- 1Translational Gastroenterology Unit, Nuffield Department of Medicine, University of Oxford, Oxford, United Kingdom
- 2Peter Medawar Building for Pathogen Research, University of Oxford, Oxford, United Kingdom
Mucosal-associated invariant T (MAIT) cells are innate-like T cells present at considerable frequencies in human blood and barrier tissues, armed with an expanding array of effector functions in response to homeostatic perturbations. Analogous to other barrier immune cells, their phenotype and function is driven by crosstalk with host and dynamic environmental factors, most pertinently the microbiome. Given their distribution, they must function in diverse extracellular milieus. Tissue-specific and adapted functions of barrier immune cells are shaped by transcriptional programs and regulated through a blend of local cellular, inflammatory, physiological, and metabolic mediators unique to each microenvironment. This review compares the phenotype and function of MAIT cells with other barrier immune cells, highlighting potential areas for future exploration. Appreciation of MAIT cell biology within tissues is crucial to understanding their niche in health and disease.
Introduction
Mucosal-associated invariant T (MAIT) cells have been intertwined with barrier immunity ever since they were coined (1, 2). Abundant in human blood, making up to 10% of peripheral T cells, they are also enriched in tissues (2–4). MAIT cells are defined as TRAV1-2+ (TCR Vα7.2+) T cells restricted by the MHC class I-related molecule (MR1), which recognizes non-peptide riboflavin biosynthesis intermediates conserved among bacteria and fungi (5–10). All mammalian barrier surfaces are colonized by riboflavin-synthesizing commensals, and MR1 has co-evolved with MAIT cells through most mammalian evolution (11), thus being an example of barrier surfaces imprinting human immunity.
Barrier surfaces are sites of cross-talk between the host and diverse external environments. MAIT cells are found in the intestine, skin, respiratory, oral and female genital mucosa, which all house microbial communities adapted to the local environment and in symbiosis with the host (12) (Table 1). MAIT cells are dependent on this microbiome and are part of a community of tissue immune cells anatomically close to epithelial surfaces (20, 35), all poised for rapid effector functions to maintain tissue homeostasis (36, 37).
The range of MAIT cell effector functions is only just being explored. It is increasingly appreciated that resident macrophages and lymphocytes are in constant cross-talk with tissues, integrating cues from the local microbiome, cellular, environmental and metabolic milieus for their development and function (38–44). In this review, we show that MAIT cells occupy a similar niche, engage in similar cross-talk and could sense similar factors (Figure 1, Table 2). Furthermore, we draw parallels with other barrier lymphocytes to explore tissue-specific factors which could modulate their function at mucosal surfaces, with the hypothesis that there are unexplored functional and metabolic adaptions for their survival and execution of tissue-specific effector functions. Understanding these factors is important to expand our knowledge of their role in shaping tissue function in health and disease.
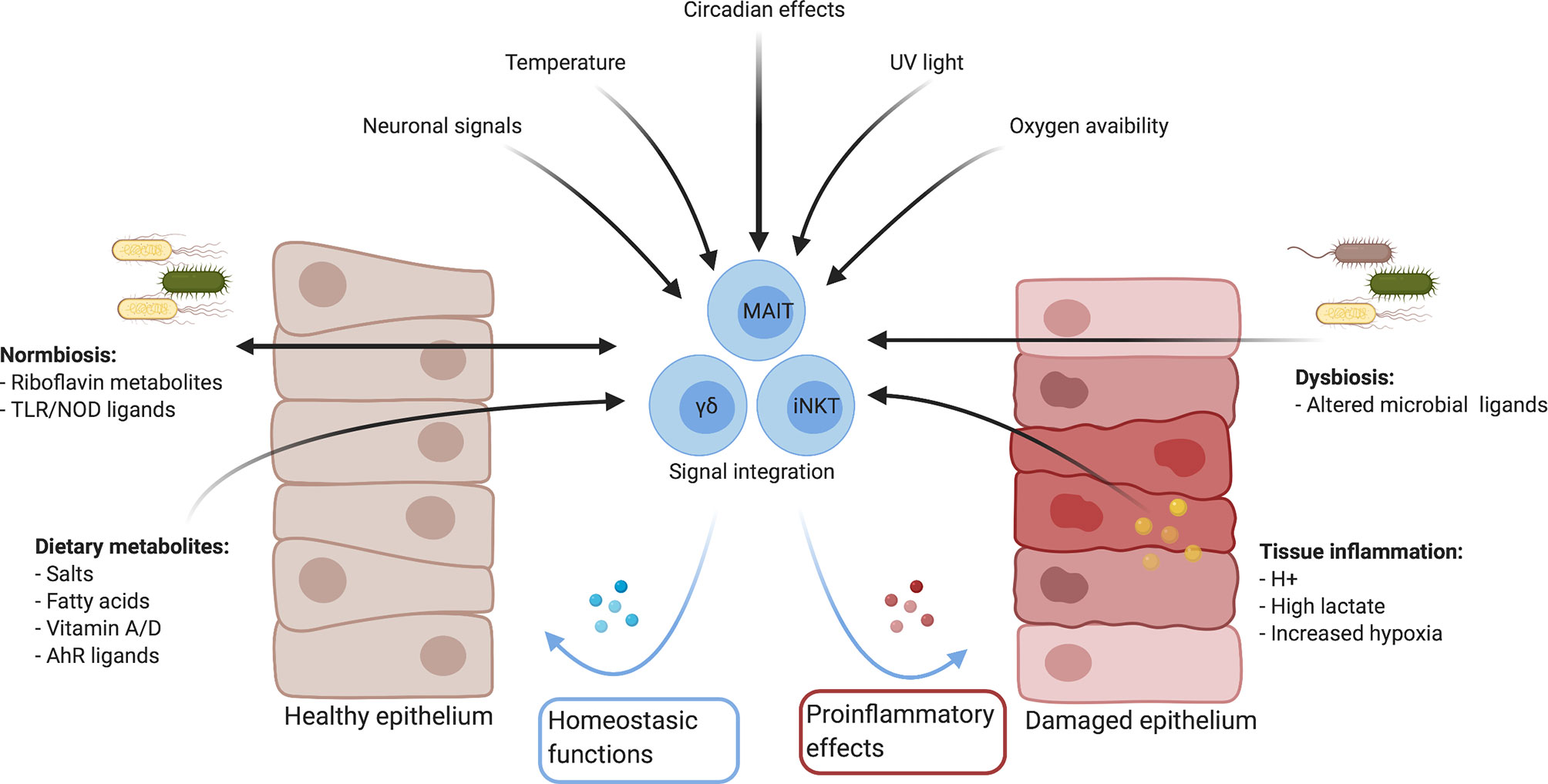
Figure 1 Summary of mucosal environmental factors which could influence immune responses directly and indirectly. Created with Biorender.com.
Barrier Tissues
Mammalian barrier surfaces are diverse physiological, chemical and cellular niches. The skin is dry, lipid-rich and acidic, primarily due to epidermal fatty acids, with unique exposures to high-salt content, UV-radiation and large fluctuations in temperature (51, 52). The female genital tract is even more stressed, driven by Lactobacillus sp.-derived lactic acid and hydrogen peroxide, with additional structural and immunological changes with the menstrual cycle, pregnancy and age (12, 53). The digestive system varies along its length, both in terms of physical factors and the unique composition of the microbiome: the oral mucosa is subject to occasional physical trauma from mastication, while the gut has the largest microbial biomass in the body in addition to a nutrient rich environment consisting of bile acids and diet-derived metabolites. Finally, the lungs are subject to fluctuating mechanical shear stress from ventilation and gravitational posture dependent changes, and also differ in structure and microbial sterility from higher to lower order bronchi and alveoli (54).
Most sites have underlying dense neural networks and varying concentrations of physiological parameters such as oxygen tension, lactate, glucose and amino acids depending on the specific location and inflammatory context; relative hypoxia is physiological in healthy skin hair follicles and the intestinal lumen, and induced in the face of increasing tissue demand during inflammation in all tissues. The inflammatory tissue microenvironment is further dysregulated by the catabolic processes necessary for generating an immune response, through excess nutrient consumption and generation of potentially toxic metabolic by-products. Moreover, these physical and biochemical changes interact with and shape the microbiome, therefore directly and indirectly interacting with resident immune cells, including MAIT cells.
Barrier MAIT Cells
Barrier tissue lymphocytes include innate, innate-like and adaptive immune cells (37). Activation is antigen-independent in natural killer (NK) and innate lymphoid cells (ILCs), and predominantly mediated through cytokines. Antigen-dependent mucosal protection is provided by CD4+ T helper 17 (TH17), regulatory T (Treg), and CD8+ tissue-resident memory (TRM) and Tc17 cells. MAIT cells and other unconventional T cells, such as invariant natural killer T (iNKT) and gamma-delta T (γδT) cells, share features with both innate and adaptive cells. They can be activated in a TCR-dependent or independent manner (20, 48, 49, 55), and are important for preserving tissue integrity and function in homeostasis.
Ontogeny and Tissue Residency
Human MAIT cells in blood have a tissue-homing effector-memory phenotype (3), but the ontogeny of cells in barrier surfaces is less clear. Murine tissue MAIT cells and iNKT are resident populations in homeostasis and following infection, expressing the transcription factor retinoic acid-related orphan receptor (ROR) γt (RORγt). After parabiosis, almost all thymic, and the majority of splenic, hepatic, lymph node and RORγt+ lung MAIT cells are host-derived, with some recirculation of RORγt− lung MAIT cells (47, 56). Following Staphylococcus epidermidis (S. epidermidis) challenge similar frequencies of murine skin MAIT, γδT, and iNKT cells are host-derived and thus tissue-resident (35).
In humans however, it is unclear to what extent tissue MAIT cells are permanently resident. Expression of αE-integrin (CD103) associated with CD8+ TRM cells is rare in blood MAIT cells (<3%) and common, but not universal, among MAIT cells within the oral and gastric mucosa (13, 15), skin (31, 57), and lungs (27–29) (Table 1). Thus tissues in health may represent a mixture of resident and recirculating MAIT cells, which could vary micro-anatomically: although most epidermal MAIT cells express CD103 and CLA (cutaneous lymphocyte antigen), only half of dermal MAIT cells express CD103 (31). Resident populations may behave differentially in disease – for example, among bronchoalveolar CD8+CD161++Vα7.2+ cells, which are mostly MAIT cells, only the CD103+ fraction is depleted in HIV infection (28).
Studies on the formation and longevity of tissue MAIT cells in humans are challenging. Remarkably, some MAIT cells do migrate into fetal small intestine, liver and lung as early as the 2nd trimester - before exposure to the conventional commensal flora (25). Fetal tissue MAIT cells, unlike circulating MAIT cells in adults, are cycling in the steady state with appreciable Ki67 expression (25). It is unclear if these early tissue resident MAIT cells could persist into adult life, similar to other fetal tissue-resident cells (58). One study using HLA allele mismatching to discriminate between donor- and recipient-derived T cells following small bowel transplantation showed that although the majority of tissue MAIT (CD161+Vα7.2+) cells are derived from the host long term, donor-derived cells can be found over a year after transplantation (59); this suggests that adult mucosal MAIT cells can persist for prolonged periods. The dynamics may of course vary in tissues with different cellular turnover, and analogous to the differential proliferative capacity and function of resident and monocyte-derived macrophages in tissues (60), the function of long-lived and newly formed MAIT cells may be distinct.
It is also unclear if MAIT cells can leave tissues. Thoracic duct lymph-derived and blood MAIT cells are CCR7− and present at comparable frequencies with overlapping TCR clonotypes (61), which suggests that MAIT cells in lymph directly derive from blood. This could imply either migration after transit through tissues, or direct CCR7-independent migration from blood through high endothelial venules. Further work is needed to understand the drivers for tissue migration, residency and persistence, including potential functional differences between long lived and nascent tissue MAIT cells.
Tissue Phenotype and Cytokine Production
MAIT cell expression of the NK-cell marker CD161 and the transcription factor RORγt (RORC) are features shared with other barrier tissue-resident cells, including TH17, peripherally derived Treg (pTreg), ILC3, iNKT and γδT cells (62–67). CD161+ T cells share a transcriptional program for innate-like cytokine-responsiveness in the absence of TCR triggering, through high expression of cytokine receptors (e.g., IL-12R, IL-18R) driven by the transcription factor promyelocytic leukemia zinc finger protein (PLZF) (45, 55). RORγt is crucial for barrier protective IL-17 production common to many mucosal lymphocytes (43, 68). Unusually, in homeostasis many human MAIT cells co-express the type-1 transcription factor T-bet (TBX21) and RORγt, whereas naïve SPF mice have two almost mutually exclusive populations (69, 70): nearly all murine tissue resident MAIT cells are RORγt+T-bet− IL-17A producing MAIT17, with less numerous T-bet+RORγt− IFN-γ producing MAIT1 found in the circulation (47). Following infection with intranasal Salmonella or Legionella, an increase in RORγt+T-bet+ lung MAIT cells is observed, suggesting that the diverse stimuli that human MAIT cells receive may explain part of the differences between species (71).
Functionally, human tissue MAIT cells are usually more activated than their circulating counterparts in homeostasis and capable of increased cytokine production (19, 23, 72) (Table 1). Additionally, barrier MAIT cells in the female genital tract and oral mucosa are predominantly CD8+ cells biased towards type-17 function (13, 32). Another study found that CD4−CD8− MAIT cells in healthy endometrium have a more mature phenotype with higher RORγt and lower T-bet expression (33). This skewed tissue biased phenotype is present from early development as fetal small intestine MAIT cells produce more cytokines (IFNγ, IL-22) than their circulating counterparts in response to Escherichia coli (E. coli) (25). It is unclear however if this phenotype varies between barrier tissues in adults. Barrier specific heterogeneity is observed in mice: murine skin MAIT cells display a transcriptional profile distinct from lung or liver (35), and both colonic and lung MAIT cells produce higher IL-17 than non-barrier tissues, such as the liver and spleen (73). Ultimately, as IL-17A and IL-22 promote barrier integrity, it will be important to understand the relative contributions of pre-programmed transcriptional and environmental cues to MAIT cell heterogeneity observed in human tissues.
Tissue Homeostasis and Tissue Repair
Given their anatomical location and similarity to other tissue-resident lymphocytes, it is perhaps unsurprising that MAIT cell effector function is important in tissue-homeostasis. This parallels tissue homeostatic roles for H2-M3 restricted CD8+ T cells (and Tregs) in mouse skin (74, 75), and γδT cell populations in the lung and gut (76–78). In NOD mice, Mr1-deficient animals have impaired intestinal barrier integrity, implicating MAIT cells in maintaining surface homeostasis (79). This concept was further supported in a model of colonic graft versus host disease (GvHD); Mr1-deficient animals had increased proinflammatory donor-derived CD4+ T cell expansion and reduced tight junction expression (73). GvHD in human bone marrow transplant recipients is also associated with lower MAIT cell frequency (80, 81), again potentially implicating a role in maintaining mucosal health.
Further recent data from both mouse and human studies has identified an important role for MAIT cells in tissue repair. Constantinides et al. found that murine skin resident MAIT cells are enriched for a distinct tissue repair transcriptional signature, similar to that observed in the previously described H2-M3 restricted CD8+ T cells responsive to S. epidermidis-derived N-formylated peptides (35). To assess MAIT cell specific tissue repair in vivo without confounding skin γδT and H2-M3 restricted CD8+ cells which can perform analogous functions, Tcrd−/− mice were infected with a strain of S. epidermidis that fails to induce CD8+ H2-M3-recognizing T cells. Tissue repair in response to S. epidermidis, measured by epidermal tongue length growth after skin punch biopsy, was higher in Tcrd−/− compared to Mr1−/−Tcrd−/− mice, implicating the additional MAIT cell deficiency.
How do these data relate to human MAIT cells? Recently, murine and human MAIT cells were also found to have a shared tissue repair transcriptional profile (as seen with the H2-M3 restricted CD8+ T cells) on resolution of infection from Legionella longbeachae and with re-infection, suggesting significant functional parallels (48). Two additional studies in human MAIT cells showed activation of such tissue repair gene expression patterns predominantly following TCR-mediated triggering (20, 49). In vitro assays of wound healing also revealed a functional repair role for MAIT cell derived soluble factors, which could be blocked using anti-MR1 antibodies (20). Taken together all these studies suggest that a local repair program analogous to other tissue resident cell types is active in MAIT cells and likely triggered in vitro through encounter with microbiota. This is supported by the remarkable observation in vivo that direct topical application of the MAIT cell TCR ligand 5-OP-RU alone prior to skin injury, in the absence of additional cytokines, is sufficient to selectively induce cutaneous MAIT cell expansion and expedite tissue repair (35). Much more work is needed to define the importance of this in human disease and also the exact mechanisms through which this large panel of soluble mediators exert their impact.
In addition to tissue-repair functions in response to commensals in the absence of inflammation, there is evidence that innate-like T cells can regulate barrier surface homeostasis by shaping the microbial landscape. CD1d and intestinal iNKT cells influence murine intestinal homeostasis and microbial colonization, with reduced Bacteriodales colonization in iNKT-deficient mice (82). Mr1-deficient animals, which lack MAIT cells, have reduced intestinal microbial diversity, similar to that found in IL-17A deficient animals (73). Conversely, in obese mice MAIT cells seem to promote microbial dysbiosis and ileal barrier dysfunction. Fecal transplantation from obese Mr1-deficient animals reduces barrier permeability in mice fed a high-fat diet, and the microbiome of obese Vα19+/− mice with a high MAIT cell frequency has lower Bifidobacteriaceae and Lactobacillaceae species (83). Given the importance of a diverse microbiome to human health, further work is needed on the interactions of MAIT cells and a healthy microbiome in maintaining tissue homeostasis.
These expanding tissue-specific functions raise the tantalizing possibility that similar to other innate-like lymphocytes, MAIT cell barrier functions may be more diverse than initially appreciated (84, 85). We know that γδT cells can remarkably promote stem-cell remodelling (86), adaptive thermoregulation in response to cold stress (87), and sympathetic nervous innervation (88). Furthermore, cytokine activated ILC3 can promote antigen specific CD4+ T cells responses directly in vitro through cell surface MHCII and co-stimulatory molecule expression (89). Indeed MAIT cells have the capacity to indirectly manipulate tissue adaptive responses, through dendritic cell maturation (90), and could potentially act as a sink for IL-7 similar to IL-7R+ ILC to limit homeostatic proliferation and preserve TCR diversity in neighboring tissue T cells (91).
In summary, MAIT cells in tissues are distinct from the population most frequently studied thus far in blood. The array of effector functions is expanding beyond the traditional cytotoxicity and cytokine production first described, and further understanding of the context in which these effector programs are engaged together with knowledge of how to modulate them are key to enable translation of MAIT cell biology to effective human therapeutics.
Barrier Adaptation
Metabolism
T cell development, differentiation, activation, function and survival are regulated by the cell intrinsic metabolism of glucose, amino acids and lipids (92–94). Nutrient availability differs between T cell compartments: circulating lymphocytes function in secondary lymphoid organs with high glucose and amino acid concentrations, whereas barrier tissues are more restrictive anatomically with variable nutrient composition. Permanent tissue-resident cells must therefore adapt to these niches for continued survival and proliferation which are also required for their tissue-specific effector functions.
Activated peripheral blood MAIT cells upregulate glucose uptake as glycolysis is required for their cytotoxicity and IFN-γ production (95, 96), and human circulating MAIT cells have reduced mitochondrial activity compared to CD161−CD8+ T cells (95). Amino acid metabolism is also crucial - peripheral MAIT cells express high levels of the L-type amino acid transporter, SLC7A5, and L-amino acid oxidase (96, 97). The metabolism of tissue MAIT cells has however not been explored.
Tissue MAIT cells may rely on OXPHOS, which correlates with TNF and IL-17 production in their circulating counterparts (96, 98). Given low tissue glucose concentrations, many resident immune cells are adapted to oxidative phosphorylation of fatty acids abundant in the skin and intestine (99). Tissue-resident but not circulating memory CD8+ T cells require these exogenous fatty acids for their survival, and upregulate transporters and fatty acid binding proteins (FABP) necessary for long term maintenance (100, 101). FABP isoform expression is tissue-specific, shared among resident TRM, IEL, ILC and γδT cells, with TRM able to modulate isotype specific expression on relocation to a new environment (102); Fabp4 and Fabp5 expression are enriched among skin resident cells, whereas Fabp1 is enriched among liver resident cells, including invariant NKT cells. Endogenous fatty acid metabolism is also important in IL-17 producing TH17 (103), with murine Tc17 cells and human IL-17 producing bronchoalveolar MAIT cells also enriched for genes in fatty acid and lipid metabolism (29, 104). It would therefore be logical to explore tissue MAIT cell mitochondrial and lipid metabolism in understanding their barrier specific effector functions.
Tissue Stress
Tissue stress includes homeostatic perturbations in metabolic or environmental factors. Examples include insufficient nutrients or accumulations of potentially toxic byproducts, including oxidative stress from excessive free radicals. Barrier tissues with an active immune response frequently have minor homeostatic perturbations tolerated by resident immune cells.
Autophagy is a metabolic stress response that recycles intracellular proteins and provides an additional nutrient source advantageous in tissues and activating environments (93, 105). MAIT cells in the liver have higher basal autophagy compared to their circulating counterparts, which may be required given the higher mitochondrial depolarization observed in stressed tissue-resident cells (106). In vitro, inhibition of autophagy reduces acquisition of a tissue-resident phenotype in circulating CD8+ T cells (106), thus enhanced autophagy may be a requirement for MAIT cell tissue survival.
Tissue oxidative stress can also be mitigated in barrier tissues by xenobiotic transporters, such as multidrug resistance protein 1 (MDR1). MDR1 (ABCB1) is an ATP-binding cassette B1 drug resistance transporter expressed on IL-17 producing CD4+ T cells in the ileum, which protects against bile acid induced oxidative stress to maintain intestinal homeostasis (107); a subset of patients with ileal Crohn’s have loss of function in MDR1, highlighting an important role in controlling tissue inflammation. MAIT cells and other CD161+ T cells also express high levels of MDR1 (3, 45, 108), and it would be interesting to evaluate in future studies whether this has similar implications for their survival and function in toxin rich niches.
Tissue oxidative stress also produces free radicals and hydrogen peroxide (H2O2). H2O2 can be transported by the plasma membrane water channel, aquaporin 3 (AQP3), which is part of a core transcriptional signature shared among type-17 secreting NKT17, ILC3, γδT, and TH17 cells in mice (109). Aqp3-deficient T cells have impaired chemokine mediated trafficking to the skin (110), and AQP3 expression is higher in human CD161+Vα7.2+ MAIT cells compared to circulating CD161− cells expressing the same TCRα (46). It is therefore possible that tissue stress regulates MAIT cell migration and survival in inflammatory tissues such as the skin.
Finally, given the often overlapping functions, some adaptations may serve to prune the tissue response to only the most appropriate cells. Murine TH17, iNKT and TRM are enriched for the purinergic receptor, P2RX7, which recognizes extracellular purines (ATP, NAD+) released after cell lysis and has cell type specific effects (111–113). Purines released from high microbial turnover can regulate barrier specific immune cell function, promoting murine TH17 differentiation (111) but inhibiting human ILC3 IL-22 production (114). P2RX7 expression on tissue iNKT and TRM however induces pyroptosis and limits immunopathology from bystander activation (113, 115). As purinergic receptor expression is downregulated on TCR engagement, release of purines from tissue damage preferentially depletes bystander activated TRM (115). Over time this could serve to shape the barrier immune response by conserving predominantly T cells specific to regularly encountered antigens. Given their presence in tissues and potential for bystander activation in response to cytokines, MAIT cell function may also be shaped by variable adaptations to tissue damage. Indeed, one could imagine that as a mammal ages, MAIT cell TCR-dependent responses could be superseded by antigen-specific TRM populations.
Barrier Sensing
Microbiome
Barrier surveillance of the commensal microbiome is essential for tissue immunity in homeostasis through shaping the composition and phenotype of innate and adaptive cells (116–118). Numerous mucosal cell types are perturbed in germ-free (GF) and antibiotic treated mice, including RORγt-expressing tissue Treg, TH17 and innate-like IL-17 producing γδT cells (119). Conversely cohousing laboratory mice with wild mice to induce a more diverse microbiome promotes a human adult-like immune composition, with increased tissue innate and differentiated memory CD8+ T cell populations (120).
We have known MAIT cells are also reliant on the microbiome since Treiner et al. discovered that they were absent in the lamina propria of GF mice (2). It was subsequently found that metabolites from riboflavin-synthesizing commensals, which engage the MAIT cell TCR, are necessary for most stages of MAIT cell intra-thymic development and subsequent peripheral expansion (56, 121). Accordingly, the dominant murine population of RORγt+ MAIT17 dependent on TCR-triggering for proliferation and function are depleted in the thymus and tissues of GF-mice, skewing the response to IFN-γ production (56). Recolonization of GF mice with microbes can rapidly restore this RORγt+ MAIT population. Remarkably, metabolites from skin riboflavin-synthesizing commensals, even in the absence of bacteria, can drive intra-thymic MAIT cell development remotely (56), in addition to sustaining the development and function of local skin-resident MAIT cells (35). There is however a narrow neonatal window until 3 weeks of age where recolonization of GF mice can restore MAIT cell development; recolonization of adult mice with bacteria after 7 weeks does not increase MAIT cell frequencies in the skin. Although they have complementary functions, this may be due to a finite niche for competing innate-like T cells shaped by the early microbiome; Tcrd-deficient animals have increased tissue-resident iNKT and MAIT cell populations (35), Cd1d-deficient animals have increased splenic and thymic MAIT cells (121), while GF mice have increased iNKT cells (122). A competing or compensatory interaction is also supported by the massive expansion of Vδ2+ T cells in a patient with a homozygous point mutation in MR1 and MAIT cell deficiency (123). Supporting complementary functions, human blood MAIT cell and iNKT cell frequencies positively correlate (124, 125). Additional work is needed to clarify the relationship between innate-like T cells, and whether this is influenced by age, disease and ligand abundance.
In humans, with a much lower frequency of tissue iNKT and γδT cells, it remains to be seen if this window period for reconstitution and niche exist in adulthood. BAL MAIT cells depleted in HIV are increased with ART; as ART partially restores a dysregulated lung microbiome (126), it is tempting to speculate this contributes to MAIT cell reconstitution. Peripheral MAIT cells however are not reconstituted by ART (21, 24). Long term reconstitution is also seen after allogeneic hematopoietic stem cell transplantation (81), dependent on the microbiome and potentially continued thymic output given the negative correlation with age.
Microbial Diversity and Pathogenicity
The mammalian microbiome is diverse and heterogeneous, varying between sites with different microenvironments. Skin hair follicles, sweat glands and sebum promote distinct commensals and immune responses compared to the intestine (52, 127, 128); diet, age and antimicrobials all shape the gut microbial landscape (129). Furthermore, dysbiosis can result from changes in the tissue microenvironment which drives the expansion of more suitably adapted commensals.
The appreciation of different microbial phyla to MAIT cell expansion and function is expanding. A screen of bacterial species in vitro found that the capacity to stimulate MAIT cells correlated with riboflavin secretion (130). Colonization of GF mice with Proteus mirabilis alone in the neonatal period is sufficient for MAIT cell expansion in the skin and lungs (35). In reality we have communities of microbes and there is evidence that increased microbial diversity is associated with improved MAIT cell reconstitution after allogeneic hematopoietic stem cell transplantation (81). This could partly be through a reduction in their activation induced cell death as in vitro microbial diversity has been shown to reduce MAIT cell activation (131). Testing common intestinal commensals in vitro has demonstrated that MAIT cell activation correlates with net riboflavin secretion, with higher diversity resulting in predominant riboflavin uptake and thus lower presentation to MAIT cells (131). This is supported by observations in apical periodontitis oral mucosa, where prominent riboflavin-expressing taxa correlate negatively with Vα7.2-Jα33 and IL17A transcripts (132). Furthermore, Il17a-deficient mice, which have microbial dysbiosis and reduced barrier protection, actually have increased MAIT cell frequencies in the lung and colon. As IL17a-deficient mice have increased Candidatus Homeothermaceae and Bacteriodaceae, it is tempting to speculate that the composition of the microbiome is crucial for MAIT cell expansion (73). As microbial diversity varies between tissues in health and disease, normally high in healthy colon and reduced in dysbiosis and metabolic diseases, this could be a mechanism to manipulate MAIT cell function and through which they may contribute to pathology.
In addition to diversity, healthy tissues are characterized by an intact barrier, disruption of which induces inflammation and could impact MAIT cell function. Most microbes are commensals living in symbiosis with the host; pathogens induce barrier disruption, inflammation and cytokine production. For example, colonization with commensal S. epidermidis does not induce inflammation and is important for tissue homeostasis and a MAIT cell tissue repair signature (35). However, stimulation of human MAIT cells in vitro with cytokines in addition to TCR engagement promotes an antimicrobial program, including the cytokine IL-26, capable of directly killing extracellular bacteria (133), and effector recruiting chemokines CXCL9 and CXCL10 (20, 49). Thus, the pathogenicity of the human microbiome could tune the MAIT cell response, and further studies should assess the various pathogen-specific factors which induce antimicrobial rather than more tolerant repair effector programs.
Microbial Metabolism and Environment
Microbial metabolism is also shaped by the tissue microenvironment and could tune MAIT cell activation. One mechanism is through availability of TCR ligands derived from riboflavin synthesis: heat stress in Streptococcus pneumoniae induces expression of the riboflavin operon (134); and acid stress increases purine and folate metabolism, as well riboflavin uptake (19, 131). Bacterial co-culture conditions influence their capacity to activate human MAIT cells in vitro (19); hypoxia, simulating the low oxygen tension in colonic crypts, stationary growth phase and hypercarbia increase MAIT cell activation, whereas hypoglycemia, acetate and pyruvate inhibit bacterial control (19). Even chemicals and pesticides, which cause gut dysbiosis on ingestion of food, have been found to increase E. coli-induced MAIT activation (135). MAIT cells could therefore survey the nature and state of the microbiome as a proxy measure of tissue health.
Non-riboflavin microbial metabolites could also modulate tissue MAIT cells, contributing to tissue homeostasis or pathogenic inflammation. Lactobacilli, enriched in the female genital tract, produce high levels of L(+)-lactate; and both lactate and Lactobacilli-derived factors dampen Staphylococcus aureus (S. aureus)-induced MAIT cell activation in whole PBMC (136). Given that the antibacterial response of MAIT cells resident in the female genital tract is biased towards IL-17 and IL-22 production compared to their circulating counterparts (32), it is tempting to speculate that microbial derived factors at barrier surfaces might directly skew MAIT cell responses.
There is evidence that other products of bacterial metabolism, short chain fatty acids (SCFA), can directly modulate barrier RORγt+ immune cell responses (137). Acetate, propionate and butyrate are products of dietary fiber fermentation which signal via G-protein coupled receptors (GPCR) to inhibit histone deacetylases (HDACs) (137). These SCFA directly promote barrier preservation and can reverse some of the immune dysregulation in GF-mice; SCFA rescue the colonic Treg depletion seen in GF mice (138) and are capable of augmenting RORγt+Treg expansion (139–141) and ILC3 IL-22 production (142). Barrier cells such as ILC3 can directly sense acetate through Ffar2 (free fatty acid receptor 2) (142), which interestingly is also enriched transcriptionally in murine skin MAIT cells compared to CD4+ T cells (35). As SCFA reduce MAIT cell antimicrobial function in vitro (19), it would be of interest to determine if microbial metabolites could be sensed and tune MAIT cell responses in a TCR-independent manner.
Dietary Factors
In addition to the microbiome, mucosal immune cells are capable of directly sensing chemicals, including nutrients in the mammalian diet (39). Dietary-derived metabolites, particularly lipophilic compounds, rapidly diffuse and bind to intracellular ligand-dependent transcription factors and can regulate tissue resident cells (143); these include receptors for vitamin A (retinoic acid receptor, RAR), vitamin D (vitamin D receptor, VDR), and tryptophan metabolites (aryl-hydrocarbon receptor, AhR). Given their often-shared function and location, MAIT cells may also be regulated by these dietary factors.
Vitamin A
The fat soluble Vitamin A is enriched in human intestine and is important for mucosal health (39). Dietary vitamin A, as all-trans-retinol, retinyl esters, or β-carotene, is metabolized to bioactive all-trans-retinoic acid (ATRA) and 9-cis-retinoic acid (144), which bind to nuclear retinoic acid receptors RARα (RARA), RARβ (RARB), and RARγ (RARG) (39). In mice RA maintains barrier homeostasis by directly modulating Treg, TH17 and ILC3 function. RORγt+pTreg development, homing and differentiation are RA dependent (145–148), with dietary deficiency promoting TH17 mediated tissue pathology (141). Similarly ILC3 gut homing (149), plasticity (150), and IL-22 mediated protection in DSS colitis are RA dependent (151). In humans, ATRA directly increases CD161 and gut homing CCR9 expression in a population of RORC+CD161+ colonic Treg associated with tissue repair (64). Vitamin A deficiency is also strongly associated epidemiologically with severe mucosal infections, which may partly be through direct effects on barrier protective immune cells.
The role of RA in innate-like T cells is less clear. RA reduces invariant NKT induced-sterile tissue damage by inducing P2RX7 expression, rendering bystander but not TCR-activated cells more susceptible to extracellular ATP-induced pyroptosis (152). γδT cell function is also reduced: CD27−γδT cell IL-17 production is inhibited by RA through reduction in IL-1R, IL-23R, and pSTAT3 expression (153). In IBD tissue however, RA levels correlate with increased γδT and MAIT cell function (IL-17, IFN-γ) (154). As RARG is upregulated in blood MAIT cells relative to conventional T cells (46), vitamin A could conceivably modulate intestinal MAIT cell migration and function to ultimately maintain mucosal integrity in an analogous manner to neighboring CD161+ T cells.
Vitamin D and Cholesterol Metabolites
The lipophilic oxysterol derivate Vitamin D can be derived from the diet or photochemically synthesized in the skin (39), and binds to its heterodimeric receptor, composed of VDR and the retinoid X receptor (RXR). Immune cells, particularly those in the intestine and skin, are enriched for expression of the nuclear VDR which is reduced in inflammatory bowel disease and implicated in the moderation of mucosal inflammation (39, 155). VDR expression is upregulated on TCR signaling and decreases type 17 associated immunopathology in humans and mice, increasing the ratio of Treg: TH17 (156, 157), inhibiting ILC3 IL-23R expression (158), and directly competing with NFATc1 for binding to the IL17A promoter (159). Vdr−/− mice also have impaired iNKT and CD8αα+ IEL development, suggesting a broad role in tissue immunity (159–161).
MAIT cell frequency and function may also be subject to regulation by vitamin D. MAIT cells triggered through their TCR upregulate VDR, either in vitro in humans or during acute Legionella longbeachae infection in mice (48). In asthmatic patients, seasonal fluctuations in peripheral MAIT cell frequency correlate with serum phytochemically derived vitamin D3 levels and peak in August (162). In cystic fibrosis patients however, although baseline serum vitamin D3 correlates with peripheral MAIT cell CD38 expression, there was a trend for reduced MAIT cell frequency in those receiving oral vitamin D supplementation (163). Dietary and photochemically-derived vitamin D may differentially regulate MAIT cells in different compartments and activation states – whether responding to commensals in homeostasis or during active inflammation.
Other cholesterol derivatives of host or microbial metabolism, including oxysterols, are abundant in the intestine and can act as RORγt ligands to promote development and function of RORγt+ intestinal cells (164). Stromal cells produce 7-α,25-hydrocycholesterol, which binds to GPR183 expressing ILC3 to promote their migration in homeostasis (165). As tissue IFN-γ producing MAIT cells transcriptionally express the oxysterol receptor GPR183 (29), oxysterol sensing may also functionally regulate MAIT cells.
Bile acids are cholesterol-derived surfactants crucial for fat digestion that bathe the ileum as part of the enterohepatic circulation and regulate both the microbiota and mucosal immunity (166). Secondary bile acids derived from microbial metabolism, including deoxycholic acid (DCA) and lithocholic acid (LCA), can directly promote mucosal homeostasis by increasing colonic FOXP3+ RORγt+ Treg (167). A screen of secondary bile acids also found that LCA derivatives can reduce the TH17:Treg balance in the intestinal lamina propria, by directly blocking RORγt-induced TH17 differentiation and promoting Treg Foxp3 expression and differentiation in a mitochondrial ROS-dependent manner (168). MAIT cell activation and PLZF expression negatively correlate with serum concentrations of conjugated bile acids in teenage children, and in vitro bile acids inhibit MAIT cell activation in response to E. coli (169), so it would be important to explore whether intestinal bile acids promote homeostatic MAIT cell responses against commensals within a healthy functioning symbiotic intestinal environment.
Aryl Hydrocarbon Receptor
Aryl hydrocarbon receptor (AhR) is a conserved ligand activated transcription factor highly expressed by cell types at barrier surfaces, in keeping with its role as an environmental sensor promoting mucosal integrity. Physiological AhR ligands include: indole-derived ligands from dietary cruciferous vegetables; host and microbe-derived tryptophan-metabolites (e.g., kynurenine); and exogenous chemicals (40). Initially discovered and enriched in intestinal TH17 and Treg (41, 170), AhR signaling is also important for the function of mucosal IL-17 producing γδT, iNKT and ILC3 (66, 171, 172). Sensing of diverse environmental signals promotes Treg differentiation, IL-22 production, ILC3 survival and IEL homeostasis, thus promoting barrier integrity (173). Ahr-deficient mice have dysfunctional skin and intestinal γδT cells and absent IEL (172, 174), with reduced capacity for TH17 differentiation and IL-22 secretion. AhR expression in CD8+ T cells is crucial for TRM and IEL persistence in tissues (175, 176), while cytokines upregulate AhR expression in NK cells and iNKT to promote cytotoxicity and IL-22 production respectively (66, 177).
The role of AhR in MAIT cells has only been tentatively explored. Ahr is dispensable for MAIT cell thymic development in mice (56). In HIV patients on ART, increased tryptophan catabolism and generation of the AhR ligand, kynurenine correlates with lower peripheral blood MAIT cell frequency and higher frequency of Treg (178). As AHR expression is higher in bronchoalveolar MAIT cells compared to matched circulating cells in children with pneumonia (29), further work should explore specifically whether tissue MAIT cells are selectively regulated by AhR in a similar manner to other IL-22 producing cells in particular.
Lipids
The predominant calorie source of diet can influence barrier immunity in mice. A high glucose diet exacerbates colitis by increasing mitochondrial metabolism to drive TH17 differentiation (179). Mice fed a high fat diet also have increased TH17 differentiation through induction of the lipid sensitive kinase, acetyl co-A carboxylase 1, crucial for de novo FA synthesis and oxidative phosphorylation (103, 180). A ketogenic high fat diet however protects against influenza challenge and promotes improved lung barrier integrity associated with early lung γδT cell recruitment, expansion and barrier type-17 function (181). In addition to diet, tissue free fatty acids have also been shown to induce a regulatory phenotype in iNKT (182). Lung type-17 MAIT cells in the context of pneumonia are enriched in genes for OXPHOS, glycolysis, lipid efflux and translocation, while other MAIT cells are enriched in genes for steroid metabolism, fatty acid synthesis and lipid uptake (29). Further studies should investigate the regulation of MAIT cell function by lipids and metabolism.
Tissue Environment
Tissue immune cellular and soluble mediators, particularly cytokines, manipulate the function of MAIT cells and other resident populations (4). This is further nuanced by the confined shared niche occupied by resident cells which compete for space and local survival signals (175). Tissue inflammation and infiltration of metabolically active, cytotoxic cells into this niche can disrupt homeostatic regulation by depleting nutrients (glucose, amino acids) and oxygen, producing waste products such as reaction oxygen species and lactate which contributes to tissue acidosis (183, 184). Resident immune cells are themselves in turn tuned by these non-immune tissue parameters.
Oxygen Sensing
Oxygen tension and regulation varies in vivo: blood and primary lymphoid organs have tightly regulated levels, whereas physiological hypoxia is observed in tissues such as the skin and intestine (38, 52, 185). Microbes can also indirectly induce colonic oxygen consumption through SCFA (186). Hypoxia regulates immune cells directly by preventing cytosolic degradation of the oxygen sensing transcription factor, hypoxia-inducible factor (HIF1A). T cell upregulation of HIF1A expression is STAT3-dependent and promotes CD8+ T cell effector functions (187), Treg plasticity (188, 189), and TH17 differentiation through induction of glycolysis, Rorγt expression and Foxp3 proteasomal degradation (190, 191).
In vitro sorted human MAIT cells co-cultured with proximal tubular epithelial cells are more activated in hypoxic conditions, with increased cytotoxicity albeit no difference in cytokine production (192). In children with pneumonia, bronchoalveolar MAIT cells have higher HIF1A expression compared to their blood counterparts which correlates with their capacity for increased IL-17 production (29). Furthermore, the tissue repair signature enriched in MAIT cells engaged through their TCR includes upregulation of HIF1A in addition to factors associated with angiogenesis (VEGFA, PDGF2, CSF2) (48). It would seem plausible that tissue MAIT cells, likely to experience local hypoxia during the course of an immune response, could tune their effector functions accordingly to ultimately induce tissue repair and improve oxygenation.
pH
Although circulating pH is homeostatically maintained around pH 7.4, deviations are seen in tissues: healthy skin is acidic due to a high free-fatty acid content; and inflammation drives tissue acidosis through glycolytic products (52). Many immune cells possess mechanisms for proton sensing, including acid-sensing ion channels (ASIC), transient receptor potential (TRP) channels, and GPCRs (193). Among T cells, human MAIT cells and other CD161+ T cells share functionality and a conserved transcriptional signature by bulk microarray, which includes enrichment for two candidate GPCR proton sensors, GPR65 and GPR68 (45, 194, 195). GPR65 may play an important role in RORγt+ T cells; the Gpr65 promoter has a RORγt binding site (196), and Gpr65 expressing T cells regulate the development of EAE in mice which is driven by type 17 inflammation (197). Naïve Gpr65−/− CD4+ T cells differentiated under TH17 conditions, or memory Gpr65−/− CD4+ T cells reactivated with IL-23 produce less IL-17A in vitro, and the adoptive transfer of Gpr65−/−CD4+ T cells into Rag1−/− recipients prior to EAE induction markedly delays and reduces disease (197). Another study however found that Gpr65-deficient mice develop exacerbated EAE, which was lost in the absence of iNKT (198); functionally deficient Gpr65gfp/gfp but not Cd1d–/– Gpr65gfp/gfp mice develop more severe disease compared to wild-type. It is particularly interesting to note that murine Gpr65 expression is important for CD4+ T survival in culture and highest in iNKT, followed by γδT and NK cells, suggesting a homeostatic role for acid sensing in innate-lymphoid cells. MAIT cells were not assessed in this study, but in humans share similarities with and are a hundred times more common than iNKT (67), thus may represent the most prominent GPR65 expressing cell. Indeed type 17 bronchoalveolar MAIT cells in children with pneumonia are enriched for GPR65 expression so future studies should explore whether acid-sensing can promote MAIT cell mucosal function (29).
Lactate
Increased lactate is concomitantly seen with acidosis in inflammation, and can be directly sensed by CD4+ T and CD8+ T cells through SLC5A12 and SLC16A1 transporters respectively; these function to inhibit T cell migration and potentially promote tissue retention (199–201). However in mice, cells with low glycolytic capability, including murine iNKT, show reduced survival under high lactate conditions in vitro (202). MAIT cells and other IL-23R+ lymphocytes could also be indirectly regulated by lactic acid augmentation of TLR-induced IL-23p19 production (203), which would skew towards type 17 responses in tissues (32, 204).
Temperature
Fever is a conserved response to infection and autoinflammatory disease across species. Although core temperature is rigorously regulated, peripheral tissues such as the skin where MAIT cells reside, are prone to deviations (205). High temperatures have long been known to enhance human lymphocyte proliferation and cytotoxicity in vitro (206), as well as CD8+ T cell differentiation and CD4+ T cell activation through increased membrane fluidity and reduced co-stimulation thresholds (207, 208). In mice, antipyretics (aspirin, ibuprofen) inhibit TH17 differentiation, with high temperatures selectively promoting inflammatory TH17 differentiation and increased lung neutrophil recruitment (209). Pulmonary MAIT cells and IL-17A producing innate-like T cells clearly protect against bacterial and viral infections in mice, which become pyrexial during the course of a normal immune response (210–212). It is unclear if pharmacological or pathological alteration of this normal febrile response, or significant exposure to cold environments, could modulate the response of tissue MAIT cells.
Electrolytes and Osmotic Stress
Similar to pH, electrolytes such as sodium, potassium, and chloride are normally tightly regulated in blood. Elevated extracellular potassium is, however, found in necrotic tissues and tumors, which paralyses human cytotoxic T cell responses (213). It is also appreciated that salt (NaCl) concentration can be enriched in barrier tissues such as the skin, particularly during inflammation (44, 52, 214). High salt diet increases EAE severity in mice due to increased TH17 differentiation from naïve precursors; direct salt-sensing ultimately induces TH17 IL-23R expression (215, 216) and inhibits Treg differentiation (217, 218).
In humans, high salt in vitro augments both naïve CD4+ TH17 polarization and memory CD8+ T cell IL-17A production in response to TCR-activation in the absence of polarizing cytokines (219). Interestingly, the skin of patients with atopic dermatitis has higher salt concentrations, and salt promotes both TH2 and TH17 cytokine production and skin-homing CCR8 expression by TCR-activated memory CD4+ T cells, thus potentially linking the environment with pathogenic mucosal T cell responses (219). Salt also indirectly regulates mucosal T cell function through differential production of polarizing cytokines: osmotic stress increases macrophage IL-1β production and Th17 generation in mice (220); and humans with a fixed high salt diet have increased plasma IL-23 (221). As MAIT cells are IL-23+ T cells in the skin, it would be interesting to determine whether their responses are also skewed in a similar way by the tissue electrolyte composition and if this contributes to disease.
Neuroendocrine System
The dense peripheral neuronal network underlying barrier surfaces co-ordinate rapid often reflex responses to external insults, such as itch, pain or cough reflex. Remarkably peripheral nerves directly regulate tissue immunity through soluble factors, including neuropeptides: neuromedin U (NMU) modulates ILC2-mediated tissue protection (222, 223); vasoactive intestinal peptide (VIP) increases ILC3-mediated epithelial barrier protection (224, 225); and catecholamines have inhibitory and stimulatory effects on ILC2 and NK cells respectively via adrenoceptor beta-2 (ADRB2) (226). The enteric nervous system can also indirectly control resident lymphocytes, through nociceptor induced IL-18 and IL-23 expression (227, 228). Given their location within this neuroimmune network, and expression of relevant receptors for cytokines and neuropeptides transcriptionally, MAIT cells could be subject to rapid manipulation by the nervous system.
Growth factors also regulate tissues and could influence MAIT cells. One example is insulin-like growth factor 1 (IGF-1) signaling, which promotes STAT3 signaling and aerobic glycolysis to increase type-17 effector functionality of TH17 and ILC3 (229). IGFbp4, an important modulator of IGF1 signaling, is enriched in murine RORγt+ TH17, Treg, and ILC3. In humans, IGFBP4 is enriched in CD161+ T cells, so may be an important regulator of MAIT cell type-17 functionality (45). Indeed insulin resistance and fasting insulin levels in obese children correlate with circulating IL-17A producing MAIT cells (230), which may imply that feedback circuits regulating tissue glucose metabolism play an important role in skewing MAIT cell function.
External Environment
UV-light can regulate the immune system (231), partly through photochemical synthesis of vitamin D. Additionally, UV light dampens inflammatory pathology in psoriasis and has been shown to degrade numerous photosensitive MAIT cell ligands, including folic-acid derived 6-FP (6-formyl-pterin) (232). Given the unstable nature of MAIT cell ligands, the impact of light on skin MAIT cell responses to commensals in particular deserves further attention.
Finally, the circadian rhythm has a role in entraining barrier RORγt+ cells (233). Clock genes regulate the RORC promoter to dictate TH17 differentiation (234) and ILC3 function (235–237); and disruption of the light-dark cycle in mice exacerbates TH17 IL-17A-dependent DSS colitis. Pathway enrichment for innate-like T cells suggests that circadian clock regulation is a shared feature among human innate-like T cells, with transcription factors ARNTL (encoding BMAL), RORA, PER1, and CRY1 enriched among MAIT, iNKT, and Vδ2+ γδT cells (238). As circadian biology regulates mammalian behavior and exposure to environmental factors, including food, this could be particularly relevant to mucosal MAIT cell function.
Conclusion
MAIT cells serve an increasingly appreciated role in barrier tissues, yet the full range of effector functions remain to be determined. Similar to other mucosal lymphocytes, they are engaged in cross-talk with the tissues and microbiome via their TCR and through cytokine receptors (Figure 2). The context of this cross-talk in tissues, in addition to the array of increasingly recognized signals sensed by resident lymphocytes, suggest that other factors may influence MAIT cell activation, function, and plasticity. Indeed, these environmental factors were identified with mouse models that may have missed the impact on MAIT cell biology as these cells are infrequent in murine mucosal tissues. Humans, however, have an abundance of MAIT cells and in contrast to laboratory mice, are exposed to phenomenally diverse environmental factors unique to each individual. Exploring local environmental factors in addition to fixed pre-programmed factors in the investigation of MAIT cell tissue biology will be crucial to understanding the variability in humans and could pave the way for personalized therapies in the context of disease.
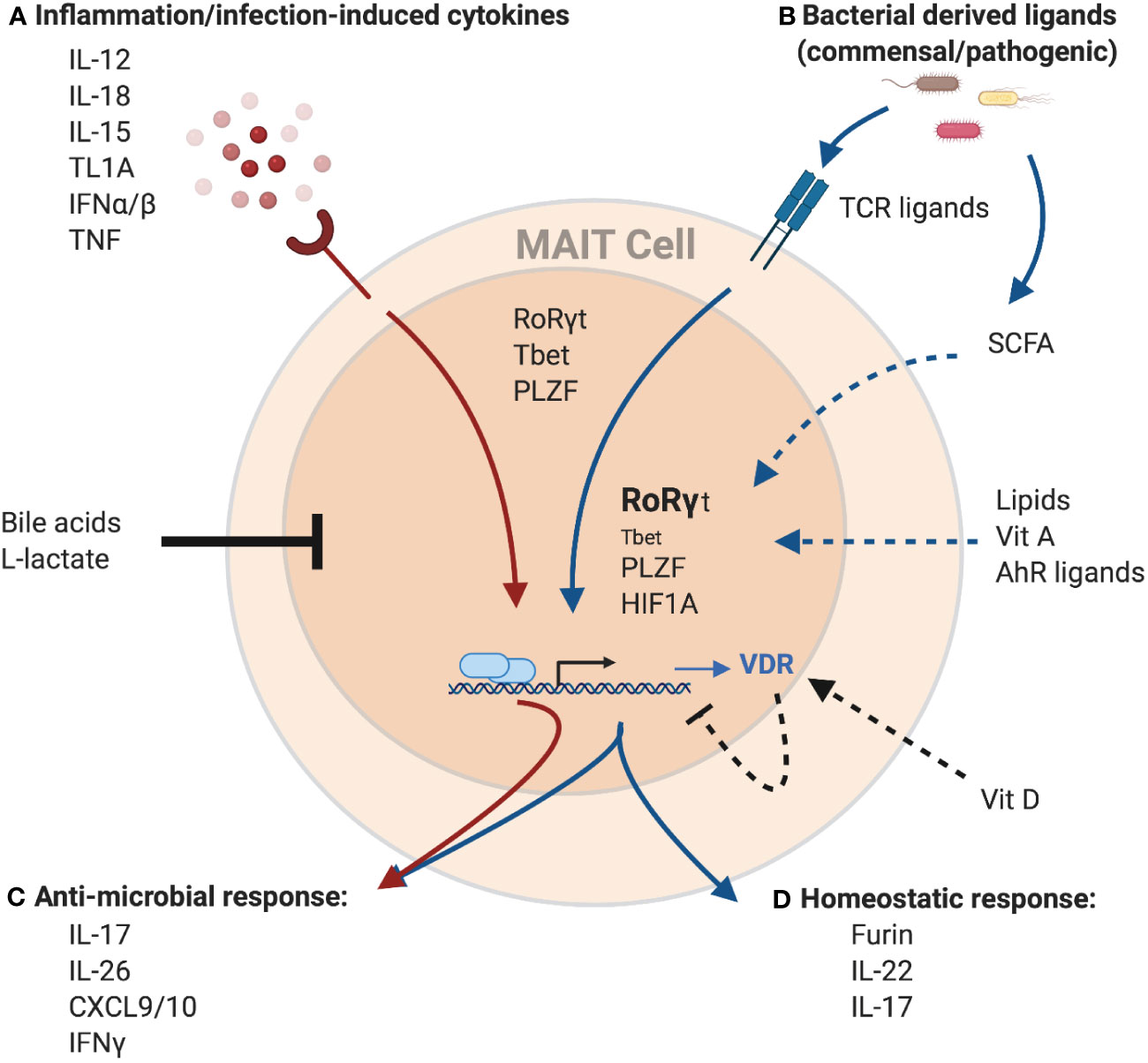
Figure 2 Potential regulation of the MAIT cell transcriptome and effector function by environmental cues. MAIT cells can be activated (A) independent of TCR-ligands by cytokines, or (B) through TCR-mediated recognition of microbial-derived riboflavin derivates presented by MR1. These signals can work both independently and synergistically to induce a spectrum of different effector programs. Cytokine-mediated MAIT activation results in the induction of a strong anti-microbial program (C), including the production of cytokines like IFNγ, IL-26 and members of the IL-17 family as well as pro-inflammatory chemokines like CXCL9 and CXCL10. These antimicrobial functions are further amplified with concurrent TCR signaling. TCR signals result in the induction of a homeostatic response (D), including cytokines associated with barrier maintenance (IL-22, IL-17), and proteins associated with tissue repair, such as the endoprotease furin. MAIT cells effector functions are controlled by the transcription factors PLZF, RoRγt, and Tbet. Importantly, while PLZF expression within MAITs is stable, expression of the homeostatic effector program is associated with increased expression of RoRγt and decreased expression of Tbet. Finally, TCR-mediated MAIT cell activation also leads to expression of HIF1A, another transcription factor associated with tissue repair. In addition to TCR-ligands and cytokines, several other factors have the potential to modulate MAIT cell activation. Bile acids and L-lactate were shown to generally reduce MAIT cell responses, while binding of Vitamin D to its receptor (VDR), the expression of which is upregulated in MAIT cells in response to TCR-signaling, has the potential to specifically inhibit the homeostatic response. In contrast, recognition of several other metabolites including AhR ligands, Vitamin A and lipids was associated with the expression of homeostatic effector molecules in other T cell populations and hence, could positively influence the expression of these molecules in MAIT cells as well. Similarly, short-chain fatty acids (SCFA), a product of bacterial metabolism, were shown to stimulate production of IL-22 and expansion of RORγt-expression lymphocytes in other immune cells, while reducing the antimicrobial function of MAIT cells, which could overall present a mechanism to preserve tissue homeostasis. Created with Biorender.com.
Author Contributions
AA conceived the review, conducted the literature review, and wrote the bulk of the manuscript. DP contributed to writing of the manuscript. C-PH edited and revised the manuscript, and created the figures. PK contributed to the planning, editing, and scope of the review. All authors contributed to the article and approved the submitted version.
Funding
AA is supported by a Wellcome Trust Clinical Research Training Fellowship [216417/Z/19/Z]. C-PH is supported by the Wellcome Trust [WT109965MA, awarded to PK] and the Deutsche Forschungsgemeinschaft (project number 403193363). PK is funded by the Wellcome Trust [WT109965MA]; the Medical Research Council (STOP-HCV); a National Institute of Health Research Senior Fellowship, the National Institute of Health Research Oxford Biomedical Research Centre, and the National Institutes of Health (U19 I082360).
Conflict of Interest
The authors declare that the research was conducted in the absence of any commercial or financial relationships that could be construed as a potential conflict of interest.
References
1. Porcelli S, Yockey CE, Brenner MB, Balk SP. Analysis of T cell antigen receptor (TCR) expression by human peripheral blood CD4-8- alpha/beta T cells demonstrates preferential use of several V beta genes and an invariant TCR alpha chain. J Exp Med (1993) 178(1):1–16. doi: 10.1084/jem.178.1.1
2. Treiner E, Duban L, Bahram S, Radosavljevic M, Wanner V, Tilloy F, et al. Selection of evolutionarily conserved mucosal-associated invariant T cells by MR1. Nature (2003) 422(6928):164–9. doi: 10.1038/nature01433
3. Dusseaux M, Martin E, Serriari N, Peguillet I, Premel V, Louis D, et al. Human MAIT cells are xenobiotic-resistant, tissue-targeted, CD161hi IL-17-secreting T cells. Blood (2011) 117(4):1250–9. doi: 10.1182/blood-2010-08-303339
4. Provine NM, Klenerman P. MAIT Cells in Health and Disease. Annu Rev Immunol (2020) 38(1):203–28. doi: 10.1146/annurev-immunol-080719-015428
5. Tilloy F, Treiner E, Park SH, Garcia C, Lemonnier F, la Salle de H, et al. An invariant T cell receptor alpha chain defines a novel TAP-independent major histocompatibility complex class Ib-restricted alpha/beta T cell subpopulation in mammals. J Exp Med (1999) 189(12):1907–21. doi: 10.1084/jem.189.12.1907
6. Reantragoon R, Corbett AJ, Sakala IG, Gherardin NA, Furness JB, Chen Z, et al. Antigen-loaded MR1 tetramers define T cell receptor heterogeneity in mucosal-associated invariant T cells. J Exp Med (2013) 210(11):2305–20. doi: 10.1084/jem.20130958
7. Le Bourhis L, Martin E, Peguillet I, Guihot A, Froux N, Core M, et al. Antimicrobial activity of mucosal-associated invariant T cells. Nat Immunol (2010) 11(8):701–8. doi: 10.1038/ni.1890
8. Gold MC, Cerri S, Smyk-Pearson S, Cansler ME, Vogt TM, Delepine J, et al. Human mucosal associated invariant T cells detect bacterially infected cells. PloS Biol (2010) 8(6):e1000407. doi: 10.1371/journal.pbio.1000407
9. Kjer-Nielsen L, Patel O, Corbett AJ, Le Nours J, Meehan B, Liu L, et al. MR1 presents microbial vitamin B metabolites to MAIT cells. Nature Nat Publishing Group (2012) 491(7426):717–23. doi: 10.1038/nature11605
10. Godfrey DI, Koay HF, McCluskey J, Gherardin NA. The biology and functional importance of MAIT cells. Nat Immunol (2019) 20(9):1110–28. doi: 10.1038/s41590-019-0444-8
11. Boudinot P, Mondot S, Jouneau L, Teyton L, Lefranc M-P, Lantz O. Restricting nonclassical MHC genes coevolve with TRAV genes used by innate-like T cells in mammals. Proc Natl Acad Sci USA (2016) 113(21):E2983–92. doi: 10.1073/pnas.1600674113
12. Belkaid Y, Naik S. Compartmentalized and systemic control of tissue immunity by commensals. Nat Immunol (2013) 14(7):646–53. doi: 10.1038/ni.2604
13. Sobkowiak MJ, Davanian H, Heymann R, Gibbs A, Emgård J, Dias J, et al. Tissue-resident MAIT cell populations in human oral mucosa exhibit an activated profile and produce IL-17. Eur J Immunol (2018) 49(1):133–43. doi: 10.1002/eji.201847759
14. Berkson JD, Slichter CK, DeBerg HA, Delaney MA, Woodward Davis AS, Maurice NJ, et al. Inflammatory Cytokines Induce Sustained CTLA-4 Cell Surface Expression on Human MAIT Cells. Immunohorizons ImmunoHorizons (2020) 4(1):14–22. doi: 10.4049/immunohorizons.1900061
15. Booth JS, Salerno-Goncalves R, Blanchard TG, Patil SA, Kader HA, Safta AM, et al. Mucosal-Associated Invariant T Cells in the Human Gastric Mucosa and Blood: Role in Helicobacter pylori Infection. Front Immunol (2015) 6(8–9):466. doi: 10.3389/fimmu.2015.00466
16. D’Souza C, Pediongco T, Wang H, Scheerlinck J-PY, Kostenko L, Esterbauer R, et al. Mucosal-Associated Invariant T Cells Augment Immunopathology and Gastritis in Chronic Helicobacter pylori Infection. J Immunol (2018) 200(5):1901–16. doi: 10.4049/jimmunol.1701512
17. Provine NM, Binder B, FitzPatrick MEB, Schuch A, Garner LC, Williamson KD, et al. Unique and Common Features of Innate-Like Human Vδ2+ γδT Cells and Mucosal-Associated Invariant T Cells. Front Immunol (2018) 9:756. doi: 10.3389/fimmu.2018.00756
18. Dunne MR, Elliott L, Hussey S, Mahmud N, Kelly J, Doherty DG, et al. Persistent changes in circulating and intestinal γδ T cell subsets, invariant natural killer T cells and mucosal-associated invariant T cells in children and adults with coeliac disease. PloS One (2013) 8(10):e76008. doi: 10.1371/journal.pone.0076008
19. Schmaler M, Colone A, Spagnuolo J, Zimmermann M, Lepore M, Kalinichenko A, et al. Modulation of bacterial metabolism by the microenvironment controls MAIT cell stimulation. Mucosal Immunol (2018) 11(4):1060–70. doi: 10.1038/s41385-018-0020-9
20. Leng T, Akther HD, Hackstein CP, Powell K, King T, Friedrich M, et al. TCR and Inflammatory Signals Tune Human MAIT Cells to Exert Specific Tissue Repair and Effector Functions. CellReports (2019) 28(12):3077–3091.e5. doi: 10.1016/j.celrep.2019.08.050
21. Cosgrove C, Ussher JE, Rauch A, Gärtner K, Kurioka A, Hühn MH, et al. Early and nonreversible decrease of CD161++ /MAIT cells in HIV infection. Blood (2013) 121(6):951–61. doi: 10.1182/blood-2012-06-436436
22. Haga K, Chiba A, Shibuya T, Osada T, Ishikawa D, Kodani T, et al. MAIT cells are activated and accumulated in the inflamed mucosa of ulcerative colitis. J Gastroenterol Hepatol (2016) 31(5):965–72. doi: 10.1111/jgh.13242
23. Slichter CK, McDavid A, Miller HW, Finak G, Seymour BJ, McNevin JP, et al. Distinct activation thresholds of human conventional and innate-like memory T cells. In: JCI Insight, vol. 1. American Society for Clinical Investigation. JCI Insight (2016) 1(8):e86292. doi: 10.1172/jci.insight.86292
24. Leeansyah E, Ganesh A, Quigley MF, Sönnerborg A, Andersson J, Hunt PW, et al. Activation, exhaustion, and persistent decline of the antimicrobial MR1-restricted MAIT-cell population in chronic HIV-1 infection. Blood (2013) 121(7):1124–35. doi: 10.1182/blood-2012-07-445429
25. Leeansyah E, Loh L, Nixon DF, Sandberg JK. Acquisition of innate-like microbial reactivity in mucosal tissues during human fetal MAIT-cell development. Nat Commun (2014) 5:3143. doi: 10.1038/ncomms4143
26. Hinks TSC, Wallington JC, Williams AP, Djukanović R, Staples KJ, Wilkinson TMA. Steroid-induced Deficiency of Mucosal-associated Invariant T Cells in the Chronic Obstructive Pulmonary Disease Lung. Implications for Nontypeable Haemophilus influenzae Infection. Am J Respir Crit Care Med (2016) 194(10):1208–18. doi: 10.1164/rccm.201601-0002OC
27. Wong EB, Gold MC, Meermeier EW, Xulu BZ, Khuzwayo S, Sullivan ZA, et al. TRAV1-2+ CD8+ T-cells including oligoconal expansions of MAIT cells are enriched in the airways in human tuberculosis. Commun Biol (2019) 2(1):203. doi: 10.1038/s42003-019-0442-2
28. Mvaya L, Mwale A, Hummel A, Phiri J, Kamng’ona R, Mzinza D, et al. Airway CD8+CD161++TCRvα7.2+ T Cell Depletion During Untreated HIV Infection Targets CD103 Expressing Cells. Front Immunol (2019) 10:2003. doi: 10.3389/fimmu.2019.02003
29. Lu B, Liu M, Wang J, Fan H, Yang D, Zhang L, et al. IL-17 production by tissue-resident MAIT cells is locally induced in children with pneumonia. Mucosal Immunol (2020) 117:1250. doi: 10.1038/s41385-020-0273-y
30. Teunissen MBM, Yeremenko NG, Baeten DLP, Chielie S, Spuls PI, de Rie MA, et al. The IL-17A-producing CD8+ T-cell population in psoriatic lesional skin comprises mucosa-associated invariant T cells and conventional T cells. J Invest Dermatol (2014) 134(12):2898–907. doi: 10.1038/jid.2014.261
31. Li J, Reantragoon R, Kostenko L, Corbett AJ, Varigos G, Carbone FR. The frequency of mucosal-associated invariant T cells is selectively increased in dermatitis herpetiformis. Australas J Dermatol (2017) 58(3):200–4. doi: 10.1111/ajd.12456
32. Gibbs A, Leeansyah E, Introini A, Paquin-Proulx D, Hasselrot K, Andersson E, et al. MAIT cells reside in the female genital mucosa and are biased towards IL-17 and IL-22 production in response to bacterial stimulation. Mucosal Immunol (2017) 10(1):35–45. doi: 10.1038/mi.2016.30
33. Dias J, Boulouis C, Gorin J-B, van den Biggelaar RHGA, Lal KG, Gibbs A, et al. The CD4-CD8- MAIT cell subpopulation is a functionally distinct subset developmentally related to the main CD8+ MAIT cell pool. Proc Natl Acad Sci USA (2018) 5(49):201812273–E11522. doi: 10.1073/pnas.1812273115
34. Solders M, Gorchs L, Erkers T, Lundell A-C, Nava S, Gidlöf S, et al. MAIT cells accumulate in placental intervillous space and display a highly cytotoxic phenotype upon bacterial stimulation. Sci Rep (2017) 7(1):6123. doi: 10.1038/s41598-017-06430-6
35. Constantinides MG, Link VM, Tamoutounour S, Wong AC, Perez-Chaparro PJ, Han S-J, et al. MAIT cells are imprinted by the microbiota in early life and promote tissue repair. Science (2019) 366(6464):eaax6624. doi: 10.1126/science.aax6624
36. Okabe Y, Medzhitov R. Tissue biology perspective on macrophages. Nat Immunol (2016) 17(1):9–17. doi: 10.1038/ni.3320
37. Fan X, Rudensky AY. Hallmarks of Tissue-Resident Lymphocytes. Cell (2016) 164(6):1198–211. doi: 10.1016/j.cell.2016.02.048
38. Ramsay G, Cantrell D. Environmental and metabolic sensors that control T cell biology. Front Immunol (2015) 6(2):99. doi: 10.3389/fimmu.2015.00099
39. Veldhoen M, Brucklacher-Waldert V. Dietary influences on intestinal immunity. Nat Rev Immunol (2012) 12(10):696–708. doi: 10.1038/nri3299
40. Gutiérrez-Vázquez C, Quintana FJ. Regulation of the Immune Response by the Aryl Hydrocarbon Receptor. Immunity (2018) 48(1):19–33. doi: 10.1016/j.immuni.2017.12.012
41. Quintana FJ, Basso AS, Iglesias AH, Korn T, Farez MF, Bettelli E, et al. Control of T(reg) and T(H)17 cell differentiation by the aryl hydrocarbon receptor. Nature (2008) 453(7191):65–71. doi: 10.1038/nature06880
42. Rothhammer V, Quintana FJ. The aryl hydrocarbon receptor: an environmental sensor integrating immune responses in health and disease. Nat Rev Immunol (2019) 19(3):184–97. doi: 10.1038/s41577-019-0125-8
43. Cua DJ, Tato CM. Innate IL-17-producing cells: The sentinels of the immune system. Nat Rev Immunol (2010) 10(7):479–89. doi: 10.1038/nri2800
44. Müller DN, Wilck N, Haase S, Kleinewietfeld M, Linker RA. Sodium in the microenvironment regulates immune responses and tissue homeostasis. Nat Rev Immunol (2019) 19(4):243–54. doi: 10.1038/s41577-018-0113-4
45. Fergusson JR, Smith KE, Fleming VM, Rajoriya N, Newell EW, Simmons R, et al. CD161 defines a transcriptional and functional phenotype across distinct human T cell lineages. Cell Rep (2014) 9(3):1075–88. doi: 10.1016/j.celrep.2014.09.045
46. Park D, Kim HG, Kim M, Park T, Ha H-H, Lee DH, et al. Differences in the molecular signatures of mucosal-associated invariant T cells and conventional T cells. Sci Rep (2019) 9(1):7094. doi: 10.1038/s41598-019-43578-9
47. Salou M, Legoux F, Gilet J, Darbois A, Halgouet du A, Alonso R, et al. A common transcriptomic program acquired in the thymus defines tissue residency of MAIT and NKT subsets. J Exp Med (2019) 216(1):133–51. doi: 10.1084/jem.20181483
48. Hinks TSC, Marchi E, Jabeen M, Olshansky M, Kurioka A, Pediongco TJ, et al. Activation and In Vivo Evolution of the MAIT Cell Transcriptome in Mice and Humans Reveals Tissue Repair Functionality. CellReports (2019) 28(12):3249–3262.e5. doi: 10.1016/j.celrep.2019.07.039
49. Lamichhane R, Schneider M, la Harpe de SM, Harrop TWR, Hannaway RF, Dearden PK, et al. TCR- or Cytokine-Activated CD8+ Mucosal-Associated Invariant T Cells Are Rapid Polyfunctional Effectors That Can Coordinate Immune Responses. Cell Rep (2019) 28(12):3061–5. doi: 10.1016/j.celrep.2019.08.054
50. Sharma M, Zhang S, Niu L, Lewinsohn DM, Zhang X, Huang S. Mucosal-Associated Invariant T Cells Develop an Innate-Like Transcriptomic Program in Anti-mycobacterial Responses. Front Immunol (2020) 11:1136. doi: 10.3389/fimmu.2020.01136
51. Belkaid Y, Segre JA. Dialogue between skin microbiota and immunity. Science (2014) 346(6212):954–9. doi: 10.1126/science.1260144
52. Chen YE, Fischbach MA, Belkaid Y. Skin microbiota-host interactions. Nature (2018) 553(7689):427–36. doi: 10.1038/nature25177
53. Monin L, Whettlock EM, Male V. Immune responses in the human female reproductive tract. Immunology (2020) 160(2):106–15. doi: 10.1111/imm.13136
54. Solis AG, Bielecki P, Steach HR, Sharma L, Harman CCD, Yun S, et al. Mechanosensation of cyclical force by PIEZO1 is essential for innate immunity. Nature (2019) 78:630. doi: 10.1038/s41586-019-1755-5
55. Ussher JE, Bilton M, Attwod E, Shadwell J, Richardson R, de Lara C, et al. CD161++ CD8+ T cells, including the MAIT cell subset, are specifically activated by IL-12+IL-18 in a TCR-independent manner. Eur J Immunol (2014) 44(1):195–203. doi: 10.1002/eji.201343509
56. Legoux F, Bellet D, Daviaud C, Morr El Y, Darbois A, Niort K, et al. Microbial metabolites control the thymic development of mucosal-associated invariant T cells. Science (2019) 366(6464):494–9. doi: 10.1126/science.aaw2719
57. Cheuk S, Schlums H, Gallais Sérézal I, Martini E, Chiang SC, Marquardt N, et al. CD49a Expression Defines Tissue-Resident CD8+ T Cells Poised for Cytotoxic Function in Human Skin. Immunity (2017) 46(2):287–300. doi: 10.1016/j.immuni.2017.01.009
58. Ginhoux F, Jung S. Monocytes and macrophages: developmental pathways and tissue homeostasis. Nat Rev Immunol (2014) 14(6):392–404. doi: 10.1038/nri3671
59. FitzPatrick MEB, Provine NM, Garner LC, Powell K, Amini A, Irwin S, et al. Human intestinal tissue-resident memory CD8+ T cells comprise transcriptionally and functionally distinct subsets. bioRxiv (2019) 869917. doi: 10.1101/869917
60. Perdiguero EG, Geissmann F. The development and maintenance of resident macrophages. Nat Immunol (2016) 17(1):2–8. doi: 10.1038/ni.3341
61. Voillet V, Buggert M, Slichter CK, Berkson JD, Mair F, Addison MM, et al. Human MAIT cells exit peripheral tissues and recirculate via lymph in steady state conditions. JCI Insight (2018) 3(7):380. doi: 10.1172/jci.insight.98487
62. Maggi L, Santarlasci V, Capone M, Peired A, Frosali F, Crome SQ, et al. CD161 is a marker of all human IL-17-producing T-cell subsets and is induced by RORC. Eur J Immunol (2010) 40(8):2174–81. doi: 10.1002/eji.200940257
63. Pesenacker AM, Bending D, Ursu S, Wu Q, Nistala K, Wedderburn LR. CD161 defines the subset of FoxP3+ T cells capable of producing proinflammatory cytokines. Blood (2013) 121(14):2647–58. doi: 10.1182/blood-2012-08-443473
64. Povoleri GAM, Nova-Lamperti E, Scotta C, Fanelli G, Chen Y-C, Becker PD, et al. Human retinoic acid-regulated CD161+ regulatory T cells support wound repair in intestinal mucosa. Nat Immunol (2018) 19(12):1403–14. doi: 10.1038/s41590-018-0230-z
65. Simoni Y, Fehlings M, Kloverpris HN, McGovern N, Koo S-L, Loh CY, et al. Human Innate Lymphoid Cell Subsets Possess Tissue-Type Based Heterogeneity in Phenotype and Frequency. Immunity (2017) 46(1):148–61. doi: 10.1016/j.immuni.2016.11.005
66. Moreira-Teixeira L, Resende M, Coffre M, Devergne O, Herbeuval J-P, Hermine O, et al. Proinflammatory environment dictates the IL-17-producing capacity of human invariant NKT cells. J Immunol (2011) 186(10):5758–65. doi: 10.4049/jimmunol.1003043
67. Garner LC, Klenerman P, Provine NM. Insights Into Mucosal-Associated Invariant T Cell Biology From Studies of Invariant Natural Killer T Cells. Front Immunol (2018) 9:1478. doi: 10.3389/fimmu.2018.01478
68. Veldhoen M. Interleukin 17 is a chief orchestrator of immunity. Nat Immunol (2017) 18(6):612–21. doi: 10.1038/ni.3742
69. Rahimpour A, Koay HF, Enders A, Clanchy R, Eckle SBG, Meehan B, et al. Identification of phenotypically and functionally heterogeneous mouse mucosal-associated invariant T cells using MR1 tetramers. J Exp Med (2015) 212(7):1095–108. doi: 10.1084/jem.20142110
70. Leeansyah E, Svärd J, Dias J, Buggert M, Nyström J, Quigley MF, et al. Arming of MAIT Cell Cytolytic Antimicrobial Activity Is Induced by IL-7 and Defective in HIV-1 Infection. PloS Pathog (2015) 11(8):e1005072. doi: 10.1371/journal.ppat.1005072
71. Wang H, Kjer-Nielsen L, Shi M, D’Souza C, Pediongco TJ, Cao H, et al. IL-23 costimulates antigen-specific MAIT cell activation and enables vaccination against bacterial infection. Sci Immunol Sci Immunol (2019) 4(41):eaaw0402. doi: 10.1126/sciimmunol.aaw0402
72. Tang X-Z, Jo J, Tan AT, Sandalova E, Chia A, Tan KC, et al. IL-7 licenses activation of human liver intrasinusoidal mucosal-associated invariant T cells. J Immunol (2013) 190(7):3142–52. doi: 10.4049/jimmunol.1203218
73. Varelias A, Bunting MD, Ormerod KL, Koyama M, Olver SD, Straube J, et al. Recipient mucosal-associated invariant T cells control GVHD within the colon. J Clin Invest (2018) 128(5):1919–36. doi: 10.1172/JCI91646
74. Linehan JL, Harrison OJ, Han S-J, Byrd AL, Vujkovic-Cvijin I, Villarino AV, et al. Non-classical Immunity Controls Microbiota Impact on Skin Immunity and Tissue Repair. Cell (2018) 172(4):784–796.e18. doi: 10.1016/j.cell.2017.12.033
75. Nosbaum A, Prevel N, Truong H-A, Mehta P, Ettinger M, Scharschmidt TC, et al. Cutting Edge: Regulatory T Cells Facilitate Cutaneous Wound Healing. J Immunol (2016) 196(5):2010–4. doi: 10.4049/jimmunol.1502139
76. Chen Y, Chou K, Fuchs E, Havran WL, Boismenu R. Protection of the intestinal mucosa by intraepithelial gamma delta T cells. Proc Natl Acad Sci (2002) 99(22):14338–43. doi: 10.1073/pnas.212290499
77. King DP, Hyde DM, Jackson KA, Novosad DM, Ellis TN, Putney L, et al. Cutting edge: protective response to pulmonary injury requires gamma delta T lymphocytes. J Immunol J Immunol (1999) 162(9):5033–6.
78. Guo X-ZJ, Dash P, Crawford JC, Allen EK, Zamora AE, Boyd DF, et al. Lung γδ T Cells Mediate Protective Responses during Neonatal Influenza Infection that Are Associated with Type 2 Immunity. Immunity (2018) 49(3):531–6. doi: 10.1016/j.immuni.2018.07.011
79. Rouxel O, Da Silva J, Beaudoin L, Nel I, Tard C, Cagninacci L, et al. Cytotoxic and regulatory roles of mucosal-associated invariant T cells in type 1 diabetes. Nat Immunol (2017) 491:717. doi: 10.1038/ni.3854
80. Bhattacharyya A, Hanafi L-A, Sheih A, Golob JL, Srinivasan S, Boeckh MJ, et al. Graft-Derived Reconstitution of Mucosal-Associated Invariant T Cells after Allogeneic Hematopoietic Cell Transplantation. Biol Blood Marrow Transplant (2018) 24(2):242–51. doi: 10.1016/j.bbmt.2017.10.003
81. Konuma T, Kohara C, Watanabe E, Takahashi S, Ozawa G, Suzuki K, et al. Reconstitution of Circulating Mucosal-Associated Invariant T Cells after Allogeneic Hematopoietic Cell Transplantation: Its Association with the Riboflavin Synthetic Pathway of Gut Microbiota in Cord Blood Transplant Recipients. J Immunol (2020) 204(6):1462–73. doi: 10.4049/jimmunol.1900681
82. Sáez de Guinoa J, Jimeno R, Gaya M, Kipling D, Garzón MJ, Dunn-Walters D, et al. CD1d-mediated lipid presentation by CD11c+ cells regulates intestinal homeostasis. EMBO J (2018) 37(5):e97537. doi: 10.15252/embj.201797537
83. Toubal A, Kiaf B, Beaudoin L, Cagninacci L, Rhimi M, Fruchet B, et al. Mucosal-associated invariant T cells promote inflammation and intestinal dysbiosis leading to metabolic dysfunction during obesity. Nat Commun (2020) 11(1):3755–20. doi: 10.1038/s41467-020-17307-0
84. Nielsen MM, Witherden DA, Havran WL. γδ T cells in homeostasis and host defence of epithelial barrier tissues. Nat Rev Immunol (2017) 17(12):733–45. doi: 10.1038/nri.2017.101
85. Bonneville M, O’Brien RL, Born WK. Gammadelta T cell effector functions: a blend of innate programming and acquired plasticity. Nat Rev Immunol (2010) 10(7):467–78. doi: 10.1038/nri2781
86. Ali N, Zirak B, Rodriguez RS, Pauli ML, Truong H-A, Lai K, et al. Regulatory T Cells in Skin Facilitate Epithelial Stem Cell Differentiation. Cell (2017) 169(6):1119–29. doi: 10.1016/j.cell.2017.05.002
87. Kohlgruber AC, Gal-Oz ST, LaMarche NM, Shimazaki M, Duquette D, Koay HF, et al. γδ T cells producing interleukin-17A regulate adipose regulatory T cell homeostasis and thermogenesis. Nat Immunol (2018) 19(5):464–74. doi: 10.1038/s41590-018-0094-2
88. Hu B, Jin C, Zeng X, Resch JM, Jedrychowski MP, Yang Z, et al. γδ T cells and adipocyte IL-17RC control fat innervation and thermogenesis. Nature (2020) Feb 19578(7796):610–4. doi: 10.1038/s41586-020-2028-z
89. Burg von N, Chappaz S, Baerenwaldt A, Horvath E, Bose Dasgupta S, Ashok D, et al. Activated group 3 innate lymphoid cells promote T-cell-mediated immune responses. Proc Natl Acad Sci USA (2014) 111(35):12835–40. doi: 10.1073/pnas.1406908111
90. Salio M, Gasser O, Gonzalez-Lopez C, Martens A, Veerapen N, Gileadi U, et al. Activation of Human Mucosal-Associated Invariant T Cells Induces CD40L-Dependent Maturation of Monocyte-Derived and Primary Dendritic Cells. J Immunol (2017) 199(8):2631–8. doi: 10.4049/jimmunol.1700615
91. Martin CE, Spasova DS, Frimpong-Boateng K, Kim H-O, Lee M, Kim KS, et al. Interleukin-7 Availability Is Maintained by a Hematopoietic Cytokine Sink Comprising Innate Lymphoid Cells and T Cells. Immunity (2017) 47(1):171–4. doi: 10.1016/j.immuni.2017.07.005
92. Buck MD, O’Sullivan D, Pearce EL. T cell metabolism drives immunity. J Exp Med (2015) 212(9):1345–60. doi: 10.1084/jem.20151159
93. MacIver NJ, Michalek RD, Rathmell JC. Metabolic regulation of T lymphocytes. Annu Rev Immunol (2013) 31(1):259–83. doi: 10.1146/annurev-immunol-032712-095956
94. Chapman NM, Boothby MR, Chi H. Metabolic coordination of T cell quiescence and activation. Nat Rev Immunol (2020) 20(1):55–70. doi: 10.1038/s41577-019-0203-y
95. Zinser ME, Highton AJ, Kurioka A, Kronsteiner B, Hagel J, Leng T, et al. Human MAIT cells show metabolic quiescence with rapid glucose-dependent upregulation of granzyme B upon stimulation. Immunol Cell Biol (2018) Feb 9117(6):1250–674. doi: 10.26226/morressier.5aeaf2d45800a000190980a8
96. O’Brien A, Loftus RM, Pisarska MM, Tobin LM, Bergin R, Wood NAW, et al. Obesity Reduces mTORC1 Activity in Mucosal-Associated Invariant T Cells, Driving Defective Metabolic and Functional Responses. J Immunol (2019) 202(12):ji1801600–3411. doi: 10.4049/jimmunol.1801600
97. Bulitta B, Zuschratter W, Bernal I, Bruder D, Klawonn F, Bergen von M, et al. Proteomic definition of human mucosal-associated invariant T cells determines their unique molecular effector phenotype. Eur J Immunol (2018) 192:4475. doi: 10.1002/eji.201747398
98. O’Brien A, Kedia-Mehta N, Tobin L, Veerapen N, Besra GS, Shea DO, et al. Targeting mitochondrial dysfunction in MAIT cells limits IL-17 production in obesity. Cell Mol Immunol (2020) 17(11):1193–5. doi: 10.1038/s41423-020-0375-1
99. Konjar Š, Veldhoen M. Dynamic Metabolic State of Tissue Resident CD8 T Cells. Front Immunol (2019) 10:1683. doi: 10.3389/fimmu.2019.01683
100. Phan AT, Goldrath AW, Glass CK. Metabolic and Epigenetic Coordination of T Cell and Macrophage Immunity. Immunity (2017) 46(5):714–29. doi: 10.1016/j.immuni.2017.04.016
101. Pan Y, Tian T, Park CO, Lofftus SY, Mei S, Liu X, et al. Survival of tissue-resident memory T cells requires exogenous lipid uptake and metabolism. Nature Nat Publishing Group (2017) 543(7644):252–6. doi: 10.1038/nature21379
102. Frizzell H, Fonseca R, Christo SN, Evrard M, Cruz-Gomez S, Zanluqui NG, et al. Organ-specific isoform selection of fatty acid-binding proteins in tissue-resident lymphocytes. Sci Immunol Sci Immunol (2020) 5(46):eaay9283. doi: 10.1126/sciimmunol.aay9283
103. Berod L, Friedrich C, Nandan A, Freitag J, Hagemann S, Harmrolfs K, et al. De novo fatty acid synthesis controls the fate between regulatory T and T helper 17 cells. Nat Med (2014) 20(11):1327–33. doi: 10.1038/nm.3704
104. Mielke LA, Liao Y, Clemens EB, Firth MA, Duckworth B, Huang Q, et al. TCF-1 limits the formation of Tc17 cells via repression of the MAF-RORγt axis. J Exp Med (2019) 216(7):1682–99. doi: 10.1084/jem.20181778
105. Wei J, Long L, Yang K, Guy C, Shrestha S, Chen Z, et al. Autophagy enforces functional integrity of regulatory T cells by coupling environmental cues and metabolic homeostasis. Nat Immunol (2016) 17(3):277–85. doi: 10.1038/ni.3365
106. Swadling L, Pallett LJ, Diniz MO, Baker JM, Amin OE, Stegmann KA, et al. Human Liver Memory CD8+ T Cells Use Autophagy for Tissue Residence. Cell Rep (2020) 30(3):687–98. doi: 10.1016/j.celrep.2019.12.050
107. Cao W, Kayama H, Chen ML, Delmas A, Sun A, Kim SY, et al. The Xenobiotic Transporter Mdr1 Enforces T Cell Homeostasis in the Presence of Intestinal Bile Acids. Immunity (2017) 47(6):1182–96. doi: 10.1016/j.immuni.2020.01.019
108. Fergusson JR, Ussher JE, Kurioka A, Klenerman P, Walker LJ. High MDR-1 expression by MAIT cells confers resistance to cytotoxic but not immunosuppressive MDR-1 substrates. Clin Exp Immunol (2018) 5(21):e98–191. doi: 10.1111/cei.13165
109. Lee YJ, Starrett GJ, Lee ST, Yang R, Henzler CM, Jameson SC, et al. Lineage-Specific Effector Signatures of Invariant NKT Cells Are Shared amongst γδ T, Innate Lymphoid, and Th Cells. J Immunol (2016) 197(4):1460–70. doi: 10.4049/jimmunol.1600643
110. Hara-Chikuma M, Chikuma S, Sugiyama Y, Kabashima K, Verkman AS, Inoue S, et al. Chemokine-dependent T cell migration requires aquaporin-3-mediated hydrogen peroxide uptake. J Exp Med (2012) 209(10):1743–52. doi: 10.1084/jem.20112398
111. Atarashi K, Nishimura J, Shima T, Umesaki Y, Yamamoto M, Onoue M, et al. ATP drives lamina propria T(H)17 cell differentiation. Nature (2008) 455(7214):808–12. doi: 10.1038/nature07240
112. Hubert S, Rissiek B, Klages K, Huehn J, Sparwasser T, Haag F, et al. Extracellular NAD+ shapes the Foxp3+ regulatory T cell compartment through the ART2-P2X7 pathway. J Exp Med (2010) 207(12):2561–8. doi: 10.1084/jem.20091154
113. Bovens AA, Wesselink TH, Behr FM, Kragten NAM, van Lier RAW, van Gisbergen KPJM, et al. Murine iNKT cells are depleted by liver damage via activation of P2RX7. Eur J Immunol (2020) 50(10):1515–24. doi: 10.1002/eji.201948509
114. Crittenden S, Cheyne A, Adams A, Forster T, Robb CT, Felton J, et al. Purine metabolism controls innate lymphoid cell function and protects against intestinal injury. Immunol Cell Biol (2018) 96(10):1049–59. doi: 10.1111/imcb.12167
115. Stark R, Wesselink TH, Behr FM, Kragten NAM, Arens R, Koch-Nolte F, et al. TRMmaintenance is regulated by tissue damage via P2RX7. Sci Immunol Sci Immunol (2018) 3(30):eaau1022. doi: 10.1126/sciimmunol.aau1022
116. Rakoff-Nahoum S, Paglino J, Eslami-Varzaneh F, Edberg S, Medzhitov R. Recognition of commensal microflora by toll-like receptors is required for intestinal homeostasis. Cell (2004) 118(2):229–41. doi: 10.1016/j.cell.2004.07.002
117. Belkaid Y, Tamoutounour S. The influence of skin microorganisms on cutaneous immunity. Nat Rev Immunol (2016) 16(6):353–66. doi: 10.1038/nri.2016.48
118. Naik S, Bouladoux N, Wilhelm C, Molloy MJ, Salcedo R, Kastenmüller W, et al. Compartmentalized control of skin immunity by resident commensals. Science (2012) 337(6098):1115–9. doi: 10.1126/science.1225152
119. Kennedy EA, King KY, Baldridge MT. Mouse Microbiota Models: Comparing Germ-Free Mice and Antibiotics Treatment as Tools for Modifying Gut Bacteria. Front Physiol (2018) 9:1534. doi: 10.3389/fphys.2018.01534
120. Beura LK, Hamilton SE, Bi K, Schenkel JM, Odumade OA, Casey KA, et al. Normalizing the environment recapitulates adult human immune traits in laboratory mice. Nature (2016) 532(7600):512–6. doi: 10.1038/nature17655
121. Koay HF, Gherardin NA, Enders A, Loh L, Mackay LK, Almeida CF, et al. A three-stage intrathymic development pathway for the mucosal-associated invariant T cell lineage. Nat Immunol (2016) 17(11):1300–11. doi: 10.1038/ni.3565
122. Olszak T, An D, Zeissig S, Vera MP, Richter J, Franke A, et al. Microbial exposure during early life has persistent effects on natural killer T cell function. Science (2012) Apr 27336(6080):489–93. doi: 10.1126/science.1219328
123. Howson LJ, Awad W, Borstel von A, Lim HJ, McWilliam HEG, Sandoval-Romero ML, et al. Absence of mucosal-associated invariant T cells in a person with a homozygous point mutation in MR1. Sci Immunol Sci Immunol (2020) 5(49):eabc9492. doi: 10.1126/sciimmunol.abc9492
124. Gherardin NA, Souter MN, Koay HF, Mangas KM, Seemann T, Stinear TP, et al. Human blood MAIT cell subsets defined using MR1 tetramers. Immunol Cell Biol (2018) 16(5):1114–525. doi: 10.1111/imcb.12021
125. Ben Youssef G, Tourret M, Salou M, Ghazarian L, Houdouin V, Mondot S, et al. Ontogeny of human mucosal-associated invariant T cells and related T cell subsets. J Exp Med (2018) 215(2):459–79. doi: 10.1084/jem.20171739
126. Twigg HL, Knox KS, Zhou J, Crothers KA, Nelson DE, Toh E, et al. Effect of Advanced HIV Infection on the Respiratory Microbiome. Am J Respir Crit Care Med (2016) 194(2):226–35. doi: 10.1164/rccm.201509-1875OC
127. Lathrop SK, Bloom SM, Rao SM, Nutsch K, Lio C-W, Santacruz N, et al. Peripheral education of the immune system by colonic commensal microbiota. Nature (2011) 478(7368):250–4. doi: 10.1038/nature10434
128. Grice EA, Kong HH, Renaud G, Young AC, NISC Comparative Sequencing Program, Bouffard GG, et al. A Diversity Profile Hum Skin Microbiota. Genome Res (2008) 18(7):1043–50. doi: 10.1101/gr.075549.107
129. Claesson MJ, Jeffery IB, Conde S, Power SE, O’Connor EM, Cusack S, et al. Gut microbiota composition correlates with diet and health in the elderly. Nature (2012) 488(7410):178–84. doi: 10.1038/nature11319
130. Tastan C, Karhan E, Zhou W, Fleming E, Voigt AY, Yao X, et al. Tuning of human MAIT cell activation by commensal bacteria species and MR1-dependent T-cell presentation. Mucosal Immunol (2018) 117(Database issue):1250–605. doi: 10.1038/s41385-018-0072-x
131. Krause JL, Schäpe SS, Schattenberg F, Müller S, Ackermann G, Rolle-Kampczyk UE, et al. The Activation of Mucosal-Associated Invariant T (MAIT) Cells Is Affected by Microbial Diversity and Riboflavin Utilization in vitro. Front Microbiol (2020) 11:755. doi: 10.3389/fmicb.2020.00755
132. Davanian H, Gaiser RA, Silfverberg M, Hugerth LW, Sobkowiak MJ, Lu L, et al. Mucosal-associated invariant T cells and oral microbiome in persistent apical periodontitis. Int J Oral Sci (2019) 11(2):16. doi: 10.1038/s41368-019-0049-y
133. Meller S, Di Domizio J, Voo KS, Friedrich HC, Chamilos G, Ganguly D, et al. T(H)17 cells promote microbial killing and innate immune sensing of DNA via interleukin 26. Nat Immunol (2015) 16(9):970–9. doi: 10.1038/ni.3211
134. Kurioka A, van Wilgenburg B, Javan RR, Hoyle R, van Tonder AJ, Harrold CL, et al. Diverse Streptococcus pneumoniae Strains Drive a Mucosal-Associated Invariant T-Cell Response Through Major Histocompatibility Complex class I-Related Molecule-Dependent and Cytokine-Driven Pathways. J Infect Dis (2018) 217(6):988–99. doi: 10.1093/infdis/jix647
135. Mendler A, Geier F, Haange S-B, Pierzchalski A, Krause JL, Nijenhuis I, et al. Mucosal-associated invariant T-Cell (MAIT) activation is altered by chlorpyrifos- and glyphosate-treated commensal gut bacteria. J Immunotoxicol (2020) 17(1):10–20. doi: 10.1080/1547691X.2019.1706672
136. Johansson MA, Björkander S, Mata Forsberg M, Qazi KR, Salvany Celades M, Bittmann J, et al. Probiotic Lactobacilli Modulate Staphylococcus aureus-Induced Activation of Conventional and Unconventional T cells and NK Cells. Front Immunol (2016) 7(JUL):273. doi: 10.3389/fimmu.2016.00273
137. Koh A, De Vadder F, Kovatcheva-Datchary P, Bäckhed F. From Dietary Fiber to Host Physiology: Short-Chain Fatty Acids as Key Bacterial Metabolites. Cell (2016) 165(6):1332–45. doi: 10.1016/j.cell.2016.05.041
138. Smith PM, Howitt MR, Panikov N, Michaud M, Gallini CA. Bohlooly-Y MThe microbial metabolites, short-chain fatty acids, regulate colonic Treg cell homeostasis. Science (2013) 341(6145):569–73. doi: 10.1126/science.1241165
139. Arpaia N, Campbell C, Fan X, Dikiy S, van der Veeken J, deRoos P, et al. Metabolites produced by commensal bacteria promote peripheral regulatory T-cell generation. Nature (2013) 504(7480):451–5. doi: 10.1038/nature12726
140. Furusawa Y, Obata Y, Fukuda S, Endo TA, Nakato G, Takahashi D, et al. Commensal microbe-derived butyrate induces the differentiation of colonic regulatory T cells. Nature (2013) 504(7480):446–50. doi: 10.1038/nature12721
141. Ohnmacht C, Park J-H, Cording S, Wing JB, Atarashi K, Obata Y, et al. MUCOSAL IMMUNOLOGYThe microbiota regulates type 2 immunity through RORγt⁺ T cells. Science (2015) 349(6251):989–93. doi: 10.1126/science.aac4263
142. Fachi JL, Sécca C, Rodrigues PB, Mato FCP, Di Luccia B, Felipe J de S, et al. Acetate coordinates neutrophil and ILC3 responses against C. difficile through FFAR2. J Exp Med (2020) 217(3):27. doi: 10.1084/jem.20190489
143. Kim KS, Hong S-W, Han D, Yi J, Jung J, Yang B-G, et al. Dietary antigens limit mucosal immunity by inducing regulatory T cells in the small intestine. Science (2016) 351(6275):858–63. doi: 10.1126/science.aac5560
144. Mora JR, Iwata M, Andrian von UH. Vitamin effects on the immune system: Vitamins A and D take centre stage. Nat Rev Immunol (2008) 8(9):685–98. doi: 10.1038/nri2378
145. Tanoue T, Atarashi K, Honda K. Development and maintenance of intestinal regulatory T cells. Nat Rev Immunol (2016) 16(5):295–309. doi: 10.1038/nri.2016.36
146. Coombes JL, Siddiqui KRR, Arancibia-Cárcamo CV, Hall J, Sun CM, Belkaid Y, et al. A functionally specialized population of mucosal CD103+ DCs induces Foxp3+ regulatory T cells via a TGF-beta and retinoic acid-dependent mechanism. J Exp Med (2007) 204(8):1757–64. doi: 10.1084/jem.20070590
147. Sun CM, Hall JA, Blank RB, Bouladoux N, Oukka M, Mora JR, et al. Small intestine lamina propria dendritic cells promote de novo generation of Foxp3 T reg cells via retinoic acid. J Exp Med (2007) 204(8):1775–85. doi: 10.1084/jem.20070602
148. Mucida D, Park Y, Kim G, Turovskaya O, Scott I, Kronenberg M, et al. Reciprocal TH17 and regulatory T cell differentiation mediated by retinoic acid. Science (2007) 317(5835):256–60. doi: 10.1126/science.1145697
149. Kim MH, Taparowsky EJ, Kim CH. Retinoic Acid Differentially Regulates the Migration of Innate Lymphoid Cell Subsets to the Gut. Immunity (2015) 43(1):107–19. doi: 10.1016/j.immuni.2015.06.009
150. Bernink JH, Krabbendam L, Germar K, de Jong E, Gronke K, Kofoed-Nielsen M, et al. Interleukin-12 and -23 Control Plasticity of CD127(+) Group 1 and Group 3 Innate Lymphoid Cells in the Intestinal Lamina Propria. Immunity (2015) 43(1):146–60. doi: 10.1016/j.immuni.2015.06.019
151. Mielke LA, Jones SA, Raverdeau M, Higgs R, Stefanska A, Groom JR, et al. Retinoic acid expression associates with enhanced IL-22 production by γδ T cells and innate lymphoid cells and attenuation of intestinal inflammation. J Exp Med (2013) 210(6):1117–24. doi: 10.1084/jem.20121588
152. Liu Q, Kim CH. Control of Tissue-Resident Invariant NKT Cells by Vitamin A Metabolites and P2X7-Mediated Cell Death. J Immunol (2019) 203(5):1189–97. doi: 10.4049/jimmunol.1900398
153. Raverdeau M, Breen CJ, Misiak A, Mills KH. Retinoic acid suppresses IL-17 production and pathogenic activity of γδ T cells in CNS autoimmunity. Immunol Cell Biol (2016) 94(8):763–73. doi: 10.1038/icb.2016.39
154. Rampal R, Wari N, Singh AK, Das U, Bopanna S, Gupta V, et al. Retinoic Acid Is Elevated in the Mucosa of Patients With Active Ulcerative Colitis and Displays a Proinflammatory Role by Augmenting IL-17 and IFNγ Production. Inflammation Bowel Dis (2020) 448(2):427. doi: 10.1093/ibd/izaa121
155. Liu W, Chen Y, Golan MA, Annunziata ML, Du J, Dougherty U, et al. Intestinal epithelial vitamin D receptor signaling inhibits experimental colitis. J Clin Invest (2013) 123(9):3983–96. doi: 10.1172/JCI65842
156. Essen von MR, Kongsbak M, Schjerling P, Olgaard K, Ødum N, Geisler C. Vitamin D controls T cell antigen receptor signaling and activation of human T cells. Nat Immunol (2010) 11(4):344–9. doi: 10.1038/ni.1851
157. Palmer MT, Lee YK, Maynard CL, Oliver JR, Bikle DD, Jetten AM, et al. Lineage-specific effects of 1,25-dihydroxyvitamin D3 on the development of effector CD4 T cells. J Biol Chem (2011) 286(2):997–1004. doi: 10.1074/jbc.M110.163790
158. Konya V, Czarnewski P, Forkel M, Rao A, Kokkinou E, Villablanca EJ, et al. Vitamin D downregulates the IL-23 receptor pathway in human mucosal group 3 innate lymphoid cells. J Allergy Clin Immunol (2018) 141(1):279–92. doi: 10.1016/j.jaci.2017.01.045
159. Joshi S, Pantalena L-C, Liu XK, Gaffen SL, Liu H, Rohowsky-Kochan C, et al. 1,25-dihydroxyvitamin D(3) ameliorates Th17 autoimmunity via transcriptional modulation of interleukin-17A. Mol Cell Biol (2011) 31(17):3653–69. doi: 10.1128/MCB.05020-11
160. Yu S, Cantorna MT. Epigenetic reduction in invariant NKT cells following in utero vitamin D deficiency in mice. J Immunol (2011) 186(3):1384–90. doi: 10.4049/jimmunol.1002545
161. Bruce D, Cantorna MT. Intrinsic requirement for the vitamin D receptor in the development of CD8αα-expressing T cells. J Immunol (2011) 186(5):2819–25. doi: 10.4049/jimmunol.1003444
162. Hinks TSC, Zhou X, Staples KJ, Dimitrov BD, Manta A, Petrossian T, et al. Innate and adaptive T cells in asthmatic patients: Relationship to severity and disease mechanisms. J Allergy Clin Immunol (2015) 136(2):323–33. doi: 10.1016/j.jaci.2015.01.014
163. Pincikova T, Paquin-Proulx D, Sandberg JK, Flodström-Tullberg M, Hjelte L. Vitamin D treatment modulates immune activation in cystic fibrosis. Clin Exp Immunol (2017) 189(3):359–71. doi: 10.1111/cei.12984
164. Santori FR, Huang P, van de Pavert SA, Douglass EF, Leaver DJ, Haubrich BA, et al. Identification of natural RORγ ligands that regulate the development of lymphoid cells. Cell Metab (2015) 21(2):286–98. doi: 10.1016/j.cmet.2015.01.004
165. Emgård J, Kammoun H, García-Cassani B, Chesné J, Parigi SM, Jacob J-M, et al. Oxysterol Sensing through the Receptor GPR183 Promotes the Lymphoid-Tissue-Inducing Function of Innate Lymphoid Cells and Colonic Inflammation. Immunity (2018) 48(1):120–8. doi: 10.1016/j.immuni.2017.11.020
166. Chen ML, Takeda K, Sundrud MS. Emerging roles of bile acids in mucosal immunity and inflammation. Mucosal Immunol (2019) 12(4):851–61. doi: 10.1038/s41385-019-0162-4
167. Song X, Sun X, Oh SF, Wu M, Zhang Y, Zheng W, et al. Microbial bile acid metabolites modulate gut RORγ+ regulatory T cell homeostasis. Nature (2020) 577(7790):410–15. doi: 10.1038/s41586-019-1865-0
168. Hang S, Paik D, Yao L, Kim E, Jamma T, Lu J, et al. Bile acid metabolites control TH17 and Treg cell differentiation. Nature (2019) 576(7785):143–8. doi: 10.1038/s41586-019-1785-z
169. Mendler A, Pierzchalski A, Bauer M, Röder S, Sattler A, Standl M, et al. MAIT cell activation in adolescents is impacted by bile acid concentrations and body weight. Clin Exp Immunol (2020) 200(2):199–213. doi: 10.1111/cei.13423
170. Veldhoen M, Hirota K, Westendorf AM, Buer J, Dumoutier L, Renauld J-C, et al. The aryl hydrocarbon receptor links TH17-cell-mediated autoimmunity to environmental toxins. Nature (2008) 453(7191):106–9. doi: 10.1038/nature06881
171. Martin B, Hirota K, Cua DJ, Stockinger B, Veldhoen M. Interleukin-17-producing gammadelta T cells selectively expand in response to pathogen products and environmental signals. Immunity (2009) 31(2):321–30. doi: 10.1016/j.immuni.2009.06.020
172. Li Y, Innocentin S, Withers DR, Roberts NA, Gallagher AR, Grigorieva EF, et al. Exogenous stimuli maintain intraepithelial lymphocytes via aryl hydrocarbon receptor activation. Cell (2011) 147(3):629–40. doi: 10.1016/j.cell.2011.09.025
173. Stockinger B, Di Meglio P, Gialitakis M, Duarte JH. The aryl hydrocarbon receptor: multitasking in the immune system. Annu Rev Immunol (2014) 32(1):403–32. doi: 10.1146/annurev-immunol-032713-120245
174. Kadow S, Jux B, Zahner SP, Wingerath B, Chmill S, Clausen BE, et al. Aryl hydrocarbon receptor is critical for homeostasis of invariant gammadelta T cells in the murine epidermis. J Immunol (2011) 187(6):3104–10. doi: 10.4049/jimmunol.1100912
175. Zaid A, Mackay LK, Rahimpour A, Braun A, Veldhoen M, Carbone FR, et al. Persistence of skin-resident memory T cells within an epidermal niche. Proc Natl Acad Sci USA (2014) 111(14):5307–12. doi: 10.1073/pnas.1322292111
176. Kurd NS, He Z, Louis TL, Milner JJ, Omilusik KD, Jin W, et al. Early precursors and molecular determinants of tissue-resident memory CD8+ T lymphocytes revealed by single-cell RNA sequencing. Sci Immunol Sci Immunol (2020) 5(47):eaaz6894. doi: 10.1126/sciimmunol.aaz6894
177. Shin JH, Zhang L, Murillo-Sauca O, Kim J, Kohrt HEK, Bui JD, et al. Modulation of natural killer cell antitumor activity by the aryl hydrocarbon receptor. Proc Natl Acad Sci USA (2013) 110(30):12391–6. doi: 10.1073/pnas.1302856110
178. Gaardbo JC, Trosied M, Stiksrud B, Midttun O, Ueland PM, Ullum H, et al. Increased Tryptophan Catabolism Is Associated With Increased Frequency of CD161+Tc17/MAIT Cells and Lower CD4+ T-Cell Count in HIV-1 Infected Patients on cART After 2 Years of Follow-Up. J Acquir Immune Defic Syndr (2015) 70(3):228–35. doi: 10.1097/QAI.0000000000000758
179. Zhang D, Jin W, Wu R, Li J, Park S-A, Tu E, et al. High Glucose Intake Exacerbates Autoimmunity through Reactive-Oxygen-Species-Mediated TGF-β Cytokine Activation. Immunity (2019) 51(4):671–5. doi: 10.1016/j.immuni.2019.08.001
180. Endo Y, Asou HK, Matsugae N, Hirahara K, Shinoda K, Tumes DJ, et al. Obesity Drives Th17 Cell Differentiation by Inducing the Lipid Metabolic Kinase, ACC1. Cell Rep (2015) 12(6):1042–55. doi: 10.1016/j.celrep.2015.07.014
181. Goldberg EL, Molony RD, Kudo E, Sidorov S, Kong Y, Dixit VD, et al. Ketogenic diet activates protective γδ T cell responses against influenza virus infection. Sci Immunol Sci Immunol (2019) 4(41):eaav2026. doi: 10.1126/sciimmunol.aav2026
182. LaMarche NM, Kane H, Kohlgruber AC, Dong H, Lynch L, Brenner MB. Distinct iNKT Cell Populations Use IFNγ or ER Stress-Induced IL-10 to Control Adipose Tissue Homeostasis. Cell Metab (2020) 32(2):243–6. doi: 10.1016/j.cmet.2020.05.017
183. Medzhitov R. Origin and physiological roles of inflammation. Nature (2008) 454(7203):428–35. doi: 10.1038/nature07201
184. Haas R, Marelli-Berg F, Mauro C. In the eye of the storm: T cell behavior in the inflammatory microenvironment. Am J Clin Exp Immunol (2013) 2(2):146–55.
185. Colgan SP, Furuta GT, Taylor CT. Hypoxia and Innate Immunity: Keeping Up with the HIFsters. Annu Rev Immunol (2020) 38(1):341–63. doi: 10.1146/annurev-immunol-100819-121537
186. Kelly CJ, Zheng L, Campbell EL, Saeedi B, Scholz CC, Bayless AJ, et al. Crosstalk between Microbiota-Derived Short-Chain Fatty Acids and Intestinal Epithelial HIF Augments Tissue Barrier Function. Cell Host Microbe (2015) 17(5):662–71. doi: 10.1016/j.chom.2015.03.005
187. Doedens AL, Phan AT, Stradner MH, Fujimoto JK, Nguyen JV, Yang E, et al. Hypoxia-inducible factors enhance the effector responses of CD8(+) T cells to persistent antigen. Nat Immunol (2013) 14(11):1173–82. doi: 10.1038/ni.2714
188. Lee JH, Elly C, Park Y, Liu Y-C. E3 Ubiquitin Ligase VHL Regulates Hypoxia-Inducible Factor-1α to Maintain Regulatory T Cell Stability and Suppressive Capacity. Immunity (2015) 42(6):1062–74. doi: 10.1016/j.immuni.2015.05.016
189. Westendorf AM, Skibbe K, Adamczyk A, Buer J, Geffers R, Hansen W, et al. Hypoxia Enhances Immunosuppression by Inhibiting CD4+ Effector T Cell Function and Promoting Treg Activity. Cell Physiol Biochem (2017) 41(4):1271–84. doi: 10.1159/000464429
190. Dang EV, Barbi J, Yang H-Y, Jinasena D, Yu H, Zheng Y, et al. Control of T(H)17/T(reg) balance by hypoxia-inducible factor 1. Cell (2011) 146(5):772–84. doi: 10.1016/j.cell.2011.07.033
191. Shi LZ, Wang R, Huang G, Vogel P, Neale G, Green DR, et al. HIF1alpha-dependent glycolytic pathway orchestrates a metabolic checkpoint for the differentiation of TH17 and Treg cells. J Exp Med (2011) 208(7):1367–76. doi: 10.1084/jem.20110278
192. Law BMP, Wilkinson R, Wang X, Kildey K, Giuliani K, Beagley KW, et al. Human Tissue-Resident Mucosal-Associated Invariant T (MAIT) Cells in Renal Fibrosis and CKD. J Am Soc Nephrol (2019) 30(7):1322–35. doi: 10.1681/ASN.2018101064
193. Pattison LA, Callejo G, St John Smith E. Evolution of acid nociception: ion channels and receptors for detecting acid. Philos Trans R Soc Lond B (2019) 374(1785):20190291. doi: 10.1098/rstb.2019.0291
194. Ishii S, Kihara Y, Shimizu T. Identification of T cell death-associated gene 8 (TDAG8) as a novel acid sensing g-protein-coupled receptor. J Biol Chem (2005) 280(10):9083–7. doi: 10.1074/jbc.M407832200
195. Ihara Y, Kihara Y, Hamano F, Yanagida K, Morishita Y, Kunita A, et al. The G protein-coupled receptor T-cell death-associated gene 8 (TDAG8) facilitates tumor development by serving as an extracellular pH sensor. Proc Natl Acad Sci USA (2010) 107(40):17309–14. doi: 10.1073/pnas.1001165107
196. Ciofani M, Madar A, Galan C, Sellars M, Mace K, Pauli F, et al. A validated regulatory network for Th17 cell specification. Cell (2012) 151(2):289–303. doi: 10.1016/j.cell.2012.09.016
197. Gaublomme JT, Yosef N, Lee Y, Gertner RS, Yang LV, Wu C, et al. Single-Cell Genomics Unveils Critical Regulators of Th17 Cell Pathogenicity. Cell (2015) 163(6):1400–12. doi: 10.1016/j.cell.2015.11.009
198. Wirasinha RC, Vijayan D, Smith NJ, Parnell GP, Swarbrick A, Brink R, et al. GPR65 inhibits experimental autoimmune encephalomyelitis through CD4+ T cell independent mechanisms that include effects on iNKT cells. Immunol Cell Biol (2018) 96(2):128–36. doi: 10.1111/imcb.1031
199. Haas R, Smith J, Rocher-Ros V, Nadkarni S, Montero-Melendez T, D’Acquisto F, et al. Lactate Regulates Metabolic and Pro-inflammatory Circuits in Control of T Cell Migration and Effector Functions. PloS Biol (2015) 13(7):e1002202. doi: 10.1371/journal.pbio.1002202
200. Pucino V, Certo M, Bulusu V, Cucchi D, Goldmann K, Pontarini E, et al. Lactate Buildup at the Site of Chronic Inflammation Promotes Disease by Inducing CD4+ T Cell Metabolic Rewiring. Cell Metab (2019) 30(6):1055–8. doi: 10.1016/j.cmet.2019.10.004
201. Pucino V, Bombardieri M, Pitzalis C, Mauro C. Lactate at the crossroads of metabolism, inflammation, and autoimmunity. Eur J Immunol (2017) 47(1):14–21. doi: 10.1002/eji.201646477
202. Kumar A, Pyaram K, Yarosz EL, Hong H, Lyssiotis CA, Giri S, et al. Enhanced oxidative phosphorylation in NKT cells is essential for their survival and function. Proc Natl Acad Sci USA (2019) 116(15):7439–48. doi: 10.1073/pnas.1901376116
203. Shime H, Yabu M, Akazawa T, Kodama K, Matsumoto M, Seya T, et al. Tumor-secreted lactic acid promotes IL-23/IL-17 proinflammatory pathway. J Immunol (2008) 180(11):7175–83. doi: 10.4049/jimmunol.180.11.7175
204. Witkin SS, Alvi S, Bongiovanni AM, Linhares IM, Ledger WJ. Lactic acid stimulates interleukin-23 production by peripheral blood mononuclear cells exposed to bacterial lipopolysaccharide. FEMS Immunol Med Microbiol (2011) 61(2):153–8. doi: 10.1111/j.1574-695X.2010.00757.x
205. Hanson DF. Fever and the immune response. The effects of physiological temperatures on primary murine splenic T-cell responses in vitro. J Immunol J Immunol (1993) 151(1):436–48.
206. Smith JB, Knowlton RP, Agarwal SS. Human lymphocyte responses are enhanced by culture at 40 degrees C. J Immunol (1978) 121(2):691–4.
207. Zynda ER, Grimm MJ, Yuan M, Zhong L, Mace TA, Capitano M, et al. A role for the thermal environment in defining co-stimulation requirements for CD4(+) T cell activation. Cell Cycle (2015) 14(14):2340–54. doi: 10.1080/15384101.2015.1049782
208. Mace TA, Zhong L, Kilpatrick C, Zynda E, Lee C-T, Capitano M, et al. Differentiation of CD8+ T cells into effector cells is enhanced by physiological range hyperthermia. J Leukocyte Biol (2011) 90(5):951–62. doi: 10.1189/jlb.0511229
209. Wang X, Ni L, Wan S, Zhao X, Ding X, Dejean A, et al. Febrile Temperature Critically Controls the Differentiation and Pathogenicity of T Helper 17 Cells. Immunity (2020) 52(2):328–41. doi: 10.1016/j.immuni.2020.01.006
210. Wang H, D’Souza C, Lim XY, Kostenko L, Pediongco TJ, Eckle SBG, et al. MAIT cells protect against pulmonary Legionella longbeachae infection. Nat Commun (2018) 9(1):3350. doi: 10.1038/s41467-018-05202-8
211. Wilgenburg BV, Loh L, Chen Z, Pediongco TJ, Wang H, Shi M, et al. MAIT cells contribute to protection against lethal influenza infection in vivo. Nat Commun (2018) 9(1):4706. doi: 10.1038/s41467-018-07207-9
212. Hassane M, Jouan Y, Creusat F, Soulard D, Boisseau C, Gonzalez L, et al. Interleukin-7 protects against bacterial respiratory infection by promoting IL-17A-producing innate T-cell response. Mucosal Immunol (2020) 13(1):128–39. doi: 10.1038/s41385-019-0212-y
213. Eil R, Vodnala SK, Clever D, Klebanoff CA, Sukumar M, Pan JH, et al. Ionic immune suppression within the tumour microenvironment limits T cell effector function. Nature (2016) 537(7621):539–43. doi: 10.1038/nature19364
214. Kopp C, Linz P, Dahlmann A, Hammon M, Jantsch J, Müller DN, et al. 23Na magnetic resonance imaging-determined tissue sodium in healthy subjects and hypertensive patients. Hypertension (2013) Mar61(3):635–40. doi: 10.1161/HYPERTENSIONAHA.111.00566
215. Wu C, Yosef N, Thalhamer T, Zhu C, Xiao S, Kishi Y, et al. Induction of pathogenic TH17 cells by inducible salt-sensing kinase SGK1. Nature (2013) 496(7446):513–7. doi: 10.1038/nature11984
216. Kleinewietfeld M, Manzel A, Titze J, Kvakan H, Yosef N, Linker RA, et al. Sodium chloride drives autoimmune disease by the induction of pathogenic TH17 cells. Nature (2013) 496(7446):518–22. doi: 10.1038/nature11868
217. Hernandez AL, Kitz A, Wu C, Lowther DE, Rodriguez DM, Vudattu N, et al. Sodium chloride inhibits the suppressive function of FOXP3+ regulatory T cells. J Clin Invest (2015) 125(11):4212–22. doi: 10.1172/JCI81151
218. Yang YH, Istomine R, Alvarez F, Al-Aubodah T-A, Shi XQ, Takano T, et al. Salt Sensing by Serum/Glucocorticoid-Regulated Kinase 1 Promotes Th17-like Inflammatory Adaptation of Foxp3+ Regulatory T Cells. Cell Rep (2020) 30(5):1515–1529.e4. doi: 10.1016/j.celrep.2020.01.002
219. Matthias J, Maul J, Noster R, Meinl H, Chao Y-Y, Gerstenberg H, et al. Sodium chloride is an ionic checkpoint for human TH2 cells and shapes the atopic skin microenvironment. Sci Transl Med (2019) 11(480):eaau0683. doi: 10.1126/scitranslmed.aau0683
220. Ip WKE, Medzhitov R. Macrophages monitor tissue osmolarity and induce inflammatory response through NLRP3 and NLRC4 inflammasome activation. Nat Commun (2015) 6(1):6931. doi: 10.1038/ncomms7931
221. Yi B, Titze J, Rykova M, Feuerecker M, Vassilieva G, Nichiporuk I, et al. Effects of dietary salt levels on monocytic cells and immune responses in healthy human subjects: a longitudinal study. Transl Res (2015) 166(1):103–10. doi: 10.1016/j.trsl.2014.11.007
222. Cardoso V, Chesné J, Ribeiro H, García-Cassani B, Carvalho T, Bouchery T, et al. Neuronal regulation of type 2 innate lymphoid cells via neuromedin U. Nature (2017) 549(7671):277–81. doi: 10.1038/nature23469
223. Klose CSN, Mahlakoiv T, Moeller JB, Rankin LC, Flamar A-L, Kabata H, et al. The neuropeptide neuromedin U stimulates innate lymphoid cells and type 2 inflammation. Nature (2017) 549(7671):282–6. doi: 10.1038/nature23676
224. Talbot J, Hahn P, Kroehling L, Nguyen H, Li D, Littman DR. Feeding-dependent VIP neuron-ILC3 circuit regulates the intestinal barrier. Nature (2020) 579(7800):575–80. doi: 10.1038/s41586-020-2039-9
225. Seillet C, Luong K, Tellier J, Jacquelot N, Shen RD, Hickey P, et al. The neuropeptide VIP confers anticipatory mucosal immunity by regulating ILC3 activity. Nat Immunol (2020) 21(2):168–77. doi: 10.1038/s41590-019-0567-y
226. Wieduwild E, Girard-Madoux MJ, Quatrini L, Laprie C, Chasson L, Rossignol R, et al. β2-adrenergic signals downregulate the innate immune response and reduce host resistance to viral infection. J Exp Med (2020) 217(4):89. doi: 10.1084/jem.20190554
227. Jarret A, Jackson R, Duizer C, Healy ME, Zhao J, Rone JM, et al. Enteric Nervous System-Derived IL-18 Orchestrates Mucosal Barrier Immunity. Cell (2020) 180(1):50–63. doi: 10.1016/j.cell.2019.12.016
228. Riol-Blanco L, Ordovas-Montanes J, Perro M, Naval E, Thiriot A, Alvarez D, et al. Nociceptive sensory neurons drive interleukin-23-mediated psoriasiform skin inflammation. Nature (2014) 510(7503):157–61. doi: 10.1038/nature13199
229. DiToro D, Harbour SN, Bando JK, Benavides G, Witte S, Laufer VA, et al. Insulin-Like Growth Factors Are Key Regulators of T Helper 17 Regulatory T Cell Balance in Autoimmunity. Immunity (2020) 52(4):650–67. doi: 10.1016/j.immuni.2020.03.013
230. Carolan E, Tobin LM, Mangan BA, Corrigan M, Gaoatswe G, Byrne G, et al. Altered distribution and increased IL-17 production by mucosal-associated invariant T cells in adult and childhood obesity. J Immunol (2015) 194(12):5775–80. doi: 10.4049/jimmunol.1402945
231. Bernard JJ, Gallo RL, Krutmann J. Photoimmunology: how ultraviolet radiation affects the immune system. Nat Rev Immunol (2019) 19(11):688–701. doi: 10.1038/s41577-019-0185-9
232. Corbett AJ, Eckle SBG, Birkinshaw RW, Liu L, Patel O, Mahony J, et al. T-cell activation by transitory neo-antigens derived from distinct microbial pathways. Nature (2014) 509(7500):361–5. doi: 10.1038/nature13160
233. Scheiermann C, Kunisaki Y, Frenette PS. Circadian control of the immune system. Nat Rev Immunol (2013) 13(3):190–8. doi: 10.1038/nri3386
234. Yu X, Rollins D, Ruhn KA, Stubblefield JJ, Green CB, Kashiwada M, et al. TH17 cell differentiation is regulated by the circadian clock. Science (2013) 342(6159):727–30. doi: 10.1126/science.1243884
235. Godinho-Silva C, Domingues RG, Rendas M, Raposo B, Ribeiro H, da Silva JA, et al. Light-entrained and brain-tuned circadian circuits regulate ILC3s and gut homeostasis. Nature (2019) 574(7777):254–8. doi: 10.1038/s41586-019-1579-3
236. Teng F, Goc J, Zhou L, Chu C, Shah MA, Eberl G, et al. A circadian clock is essential for homeostasis of group 3 innate lymphoid cells in the gut. Sci Immunol Sci Immunol (2019) 4(40):eaax1215. doi: 10.1126/sciimmunol.aax1215
237. Wang Q, Robinette ML, Billon C, Collins PL, Bando JK, Fachi JL, et al. Circadian rhythm-dependent and circadian rhythm-independent impacts of the molecular clock on type 3 innate lymphoid cells. Sci Immunol Sci Immunol (2019) 4(40):eaay7501. doi: 10.1126/sciimmunol.aay7501
Keywords: mucosal-associated invariant T cells, microenvironment, microbiome, metabolism, tissue resident cells, mucosal immunology, diet
Citation: Amini A, Pang D, Hackstein CP and Klenerman P (2020) MAIT Cells in Barrier Tissues: Lessons from Immediate Neighbors. Front. Immunol. 11:584521. doi: 10.3389/fimmu.2020.584521
Received: 17 July 2020; Accepted: 26 October 2020;
Published: 30 November 2020.
Edited by:
Edwin Leeansyah, Tsinghua-Berkeley Shenzhen Institute, ChinaReviewed by:
Megan K. L. MacLeod, University of Glasgow, United KingdomDominic Paquin Proulx, United States Military HIV Research Program, United States
Shouxiong Huang, University of Cincinnati, United States
Copyright © 2020 Amini, Pang, Hackstein and Klenerman. This is an open-access article distributed under the terms of the Creative Commons Attribution License (CC BY). The use, distribution or reproduction in other forums is permitted, provided the original author(s) and the copyright owner(s) are credited and that the original publication in this journal is cited, in accordance with accepted academic practice. No use, distribution or reproduction is permitted which does not comply with these terms.
*Correspondence: Ali Amini, YWxpLmFtaW5pQHNqYy5veC5hYy51aw==; Paul Klenerman, cGF1bC5rbGVuZXJtYW5AbWVkYXdhci5veC5hYy51aw==