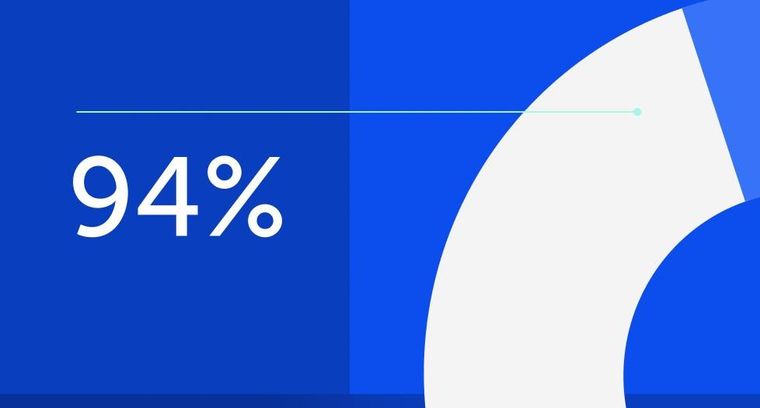
94% of researchers rate our articles as excellent or good
Learn more about the work of our research integrity team to safeguard the quality of each article we publish.
Find out more
REVIEW article
Front. Immunol., 21 January 2021
Sec. Immunological Memory
Volume 11 - 2020 | https://doi.org/10.3389/fimmu.2020.595297
This article is part of the Research TopicImmunity in Infancy and Impact on Immediate and Long-Term HealthView all 16 articles
Infants are capable of mounting adaptive immune responses, but their ability to develop long-lasting immunity is limited. Understanding the particularities of the neonatal adaptive immune system is therefore critical to guide the design of immune-based interventions, including vaccines, in early life. In this review, we present a thorough summary of T cell, B cell, and humoral immunity in early life and discuss infant adaptive immune responses to pathogens and vaccines. We focus on the differences between T and B cell responses in early life and adulthood, which hinder the generation of long-lasting adaptive immune responses in infancy. We discuss how knowledge of early life adaptive immunity can be applied when developing vaccine strategies for this unique period of immune development. In particular, we emphasize the use of novel vaccine adjuvants and optimization of infant vaccine schedules. We also propose integrating maternal and infant immunization strategies to ensure optimal neonatal protection through passive maternal antibody transfer while avoiding hindering infant vaccine responses. Our review highlights that the infant adaptive immune system is functionally distinct and uniquely regulated compared to later life and that these particularities should be considered when designing interventions to promote pediatric health.
Despite tremendous progress in recent decades, infectious diseases remain a leading cause of morbidity and mortality in pediatric populations worldwide (1–3). Infectious diseases cause nearly 25% of deaths in the neonatal period (from birth to one month of age) and up to one third of deaths in children under 5 years of age (1–3). Vaccines are one of the most cost-effective interventions to address the global burden of pediatric infectious diseases, and the implementation of early life immunizations has reduced deaths in neonates and children across the world (4). Due to differences in the early life and adult immune systems, it is increasingly appreciated that an in-depth understanding of early life immunity is crucial for the development of effective pediatric vaccines and for optimizing pediatric vaccine schedules.
While recent reviews have outlined differences between the infant and adult immune system, they focus on innate immunity given its key role in defense in early life [reviewed in (5–7)]. Because vaccine-induced protection largely depends on acquired immunity, we have instead focused this review on early life adaptive immunity. Herein, we present a detailed overview of T and B cell development as well as the unique factors regulating early life adaptive immunity. We review T cell and humoral responses to pathogens and vaccines in early life, which reveal that adaptive immune responses can be generated in infancy but that these are generally attenuated compared to later in life. We also discuss how passive maternal antibody transfer impacts early life adaptive immunity and may be harnessed to protect neonates. Our review focuses on human studies of early life adaptive immunity, yet we have also integrated evidence from animal models where appropriate (see Box 1). Our goal is that this review can inform the rational design of vaccines and other immune-based interventions to combat pediatric infectious diseases. Understanding early life immunity is particularly critical for vaccine development since vaccines and adjuvants traditionally have not been tailored to engage the neonatal or infant adaptive immune response. Therefore, applying knowledge of early life adaptive immunity in vaccine development can substantially improve the efficacy and impact of pediatric vaccines.
Box 1. Key considerations for studies of early life immunity. Many studies of early life immunity use neonatal mice or human umbilical cord blood, yet these model systems have important caveats: 1) murine and human immune cell ontogeny differ substantially, especially regarding T cell development [(8, 9) reviewed in (10–12)], 2) immune responses measured in umbilical cord blood are distinct from those detected during prenatal and postnatal life (13), and 3) the milieu of cytokines, corticosteroids, and metabolites generated during labor can significantly modulate immune responses detected in cord blood [reviewed in (14, 15)]. While the use of model systems is critical to complement human studies, it is important to consider possible limitations when interpreting different studies of early life immunity.
The T cell compartment in early life is uniquely positioned to respond to diverse immunological demands, such as balancing immunotolerance in utero and during microbial colonization with defense against pathogens (12). These divergent demands result in highly stimulus dependent T cell responses in early life (Figure 1). Human T cell development begins in utero, as the thymus starts producing T cells at 12–14 weeks of gestation, and the neonatal T cell receptor (TCR) repertoire is diversified by 26 weeks of gestation (16–19). Distinct waves of human T cell development occur in utero, yet the T cell compartment continues to evolve dynamically after birth. In contrast to other immune cell compartments (e.g., B, natural killer, and dendritic cells) that converge with adult immunophenotypes by 3 months of age, infant and adult T cell compartments remain phenotypically distinct at least for the first 2 years of life (13, 20).
Figure 1 Summary of the T cell compartment in early life. This overview of the infant T cell compartment summarizes evidence from human studies unless otherwise stated. Infants rely on “innate-like” CD4+ and CD8+ T cells, which are more likely to be RTEs and to signal through innate immune pathways such as complement receptor and TLR signaling, promote inflammation, and respond with antimicrobial peptides rather than classic antigen-specific adaptive immune responses. T helper type 1 (Th1) cells are generated in response to certain pathogens and vaccines in early life; however, epigenetic regulation of cytokine loci may limit these responses. T helper type 2 (Th2) cells are generated in response to allergens and common environmental antigens encountered in early life and only rarely in response to pathogens or vaccines. Regulatory T cells (Tregs) maintain fetal immunotolerance in utero and are present in higher numbers and proportions in early life blood and peripheral tissues, which may aid in promoting tolerance to microbial colonization but may impair mucosal and systemic T cell activation and adaptive immune responses. T follicular helper cell (TFH) responses are impaired in neonatal murine models but have not been studied extensively in human neonates. The restricted function of TFH cells in early life may be due to immaturity in co-activation signals required for B and T cell crosstalk and may impair generation of germinal center responses. *data shown in mice. RTEs, recent thymic emigrants; CMV, cytomegalovirus; RSV, respiratory syncytial virus; P. falciparum, plasmodium falciparum; T. cruzi, Trypanosoma cruzi.
Infants have high inter-individual variability in CD4+ and CD8+ subsets [i.e., proportion of naïve, central memory (TCM), effector memory (TEM), and tissue-resident effector memory T cells (TEMRA)], yet overall the neonatal T cell compartment is dominated by naïve T cells (13). The majority of these naïve T cells are recent thymic emigrants (RTEs), which harbor unique effector functions (18, 21–23). RTEs are biased towards innate-like immune signaling and preferentially differentiate into induced regulatory T cells (iTregs), which limits their role in adaptive T cell responses (22, 24). Despite the predominance of naïve T cells, TEM are present in cord blood even in the absence of intrauterine infection, suggesting that T cell memory is generated during normal fetal development (25, 26).
T cells from pediatric organ donors (2 months to 2 years of age) are also more likely to be naïve and RTEs compared to T cells from adults (20). This increased proportion of naïve T cells and RTEs has been observed across T cell compartments in blood, lymphoid tissue, lung, and the intestine (20). In general, infants have fewer TEM cells compared to adults except in the lung and small intestine where proportions are comparable (20). Given the high burden of respiratory and diarrheal diseases in infants, it is interesting to note that effector T cell memory may be relatively enhanced in the lung and small intestine in early life compared to other tissues; however, the functional capabilities of these TEM populations are largely unknown. Moreover, the increased ratio of Tregs : TEM cells in these tissues may hinder the ability to mount an effector-memory response (20). Thus, while TEM responses are generated in utero and present in mucosal tissues (i.e., lung and small intestine) in early life, it is unclear how effective they are in mounting adaptive responses. Overall, the relative enrichment of antigenically naïve T cells and RTEs compared to memory and effector T cells likely contributes to blunted adaptive T cell responses in infancy.
In addition to phenotypic differences, adult and infant T cells have distinct functional responses. Notably, cord and neonatal peripheral blood contain fewer IFN-γ and IL-17A producing but significantly more CXCL-8 producing T cells compared to adult blood (23, 27, 28). CXCL-8 is an innate immune effector, and CXCL-8 producing T cells can co-express complement receptors (CR), which also function in innate immune cell signaling (23). These CXCL-8 producing T cells are enriched in the neonatal T cell compartment, more likely to be RTEs, and may act as innate-like precursors to the classic adaptive proinflammatory IFN-γ producing T cells (22).
Over the first 3 months of life, CXCL-8 plasma concentrations decrease whereas IL-17A concentrations increase, reflecting a shift from innate-like to canonical adaptive cytokine production (13). However, T cells from older children (aged 5–16 years) still show decreased proinflammatory cytokine secretion (e.g., IFN-γ and IL-17A) compared to adults following ex vivo stimulation (29). These differences in early life cytokine production highlight the importance of CXCL-8 and suggest that neonatal T cells respond preferentially with innate rather than adaptive responses favored in adulthood (Figure 1). This skewing of the early life T cell compartment towards innate-like responses may need to be overcome in order to elicit durable adaptive T cell responses in infancy.
Cell-intrinsic (i.e., transcriptional, epigenetic) and cell-extrinsic (i.e., cytokines, cell-to-cell communication) mechanisms that regulate T cell responses are distinct in early life compared to adulthood. For instance, fetal and neonatal T cells have a transcriptional landscape that favors tolerogenic and innate-like cytokine production over proinflammatory T cell responses (30). Moreover, when comparing transcriptional pathways in preterm, term, and 3 month old infants, younger neonates exhibit a downregulation of IFN-γ production and T cell proliferation, and upregulation of IL-10 and CXCL-8 biosynthesis (13). These results suggest that neonatal T cells are biased towards innate immune signaling and immunosuppression, as IL-10 promotes Treg differentiation and suppresses T cell activation. Transcriptional differences between naïve CD8+ T cells from cord and adult blood further supports the hypothesis that T cells in early life harbor a gene expression program favoring innate over adaptive T cell functions (i.e., antigen recognition) (31). Naïve CD8+ T cells from cord blood upregulate innate immune genes in toll-like receptor (TLR) signaling, inflammation, and antimicrobial peptides whereas those from adults highly express cell cytotoxicity and TCR-signaling genes (31). Mechanistic work in mouse models further supports the notion that early life T cells employ transcriptional programs to promote rapid innate immune responses over antigen-specific memory responses (32, 33).
Neonatal T cells also have a distinct epigenetic landscape compared to adult T cells, as reflected by differences in DNA methylation, chromatin, histone modifications, and micro RNA (miRNA) expression [reviewed in (12)]. In fetal CD4+ T cells, IL-2 and IFN-γ production is blunted by expression of the transcription factor Helios (34). Moreover, in cord blood, the IFN-γ promoter of naïve CD4+ T cells is heavily methylated, which correlates with lower levels of IFN-γ secretion (35). Chromatin accessibility at other cytokine loci (e.g., IL-12 and IL-13) in neonatal T and dendritic cells differs from adults, suggesting that chromatin remodeling occurs during the transition from neonatal to adult T cell phenotypes (36, 37). Epigenetic differences in chromatin, histone modifications, and miRNA expression in cord versus adult T cells likely drive differences in gene expression and function [(31, 38–40), reviewed in (12)]. Most of these findings are from cord blood and mouse studies, thus additional studies are needed to understand the transcriptional and epigenetic mechanisms governing T cells postnatally. Such work may reveal novel therapeutic targets for modulating infant T cells to promote long-lasting adaptive responses.
Mounting evidence suggests that a strong bias towards Treg development in early life tempers adaptive immune responses. Fetal immunotolerance in utero is essential and is maintained by immunosuppressive Tregs, which hamper proinflammatory T cell activity and promote tolerance to the maternal host [reviewed in (41, 42)]. Tregs are enriched in fetal lymphoid tissues and they suppress non-Treg T cell proliferation, activation, and IFN-γ production (30, 43). Moreover, expression of Helios in fetal naïve CD4+ T cells contributes to an epigenetic predisposition to Treg differentiation (34). Both fetal, and to a lesser extent, neonatal CD4+ T cells are poised to differentiate into Tregs upon TCR engagement (30, 40, 44).
Treg persistence postnatally and their role in modulating adaptive immune responses in infancy is unclear. During fetal development, the proportion of Tregs declines with gestational age from 15–20% in the second trimester to ~5% of total CD4+ T cells in cord blood, which is comparable to adult blood (44, 45). However, recent work has demonstrated that Tregs are a highly enriched and compartmentalized T cell subset in early postnatal life (20). When comparing T cell compartments from pediatric (2 months to 2 years of age) to adult organ donors, Thome et al. found that Tregs accounted for 10-20% of total CD4+ T cells in pediatric blood, lung, intestine, and lymphoid tissues versus only ~5% in adult donor tissues (20). This predominance of Tregs was directly correlated with age, as highlighted by decreasing proportions of Tregs over time. The importance of Tregs in modulating infant immune responses across diverse sites (e.g., blood, lymphoid, and mucosal tissues) has been underexplored. Tregs likely regulate appropriate local mucosal immune responses during microbial colonization, as Tregs may protect against necrotizing enterocolitis in preterm infants by promoting tolerance during newborn gut microbial colonization (46). Because the immunosuppressive effects of these Tregs may increase infectious disease susceptibility and could dampen vaccine responsiveness in infancy, further research is crucial to gain a deeper understanding of their abundance and function in early life.
It is generally believed that CD4+ T cells are biased towards T helper type 2 (Th2) differentiation in early life [reviewed in (11, 47, 48)]. This tendency towards Th2 differentiation was first observed in neonatal mice, but the evidence for Th2 bias in human neonates is less clear. Naïve CD4+ T cells can differentiate into Th subsets including Th1, Th2, and Th17 cells, which produce IFN-γ, IL-4/IL-5/IL-13, and IL-17/IL-22, respectively. Th1 cells are proinflammatory and defend against intracellular pathogens (e.g., viruses), whereas Th2 cells protect against parasitic infections and have been implicated in atopic diseases (e.g., allergy and asthma), and Th17 cells protect against extracellular pathogens (Figure 1). While neonatal innate immune cells produce more Th2 than Th1-polarizing cytokines (49, 50), Th responses in early life appear to be highly stimulus dependent.
Recently, a population of naïve CD4+ T cells has been identified in cord blood and infant adenoids that highly express IL-4 when stimulated under multiple conditions (e.g., anti-CD3/anti-CD28, PMA/Ionomycin, TGF-β). However, IFN-γ is also expressed by these T cells under some conditions (e.g., PMA/Ionomycin, IL-1β, IL-12), indicating that these cells generate both Th1 and Th2 type responses (51). Additional evidence that the stimuli and cytokine milieu strongly dictate early life Th differentiation is apparent from studies of neonatal T cell responses to allergen. T cells isolated from cord blood produce Th2 type cytokines when stimulated by environmental allergens ex vivo (52). Similarly, T cells from infants (<2 years of age) stimulated with allergens favor Th2 over Th1 responses (53). Infants (<3 months of age) infected with RSV also generate high levels of IL-4 and no detectable IFN-γ, suggesting a Th2 biased response (54). RSV also elicits a Th2 type response in neonatal mice, and early life RSV infections in humans have been implicated in the development of atopy and asthma [(55) reviewed in (56)]. Thus, Th2 responses to RSV infection may be pathogen-specific and reflective of a complex interplay with Th2-mediated disease risk. Overall, it is clear that infants mount Th2 responses against allergens and RSV in early life but less apparent whether this represents a global Th2 bias.
Infants (<3 months of age) infected with influenza or parainfluenza virus generate IFN-γ and IL-4/IL-5, suggesting they mount both Th1 and Th2 responses (54). Moreover, a prospective analysis of Th1 and Th2 cytokine levels in newborns followed until 3 months of age did not show Th2 skewing (57). T cells from lymph nodes of pediatric organ donors (<2 years of age) also do not demonstrate a Th2 bias after stimulation (20). Importantly, it is unclear if Th responses studied ex vivo recapitulate in vivo function. However, both in vivo and ex vivo evidence suggests that the stimulation (e.g., allergen, pathogen, TCR/co-stimulation, mitogen) and cytokine milieu (e.g., strongly Th-polarizing, proinflammatory, anti-inflammatory) largely dictate Th responses in early life (Figure 1).
Studies of fetal and neonatal immune responses to infections have greatly advanced our understanding of the functional capabilities of T cells in early life [reviewed in (58)]. Fetuses mount pathogen-specific CD8+ and CD4+ T cell responses against human immunodeficiency virus (HIV), cytomegalovirus (CMV), Trypanosoma cruzi, and Plasmodium falciparum [(59–63) reviewed in (64–66)]. Fetuses exposed to P. falciparum and T. cruzi generate CD4+ T cell responses that release proinflammatory cytokines when re-stimulated, highlighting that adaptive T cell memory is elicited in utero (62, 67). Moreover, infants exposed to P. falciparum in utero produce antigen-specific CD4+ and CD8+ T cell responses that undergo memory differentiation (63). These P. falciparum specific CD4+ T cell responses in cord blood correlate with protection against malaria infection in the first 2 years of life, suggesting that priming of pathogen-specific CD4+ T cell responses in utero may confer protection later in life (63).
Infectious exposures can impact the developing T cell compartment broadly in addition to eliciting pathogen-specific T cell responses. Congenital CMV infection causes widespread immune activation and differentiation of the developing T cell compartment [(68, 69), reviewed in (65)]. Moreover, infants exposed to pathogens in utero but not infected (e.g., born to mothers infected with P. falciparum, T. cruzi, HIV, or hepatitis C virus) have global changes in their T cell immunophenotypes and altered T cell responses to stimulation [(70), reviewed in (71)]. These exposed yet uninfected infants can have reduced T cell responses to vaccines and increased susceptibility to homologous and heterologous infections, perhaps due to inappropriate tolerogenic T cell responses generated in utero (71). Thus, prenatal exposure to pathogens can broadly shape the neonatal T cell compartment with long-term consequences for adaptive immune responses, which should be considered when designing vaccine strategies.
Although capable of generating antigen-specific T cell memory, infant T cell responses are limited compared to adults. Infants have impaired CD4+ T cell responses to P. vivax (72), HIV (73), and CMV (74), which may contribute to poor pathogen control. Furthermore, neonates with viral respiratory tract infections (e.g., RSV, influenza, and coronaviruses) preferentially generate TEM rather than tissue-resident memory T cells (TRM), which may impair the development of long-lasting memory (75). Given the ongoing outbreak of SARS-CoV-2, a newly emergent coronavirus and ongoing infectious threat to pediatric health, it is important to consider how T cell responses may be age-dependent with distinct implications for disease pathogenesis and vaccine interventions [reviewed in (76)]. These studies suggest that antigen-specific T cell responses are generated in response to certain pathogens, yet effector T cell responses may be favored over memory T cell responses during early life.
Infant T cell responses to vaccines have also demonstrated that the T cell compartment is equipped to mount pathogen-specific adaptive responses in early life, but suggests that these are usually diminished compared to adulthood. Infants generate T cell responses following measles vaccination, yet immunization before 6 months of age produce less IFN-γ than adults (77, 78). Moreover, infants receiving the hepatitis B (HepB) vaccine have impaired T cell cytokine production and differentiation compared adults (79). Although the T cell responses to the measles and HepB vaccines are attenuated in infancy compared to adulthood, there is also evidence that infants can mount more adult-like responses to some vaccines.
Infants receiving the Bacillus Calmette–Guérin (BCG) vaccine against Mycobacterium tuberculosis have more robust Th1 and pathogen-specific CD8+ T cell responses than adults (80–82). Moreover, priming infants with the BCG vaccine in infancy appears to boost T cell responses to heterologous vaccines, including HepB and oral poliovirus later in life [(83), reviewed in (84)]. Bordetella pertussis vaccination also induces a potent Th1 response in young infants and can enhance overall T cell activation (85). Thus, infants can mount vigorous T cell responses following some vaccines despite inherent maturational differences in the early life T cell compartment. Therefore, targeted intervention strategies that account for the distinct nature of the neonatal T cell compartment should be employed to effectively engage T cells in adaptive responses.
Immunoglobulins (Ig) i.e., antibodies, encoded by the B cell receptor (BCR) genes, are the key mediators of adaptive humoral immunity and are composed of different isotypes with distinct functions (e.g., IgM, IgG, IgA, and IgE). While fetal IgM production begins in utero and increases substantially postnatally, endogenous IgG and IgA production, which requires B cell class-switching, remains limited until 6 months of age (86). In order to respond to pathogens, the mammalian adaptive immune system has evolved multiple mechanisms to recognize diverse antigens. BCR combinatorial diversity is achieved when different genes segments in V(D)J loci are recombined to form the V region and junctional diversity is formed when the enzyme terminal deoxynucleotidyl transferase (TdT) adds random N nucleotides at the joints between V(D)J gene segments during DNA recombination. These two sources of diversity can generate, approximately 1011 different receptors in the naïve BCR repertoire (87), and more diversity is added when different heavy- and light-chain V regions pair to form the antigen-binding site. Once a BCR recognizes its cognate antigen, somatic hypermutation (SHM) occurs in germinal centers and mutations are introduced to the antibody V regions to improve binding to antigens. Although fetal mature B cells can be detected as early as 9 weeks of gestation, the formation of germinal centers and adaptive humoral responses is greatly attenuated until after birth (10).
By detecting the products of V(D)J DNA recombination generated during B cell development, Rechavi et al. showed that BCR combinatorial diversity begins around 12 weeks of gestation (19). At birth, infants have low levels of SHM, but over time, SHM rates increase. By 2 years of age, SHM rates in non-class-switched IgM and IgD-expressing B cells reach adult levels. However, by 3 years of age, class-switched IgG and IgA-producing B cells only achieve 60 to 75% of adult SHM levels and only reach adult levels at 6 years of age (88, 89). The lower rates of SHM in infants can lead to reduced antibody binding to the antigen. Less differentiated CD27dull memory B cells (which are mostly IgM-expressing) predominate in the infant B cell compartment; the more differentiated CD27bright memory B cells are absent until 3–4 years of age (90). In fact, these CD27dull memory B cells seem to be the progenitor of CD27bright memory B cells, and thus are relatively enriched in early life compared to later in life (90). Functionally, CD27bright memory B cell populations have higher SHM rates, contain more class-switched memory B cells, and generate more potent response to antigens. Differences between adult and infant B cells have also been reported in the Ig complementarity-determining region 3 (CDR3), which is critical for antigen binding. The Ig heavy chain CDR3 (HCDR3) is significantly shorter in fetuses due to fewer N nucleotides at the VDJ junctions compared to term infants, which could be due to lower TdT expression in fetal life (19). HCDR3 maturation is initiated in the third trimester of pregnancy, but HCDR3 lengths do not reach adult levels until 5 months of age (91). Overall, the composition of the memory B cell compartment, limited SHM rates, and shorter HCDR3 lengths during B cell development highlight why infants often have less potent humoral responses to antigens.
Overall, early life B cell responses to T cell-dependent (TD) and T cell-independent (TI) antigens are weaker than in adulthood except for a few pathogens and vaccines discussed later (86). As summarized in Figure 2, multiple factors contribute to the diminished antibody response in infancy, including cell-intrinsic and cell-extrinsic factors. The gene expression profile of neonatal B cells is distinct from adult B cells, which limits the activation signals neonatal B cells receive from CD4+ T cells upon exposure to TD antigens. Specifically, human neonatal B cells express lower levels of the co-stimulatory receptors CD40, CD80, and CD84, resulting in dampened responses to CD40 ligand (CD40L) expressed by T cells (86).
Figure 2 Summary of the B cell compartment and antibody responses in early life. Dampened germinal center reaction is generally observed in infants, and differentiation of activated B cells is skewed toward short-lived memory B cells over plasma cells. Bone marrow in early life is unable to provide an optimal niche for the differentiation of long-lasting plasma cells. Transplacental maternal antibodies influence the neonatal B cell-mediated response by the illustrated mechanisms. *data shown in mice. FDC, follicular dendritic cell.
To elicit a potent and durable antibody response, three key structures are involved (Figure 2) including: 1) the follicular dendritic cell (FDC) network, 2) germinal centers, and 3) the bone marrow. FDCs capture and retain antigens in immune complexes with complement or antibodies (92). By presenting native antigens to B cells, FDCs play an important role in the nucleation of germinal centers. However, in neonatal mice, FDC precursors fail to differentiate into mature FDCs following B cell-mediated signaling, leading to the absence of effective germinal center responses in neonatal mice until 3 weeks of age (93). Despite no direct evidence in humans, the lack of mature germinal centers in mouse models suggests that immature interactions between FDC and B cells may contribute to the attenuated B cell and humoral responses in early life (94). Another mechanism that may limit early life humoral responses could be the lower expression levels of CD73 in neonatal naïve B cells, as CD73 converts adenosine mono-phosphate into adenosine, an essential immunoregulatory molecule (95). Lower CD73 expression in B cells has also been observed in patients with common variable immunodeficiency, which have limited antibody production, suggesting that decreased CD73 expression in infant B cells may limit humoral responses (96).
Infants generally have lower magnitude and limited persistence of antibody responses following natural infection and vaccination (97, 98), which may result from the inability of early-life bone marrow to establish a pool of long-lived plasma cells. In adults, plasmablasts migrate to the bone marrow after exiting germinal centers, and, with survival signaling from the stromal cells, then differentiate into long-lived plasma cells. However, due to insufficient signals from immature stromal cells, plasmablasts in neonatal mice fail to differentiate into long-lived plasma cells in the bone marrow (98, 99). Furthermore, upon antigen exposure, both human and mouse neonatal B cells show preferential differentiation toward memory B cells instead of long-lived plasma cells (86), possibly due to lower expression of CD40 and CD21 in infant B cells (100, 101).
TI antigens include repetitive, highly valent structures such as polysaccharides, thus B cell responses to TI antigens are key for host defense against encapsulated bacteria. Humans do not develop humoral responses to TI polysaccharides until 1–2 years of age, which is further supported by mouse studies showing that mice (<3 weeks of age) do not respond to immunization with pure pneumococcal polysaccharides (102–104). Due to this impaired TI humoral response, neonates are more susceptible to infections with encapsulated bacteria such as Haemophilus influenzae type b (Hib) and Streptoccoccus pneumoniae. Neonatal hyporesponsiveness to TI antigens may be due to immaturity of marginal zone B cells and lower numbers of CD27+ memory B cells in early life, as splenic marginal zone B cells are the major subpopulation responding to TI antigens (105). Lower B cell expression of the C3 complement receptor 2 (CR2 or CD21) and decreased levels of C3 complement may further impair humoral responses to TI antigens in early life (105–108). CD21 aids B cell signaling by ligating C3 fragments that opsonize bacterial polysaccharide capsules, so reduced C3 and CD21 levels may render neonatal B cells less sensitive to TI antigens (109). To overcome this limitation of the early life immune system, pediatric vaccines against encapsulated pathogens are conjugated to proteins to recruit T cell help and improve humoral vaccine responses (110–112). Therefore, factors limiting humoral responses to both TD and TI antigens in early life and the nature of the antigen must be considered when designing pediatric vaccines.
Early life antibody responses can be substantially shaped by pathogens, maternal antibodies, and microbial colonization (88, 113–115). The factors most unique to early life that shape humoral responses include maternally transferred antibodies and colonization of the newborn gut microbiota. Due to the limitations to generating antibody responses in early life, neonates rely heavily on passive maternal antibody transfer for protection against infections [reviewed in (116)]. Transplacental IgG transfer, mediated by the neonatal Fc receptor (FcRn), begins during the second trimester and increases throughout gestation (117). Maternal IgG persists in infant circulation for months postnatally, and the importance of maternal antibodies in protecting neonates is underscored by diseases in which transplacental antibody transfer is disrupted. For instance, maternal HIV infection can impair transplacental antibody transfer [reviewed in (118)], which may increase the susceptibility of HIV-exposed yet uninfected infants to heterologous infections (119–122). Passive maternal antibody transfer of mostly secretory IgA (sIgA) and some IgG postnatally through breastmilk also provides protection of the neonate particularly at mucosal surfaces.
Despite the important protection conferred by maternal antibodies, the presence of maternal antibodies can also dampen B cell responses to vaccines in human neonates [reviewed in (123)]. Mechanistic studies on maternal antibody-mediated interference in humans have been lacking, yet these mechanisms have been explored in rodent models [reviewed in (123, 124)]. Proposed mechanisms of interference include: 1) live viral vaccine neutralization, 2) epitope masking, 3) clearance of immune complex through Fc-dependent phagocytosis, 4) binding of inhibitory Fc receptor FcγRIIB, 5) inhibition of the differentiation of plasma cell and memory B cell differentiation (114, 125–127) (Figure 2). Maternal antibodies can neutralize live vaccines such as the measles and polio vaccines, which may contribute to the low antibody responses observed in human neonates (124). Maternal antibodies may also inhibit the activation of B cell clones by blocking immunodominant epitopes, which could explain the attenuated neonatal antibody response to vaccines that do not involve live virus (e.g., inactivated and subunit vaccines) (124). Moreover, opsonization by maternal antibodies may facilitate the clearance of vaccine antigen, limiting its availability for infant B cell recognition. Kim et al. also demonstrated in cotton rats that vaccine-specific maternal antibodies may inhibit neonatal B cell activation by crosslinking inhibitory FcγRIIB receptors on B cells (127). Vono et al. demonstrated that high titers of antigen-specific maternal antibodies inhibited the antibody response to autologous immunization in the offspring of vaccinated dams by preventing germinal center B cells from differentiating into plasma and memory B cells, though the formation of germinal centers was not affected (114). Another study of neonatal mice also demonstrated that high but not low or moderate antigen-specific maternal antibody levels hindered pup antibody responses to pneumococcal immunization (128). Thus, maternal antibodies may impact neonatal B cell immunity in many complex ways, perhaps more profoundly than previously appreciated. Understanding the mechanisms regulating maternal antibody transfer and interference with neonatal humoral responses is key for the development of vaccines leveraging passive maternal immunization and for designing pediatric vaccine schedules.
The newborn gut microbiome is primarily colonized shortly after birth, yet a stable, adult-like community is not established until around 3 years of age (129). The microbiome can substantially modulate the host antibody repertoire and responses to infections and vaccines in early life [reviewed in (113)]. Germ-free mice have limited sIgA production, as evidence by smaller Peyer’s patches, lower numbers of CD4+ T cells and IgA-producing plasma cells (130), and elevated levels of serum IgE and anaphylaxis (129). Notably, this phenotype can be reversed by introducing commensal microbes before two weeks of age but not after, implying that microbial colonization must be achieved within a narrow perinatal period known as the “window of opportunity” (129–132). However, more studies into how the gut microbiome influences human neonatal humoral responses are needed. Due to differences in microbial colonization, infants and adults can mount distinct antibody response to pathogens. A bias towards HIV envelope (i.e., gp41) specific antibodies that cross react with commensal bacteria has been observed in HIV infected and uninfected adults, but gp41 dominant antibody responses are not observed during pediatric HIV infection (133, 134). This highlights how immune imprinting by the microbiome can modulate antibody specificities and that targeting the microbiome could be a novel strategy for engineering humoral responses to vaccination in early life.
Despite the attenuated humoral responses in early life to encapsulated bacteria such as Hib and Streptococcus pneumoniae, infants and young children are able mount robust antibody responses to some pathogens. For instance, infants generate antibodies that neutralize diverse HIV strains by targeting conserved epitopes (known as broadly neutralizing antibodies, bnAbs). BnAbs develop in a small proportion (10–25%) of HIV-infected adults after years of infection, yet studies have revealed that bnAbs can be detected within 1 year of infection in HIV-infected infants (135, 136). Unlike in HIV-infected adults where plasma neutralization breadth is usually mediated by antibodies against one or two specificities, a high portion of children (63%) develop polyclonal broadly neutralizing antibody responses against HIV (137). Adult bnAbs often have unusual characteristics such as high SHM rates, which may contribute to their delayed development in adults (133). Strikingly, high levels of SHM seem to be less important in pediatric bnAbs as two bnAbs, BF520.1 and AIIMS-P01, isolated from HIV-infected children demonstrate neutralizing potency comparable to adult bnAbs but have much lower SHM rates (6.6 and 7% versus 15.8 to 23.1%) (138, 139). Wiehe et al. compared the number of rare i.e., improbable mutations required for different antibody lineages to acquire heterologous virus neutralization capacity and showed that BF520.1 had fewer improbable mutations than adult bnAbs; however, an improbable mutation, N52A, was essential for neutralization potency, highlighting that such mutation events may be important for generating bnAbs in HIV-infected infants (140). These studies on HIV bnAbs highlight that the developmental pathways for bnAbs in infants may differ from adults and young children may even be better at generating bnAbs (136).
Potently neutralizing antibodies with limited rates of SHM have been observed in RSV infections in early life as well. Goodwin et al. analyzed antibodies isolated from memory B cells of infants (<3 months of age) hospitalized for RSV infection and observed that the majority of the RSV-specific antibodies did not have detectable SHM. Yet, antibodies from each of the infants demonstrated neutralizing activity against RSV and 4 out of 5 had highly potent neutralizing activity (141). Although the authors did not test whether these antibodies prevented RSV reinfection, this study demonstrated that, even with limited levels of SHM, antibodies generated in early life may still have substantial anti-pathogenic i.e., neutralizing capabilities.
Due to immaturity, fewer co-stimulatory receptors, lower SHM rates, limited class-switching, and maternal antibody interference, infant humoral responses to vaccination are often attenuated compared to adulthood. Neonatal immunizations often result in low-titer responses, reduced durability, and poor seroconversion rates. An ideal neonatal vaccine should induce a robust and durable immune response with a single dose at birth, thereby minimizing vulnerability to infections. Yet, only 50% of infants generate a weak neutralizing antibody titer following one dose of the oral polio vaccine, and infants have limited responses to one dose of the diphtheria, pertussis, tetanus (DTaP) and Hib vaccines [reviewed in (124)]. Overall, vaccine-induced antibodies wane to low or undetectable levels 6 to 9 months after the first dose in most infants, Thus, except for the BCG and HepB vaccines, most pediatric vaccines are administered after 2 months of age in a series of booster doses including: the rotavirus vaccine (2 and 4 months), DTaP vaccine (2, 4, 6, and 15–18 months), Hib conjugate vaccine (2, 4, 6, 12–15 months), pneumococcal conjugate vaccine (2, 4, 6, 12–15 months), polio vaccine (2, 4, 6–18 months), seasonal influenza vaccine (>6 months), measles, mumps, and rubella vaccine (12 months), varicella vaccine (12 months), and hepatitis A vaccine (12–18 months) [reviewed in (142)].
Notably, newborns can mount adult-like antibody responses following HepB vaccination (79). This highlights that certain stimuli may be better able to induce a protective immune response via vaccination in early life. In fact, when comparing adult and infant responses to HIV vaccines, we observed that children immunized with an oil-in-water emulsion adjuvant (MF59) developed higher antibody titers than adults immunized with the same vaccine, yet no difference was observed between adults and infants when immunized with an Alum-adjuvanted vaccine (143). These results suggest that the choice of the vaccine adjuvant can significantly improve antibody responses early in life, as discussed below.
Given the particularities of early life adaptive immunity, vaccination strategies must be specifically tailored to this stage of immune development [reviewed in (144–146)]. As discussed, early life T cell responses are limited due to differences favoring innate and effector over adaptive memory responses, immunotolerance, and an enrichment of RTEs and Tregs. Moreover, the ability to generate long-lived plasma B cells and antibody responses in early life is limited due to immaturity, maternal antibody interference, poor germinal center formation, and reduced T and B cell crosstalk. Work in mouse models suggests that T follicular helper cell (TFH) interactions with germinal center B cells are impaired in neonatal immunization [(147–149) reviewed in (145)]; however, studies on early life germinal center responses and TFH cells in humans are lacking [reviewed in (146)].
A promising approach to improve adaptive immune responses to vaccines in early life is through the informed choice of vaccine adjuvants and novel adjuvant combinations, which can stimulate both adaptive immune cells and recruit innate antigen-presenting cells [reviewed in (150)]. For instance, the vaccine adjuvant MF59 elicited robust antigen-specific T cell responses and prevented disease acquisition in infants vaccinated against influenza (151, 152). Studies in neonatal mice suggest that MF59 may improve effecter CD4+ T cell but not TFH responses, though MF59 may enhance germinal center responses compared to Alum (153, 154). Moreover, adjuvants targeting TLRs in early life have been shown to boost early life adaptive immunity in humans and animal models. Adjuvants combining Alum with TLR4 (GLA-squalene emulsion (SE)] or TLR9 (CpG-1826 and IC31) were able to induce TFH responses in neonatal mice (149, 155). Indeed, a TLR7/8 agonist adjuvant 3M-052 overcame the hyporesponsiveness to the pneumococcal conjugate vaccine at birth (156). The TLR4 agonist, monophosphoryl lipid A (MPL), combined with Quillaja saponaria (QS-21), an adjuvant called AS01, also enhanced polyfunctional T cell responses in a recent malaria vaccine trial in young infants (157, 158). Furthermore, infant rhesus macaques immunized against HIV with adjuvants such as MF59, AS01, and 3M-052/SE had higher magnitude and avidity antibody responses compared to those immunized with Alum (159). Infant rhesus macaques immunized with the 3M-052/SE TLR activating adjuvant also had higher HIV-specific B cell responses, showing that these adjuvants can elicit strong B cell and humoral responses in early life (159). Moreover, a novel C-type lectin agonist (CAF01) and other pathogen-derived adjuvants (LT-K63 and mmCT) were able to enhance germinal center responses in neonatal mice (154, 155, 160). These studies highlight that newer adjuvants can greatly improve adaptive T cell and humoral immune responses in early life.
Induction of long-lasting vaccine-elicited immunity in early life must also involve optimization of the infant immunization schedule. We have demonstrated in infant rhesus macaques that extending the interval between HIV immunizations from 3 to 6 weeks increased the durability of antibody responses and promoted the generation of high avidity HIV-specific antibodies (159). Increasing the time between immunizations may allow for improved long-lived plasma cell and antigen-specific memory B cell development. However, human studies comparing different immunization intervals for eliciting antigen-specific T cell and humoral responses in early life are lacking, highlighting the need for more research as to the most efficacious interval between booster doses. Moreover, the timing of certain vaccines and coordination of vaccine regimens must be considered given that certain early life immunizations (e.g., BCG and pertussis) may also improve subsequent vaccine responses by boosting adaptive immunity broadly.
Another way to overcome the challenges to generating persistent humoral responses in neonates is to leverage passive maternal antibody transfer. Vaccine-elicited IgG transferred transplacentally and sIgA transferred via breast milk can protect neonates while adaptive immunity matures. Maternal vaccination against influenza and pertussis during pregnancy has been shown to be effective in protecting infants (161, 162). Moreover, we recently showed that maternal tetanus, diphtheria and pertussis (Tdap) vaccination during pregnancy improved infant vaccine-specific antibody levels when compared to prenatal vaccination (163). Importantly, additional research into the antibody characteristics, such as subtype and Fc glycosylation, that are most efficiently transferred and that best enhance infant immunity is necessary to maximize the benefits of such interventions [reviewed in (125)].
Considerations of maternal antibody interference with infant B cell development and antibody responses must be considered when integrating maternal vaccination strategies into the pediatric vaccine schedule. A recent randomized control trial of maternal Tdap vaccination suggested that infant vaccination may need to be delayed in the setting of maternal immunization (164, 165). Moreover, maternal immunization in the late secondary trimester led to improved infant antibody levels compared to third trimester vaccination (163). Thus, strategies to improve early life immunity through interventions aimed directly at the neonate and indirectly though maternal immunization must be coordinated.
Though early life adaptive immune responses to pathogens and vaccinations are limited, the neonatal immune system is clearly capable of generating antigen-specific T and B cell responses. Importantly, the immunologic milieu, immune stimulus, and immune cross-talk from maternal antibodies and microbial colonization are key regulators of infant adaptive immune responses, which must be considered when developing immune-based interventions for early life. In order to harness infant adaptive immunity through vaccination, the informed use adjuvants and optimization of vaccine schedules will be essential. Moreover, immunization strategies targeting mother-infant dyads and passive maternal antibody transfer are a promising strategy for protecting neonates during this vulnerable period of immune development. Significant work remains in this specialized area of human immunology, yet the unique nature of the infant immune system need not be viewed as a barrier to developing effective therapies. By translating our knowledge of early life adaptive immunity, we can develop targeted interventions that improve pediatric health on a global scale.
ECS and JLC completed the literature review. ECS, JLC, and RG wrote the first draft of the manuscript. TDB, SRP, and GGF helped conceptualize the manuscript. ECS, JLC, RG, TDB, SRP, and GGF all revised and edited the manuscript. All authors contributed to the article and approved the submitted version.
This study was supported by NIH NIAID 2P01AI117915 “Early Life Vaccination to Prevent HIV Acquisition during Adolescence” (SP, GF), NIH NIAID R01AI131978 “Functional Profile to HIV Vaccine Elicited Antibodies in Infants” (GF).
SP provides consulting services to Moderna, Merck and Co Vaccines, Pfizer Inc. and Sanofi for their preclinical CMV vaccine programs.
The remaining authors declare that the research was conducted in the absence of any commercial or financial relationships that could be construed as a potential conflict of interest.
Figures were created with BioRender.com.
1. Bhutta ZA, Black RE. Global maternal, newborn, and child health - So near and yet so far. N Engl J Med (2013) 369:2226–35. doi: 10.1056/NEJMra1111853
2. Liu L, Oza S, Hogan D, Perin J, Rudan I, Lawn JE, et al. Global, regional, and national causes of child mortality in 2000-13, with projections to inform post-2015 priorities: an updated systematic analysis. Lancet (2015) 385(9966):430–40. doi: 10.1016/S0140-6736(14)61698-6
3. Hug L, Alexander M, You D, Alkema L. UN Inter-agency Group for Child Mortality Estimation. National, regional, and global levels and trends in neonatal mortality between 1990 and 2017, with scenario-based projections to 2030: a systematic analysis. Lancet Glob Health (2019) 7(6):e710–20. doi: 10.1016/S2214-109X(19)30163-9
4. Levine OS, Bloom DE, Cherian T, De Quadros C, Sow S, Wecker J, et al. The future of immunisation policy, implementation, and financing. Lancet (2011) 378:439. doi: 10.1016/S0140. www.thelancet.com.
5. Marchant A, Kollmann TR. Understanding the Ontogeny of the Immune System to Promote Immune-Mediated Health for Life. Front Immunol (2015) 6:77 doi: 10.3389/fimmu.2015.00077
6. Kollmann TR, Kampmann B, Mazmanian SK, Marchant A, Levy O. Protecting the Newborn and Young Infant from Infectious Diseases: Lessons from Immune Ontogeny. Immunity (2017) 46:350–63. doi: 10.1016/j.immuni.2017.03.009
7. Zhang X, Zhivaki D, Lo-Man R. Unique aspects of the perinatal immune system. Nat Rev Immunol (2017) 17:495–507. doi: 10.1038/nri.2017.54
8. Mold JE, McCune JM. Immunological Tolerance During Fetal Development. From Mouse to Man. In: Advances in Immunology. San Diego, CA: Academic Press Inc. (2012). p. 73–111. doi: 10.1016/B978-0-12-394299-9.00003-5
9. den Braber I, Mugwagwa T, Vrisekoop N, Westera L, Mögling R, Bregje de Boer A, et al. Maintenance of Peripheral Naive T Cells Is Sustained by Thymus Output in Mice but Not Humans. Immunity (2012) 36:288–97. doi: 10.1016/j.immuni.2012.02.006
10. Park J-E, Jardine L, Gottgens B, Teichmann SA, Haniffa M. Prenatal development of human immunity. Science (80- ) (2020) 368:600–3. doi: 10.1126/science.aaz9330
11. Adkins B, Leclerc C, Marshall-Clarke S. Neonatal adaptive immunity comes of age. Nat Rev Immunol (2004) 4:553–64. doi: 10.1038/nri1394
12. Rudd BD. Neonatal T Cells: A Reinterpretation. Annu Rev Immunol (2020) 38:229–47. doi: 10.1146/annurev-immunol-091319-083608
13. Olin A, Henckel E, Chen Y, Lakshmikanth T, Pou C, Mikes J, et al. Stereotypic Immune System Development in Newborn Children. Cell (2018) 174:1277–92.e14. doi: 10.1016/j.cell.2018.06.045
14. Hodgins DC, Shewen PE. Vaccination of neonates: Problem and issues. Vaccine (2012) 30:1541–59. doi: 10.1016/j.vaccine.2011.12.047
15. Belderbos ME, Levy O, Meyaard L, Bont L. Plasma-mediated immune suppression: A neonatal perspective. Pediatr Allergy Immunol (2013) 24:102–13. doi: 10.1111/pai.12023
16. Haynes BF, Heinly CS. Early human T cell development: Analysis of the human thymus at the time of initial entry of hematopoietic stem cells into the fetal thymic microenvironment. J Exp Med (1995) 181:1445–58. doi: 10.1084/jem.181.4.1445
17. Haynes BE, Martin ME, Kay HH, Kurtzberg J. Early events in human T cell ontogeny: Phenotypic characterization and immunohistologic localization of t cell precursors in early human fetal tissues. J Exp Med (1988) 168:1061–810. doi: 10.1084/jem.168.3.1061
18. Schönland SO, Zimmer JK, Lopez-Benitez CM, Widmann T, Ramin KD, Goronzy JJ, et al. Homeostatic control of T-cell generation in neonates. Blood (2003) 102:1428–34. doi: 10.1182/blood-2002-11-3591
19. Rechavi E, Lev A, Lee YN, Simon AJ, Yinon Y, Lipitz S, et al. Timely and spatially regulated maturation of B and T cell repertoire during human fetal development. Sci Transl Med (2015) 7:276ra25. doi: 10.1126/scitranslmed.aaa0072
20. Thome JJC, Bickham KL, Ohmura Y, Kubota M, Matsuoka N, Gordon C, et al. Early-life compartmentalization of human T cell differentiation and regulatory function in mucosal and lymphoid tissues. Nat Med (2016) 22:72–7. doi: 10.1038/nm.4008
21. Haines CJ, Giffon TD, Lu LS, Lu X, Tessier-Lavigne M, Ross DT, et al. Human CD4 + T cell recent thymic emigrants are identified by protein tyrosine kinase 7 and have reduced immune function. J Exp Med (2009) 206:275–85. doi: 10.1084/jem.20080996
22. Das A, Rouault-Pierre K, Kamdar S, Gomez-Tourino I, Wood K, Donaldson I, et al. Adaptive from Innate: Human IFN-γ + CD4 + T Cells Can Arise Directly from CXCL8-Producing Recent Thymic Emigrants in Babies and Adults. J Immunol (2017) 199:1696–705. doi: 10.4049/jimmunol.1700551
23. Pekalski ML, García AR, Ferreira RC, Rainbow DB, Smyth DJ, Mashar M, et al. Neonatal and adult recent thymic emigrants produce IL-8 and express complement receptors CR1 and CR2. JCI Insight (2017) 2:e93739. doi: 10.1172/jci.insight.93739
24. Paiva RS, Lino AC, Bergman ML, Caramalho Í, Sousa AE, Zelenay S, et al. Recent thymic emigrants are the preferential precursors of regulatory T cells differentiated in the periphery. Proc Natl Acad Sci U S A (2013) 110:6494–9. doi: 10.1073/pnas.1221955110
25. Zhang X, Mozeleski B, Lemoine S, Deŕiaud E, Lim A, Zhivaki D, et al. CD4 T cells with effector memory phenotype and function develop in the sterile environment of the fetus. Sci Transl Med (2014) 6:238ra72. doi: 10.1126/scitranslmed.3008748
26. Halkias J, Rackaityte E, Hillman SL, Aran D, Mendoza VF, Marshall LR, et al. CD161 contributes to prenatal immune suppression of IFN-γ-producing PLZF+ T cells. J Clin Invest (2019) 129:3562–77. doi: 10.1172/JCI125957
27. Gibbons D, Fleming P, Virasami A, Michel ML, Sebire NJ, Costeloe K, et al. Interleukin-8 (CXCL8) production is a signatory T cell effector function of human newborn infants. Nat Med (2014) 20:1206–10. doi: 10.1038/nm.3670
28. Scheible KM, Emo J, Laniewski N, Baran AM, Peterson DR, Holden-Wiltse J, et al. T cell developmental arrest in former premature infants increases risk of respiratory morbidity later in infancy. JCI Insight (2018) 3:e96724. doi: 10.1172/jci.insight.96724
29. Rudolph ME, McArthur MA, Barnes RS, Magder LS, Chen WH, Sztein MB. Differences between pediatric and adult T Cell Responses to in vitro staphylococcal enterotoxin B stimulation. Front Immunol (2018) 9:498. doi: 10.3389/fimmu.2018.00498
30. Mold JE, Michaëlsson J, Burt TD, Muench MO, Beckerman KP, Busch MP, et al. Maternal alloantigens promote the development of tolerogenic fetal regulatory T cells in utero. Science (80- ) (2008) 322:1562–5. doi: 10.1126/science.1164511
31. Galindo-Albarrán AO, López-Portales OH, Gutiérrez-Reyna DY, Rodríguez-Jorge O, Sánchez-Villanueva JA, Ramírez-Pliego O, et al. CD8+ T Cells from Human Neonates Are Biased toward an Innate Immune Response. Cell Rep (2016) 17:2151–60. doi: 10.1016/j.celrep.2016.10.056
32. Wang J, Wissink EM, Watson NB, Smith NL, Grimson A, Rudd BD. Fetal and adult progenitors give rise to unique populations of CD8+ T cells. Blood (2016) 128:3073–82. doi: 10.1182/blood-2016-06-725366
33. Smith NL, Patel RK, Reynaldi A, Grenier JK, Wang J, Watson NB, et al. Developmental Origin Governs CD8 + T Cell Fate Decisions during Infection. Cell (2018) 174:117–30.e14. doi: 10.1016/j.cell.2018.05.029
34. Ng MSF, Roth TL, Mendoza VF, Marson A, Burt TD. Helios enhances the preferential differentiation of human fetal CD4+ naïve T cells into regulatory T cells. Sci Immunol (2019) 4:eaav5947. doi: 10.1126/sciimmunol.aav5947
35. White GP, Watt PM, Holt BJ, Holt PG. Differential Patterns of Methylation of the IFN-γ Promoter at CpG and Non-CpG Sites Underlie Differences in IFN-γ Gene Expression Between Human Neonatal and Adult CD45RO – T Cells. J Immunol (2002) 168:2820–7. doi: 10.4049/jimmunol.168.6.2820
36. Goriely S, Van Lint C, Dadkhah R, Libin M, De Wit D, Demonté D, et al. A Defect in Nucleosome Remodeling Prevents IL-12(p35) Gene Transcription in Neonatal Dendritic Cells. J Exp Med (2004) 199:1011–6. doi: 10.1084/jem.20031272
37. Webster RB, Rodriguez Y, Klimecki WT, Vercelli D. The human IL-13 locus in neonatal CD4+ T cells is refractory to the acquisition of a repressive chromatin architecture. J Biol Chem (2007) 282:700–9. doi: 10.1074/jbc.M609501200
38. Palin AC, Ramachandran V, Acharya S, Lewis DB. Human Neonatal Naive CD4 + T Cells Have Enhanced Activation-Dependent Signaling Regulated by the MicroRNA miR-181a. J Immunol (2013) 190:2682–91. doi: 10.4049/jimmunol.1202534
39. Yu HR, Hsu TY, Huang HC, Kuo HC, Li SC, Yang KD, et al. Comparison of the functional microRNA expression in immune cell subsets of neonates and adults. Front Immunol (2016) 7:615. doi: 10.3389/fimmu.2016.00615
40. Bronevetsky Y, Burt TD, McCune JM. Lin28b Regulates Fetal Regulatory T Cell Differentiation through Modulation of TGF-β Signaling. J Immunol (2016) 197:4344–50. doi: 10.4049/jimmunol.1601070
41. Burt TD. Fetal Regulatory T Cells and Peripheral Immune Tolerance In Utero: Implications for Development and Disease. Am J Reprod Immunol (2013) 69:346–58. doi: 10.1111/aji.12083
42. Rackaityte E, Halkias J. Mechanisms of Fetal T Cell Tolerance and Immune Regulation. Front Immunol (2020) 11:588. doi: 10.3389/fimmu.2020.00588
43. Michaëlsson J, Mold JE, McCune JM, Nixon DF. Regulation of T Cell Responses in the Developing Human Fetus. J Immunol (2006) 176:5741–8. doi: 10.4049/jimmunol.176.10.5741
44. De Roock S, Hoeks SBEA, Meurs L, Steur A, Hoekstra MO, Prakken BJ, et al. Critical role for programmed death 1 signaling and protein kinase B in augmented regulatory T-cell induction in cord blood. J Allergy Clin Immunol (2011) 128:1369–71. doi: 10.1016/j.jaci.2011.08.006
45. Takahata Y, Nomura A, Takada H, Ohga S, Furuno K, Hikino S, et al. CD25+CD4+ T cells in human cord blood: An immunoregulatory subset with naive phenotype and specific expression of forkhead box p3 (Foxp3) gene. Exp Hematol (2004) 32:622–9. doi: 10.1016/j.exphem.2004.03.012
46. Qazi KR, Bach Jensen G, van der Heiden M, Björkander S, Holmlund U, Haileselassie Y, et al. Extremely Preterm Infants Have Significant Alterations in Their Conventional T Cell Compartment during the First Weeks of Life. J Immunol (2020) 204:68–77. doi: 10.4049/jimmunol.1900941
47. Debock I, Flamand V. Unbalanced neonatal CD4+ T-cell immunity. Front Immunol (2014) 5:393. doi: 10.3389/fimmu.2014.00393
48. Pettengill MA, van Haren SD, Levy O. Soluble mediators regulating immunity in early life. Front Immunol (2014) 5:457. doi: 10.3389/fimmu.2014.00457
49. Kollmann TR, Crabtree J, Rein-Weston A, Blimkie D, Thommai F, Wang XY, et al. Neonatal Innate TLR-Mediated Responses Are Distinct from Those of Adults. J Immunol (2009) 183:7150–60. doi: 10.4049/jimmunol.0901481
50. Belderbos ME, van Bleek GM, Levy O, Blanken MO, Houben ML, Schuijff L, et al. Skewed pattern of Toll-like receptor 4-mediated cytokine production in human neonatal blood: Low LPS-induced IL-12p70 and high IL-10 persist throughout the first month of life. Clin Immunol (2009) 133:228–37. doi: 10.1016/j.clim.2009.07.003
51. Hebel K, Weinert S, Kuropka B, Knolle J, Kosak B, Jorch G, et al. CD4 + T Cells from Human Neonates and Infants Are Poised Spontaneously To Run a Nonclassical IL-4 Program. J Immunol (2014) 192:5160–70. doi: 10.4049/jimmunol.1302539
52. Prescott SL, Macaubas C, Holt BJ, Smallacombe TB, Loh R, Sly PD, et al. Transplacental priming of the human immune system to environmental allergens: universal skewing of initial T cell responses toward the Th2 cytokine profile. J Immunol (1998) 160:4730–7.
53. Prescott SL, Macaubas C, Smallacombe T, Holt BJ, Sly PD, Holt PG. Development of allergen-specific T-cell memory in atopic and normal children. Lancet (1999) 353:196–200. doi: 10.1016/S0140-6736(98)05104-6
54. Kristjansson S, Bjarnarson SP, Wennergren G, Palsdottir AH, Arnadottir T, Haraldsson A, et al. Respiratory syncytial virus and other respiratory viruses during the first 3 months of life promote a local TH2-like response. J Allergy Clin Immunol (2005) 116:805–11. doi: 10.1016/j.jaci.2005.07.012
55. You D, Marr N, Saravia J, Shrestha B, Lee GI, Turvey SE, et al. IL-4Rα on CD4 + T cells plays a pathogenic role in respiratory syncytial virus reinfection in mice infected initially as neonates. J Leukoc Biol (2013) 93:933–42. doi: 10.1189/jlb.1012498
56. Restori KH, Srinivasa BT, Ward BJ, Fixman ED. Neonatal immunity, respiratory virus infections, and the development of asthma. Front Immunol (2018) 9:1249. doi: 10.3389/fimmu.2018.01249
57. Halonen M, Lohman IC, Stern DA, Spangenberg A, Anderson D, Mobley S, et al. Th1/Th2 Patterns and Balance in Cytokine Production in the Parents and Infants of a Large Birth Cohort. J Immunol (2009) 182:3285–93. doi: 10.4049/jimmunol.0711996
58. Yockey LJ, Lucas C, Iwasaki A. Contributions of maternal and fetal antiviral immunity in congenital disease. Science (80- ) (2020) 368:608–12. doi: 10.1126/science.aaz1960
59. Luzuriaga K, Holmes D, Hereema A, Wong J, Panicali DL, Sullivan JL. HIV-1-specific cytotoxic T lymphocyte responses in the first year of life. J Immunol (1995) 154:433–43.
60. Thobakgale CF, Ramduth D, Reddy S, Mkhwanazi N, de Pierres C, Moodley E, et al. Human Immunodeficiency Virus-Specific CD8+ T-Cell Activity Is Detectable from Birth in the Majority of In Utero-Infected Infants. J Virol (2007) 81:12775–84. doi: 10.1128/jvi.00624-07
61. Marchant A, Appay V, van der Sande M, Dulphy N, Liesnard C, Kidd M, et al. Mature CD8+ T lymphocyte response to viral infection during fetal life. J Clin Invest (2003) 111:1747–55. doi: 10.1172/jci17470
62. Hermann E, Truyens C, Alonso-Vega C, Even J, Rodriguez P, Berthe A, et al. Human fetuses are able to mount an adultlike CD8 T-cell response. Blood (2002) 100:2153–8. doi: 10.1182/blood.V100.6.2153
63. Odorizzi PM, Jagannathan P, McIntyre TI, Budker R, Prahl M, Auma A, et al. In utero priming of highly functional effector T cell responses to human malaria. Sci Transl Med (2018) 10:eaat6176. doi: 10.1126/scitranslmed.aat6176
64. Muenchhoff M, Prendergast JAJ, Goulder PJR. Immunity to HIV in early life. Front Immunol (2014) 5:391. doi: 10.3389/fimmu.2014.00391
65. Huygens A, Dauby N, Vermijlen D, Marchant A. Immunity to cytomegalovirus in early life. Front Immunol (2014) 5:552. doi: 10.3389/fimmu.2014.00552
66. Rechavi E, Somech R. Maturation of the immune system in the fetus and the implications for congenital CMV. Best Pract Res Clin Obstet Gynaecol (2019) 60:35–41. doi: 10.1016/j.bpobgyn.2019.03.002
67. King CL, Malhotra I, Wamachi A, Kioko J, Mungai P, Wahab SA, et al. Acquired Immune Responses to Plasmodium falciparum Merozoite Surface Protein-1 in the Human Fetus. J Immunol (2002) 168:356–64. doi: 10.4049/jimmunol.168.1.356
68. Vermijlen D, Brouwer M, Donner C, Liesnard C, Tackoen M, Van Rysselberge M, et al. Human cytomegalovirus elicits fetal γδ T cell responses in utero. J Exp Med (2010) 207:807–21. doi: 10.1084/jem.20090348
69. Huygens A, Lecomte S, Tackoen M, Olislagers V, Delmarcelle Y, Burny W, et al. Functional Exhaustion Limits CD4 + and CD8 + T-Cell Responses to Congenital Cytomegalovirus Infection. J Infect Dis (2015) 212(3):484–94. doi: 10.1093/infdis/jiv071
70. Babik JM, Cohan D, Monto A, Hartigan-O’connor DJ, Mccune JM. The Human Fetal Immune Response to Hepatitis C Virus Exposure in Utero. J Infect Dis (2011) 203(2):196–206. doi: 10.1093/infdis/jiq044
71. Dauby N, Goetghebuer T, Kollmann TR, Levy J, Marchant A. Uninfected but not unaffected: Chronic maternal infections during pregnancy, fetal immunity, and susceptibility to postnatal infections. Lancet Infect Dis (2012) 12:330–40. doi: 10.1016/S1473-3099(11)70341-3
72. Xainli J, Baisor M, Kastens W, Bockarie M, Adams JH, King CL. Age-Dependent Cellular Immune Responses to Plasmodium vivax Duffy Binding Protein in Humans. J Immunol (2002) 169:3200–7. doi: 10.4049/jimmunol.169.6.3200
73. Huang S, Dunkley-Thompson J, Tang Y, Macklin EA, Steel-Duncan J, Singh-Minott I, et al. Deficiency of HIV-Gag-Specific T Cells in Early Childhood Correlates with Poor Viral Containment. J Immunol (2008) 181:8103–11. doi: 10.4049/jimmunol.181.11.8103
74. Miles DJC, van der Sande M, Kaye S, Crozier S, Ojuola O, Palmero MS, et al. CD4 + T Cell Responses to Cytomegalovirus in Early Life: A Prospective Birth Cohort Study. J Infect Dis (2008) 197:658–62. doi: 10.1086/527418
75. Connors TJ, Baird JS, Yopes MC, Zens KD, Pethe K, Ravindranath TM, et al. Developmental Regulation of Effector and Resident Memory T Cell Generation during Pediatric Viral Respiratory Tract Infection. J Immunol (2018) 201:432–9. doi: 10.4049/jimmunol.1800396
76. Singh T, Heston SM, Langel SN, Blasi M, Hurst JH, Fouda GG, et al. Lessons from COVID-19 in children: Key hypotheses to guide preventative and therapeutic strategies. Clin Infect Dis (2020) 71:2006–13. doi: 10.1093/cid/ciaa547
77. Gans HA, Maldonado Y, Yasukawa LL, Beeler J, Audet S, Rinki MM, et al. IL-12, IFN-gamma, and T cell proliferation to measles in immunized infants. J Immunol (1999) 162:5569–75.
78. Gans H, Yasukawa L, Rinki M, DeHovitz R, Forghani B, Beeler J, et al. Immune Responses to Measles and Mumps Vaccination of Infants at 6, 9, and 12 Months. J Infect Dis (2001) 184:817–26. doi: 10.1086/323346
79. Ota MOC, Vekemans J, Schlegel-Haueter SE, Fielding K, Whittle H, Lambert PH, et al. Hepatitis B immunisation induces higher antibody and memory Th2 responses in new-borns than in adults. Vaccine (2004) 22:511–9. doi: 10.1016/j.vaccine.2003.07.020
80. Marchant A, Goetghebuer T, Ota MO, Wolfe I, Ceesay SJ, De Groote D, et al. Newborns develop a Th1-type immune response to Mycobacterium bovis bacillus Calmette-Guérin vaccination. J Immunol (1999) 163:2249–55.
81. Vekemans J, Amedei A, Ota MO, D'Elios MM, Goetghebuer T, Ismaili J, et al. Neonatal Bacillus Calmette-Guérin Vaccination Induces Adult-Like IFN-gamma Production by CD4+ T Lymphocytes. Eur J Immunol (2001) 31:1531–5. doi: 10.1002/1521-4141(200105)31:5<1531::AID-IMMU1531>3.0.CO;2-1
82. Hussey GD, Watkins MLV, Goddard EA, Gottschalk S, Hughes EJ, Iloni K, et al. Neonatal mycobacterial specific cytotoxic T-lymphocyte and cytokine profiles in response to distinct BCG vaccination strategies. Immunology (2002) 105:314–24. doi: 10.1046/j.1365-2567.2002.01366.x
83. Ota MOC, Vekemans J, Schlegel-Haueter SE, Fielding K, Sanneh M, Kidd M, et al. Influence of Mycobacterium bovis Bacillus Calmette-Guérin on Antibody and Cytokine Responses to Human Neonatal Vaccination. J Immunol (2002) 168:919–25. doi: 10.4049/jimmunol.168.2.919
84. Whittaker E, Goldblatt D, McIntyre P, Levy O. Neonatal immunization: Rationale, current state, and future prospects. Front Immunol (2018) 9:532. doi: 10.3389/fimmu.2018.00532
85. Mascart F, Verscheure V, Malfroot A, Hainaut M, Piérard D, Temerman S, et al. Bordetella pertussis Infection in 2-Month-Old Infants Promotes Type 1 T Cell Responses. J Immunol (2003) 170:1504–9. doi: 10.4049/jimmunol.170.3.1504
86. Siegrist CA, Aspinall R. B-cell responses to vaccination at the extremes of age. Nat Rev Immunol (2009) 9:185–94. doi: 10.1038/nri2508
87. Weigert M, Perry R, Kelley D, Hunkapiller T, Schilling J, Hood L. The joining of V and J gene segments creates antibody diversity. Nature (1980) 283(5746):497–9. doi: 10.1038/283497a0
88. Nielsen SCA, Roskin KM, Jackson KJL, Joshi SA, Nejad P, Lee JY, et al. Shaping of infant B cell receptor repertoires by environmental factors and infectious disease. Sci Transl Med (2019) 11:1–14. doi: 10.1126/scitranslmed.aat2004
89. IJspeert H, van Schouwenburg PA, van Zessen D, Pico-Knijnenburg I, Driessen GJ, Stubbs AP, et al. Evaluation of the antigen-experienced B-cell receptor repertoire in healthy children and adults. Front Immunol (2016) 7:410. doi: 10.3389/fimmu.2016.00410
90. Grimsholm O, Piano Mortari E, Davydov AN, Shugay M, Obraztsova AS, Bocci C, et al. The Interplay between CD27dull and CD27bright B Cells Ensures the Flexibility, Stability, and Resilience of Human B Cell Memory. Cell Rep (2020) 30:2963–77.e6. doi: 10.1016/j.celrep.2020.02.022
91. Schroeder HW, Zhang L, Philips JB. Slow, programmed maturation of the immunoglobulin HCDR3 repertoire during the third trimester of fetal life. Blood (2001) 98:2745–51. doi: 10.1182/blood.V98.9.2745
92. Kranich J, Krautler NJ. How follicular dendritic cells shape the B-cell antigenome. Front Immunol (2016) 7:225. doi: 10.3389/fimmu.2016.00225
93. Pihlgren M, Tougne C, Bozzotti P, Fulurija A, Duchosal MA, Lambert P-H, et al. Unresponsiveness to Lymphoid-Mediated Signals at the Neonatal Follicular Dendritic Cell Precursor Level Contributes to Delayed Germinal Center Induction and Limitations of Neonatal Antibody Responses to T-Dependent Antigens. J Immunol (2003) 170:2824–32. doi: 10.4049/jimmunol.170.6.2824
94. Kruschinski C, Zidan M, Debertin AS, Von Hörsten S, Pabst R. Age-Dependent Development of the Splenic Marginal Zone in Human Infants Is Associated with Different Causes of Death. Hum Pathol (2004) 35:113–21. doi: 10.1016/S0046-8177(03)00422-2
95. Pettengill MA, Levy O. Circulating human neonatal naïve B cells are deficient in CD73 impairing purine salvage. Front Immunol (2016) 7:121. doi: 10.3389/fimmu.2016.00121
96. Schena F, Volpi S, Faliti CE, Penco F, Santi S, Proietti M, et al. Dependence of Immunoglobulin Class Switch Recombination in B Cells on Vesicular Release of ATP and CD73 Ectonucleotidase Activity. Cell Rep (2013) 3:1824–31. doi: 10.1016/j.celrep.2013.05.022
97. Saso A, Kampmann B. Vaccine responses in newborns. Semin Immunopathol (2017) 39:627–42. doi: 10.1007/s00281-017-0654-9
98. Pihlgren M, Friedli M, Tougne C, Rochat A-F, Lambert P-H, Siegrist C-A. Reduced Ability of Neonatal and Early-Life Bone Marrow Stromal Cells to Support Plasmablast Survival. J Immunol (2006) 176:165–72. doi: 10.4049/jimmunol.176.1.165
99. Belnoue E, Pihlgren M, McGaha TL, Tougne C, Rochat AF, Bossen C, et al. APRIL is critical for plasmablast survival in the bone marrow and poorly expressed by early-life bone marrow stromal cells. Blood (2008) 111:2755–64. doi: 10.1182/blood-2007-09-110858
100. Benson MJ, Erickson LD, Gleeson MW, Noelle RJ. Affinity of antigen encounter and other early B-cell signals determine B-cell fate. Curr Opin Immunol (2007) 19:275–80. doi: 10.1016/j.coi.2007.04.009
101. Gatto D, Pfister T, Jegerlehner A, Martin SW, Kopf M, Bachmann MF. Complement receptors regulate differentiation of bone marrow plasma cell precursors expressing transcription factors Blimp-1 and XBP-1. J Exp Med (2005) 201:993–1005. doi: 10.1084/jem.20042239
102. Rijkers GT, Sanders EAM, Breukels MA, Zegers BJM. Infant B cell responses to polysaccharide determinants. Vaccine (1998) 16:1396–400. doi: 10.1016/S0264-410X(98)00098-X
103. Mosier DE, Johnson BM. Ontogeny of mouse lymphocyte function. II. Development of the ability to produce antibody is modulated by T lymphocytes. J Exp Med (1975) 141:216–26. doi: 10.1084/jem.141.1.216
104. Jakobsen H, Bjarnarson S, Del Giudice G, Moreau M, Siegrist CA, Jonsdottir I. Intranasal immunization with pneumococcal conjugate vaccines with LT-K63, a nontoxic mutant of heat-labile enterotoxin, as adjuvant rapidly induces protective immunity against lethal pneumococcal infections in neonatal mice. Infect Immun (2002) 70:1443–52. doi: 10.1128/IAI.70.3.1443-1452.2002
105. Zandvoort A, Lodewijk ME, De Boer NK, Dammers PM, Kroese FGM, Timens W. CD27 expression in the human splenic marginal zone: The infant marginal zone is populated by naive B cells. Tissue Antigens (2001) 58:234–42. doi: 10.1034/j.1399-0039.2001.580403.x
106. Timens W, Boes A, Rozeboom-Uiterwijk T, Poppema S. Immaturity of the human splenic marginal zone in infancy. Possible contribution to the deficient infant immune response. J Immunol (1989) 143:3200 LP–3206.
107. Davis CA, Vallota EH, Forristal J. Serum complement levels in infancy: Age related changes. Pediatr Res (1979) 13:1043–6. doi: 10.1203/00006450-197909000-00019
108. Tasker L, Marshall-Clarke S. Functional responses of human neonatal B lymphocytes to antigen receptor cross-linking and CpG DNA. Clin Exp Immunol (2003) 134:409–19. doi: 10.1111/j.1365-2249.2003.02318.x
109. Cherukuri A, Cheng PC, Pierce SK. The Role of the CD19/CD21 Complex in B Cell Processing and Presentation of Complement-Tagged Antigens. J Immunol (2001) 167:163–72. doi: 10.4049/jimmunol.167.1.163
110. Rappuoli R, De Gregorio E, Costantino P. On the mechanisms of conjugate vaccines. Proc Natl Acad Sci U S A (2019) 116:14–6. doi: 10.1073/pnas.1819612116
111. Rappuoli R. Glycoconjugate vaccines: Principles and mechanisms. Sci Transl Med (2018) 10:1–7. doi: 10.1126/scitranslmed.aat4615
112. Pollard AJ, Perrett KP, Beverley PC. Maintaining protection against invasive bacteria with protein- polysaccharide conjugate vaccines. Nat Rev Immunol (2009) 9:213–20. doi: 10.1038/nri2494
113. Nguyen QN, Himes JE, Martinez DR, Permar SR. The Impact of the Gut Microbiota on Humoral Immunity to Pathogens and Vaccination in Early Infancy. PLoS Pathog (2016) 12:1–6. doi: 10.1371/journal.ppat.1005997
114. Vono M, Eberhardt CS, Auderset F, Mastelic-Gavillet B, Lemeille S, Christensen D, et al. Maternal Antibodies Inhibit Neonatal and Infant Responses to Vaccination by Shaping the Early-Life B Cell Repertoire within Germinal Centers. Cell Rep (2019) 28:1773–84.e5. doi: 10.1016/j.celrep.2019.07.047
115. Mina MJ, Kula T, Leng Y, Li M, De Vries RD, Knip M, et al. Measles virus infection diminishes preexisting antibodies that offer protection from other pathogens. Science (2019) 606:599–606. doi: 10.1126/science.aay6485
116. Albrecht M, Arck PC. Vertically Transferred Immunity in Neonates: Mothers, Mechanisms and Mediators. Front Immunol (2020) 11:555. doi: 10.3389/fimmu.2020.00555
117. Ghazal P, Dickinson P, Smith CL. Early life response to infection. Curr Opin Infect Dis (2013) 26:213–8. doi: 10.1097/QCO.0b013e32835fb8bf
118. Abu-Raya B, Smolen KK, Willems F, Kollmann TR, Marchant A. Transfer of maternal antimicrobial immunity to HIV-exposed uninfected newborns. Front Immunol (2016) 7:338. doi: 10.3389/fimmu.2016.00338
119. De Moraes-Pinto MI, Verhoeff F, Chimsuku L, Milligan PJM, Wesumperuma L, Broadhead RL, et al. Placental antibody transfer: Influence of maternal HIV infection and placental malaria. Arch Dis Child Fetal Neonatal Ed (1998) 79:202–5. doi: 10.1136/fn.79.3.F202
120. Dangor Z, Kwatra G, Izu A, Adrian P, Van Niekerk N, Cutland CL, et al. HIV-1 Is Associated with Lower Group B Streptococcus Capsular and Surface-Protein IgG Antibody Levels and Reduced Transplacental Antibody Transfer in Pregnant Women. J Infect Dis (2015) 212:453–62. doi: 10.1093/infdis/jiv064
121. Cumberland P, Shulman CE, Maple PAC, Bulmer JN, Dorman EK, Kawuondo K, et al. Maternal HIV Infection and Placental Malaria Reduce Transplacental Antibody Transfer and Tetanus Antibody Levels in Newborns in Kenya. J Infect Dis (2007) 196:550–7. doi: 10.1086/519845
122. Evans C, Jones CE, Prendergast AJ. HIV-exposed, uninfected infants: new global challenges in the era of paediatric HIV elimination. Lancet Infect Dis (2016) 16:e92–e107. doi: 10.1016/S1473-3099(16)00055-4
123. Niewiesk S. Maternal antibodies: Clinical significance, mechanism of interference with immune responses, and possible vaccination strategies. Front Immunol (2014) 5:446. doi: 10.3389/fimmu.2014.00446
124. Siegrist CA. Neonatal and early life vaccinology. Vaccine (2001) 19:3331–46. doi: 10.1016/S0264-410X(01)00028-7
125. Langel SN, Otero CE, Martinez DR, Permar SR. Maternal gatekeepers: How maternal antibody Fc characteristics influence passive transfer and infant protection. PLoS Pathog (2020) 16:1–8. doi: 10.1371/journal.ppat.1008303
126. Siegrist CA. Mechanisms by which maternal antibodies influence infant vaccine responses: Review of hypotheses and definition of main determinants. Vaccine (2003) 21:3406–12. doi: 10.1016/S0264-410X(03)00342-6
127. Kim D, Huey D, Oglesbee M, Niewiesk S. Insights into the regulatory mechanism controlling the inhibition of vaccine-induced seroconversion by maternal antibodies. Blood (2011) 117:6143–51. doi: 10.1182/blood-2010-11-320317
128. Richter MY, Jakobsen H, Haeuw JF, Power UF, Jonsdottir I. Protective levels of polysaccharide-specific maternal antibodies may enhance the immune response elicited by pneumococcal conjugates in neonatal and infant mice. Infect Immun (2005) 73:956–64. doi: 10.1128/IAI.73.2.956-964.2005
129. Gensollen T, Iyer SS, Kasper DL, Blumberg RS. How colonization by microbiota in early life shapes the immune system. Science (80- ) (2016) 352:539–44. doi: 10.1126/science.aad9378
130. Belkaid Y, Hand TW. Role of the microbiota in immunity and inflammation. Cell (2014) 157:121–41. doi: 10.1016/j.cell.2014.03.011
131. Al Nabhani Z, Eberl G. Imprinting of the immune system by the microbiota early in life. Mucosal Immunol (2020) 13:183–9. doi: 10.1038/s41385-020-0257-y
132. Gensollen T, Blumberg RS. Correlation between early-life regulation of the immune system by microbiota and allergy development. J Allergy Clin Immunol (2017) 139:1084–91. doi: 10.1016/j.jaci.2017.02.011
133. Burton DR, Hangartner L. Broadly Neutralizing Antibodies to HIV and Their Role in Vaccine Design. Annu Rev Immunol (2016) 34:635–59. doi: 10.1146/annurev-immunol-041015-055515
134. Williams WB, Liao HX, Moody MA, Kepler TB, Alam SM, Gao F, et al. Diversion of HIV-1 vaccine-induced immunity by gp41-microbiota cross-reactive antibodies. Science (80- ) (2015) 349:aab1253. doi: 10.1126/science.aab1253
135. Martinez DR, Permar SR, Fouda GG. Contrasting Adult and Infant Immune Responses to HIV Infection and Vaccination. Clin Vaccine Immunol (2016) 23:84–94. doi: 10.1128/CVI.00565-15
136. Goo L, Chohan V, Nduati R, Overbaugh J. Early development of broadly neutralizing antibodies in HIV-1-infected infants. Nat Med (2014) 20:655–8. doi: 10.1038/nm.3565
137. Ditse Z, Muenchhoff M, Adland E, Jooste P, Goulder P, Moore PL, et al. HIV-1 Subtype C-Infected Children with Exceptional Neutralization Breadth Exhibit Polyclonal Responses Targeting Known Epitopes. J Virol 92(17):e00878–18. doi: 10.1128/JVI.00878-18
138. Simonich CA, Williams KL, Verkerke HP, Williams JA, Nduati R, Lee KK, et al. HIV-1 Neutralizing Antibodies with Limited Hypermutation from an Infant. Cell (2016) 166(1):77–87. doi: 10.1016/j.cell.2016.05.055
139. Kumar S, Panda H, Makhdoomi MA, Mishra N, Safdari HA, Chawla H, et al. An HIV-1 Broadly Neutralizing Antibody from a Clade C-Infected Pediatric Elite Neutralizer Potently Neutralizes the Contemporaneous and Autologous Evolving Viruses. J Virol (2019) 93(4):e01495–18. doi: 10.1128/JVI.01495-18
140. Wiehe K, Bradley T, Meyerhoff RR, Hart C, Williams WB, Easterhoff D, et al. Functional Relevance of Improbable Antibody Mutations for HIV Broadly Neutralizing Antibody Development. Cell Host Microbe (2018) 23:759–65.e6. doi: 10.1016/j.chom.2018.04.018
141. Goodwin E, Gilman MSA, Wrapp D, Chen M, Ngwuta JO, Moin SM, et al. Infants Infected with Respiratory Syncytial Virus Generate Potent Neutralizing Antibodies that Lack Somatic Hypermutation. Immunity (2018) 48:339–49.e5. doi: 10.1016/j.immuni.2018.01.005
142. Sakala IG, Eichinger KM, Petrovsky N. Neonatal vaccine effectiveness and the role of adjuvants. Expert Rev Clin Immunol (2019) 15:869–78. doi: 10.1080/1744666X.2019.1642748
143. Fouda GG, Cunningham CK, McFarland EJ, Borkowsky W, Muresan P, Pollara J, et al. Infant HIV type 1 gp120 vaccination elicits robust and durable anti-V1V2 immunoglobulin G responses and only rare envelope-specific immunoglobulin a responses. J Infect Dis (2015) 211:508–17. doi: 10.1093/infdis/jiu444
144. Prabhudas M, Adkins B, Gans H, King C, Levy O, Ramilo O, et al. Challenges in infant immunity: Implications for responses to infection and vaccines. Nat Immunol (2011) 12:189–94. doi: 10.1038/ni0311-189
145. Mohr E, Siegrist CA. Vaccination in early life: Standing up to the challenges. Curr Opin Immunol (2016) 41:1–8. doi: 10.1016/j.coi.2016.04.004
146. Kollmann TR, Marchant A, Way SS. Vaccination strategies to enhance immunity in neonates. Science (80- ) (2020) 368:612–5. doi: 10.1126/science.aaz9447
147. Mastelic B, Kamath AT, Fontannaz P, Tougne C, Rochat A-F, Belnoue E, et al. Environmental and T Cell–Intrinsic Factors Limit the Expansion of Neonatal Follicular T Helper Cells but May Be Circumvented by Specific Adjuvants. J Immunol (2012) 189:5764–72. doi: 10.4049/jimmunol.1201143
148. Debock I, Jaworski K, Chadlaoui H, Delbauve S, Passon N, Twyffels L, et al. Neonatal Follicular Th Cell Responses Are Impaired and Modulated by IL-4. J Immunol (2013) 191:1231–9. doi: 10.4049/jimmunol.1203288
149. Mastelic-Gavillet B, Vono M, Gonzalez-Dias P, Ferreira FM, Cardozo L, Lambert PH, et al. Neonatal T follicular helper cells are lodged in a pre-T follicular helper stage favoring innate over adaptive germinal center responses. Front Immunol (2019) 10:1845. doi: 10.3389/fimmu.2019.01845
150. Levy O, Goriely S, Kollmann TR. Immune response to vaccine adjuvants during the first year of life. Vaccine (2013) 31:2500–5. doi: 10.1016/j.vaccine.2012.10.016
151. Nakaya HI, Clutterbuck E, Kazmin D, Wang L, Cortese M, Bosinger SE, et al. Systems biology of immunity to MF59-adjuvanted versus nonadjuvanted trivalent seasonal influenza vaccines in early childhood. Proc Natl Acad Sci U S A (2016) 113:1853–8. doi: 10.1073/pnas.1519690113
152. Vesikari T, Knuf M, Wutzler P, Karvonen A, Kieninger-Baum D, Schmitt HJ, et al. Oil-in-water emulsion adjuvant with influenza vaccine in young children. N Engl J Med (2011) 365:1406–16. doi: 10.1056/NEJMoa1010331
153. Mastelic Gavillet B, Eberhardt CS, Auderset F, Castellino F, Seubert A, Tregoning JS, et al. MF59 Mediates Its B Cell Adjuvanticity by Promoting T Follicular Helper Cells and Thus Germinal Center Responses in Adult and Early Life. J Immunol (2015) 194:4836–45. doi: 10.4049/jimmunol.1402071
154. Aradottir Pind AA, Dubik M, Thorsdottir S, Meinke A, Harandi AM, Holmgren J, et al. Adjuvants Enhance the Induction of Germinal Center and Antibody Secreting Cells in Spleen and Their Persistence in Bone Marrow of Neonatal Mice. Front Immunol (2019) 10:2214. doi: 10.3389/fimmu.2019.02214
155. Vono M, Eberhardt CS, Mohr E, Auderset F, Christensen D, Schmolke M, et al. Overcoming the neonatal limitations of inducing germinal centers through liposome-based adjuvants including C-type lectin agonists trehalose dibehenate or Curdlan. Front Immunol (2018) 9:381. doi: 10.3389/fimmu.2018.00381
156. Dowling DJ, van Haren SD, Scheid A, Bergelson I, Kim D, Mancuso CJ, et al. TLR7/8 adjuvant overcomes newborn hyporesponsiveness to pneumococcal conjugate vaccine at birth. JCI Insight (2017) 2:e91020. doi: 10.1172/jci.insight.91020
157. RTS, S Clinical Trials Partnership. Efficacy and safety of RTS,S/AS01 malaria vaccine with or without a booster dose in infants and children in Africa: Final results of a phase 3, individually randomised, controlled trial. Lancet (2015) 386:31–45. doi: 10.1016/S0140-6736(15)60721-8
158. Moncunill G, De Rosa SC, Ayestaran A, Nhabomba AJ, Mpina M, Cohen KW, et al. RTS,S/AS01E malaria vaccine induces memory and polyfunctional T cell responses in a pediatric African phase iii trial. Front Immunol (2017) 8:1008 doi: 10.3389/fimmu.2017.01008
159. Phillips B, Van Rompay KKA, Rodriguez-Nieves J, Lorin C, Koutsoukos M, Tomai M, et al. Adjuvant-Dependent Enhancement of HIV Env-Specific Antibody Responses in Infant Rhesus Macaques. J Virol (2018) 92:1051–69. doi: 10.1128/jvi.01051-18
160. Bjarnarson SP, Adarna BC, Benonisson H, Del Giudice G, Jonsdottir I. The Adjuvant LT-K63 Can Restore Delayed Maturation of Follicular Dendritic Cells and Poor Persistence of Both Protein- and Polysaccharide-Specific Antibody-Secreting Cells in Neonatal Mice. J Immunol (2012) 189:1265–73. doi: 10.4049/jimmunol.1200761
161. Madhi SA, Cutland CL, Kuwanda L, Weinberg A, Hugo A, Jones S, et al. Influenza vaccination of pregnant women and protection of their infants. N Engl J Med (2014) 371:918–31. doi: 10.1056/NEJMoa1401480
162. Amirthalingam G, Andrews N, Campbell H, Ribeiro S, Kara E, Donegan K, et al. Effectiveness of maternal pertussis vaccination in England: An observational study. Lancet (2014) 384:1521–8. doi: 10.1016/S0140-6736(14)60686-3
163. Eberhardt CS, Blanchard-Rohner G, Lemaître B, Boukrid M, Combescure C, Othenin-Girard V, et al. Maternal immunization earlier in pregnancy maximizes antibody transfer and expected infant seropositivity against pertussis. Clin Infect Dis (2016) 62:829–36. doi: 10.1093/cid/ciw027
164. Barug D, Pronk I, van Houten MA, Versteegh FGA, Knol MJ, van de Kassteele J, et al. Maternal pertussis vaccination and its effects on the immune response of infants aged up to 12 months in the Netherlands: an open-label, parallel, randomised controlled trial. Lancet Infect Dis (2019) 19:392–401. doi: 10.1016/S1473-3099(18)30717-5
Keywords: infant immunity, adaptive immunity, pediatric infectious diseases, neonatal T cells, vaccines, neonatal B cells, adjuvants, maternal antibody transfer
Citation: Semmes EC, Chen J-L, Goswami R, Burt TD, Permar SR and Fouda GG (2021) Understanding Early-Life Adaptive Immunity to Guide Interventions for Pediatric Health. Front. Immunol. 11:595297. doi: 10.3389/fimmu.2020.595297
Received: 15 August 2020; Accepted: 04 December 2020;
Published: 21 January 2021.
Edited by:
David J. Dowling, Boston Children’s Hospital, United StatesReviewed by:
Stefanía P. Bjarnarson, University of Iceland, IcelandCopyright © 2021 Semmes, Chen, Goswami, Burt, Permar and Fouda. This is an open-access article distributed under the terms of the Creative Commons Attribution License (CC BY). The use, distribution or reproduction in other forums is permitted, provided the original author(s) and the copyright owner(s) are credited and that the original publication in this journal is cited, in accordance with accepted academic practice. No use, distribution or reproduction is permitted which does not comply with these terms.
*Correspondence: Genevieve G. Fouda, R2VuZXZpZXZlLmZvdWRhQGR1a2UuZWR1
†These authors share first authorship
Disclaimer: All claims expressed in this article are solely those of the authors and do not necessarily represent those of their affiliated organizations, or those of the publisher, the editors and the reviewers. Any product that may be evaluated in this article or claim that may be made by its manufacturer is not guaranteed or endorsed by the publisher.
Research integrity at Frontiers
Learn more about the work of our research integrity team to safeguard the quality of each article we publish.