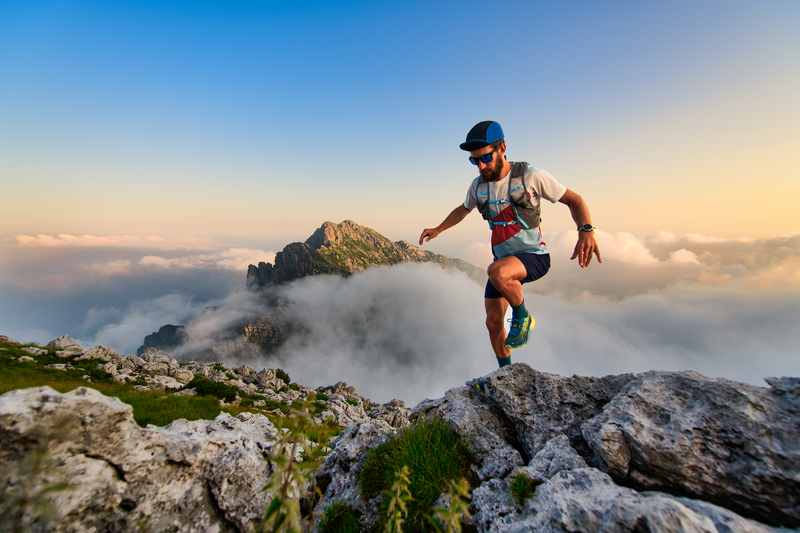
94% of researchers rate our articles as excellent or good
Learn more about the work of our research integrity team to safeguard the quality of each article we publish.
Find out more
ORIGINAL RESEARCH article
Front. Immunol. , 10 December 2020
Sec. Microbial Immunology
Volume 11 - 2020 | https://doi.org/10.3389/fimmu.2020.619574
This article is part of the Research Topic The Role of Dietary Interventions in The Regulation of Host-Microbe Interactions View all 24 articles
Cadmium (Cd) is a toxic metal inducing a range of adverse effects on organs including liver and kidneys. However, the underlying molecular mechanisms of Cd-induced intestinal toxicity through dietary intake is poorly studied. This study evaluated the toxic effects of Cd on intestinal physiology and confirmed the effectiveness of the protective mechanism of the probiotic Lactobacillus plantarum CCFM8610 against chronic Cd toxicity. After treatment with Cd, the HT-29 cell line was subjected to iTRAQ analysis, which revealed that changes in the proteomic profiles after Cd exposure were related to pathways involved in the stress response and carbohydrate metabolism. The results of an animal trial also indicated that 10 weeks of Cd exposure decreased the fecal water content and contractile response of colonic muscle strips in mice, and delayed the excretion time of the first black feces. L. plantarum CCFM8610 treatment provided protective effects against these Cd-induced intestinal motility dysfunctions by recovering the levels of neurotransmitters, including substance P, acetyl cholinesterase, vasoactive intestinal peptide, 5-hydroxytryptamine, calcitonin gene-related peptide, and nitric oxide, and suppressing the cellular stress response in mice (e.g., the inhibition of mitogen-activated protein kinase pathways). The administration of this probiotic was also observed to reduce Cd levels in the tissues and blood of the mice. Our results suggest a newly identified protective mechanism of probiotics against Cd toxicity that involves the recovery of intestinal motility and increase in fecal cadmium excretion.
Cadmium (Cd) is a heavy metal widely distributed in the environment. Once Cd has accumulated in the host, it can induce a range of adverse effects on the liver, kidney, brain, bone, and reproductive systems (1–4). The mechanisms of Cd toxicity have been well studied, including its competitive interference with the metabolism of essential divalent metals such as zinc and manganese, cytotoxicity induced by the abnormal production of reactive oxide species, and disruption of protein structures by the binding of Cd to sulfhydryl groups (5–7).
For the human population, food and drinking water are the main sources of Cd exposure (8). Research has shown that after oral intake, a major fraction of Cd (approximately 60%) is absorbed by the gut as a result of mucociliary clearance and subsequent ingestion (9). Although a number of studies have demonstrated that the liver, kidneys, and lungs are the main targets of long-term Cd exposure (10), reports on the toxic effects of this heavy metal on the gut are relatively limited. Animal studies have shown that the oral administration of Cd induce pathological changes in gastric mucosa and intestinal villi (11). Cross-sectional epidemiological studies have reported Cd exposure to be accompanied by increased incidence of intestinal abnormalities such as constipation, vomiting, nausea, anorexia, and indigestion (12, 13). Cd exposure has also been shown to significantly affect intestinal motility, which delays fecal Cd excretion and exacerbates intestinal Cd absorption (11). Several studies have indicated that Cd may strongly stimulate the intestinal mucosa, inhibit digestive enzymes, disrupt gut barrier function, and adversely impact gut immunity (11, 14, 15). However, the underlying molecular mechanisms of Cd-induced intestinal toxicity are not thoroughly understood.
A number of animal trials showed that probiotics, including Lactobacillus rhamnosus GG, L. rhamnosus LC705 (16), and a cocktail of Lactobacillus and Bifidobacterium strains (17) can alleviate Cd toxicity in vivo. The protective effects of these strains may be due to their Cd-binding ability, which promotes fecal excretion of this metal (18). In our previous studies, L. plantarum CCFM8610, a probiotic isolated from fermented Chinese food, showed good Cd-binding and Cd-tolerance abilities in vitro (19). Subsequent animal and human trials confirmed that the administration of this probiotic can significantly reduce the Cd burden in vivo (20, 21). Interestingly, we found this strain to also markedly alleviate Cd-induced constipation in mice (22). Cd exposure may be detrimental to host health through increasing intestinal permeability and inducing dysfunctions in gut microbiota (23). A recent study showed that Cd treatment could markedly decreased the microbial richness and the abundance of short chain fatty acid-producing bacteria in the gut of mice (24). Furthermore, it has been reported that the intestinal bacterial species diversity of mice decreased gradually with the increase of Cd concentration (25). A number of studies have reported that probiotics can promote intestinal motility by modulating the gut microbiota to produce more short-chain fatty acids and regulate bile acid metabolism (20, 26, 27). Therefore, we hypothesized that specific probiotics may inhibit the intestinal absorption of Cd and increase the fecal excretion of Cd by the recovery of intestinal motility. This represents a new understanding of the mechanism underlying the protective effects of probiotics against Cd toxicity.
Based on these analyses, the aims of the present study were to perform a proteomic analysis to investigate the toxic mechanism of Cd on intestinal physiology. Experiments were also conducted to evaluate the protective effects of L. plantarum CCFM8610 against the Cd-induced depression of gut motility in mice.
Fetal bovine serum (FBS), RPMI-1640 medium, trypsin, penicillin, and streptomycin were obtained from Gibco (Waltham, MA, USA). Cadmium chloride (CdCl2) and other analytical-grade laboratory reagents were obtained from Sinopharm Chemical Reagent Company (Shanghai, China). iTRAQ reagents were purchased from Applied Biosystems (Waltham, MA, USA).
Kits purchased from SenBeiJia Biotechnology (Nanjing, China) were used to determine the contents of nitric oxide (NO), 5-hydroxytryptamine (5-HT), substance P, acetylcholinesterase (AChE), vasoactive intestinal peptide (VIP), and calcitonin gene-related peptide (CGRP). TRIzol reagent was obtained from Life Technologies (Waltham, MA, USA). Reverse transcription was performed using a kit purchased from Takara Bio (Beijing, China).
HT-29 cells were conventionally incubated in RPMI-1640 medium containing 1% penicillin/streptomycin and 10% FBS. Cells were cultured at 37°C in a 5% CO2 moist atmosphere, and the medium was refreshed every 2 days. The cells were digested with trypsin and diluted to a 1:3 ratio for passage when the confluence reached 80–90%.
In the logarithmic growth phase, cells were grown in a six-well plate at a density of 1 × 106 cells per well. After cell adhesion, the cells were divided into Control and Cd groups for treatment, as shown in Table 1. The dose of Cd exposure (50 μM CdCl2) was selected to mimic a moderate toxic effect of Cd on intestinal epithelial cells (28). After 24-h incubation, cells were collected for iTRAQ proteomic analysis by the method shown in Supplemental Figure 1 (29). Protein Pilot Software V. 5.0 (AB Sciex, Beijing, China) was used for data processing. The identification of proteins and screening of differentially expressed proteins were performed as described in a previous study (29). The mass spectrometry proteomics data have been deposited to the ProteomeXchange Consortium (http://proteomecentral.proteomexchange.org) via the iProX partner repository (30) with the dataset identifier PXD022209.
The incubation and lyophilization of L. plantarum CCFM8610 were performed as previously reported (21).
C57BL/6 mice (4 weeks of age) obtained from the Shanghai Laboratory Animal Center (Shanghai, China) were used in the experiments. The mice were kept in a temperature- and humidity-controlled room that was set to maintain a 12-h light/12-h dark cycle. The mice were fed standard commercial rat chow, and water was provided ad libitum. All the protocols of the study were approved by the Ethics Committee of Jiangnan University, China (JN. No. 20170618-20170918), and all the study procedures were performed in accordance with European Union guidelines (Directive 2010/63/EU) for the care and use of experimental animals.
After a 1-week adaptation period, 30 mice were divided into three groups, including the Control group, Cd group, and Cd plus CCFM8610 group (Table 2). The concentration of CdCl2 was selected based on the results of our previous study to mimic chronic oral Cd exposure (19). After a 10-week treatment, the mice were anesthetized and sacrificed. The blood, feces, and tissues of the mice were then collected for analysis.
During the experiment, each mouse was housed in a pathogen-free cage and fecal samples were collected weekly. The fecal water content was determined using a method previously described (31).
The mice were fasted overnight and then gavaged with 0.2 ml of activated carbon solution. They were then given free access to food and water, and the time between the gavage and the appearance of their first darkened feces was recorded.
At the end of the experiment, each mouse was fasted overnight and the GI transit time was determined by recording the length of the small intestine and the distance traveled by the activated carbon in the intestine (32).
The concentrations of NO, 5-HT, substance P, AchE, VIP, and CGRP in the colon and jejunum were determined using the kits, according to the manufacturer’s instructions.
A quantitative reverse transcription polymerase chain reaction (RT-qPCR) was performed using a Bio-Rad CFX96TM Real-Time System (Shanghai, China), as previously reported (29). All the primers used in this test are listed in Supplemental Table 1 (33). All assays were repeated three times, and the 2-△△Ct method was used to analyze the data.
The concentrations of Cd in the liver, kidneys, and blood were measured using a graphite furnace atomic absorption spectrophotometer (Varian, USA) (22).
The data are presented as means ± SD. A one-way analysis of variance was used for the intergroup analysis, with the differences considered to be statistically significant at P < 0.05. GraphPad Prism 8.0 (GraphPad Software Inc., San Diego, CA, USA) and OriginPro 8.5 (OriginLab Corporation, Northampton, MA, USA) were used for statistical analysis and construction.
A total of 4,089 proteins were detected and identified by iTRAQ analysis (Figure 1A). Based on the screening criteria of a fold change >2.0 and P value <0.05, Cd exposure was found to induce the differential expression of 168 proteins (Figure 1B). Gene ontology (GO) analysis (Figure 2) indicated that the differentially expressed proteins after Cd exposure had a wide range of functions that could be categorized as biological processes, cellular components, and molecular functions. The relevant metabolic pathways were related to stress response (19 proteins), membrane protein and other cell structure proteins (34 proteins), carbohydrate metabolism (13 proteins), lipid metabolism (10 proteins), amino acid metabolism (8 proteins), translation (8 proteins), etc. The Kyoto Encyclopedia of Genes and Genomes (KEGG) classification (Supplemental Figure 2) showed similar trends in the Cd-induced alterations in protein expression.
Figure 1 Differentially expressed proteins in HT-29 cells after Cd exposure. (A) volcano plots showing all detected proteins. The red dots represent the differentially expressed proteins after Cd exposure P-value of <0.05 with fold change of >2 or fold change of <0.5. The black dots are proteins with no significant changes. (B) the number of up-regulated and down-regulated genes responsible for differentially expressed proteins.
Figure 2 Functional categories of differentially expressed proteins after Cd-exposure by GO analysis.
Of the proteins altered in the global stress response (Supplemental Table 2), eight heat-shock proteins were upregulated by a factor of 2–3 after Cd exposure. Cd also induced a 6.2-fold increase in the expression of a heme oxygenase protein HMOX1 (P09601). The expression of M6PR (P20645) protein, a mannose-6-phosphate receptor related to lysosomal transport and cell apoptosis, increased by a factor of 8.8 after Cd exposure. Some proteins responsible for protein synthesis and hydrolysis (e.g. TGM2, PSMG2, and PREP), the glycolytic pathway (e.g. ACSS1 and ALDOC), and lipid metabolism (e.g. PEBP1, CMBL, and PEBP1) were downregulated in the Cd-treated group. Other proteins associated with cellular ribosome synthesis (e.g., TCOF1 and GTPBP4) and the maintenance of cellular morphology (e.g., FLNB and FLNA) were upregulated in response to Cd stimulation.
A chronic oral Cd exposure induced decreases in fecal water content and inhibited the GI transit ability of the mice (Figure 3). Treatment with L. plantarum CCFM8610 caused some recovery of the gut motility-related parameters of the mice, especially the time of the excretion of their first black feces (P < 0.05).
Figure 3 Effects of L. plantarum CCFM8610 on Cd-induced alterations in intestinal motility of mice. (A) Fecal water content of mice. (B) Small intestinal transit rate of mice. (C) First black stool defecation time of mice. The letters a, b and c mean the data of groups with different letters differ significantly (P < 0.05).
The levels of the neurotransmitters AchE, VIP, and 5-HT in the colon were significantly reduced by Cd exposure, whereas the CGRP and NO levels were increased (P < 0.05, Figure 4). These alterations were reversed after the administration of L. plantarum CCFM8610. Similar protective effects on the levels of VIP and CGRP of this probiotic were observed in the jejunum (Supplemental Figure 3).
Figure 4 Effects of L. plantarum CCFM8610 on Cd-induced alterations in the levels of AchE (A), VIP (B), 5-HT (C), CGRP (D), and NO (E) in the colon of mice. The letters a and b mean the data of groups with different letters differ significantly P < 0.05). AchE, acetylcholinesterase; VIP, vasoactive intestinal peptide; 5-HT, 5-hydroxytryptamine; CGRP, calcitonin gene-related peptide; NO, nitric oxide.
Cd exposure induced significant extracellular regulated protein kinases (ERK) and c-Jun N-terminal kinase (JNK) expressions in the colon and jejunum of the mice (P < 0.05, Figures 5A, B, and Supplemental Figures 4A, B). The L. plantarum CCFM8610 treatment induced marked recovery of the alterations in these two genes, and increased the expression of B-cell lymphoma-2 (bcl-2) in the gut (Figure 5C, Supplemental Figure 3).
Figure 5 Effects of L. plantarum CCFM8610 on Cd-induced alterations in the expression of ERK (A), JNK (B), and bcl-2 (C) in the colon of mice. The letters a and b mean the data of groups with different letters differ significantly (P < 0.05). ERK, extracellular regulated protein kinases; JNK, c-Jun N-terminal kinase; Bcl-2, B-cell lymphoma-2.
Compared with the control group, Cd exposure caused significant increases in the Cd levels in the tissues and blood of the mice (P < 0.05, Figure 6). The L. plantarum CCFM8610 treatment led to a marked reduction in the levels of Cd in the blood, liver, and kidneys of the mice (Figure 6).
Figure 6 Effects of L. plantarum CCFM8610 on the concentrations of Cd in the liver (A), kidney (B), and blood (C) of mice after Cd exposure. The letters a, b and c mean the data of groups with different letters differ significantly (P < 0.05).
The toxicity of Cd in the gut is attracting increasing attention because food-borne Cd is the main source of exposure to this environmental hazard (15). Besides, iTRAQ is a widely used approach for proteomic analysis that identifies and quantifies proteins using labeled peptides identifiable by sensitive mass spectrometers (34). This analysis is further strengthened by using robust bioinformatic tools and statistical analyses to support the observations (35). Some reviews have summarized the application of proteomic analysis on the studies of the toxicity of heavy metals including lead, arsenic and Cd (36, 37). Using the proteomic techniques is helpful for the screening of highly sensitive and specific biomarkers of metal exposure. To understand the molecular toxicity mechanism of Cd on enterocytes, alterations in the proteomic profiles after Cd exposure were evaluated (Figures 1, 2, and Supplemental Table 2). The results of iTRAQ analysis revealed that Cd treatment affected the expression of proteins involved in the stress response and cell apoptosis in enterocytes, which is consistent with previous findings regarding the cytotoxic effects of Cd exposure (38, 39). In support of these results, our animal trial showed that oral Cd exposure induced a significant toxic effect on intestinal physiology (Figure 3–6, Supplemental Figure 3, 4). Based on previous studies, heavy metals including copper and mercury can adversely affect gut physiology via inducing oxidative stress and inflammation and disrupting the metabolic homeostasis of the gut microbiota (40, 41). Mycotoxins such as deoxynivalenol, aflatoxin and ochratoxin have been reported to inhibit the production of intestinal mucins and damage the gut barrier (14, 42, 43). Some of these studies reported that the effects on MAPK pathway may be an important mechanism for the toxicity of these hazards.
Abnormal increase in the expression of heat-shock proteins may be accompanied by enhanced cell proliferation and increased tumor risk (44). The induction of heme oxygenase expression, which is reported to be beneficial against inflammation and tissue injury, may be a self-protective mechanism of the enterocytes against Cd exposure (45). Previous studies have indicated that NO can induce the expression of heme oxygenase-1 via activation of the ERK pathway (46, 47). This finding was confirmed by our in vivo results, which showed that Cd exposure increased the level of NO and the expression of ERK in the gut of mice (Figures 4 and 5). The most drastic changes in expression were observed in the cation-dependent mannose-6-phosphate receptor (increase by 8.8-fold), a protein associated with lysosomal transport and cell apoptosis (48), which indicates a strong Cd-induced stress response in enterocytes.
Among other proteins significantly affected by Cd exposure, the aldo-keto reductase family 1 member B10 has been reported to be involved in the CRAF-MEK-ERK pathway (49), which is consistent with the results of our animal experiment (Figure 5). Some proteins related to amino acid metabolism, such as N(G),N(G)-dimethylarginine dimethylaminohydrolase 1 and 2, were downregulated by the Cd exposure (Supplemental Table 2). This can explain the increase in the intestinal NO levels in the gut of Cd-exposed mice, as these enzymes play a role in reducing the production and bioavailability of NO (50).
Previous studies have shown that specific probiotics can provide significant protective effects against Cd toxicity in vivo (17, 22). The reported mechanisms include intestinal Cd sequestration, anti-oxidative stress action, and bile acid modulation (19, 21). In the present study, we also noticed that the administration of L. plantarum CCFM8610 alleviated Cd-induced intestinal motility disorders (Figure 3A). Such protective effects may increase the fecal excretion of Cd and inhibit the absorption of this metal in the gut (11). As such, it may represent an additional mechanism by which this strain reduces the Cd burden in the tissues and blood of mice (Figure 6). Previous studies have revealed the potential mechanism for the modulation of neurotransmitters by specific gut microbes. Gut microbiota promote colonic 5-HT production through an effect of short-chain fatty acids on enterochromaffin cells (51). Moreover, secondary bile acids produced by microbial biotransformation of cholate can stimulate neurotransmitters biosynthesis (52). Some lactobacillus strains such as L. reuteri and L. rhamnosus have been reported to activate GABA and 5-HT receptors in the gut, which can also explain the effects of probiotics on the levels of neurotransmitters (53, 54). In support of the proteomic analysis results, Cd exposure was also observed to increase the NO level in the intestines of mice (Figure 4E, Supplemental Figure 3E). This nitroso compound is reported to serve as an inhibitory transmitter in the gut of humans and animals (55, 56). Clinical studies have shown that the levels of NO and CGRP (a neuropeptide affecting a variety of gastrointestinal functions) are increased in patients with constipation (57, 58). The recovery of these two neurotransmitters by L. plantarum CCFM8610 treatment may therefore relieve the inhibitory effects of Cd on intestinal motility.
Substance P, AchE, VIP, and 5-HP play important roles in stimulating intestinal motility, relaxing enteric smooth muscle, and promoting defecation (59). Previous studies have reported decreased levels of these neurotransmitters in patients with idiopathic chronic constipation and constipation-predominant irritable bowel syndrome (60–62). The protective effects of L. plantarum CCFM8610 against Cd-induced intestinal motility disorders may also be due to the strain’s ability to increase the levels of these parameters. In support of our hypothesis, previous studies have shown that other probiotics, including L. plantarum 90sk and L. casei Qian, can modulate the expression of these neurotransmitters in rodents and humans (63–65).
Cd exposure has been reported to induce oxidative stress, which activates the mitogen-activated protein kinase (MAPK) signaling pathway and results in programmed cell death (66). This finding is consistent with our proteomic analysis results, as we found that proteins related to MAPK pathways (such as heme oxygenase-1 and heat shock protein-70) increased after Cd treatment. As shown in Figures 5A, B, and Supplemental Figures 4A, B, the expressions of protein kinases ERK and JNK were significantly increased after Cd exposure, further confirming the cytotoxic effects of Cd on enterocytes. Bcl-2 is known to be a key regulator of cell apoptosis and death (67). A previous study reported that the expression of bcl-2 can suppress Cd-induced cell death (68). L. plantarum CCFM8610 treatment induced the recovery of Cd-induced alterations in the expression of ERK and bcl-2, which indicates that this strain may inhibit Cd-activated programmed cell death and thereby protect intestinal neuronal cells. This finding may also explain the L. plantarum CCFM8610-mediated alleviation of the Cd-induced depression of intestinal motility. Consistent with our results, previous studies have shown that probiotics such as L. reuteri 6475 can suppress the ERK1/2 pathway in tumor necrosis factor-treated human myeloid leukemia-derived cells (69), and that a multispecies probiotic product increased the bcl-2 levels in constipated mice (70).
In this study, iTRAQ analyses were performed to evaluate the toxic effects of Cd on enterocytes. The results revealed that changes in the proteomic profiles after Cd exposure were related to pathways involved in the stress response and carbohydrate metabolism. The results of animal trials indicated that L. plantarum CCFM8610 treatment provided protective effects against dysfunctions of Cd-induced intestinal motility via the recovery of neurotransmitter levels and suppression of the cellular stress response in mice.
The datasets presented in this study can be found in online repositories. The names of the repository/repositories and accession number(s) can be found in the article/Supplementary Material.
The animal study was reviewed and approved by the Ethics Committee of Jiangnan University, China (JN. No. 20170618-20170918).
YL: Methodology, Software, Formal analysis, Visualization, Writing—original draft. JW: Writing—original draft, Methodology, Software. YX: Investigation, Formal analysis. QL: Software, Formal analysis. LY: Validation, Methodology, Investigation. FT: Validation, Investigation. JZ: Validation, Investigation. HZ: Validation, Investigation. QZ: Conceptualization, Writing—review and editing, Supervision, Funding acquisition. WC: Project administration, Funding acquisition. All authors contributed to the article and approved the submitted version.
This work was supported by the National Natural Science Foundation of China Program (No. 31871773, 32001665 and No. 31820103010); National First-Class Discipline Program of Food Science and Technology (JUFSTR20180102); the BBSRC Newton Fund Joint Centre Award; and Collaborative Innovation Center of Food Safety and Quality Control in Jiangsu Province.
The authors declare that the research was conducted in the absence of any commercial or financial relationships that could be construed as a potential conflict of interest.
The Supplementary Material for this article can be found online at: https://www.frontiersin.org/articles/10.3389/fimmu.2020.619574/full#supplementary-material
1. Andjelkovic M, Djordjevic AB, Antonijevic E, Antonijevic B, Stanic M, Kotur-Stevuljevic J, et al. Toxic Effect of Acute Cadmium and Lead Exposure in Rat Blood, Liver, and Kidney. Int J Env Res Pub He (2019) 16:1–21. doi: 10.3390/ijerph16020274
2. Ciesielski T, Weuve J, Bellinger DC, Schwartz J, Lanphear B, Wright RO. Cadmium exposure and neurodevelopmental outcomes in U.S. children. Environ Health Persp (2012) 120:758–63. doi: 10.1289/ehp.1104152
3. Kumar S, Sharma A. Cadmium toxicity: effects on human reproduction and fertility. Rev Environ Health (2019) 34:327–38. doi: 10.1515/reveh-2019-0016
4. Reyes-Hinojosa D, Lozada-Perez CA, Cuevas YZ, Lopez-Reyes A, Martinez-Nava G, Fernandez-Torres J, et al. Toxicity of cadmium in musculoskeletal diseases. Environ Toxicol Phar (2019) 72:103219. doi: 10.1016/j.etap.2019.103219
5. Cuypers A, Plusquin M, Remans T, Jozefczak M, Keunen E, Gielen H, et al. Cadmium stress: an oxidative challenge. Biometals (2010) 23:927–40. doi: 10.1007/s10534-010-9329-x
6. Moulis JM. Cellular mechanisms of cadmium toxicity related to the homeostasis of essential metals. Biometals (2010) 23:877–96. doi: 10.1007/s10534-010-9336-y
7. Valko M, Morris H, Cronin MT. Metals, toxicity and oxidative stress. Curr Med Chem (2005) 12:1161–208. doi: 10.2174/0929867053764635
8. Godt J, Scheidig F, Grosse-Siestrup C, Esche V, Brandenburg P, Reich A, et al. The toxicity of cadmium and resulting hazards for human health. J Occup Med Toxicol (2006) 1:22. doi: 10.1186/1745-6673-1-22
9. Breton J, Daniel C, Dewulf J, Pothion S, Froux N, Sauty M, et al. Gut microbiota limits heavy metals burden caused by chronic oral exposure. Toxicol Lett (2013) 222:132–8. doi: 10.1016/j.toxlet.2013.07.021
10. Zhang S, Jin Y, Zeng Z, Liu Z, Fu Z. Subchronic Exposure of Mice to Cadmium Perturbs Their Hepatic Energy Metabolism and Gut Microbiome. Chem Res Toxicol (2015) 28:2000–9. doi: 10.1021/acs.chemrestox.5b00237
11. Andersen O, Nielsen JB, Svendsen P. Oral cadmium chloride intoxication in mice: effects of dose on tissue damage, intestinal absorption and relative organ distribution. Toxicology (1988) 48:225–36. doi: 10.1016/0300-483x(88)90103-5
12. Landrigan PJ. Occupational and community exposures to toxic metals: lead, cadmium, mercury and arsenic. West J Med (1982) 137:531–9. doi: 10.0000/PMID7164433
13. Viaene MK, Masschelein R, Leenders J, De Groof M, Swerts LJ, Roels HA. Neurobehavioural effects of occupational exposure to cadmium: a cross sectional epidemiological study. Occup Environ Med (2000) 57:19–27. doi: 10.1136/oem.57.1.19
14. Luo S, Terciolo C, Bracarense A, Payros D, Pinton P, Oswald IP. In vitro and in vivo effects of a mycotoxin, deoxynivalenol, and a trace metal, cadmium, alone or in a mixture on the intestinal barrier. Environ Int (2019) 132:5082. doi: 10.1016/j.envint.2019.105082
15. Ninkov M, Popov AA, Demenesku J, Mirkov I, Mileusnic D, Petrovic A, et al. Toxicity of oral cadmium intake: Impact on gut immunity. Toxicol Lett (2015) 237:89–99. doi: 10.1016/j.toxlet.2015.06.002
16. Halttunen T, Salminen S, Tahvonen R. Rapid removal of lead and cadmium from water by specific lactic acid bacteria. Int J Food Microbiol (2007) 114:30–5. doi: 10.1016/j.ijfoodmicro.2006.10.040
17. Jama A, Mitic-Culafic D, Kolarevic S, Djurasevic S, Knezevic-Vukcevic J. Protective effect of probiotic bacteria against cadmium-induced genotoxicity in rat hepatocytes in vivo and in vitro. Arch Biol Sci (2012) 64:1197–206. doi: 10.2298/abs1203197j
18. Monachese M, Burton JP, Reid G. Bioremediation and tolerance of humans to heavy metals through microbial processes: a potential role for probiotics? Appl Environ Microbiol (2012) 78:6397–404. doi: 10.1128/AEM.01665-12
19. Zhai Q, Wang G, Zhao J, Liu X, Narbad A, Chen YQ, et al. Protective effects of Lactobacillus plantarum CCFM8610 against chronic cadmium toxicity in mice indicate routes of protection besides intestinal sequestration. Appl Environ Microbiol (2014) 80:4063–71. doi: 10.1128/AEM.00762-14
20. Bekkali NL, Bongers ME, Van den Berg MM, Liem O, Benninga MA. The role of a probiotics mixture in the treatment of childhood constipation: a pilot study. Nutr J (2007) 6:17. doi: 10.1186/1475-2891-6-17
21. Zhai Q, Liu Y, Wang C, Zhao J, Zhang H, Tian F, et al. Increased Cadmium Excretion Due to oral administration of lactobacillus plantarum strains by regulating enterohepatic circulation in mice. J Agric Food Chem (2019) 67:3956–65. doi: 10.1021/acs.jafc.9b01004
22. Zhai Q, Wang G, Zhao J, Liu X, Tian F, Zhang H, et al. Protective effects of Lactobacillus plantarum CCFM8610 against acute cadmium toxicity in mice. Appl Environ Microbiol (2013) 79:1508–15. doi: 10.1128/AEM.03417-12
23. Ya J, Ju ZQ, Wang HY, Zhao HF. Exposure to cadmium induced gut histopathological damages and microbiota alterations of Chinese toad (Bufo gargarizans) larvae. Ecotox Environ Safe (2019) 180:449–56. doi: 10.1016/j.ecoenv.2019.05.038
24. He XW, Qi ZD, Hou H, Qian L, Gao J, Zhang XX. Structural and functional alterations of gut microbiome in mice induced by chronic cadmium exposure. Chemosphere (2020) 246:125747. doi: 10.1016/j.chemosphere.2019.125747
25. Liu YH, Li YH, Xia YH, Liu KY, Ren LL, Ji YL. The dysbiosis of gut microbiota caused by low-dose cadmium aggravate the injury of mice liver through increasing intestinal permeability. Microorganisms (2020) 8:211. doi: 10.3390/microorganisms8020211
26. Dimidi E, Christodoulides S, Fragkos KC, Scott SM, Whelan K. The effect of probiotics on functional constipation in adults: a systematic review and meta-analysis of randomized controlled trials. Am J Clin Nutr (2014) 100:1075–84. doi: 10.3945/ajcn.114.089151
27. Ouwehand AC, Lagstrom H, Suomalainen T, Salminen S. Effect of probiotics on constipation, fecal azoreductase activity and fecal mucin content in the elderly. Ann Nutr Metab (2002) 46:159–62. doi: 10.1159/000063075
28. Zhai QX, Tian FW, Zhao JX, Zhang H, Narbad A, Chen W. Oral Administration of Probiotics Inhibits Absorption of the Heavy Metal Cadmium by Protecting the Intestinal Barrier. Appl Environ Microbiol (2016) 82:4429–40. doi: 10.1128/Aem.00695-16
29. Zhai Q, Xiao Y, Zhao J, Tian F, Zhang H, Narbad A, et al. Identification of key proteins and pathways in cadmium tolerance of Lactobacillus plantarum strains by proteomic analysis. Sci Rep (2017) 7:1182. doi: 10.1038/s41598-017-01180-x
30. Ma J, Chen T, Wu SF, Yang CY, Bai MZ, Shu KX, et al. iProX: an integrated proteome resource. Nucleic Acids Res (2019) 47:D1211–7. doi: 10.1093/nar/gky869
31. Lee DK, Jang S, Baek EH, Kim MJ, Lee KS, Shin HS, et al. Lactic acid bacteria affect serum cholesterol levels, harmful fecal enzyme activity, and fecal water content. Lipids Health Dis (2009) 8:21. doi: 10.1186/1476-511X-8-21
32. Wang L, Hu L, Xu Q, Yin B, Fang D, Wang G, et al. Bifidobacterium adolescentis Exerts Strain-Specific Effects on Constipation Induced by Loperamide in BALB/c Mice. Int J Mol Sci (2017) 18:318. doi: 10.3390/ijms18020318
33. Ye J, Coulouris G, Zaretskaya I, Cutcutache I, Rozen S, Madden TL. Primer-BLAST: a tool to design target-specific primers for polymerase chain reaction. BMC Bioinf (2012) 13:134. doi: 10.1186/1471-2105-13-134
34. Evans C, Noirel J, Ow SY, Salim M, Pereira-Medrano AG, Couto N, et al. An insight into iTRAQ: where do we stand now? Anal Bioanal Chem (2012) 404:1011–27. doi: 10.1007/s00216-012-5918-6
35. Herbrich SM, Cole RN, West KP, Schulze K, Yager JD, Groopman JD, et al. Statistical Inference from Multiple iTRAQ Experiments without Using Common Reference Standards. J Proteome Res (2013) 12:594–604. doi: 10.1021/pr300624g
36. Kossowska B, Dudka I, Gancarz R, Antonowicz-Juchniewicz J. Application of classic epidemiological studies and proteomics in research of occupational and environmental exposure to lead, cadmium and arsenic. Int Int J Hyg Envir Heal (2013) 216:1–7. doi: 10.1016/j.ijheh.2012.03.002
37. Luque-Garcia JL, Cabezas-Sanchez P, Camara C. Proteomics as a tool for examining the toxicity of heavy metals. Trac-Trends Anal Chem (2011) 30:703–16. doi: 10.1016/j.trac.2011.01.014
38. Liu J, Qu W, Kadiiska MB. Role of oxidative stress in cadmium toxicity and carcinogenesis. Toxicol Appl Pharmacol (2009) 238:209–14. doi: 10.1016/j.taap.2009.01.029
39. Waisberg M, Joseph P, Hale B, Beyersmann D. Molecular and cellular mechanisms of cadmium carcinogenesis. Toxicology (2003) 192:95–117. doi: 10.1016/s0300-483x(03)00305-6
40. Jiang X, Gu S, Liu D, Zhao L, Xia S, He X, et al. Lactobacillus brevis 23017 Relieves Mercury Toxicity in the Colon by Modulation of Oxidative Stress and Inflammation Through the Interplay of MAPK and NF-κB Signaling Cascades. Front Microbiol (2018) 9:2425:2425. doi: 10.3389/fmicb.2018.02425
41. Wei XL, Song M, Yin XM, Schuschke DA, Koo I, McClain CJ, et al. Effects of Dietary Different Doses of Copper and High Fructose Feeding on Rat Fecal Metabolome. J Proteome Res (2015) 14:4050–8. doi: 10.1021/acs.jproteome.5b00596
42. Huang X, Gao YN, Li SL, Wu CQ, Wang JQ, Zheng N. Modulation of Mucin (MUC2, MUC5AC and MUC5B) mRNA Expression and Protein Production and Secretion in Caco-2/HT29-MTX Co-Cultures Following Exposure to Individual and Combined Aflatoxin M1 and Ochratoxin A. Toxins (2019) 11:132–2. doi: 10.3390/toxins11020132
43. Pinton P, Graziani F, Pujol A, Nicoletti C, Paris O, Ernouf P, et al. Deoxynivalenol inhibits the expression by goblet cells of intestinal mucins through a PKR and MAP kinase dependent repression of the resistin-like molecule. Mol Nutr Res (2015) 59:1076–87. doi: 10.1002/mnfr.201500005
44. Choi DW, Lim MS, Lee JW, Chun W, Lee SH, Nam YH, et al. The Cytotoxicity of Kahweol in HT-29 Human Colorectal Cancer Cells Is Mediated by Apoptosis and Suppression of Heat Shock Protein 70 Expression. Biomol Ther (2015) 23:128–33. doi: 10.4062/biomolther.2014.133
45. Abraham NG, Kappas A. Pharmacological and clinical aspects of heme oxygenase. Pharmacol Rev (2008) 60:79–127. doi: 10.1124/pr.107.07104
46. Carter EP, Hartsfield CL, Miyazono M, Jakkula M, Morris KG, McMurtry IF. Regulation of heme oxygenase-1 by nitric oxide during hepatopulmonary syndrome. Am J Physiol Lung Cell Mol Physiol (2002) 283:346–53. doi: 10.1152/ajplung.00385.2001
47. Takeda K, Yu ZX, Qian S, Chin TK, Adelstein RS, Ferrans VJ. Nonmuscle myosin II localizes to the Z-lines and intercalated discs of cardiac muscle and to the Z-lines of skeletal muscle. Cell Motil Cytoskeleton (2000) 46:59–68. doi: 10.1002/(SICI)1097-0169(200005)46:1<59::AID-CM6>3.0.CO;2-Q
48. Zhou G, Roizman B. Cation-independent mannose 6-phosphate receptor blocks apoptosis induced by herpes simplex virus 1 mutants lacking glycoprotein D and is likely the target of antiapoptotic activity of the glycoprotein. J Virol (2002) 76:6197–204. doi: 10.1128/jvi.76.12.6197-6204.2002
49. Zhang W, Li H, Yang Y, Liao J, Yang GY. Knockdown or inhibition of aldo-keto reductase 1B10 inhibits pancreatic carcinoma growth via modulating Kras-E-cadherin pathway. Cancer Lett (2014) 355:273–80. doi: 10.1016/j.canlet.2014.09.031
50. Amir M, Hassanein SI, Abdel Rahman MF, Gad MZ. AGXT2 and DDAH-1 genetic variants are highly correlated with serum ADMA and SDMA levels and with incidence of coronary artery disease in Egyptians. Mol Biol Rep (2018) 45:2411–9. doi: 10.1007/s11033-018-4407-1
51. Reigstad CS, Salmonson CE, Rainey JF, Szurszewski JH, Linden DR, Sonnenburg JL, et al. Gut microbes promote colonic serotonin production through an effect of short-chain fatty acids on enterochromaffin cells. FASEB J (2015) 29:1395–403. doi: 10.1096/fj.14-259598
52. Yano JM, Yu K, Donaldson GP, Shastri GG, Ann P, Ma L, et al. Indigenous Bacteria from the Gut Microbiota Regulate Host Serotonin Biosynthesis. Cell (2015) 163:258–8. doi: 10.1016/j.cell.2015.09.017
53. Kannampalli P, Pochiraju S, Chichlowski M, Berg BM, Rudolph C, Bruckert M, et al. Probiotic Lactobacillus rhamnosus GG (LGG) and prebiotic prevent neonatal inflammation-induced visceral hypersensitivity in adult rats. Neurogastroent Motil (2014) 26:1694–704. doi: 10.1111/nmo.12450
54. Mu QH, Tavella VJ, Luo XM. Role of Lactobacillus reuteri in Human Health and Diseases. Front Microbiol (2018) 9:757:757. doi: 10.3389/fmicb.2018.00757
55. Gustafsson BI, Delbro DS. Tonic inhibition of small intestinal motility by nitric oxide. J Auton Nerv Syst (1993) 44:179–87. doi: 10.1016/0165-1838(93)90030-x
56. Russo A, Fraser R, Adachi K, Horowitz M, Boeckxstaens G. Evidence that nitric oxide mechanisms regulate small intestinal motility in humans. Gut (1999) 44:72–6. doi: 10.1136/gut.44.1.72
57. Cortesini C, Cianchi F, Infantino A, Lise M. Nitric oxide synthase and VIP distribution in enteric nervous system in idiopathic chronic constipation. Dig Dis Sci (1995) 40:2450–5. doi: 10.1007/BF02063253
58. Numata T, Takayama S, Tobita M, Ishida S, Katayose D, Shinkawa M, et al. Traditional Japanese medicine daikenchuto improves functional constipation in poststroke patients. Evid Based Complement Alternat Med (2014) 2014:231258. doi: 10.1155/2014/231258
59. Furness JB. The enteric nervous system and neurogastroenterology. Nat Rev Gastroenterol Hepatol (2012) 9:286–94. doi: 10.1038/nrgastro.2012.32
60. Camilleri M. Serotonin in the gastrointestinal tract. Curr Opin Endocrinol Diabetes Obes (2009) 16:53–9. doi: 10.1097/med.0b013e32831e9c8e
61. Koch TR, Carney JA, Go L, Go VLW. Idiopathic chronic constipation is associated with decreased colonic vasoactive intestinal peptide. Gastroenterology (1988) 94:300–10. doi: 10.1016/0016-5085(88)90416-7
62. Tzavella K, Riepl RL, Klauser AG, Voderholzer WA, Schindlbeck NE, Muller-Lissner SA. Decreased substance P levels in rectal biopsies from patients with slow transit constipation. Eur J Gastroenterol Hepatol (1996) 8:1207–11. doi: 10.1097/00042737-199612000-00014
63. Horii Y, Nakakita Y, Fujisaki Y, Yamamoto S, Itoh N, Miyazaki K, et al. Effects of intraduodenal injection of Lactobacillus brevis SBC8803 on autonomic neurotransmission and appetite in rodents. Neurosci Lett (2013) 539:32–7. doi: 10.1016/j.neulet.2013.01.037
64. Strandwitz P. Neurotransmitter modulation by the gut microbiota. Brain Res (2018) 1693:128–33. doi: 10.1016/j.brainres.2018.03.015
65. Zhao X, Suo HY, Qian Y, Li GJ, Liu ZH, Li J. Therapeutic effects of Lactobacillus casei Qian treatment in activated carbon-induced constipated mice. Mol Med Rep (2015) 12:3191–9. doi: 10.3892/mmr.2015.3737
66. Liu Zhou G, Roizman B. Cation-independent mannose 6-phosphate receptor blocks apoptosis induced by herpes simplex virus 1 mutants lacking glycoprotein D and is likely the target of antiapoptotic activity of the glycoprotein. J Virol (2002) 76:6197–204. doi: 10.1128/jvi.76.12.6197-6204.2002
67. Chao DT, Korsmeyer SJ. BCL-2 family: regulators of cell death. Annu Rev Immunol (1998) 16:395–419. doi: 10.1146/annurev.immunol.16.1.395
68. Kim MS, Kim BJ, Woo HN, Kim KW, Kim KB, Kim IK, et al. Cadmium induces caspase-mediated cell death: suppression by Bcl-2. Toxicology (2000) 145:27–37. doi: 10.1016/s0300-483x(99)00176-6
69. Iyer C, Kosters A, Sethi G, Kunnumakkara AB, Aggarwal BB, Versalovic J. Probiotic Lactobacillus reuteri promotes TNF-induced apoptosis in human myeloid leukemia-derived cells by modulation of NF-kappaB and MAPK signalling. Cell Microbiol (2008) 10:1442–52. doi: 10.1111/j.1462-5822.2008.01137.x
Keywords: Lactobacillus plantarum, cadmium, intestinal motility, proteomic analysis, neurotransmitter, cellular stress
Citation: Liu Y, Wu J, Xiao Y, Liu Q, Yu L, Tian F, Zhao J, Zhang H, Chen W and Zhai Q (2020) Relief of Cadmium-Induced Intestinal Motility Disorder in Mice by Lactobacillus plantarum CCFM8610. Front. Immunol. 11:619574. doi: 10.3389/fimmu.2020.619574
Received: 20 October 2020; Accepted: 10 November 2020;
Published: 10 December 2020.
Edited by:
Tingtao Chen, Nanchang University, ChinaReviewed by:
Jiachao Zhang, Hainan University, ChinaCopyright © 2020 Liu, Wu, Xiao, Liu, Yu, Tian, Zhao, Zhang, Chen and Zhai. This is an open-access article distributed under the terms of the Creative Commons Attribution License (CC BY). The use, distribution or reproduction in other forums is permitted, provided the original author(s) and the copyright owner(s) are credited and that the original publication in this journal is cited, in accordance with accepted academic practice. No use, distribution or reproduction is permitted which does not comply with these terms.
*Correspondence: Qixiao Zhai, emhhaXFpeGlhb0BzaW5hLmNvbQ==; emhhaXFpeGlhb0BqaWFuZ25hbi5lZHUuY24=
†These authors have contributed equally to this work
Disclaimer: All claims expressed in this article are solely those of the authors and do not necessarily represent those of their affiliated organizations, or those of the publisher, the editors and the reviewers. Any product that may be evaluated in this article or claim that may be made by its manufacturer is not guaranteed or endorsed by the publisher.
Research integrity at Frontiers
Learn more about the work of our research integrity team to safeguard the quality of each article we publish.