable O, et al. The kinase IKKβ regulates a STING- and NF-κB-dependent antiviral response pathway in Drosophila. Immunity (2018) 49:225–34.e4. doi: 10.1016/j.immuni.2018.07.013
44. Olson KE, Paradkar PN, Duchemin J-B, Voysey R, Walker PJ. Dicer-2-dependent activation of Culex Vago occurs via the TRAF-Rel2 signaling pathway. PloS Negl Trop Dis (2014) 8:e2823. doi: 10.1371/journal.pntd.0002823
45. Paradkar PN, Trinidad L, Voysey R, Duchemin JB, Walker PJ. Secreted Vago restricts West Nile virus infection in Culex mosquito cells by activating the Jak-STAT pathway. Proc Natl Acad Sci USA (2012) 109:18915–20. doi: 10.1073/pnas.1205231109
46. Avadhanula V, Weasner BP, Hardy GG, Kumar JP, Hardy RW. A novel system for the launch of alphavirus RNA synthesis reveals a role for the IMD pathway in arthropod antiviral response. PloS Pathog (2009) 5:e1000582. doi: 10.1371/journal.ppat.1000582
47. Wang F, Li X, Hua X, Xia Q. Screening and analysis of anti-BmNPV cytokines in silkworm (Bombyx mori). Sci Agricult Sinica (2018) 51:789–99. doi: 10.3864/j.issn.0578-1752.2018.04.018
48. Kanthong N, Laosutthipong C, Flegel TW. Response to Dengue virus infections altered by cytokine-like substances from mosquito cell cultures. BMC Microbiol (2010) 10:290. doi: 10.1186/1471-2180-10-290
49. Deddouche S, Matt N, Budd A, Mueller S, Kemp C, Galiana-Arnoux D, et al. The DExD/H-box helicase Dicer-2 mediates the induction of antiviral activity in drosophila. Nat Immunol (2008) 9:1425–32. doi: 10.1038/ni.1664
50. Paro S, Imler J-L, Meignin C. Sensing viral RNAs by Dicer/RIG-I like ATPases across species. Curr Opin Immunol (2015) 32:106–13. doi: 10.1016/j.coi.2015.01.009
51. Poirier EZ, Goic B, Tomé-Poderti L, Frangeul L, Boussier J, Gausson V, et al. Dicer-2-dependent generation of viral DNA from defective genomes of RNA viruses modulates antiviral immunity in insects. Cell Host Microbe (2018) 23:353–65.e8. doi: 10.1016/j.chom.2018.02.001
52. Lamiable O, Kellenberger C, Kemp C, Troxler L, Pelte N, Boutros M, et al. Cytokine Diedel and a viral homologue suppress the IMD pathway in Drosophila. Proc Natl Acad Sci USA (2016) 113:698–703. doi: 10.1073/pnas.1516122113
53. Gould A, Mlih M, Khericha M, Birdwell C, West AP. Karpac J. A virus-acquired host cytokine controls systemic aging by antagonizing apoptosis. PloS Biol (2018) 16:e2005796. doi: 10.1371/journal.pbio.2005796
54. Zaghloul HAH, Hice R, Arensburger P, Federici BA. Transcriptome analysis of the Spodoptera frugiperda ascovirus in vivo provides insights into how its apoptosis inhibitors and caspase promote increased synthesis of viral vesicles and virion progeny. J Virol (2017) 91:e00874–17. doi: 10.1128/JVI.00874-17
55. Rosas-Diaz T, Zhang D, Fan PF, Wang LP, Ding X, Jiang YL, et al. A virus-targeted plant receptor-like kinase promotes cell-to-cell spread of RNAi. Proc Natl Acad Sci USA (2018) 115:1388–93. doi: 10.1073/pnas.1715556115
56. Kalamvoki M, Du T, Roizman B. Cells infected with herpes simplex virus 1 export to uninfected cells exosomes containing STING, viral mRNAs, and microRNAs. Proc Natl Acad Sci USA (2014) 111:E4991–6. doi: 10.1073/pnas.1419338111
57. Baroja-Mazo A, Martin-Sanchez F, Gomez AI, Martinez CM, Amores-Iniesta J, Compan V, et al. The NLRP3 inflammasome is released as a particulate danger signal that amplifies the inflammatory response. Nat Immunol (2014) 15:738–48. doi: 10.1038/ni.2919
58. Patel SJ, King KR, Casali M, Yarmush ML. DNA-triggered innate immune responses are propagated by gap junction communication. Proc Natl Acad Sci USA (2009) 106:12867–72. doi: 10.1073/pnas.0809292106
59. Saleh M-C, Tassetto M, van Rij RP, Goic B, Gausson V, Berry B, et al. Antiviral immunity in Drosophila requires systemic RNA interference spread. Nature (2009) 458:346–50. doi: 10.1038/nature07712
60. Karlikow M, Goic B, Mongelli V, Salles A, Schmitt C, Bonne I, et al. Drosophila cells use nanotube-like structures to transfer dsRNA and RNAi machinery between cells. Sci Rep (2016) 6:27085. doi: 10.1038/srep27085
61. Tassetto M, Kunitomi M, Andino R. Circulating immune cells mediate a systemic RNAi-based adaptive antiviral response in Drosophila. Cell (2017) 169:314–25.e13. doi: 10.1016/j.cell.2017.03.033
62. Doucet D, Coste F, Kemp C, Bobezeau V, Hetru C, Kellenberger C, et al. Crystal structure of Diedel, a marker of the immune response of Drosophila melanogaster. PloS One (2012) 7:e33416. doi: 10.1371/journal.pone.0033416
63. Malagoli D, Sacchi S, Ottaviani E. Unpaired (upd)-3 expression and other immune-related functions are stimulated by interleukin-8 in Drosophila melanogaster SL2 cell line. Cytokine (2008) 44:269–74. doi: 10.1016/j.cyto.2008.08.011
64. Abdul-Cader MS, Amarasinghe A, Abdul-Careem MF. Activation of toll-like receptor signaling pathways leading to nitric oxide-mediated antiviral responses. Arch Virol (2016) 161:2075–86. doi: 10.1007/s00705-016-2904-x
65. Nappi AJ, Vass E, Frey F, Carton Y. Nitric oxide involvement in Drosophila immunity. Nitric Oxide (2000) 4:423–30. doi: 10.1006/niox.2000.0294
66. Eleftherianos I, More K, Spivack S, Paulin E, Khojandi A, Shukla S. Nitric oxide levels regulate the immune response of Drosophila melanogaster reference laboratory strains to bacterial infections. Infect Immun (2014) 82:4169–81. doi: 10.1128/Iai.02318-14
67. Foley E, O’Farrell PH. Nitric oxide contributes to induction of innate immune responses to gram-negative bacteria in Drosophila. Gene Dev (2003) 17:115–25. doi: 10.1101/gad.1018503
68. Herrera-Ortiz A, Martinez-Barnetche J, Smit N, Rodriguez MH, Lanz-Mendoza H. The effect of nitric oxide and hydrogen peroxide in the activation of the systemic immune response of Anopheles albimanus infected with Plasmodium berghei. Dev Comp Immunol (2011) 35:44–50. doi: 10.1016/j.dci.2010.08.004
69. Takhampunya R, Padmanabhan R, Ubol S. Antiviral action of nitric oxide on dengue virus type 2 replication. J Gen Virol (2006) 87:3003–11. doi: 10.1099/vir.0.81880-0
70. Srinivasan N, Gordon O, Ahrens S, Franz A, Deddouche S, Chakravarty P, et al. Actin is an evolutionarily-conserved damage-associated molecular pattern that signals tissue injury in Drosophila melanogaster. Elife (2016) 5:e19662. doi: 10.7554/eLife.19662
71. Niu J, Meeus I, Smagghe G. Differential expression pattern of Vago in bumblebee (Bombus terrestris), induced by virulent and avirulent virus infections. Sci Rep (2016) 6:34200. doi: 10.1038/srep34200
72. Xi Z, Ramirez JI, Dimopoulos G. The Aedes aegypti Toll pathway controls dengue virus infection. PloS Pathog (2008) 4:e1000098. doi: 10.1371/journal.ppat.1000098
73. Martin M, Hiroyasu A, Guzman RM, Roberts SA, Goodman AG. Analysis of Drosophila STING reveals an evolutionarily conserved antimicrobial function. Cell Rep (2018) 23:3537–50.e6. doi: 10.1016/j.celrep.2018.05.029
74. Hua X, Xu W, Ma S, Xia Q. STING-dependent autophagy suppresses Nosema bombycis infection in silkworms, Bombyx mori. Dev Comp Immunol (2020) 115:103862. doi: 10.1016/j.dci.2020.103862
75. Ishii K, Hamamoto H, Kamimura M, Nakamura Y, Noda H, Imamura K, et al. Insect cytokine paralytic peptide (PP) induces cellular and humoral immune responses in the silkworm Bombyx mori. J Biol Chem (2010) 285:28635–42. doi: 10.1074/jbc.M110.138446
76. Tsuzuki S, Matsumoto H, Furihata S, Ryuda M, Tanaka H, Jae Sung E, et al. Switching between humoral and cellular immune responses in Drosophila is guided by the cytokine GBP. Nat Commun (2014) 5:4628. doi: 10.1038/ncomms5628
77. Schneider DS, Ayres JS, Brandt SM, Costa A, Dionne MS, Gordon MD, et al. Drosophila eiger mutants are sensitve to extracellular pathogens. PloS Pathog (2007) 3:e24. doi: 10.1371/journal.ppat.0030041
78. Igaki T, Kanda H, Yamamoto-Goto Y, Kanuka H, Kuranaga E, Aigaki T, et al. Eiger, a TNF superfamily ligand that triggers the Drosophila JNK pathway. EMBO J (2002) 21:3009–18. doi: 10.1093/emboj/cdf306
79. Trammell CE, Goodman AG. Emerging mechanisms of insulin-mediated antiviral immunity in Drosophila melanogaster. Front Immunol (2019) 10:2973:2973. doi: 10.3389/fimmu.2019.02973
Keywords: insect, antiviral immunity, cytokine, Dicer-2, intercellular transfer
Citation: Wang F (2021) Sending Out Alarms: A Perspective on Intercellular Communications in Insect Antiviral Immune Response. Front. Immunol. 12:613729. doi: 10.3389/fimmu.2021.613729
Received: 03 October 2020; Accepted: 12 January 2021;
Published: 23 February 2021.
Edited by:
Luc Swevers, National Centre of Scientific Research Demokritos, GreeceReviewed by:
Humberto Lanz-Mendoza, National Institute of Public Health, MexicoCopyright © 2021 Wang. This is an open-access article distributed under the terms of the Creative Commons Attribution License (CC BY). The use, distribution or reproduction in other forums is permitted, provided the original author(s) and the copyright owner(s) are credited and that the original publication in this journal is cited, in accordance with accepted academic practice. No use, distribution or reproduction is permitted which does not comply with these terms.
*Correspondence: Fei Wang, bWFzc21vY2FAc3d1LmVkdS5jbg==
Disclaimer: All claims expressed in this article are solely those of the authors and do not necessarily represent those of their affiliated organizations, or those of the publisher, the editors and the reviewers. Any product that may be evaluated in this article or claim that may be made by its manufacturer is not guaranteed or endorsed by the publisher.
Research integrity at Frontiers
Learn more about the work of our research integrity team to safeguard the quality of each article we publish.