- Department of Microbiology and Immunology, Program in Infectious Diseases and Immunity in Global Health, The Research Institute of the McGill University Health Centre, McGill University, Montreal, QC, Canada
One of the major challenges the scientific community faces today is the lack of translational data generated from mouse trials for human health application. Housing temperature-dependent chronic cold stress in laboratory rodents is one of the key factors contributing to lack of translatability because it reveals major metabolic differences between humans and rodents. While humans tend to operate at temperatures within their thermoneutral zone, most laboratory rodents are housed at temperatures below this zone and have an increased energy demand to generate heat. This has an impact on the immune system of mice and thus affects results obtained using murine models of human diseases. A limited number of studies and reviews have shown that results obtained on mice housed at thermoneutrality were different from those obtained from mice housed in traditional housing conditions. Most of those studies, focused on obesity and cancer, found that housing mice at thermoneutrality changed the outcomes of the diseases negatively and positively, respectively. In this review, we describe how thermoneutrality impacts the immune system of rodents generally and in the context of different disease models. We show that thermoneutrality exacerbates cardiovascular and auto-immune diseases; alleviates asthma and Alzheimer’s disease; and, changes gut microbiome populations. We also show that thermoneutrality can have exacerbating or alleviating effects on the outcome of infectious diseases. Thus, we join the call of others in this field to urge researchers to refine murine models of disease and increase their translational capacity by considering housing at thermoneutrality for trials involving rodents.
Introduction
Thermoneutrality (TN) has been defined as the metabolic state of an organism in an environmental temperature at which it does not have to generate or lose heat (1). It is a relatively recent term in the scientific literature with the first reference dating to 1968 (2). The general interest in this concept is still under the radar. Despite this, TN is an important concept to consider when conducting research using one of the most common human disease models: the mouse (1, 3–7). Mice are frequently used in research because of ease in maintenance and genetic manipulation (3, 8). However, much of the research conducted on mice models does not translate well to important impact in human clinical trials due to differences in data reporting; study set-up parameters; and, mouse vs. human metabolism (9–11). A substantial improvement and refining of murine models is required in order to reduce the variability of findings due to metabolic differences (11). Some problems in metabolic reproducibility stem from circadian rhythm differences with humans; social stressors; and, facility-based differences in the gut microbiome, but another important factor is housing temperature (5, 6, 11–16). In the laboratory setting, mice are routinely housed at temperatures (20–22°C) below their thermoneutral zone (TNZ) (29–34°C) (5). In this review, we will refer to the standard temperature used in most research settings as sub-optimal temperature (ST) because it subjects mice to a constant metabolic stress (5). Rodents are particularly affected by exposure to ST because they rely heavily on non-shivering thermogenesis for heat generation and possess a greater thermogenic demand due to their greater surface area to volume (SA/V) ratio than other animals (1, 5). Consequently, mice lose heat more quickly and need more energy to maintain their body core temperature (BCT) (3). Behavioral signs of cold stress can be observed in traditional housing systems: mice tend to huddle together or use nesting material in order to prevent heat loss (17). Mice pups also vocalize more to indicate distress when they are housed below their thermoneutral temperature (TT) (18). In addition, given the choice, mice preferentially choose an area in their housing that is closer to their TT, regardless of the availability of nesting material (1, 19). The goal of this review is to present a thorough overview of what is currently known about TN and its effect on murine models of disease.
Role of Environmental Temperature on Mouse Metabolism
The rodent response to cold stress through brown adipose tissue (BAT)-mediated non-shivering thermogenesis is well understood (11). The environmental ST sensed by the brain (Figure 1A) causes the release of norepinephrine (NE) from the sympathetic nervous system (Figure 1B) (11). The released NE interacts with β-adrenergic receptors (β-AR) on the surface of brown adipocytes (Figure 1C) (11), resulting in break-down of triglycerides (TG) within the BAT into free-fatty acids (FFA) (Figure 1D). The FFA interact with uncoupling protein-1 (UCP-1) present in the cytosol (Figure 1E) of BAT cells and stimulate the mitochondria to produce energy in the form of heat (Figure 1F) (11). The thyroid hormone (TH) is thought to play a role in this metabolic process by stimulating the brain regions responsible for BAT activation (Figure 1G) (11).
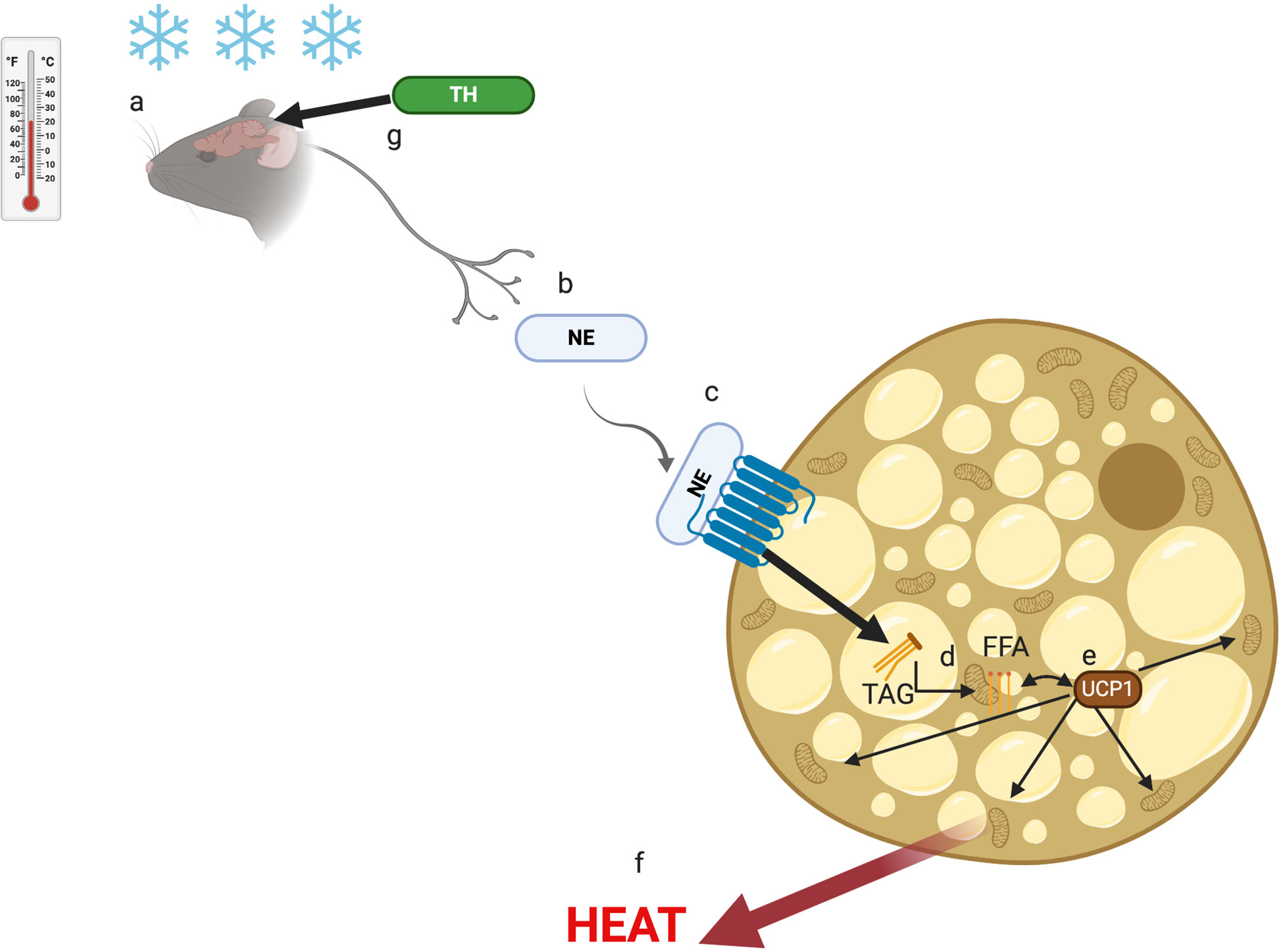
Figure 1 The non-shivering thermogenesis activation pathway. Cold stress is sensed by the brain (A) and signals the sympathetic nervous system to release norepinephrine (NE) (B) that interact with b-adrenergic receptor (b-AR) on brown adipocytes (C). As a result, triglycerides (TAG) are broken down to free fatty acids (FFA) (D) that interact with uncoupling protein-1 (UCP-1) (E) to induce the mitochondria to generate heat (E). The thyroid hormone (TH) plays a role in the cold stress (F) sensor region of the brain (G).
Various physiological and metabolic effects of housing mice at TT compared to ST have been documented in a number of publications (20–29). At TT, mice have lower energy expenditure in light and dark cycles; higher energy efficiency; and decreased nutrient mobilization and metabolization due to the removal of the cold stressor (21–24). They also spend less time awake, suggesting that mice housed at ST experience sleep deprivation (3, 22). Mice housed at TT exhibit less cancellous bone loss and are better adapted to deal with metabolic stressors such as sleep deprivation or heat stress (20, 26, 27, 30). Most mouse models of longevity are more resistant to ST due to their lower BCT (3). In addition, the effects observed on mice housed at TT can often be mimicked at ST by treating the mice with β-AR antagonists, which suppresses the NE pathway for heat generation (27–29).
Role of Environmental Temperature on the Immune System
Another major consequence of ST housing is decreased energy availability for mice immune system. Energy needed to drive immune processes is instead redirected toward heat generation (8), resulting in clear differences in the immune response of mice at ST compared to TT. Some studies have reported an increase in thermogenesis-associated M2 macrophages at ST (31). These macrophages are associated with adipose tissue and provide the same function as NE during thermogenesis (31). Other studies have reported an increase in BAT inflammation with production of pro-inflammatory cytokines (MCP1, IFNγ, TNFα, IL-1β, and IL-6) during exposure to ST (32). These alternatively activated macrophages have been shown to be associated with expression of IL-4 and IL-13 and are involved in the expression of catecholamines by BAT (33). T cell proliferation has been shown to be suppressed in part by myeloid-derived suppressor cells (MDSCs) that are generated in greater number via β-AR signaling under ST conditions (34, 35). Another study showed that BAT inflammation at ST is mediated by the type 2 immune response in mice (36). Treatment of mice at TT with IL-33, which triggers ILC2s that are involved in the type-2 response, resulted in an increase in BAT size, activity, and energy expenditure (36). In addition, mice and rats housed at ST tend to experience latent hypothermia after immune challenge with LPS, while those at TT undergo a fever response (37, 38). This has also been documented in other animals (e.g., pigs and cattle) (39). In rats, the ST-hypothermic response is age-dependent, with a reduced effect observed in older animals (40, 41). At TT, a reduction in activity is generally sufficient to fight an immune challenge compared to the need for increases in processes such as oxygen consumption; blood glucose metabolism; and, FA metabolism at ST (42). Some immune factors, such as TNF-α, Il-6, and Il-1β, have been implicated in the differential response to immune challenge at TT compared to ST due to their function as pyrogens in the fever response (27, 41, 43). In addition, high mobility group box 1 (HMGB1), the macrophage-derived factor involved in tissue repair and tissue fibrosis, was found at a higher level in circulation at TT and higher liver inflammation was observed (44). Interestingly, immune-challenged rodents tend to prefer environmental temperatures closer to their TT, indicating that TT is beneficial in the response to various pathogens (40). However, in this review we will outline cases in which housing at TT can exacerbate disease presentation (see Table 1). Figure 2 outlines the effect of thermoneutrality on different models of disease.
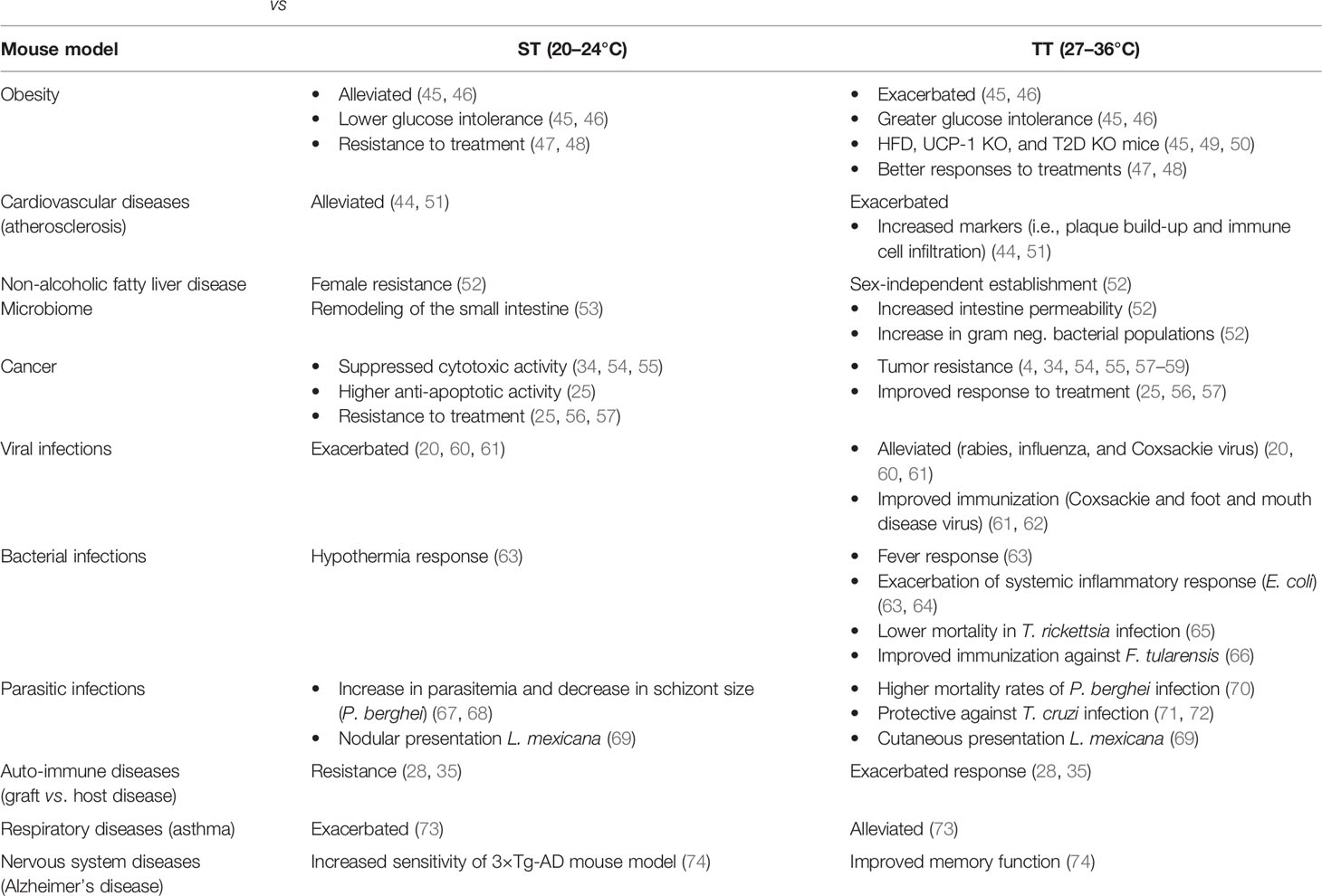
Table 1 Effects of sub-optimal vs. thermoneutral environmental temperatures on the presentation of murine disease models.
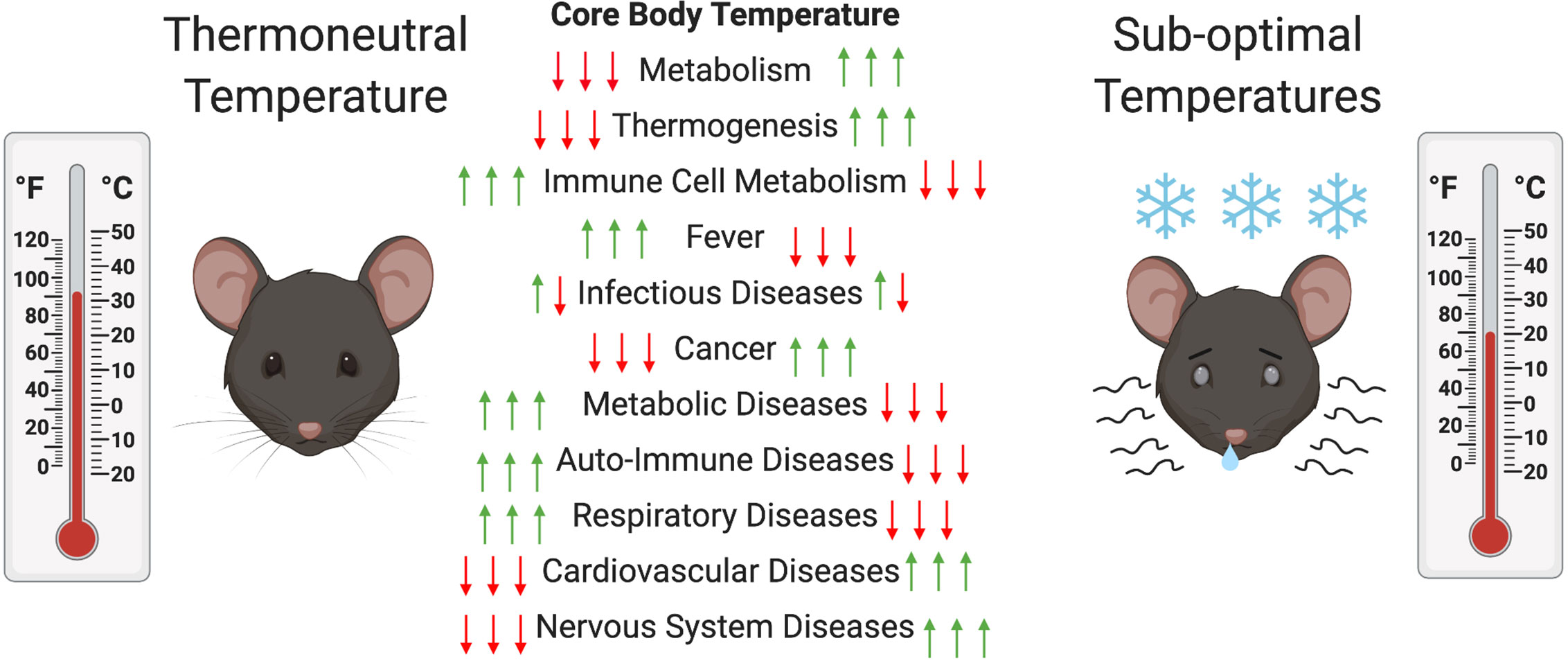
Figure 2 Changes in mouse metabolism and diseases at thermoneutral (TT) compared to suboptimal temperatures (ST). In order to maintain their core body temperatures (CBT) mice have to increase and decrease their metabolic activity to increase or decrease thermogenesis at ST compared to TT, respectively. This can decrease or increase the metabolic activity of their immune cells and have alleviating (red arrow) or exacerbating (green arrow) effects on various disease models. In the case of infectious diseases both can occur depending on the type of pathogen.
Respiratory Diseases
The effect of TN on the respiratory system of mice has only been investigated in the context of the ovalbumin-induced asthma disease model (73). Liao et al. observed lower inflammatory cell counts driven by a decrease in eosinophil populations in bronchoalveolar lavage from these mice (73). The airway hyperresponsiveness to asthma was also reduced in mice at TT compared to ST, characterized by lower IL-4, IL-10, and IgGE levels and higher IFNγ levels (73). These results were attributed to an increase in regulatory T cell infiltration in the lungs at the higher temperature, which dampens the inflammatory response (73). In this context, TN mediates a protective immune response by promoting tight regulation of immune cells at the site of inflammation compared to ST. However, this phenomenon should be tested on other respiratory disease models.
Graft vs. Host disease
Another disease in which TN housing has revealed a gap in the understanding of murine models is in the context of graft vs. host disease (GVHD) (28, 35). This disease occurs when the immune system of an individual grafted with cells or tissue from a genetically distinct individual recognizes it as foreign and induces a fulgurant immune response. It was previously thought that congenic mice were resistant to the establishment of GVHD but Leigh et al. (27) found that grafting mice with bone marrow-derived T cells caused severe GVHD characterized by lesions in the intestines and liver of mice housed at TT (28). They attributed the resistance in mice housed at ST to high NE levels that impaired their immune response. Resistance at ST was reversed when the mice were treated with a β-AR antagonist or the gene encoding β-AR was knocked-out (28). Contrarily, when mice were treated with a β-AR at TT agonist, GVHD was decreased (28). In another study, the same group demonstrated that mice with T cells lacking β-AR housed at ST experienced a Th1 response when they were implanted with an allo-graft, suggesting that β-AR signaling plays a direct role in the severity of GVHD (35). They also showed that treating mice with a β-AR agonist decreased the severity of GVHD (35). These two studies demonstrated a clear link between TN and a metabolically activated immune system mediated by β-AR signaling.
Bacterial Infections
In the context of the fever-response to bacterial agents, housing temperatures closer to TN may alleviate or exacerbate disease presentation. As mentioned previously, rodents challenged with LPS develop hypothermia when housed at ST and fever when housed at TT (63). This was also observed in rats treated with a dose of Escherichia coli which induced a systemic inflammatory response syndrome (63). Hypothermic rats housed at ST also had decreased neutrophil infiltration in lungs; lower arterial pressure; decreased endotoxemia; increased liver bacterial load; lower organ dysfunction; and, lower mortality rates (63). This demonstrates that fever in rodents housed at TT can exacerbate disease, probably due to increased sensitivity to infectious agents and immune-cell mediated bacteriolysis followed by sepsis (63, 64). Interestingly, changes were not seen in levels of the major fever-associated cytokines (63).
It is important to note that an exacerbated response does not occur in all bacterial infections. Mice injected with Typhus rickettsia, which develops more favorably in a colder environment, had a 75% decrease in mortality rates at TT compared to ST (65). Importantly, increased immune sensitivity at TT can be beneficial in vaccination against bacterial disease. Balb/c mice housed at TT vaccinated with live attenuated Francisella tularensis had a higher IFNγ-mediated antigen-specific T-cell response when challenged with the pathogen (66). Unfortunately, information on the effect of TT on bacterial diseases in mice is very limited. The studies cited above show variation in the bacterial species or strain; animal species, strain, age, and sex; acclimatization time; type of cage system; and, type of inoculum tested (63, 65, 66). This makes it difficult to draw solid conclusions pertaining to the specific role of TN in the context of bacterial infections.
Viral Infections
A TN environment may also be protective for the murine host experiencing viral infection. Lower inflammation (i.e., lower leucopenia and lower cytokine induction) was reported in mice infected with influenza virus at TT compared to ST, despite the presence of equivalent viral titers in both groups (20). The animals at TT also exhibited a fever-response and better sleep recuperation (20). It has also been shown that mice infected with rabies virus at 37°C had lower mortality rates and lower brain-viral burden than those at 24°C (60). Similar results were obtained with mice infected with Coxsackie virus at 36°C compared to 25°C (61). In addition, mice housed at 36°C responded better to a pre-challenge live-vaccine (61). Interestingly, the effect observed at TT was absent in animals treated with an immune-suppressor, suggesting that the change in survival and tissue-burden was mediated by increased immune cell competence at TT (61). In another study, mice at TT that received a foot-and-mouth DNA vaccine had a superior immune response, with increased IgG levels, T cell proliferation, IFNγ production in both CD4+ and CD8+ T cells, and IL-4 production in CD4+ T cells, compared to heat-stressed mice at 38°C (62). However, this response was not tested at ST and the temperature defined by researchers as TT (24°C) was lower than the generally recognized TN range for mice (5, 62). There may be viral infections in which TN is detrimental for the host, but these have not been reported in the literature.
There are important limitations in the investigations of the TN effect on the response to viral infections. The first is the wide range of temperatures defined as being thermoneutral (20, 62). In addition, as with studies on bacterial infections, mouse strain, age, sex, and acclimatization time should be taken into account in future studies. Use of additional viral disease models will be required to gain a better understanding of TN on the immune response to viruses.
Parasite Infections
The effects of TN on the outcome of parasitic infections are still largely unraveled. However, a few studies investigating the role of temperature on parasitized murine hosts offer insights into potential consequences of TN housing on parasitic disease models (67–72).
Malaria-causing parasites belonging to the Plasmodium genus have a complex life cycle (75). The species that infect mammals incorporate temperature changes that occur during transmission between invertebrate to vertebrate hosts into their development (75). The blood-stage of these parasites causes the majority of malaria-associated symptoms, including fever, which also has consequences on the parasite (75). Thus, housing Plasmodium-infected mice at TT could have significantly different clinical presentation compared to those housed at ST. There is some evidence to support this possibility in the literature. For example, mice exposed to cold temperatures had an increase in parasitemia after 4 days, suggesting that parasitemia may diminish at TT (67). In addition, blood-stage parasite development may be affected by TT. Rats infected with two different strains of Plasmodium berghei had schizont-stage parasites of smaller sizes when exposed to colder temperatures (12°C) compared to TT, highlighting a potential protective effect of lower temperatures (68).This difference was not observed in rats housed at 20°C (68). Housing density also seems to be a factor in the outcome of Plasmodium infection. Mice housed in groups of five at TT (27°C) had higher mortality rates than those housed individually. The latter had the same mortality rates as mice housed at ST (21°C) in groups of five, but higher rates than those housed individually at 21°C (70). The study investigators concluded that the group-housed mice were probably more susceptible to disease at both temperatures because of their inability to dissipate body heat during the fever-response induced by the blood-stage of malaria (70). In this context, TT would be expected to exacerbate the malarial symptoms. In order to gain a better understanding of TN and malarial disease, other aspects of this disease such as its role on sequestration or spleen megalopathy should be investigated.
It is unclear whether malarial disease clinical signs would be diminished or exacerbated at TN. However, higher temperatures seem to have a protective effect against infections with Trypanosoma cruzi, the causative agent of Chagas disease. Mice infected with the parasite at 35°C survived and overcame the infection, whereas those infected at 26°C experienced chronic infections (71). Similar results were obtained in a different mouse strain, where a reduction of parasitemia and tissue pathology, and an increase in immune responsiveness was observed (72). In both studies, the protective effects of high temperatures were completely reversed by treatment with immunosuppressors or depletion of CD8+ immune cells, implicating the competence of immune cells at TT (71, 72).
Finally, the environmental temperature of the host has been tested on an infection caused by a third type of parasite: Leishmania mexicana (69). Balb/C mice at 32°C had inoculation site nodular inflammation with macrophage infiltration and phagocytosis presentation whereas those at 22°C had a more cutaneous presentation with mast cell infiltration (69). Both showed visceralization as confirmed by parasite culture from lesions, immunohistology, and PCR (69). However, the mice were not conditioned to the different temperatures beforehand (69).
The parasitic infections described above only represent a minor fraction of all parasitic diseases that could be impacted by TT. For each of those diseases, a wide variety of models exist, constituted of various mouse strain and parasite species/strain combinations that can be investigated at TN. In addition, many of the studies described above did not acclimatize the animals to the temperature conditions, which could bias the results (68, 69, 72). Murine host sex and age differences, as well as difference in acclimatization time and caging system will also have to be taken into consideration when conducting future experiments.
Cancer
TN has been investigated more frequently in cancer than in other non-metabolic diseases. Housing at TT has been shown to confer tumor-growth resistance in mice with various types of cancers (34, 54, 55). For example, mice inoculated with skin melanoma, colon carcinoma, pancreatic carcinoma, or mammary gland adenoma tumor-cells all had lower tumor formation, growth, and metastasis at TT compared to ST (54). In another study, mice inoculated with mammary gland adenoma had smaller tumors size at TT compared to ST (55). This effect was determined to involve a change in number, activity and function of CD8+ T cells and dendritic cells at higher temperatures (54, 55). A ST environment increases the number of immature or incomplete phenotype DCs, while at TT, CD8+ T cells are more likely to be activated and express IFNγ (54, 55). Also, mice housed at ST exhibited cold stress accompanied by elevated levels of NE that decreased the energy available for their immune system and reduced their response to tumor cells (4, 56, 58, 59, 76). Mohammadpour et al., showed that mammary gland adenoma murine models, circulating NE released under ST conditions activated MDSCs through their β-AR, resulting in decreased T cells proliferation and increased tumor growth (34). This effect was reversed, in β-AR knockout mice at ST or with mice treated with β-AR agonists at TT (34). The beneficial effect of TN housing was further demonstrated by the preference for that temperature in tumor-bearing mice when given the choice (54). The effect of cold stress at ST is even stronger in individually ventilated cages (IVC), a system that is widely used in the current laboratory settings (77). Mice housed in IVCs had smaller tumors, lower tumor metabolism, larger adrenal weights, and more signs of cold stress than those housed in a static system at the same temperature (77). This was somewhat reduced when the mice were given the opportunity to shelter with nesting material (77).
TN may have a positive influence on various cancer therapies. Mice with colon adenocarcinoma had lower responses to irradiation and chemotherapy at ST compared to TT (56). Mice bearing pancreatic tumors had higher sensitivity to cytotoxic treatments at TT compared to ST (25). Melanoma tumor-bearing mice had lower tumor growth after 3 weeks of aquatic exercise at TT compared to ST (57). This response was attributed to an enhanced cytotoxic-cell mediated immune response characterized by increases in populations of natural killer cells; natural killer T cells; γδT cells; CD8+ T cells; and, IFN-γ at the higher temperatures (57). In addition, resistance to treatments at ST was mostly attributed to elevated levels of NE because treatment with beta-blockers resulted in responses similar to those observed at TT (4, 25, 56, 58, 59, 76). Interestingly, higher levels of anti-apoptotic molecules (BAD, MCL-1, BCL-2, and BCLxL) and CREB, a transcription factor involved in the survival of these molecules were seen at ST compared to TT, which may also contributed to lower treatment efficacy at ST (25).
An irradiation-induced cancer model displayed a higher propensity for hematopoietic stem cells to undergo apoptosis when the animal received total body irradiation at TT compared to ST (78). Therefore, investigation of tumorigenesis in mouse models only at ST is likely to limit our understanding of this process.
The studies described above were limited to certain types of cancers and do not exclude that some cancer types would react differently to a TT environment. Also, different acclimatization times for the cage temperatures in the studies were likely to affect mouse metabolism differently (25, 54–56, 76, 78).
Metabolic Diseases
Most studies conducted at TT have been on obesity-related research. Obesity can be induced in mice by providing them with an ad libitum high fat diet (HFD) (79). As mentioned in the introduction, the mouse metabolism is less active at TT with evidence of lower fasting glucose levels, decreased food intake, lower energy expenditure, and decreased BAT activation because the animal does not have to generate heat (21–24, 45). These diet-induced changes occur independently of the type of diet fed to mice (45). However, some diet-based differences occur at TT. In most HFD-obese mice models, mice housed at TT have lower glucose clearance, higher adiposity, increased hepatic fat accumulation, increased adipose inflammation, and greater glucose intolerance than mice at ST fed the same diet (45, 46). This suggests that housing at TT exacerbates the establishment of obesity.
Diet-dependent metabolic changes are closely related to the reduction of the NE-mediated BAT activation pathway at TT. Disruption of the pathway by gene knock-out or treatment with exogenous disruptors in mice housed at ST leads to similar results (29, 32, 49, 50, 80–82). For example, when C57BL6 mice were treated with BMP7, an inducer of BAT differentiation, they displayed increased food intake; increased BAT weights; increased expression of ucp-1, CD36 and lipase; increased energy expenditure; lower WAT weights, and higher lipolysis activity at ST, but not at TT (80). UCP-1 KO mice have been used to study the establishment of obesity because they were thought to be resistant to it when fed an HFD (50). However, it was shown that this resistance disappeared at TT because of a decrease in metabolic efficiency (81, 82). However, these KO mice did not exhibit adipose tissue inflammation at TT compared to ST, which suggests that BAT inflammation is due to exposure to cold and not to accumulation of fat (32). In addition, disruption of TH activity by knocking-out the type 2 deiodinase enzyme required for its activation had a similar effect as knocking-out UCP-1 (49). The obesity phenotype was decreased at ST, but increased at TT due to the inability of those mice to activate diet-induced thermogenesis compared to WT mice (49).
Thermoneutrality also has an effect on obesity-related disease presentations such as atherosclerosis and non-alcoholic fatty liver disease (NAFLD) (44, 51, 52, 83). Atherosclerosis is defined as plaque build-up coupled with immune cell infiltration in the blood vessel wall and is one of the leading causes of cardiovascular disease (CVD) (83). It occurs when there is an increase of lipids in the circulation as a result of changes in the metabolic environment (83). When housed at TT, mice display two metabolic markers of atherosclerosis (i.e., adipose and vasculature inflammation) faster than at ST, even if there is no change in insulin resistance (44, 51). Macrophage infiltration in the aorta and upregulation of pro-inflammatory cytokines, chemokines and other inflammatory mediators were observed at TT (51). In addition, the combination of a low metabolism at TN and a HFD initiated atherosclerosis in WT mice by altering blood lipid profiles and increasing aortic plaque size (83). In mice that are predisposed to atherosclerosis, the disease was exacerbated by the same combination (83). These results suggest that the link between adipose tissue inflammation and insulin-resistance only occur in cold stressed mice and thus are not applicable to our understanding of human atherosclerosis (51). Diet-induced NAFLD is the most common chronic liver disease and may lead to complications, the need for liver transplantation, and death (52). Female mice were thought to be resistant to the establishment of the disease due to hormonal differences (52). However, multiple strains of mice housed at TT and fed a HFD had lower corticosterone production; upregulated pro-inflammatory cytokines; and, exacerbated diet-induced NAFLD, independently of sex (52). Sex-independent increases in intestinal permeability and an altered microbiome were also observed at TT. Depletion of gram-negative bacteria, TLR4 deletion, or IL-17 axis activation reversed all TT-associated effects (52). This strongly implicates a role for environmental temperature on the composition of the gut microbiome in the establishment of NAFLD because the skewing of gram-negative bacteria at TT increases intestinal permeability, which in turn causes more LPS to interact with TLR4 to increase inflammation (52). This is particularly relevant because the small intestine is remodeled by the microbiome during cold stress acclimatization (53). These results led some researchers to speculate that the mouse microbiome is different at TT compared to ST, but more studies should be conducted to establish the effect of TN on the microbiome (8). Housing temperature does not seem to influence joint replacement therapy failure models (84). Joint replacement is used to treat joint degenerative disease. Wear and tear on the joint as well as lower circulating levels of leptin in obese individuals are thought to play a role on osteolysis leading to joint replacement failure (84). However, obese (ob/ob) mouse models are resistant to the development of osteolysis at ST and TT suggesting that this effect is independent of TN (84).
Finally, some of the proposed obesity treatments have different outcomes at TT compared to ST (47, 48). Because obese mice treated with DNP, a weight loss drug, had no reduction in obesity phenotype at ST, the drug was initially thought to be ineffective (47). However, although treatment with DNP for 4 weeks at TT did not affect food intake, energy expenditure increased; body weight and fat mass decreased; glucose tolerance improved; steatosis was reduced; and decreases were observed in TH levels, UCP-1 expression and BAT thermogenesis (47). In addition, when mice underwent weight reduction surgery, they showed similar energy reduction at ST and TT, but those at TT showed lower energy basal demand at TT (48). They also exhibited less torpor, defined as a depression in all physiological functions at TT, suggesting that TN improved the outcomes of surgery by enabling mice to recover better (48).
The limitations of these studies include different temperatures; mice models; and, acclimatization times used in different experiments. These difference may explain why some contradictory effects where observed in different studies such as the presence or absence of temperature-dependent glucose tolerance at TT (45, 46).
Cardiovascular Diseases
There are clear links in rodents between metabolic activity and changes in cardiovascular system activity that can predispose to CVD. Several studies showed that the metabolism of rodents at TT changes some of their cardiovascular parameters (22, 85–87). Mice and rats housed at temperatures oscillating between ST and TT, had lower mean blood pressure; heart rate; and, pulse pressure when the cage temperature was closer to TT as a consequence of lower thermogenic demands at TT (11, 85). The differences were more significant in mice than rats, most likely due to their greater metabolic demands (69). Similar results were also obtained for mice and rats housed at TT compared to ST (22, 87). These changes are clearly representative of a change in metabolism since they were observed with greater intensity when mice at TT were fasting (86). Oxygen consumption was unaffected at TT for mice but decreased for rats (86). These changes can predispose rodents to cardiovascular events like coronary artery disease or atherosclerosis, described in the Metabolic Diseases section (44, 51). It is not yet clear whether TN has a protective effect on other CVDs.
Alzheimer’s Disease
The impact of TN on the central nervous system has been studied in the context of Alzheimer’s disease (AD) (74). Vandal et al. found that the triple-transgenic mouse model of AD (3×Tg-AD) was more susceptible to a cold environment despite having a higher non-shivering thermogenesis activity than WT mice, thus suggesting that ST would play a greater impact on these mice (74). At TT, the memory function and neuropathology of 3xTg-AD mice was improved within a week compared to 3xTg-AD mice housed at ST (74).
Opposing Views to the Thermoneutral Model
Most of the current literature on TN advocates for conducting experiments on mice in housing at approximately 30°C since it is within the TNZ of mice (1, 3–6, 8, 13, 58, 88, 89). Thus results obtained at this temperature are more likely to be consistent with the metabolic state experienced by humans (1, 3–6, 8, 13, 58, 88, 89). However, some investigators have argued that the state of mice at this temperature is not representative of the average human metabolic state and experiments conducted at 30°C cannot be extrapolated to humans (90). In their review, Speakman et al. state that most humans function in environments that are on average 3°C below their lower critical temperature (Tlc) (90). This means that they occupy a space at a temperature that is below the lowest temperature within their TNZ (90). This metabolic state in humans corresponds to about 23–25°C in mice (90). In addition, Speakman et al. argue that mice are routinely given the opportunity to shelter and huddle in group housing, which increases their BCT (90). In this context, the researchers claim that the Tlc of the mice is further reduced to 20–22°C (90). One of the assumptions of this argument is that the murine response to change in environmental temperature is the same as the human response (90). However, as we mentioned in the introductory section of this paper, the metabolism of mice and humans is very different. Mice have a higher metabolic demand due to their smaller SA/V ratio and they rely more heavily on non-shivering thermogenesis than humans (1, 5). In addition, there is substantial evidence that humans mostly operate at a temperature within their TNZ (3, 11, 13). Thus, we are in agreement with the belief held by other investigators that more murine studies should be conducted at TT and the results compared to those obtained at ST. This will provide a better understanding of mouse disease models and refine their use for application to human health.
Conclusion
As outlined in the Introduction, very few results obtained in mouse studies translate to human trials (9, 12, 16). This is generally attributed to biological differences between mice and humans and has led to calls by the scientific community to improve the murine models used to study human diseases (9, 12). One major aspect of studies with murine models that has been overlooked is the housing environment. Besides the consequences due to social stressors and microbiome differences, housing temperature also plays a role in the response to immune challenge (12, 16). Sub-optimal housing temperatures routinely used in the laboratory setting induce metabolic activity for heat generation, reducing energy available for metabolism of other biological functions like the immune system (5). The differing metabolic states of mice housed at ST and TT are likely to complicate our understanding of disease in murine models (Figure 2) (5). In this review, we illustrated that housing at TN could either exacerbate or have protective effects in the context of bacterial, viral and parasitic infections, as well as in metabolic, cardiovascular and cancer diseases. We also showed the differential effect of TT compared to ST housing in Alzheimer’s disease, respiratory disease, and graft vs. host disease. Taking into consideration the various original research articles and independent reviews done on the subject, we feel that it is safe to assume that housing temperature can influence the outcome of studies on murine models of disease and therefore affect our understanding of human diseases, in spite of confounding factors linked to sheltering material and group “huddling” (17, 90). We conclude that there is an advantage to investigating the role of TN in various disease models and comparing results to those obtained at ST in an effort to improve our understanding of human diseases.
Author Contributions
FV and MO discussed and prepared the plan of the review. FV did the majority of the literature search and wrote the first draft of the review. FV and MO developed and drew the figures and table together. FV and MO edited the text and the overall presentation of the review. All authors contributed to the article and approved the submitted version.
Funding
Research in the MO laboratory is supported by grants from the Canadian Institute for Health Research (Grant #159765) and the Natural Science and Engineering Research Council of Canada (Grant RGPIN/03863-2017).
Conflict of Interest
The authors declare that the research was conducted in the absence of any commercial or financial relationships that could be construed as a potential conflict of interest.
Acknowledgments
FV is the recipient of a Banting and Best M.Sc. Studentship from the Canadian Institute of Health Research.
References
1. Gordon CJ. Thermal physiology of laboratory mice: defining thermoneutrality. J Thermal Biol (2012) 37(8):654–85. doi: 10.1016/j.jtherbio.2012.08.004
2. Poczopko P. Studies on the effect of environmental thermal conditions on geese: deep and external body temperature in goslings at thermoneutrality and during short exposures to cold. Acta Physiol Pol (1968) 19(5):719–35.
3. Maloney SK, Fuller A, Mitchell D, Gordon C, Overton JM. Translating animal model research: does it matter that our rodents are cold? Physiol (Bethesda) (2014) 29(6):413–20. doi: 10.1152/physiol.00029.2014
4. Hylander BL, Repasky EA. Thermoneutrality, mice, and cancer: a heated opinion. Trends Cancer (2016) 2(4):166–75. doi: 10.1016/j.trecan.2016.03.005
5. Gordon CJ. The mouse thermoregulatory system: its impact on translating biomedical data to humans. Physiol Behav (2017) 179:55–66. doi: 10.1016/j.physbeh.2017.05.026
6. Overton JM. Phenotyping small animals as models for the human metabolic syndrome: thermoneutrality matters. Int J Obes (Lond) (2010) 34 Suppl 2:S53–8. doi: 10.1038/ijo.2010.240
7. Kingma B, Frijns A, van Marken Lichtenbelt W. The thermoneutral zone: implications for metabolic studies. Front Biosci (Elite Ed) (2012) 4:1975–85. doi: 10.2741/e518
8. Ganeshan K, Chawla A. Warming the mouse to model human diseases. Nat Rev Endocrinol (2017) 13(8):458–65. doi: 10.1038/nrendo.2017.48
9. Landis SC, Amara SG, Asadullah K, Austin CP, Blumenstein R, Bradley EW, et al. A call for transparent reporting to optimize the predictive value of preclinical research. Nat (2012) 490(7419):187–91. doi: 10.1038/nature11556
10. Richter SH, Garner JP, Würbel H. Environmental standardization: cure or cause of poor reproducibility in animal experiments? Nat Methods (2009) 6(4):257–61. doi: 10.1038/nmeth.1312
11. Cannon B, Nedergaard J. Nonshivering thermogenesis and its adequate measurement in metabolic studies. J Exp Biol (2011) 214(2):242–53. doi: 10.1242/jeb.050989
12. Troublesome variability in mouse studies. Nat Neurosci (2009) 12(9):1075. doi: 10.1038/nn0909-1075
13. Karp CL. Unstressing intemperate models: how cold stress undermines mouse modeling. J Exp Med (2012) 209(6):1069–74. doi: 10.1084/jem.20120988
14. Bass J, Lazar MA. Circadian time signatures of fitness and disease. Science (2016) 354(6315):994–9. doi: 10.1126/science.aah4965
15. Longo VD, Panda S. Fasting, circadian rhythms, and time-restricted feeding in healthy lifespan. Cell Metab (2016) 23(6):1048–59. doi: 10.1016/j.cmet.2016.06.001
16. Turner PV. The role of the gut microbiota on animal model reproducibility. Anim Models Exp Med (2018) 1(2):109–15. doi: 10.1002/ame2.12022
17. Gordon CJ, Aydin C, Repasky EA, Kokolus KM, Dheyongera G, Johnstone AFM. Behaviorally mediated, warm adaptation: a physiological strategy when mice behaviorally thermoregulate. J Thermal Biol (2014) 44:41–6. doi: 10.1016/j.jtherbio.2014.06.006
18. Okon EE. The effect of environmental temperature on the production of ultrasounds by isolated non-handled albino mouse pups. J Zool (1970) 162(1):71–83. doi: 10.1111/j.1469-7998.1970.tb01258.x
19. Gaskill BN, Rohr SA, Pajor EA, Lucas JR, Garner JP. Some like it hot: mouse temperature preferences in laboratory housing. Appl Anim Behav Sci (2009) 116(2):279–85. doi: 10.1016/j.applanim.2008.10.002
20. Jhaveri KA, Trammell RA, Toth LA. Effect of environmental temperature on sleep, locomotor activity, core body temperature and immune responses of C57BL/6J mice. Brain Behav Immun (2007) 21(7):975–87. doi: 10.1016/j.bbi.2007.03.007
21. Kaiyala KJ, Morton GJ, Thaler JP, Meek TH, Tylee T, Ogimoto K, et al. Acutely decreased thermoregulatory energy expenditure or decreased activity energy expenditure both acutely reduce food intake in mice. PloS One (2012) 7(8):e41473. doi: 10.1371/journal.pone.0041473
22. Lo Martire V, Silvani A, Bastianini S, Berteotti C, Zoccoli G. Effects of ambient temperature on sleep and cardiovascular regulation in mice: the role of hypocretin/orexin neurons. PloS One (2012) 7(10):e47032. doi: 10.1371/journal.pone.0047032
23. David JM, Chatziioannou AF, Taschereau R, Wang H, Stout DB. The hidden cost of housing practices: using noninvasive imaging to quantify the metabolic demands of chronic cold stress of laboratory mice. Comp Med (2013) 63(5):386–91.
24. Jun JC, Shin MK, Yao Q, Devera R, Fonti-Bevans S, Polotsky VY. Thermoneutrality modifies the impact of hypoxia on lipid metabolism. Am J Physiol Endocrinol Metab (2013) 304(4):E424–35. doi: 10.1152/ajpendo.00515.2012
25. Eng JW, Reed CB, Kokolus KM, Pitoniak R, Utley A, Bucsek MJ, et al. Housing temperature-induced stress drives therapeutic resistance in murine tumour models through beta2-adrenergic receptor activation. Nat Commun (2015) 6:6426. doi: 10.1038/ncomms7426
26. Iwaniec UT, Philbrick KA, Wong CP, Gordon JL, Kahler-Quesada AM, Olson DA, et al. Room temperature housing results in premature cancellous bone loss in growing female mice: implications for the mouse as a preclinical model for age-related bone loss. Osteoporosis Int (2016) 27(10):3091–101. doi: 10.1007/s00198-016-3634-3
27. Szentirmai E, Kapas L. Sleep and body temperature in TNFalpha knockout mice: the effects of sleep deprivation, beta3-AR stimulation and exogenous TNFalpha. Brain Behav Immun (2019) 81:260–71. doi: 10.1016/j.bbi.2019.06.022
28. Leigh ND, Kokolus KM, O’Neill RE, Du W, Eng JW, Qiu J, et al. Housing temperature-induced stress is suppressing murine graft-versus-host disease through beta2-adrenergic receptor signaling. J Immunol (2015) 195(10):5045–54. doi: 10.4049/jimmunol.1500700
29. Xiao C, Goldgof M, Gavrilova O, Reitman ML. Anti-obesity and metabolic efficacy of the beta3-adrenergic agonist, CL316243, in mice at thermoneutrality compared to 22 degrees C. Obes (Silver Spring) (2015) 23(7):1450–9. doi: 10.1002/oby.21124
30. Eng JW, Reed CB, Kokolus KM, Repasky EA. Housing temperature influences the pattern of heat shock protein induction in mice following mild whole body hyperthermia. Int J Hyperthermia (2014) 30(8):540–6. doi: 10.3109/02656736.2014.981300
31. Ruiz de Azua I, Mancini G, Srivastava RK, Rey AA, Cardinal P, Tedesco L, et al. Adipocyte cannabinoid receptor CB1 regulates energy homeostasis and alternatively activated macrophages. J Clin Invest (2017) 127(11):4148–62. doi: 10.1172/JCI83626
32. Bond LM, Burhans MS, Ntambi JM. Uncoupling protein-1 deficiency promotes brown adipose tissue inflammation and ER stress. PloS One (2018) 13(11):e0205726. doi: 10.1371/journal.pone.0205726
33. Nguyen KD, Qiu Y, Cui X, Goh YP, Mwangi J, David T, et al. Alternatively activated macrophages produce catecholamines to sustain adaptive thermogenesis. Nature (2011) 480(7375):104–8. doi: 10.1038/nature10653
34. Mohammadpour H, MacDonald CR, Qiao G, Chen M, Dong B, Hylander BL, et al. β2 adrenergic receptor–mediated signaling regulates the immunosuppressive potential of myeloid-derived suppressor cells. J Clin Invest (2019) 129(12):5537–52. doi: 10.1172/JCI129502
35. Mohammadpour H, Sarow JL, Chen GL, MacDonald CR, Sharma U, Herr MM, et al. β2- Adrenergic Signaling Regulates Graft Versus Host Disease after Allogenic Transplantation While Preserving Graft Versus Leukemia Effect. Blood (2019) 134(Supplement_1):1915–. doi: 10.1182/blood-2019-123139
36. Lee MW, Odegaard JI, Mukundan L, Qiu Y, Molofsky AB, Nussbaum JC, et al. Activated type 2 innate lymphoid cells regulate beige fat biogenesis. Cell (2015) 160(1-2):74–87. doi: 10.1016/j.cell.2014.12.011
37. Rudaya AY, Steiner AA, Robbins JR, Dragic AS, Romanovsky AA. Thermoregulatory responses to lipopolysaccharide in the mouse: dependence on the dose and ambient temperature. Am J Physiol Regul Integr Comp Physiol (2005) 289(5):R1244–52. doi: 10.1152/ajpregu.00370.2005
38. Wanner SP, Yoshida K, Kulchitsky VA, Ivanov AI, Kanosue K, Romanovsky AA. Lipopolysaccharide-induced neuronal activation in the paraventricular and dorsomedial hypothalamus depends on ambient temperature. PloS One (2013) 8(9):e75733. doi: 10.1371/journal.pone.0075733
39. Carroll JA, Burdick NC, Chase CC Jr., Coleman SW, Spiers DE. Influence of environmental temperature on the physiological, endocrine, and immune responses in livestock exposed to a provocative immune challenge. Domest Anim Endocrinol (2012) 43(2):146–53. doi: 10.1016/j.domaniend.2011.12.008
40. Peloso ED, Florez-Duquet M, Buchanan JB, Satinoff E. LPS fever in old rats depends on the ambient temperature. Physiol Behav (2003) 78(4-5):651–4. doi: 10.1016/S0031-9384(03)00046-5
41. Buchanan JB, Peloso E, Satinoff E. A warmer ambient temperature increases the passage of interleukin-1beta into the brains of old rats. Am J Physiol Regul Integr Comp Physiol (2008) 295(1):R361–8. doi: 10.1152/ajpregu.00104.2007
42. Ganeshan K, Nikkanen J, Man K, Leong YA, Sogawa Y, Maschek JA, et al. Energetic trade-offs and hypometabolic states promote disease tolerance. Cell (2019) 177(2):399–413.e12. doi: 10.1016/j.cell.2019.01.050
43. Hamzic N, Blomqvist A, Nilsberth C. Immune-induced expression of lipocalin-2 in brain endothelial cells: relationship with interleukin-6, cyclooxygenase-2 and the febrile response. J Neuroendocrinol (2013) 25(3):271–80. doi: 10.1111/jne.12000
44. Personnaz J, Piccolo E, Branchereau M, Filliol A, Paccoud R, Moreau E, et al. Macrophage-derived HMGB1 is dispensable for tissue fibrogenesis. FASEB Bioadv (2019) 1(4):227–45. doi: 10.1096/fba.2018-00035
45. Clayton ZS, McCurdy CE. Short-term thermoneutral housing alters glucose metabolism and markers of adipose tissue browning in response to a high-fat diet in lean mice. Am J Physiol Regul Integr Comp Physiol (2018) 315(4):R627–r37. doi: 10.1152/ajpregu.00364.2017
46. Stemmer K, Kotzbeck P, Zani F, Bauer M, Neff C, Muller TD, et al. Thermoneutral housing is a critical factor for immune function and diet-induced obesity in C57BL/6 nude mice. Int J Obes (Lond) (2015) 39(5):791–7. doi: 10.1038/ijo.2014.187
47. Goldgof M, Xiao C, Chanturiya T, Jou W, Gavrilova O, Reitman ML. The chemical uncoupler 2,4-dinitrophenol (DNP) protects against diet-induced obesity and improves energy homeostasis in mice at thermoneutrality. J Biol Chem (2014) 289(28):19341–50. doi: 10.1074/jbc.M114.568204
48. Ravussin Y, LeDuc CA, Watanabe K, Leibel RL. Effects of ambient temperature on adaptive thermogenesis during maintenance of reduced body weight in mice. Am J Physiol Regul Integr Comp Physiol (2012) 303(4):R438–48. doi: 10.1152/ajpregu.00092.2012
49. Castillo M, Hall JA, Correa-Medina M, Ueta C, Kang HW, Cohen DE, et al. Disruption of thyroid hormone activation in type 2 deiodinase knockout mice causes obesity with glucose intolerance and liver steatosis only at thermoneutrality. Diabetes (2011) 60(4):1082–9. doi: 10.2337/db10-0758
50. Liu X, Rossmeisl M, McClaine J, Riachi M, Harper M-E, Kozak LP. Paradoxical resistance to diet-induced obesity in UCP1-deficient mice. J Clin Invest (2003) 111(3):399–407. doi: 10.1172/JCI200315737
51. Tian XY, Ganeshan K, Hong C, Nguyen KD, Qiu Y, Kim J, et al. Thermoneutral housing accelerates metabolic inflammation to potentiate atherosclerosis but not insulin resistance. Cell Metab (2016) 23(1):165–78. doi: 10.1016/j.cmet.2015.10.003
52. Giles DA, Moreno-Fernandez ME, Stankiewicz TE, Graspeuntner S, Cappelletti M, Wu D, et al. Thermoneutral housing exacerbates nonalcoholic fatty liver disease in mice and allows for sex-independent disease modeling. Nat Med (2017) 23(7):829–38. doi: 10.1038/nm.4346
53. Chevalier C, Stojanović O, Colin DJ, Suarez-Zamorano N, Tarallo V, Veyrat-Durebex C, et al. Gut microbiota orchestrates energy homeostasis during cold. Cell (2015) 163(6):1360–74. doi: 10.1016/j.cell.2015.11.004
54. Kokolus KM, Capitano ML, Lee CT, Eng JW, Waight JD, Hylander BL, et al. Baseline tumor growth and immune control in laboratory mice are significantly influenced by subthermoneutral housing temperature. Proc Natl Acad Sci USA (2013) 110(50):20176–81. doi: 10.1073/pnas.1304291110
55. Kokolus KM, Spangler HM, Povinelli BJ, Farren MR, Lee KP, Repasky EA. Stressful presentations: mild cold stress in laboratory mice influences phenotype of dendritic cells in naive and tumor-bearing mice. Front Immunol (2014) 5:23 doi: 10.3389/fimmu.2014.00023.
56. MacDonald CR, Bucsek MJ, Qiao G, Chen M, Evans L, Greenberg DJ, et al. Adrenergic receptor signaling regulates the response of tumors to ionizing radiation. Radiat Res (2019) 191(6):585–9. doi: 10.1667/RR15193.1
57. Lee B, Kim G, Jo Y, Lee B, Shin YI, Hong C. Aquatic exercise at thermoneutral water temperature enhances antitumor immune responses. Immune Netw (2019) 19(2):e10. doi: 10.4110/in.2019.19.e10
58. Hylander BL, Eng JW, Repasky EA. The impact of housing temperature-induced chronic stress on preclinical mouse tumor models and therapeutic responses: an important role for the nervous system. Adv Exp Med Biol (2017) 1036:173–89. doi: 10.1007/978-3-319-67577-0_12
59. Hylander BL, Gordon CJ, Repasky EA. Manipulation of ambient housing temperature to study the impact of chronic stress on immunity and cancer in mice. J Immunol (2019) 202(3):631–6. doi: 10.4049/jimmunol.1800621
60. Bell JF, Moore GJ. Effects of high ambient temperature on various stages of rabies virus infection in mice. Infect Immun (1974) 10(3):510–5. doi: 10.1128/IAI.10.3.510-515.1974
61. Underwood GE, Baker CA, Weed SD. Protective effect of elevated temperature on mice infected with Coe virus. J Immunol (1966) 96(6):1006–12.
62. Hu Y, Jin H, Du X, Xiao C, Luo D, Wang B, et al. Effects of chronic heat stress on immune responses of the foot-and-mouth disease DNA vaccination. DNA Cell Biol (2007) 26(8):619–26. doi: 10.1089/dna.2007.0581
63. Liu E, Lewis K, Al-Saffar H, Krall CM, Singh A, Kulchitsky VA, et al. Naturally occurring hypothermia is more advantageous than fever in severe forms of lipopolysaccharide- and Escherichia coli-induced systemic inflammation. Am J Physiol Regul Integr Comp Physiol (2012) 302(12):R1372–83. doi: 10.1152/ajpregu.00023.2012
64. Previte JJ, Berry LJ. The effect of environmental temperature on the host-parasite relationship in mice. J Infect Dis (1962) 110(3):201–9. doi: 10.1093/infdis/110.3.201
65. Moragues V, Pinkerton H. Variation in morbidity and mortality of murine typhus infection in mice with changes in the environmental temperature. J Exp Med (1944) 79(1):41–3. doi: 10.1084/jem.79.1.41
66. Rubin RL. Mice housed at elevated vivarium temperatures display enhanced T-cell response and survival to Francisella tularensis. Comp Med (2017) 67(6):491–7.
67. McQuisition TE. Effect of temperature and clofibrate on Plasmodium berghei infection in mice. Am J Trop Med Hyg (1979) 28(1):12–4. doi: 10.4269/ajtmh.1979.28.12
68. Yoeli M, Young C, Jadin JB. Effects of lowered environmental temperature on the growth of exoerythrocytic stages of Plasmodium berghei. Am J Trop Med Hyg (1975) 24(5):769–75. doi: 10.4269/ajtmh.1975.24.769
69. Quinonez-Diaz L, Mancilla-Ramirez J, Avila-Garcia M, Ortiz-Avalos J, Berron A, Gonzalez S, et al. Effect of ambient temperature on the clinical manifestations of experimental diffuse cutaneous leishmaniasis in a rodent model. Vector Borne Zoonotic Dis (2012) 12(10):851–60. doi: 10.1089/vbz.2011.0844
70. Plaut SM, Ader R, Friedman SB, Ritterson AL. Social factors and resistance to malaria in the mouse: effects of group vs individual housing on resistance to Plasmodium berghei infection. Psychosom Med (1969) 31(6):536–52. doi: 10.1097/00006842-196911000-00007
71. Amrein YU. Effects of environmental temperature on Trypanosoma cruzi infection in mice. J Parasitology (1967) 53(6):1160–. doi: 10.2307/3276673
72. Ming Z, Davis CD. CD8+ T lymphocytes required for enhanced survival of Trypanosoma cruzi-infected mice at elevated environmental temperature. J Parasitol (2003) 89(3):630–2. doi: 10.1645/0022-3395(2003)089[0630:CTLRFE]2.0.CO;2
73. Liao W, Zhou L, Zhao X, Song L, Lu Y, Zhong N, et al. Thermoneutral housing temperature regulates T-regulatory cell function and inhibits ovabumin-induced asthma development in mice. Sci Rep (2017) 7(1):7123. doi: 10.1038/s41598-017-07471-7
74. Vandal M, White PJ, Tournissac M, Tremblay C, St-Amour I, Drouin-Ouellet J, et al. Impaired thermoregulation and beneficial effects of thermoneutrality in the 3xTg-AD model of Alzheimer’s disease. Neurobiol Aging (2016) 43:47–57. doi: 10.1016/j.neurobiolaging.2016.03.024
75. Oakley MS, Gerald N, McCutchan TF, Aravind L, Kumar S. Clinical and molecular aspects of malaria fever. Trends Parasitol (2011) 27(10):442–9. doi: 10.1016/j.pt.2011.06.004
76. Qiao G, Chen M, Bucsek MJ, Repasky EA, Hylander BL. Adrenergic signaling: a targetable checkpoint limiting development of the antitumor immune response. Front Immunol (2018) 9:164. doi: 10.3389/fimmu.2018.00164
77. David JM, Knowles S, Lamkin DM, Stout DB. Individually ventilated cages impose cold stress on laboratory mice: a source of systemic experimental variability. J Am Assoc Lab Anim Sci (2013) 52(6):738–44.
78. Povinelli BJ, Kokolus KM, Eng JW, Dougher CW, Curtin L, Capitano ML, et al. Standard sub-thermoneutral caging temperature influences radiosensitivity of hematopoietic stem and progenitor cells. PloS One (2015) 10(3):e0120078. doi: 10.1371/journal.pone.0120078
79. Bell RR. Exercise and Diet-Induced Obesity in Mice. In: Owen JB, Treasure JL, Collier DA, editors. Animal models — disorders of eating behaviour and body composition. Dordrecht: Springer Netherlands (2001). p. 97–116.
80. Boon MR, van den Berg SA, Wang Y, van den Bossche J, Karkampouna S, Bauwens M, et al. BMP7 activates brown adipose tissue and reduces diet-induced obesity only at subthermoneutrality. PloS One (2013) 8(9):e74083. doi: 10.1371/journal.pone.0074083
81. Feldmann HM, Golozoubova V, Cannon B, Nedergaard J. UCP1 ablation induces obesity and abolishes diet-induced thermogenesis in mice exempt from thermal stress by living at thermoneutrality. Cell Metab (2009) 9(2):203–9. doi: 10.1016/j.cmet.2008.12.014
82. Klaus S, Munzberg H, Truloff C, Heldmaier G. Physiology of transgenic mice with brown fat ablation: obesity is due to lowered body temperature. Am J Physiol (1998) 274(2):R287–93. doi: 10.1152/ajpregu.1998.274.2.R287
83. Giles DA, Ramkhelawon B, Donelan EM, Stankiewicz TE, Hutchison SB, Mukherjee R, et al. Modulation of ambient temperature promotes inflammation and initiates atherosclerosis in wild type C57BL/6 mice. Mol Metab (2016) 5(11):1121–30. doi: 10.1016/j.molmet.2016.09.008
84. Philbrick KA, Branscum AJ, Wong CP, Turner RT, Iwaniec UT. Leptin increases particle-induced osteolysis in female ob/ob mice. Sci Rep (2018) 8(1):14790. doi: 10.1038/s41598-018-33173-9
85. Swoap SJ, Overton JM, Garber G. Effect of ambient temperature on cardiovascular parameters in rats and mice: a comparative approach. Am J Physiol Regul Integr Comp Physiol (2004) 287(2):R391–6. doi: 10.1152/ajpregu.00731.2003
86. Williams TD, Chambers JB, Henderson RP, Rashotte ME, Overton JM. Cardiovascular responses to caloric restriction and thermoneutrality in C57BL/6J mice. Am J Physiol Regul Integr Comp Physiol (2002) 282(5):R1459–67. doi: 10.1152/ajpregu.00612.2001
87. Overton JM, Williams TD, Chambers JB, Rashotte ME. Cardiovascular and metabolic responses to fasting and thermoneutrality are conserved in obese Zucker rats. Am J Physiol Regul Integr Comp Physiol (2001) 280(4):R1007–15. doi: 10.1152/ajpregu.2001.280.4.R1007
88. Hankenson FC, Marx JO, Gordon CJ, David JM. Effects of rodent thermoregulation on animal models in the research environment. Comp Med (2018) 68(6):425–38. doi: 10.30802/AALAS-CM-18-000049
89. Messmer MN, Kokolus KM, Eng JW, Abrams SI, Repasky EA. Mild cold-stress depresses immune responses: implications for cancer models involving laboratory mice. Bioessays (2014) 36(9):884–91. doi: 10.1002/bies.201400066
Keywords: thermoneutrality, murine model, immune functions, infectious diseases, metabolism, body temperature, immunity
Citation: Vialard F and Olivier M (2020) Thermoneutrality and Immunity: How Does Cold Stress Affect Disease? Front. Immunol. 11:588387. doi: 10.3389/fimmu.2020.588387
Received: 28 July 2020; Accepted: 26 October 2020;
Published: 20 November 2020.
Edited by:
Irun R. Cohen, Weizmann Institute of Science, IsraelReviewed by:
Elizabeth Ann Repasky, University at Buffalo, United StatesMarie-Claude Gaudreau, Bristol Myers Squibb, United States
Copyright © 2020 Vialard and Olivier. This is an open-access article distributed under the terms of the Creative Commons Attribution License (CC BY). The use, distribution or reproduction in other forums is permitted, provided the original author(s) and the copyright owner(s) are credited and that the original publication in this journal is cited, in accordance with accepted academic practice. No use, distribution or reproduction is permitted which does not comply with these terms.
*Correspondence: Martin Olivier, bWFydGluLm9saXZpZXJAbWNnaWxsLmNh