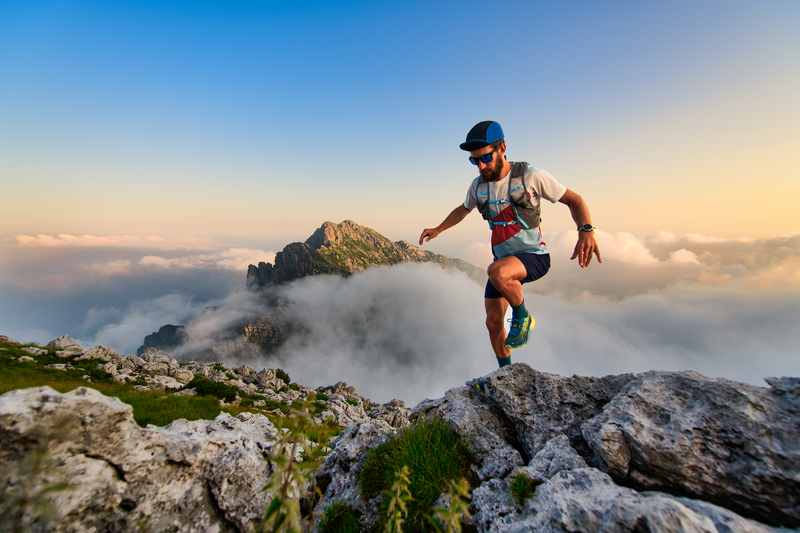
94% of researchers rate our articles as excellent or good
Learn more about the work of our research integrity team to safeguard the quality of each article we publish.
Find out more
HYPOTHESIS AND THEORY article
Front. Immunol. , 08 December 2020
Sec. Nutritional Immunology
Volume 11 - 2020 | https://doi.org/10.3389/fimmu.2020.590685
This article is part of the Research Topic Beyond Probiotics: Dietary Microbial Modulators of the Immune System - Effects and Mechanisms View all 12 articles
Converging evidences showed that people with diabetes mellitus (DM) have significantly higher risk for different cancers, of which the exact mechanism underlying the association has not been fully realized. Short-chain fatty acids (SCFAs), the fermentation products of the intestinal microbiota, are an essential source for energy supply in gut epithelial cells. They have been reported to improve intestinal barrier integrity, prevent microbial translocation, and further dampen inflammation. Gut dysbiosis and reduction in SCFA-producing bacteria as well as SCFAs production in the intestine are commonly seen in metabolic disorders including DM and obesity. Moreover, inflammation can contribute to tumor initiation and progression through multiple pathways, such as enhancing DNA damage, accumulating mutations in tumor suppressor genes Tp53, and activating nuclear factor-kappa B (NF-κB) signaling pathways. Based on these facts, we hypothesize that lower levels of microbial SCFAs resulted from gut dysbiosis in diabetic individuals, enhance microbial translocation, and increase the inflammatory responses, inducing tumorigenesis ulteriorly. To this end, we will discuss protective properties of microbial SCFAs and explore the pivotal roles SCFAs played in the link of DM with cancer, so as to take early precautions to reduce the risk of cancer in patients with DM.
Diabetes mellitus (DM) is characterized by different metabolic abnormalities, including hyperglycemia, insulin secretion deficiency, insulin dysfunction, and energy metabolism disturbances (1, 2). The World Health Organization (WHO) reported about 422 million people have DM in the world. Morbidity and prevalence of DM have been steadily rising over the past few decades. Aside from DM, cancer ranks as the second main cause of death in the developed world and the third cause for death in developing nations (3). DM and cancer share several common characteristics, such as microbial imbalance and bacterial translocation (4–6). Unfortunately, these two diseases are frequently coexistent and can affect each other, worsening the prognosis of patients.
Generally compared with non-diabetic people, the incidence of cancer in diabetic patients increased by approximately 20–25%, depending on specific cancer type and site (7, 8), which has been observed in both Asian and Western populations (9). People with diabetes accompanied by cancer also experienced higher mortality risk, in contrast with the group with only cancer (10). Practically speaking, when comparing to the general population, the significantly increased risk of cancer in diabetic individuals has been proved in hepatocellular and pancreatic carcinomas, which were 2.5 and 1.94 times higher respectively (11, 12). Friberg et al. demonstrated that in diabetic women, the risk for endometrial cancer nearly doubled (13). The epidemiological literature indicates that patients with DM are at modestly increased risk (by 19–42%) of developing colorectal, gastric, kidney, breast, and bladder cancers (14–16). Numerous studies have also declared the positive associations of DM with non-Hodgkin lymphoma, leukemia, and myeloma, which is about 19–22% higher in patients with only type II DM (17–19). Regarding type I DM cohorts, in comparison with the non-diabetics, increased risk of several cancers might be reported in multiple research cohorts, yet inconsistent if all studies are considered (20–22).
However, regarding the existing correlation of DM with cancer, the mechanisms remain to be established. Identifying and utilizing the intermediate links between the two diseases are essential to reduce the risk of cancer and improve the symptoms of DM. Short-chain fatty acids (SCFAs), as functional microbial metabolites (23), might be a possibility to unlock the tie. Herein, we will go further behind this correlation and focus on SCFAs, which may be involved in the progress of DM developing into cancer.
Based on recent evidences, we hypothesize that lower abundance of SCFAs resulted from gut microbial dysbiosis in diabetics could induce the destruction of the gut mucosal barrier, increase microbial translocation, and promote inflammation responses, further boosting inflammatory-malignant transformation and enhancing risk of tumorigenesis (Figure 1).
Figure 1 Flowcharts representing the association of diabetes, short-chain fatty acids (SCFAs), inflammation, and tumor. Due to gut microbiota dysbiosis in people with diabetes, both SCFAs-producing bacteria and the produced SCFAs decreased. Reduction in SCFAs could weaken anti-inflammatory responses in diabetics through diverse mechanisms, such as increasing gut permeability, inducing microbial translocation, and attenuating binding capabilities of SCFAs to both GPCRs and HDAC. Hence, the inflammation was exacerbated in diabetic patients. Increased inflammation may lead to tumorigenesis in normal cells through accumulated genetic mutations, which proceeded from DNA damage. Moreover, various inflammatory immune cells can secrete abundant inflammatory mediators such as cytokines, chemokines, and growth factors, which may activate key transcription factors, including NF-κB and STAT3. This could initiate tumor growth in normal cells, or boost malignant processes in tumor cells including angiogenesis, dissemination, and invasion. In turn, inflammatory reactions were aggravated under sustained tumor-associated inflammation, thus forming a vicious and positive feedback loop between inflammation and tumor.
The intestinal barrier is the functional defense line, consisting of microbiota, intestinal epithelial cells (IECs), and mucosal immunity (24). The gut microbiota are collectively referred to as eukaryotes, archaea, and bacteria, among which bacterial phyla are the most abundant (25). Investigations identified that keeping the diversity and quantity of human gut microbiota, which can protect the host against pathogens by competition for niches and nutritive supply, improvement of immune functions, and regulation of metabolism, is of critical importance in maintaining intestinal homeostasis (26, 27). Akkermansia muciniphila was reported to improve intestinal epithelial monolayer integrity by stimulating colonic mucin secretion, increasing the thickness of the gut mucus layer, and binding directly to intestinal cells (28, 29). On the contrary, dysregulation of gut microbiota composition, also called dysbiosis, could impair the balance between the commensal species and various pathogens, as well as decrease the release of metabolic SCFAs and antimicrobial molecules such as bacteriocins (30). Depleted Firmicutes and increased Proteobacteria such as Enterobacter cloacae and Enterobacter species contribute to the disruption of IECs, which in turn induces the translocation of intestinal microbiota and their toxins, leading to infectious threats (31, 32). Dysbiosis may also regulate IECs to release intestinal miRNAs, which could orchestrate the immune responses via TLR dependent pathways or PRR families to fight against pathogens (33). Other than SCFAs and bacterial toxins, microbiota might influence the intestinal barrier through other signal pathways, including bile acids metabolism and endocannabinoid system (34, 35).
Emerging evidences indicated DM is significantly correlated with gut microbiota dysbiosis, which harms the integrity of the gut wall and promotes the shift of endotoxemia from the intestinal cavity into the circulatory system, hence triggering inflammation, autoimmune responses, and oxidative stress (36). Larsen et al. demonstrated that microbial dysbiosis could provoke changes in composition and distribution of gut microorganisms, particularly intestinal bacterial species in the mucosa, and metabolic activities (37). Compared to non-diabetics, Lactobacillus spp and Betaproteobacteria groups were highly enriched in diabetics, which was positively connected to plasma glucose and could trigger inflammation effect in DM (38–40). Considerable studies indicated that the abundance of Akkermansia muciniphila, Firmicutes, and Clostridium spp was significantly decreased among individuals with DM, inducing unfavorable effects on nutrient metabolic control, glucose tolerance, and inflammation responses (41–43). In addition, the diabetics possessed lower abundance of SCFA-producing organisms, including Roseburia intestinalis and Faecalibacterium prausnitzii, which led to reduced anti-inflammatory SCFAs levels, especially butyrate (37, 44, 45). Similar results have been observed in animal studies describing that the levels of SCFA-producing bacteria and SCFA production declined remarkably in diabetic mice versus the diabetes-free group (46, 47). Deficiency in SCFA production could promote intestine microbes to spread into the systemic circulation, thereby inducing or aggravating systemic inflammatory responses (48, 49).
Notably, intestinal SCFA producers and their metabolites SCFAs are reported to play crucial roles in the pathophysiology of DM. Compared to healthy controls, type 1 diabetes mellitus (T1DM) patients harbored decreased population of SCFA-producing bacteria and circulating SCFAs, in line with markedly decreased genes contributing to SCFAs synthesis, which is associated with disturbed microbiota composition (50–52). Gut metagenome data also revealed that subjects with type 2 diabetes mellitus (T2DM) were characterized by a decrease in SCFA-producing microbiota and SCFA abundance, issued from altered gut microbiota, impaired intestinal barrier, and increased plasma levels of lipopolysaccharides (LPS) (53–55). For diabetics, the favorable effects of SCFAs were mainly identified in reducing serum glucose levels, improving insulin resistance, mitigating inflammation, and enhancing secretion of protective glucagon-like peptide 1 (GLP-1) (56–58). Conversely, dietary interventions with SCFAs were shown to alleviate T1DM in mice models, as evidenced by decreased auto-immune T cell counts, suppressed B cell proliferation, and expanded autoimmune FoxP3+ Treg cell repertoire in the colon, spleen, and systemic lymph nodes (46, 58). By providing SCFA-releasing diets to T2DM patients, it could significantly improve metabolic disorders by enriching SCFA-producing microbes mass, ameliorating individual glucose tolerance, and reducing levels of hemoglobin A1c (59–61). Herein, we discuss the latest developments in the protective effects of SCFAs and appreciate their potential involvement in diseases.
SCFAs, predominantly acetate, butyrate, and propionate, are anaerobic fermentation metabolites of fiber produced by intestinal microorganisms (62). SCFAs act as the principal energy source for colorectal cells, enhancing intestine epithelium integrity (63). Besides, as leading messenger molecules between gut microbiota and host health, SCFAs can enter the blood circulation, protect the gut barrier, regulate inflammatory responses, and influence immune functions of distal tissues (64).
SCFAs can promote the intestinal epithelium function, mainly by hypoxia-inducible factor-1 (HIF-1), which is a transcription factor stabilizing intestinal epithelial barrier (65). Antibiotic-mediated microbiota depletion reduces intestinal levels of HIF and causes epithelium impairment, which could be restored by supplementing butyrate. However, the effects of butyrate on gut epithelial barrier protection vanished when lacking HIF (29). In addition, SCFAs can restore the intestinal barrier integrity by inducing gene expressions of inter-epithelial junction protein Claudin-1, and activating other transcription factors such as STAT3 and SP1, even in inflammatory conditions (66, 67). Another involved mechanism is that SCFAs could induce IECs to produce antimicrobial peptides (AMPs), recruit neutrophils and anti-inflammatory cytokines, suppress activation of NLRP3 inflammasome, and further protect the intestinal barrier from damage by pathogens (68, 69).
When SCFAs were supplemented in vivo, they were shown to preserve the molecular barrier of intestinal epithelium. Vieira et al. reported that oral SCFAs could significantly improve intestinal trophism and inhibit leukocytes and other immunocytes infiltration, further attenuating the inflammatory state of gut barrier in rats with acute ulcerative colitis (70). SCFAs treatment may also constitute substrates for gut mucosa and ameliorate intestinal damage, by restoration of glutathione levels and reduction in proinflammatory mediators, including nitric oxide (NO) and tumor necrosis factor-α (TNF-α) in rat models of colitis (71). In addition, rectal administration of SCFAs could promote mucus secretion and intestinal repair, by increasing abundance of mucus-associated bacteria and expression of genes required for pathogens elimination, such as IL-17A and IL-1β in mice with enteritis (72, 73).
Säemann et al. reported that administration of SCFAs could not only reduce secretion of pro-inflammatory markers such as TNF-α, IL-6, and IL-12 but also increase release of anti-inflammatory markers including IL-10 in cultured human peripheral blood mononuclear cell (PBMCs) in vitro (74), providing important clues to cognize the anti-inflammatory actions of SCFAs. With evidence-based potentiated generation of anti-inflammatory regulatory T cells, inhibited release of reactive oxygen species, and suppressed production of pro-inflammatory cytokines including TNF-α and IL-1β, anti-inflammatory effects of dietary SCFAs have been confirmed in different animal models, such as steatosis, allergic airway inflammation, and chronic kidney disease (CKD) (64, 75, 76). In addition, clinical investigations revealed that the inflammatory potential, analyzed by the TNF-α/IL-10 ratio, was significantly higher in the diabetic group than the healthy ones, which may be counteracted by dietary fiber that can produce SCFAs (77, 78), corroborating the inflammation inhibitory potential of SCFAs. In most cases, reduced inflammatory conditions and improved immunity are inseparable.
Several mechanisms have already been explored on the anti-inflammation influences of SCFAs (1). Permeability: Increased permeability is believed to induce microbial translocation, which could trigger an inflammatory cascade (79). Chen et al. confirmed that the representative SCFA, butyrate, is a crucial substrate for promoting epithelial cell growth, which can maintain the colonic epithelium, prevent excessive gut permeability, and even induce innate immune responses to injury and invasive microorganisms if necessary (80) (2). Nuclear factor-kappa B (NF-κB): NF-κB is a critical transcription factor for inducing expressions of multiple inflammation related genes. Studies have shown that butyrate can inhibit NF-κB activation in human macrophages and epithelial cells (81, 82) (3). Histone deacetylation (HDAC): HDAC inhibitors were initially developed as cancer-combating agents. Nowadays HDACs inhibitors are attracting much more interest as anti-inflammatory agents, independent of their known proapoptotic or cell cycle arrest actions on malignant cells (83). SCFAs are natural HDACs inhibitors, facilitating expressions of anti-inflammatory genes in the immune cell, promoting T lymphocytes as they differentiate into effector T cells such as Th1 and Th17 cells subsets, and boosting their immune responses in inflammation (84, 85). Kim et al. also revealed that by promoting activation and differentiation of B cells to plasma B cells, SCFAs accelerated the production of most antibodies types, including IgG and IgA (86) (4). G-protein coupled receptors (GPCRs): SCFAs could activate GPR41 and GPR43 in intestinal epithelial cells, leading to transmission of mitogen-activated protein kinase signaling, and rapid secretion of chemokines and cytokines (87, 88). Singh et al. showed that via activating GPR109A in macrophages and dendritic cells, SCFAs make them highly efficient inducers of regulatory T cells, particularly FoxP3+ T cells, to limit inflammation and control carcinogenesis (88). So SCFAs not only act as anti-inflammatory bacterial metabolites but also function as immune boosters to prepare the host to better exterminate pathogens.
Furthermore, SCFAs are among the most extensively studied microbial metabolites that intervene in host metabolism. By binding to GPR43 and GPR41, SCFAs are able to raise plasma levels of GLP-1, peptide YY (PYY), and leptin, resulting in reduced food intake, enhanced glucose metabolism, and improved glucose homeostasis (89–91). Fascinating animals researches also indicated that butyrate and propionate may both activate gene expression germane to intestinal gluconeogenesis, through cAMP-dependent pathway and GPR41-dependent gut-brain circuits respectively, eventually improving glycaemia control and ameliorating insulin sensitivity (92, 93). Another vital function of SCFAs is intracellular metabolic integration to produce energy such as adenosine triphosphate (ATP) (94). Canfora et al. demonstrated that infusions of SCFA mixtures in the colon boosted energy consumption, enhanced fat oxidation, and decreased lipolysis in metabolic profiles (95).
In order to achieve these benefits, the production of SCFAs can be encouraged in various ways, such as natural sugar and high-fiber intake (96, 97). However, it is necessary to determine the dosage range of SCFAs before they can be applied in clinic, as superfluous SCFAs might exert some adverse effects, such as accelerating cholesterol synthesis and lipid accumulation within the liver (98, 99). Excessive acetate and butyrate may also be involved in host fat storage by enhancing energy intake and intestinal polysaccharide degradation, thereby contributing to weight gain and obesity phenotype in obese mice (100, 101).
Considering their extreme importance in resisting inflammation in the gut, SCFAs are regarded with mighty potential for certain diseases including metabolic conditions and cancer, providing strong theoretic foundation to launch SCFAs-based clinical trials for cancer treatment. However, the causative role of SCFAs abundance in diabetics with respect to carcinogenesis needs further elucidation.
Inflammation usually involves the activation, recruitment, and functioning of innate and adaptive immune cells, which is essential for the host to defend against pathogens, repair damaged tissues, and regulate tissue homeostasis (102). Acute inflammation is protective and normally self-limited, which would be terminated after elimination of harmful triggers or completion of the restorative process (103). This self-limiting property of acute inflammation was also verified by Bannenberg and his colleagues in mouse peritonitis models, induced by zymosan (104). Once acute inflammatory responses are out of control and cause tissue damage, it will amplify inflammation and progress to chronicity (105), hence predisposing people to cancer development. More importantly, inflammatory immune cells together with fibroblasts and vascular endothelial cells constitute the stroma network for cancer cell survival, namely the tumor microenvironment (TME) (106), which can boost tumor occurrence, tumor promotion, malignant transformation, and metastatic transmission (107, 108). In general, tumor extrinsic inflammation can be caused by certain factors including infection, metabolic diseases, autoimmune diseases, and smoking, while tumor intrinsic inflammation can be triggered by cancer-related gene mutations or by recruiting and activating inflammation-fighting cells (109). Inflammation, irrespectively of its inducement or appearance, owns a significant effect on carcinogenesis. Typical pro-inflammatory cytokines IL-17 and IL-23, which can promote tumor development and progression respectively, were both upregulated in mouse models of colorectal cancer (CRC) (110, 111). In turn, tumorigenesis may enhance pro-tumorigenic inflammation, illustrating the essential circle between inflammation and cancer. Collectively, epidemiological studies showed that inflammation is linked to the initiation of around 20% of cancers (112). To be specific, chronic inflammations caused by the hepatitis virus and Helicobacter pylori are associated with the majority of hepatocellular and gastric carcinomas respectively (113–115). Approximately 2% of CRC develops in patients with ulcerative colitis (UC) (116, 117). Chronic airway inflammations with tobacco smoke and airborne particulates are the major risk factors for lung cancer (118, 119).
Although the predisposing factors or sources may vary, inflammation always goes together with increased risk of cancer, oncogenic transformation, and malignant progression via multiple approaches (1). Enhancement of DNA damage. During inflammatory conditions, both inflammatory and epithelial cells can release chemicals like reactive oxygen and nitrogen species (RONS), which may cause DNA lesions (120). Furthermore, the generated DNA damage signals and cytotoxicity can promote inflammation, inducing a continuous vicious circle between DNA lesions and DNA repair, which can further enhance DNA damage. Subsequently, this feedback loop induces genetic mutations, genome instability, and eventual tumorigenesis (121, 122). Chen et al. reported that 7,12-Dimethylbenz[a]anthracene (DMBA), a mighty genotoxic agent, could activate the cGAS–cGAMP–STING pathway, inducing inflammation-driven cutaneous carcinogenesis in mouse models (123). Singhal et al. have manifested that DMBA was engaged in mammary carcinogenesis as well as the distant metastasis in skin-tumor-sensitive (STS) female mouse strains, mimicking DMBA‐induced human breast cancer (124) (2). Inactivation of anti-oncogene and activation of oncogenes. Without additional inducers, chronic inflammation can induce accumulated mutational in-activations of tumor suppressor genes such as Tp53 in the epithelial cells, leading to upregulation of microtubule modulin stathmin1 and increased chemoresistance in breast and colon cancer cell lines or patient specimens (125–127). Moreover, accumulation of activated mutations in epithelial oncogenes (K-ras and c-Myc) can be promoted by inflammation-induced DNA damage or inflammatory cytokines, which can also cooperate with other inflammatory stimuli to manifest cancerogenic activities, such as enhancing mutagenesis, promoting tissue injury, and ultimately carcinogenesis (128, 129) (3). Regulation of signaling pathways. Both NF-κB and signal transducer and activator of transcription 3 (STAT3) have been identified as cardinal inflammatory signaling molecules during carcinogenesis. Survival signals activated by NF-κB and STAT3 were enhanced in mutated epithelial cells, which protected cells against the attack of cytotoxic T lymphocytes, boosted malignant clones, and enabled tumor outgrowth (130–132). Otherwise, programmed cell death protein-1 (PD-1) pathway was activated to deliver inhibitory signals, which may be evoked by inflammatory signals interferon‐gamma (IFN‐γ), K-ras mutations, or K-ras/Tp53 co-mutations. This could drive T cell exhaustion, generate a tolerant microenvironment, and help cancerous cells escape immune surveillance and survive, particularly for patients with lung adenocarcinoma (133–135) (4). Modulation of inflammatory mediators. Cytokines, chemokines, and growth factors are the predominant cell-signaling molecules produced by multiple cells in the inflammatory microenvironment and are fundamental to tumor development in different stages (136). Dash reported that TNF-α was among the chief cytokines in inflammatory responses, which rendered epithelial to mesenchymal transition (EMT), accelerated cancerous cells invading process, and elicited other inflammatory proteases to orchestrate inflammatory conditions (137). Tumorigenic cytokines such as IL-6 and IL-11 could directly act on tumor cells, enhancing cell proliferation, stimulating angiogenesis, and expanding cancer stem cell (CSC) population, in mouse xenograft models of gastrointestinal and breast cancers (138, 139). Calon et al. identified that IL-11 was also involved in summoning myeloid cells such as fibroblasts and tumor growth factor-β (TGF-β), which could facilitate cancer cell migration, promote tumor invasion, and assist metastatic transition in colorectal carcinoma of mice (140). Regarding the similarity of inflammatory processes in distinct cancers, preventing or controlling inflammation may be an imperative treatment for cancer.
Numerous studies have demonstrated that some bacteria can secrete tumor-boosting metabolites like secondary bile acids, whereas some other species can generate tumor-suppressing metabolites such as SCFAs (141, 142). Echoing previously demonstrated anti-inflammation significance of SCFAs, substantial epidemiological data established that increased incidence of inflammatory diseases and cancer was linked to subjects with diets poor in SCFAs or decreased concentration of fecal SCFAs, typically for gastric and breast cancers (143–145). SCFAs in the gut and other organs can extensively reduce carcinogenesis as well as prevent and treat gastrointestinal and lung cancers, by inhibiting cell growth and migration, suppressing histone deacetylase, and inducing apoptosis (146–148). Specifically, Ohara et al. reported that increased CRC risk correlated tightly with altered gut microbiota, reduced output of SCFAs, and worse inflammation state (149). Therefore, increasing SCFAs production via regulation of gut microbiota should have a bright future in anti-cancer therapy.
Other than SCFAs, evidences also indicate that low-dose aspirin could reduce the risk of tumorigenesis, particularly in CRC. As an inhibitor of cyclooxygenase (COX)-1, aspirin exerts its significant chemo-preventive effects in CRC through inhibition of NF-κB dependent pathways, Wnt/β-catenin signaling, and additional COX proteins acetylation (150, 151). The notable anti-tumor effects of aspirin have been observed by reduction in platelet activation, inhibition of tumor angiogenesis, and decrease of pro-inflammatory agents (152). In addition, low-dose aspirin could reduce metastasis in colon cancer, mainly due to its effective inhibition of prostaglandin E2 (PGE2) formation and platelet-tumor cell aggregation (153). Different from aspirin and other modulators of NF-κB, SCFAs could not only inhibit NF-κB signaling pathway but also promote gut microbial ecology and enhance intestinal integrity, further fighting against inflammation and reducing tumorigenesis through multiple pathways. Moreover, as SCFAs can be supplemented by dietary interventions, they were considered to be safer and more obtainable. However, aspirin can cause several severe adverse effects, such as gastrointestinal hemorrhage, intracranial bleeding, and hypersensitivity reactions (154, 155). Even aspirin in a low dose can lead to bleeding in diabetics (156). These advantages push SCFAs to be potential agents to lower cancer risk.
Low levels of SCFAs, fermented by gut microbiota, have been linked with DM-related intestinal barrier dysfunction, gut dysbiosis, and aggravated inflammation. Mostly diabetic patients exhibited higher incidence of various tumors. In addition, an inverse relationship between decreased production of SCFAs and increased risk of cancer has also been discovered, showing that SCFAs have the potential of associating DM with tumorigenesis. Accordingly, diets high in fiber and transferring fecal microbiota, aiming at increasing SCFAs production or strains of SCFA-producing species, can attenuate the progression of inflammatory disorders by altering gut microbiota composition and suppressing inflammation (157), which may provide a new paradigm in cancer prevention and treatment in diabetes. However, the effectiveness and security of utilizing SCFAs to dampen inflammation and decrease the incidence and mortality of tumors in diabetic individuals require more research to confirm. Immediate evidences are still needed to validate and recognize the favorable influences of SCFAs as a capable regulator of cancer-related inflammation in DM. Efforts of collaboration encompassing metabolism, microbiology, immunology, oncology, and dietotherapy will define routes of administration and dosage to obtain the optimum benefits of SCFAs as an anti-inflammatory and anticarcinogenic soldier in DM.
QY and JO wrote the first draft of the manuscript. FS provided critical revision of the manuscript. JY conceived and designed the manuscript. All authors contributed to the article and approved the submitted version
Our work was funded by Chongqing Basic and Frontier Research project (grant number: cstc2018jcyjAX0652) and Chongqing Technological Innovation and Application Development project (grant number: cstc2019jscx-msxmX0190).
The authors declare that the research was conducted in the absence of any commercial or financial relationships that could be construed as a potential conflict of interest.
1. Asmat U, Abad K, Ismail K. Diabetes mellitus and oxidative stress—A concise review. Saudi Pharm J (2016) 24:547–53. doi: 10.1016/j.jsps.2015.03.013
4. Gomes S, Oliveira C, Azevedo-Silva J, Casanova M, Barreto J, Pereira H, et al. The role of diet-related short-chain fatty acids in colorectal cancer metabolism and survival: prevention and therapeutic implications. Curr Med Chem (2020) 27:4087–108. doi: 10.2174/0929867325666180530102050
5. Oguntibeju OO. Type 2 diabetes mellitus, oxidative stress and inflammation: examining the links. Int J Physiol Pathophysiol Pharmacol (2019) 11:45.
6. Wang G, Yu Y, Wang YZ, Wang JJ, Guan R, Sun Y, et al. Role of SCFAs in gut microbiome and glycolysis for colorectal cancer therapy. J Cell Physiol (2019) 234:17023–49. doi: 10.1002/jcp.28436
7. Handelsman Y, LeRoith D, Bloomgarden Z, Dagogo-Jack S, Einhorn D, Garber A, et al. Diabetes and cancer—an AACE/ACE consensus statement. Endocr Pract (2013) 19:675–93. doi: 10.4158/EP13248.CS
8. Satija A, Spiegelman D, Giovannucci E, Hu FB. Type 2 diabetes and risk of cancer. Br Med J (2015) 350:g7707. doi: 10.1136/bmj.g7707
9. Noto H, Osame K, Sasazuki T, Noda M. Substantially increased risk of cancer in patients with diabetes mellitus: a systematic review and meta-analysis of epidemiologic evidence in Japan. J Diabetes its Complications (2010) 24:345–53. doi: 10.1016/j.jdiacomp.2010.06.004
10. Bensimon L, Yin H, Suissa S, Pollak MN, Azoulay L. Type 2 diabetes and the risk of mortality among patients with prostate cancer. Cancer Causes Control (2014) 25:329–38. doi: 10.1007/s10552-013-0334-6
11. El–Serag HB, Hampel H, Javadi F. The association between diabetes and hepatocellular carcinoma: a systematic review of epidemiologic evidence. Clin Gastroenterol Hepatol (2006) 4:369–80. doi: 10.1016/j.cgh.2005.12.007
12. Ben Q, Xu M, Ning X, Liu J, Hong S, Huang W, et al. Diabetes mellitus and risk of pancreatic cancer: a meta-analysis of cohort studies. Eur J Cancer (2011) 47:1928–37. doi: 10.1016/j.ejca.2011.03.003
13. Friberg E, Orsini N, Mantzoros C, Wolk A. Diabetes mellitus and risk of endometrial cancer: a meta-analysis. Springer (2007) 50:1365–74. doi: 10.1007/s00125-007-0681-5
14. Sun L, Yu S. Diabetes mellitus is an independent risk factor for colorectal cancer. Dig Dis Sci (2012) 57:1586–97. doi: 10.1007/s10620-012-2059-x
15. Bao C, Yang X, Xu W, Luo H, Xu Z, Su C, et al. Diabetes mellitus and incidence and mortality of kidney cancer: a meta-analysis. J Diabetes its Complications (2013) 27:357–64. doi: 10.1016/j.jdiacomp.2013.01.004
16. Boyle P, Boniol M, Koechlin A, Robertson C, Valentini F, Coppens K, et al. Diabetes and breast cancer risk: a meta-analysis. Br J Cancer (2012) 107:1608–17. doi: 10.1038/bjc.2012.414
17. Castillo JJ, Mull N, Reagan JL, Nemr S, Mitri J. Increased incidence of non-Hodgkin lymphoma, leukemia, and myeloma in patients with diabetes mellitus type 2: a meta-analysis of observational studies. Blood J Am Soc Hematol (2012) 119:4845–50. doi: 10.1182/blood-2011-06-362830
18. Mitri J, Castillo J, Pittas AG. Diabetes and risk of Non-Hodgkin’s lymphoma: a meta-analysis of observational studies. Diabetes Care (2008) 31:2391–7. doi: 10.2337/dc08-1034
19. Giovannucci E, Harlan DM, Archer MC, Bergenstal RM, Gapstur SM, Habel LA, et al. Diabetes and cancer: a consensus report. Diabetes Care (2010) 33:1674–85. doi: 10.2337/dc10-0666
20. Hjalgrim H, Frisch M, Ekbom A, Kyvik K, Melbye M, Green A. Cancer and diabetes-a follow-up study of two population based cohorts of diabetic patients. J Internal Med (1997) 241:471–5. doi: 10.1111/j.1365-2796.1997.tb00004.x
21. Shu X, Ji J, Li X, Sundquist J, Sundquist K, Hemminki K. Cancer risk among patients hospitalized for Type 1 diabetes mellitus: a population-based cohort study in Sweden. Diabet Med (2010) 27:791–7. doi: 10.1111/j.1464-5491.2010.03011.x
22. Zendehdel K, Nyrén O, Östenson C-G, Adami H-O, Ekbom A, Ye W. Cancer incidence in patients with type 1 diabetes mellitus: a population-based cohort study in Sweden. J Natl Cancer Inst (2003) 95:1797–800. doi: 10.1093/jnci/djg105
23. Li L, Ma L, Fu P. Gut microbiota-derived short-chain fatty acids and kidney diseases. Drug Des Devel Ther (2017) 11:3531–42. doi: 10.2147/DDDT.S150825
24. Meng M, Klingensmith NJ, Coopersmith CM. New insights into the gut as the driver of critical illness and organ failure. Curr Opin Crit Care (2017) 23:143–8. doi: 10.1097/mcc.0000000000000386
25. Sharma S, Tripathi P. Gut microbiome and type 2 diabetes: where we are and where to go? J Nutr Biochem (2019) 63:101–8. doi: 10.1016/j.jnutbio.2018.10.003
26. Xu W-T, Nie Y-Z, Yang Z, Lu N-H. The crosstalk between gut microbiota and obesity and related metabolic disorders. Future Microbiol (2016) 11:825–36. doi: 10.2217/fmb-2015-0024
27. Singer-Englar T, Barlow G, Mathur R. Obesity, diabetes, and the gut microbiome: an updated review. Expert Rev Gastroenterol Hepatol (2019) 13:3–15. doi: 10.1080/17474124.2019.1543023
28. van der Lugt B, van Beek AA, Aalvink S, Meijer B, Sovran B, Vermeij WP, et al. Akkermansia muciniphila ameliorates the age-related decline in colonic mucus thickness and attenuates immune activation in accelerated aging Ercc1 (-/Δ7) mice. Immun Ageing (2019) 16:6. doi: 10.1186/s12979-019-0145-z
29. Kelly CJ, Zheng L, Campbell EL, Saeedi B, Scholz CC, Bayless AJ, et al. Crosstalk between Microbiota-Derived Short-Chain Fatty Acids and Intestinal Epithelial HIF Augments Tissue Barrier Function. Cell Host Microbe (2015) 17:662–71. doi: 10.1016/j.chom.2015.03.005
30. Drissi F, Buffet S, Raoult D, Merhej V. Common occurrence of antibacterial agents in human intestinal microbiota. Front Microbiol (2015) 6:441. doi: 10.3389/fmicb.2015.00441
31. Nagpal R, Yadav H. Bacterial Translocation from the Gut to the Distant Organs: An Overview. Ann Nutr Metab (2017) 71(Suppl 1):11–6. doi: 10.1159/000479918
32. Li Q, Wang C, Tang C, He Q, Zhao X, Li N, et al. Successful treatment of severe sepsis and diarrhea after vagotomy utilizing fecal microbiota transplantation: a case report. Crit Care (2015) 19:37. doi: 10.1186/s13054-015-0738-7
33. Liu S, da Cunha AP, Rezende RM, Cialic R, Wei Z, Bry L, et al. The Host Shapes the Gut Microbiota via Fecal MicroRNA. Cell Host Microbe (2016) 19:32–43. doi: 10.1016/j.chom.2015.12.005
34. Fiorucci S, Distrutti E. Bile Acid-Activated Receptors, Intestinal Microbiota, and the Treatment of Metabolic Disorders. Trends Mol Med (2015) 21:702–14. doi: 10.1016/j.molmed.2015.09.001
35. Cani PD, Plovier H, Van Hul M, Geurts L, Delzenne NM, Druart C, et al. Endocannabinoids–at the crossroads between the gut microbiota and host metabolism. Nat Rev Endocrinol (2016) 12:133–43. doi: 10.1038/nrendo.2015.211
36. Sohail MU, Althani A, Anwar H, Rizzi R, Marei HE. Role of the gastrointestinal tract microbiome in the pathophysiology of diabetes mellitus. J Diabetes Res (2017) 2017:9631435. doi: 10.1155/2017/9631435
37. Larsen N, Vogensen FK, Van Den Berg FW, Nielsen DS, Andreasen AS, Pedersen BK, et al. Gut microbiota in human adults with type 2 diabetes differs from non-diabetic adults. PLoS One (2010) 5:e9085. doi: 10.1371/journal.pone.0009085
38. Lê K-A, Li Y, Xu X, Liu T, Yang W, He F, et al. Alterations in fecal Lactobacillus and Bifidobacterium species in type 2 diabetic patients in Southern China population. Front Physiol (2013) 3:496. doi: 10.3389/fphys.2012.00496
39. Hänninen A, Nurmela R, Maksimow M, Heino J, Jalkanen S, Kurts C. Islet β-cell-specific T cells can use different homing mechanisms to infiltrate and destroy pancreatic islets. Am J Pathol (2007) 170:240–50. doi: 10.2353/ajpath.2007.060142
40. Wen L, Ley RE, Volchkov PY, Stranges PB, Avanesyan L, Stonebraker AC, et al. Innate immunity and intestinal microbiota in the development of Type 1 diabetes. Nature (2008) 455:1109–13. doi: 10.1038/nature07336
41. Everard A, Belzer C, Geurts L, Ouwerkerk JP, Druart C, Bindels LB, et al. Cross-talk between Akkermansia muciniphila and intestinal epithelium controls diet-induced obesity. Proc Natl Acad Sci (2013) 110:9066–71. doi: 10.1073/pnas.1219451110
42. Hansen CHF, Krych L, Nielsen DS, Vogensen FK, Hansen LH, Sørensen SJ, et al. Early life treatment with vancomycin propagates Akkermansia muciniphila and reduces diabetes incidence in the NOD mouse. Diabetologia (2012) 55:2285–94. doi: 10.1007/s00125-012-2564-7
43. Lau K, Benitez P, Ardissone A, Wilson TD, Collins EL, Lorca G, et al. Inhibition of type 1 diabetes correlated to a Lactobacillus johnsonii N6. 2-mediated Th17 bias. J Immunol (2011) 186:3538–46. doi: 10.4049/jimmunol.1001864
44. Harbison JE, Roth-Schulze AJ, Giles LC, Tran CD, Ngui KM, Penno MA, et al. Gut microbiome dysbiosis and increased intestinal permeability in children with islet autoimmunity and type 1 diabetes: A prospective cohort study. Pediatr Diabet (2019) 20:574–83. doi: 10.1111/pedi.12865
45. Forslund K, Hildebrand F, Nielsen T, Falony G, Le Chatelier E, Sunagawa S, et al. Disentangling type 2 diabetes and metformin treatment signatures in the human gut microbiota. Nature (2015) 528:262–6. doi: 10.1038/nature15766
46. Mariño E, Richards JL, McLeod KH, Stanley D, Yap YA, Knight J, et al. Gut microbial metabolites limit the frequency of autoimmune T cells and protect against type 1 diabetes. Nat Immunol (2017) 18:552–62. doi: 10.1038/ni.3713
47. Garcia-Mazcorro JF, Lage NN, Mertens-Talcott S, Talcott S, Chew B, Dowd SE, et al. Effect of dark sweet cherry powder consumption on the gut microbiota, short-chain fatty acids, and biomarkers of gut health in obese db/db mice. PeerJ (2018) 6:e4195. doi: 10.7717/peerj.4195
48. Van Passel MW, Kant R, Zoetendal EG, Plugge CM, Derrien M, Malfatti SA, et al. The genome of Akkermansia muciniphila, a dedicated intestinal mucin degrader, and its use in exploring intestinal metagenomes. PLoS One (2011) 6:e16876. doi: 10.1371/journal.pone.0016876
49. Schwiertz A, Taras D, Schäfer K, Beijer S, Bos NA, Donus C, et al. Microbiota and SCFA in lean and overweight healthy subjects. Obesity (2010) 18:190–5. doi: 10.1038/oby.2009.167
50. Huang J, Pearson JA, Peng J, Hu Y, Sha S, Xing Y, et al. Gut microbial metabolites alter IgA immunity in type 1 diabetes. JCI Insight (2020) 5:e135718. doi: 10.1172/jci.insight.135718
51. Vatanen T, Franzosa EA, Schwager R, Tripathi S, Arthur TD, Vehik K, et al. The human gut microbiome in early-onset type 1 diabetes from the TEDDY study. Nature (2018) 562:589–94. doi: 10.1038/s41586-018-0620-2
52. de Goffau MC, Fuentes S, van den Bogert B, Honkanen H, de Vos WM, Welling GW, et al. Aberrant gut microbiota composition at the onset of type 1 diabetes in young children. Diabetologia (2014) 57:1569–77. doi: 10.1007/s00125-014-3274-0
53. Adachi K, Sugiyama T, Yamaguchi Y, Tamura Y, Izawa S, Hijikata Y, et al. Gut microbiota disorders cause type 2 diabetes mellitus and homeostatic disturbances in gut-related metabolism in Japanese subjects. J Clin Biochem Nutr (2019) 64:231–8. doi: 10.3164/jcbn.18-101
54. Karlsson FH, Tremaroli V, Nookaew I, Bergström G, Behre CJ, Fagerberg B, et al. Gut metagenome in European women with normal, impaired and diabetic glucose control. Nature (2013) 498:99–103. doi: 10.1038/nature12198
55. Le Chatelier E, Nielsen T, Qin J, Prifti E, Hildebrand F, Falony G, et al. Richness of human gut microbiome correlates with metabolic markers. Nature (2013) 500:541–6. doi: 10.1038/nature12506
56. Zhao F, Liu Q, Cao J, Xu Y, Pei Z, Fan H, et al. A sea cucumber (Holothuria leucospilota) polysaccharide improves the gut microbiome to alleviate the symptoms of type 2 diabetes mellitus in Goto-Kakizaki rats. Food Chem Toxicol (2020) 135:110886. doi: 10.1016/j.fct.2019.110886
57. Ahmadi S, Mainali R, Nagpal R, Sheikh-Zeinoddin M, Soleimanian-Zad S, Wang S, et al. Dietary polysaccharides in the amelioration of gut microbiome dysbiosis and metabolic diseases. Obes Control Ther: Open Access (2017) 4:10. doi: 10.15226/2374-8354/4/2/00140
58. Wen L, Wong FS. Dietary short-chain fatty acids protect against type 1 diabetes. Nat Immunol (2017) 18:484–6. doi: 10.1038/ni.3730
59. Zhao L, Zhang F, Ding X, Wu G, Lam YY, Wang X, et al. Gut bacteria selectively promoted by dietary fibers alleviate type 2 diabetes. Science (2018) 359:1151–6. doi: 10.1126/science.aao5774
60. Zhao L, Lou H, Peng Y, Chen S, Zhang Y, Li X. Comprehensive relationships between gut microbiome and faecal metabolome in individuals with type 2 diabetes and its complications. Endocrine (2019) 66:526–37. doi: 10.1007/s12020-019-02103-8
61. Yao Y, Yan L, Chen H, Wu N, Wang W, Pro DW. Cyclocarya paliurus polysaccharides alleviate type 2 diabetic symptoms by modulating gut microbiota and short-chain fatty acids. Phytomedicine (2020) 77:153268. doi: 10.1016/j.phymed.2020.153268
62. Roy CC, Kien CL, Bouthillier L, Levy E. Short-chain fatty acids: ready for prime time? Nutr Clin Pract (2006) 21:351–66. doi: 10.1177/0115426506021004351
63. Donohoe DR, Garge N, Zhang X, Sun W, O’Connell TM, Bunger MK, et al. The microbiome and butyrate regulate energy metabolism and autophagy in the mammalian colon. Cell Metab (2011) 13:517–26. doi: 10.1016/j.cmet.2011.02.018
64. Trompette A, Gollwitzer ES, Yadava K, Sichelstiel AK, Sprenger N, Ngom-Bru C, et al. Gut microbiota metabolism of dietary fiber influences allergic airway disease and hematopoiesis. Nat Med (2014) 20:159–66. doi: 10.1038/nm.3444
65. Fachi JL, Felipe JS, Pral LP, da Silva BK, Corrêa RO, de Andrade MCP, et al. Butyrate Protects Mice from Clostridium difficile-Induced Colitis through an HIF-1-Dependent Mechanism. Cell Rep (2019) 27:750–61.e7. doi: 10.1016/j.celrep.2019.03.054
66. Chen J, Zhao KN, Vitetta L. Effects of Intestinal Microbial⁻Elaborated Butyrate on Oncogenic Signaling Pathways. Nutrients (2019) 11:1026. doi: 10.3390/nu11051026
67. Wang HB, Wang PY, Wang X, Wan YL, Liu YC. Butyrate enhances intestinal epithelial barrier function via up-regulation of tight junction protein Claudin-1 transcription. Dig Dis Sci (2012) 57:3126–35. doi: 10.1007/s10620-012-2259-4
68. Zhao Y, Chen F, Wu W, Sun M, Bilotta AJ, Yao S, et al. GPR43 mediates microbiota metabolite SCFA regulation of antimicrobial peptide expression in intestinal epithelial cells via activation of mTOR and STAT3. Mucosal Immunol (2018) 11:752–62. doi: 10.1038/mi.2017.118
69. Feng Y, Wang Y, Wang P, Huang Y, Wang F. Short-Chain Fatty Acids Manifest Stimulative and Protective Effects on Intestinal Barrier Function Through the Inhibition of NLRP3 Inflammasome and Autophagy. Cell Physiol Biochem (2018) 49:190–205. doi: 10.1159/000492853
70. Vieira EL, Leonel AJ, Sad AP, Beltrão NR, Costa TF, Ferreira TM, et al. Oral administration of sodium butyrate attenuates inflammation and mucosal lesion in experimental acute ulcerative colitis. J Nutr Biochem (2012) 23:430–6. doi: 10.1016/j.jnutbio.2011.01.007
71. Rodríguez-Cabezas ME, Gálvez J, Lorente MD, Concha A, Camuesco D, Azzouz S, et al. Dietary fiber down-regulates colonic tumor necrosis factor alpha and nitric oxide production in trinitrobenzenesulfonic acid-induced colitic rats. J Nutr (2002) 132:3263–71. doi: 10.1093/jn/132.11.3263
72. Jiminez JA, Uwiera TC, Abbott DW, Uwiera RRE, Inglis GD. Butyrate Supplementation at High Concentrations Alters Enteric Bacterial Communities and Reduces Intestinal Inflammation in Mice Infected with Citrobacter rodentium. mSphere (2017) 2:e00243-17. doi: 10.1128/mSphere.00243-17
73. Derrien M, Van Baarlen P, Hooiveld G, Norin E, Müller M, de Vos WM. Modulation of Mucosal Immune Response, Tolerance, and Proliferation in Mice Colonized by the Mucin-Degrader Akkermansia muciniphila. Front Microbiol (2011) 2:166. doi: 10.3389/fmicb.2011.00166
74. Säemann MD, Böhmig GA, Österreicher CH, Burtscher H, Parolini O, Diakos C, et al. Anti-inflammatory effects of sodium butyrate on human monocytes: potent inhibition of IL-12 and up-regulation of IL-10 production. FASEB J (2000) 14:2380–2. doi: 10.1096/fj.00-0359fje
75. Raso GM, Simeoli R, Russo R, Iacono A, Santoro A, Paciello O, et al. Effects of sodium butyrate and its synthetic amide derivative on liver inflammation and glucose tolerance in an animal model of steatosis induced by high fat diet. PLoS One (2013) 8:e68626. doi: 10.1371/journal.pone.0068626
76. Vaziri ND, Liu S-M, Lau WL, Khazaeli M, Nazertehrani S, Farzaneh SH, et al. High amylose resistant starch diet ameliorates oxidative stress, inflammation, and progression of chronic kidney disease. PLoS One (2014) 9:e114881. doi: 10.1371/journal.pone.0114881
77. Roelofsen H, Priebe M, Vonk R. The interaction of short-chain fatty acids with adipose tissue: relevance for prevention of type 2 diabetes. Benef Microbes.= (2010) 1:433–7. doi: 10.3920/BM2010.0028
78. Larasati RA, Harbuwono DS, Rahajeng E, Pradipta S, Nuraeni HS, Susilowati A, et al. The role of butyrate on monocyte migration and inflammation response in patient with type 2 diabetes mellitus. Biomedicines (2019) 7:74. doi: 10.3390/biomedicines7040074
79. Geirnaert A, Calatayud M, Grootaert C, Laukens D, Devriese S, Smagghe G, et al. Butyrate-producing bacteria supplemented in vitro to Crohn’s disease patient microbiota increased butyrate production and enhanced intestinal epithelial barrier integrity. Sci Rep (2017) 7:1–14. doi: 10.1038/s41598-017-11734-8
80. Chen G, Ran X, Li B, Li Y, He D, Huang B, et al. Sodium butyrate inhibits inflammation and maintains epithelium barrier integrity in a TNBS-induced inflammatory bowel disease mice model. EBioMedicine (2018) 30:317–25. doi: 10.1016/j.ebiom.2018.03.030
81. Luhrs H, Gerke T, Boxberger F, Backhaus K, Melcher R, Scheppach W, et al. Butyrate inhibits interleukin-1-mediated nuclear factor-kappa B activation in human epithelial cells. Dig Dis Sci (2001) 46:1968–73. doi: 10.1023/a:1010699418024
82. Luhrs H, Gerke T, Muller JG, Melcher R, Schauber J, Boxberge F, et al. Butyrate inhibits NF-kappaB activation in lamina propria macrophages of patients with ulcerative colitis. Scand J Gastroenterol (2002) 37:458–66. doi: 10.1080/003655202317316105
83. Akimova T, Beier UH, Liu Y, Wang L, Hancock WW. Histone/protein deacetylases and T-cell immune responses. Blood (2012) 119:2443–51. doi: 10.1182/blood-2011-10-292003
84. Kim CH. Microbiota or short-chain fatty acids: which regulates diabetes? Cell Mol Immunol (2018) 15:88–91. doi: 10.1038/cmi.2017.57
85. Park J, Kim M, Kang SG, Jannasch AH, Cooper B, Patterson J, et al. Short-chain fatty acids induce both effector and regulatory T cells by suppression of histone deacetylases and regulation of the mTOR–S6K pathway. Mucosal Immunol (2015) 8:80–93. doi: 10.1038/mi.2014.44
86. Kim M, Qie Y, Park J, Kim CH. Gut microbial metabolites fuel host antibody responses. Cell Host Microbe (2016) 20:202–14. doi: 10.1016/j.chom.2016.07.001
87. Kim MH, Kang SG, Park JH, Yanagisawa M, Kim CH. Short-chain fatty acids activate GPR41 and GPR43 on intestinal epithelial cells to promote inflammatory responses in mice. Gastroenterology (2013) 145:396–406.e1-10. doi: 10.1053/j.gastro.2013.04.056
88. Singh N, Gurav A, Sivaprakasam S, Brady E, Padia R, Shi H, et al. Activation of Gpr109a, receptor for niacin and the commensal metabolite butyrate, suppresses colonic inflammation and carcinogenesis. Immunity (2014) 40:128–39. doi: 10.1016/j.immuni.2013.12.007
89. Everard A, Cani PD. Gut microbiota and GLP-1. Rev Endocr Metab Disord (2014) 15:189–96. doi: 10.1007/s11154-014-9288-6
90. Reimann F, Tolhurst G. Gribble FM. G-protein-coupled receptors in intestinal chemosensation. Cell Metab (2012) 15:421–31. doi: 10.1016/j.cmet.2011.12.019
91. Tolhurst G, Heffron H, Lam YS, Parker HE, Habib AM, Diakogiannaki E, et al. Short-chain fatty acids stimulate glucagon-like peptide-1 secretion via the G-protein–coupled receptor FFAR2. Diabetes (2012) 61:364–71. doi: 10.2337/db11-1019
92. De Vadder F, Kovatcheva-Datchary P, Goncalves D, Vinera J, Zitoun C, Duchampt A, et al. Microbiota-generated metabolites promote metabolic benefits via gut-brain neural circuits. Cell (2014) 156:84–96. doi: 10.1016/j.cell.2013.12.016
93. Bouter KE, van Raalte DH, Groen AK, Nieuwdorp M. Role of the gut microbiome in the pathogenesis of obesity and obesity-related metabolic dysfunction. Gastroenterology (2017) 152:1671–8. doi: 10.1053/j.gastro.2016.12.048
94. Hu J, Lin S, Zheng B, Cheung PC. Short-chain fatty acids in control of energy metabolism. Crit Rev Food Sci Nutr (2018) 58:1243–9. doi: 10.1080/10408398.2016.1245650
95. Canfora EE, van der Beek CM, Jocken JW, Goossens GH, Holst JJ, Damink SWO, et al. Colonic infusions of short-chain fatty acid mixtures promote energy metabolism in overweight/obese men: a randomized crossover trial. Sci Rep (2017) 7:1–12. doi: 10.1038/s41598-017-02546-x
96. Fehlbaum S, Prudence K, Kieboom J, Heerikhuisen M, van den Broek T, Schuren FHJ, et al. In Vitro Fermentation of Selected Prebiotics and Their Effects on the Composition and Activity of the Adult Gut Microbiota. Int J Mol Sci (2018) 19:3097. doi: 10.3390/ijms19103097
97. Zhang C, Björkman A, Cai K, Liu G, Wang C, Li Y, et al. Impact of a 3-Months Vegetarian Diet on the Gut Microbiota and Immune Repertoire. Front Immunol (2018) 9:908. doi: 10.3389/fimmu.2018.00908
98. Perry RJ, Peng L, Barry NA, Cline GW, Zhang D, Cardone RL, et al. Acetate mediates a microbiome-brain-β-cell axis to promote metabolic syndrome. Nature (2016) 534:213–7. doi: 10.1038/nature18309
99. Rahat-Rozenbloom S, Fernandes J, Cheng J, Wolever TMS. Acute increases in serum colonic short-chain fatty acids elicited by inulin do not increase GLP-1 or PYY responses but may reduce ghrelin in lean and overweight humans. Eur J Clin Nutr (2017) 71:953–8. doi: 10.1038/ejcn.2016.249
100. Turnbaugh PJ, Ley RE, Mahowald MA, Magrini V, Mardis ER, Gordon JI. An obesity-associated gut microbiome with increased capacity for energy harvest. Nature (2006) 444:1027–31. doi: 10.1038/nature05414
101. Tan J, McKenzie C, Potamitis M, Thorburn AN, Mackay CR, Macia L. The role of short-chain fatty acids in health and disease. Adv Immunol (2014) 121:91–119. doi: 10.1016/b978-0-12-800100-4.00003-9
102. Medzhitov R. Origin and physiological roles of inflammation. Nature (2008) 454:428–35. doi: 10.1038/nature07201
103. Kumar V, Abbas AK, Fausto N, Aster JC. Robbins and Cotran pathologic basis of disease, professional edition e-book: Elsevier health sciences. World Health Organization (2014).
104. Bannenberg GL, Chiang N, Ariel A, Arita M, Tjonahen E, Gotlinger KH, et al. Molecular circuits of resolution: formation and actions of resolvins and protectins. J Immunol (2005) 174:4345–55. doi: 10.4049/jimmunol.174.7.4345
105. Gordon S. Phagocytosis: an immunobiologic process. Immunity (2016) 44:463–75. doi: 10.1016/j.immuni.2016.02.026
106. Greten FR, Grivennikov SI. Inflammation and cancer: triggers, mechanisms, and consequences. Immunity (2019) 51:27–41. doi: 10.1016/j.immuni.2019.06.025
107. Bishehsari F, Engen PA, Preite NZ, Tuncil YE, Naqib A, Shaikh M, et al. Dietary fiber treatment corrects the composition of gut microbiota, promotes SCFA production, and suppresses colon carcinogenesis. Genes (2018) 9:102. doi: 10.3390/genes9020102
109. Todoric J, Antonucci L, Karin M. Targeting inflammation in cancer prevention and therapy. Cancer Prev Res (2016) 9:895–905. doi: 10.1158/1940-6207.CAPR-16-0209
110. Wang K, Kim MK, Di Caro G, Wong J, Shalapour S, Wan J, et al. Interleukin-17 receptor a signaling in transformed enterocytes promotes early colorectal tumorigenesis. Immunity (2014) 41:1052–63. doi: 10.1016/j.immuni.2014.11.009
111. Grivennikov SI, Wang K, Mucida D, Stewart CA, Schnabl B, Jauch D, et al. Adenoma-linked barrier defects and microbial products drive IL-23/IL-17-mediated tumour growth. Nature (2012) 491:254–8. doi: 10.1038/nature11465
112. Siegel RL, Miller KD, Jemal A. Cancer statistics, 2020. CA: Cancer J Clin (2020) 70:7–30. doi: 10.3322/caac.21590
113. Rawla P, Barsouk A. Epidemiology of gastric cancer: global trends, risk factors and prevention. Prz Gastroenterol (2019) 14:26. doi: 10.5114/pg.2018.80001
114. Mahale P, Engels EA, Koshiol J. Hepatitis B virus infection and the risk of cancer in the elderly US population. Int J Cancer (2019) 144:431–9. doi: 10.1002/ijc.31643
115. Wong A, Le A, Lee M-H, Lin Y-J, Nguyen P, Trinh S, et al. Higher risk of hepatocellular carcinoma in Hispanic patients with hepatitis C cirrhosis and metabolic risk factors. Sci Rep (2018) 8:1–8. doi: 10.1038/s41598-018-25533-2
116. Olén O, Erichsen R, Sachs MC, Pedersen L, Halfvarson J, Askling J, et al. Colorectal cancer in ulcerative colitis: a Scandinavian population-based cohort study. Lancet (2020) 395:123–31. doi: 10.1016/S0140-6736(19)32545-0
117. Bopanna S, Ananthakrishnan AN, Kedia S, Yajnik V, Ahuja V. Risk of colorectal cancer in Asian patients with ulcerative colitis: a systematic review and meta-analysis. Lancet Gastroenterol Hepatol (2017) 2:269–76. doi: 10.1016/S2468-1253(17)30004-3
118. Consonni D, Carugno M, De Matteis S, Nordio F, Randi G, Bazzano M, et al. Outdoor particulate matter (PM10) exposure and lung cancer risk in the EAGLE study. PLoS One (2018) 13:e0203539. doi: 10.1371/journal.pone.0203539
119. Zhou G. Tobacco, air pollution, environmental carcinogenesis, and thoughts on conquering strategies of lung cancer. Cancer Biol Med (2019) 16:700. doi: 10.20892/j.issn.2095-3941.2019.0180
120. Berti M, Vindigni A. Replication stress: getting back on track. Nat Struct Mol Biol (2016) 23:103–9. doi: 10.1038/nsmb.3163
121. Kawanishi S, Ohnishi S, Ma N, Hiraku Y, Murata M. Crosstalk between DNA Damage and Inflammation in the Multiple Steps of Carcinogenesis. Int J Mol Sci (2017) 18:1808. doi: 10.3390/ijms18081808
122. Jaiswal M, LaRusso NF, Burgart LJ, Gores GJ. Inflammatory cytokines induce DNA damage and inhibit DNA repair in cholangiocarcinoma cells by a nitric oxide-dependent mechanism. Cancer Res (2000) 60:184–90. doi: 10.1053/gast.2001.20875
123. Ahn J, Xia T, Konno H, Konno K, Ruiz P, Barber GN. Inflammation-driven carcinogenesis is mediated through STING. Nat Commun (2014) 5:1–9. doi: 10.1038/ncomms6166
124. Singhal SS, Horne D, Singhal J, Vonderfecht S, Salgia R, Awasthi S. Synergistic efficacy of RLIP inhibition and 2′-hydroxyflavanone against DMBA-induced mammary carcinogenesis in SENCAR mice. Mol Carcinogenesis (2019) 58:1438–49. doi: 10.1002/mc.23026
125. Canli Ö, Nicolas AM, Gupta J, Finkelmeier F, Goncharova O, Pesic M, et al. Myeloid cell-derived reactive oxygen species induce epithelial mutagenesis. Cancer Cell (2017) 32:869–83.e5. doi: 10.1016/j.ccell.2017.11.004
126. Robles AI, Traverso G, Zhang M, Roberts NJ, Khan MA, Joseph C, et al. Whole-exome sequencing analyses of inflammatory bowel Disease– Associated colorectal cancers. Gastroenterology (2016) 150:931–43. doi: 10.1053/j.gastro.2015.12.036
127. Masciarelli S, Fontemaggi G, Di Agostino S, Donzelli S, Carcarino E, Strano S, et al. Gain-of-function mutant p53 downregulates miR-223 contributing to chemoresistance of cultured tumor cells. Oncogene (2014) 33:1601–8. doi: 10.1038/onc.2013.106
128. Liao W, Overman MJ, Boutin AT, Shang X, Zhao D, Dey P, et al. KRAS-IRF2 axis drives immune suppression and immune therapy resistance in colorectal cancer. Cancer Cell (2019) 35:559–72.e7. doi: 10.1016/j.ccell.2019.02.008
129. Kortlever RM, Sodir NM, Wilson CH, Burkhart DL, Pellegrinet L, Swigart LB, et al. Myc cooperates with Ras by programming inflammation and immune suppression. Cell (2017) 171:1301–15.e14. doi: 10.1016/j.cell.2017.11.013
130. Nguyen AV, Wu Y-Y, Liu Q, Wang D, Nguyen S, Loh R, et al. STAT3 in epithelial cells regulates inflammation and tumor progression to malignant state in colon. Neoplasia (2013) 15:998–1008. doi: 10.1593/neo.13952
131. Taniguchi K, Karin M. NF-κB, inflammation, immunity and cancer: coming of age. Nat Rev Immunol (2018) 18:309–24. doi: 10.1038/nri.2017.142
132. Ziegler PK, Bollrath J, Pallangyo CK, Matsutani T, Canli Ö, De Oliveira T, et al. Mitophagy in intestinal epithelial cells triggers adaptive immunity during tumorigenesis. Cell (2018) 174:88–101.e16. doi: 10.1016/j.cell.2018.05.028
133. Chihara N, Madi A, Kondo T, Zhang H, Acharya N, Singer M, et al. Induction and transcriptional regulation of the co-inhibitory gene module in T cells. Nature (2018) 558:454–9. doi: 10.1038/s41586-018-0206-z
134. Lee CK, Man J, Lord S, Cooper W, Links M, Gebski V, et al. Clinical and molecular characteristics associated with survival among patients treated with checkpoint inhibitors for advanced non–small cell lung carcinoma: a systematic review and meta-analysis. JAMA Oncol (2018) 4:210–6. doi: 10.1001/jamaoncol.2017.4427
135. Dong Z-Y, Zhong W-Z, Zhang X-C, Su J, Xie Z, Liu S-Y, et al. Potential predictive value of TP53 and KRAS mutation status for response to PD-1 blockade immunotherapy in lung adenocarcinoma. Clin Cancer Res (2017) 23:3012–24. doi: 10.1016/j.jtho.2016.11.504
136. Wang J, Li D, Cang H, Guo B. Crosstalk between cancer and immune cells: Role of tumor-associated macrophages in the tumor microenvironment. Cancer Med (2019) 8:4709–21. doi: 10.1002/cam4.2327
137. Dash S. Analyzing the Role of TNF-α and Autophagy in Regulation of TGF-β Induced Epithelial to Mesenchymal Transition in Cancer Cells. BITS Pilani (2018).
138. Putoczki TL, Thiem S, Loving A, Busuttil RA, Wilson NJ, Ziegler PK, et al. Interleukin-11 is the dominant IL-6 family cytokine during gastrointestinal tumorigenesis and can be targeted therapeutically. Cancer Cell (2013) 24:257–71. doi: 10.1016/j.ccr.2013.06.017
139. Korkaya H, G-i K, Davis A, Malik F, Henry NL, Ithimakin S, et al. Activation of an IL6 inflammatory loop mediates trastuzumab resistance in HER2+ breast cancer by expanding the cancer stem cell population. Mol Cell (2012) 47:570–84. doi: 10.1016/j.molcel.2012.06.014
140. Calon A, Espinet E, Palomo-Ponce S, Tauriello DV, Iglesias M, Céspedes MV, et al. Dependency of colorectal cancer on a TGF-β-driven program in stromal cells for metastasis initiation. Cancer Cell (2012) 22:571–84. doi: 10.1016/j.ccr.2012.08.013
141. Louis P, Hold GL, Flint HJ. The gut microbiota, bacterial metabolites and colorectal cancer. Nat Rev Microbiol (2014) 12:661–72. doi: 10.1038/nrmicro3344
142. O’keefe SJ. Diet, microorganisms and their metabolites, and colon cancer. Nat Rev Gastroenterol Hepatol (2016) 13:691. doi: 10.1038/nrgastro.2016.165
143. Liang W, Yang Y, Wang H, Wang H, Yu X, Lu Y, et al. Gut microbiota shifts in patients with gastric cancer in perioperative period. Medicine (2019) 98:e16626. doi: 10.1097/MD.0000000000016626
144. Seethaler B, Beutel J, Kogel M, Basrai M, Walter J, Laville M, et al. Influence of the Mediterranean diet on the production of short-chain fatty acids in women at risk for breast cancer (LIBRE). Proc Nutr Soc (2020) 79:e180. doi: 10.1017/S0029665120001287
145. Yusuf F, Adewiah S, Fatchiyah F. The level short chain fatty acids and HSP 70 in colorectal cancer and non-colorectal cancer. Acta Inform Medica (2018) 26:160. doi: 10.5455/aim.2018.26.160-163
146. Keku TO, Dulal S, Deveaux A, Jovov B, Han X. The gastrointestinal microbiota and colorectal cancer. Am J Physiol-Gastrointest Liver Physiol (2015) 308:G351–63. doi: 10.1152/ajpgi.00360.2012
147. Kim K, Kwon O, Ryu TY, Jung CR, Kim J, Min JK, et al. Propionate of a microbiota metabolite induces cell apoptosis and cell cycle arrest in lung cancer. Mol Med Rep (2019) 20:1569–74. doi: 10.3892/mmr.2019.10431
148. Wu X, Wu Y, He L, Wu L, Wang X, Liu Z. Effects of the intestinal microbial metabolite butyrate on the development of colorectal cancer. J Cancer (2018) 9:2510. doi: 10.7150/jca.25324
149. Ohara T, Mori T. Antiproliferative effects of short-chain fatty acids on human colorectal cancer cells via gene expression inhibition. Anticancer Res (2019) 39:4659–66. doi: 10.21873/anticanres.13647
150. Bos CL, Kodach LL, van den Brink GR, Diks SH, van Santen MM, Richel DJ, et al. Effect of aspirin on the Wnt/beta-catenin pathway is mediated via protein phosphatase 2A. Oncogene (2006) 25:6447–56. doi: 10.1038/sj.onc.1209658
151. Takada Y, Singh S, Aggarwal BB. Identification of a p65 peptide that selectively inhibits NF-kappa B activation induced by various inflammatory stimuli and its role in down-regulation of NF-kappaB-mediated gene expression and up-regulation of apoptosis. J Biol Chem (2004) 279:15096–104. doi: 10.1074/jbc.M311192200
152. Rohwer N, Kühl AA, Ostermann AI, Hartung NM, Schebb NH, Zopf D, et al. Effects of chronic low-dose aspirin treatment on tumor prevention in three mouse models of intestinal tumorigenesis. Cancer Med (2020) 9:2535–50. doi: 10.1002/cam4.2881
153. Boutaud O, Sosa IR, Amin T, Oram D, Adler D, Hwang HS, et al. Inhibition of the Biosynthesis of Prostaglandin E2 By Low-Dose Aspirin: Implications for Adenocarcinoma Metastasis. Cancer Prev Res (Phila) (2016) 9:855–65. doi: 10.1158/1940-6207.Capr-16-0094
154. Laidlaw TM, Cahill KN. Current Knowledge and Management of Hypersensitivity to Aspirin and NSAIDs. J Allergy Clin Immunol Pract (2017) 5:537–45. doi: 10.1016/j.jaip.2016.10.021
155. McQuaid KR, Laine L. Systematic review and meta-analysis of adverse events of low-dose aspirin and clopidogrel in randomized controlled trials. Am J Med (2006) 119:624–38. doi: 10.1016/j.amjmed.2005.10.039
156. Leggio M, Bendini MG, Caldarone E, Lombardi M, Severi P, D’Emidio S, et al. Low-dose aspirin for primary prevention of cardiovascular events in patients with diabetes: Benefit or risk? Diabetes Metab (2018) 44:217–25. doi: 10.1016/j.diabet.2017.11.002
Keywords: short-chain fatty acids, gut dysbiosis, inflammation, diabetes, cancer
Citation: Yang Q, Ouyang J, Sun F and Yang J (2020) Short-Chain Fatty Acids: A Soldier Fighting Against Inflammation and Protecting From Tumorigenesis in People With Diabetes. Front. Immunol. 11:590685. doi: 10.3389/fimmu.2020.590685
Received: 02 September 2020; Accepted: 03 November 2020;
Published: 08 December 2020.
Edited by:
Francisco José Pérez-Cano, University of Barcelona, SpainReviewed by:
Matteo A. Russo, San Raffaele Pisana (IRCCS), ItalyCopyright © 2020 Yang, Ouyang, Sun and Yang. This is an open-access article distributed under the terms of the Creative Commons Attribution License (CC BY). The use, distribution or reproduction in other forums is permitted, provided the original author(s) and the copyright owner(s) are credited and that the original publication in this journal is cited, in accordance with accepted academic practice. No use, distribution or reproduction is permitted which does not comply with these terms.
*Correspondence: Jiadan Yang, eWFuZ2ppYWRhbjEwMjZAMTYzLmNvbQ==
†These authors have contributed equally to this work
Disclaimer: All claims expressed in this article are solely those of the authors and do not necessarily represent those of their affiliated organizations, or those of the publisher, the editors and the reviewers. Any product that may be evaluated in this article or claim that may be made by its manufacturer is not guaranteed or endorsed by the publisher.
Research integrity at Frontiers
Learn more about the work of our research integrity team to safeguard the quality of each article we publish.