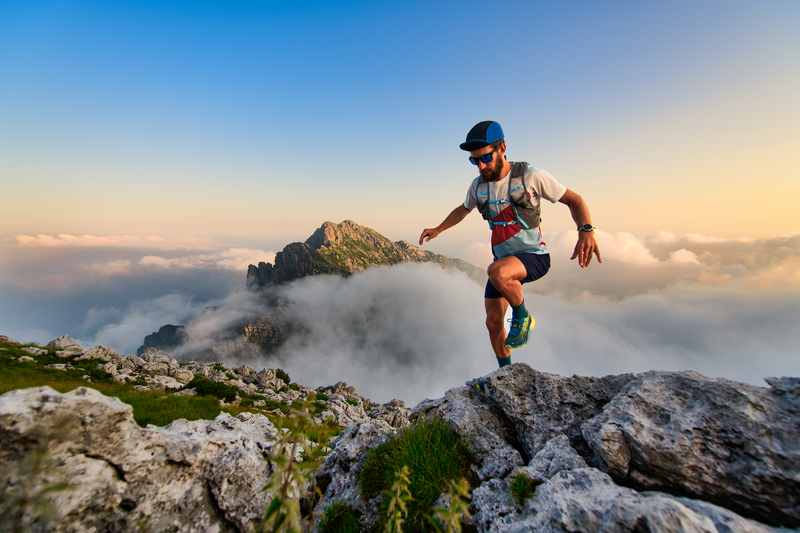
94% of researchers rate our articles as excellent or good
Learn more about the work of our research integrity team to safeguard the quality of each article we publish.
Find out more
MINI REVIEW article
Front. Immunol. , 07 December 2020
Sec. Immunological Tolerance and Regulation
Volume 11 - 2020 | https://doi.org/10.3389/fimmu.2020.609277
This article is part of the Research Topic The Role of Metabolism in MSC-Mediated Immunomodulation View all 9 articles
Mesenchymal stromal cells (MSCs) are spindle-shaped, plastic-adherent cells in vitro with potent immunosuppressive activity both in vitro and in vivo. MSCs have been employed as a cellular immunotherapy in diverse preclinical models and clinical trials, but most commonly as agents for the prophylaxis or therapy of graft versus host disease after hematopoietic cell transplantation. In addition to the oft studied secreted cytokines, several metabolic pathways intrinsic to MSCs, notably indoleamine 2,3-dioxygenase, prostaglandin E2, hypoxia-inducible factor 1 α, heme oxygenase-1, as well as energy-generating metabolism, have been shown to play roles in the immunomodulatory activity of MSCs. In this review, we discuss these key metabolic pathways in MSCs which have been reported to contribute to MSC therapeutic effects in the setting of hematopoietic cell transplantation and graft versus host disease. Understanding the contribution of MSC metabolism to immunomodulatory activity may substantially inform the development of future clinical applications of MSCs.
First identified in bone marrow in 1968 (1, 2), mesenchymal stromal cells (MSCs) are spindle-shaped, adherent in cell culture conditions, and widely investigated for their immunoregulatory properties and ability to contribute to tissue regeneration (3, 4). MSCs have been identified in a variety of tissue sources including bone marrow, adipose tissue, amniotic membrane and fluid, placental and fetal tissues, umbilical cord tissues, endometrium, blood, and synovial fluid (3). MSCs are present in relatively low numbers in any given tissue, and thus, prior to research or clinical use, are isolated and expanded ex vivo in cell culture media (3). Since MSCs may display several morphological and physiological characteristics in culture (3, 5), the minimum necessary criteria for MSC definition have been outlined by the International Society for Cellular Therapy (6, 7).
Indeed, MSCs are known to differentiate into chondrocytes, adipocytes, and osteocytes in vitro, suggesting that perhaps MSCs perform stem-like functions (8–12). Although tissue repair and regeneration has been reported after intravenous infusion of MSCs in a variety of disease settings (e.g., and late-onset hemorrhagic cystitis) (13–17), there are currently no in vivo data demonstrating that MSCs are true stem cells, differentiating to resident cells; however, it is possible that MSCs indirectly mediate endogenous tissue regeneration and repair mechanisms, perhaps by secreting soluble factors, via paracrine mechanisms or metabolic activity (18).
MSCs have also been shown to modulate adaptive and innate immunity in vitro and in vivo, usually after cytokine activation (e.g., IFN-γ) (4). MSCs may suppress both T and B cell proliferation as well as T cell effector activity (19–23), and MSCs are reported to inhibit proliferation by arresting T cells in the G0/G1 phases of the cell cycle or by promoting lymphocyte apoptotic pathways (24–28). Mechanistically, MSCs likely contribute to immunomodulation through cell-to-cell contact or paracrine and metabolic mechanisms (e.g., TGF-β, hepatocyte growth factor, prostaglandin E2; PGE2, and indoleamine 2,3-dioxygenase; IDO pathways), as immunosuppression has been reported in co-cultures and when cells are separated by a Transwell (26, 27, 29–32). Regarding the innate immune system, MSCs activated by monocytes or cytokines signal macrophages to promote pro- or anti-inflammatory pathways, by inducing the polarization of M2 macrophages (30, 32, 33). Moreover, inactive or apoptotic MSCs, those engulfed by phagocytic cells, or suppressed by host cytotoxic cells contribute to immunosuppression in in vitro and in vivo (34–36).
On account of this immunosuppressive activity, MSCs have been identified as promising candidates for immunosuppressive cell therapies and have been especially studied in the context of treating and preventing acute graft versus host disease (aGVHD) during hematologic cell transplantation (HCT), which occurs when donor immune cells attack recipient tissue (usually liver, gut, and skin) (37, 38). MSC-based cell products have been approved or conditionally approved for the treatment and prophylaxis of aGVHD in pediatric patients in Japan (TEMCELL), Canada, and New Zealand (Prochymal) (39). Recently, Mesoblast conducted a phase III clinical trial using a donor-derived bone marrow MSC cell therapy (RYONCIL™) to treat pediatric steroid refractory aGVHD (40). Notwithstanding these approved and pre-approved MSC-based cell products for treatment and prophylaxis of GVHD, many clinical trials have generated mixed results (40–44).
Although cell contact-dependent and secretory mechanisms have been established as the primary immunoregulatory modes of action of MSCs, recently, metabolic stress and activity have been shown to be involved in MSC immunomodulatory functions. Indeed, a great many metabolic pathways are known known to be involved in MSC physiologic mechanisms. However, the IDO, PGE2, hypoxia-inducible factor 1 α (HIF1α), heme oxygenase-1 (HO-1), and energy metabolic pathways have been especially implicated in the literature to play key roles in the immunosuppressive activity of MSCs (Figure 1). Herein, we review recent and notable scientific advances that indicate how the aforementioned metabolic pathways endogenous to MSCs, among others, may contribute to immunomodulation in the context of HCT and GVHD.
Figure 1 Schematic of proposed MSC-mediated metabolic immunomodulatory mechanisms reviewed herein. Dotted lines indicate incompletely understood mechanisms. Tryp, tryptophan; Kyn, kynurenines; CO, carbon monoxide; AA, arachidonic acid; mito, mitochondria; met, metabolism.
The metabolic activity of IDO seemingly plays a key role in the immunosuppressive activity of MSCs on T cells and other lymphocytes (45, 46). Naïve MSCs do not normally synthesize IDO; however, after cytokine activation (usually by IFN-γ or TNF-α), MSCs express high levels of IDO (22, 47) The enzymatic activity of IDO acts on the kynurenine pathway of tryptophan metabolism. An essential amino acid, tryptophan is recruited by the cell for protein synthesis, or may be metabolized in the serotonin or tryptamine pathways (48, 49). After induction of IDO expression, the kynurenine pathway is activated and L-tryptophan is metabolically converted into kynurenine, which may be further metabolized into biologically-active kynurenine derivatives including kynurenic acid, 3-hydroxyanthranilic acid, picolinic acid, quinolinic acid (48, 49). Notably, quinolinic acid and 3-hydroxyanthranilic acid are known to target lymphocytes and contribute to suppression of T cell proliferation (50, 51), and kynurenic acid may also modulate the immune system by agonizing aryl hydrocarbon receptor, G-protein-coupled receptor 35, and promoting anti-inflammatory cytokines (e.g., TNF-α, IL6, IL1β, and IL10) (52). Moreover, the addition of tryptophan significantly restores allogeneic T-cell proliferation (53), while adding kynurenine suppresses allogeneic T-cell proliferation (46). Although IDO-mediated catabolism of tryptophan contributes to the MSC-induced immunosuppression, additional investigation is needed in order to uncover the mechanism of action, especially in vivo.
It is known that MSCs elicit immunosuppressive effects when first primed with IFN-γ or a combination of IFN-γ with TNF-α, IL-1α or IL-1β (22, 47). Given that the IDO pathway is significantly upregulated with IFN-γ treatment, IDO expression and tryptophan metabolism has been implicated in suppressing T cells and controlling GVHD (54). Kim et al. recently demonstrated that compared to naïve MSCs, human MSCs primed with IFN-γ significantly upregulated IDO expression, increased immunosuppressive activity in vitro, and reduced GVHD symptoms and mortality a NOD-SCID PBMC-transplanted mouse model (55). In addition, the investigators showed that downregulating IDO in IFN-γ-primed MSCs decreased this activity, and IDO expression was driven by the JAK/STAT1 signaling pathway. In another recent study, human gingival MSCs stimulated were reported to inhibit T cell and PBMC proliferation in vitro and improve survival in a xenogenic GVHD model in the NOD/SCID mice via a combination of CD39, CD73, adenosine, and IDO signals (56).
Inhibition of T cell proliferation by IFN-γ-licensed MSCs is widely believed to be IDO-dependent. However, there is additional evidence that IDO metabolism and signaling may not be involved with MSC activity on effector T cell effector functions (e.g., cytokine production). Chinnadurai et al. have shown, for example, that IFN-γ-primed MSCs inhibit T cell (Th1) effector production of IFN-γ, TNF- α, and IL-2 independent of IDO. Inhibition of T cell effector function was instead mediated by B7H1 and B7DC/PD1 pathways (23). In an analysis of a MSC-based off-the-shelf cell product, intravenous infusions of Cymerus™ MSCs (Cynata Therapeutics) ameliorated disease and prolonged survival in a humanized GVHD mouse model after treating cells with IFN-γ (57). Although activating Cymerus™ MSCs with IFN-γ increased IDO expression 5-fold after a 48 h incubation, upregulation of the immune checkpoint inhibitor PD-L1, which contributes to the PD1-PDL1 signaling axis was also observed (26, 58). Therefore, while IDO-meditated metabolic activity plays a role in the immunomodulatory properties of IFN-γ-primed MSCs, other factors such as PD1 signaling and IDO-independent T cell effector activity seem to also be involved in immunosuppression induced by MSCs. Moreover, the relative contributions of each mechanism have yet to be determined.
Despite the potent immunosuppressive activity observed in IFN-γ-primed MSCs, largely due to the IDO pathway, no clinical trials to date have employed IFN-γ-primed MSCs for the treatment and prophylaxis of GVHD. However, Horwitz and colleagues have recently registered a phase I clinical trial with the NIH aimed at using IFN-γ-primed MSCs as prophylaxis for aGVHD after patients with hematologic malignancies and myelodysplasia have received HCT (NCT04328714).
PGE2 metabolic activity has been implicated in MSC-based immunosuppressive activity. PGE2 is an arachidonic acid derivative synthesized by cyclooxygenases COX1, COX2, and prostaglandin synthetase (59). MSC secretion of PGE2 correlates with suppression of lymphocyte proliferation (60), and PGE2 is known to promote induction of immunosuppressive interleukins (IL4, IL10, and IL6), proliferation and cytotoxicity of natural killer cells, and differentiation of Treg cells, and suppress differentiation of dendritic cells and naive T cells to Th17 cells (61–64),. Additionally, IL6 may contribute to PGE2-mediated immunomodulation in MSCs by positively regulating the COX2 function and synthesis of PGE2 (65, 66). IL6-dependent PGE2 has also been shown to promote immunosuppression via changing Th1 and Th2 ratios, inhibiting maturation of dendritic cells and stimulating of Treg cells (67, 68). Thus, synthesis and secretion of PGE2 contributes to MSC immunomodulation and have immunosuppressive potential in the context of GVHD.
In vivo, MSC infusions significantly increase secretion of PGE2 both before and after the onset of GVHD (69). Auletta et al. reported that of indomethacin (IM), a COX inhibitor which decrease PGE2 synthesis, and direct pharmacologic inhibition of PGE2-EP receptor interaction reversed T cell suppression induced my BM MSCs in vitro (70). In an allogeneic BMT mouse model, the investigators show that the survival advantage of animals treated with intravenous BM MSC injections was also reversed with a 7-day dose of IM. Similar results have been reported with MSCs isolated from other tissue sources, such as umbilical cord tissue (71). More recently, Kim et al. showed that treating BM MSCs with IM or downregulating expression of prostaglandin E synthetase (PGES) via siRNA reduced proliferation of human PBMCs, and PGES knock down MSCs were unable to reduce mortality in mice with GVHD (72). These studies present evidence that PGE2 may be a key effector of immunosuppression in GVHD and HCT clinical settings.
Heme oxygenase intracellularly metabolizes heme to biliverdin, CO, and free divalent iron, and HO-1 is reported to have anti-inflammatory and immunosuppressive properties (73–75). Chabannes et al. (76). were the first to demonstrate that HO-1 may play a role in MSC-mediated immunosuppression, and report a reversal of PBMC suppression after adding the HO-1-specific inhibiter tin protoporphyrin (SnPP) (76). Reduced T cell suppression in vitro and improved survival in vivo was also observed after inhibiting rat MSC HO-1 in combination with nitric oxide synthase (NOS). Human MSCs displayed similar results in vitro, but were not tested in vivo. Interestingly, the nitric oxide synthesizing pathway, which, like the IDO pathway, may also be stimulated in MSCs by IFN-γ, is implicated in rodent rather than human systems (77–79). Infusion of murine MSCs transduced with murine HO-1 have also been shown to increase the number of Treg cells in spleen and lymph nodes, and significantly reduce severity of clinical aGVHD in mice (80).
In contrast, Galipeau and colleagues have reported that human BM MSCs express low levels of HO-1 both before and after priming with IFN-γ, TNF-α, and/or TGF-β, and MSCs treated with SnPP had no effect on T cell suppression, possibly due to the notion that the IDO pathway may require heme as a cofactor (81). Therefore, the role of HO-1 in MSC-mediated immunomodulation, notably in the context of suppressing the immune system, is inconsistent in the literature. It is possible that this inconsistency may be an in vitro artifact, or a result of mixing experimental approaches. For example, studies described herein that reported an effect of HO-1 on MSC-mediated immune suppression, largely studied animal MSCs, while Galipeau et al. investigated humans MSCs. In any case, additional investigation is required in order to understand the role of HO-1 in MSC-mediated immunomodulation, especially in vivo.
In vivo, MSCs are thought to be located in perivascular niches under relatively hypoxic conditions, where oxygen tension is low. Hypoxia plays a crucial role in maintaining homeostasis throughout the body from early stages of embryonic development, and the metabolic regulatory mechanisms of hypoxia are largely driven by oxygen-sensitive transcription factors, including hypoxia-inducible factor 1 (HIF-1) (82). HIF-1 is a heterodimer consisting of an oxygen-regulated α-subunit and a constitutively expressed β-subunit. Under hypoxic conditions, hydroxylation of HIF-1 α by prolyl hydroxylase is suppressed, leading to the accumulation and nuclear translocation of HIF-1 α (83, 84). Activation of HIF-1 α has been shown to regulate transcription of genes necessary for carbohydrate, fatty acid, and other metabolic pathways involved with energy production (85). Metabolic activity and regulation of HIF-1 α in MSCs has been implicated in MSC differentiation potential (86–89), migration and chemotactic localization (90), the inflammatory response (91), tissue repair, and angiogenesis (92–94)
Hypoxia and HIF-1 α metabolism in MSCs may also play a role in immunomodulation. In response to hypoxia, it has been shown that MSCs produce an increased level of anti-inflammatory cytokines (e.g., IL-10), decreased pro- inflammatory cytokines (e.g., TNF α), and demonstrate enhanced suppression of PBMCs (95–97). Recently, Kim and colleagues have shown that human MSCs expanded under hypoxia, promoted T cell suppression, and when IV-administered to a humanized mouse GVHD model, improved survival and reduced symptoms of GVHD were also observed (97). These data support the notion that hypoxia priming or increased expression HIF-1 α in MSCs could be a viable strategy to promote donor and host immunomodulation and reduce GVHD during HCT. However, hypoxia- and HIF-1 α-mediated immunoregulation by MSCs is a relatively new avenue of research, and this transcription factor regulates many metabolically active genes. Thus, additional studies aimed at uncovering a mechanism of action are needed.
Energy-generating metabolic pathways (i.e., lipid and carbohydrate metabolism) have also been implicated in MSC-mediated immunomodulation. Contreras-Lopez et al. recently demonstrated that metabolism of peroxisome proliferator-activated receptor (PPAR) β/δ, which plays and key role in lipid and glucose metabolism and homeostasis, may be important for MSC immunomodulation of T cells (98). The investigators reported that PPARβ/δ knock out MSCs had enhanced suppression of Th1 and Th17 proliferation via enhancement of glycolysis, and inhibition of mitochondrial generation of ATP promoted aerobic glycolysis in WT MSCs and consequentially improved immunosuppressive activity. Similarly, Lui et al. recently demonstrated that priming MSCs with IFN-γ induces a metabolic switch towards aerobic glycolysis and strengthens T cell suppression (99). Moreover, oxidative glucose and lipid metabolism have been shown to contribute only 3% of ATP production in MSCs, while glycolysis generates 97% of cellular ATP (100). The studies together suggest that regulation of energy metabolism in MSCs, notably by reprogramming a switch from mitochondrial activity towards glycolysis, promotes immunosuppression.
MSCs contribute to adaptive and innate immunomodulation through cell-to-cell contact, secretory and paracrine signaling mechanisms, as well as intracellular metabolic pathways. IFN-γ, IDO and kynurenine, PGE2, HIF1α, HO-1, as well as energy-generating metabolic pathways have been implicated in MSC-mediated immunosuppression. Some studies have reported conflicting results, particularly regarding specific mechanisms of action and downstream targets. Moreover, the role of HO-1 in immunomodulation by MSCs remains an open question. IDO-kynurenine metabolism presents one of the most compelling mechanisms by which MSCs suppress the immune system. However, given that IDO is only expressed after MSCs are primed with IFN-γ or other cytokine combinations, which may regulate expression and activation of other factors (e.g., PDL-1), IDO may be only one of many contributors of MSC-based immunoregulation. Interestingly, aerobic glycolytic pathways, rather than oxidation of energy-generating substrates via mitochondria, have recently been hypothesized to play a key role in MSC immunomodulation, adding to the studies that promote the importance of IDO and kynurenine metabolism via IFN-γ licensing. Understanding how MSC metabolism modulates immune cell activity may have significant applications in the development of MSC-based therapeutics, especially in the context of HCT and aGVHD.
AJB and EMH conceived and designed the review. AJB wrote the original manuscript draft. EMH, EMF, and AJB edited and approved the final version of the manuscript. All authors contributed to the article and approved the submitted version.
This work was supported by the National Institutes of Health (grant R56HL147867) and Aflac Cancer & Blood Disorders Center.
The authors declare that the research was conducted in the absence of any commercial or financial relationships that could be construed as a potential conflict of interest.
Figure graphics were generated at Biorender.com.
1. Friedenstein AJ, Deriglasova UF, Kulagina NN, Panasuk AF, Rudakowa SF, Luriá EA, et al. Precursors for fibroblasts in different populations of hematopoietic cells as detected by the in vitro colony assay method. Exp Hematol (1974) 2:83–92.
2. Friedenstein AJ, Petrakova KV, Kurolesova AI, Frolova GP. Heterotopic transplants of bone marrow. Transplantation (1968) 6:230–47. doi: 10.1097/00007890-196803000-00009
3. Ullah I, Subbarao RB, Rho GJ. Human mesenchymal stem cells - Current trends and future prospective. Biosci Rep (2015) 35. doi: 10.1042/BSR20150025
4. Gao F, Chiu SM, Motan DAL, Zhang Z, Chen L, Ji HL, et al. Mesenchymal stem cells and immunomodulation: Current status and future prospects. Cell Death Dis (2016) 7. doi: 10.1038/cddis.2015.327
5. Kozlowska U, Krawczenko A, Futoma K, Jurek T, Rorat M, Patrzalek D, et al. Similarities and differences between mesenchymal stem/progenitor cells derived from various human tissues. World J Stem Cells (2019) 11:347–74. doi: 10.4252/wjsc.v11.i6.347
6. Horwitz EM, Le Blanc K, Dominici M, Mueller I, Slaper-Cortenbach I, Marini FC, et al. Clarification of the nomenclature for MSC: The International Society for Cellular Therapy position statement. Cytotherapy (2005) 7:393–5. doi: 10.1080/14653240500319234
7. Dominici M, Le Blanc K, Mueller I, Slaper-Cortenbach I, Marini FC, Krause DS, et al. Minimal criteria for defining multipotent mesenchymal stromal cells. The International Society for Cellular Therapy position statement. Cytotherapy (2006) 8:315–7. doi: 10.1080/14653240600855905
8. Prockop DJ. Repair of tissues by adult stem/progenitor cells (MSCs): Controversies, myths, and changing paradigms. Mol Ther (2009) 17:939–46. doi: 10.1038/mt.2009.62
9. Sagar R, Walther-Jallow L, David AL, Götherström C, Westgren M. Fetal Mesenchymal Stromal Cells: an Opportunity for Prenatal Cellular Therapy. Curr Stem Cell Rep (2018) 4:61–8. doi: 10.1007/s40778-018-0118-8
10. Sueblinvong V, Loi R, Eisenhauer PL, Bernstein IM, Suratt BT, Spees JL, et al. Derivation of lung epithelium from human cord blood-derived mesenchymal stem cells. Am J Respir Crit Care Med (2008) 177:701–11. doi: 10.1164/rccm.200706-859OC
11. Spees JL, Olson SD, Ylostalo J, Lynch PJ, Smith J, Perry A, et al. Differentiation, cell fusion, and nuclear fusion during ex vivo repair of epithelium by human adult stem cells from bone marrow stroma. Proc Natl Acad Sci U S A (2003) 100:2397–402. doi: 10.1073/pnas.0437997100
12. Yue WM, Liu W, Bi YW, He XP, Sun WY, Pang XY, et al. Mesenchymal stem cells differentiate into an endothelial phenotype, reduce neointimal formation, and enhance endothelial function in a rat vein grafting model. Stem Cells Dev (2008) 17:785–93. doi: 10.1089/scd.2008.0243
13. Zhu S, Li H, Lyu C, Liang J, Zeng L, Xu K. Infusion of Mesenchymal Stem Cells Combined with Epithelial Progenitor Cells Promotes Injured Intestinal Repairing after Hematopoietic Cell Transplantation. Blood (2019) 134:5604–4. doi: 10.1182/blood-2019-127584
14. DiMarino AM, Caplan AI, Bonfield TL. Mesenchymal stem cells in tissue repair. Front Immunol (2013) 4:201. doi: 10.3389/fimmu.2013.00201
15. Wang Y, Chen F, Gu B, Chen G, Chang H, Wu D. Mesenchymal stromal cells as an adjuvant treatment for severe late-onset hemorrhagic cystitis after allogeneic hematopoietic stem cell transplantation. Acta Haematol (2015) 133:72–7. doi: 10.1159/000362530
16. Hassan Z, Remberger M, Svenberg P, Elbander M, Omazic B, Mattsson J, et al. Hemorrhagic cystitis: A retrospective single-center survey. Clin Transplant (2007) 21:659–67. doi: 10.1111/j.1399-0012.2007.00705.x
17. Wang LT, Ting CH, Yen ML, Liu KJ, Sytwu HK, Wu KK, et al. Human mesenchymal stem cells (MSCs) for treatment towards immune- and inflammation-mediated diseases: review of current clinical trials. J BioMed Sci (2016) 23:1–13. doi: 10.1186/s12929-016-0289-5
18. Prockop DJ, Olson SD. Clinical trials with adult stem/progenitor cells for tissue repair: Let’s not overlook some essential precautions. Blood (2007) 109:3147–51. doi: 10.1182/blood-2006-03-013433
19. Rasmusson I, Ringdén O, Sundberg B, Le Blanc K. Mesenchymal stem cells inhibit the formation of cytotoxic T lymphocytes, but not activated cytotoxic T lymphocytes or natural killer cells. Transplantation (2003) 76:1208–13. doi: 10.1097/01.TP.0000082540.43730.80
20. Di Nicola M, Carlo-Stella C, Magni M, Milanesi M, Longoni PD, Matteucci P, et al. Human bone marrow stromal cells suppress T-lymphocyte proliferation induced by cellular or nonspecific mitogenic stimuli. Blood (2002) 99:3838–43. doi: 10.1182/blood.V99.10.3838
21. Corcione A, Benvenuto F, Ferretti E, Giunti D, Cappiello V, Cazzanti F, et al. Human mesenchymal stem cells modulate B-cell functions. Blood (2006) 107:367–72. doi: 10.1182/blood-2005-07-2657
22. Krampera M, Cosmi L, Angeli R, Pasini A, Liotta F, Andreini A, et al. Role for Interferon-γ in the Immunomodulatory Activity of Human Bone Marrow Mesenchymal Stem Cells. Stem Cells (2006) 24:386–98. doi: 10.1634/stemcells.2005-0008
23. Chinnadurai R, Copland IB, Patel SR, Galipeau J. IDO-Independent Suppression of T Cell Effector Function by IFN-γ–Licensed Human Mesenchymal Stromal Cells. J Immunol (2014) 192:1491–501. doi: 10.4049/jimmunol.1301828
24. Le Blanc K, Tammik L, Sundberg B, Haynesworth SE, Ringdén O. Mesenchymal Stem Cells Inhibit and Stimulate Mixed Lymphocyte Cultures and Mitogenic Responses Independently of the Major Histocompatibility Complex. Scand J Immunol (2003) 57:11–20. doi: 10.1046/j.1365-3083.2003.01176.x
25. Tse WT, Pendleton JD, Beyer WM, Egalka MC, Guinan EC. Suppression of allogeneic T-cell proliferation by human marrow stromal cells: Implications in transplantation. Transplantation (2003) 75:389–97. doi: 10.1097/01.TP.0000045055.63901.A9
26. Davies LC, Heldring N, Kadri N, Le Blanc K. Mesenchymal Stromal Cell Secretion of Programmed Death-1 Ligands Regulates T Cell Mediated Immunosuppression. Stem Cells (2017) 35:766–76. doi: 10.1002/stem.2509
27. Han KH, Ro H, Hong JH, Lee EM, Cho B, Yeom HJ, et al. Immunosuppressive mechanisms of embryonic stem cells and mesenchymal stem cells in alloimmune response. Transpl Immunol (2011) 25:7–15. doi: 10.1016/j.trim.2011.05.004
28. Plumas J, Chaperot L, Richard MJ, Molens JP, Bensa JC, Favrot MC. Mesenchymal stem cells induce apoptosis of activated T cells. Leukemia (2005) 19:1597–604. doi: 10.1038/sj.leu.2403871
29. Nauta AJ, Fibbe WE. Immunomodulatory properties of mesenchymal stromal cells. Blood (2007) 110:3499–506. doi: 10.1182/blood-2007-02-069716
30. Le Blanc K, Mougiakakos D. Multipotent mesenchymal stromal cells and the innate immune system. Nat Rev Immunol (2012) 12:383–96. doi: 10.1038/nri3209
31. Krampera M, Glennie S, Dyson J, Scott D, Laylor R, Simpson E, et al. Bone marrow mesenchymal stem cells inhibit the response of naive and memory antigen-specific T cells to their cognate peptide. Blood (2003) 101:3722–9. doi: 10.1182/blood-2002-07-2104
32. Bernardo ME, Fibbe WE. Mesenchymal stromal cells: Sensors and switchers of inflammation. Cell Stem Cell (2013) 13:392–402. doi: 10.1016/j.stem.2013.09.006
33. Eggenhofer E, Hoogduijn MJ. Mesenchymal stem cell-educated macrophages. Transplant Res (2012) 1. doi: 10.1186/2047-1440-1-12
34. de Witte SFH, Luk F, Sierra Parraga JM, Gargesha M, Merino A, Korevaar SS, et al. Immunomodulation By Therapeutic Mesenchymal Stromal Cells (MSC) Is Triggered Through Phagocytosis of MSC By Monocytic Cells. Stem Cells (2018) 36:602–15. doi: 10.1002/stem.2779
35. Galleu A, Riffo-Vasquez Y, Trento C, Lomas C, Dolcetti L, Cheung TS, et al. Apoptosis in mesenchymal stromal cells induces in vivo recipient-mediated immunomodulation. Sci Transl Med (2017) 9. doi: 10.1126/scitranslmed.aam7828
36. Luk F, De Witte SFH, Korevaar SS, Roemeling-Van Rhijn M, Franquesa M, Strini T, et al. Inactivated mesenchymal stem cells maintain immunomodulatory capacity. Stem Cells Dev (2016) 25:1342–54. doi: 10.1089/scd.2016.0068
37. Hill GR, Ferrara JLM. The primacy of the gastrointestinal tract as a target organ of acute graft-versus-host disease: Rationale for the use of cytokine shields in allogeneic bone marrow transplantation. Blood (2000) 95:2754–9. doi: 10.1182/blood.v95.9.2754.009k25_2754_2759
38. Sung AD, Chao NJ. Concise Review: Acute Graft-Versus-Host Disease: Immunobiology, Prevention, and Treatment. Stem Cells Transl Med (2013) 2:25–32. doi: 10.5966/sctm.2012-0115
39. Galipeau J, Sensébé L. Mesenchymal Stromal Cells: Clinical Challenges and Therapeutic Opportunities. Cell Stem Cell (2018) 22:824–33. doi: 10.1016/j.stem.2018.05.004
40. Kurtzberg J, Prockop S, Chaudhury S, Horn B, Nemecek E, Prasad V, et al. Study 275: Updated Expanded Access Program for Remestemcel-L in Steroid-Refractory Acute Graft-versus-Host Disease in Children. Biol Blood Marrow Transplant (2020) 26:855–64. doi: 10.1016/j.bbmt.2020.01.026
41. Le Blanc K, Frassoni F, Ball L, Locatelli F, Roelofs H, Lewis I, et al. Mesenchymal stem cells for treatment of steroid-resistant, severe, acute graft-versus-host disease: a phase II study. Lancet (2008) 371:1579–86. doi: 10.1016/S0140-6736(08)60690-X
42. Bernardo ME, Ball LM, Cometa AM, Roelofs H, Zecca M, Avanzini MA, et al. Co-infusion of ex vivo-expanded, parental MSCs prevents life-threatening acute GVHD, but does not reduce the risk of graft failure in pediatric patients undergoing allogeneic umbilical cord blood transplantation. Bone Marrow Transplant (2011) 46:200–7. doi: 10.1038/bmt.2010.87
43. Salmenniemi U, Itälä-Remes M, Nystedt J, Putkonen M, Niittyvuopio R, Vettenranta K, et al. Good responses but high TRM in adult patients after MSC therapy for GvHD. Bone Marrow Transplant (2017) 52:606–8. doi: 10.1038/bmt.2016.317
44. Galipeau J. Concerns arising from MSC retrieval from cryostorage and effect on immune suppressive function and pharmaceutical usage in clinical trials. ISBT Sci Ser (2013) 8:100–1. doi: 10.1111/voxs.12022
45. Mbongue JC, Nicholas DA, Torrez TW, Kim NS, Firek AF, Langridge WHR. The role of indoleamine 2, 3-dioxygenase in immune suppression and autoimmunity. Vaccines (2015) 3:703–29. doi: 10.3390/vaccines3030703
46. Ryan JM, Barry F, Murphy JM, Mahon BP. Interferon-γ does not break, but promotes the immunosuppressive capacity of adult human mesenchymal stem cells. Clin Exp Immunol (2007) 149:353–63. doi: 10.1111/j.1365-2249.2007.03422.x
47. Shi Y, Hu G, Su J, Li W, Chen Q, Shou P, et al. Mesenchymal stem cells: A new strategy for immunosuppression and tissue repair. Cell Res (2010) 20:510–8. doi: 10.1038/cr.2010.44
48. Höglund E, Øverli Ø, Winberg S. Tryptophan metabolic pathways and brain serotonergic activity: A comparative review. Front Endocrinol (Lausanne) (2019) 10:158. doi: 10.3389/fendo.2019.00158
49. Jones SP, Guillemin GJ, Brew BJ. The kynurenine pathway in stem cell biology. Int J Tryptophan Res (2013) 6:57–66. doi: 10.4137/IJTR.S12626
50. Frumento G, Rotondo R, Tonetti M, Damonte G, Benatti U, Ferrara GB. Tryptophan-derived catabolites are responsible for inhibition of T and natural killer cell proliferation induced by indoleamine 2,3-dioxygenase. J Exp Med (2002) 196:459–68. doi: 10.1084/jem.20020121
51. Fallarino F, Grohmann U, Hwang KW, Orabona C, Vacca C, Bianchi R, et al. Modulation of tryptophan catabolism by regulatory T cells. Nat Immunol (2003) 4:1206–12. doi: 10.1038/ni1003
52. Wirthgen E, Hoeflich A, Rebl A, Günther J. Kynurenic Acid: The Janus-faced role of an immunomodulatory tryptophan metabolite and its link to pathological conditions. Front Immunol (2018) 8:1957. doi: 10.3389/fimmu.2017.01957
53. Meisel R, Zibert A, Laryea M, Göbel U, Däubener W, Dilloo D. Human bone marrow stromal cells inhibit allogeneic T-cell responses by indoleamine 2,3-dioxygenase-mediated tryptophan degradation. Blood (2004) 103:4619–21. doi: 10.1182/blood-2003-11-3909
54. Godoy JAP, Paiva RMA, Souza AM, Kondo AT, Kutner JM, Okamoto OK. Clinical Translation of Mesenchymal Stromal Cell Therapy for Graft Versus Host Disease. Front Cell Dev Biol (2019) 7:255. doi: 10.3389/fcell.2019.00255
55. Kim DS, Jang IK, Lee MW, Ko YJ, Lee DH, Lee JW, et al. Enhanced Immunosuppressive Properties of Human Mesenchymal Stem Cells Primed by Interferon-γ. EBioMedicine (2018) 28:261–73. doi: 10.1016/j.ebiom.2018.01.002
56. Huang F, Chen M, Chen W, Gu J, Yuan J, Xue Y, et al. Human gingiva-derived mesenchymal stem cells inhibit xeno-graft-versus-host disease via CD39-CD73-adenosine and IDO signals. Front Immunol (2017) 8:68. doi: 10.3389/fimmu.2017.00068
57. Ozay EI, Vijayaraghavan J, Gonzalez-Perez G, Shanthalingam S, Sherman HL, Garrigan DT, et al. Cymerus™ iPSC-MSCs significantly prolong survival in a pre-clinical, humanized mouse model of Graft-vs-host disease. Stem Cell Res (2019) 35. doi: 10.1016/j.scr.2019.101401
58. Yan Z, Zhuansun Y, Liu G, Chen R, Li J, Ran P. Mesenchymal stem cells suppress T cells by inducing apoptosis and through PD-1/B7-H1 interactions. Immunol Lett (2015) 162:248–55. doi: 10.1016/j.imlet.2014.09.013
59. Kalinski P. Regulation of Immune Responses by Prostaglandin E 2. J Immunol (2012) 188:21–8. doi: 10.4049/jimmunol.1101029
60. Carter D, Tyrell A, Bubnic S, Marcelino M, Kedzierski K, Monroy R, et al. Characterization of MSC Potential To Treat GVHD Using Molecular Markers Linked to MSC-Mediated Immunosuppression In Vitro. Blood (2005) 106:4322–2. doi: 10.1182/blood.v106.11.4322.4322
61. Williams JA, Pontzer CH, Shacter E. Regulation of macrophage interleukin-6 (IL-6) and IL-10 expression by prostaglandin E2: The role of p38 mitogen-activated protein kinase. J Interferon Cytokine Res (2000) 20:291–8. doi: 10.1089/107999000312423
62. Baratelli F, Lin Y, Zhu L, Yang S-C, Heuzé-Vourc’h N, Zeng G, et al. Prostaglandin E 2 Induces FOXP3 Gene Expression and T Regulatory Cell Function in Human CD4 + T Cells. J Immunol (2005) 175:1483–90. doi: 10.4049/jimmunol.175.3.1483
63. Follin B, Juhl M, Cohen S, Perdersen AE, Kastrup J, Ekblond A. Increased Paracrine Immunomodulatory Potential of Mesenchymal Stromal Cells in Three-Dimensional Culture. Tissue Eng Part B Rev (2016) 22:322–9. doi: 10.1089/ten.teb.2015.0532
64. Najar M, Raicevic G, Fayyad-Kazan H, Bron D, Toungouz M, Lagneaux L. Mesenchymal stromal cells and immunomodulation: A gathering of regulatory immune cells. Cytotherapy (2016) 18:160–71. doi: 10.1016/j.jcyt.2015.10.011
65. Bouffi C, Bony C, Courties G, Jorgensen C, Noël D. IL-6-dependent PGE2 secretion by mesenchymal stem cells inhibits local inflammation in experimental arthritis. PloS One (2010) 5. doi: 10.1371/journal.pone.0014247
66. Rummel C, Sachot C, Poole S, Luheshi GN. Circulating interleukin-6 induces fever through a STAT3-linked activation of COX-2 in the brain. Am J Physiol Regul Integr Comp Physiol (2006) 291. doi: 10.1152/ajpregu.00301.2006
67. Najar M, Rouas R, Raicevic G, Boufker HI, Lewalle P, Meuleman N, et al. Mesenchymal stromal cells promote or suppress the proliferation of T lymphocytes from cord blood and peripheral blood: The importance of low cell ratio and role of interleukin-6. Cytotherapy (2009) 11:570–83. doi: 10.1080/14653240903079377
68. Nauta AJ, Kruisselbrink AB, Lurvink E, Willemze R, Fibbe WE. Mesenchymal Stem Cells Inhibit Generation and Function of Both CD34 + -Derived and Monocyte-Derived Dendritic Cells. J Immunol (2006) 177:2080–7. doi: 10.4049/jimmunol.177.4.2080
69. Jang YK, Kim M, Lee YH, Oh W, Yang YS, Choi SJ. Optimization of the therapeutic efficacy of human umbilical cord blood-mesenchymal stromal cells in an NSG mouse xenograft model of graft-versus-host disease. Cytotherapy (2014) 16:298–308. doi: 10.1016/j.jcyt.2013.10.012
70. Auletta JJ, Eid SK, Wuttisarnwattana P, Silva I, Metheny L, Keller MD, et al. Human mesenchymal stromal cells attenuate graft-versus-host disease and maintain graft-versus-leukemia activity following experimental allogeneic bone marrow transplantation. Stem Cells (2015) 33:601–14. doi: 10.1002/stem.1867
71. Cutler AJ, Limbani V, Girdlestone J, Navarrete CV. Umbilical Cord-Derived Mesenchymal Stromal Cells Modulate Monocyte Function to Suppress T Cell Proliferation. J Immunol (2010) 185:6617–23. doi: 10.4049/jimmunol.1002239
72. Kim DS, Lee WH, Lee MW, Park HJ, Jang IK, Lee JW, et al. Involvement of TLR3-Dependent PGES Expression in Immunosuppression by Human Bone Marrow Mesenchymal Stem Cells. Stem Cell Rev Rep (2018) 14:286–93. doi: 10.1007/s12015-017-9793-6
73. Otterbein LE, Choi AMK. Heme oxygenase: Colors of defense against cellular stress. Am J Physiol Lung Cell Mol Physiol (2000) 279. doi: 10.1152/ajplung.2000.279.6.l1029
74. Chauveau C, Rémy S, Royer PJ, Hill M, Tanguy-Royer S, Hubert FX, et al. Heme oxygenase-1 expression inhibits dendritic cell maturation and proinflammatory function but conserves IL-10 expression. Blood (2005) 106:1694–702. doi: 10.1182/blood-2005-02-0494
75. Vijayan V, Wagener FADTG, Immenschuh S. The macrophage heme-heme oxygenase-1 system and its role in inflammation. Biochem Pharmacol (2018) 153:159–67. doi: 10.1016/j.bcp.2018.02.010
76. Chabannes D, Hill M, Merieau E, Rossignol J, Brion R, Soulillou JP, et al. A role for heme oxygenase-1 in the immunosuppressive effect of adult rat and human mesenchymal stem cells. Blood (2007) 110:3691–4. doi: 10.1182/blood-2007-02-075481
77. Albina JE, Abate JA, Henry WL. Nitric oxide production is required for murine resident peritoneal macrophages to suppress mitogen-stimulated T cell proliferation. Role of IFN-gamma in the induction of the nitric oxide-synthesizing pathway. J Immunol (1991) 147:144–8. doi: 10.1161/01.HYP.21.2.185
78. Ren G, Su J, Zhang L, Zhao X, Ling W, L’Huillie A, et al. Species variation in the mechanisms of mesenchymal stem cell-mediated immunosuppression. Stem Cells (2009) 27:1954–62. doi: 10.1002/stem.118
79. Su J, Chen X, Huang Y, Li W, Li J, Cao K, et al. Phylogenetic distinction of iNOS and IDO function in mesenchymal stem cell-mediated immunosuppression in mammalian species. Cell Death Differ (2014) 21:388–96. doi: 10.1038/cdd.2013.149
80. Yu M, Wang J, Fang Q, Liu P, Chen S, Zhe N, et al. High expression of heme oxygenase-1 in target organs may attenuate acute graft-versus-host disease through regulation of immune balance of TH17/Treg. Transpl Immunol (2016) 37:10–7. doi: 10.1016/j.trim.2016.05.002
81. Patel SR, Copland IB, Garcia MA, Metz R, Galipeau J. Human mesenchymal stromal cells suppress T-cell proliferation independent of heme oxygenase-1. Cytotherapy (2015) 17:382–91. doi: 10.1016/j.jcyt.2014.11.010
82. Stamati K, Mudera V, Cheema U. Evolution of oxygen utilization in multicellular organisms and implications for cell signalling in tissue engineering. J Tissue Eng (2011) 2:1–12. doi: 10.1177/2041731411432365
83. Weidemann A, Johnson RS. Biology of HIF-1α. Cell Death Differ (2008) 15:621–7. doi: 10.1038/cdd.2008.12
84. Dengler VL, Galbraith MD, Espinosa JM. Transcriptional regulation by hypoxia inducible factors. Crit Rev Biochem Mol Biol (2014) 49:1–15. doi: 10.3109/10409238.2013.838205
85. Gaspar JM, Velloso LA. Hypoxia inducible factor as a central regulator of metabolism ⇓ implications for the development of obesity. Front Neurosci (2018) 12:813. doi: 10.3389/fnins.2018.00813
86. Keith B, Simon MC. Hypoxia-Inducible Factors, Stem Cells, and Cancer. Cell (2007) 129:465–72. doi: 10.1016/j.cell.2007.04.019
87. Zhang C, Yang F, Cornelia R, Tang W, Swisher S, Kim H. Hypoxia-inducible factor-1 is a positive regulator of Sox9 activity in femoral head osteonecrosis. Bone (2011) 48:507–13. doi: 10.1016/j.bone.2010.10.006
88. Robins JC, Akeno N, Mukherjee A, Dalal RR, Aronow BJ, Koopman P, et al. Hypoxia induces chondrocyte-specific gene expression in mesenchymal cells in association with transcriptional activation of Sox9. Bone (2005) 37:313–22. doi: 10.1016/j.bone.2005.04.040
89. Wagegg M, Gaber T, Lohanatha FL, Hahne M, Strehl C, Fangradt M, et al. Hypoxia Promotes Osteogenesis but Suppresses Adipogenesis of Human Mesenchymal Stromal Cells in a Hypoxia-Inducible Factor-1 Dependent Manner. PloS One (2012) 7. doi: 10.1371/journal.pone.0046483
90. Hung SC, Pochampally RR, Hsu SC, Sanchez CC, Chen SC, Spees J, et al. Short-term exposure of multipotent stromal cells to low oxygen increases their expression of CX3CR1 and CXCR4 and their engraftment in vivo. PloS One (2007) 2. doi: 10.1371/journal.pone.0000416
91. Wang GL, Semenza GL. Purification and characterization of hypoxia-inducible factor. J Biol Chem (1995) 270:1230–7. doi: 10.1074/jbc.270.3.1230
92. Razban V, Lotfi AS, Soleimani M, Ahmadi H, Massumi M, Khajeh S, et al. HIF-1α overexpression induces angiogenesis in mesenchymal stem cells. Biores Open Access (2012) 1:174–83. doi: 10.1089/biores.2012.9905
93. Shi X, Zhang G, Sun H, Bai Y, Liu Y, Zhang W, et al. Effects of over-expression of HIF-1alpha in bone marrow-derived mesenchymal stem cells on traumatic brain injury. Eng Life Sci (2018) 18:401–7. doi: 10.1002/elsc.201800015
94. Noronha Nc NDC, Mizukami A, Caliári-Oliveira C, Cominal JG, Rocha JLM, Covas DT, et al. Priming approaches to improve the efficacy of mesenchymal stromal cell-based therapies. Stem Cell Res Ther (2019) 10. doi: 10.1186/s13287-019-1224-y
95. Lan YW, Choo KB, Chen CM, Hung TH, Bin CY, CH H, et al. Hypoxia-preconditioned mesenchymal stem cells attenuate bleomycin-induced pulmonary fibrosis. Stem Cell Res Ther (2015) 6. doi: 10.1186/s13287-015-0081-6
96. Jiang CM, Liu J, Zhao JY, Xiao L, An S, Gou YC, et al. Effects of hypoxia on the immunomodulatory properties of human gingiva-derived mesenchymal stem cells. J Dent Res (2015) 94:69–77. doi: 10.1177/0022034514557671
97. Kim YH, Jin HJ, Heo J, Ju H, Lee HY, Kim S, et al. Small hypoxia-primed mesenchymal stem cells attenuate graft-versus-host disease. Leukemia (2018) 32:2672–84. doi: 10.1038/s41375-018-0151-8
98. Contreras-Lopez RA, Elizondo-Vega R, Torres MJ, Vega-Letter AM, Luque-Campos N, Paredes-Martinez MJ, et al. PPARβ/δ-dependent MSC metabolism determines their immunoregulatory properties. Sci Rep (2020) 10. doi: 10.1038/s41598-020-68347-x
99. Liu Y, Yuan X, Muñoz N, Logan TM, Ma T. Commitment to Aerobic Glycolysis Sustains Immunosuppression of Human Mesenchymal Stem Cells. Stem Cells Transl Med (2019) 8:93–106. doi: 10.1002/sctm.18-0070
Keywords: kynurenine, PGE2, heme oxygenase-1, hypoxia-inducible factor 1 α, indoleamine 2,3-dioxygenase, graft versus host disease, mesenchymal stromal cell, aerobic glycolysis
Citation: Burnham AJ, Foppiani EM and Horwitz EM (2020) Key Metabolic Pathways in MSC-Mediated Immunomodulation: Implications for the Prophylaxis and Treatment of Graft Versus Host Disease. Front. Immunol. 11:609277. doi: 10.3389/fimmu.2020.609277
Received: 23 September 2020; Accepted: 10 November 2020;
Published: 07 December 2020.
Edited by:
Yves-Marie Pers, INSERM U1183 Cellules Souches, Plasticité Cellulaire, Médecine Régénératrice Et Immunothérapies, FranceReviewed by:
Qifa Liu, Southern Medical University, ChinaCopyright © 2020 Burnham, Foppiani and Horwitz. This is an open-access article distributed under the terms of the Creative Commons Attribution License (CC BY). The use, distribution or reproduction in other forums is permitted, provided the original author(s) and the copyright owner(s) are credited and that the original publication in this journal is cited, in accordance with accepted academic practice. No use, distribution or reproduction is permitted which does not comply with these terms.
*Correspondence: Andre J. Burnham, YW5kcmUuam9zZXBoLmJ1cm5oYW1AZW1vcnkuZWR1; Edwin M. Horwitz, ZWR3aW4uaG9yd2l0ekBlbW9yeS5lZHU=
Disclaimer: All claims expressed in this article are solely those of the authors and do not necessarily represent those of their affiliated organizations, or those of the publisher, the editors and the reviewers. Any product that may be evaluated in this article or claim that may be made by its manufacturer is not guaranteed or endorsed by the publisher.
Research integrity at Frontiers
Learn more about the work of our research integrity team to safeguard the quality of each article we publish.