- 1Department of Stem Cell and Infectious Diseases, KaviKrishna Laboratory, Guwahati Biotech Park, Indian Institute of Technology, Guwahati, India
- 2KaviKrishna Telemedicine Care, Sualkuchi, India
- 3Department of Stem Cell and Infection, Thoreau Laboratory for Global Health, M2D2, University of Massachusetts, Lowell, MA, United States
Mycobacterium tuberculosis (Mtb), the causative organism of pulmonary tuberculosis (PTB) now infects more than half of the world population. The efficient transmission strategy of the pathogen includes first remaining dormant inside the infected host, next undergoing reactivation to cause post-primary tuberculosis of the lungs (PPTBL) and then transmit via aerosol to the community. In this review, we are exploring recent findings on the role of bone marrow (BM) stem cell niche in Mtb dormancy and reactivation that may underlie the mechanisms of PPTBL development. We suggest that pathogen’s interaction with the stem cell niche may be relevant in potential inflammation induced PPTBL reactivation, which need significant research attention for the future development of novel preventive and therapeutic strategies for PPTBL, especially in a post COVID-19 pandemic world. Finally, we put forward potential animal models to study the stem cell basis of Mtb dormancy and reactivation.
Introduction
Pulmonary tuberculosis (PTB) is a major global health disease. Each year nearly 10 million new PTB cases are reported as estimated by the world health organization (1). Then, these infected cases spread the disease in the community via aerosol, thus the bacterial transmission is maintained (2). Noticeably, humans are the only host in the entire animal kingdom where the bacteria can complete its transmission cycle under natural conditions (3). Therefore, any attempt to develop an effective policy to eradicate this pathogen from humans needs an appreciation of how the pathogen exploits immunocompetent adults to maximize its transmission success.
Mtb enters into the human host via aerosol, initiates a primary infection in the lungs, which generate active TB lesions including caseating granuloma formation (4–7). A vigorous cell-mediated immune response leads to eventual calcification of the granuloma, and the infected person develops a robust, life-long immunity against primary TB (8, 9). However, after 10–30 years of dormancy or latency, active TB lesions reappear in the apical part of the lungs as post-primary tuberculosis of the lungs (PPTBL) (2, 10, 11). Importantly, these PPTBL infected adults exhibit vigorous cell-mediated immunity (CMI) against Mtb as confirmed by a positive tuberculin test (8, 10–13). As the PPTBL progresses, pulmonary tissues are filled with heterogeneous types of granulomas that include active cavities, as well as fibrotic, non-progressive, sterile granulomas (14). These non-progressive granulomas are a highly organized structure (14), where the dormant bacilli remain in a standoff with the immune cells (9, 15, 16). This highly organized non-progressive granuloma structure is unique to human TB infection and is not present in mouse models of PTB (17–19). The active granulomas expand into nearby bronchioles, allowing the bacteria to enter into the sputum. Then, the infected person spreads aerosols containing live Mtb into the community by the process of vigorous coughing. The bacteria enter into a new host, initiate primary TB infection in the lungs, undergo latency for years and initiate PPTBL. Thus, the initiation of PPTBL occurs in the lungs of an adult with latent TB infection (LTBI) (the time period between primary infection and the clinical manifestation of PPTBL). Hence the disease is named as post-primary TB of the lungs or PPTBL (8, 20) and only by causing PPTBL, Mtb maintains transmission in human (2, 21).
The source of Mtb in the adult that initiate PPTBL is not yet clearly known, which limits our ability to target the transmission strategy of the pathogen in the community. From the perspective of Mtb transmission, the effective means for PPTBL development would be to hide in dormant state intracellular to a host cell type; which is immunosuppressed (21) and has self-renewal and migratory ability. Such a strategic approach would then permit the pathogen to migrate to apical part of the lungs to initiate a pneumonia-like exudative early phase of PPTBL. Previously, we proposed that the human adult stem cell niche in bone marrow (BM) might serve as a protective niche for dormant Mtb (22), and these cells would then migrate to lungs for PPTBL development (Figure 1). Surprisingly, many clinicians had already provided anecdotal evidence of finding Mtb in BM including our experience in Bhutan during 1995–1998 (23, 29) provide “bed to benchside” rationale to examine whether Mtb may hijack the BM-stem cell niche for its transmission strategy (22). However, skeptics will point out that hijacking of the stem cell niche will lead to widespread hematological and other stem cell-related disorders, often not seen in patients with PPTBL. We demonstrated that Mtb infects a rare population of human BM-stem cell to remain dormant (22). This may explain why hematological disorders are not widespread in PPTBL subjects. Following our initial findings, many laboratories not only reproduced our findings but also added important information about the Mtb/human BM-stem cell host-pathogen interaction (24–26). These findings raise human bone marrow derived stem cells as the site of Mtb latency (27). Recently, we have shown that stem cell altruism may be involved in Mtb-reactivation (28), and in the aerosol-induced immunity (29). These recent studies indicate the emerging role of BM stem cell niche in the pathogenesis and community transmission of Mtb.
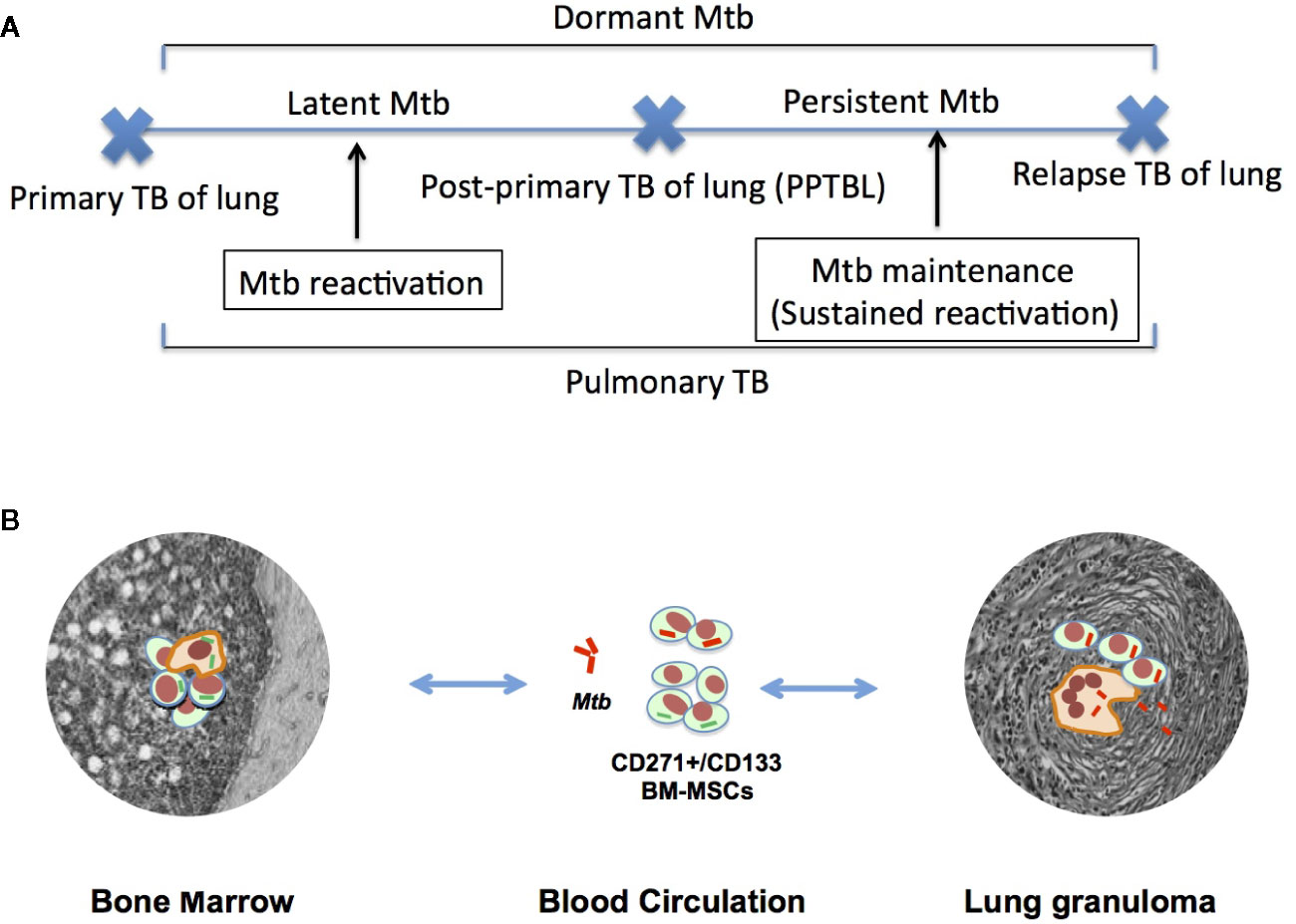
Figure 1 A hypothetical model of MSC mediated PPTBL development. (A) A schematic model of Mtb dormancy and reactivation showing the meaning of different terminologies used in the review article. (B) Hypothetical model showing the role of CD271+BM-MSC in PPTBL development. The model introduces a new self-renewing cell type, the CD271+BM-MSCs as a potential reservoir of dormant Mtb (dMtb). Following primary TB infection, dMtb hide in BM. In adults, inflammation in the lungs mobilizes BM-MSCs. The mobilization process facilitates homing of dMtb harboring BM-MSCs into the lungs leading to PPTBL development [adapted from reference no. (22)].
The primary objective of this review is to discuss the significance of the adult stem cell niche as a protective site of TB dormancy and reactivation that allows the pathogen to initiate PPTBL in an immunocompetent adult and subsequently to transmit Mtb in the community. We speculate that during early phase of PPTBL initiation, Mtb harboring BM stem cells may mobilize, and home to lung for initiating PPTBL, and then exporting the bacteria to the community via sputum, thus completing the bacterial transmission cycle. Thus, in this review, we will put forward a new model of Mtb transmission in the community, and discuss the ways for the Mtb transmission cycle to be blocked by modulating the process of BM stem cell mobilization to lung. Furthermore, we put forward potential animal models to study the interaction between pathogen and stem cell niche so that critical mechanisms including putative stem cell niche defense mechanism can be studied and can be targeted to eliminate latent Mtb infection.
The Enigmatic Source of Dormant MTB for the Initiation of PPTBL
A potential source of PPTBL is the exogenous re-infection with a different Mtb strain (30, 31), particularly in the geographical area of high TB incidence (31, 32). To explain the mechanism of PPTBL initiation in LTBI subjects, Medlar proposed the “allergic soil” hypothesis. Medlar suggested that the primary TB infection pre-conditions the apical part of the lungs for later migration of exogenous, re-infecting Mtb (5). However, the “allergic soil” hypothesis was ruled out in a guinea pig model of re-infection (33). Recent genome-based studies showed that endogenous or exogenous reactivation of Mtb could be evaluated by performing genotyping and whole genome sequencing (WGS) (34–36). However, it is not clear, how the bacteria from the exogenous re-infection would survive and initiate PPTBL in an immunocompetent adult host having vigorous cell mediated immunity against Mtb (21, 37–39). In this context, PPTBL initiation may rather be caused by endogenous Mtb strain that remained dormant in the human host after primary infection.
It is presumed that the endogenous source of the replicating Mtb in the PPTBL is the dormant Mtb hiding in the granulomas of lungs, and/or in the extra-granuloma sites in the lungs (3, 11, 12, 40–42). This presumption is supported by numerous animal models of Mtb infection, where, the dormant state is achieved as a result of Mtb interaction with the immune cells present in the granuloma (9, 19, 37, 43, 44) and the hypoxic microenvironment prevailing in the granuloma (45–49). However, the site of dormant Mtb in the lungs of LTBI subjects is not clearly known (4–6, 21, 39), although several probable sites have been proposed based on clinical findings (Table 1).
An obvious first site to look for the dormant Mtb would be the primary TB associated site, known as the “ghon complex”, a fibrotic granuloma located mostly in the lower lungs pleural area, and calcified hilar lymph nodes (2, 50). The granuloma becomes calcified and sterile 2–5 years after development of primary TB (2, 4, 21, 38, 50, 51). The site does not show any Mtb reactivation activity in PPTBL, which occurs 10–30 years after the primary TB (5, 20, 39). In fact, PPTBL occurs mostly in the apical part of the lungs (4, 21, 37, 50) instead in the primary TB infection site. Therefore, it is most unlikely that the primary granuloma is the site of Mtb dormancy and reactivation.
The second possible site would be the apical part of the lungs, the most common site of PPTBL. However, during the LTBI period, no dormant granulomas could be found at this site (4, 20, 34, 37, 50, 59). The third probable site is the normal lungs tissues. However, during LTB, no dormant granulomas containing viable Mtb could be found in the lungs (4, 20, 37, 42, 50, 59). Instead, human autopsy reports found a small amount of Mtb-DNA scattered intracellular and extracellular in the normal tissues of the lungs (21, 37, 53). Surprisingly, viable Mtb were isolated from normal lungs tissue of LTBI subjects in one report; however subsequent studies were not done to confirm whether the LTBI subjects were suffering from subclinical PPTBL infection (52). Some of the bacilli remain intracellular in alveolar macrophages (53). Mtb has been found to re-program macrophages to remain in a relatively non-replicative dormant state (9, 60); however, the viability of intracellular Mtb is poor (54), and so far, no direct isolation of viable Mtb from macrophages of LTBI subject has been reported (22, 37). Importantly, latent or dormant Mtb are highly immunogenic intracellular to macrophages as the bacteria fail to arrest phagosome maturation and are mostly processed for efficient antigen presentation to stimulate adaptive immunity (61). Thus, in LTBI subjects having vigorous T cell mediated immunity, it is highly unlikely that latent Mtb can persists intracellular to macrophages, as the pathogen will be killed by macrophage with the help of specific T cells mediated interferon gamma response (62).
The fourth potential site of dormancy is the putative alternate form of Mtb that includes endospores. However, such a phenotype of Mtb has not yet been identified, despite a century of research (37, 63).
The fifth potential source of PPTBL is the extra-pulmonary host cell types including adipocytes, fibroblasts and macrophages, where Mtb-DNA has been found (21, 37, 55). However, viable and dormant Mtb have not been recovered from these cell types. Furthermore, it is not clear how would Mtb migrate from these sites to the apical part of the lungs (59), because these cell types are differentiated and resident cells (22, 37).
Bone Marrow (BM) Stem Cell Niche as the Site of Dormant MTB
BM is an important site of the adult stem cell niche, where hematopoietic, mesenchymal and endothelial stem cells reside in their quiescent state (64). The BM stem cell niche is immunopriviledged (65, 66). These stem cells have the potential to self-renew (64, 67–70) and expand in the hypoxia/oxidative stress microenvironment (71–75), prevalent in the area of inflammation. The hypoxic microenvironment of stem cell niche may favor Mtb dormancy (76, 77) and resistance against anti TB drugs (56, 57). All these reasons unite to make BM stem cells a potential niche for Mtb during LTBI (27). Interestingly, stem cell’s self-renewal property is utilized by other bacteria, including Mycobacterium leprae (78) and Wolbachia (79) for effective transmission. Thus, it is presumed that BM stem cells’ self-renewal property could be utilized by dormant Mtb for initiation and transmission of PPTBL.
To investigate BM-stem cells as the potential host cells for dormant Mtb, we first focused on developing an in vitro model of Mtb and human BM-stem cell host pathogen interaction. We used a serum free media to culture CD133+BM cells (22, 73). The CD133+BM cells are enriched in HSCs, endothelial stem cells (22, 58, 80, 81) and naïve CD271+MSCs (22, 73). We found that Mtb infect CD271+BM-MSCs and CD34+BM-HSCs but maintain a non-replicating dormant status mainly in the CD271+BM-MSCs for 2 weeks. These preliminary in vitro results helped us to focus on the CD271+ BM-MSC as a possible Mtb target for dormancy (22).
CD271+BM-MSC is a type of multipotent mesenchymal stem cell (66, 82–84) that is very rare, comprising only 0.0017–0.0201% of BM mononuclear cell compartment (85). The cell type has potent immunosuppressive activity (84) and resides in the immunoprivileged and hypoxic niche in BM (65, 66). The CD271+ BM-MSCs are highly heterogeneous, and co-express two hematopoietic stem cell markers, CD133 and CD34 (22, 66, 86). Several recent studies have demonstrated the potential in vivo self-renewal property of CD271+ BM-MSCs. In these studies, the CD271+ BM-MSCs were directly isolated from human BM by flow cytometry or magnetic sorting technique, and their multipotent differentiation capacity was confirmed (22, 66, 86). The in vivo BM niche of this cell type was also identified, where they remain in a quiescent state (66, 87), and then self-renew during mobilization in response to tissue damage (86).
We speculated that the stemness state (the stem cell state of undifferentiating and self-renewal) of stem cell could be one of the key mechanisms of Mtb dormancy in stem cells. The CD271+BM-MSCs, when grown in vitro in high serum media or with adipogenic agents, differentiate to mesenchymal stromal cells, including the loss of stemness markers CD271, CD133, ABCG2 and HIF-2alpha expression (22, 73). It also significantly reduces the viability of intracellular dormant Mtb (dMtb) (22). These findings confirm the maintenance of the stemness state of stem cells is essential for Mtb dormancy.
To investigate if the CD271+BM-MSCs are the dormancy site for Mtb in PPTBL cases, we recruited patients through our KaviKrishna Telemedicine care (22, 29) located in NE India. These were successfully treated subjects for PPTBL, who donated 6–7 ml of BM for immunomagnetic separation of CD271+BM-MSCs. In the CD271+BM-MSCs from 9/11 subjects, Mtb-DNA was recovered, and two of these samples showed the presence of viable Mtb (22) (Table 1). Later, Tornack et al. also recovered Mtb-DNA from CD271+BM-MSCs of LTBI subjects (24). Thus, recovery of viable Mtb from CD271+BM-MSCs of PPTBL subjects successfully treated with anti-TB drugs indicates that BM stem cells may serve as a protective niche for Mtb against antibiotic treatment (22). Indeed, in a Cornell model of dormancy/reactivation, we recovered viable Mtb from the ABCG2+ expressing CD271+BM-MSCs despite prolonged anti-TB drug therapy (22, 56, 57). The MSCs expressing drug efflux pump, e.g. ABCG2 (22, 88, 89) might help intracellular Mtb to escape drug toxicity. Subsequently, we found that the Mtb-DNA harboring CD271+BM-MSCs of post-PPTBL subjects exhibited high expression of hypoxia inducible factor 1 alpha (HIF1alpha) and low expression of CD146 (a hypoxia down regulated cell surface marker) (56), suggesting that these Mtb infected BM-MSCs resided in the hypoxic niche of BM (56). Moreover, the hypoxic localization of these Mtb-harboring stem cells in the BM niche might make these Mtb unreachable by the current anti-TB therapy (56). Interestingly, hypoxia is known to induce dormancy in Mtb (45), and researchers found that Mtb intracellular to BM-stem cells of LTBI subjects express hypoxia induced dormancy genes DosR, hspX and c-lat (24). Overall, these findings suggest hypoxic niche of human BM-stem cells could be an important mechanism for Mtb dormancy.
Like human BM-MSCs, mouse BM-MSCs has also been found to contain dormant Mtb (22, 25). In a mouse model of Mtb dormancy, we recovered non-replicating dormant Mtb intracellular to CD271+BM-MSCs even after 6 months of primary TB infection. To confirm the long-term viability and re-infection capacity of these dormant Mtb, we performed the in vivo serial transplantation assay, where non-replicating Mtb harboring CD271+BM-MSCs from primary infected mice were injected into the secondary recipient mice. We showed that only a few of recovered dormant Mtb-m18b (~40) harboring CD271+BM-MSCs were enough to cause tubercular lesions in the lungs of secondary recipient mice (22), thus confirming the re-infection potential of dormant Mtb intracellular to CD271+BM-MSCs (22). Another study performed the expression of dormancy related genes in the Mtb recovered from CD45-Sca1+BM-MSCs; these cells were recovered from Mtb infected mice. The study found that recovered Mtb expresses dormancy related genes; thus confirming the dormancy status of the pathogen intracellular to Sca-1+BM-MSCs (25). However, in vivo transplantation assay was not performed to demonstrate the long-term viability of these dormant Mtb intracellular to Sca1+BM-MSCs.
The mechanism of Mtb dormancy intracellular to MSCs is now the subject of intense research. Using human and mouse mesenchymal stromal cells grown in the high serum media, several laboratories studied mechanisms of survival, adaptation and dormancy of Mtb intracellular to MSCs (24–26, 90). One of these studies found that Mtb remains in the lipid droplets inside the cytosolic fraction of MSCs induce lipid synthesis (25). Another study found that the cytosolic localization provides resistance capacity to Mtb against host cellular autophagy (91). Yet, another study found that virulent but not avirulent Mtb may reprogram BM-MSCs and remain viable inside them to escape cytotoxicity of antimicrobial peptide cathelicidin (90). A recent study found Mtb intracellular to BM-MSC exhibit increased expression of dormancy gene hspX with simultaneous increase in tolerance to anti TB therapy (26). Various studies showed that nitric oxide synthase 2 kills intracellular Mtb of MSCs by nitric oxide production (92–94), confirming the innate defense mechanism of MSC that may play important role in immune response against Mtb (95). In a model of human adipose tissue derived MSCs, virulent Mtb strain H37Rv exhibit a drug and inflammatory cytokine tolerant phenotype by modulating PGE2 signaling (96). These studies indicate the emerging significance of MSCs as a host cell for Mtb and also other pathogens (95, 97). Further studies using the in vivo naïve MSCs will be needed to determine the mechanism of Mtb dormancy intracellular to naïve human MSCs (73).
In addition to MSCs, HSCs may also be a potential niche for dormant Mtb (22, 24). Studies also showed that nitric oxide synthase 2 (Nos2) could play an important role in Mtb dormancy intracellular to HSCs (98). HSCs are the multipotent, self-renewing progenitor cells that reside in the BM niche in their quiescent state like MSCs (64, 65). In an invitro assay, we found that Mtb can infect CD34+HSCs (22). Furthermore, we found that HSCs of some of the previously treated PPTBL subjects contain dormant Mtb (22) (Table 1). Our finding was confirmed by Tornack et al. who recovered dormant Mtb in HSCs of LTBI human peripheral blood (24) (Table 1). When these human CD34+HSCs and mouse CD150+HSCs containing non-replicating Mtb were administered intratracheally to recipient immune-deficient mice, animals formed lesions in the lungs (24) suggesting that these dormant Mtb retained viability and re-infection capability. However, in mouse model of Mtb infection, HSCs has been found to resist internalization during acute infection (99).
Thus, we and others have identified MSCs and HSCs harboring dormant Mtb, and confirm their infectious and re-activating potential in a very limited number of subjects. However, a detailed study encompassing a larger group of individuals is required to test the hypothesis (22) that viable bacteria could be recovered from the BM-MSCs and HSCs of subjects with an early sub-clinical case of PPTBL.
Bone Marrow Derived Stem Cells’ Potential Role in PPTBL: A Testable Hypothesis
The development of PPTBL occurs in adult LTBI subjects positive for IGRA (Interferon-Gamma Release Assays) or TST (tuberculin skin test). The clinical presentation of PPTBL occurs in seemingly healthy adults who had an episode of acute respiratory tract infection (ARI) for more than 2 weeks. Medlar tried to explain the development of PPTBL by the “allergic soil” hypothesis (4, 5). Accordingly, primary TB infection pre-condition the apical part of the lungs for migration and homing of bacteria (4, 5). Medlar hypothesis resembles the site-specific homing and niche to niche migration of MSCs (64, 67, 100–102) and HSCs (103–105). Accordingly, the primary TB infection may pre-condition the apical part of the lungs for migration and homing of Mtb infected MSCs and or HSCs in appropriate conditions. Such a possibility is gaining significance as our understanding of BM-stem cells migration and homing to distant organs is growing.
Recent advances in stem cell research suggest that BM-derived stem cells migrate to the area of inflammation, including lungs (106–109). The CD271+ BM-MSCs’ migration from BM niche into the circulation (104, 108, 109) following tissue damage/inflammation associated with acute myocardial infarction (86) and acute Ischemic Stroke (110) suggesting the mobilizations of these cells to the site of inflammation/injury. Additionally, BM-stem cells exhibit age-specific mobilization to specific tissues (104, 111). Moreover, CD271+ BM-MSCs are significantly mobilized in adult/elderly versus children (86). Interestingly, this is the age group of PPTBL development.
Hence, based on these stem cells’ sites and age specific migration/homing potential, we have proposed a model of PPTBL development in an immunocompetent adult subject (22) (Figure 1). In this model, BM-stem cells harboring dormant Mtb may migrate to lungs in response to tissue inflammation likely due to ARI. These migratory stem cells will localize to the apical part of the lungs as per the site specific migration of CD271+ BM-MSCs and thus transfer Mtb to resident alveolar macrophages, as well as resident lung MSCs (Figure 1). As described in Figure 2A, periodic bouts of ARI may send signals from lungs to BM for stem cell mobilization as a part of BM-pulmonary niche to niche interaction. We hypothesize that dMtb harboring MSCs will migrate to area of inflammation in the lung, and reprogram to the “enhanced stemness” a transient phenotype of stem cells characterized by ability to maintain stemness, as well as secret cytoprotective agents in the microenvironment of extreme oxidative stress/inflammation (72, 112). Stem cells that reprogram to “enhanced stemness” phenotype activate a HIF-2alpha stemness pathway, and exhibit altruistic behavior (112) i.e. sacrificing self-fitness to enhance group fitness during stress, and therefore, these transient stem cells can be termed as altruistic stem cells (ASCs) (73, 112) in contrast to competitive stem cells that eliminate weak neighbors during stress (114). ES cell derived ASCs exhibited intrinsic stemness (niche independent stemness i.e. autocrine regulation of stemness) having an altruistic component i.e. ability to modulate the niche to enhance group fitness in the microenvironment of hypoxia/oxidative stress (Figure 3) (112). Thus, ASCs exhibit niche modulatory or altruistic stemness in the microenvironment of hypoxia/oxidative stress, and therefore serve as niche defense mechanism (73). We suggest that pathogen may exploit stem cell altruism (112) to enhance their fitness in the hostile microenvironment of lung. Thus, intracellular dMtb may facilitate ASC reprograming of MSCs in the ARI lung. The reprogrammed ASCs may then stimulate replicating of dMtb and their subsequent release to neighboring MSCs and or macrophages. This process will lead to PPTBL development (Figures 2B, C). This model depicted in Figure 2 can explain the clinical presentation of PPTBL in seemingly healthy adults who had an episode of ARI for more than 2 weeks before being diagnosed as a case of either sputum positive or negative PPTBL. The model can also explain the reactivation of dormant Mtb in immunocompetent adult despite having strong Mtb specific IFN-gamma producing CD4+/CD8+ T cells. We and others found that dMtb harboring MSCs are cytoprotective (56) and can resist IFN-gamma mediated toxicity (96), which may protect the reactivating Mtb from immune onslaught.
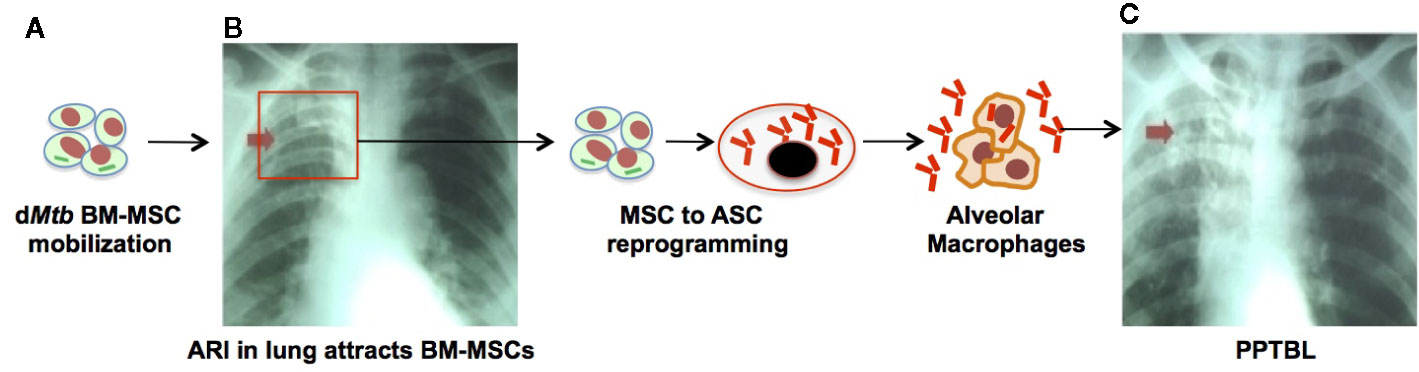
Figure 2 The emerging role of stem cell altruism in PPTBL development. (A) Inflammation such as acute respiratory tract infection (ARI) in lungs causes mobilization and homing of dMtb harboring BM-MSCs to the lungs. (B) Inside the lungs, dMtb-BM-MSCs self-renew and reprogram from MSCs to altruistic stem cells (ASCs) by the process of stem cell altruism (73, 112, 113). These ASCs undergo clonal proliferation and become permissible for intracellular replication of dMtb. Replicating Mtb exit ASCs into extracellular space to infect alveolar macrophages. During this phase, patient exhibit subclinical exudative, focal pneumonia like phase of PPTBL as shown in chest X-ray of a patient with sub-clinical PPTBL (arrow). (C) A host immune response surrounds the infected alveolar macrophages, and eventually leads to granuloma and cavity formation, thus developing clinical PPTBL as shown in chest X-ray of a PPTBL patient (arrow).
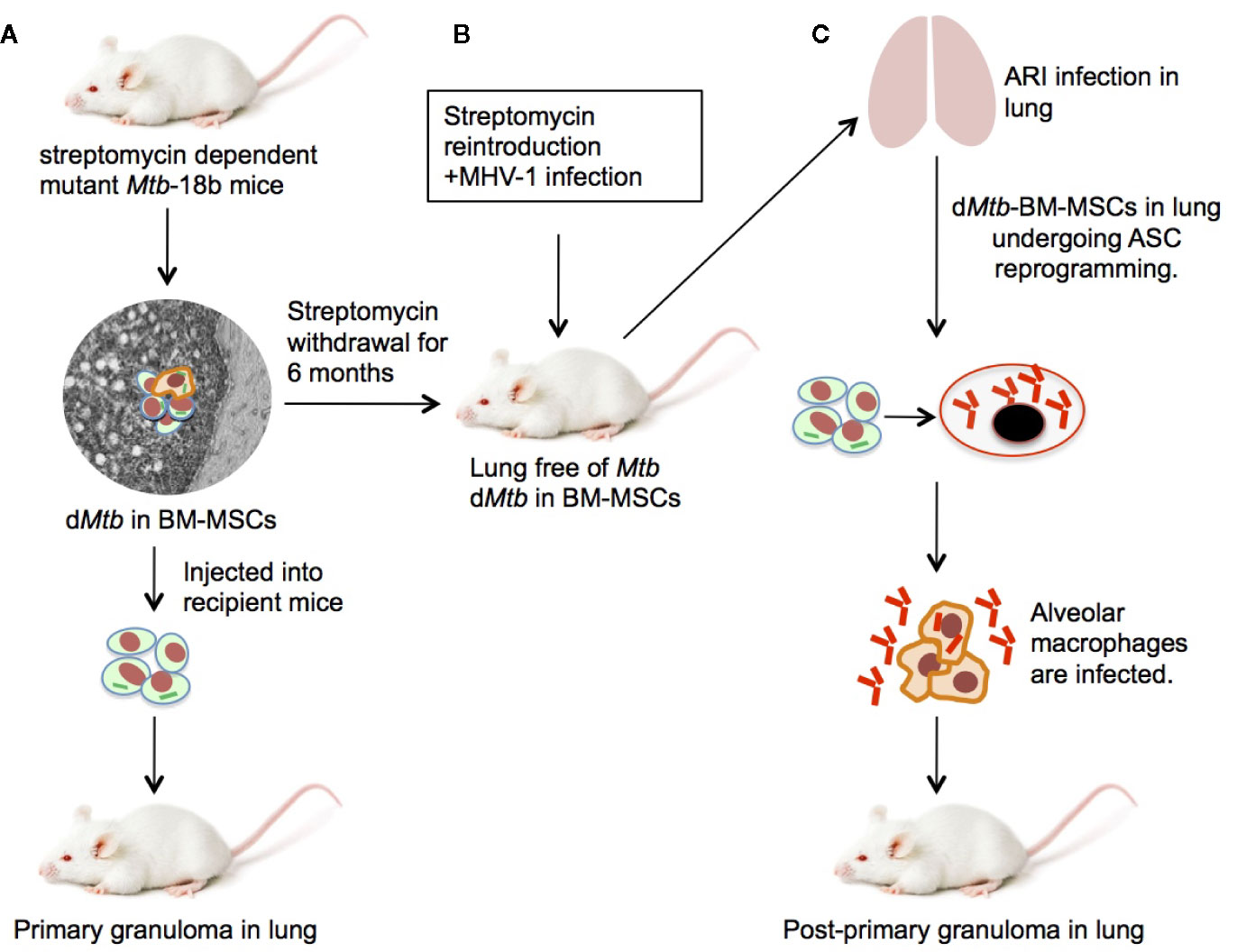
Figure 3 An experimental mouse model of stem cell mediated development of PPTBL. (A) Mouse model of streptomycin dependent mutant 18b-Mtb strain dormancy intracellular to BM-MSCs (22). (B) After 6 months of streptomycin starvation, streptomycin is re-introduced and mice are infected with MHV-1 intranasally to cause acute respiratory tract infection (ARI) inducing BM-MSC mobilization to lungs. (C) The dMtb harboring BM-MSCs in MHV-1 infected lungs expands and reprogram to altruistic stem cells (ASCs; see text) that permit Mtb replication and exit to extracellular space. The reactivated Mtb then infect nearby alveolar macrophages, leading to PPTBL development.
The stem cell model of PPTBL may also help us to gain insight about sputum negative (Mtb negative for Acid Fast Bacilli stain and culture) PPTBL, where the lungs contain fibrotic, and non-progressive granulomas (14). These granulomas may contain mostly dead or deep-dormant Mtb that fails to stain for acid fast bacilli (AFB) (115), probably due to hypoxia/oxidative stress prevalent in the granuloma. These deep dormant Mtb are viable but non-culturable and require resuscitation factors (116). Clinical subjects of PPTBL harboring these granulomas may present in the clinic as sputum negative but Chest X-ray positive pulmonary TB (PTB) (117). Eventually, as the disease progress, the deep dormant bacteria in the granuloma in these patients may undergo resuscitation and therefore, patients turn into smear positive PTB. Thus, PPTBL progression and maintenance may involve a mechanism of resuscitating deep dormant bacteria. In this context, sputum of smear negative subjects contains viable but non-culturable (VBNC) Mtb (118); the source of this VBNC-Mtb may be lung granuloma as bacteria in sputum reflect active pulmonary lesions (119). Our preliminary study indicates that CD271+BM-MSCs may resuscitate VBNC deep dormant Mtb (29), suggesting the ability of stem cells to contribute to resuscitating dying granulomas. Recently, CD73+ cells containing Mtb antigen Ag85B has been detected in human lung granulomas (96), suggesting the potential presence of Mtb infected MSCs in active granuloma lesions of PTB, although further testing is required to confirm the MSC phenotype of the cells by direct sorting, as well as the viability of the intracellular Mtb. Thus, we propose that studying stem cell mediated resuscitation of deep dormant Mtb may contribute in understanding the pathogenesis of smear negative PTB.
Developing Mouse Models of Stem Cell Mediated Initiation/Maintenance of PPTBL
Animal models are essential to gain insight about the role of BM stem cells in the endogenous reactivation of dMtb leading to PPTBL. Guinea pigs, monkey, pigs, mice and rabbit are used to model TB infection. Among these animals, only in mice, the BM stem cell niche and resident lungs stem cells have been extensively studied. Hence, mice could be an appropriate animal model to study stem cells’ contribution to PPTBL initiation. Indeed, mouse model has been extensively used to study the immune equilibrium of lung granuloma (120), where MSC may contribute to favor Mtb growth (121). However, Mtb infected mice succumb to primary infection (22); therefore, PPTBL cannot be studied in this animal type. Additionally, Cornel model of dormancy is not considered as an appropriate model for PPTBL, as unlike in human, where immune cells induces dormancy, in the Cornell model, dormancy is achieved by treating animals with anti-TB drugs.
Nevertheless, we have used a streptomycin mutant strain of 18b (m18b) (122) infected mouse model to study PPTBL initiation. In this model, Mtb-m18b remains in viable and non-replicating state for 6 months intracellular to CD271+ BM-MSC population (22), mainly in the hypoxic niche (27). This mouse model is suitable to study stem cell mediated endogenous PPTBL, as the animal’s lungs become Mtb free after 6 months of streptomycin starvation; only a few Mtb in dormant state can be detected intracellular to lungs and BM CD271+MSCs (22), a situation similar to human spectrum of latent TB. Additionally, in this mouse model, we have fully characterized dormant Mtb harboring naïve MSCs in the lung and confirmed their stemness (self-renewal and differentiation). Therefore, in this mouse model, BM and lung MSC interaction can be evaluated during PPTBL development and maintenance. We have further improved this model by testing the idea of ARI mediated MSC to ASC reprograming and consequent Mtb reactivation as depicted in Figures 2 to 3. To investigate this possibility, dormant Mtb-m18b harboring mice were infected with an ARI causing corona virus, the MHV-1 (murine hepatitis virus strain-1) (123), to mimic oxidative/inflammatory stress in the lung. MHV-1 infection led to the ASC reprogramming of Mtb harboring CD271+MSCs. Importantly, ASC reprogramming led to Mtb reactivation/replication was reactivated in the lungs of 9/10 MHV-1 infected mice versus 1/10-control mice (28). Notably, the virus infected animal exhibited circulatory CD271+MSCs that contain Mtb (28) (Figure 3). We suggest that this MHV-1 infected mouse model of Mtb-m18b can be further improved as a putative mouse model of PPTBL. However, further studies are needed to confirm the usefulness of the mouse model to decipher the cellular and molecular mechanisms of Mtb dormancy in BM stem cells. Another approach would be to develop humanized mouse model that contains human immune system, so that dormant Mtb containing BM- stem cells of latent subjects can be injected to these animals. However, our last few years of attempt to develop this model were not successful as the humanized mouse model succumbs to Mtb infection within 2–3 months of infection.
Future Direction
Numerous studies suggest that source of PPTBL initiation is the endogenous reactivation (34–36) although the source has not been clearly known. Recent studies suggest that human BM-stem cells may serve as a protective niche for dormant Mtb having reactivation potential (22, 24). Hence, future studies should be directed to confirm the role of BM-stem cells in endogenous reactivation of dormant Mtb. For clinical context, endogenous reactivation can be confirmed by collecting Mtb in stem cells of LTBI patients, and the Mtb obtained from sputum of the same patient following PPTBL, and then subjecting these Mtb to genotyping and WGS (34–36). This approach will confirm either the PPTBL is due to endogenous Mtb reactivation or exogenous Mtb strain. Such an approach may also be helpful to screen potential HSC transplantation donors. Reports showing Mtb infection after allogeneic HSC transplantation (124) suggest that it is necessary to confirm intracellular Mtb dormancy status of the donor to avoid risk of future dMtb reactivation in the acceptor. In this context, we suggest that genotyping and WGS approaches may help to confirm the reactivation potential of dormant Mtb residing in donor’s BM-stem cells.
Future clinical studies should also be directed to examine if a dynamic interaction between the BM stem cell niche and lungs granulomas may contribute to PTB progression and drug-resistance. Stem cell model of Mtb dormancy/reactivation predicts that PPTBL mediated inflammation in the lungs will sustain the dynamic niche-to-niche interaction between BM-stem cells and lungs (Figures 1 to 2), thereby keeping granulomas alive. Pulmonary inflammation, especially in chronic obstructive pulmonary disease (COPD) and viral infection induced ARI may enhance the dynamic niche-to-niche interaction of BM stem cells and lungs niche thereby initiating and sustaining Mtb reactivation (28). Epidemiological data indicate that both smoking and COPD are two important risk factors for PPTBL initiation and relapse (125). Urban air pollution increases the incidence of COPD (126, 127), as well as pandemic such as COVID-19 related ARI (128). Importantly, both COPD and ARI may sustain and or aggravate the pulmonary inflammation. Hence, it is important to address the inflammatory aspect of PTB. Unfortunately, the inflammatory aspects of PTB are not well addressed clinically, and patients are left with inflammation, that may aid in COPD even after successful anti-TB therapy (125, 129, 130). In fact, millions of TB patients are suffering in their impoverished state in the developing world, mostly suffering from chronic lungs diseases including COPD related inflammation (126, 127) and ARI. Our two and half-decades of anecdotal experience in managing PPTBL subjects in Bhutan and India (23) through KaviKrishna telemedicine care (22, 29) (https://www.kavikrishnalab.org/ktc/) suggest that managing inflammation and improving the overall nutrition can reduce PPTBL development and relapse, consistent with published studies (131). Interestingly, a recent finding shows that anti-inflammatory drug celecoxib reduces the survival of Mtb intracellular to CD73+/Sca-1+ MSCs in the lung of INH treated mice with PTB (96) suggesting the potential benefit of managing inflammation to treat PPTBL. Whether such affordable intervention of anti-inflammatory agents reduces the dynamic interaction between stem cell niche and lungs granulomas is now under active investigation.
Further advance in the stem cell field is required to gain insight in to the mechanism of stem cell mediated Mtb dormancy and reactivation. It will be important to find out how the pathogen modulates stemness to maintain the immunosuppressive phenotype. Interestingly, Mtb infected adipose tissue derived MSCs has shown the activation of the autocrine pathway of PGE2 (96), which was earlier found to be involved in the stemness of MSCs. PGE2 may enhance the niche independent stemness of immunosuppressive MSCs (73), thus benefiting intracellular pathogen. BM stem cell niche may exert innate defense against pathogens by modulating the niche (73). Indeed, HSCs and endothelial progenitor cells modulate BM niche to prevent pathogen infection (132). We found that after intravenous injection of Mtb to mice, only a small fraction of BM-MSCs harbor the pathogen, suggesting that the stem cell may resist pathogen’s invasion. Indeed, in vitro studies found that intracellular Mtb are killed by autophagy and phagocytosis mechanism of MSCs (92). Also, Mtb infected MSCs secret nitric oxide (92) that kills the intracellular Mtb (93). Another in vitro study showed that rapamycin addition reduces the dormant Mtb load inside MSCs by inducing autophagy (25). These emerging data indicate a potential MSC mediated defense against Mtb invasion. Interestingly, pathogen including Mycobacterium avium may exhaust quiescence HSCs in BM niche by IFN-gamma mediated proliferation (133). Additionally, BCG or Mtb-H37Rv infection in mice BM causes HSC expansion (99, 134). These works suggest that pathogen may disturb the long-term self-renewal capacity of HSCs. In this context, we speculate that BM-stem cell niche has evolved niche defense mechanism to resist pathogen mediated HSC exhaustion. Mtb-H37Rv infection in BM causes the expansion of hypoxic MSCs (56), indicating the potential existence of a stem cell niche defense (73). However, in our Cornell model of dormancy study, Mtb seems to escape these mechanisms of stem cell niche defense to successfully reside inside BM-MSCs, while maintaining the long-term health of the animal (56), suggesting that HSCs were not exhausted. Thus, it appears that BM niche defense may have the ability to maintain HSC self-renewal despite pathogen invasion.
Understanding the mechanism of stem cell mediated defense against Mtb invasion will facilitate vaccine development against dormant Mtb (135). Our ongoing work on stem cell altruism (72, 73, 112) may help us to further gain insight about the putative ASC based stem cell niche defense and its role in Mtb dormancy and reactivation. First, we found that MHV-1 viral infection (a model of ARI) activates an innate ASC defense mechanism against the virus, and in the process, reactivation of dMtb occur (Figure 3). Interestingly, MHV-1 infection serve as a mouse model of clinically relevant human infecting severe acute respiratory syndrome corona virus 1 (SARS-CoV-1) strain (123) and possibly SARS-CoV-2 mediated COVID-19. Thus, MHV-1 mouse model may be useful to study whether SARS-CoV-2 infection would reactivate dormant TB infection (28). Second, ASC reprograming mechanism may be of relevance in the resuscitation of deep dormant Mtb (29), which are VBNC (118). Importantly, we showed that VBNC obtained from Mtb-m18b strain present in the sputum of smear negative PTB subjects could be resuscitated by BM-MSCs and during the resuscitation process, MSCs reprogram to ASCs (29). Third, in a mouse model of Mtb infection, we found that VBNC harboring ASCs in the lung were identified and found to export extracellular vesicle (EV) into the broncho-alveolar (BAL) fluid. The EVs are rich in ESAT-6 antigen and therefore, may serve as natural aerosol based vaccine. Fourth, we also isolated such ESAT-6 rich EVs in the aerosol of subjects with smear-negative PTB. Notably, the EVs rich aerosols did not contain live Mtb. These findings indicate that aerosols of PTB subjects may transmit antigens into the community without spreading the pathogen, a potential natural vaccination process of herd immunity (29). We propose that studying ASC reprogramming may reveal an already existed natural immunity mechanism in the community against Mtb, which may further be utilized to develop an improved control program for TB or other pathogens (29).
We speculate that the work on Mtb/BM-stem cell host/pathogen interaction may also provide insight about the memory component of BM-stem cell niche defense. It is conceivable that stem cell niche has evolved sophisticated mechanism to defend their niche, including the retention of specific memory of a given pathogen. It has been known that innate defense mechanism is capable of specific memory (136) even in a thymic mice (137), and this type of innate immune memory is now known as trained immunity (138), and the mechanisms include the imprinting of pathogen specific epigenetic signature in innate immune cells and resident stem cells (138). Trained immunity has been largely characterized in BCG-vaccinated mouse model of memory macrophages (139) and memory NK cells (140). Importantly, in a stem cell model of trained immunity, BCG trained HSCs in BM may differentiate to monocyte and contribute to lung-alveolar macrophages defense against the invasion of virulent Mtb strain H37Rv (134). This indicates the role of trained immunity in the interaction between BM-stem cell niche and alveolar-macrophage compartment. Notably, virulent Mtb strain H37Rv modulates trained immunity of HSCs (99) and also modulate the hypoxic microenvironment of BM niche (56). Thus, virulent Mtb may have evolved mechanisms to evade trained immunity to remain dormant intracellular to MSCs or HSCs. Studying the mechanisms of Mtb mediated evasion of trained immunity to persist intracellular to HSC/MSCs may help to develop innovative vaccine strategies against tuberculosis. In this context, it will be interesting to study potential of Mtb-induced ASCs (28, 29) as a part of trained immunity.
The pathogenesis and the immune response in PPTBL is complex with multiple players often having double roles of pathology versus protection (141, 142). It is possible that stem cells may have double role: on one hand, stem cells may protect a community by spreading herd immunity (29), and on the other hand, stem cells may serve as a protective niche for dormant Mtb. To decipher the complex role of stem cells in pathogenesis and the immune response to PPTBL, future studies of BM-stem cell biology need to further address the i) stem cell niche based defense mechanism ii) stem cells’ niche to niche interaction between bone marrow and lungs iii) the role of stem cell niche defense in trained immunity.
Discussion
In summary, emerging laboratory and clinical results now provide a conceptual framework of the potential role of adult stem cell niche in the dormant Mtb infection. Failure to eradicate TB, despite decades of TB control programs (143) may be due to dormant Mtb infection. The recent identification of CD271+ BM-MSCs, its localization in the hypoxic BM-niche (5, 66, 89), mobilization in response to tissue damage (88), and its ability to harbor dormant Mtb (9, 22) provide experimental support to this hypothesis. Importantly, our initial observations have been reproduced in many laboratories, which further strengthen our hypothesis. These findings suggest a deep evolutionary interaction between stem cells and Mtb, where the pathogen exploits self-renewal mechanism of stem cells to remain dormant and then induce PPTBL in healthy and immunocompetent hosts for their robust transmission in the human community. Advances in basic and translational biology research in stem cell and Mtb host/pathogen interaction is necessary in order to develop effective courses to eliminate this pathogen from human host.
Author Contributions
BD has conceptualized, wrote and edited the manuscript. LP has wrote, edited the manuscript and created the figures and table. All authors contributed to the article and approved the submitted version.
Funding
The work was supported by KaviKrishna Foundation, Sualkuchi, Assam (LP) (KKL/2018-4_MHV-1), Department of Biotechnology (DBT), Govt. of India (BD) (BT/PR22952/NER/95/572/2017) and KaviKrishna USA Foundation, Lincoln, MA (BD) (KUF/2019-ASC-BD).
Conflict of Interest
The authors declare that the research was conducted in the absence of any commercial or financial relationships that could be construed as a potential conflict of interest.
Acknowledgments
The authors are grateful to the following clinicians and researchers for their extensive support and contributions that helped to formulate the hypothesis described here. They are the late G.N. Gyi, MS, FRCS, and B. R. Giri, MD, Mongar Hospital, Bhutan, Herman Yeger, PhD, Hospital for Sick Children, Toronto, Antonio Campos-Neto, Forsyth Institute, Boston, Dean W. Felsher, MD, PhD, Stanford University, Rituraj Kalita, PhD, Cotton University, PC Bhattacharya, MD, Naren Barman, MD and my colleagues from KaviKrishna laboratory and KaviKrishna Telemedicine Care.
References
1. Global tuberculosis report. Geneva: World Health Organization (2019). p. 1. Available at: https://apps.who.int/iris/bitstream/handle/10665/329368/9789241565714-eng.pdf.
2. Dubos R, Dubos J. The white plague: Tuberculosis, man, and society. 2nd ed. New Brunswick, N.J: Rutgers University Press (1987).
3. Hunter RL, Jagannath C, Actor JK. Pathology of post primary tuberculosis in humans and mice: the contradiction of long-held beliefs. Tuberc (Edinb) (2007) 87(4):267–78. doi: 10.1016/j.tube.2006.11.003
4. Medlar EM. The pathogenesis of minimal pulmonary tuberculosis; a study of 1,225 necropsies in cases of sudden and unexpected death. Am Rev Tuberc (1948) 58(6):583–611. doi: 10.1164/art.1948.58.6.583
5. Medlar EM. The behavior of pulmonary tuberculous lesions; a pathological study. Am Rev Tuberc (1955) 71(3):1–244.
7. Hunter RL. The pathogenesis of tuberculosis: The early infiltrate of Post-primary (Adult pulmonary) Tuberculosis: A Distinct Disease Entity. Front Immunol (2018) 9:2108. doi: 10.3389/fimmu.2018.02108
8. North RJ, Jung YJ. Immunity to tuberculosis. Annu Rev Immunol (2004) 22:599–623. doi: 10.1146/annurev.immunol.22.012703.104635
9. Gengenbacher M, Kaufmann SH. Mycobacterium tuberculosis: success through dormancy. FEMS Microbiol Rev (2012) 36(3):514–32. doi: 10.1111/j.1574-6976.2012.00331.x
10. Cheeseman EA. The age distribution of tuberculosis mortality in Northern Ireland. Ulster Med J (1952) 21(1):15–243.
11. Korzeniewska-Kosela M, Krysl J, Muller N, Black W, Allen E, FitzGerald JM. Tuberculosis in young adults and the elderly: A prospective comparison study. Chest (1994) 106(1):28–32. doi: 10.1378/chest.106.1.28
12. Donald PR, Marais BJ, Barry CE,3. Age and the epidemiology and pathogenesis of tuberculosis. Lancet (2010) 375(9729):1852–4. doi: 10.1016/S0140-6736(10)60580-6
13. Lawn SD, Acheampong JW. Pulmonary tuberculosis in adults: factors associated with mortality at a Ghanaian teaching hospital. West Afr J Med (1999) 18(4):270–4.
14. Ulrichs T, Kaufmann SH. New insights into the function of granulomas in human tuberculosis. J Pathol (2006) 208(2):261–9. doi: 10.1002/path.1906
15. Agarwal N, Lamichhane G, Gupta R, Nolan S, Bishai WR. Cyclic AMP intoxication of macrophages by a Mycobacterium tuberculosis adenylatecyclase. Nature (2009) 460(7251):98–102. doi: 10.1038/nature08123
16. Parrish NM, Dick JD, Bishai WR. Mechanisms of latency in Mycobacterium tuberculosis. Trends Microbio (1998) l6(3):107–12. doi: 10.1016/S0966-842X(98)01216-5
17. Russell DG. Who puts the tubercle in tuberculosis? Nat Rev Microbiol (2007) 5(1):39–47. doi: 10.1038/nrmicro1538
18. Huynh KK, Joshi SA, Brown EJ. A delicate dance: host response to mycobacteria. Curr Opin Immunol (2011) 23(4):464–72. doi: 10.1016/j.coi.2011.06.002
19. Silva Miranda M, Breiman A, Allain S, Deknuydt F, Altare F. The tuberculous granuloma: an unsuccessful host defence mechanism providing a safe shelter for the bacteria? Clin Dev Immunol (2012) 2012:139127. doi: 10.1155/2012/139127
20. Hunter RL. Pathology of post-primary tuberculosis of the lungs: an illustrated critical review. Tuberc (Edinb) (2011) 91(6):497–509. doi: 10.1016/j.tube.2011.03.007
21. Balasubramanian V, Wiegeshaus EH, Taylor BT, Smith DW. Pathogenesis of tuberculosis: a pathway to apical localization. Tuber Lungs Dis (1994) 75(3):168–78. doi: 10.1016/0962-8479(94)90002-7
22. Das B, Kashino SS, Pulu I, Kalita D, Swami V, Yeger H, et al. CD271(+) bone marrow mesenchymal stem cells may provide a niche for dormant Mycobacterium tuberculosis. Sci Transl Med (2013) 5(170):170ra13. doi: 10.1126/scitranslmed.3004912
23. Das B. The Science Behind Squalene: The Human Antioxidant. Toronto, Canada: Toronto Medical Pub., for the International Council for Bionutrient Research (2000).
24. Tornack J, Reece ST, Bauer WM, Vogelzang A, Bandermann S, Zedler U, et al. Human and Mouse Hematopoietic Stem Cells Are a Depot for Dormant Mycobacterium tuberculosis. PloS One (2017) 12(1):e0169119. doi: 10.1371/journal.pone.0169119
25. Fatima S, Kamble SS, Dwivedi VP, Bhattacharya D, Kumar S, Ranganathan A, et al. Mycobacterium tuberculosis programs mesenchymal stem cells to establish dormancy and persistence. J Clin Invest (2020) 130(2):655–61. doi: 10.1172/JCI128043
26. Singh VK, Mishra A, Bark S, Mani A, Subbian S, Hunter RL, et al. Human mesenchymal stem cell based intracellular dormancy model of Mycobacterium tuberculosis. Microbes Infect (2020) 22(9):423–31. doi: 10.1016/j.micinf.2020.05.015
27. Mayito J, Andia I, Belay M, Jolliffe DA, Kateete DP, Reece ST, et al. Anatomic and Cellular Niches for Mycobacterium tuberculosis in Latent Tuberculosis Infection. J Infect Dis (2019) 219(5):685–94. doi: 10.1093/infdis/jiy579
28. Pathak L, Gayan S, Pal B, Talukdar J, Bhuyan S, Sandhya S, et al. Corona virus activates a stem cell mediated defense mechanism that accelerates activation of dormant tuberculosis: implications for the COVID-19 pandemic. bioRxiv (2020) 2020.05.06.077883. doi: 10.1101/2020.05.06.077883
29. Das B, Pathak L, Gayan S, Pal B, Saikia P, Baishya T, et al. Stem cell basis of a host driven transmission of antigen packed aerosols: a novel mechanism of natural vaccination for tuberculosis. bioRxiv (2020) 2020:11.14.382572. doi: 10.1101/2020.11.14.382572
30. vanRie A, Warren R, Richardson M, Victor TC, Gie RP, Enarson DA, et al. Exogenous reinfection as a cause of recurrent tuberculosis after curative treatment. N Engl J Med (1999) 341(16):1174–9. doi: 10.1056/NEJM199910143411602
31. Canetti G, Robert M. Exogenous Reinfection in Healed Tuberculosis. Revue de la tuberculose (1950) 14(5-6):451–7.
32. Kalema N, Lindan C, Glidden D, Yoo SD, Katamba A, Alfred A, et al. Predictors and short-term outcomes of recurrent pulmonary tuberculosis, Uganda: a cohort study. S Afr Respir J (2017) 23(4):106–12.
33. Ziegler JE, Edwards ML, Smith DW. Exogenous reinfection in experimental airborne tuberculosis. Tubercle (1985) 66(2):121–8. doi: 10.1016/0041-3879(85)90077-7
34. Interrante JD, Haddad MB, Kim L, Gandhi NR. Exogenous Reinfection as a Cause of Late Recurrent Tuberculosis in the United States. Ann Am ThoracSoc (2015) 12(11):1619–26. doi: 10.1513/AnnalsATS.201507-429OC
35. Parvaresh L, Crighton T, Martinez E, Bustamante A, Chen S, Sintchenko V. Recurrence of tuberculosis in a low-incidence setting: a retrospective cross-sectional study augmented by whole genome sequencing. BMC Infect Dis (2018) 18(1):265. doi: 10.1186/s12879-018-3164-z
36. Zong Z, Huo F, Shi J, Jing W, Ma Y, Liang Q, et al. Relapse versus reinfection of recurrent tuberculosis patients in a national tuberculosis specialized hospital in Beijing, China. Front Microbiol (2018) 9:1858. doi: 10.3389/fmicb.2018.01858
37. Gomez JE, McKinney JD. M. tuberculosis persistence, latency, and drug tolerance. Tuberc (Edinb) (2004) 84(1-2):29–44. doi: 10.1016/j.tube.2003.08.003
38. Bloom BR, McKinney JD. The death and resurrection of tuberculosis. Nat Med (1999) 5(8):872–4. doi: 10.1038/11309
39. Young DB, Gideon HP, Wilkinson RJ. Eliminating latent tuberculosis. Trends Microbiol (2009) 17(5):183–8. doi: 10.1016/j.tim.2009.02.005
40. Collins FM. Mycobacterial disease, immunosuppression, and acquired immunodeficiency syndrome. Clin Microbiol Rev (1989) 2(4):360–77. doi: 10.1128/CMR.2.4.360
41. Russell DG, Cardona PJ, Kim MJ, Allain S, Altare F. Foamy macrophages and the progression of the human tuberculosis granuloma. Nat Immunol (2009) 10(9):943–8. doi: 10.1038/ni.1781
42. Ramakrishnan L. Revisiting the role of the granuloma in tuberculosis. Nat Rev Immunol (2012) 12(5):352–66. doi: 10.1038/nri3211
43. Barry CE,3, Boshoff HI, Dartois V, Dick T, Ehrt S, Flynn J, et al. The spectrum of latent tuberculosis: rethinking the biology and intervention strategies. Nat Rev Microbiol (2009) 7(12):845–55. doi: 10.1038/nrmicro2236
44. Gupta A, Kaul A, Tsolaki AG, Kishore U, Bhakta S. Mycobacterium tuberculosis: immune evasion, latency and reactivation. Immunobiology (2012) 217(3):363–74. doi: 10.1016/j.imbio.2011.07.008
45. Rustad TR, Sherrid AM, Minch KJ, Sherman DR. Hypoxia: a window into Mycobacterium tuberculosis latency. Cell Microbiol (2009) 11(8):1151–9. doi: 10.1111/j.1462-5822.2009.01325.x
46. Wayne LG, Hayes LG. An in vitro model for the sequential study of shift down of Mycobacterium tuberculosis through two stages of nonreplicating persistence. Infect Immun (1996) 64(6):2062–9. doi: 10.1128/IAI.64.6.2062-2069.1996
47. Stewart GR, Robertson BD, Young DB. Tuberculosis: a problem with persistence. Nat Rev Microbiol (2003) 1(2):97–105. doi: 10.1038/nrmicro749
48. Remot A, Doz E, Winter N. Neutrophils and Close Relatives in the Hypoxic Environment of the Tuberculous Granuloma: New Avenues for Host-Directed Therapies? Front Immunol (2019) 12(10):417. doi: 10.3389/fimmu.2019.00417
49. Via LE, Lin PL, Ray SM, Carrillo J, Allen SS, Eum SY, et al. Tuberculous granulomas are hypoxic in guinea pigs, rabbits, and nonhuman primates. Infect Immun (2008) 76(6):2333–40. doi: 10.1128/IAI.01515-07
50. Gunn F. Tuberculosis. In: Anderson WAD, editor. Pathology, 4th ed. St Louis, MI: C. V. Mosby Company (1961). p. 243–63.
51. Loomis HP. Some facts in the etiology of tuberculosis, evidenced by thirty autopsies and experiments upon animals. Med Record (1890) 38:689–98.
52. Aronson D, Caroline WE. The Types of Tubercle Bacilli Found in Tuberculous Lesions and in Non tuberculous Tissue in Man. J Infect Dis (1930) 47(1):30–55. doi: 10.1093/infdis/47.1.30
53. Hernandez-Pando R, Jeyanathan M, Mengistu G, Aguilar D, Orozco H, Harboe M, et al. Persistence of DNA from Mycobacterium tuberculosis in superficially normal lungs tissue during latent infection. Lancet (2000) 356(9248):2133–8. doi: 10.1016/S0140-6736(00)03493-0
54. Biketov S, Mukamolova GV, Potapov V, Gilenkov E, Vostroknutova G, Kell DB, et al. Culturability of Mycobacterium tuberculosis cells isolated from murine macrophages: a bacterial growth factor promotes recovery. FEMS Immunol Med Microbiol (2000) 29(4):233–40. doi: 10.1111/j.1574-695X.2000.tb01528.x
55. Neyrolles O, Hernandez-Pando R, Pietri-Rouxel F, Fornes P, Tailleux L, Barrios Payan JA, et al. Is adipose tissue a place for Mycobacterium tuberculosis persistence? PLoS One (2006) 1(1):e43. doi: 10.1371/journal.pone.0000043
56. Garhyan J, Bhuyan S, Pulu I, Kalita D, Das B, Bhatnagar R. Preclinical and Clinical Evidence of Mycobacterium tuberculosis Persistence in the Hypoxic Niche of Bone Marrow Mesenchymal Stem Cells after Therapy. Am J Pathol (2015) 185(7):1924–34. doi: 10.1016/j.ajpath.2015.03.028
57. Beamer G, Major S, Das B, Campos-Neto A. Bone marrow mesenchymal stem cells provide an antibiotic-protective niche for persistent viable Mycobacterium tuberculosis that survive antibiotic treatment. Am J Pathol (2014) 184(12):3170–5. doi: 10.1016/j.ajpath.2014.08.024
58. Charrier S, Boiret N, Fouassier M, Berger J, Rapatel C, Pigeon P, et al. Normal human bone marrow CD34(+)CD133(+) cells contain primitive cells able to produce different categories of colony-forming unit megakaryocytes in vitro. ExpHematol (2002) 30(9):1051–60. doi: 10.1016/S0301-472X(02)00882-2
59. Hunter RL, Actor JK, Hwang SA, Karev V, Jagannath C. Pathogenesis of post primary tuberculosis: immunity and hypersensitivity in the development of cavities. Ann Clin Lab Sci (2014) 44(4):365–87.
60. Zimmermann M, Kogadeeva M, Gengenbacher M, McEwen G, Mollenkopf HJ, Zamboni N, et al. Integration of Metabolomics and Transcriptomics Reveals a Complex Diet of Mycobacterium tuberculosis during Early Macrophage Infection. mSystems (2017) 2(4):pii: e00057–17. doi: 10.1128/mSystems.00057-17
61. Mariotti S, Pardini M, Gagliardi MC, Teloni R, Giannoni F, Fraziano M, et al. Dormant Mycobacterium tuberculosis fails to block phagosome maturation and shows unexpected capacity to stimulate specific human T lymphocytes. J Immunol (2013) 191(1):274–82. doi: 10.4049/jimmunol.1202900
62. Flynn JL, Chan J, Triebold KJ, Dalton DK, Stewart TA, Bloom BR. An essential role for interferon gamma in resistance to Mycobacterium tuberculosis infection. J Exp Med (1993) 178(6):2249–54. doi: 10.1084/jem.178.6.2249
63. Traag BA, Driks A, Stragier P, Bitter W, Broussard G, Hatfull G, et al. Do mycobacteria produce endospores? Proc Natl Acad Sci USA (2010) 107(2):878–81. doi: 10.1073/pnas.0911299107
64. Aria F, Suda T. Quiescent stem cells in the niche. Cambridge, MA: Harvard Stem Cell Institute (2008).
65. Fujisaki J, Wu J, Carlson AL, Silberstein L, Putheti P, Larocca R, et al. In vivo imaging of Treg cells providing the immune privilege to the hematopoietic stem-cell niche. Nature (2011) 474(7350):216–9. doi: 10.1038/nature10160
66. Tormin A, Li O, Brune JC, Walsh S, Schutz B, Ehinger M, et al. CD146 expression on primary nonhematopoietic bone marrow stem cells is correlated with in situ localization. Blood (2011) 117(19):5067–77. doi: 10.1182/blood-2010-08-304287
67. Jones DL, Wagers AJ. No place like home: anatomy and function of the stem cell niche. Nat Rev Mol Cell Biol (2008) 9(1):11–21. doi: 10.1038/nrm2319
68. Jones E, McGonagle D. Human bone marrow mesenchymal stem cells in vivo. Rheumatol (Oxford) (2008) 47(2):126–31. doi: 10.1093/rheumatology/kem206
69. Kubota Y, Takubo K, Suda T. Bone marrow long label-retaining cells reside in the sinusoidal hypoxic niche. Biochem Biophys Res Commun (2008) 366(2):335–9. doi: 10.1016/j.bbrc.2007.11.086
70. Larochelle A, Vormoor J, Hanenberg H, Wang JC, Bhatia M, Lapidot T, et al. Identification of primitive human hematopoietic cells capable of repopulating NOD/SCID mouse bone marrow: implications for gene therapy. Nat Med (1996) 2(12):1329–37. doi: 10.1038/nm1296-1329
71. Danet GH, Pan Y, Luongo JL, Bonnet DA, Simon MC. Expansion of human SCID-repopulating cells under hypoxic conditions. J Clin Invest (2003) 112(1):126–35. doi: 10.1172/JCI17669
72. Das B, Tsuchida R, Baruchel S, Malkin DYH. The Idea and Evidence for the Tumor Stemness Switch. Rajasekhar VK, Vemuri MC, editors. New Jersey, United States: Regul Networks Stem Cells Stem Cell Biol Regen Med Humana Press (2009). doi: 10.1007/978-1-60327-227-8_35
73. Pal B, Das B. In vitro Culture of Naïve Human Bone Marrow Mesenchymal Stem Cells: A Stemness Based Approach. Front Cell Dev Biol (2017) 23(5):69. doi: 10.3389/fcell.2017.00069
74. Grayson WL, Zhao F, Bunnell B, Ma T. Hypoxia enhances proliferation and tissue formation of human mesenchymal stem cells. Biochem Biophys Res Commun (2007) 358(3):948–53. doi: 10.1016/j.bbrc.2007.05.054
75. Parmar K, Mauch P, Vergilio JA, Sackstein R, Down JD. Distribution of hematopoietic stem cells in the bone marrow according to regional hypoxia. Proc Natl Acad Sci USA (2007) 104(13):5431–6. doi: 10.1073/pnas.0701152104
76. Kumar A, Toledo JC, Patel RP, Lancaster JR Jr, Steyn AJ. Mycobacterium tuberculosis DosS is a redox sensor and DosT is a hypoxia sensor. Proc Natl Acad Sci USA (2007) 104:11568e11573. doi: 10.1073/pnas.0705054104
77. Wayne LG, Sohaskey CD. Nonreplicating persistence of mycobacterium tuberculosis. Annu Rev Microbiol (2001) 55:139e163. doi: 10.1146/annurev.micro.55.1.139
78. Masaki T, Qu J, Cholewa-Waclaw J, Burr K, Raaum R, Rambukkana A. Reprogramming adult Schwann cells to stem cell-like cells by leprosy bacilli promotes dissemination of infection. Cell (2013) 152(1-2):51–67. doi: 10.1016/j.cell.2012.12.014
79. Frydman HM, Li JM, Robson DN, Wieschaus E. Somatic stem cell niche tropism in Wolbachia. Nature (2006) 441(7092):509–12. doi: 10.1038/nature04756
80. Hess DA, Wirthlin L, Craft TP, Herrbrich PE, Hohm SA, Lahey R, et al. Selection based on CD133 and high aldehyde dehydrogenase activity isolates long-term reconstituting human hematopoietic stem cells. Blood (2006) 107(5):2162–9. doi: 10.1182/blood-2005-06-2284
81. Tondreau T, Meuleman N, Delforge A, Dejeneffe M, Leroy R, Massy M, et al. Mesenchymal stem cells derived from CD133-positive cells in mobilized peripheral blood and cord blood: proliferation, Oct4 expression, and plasticity. Stem Cells (2005) 23(8):1105–12. doi: 10.1634/stemcells.2004-0330
82. Buhring HJ, Battula VL, Treml S, Schewe B, Kanz L, Vogel W. Novel markers for the prospective isolation of human MSC. Ann N Y Acad Sci (2007) 1106:262–71. doi: 10.1196/annals.1392.000
83. Churchman SM, Ponchel F, Boxall SA, Cuthbert R, Kouroupis D, Roshdy T, et al. Transcriptional profile of native CD271+ multipotential stromal cells: evidence for multiple fates, with prominent osteogenic and Wnt pathway signaling activity. Arthritis Rheum (2012) 64(8):2632–43. doi: 10.1002/art.34434
84. Kuci S, Kuci Z, Kreyenberg H, Deak E, Putsch K, Huenecke S, et al. CD271 antigen defines a subset of multipotent stromal cells with immunosuppressive and lymphohematopoietic engraftment-promoting properties. Haematologica (2010) 95(4):651–9. doi: 10.3324/haematol.2009.015065
85. Alvarez-Viejo M, Menendez-Menendez Y, Blanco-Gelaz MA, Ferrero-Gutierrez A, Fernandez-Rodriguez MA, Gala J, et al. Quantifying mesenchymal stem cells in the mononuclear cell fraction of bone marrow samples obtained for cell therapy. Transplant Proc (2013) 45(1):434–9. doi: 10.1016/j.transproceed.2012.05.091
86. Iso Y, Yamaya S, Sato T, Poole CN, Isoyama K, Mimura M, et al. Distinct mobilization of circulating CD271+ mesenchymal progenitors from hematopoietic progenitors during aging and after myocardial infarction. Stem Cells Transl Med (2012) 1(6):462–8. doi: 10.5966/sctm.2011-0051
87. Qian H, Le Blanc K, Sigvardsson M. Primary Mesenchymal Stem and Progenitor Cells from Bone Marrow Lack Expression of CD44. J Biol Chem (2012) 287:25796–807. doi: 10.1074/jbc.M112.339622
88. Haimeur A, Conseil G, Deeley RG, Cole SP. The MRP-related and BCRP/ABCG2 multidrug resistance proteins: biology, substrate specificity and regulation. Curr Drug Metab (2004) 5(1):21–53. doi: 10.2174/1389200043489199
89. Suzuki M, Suzuki H, Sugimoto Y, Sugiyama Y. ABCG2 transports sulfated conjugates of steroids and xenobiotics. J Biol Chem (2003) 278(25):22644–9. doi: 10.1074/jbc.M212399200
90. Naik SK, Padhi A, Ganguli G, Sengupta S, Pati S, Das D, et al. Mouse Bone Marrow Sca-1+ CD44+ Mesenchymal Stem Cells Kill Avirulent Mycobacteria but Not Mycobacterium tuberculosis through Modulation of Cathelicidin Expression via the p38 Mitogen-Activated Protein Kinase-Dependent Pathway. Infect Immun (2017) 85(10):e00471–17. doi: 10.1128/IAI.00471-17
91. Jamwal SV, Mehotra P, Singh A, Siddiqui Z, Basu A, Rao KV. Mycobacterial escape from macrophage phagosomes to the cytoplasm represents an alternate adaptation mechanism. Sci Rep (2016) 6:23089. doi: 10.1038/srep23089
92. Khan A, Mann L, Papanna R, Lyu MA, Singh CR, Olson S, et al. Mesenchymal stem cells internalize Mycobacterium tuberculosis through scavenger receptors and restrict bacterial growth through autophagy. Sci Rep (2017) 7(1):15010. doi: 10.1038/s41598-017-15290-z
93. Bogdan C. Nitric oxide synthase in innate and adaptive immunity: an update. Trends Immunol (2015) 36(3):161–78. doi: 10.1016/j.it.2015.01.003
94. Yang K, Wu Y, Xie H, Li M, Ming S, Li L, et al. Macrophage-mediated inflammatory response decreases mycobacterial survival in mouse MSCs by augmenting NO production. Sci Rep (2016) 6:27326. doi: 10.1038/srep27326
95. Khan A, Hunter RL, Jagannath C. Emerging role of mesenchymal stem cells during tuberculosis: The fifth element in cell mediated immunity. Tuberc (Edinb) (2016) 101S:S45–52. doi: 10.1016/j.tube.2016.09.019
96. Jain N, Kalam H, Singh L, Sharma V, Kedia S, Das P, et al. Mesenchymal stem cells offer a drug-tolerant and immune-privileged niche to Mycobacterium tuberculosis. Nat Commun (2020) 11(1):3062. doi: 10.1038/s41467-020-16877-3
97. Kohli S, Singh Y, Sowpati DT, Ehtesham NZ, Dobrindt U, Hacker J, et al. Human mesenchymal stem cells: New sojourn of bacterial pathogens. Int J Med Microbiol (2015) 305(3):322–6. doi: 10.1016/j.ijmm.2015.01.001
98. Reece ST, Vogelzang A, Tornack J, Bauer W, Zedler U, Schommer-Leitner S, et al. Mycobacterium tuberculosis-Infected Hematopoietic Stem and Progenitor Cells Unable to Express Inducible Nitric Oxide Synthase Propagate Tuberculosis in Mice. J Infect Dis (2018) 217(10):1667–71. doi: 10.1093/infdis/jiy041
99. Khan N, Downey J, Sanz J, Kaufmann E, Blankenhaus B, Pacis A, et al. M. tuberculosis Reprograms Hematopoietic Stem Cells to Limit Myelopoiesis and Impair Trained Immunity. Cell (2020) 183(3):752–70.e22. doi: 10.1016/j.cell.2020.09.062
100. Ema H. Two anatomically distinct niches regulate stem cell activity. Blood (2012) 120(11):2174–81. doi: 10.1182/blood-2012-04-424507
101. Rizvi AZ, Wong MH. Epithelial stem cells and their niche: there’s no place like home. Stem Cells (2005) 23(2):150–65. doi: 10.1634/stemcells.2004-0096
102. Fu X, Liu G, Halim A, Ju Y, Luo Q, Song G. Mesenchymal Stem Cell Migration and Tissue Repair. Cells (2019) 8(8):784. doi: 10.3390/cells8080784
103. Lo Celso C, Scadden DT. The hematopoietic stem cell niche at a glance. J Cell Sci (2011) 124(Pt 21):3529–35. doi: 10.1242/jcs.074112
104. Whetton AD, Graham GJ. Homing and mobilization in the stem cell niche. Trends Cell Biol (1999) 9(6):233–8. doi: 10.1016/S0962-8924(99)01559-7
105. He XC, Li Z, Sugimura R, Ross J, Zhao M, Li L. Homing and migration assays of hematopoietic stem/progenitor cells. Methods Mol Biol (2014) 1185:279–84. doi: 10.1007/978-1-4939-1133-2_19
106. Crosby LM, Waters CM. Epithelial repair mechanisms in the lungs. Am J Physiol Lungs Cell Mol Physiol (2010) 298(6):L715–31. doi: 10.1152/ajplung.00361.2009
107. Sage EK, Loebinger MR, Polak J, Janes SM. The role of bone marrow-derived stem cells in lungs regeneration and repair. In: Bhatia S, editor. StemBook Cambridge (MA): Stembook, Harvard stem cell institute (2008). https://www.ncbi.nlm.nih.gov/books/NBK27055/
108. Spaeth EL, Kidd S, Marini FC. Tracking inflammation-induced mobilization of mesenchymal stem cells. Methods MolBiol (2012) 904:173–90. doi: 10.1007/978-1-61779-943-3_15
109. MahdaviGorabi A, Banach M, Reiner Ž, Pirro M, Hajighasemi S, Johnston TP, et al. The Role of Mesenchymal Stem Cells in Atherosclerosis: Prospects for Therapy via the Modulation of Inflammatory Milieu. J Clin Med (2019) 8(9):pii: E1413. doi: 10.3390/jcm8091413
110. Gójska-Grymajło A, Zieliński M, Gąsecki D, Kowalczyk K, Kwarciany M, Seroczyńska B, et al. CD271+, CXCR7+, CXCR4+, and CD133+ Stem/Progenitor Cells and Clinical Characteristics of Acute Ischemic Stroke Patients. Neuromolecular Med (2018) 20(3):301–11. doi: 10.1007/s12017-018-8494-x
111. Mozid AM, Jones D, Arnous S, Saunders N, Wragg A, Martin J, et al. The effects of age, disease state, and granulocyte colony-stimulating factor on progenitor cell count and function in patients undergoing cell therapy for cardiac disease. Stem Cells Dev (2013) 22(2):216–23. doi: 10.1089/scd.2012.0139
112. Das B, Bayat-Mokhtari R, Tsui M, Lotfi S, Tsuchida R, Felsher DW, et al. HIF-2α suppresses p53 to enhance the stemness and regenerative potential of human embryonic stem cells. Stem Cells (2012) 30(8):1685–95. doi: 10.1002/stem.1142
113. Das B. Altruistic stem cell and cancer stem cells. In: Rajasekhar VK, editor. Cancer stem cells. Hoboken, NJ: Wiley Press (2014). p. 89–105. doi: 10.1002/9781118356203.ch7
114. Di Gregorio A, Bowling S, Rodriguez TA. Cell Competition and Its Role in the Regulation of Cell Fitness from Development to Cancer. Dev Cell (2016) 38(6):621–34. doi: 10.1016/j.devcel.2016.08.012
115. Ehlers S. Lazy, dynamic or minimally recrudescent? On the elusive nature and location of the mycobacterium responsible for latent tuberculosis. Infection (2009) 37:87–95. doi: 10.1007/s15010-009-8450-7
116. Rosser A, Stover C, Pareek M, Mukamolova GV. Resuscitation-promoting factors are important determinants of the pathophysiology in Mycobacterium tuberculosis infection. Crit Rev Microbiol (2017) 43:621–30. doi: 10.1080/1040841X.2017.1283485
117. Sia IG, Wieland ML. Current concepts in the management of tuberculosis. Mayo Clin Proc (2011) 86(4):348–61. doi: 10.4065/mcp.2010.0820
118. Dusthackeer A, Balasubramanian M, Shanmugam G, Priya S, Nirmal CR, Sam Ebenezer R, et al. Differential Culturability of Mycobacterium tuberculosis in Culture-Negative Sputum of Patients With Pulmonary Tuberculosis and in a Simulated Model of Dormancy. Front Microbiol (2019) 10:2381. doi: 10.3389/fmicb.2019.02381
119. Garton NJ, Waddell SJ, Sherratt AL, Lee S-M, Smith RJ, Senner C, et al. Cytological and transcript analyses reveal fat and lazy persister-like bacilli in tuberculous sputum. PLoS Med (2008) 5(4):e75. doi: 10.1371/journal.pmed.0050075
120. Guirado E, Schlesinger LS. Modeling the Mycobacterium tuberculosis Granuloma - the Critical Battlefield in Host Immunity and Disease. Front Immunol (2013) 4:98. doi: 10.3389/fimmu.2013.00098
121. Raghuvanshi S, Sharma P, Singh S, Van Kaer L, Das G. Mycobacterium tuberculosis evades host immunity by recruiting mesenchymal stem cells. Proc Natl Acad Sci USA (2010) 107(50):21653–8. doi: 10.1073/pnas.1007967107
122. Kashino SS, Ovendale P, Izzo A, Campos-Neto A. Unique model of dormant infection for tuberculosis vaccine development. Clin Vaccine Immunol (2006) 13(9):1014–21. doi: 10.1128/CVI.00120-06
123. De Albuquerque N, Baig E, Ma X, Zhang J, He W, Rowe A, et al. Murine hepatitis virus strain 1 produces a clinically relevant model of severe acute respiratory syndrome in A/J mice. J Virol (2006) 80(21):10382–94. doi: 10.1128/JVI.00747-06
124. Abad CLR, Razonable RR. An update on Mycobacterium tuberculosis infection after hematopoietic stem cell transplantation in adults. Clin Transpl (2018) 32(12):e13430. doi: 10.1111/ctr.13430
125. Chakrabarti B, Calverley PM, Davies PD. Tuberculosis and its incidence, special nature, and relationship with chronic obstructive pulmonary disease. Int J Chron Obstruct Pulmon Dis (2007) 2(3):263–72.
126. Salvi SS, Barnes PJ. Chronic obstructive pulmonary disease in non-smokers. Lancet (2009) 374(9691):733–43. doi: 10.1016/S0140-6736(09)61303-9
127. Eisner MD, Anthonisen N, Coultas D, Kuenzli N, Perez-Padilla R, Postma D, et al. An official American Thoracic Society public policy statement: Novel risk factors and the global burden of chronic obstructive pulmonary disease. Am J Respir Crit Care Med (2010) 182(5):693–718. doi: 10.1164/rccm.200811-1757ST
128. Liang D, Shi L, Zhao J, Liu P, Schwartz J, Gao S, et al. Urban Air Pollution May Enhance COVID-19 Case-Fatality and Mortality Rates in the United States. medRxiv: preprint server Health Sci (2020). https://www.medrxiv.org/content/10.1101/2020.05.04.20090746v1 doi: 10.1101/2020.05.04.20090746
129. Jordan TS, Spencer EM, Davies P. Tuberculosis, bronchiectasis, and chronic airflow obstruction. Respirology (2010) 15(4):623–8. doi: 10.1111/j.1440-1843.2010.01749.x
130. Ehrlich RI, Adams S, Baatjies R, Jeebhay MF. Chronic airflow obstruction and respiratory symptoms following tuberculosis: a review of South African studies. Int J Tuberc Lungs Dis (2011) 15(7):886–91. doi: 10.5588/ijtld.10.0526
131. Chandrasekaran P, Saravanan N, Bethunaickan R, Tripathy S. Malnutrition: Modulator of Immune Responses in Tuberculosis. Front Immunol (2017) 8:1316. doi: 10.3389/fimmu.2017.01316
132. Nombela-Arrieta C, Isringhausen S. The Role of the Bone Marrow Stromal Compartment in the Hematopoietic Response to Microbial Infections. Front Immunol (2016) 7:689. doi: 10.3389/fimmu.2016.00689
133. Baldridge MT, King KY, Boles NC, Weksberg DC, Goodell MA. Quiescent haematopoietic stem cells are activated by IFN-gamma in response to chronic infection. Nature (2010) 465(7299):793–7. doi: 10.1038/nature09135
134. Kaufmann E, Sanz J, Dunn JL, Khan N, Mendonça LE, Pacis A, et al. BCG Educates Hematopoietic Stem Cells to Generate Protective Innate Immunity against Tuberculosis. Cell (2018) 172(1-2):176–90.e19. doi: 10.1016/j.cell.2017.12.031
135. Caño-Muñiz S, Anthony R, Niemann S, Alffenaar JC. New Approaches and Therapeutic Options for Mycobacterium tuberculosis in a Dormant State. Clin Microbiol Rev (2017) 31(1):pii: e00060–17. doi: 10.1128/CMR.00060-17
136. Kurtz J, Franz K. Innate defence: evidence for memory in invertebrate immunity. Nature (2003) 425(6953):37–8. doi: 10.1038/425037a
137. Tribouley J, Tribouley-Duret J, Appriou M. [Effect of Bacillus Callmette Guerin (BCG) on the receptivity of nude mice to Schistosoma mansoni]. C R Seances Soc Biol Fil (1978) 172(5):902–4.
138. Netea MG, Quintin J, van der Meer JWM. Trained immunity: a memory for innate host defense. Cell Host Microbe (2011) 9(5):355–61. doi: 10.1016/j.chom.2011.04.006
139. Kleinnijenhuis J, Quintin J, Preijers F, Joosten LAB, Ifrim DC, Saeed S, et al. Bacille Calmette-Guerin induces NOD2-dependent nonspecific protection from reinfection via epigenetic reprogramming of monocytes. Proc Natl Acad Sci U S A (2012) 109(43):17537–42. doi: 10.1073/pnas.1202870109
140. Venkatasubramanian S, Cheekatla S, Paidipally P, Tripathi D, Welch E, Tvinnereim AR, et al. IL-21-dependent expansion of memory-like NK cells enhances protective immune responses against Mycobacterium tuberculosis. Mucosal Immunol (2017) 10(4):1031–42. doi: 10.1038/mi.2016.105
141. Dorhoi A, Reece ST, Kaufmann SHE. For better or for worse: the immune response against Mycobacterium tuberculosis balances pathology and protection. Immunol Rev (2011) 240(1):235–51. doi: 10.1111/j.1600-065X.2010.00994.x
142. Ernst JD. The immunological life cycle of tuberculosis. Nat Rev Immunol (2012) 12(8):581–91. doi: 10.1038/nri3259
Keywords: post-primary tuberculosis of the lungs, dormancy, reactivation, stem cell niche, bone marrow derived stem cells, altruistic stem cells, Mycobacterium tuberculosis
Citation: Pathak L and Das B (2021) Initiation of Post-Primary Tuberculosis of the Lungs: Exploring the Secret Role of Bone Marrow Derived Stem Cells. Front. Immunol. 11:594572. doi: 10.3389/fimmu.2020.594572
Received: 28 August 2020; Accepted: 03 December 2020;
Published: 21 January 2021.
Edited by:
Francesca Di Rosa, Italian National Research Council, ItalyReviewed by:
Kerry L. Hilligan, National Institutes of Health (NIH), United StatesMaria Cristina Gagliardi, National Institute of Health (ISS), Italy
Copyright © 2021 Pathak and Das. This is an open-access article distributed under the terms of the Creative Commons Attribution License (CC BY). The use, distribution or reproduction in other forums is permitted, provided the original author(s) and the copyright owner(s) are credited and that the original publication in this journal is cited, in accordance with accepted academic practice. No use, distribution or reproduction is permitted which does not comply with these terms.
*Correspondence: Bikul Das, YmRhc0BrYXZpa3Jpc2huYWxhYi5vcmc=