- 1Laboratory of Cellular Immunology and Pharmacology, National Institute for infectious Diseases “Lazzaro Spallanzani”-IRCCS, Rome, Italy
- 2Laboratory of Translational Research, National Institute for infectious Diseases “Lazzaro Spallanzani”-IRCCS, Rome, Italy
- 3UOS Professioni Sanitarie Tecniche National Institute for Infectious Diseases Lazzaro Spallanzani-IRCCS, Rome, Italy
- 4Department of Biology, University of Rome “Tor Vergata”, Rome, Italy
- 5Department of Epidemiology, Preclinical Research, and Advanced Diagnostics, National Institute for Infectious Diseases ‘Lazzaro Spallanzani’-IRCCS, Rome, Italy
- 6Respiratory Infectious Diseases Unit, National Institute for infectious Diseases “Lazzaro Spallanzani”-IRCCS, Rome, Italy
Tuberculosis (TB), due to Mycobacterium tuberculosis infection, is still the principal cause of death caused by a single infectious agent. The balance between the bacillus and host defense mechanisms reflects the different manifestations of the pathology. Factors defining this variety are unclear and likely involve both mycobacterial and immunological components. Myeloid derived suppressor cells (MDSC) have been shown to be expanded during TB, but their role in human TB pathogenesis is not clear. We evaluated the frequency of circulating MDSC by flow-cytometry in 19 patients with active TB, 18 with latent TB infection (LTBI), and 12 healthy donors (HD) as control. Moreover, we investigated the capacity of MDSC to modulate the mycobactericidal activity of monocytes. The association between MDSC level and TB chest X-ray severity score was analyzed. We observed that, unlike active TB, polymorphonuclear (PMN)-MDSC are not expanded in LTBI patients, and, by performing a receiver operating characteristic (ROC) curve analysis, we found that PMN-MDSC frequency supported the discrimination between active disease and LTBI. Interestingly, we observed an association between PMN-MDSC levels and the severity of TB disease evaluated by chest X-ray. Specifically, PMN-MDSC frequency was higher in those classified with a low/mild severity score compared to those classified with a high severity score. Moreover, PMN-MDSC can impact mycobacterial growth by inducing ROS production in Bacillus Calmette et Guerin (BCG)-infected monocytes. This effect was lost when tested with M. tuberculosis (MTB), In conclusion, our data indicate that the elevated frequency of PMN-MDSC in IGRA-positive individuals is associated with active TB. Our findings also pointed out a beneficial role of PMN-MDSC during human active TB, most likely associated with the limitation of inflammation-induced tissue damage.
Introduction
Tuberculosis (TB) remains a leading cause of global mortality (World Health Organization. Global Tuberculosis Report 2020) and the concerns about its elimination is related to a poor understanding of TB disease mechanisms.
The course of infection is extremely variable in humans. Once infected, most people control the initial infection. The individuals with a positive response to an immunologic test [tuberculin skin test (TST) or interferon-γ release assay (IGRA)] without signs or symptoms of active TB are classified as having a “latent TB infection” (LTBI). A small proportion (5–15%) of these individuals progress to active, symptomatic, and transmissible TB within 2 years of infection, likely representing a lack of initial control of infection; this is termed primary TB. Active TB develops in a variety of ways. Pulmonary TB can present with mild, moderate, or severe respiratory and systemic symptoms, with involvement of single or multiple lung lobes. The factors defining this variety are unclear and likely involve both microbial and immunologic components. Although the identification of protective markers of immune response are still lacking, a number of studies have shown that CD8 T cell–mediated killing of infected host cells is crucial for the defense against Mycobacterium tuberculosis (MTB) infection (1, 2). Suppression of cell-mediated immunity is associated with the development of TB disease (3). Regulatory T cells (Tregs) are increased in active TB (4), and limit potentially protective immune responses and facilitate microbial replication during TB as well as in other diseases (5, 6). In addition to Tregs, myeloid-derived suppressor cells (MDSC) is another immunosuppressive cell population known to be increased during TB disease (7, 8). MDSC were initially observed in tumor‐bearing mice, and two main subset were described, polymorphonuclear (PMN) and monocytic MDSC. PMN-MDSC are clearly defined as CD11b++ Ly-6G+Ly6Clow cells, while M-MDSC as CD11b+Ly6G−Ly6Chigh. Since the lack of a specific markers for human MDSC, recently the standard characterization have been suggested to identify MDSC: in human peripheral blood mononuclear cell (PBMC) the equivalent to PMN-MDSC are defined as HLA-DR-/low CD11b+ CD14- CD15+ (or CD66b+) and M-MDSC as HLA-DR-/low CD11b+ (or CD33+) CD14+ CD15-. A third group of MDSC defined early-stage MDSC (e-MDSC) has been identified as HLA-DR- CD33+ CD15- Lin- (CD3- CD56- CD19- CD14-) (9).
MDSC are known to have the remarkable ability to suppress T-cell responses through several mechanisms, including inducible nitric oxide synthetase (iNOS), arginase-1(Arg-1), nicotinamide adenine dinucleotide phosphate oxidase (NOX2), and transforming growth factor β metabolism (10–13).
In murine model, it has been demonstrated a detrimental role of MDSC during TB (14). However, in TB patients, the role of MDSC has not been yet elucidated, and the MDSC frequency has not yet been clearly linked with the severity of disease, namely, the extension of the lung lesions evaluated by radiological score and/or smear grading. Further, contrasting data were reported on the levels of circulating MDSC in household contacts (7, 8).
In the present study we demonstrate that, unlike active TB, polymorphonuclear (PMN)-MDSC are not expanded in LTBI patients, and therefore the PMN-MDSC frequency might be used to discriminate between active disease and LTBI. Furthermore, we show an association between the PMN-MDSC level and the severity of the TB disease, possibly due to the capacity to control the inflammation level, thus counteracting lung tissue damage.
Materials and Methods
Study Population
Nineteen subjects with active TB, 18 with LTBI, and 12 TST negative healthy donors (HD) were enrolled at the respiratory disease wards and outpatient clinic at the “Lazzaro Spallanzani” National Institute for Infectious Diseases in Rome. All enrolled subjects were HIV-uninfected and not undergoing immune-suppressive therapy. The patient characteristics are reported in Table 1. Among those classified as active TB, 18 were pulmonary TB (12 MTB culture positive, 5 with a clinical diagnosis, 1 with a histology diagnosis), and 1 extra-pulmonary TB. Among active TB patients, 18 were recruited within 9 days from treatment initiation (Isoniazid, rifampicin, ethambutol, pyrazinamide), and 1 before therapy.
Evaluation of chest X-ray severity score was performed: 0 normal chest X-ray; 1: mild grade (nodules and or infiltrates with proportion of lung affected <30%); 2: intermediate grade (infiltrates with proportion of lung affected >30%, and/or cavitation <4cm in diameter); 3: high grade (infiltrates of any percentage of extension, with cavitation >4 cm in diameter and/or bronchial spread and/or miliarity and/or pleural effusion and/or adenopathy).
Among patients classified as LTBI, 9 were recent and 9 remote LTBI. LTBI diagnosis was based on a positive QuantiFERON Plus test (QFT-P) (Qiagen) in the absence of radiological signs of active TB and clinical symptoms of TB disease. Seventeen LTBI did not receive any preventive therapy before enrolment; one LTBI was recruited 4 days after preventive therapy initiation (Isoniazid).
The study was approved by the INMI Ethical Committee (approval number 72/2015). Informed written consent was required to participate in the study.
PBMC Isolation and Flow Cytometry Analysis
Peripheral venous blood was collected in 10 ml lithium-heparin BD Vacutainer® blood collection tubes (Becton Dickinson). Peripheral blood mononuclear cells (PBMC) were isolated from peripheral blood by density gradient centrifugation (Lympholyte-H; Cederlane). After separation, PBMC were resuspended in RPMI 1640 (EuroClone) supplemented with 10% heat-inactivated fetal bovine serum (FBS) (EuroClone), 2 mmol/L L-glutamine, 10 mmol/L HEPES buffer (N-2-hydroxyethylpiperazine-N-2-ethane sulfonic acid), hereafter termed R10, and 2 mmol/L penicillin, and 50 µg/mL streptomycin (EuroClone).
Evaluation of MDSC percentage was performed using 0.5 x 106 PBMC stained with anti-CD15 FITC (clone MMA), anti-CD33 PE (clone WM53), anti-CD14 PC5.5 (clone M5E2), anti-CD11b PE-Cy7 (clone M1/70), anti-CD16 pacific blue (clone 3G8) from BD Biosciences, and anti-HLA-DR ECD (clone IMMU-357), cocktail of antibodies anti-CD3 APC-A700 (clone UCHT1), -CD56 APC-A700 (N901), -CD19 APC-A700 (clone J3-119) (Lin) from Beckman Coulter. Monocyte absolute number was evaluated by routine blood count. Monocyte percentage subsets were evaluated using anti-CD14 FITC (clone M5E2), anti-CD16 pacific blue (clone 3G8) monoclonal antibodies (BD Biosciences). Single staining and compensation controls were used to set up flow cytometry experiments. Acquisition of 100,000 events was performed in the leukocyte-gated population on Navios and analyzed with Kaluza software (Beckman Coulter).
PMN-MDSC and Monocytes Purification
PMN-MDSC and CD14+ monocytes were purified from PBMC of HD by immunomagnetic sorting by using CD15 and CD14 microbeads respectively (Miltenyi Biotec) following the manufacturer’s procedure. The purity of PMN-MDSC and monocytes were >90% and 95% respectively, as verified by flow cytometry (data not shown). Purified cells were rested for 18 hours in R10.
To evaluate the immune suppressive ability of PMN-MDSC from HD, PBMC from the same donors were labeled with CFDA-SE (Vibrant CFDA SE cell tracer kit, Invitrogen) according with manufacturer’s instructions, and cultured with purified PMN-MDSC at 1:1 ratio. Cells were stimulated with Staphylococcus Enterotoxin 200ng/ml (SEB, Sigma Aldrich), and after 5 days lymphocytes proliferation was evaluated by flow cytometry.
Bacteria
Mycobacterium bovis BCG Pasteur strain (TMC1011) were grown in Middlebrook 7H9 (Difco, Sparks, MD) supplemented with 10% (vol/vol) oleic acid-albumin-dextrose-catalase (OADC; Difco), with 0.2% glycerol (Microbiol, Cagliari, Italy) and 0.05% Tween 80 (Sigma-Aldrich, St. Louis, MO) at 37°C, and titred by CFU assay, performed in plates with Middlebrook 7H10 (Difco) supplemented with 10% OADC (oleic acid, albumin, dextrose and catalase), as described (14, 15).
Mycobacterium bovis (BCG) transformed with the plasmid carrying Vibrio harveyi luciferase gene, LuxAB, in shuttle plasmid pSMT1 (BCGlux) was kindly provided by Prof. R. Reljic from S. George’s University of London (UK), grown in in Middlebrook 7H9 (Difco) broth supplemented with 10% ADC (albumin, dextrose and catalase), 0.05% Tween 80, and 50 μg/ml hygromycin B and used to evaluate intracellular mycobacterial viability, as described (15). MTB (H37Rv) was grown in Middlebrook 7H9 (Difco, Sparks, MD) supplemented with 10% (vol/vol) oleic acid-albumin-dextrose-catalase (OADC; Difco), with 0.2% glycerol (Microbiol, Cagliari, Italy) and 0.05% Tween 80 (Sigma-Aldrich, St. Louis, MO) at 37°C.
Monocytes Infection and PMN-MDSC Co-Culture
Monocytes (2.5x105) were infected in a final volume of 250µl for 3 hours at the multiplicity of infection (MOI) of 5 with BCG, BCG-lux, or MTB (H37Rv) previously sonicated in a bath sonicator for 3 minutes for removing mycobacterial clumps. Thereafter, infected cells were centrifuged three times at 558g for 5 minutes to remove extracellular bacilli and cultured for 72h at the ratio 1:1, with 2.5x105 uninfected purified autologous PMN-MDSC or monocytes. Single cultures of monocytes or PMN-MDSC (5 x 105, in order to maintain the equal cell number for each well during 3 day-stimulation) were infected with BCGlux and used as control of infection.
Infected cells were cultured in 48-well plate at the concentration of 2.5x105/500µl, in RPMI 1640 supplemented with 10% heat-inactivated fetal bovine serum, 2 mM L-Glutamine and, where indicated, with 100U/ml polyethylene glycol-Catalase (PEG-Cat), to block intracellular Reactive Oxygen Species (ROS).
Intracellular BCG-lux growth was assessed by luminometric assay, as described (16, 17). Briefly, after 3 day of co-culture, cells were lysed by adding 0.1% saponin directly into the well and cells were incubated at 37°C for 30 min. Luminometric analysis was performed using PBS, mycobacterial suspension and 1% decanal (used as luciferase substrate), at the ratio 8:1:1 in a 24-well white plate. Data are expressed as means ± standard error (SEM) of Relative Luminescence Units (RLU) values of triplicate cultures, obtained 72 hours post infection, and as replication index obtained as the ratio between the single values of culture triplicate at 72 hours and the mean of the values obtained immediately after 3 hours infection. Luminescence has been evaluated by Varioskan LUX Multimode Microplate reader (Thermo Fisher Scientific).
Intracellular BCG and H37Rv growth were assessed by Colony Forming Units (CFU) assay. Briefly, BCG infected cells were lysed, as described above, and samples were diluted in PBS-Tween80 0.05% and plated in triplicate. H37Rv infected cells were lysed in 0.1% Triton-X100 (Sigma-Aldrich, St. Louis, MO) and intracellular bacteria determined by CFU counting by serially diluting lysates in PBS containing Tween80 (0.05%) and plating on 7H11/OADC agar plates. After 21 days, CFU were enumerated.
MTT Assay
In order to analyze the possible alteration of cell viability after BCG infection, the MTT (Molecular Probe) assay was executed. Briefly, monocytes, seeded in 96-well plate (1.25 x 105/well), were infected with BCG (MOI 5) as described above. After 3 days of co-cultured with 1.25 x 105 uninfected purified PMN-MDSC or monocytes, MTT assay was performed, according to the manufacturer’s instructions. Infected cells were treated or not with 0.1% saponin at 37°C for 30 min and used as a negative and positive control, respectively. The concentration of solubilized formazan was determined by optical density at 540 nm, and measured by using Varioskan LUX Multimode Microplate reader (Thermo Fisher Scientific).
Statistical Analysis
GraphPad Prism version 4.00 for Windows (GraphPad Software) has been used to carry out the statistical analyses. The non-parametric Kruskal-Wallis with Dunn’s posttest, or Mann-Whitney test have been used to compare continuous variables. If appropriate, a paired T test was also used and p <0.05 was considered statistically significant. Correlations were evaluated with the non-parametric Spearman test. To evaluate the accuracy of PMN-MDSC frequency as candidate biomarker in discriminating between patients with active disease and LTBI subjects, a receiver operating characteristic (ROC) curve analysis was performed.
Results
PMN-MDSC Frequency Associated With Active TB
We firstly evaluated the frequency of MDSC in patients with active TB, LTBI and healthy donors (HD). As previously demonstrated (8), PMN-MDSC frequency in TB patients was significantly higher than LTBI and HD (Figures 1A, B). Moreover, the percentage of PMN-MDSC in LTBI individuals was comparable with HD (Figure 1B). We did not detect monocytic-MDSC in TB patients, LTBI, or in HD. We also found no correlation between PMN-MDSC frequency and number of days from therapy initiation (data not shown).
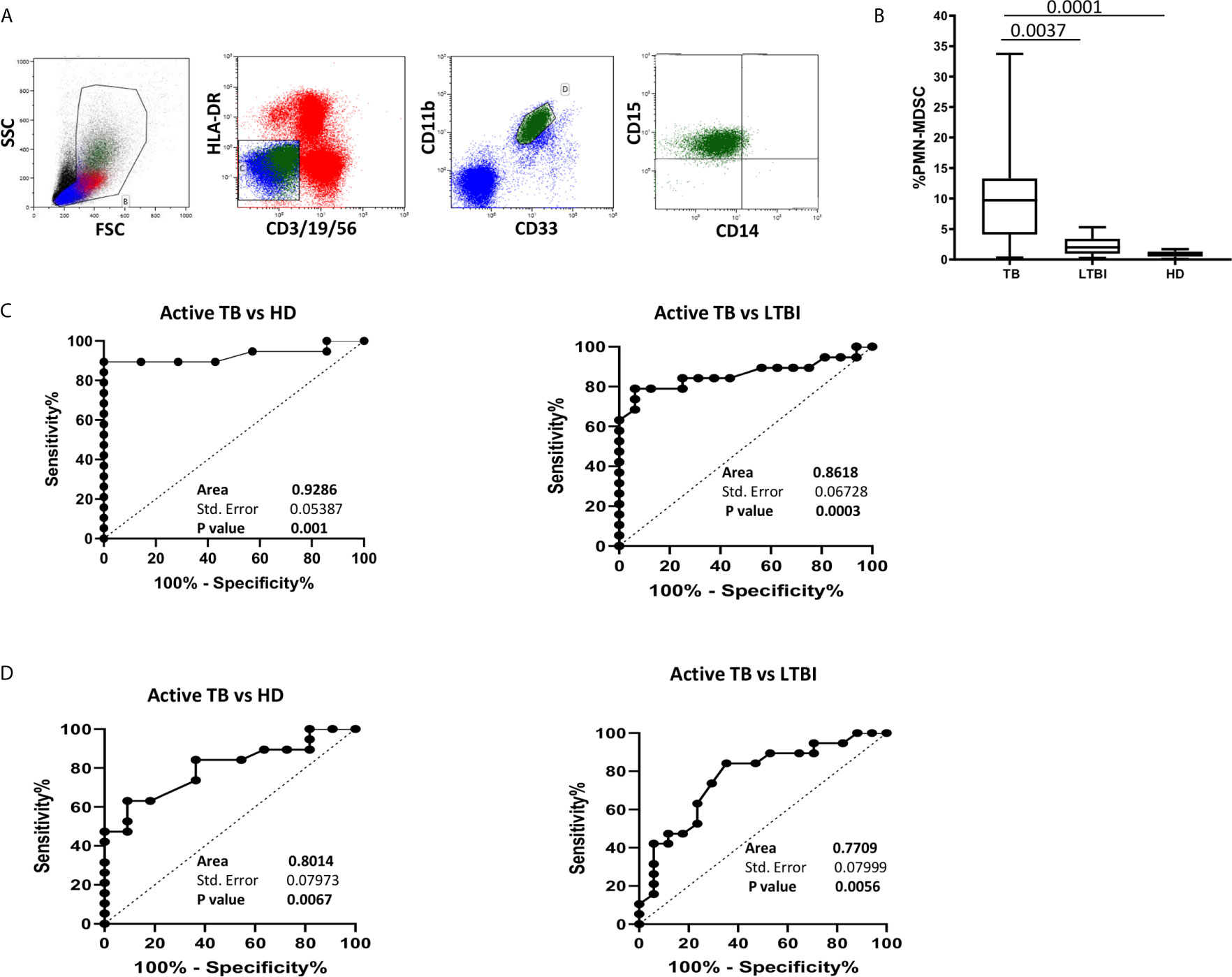
Figure 1 PMN-MDSC discriminate active TB and LTBI. (A) Gating strategy used to identify MDSC: in the morphological gate (FSC/SSC) we excluded debris, then we gated Lin-/HLA DRlow/- cells. In this gate, we selected CD11b+CD33+ cells (MDSC). Then, the expression of CD14, CD15 is shown. The percentage of Lin-/HLA-DR-/CD11b+/CD33+ has been calculated in the morphological gate. (B) PMN-MDSC frequency in active TB (18), LTBI (19) and HD (12). Results are shown as Box and Whiskers. The Kruskall-Wallis test (with Dunn’s multiple comparison posttest) was applied. (C) Receiver operating characteristic (ROC) curve for the PMN-MDSC frequency. Right panel TB vs HD, left panel TB vs LTBI. (D) Receiver operating characteristic (ROC) curve for the Mo/Ly ratio. Right panel TB vs HD, left panel TB vs LTBI. PMN-MDSC: polymorphonuclear-myeloid-derived suppressor cells; TB, tuberculosis; LTBI, latent tuberculosis infection; HD, healthy donors.
The results from the ROC analysis indicated that the frequency value of this cell population discriminated between LTBI and active TB (AUC: 0.86, p = 0.0003) identifying a cut off value of 4% (78.95% and 93.75% of sensibility and specificity respectively). PMN-MDSC frequency also distinguished active TB from HD (AUC: 0.93, p = 0.001) (Figure 1C).
It has been recently shown that the monocytes/lymphocytes ratio (Mo/Ly ratio) is associated with active TB (18, 19). We than calculated the ROC curves for Mo/Ly ratio in the same patients’ group and found that, although the Mo/Ly ratio discriminated between LTBI and active TB (AUC: 0.77, p = 0.0056), the PMN-MDSC frequency detection was a more robust tool (Figure 1D).
PMN-MDSC Frequency Correlate With Monocyte Percentage and Number
Since an increase of monocytes and monocyte/lymphocyte (Mo/Ly) ratio have been reported during active TB, we wondered whether a crosstalk between MDSC and monocytes occurred during TB. We firstly confirmed a higher percentage and number of circulating monocytes and Mo/Ly ratio in our setting (Figure 2A). Moreover, we found a direct correlation between PMN-MDSC frequency and the monocytes proportion and absolute number, and with the Mo/Ly ratio (Figure 2B). We then analyzed the circulating monocyte subsets by evaluating the expression of CD14 and CD16 molecules (Figure 3A). A direct correlation between PMN-MDSC and CD14++CD16- monocytes (Figure 3B) was found, suggesting a possible interplay among these myeloid cell populations. We did not find significant correlations with non-classical CD14-CD16+ and intermediate CD14+CD16+ monocytes (Figures 3C, D).
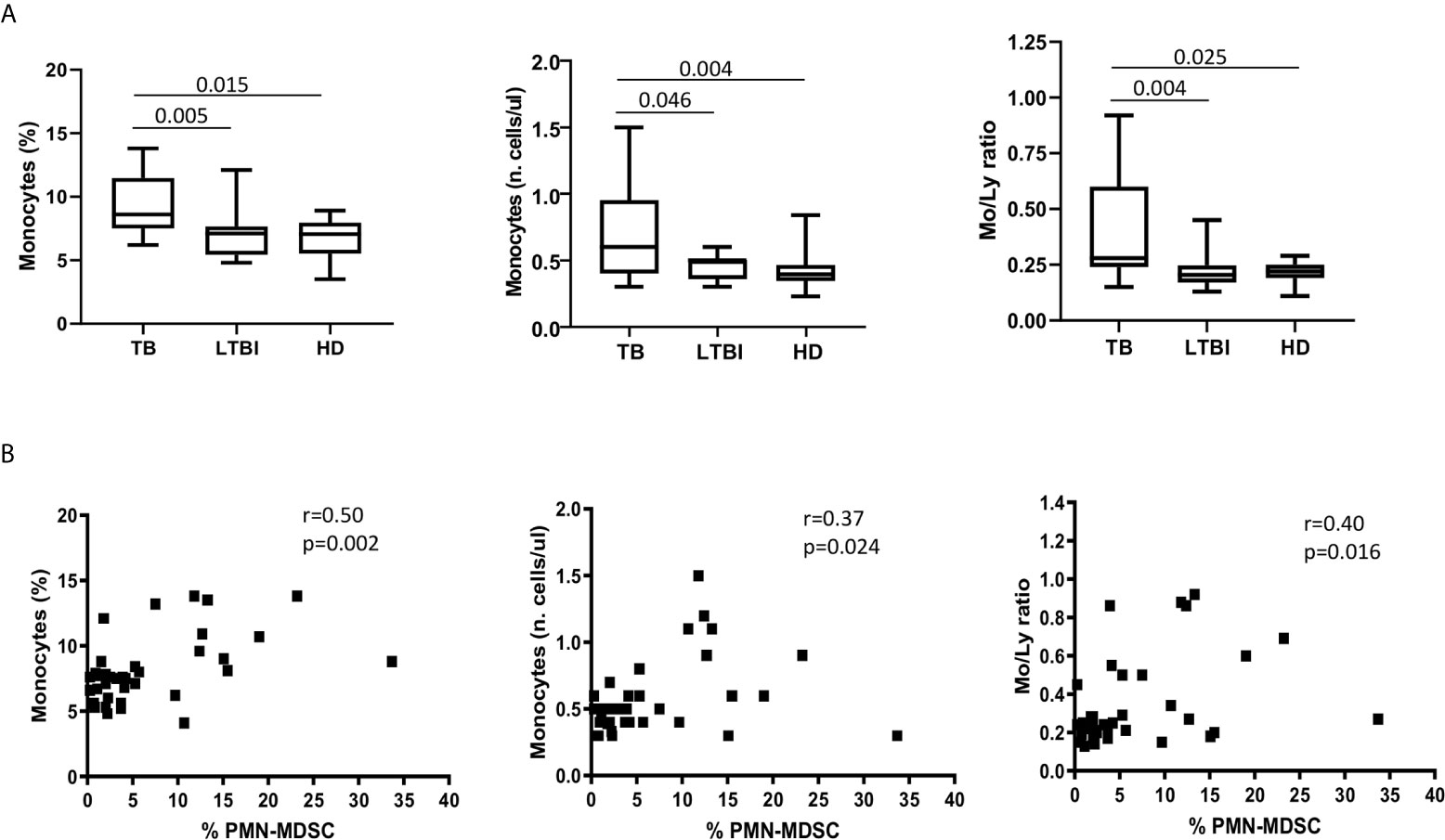
Figure 2 PMN-MDSC correlates with monocyte percentage and number, and with Mo/Ly ratio. (A) The monocytes percentage and absolute number, and Mo/Ly ration in active TB (18), LTBI (19), and HD (12). Results are shown as Box and Whiskers. The Kruskall-Wallis test (with Dunn’s multiple comparison posttest) was applied. (B) Correlation between PMN-MDSC frequency and percentage and number of monocytes, and with Mo/Ly ratio from TB (18) and LTBI (19) individuals. The Spearman test was applied to evaluate correlations. The p <0.05 was considered statistically significant. TB, tuberculosis; LTBI, latent tuberculosis infection; HD, healthy donors.
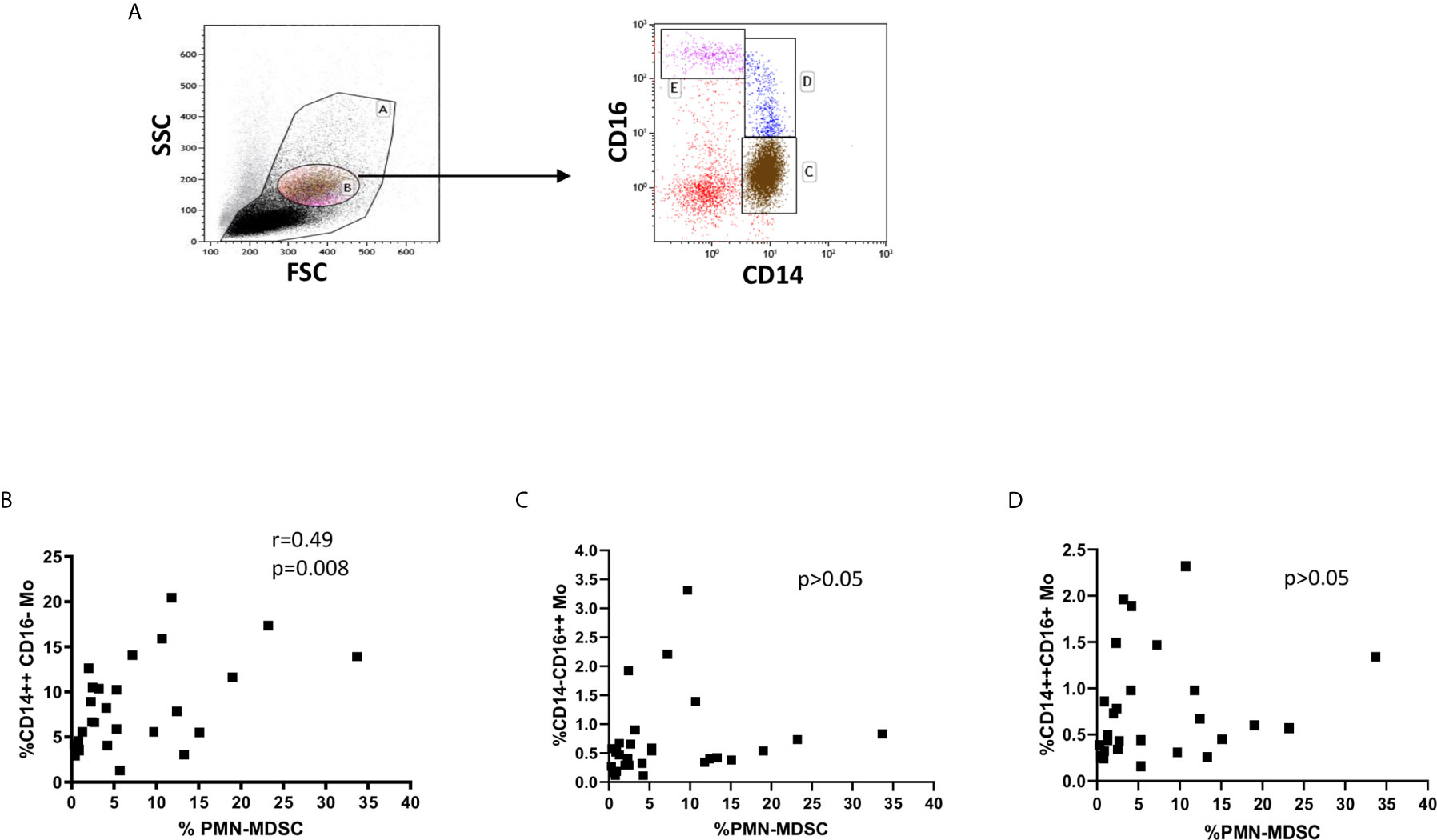
Figure 3 PMN-MDSC frequency correlates with the CD14++CD16- monocyte proportion. (A) Gating strategy used to identify monocyte subsets: in the morphological gate (FSC/SSC) we excluded debris gate (A), and morphologically identified monocytes gate (B). In gate B, we selected CD14++CD16, CD14++CD16+, and CD14-CD16++ monocytes. The percentage of monocyte subsets was calculated in the gate (A) Correlation between PMN-MDSC frequency and CD14++CD16 (B), CD14++CD16+ (C), and CD14-CD16++ (D) monocytes from active TB and LTBI subjects. The Spearman test was applied to evaluate correlations. The p <0.05 was considered statistically significant.
PMN-MDSC Are Associated With a Low Disease Severity
The role of MDSC during human tuberculosis is not clear. To understand whether in vivo PMN-MDSC might associate with the disease severity, we evaluated the severity radiological score. Three out of 19 TB patients have a score 1, 4 a score 2, and 12 a score 3. Due to the limited number of patients, we grouped patients with a radiological score 1 and 2 in the same group (group A). Therefore, we analyzed the proportion of PMN-MDSC in 2 groups, based on the radiological severity of the patients, group A (radiological score 1 and 2) and group B (radiological score 3). Interestingly, we found that those with a mild/moderate radiological TB had an almost significant higher PMN-MDSC proportion than those with a more severe radiological TB (Figure 4), indicating that PMN-MDSC may play a beneficial role during active TB.
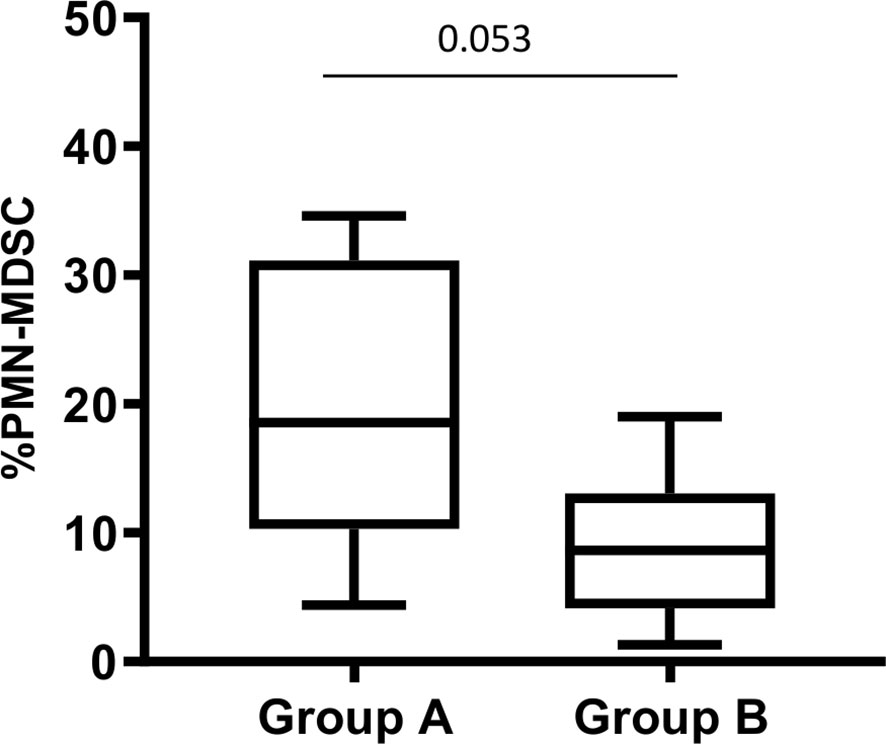
Figure 4 PMN-MDSC frequency is associated with chest X-ray severity score. PMN-MDSC frequency in group A (score 1 and 2) and B (score 3) stratified based on the chest X-ray severity score. The Mann-Whitney test was applied, and the p <0.05 was considered significant. PMN-MDSC, polymorphonuclear-myeloid-derived suppressor cells.
PMN-MDSC Induce a ROS-Mediated BCG but Not MTB Inhibition by Monocytes
Since we observed a correlation between PMN-MDSC and CD14++CD16- monocytes frequencies, and found a higher PMN-MDSC frequency in patients with low severity score, we evaluated whether PMN-MDSC may affect the capacity of monocytes to control the mycobacterial replication. To this aim, we purified monocytes and CD15+ PMN-MDSC from PBMC of healthy donors, and verified the immune suppressive ability of the isolated PMN-MDSC by evaluating their impact on the proliferation of CD3 T cells upon stimulation with SEB (Supplementary Figure 1). We then infected purified monocytes with BCGlux (MOI 1:5) and co-cultured with purified syngeneic PMN-MDSC. Intracellular BCGlux replication was evaluated after 3 days from infection by luminometric analysis. We found that addition of PMN-MDSC inhibited the BCG replication in monocytes (Figures 5A, B). Similar results were obtained by using wild type BCG and by enumerating colony-forming units (CFU) (Figure 5D). As previously reported in the mouse model, we found that human PMN-MDSC are susceptible to BCG infection and sustain BCG replication as monocytes (Figures 5A, B). To evaluate whether PMN-MDSC induced a decrease of BCG-infected monocytes viability, after 72 hours of culture we performed a MTT assay and found that PMN-MDSC did not alter the viability of infected monocytes (Supplementary Figure 2).
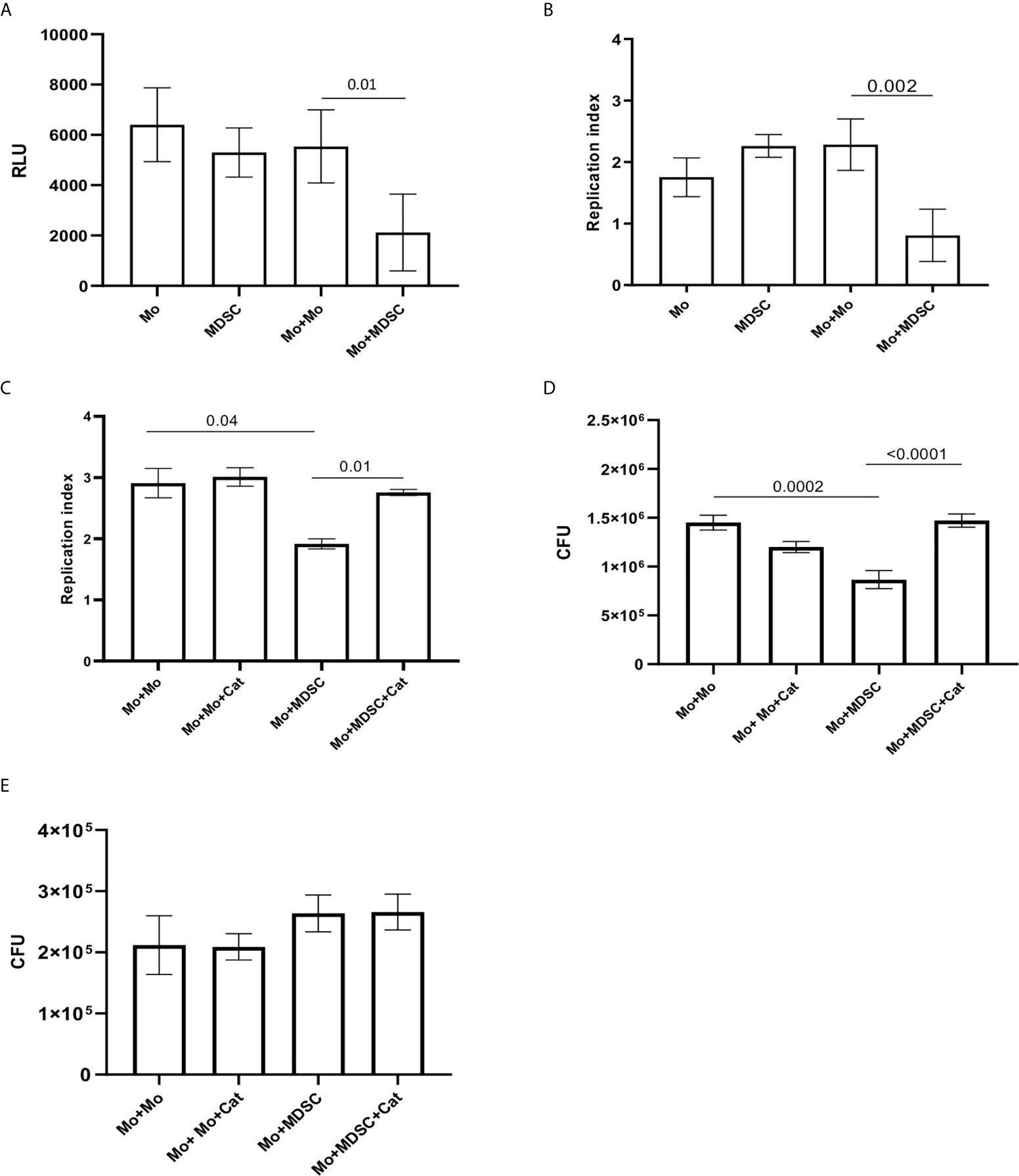
Figure 5 PMN-MDSC induce BCG and not MTB growth inhibition into monocytes. Relative Luminescence Unit (A) and Replication index (B) of BCGlux 3 days post infection into purified monocytes alone (Mo) or cultured with not infected purified PMN-MDSC (ratio 1/1, Mo+MDSC), or with not infected monocytes as control (ratio 1/1, Mo+Mo). PMN-MDSC infected with BCGlux alone was also evaluate (MDSC). Results are shown as mean ± SE of four independent experiments. (C) Replication index of BCGlux after 3 days of infection of purified monocytes (Mo) cultured with not infected purified PMN-MDSC (ratio 1/1, Mo+MDSC), or with not infected monocytes as control (ratio 1/1, Mo+Mo) in the presence or absence of polyethylene glycol-Catalase (Cat). Results are show as mean ± SE of replicates of one experiment (cells from one donor). (D) Colony Forming Unit (CFU) of wild type BCG after 3 days of infection of purified monocytes (Mo) cultured with not infected purified PMN-MDSC (ratio 1/1, Mo+MDSC), or with not infected monocytes as control (ratio 1/1, Mo+Mo) in the presence or absence of polyethylene glycol-Catalase (Cat). Results are show as mean ± SE of replicates of one out of two independent experiments (cells from two donors). A paired T test was used, and p <0.05 was considered statistically significant. Mo, monocytes; PMN-MDSC, polymorphonuclear-myeloid-derived suppressor cells. (E) Colony Forming Unit (CFU) of MTB (H37Rv) after 3 days of infection of purified monocytes (Mo) cultured with not infected purified PMN-MDSC (ratio 1/1, Mo+MDSC), or with not infected monocytes as control (ratio 1/1, Mo+Mo) in the presence or absence of polyethylene glycol-Catalase (Cat). Results are show as mean ± SE of three independent experiments.
Reactive Oxygen Species (ROS) play a pivotal role in the control of mycobacteria replication in macrophages (20, 21). We found that the addition of an inhibitor of ROS (catalase) increased both BCGlux (Figure 5C) and BCG wild type (Figure 5D) replication into monocytes cultured with PMN-MDSC. Differently, catalase was not able to modulate BCG replication when PMN-MDSC were not present (Figures 5C, D). We then asked whether PMN-MDSC were able to reduce also MTB growth in the infected monocytes. Differently from BCG, PMN-MDSC were not able to affect the capacity of monocyte to restrain MTB replication; accordingly, no effect was observed upon catalase treatment (Figure 5E).
Discussion
The dogma of the binary nature of TB infection (active TB vs LTBI) is an oversimplified concept. Indeed, the course of TB is extremely variable in humans. Once infection is established, most people control, but do not eliminate the infection (LTBI), while others are unable to restrict MTB replication and active disease develops. Active TB is variable as well: pulmonary TB can present with mild, moderate, or severe respiratory and systemic symptoms, with involvement of single or multiple lung lobes. Cavitary TB is present in some, but not all, individuals with pulmonary TB. Thus, the identification of mechanisms associated with protection from infection or progression to disease is crucial to develop efficient diagnostic and therapeutic tools to improve the management of TB patients (22, 23). In the present study, we investigated the role of MDSC during TB and the possible use of their frequency as biomarker of disease stratification. As previously reported, PMN-MDSC frequency significantly increases during active TB, while no expansion was observed in LTBI. Indeed, we found an association between high PMN-MDSC frequency and active TB. Some reports showed a higher frequency of Mo-MDSC during TB (7, 24, 25). Differently, we did not detect Mo-MDSC in our patients. This discrepancy could be explained by the use of cryopreserved PBMC in the previous papers. Cryopreservation strongly affect PMN-MDSC (26), possibly altering the proportion of MDSC subsets.
IGRA are, with the TST, the only tools available for the diagnosis of LTBI. However, these assays are very limited in their capacity to discriminate LTBI and active TB, in the monitoring of treatment response, and in the capacity to identify LTBI patients who will progress to active TB (27, 28). Several markers have been investigated as biomarker of active TB. IFN-γ-and IP-10 were reported to be increased in the unstimulated plasma of children and adults with active TB (29, 30). Effector T cells phenotype expansion was observed during active TB, whereas memory T-cells are associated with infection control (31–33). The Mo/Ly ratio was also investigated as marker of TB infection progression, demonstrating its association with the active phase of the disease (18, 34). Our data indicate that PMN-MDSC frequency may be used to discriminate active TB and LTBI, even with a better accuracy than the Mo/Ly ratio. It has been previously demonstrated that PMN-MDSC drastically decreased in cured TB patients, indicating that this could be a good biomarker of treatment response (22, 23). Differently from other markers such as IFN-γ, once MTB is eradicated, MTB-induced stimuli that mediate PMN-MDSC expansion decrease, thus inducing a contraction of these suppressive cell population. Since the limited number of patients included in this work, a larger study is needed to validate the PMN-MDSC frequency as new marker of active TB. Interestingly, a high PMN-MDSC frequency was observed in active TB patients with a low/mild severity score, suggesting that PMN-MDSC could exert a beneficial role in controlling the inflammation-induced tissue damage in human active TB. The suppressive capacity of PMN-MDSC on T cell response has been clearly demonstrated during TB (35). However, it has been recently demonstrated that, in a murine model of BCG-induced pleurisy, the interaction of transmembrane TNF and TNFR2 plays a critical role for MDSC suppressive activity on T cells, which allows attenuation of the inflammation within the pleural cavity (36). Immune-mediated tissue injury may be more detrimental than the pathogen itself. Therefore, mechanisms to counter regulate pro-inflammatory immune cells and prevent the harmful effects of excessive inflammation are pivotal.
In a murine model of tuberculosis, the accumulation of Gr-1dimCD11b+ cells was associated with fatal TB infection (37, 38), and a detrimental role of MDSC was shown (14). However, the discrepancy between our and previous works could be explained by the different TB pathology between humans and mice (39), as the absence of caseation and lung cavitation in the animal model (40).
Monocytes/macrophages represent one of the targets of MTB infection and play a central role in containing MTB replication. MDSC may modulate the polarization of monocytes (41), but their ability to affect the monocyte bactericidal ability was not still explored. We found that in vitro PMN-MDSC may augment BCG killing activity of monocytes, possibly mediate by ROS production. Indeed, ROS are capable to induce microtubule associated protein 1A/1B-LC3 translocation to the phagosome, enhancing phagosome maturation (21, 42). Moreover, it has been demonstrated that ROS are able to induce autophagy (43), which represents a defense mechanism inhibiting BCG and Mycobacterium tuberculosis survival (44). ROS production is mediated by NADPH oxidases, which was demonstrated to be increased by TGF-beta in different cellular systems (45, 46). TGF-beta is highly expressed by MDSC, and could involve in the ROS-mediate BCG replication control by monocytes. Differently from BCG, PMN-MDSC did not induce MTB growth inhibition by monocytes. This difference may be due to the ability of MTB in subverting the macrophage’s mechanisms of intracellular killing, in particular, to inhibit ROS production (47).
Therefore, these data suggest that the possible PMN-MDSC beneficial role observed in patients could be not significantly associated with their mycobactericidal activity but rather with their ability to modify the lung microenvironment. The in vitro infection is a very simplified system that does not consider the lung complexity and the inflammatory microenvironment. Moreover, in our in vitro experiments, monocytes and PMN-MDSC were isolated from healthy donors. Two-signal model has been described for the differentiation of MDSC. This model includes 2 phases: the expansion of immature myeloid cells, and the activation phase converting immature myeloid cells into suppressive MDSC (48). We showed that PMN-MDSC we purified from healthy donors had suppressive function, thus belonging to the MDSC cell population; however, we cannot exclude that cells from TB patients could have different behavior. We did not find any correlation with PMN-MDSC frequency and the number of acid-alcohol resistant bacilli (data not shown), corroborating the hypothesis that PMN-MDSC could limit the inflammation-induced lung damage rather than MTB replication.
We found a correlation between circulating PMN-MDSC and CD14++ monocytes frequency, and in particular with the classical CD14++CD16- subset. The activated monocytes have a potent anti-bacterial capacity; in particular, it has been shown that classical CD14++CD16- monocytes are involved in the protection against TB (49). Whether PMN-MDSC expansion, by limiting excessive inflammation, may favor the classical monocyte differentiation and functions needs to be investigated.
In conclusion, our data indicate that elevated frequency of PMN-MDSC in IGRA-positive individuals is associated with active TB. Our findings also point out a beneficial role of PMN-MDSC during human active TB most likely exerted by controlling the inflammation level, by limiting T cell response, thus counteracting tissue damage. Wider studies are mandatory to confirm the efficacy of circulating PMN-MDSC as a biomarker of active TB, and to deeply dissect their contribution in the balance between protection and pathogenesis of the human TB disease.
Data Availability Statement
The original contributions presented in the study are included in the article/Supplementary Material. Further inquiries can be directed to the corresponding author.
Ethics Statement
The studies involving human participants were reviewed and approved by INMI Ethical Committee (approval number 72/2015). Istituto Nazionale per le Malattie Infettive “Lazzaro Spallanzani” IRCCS. The patients/participants provided their written informed consent to participate in this study.
Author Contributions
Conceptualization: AS, CA. Experimental design: AS, CA, MF. Flow cytometry analysis: GGr, VB, EC, RC. Functional experiments: GGr, AR, AA, FS, SN. Statistical analysis: GGr, AS. Patient management: FP, DG, GGu, SM. Patients categorization: DG, GC. Writing the paper: AS, GGr, CA. All authors contributed to the article and approved the submitted version.
Funding
The study was supported by Italian Ministry of Heath, Ricerca Corrente, and by Fondazione Italiana Fibrosi Cistica 2019. The funders had no role in study design, data collection, and analysis, decision to publish, or preparation of the manuscript.
Conflict of Interest
The authors declare that the research was conducted in the absence of any commercial or financial relationships that could be construed as a potential conflict of interest.
Acknowledgments
We thank E. Ercoli and all the nursing staff and socio-health operators of the Respiratory Infectious Diseases Unit, who provided expertise and care for patients.
Supplementary Material
The Supplementary Material for this article can be found online at: https://www.frontiersin.org/articles/10.3389/fimmu.2021.594376/full#supplementary-material
Supplementary Figure 1 | PMN-MDSC purified from HD inhibit T cell proliferation. PBMC labeled with CFDA-SE were stimulated with SEB in the presence of PMN-MDSC (1:1 ratio). After 5 days, CD3+ T cells proliferation was evaluated by flow cytometry. Representative gating strategy and histogram plots of one out of 3 independent experiments CD3+ T cells proliferation in the indicated conditions. MDSC: myeloid-derived suppressor cells; SEB, Staphylococcus Enterotoxin; PBMC, Peripheral blood mononuclear cells.
Supplementary Figure 2 | PMN-MDSC did not alter BCG-infected monocyte viability. BCG-infected monocytes (1.25x105) were cultured with uninfected purified PMN-MDSC (at the ratio 1:1, Mo+MDSC) or with uninfected monocytes (ratio 1:1, Mo+Mo). After 3 days of culture, MTT assay was performed. Negative control consisted of infected cells treated with 0.1% saponin at 37˚C for 30 min. Results are shown as means ± SE of OD 540nm values of quadruplicate cultures. Data are representative of 2 independent experiments performed on cells from different donors. Mo, monocytes; PMN-MDSC, polymorphonuclear-myeloid-derived suppressor cells; Sap, saponin.
References
1. van Pinxteren LA, Cassidy JP, Smedegaard BH, Agger EM, Andersen P. Control of Latent Mycobacterium Tuberculosis Infection is Dependent on CD8 T Cells. Eur J Immunol (2000) 30:3689–98. doi: 10.1002/1521-4141(200012)30:123.0.CO;2-4
2. Canaday DH, Wilkinson RJ, Li Q, Harding CV, Silver RF, Boom WH. CD4(+) and CD8(+) T Cells Kill Intracellular Mycobacterium Tuberculosis by a Perforin and Fas/Fas Ligand-Independent Mechanism. J Immunol (2001) 167:2734–42. doi: 10.4049/jimmunol.167.5.2734
3. Cooper AM. Cell-Mediated Immune Responses in Tuberculosis. Annu Rev Immunol (2009) 27:393–422. doi: 10.1146/annurev.immunol.021908.132703
4. Chiacchio T, Casetti R, Butera O, Vanini V, Carrara S, Girardi E, et al. Characterization of Regulatory T Cells Identified as CD4(+)CD25(High)CD39(+) in Patients With Active Tuberculosis. Clin Exp Immunol (2009) 156:463–70. doi: 10.1111/j.1365-2249.2009.03908.x
5. Scott-Browne JP, Shafiani S, Tucker-Heard G, Ishida-Tsubota K, Fontenot JD, Rudensky AY, et al. Expansion and Function of Foxp3-Expressing T Regulatory Cells During Tuberculosis. J Exp Med (2007) 204:2159–69. doi: 10.1084/jem.20062105
6. Kursar M, Koch M, Mittrucker HW, Nouailles G, Bonhagen K, Kamradt T, et al. Cutting Edge: Regulatory T Cells Prevent Efficient Clearance of Mycobacterium Tuberculosis. J Immunol (2007) 178:2661–5. doi: 10.4049/jimmunol.178.5.2661
7. du Plessis N, Loebenberg L, Kriel M, von Groote-Bidlingmaier F, Ribechini E, Loxton AG, et al. Increased Frequency of Myeloid-Derived Suppressor Cells During Active Tuberculosis and After Recent Mycobacterium Tuberculosis Infection Suppresses T-Cell Function. Am J Respir Crit Care Med (2013) 188:724–32. doi: 10.1164/rccm.201302-0249OC
8. El Daker S, Sacchi A, Tempestilli M, Carducci C, Goletti D, Vanini V, et al. Granulocytic Myeloid Derived Suppressor Cells Expansion During Active Pulmonary Tuberculosis is Associated With High Nitric Oxide Plasma Level. PLoS One (2015) 10:e0123772. doi: 10.1371/journal.pone.0123772
9. Bronte V, Brandau S, Chen SH, Colombo MP, Frey AB, Greten TF, et al. Recommendations for Myeloid-Derived Suppressor Cell Nomenclature and Characterization Standards. Nat Commun (2016) 7:12150. doi: 10.1038/ncomms12150
10. Solito S, Falisi E, Diaz-Montero CM, Doni A, Pinton L, Rosato A, et al. A Human Promyelocytic-Like Population is Responsible for the Immune Suppression Mediated by Myeloid-Derived Suppressor Cells. Blood (2011) 118:2254–65. doi: 10.1182/blood-2010-12-325753
11. Rodriguez PC, Hernandez CP, Quiceno D, Dubinett SM, Zabaleta J, Ochoa JB, et al. Arginase I in Myeloid Suppressor Cells is Induced by COX-2 in Lung Carcinoma. J Exp Med (2005) 202:931–9. doi: 10.1084/jem.20050715
12. Lee BR, Chang SY, Hong EH, Kwon BE, Kim HM, Kim YJ, et al. Elevated Endoplasmic Reticulum Stress Reinforced Immunosuppression in the Tumor Microenvironment Via Myeloid-Derived Suppressor Cells. Oncotarget (2014) 5:12331–45. doi: 10.18632/oncotarget.2589
13. Li H, Han Y, Guo Q, Zhang M, Cao X. Cancer-Expanded Myeloid-Derived Suppressor Cells Induce Anergy of NK Cells Through Membrane-Bound TGF-Beta 1. J Immunol (2009) 182:240–9. doi: 10.4049/jimmunol.182.1.240
14. Knaul JK, Jörg S, Oberbeck-Mueller D, Heinemann E, Scheuermann L, Brinkmann V, et al. Lung-Residing Myeloid-Derived Suppressors Display Dual Functionality in Murine Pulmonary Tuberculosis. Am J Respir Crit Care Med (2014) 190:1053–66. doi: 10.1164/rccm.201405-0828OC
15. De Santis F, Poerio N, Gismondi A, Nanni V, Di Marco G, Nisini R, et al. Hydroalcoholic Extract From Origanum Vulgare Induces a Combined Anti-Mycobacterial and Anti-Inflammatory Response in Innate Immune Cells. PLoS One (2019) 14:e0213150. doi: 10.1371/journal.pone.0213150
16. Poerio N, Bugli F, Taus F, Santucci MB, Rodolfo C, Cecconi F, et al. Liposomes Loaded With Bioactive Lipids Enhance Antibacterial Innate Immunity Irrespective of Drug Resistance. Sci Rep (2017) 7:45120. doi: 10.1038/srep45120
17. Kampmann B, Tena GN, Mzazi S, Eley B, Young DB, Levin M. Novel Human In Vitro System for Evaluating Antimycobacterial Vaccines. Infect Immun (2004) 72:6401–7. doi: 10.1128/IAI.72.11.6401-6407.2004
18. La Manna MP, Orlando V, Dieli F, Di Carlo P, Cascio A, Cuzzi G, et al. Quantitative and Qualitative Profiles of Circulating Monocytes may Help Identifying Tuberculosis Infection and Disease Stages. PLoS One (2017) 12:e0171358. doi: 10.1371/journal.pone.0171358
19. Naranbhai V, Hill AV, Abdool Karim SS, Naidoo K, Abdool Karim Q, Warimwe GM, et al. Ratio of Monocytes to Lymphocytes in Peripheral Blood Identifies Adults At Risk of Incident Tuberculosis Among HIV-Infected Adults Initiating Antiretroviral Therapy. J Infect Dis (2014) 209:500–9. doi: 10.1093/infdis/jit494
20. Roca FJ, Ramakrishnan L. TNF Dually Mediates Resistance and Susceptibility to Mycobacteria Via Mitochondrial Reactive Oxygen Species. Cell (2013) 153:521–34. doi: 10.1016/j.cell.2013.03.022
21. Martinez J, Malireddi RK, Lu Q, Cunha LD, Pelletier S, Gingras S, et al. Molecular Characterization of LC3-Associated Phagocytosis Reveals Distinct Roles for Rubicon, NOX2 and Autophagy Proteins. Nat Cell Biol (2015) 17:893–906. doi: 10.1038/ncb3192
22. Goletti D, Lindestam Arlehamn CS, Scriba TJ, Anthony R, Cirillo DM, Alonzi T, et al. Can We Predict Tuberculosis Cure? What Tools are Available? Eur Respir J (2018) 52:1801089. doi: 10.1183/13993003.01089-2018
23. Goletti D, Lee MR, Wang JY, Walter N, Ottenhoff THM. Update on Tuberculosis Biomarkers: From Correlates of Risk, to Correlates of Active Disease and of Cure From Disease. Respirology (2018) 23:455–66. doi: 10.1111/resp.13272
24. Du Plessis N, Jacobs R, Gutschmidt A, Fang Z, van Helden PD, Lutz MB, et al. Phenotypically Resembling Myeloid Derived Suppressor Cells are Increased in Children With HIV and Exposed/Infected With Mycobacterium Tuberculosis. Eur J Immunol (2017) 47:107–18. doi: 10.1002/eji.201646658
25. Jontvedt Jorgensen M, Jenum S, Tonby K, Mortensen R, Walzl G, Du Plessis N, et al. Monocytic Myeloid-Derived Suppressor Cells Reflect Tuberculosis Severity and are Influenced by Cyclooxygenase-2 Inhibitors. J Leukoc Biol (2020) 32:1–10. doi: 10.1002/JLB.4A0720-409RR
26. Kotsakis A, Harasymczuk M, Schilling B, Georgoulias V, Argiris A, Whiteside TL. Myeloid-Derived Suppressor Cell Measurements in Fresh and Cryopreserved Blood Samples. J Immunol Methods (2012) 38:14–22. doi: 10.1016/j.jim.2012.04.004
27. Sester M, Sotgiu G, Lange C, Giehl C, Girardi E, Migliori GB, et al. Interferon-Gamma Release Assays for the Diagnosis of Active Tuberculosis: A Systematic Review and Meta-Analysis. Eur Respir J (2011) 37:100–11. doi: 10.1183/09031936.00114810
28. Esmail H, Cobelens F, Goletti D. Transcriptional Biomarkers for Predicting Development of Tuberculosis: Progress and Clinical Considerations. Eur Respir J (2020) 55:1901957. doi: 10.1183/13993003.01957-2019
29. Cannas A, Calvo L, Chiacchio T, Cuzzi G, Vanini V, Lauria FN, et al. IP-10 Detection in Urine is Associated With Lung Diseases. BMC Infect Dis (2010) 10:333–2334-10-333. doi: 10.1186/1471-2334-10-333
30. Azzurri A, Sow OY, Amedei A, Bah B, Diallo S, Peri G, et al. IFN-Gamma-Inducible Protein 10 and Pentraxin 3 Plasma Levels are Tools for Monitoring Inflammation and Disease Activity in Mycobacterium Tuberculosis Infection. Microbes Infect (2005) 7:1–8. doi: 10.1016/j.micinf.2004.09.004
31. Petruccioli E, Petrone L, Vanini V, Sampaolesi A, Gualano G, Girardi E, et al. Ifngamma/Tnfalpha Specific-Cells and Effector Memory Phenotype Associate With Active Tuberculosis. J Infect (2013) 66:475–86. doi: 10.1016/j.jinf.2013.02.004
32. Pollock KM, Whitworth HS, Montamat-Sicotte DJ, Grass L, Cooke GS, Kapembwa MS, et al. T-Cell Immunophenotyping Distinguishes Active From Latent Tuberculosis. J Infect Dis (2013) 208:952–68. doi: 10.1093/infdis/jit265
33. Goletti D, Butera O, Bizzoni F, Casetti R, Girardi E, Poccia F. Region of Difference 1 Antigen-Specific CD4+ Memory T Cells Correlate With a Favorable Outcome of Tuberculosis. J Infect Dis (2006) 194:984–92. doi: 10.1086/507427
34. Naranbhai V, Fletcher HA, Tanner R, O’Shea MK, McShane H, Fairfax BP, et al. Distinct Transcriptional and Anti-Mycobacterial Profiles of Peripheral Blood Monocytes Dependent on the Ratio of Monocytes: Lymphocytes. EBioMedicine (2015) 2:1619–26. doi: 10.1016/j.ebiom.2015.09.027
35. Hmama Z, Peña-Díaz S, Joseph S, Av-Gay Y. Immunoevasion and Immunosuppression of the Macrophage by Mycobacterium Tuberculosis. Immunol Rev (2015) 264:220–32. doi: 10.1111/imr.12268
36. Magcwebeba T, Dorhoi A, du Plessis N. The Emerging Role of Myeloid-Derived Suppressor Cells in Tuberculosis. Front Immunol (2019) 10:917. doi: 10.3389/fimmu.2019.00917
37. Tsiganov EN, Verbina EM, Radaeva TV, Sosunov VV, Kosmiadi GA, Nikitina IY, et al. Gr-1dimcd11b+ Immature Myeloid-Derived Suppressor Cells But Not Neutrophils are Markers of Lethal Tuberculosis Infection in Mice. J Immunol (2014) 192:4718–27. doi: 10.4049/jimmunol.1301365
38. Lyadova IV, Tsiganov EN, Kapina MA, Shepelkova GS, Sosunov VV, Radaeva TV, et al. In Mice, Tuberculosis Progression is Associated With Intensive Inflammatory Response and the Accumulation of Gr-1 Cells in the Lungs. PLoS One (2010) 5:e10469. doi: 10.1371/journal.pone.0010469
39. Apt A, Kramnik I. Man and Mouse TB: Contradictions and Solutions. Tuberc (Edinb) (2009) 89:195–8. doi: 10.1016/j.tube.2009.02.002
40. Ordonez AA, Tasneen R, Pokkali S, Xu Z, Converse PJ, Klunk MH, et al. Mouse Model of Pulmonary Cavitary Tuberculosis and Expression of Matrix Metalloproteinase-9. Dis Model Mech (2016) 9:779–88. doi: 10.1242/dmm.025643
41. Sinha P, Clements VK, Bunt SK, Albelda SM, Ostrand-Rosenberg S. Cross-Talk Between Myeloid-Derived Suppressor Cells and Macrophages Subverts Tumor Immunity Toward a Type 2 Response. J Immunol (2007) 179:977–83. doi: 10.4049/jimmunol.179.2.977
42. Nunes P, Demaurex N. The Role of Calcium Signaling in Phagocytosis. J Leukoc Biol (2010) 88:57–68. doi: 10.1189/jlb.0110028
43. Scherz-Shouval R, Elazar Z. Regulation of Autophagy by ROS: Physiology and Pathology. Trends Biochem Sci (2011) 36:30–8. doi: 10.1016/j.tibs.2010.07.007
44. Gutierrez MG, Master SS, Singh SB, Taylor GA, Colombo MI, Deretic V. Autophagy is a Defense Mechanism Inhibiting BCG and Mycobacterium Tuberculosis Survival in Infected Macrophages. Cell (2004) 119:753–66. doi: 10.1016/j.cell.2004.11.038
45. Parsanathan R, Jain SK. Glucose-6-Phosphate Dehydrogenase Deficiency Activates Endothelial Cell and Leukocyte Adhesion Mediated Via the Tgfβ/Nadph Oxidases/Ros Signaling Pathway. Int J Mol Sci (2020) 21:7474. doi: 10.3390/ijms21207474
46. Lou Z, Wang A-P, Duan X-M, Hu G-H, Song G-L, Zuo M-L, et al. Upregulation of NOX2 and NOX4 Mediated by TGF-β Signaling Pathway Exacerbates Cerebral Ischemia/Reperfusion Oxidative Stress Injury. Cell Physiol Biochem (2018) 46:2103–13. doi: 10.1159/000489450
47. Chavez-Galan L, Vesin D, Uysal H, Blaser G, Benkhoucha M, Ryffel B, et al. Transmembrane Tumor Necrosis Factor Controls Myeloid-Derived Suppressor Cell Activity Via TNF Receptor 2 and Protects From Excessive Inflammation During BCG-Induced Pleurisy. Front Immunol (2017) 8:999. doi: 10.3389/fimmu.2017.00999
48. Condamine T, Mastio J, Gabrilovich DI. Transcriptional Regulation of Myeloid-Derived Suppressor Cells. J Leukoc Biol (2015) 98:913–22. doi: 10.1189/jlb.4RI0515-204R
Keywords: MDSC (myeloid-derived suppressor cell), tuberculosis, LTBI (latent TB infections), active TB, monocytes
Citation: Grassi G, Vanini V, De Santis F, Romagnoli A, Aiello A, Casetti R, Cimini E, Bordoni V, Notari S, Cuzzi G, Mosti S, Gualano G, Palmieri F, Fraziano M, Goletti D, Agrati C and Sacchi A (2021) PMN-MDSC Frequency Discriminates Active Versus Latent Tuberculosis and Could Play a Role in Counteracting the Immune-Mediated Lung Damage in Active Disease. Front. Immunol. 12:594376. doi: 10.3389/fimmu.2021.594376
Received: 13 August 2020; Accepted: 06 April 2021;
Published: 26 April 2021.
Edited by:
Anca Dorhoi, Friedrich-Loeffler-Institute, GermanyReviewed by:
Irina V. Lyadova, Russian Academy of Sciences, RussiaTobias Dallenga, Research Center Borstel (LG), Germany
Copyright © 2021 Grassi, Vanini, De Santis, Romagnoli, Aiello, Casetti, Cimini, Bordoni, Notari, Cuzzi, Mosti, Gualano, Palmieri, Fraziano, Goletti, Agrati and Sacchi. This is an open-access article distributed under the terms of the Creative Commons Attribution License (CC BY). The use, distribution or reproduction in other forums is permitted, provided the original author(s) and the copyright owner(s) are credited and that the original publication in this journal is cited, in accordance with accepted academic practice. No use, distribution or reproduction is permitted which does not comply with these terms.
*Correspondence: Chiara Agrati, Y2hpYXJhLmFncmF0aUBpbm1pLml0