- 1Department of Electrical and Computer Engineering, College of Engineering, Carnegie Mellon University, Pittsburgh, PA, United States
- 2Department of Chemical Engineering, College of Engineering, Carnegie Mellon University, Pittsburgh, PA, United States
Extracellular vesicles (EVs) are important players in autoimmune diseases, both in disease pathogenesis and as potential treatments. EVs can transport autoimmune triggers throughout the body, facilitating the process of antigen presentation. Understanding the link between cellular stress and EV biogenesis and intercellular trafficking will advance our understanding of autoimmune diseases. In addition, EVs can also be effective treatments for autoimmune diseases. The diversity of cell types that produce EVs leads to a wide range of molecules to be present in EVs, and thus EVs have a wide range of physiological effects. EVs derived from dendritic cells or mesenchymal stem cells have been shown to reduce inflammation. Since many autoimmune treatments are focused only on symptom management, EVs present a promising avenue for potential treatments. This review looks at the different roles EVs can play in autoimmune diseases, from disease pathology to diagnosis and treatment. We also overview various methodologies in isolating or generating EVs and look to the future for possible applications of EVs in autoimmune diseases.
Introduction to Extracellular Vesicle (EV) Biology
Our understanding of the role of extracellular vesicles (EVs) in biological processes is rapidly evolving. There is substantial literature connecting cellular stress to autoimmunity, but surprisingly there are few that conceptualize EVs as the communication link (1–3). When EVs were first discovered, they were believed to be a final product, a mechanism for discarding unnecessary cellular materials (4). Subsequent studies showed that EVs could also function to transmit instructions for cell migration, proliferation, and differentiation (5). Later on, the role of EVs modulating immune responses through antigen presentation was uncovered (6). Immune cells secrete EVs and also receive cellular instructions from EVs that guide further immune reactions (7). We believe this is an emerging trend that should be evaluated when considering diagnostic and therapeutic applications.
EVs are produced by a variety of cells, and their content resembles their host cells—the lipid composition of the outer cell membrane makes up the outer membrane of the EV. In addition, EVs contain a mixture of bioactive molecules—i.e. proteins, nucleic acids, metabolites—derived from the cytoplasmic content of the host cell. Importantly, however, the concentration may not be similar, and studies have shown enrichment of proteins in EVs that was not apparent from the host cells (6, 8).
While exosome is the classical term, EV has become the more general term to encapsulate both the diversity and complexity of vesicles observed (9, 10). EVs range in size from 30 nm nanovesicles to ectosomes (100–1,000 nm) to 5 µm microparticles or microvesicles. Nanovesicles—the EV population most commonly thought of as exosomes—are thought to be derived from the fusion of specialized trafficking multi-vesicular endosomes within the cell membrane (11). In contrast, microvesicles are thought to be pinched or shed from the cell membrane (7). Microvesicles also include vesicles derived from apoptotic cells (ApoEVs) (12–14), which were formerly considered as debris rather than purposefully packaged compartments with immunoregulatory functions. The heterogeneity in nomenclature combined with historical EV isolation techniques that filtered out larger vesicles makes it difficult to compare studies (15). Moreover, some ApoEVs resemble nanovesicles in size, morphology, and surface markers (16). Nonetheless, these differences in biogenesis yield significant information about the host cells as well as the potential purpose of the resulting EVs.
Communication of EVs with immune cells occurs through a variety of mechanisms that are tightly paired with the intended downstream function. For example, EVs can be transmitted via direct cell-to-cell contact in the immune synapses, which are junctions between antigen-presenting cells (APCs) and T cells that mediate the antigen presentation process (17–19). EV membranes can include surface molecules that facilitate binding with the extracellular matrix (ECM) (20). The location of EVs within the ECM can also dictate certain physiological processes such as differentiation and angiogenesis (21). During skeletal regeneration, matrix-bound EVs guide macrophage differentiation and downstream myogenesis of skeletal muscle progenitor cells (22, 23). EVs can also be freely shed to travel through interstitial fluid to the lymph nodes or diffuse into the bloodstream (24). Each of these mechanisms becomes important for understanding the development of immune response leading to autoimmune diseases.
Proteomic, genomic, and metabolic data indicate that EVs from distressed cells differ in size, content, quantity, and biogenesis from healthy cells (12, 25). Healthy cells exposed to cellular stress show increases in EV production, content, and uptake. Bovine granulosa cells that were exposed to H2O2 oxidative stress released exosomes containing anti-oxidative molecules (26). Serum-starved mesenchymal stem cells (MSCs) produces exosomes that traffic to neurons 22-fold more efficently than non-serum deprived MSCs (27). While EV content and production were modulated when endothelial cells were exposed to hypoxic conditions, the properties were not reflected when exposed to high glucose (28). These suggest that EVs can modulate communication of stress signals, although not communicate all stresses equally.
EV Production, Cellular Stress, and Autoimmunity
In the context of autoimmunity, the relationship between cellular stress and EV biogenesis is especially significant. Cellular stress is implicated in the initiation of many autoimmune diseases (3). While there are genetic factors that contribute to autoimmunity, genotype alone does not explain all the incidences. EV content varies widely based on cell type as well as the amount of cellular stress. It is unclear whether there is a consistent purpose for EV communication in autoimmunity. In some cases, EVs could function as a warning or protective signal to surrounding cells. On the other hand, it could simply be a disposal mechanism gone wrong. For example, ineffective clearing of ApoEVs can result in necrosis, causing the release of autoantigens with other pro-inflammatory signals, thereby contributing to autoimmune disease (12).
EV-mediated intercellular communication has garnered increasing attention and is thought to have a critical role in the development and progression of autoimmunity. In the same manner that tumor-derived EVs can condition bone marrow-derived cells to prepare distal organs for the arrival of metastatic cells, EVs from autoimmune organs can educate the immune system on how to react (24, 29). EVs can carry autoantigens, a “self” protein or protein complex that triggers an immune response, which then facilitates an autoimmune disease (Table 1).
The origin of autoimmunity is not known for many diseases, and clinical treatments frequently rely on addressing the symptoms rather than the root cause. For example, the standard treatment for Type 1 Diabetes is delivery of exogenously produced insulin, and rheumatoid arthritis is treated via delivery of systemic immunosuppressant drugs such as steroids. The growing literature about EVs’ role in antigen presentation may bring the fields closer to not only explanations of disease initiation, but also methods to diagnose and treat autoimmunity.
Systemic Lupus Erythematosus (SLE)
One common autoimmune disorder associated with EVs is SLE. SLE is characterized with the hyperproduction of autoantibodies and the accumulation of immune-complexes (ICs), leading to inflammation and tissue damage (Figure 1) (31). ICs are formed when EVs containing surface antigens bind to circulating immunoglobulins or ECM proteins, thereby facilitating immune recognition (50, 51). These ICs induce complement-mediated immune responses (52). Apoptosis and immunogenic apoptotic bodies are commonly found in SLE, and may contain dsDNA or other nucleic acids (32, 36). These EVs may stimulate type-1 interferon (IFN) production, which is another major disease contributor in SLE (31). These EVs may also contain autoantigens such as galectin-3-binding protein (G3BP), which is strongly correlated with IC production and increased adherence to the endothelium and basement membrane (32–34).
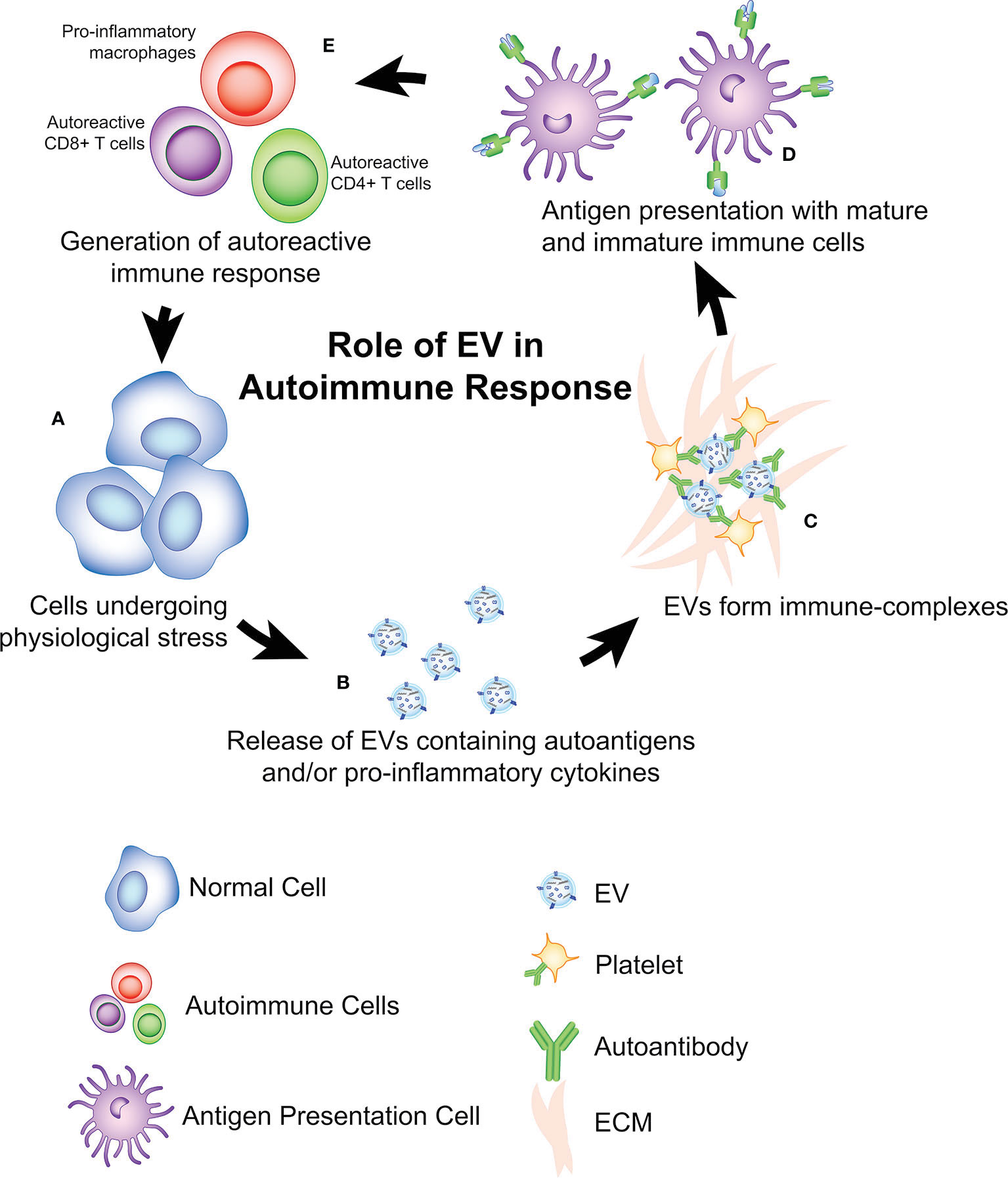
Figure 1 Role of EVs in antigen presentation and initiation of autoimmune diseases. (A) a potential theory is that cells undergoing physiological stress initiation autoimmunity via release of (B) EVs containing autoantigens, pro-inflammatory cytokines, and/or genetic material (i.e. miRNA, DNA). (C) In some cases, these EVs can interact with ECM, antibodies, platelets to form immune-complexes (ICs) that can also facilitate presentation to (D) immature and mature antigen presenting cells. After antigen presentation, an adaptive autoimmune response is developed (E) as indicated by the expansion of autoreactive CD8+ T Cells, CD4+ T cells, autoreactive B cells, and the pro-inflammatory activation of innate immune cells (i.e. macrophages, neutrophils). This process produces a positive feedback loop, contributing to more cellular damage within the autoimmune microenvironment which leads to the production of more EVs.
Diabetes
Type 1 diabetes (T1D) is a tissue-specific autoimmune disease caused by the autoreactive T cell–mediated destruction of insulin-producing β-cells in the pancreas. EVs are thought to contribute to T1D development in several ways. First, there is a working hypothesis that EVs containing autoantigens are released from stressed cells (Figure 1), leading to the activation of autoreactive T cells (40). Autoantigens (ZnT8, insulin, GAD65, and IA-2) have been found in EVs derived from pancreatic islet cells, in both human and rat models (37, 38, 53). These EVs are subsequently endocytosed by dendritic cells (DCs) followed with the activation of the APCs. Aside from the autoantigens, EVs derived from islets also contain immunostimulatory cytokines such as IFN-γ. These cytokines are able to stimulate monocytes, autoreactive B and T cells, which further contribute to the destruction of pancreatic cells (38, 54, 55). In this manner, EVs serve as an autoimmune trigger by facilitating the antigen presentation and activation of autoreactive immune cells (37).
EVs are also involved in the development of Type 2 Diabetes (T2D) by promoting insulin resistance via downregulation of glucose transporter type 4 (GLUT4) (56, 57). Microvesicles derived from pro-inflammatory macrophages have been shown to reduce insulin signal transduction and GLUT4 activity (57). Moreover, ApoEVs may induce pro-inflammatory macrophage polarization via secretion of mir-155, a potent circulating microRNA (miRNA) that is clinically elevated in T2D studies (58, 59).
Rheumatoid Arthritis (RA)
RA is a systemic autoimmune disease characterized by chronic inflammation of the synovial joints and the erosion of cartilage (60). EVs are also implicated in RA pathogenesis. Clinically, RA patients have increased EV concentration in synovial fluid and the joint space in comparison to healthy controls (41, 61, 62). The main sources of EVs in RA are platelets, leukocytes and fibroblast-like synoviocytes (FLS) (41–44). Platelet-derived EVs (PEVs) are of particular interest as their higher concentrations in patients with RA and are correlated with disease severity (61, 63). PEVs present interleukin-1 (IL-1) on their surface, which stimulate FLS to release pro-inflammatory IL-6 and IL-8 (44). Stimulated FLSs are also known to release a number of ECM-degrading enzymes that contribute to joint destruction (41). RA-associated EVs also frequently express citrullinated proteins including fibrinogen, fibrin, fibronectin and vimentin which can act as autoantigens (42, 45). EVs containing citrullinated proteins are of particular interest, given that anti-cyclic citrullinated peptide (anti-CCP) antibodies have a 96% specificity for the diagnosis of RA (63). These EVs can also form ICs which are pro-pro-inflammatory and stimulate neutrophils to release leukotrienes, which further perturbate the inflammation in the joint (42).
Vitiligo
Vitiligo is a disorder consisting of the destruction of melanocytes leading to the depigmentation of the skin (64). Oxidative stress via reactive oxygen species (ROS) causes damage to melanocytes which then release antigens through a number of pathways, including EVs (65). When exposed to ROS, melanocytes release EVs containing tyrosinase and Melan-A (MART-1), a melanosome antigen recognized by T cells, thus leading to activation of the autoimmune response (46, 66). Vitiligo also develops as an immune related adverse event (irAEs) following checkpoint inhibitor cancer immunotherapy (67, 68). Interestingly, in the case of melanoma, vitiligo is associated with successful treatment, and individuals with pre-existing vitiligo have lower frequencies of melanoma cancer development (69, 70). This suggests a dynamic relationship between T cell activation and melanocyte destruction.
Pre-Eclampsia (PE)
PE is a leading cause of maternal death, affecting 2–8% of pregnancies (71, 72). It manifests in two stages, the first being incomplete development of the placenta, while the second stage consists of the main pathology of the disease due to systemic inflammation in the mother (47). It is believed that EVs are directly involved in both stages of PE pathogenesis (73, 74).
During normal pregnancy, exosomes influence the spiral artery remodeling in the uterus, forming the blood vessels that allow for sufficient delivery of nutrients and oxygen from the mother to the fetus (75). Over the course of spiral artery remodeling, uterine arteries expand from 200 μm to 2 mm in diameter. This process occurs through the sequential steps of vessel dilation, vascular smooth muscle cell separation, endothelial cell swelling, extravillous trophoblast (EVT) cell invasion and fibrinoid deposition (75). In early-onset PE, poor placentation occurs due to insufficient spiral artery remodeling. The intrauterine oxygen tension in PE remains at hypoxic levels (1%-3%), leading to a 7-fold increase in exosome release (75, 76). In addition, bioactivity of the exosomes showed increased levels of hypoxia-induced factor 1-alpha (HIF-1α) and IL-8 (75).
The release of these exosomes is dependent upon the oxygen tension within the uterus. During the first trimester, intrauterine oxygen tension as low as 3%. The lower oxygen tension causes an increase in exosomal release and bioactivity, stimulating the invasion of the EVTs to perform proper placentation (75). Cytotrophoblast exosomes regulate this invasion in both a time and dose-dependent manner (75). After adequate spiral artery remodeling occurs, the oxygen tension increases to 8% (76). It has been hypothesized that the increase in exosomal release under hypoxia is an adaptive measure taken by the body to induce the proliferation and invasion of the EVTs to correct the poor placentation while sufficiently remodel the spiral arteries (77). However, the mechansims underlying why this process leads to normal pregnancy in some and PE in others is still unclear.
Much like in other autoimmune diseases summarized in this review, EVs generated via cellular stress contribute to PE. In vitro studies reveal that human placental cells (BeWo) undergoing endoplasmic reticulum stress (ER-stress) produce EVs that contain damage-associated molecular patterns (DAMPs) thought to contribute to the development of PE (78). EVs from the syncytiotrophoblast, the epithelial covering of the placenta interfacing with maternal blood, shed into the maternal blood carrying antigens (47, 74). EVs collected from patients with PE show significantly higher neprilysin and human leukocyte antigen (HLA-DR), with significantly lower synctin-2 compared to EVs from normal pregnancies (47, 79). Syncytiotropoblast-derived EVs stimulate monocytes, neutrophils, B and T lymphocytes which then release IL-6, IL-8 and tumor necrosis factor-α (TNF-α), leading to a pro-inflammatory response (74).
EV-Facilitated Therapies to Treat Autoimmune Disease
MSC-Derived EVs as Autoimmune Diseases Therapies
Mesenchymal stem cells (MSCs) are key sources of immunosuppressive soluble factors that are critical to tissue repair and regeneration (80). Because of their unique function, MSCs or MSC-derived EVs (MSC-EVs) have been therapeutically administered to heal wounds and repair tissue after myocardial infarction (80, 81). Current autoimmune therapeutic strategies involving MSC-EVs have shown efficacy in reducing inflammation in several animal models including experimental autoimmune encephalomyelitis (EAE) mice, which can serve as a model for multiple sclerosis (MS). EAE mice treated with EVs from stimulated MSCs showed reduction in the clinical score, demyelination and neuroinflammation, with increased secretion of anti-inflammatory cytokines as well as upregulated regulatory T cells (82, 83). In a rat experimental autoimmune uveitis (EAU) model, MSC-EVs lead to a reduction of EAU in the eyes (84).
In addition to reducing inflammation, MSCs have been clinically explored to prevent tissue damage and promote regeneration. Tao et al., used human embryonic MSC-EVs to regenerate tissue following osteoarthritis-induced cartilage damage (85). The group found MSC-EVs improved histological scores and gross appearance of the cartilages over controls, with restoration of cartilage as well as subchondral bone by week 12. The characteristics of hyaline cartilage closely resembled the unoperated control. In addition to this, exosomes from human synovial MSC (SMSC) have been shown to promote cartilage regeneration with preventive effect in a rat osteoarthritis model (85). Bone marrow-derived MSC-EVs were shown to delay the inflammatory response in RA, measured through reduction in joint damage and pro-inflammatory gene expression (86). Interestingly, both MSC-EVs classified as exosomes and microvesicles can protect mice from joint damage (87).
Induced pluripotent MSC (iMSCs) are a valuable cell source for EV treatments due to their compatibility with autologous transplantation (88). Moreover, iMSCs pocesses the potential for scalability, which is vital due to the enourmous amounts of cells needed to produce therapeutic quantities of EVs and the limited population of source cells from which to derive. When tested for their ability to promote proliferation in a skin wound healing model, EVs derived from iMSCs performed similarly to EVs derived from native MSCs (89). Comparable studies show similar therapeutic results in osteoarthritis (90).
DC-Derived EVs as Autoimmune Diseases Therapies
DCs can regulate adaptive immunuity through antigen presentation to T cells and in this manner, DC derived EVs (DC-EVs) have also been explored for treatment of autoimmune diseases. DCs differ from MSCs slightly in that DCs can perform either pro-inflammatory or anti-inflammatory functions (91), thus DC-EVs must be harvested from DCs that have been conditioned to an immunosuppressive phenotype to illicit an anti-inflammatory effect. Nonetheless, DC-EVs have been assessed for their therapeutic potential to treat autoimmune diseases with cheerful efficacy across several animal models. DC-EVs delayed the onset of murine collagenase-induced arthritis (CIA) and dampened the severity of established arthritis (92). Mice that received EVs from engineered bone marrow DCs (BMDCs) even showed a reversal effect of established CIA, with the exosomes having a stronger immunosuppressing effect than the parental DCs (92). DC-EVs have also been studied for Irritable Bowel Disease (IBD), with showing a protective effective through induction of regulatory T cells (93).
EVs as A Drug Delivery Vehicle
Most of the current EV treatment strategies involve harvesting EVs from stimulated MSCs or DCs (Table 2). There are fewer studies that load harvested native EVs with therapeutic reagents for treatment in autoimmune disease, which could be a future growth opportunity. Various methods have been explored to utilize EVs to deliver drugs for septic shock, breast cancer, prostate cancer, hepatocarcinoma, and Parkinson’s disease (94–99). EVs can be loaded externally, i.e. drugs are loaded via membrane manipluations. Sun et al. mixing the exosomes with curcumin and demonstrated an enhanced anti-inflammatory effect of curcumin in septic shock treatment (97). Loading EVs with aptamers through mechanical extrusion of breast cancer cells enhances breast cancer targeting effect of the EVs (94). Chemotherapy may also be improved with EVs, as co-incubated prostate cancer-derived EVs with paclitaxel showed a stronger therapeutic effect (98) than paclitaxel alone. EVs have also been loaded using internal mechanisms, i.e.loading cells and harvesting the drug-laden EVs from cell culture media. The Batrakova group has successfully used this strategy to delivery nucleic acids in Parkinson’s Disease, cancer, and Batten’s Disease (100–102). Other groups transfect cells with vectors encoding for nanobodies to enhance the EVs targeting capacity (103).
EV Isolation Methods
The manner in which EVs are isolated and characterized via size, morphology, and composition is well developed (8, 10, 104–107). However there is a missing connection between how the field characterizes EVs and their classifications via biogenesis. For example, ApoEVs can be as large as 5 µm but also as small as 50 nm (12). In the context of autoimmune diseaases, the isolation and classification of EVs are particularly relevant, considering their diversed contribution to disease initiation and propagation. Various EV isolation methods have been used for autoimmune diseases, with each of them targeting specific EV populations.
Differential centrifugation is arguably the most commonly used technique for EV isolation (96, 108–110). This method usually involves a series of low-speed centrifugation steps to remove the cellular debris, followed by ultracentrifugation to pellet down the sub-micron size vesicles. Following differential centrifugation, density gradient centrifugation may further purify EVs (96, 108, 111). Tris/sucrose/D2O mixture is frequently used as a cushion, which is then topped with the partially isolated EVs. After centrifuging the combination at 100,000 x g for 75 min, the cushion is transferred into a new container and diluted, followed by another ultracentrifugation. For further characterization, ultrafiltration can be used. Ultrafiltration passes the EV suspension through one or a series of membrane filters with defined pore sizes, which separates EVs into subtypes based on sizes (110).
Chromatography is also used to isolate EVs. This includes size-exclusion chromatography where porous beads separate the particles based on the hydrodynamic radius. The fraction containing particles with the size of EVs are selectively collected (112–114). Alternatively, as EVs have negative charges, an anion exchange chromatography isolation protocol has been established. Culture medium containing EVs is passed through a solid-phase monolithic ion exchange column and then eluted with NaCl (115).
Precipitation methods are simple but with potentially compromised performance. Polymer precipitation with polyethylene glycol (PEG) is frequently adopted by the commercial kits to eliminate the usage of ultracentrifugation (116). Adding PEG or other super hydrophilic polymers into the EV-containing samples decreases the solubility of EVs, allowing pellet formation at 1,500 x g. Chemical precipitation includes organic solvents, sodium acetate, and protamine (117). Organic solvents precipitate EVs out of the solution through ion-pairing effect, while sodium acetate disturbs EV hydration and pellets by hydrophobic effect. Protamine, as a positively charged molecule, pellets the EVs in a similar manner, and is removed by gel filtration.
Affinity-based separation is another important approach, particularly because of the capacity to isolate EVs based on subpopulations. Here, antibody, aptamer or other affinity reagents are used to isolate EVs with a specific surface expression profile (118–121). Immunoprecipitation takes advantage of the markers commonly enriched on EV surface. The EV suspension is mixed with magnetic beads coupled with the corresponding antibodies, then isolated with a magnetic field. For total exosome isolation, tetraspanins CD63, CD9, and CD81 are normally used as surface antigen targets (96, 122).
Microfluidics are gaining interests in isolating EVs. Microfluidics isolate EVs from human sera or cell culture media in a lab-on-a-chip fashion (123). Chen et al. demonstrated the concept with herringbone groves in 50-μm-wide channels to increase surface area, while attached anti-CD63 antibodies to the surface via biotin-NeutrAvidin conjugation for EV capturing. As CD63 is not ubiquitously expressed in all EV population, Wan et al. first designed a lipid nanoprobe that targets the EV membrane, then incorporated it onto a silica herringbone nanostructure microfluidic device for total EV isolation (124).
Artificial EV Generation Methods
The biocompatibility and functionality of EVs make them an advantageous drug delivery vehicle. However, cells secrete EV at a relatively low rate. Therefore, multiple approaches have been explored to generate EVs artificially (Figure 2), with a common method being mechanical extrusion (Figure 2A). Mechanical extrusion methods pass whole cells or membrane lysates through channels of 1–10 μm in diameter (94, 125–127). The channels can be created with tracked-etched membranes or microfluidic devices. Membrane decoration is an efficient method for EV drug loading, which encapsulates a core into lysed cellular or EV membranes (Figure 2B) (95, 128, 129). The core could be a metal-organic framework or nanoparticles.
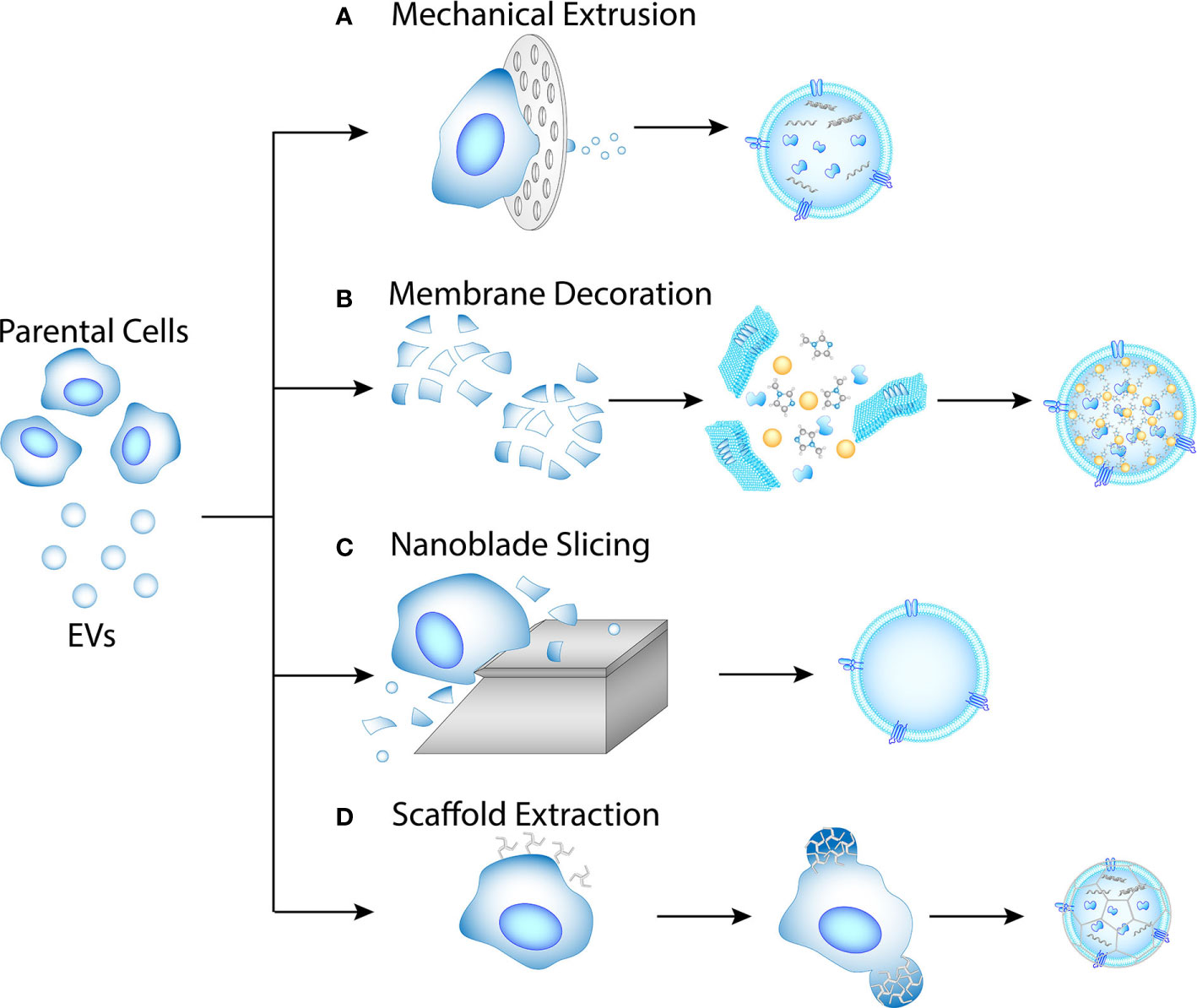
Figure 2 Methods for artificial EV production. (A) Mechanical extrusion. Intracellular contents are pertained in the EVs. (B) Membrane decoration. Membrane fragments are obtained from lysed cell or EV, then mixed with nanoparticle or MOF cores. (C) Nanoblade slicing. Cellular membranes are sliced off the cells and allowed to self-assemble into EVs. (D) Scaffold extraction. Clathrin-like nanoparticles may extract EVs from cellular membrane by pinching.
Some other methods for artificial EV generation that have been investigated include nanoblade slicing (Figure 2C) and scaffold extraction (Figure 2D). Nanoblade slicing rolls cells over microfabricated 500 nm-thick silicon nitride blades (130). The cellular membrane is sliced into fragments during the process, which then self-assemble into nanovesicles with diameters of 100-300 nm. Substances such as nanobeads can be flowed simultaneously for encapsulation, with an efficiency around 30%. Scaffold extraction is potentially a method for extracting EVs directly from the cellular membrane (131). Using dissipative particle dynamics model, Li et al. simulated the extraction process with clathrin-like nanoparticles, and concluded that the size of the resulting vesicles depends on the concentration of the nanoparticles as wells as membrane surface tension.
Use of EVs in Clinical Trials for Auto-Immune Diseases
At the time of this writing there were 560 registered trials that involved EVs or derivative terms such as exosomes, microvesicles, and/or microparticles. Of these, 47 trials aligned with autoimmune diseases (Table 2). A subset of these clinical trials categorize the potential for EVs as autoimmune disease biomarkers. One such study is NCT04164966, an observational study to detect circulating beta-cell exosomes in early onset T1D in a pediatric population. Microparticles are also being studied as a prognostic indicator in SLE (NCT03575156) and arthritis (NCT02333708), highlighting the need for investigation of multiple EV populations. In addition, some trial studies measure EVs concentration as a monitoring tool to quantify the success of their drug intervention. An example of this is NCT01397513, a trial that correlates platelet microparticles to the effect of aspirin dosage on fibrin formation in T1D patients (132).
Of those, there were currently five clinical trials using EVs to treat autoimmune-related diseases. While this seems sparse, it should be noted that these numbers are likely due to the prevalence of cell-based treatments. Of the roughly 10,000 clinical trials related to autoimmune disease, ~300 trials are using MSCs or T cells as a treatment. As the scientific capacity to produce clinical-grade EVs increases, there will likely be an increase in EVs-based therapies for all diseases. As it stands, the results for EVs to treat autoimmune diseases is compelling but limited (Table 2). While not classified as such, the clinical manifestation and treatment strategies of graft-versus-host-disease (GvHD) are similar to textbook autoimmune disease (133). Kordelas et al. have demonstrated the feasibility of using the exosome to ameliorate GvHD in one patient that received allogeneic hematopoietic stem cell transplantation (134). Within two weeks, the cutaneous and mucosal GvHD syndromes were remarkably improved, which consequently reduced the dosage of steroid treatment from 125 mg/d to 30 mg/d after exosome therapy. Diarrhea volume of the patient was also objectively reduced.
Interestingly, another clinical trial used two distinct populations of MSC-EVs to treat T1D. The first dose included EVs that were 40–180 nm in size with CD63, CD9, Alix, TSG101 and HSP70 as markers. The second dose consisted of microvesicles of 180-1000 nm in size purified from same MSCs but characterized via with annexin V, flotillin-2, selectin, integrin, cd40 and metalloproteinase markers. Unfortunately, the publications listed by the study principal invesetigators do not include data on EVs but rather the delivery of MSCs, making it unclear whether either population of EVs would have had any promising effect (135, 136).
Conclusion: Future Growth Areas
There are many opportunities for EVs therapies in autoimmune disease. MSC and DC derived EVs are demonstrably efficacious for mounting anti-inflammatory response that promote regeneration, wound-healing, and ameliorating autoimmune disease. However, they are difficult to produce, they require large volumes of cells/media and their batch-to-batch variability lacks the consistency of traditional small molecule therapies. Artificial production of EVs can enable scalable solutions to production, purification, and modification challenges. Currently, the biomarker and diagnostics capabilities of EVs is more advanced than treatments used for autoimmune diseases. New techniques for producing and characterizing artificial EVs could facilitate treatment for autoimmune diseases.
While there has been a large amount of research done on the role of MSC-EVs and DC-EVs, there is comparatively little known about the role of EVs from other immune cells such as macrophages and B cells. Given their role in the pathogenesis of autoimmune disease, it is likely that the EVs from these cells play a significant part in the immune response and could be used in downstream treatment and diagnosis strategies.
Due to the increase of immunotherapy treatments (i.e. checkpoint inhibitors, CAR-T), there will likely be an increase in autoimmune disease events due to irAEs. One example is a treatment using checkpoint inhibitors designed to increase cytotoxic T cell activity in tumors gave rise to cases of vitiligo and hypo/hyper-thyroidism (67, 70). irAEs can range from mild skin reactions to life-long autoimmune-related diseases such as arthritis (137). This convergence in patient populations being treated for irAEs and autoimmune diseases creates an opportunity to gain insight into autoimmune pathology, which is still not universally understood. Investigating EVs in autoimmune disease also presents a unique opportunity to explore bidirectional relationships between diseases. A great example of this are findings that COVID-19 can initiate new-onset diabetes or enhance metabolic complications of pre-existing diabetes (138).
In conclusion, EVs play a significant role in autoimmune disease initiation and progression. Though the role of EVs vary throughout each autoimmune disease summarized, the common themes encourage further investigation of EVs as diagnostic and therapeutic tools. EVs facilitate autoimmune responses by presenting autoantigens from cellularly stressed cells to immune cells. In addition, the EVs can form immune complexes that trigger inflammatory responses. Deciphering the linkage between EVs biogenesis and trafficking may reveal insights into unanswered questions in autoimmune initiation and hold the key to effective treatment.
Author Contributions
ML, ED, EP, HF, S-YZ, and EW wrote and edited the manuscript. EW conceived and oversaw the project. All authors contributed to the article and approved the submitted version.
Funding
ML was supported through NIH 7R01CA230339. ED was supported by the Molecular Biophysics and Structural Biology graduate program at the University of Pittsburgh and Carnegie Mellon University.
Conflict of Interest
The authors declare that the research was conducted in the absence of any commercial or financial relationships that could be construed as a potential conflict of interest.
References
1. Morito D, Nagata K. ER Stress Proteins in Autoimmune and Inflammatory Diseases. Front Immunol (2012) 3:48:48. doi: 10.3389/fimmu.2012.00048
2. Smallwood MJ, Nissim A, Knight AR, Whiteman M, Haigh R, Winyard PG. Oxidative stress in autoimmune rheumatic diseases. Free Radic Biol Med (2018) 125:3–14. doi: 10.1016/j.freeradbiomed.2018.05.086
3. Harris JE. Cellular stress and innate inflammation in organ-specific autoimmunity: lessons learned from vitiligo. Immunol Rev (2016) 269(1):11–25. doi: 10.1111/imr.12369
4. Johnstone RM. Maturation of reticulocytes: formation of exosomes as a mechanism for shedding membrane proteins. Biochem Cell Biol (1992) 70(3-4):179–90. doi: 10.1139/o92-028
5. Gangoda L, Boukouris S, Liem M, Kalra H, Mathivanan S. Extracellular vesicles including exosomes are mediators of signal transduction: are they protective or pathogenic? Proteomics (2015) 15(2-3):260–71. doi: 10.1002/pmic.201400234
6. Robbins PD, Morelli AE. Regulation of immune responses by extracellular vesicles. Nat Rev Immunol (2014) 14(3):195–208. doi: 10.1038/nri3622
7. Lindenbergh MFS, Stoorvogel W. Antigen Presentation by Extracellular Vesicles from Professional Antigen-Presenting Cells. Annu Rev Immunol (2018) 36(1):435–59. doi: 10.1146/annurev-immunol-041015-055700
8. Xu R, Greening DW, Zhu HJ, Takahashi N, Simpson RJ. Extracellular vesicle isolation and characterization: toward clinical application. J Clin Invest (2016) 126(4):1152–62. doi: 10.1172/JCI81129
9. Witwer KW, Thery C. Extracellular vesicles or exosomes? On primacy, precision, and popularity influencing a choice of nomenclature. J Extracell Vesicles (2019) 8(1):1648167. doi: 10.1080/20013078.2019.1648167
10. Thery C, Witwer KW, Aikawa E, Alcaraz MJ, Anderson JD, Andriantsitohaina R, et al. Minimal information for studies of extracellular vesicles 2018 (MISEV2018): a position statement of the International Society for Extracellular Vesicles and update of the MISEV2014 guidelines. J Extracell Vesicles (2018) 7(1):1535750. doi: 10.1080/20013078.2018.1535750
11. Turchinovich A, Drapkina O, Tonevitsky A. Transcriptome of Extracellular Vesicles: State-of-the-Art. Front Immunol (2019) 10:202. doi: 10.3389/fimmu.2019.00202
12. Apoptotic Cell-Derived Extracellular Vesicles: More Than Just Debris. Front Immunol (2018) 9:1486:1486. doi: 10.3389/fimmu.2018.01486
13. Niessen A, Heyder P, Krienke S, Blank N, Tykocinski LO, Lorenz HM, et al. Apoptotic-cell-derived membrane microparticles and IFN-alpha induce an inflammatory immune response. J Cell Sci (2015) 128(14):2443–53. doi: 10.1242/jcs.162735
14. Kato Y, Park J, Takamatsu H, Konaka H, Aoki W, Aburaya S, et al. Apoptosis-derived membrane vesicles drive the cGAS-STING pathway and enhance type I IFN production in systemic lupus erythematosus. Ann Rheum Dis (2018) 77(10):1507–15. doi: 10.1136/annrheumdis-2018-212988
15. Tkach M, Kowal J, Thery C. Why the need and how to approach the functional diversity of extracellular vesicles. Philos Trans R Soc Lond B Biol Sci (2018) 373(1737):20160479. doi: 10.1098/rstb.2016.0479
16. Park SJ, Kim JM, Kim J, Hur J, Park S, Kim K, et al. Molecular mechanisms of biogenesis of apoptotic exosome-like vesicles and their roles as damage-associated molecular patterns. Proc Natl Acad Sci U S A (2018) 115(50):E11721–30. doi: 10.1073/pnas.1811432115
17. Mittelbrunn M, Gutierrez-Vazquez C, Villarroya-Beltri C, Gonzalez S, Sanchez-Cabo F, Gonzalez MA, et al. Unidirectional transfer of microRNA-loaded exosomes from T cells to antigen-presenting cells. Nat Commun (2011) 2:282. doi: 10.1038/ncomms1285
18. Gutiérrez-Vázquez C, Villarroya-Beltri C, Mittelbrunn M, Sánchez-Madrid F. Transfer of extracellular vesicles during immune cell-cell interactions. Immunol Rev (2013) 251(1):125–42. doi: 10.1111/imr.12013
19. Mittelbrunn M, Vicente Manzanares M, Sanchez-Madrid F. Organizing polarized delivery of exosomes at synapses. Traffic (2015) 16(4):327–37. doi: 10.1111/tra.12258
20. Németh A, Orgovan N, Sódar BW, Osteikoetxea X, Pálóczi K, Szabó-Taylor KÉ, et al. Antibiotic-induced release of small extracellular vesicles (exosomes) with surface-associated DNA. Sci Rep (2017) 7(1):8202. doi: 10.1038/s41598-017-08392-1
21. Todorova D, Simoncini S, Lacroix R, Sabatier F, Dignat-George F. Extracellular Vesicles in Angiogenesis. Circ Res (2017) 120(10):1658–73. doi: 10.1161/CIRCRESAHA.117.309681
22. Hussey GS, Dziki JL, Lee YC, Bartolacci JG, Behun M, Turnquist HR, et al. Matrix bound nanovesicle-associated IL-33 activates a pro-remodeling macrophage phenotype via a non-canonical, ST2-independent pathway. J Immunol Regener Med (2019) 3:26–35. doi: 10.1016/j.regen.2019.01.001
23. Huleihel L. HG, Naranjo JD, Zhang L, Dziki JL, Turner NJ, Stolz DB, et al. Matrix-bound nanovesicles within ECM bioscaffolds. Sci Adv (2016) 2(6):e1600502. doi: 10.1126/sciadv.1600502
24. Hood JL, San RS, Wickline SA. Exosomes released by melanoma cells prepare sentinel lymph nodes for tumor metastasis. Cancer Res (2011) 71(11):3792–801. doi: 10.1158/0008-5472.CAN-10-4455
25. Abramowicz A, Widlak P, Pietrowska M. Different Types of Cellular Stress Affect the Proteome Composition of Small Extracellular Vesicles: A Mini Review. Proteomes (2019) 7(2):23. doi: 10.3390/proteomes7020023
26. Saeed-Zidane M, Linden L, Salilew-Wondim D, Held E, Neuhoff C, Tholen E, et al. Cellular and exosome mediated molecular defense mechanism in bovine granulosa cells exposed to oxidative stress. PloS One (2017) 12(11):e0187569. doi: 10.1371/journal.pone.0187569
27. Haraszti RA, Miller R, Dubuke ML, Rockwell HE, Coles AH, Sapp E, et al. Serum Deprivation of Mesenchymal Stem Cells Improves Exosome Activity and Alters Lipid and Protein Composition. iScience (2019) 16:230–41. doi: 10.1016/j.isci.2019.05.029
28. de Jong OG, Verhaar MC, Chen Y, Vader P, Gremmels H, Posthuma G, et al. Cellular stress conditions are reflected in the protein and RNA content of endothelial cell-derived exosomes. J Extracell Vesicles (2012) 1:18396. doi: 10.3402/jev.v1i0.18396
29. Peinado H, Aleckovic M, Lavotshkin S, Matei I, Costa-Silva B, Moreno-Bueno G, et al. Melanoma exosomes educate bone marrow progenitor cells toward a pro-metastatic phenotype through MET. Nat Med (2012) 18(6):883–91. doi: 10.1038/nm.2753
30. Kapsogeorgou EK, Abu-Helu RF, Moutsopoulos HM, Manoussakis MN. Salivary gland epithelial cell exosomes: A source of autoantigenic ribonucleoproteins. Arthritis Rheum (2005) 52(5):1517–21. doi: 10.1002/art.21005
31. Rasmussen NS, Jacobsen S. Microparticles - culprits in the pathogenesis of systemic lupus erythematosus? Expert Rev Clin Immunol (2018) 14(6):443–5. doi: 10.1080/1744666X.2018.1474100
32. Rasmussen NS, Nielsen CT, Jacobsen S, Nielsen CH. Stimulation of Mononuclear Cells Through Toll-Like Receptor 9 Induces Release of Microvesicles Expressing Double-Stranded DNA and Galectin 3-Binding Protein in an Interferon-alpha-Dependent Manner. Front Immunol (2019) 10:2391:2391. doi: 10.3389/fimmu.2019.02391
33. Nielsen CT, Ostergaard O, Rasmussen NS, Jacobsen S, Heegaard NHH. A review of studies of the proteomes of circulating microparticles: key roles for galectin-3-binding protein-expressing microparticles in vascular diseases and systemic lupus erythematosus. Clin Proteomics (2017) 14:11. doi: 10.1186/s12014-017-9146-0
34. Ostergaard O, Nielsen CT, Tanassi JT, Iversen LV, Jacobsen S, Heegaard NHH. Distinct proteome pathology of circulating microparticles in systemic lupus erythematosus. Clin Proteomics (2017) 14:23. doi: 10.1186/s12014-017-9159-8
35. Ostergaard O, Nielsen CT, Iversen LV, Tanassi JT, Knudsen S, Jacobsen S, et al. Unique protein signature of circulating microparticles in systemic lupus erythematosus. Arthritis Rheum (2013) 65(10):2680–90. doi: 10.1002/art.38065
36. Mobarrez F, Fuzzi E, Gunnarsson I, Larsson A, Eketjall S, Pisetsky DS, et al. Microparticles in the blood of patients with SLE: Size, content of mitochondria and role in circulating immune complexes. J Autoimmun (2019) 102:142–9. doi: 10.1016/j.jaut.2019.05.003
37. Cianciaruso C, Phelps EA, Pasquier M, Hamelin R, Demurtas D, Alibashe Ahmed M, et al. Primary Human and Rat beta-Cells Release the Intracellular Autoantigens GAD65, IA-2, and Proinsulin in Exosomes Together With Cytokine-Induced Enhancers of Immunity. Diabetes (2017) 66(2):460–73. doi: 10.2337/db16-0671
38. Bashratyan R, Sheng H, Regn D, Rahman MJ, Dai YD. Insulinoma-released exosomes activate autoreactive marginal zone-like B cells that expand endogenously in prediabetic NOD mice. Eur J Immunol (2013) 43(10):2588–97. doi: 10.1002/eji.201343376
39. Bashratyan R, Regn D, Rahman MJ, Marquardt K, Fink E, Hu WY, et al. Type 1 diabetes pathogenesis is modulated by spontaneous autoimmune responses to endogenous retrovirus antigens in NOD mice. Eur J Immunol (2017) 47(3):575–84. doi: 10.1002/eji.201646755
40. Dai YD, Sheng H, Dias P, Jubayer Rahman M, Bashratyan R, Regn D, et al. Autoimmune Responses to Exosomes and Candidate Antigens Contribute to Type 1 Diabetes in Non-Obese Diabetic Mice. Curr Diabetes Rep (2017) 17(12):130. doi: 10.1007/s11892-017-0962-4
41. Fu H, Hu D, Zhang L, Tang P. Role of extracellular vesicles in rheumatoid arthritis. Mol Immunol (2018) 93:125–32. doi: 10.1016/j.molimm.2017.11.016
42. Cloutier N, Tan S, Boudreau LH, Cramb C, Subbaiah R, Lahey L, et al. The exposure of autoantigens by microparticles underlies the formation of potent inflammatory components: the microparticle-associated immune complexes. EMBO Mol Med (2013) 5(2):235–49. doi: 10.1002/emmm.201201846
43. Distler JH, Jungel A, Huber LC, Seemayer CA, Reich CF, Gay RE, et al. The induction of matrix metalloproteinase and cytokine expression in synovial fibroblasts stimulated with immune cell microparticles. Proc Natl Acad Sci U S A (2005) 102(8):2892–7. doi: 10.1073/pnas.0409781102
44. Boilard E, Nigrovic PA, Larabee K, Watts GF, Coblyn JS, Weinblatt ME, et al. Platelets amplify inflammation in arthritis via collagen-dependent microparticle production. Science (2010) 327(5965):580–3. doi: 10.1126/science.1181928
45. Skriner K, Adolph K, Jungblut PR, Burmester GR. Association of citrullinated proteins with synovial exosomes. Arthritis Rheum (2006) 54(12):3809–14. doi: 10.1002/art.22276
46. van den Boorn JG, Picavet DI, van Swieten PF, van Veen HA, Konijnenberg D, van Veelen PA, et al. Skin-depigmenting agent monobenzone induces potent T-cell autoimmunity toward pigmented cells by tyrosinase haptenation and melanosome autophagy. J Invest Dermatol (2011) 131(6):1240–51. doi: 10.1038/jid.2011.16
47. Tersigni C, Redman CW, Dragovic R, Tannetta D, Scambia G, Di Simone N, et al. HLA-DR is aberrantly expressed at feto-maternal interface in pre-eclampsia. J Reprod Immunol (2018) 129:48–52. doi: 10.1016/j.jri.2018.06.024
48. Xiao X, Xiao F, Zhao M, Tong M, Wise MR, Stone PR, et al. Treating normal early gestation placentae with preeclamptic sera produces extracellular micro and nano vesicles that activate endothelial cells. J Reprod Immunol (2017) 120:34–41. doi: 10.1016/j.jri.2017.04.004
49. Gill M, Motta-Mejia C, Kandzija N, Cooke W, Zhang W, Cerdeira AS, et al. Placental Syncytiotrophoblast-Derived Extracellular Vesicles Carry Active NEP (Neprilysin) and Are Increased in Preeclampsia. Hypertension (2019) 73(5):1112–9. doi: 10.1161/HYPERTENSIONAHA.119.12707
50. Fendl B, Eichhorn T, Weiss R, Tripisciano C, Spittler A, Fischer MB, et al. Differential Interaction of Platelet-Derived Extracellular Vesicles With Circulating Immune Cells: Roles of TAM Receptors, CD11b, and Phosphatidylserine. Front Immunol (2018) 9:2797:2797. doi: 10.3389/fimmu.2018.02797
51. Fortin PR, Cloutier N, Bissonnette V, Aghdassi E, Eder L, Simonyan D, et al. Distinct Subtypes of Microparticle-containing Immune Complexes Are Associated with Disease Activity, Damage, and Carotid Intima-media Thickness in Systemic Lupus Erythematosus. J Rheumatol (2016) 43(11):2019–25. doi: 10.3899/jrheum.160050
52. Buzas EI, Toth EA, Sodar BW, Szabo-Taylor KE. Molecular interactions at the surface of extracellular vesicles. Semin Immunopathol (2018) 40(5):453–64. doi: 10.1007/s00281-018-0682-0
53. Nakayama M, Simmons KM, Michels AW. Molecular Interactions Governing Autoantigen Presentation in Type 1 Diabetes. Curr Diabetes Rep (2015) 15(12):113. doi: 10.1007/s11892-015-0689-z
54. Rahman MJ, Regn D, Bashratyan R, Dai YD. Exosomes Released by Islet-Derived Mesenchymal Stem Cells Trigger Autoimmune Responses in NOD Mice. Diabetes (2014) 63. doi: 10.2337/db13-0859
55. Rutman AK, Negi S, Gasparrini M, Hasilo CP, Tchervenkov J, Paraskevas S. Immune Response to Extracellular Vesicles From Human Islets of Langerhans in Patients With Type 1 Diabetes. Endocrinology (2018) 159(11):3834–47. doi: 10.1210/en.2018-00649
56. Xiao Y, Zheng L, Zou X, Wang J, Zhong J, Zhong T. Extracellular vesicles in type 2 diabetes mellitus: key roles in pathogenesis, complications, and therapy. J Extracell Vesicles (2019) 8(1):1625677–1625677. doi: 10.1080/20013078.2019.1625677
57. Zhang Y, Shi L, Mei H, Zhang J, Zhu Y, Han X, et al. Inflamed macrophage microvesicles induce insulin resistance in human adipocytes. Nutr Metab (2015) 12:21–1. doi: 10.1186/s12986-015-0016-3
58. Polina ER, Oliveira FM, Sbruzzi RC, Crispim D, Canani LH, Santos KG. Gene polymorphism and plasma levels of miR-155 in diabetic retinopathy. Endocr Connect (2019) 8(12):1591–9. doi: 10.1530/EC-19-0446
59. Zhang Y, Mei H, Chang X, Chen F, Zhu Y, Han X. Adipocyte-derived microvesicles from obese mice induce M1 macrophage phenotype through secreted miR-155. J Mol Cell Biol (2016) 8(6):505–17. doi: 10.1093/jmcb/mjw040
60. Guo Q, Wang Y, Xu D, Nossent J, Pavlos NJ, Xu J. Rheumatoid arthritis: pathological mechanisms and modern pharmacologic therapies. Bone Res (2018) 6:15. doi: 10.1038/s41413-018-0016-9
61. Vinuela-Berni V, Doniz-Padilla L, Figueroa-Vega N, Portillo-Salazar H, Abud-Mendoza C, Baranda L, et al. Proportions of several types of plasma and urine microparticles are increased in patients with rheumatoid arthritis with active disease. Clin Exp Immunol (2015) 180(3):442–51. doi: 10.1111/cei.12598
62. Gyorgy B, Szabo TG, Turiak L, Wright M, Herczeg P, Ledeczi Z, et al. Improved flow cytometric assessment reveals distinct microvesicle (cell-derived microparticle) signatures in joint diseases. PloS One (2012) 7(11):e49726. doi: 10.1371/journal.pone.0049726
63. Withrow J, Murphy C, Liu Y, Hunter M, Fulzele S, Hamrick MW. Extracellular vesicles in the pathogenesis of rheumatoid arthritis and osteoarthritis. Arthritis Res Ther (2016) 18(1):286. doi: 10.1186/s13075-016-1178-8
64. Rashighi M, Harris JE. Vitiligo Pathogenesis and Emerging Treatments. Dermatol Clin (2017) 35(2):257–65. doi: 10.1016/j.det.2016.11.014
65. Xie H, Zhou F, Liu L, Zhu G, Li Q, Li C, et al. Vitiligo: How do oxidative stress-induced autoantigens trigger autoimmunity? J Dermatol Sci (2016) 81(1):3–9. doi: 10.1016/j.jdermsci.2015.09.003
66. van den Boorn JG, Konijnenberg D, Dellemijn TA, van der Veen JP, Bos JD, Melief CJ, et al. Autoimmune destruction of skin melanocytes by perilesional T cells from vitiligo patients. J Invest Dermatol (2009) 129(9):2220–32. doi: 10.1038/jid.2009.32
67. Sibaud V. Dermatologic Reactions to Immune Checkpoint Inhibitors : Skin Toxicities and Immunotherapy. Am J Clin Dermatol (2018) 19(3):345–61. doi: 10.1007/s40257-017-0336-3
68. Sosa A, Lopez Cadena E, Simon Olive C, Karachaliou N, Rosell R. Clinical assessment of immune-related adverse events. Ther Adv Med Oncol (2018) 10:1758835918764628. doi: 10.1177/1758835918764628
69. Teulings H-E, Limpens J, Jansen SN, Zwinderman AH, Reitsma JB, Spuls PI, et al. Vitiligo-Like Depigmentation in Patients With Stage III-IV Melanoma Receiving Immunotherapy and Its Association With Survival: A Systematic Review and Meta-Analysis. J Clin Oncol (2015) 33(7):773–81. doi: 10.1200/jco.2014.57.4756
70. Freeman-Keller M, Kim Y, Cronin H, Richards A, Gibney G, Weber JS. Nivolumab in Resected and Unresectable Metastatic Melanoma: Characteristics of Immune-Related Adverse Events and Association with Outcomes. Clin Cancer Res (2016) 22(4):886–94. doi: 10.1158/1078-0432.CCR-15-1136
71. Duley L. The global impact of pre-eclampsia and eclampsia. Semin Perinatol (2009) 33(3):130–7. doi: 10.1053/j.semperi.2009.02.010
72. Al-Jameil N, Aziz Khan F, Fareed Khan M, Tabassum H. A brief overview of preeclampsia. J Clin Med Res (2014) 6(1):1–7. doi: 10.4021/jocmr1682w
73. Kohli S, Ranjan S, Hoffmann J, Kashif M, Daniel EA, Al-Dabet MM, et al. Maternal extracellular vesicles and platelets promote preeclampsia via inflammasome activation in trophoblasts. Blood (2016) 128(17):2153–64. doi: 10.1182/blood-2016-03-705434
74. Han C, Han L, Huang P, Chen Y, Wang Y, Xue F. Syncytiotrophoblast-Derived Extracellular Vesicles in Pathophysiology of Preeclampsia. Front Physiol (2019) 10:1236:1236. doi: 10.3389/fphys.2019.01236
75. Salomon C, Yee SW, Mitchell MD, Rice GE. The possible role of extravillous trophoblast-derived exosomes on the uterine spiral arterial remodeling under both normal and pathological conditions. BioMed Res Int (2014) 2014:693157–7. doi: 10.1155/2014/693157
76. Salomon C, Ryan J, Sobrevia L, Kobayashi M, Ashman K, Mitchell M, et al. Exosomal signaling during hypoxia mediates microvascular endothelial cell migration and vasculogenesis. PloS One (2013) 8(7):e68451. doi: 10.1371/journal.pone.0068451
77. Salomon C, Kobayashi M, Ashman K, Sobrevia L, Mitchell MD, Rice GE. Hypoxia-induced changes in the bioactivity of cytotrophoblast-derived exosomes. PloS One (2013) 8(11):e79636. doi: 10.1371/journal.pone.0079636
78. Collett GP RC, Sargent IL, Vatish M. Endoplasmic reticulum stress stimulates the release of extracellular vesicles carrying danger-associated molecular pattern (DAMP) molecules. Oncotarget (2018) 6:6707–17. doi: 10.18632/oncotarget.24158
79. O’Neill CP, Gilligan KE, Dwyer RM. Role of Extracellular Vesicles (EVs) in Cell Stress Response and Resistance to Cancer Therapy. Cancers (Basel) (2019) 11(2):136. doi: 10.3390/cancers11020136
81. Casado-Díaz A, Quesada-Gómez JM, Dorado G. Extracellular Vesicles Derived From Mesenchymal Stem Cells (MSC) in Regenerative Medicine: Applications in Skin Wound Healing. Front Bioeng Biotechnol (2020) 8:146:146. doi: 10.3389/fbioe.2020.00146
82. Mokarizadeh A, Delirezh N, Morshedi A, Mosayebi G, Farshid AA, Mardani K. Microvesicles derived from mesenchymal stem cells: potent organelles for induction of tolerogenic signaling. Immunol Lett (2012) 147(1-2):47–54. doi: 10.1016/j.imlet.2012.06.001
83. Riazifar M, Mohammadi MR, Pone EJ, Yeri A, Lasser C, Segaliny AI, et al. Stem Cell-Derived Exosomes as Nanotherapeutics for Autoimmune and Neurodegenerative Disorders. ACS Nano (2019) 13(6):6670–88. doi: 10.1021/acsnano.9b01004
84. Bai L, Shao H, Wang H, Zhang Z, Su C, Dong L, et al. Effects of Mesenchymal Stem Cell-Derived Exosomes on Experimental Autoimmune Uveitis. Sci Rep (2017) 7(1):4323. doi: 10.1038/s41598-017-04559-y
85. Tao SC, Yuan T, Zhang YL, Yin WJ, Guo SC, Zhang CQ. Exosomes derived from miR-140-5p-overexpressing human synovial mesenchymal stem cells enhance cartilage tissue regeneration and prevent osteoarthritis of the knee in a rat model. Theranostics (2017) 7(1):180–95. doi: 10.7150/thno.17133
86. Zheng J, Zhu L, Iok In I, Chen Y, Jia N, Zhu W. Bone marrow-derived mesenchymal stem cells-secreted exosomal microRNA-192-5p delays inflammatory response in rheumatoid arthritis. Int Immunopharmacol (2020) 78:105985. doi: 10.1016/j.intimp.2019.105985
87. Cosenza S, Ruiz M, Toupet K, Jorgensen C, Noel D. Mesenchymal stem cells derived exosomes and microparticles protect cartilage and bone from degradation in osteoarthritis. Sci Rep (2017) 7(1):16214. doi: 10.1038/s41598-017-15376-8
88. Diederichs S, Tuan RS. Functional comparison of human-induced pluripotent stem cell-derived mesenchymal cells and bone marrow-derived mesenchymal stromal cells from the same donor. Stem Cells Dev (2014) 23(14):1594–610. doi: 10.1089/scd.2013.0477
89. Kim S, Lee SK, Kim H, Kim TM. Exosomes Secreted from Induced Pluripotent Stem Cell-Derived Mesenchymal Stem Cells Accelerate Skin Cell Proliferation. Int J Mol Sci (2018) 19(10):3119. doi: 10.3390/ijms19103119
90. Zhu Y, Wang Y, Zhao B, Niu X, Hu B, Li Q, et al. Comparison of exosomes secreted by induced pluripotent stem cell-derived mesenchymal stem cells and synovial membrane-derived mesenchymal stem cells for the treatment of osteoarthritis. Stem Cell Res Ther (2017) 8(1):64. doi: 10.1186/s13287-017-0510-9
91. Sousa C, Pereira I, Santos AC, Carbone C, Kovačević AB, Silva AM, et al. Targeting dendritic cells for the treatment of autoimmune disorders. Colloids Surf B Biointerfaces (2017) 158:237–48. doi: 10.1016/j.colsurfb.2017.06.050
92. Kim K. KS, Choi S, Youn B, Kim H. Extracellular Vesicles as Drug Delivery Vehicles for Rheumatoid Arthritis. Curr Stem Cell Res Ther (2016) 11(4):329–42. doi: 10.2174/1574888x11666151203223251
93. Cai Z, Zhang W, Yang F, Yu L, Yu Z, Pan J, et al. Immunosuppressive exosomes from TGF-beta1 gene-modified dendritic cells attenuate Th17-mediated inflammatory autoimmune disease by inducing regulatory T cells. Cell Res (2012) 22(3):607–10. doi: 10.1038/cr.2011.196
94. Wan Y, Wang L, Zhu C, Zheng Q, Wang G, Tong J, et al. Aptamer-conjugated extracellular nanovesicles for targeted drug delivery. Cancer Res (2017) 78(3):798–808. doi: 10.1158/0008-5472.CAN-17-2880
95. Fang RH, Hu C-MJ, Luk BT, Gao W, Copp JA, Tai Y, et al. Cancer Cell Membrane-Coated Nanoparticles for Anticancer Vaccination and Drug Delivery. Nano Lett (2014) 14(4):2181–8. doi: 10.1021/nl500618u
96. Tauro BJ, Greening DW, Mathias RA, Ji H, Mathivanan S, Scott AM, et al. Comparison of ultracentrifugation, density gradient separation, and immunoaffinity capture methods for isolating human colon cancer cell line LIM1863-derived exosomes. Methods (2012) 56(2):293–304. doi: 10.1016/j.ymeth.2012.01.002
97. Sun D, Zhuang X, Xiang X, Liu Y, Zhang S, Liu C, et al. A novel nanoparticle drug delivery system: the anti-inflammatory activity of curcumin is enhanced when encapsulated in exosomes. Mol Ther (2010) 18(9):1606–14. doi: 10.1038/mt.2010.105
98. Saari H, Lazaro-Ibanez E, Viitala T, Vuorimaa-Laukkanen E, Siljander P, Yliperttula M. Microvesicle- and exosome-mediated drug delivery enhances the cytotoxicity of Paclitaxel in autologous prostate cancer cells. J Control Release (2015) 220(Pt B):727–37. doi: 10.1016/j.jconrel.2015.09.031
99. O’Brien K, Lowry MC, Corcoran C, Martinez VG, Daly M, Rani S, et al. miR-134 in extracellular vesicles reduces triple-negative breast cancer aggression and increases drug sensitivity. Oncotarget (2015) 6(32):32774–89. doi: 10.18632/oncotarget.5192
100. Haney MJ, Zhao Y, Jin YS, Batrakova EV. Extracellular Vesicles as Drug Carriers for Enzyme Replacement Therapy to Treat CLN2 Batten Disease: Optimization of Drug Administration Routes. Cells (2020) 9(5):1273. doi: 10.3390/cells9051273
101. Haney MJ, Zhao Y, Fay J, Duhyeong H, Wang M, Wang H, et al. Genetically modified macrophages accomplish targeted gene delivery to the inflamed brain in transgenic Parkin Q311X(A) mice: importance of administration routes. Sci Rep (2020) 10(1):11818. doi: 10.1038/s41598-020-68874-7
102. Haney MJ, Zhao Y, Jin YS, Li SM, Bago JR, Klyachko NL, et al. Macrophage-Derived Extracellular Vesicles as Drug Delivery Systems for Triple Negative Breast Cancer (TNBC) Therapy. J Neuroimmune Pharmacol (2020) 15(3):487–500. doi: 10.1007/s11481-019-09884-9
103. Kooijmans SA, Aleza CG, Roffler SR, van Solinge WW, Vader P, Schiffelers RM. Display of GPI-anchored anti-EGFR nanobodies on extracellular vesicles promotes tumour cell targeting. J Extracell Vesicles (2016) 5:31053. doi: 10.3402/jev.v5.31053
104. Li P, Kaslan M, Lee SH, Yao J, Gao Z. Progress in Exosome Isolation Techniques. Theranostics (2017) 7(3):789–804. doi: 10.7150/thno.18133
105. Coumans FAW, Brisson AR, Buzas EI, Dignat-George F, Drees EEE, El-Andaloussi S, et al. Methodological Guidelines to Study Extracellular Vesicles. Circ Res (2017) 120(10):1632. doi: 10.1161/CIRCRESAHA.117.309417
106. Szatanek R, Baran J, Siedlar M, Baj-Krzyworzeka M. Isolation of extracellular vesicles: Determining the correct approach (Review). Int J Mol Med (2015) 36(1):11–7. doi: 10.3892/ijmm.2015.2194
107. Liga A, Vliegenthart ADB, Oosthuyzen W, Dear JW, Kersaudy-Kerhoas M. Exosome isolation: a microfluidic road-map. Lab Chip (2015) 15(11):2388–94. doi: 10.1039/C5LC00240K
108. Momen-Heravi F. Isolation of Extracellular Vesicles by Ultracentrifugation. In: Kuo WP, Jia S, editors. Extracellular Vesicles: Methods and Protocols. New York, NY: Springer New York (2017). doi: 10.1007/978-1-4939-7253-1_3
109. Freitas D, Balmaña M, Poças J, Campos D, Osório H, Konstantinidi A, et al. Different isolation approaches lead to diverse glycosylated extracellular vesicle populations. J Extracell Vesicles (2019) 8(1):1621131. doi: 10.1080/20013078.2019.1621131
110. Lobb RJ, Becker M, Wen SW, Wong CSF, Wiegmans AP, Leimgruber A, et al. Optimized exosome isolation protocol for cell culture supernatant and human plasma. J Extracell Vesicles (2015) 4:27031–1. doi: 10.3402/jev.v4.27031
111. Kowal J, Arras G, Colombo M, Jouve M, Morath JP, Primdal-Bengtson B, et al. Proteomic comparison defines novel markers to characterize heterogeneous populations of extracellular vesicle subtypes. Proc Natl Acad Sci (2016) 113(8):E968–77. doi: 10.1073/pnas.1521230113
112. Ludwig N, Razzo BM, Yerneni SS, Whiteside TL. Optimization of cell culture conditions for exosome isolation using mini-size exclusion chromatography (mini-SEC). Exp Cell Res (2019) 378(2):149–57. doi: 10.1016/j.yexcr.2019.03.014
113. Corso G, Mäger I, Lee Y, Görgens A, Bultema J, Giebel B, et al. Reproducible and scalable purification of extracellular vesicles using combined bind-elute and size exclusion chromatography. Sci Rep (2017) 7(1):11561. doi: 10.1038/s41598-017-10646-x
114. Böing AN, van der Pol E, Grootemaat AE, Coumans FAW, Sturk A, Nieuwland R. Single-step isolation of extracellular vesicles by size-exclusion chromatography. J Extracell Vesicles (2014) 3(1):23430. doi: 10.3402/jev.v3.23430
115. Heath N, Grant L, De Oliveira TM, Rowlinson R, Osteikoetxea X, Dekker N, et al. Rapid isolation and enrichment of extracellular vesicle preparations using anion exchange chromatography. Sci Rep (2018) 8(1):5730. doi: 10.1038/s41598-018-24163-y
116. Weng Y, Sui Z, Shan Y, Hu Y, Chen Y, Zhang L, et al. Effective isolation of exosomes with polyethylene glycol from cell culture supernatant for in-depth proteome profiling. Analyst (2016) 141(15):4640–6. doi: 10.1039/C6AN00892E
117. Gallart-Palau X, Serra A, Wong ASW, Sandin S, Lai MKP, Chen CP, et al. Extracellular vesicles are rapidly purified from human plasma by PRotein Organic Solvent PRecipitation (PROSPR). Sci Rep (2015) 5:14664–4. doi: 10.1038/srep14664
118. Gao X, Ran N, Dong X, Zuo B, Yang R, Zhou Q, et al. Anchor peptide captures, targets, and loads exosomes of diverse origins for diagnostics and therapy. Sci Trans Med (2018) 10(444):eaat0195. doi: 10.1126/scitranslmed.aat0195
119. Carney RP, Hazari S, Rojalin T, Knudson A, Gao T, Tang Y, et al. Targeting Tumor-Associated Exosomes with Integrin-Binding Peptides. Adv Biosyst (2017) 1(5):1600038–n/a. doi: 10.1002/adbi.201600038
120. Nakai W, Yoshida T, Diez D, Miyatake Y, Nishibu T, Imawaka N, et al. A novel affinity-based method for the isolation of highly purified extracellular vesicles. Sci Rep (2016) 6:33935. doi: 10.1038/srep33935
121. Melo SA, Luecke LB, Kahlert C, Fernandez AF, Gammon ST, Kaye J, et al. Glypican-1 identifies cancer exosomes and detects early pancreatic cancer. Nature (2015) 523(7559):177–82. doi: 10.1038/nature14581
122. Tian Y, Gong M, Hu Y, Liu H, Zhang W, Zhang M, et al. Quality and efficiency assessment of six extracellular vesicle isolation methods by nano-flow cytometry. J Extracell Vesicles (2020) 9(1):1697028. doi: 10.1080/20013078.2019.1697028
123. Chen C, Skog J, Hsu C-H, Lessard RT, Balaj L, Wurdinger T, et al. Microfluidic isolation and transcriptome analysis of serum microvesicles. Lab Chip (2010) 10(4):505–11. doi: 10.1039/b916199f
124. Wan Y, Maurer M, He H-Z, Xia Y-Q, Hao S-J, Zhang W-L, et al. Enrichment of extracellular vesicles with lipid nanoprobe functionalized nanostructured silica. Lab Chip (2019) 19(14):2346–55. doi: 10.1039/C8LC01359D
125. Jo W, Kim J, Yoon J, Jeong D, Cho S, Jeong H, et al. Large-scale generation of cell-derived nanovesicles. Nanoscale (2014) 6(20):12056–64. doi: 10.1039/C4NR02391A
126. Jo W, Jeong D, Kim J, Cho S, Jang SC, Han C, et al. Microfluidic fabrication of cell-derived nanovesicles as endogenous RNA carriers. Lab Chip (2014) 14(7):1261–9. doi: 10.1039/C3LC50993A
127. Jang SC, Kim OY, Yoon CM, Choi D-S, Roh T-Y, Park J, et al. Bioinspired Exosome-Mimetic Nanovesicles for Targeted Delivery of Chemotherapeutics to Malignant Tumors. ACS Nano (2013) 7(9):7698–710. doi: 10.1021/nn402232g
128. Cheng G, Li W, Ha L, Han X, Hao S, Wan Y, et al. Self-Assembly of Extracellular Vesicle-like Metal–Organic Framework Nanoparticles for Protection and Intracellular Delivery of Biofunctional Proteins. J Am Chem Soc (2018) 140(23):7282–91. doi: 10.1021/jacs.8b03584
129. Parodi A, Quattrocchi N, van de Ven AL, Chiappini C, Evangelopoulos M, Martinez JO, et al. Synthetic nanoparticles functionalized with biomimetic leukocyte membranes possess cell-like functions. Nat Nanotechnol (2013) 8(1):61–8. doi: 10.1038/nnano.2012.212
130. Yoon J, Jo W, Jeong D, Kim J, Jeong H, Park J. Generation of nanovesicles with sliced cellular membrane fragments for exogenous material delivery. Biomaterials (2015) 59:12–20. doi: 10.1016/j.biomaterials.2015.04.028
131. Li Y, Zhang X, Lin J, Li R, Yue T. Extracting lipid vesicles from plasma membranes via self-assembly of clathrin-inspired scaffolding nanoparticles. Colloids Surf B Biointerfaces (2019) 176:239–48. doi: 10.1016/j.colsurfb.2019.01.008
132. Tehrani S, Antovic A, Mobarrez F, Mageed K, Lins PE, Adamson U, et al. High-dose aspirin is required to influence plasma fibrin network structure in patients with type 1 diabetes. Diabetes Care (2012) 35(2):404–8. doi: 10.2337/dc11-1302
133. Tyndall A, Dazzi F. Chronic GVHD as an autoimmune disease. Best Pract Res Clin Haematol (2008) 21(2):281–9. doi: 10.1016/j.beha.2008.03.003
134. Kordelas L, Rebmann V, Ludwig AK, Radtke S, Ruesing J, Doeppner TR, et al. MSC-derived exosomes: a novel tool to treat therapy-refractory graft-versus-host disease. Leukemia (2014) 28:970. doi: 10.1038/leu.2014.41
135. Zhao Y, Jiang Z, Zhao T, Ye M, Hu C, Yin Z, et al. Reversal of type 1 diabetes via islet β cell regeneration following immune modulation by cord blood-derived multipotent stem cells. BMC Med (2012) 10(1):3. doi: 10.1186/1741-7015-10-3
136. Ezquer F, Ezquer M, Contador D, Ricca M, Simon V, Conget P. The antidiabetic effect of mesenchymal stem cells is unrelated to their transdifferentiation potential but to their capability to restore Th1/Th2 balance and to modify the pancreatic microenvironment. Stem Cells (2012) 30(8):1664–74. doi: 10.1002/stem.1132
137. Smith MH, Bass AR. Arthritis After Cancer Immunotherapy: Symptom Duration and Treatment Response. Arthritis Care Res (Hoboken) (2019) 71(3):362–6. doi: 10.1002/acr.23467
Keywords: extracellular vesicle, autoimmunity, antigen presentation, immune-related adverse events, therapeutic delivery
Citation: Lu M, DiBernardo E, Parks E, Fox H, Zheng S-Y and Wayne E (2021) The Role of Extracellular Vesicles in the Pathogenesis and Treatment of Autoimmune Disorders. Front. Immunol. 12:566299. doi: 10.3389/fimmu.2021.566299
Received: 27 May 2020; Accepted: 04 January 2021;
Published: 24 February 2021.
Edited by:
Ankur Singh, Georgia Institute of Technology, United StatesReviewed by:
Bergithe Eikeland Oftedal, University of Bergen, NorwayWesley H. Brooks, University of South Florida, United States
Copyright © 2021 Lu, DiBernardo, Parks, Fox, Zheng and Wayne. This is an open-access article distributed under the terms of the Creative Commons Attribution License (CC BY). The use, distribution or reproduction in other forums is permitted, provided the original author(s) and the copyright owner(s) are credited and that the original publication in this journal is cited, in accordance with accepted academic practice. No use, distribution or reproduction is permitted which does not comply with these terms.
*Correspondence: Elizabeth Wayne, ZXdheW5lQGFuZHJldy5jbXUuZWR1
†These authors have contributed equally to this work and share first authorship