- 1Critical Care Research Group, The Prince Charles Hospital, Brisbane, QLD, Australia
- 2Faculty of Medicine, University of Queensland, Brisbane, QLD, Australia
- 3Roslin Institute, University of Edinburgh, Edinburgh, United Kingdom
- 4Clinical Immunology and Allergy, and Sullivan Nicolaides Pathology, Wesley Hospital, Brisbane, QLD, Australia
- 5Department of Anaesthesia and Perfusion, The Prince Charles Hospital, Brisbane, QLD, Australia
- 6Adult Intensive Care Service, The Prince Charles Hospital, Brisbane, QLD, Australia
- 7Department of Intensive Care Unit, Guy’s and St Thomas’ Hospital NHS Foundation Trust, London, United Kingdom
- 8School of Immunology & Microbial Sciences, King’s College London, London, United Kingdom
- 9Department of Paediatrics, Chonnam National University Children’s Hospital and Medical School, Gwangju, South Korea
A plethora of leukocyte modulations have been reported in critically ill patients. Critical illnesses such as acute respiratory distress syndrome and cardiogenic shock, which potentially require extracorporeal membrane oxygenation (ECMO) support, are associated with changes in leukocyte numbers, phenotype, and functions. The changes observed in these illnesses could be compounded by exposure of blood to the non-endothelialized surfaces and non-physiological conditions of ECMO. This can result in further leukocyte activation, increased platelet-leukocyte interplay, pro-inflammatory and pro-coagulant state, alongside features of immunosuppression. However, the effects of ECMO on leukocytes, in particular their phenotypic and functional signatures, remain largely overlooked, including whether these changes have attributable mortality and morbidity. The aim of our narrative review is to highlight the importance of studying leukocyte signatures to better understand the development of complications associated with ECMO. Increased knowledge and appreciation of their probable role in ECMO-related adverse events may assist in guiding the design and establishment of targeted preventative actions.
Introduction
Extracorporeal membrane oxygenation (ECMO) is a potentially lifesaving modality that supports critically ill patients with refractory cardiac and/or respiratory failure (1–5). Over the last decade, the use and number of ECMO centers have dramatically increased (6). Beyond providing support to the heart and lungs, ECMO is used as a cardiopulmonary resuscitation tool and a bridge to transplant. The modern era of ECMO, post 2007, introduced significant advances in circuit design and improved expertise in clinical management (7). Despite these improvements, adverse events remain common. ECMO is associated with an increased risk of complications such as exacerbated systemic inflammatory response syndrome (SIRS), infection, new organ dysfunction, and thrombosis (6, 8–10).
The main cause for these complications may be alteration of blood components by their contact with the foreign and non-endothelialized surfaces of the ECMO circuit (comprises of a pump and membrane oxygenator connected by circuit tubing). However, delineation of ECMO-related changes can be challenging due to the underpinning pathophysiology of the critically unwell patients. It is further complicated by variances in technology, exertion of non-physiological conditions, and intensive use of modulatory clinical resources during ECMO. Activation of peripheral pro-inflammatory responses and the hemostatic system (platelet and coagulation) are widely recognized in the setting of ECMO thus far (11, 12). Likewise, differences in the closely regulated and inter-dependent blood leukocyte subset (namely monocytes, neutrophils, B and T lymphocytes) profiles have also been evaluated.
Existing research reported alteration of leukocyte numbers, phenotype, and functions with non-specific activation when ECMO is used (Tables 1 and 2). A similar response has been observed in other extracorporeal circulatory modalities preceding ECMO, such as cardiopulmonary bypass (CPB), renal replacement therapy, and ventricular assist devices (VAD) (40–49). The sequelae linked to inappropriate leukocyte modulation can be detrimental. This is because leukocytes are multifaceted and play a critical role in the intricate communication between innate and adaptive immunity, regulation, and stimulation of inflammatory responses and self-tolerance (50), and interaction with platelets and endothelial cells that contribute to coagulation (51–55). These changes are also common in refractory cardiac and respiratory failure patients, triggered by the underlying disease that necessitated ECMO (56–59). Therefore, it is possible that the changes observed in these illnesses could be compounded by ECMO and associated factors/stressors (Figure 1).
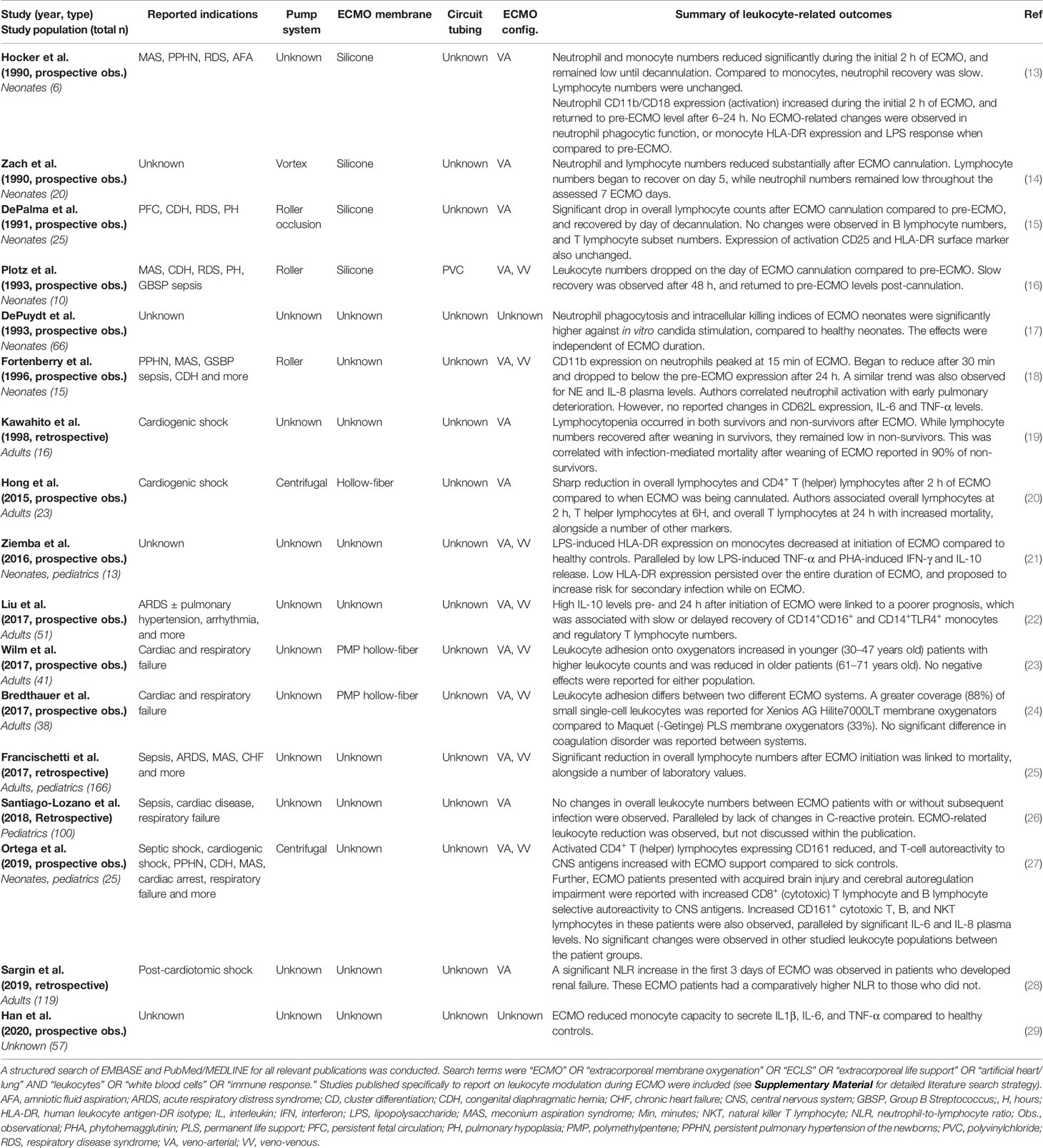
Table 1 Clinical studies investigating changes in leukocyte numbers, phenotype, and functions in the context of ECMO.
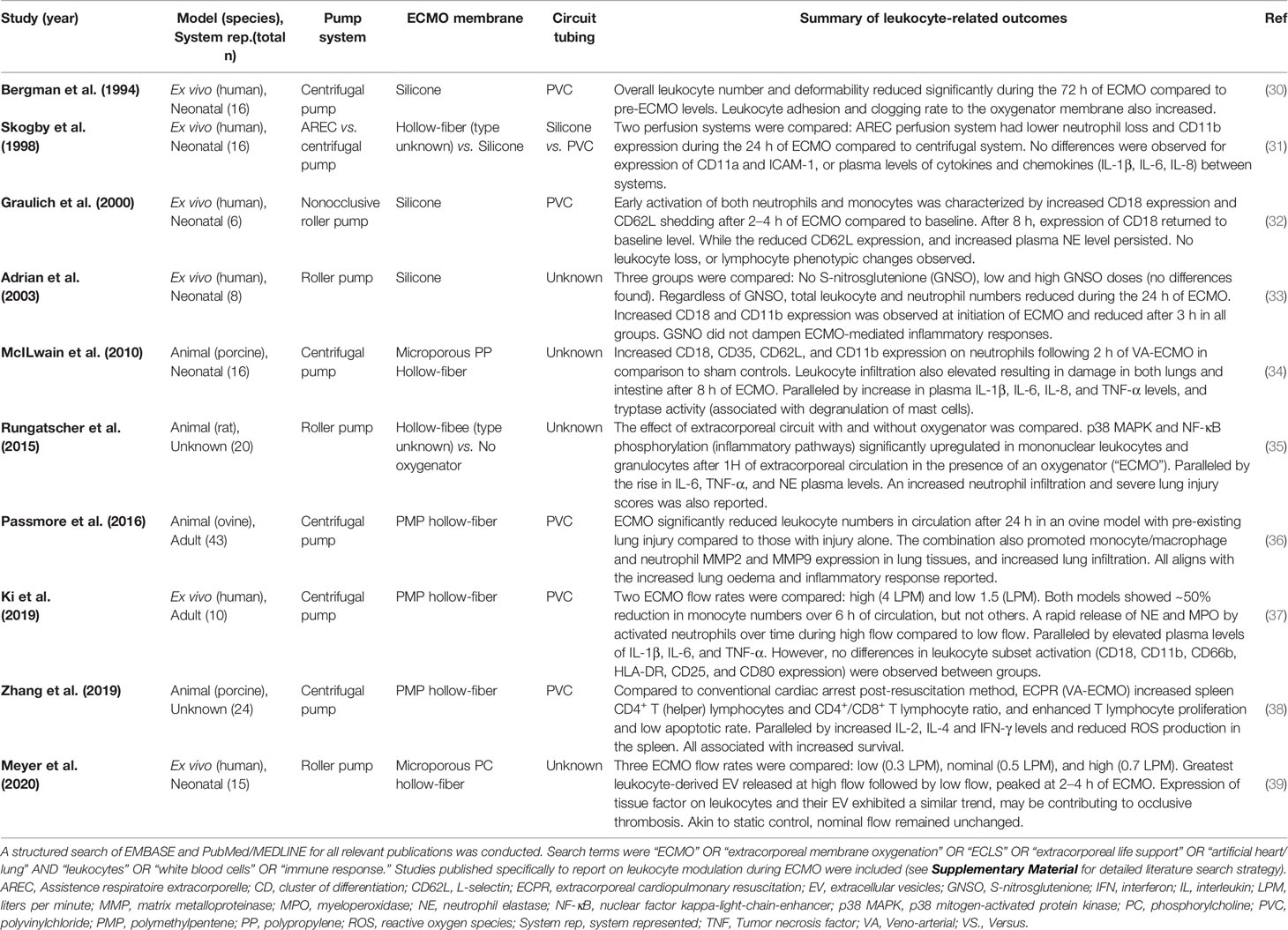
Table 2 Experimental studies investigating changes in leukocyte numbers, phenotype, and function in the context of ECMO.
Currently, there is limited knowledge regarding leukocyte phenotype and functions during ECMO, including whether these changes have attributable mortality and morbidity. Much of what we know about the effects of ECMO on leukocytes and the immune system is based on changes in the inflammatory response (11), as well as prior knowledge from CPB (41–43, 60, 61). Given the pivotal contribution leukocytes can have in both immune and hemostatic complications, better characterization of their ECMO-related phenotypic and functional signatures could be beneficial. In particular, the capacity to separate the immunologic signatures between the patients’ leukocyte-modulating primary disease burden and ECMO, could facilitate patient risk stratification and prognosis. Additionally, by optimizing ECMO circuit design we may lessen its effects on leukocytes to help reduce poor outcomes.
This review aims to provide a summary of both published clinical and experimental evidence on the effects of ECMO on the innate and adaptive leukocyte numbers, phenotype, and functions, and to highlight their potential role in ECMO outcomes. In addition, the influences of different ECMO circuit designs and factors (clinical therapies and ECMO-related stressors) that may further contribute to the occurrence of leukocyte modulation will be discussed. Finally, we address the limitations and future directions on this Research Topic.
ECMO as a Second “Hit” to Leukocyte Pathophysiology in Critically Ill Patients
Leukocyte dysfunction is common in critical illnesses such as acute respiratory distress syndrome (ARDS), cardiogenic shock, and sepsis (56–58, 62), which are also frequent indications for ECMO support. As changes in the functional immune response cannot be sufficiently characterized by the widely researched inflammatory mediators alone, due to their short half-life and exclusive origin, cellular profiling may be beneficial. Leukocyte numeric, phenotypic and functional alterations have long been recognized as important indicators of inflammation and predictors of poor outcomes. These changes are associated with increased risk of secondary infection, end organ injuries, mortality, and a prolonged length of hospital stay in critically ill patients (56–59, 63, 64). Studies have suggested that veno-arterial (VA; heart and/or lung support) and veno-venous (VV; lung support) ECMO further dysregulates the leukocyte pathophysiology in refractory neonates, pediatrics, and adults. These applications act as a second “hit” to the immune system (Figure 2).
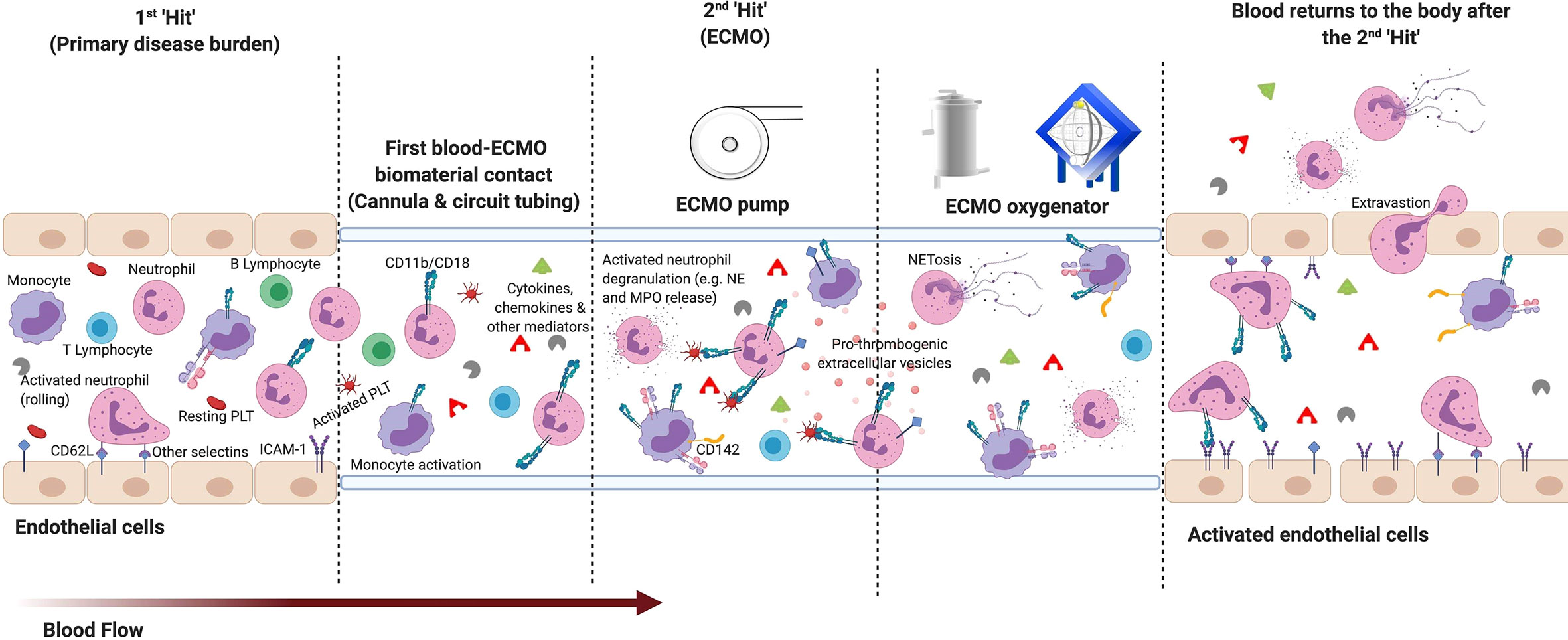
Figure 2 The two “hit” ECMO model: Basic overview of early leukocyte modulation during extracorporeal membrane oxygenation (ECMO). 1st hit: Immune modulation from the patient’s primary disease burden. 2nd hit: The inflammatory response to ECMO is proposed to activate upon contact of blood with the non-endothelialized surface of the ECMO circuit and the non-physiological conditions generated. ECMO-mediated promotion of pro-inflammatory cytokines and chemokines, and other mediators (e.g. complement products) from non-physiological exposure, contact and cellular activation can lead to further activation of leukocyte subpopulations. During early ECMO, innate immune neutrophils and monocytes become the first to activate—consistently reported with an increased expression of surface integrins cluster differentiation (CD)11b and CD18. Alongside L-selectin (CD62L), these modulated integrin expressions potentiate an increase in leukocyte adhesion to the membrane oxygenator, as well as activated endothelium (e.g. increased Intercellular Adhesion Molecule (ICAM)-1) expression) and platelets [PLT; e.g. increased P-selectin glycoprotein ligand-1 (PSGL) and glycoprotein Ib alpha polypeptide (GIbα) expression]. As blood continues to circulate through the ECMO circuit: monocyte expression of tissue factor (CD142), neutrophil degranulation, neutrophil extracellular trap (NET)osis, pro-thrombogenic extracellular vesicle release and activation of downstream lymphocytes are also upregulated. All of which can contribute to the heightened systemic inflammatory response syndrome (SIRS), leukocytopenia, infection, end-organ damage, and thrombosis frequently reported in patients. (Figure created with BioRender.com).
In this section, we provide an overview of current studies (Tables 1 and 2) published specifically to report on the effect of ECMO on leukocytes (see Supplementary Material for detailed literature search strategy). It will focus on the four major peripheral leukocyte subsets of the innate and adaptive immunity: neutrophils, monocytes, T and B lymphocytes.
Impact on the Innate Immune Leukocytes
Neutrophil and monocyte profiles are the most studied peripheral leukocytes in ECMO. It is likely due to their abundant expression, and critical role in the initiation of rapid immune response and direction of downstream adaptive immunity. During neonatal and adult ECMO, a dramatic fall of neutrophils and monocytes is consistently described. It can occur within 2 h of ECMO cannulation (13, 14, 22). In contrast to CPB where the number of leukocytes increased during open heart surgeries (40, 43), leukocytopenia has long been reported in ECMO (16, 25). Differences could be associated with the pathophysiology between ECMO and CPB patients, and the involvement of other immune triggers (e.g. induction of surgical trauma and ischemic insults in cardiac surgery patients). Studies also correlated slow or delayed recovery of monocytes with worsen prognosis and mortality in both VA and VV ECMO patients (22). Specifically, low number of cluster differentiation (CD)14+CD16+ and CD14+ toll-like receptor (TLR)4+ monocyte subsets have been linked to non-survivors (22).
In comparison, the changes in neutrophil and monocyte numbers were variable in ex vivo. While the clinical trend of reduction was reported by Adrian et al., this was not observed by Graulich et al. and partially observed by Ki et al. (32, 33, 37). The discrepancies between the outcomes could be rationalized by the utilization of different ECMO technology, model setup, and blood flow rates. In addition, the lack of endothelial lining in the current models may contribute to these differences between ex vivo studies, as well as ex vivo and clinical studies. In response to the continuous exposure to non-physiological conditions (e.g. flow rates and associated shear stress) and significant release of inflammatory mediators during ECMO (16, 18, 34, 35, 37, 39, 65–67), cells of the endothelial lining are likely to convert into an activated state (68, 69). This can subsequently elevate leukocyte adhesion [from phenotypic modulation (13, 17, 18, 34–36)] onto either the artificial surface of the ECMO circuit (23, 24, 70) or the endothelium itself (68, 69), possibly acting as a consistent regulator of cell attachment in vivo.
Furthermore, current animal and human research have characterized a number of leukocyte phenotypic profiles associated with ECMO (see Supplemental Table 1 on discussed leukocyte markers). Activation of neutrophils during ECMO is predominantly identified by the increased expression of CD11b and CD18 in both clinical and experimental ECMO settings (13, 17, 18, 31–36). Similar phenotypic modulation of neutrophils is reported in CPB patients post-operation (43, 71), but their expression begins to downregulate after 3–4 h of ECMO and can persist for a prolonged duration (18, 32, 34). This is also observed in other innate immune cells (32), which could be associated with an increased adhesion or binding with ligands as part of the activation process during ECMO. CD11b and CD18 are integrins essential to regulating adhesion and migration of leukocytes to mediate the inflammatory response. Modulation of these molecules expressed on the surface of leukocytes, including neutrophils, can have an impact on their crucial role in trans-endothelial and trans-epithelial migration to areas of inflammation as part of host immune defense (36, 72). However, the effect of ECMO on L-selectin (CD62L), another key adhesion molecule, remains debatable. Three studies independently reported either a reduced, augmented, or no change in expression of L-selectin at the initial hours of ECMO (18, 32, 33). Observed discrepancies may be associated with the differences between human, porcine and ex vivo ECMO. Activation of neutrophils is also characterized by degranulation. During ECMO, neutrophil elastase (NE) and myeloperoxidase (MPO) levels rise significantly within 2–4 h alongside other inflammatory mediators (31, 32, 34, 37). NE and MPO are pro-inflammatory granules released into the extracellular space by activated neutrophils for clearance of Gram-negative bacteria and mediate anti-microbial activities (73). It is part of an emerging concept for neutrophil function, the formation of neutrophil extracellular traps (NETs).
Alongside neutrophils, monocyte phenotype that tightly governs their functions is also altered as seen in valve replacement patients exposed to CPB. Monocytes become activated with increased CD11b, but the surface expression of human leukocyte antigen (HLA)-DR was significantly lower compared to the preoperative state (43). Indeed, such modulation is witnessed in experimental and clinical ECMO (21, 32). Monocytes expressing TLR4, an essential receptor in the recognition of bacterial pathogens, were also low during VV-ECMO. Authors speculated that it is caused by intensive endocytosis of CD14/TLR4 due to rampant damage-associated molecular pattern molecules from the pre-existing ARDS and further exacerbating by the immunological imbalance mediated by ECMO (22). These profiles are also observed in infected and septic patients (58, 63), and some are linked to mortality (22, 74).
Impact on the Adaptive Immune Leukocytes
T and B lymphocytes are essential to the adaptive immune system: if they become dysregulated, the capacity of the immune system to distinguish self from foreign and provide specialized immune defense can be hindered. Reduction in the overall lymphocyte numbers during ECMO, and the impediment of their recovery post-weaning is associated with mortality in patients (14, 15, 19, 20). A significantly lower T regulatory lymphocyte number (a subset of T lymphocytes) has been further identified in deceased patients on day 3 of ECMO, coupled with high anti-inflammatory interleukin (IL)-10 (22). A similar reduction was evident in valve replacement surgery patients using CPB (43). More recent publications have examined the potential of neutrophil-to-lymphocyte ratio (NLR) as a predictor of poor prognosis and survival in post-cardiotomic shock patients supported with ECMO. NLR is a ratio determined by dividing neutrophil absolute counts by lymphocyte absolute counts and is an indicator of perturbed leukocyte homeostasis. ECMO support is reported to increase the NLR values, and patients with high NLR have an increased risk for renal complications and mortality compared to those with a lower ratio (28). In CPB, higher NLR is associated with longer extubation time and a longer duration of ICU stay, consistent with ECMO (75). The prognostic capacity of NLR is further demonstrated across various critical illnesses (56, 76, 77).
Unlike the innate immune cells, phenotypic profiling of T and B lymphocyte modulation during ECMO is infrequent. An ex vivo ECMO study reported no changes in CD18 and CD62L expression in overall lymphocyte population (32). While CD161 expressing T helper lymphocytes were downregulated in both VA- and VV-ECMO neonates and pediatrics, changes were not observed for cytotoxic T or B lymphocytes. There remains a need for more studies to provide further indications of the role ECMO may have on the adaptive immune response. Future phenotypic research may also benefit from investigating other key subsets such as T helper 1, 2, and 17 lymphocytes over the overall lymphocyte or T helper population. Each T helper lymphocyte subset have specialized function and response required for differential adaptive immune response, thus, changes of the subsets could be overlooked when assessing the wider population.
Taken together, innate and adaptive leukocyte numbers can be a powerful prognostic tool, and development of a more comprehensive database including a larger heterogenous ECMO population could prove advantageous. However, the data generated cannot inform delineation of changes mediated by pre-existing disease and ECMO, or targeted future therapies. Based on the indicated profile of these leukocytes, increased surface molecules for adhesion and activation are associated with leukocytopenia. Therefore, characterization of leukocyte phenotype during ECMO would be complementary and important.
What Are the Causes of ECMO-Related Leukocyte Modulation?
ECMO Technology (Circuit Designs)
Direct contact of blood with the foreign surface of the ECMO circuit is widely accepted as a consequence of modulated blood physiology. While advances in ECMO technology have improved circuit biocompatibility and reduced activation of the coagulation system, significant immune changes persist, potentially contributing to SIRS, infection, new organ dysfunction, and thrombosis.
Blood Pumps
Blood pumps are a crucial component for extracorporeal blood circulation within the ECMO module. CPB and ECMO studies established the benefits of newer modified centrifugal pumps over roller pumps on blood damage reduction (78–80). However, no direct comparative investigation has been performed on ECMO specific to leukocyte modulation to accurately discern the differences. Previous CPB studies have demonstrated lower leukocyte loss, alongside reduced hemolysis, inflammatory response, and platelet activation when using centrifugal pumps (79). ECMO is assumed to follow a similar trend, and changes may lie largely with the oxygenator.
Oxygenators
Another major technological evolution of ECMO after 2007, was the implementation of polymethylpentene (PMP) hollow-fiber oxygenator membranes required for oxygenation. Early generations used bubble and silicone-based spiral coil membrane oxygenators that resulted in a significant drop in overall circulating leukocyte numbers in comparison to hollow-fiber membrane oxygenators. ECMO using The Kolobow silicone oxygenator membrane also reported increased leukocyte loss, deformability, and clogging rate to the oxygenator ex vivo, compared to hollow-fiber (30, 31). Observed enhancement for hollow-fiber membranes are associated with dampened activation of neutrophils characterized by the lower expression of CD11b adhesion molecule, and NE and superoxide anion release (30, 81).
Hollow-fiber membrane oxygenators are designed for a longer use duration (days to weeks) and have enhanced biocompatibility, however, modulatory effects are still reported (Tables 1 and 2). High cellular and coagulation factor deposits on PMP membrane oxygenators are observed (23, 24, 70). The immune modulatory influence of extracorporeal circuit with and without an hollow fiber oxygenator was compared using a rat model (35). Two major inflammatory signaling pathways of leukocytes, p38 mitogen-activated protein kinase (MAPK) and Nuclear factor kappa B (NF-κB) phosphorylation, were significantly upregulated after 1 h of extracorporeal circulation. An increase in severe lung injury scores was also reported, while no significant leukocyte changes or organ injury were observed in the absence of an oxygenator. Guided by reduced leukocyte and platelet adhesion profile, Obstals et al. proposed that optimization of the membrane surface using other non-thrombogenic polymer brushes could be beneficial (82).
Although the PMP hollow-fiber membrane oxygenators are now ubiquitous in the clinical setting, many different systems and manufactures are available. The degree of leukocyte and other cellular modulation mediated is likely to be different between centers and countries, in part, for this reason. As Bredthauer et al. have demonstrated, there are clear differences in leukocyte adhesion, as well as vWF deposition on PMP hollow-fiber oxygenator membranes between commercially available systems such as the Maquet(-Getinge) permanent life support (PLS) and Xenios AG Hilite LT (24). Variances in how a membrane is configured within the oxygenator can also influence blood flow rates and ultimately cellular and humoral responses. This is supported by the CPB literature, differential cellular deformability were observed when different oxygenators of the same microporous polypropylene hollow-fiber membrane were compared (83). However, careful consideration into the influences of other factors such as age, gender, and the patients’ underlying disease burden are essential for future studies comparing the potential impact of the different technologies (23, 24, 70).
Other ECMO-Associated Factors
Beyond ECMO technology, there are other common factors associated with ECMO that are likely to contribute to the leukocyte and blood component profile reported in patients. These factors include ECMO flow rates and the associated non-physiological shear stress exerted during extracorporeal circulation. However, current knowledge in this research area is very limited.
Two recently published ex vivo studies reported ECMO flow rates and the mediated non-physiological shear stress as potential influencing factors (37, 39). During continuous ECMO blood flow, wall shear stress within the rigid ECMO circuit increases to non-physiological levels as blood flow elevates. As illuminated by CPB, VAD, and fluid dynamics studies, variation in these parameters can differentially influence leukocyte phenotype, function, migration, and deformability (47, 84–88). At high adult and neonatal ECMO flow, significant increases in neutrophil activation, characterized by a rise in plasma neutrophil granules (NE and MPO), and leukocyte-derived extracellular vesicles were observed during 6 h of ECMO, in comparison to low flow. An upregulated expression of tissue factor on these extracellular vesicles and leukocytes was also reported, which could contribute to ECMO-related inflammation and thrombosis. The findings were further supported by a rapid increase of pro-inflammatory cytokines and chemokines, resembling SIRS (37). However, the observed differences between high and low flow rates were not detected experimentally for leukocyte activation phenotype (37), and clinically for hemolysis (89). These may be attributed to the absence of an underlying primary disease burden and an endothelium, as well as the use of cannula and the timing of blood collection ex vivo. In addition, for each oxygenator design and size there is likely an optimal flow for different cellular components that differentially minimizes non-physiological shear stress and damage, as seen in Meyer et al. study (39). Further animal and clinical research is required to validate the ex vivo effect of ECMO flow rate specific to leukocyte modulation.
Of note, the ECMO patient population is extremely diverse. This makes it challenging to characterize the effect of ECMO-related leukocyte modulation and to discern targets for intervention. In addition to their differential ECMO support, medications, anti-coagulant strategies, and premorbid health, patients are also exposed to different stressors and therapies. Therefore, investigations into other frequently used therapies with known leukocyte modulatory effects are also warranted as they are resource intensive.
What Are the Implications of Leukocyte Modulation in ECMO Patient Outcomes?
Infection
Development of infection is witnessed across various extracorporeal supports despite differences in the underlying primary disease burden in patients. Incidences are reported in cardiac patients subjected to open-heart cardiac surgery (90) and VAD implant (91), in addition to ECMO (with 3.5–64% infection rate) (8). These are often attributed to the exposure of wounds from surgeries, driveline insertion or cannulation. Santiago-Lozano et al. demonstrated that overall leukocyte numbers alone were unable to identify patients who will develop infection following VA-ECMO cannulation (26), and investigation into leukocyte subsets may provide additional information. Further phenotypic and functional immune studies revealed the potential underlying role of acquired immunoparalysis in the occurrence of infection in these patient cohorts (Figure 3).
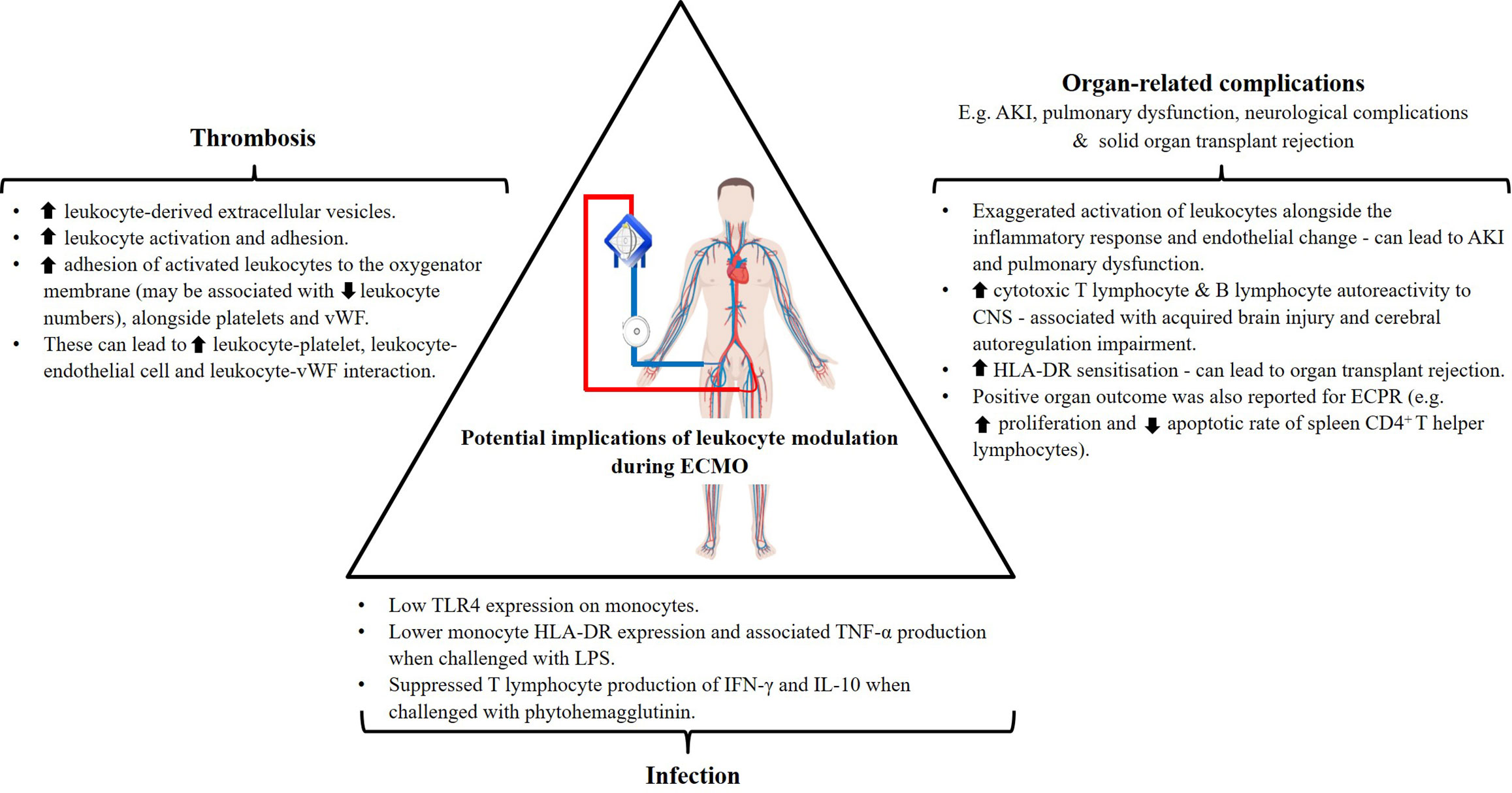
Figure 3 Potential implications of documented leukocyte modulation in ECMO patient outcomes. human leukocyte antigen (HLA)-DR, tumor necrosis factor (TNF)-α, interferon (IFN)-γ, interleukin (IL)-10, acute kidney injury (AKI), toll-like receptor (TLR)-4, lipopolysaccharides (LPS), von Willebrand factor (vWF) (Figure partially created with BioRender.com).
Monocytes and other antigen presenting cells (e.g. dendritic cells) express an abundant level of HLA-DR, which is used as a means of assessing innate immune function, as well as monocyte activation. During an infective episode, upregulation of HLA-DR expression on monocytes is anticipated to present antigens to lymphocytes, eliciting an immune response. Ziemba et al. have shown that upon endotoxin stimulation, monocyte HLA-DR expression and associated tumor necrosis factor (TNF)-α production were lower following ECMO than healthy controls (21). Low TLR4 expression on monocytes observed in non-survivors during early ECMO support may play a role in its lack of response, as it is a key pattern recognition receptor for endotoxin (22). Recently, Han et al. also proposed that reduced monocyte function is associated with increased CD71+ (transferrin receptor) immature erythrocytes found during ECMO, mediating an inhibitory effect (29). Upon depletion of these immune modulatory erythrocytes, beyond their traditional role, monocyte function was retained. In addition, ECMO patients are reported with suppressed T lymphocyte production of interferon (IFN)-γ and IL-10 following ex vivo phytohemagglutinin stimulation (21). These are consistent with Beshish et al. definition of innate immunoparalysis mediated by ECMO (92). The observed changes are also in line with profiles associated with sepsis, and nosocomial infections in post-CPB cardiac surgery and VAD implanted patients (40, 41, 49).
However, it remains speculative whether the lack of response from leukocytes is a result of ECMO use or the disease burden itself without a comparison to pre-ECMO response or sick controls. ECMO can be used to support patients who may already be immunoparalyzed such as those with sepsis and unsuccessful weaning from CPB, as shown in an earlier neonatal study (13). On the contrary, DePuydt and colleagues also reported enhanced candidacidal activity (phagocytosis and intracellular killing) in neonates (17). Differences may be associated with the variances between ECMO technology, duration, indication as well as the differential pathways of activation by fungi versus bacteria. Additional studies are necessary to determine whether the increased risk of infection in some patients is associated with the inability of leukocyte subsets to respond appropriately when immune challenges arise.
Organ-Related Complications
Acute kidney injury (AKI) affects up to 60% of ICU patients and is associated with mortality rates of between 15 and 60% (93). It is also the most common organ complication associated with ECMO, resulting in up to 30–45% of patients requiring renal replacement therapy while supported by this modality (6, 9). Despite extensive investigation in the setting of CPB, and improved ECMO circuit design to reduce hemolysis, AKI still occurs. The cause of AKI is complex, making it difficult to discern the relative contributions of the patients underlying pathology from ECMO itself. Currently there is no evidence to implicate ECMO as an independent risk factor for AKI. Two general phenomena have been proposed: ischemia- or inflammation-mediated insult (94). If ECMO support is inadequate for delivery of oxygen and to support metabolic demands, ischemic insults can occur, triggering a cascade of immunologic consequences and vasoconstriction. Alternatively, it can occur due to overwhelming activation of the systemic inflammatory response, leukocytes and endothelial cells, as seen in critically ill patients (56–59) and in those supported with ECMO (18, 32–34). The aberrant immunologic response can result in hyperdynamic states that also lead to AKI (94). Similar phenomena were also correlated with the induction of pulmonary dysfunction (34–36), seen in 1–8% of ECMO patients (6) (Figure 3).
In addition to AKI and pulmonary dysfunction, the influence of ECMO-related leukocyte modulation to potentiate other organ-related changes is proposed. Ortega and colleagues observed an increased T lymphocyte selective autoreactivity to the central nervous system components when ECMO support is used compared to sick controls (27). Further sub-analysis of the ECMO patient group revealed elevated cytotoxic T lymphocyte and B lymphocyte autoreactivity to central nervous system components in those with acquired brain injury and cerebral autoregulation impairment (27). These changes may have contributed to the subcategories of neurological complications reported by the current Extracorporeal Life Support registry (6). In contrast to other studies, Zhang et al. observed improved organ outcomes following VA-ECMO when used as extracorporeal cardiopulmonary resuscitation (ECPR) for post-resuscitation in a porcine model of cardiac arrest (38). VA-ECMO provided better stabilization of hemodynamics, tissue perfusion and oxygenation compared to the traditional approach. It was found to enhance the function of the spleen, unlike conventional management. The spleen is a T-lymphocyte-rich immune reservoir essential to immunological responses, and is a commonly affected organ following cardiac arrest and traditional post-resuscitation methods. Use of ECPR was able to reduce reactive oxygen species production and mitochondrial dysfunction, which contributed to enhanced proliferation and low apoptotic rate of spleen CD4+ T helper lymphocytes (38).
To date, there is insufficient mechanistic and clinical data to clearly delineate if the occurrence of organ dysfunction is ECMO-related or simply a secondary progression to the primary disease burden necessitating ECMO. Given the pivotal involvement of leukocytes at the initiation phase of organ injury, further dedicated mechanistic studies should be considered. This may inform a new capacity to delineate the two, and enable clinicians to predict, treat, and ameliorate the clinical sequelae. Furthermore, ECMO has been reported to increase HLA-DR sensitization in solid organ transplant patients (95, 96). As more ECMO is being used for transplantation, a better understanding of the role of ECMO-related leukocyte modulation in this setting is also necessary.
Thrombosis
Thrombosis is another serious complication of ECMO with the highest incidence recorded in neonates affecting up to 37.5% of patients (6, 10). Despite better anti-coagulation strategies using unfractionated heparin and development of heparinized circuitry, thrombosis still occurs. While the role of activated leukocytes and their upregulated expression of adhesion molecules in coagulation is acknowledged (11) (Figure 3), there is very little existing research in the setting of ECMO in comparison to the classic pathways. One study demonstrated increased production of both leukocyte- and platelet-derived pro-thrombogenic factors during ECMO circulation (39), as seen in VAD patients (48). In particular, increased tissue factor expression was reported on activated leukocytes and leukocyte-derived extracellular vesicles, acting as the activator of the tissue factor pathway for coagulation. Adhesion of these activated leukocytes to the oxygenator membrane, alongside platelets, and procoagulant factors such as von Willebrand factor (vWF), has also been hypothesized to further stimulate clot formation. A recently published study associated high level accumulation of VWF and other cellular deposits on the membrane oxygenator with elevated SOFA scores, severe thrombocytopenia, and persistent liver dysfunction in ECMO patients (70). Exposure of blood to extracorporeal circuitry and non-physiological conditions (increased shear stress), as seen in VADs and ECMO (97, 98), can also lead to alterations in vWF conformation and loss of normal pro-coagulant properties resulting in the development of acquired von Willebrand syndrome (99). In the other studies, no differences in the hemostatic parameters and clinical outcomes were observed between patients with more or less cellular deposits (23, 24). Nonetheless, the authors correlated higher deposition with younger patients (35 versus 61 years old) who had greater pre-ECMO leukocyte counts (23), and the oxygenator manufacturer/design used (24). Additional investigation into neonates and pediatrics, an even younger and thrombosis-prone population, may provide different insights. Furthermore, Bredthauer and colleagues showed that the frequently required anticoagulants used for ECMO inhibits neutrophil migration and chemotactic migration efficiency (100). The inhibitory effect of heparin and argatroban have been proposed to not only modulate neutrophil function but may also increase adhesion onto the membrane oxygenator to prompt clot formation.
Thrombosis can also occur upon adhesion of activated leukocytes to platelets and endothelial cells, two of the major players of coagulation. Complexes of leukocyte integrin (CD11b/CD18) with platelet adhesion molecules (P-selectin glycoprotein ligand-1 and glycoprotein Ib alpha polypeptide) are known to regulate thrombosis (51). The interaction has been suggested to be a better marker than P-selectin expression on platelets due to its transient expression (51, 55). In addition, thrombotic events can be initiated and propagated through interaction between activated innate immune leukocytes (neutrophil and monocyte) and endothelial cells (e.g. between CD11b/CD18 and endothelial cell Intercellular Adhesion Molecule (ICAM)-1; chemokine receptor 2 and ligand 2) (52–54).
A targeted reduction or inhibition of these surface molecules on leukocytes using antibodies may provide an additional avenue to help reduce the incidence of thrombosis (101, 102). To further address this issue, a number of new strategies are being investigated in vitro and clinically such as optimization of the oxygenator PMP membrane surface (82) and administration of supplemental nitric oxide (NECTAR-KIDS randomized control trial; ACTRN12619001518156) to help restore the balance of the immunologic and hemostatic systems. Neutrophil and monocyte assessment, as well as their extracellular vesicle thrombotic phenotype may also prove to be beneficial in these studies.
Future Directions
Current evidence underscores the importance of leukocytes and their subpopulations as biomarkers of inflammation, predictors of mortality and/or poor patient outcomes across a spectrum of critical illnesses (56, 63, 76, 103, 104). There have been numerous therapeutic interventions targeting leukocytes (102, 105, 106). However, available reports on leukocytes in the context of ECMO are rudimentary. This limits the potential for further technological solutions or targeted treatments to minimize ECMO-mediated leukocyte modulation, and the adverse events that accompany it. In addition to phenotypic and functional profiling, interrogation into the impact of ECMO on leukocyte extracellular vesicle generation, and their interaction with platelets and endothelial cells will be essential for knowledge progression. Future studies should also aim to address two major challenges of current leukocyte research: ECMO technological disparity and the lack of understanding in the compound effect of ECMO with other clinical stressors and therapies.
Addressing Technological (Circuit Designs) Disparities
The technology employed in experimental and clinical ECMO research conducted pre- and post-2007 is substantially different, necessitating a technological disclaimer when performing comparative meta-analysis. Additionally, consistent reporting of the ECMO system or circuit biomaterial used in future publications would be advantageous to assist analysis. While the biomaterial and centrifugal pump between different clinically used ECMO systems are relatively homogenous at present, the membrane configuration of the oxygenators remains quite different in some. These differences may yield diverse modulation of leukocyte profiles, and similarly for other blood components (24, 30, 31, 83), however this has yet to be thoroughly investigated. In order to address the modern era ECMO technological discrepancies, a benchmark oxygenator study would prove beneficial. This may be achieved by profiling and assessing leukocyte changes and other cellular functions when exposed to different ECMO circuit designs using PMP hollow-fiber membranes. This will allow for fair comparison between studies and will help to build a more comprehensive library of immune profiles for ECMO, and future optimization of the technology.
Understanding Factors Contributing to ECMO-Related Leukocyte Modulation
Other than the discussed non-physiological stressors of changing flow dynamics and the associated shear stress generated within the circuit, patients are also frequently exposed to therapies such as multiple blood transfusions when treating bleeding complications (107), heparinization to reduce clot formations (11, 12), supranormal administration of supplemental oxygen concerning hypoxia (108), and renal replacement therapy for ECMO-related AKI (9). These therapies have been independently reported to modulate leukocyte phenotype and function (44–46, 100, 109–111), which can result in a worsened prognosis. The cumulative impact of these therapies and stressors is likely to be significant. This provides the impetus for the development of a comprehensive mechanistic understanding of immunobiology and pathophysiology in the context of ECMO. Together, these aspects will need to be controlled in both scientific and clinical studies to make these research outcomes meaningful.
Potential Interventions and Targets for ECMO-Related Leukocyte Modulation
Given that one of the reasons for the modulated leukocyte profiles is likely initiated by the direct contact between patient blood and the non-endothelial surfaces of ECMO circuits, components with the capacity to reduce leukocyte activation and adhesion, or to boost pathogenic function, may be beneficial. Nitric oxide is an element capable of enhancing the anti-inflammatory capacity of the immune system as well as reducing cellular activation and adhesion (112–114). An early ex vivo ECMO study has also highlighted the potential importance of delivery methods when using supplemental gas to improve biological and clinical outcomes (e.g. direct infusion into circulating blood versus administrating into the oxygenator) (33). The benefit of nitric oxide administration into the oxygenator in patients undergoing cardiac surgery has been demonstrated with improved outcomes in a number of randomized control trials (115, 116). Further, adoption of specific leukocyte targets may also prove more effective. For example, use of inhibitors to dampen or transiently eradicate the inflammatory response mediated by leukocyte activation and adhesion. The β2-integrin CD11b/CD18 surface antigen is commonly used to characterize activation of neutrophils and has recently been proposed as a novel therapeutic target for controlling inflammation (101). Thus, inhibition of the surface antigen and downstream processes may alleviate patients’ resistance to sustained manifestations of neutrophil activity, interaction with endothelial cells and migration to organs resulting in MODS. Other studies have reported efficacy in blocking adhesion surface antigens of lymphocytes using antibodies and antagonists in various inflammatory diseases (102). Alternatively, the leukocyte inhibition module, a medical device that limits overshooting of neutrophils post-operative activity following CPB, could be useful for ECMO patients. The efficacy and safety of this device has been demonstrated in patients, with the capacity to limit neutrophil activity and inflammatory responses (117–119). However, until a better understanding of leukocytes in ECMO is available, attempts to create specific immune interventions to improve patient outcomes must be cautious and will remain challenging.
Conclusions
Leukocytes are central to the pathophysiology of critical illness. An increasingly detailed understanding of phenotypic and functional alterations in leukocyte populations during critical illness has opened new areas of research. This is less well developed in the context of ECMO, compared to other extracorporeal devices, where research is lacking. In adults particularly, no mechanistic data is available, despite the widespread adoption of this modality. If advances in ECMO technology are to translate into continued improvements in outcome this deficit needs to be addressed. Specifically, we need to understand the full spectrum of ECMO-related leukocyte modulation in the heterogenous patient populations, and that associated with different ECMO circuits and therapies. This knowledge could provide data to help delineate the immunologic signatures between primary disease burden and ECMO, optimize ECMO design, and lessen its effects on leukocytes to help reduce the frequently reported complications associated with ECMO. Equally, a careful selection of primary measures and appropriately powered clinical study design are essential in determining the impact of these biological perturbations on patient outcomes. With these insights, we may then facilitate risk stratification for patients to inform clinical decision-making and to optimize survival, quality of life, and resource allocation.
Author Contributions
KK and JS conceived the review, and KK wrote the original draft of the manuscript. JM, DL, MP, CM, KS, MS-H, HC, JS, and JF reviewed the manuscript, and rewrote individual sections. Both JS and JF have contributed equally to this manuscript as co-last authors. All authors contributed to the article and approved the submitted version.
Funding
Publication of this review is funded by The National Health and Medical Research Council Centre for Research Excellence in Advanced Cardio-respiratory Therapies Improving OrgaN Support (APP1079421, Australia) and The Prince Charles Hospital Foundation (The Common Good). This work was also supported by the National Institute for Health Research Clinician Scientist Award (CS-2016-16-011 for MS-H), the Metro North Hospital and Health Service, University of Queensland and Queensland Health Bionics (for KK), Advance Queensland (for JS), and the Office of Health and Medical Research, Queensland Health (for JF).
Conflict of Interest
The authors declare that the research was conducted in the absence of any commercial or financial relationships that could be construed as a potential conflict of interest.
Acknowledgments
The authors would like to thank the National Health and Medical Research Council Centre for Research Excellence, The Prince Charles Hospital Foundation (The Common Good), University of Queensland, Office of Health and Medical Research and Queensland Government for the financial assistance provided for the publication of this manuscript. This review is solely the responsibility of the authors and does not reflect the views of the above funders. Also, this work was supported by the National Institute for Health Research Clinician Scientist Award (CS-2016-16-011 for MS-H). The views expressed in this publication are those of the authors and not necessarily those of the National Health Service, the National Institute for Health Research, or the Department of Health and Social Care.
Supplementary Material
The Supplementary Material for this article can be found online at: https://www.frontiersin.org/articles/10.3389/fimmu.2020.600684/full#supplementary-material
References
1. Thiagarajan RR, Barbaro RP, Rycus PT, Mcmullan DM, Conrad SA, Fortenberry JD, et al. Extracorporeal Life Support Organization Registry International Report 2016. Asaio J (2017) 63:60–7. doi: 10.1097/mat.0000000000000475
2. Bréchot N, Hajage D, Kimmoun A, Demiselle J, Agerstrand C, Montero S, et al. Venoarterial extracorporeal membrane oxygenation to rescue sepsis-induced cardiogenic shock: a retrospective, multicentre, international cohort study. Lancet (2020) 396:545–52. doi: 10.1016/s0140-6736(20)30733-9
3. Combes A, Hajage D, Capellier G, Demoule A, Lavoue S, Guervilly C, et al. Extracorporeal Membrane Oxygenation for Severe Acute Respiratory Distress Syndrome. N Engl J Med (2018) 378:1965–75. doi: 10.1056/NEJMoa1800385
4. Noah MA, Peek GJ, Finney SJ, Griffiths MJ, Harrison DA, Grieve R, et al. Referral to an extracorporeal membrane oxygenation center and mortality among patients with severe 2009 influenza A(H1N1). JAMA (2011) 306:1659–68. doi: 10.1001/jama.2011.1471
5. Peek GJ, Mugford M, Tiruvoipati R, Wilson A, Allen E, Thalanany MM, et al. Efficacy and economic assessment of conventional ventilatory support versus extracorporeal membrane oxygenation for severe adult respiratory failure (CESAR): a multicentre randomised controlled trial. Lancet (2009) 374:1351–63. doi: 10.1016/s0140-6736(09)61069-2
6. Extracorporeal Life Support Organization. “ECLS Registry Report: Interntional Summary 2019”. Ann Arbor, MI: Extracorporeal Life Support Organization (2019).
7. Lequier L, Horton SB, Mcmullan DM, Bartlett RH. Extracorporeal Membrane Oxygenation Circuitry. Pediatr Crit Care Med (2013) 14:S7–12. doi: 10.1097/PCC.0b013e318292dd10
8. Maclaren G, Schlapbach LJ, Aiken AM. Nosocomial Infections During Extracorporeal Membrane Oxygenation in Neonatal, Pediatric, and Adult Patients: A Comprehensive Narrative Review. Pediatr Crit Care Med (2020) 21:283–90. doi: 10.1097/pcc.0000000000002190
9. Thongprayoon C, Cheungpasitporn W, Lertjitbanjong P, Aeddula NR, Bathini T, Watthanasuntorn K, et al. Incidence and Impact of Acute Kidney Injury in Patients Receiving Extracorporeal Membrane Oxygenation: A Meta-Analysis. J Clin Med (2019) 8:981. doi: 10.3390/jcm8070981
10. Dalton HJ, Reeder R, Garcia-Filion P, Holubkov R, Berg RA, Zuppa A, et al. Factors Associated with Bleeding and Thrombosis in Children Receiving Extracorporeal Membrane Oxygenation. Am J Respir Crit Care Med (2017) 196:762–71. doi: 10.1164/rccm.201609-1945OC
11. Millar JE, Fanning JP, Mcdonald CI, Mcauley DF, Fraser JF. The inflammatory response to extracorporeal membrane oxygenation (ECMO): a review of the pathophysiology. Crit Care (2016) 20:387. doi: 10.1186/s13054-016-1570-4
12. Doyle AJ, Hunt BJ. Current Understanding of How Extracorporeal Membrane Oxygenators Activate Haemostasis and Other Blood Components. Front Med (2018) 5:352. doi: 10.3389/fmed.2018.00352
13. Hocker JR, Wellhausen SR, Ward RA, Simpson PM, Cook LN. Effect of extracorporeal membrane oxygenation on leukocyte function in neonates. Artif Organs (1991) 15:23–8. doi: 10.1111/j.1525-1594.1991.tb00755.x
14. Zach TL, Steinhorn RH, Georgieff MK, Mills MM, Green TP. Leukopenia associated with extracorporeal membrane oxygenation in newborn infants. J Pediatr (1990) 116:440–4. doi: 10.1016/S0022-3476(05)82840-9
15. Depalma L, Short BL, Meurs KV, Luban NLC. A flow cytometric analysis of lymphocyte subpopulations in neonates undergoing extracorporeal membrane oxygenation. J Pediatr (1991) 118:117–20. doi: 10.1016/S0022-3476(05)81862-1
16. Plotz FB, Van Oeveren W, Bartlett RH, Wildevuur CR. Blood activation during neonatal extracorporeal life support. J Thorac Cardiovasc Surg (1993) 105:823–32. doi: 10.1016/S0022-5223(19)34156-X
17. Depuydt LE, Schuit KE, Smith SD. Effect of extracorporeal membrane oxygenation on neutrophil function in neonates. Crit Care Med (1993) 21:1324–7. doi: 10.1097/00003246-199309000-00015
18. Fortenberry JD, Bhardwaj V, Niemer P, Cornish JD, Wright JA, Bland L. Neutrophil and cytokine activation with neonatal extracorporeal membrane oxygenation. J Pediatr (1996) 128:670–8. doi: 10.1016/S0022-3476(96)80133-8
19. Kawahito K, Kobayashi E, Misawa Y, Adachi H, Fujimura A, Ino T, et al. Recovery from lymphocytopenia and prognosis after adult extracorporeal membrane oxygenation. Arch Surg (1998) 133:216–7. doi: 10.1001/archsurg.133.2.216
20. Hong TH, Hu FC, Kuo SW, Ko WJ, Chow LP, Hsu LM, et al. Predicting outcome in patients under extracorporeal membrane oxygenation due to cardiogenic shock through dynamic change of lymphocytes and interleukins. IJC Metab Endocrine (2015) 7:36–44. doi: 10.1016/j.ijcme.2014.11.001
21. Ziemba K, Jyotsna N, Hanson-Huber L, Steele L, Cismowski M, West T, et al. Innate and adaptive immune function during extracorporeal membrane oxygenation. Crit Care Med (2016) 44:232. doi: 10.1097/01.ccm.0000509302.65625.4f
22. Liu CH, Kuo SW, Ko WJ, Tsai PR, Wu SW, Lai CH, et al. Early measurement of IL-10 predicts the outcomes of patients with acute respiratory distress syndrome receiving extracorporeal membrane oxygenation. Sci Rep (2017) 7:1021. doi: 10.1038/s41598-017-01225-1
23. Wilm J, Philipp A, Muller T, Bredthauer A, Gleich O, Schmid C, et al. Leukocyte adhesion as an indicator of oxygenator thrombosis during extracorporeal membrane oxygenation therapy? ASAIO J (2017) 64:24–30. doi: 10.1097/mat.0000000000000586
24. Bredthauer A, Wilm J, Philipp A, Foltan M, Mueller T, Lehle K. The oxygenator design might influence the adhesion of leukocytes and deposits of von willebrand fibers on the surface of gas exchange membranes during ECMO. Eur J Heart Fail (2017) 19:36–7. doi: 10.1002/ejhf.869
25. Francischetti IMB, Szymanski J, Rodriguez D, Heo M, Wolgast LR. Laboratory and clinical predictors of 30-day survival for patients on Extracorporeal Membrane Oxygenation (ECMO): 8-Year experience at Albert Einstein College of Medicine, Montefiore Medical Center. J Crit Care (2017) 40:136–44. doi: 10.1016/j.jcrc.2017.03.027
26. Santiago-Lozano MJ, Barquin-Conde ML, Fuentes-Moreno L, Leon-Vela RM, Madrid-Vazquez L, Sanchez-Galindo A, et al. Infectious complications in paediatric patients treated with extracorporeal membrane oxygenation. Enferm Infecc Microbiol Clin (2018) 36:563–67. doi: 10.1016/j.eimc.2017.10.025
27. Ortega SB, Pandiyan P, Windsor J, Torres VO, Selvaraj UM, Lee A, et al. A Pilot Study Identifying Brain-Targeting Adaptive Immunity in Pediatric Extracorporeal Membrane Oxygenation Patients With Acquired Brain Injury. Crit Care Med (2019) 47:e206–e13. doi: 10.1097/ccm.0000000000003621
28. Sargin M, Mete MT, Erdogan SB, Kuplay H, Bastopcu M, Akansel S, et al. Prognostic Value of Neutrophil Lymphocyte Ratio for Early Renal Failure in ECMO Patients. J Heart Lung Transplant (2019) 38:S175. doi: 10.1016/j.healun.2019.01.420
29. Han J, Cao Y, Hao X, Fan L, Du Z, Hou X, et al. CD71+ erythroid cells inhibit inflammatory cytokines secretion of monocytes during extracorporeal membrane oxygenation. J Immunol Res (2020) 204:148.16.
30. Bergman P, Belboul A, Friberg LG, Al-Khaja N, Mellgren G, Roberts D. The effect of prolonged perfusion with a membrane oxygenator (PPMO) on white blood cells. Perfusion (1994) 9:35–40. doi: 10.1177/026765919400900106
31. Skogby M, Mellgren K, Adrian K, Friberg LG, Chevalier JY, Mellgren G. Induced cell trauma during in vitro perfusion: a comparison between two different perfusion systems. Artif Organs (1998) 22:1045–51. doi: 10.1046/j.1525-1594.1998.06064.x
32. Graulich J, Walzog B, Marcinkowski M, Bauer K, Kossel H, Fuhrmann G, et al. Leukocyte and endothelial activation in a laboratory model of extracorporeal membrane oxygenation (ECMO). Pediatr Res (2000) 48:679–84. doi: 10.1203/00006450-200011000-00021
33. Adrian K, Skogby M, Friberg LG, Mellgren K. The effect of s-nitroso-glutathione on platelet and leukocyte function during experimental extracorporeal circulation. Artif Organs (2003) 27:570–5. doi: 10.1046/j.1525-1594.2003.07106.x
34. Mcilwain RB, Timpa JG, Kurundkar AR, Holt DW, Kelly DR, Hartman YE, et al. Plasma concentrations of inflammatory cytokines rise rapidly during ECMO-related SIRS due to the release of preformed stores in the intestine. Lab Invest (2010) 90:128–39. doi: 10.1038/labinvest.2009.119
35. Rungatscher A, Tessari M, Stranieri C, Solani E, Linardi D, Milani E, et al. Oxygenator is the main responsible for leukocyte activation in experimental model of extracorporeal circulation: A cautionary tale. Mediators Inflammation (2015) 2015:7. doi: 10.1155/2015/484979
36. Passmore MR, Fung YL, Simonova G, Foley SR, Dunster KR, Diab SD, et al. Inflammation and lung injury in an ovine model of extracorporeal membrane oxygenation support. Am J Physiol Lung Cell Mol Physiol (2016) 311:L1202–l12. doi: 10.1152/ajplung.00296.2016
37. Ki KK, Passmore MR, Chan CHH, Malfertheiner MV, Bouquet M, Cho HJ, et al. Effect of ex vivo extracorporeal membrane oxygenation flow dynamics on immune response. Perfusion (2019) 34:5–14. doi: 10.1177/0267659119830012
38. Zhang Q, Liu B, Zhao L, Lian Y, Yuan X, Zhang Y, et al. Venoarterial Extracorporeal Membrane Oxygenation Increased Immune Function of Spleen and Decreased Reactive Oxygen Species During Post-Resuscitation. Artif Organs (2019) 43:377–85. doi: 10.1111/aor.13367
39. Meyer AD, Rishmawi AR, Kamucheka R, Lafleur C, Batchinsky AI, Mackman N, et al. Effect of blood flow on platelets, leukocytes, and extracellular vesicles in thrombosis of simulated neonatal extracorporeal circulation. J Thromb Haemost (2020) 18:399–410. doi: 10.1111/jth.14661
40. Perros AJ, Esguerra-Lallen A, Rooks K, Chong F, Engkilde-Pedersen S, Faddy HM, et al. Coronary artery bypass grafting is associated with immunoparalysis of monocytes and dendritic cells. J Cell Mol Med (2020) 24:4791–803. doi: 10.1111/jcmm.15154
41. Wilhelm W, Grundmann U, Rensing H, Werth M, Langemeyer J, Stracke C, et al. Monocyte deactivation in severe human sepsis or following cardiopulmonary bypass. Shock (2002) 17:354–60. doi: 10.1097/00024382-200205000-00002
42. Rossaint J, Berger C, Van Aken H, Scheld HH, Zahn PK, Rukosujew A, et al. Cardiopulmonary bypass during cardiac surgery modulates systemic inflammation by affecting different steps of the leukocyte recruitment cascade. PloS One (2012) 7:e45738–8. doi: 10.1371/journal.pone.0045738
43. Rudensky B, Yinnon AM, Shutin O, Broide E, Wiener-Well Y, Bitran D, et al. The cellular immunological responses of patients undergoing coronary artery bypass grafting compared with those of patients undergoing valve replacement. Eur J Cardiothorac Surg (2010) 37:1056–62. doi: 10.1016/j.ejcts.2009.12.002
44. Liakopoulos V, Jeron A, Shah A, Bruder D, Mertens PR, Gorny X. Hemodialysis-related changes in phenotypical features of monocytes. Sci Rep (2018) 8:13964–64. doi: 10.1038/s41598-018-31889-2
45. Griveas I, Visvardis G, Sakellariou G, Passadakis P, Thodis I, Vargemezis V, et al. Biocompatibility study based on differential sequestration kinetics of CD14+CD16+ blood monocyte subsets with different dialyzers. Ren Fail (2006) 28:493–9. doi: 10.1080/08860220600781336
46. Sester U, Sester M, Heine G, Kaul H, Girndt M, Köhler H. Strong depletion of CD14(+)CD16(+) monocytes during haemodialysis treatment. Nephrol Dial Transplant (2001) 16:1402–8. doi: 10.1093/ndt/16.7.1402
47. Radley G, Laura Pieper I, Thomas BR, Hawkins K, Thornton CA. Artificial shear stress effects on leukocytes at a biomaterial interface. Artif Organs (2019) 43:E139–e51. doi: 10.1111/aor.13409
48. Diehl P, Aleker M, Helbing T, Sossong V, Beyersdorf F, Olschewski M, et al. Enhanced microparticles in ventricular assist device patients predict platelet, leukocyte and endothelial cell activation. Interact Cardiovasc Thoracic Surg (2010) 11:133–37. doi: 10.1510/icvts.2010.232603
49. Kirsch M, Boval B, Damy T, Ghendouz S, Vermes E, Loisance D, et al. Importance of monocyte deactivation in determining early outcome after ventricular assist device implantation. Int J Artif Organs (2012) 35:169–76. doi: 10.5301/ijao.5000053
50. Rich RR, Chaplin DD. “1 - The Human Immune Response,”. In: Rich RR, Fleisher TA, Shearer WT, Schroeder HW, Frew AJ, Weyand CM, editors. Clinical Immunology, Fifth Edition. London: Content Repository Only (2019). p. 3–17.e1.
51. Wang Y, Gao H, Shi C, Erhardt PW, Pavlovsky A, A Soloviev D, et al. Leukocyte integrin Mac-1 regulates thrombosis via interaction with platelet GPIbα. Nat Commun (2017) 8:15559–59. doi: 10.1038/ncomms15559
52. Laurance S, Bertin F-R, Ebrahimian T, Kassim Y, Rys RN, Lehoux S, et al. Gas6 Promotes Inflammatory (CCR2 hi CX3CR1 lo) Monocyte Recruitment in Venous Thrombosis. Arterioscler Thromb Vasc Biol (2017) 37:1315–22. doi: 10.1161/ATVBAHA.116.308925
53. Von Brühl M-L, Stark K, Steinhart A, Chandraratne S, Konrad I, Lorenz M, et al. Monocytes, neutrophils, and platelets cooperate to initiate and propagate venous thrombosis in mice in vivo. J Exp Med (2012) 209:819–35. doi: 10.1084/jem.20112322
54. Liu X, Xue Y, Ding T, Sun J. Enhancement of proinflammatory and procoagulant responses to silica particles by monocyte-endothelial cell interactions. Part Fibre Toxicol (2012) 9:36. doi: 10.1186/1743-8977-9-36
55. Michelson AD, Barnard MR, Krueger LA, Valeri CR, Furman MI. Circulating monocyte-platelet aggregates are a more sensitive marker of in vivo platelet activation than platelet surface P-selectin: studies in baboons, human coronary intervention, and human acute myocardial infarction. Circulation (2001) 104:1533–7. doi: 10.1161/hc3801.095588
56. Li W, Ai X, Ni Y, Ye Z, Liang Z. The Association Between the Neutrophil-to-Lymphocyte Ratio and Mortality in Patients with Acute Respiratory Distress Syndrome: A Retrospective Cohort Study. Shock (2018) 51:161–7. doi: 10.1097/shk.0000000000001136
57. Peng Y, Wang J, Xiang H, Weng Y, Rong F, Xue Y, et al. Prognostic Value of Neutrophil-Lymphocyte Ratio in Cardiogenic Shock: A Cohort Study. Med Sci Mon Int Med J Exp Clin Res (2020) 26:e922167–e67. doi: 10.12659/MSM.922167
58. Conway Morris A, Anderson N, Brittan M, Wilkinson TS, Mcauley DF, Antonelli J, et al. Combined dysfunctions of immune cells predict nosocomial infection in critically ill patients. Br J Anaesth (2013) 111:778–87. doi: 10.1093/bja/aet205
59. Allen ML, Peters MJ, Goldman A, Elliott M, James I, Callard R, et al. Early postoperative monocyte deactivation predicts systemic inflammation and prolonged stay in pediatric cardiac intensive care. Crit Care Med (2002) 30:1140–5. doi: 10.1097/00003246-200205000-00031
60. Baufreton C, Intrator L, Jansen PG, Te Velthuis H, Le Besnerais P, Vonk A, et al. Inflammatory response to cardiopulmonary bypass using roller or centrifugal pumps. Ann Thorac Surg (1999) 67:972–7. doi: 10.1016/s0003-4975(98)01345-9
61. Mcbride WT, Armstrong MA, Crockard AD, Mcmurray TJ, Rea JM. Cytokine balance and immunosuppressive changes at cardiac surgery: contrasting response between patients and isolated CPB circuits. Br J Anaesth (1995) 75:724–33. doi: 10.1093/bja/75.6.724
62. Cuinet J, Garbagnati A, Rusca M, Yerly P, Schneider AG, Kirsch M, et al. Cardiogenic shock elicits acute inflammation, delayed eosinophilia, and depletion of immune cells in most severe cases. Sci Rep (2020) 10:7639–39. doi: 10.1038/s41598-020-64702-0
63. Conway Morris A, Datta D, Shankar-Hari M, Stephen J, Weir CJ, Rennie J, et al. Cell-surface signatures of immune dysfunction risk-stratify critically ill patients: INFECT study. Intensive Care Med (2018) 44:627–35. doi: 10.1007/s00134-018-5247-0
64. Pfortmueller CA, Meisel C, Fux M, Schefold JC. Assessment of immune organ dysfunction in critical illness: utility of innate immune response markers. Intensive Care Med Exp (2017) 5:49–9. doi: 10.1186/s40635-017-0163-0
65. Mildner RJ, Taub N, Vyas JR, Killer HM, Firmin RK, Field DJ, et al. Cytokine imbalance in infants receiving extracorporeal membrane oxygenation for respiratory failure. Biol Neonate (2005) 88:321–7. doi: 10.1159/000087630
66. Risnes I, Wagner K, Ueland T, Mollnes T, Aukrust P, Svennevig J. Interleukin-6 may predict survival in extracorporeal membrane oxygenation treatment. Perfusion (2008) 23:173–8. doi: 10.1177/0267659108097882
67. Chen Q, Yu W, Shi J, Shen J, Hu Y, Gao T, et al. The effect of venovenous extra-corporeal membrane oxygenation (ECMO) therapy on immune inflammatory response of cerebral tissues in porcine model. J Cardiothorac Surg (2013) 8:186–86. doi: 10.1186/1749-8090-8-186
68. Hwang J, Saha A, Boo YC, Sorescu GP, Mcnally JS, Holland SM, et al. Oscillatory shear stress stimulates endothelial production of O2- from p47phox-dependent NAD(P)H oxidases, leading to monocyte adhesion. J Biol Chem (2003) 278:47291–8. doi: 10.1074/jbc.M305150200
69. Chatterjee S. Endothelial Mechanotransduction, Redox Signaling and the Regulation of Vascular Inflammatory Pathways. Front Physiol (2018) 9:524. doi: 10.3389/fphys.2018.00524
70. Steiger T, Foltan M, Philipp A, Mueller T, Gruber M, Bredthauer A, et al. Accumulations of von Willebrand factor within ECMO oxygenators: Potential indicator of coagulation abnormalities in critically ill patients? Artif Organs (2019) 43:1065–76. doi: 10.1111/aor.13513
71. Asimakopoulos G, Kohn A, Stefanou DC, Haskard DO, Landis RC, Taylor KM. Leukocyte integrin expression in patients undergoing cardiopulmonary bypass. Ann Thorac Surg (2000) 69:1192–7. doi: 10.1016/s0003-4975(99)01553-2
72. Schnoor M, Parkos CA. Disassembly of endothelial and epithelial junctions during leukocyte transmigration. Front Biosci (2008) 13:6638–52. doi: 10.2741/3178
73. Pham CT. Neutrophil serine proteases: specific regulators of inflammation. Nat Rev Immunol (2006) 6:541–50. doi: 10.1038/nri1841
74. Delano MJ, Ward PA. Sepsis-induced immune dysfunction: can immune therapies reduce mortality? J Clin Invest (2016) 126:23–31. doi: 10.1172/JCI82224
75. Xu H, Sun Y, Zhang S. The Relationship Between Neutrophil to Lymphocyte Ratio and Clinical Outcome in Pediatric Patients After Cardiopulmonary Bypass Surgery: A Retrospective Study. Front Pediatr (2019) 7:308:308. doi: 10.3389/fped.2019.00308
76. Bhat T, Teli S, Rijal J, Bhat H, Raza M, Khoueiry G, et al. Neutrophil to lymphocyte ratio and cardiovascular diseases: a review. Expert Rev Cardiovasc Ther (2013) 11:55–9. doi: 10.1586/erc.12.159
77. Dell’aquila E, Cremolini C, Zeppola T, Lonardi S, Bergamo F, Masi G, et al. Prognostic and predictive role of neutrophil/lymphocytes ratio in metastatic colorectal cancer: a retrospective analysis of the TRIBE study by GONO. Ann Oncol (2018) 29:924–30. doi: 10.1093/annonc/mdy004
78. Byrnes J, Mckamie W, Swearingen C, Prodhan P, Bhutta A, Jaquiss R, et al. Hemolysis During Cardiac Extracorporeal Membrane Oxygenation: A Case-Control Comparison of Roller Pumps and Centrifugal Pumps in a Pediatric Population. ASAIO J (2011) 57:456–61. doi: 10.1097/MAT.0b013e31822e2475
79. Morgan IS, Codispoti M, Sanger K, Mankad PS. Superiority of centrifugal pump over roller pump in paediatric cardiac surgery: prospective randomised trial. Eur J Cardiothorac Surg (1998) 13:526–32. doi: 10.1016/S1010-7940(98)00067-0
80. Yu K, Long C, Hei F, Li J, Liu J, Ji B, et al. Clinical evaluation of two different extracorporeal membrane oxygenation systems: a single center report. Artif Organs (2011) 35:733–7. doi: 10.1111/j.1525-1594.2010.01173.x
81. Stahl RF, Fisher CA, Kucich U, Weinbaum G, Warsaw DS, Stenach N, et al. Effects of simulated extracorporeal circulation on human leukocyte elastase release, superoxide generation, and procoagulant activity. J Thorac Cardiovasc Surg (1991) 101:230–9. doi: 10.1016/S0022-5223(19)36757-1
82. Obstals F, Vorobii M, Riedel T, De Los Santos Pereira A, Bruns M, Singh S, et al. Improving Hemocompatibility of Membranes for Extracorporeal Membrane Oxygenators by Grafting Nonthrombogenic Polymer Brushes. Macromol Biosci (2018) 18:1700359. doi: 10.1002/mabi.201700359
83. Kawahito S, Maeda T, Yoshikawa M, Takano T, Nonaka K, Linneweber J, et al. Blood trauma induced by clinically accepted oxygenators. ASAIO J (2001) 47:492–5. doi: 10.1097/00002480-200109000-00019
84. Gu YJ, Boonstra PW, Graaff R, Rijnsburger AA, Mungroop H, Van Oeveren W. Pressure drop, shear stress, and activation of leukocytes during cardiopulmonary bypass: a comparison between hollow fiber and flat sheet membrane oxygenators. Artif Organs (2000) 24:43–8. doi: 10.1046/j.1525-1594.2000.06351.x
85. Mitchell MJ, Lin KS, King MR. Fluid shear stress increases neutrophil activation via platelet-activating factor. Biophys J (2014) 106:2243–53. doi: 10.1016/j.bpj.2014.04.001
86. Fukuda S, Yasu T, Predescu DN, Schmid-Schonbein GW. Mechanisms for regulation of fluid shear stress response in circulating leukocytes. Circ Res (2000) 86:E13–8. doi: 10.1161/01.res.86.1.e13
87. Chan CHH, Pieper IL, Robinson CR, Friedmann Y, Kanamarlapudi V, Thornton CA. Shear Stress-Induced Total Blood Trauma in Multiple Species. Artif Organs (2017) 41:934–47. doi: 10.1111/aor.12932
88. Lewis CS, Alsmadi NZ, Snyder TA, Schmidtke DW. Effects of Transient Exposure to High Shear on Neutrophil Rolling Behavior. Cell Mol Bioeng (2018) 11:279–90. doi: 10.1007/s12195-018-0533-z
89. Lehle K, Philipp A, Muller T, Schettler F, Bein T, Schmid C, et al. Flow dynamics of different adult ECMO systems: a clinical evaluation. Artif Organs (2014) 38:391–8. doi: 10.1111/aor.12180
90. Mazzeffi M, Gammie J, Taylor B, Cardillo S, Haldane-Lutterodt N, Amoroso A, et al. Healthcare-Associated Infections in Cardiac Surgery Patients With Prolonged Intensive Care Unit Stay. Ann Thorac Surg (2017) 103:1165–70. doi: 10.1016/j.athoracsur.2016.12.041
91. Koval CE, Stosor V. Ventricular assist device-related infections and solid organ transplantation—Guidelines from the American Society of Transplantation Infectious Diseases Community of Practice. Clin Transplant (2019) 33:e13552. doi: 10.1111/ctr.13552
92. Beshish AG, Bradley JD, Mcdonough KL, Halligan NLN, Mchugh WM, Sturza J, et al. The functional immune response of patients on extracorporeal life support. ASAIO J (2018) 65:77–83. doi: 10.1097/mat.0000000000000748
93. Hoste EA, Bagshaw SM, Bellomo R, Cely CM, Colman R, Cruz DN, et al. Epidemiology of acute kidney injury in critically ill patients: the multinational AKI-EPI study. Intensive Care Med (2015) 41:1411–23. doi: 10.1007/s00134-015-3934-7
94. Verma SK, Molitoris BA. Renal endothelial injury and microvascular dysfunction in acute kidney injury. Semin Nephrol (2015) 35:96–107. doi: 10.1016/j.semnephrol.2015.01.010
95. Hayes D Jr., Preston TJ, Kirkby S, Nicol KK. Human leukocyte antigen sensitization in lung transplant candidates supported by extracorporeal membrane oxygenation. Am J Respir Crit Care Med (2013) 188:627–8. doi: 10.1164/rccm.201303-0428LE
96. Hong BJ, Delaney M, Guynes A, Warner P, Mcmullan DM, Kemna MS, et al. Human leukocyte antigen sensitization in pediatric patients exposed to mechanical circulatory support. Asaio J (2014) 60:317–21. doi: 10.1097/mat.0000000000000053
97. Chan CHH, Pieper IL, Fleming S, Friedmann Y, Foster G, Hawkins K, et al. The Effect of Shear Stress on the Size, Structure, and Function of Human von Willebrand Factor. Artif Organs (2014) 38:741–50. doi: 10.1111/aor.12382
98. Ki KK, Passmore MR, Chan CHH, Malfertheiner MV, Fanning JP, Bouquet M, et al. Low flow rate alters haemostatic parameters in an ex-vivo extracorporeal membrane oxygenation circuit. Intensive Care Med Exp (2019) 7:51. doi: 10.1186/s40635-019-0264-z
99. Heilmann C, Geisen U, Beyersdorf F, Nakamura L, Benk C, Trummer G, et al. Acquired von Willebrand syndrome in patients with extracorporeal life support (ECLS). Intensive Care Med (2012) 38:62–8. doi: 10.1007/s00134-011-2370-6
100. Bredthauer A, Kopfmueller M, Gruber M, Pfaehler S-M, Lehle K, Petermichl W, et al. Therapeutic Anticoagulation with Argatroban and Heparins Reduces Granulocyte Migration: Possible Impact on ECLS-Therapy? Cardiovasc Ther (2020) 2020:9783630. doi: 10.1155/2020/9783630
101. Zen K, Guo YL, Li LM, Bian Z, Zhang CY, Liu Y. Cleavage of the CD11b extracellular domain by the leukocyte serprocidins is critical for neutrophil detachment during chemotaxis. Blood (2011) 117:4885–94. doi: 10.1182/blood-2010-05-287722
102. Harlan JM, Winn RK. Leukocyte-endothelial interactions: clinical trials of anti-adhesion therapy. Crit Care Med (2002) 30:S214–9. doi: 10.1097/00003246-200205001-00007
103. Hall MW, Knatz NL, Vetterly C, Tomarello S, Wewers MD, Volk HD, et al. Immunoparalysis and nosocomial infection in children with multiple organ dysfunction syndrome. Intensive Care Med (2011) 37:525–32. doi: 10.1007/s00134-010-2088-x
104. Zheng H-Y, Zhang M, Yang C-X, Zhang N, Wang X-C, Yang X-P, et al. Elevated exhaustion levels and reduced functional diversity of T cells in peripheral blood may predict severe progression in COVID-19 patients. :Cell Mol Immunol (2020) 17:541–43. doi: 10.1038/s41423-020-0401-3
105. Pinder EM, Rostron AJ, Hellyer TP, Ruchaud-Sparagano M-H, Scott J, Macfarlane JG, et al. Randomised controlled trial of GM-CSF in critically ill patients with impaired neutrophil phagocytosis. Thorax (2018) 73:918–25. doi: 10.1136/thoraxjnl-2017-211323
106. Herbst RS, Eckhardt SG, Kurzrock R, Ebbinghaus S, O’dwyer PJ, Gordon MS, et al. Phase I dose-escalation study of recombinant human Apo2L/TRAIL, a dual proapoptotic receptor agonist, in patients with advanced cancer. J Clin Oncol (2010) 28:2839–46. doi: 10.1200/jco.2009.25.1991
107. Tauber H, Streif W, Fritz J, Ott H, Weigel G, Loacker L, et al. Predicting Transfusion Requirements During Extracorporeal Membrane Oxygenation. J Cardiothorac Vasc Anesth (2016) 30:692–701. doi: 10.1053/j.jvca.2016.01.009
109. Ki KK, Faddy HM, Flower RL, Dean MM. Platelet concentrates modulate myeloid dendritic cell immune responses. Platelets (2017) 29:373–82. doi: 10.1080/09537104.2017.1306045
110. Ki KK, Faddy HM, Flower RL, Dean MM. Packed red blood cell transfusion modulates myeloid dendritic cell activation and inflammatory response. J Interferon Cytokine Res (2018) 38:111–21. doi: 10.1080/09537104.2017.1306045
111. Rodriguez-Gonzalez R, Martin-Barrasa JL, Ramos-Nuez A, Canas-Pedrosa AM, Martinez-Saavedra MT, Garcia-Bello MA, et al. Multiple system organ response induced by hyperoxia in a clinically relevant animal model of sepsis. Shock (2014) 42:148–53. doi: 10.1097/shk.0000000000000189
112. Iwata M, Suzuki S, Asai Y, Inoue T, Takagi K. Involvement of nitric oxide in a rat model of carrageenin-induced pleurisy. Mediators Inflammation (2010) 2010:682879. doi: 10.1155/2010/682879
113. Dal Secco D, Paron JA, De Oliveira SH, Ferreira SH, Silva JS, de Cunha F. Neutrophil migration in inflammation: nitric oxide inhibits rolling, adhesion and induces apoptosis. Nitric Oxide (2003) 9:153–64. doi: 10.1016/j.niox.2003.11.001
114. Carreau A, Kieda C, Grillon C. Nitric oxide modulates the expression of endothelial cell adhesion molecules involved in angiogenesis and leukocyte recruitment. Exp Cell Res (2011) 317:29–41. doi: 10.1016/j.yexcr.2010.08.011
115. James C, Millar J, Horton S, Brizard C, Molesworth C, Butt W. Nitric oxide administration during paediatric cardiopulmonary bypass: a randomised controlled trial. Intensive Care Med (2016) 42:1744–52. doi: 10.1007/s00134-016-4420-6
116. Checchia PA, Bronicki RA, Muenzer JT, Dixon D, Raithel S, Gandhi SK, et al. Nitric oxide delivery during cardiopulmonary bypass reduces postoperative morbidity in children–a randomized trial. J Thorac Cardiovasc Surg (2013) 146:530–6. doi: 10.1016/j.jtcvs.2012.09.100
117. Scholz M, Cinatl J, Barros RT, Lisboa AC, Genevcius CF, Margraf S, et al. First efficacy and safety results with the antibody containing leukocyte inhibition module in cardiac surgery patients with neutrophil hyperactivity. ASAIO J (2005) 51:144–7. doi: 10.1097/01.MAT.0000153646.20861.DE
118. Abdel-Rahman U, Margraf S, Aybek T, Lögters T, Bitu-Moreno J, Francischetti I, et al. Inhibition of neutrophil activity improves cardiac function after cardiopulmonary bypass. J Inflammation (2007) 4:21–1. doi: 10.1186/1476-9255-4-21
Keywords: leukocyte modulation, innate and adaptive immunity, extracorporeal membrane oxygenation technology, critically ill patients, extracorporeal membrane oxygenation
Citation: Ki KK, Millar JE, Langguth D, Passmore MR, McDonald CI, Shekar K, Shankar-Hari M, Cho HJ, Suen JY and Fraser JF (2021) Current Understanding of Leukocyte Phenotypic and Functional Modulation During Extracorporeal Membrane Oxygenation: A Narrative Review. Front. Immunol. 11:600684. doi: 10.3389/fimmu.2020.600684
Received: 17 September 2020; Accepted: 23 November 2020;
Published: 08 January 2021.
Edited by:
Jean-Marc Cavaillon, Institut Pasteur, FranceReviewed by:
Charles-Edouard Luyt, Hôpitaux Universitaires Pitié Salpêtrière, FranceKarla Lehle, University Hospital Regensburg, Germany
Copyright © 2021 Ki, Millar, Langguth, Passmore, McDonald, Shekar, Shankar-Hari, Cho, Suen and Fraser. This is an open-access article distributed under the terms of the Creative Commons Attribution License (CC BY). The use, distribution or reproduction in other forums is permitted, provided the original author(s) and the copyright owner(s) are credited and that the original publication in this journal is cited, in accordance with accepted academic practice. No use, distribution or reproduction is permitted which does not comply with these terms.
*Correspondence: Katrina K. Ki, ay5raUB1cS5lZHUuYXU=; Jacky Y. Suen, ai5zdWVuMUB1cS5lZHUuYXU=
†These authors have contributed equally to this work and share last authorship