- 1Infectious Diseases Research Collaboration, Kampala, Uganda
- 2Department of Infection Biology, London School of Hygiene and Tropical Medicine, London, United Kingdom
- 3Department of Medicine, University of California San Francisco, San Francisco, CA, United States
- 4School of Medicine, Makerere University, Kampala, Uganda
- 5Burnet Institute, Melbourne, VIC, Australia
- 6Central Clinical School, Monash University, Melbourne, VIC, Australia
- 7Department of Medicine, University of Melbourne, Melbourne, VIC, Australia
- 8Chan Zuckerberg Biohub, San Francisco, CA, United States
Understanding how immunity to malaria is affected by declining transmission is important to aid vaccine design and understand disease resurgence. Both IgG subclasses and avidity of antigen-specific responses are important components of an effective immune response. Using a multiplex bead array assay, we measured the total IgG, IgG subclasses, and avidity profiles of responses to 18 P. falciparum blood stage antigens in samples from 160 Ugandans collected at two time points during high malaria transmission and two time points following a dramatic reduction in transmission. Results demonstrated that, for the antigens tested, (i) the rate of decay of total IgG following infection declined with age and was driven consistently by the decrease in IgG3 and occasionally the decrease in IgG1; (ii) the proportion of IgG3 relative to IgG1 in the absence of infection increased with age; (iii) the increase in avidity index (the strength of association between the antibody and antigen) following infection was largely due to a rapid loss of non-avid compared to avid total IgG; and (iv) both avid and non-avid total IgG in the absence of infection increased with age. Further studies are required to understand the functional differences between IgG1 and IgG3 in order to determine their contribution to the longevity of protective immunity to malaria. Measuring changes in antibody avidity may be a better approach of detecting affinity maturation compared to avidity index due to the differential expansion and contraction of high and low avidity total IgG.
Introduction
IgG is an important component of immunity to malaria, with function defined by the specific recognition of antigens, and tropism of the constant region (Fc) for variant Fc receptors (1). Avidity, the sum of binding affinities between antibodies and antigenic epitopes, may reflect the functional quality of the antibody variable region (2, 3). IgG subclasses IgG1–IgG4 have differences in the Fc region that affect their affinity to variants of the Fcγ receptors, influencing their effector function, longevity, and ability to cross the placental barrier (4, 5). IgG subclass switching and affinity maturation (6, 7), are important components that dictate the quality of immunity to malaria (8–10).
Antibody levels and breadth of response to specific P. falciparum antigen targets generally diminish in the absence of re-infection, which is thought to contribute to loss of immunity (11–16). However, there are differences in the rate of decay of antibodies to different antigens (17, 18). Antibody responses to malaria are predominantly cytophilic (IgG1 and IgG3) and have been shown to mediate effector mechanisms that inhibit of parasite growth (19, 20), promote opsonic phagocytosis (21) and complement fixation (22, 23). Epidemiological studies have demonstrated associations between IgG1 and IgG3 targeting various P. falciparum antigens and different manifestations of immunity, including reductions in the risk of infection, parasite density, clinical disease, and disease severity (15, 20, 24, 25). In general, these associations were stronger for IgG3 compared to IgG1 (26–28). Furthermore, in-vitro functional assays have implicated interference by IgG2 and IgG4 in the opsonizing function of the cytophilic IgG1 and IgG3 antibodies in competition assays (29, 30).
Previous studies have shown differences in class switch bias profiles driven by different P. falciparum antigens (31–34). Other factors such as age and cumulative exposure are also thought to influence subclass switching (35). Therefore, the relative composition of the subclasses may influence the functional relevance of antibodies in antimalarial immunity.
Antibody avidity is a correlate for immune memory and protection in some infections (36–42). In malaria, studies have described an increase in affinity following resolution of clinical malaria (43), higher avidity in those with reduced risk of complicated malaria (44, 45), higher avidity in clinically immune compared to non-immune populations (43), and an association between higher avidity and reduced risk of placental malaria (10). Surprisingly, a prior study by our group demonstrated that avidity to the P. falciparum antigens AMA-1 and MSP1-19 was inversely related to transmission intensity at three sites in Uganda (46). This seemingly counterintuitive result prompted us to more measure avidity to broader array of antigens and to explicitly evaluate changes over time in individuals living in a setting of changing transmission intensity.
Few studies have combined the evaluation of IgG subclasses (IgG1-4) and avidity, and responses to only a limited number of P. falciparum antigens have been studied. We also do not fully understand the natural history of antibody waning in the absence of infection that may be important for naturally acquired immunity and its longevity. Understanding how naturally acquired immunity is maintained is important to identify populations at risk in settings with declining malaria transmission and to better inform malaria vaccine design.
In this study, we measured antibody levels to total IgG, IgG1–4 and avidity index (AI) to 18 P. falciparum blood stage antigens in the same individuals before and after decreases in malaria transmission (>90%) due to indoor residual spraying of insecticide (IRS). We compared the net changes in antibody responses during this period to gain a broader insight into how IgG subclasses and avidity index are acquired with age, and how they influence a reduction in IgG levels in the absence of infection.
Methods
Study Population
Study participants were part of a cohort in Nagongera, eastern Uganda described in detail elsewhere (47). In 2011 Nagongera had one of the highest malaria burdens in the region with an entomological inoculation rate (EIR) of 215 infectious bites per person per year (48). Malaria control interventions included the use of insecticide treated nets (ITN), malaria case management with artemisinin-based therapies and intermittent presumptive treatment during pregnancy with sulfadoxine-pyrimethamine as per the Ugandan National Malaria Control Program policy. Between December 2014 and February 2015, IRS with the carbamate bendiocarb was introduced for the first time, followed by additional rounds ~ every 6 months thereafter. This intervention led to a dramatic decrease in the burden of malaria (Figure 1).
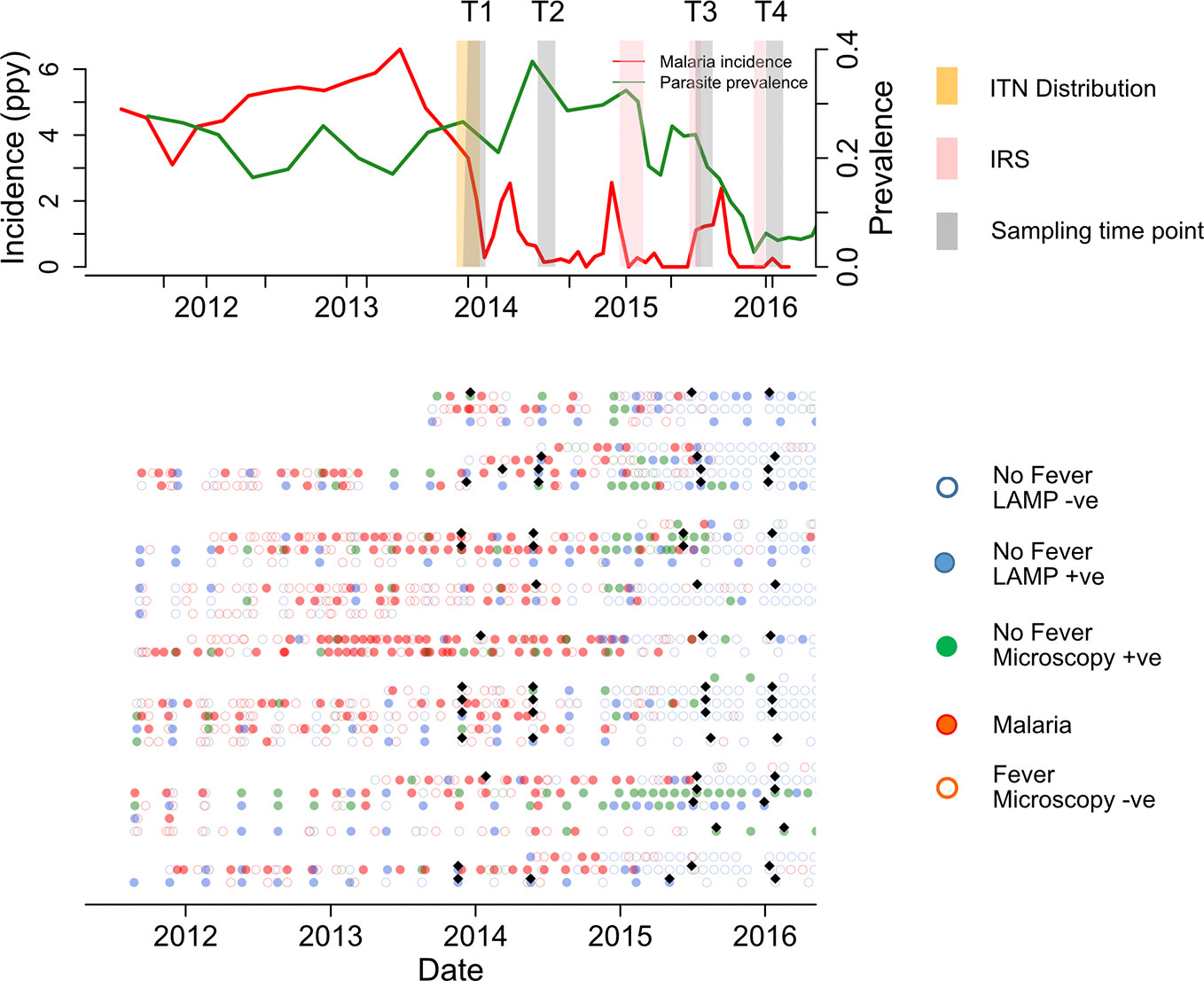
Figure 1 Summary of cohort at population and individual level. Upper panel shows malaria incidence (green line), parasite prevalence (red line), insecticide treated nets (ITN) distribution (yellow bar), and indoor residual spraying (IRS) spraying schedules (pink bars) in the full cohort. The lower panel represents the individual malaria status over 160 study sampling time points (black diamonds), at Time 1 (T1), T2, T3, and T4 (6 & 12 months before first IRS and 6 and 12 months after first IRS respectively). Participants were actively followed initially at 3 month intervals, which increased to every month in Oct 2016. Samples patent by microscopy and sub-patent by LAMP Infections are represented by green filled and blue filled circles respectively. Red filled, and red open circles represent malaria and non-malaria fever respectively. Green and blue open circles represent microscopy negative and loop mediated isothermal amplification (LAMP) negative, respectively.
Plasma samples were selected from study participants (n=160) and classified into three age groups; 1–4 (n=40), 5–11 (n=92) and >18 years (n=28). Time points were selected at 12 and 6 months pre-IRS (T1 and T2, respectively), and at 6 and 12 months post-IRS (T3 and T4, respectively). Study participants were provided with ITN at enrollment and monitored for parasite prevalence and malaria incidence (47). Malaria cases were identified via passive surveillance. P. falciparum infection was identified by active surveillance at 1–3 monthly intervals using both microscopy and LAMP (49).
Ethical Approval
Ethical approval was obtained from the Makerere University School of Medicine Research and Ethics Committee (REC REF 2011-203), the Uganda National Council for Science and Technology (HS 1074), the LSHTM ethics committee (reference # 6012), and the University of California, San Francisco Committee on Human Research (reference 027911).
Written informed consent was obtained from a parents or guardian of each child enrolled in the study and also from participating adults.
P. falciparum Antigens
A total of 18 recombinant P. falciparum blood stage antigens were assessed in addition to tetanus toxoid (TT) (National Institute of Biological Service and Control) as a non-malaria control. The P. falciparum antigens broadly fell into three categories; (i) infected red blood cell, (ii) merozoite apical organelle, and (iii) merozoite surface antigen associated proteins. The recombinant antigens were expressed in Escherichia coli either as glutathione S-transferase (GST) fusion proteins or as histidine tagged constructs (Supplementary Table 1), with the exception of AMA1 (50) which was expressed in Pichia pastoris and Rh5.1 which was expressed in HEK293 mammalian cells (51). Antigen sequences were derived from the 3D7 isolate, with the exception of MSP2 (Dd2 and CH150/9 alleles) (52), MSP1-19 (FVO) (53) and GLURP RII (F32 allele) (54).
Multiplex Bead Array Assay to Measure Total IgG and IgG1–4
Total IgG responses to 18 P. falciparum blood stage antigens and TT were assayed in plasma at 1/1,000 dilution using a multiplex bead array assay. Antigens were coupled to magnetic MagPlex microsphere beads (Luminex Corp, Austin, Texas) and assayed as previously described (55–58). Briefly, 50 µl of a pooled antigen-coupled bead suspension was added to each well of a 96-well plate, washed with PBS/Tween 20 and incubated with 50 µl of a 1/1,000 test plasma. A pool of hyperimmune Ugandan serum was used as a positive control. The plates were incubated 1.5 h at room temperature, washed (PBS/Tween 20) and goat anti-human IgG rPE labeled secondary antibody (Jackson Immuno Research Laboratories) was added and incubated for 1.5 h on a shaking platform, at room temperature. The plates were washed a final time, 1xPBS added to each well and the plates read on a MagPix machine (Luminex Corp, Austin, Texas). The results were expressed as median fluorescent intensity (MFI) and a standard curve based on the hyperimmune serum pool was included on each plate to normalize for plate to plate variations. The blank well MFI was deducted from each well to determine the net MFI.
To measure the IgG subclass responses, the total IgG assay described above was modified as follows. Test serum was used at 1/100 including a biotinylated anti-human IgG subclass (mouse anti-human IgG1: HP6069, IgG2: HP6002, IgG3: HP6050 IgG4: HP6023, Thermo fisher Scientific, UK) as the secondary antibody with a streptavidin-Phycoerythrin labeled tertiary component (Thermo fisher Scientific, UK), similar to what was previously described (59, 60). The mouse anti-human secondary antibodies were used at 1/400, 1/400, 1/1,000, and 1/400 for IgG1–4, respectively. The results were expressed as MFI was after subtracting the blank well.
Multiplex Bead Array Assay to Measure IgG Avidity Index
To measure the avidity index, the total IgG assay was modified to include a Guanidine Hydrochloride (GuHCl) step to dissociate antibodies with low avidity. Briefly, 50µl GuHCl was added to one of the duplicate wells for each sample, after the plasma incubation step. PBS was added to the second well. After washing R-Phycoerythrin-conjugated AffiniPure F (ab’) 2 Goat anti-human IgG was added and processed as per the standard MagPix assay described above. The signal detected in the well treated with GuHCl represented high avidity antibodies. Avidity index was calculated as a percentage of the high avidity antibodies of the total antibody as shown below.
Statistical Analysis
The net changes in total IgG, IgG1–4, avidity index (AI) and the avid IgG levels (the antibody remaining bound after GuHCl treatment) between the peak and lowest malaria transmission were derived from a paired difference between log10 transformed MFI and AI at T2 and T4 respectively.
Associations between antibody levels (log10 transformed MFI) for total IgG, IgG1–4, avidity index and avid IgG with 90 days since last infection were assessed using the generalized estimation equation (GEE) to allow for repeated measures per individual including all 4 time points. Infections were defined by microscopy or loop mediated isothermal amplification (LAMP) for the smear negative slides.
Associations between total IgG, IgG1–4, avidity index and avidity index with age was assessed using a GEE model, adjusted for days since infection. Age groups 1–4 years was as the reference and compared to age groups 5–11 and 18 years.
Median (and interquartile range) differences for total IgG, IgG1–4, AI and avid IgG levels between age categories where compared in a one-way ANOVA, not assuming normal distribution (using nonparametric, Kruskal-Wallis test) with pairwise comparisons between all age groups.
Analysis were performed and figures generated using Stata version 14 (StataCorp LLC, USA), GraphPad Prism version 7 (Graphpad Software Inc, USA) and R-studio (RStudio, Inc, USA).
Results
Recombinant Protein Panel
The antigen panel used in the study was comprised of previously validated markers of seroincidence (34, 61, 62). All protein constructs were based on single alleles with the exception of MSP2 which was represented by two of the dimorphic types (Dd2 and CH150/9).Target sequences selected were anchored within conserved or semi-conserved regions of each protein (63) with the exception of AMA1 which was based on the full-length sequence with N- and C-terminal truncations (50) (Supplementary Table S1).
Decline in IgG Levels Against P. falciparum Blood Stage Antigens With Decreasing Transmission
IgG responses were detected to all 18 P. falciparum antigens evaluated, with median responses higher for IgG3 compared to IgG1 to all antigens except HSP40 ag1 and MSP1-19, for which responses were similar, and to AMA-1 and Rh2_2030 which were higher for IgG1 compared to IgG3 (Supplementary Figure 1). To determine if the reduction in transmission in Nagongera was associated with antibody responses, we assessed the difference in antibody levels between T2 (6 months pre-IRS; peak transmission) and T4 (12 months post IRS; lowest transmission) (Figure 2 and Table 1). IgG levels (Log10MFI) were reduced for most of the P. falciparum antigens between the times of peak malaria transmission (T2) and lowest transmission (T4) (Figure 2A). A similar reduction was observed for almost all IgG3 responses, but this was not seen for IgG1, 2, or 4 responses. The reductions in the total IgG and IgG3 responses were similar for all the antigens tested. The largest reduction in total IgG was observed for Etramp5 ag1 and the smallest for AMA-1. The largest reduction in IgG3 was also observed for Etramp5 ag1 and the smallest for Rh5.1. IgG responses to tetanus toxoid, a non-malaria antigen control, did not change markedly between the two time points, consistent with the notion that the observed decreases were malaria specific. Taken together, these results showed that median total IgG levels declined dramatically between T2 and T4, mostly driven by reductions in IgG3 levels.
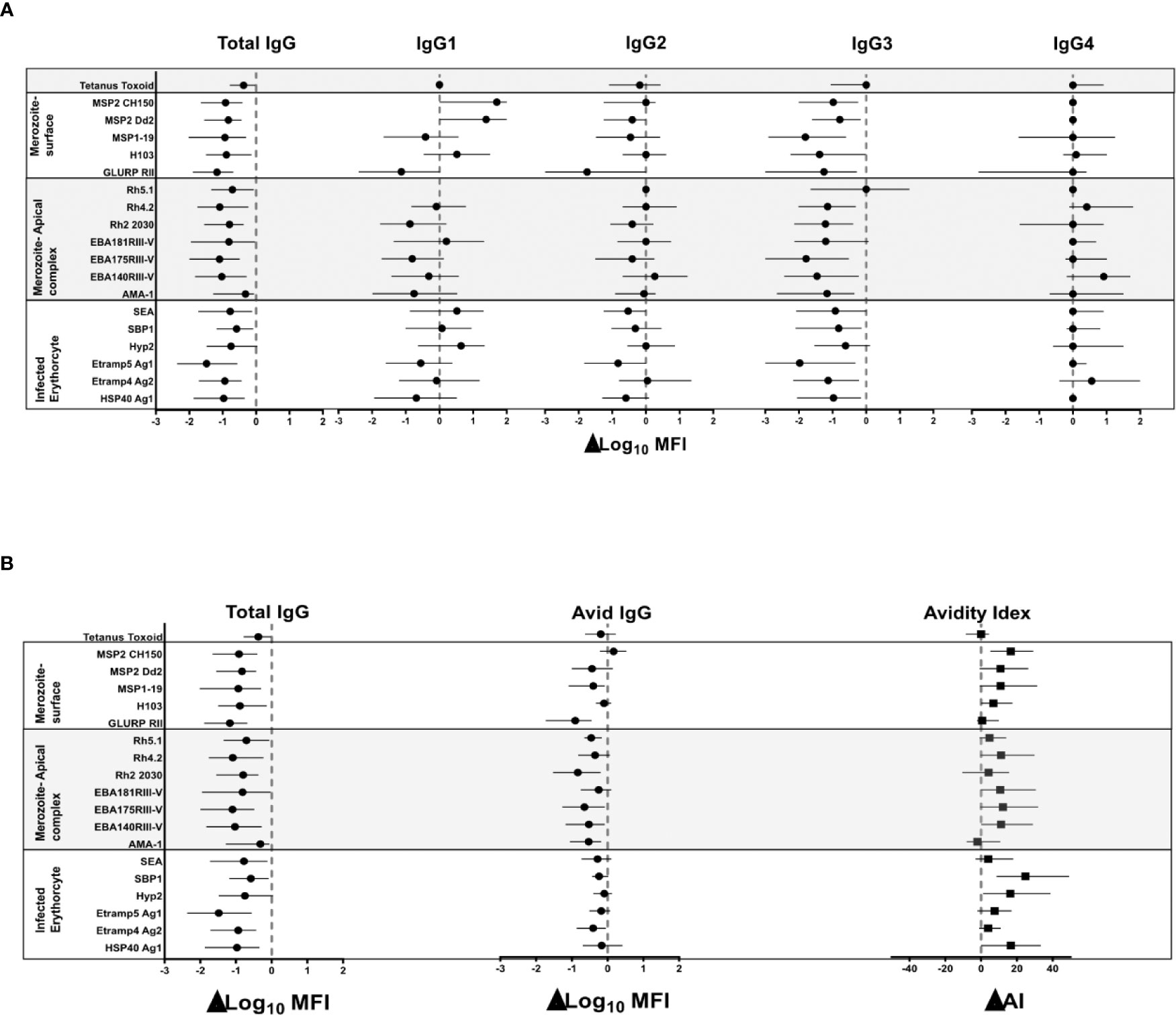
Figure 2 Net change in the median (IQR) of log10 MFI of total IgG, IgG1 – 4, avid IgG and avidity index between peak malaria transmission at T2 and near zero transmission at T4. (A) Total IgG and IgG1, IgG2, IgG3 and IgG4 (B) Total IgG and avid IgG and Avidity index. The broken red line denotes zero; no net change in responses. The black dot represents the median and the lines on either side the interquartile ranges.
Increase in Antibody Avidity Index to the P. falciparum Blood Stage Antigens With Decreasing Transmission
To determine if the reduction in malaria transmission was associated with antibody avidity, we measured differences in the avidity index and the avid total IgG (i.e. antibodies remaining after the GuHCl antibody dissociation step), between T2 and T4. The median avidity index increased for most of the P. falciparum antigens between T2 and T4, with the exception of GLURP RII, AMA-1, Rh5.1, and Etramp5 ag1 (Figure 2B). The largest net increase in avidity index was observed for antigens SBP1 and HSP40 ag1. The high avidity antibodies showed a minor decrease in the antibody responses for most antigens (Figure 2B). For GLURP RII, Rh2_2030, EBA181 RIII-V, EBA175 RIII-V, EBA140 RIII-V and AMA-1, the magnitude of the decrease in the high avidity antibodies was less than that of total IgG. There were no consistent patterns across the different categories of the P. falciparum antigens. Avidity index and avid pool to tetanus toxoid did not change between T2 and T4, as expected, again indicating that the observations were specific for P. falciparum. Since the difference between total IgG and the avid pool was an indirect measure of low avidity antibodies, these results indicated that increased avidity index was driven by a preferential loss of the pool of antibodies with lower avidity following marked reduction in transmission.
The Decline in Total IgG Responses in the Absence of P. falciparum Infection Was Driven by Preferential Decay of IgG3
We hypothesized that population level changes in antibody responses associated with a decrease in malaria transmission were due to a waning of antibodies in the absence of blood stage infection. To test this hypothesis and measure the rate of change, we took advantage of frequent active and continuous passive surveillance for P. falciparum infection to relate antibodies at all 4 time points (T1–T4) to the time since an individual’s last infection. Waning of antibodies was estimated using log-linear regression, i.e. assuming an exponential rate of decay and accounting for repeated measures using generalized estimating equations (GEE). Total IgG responses decreased following infection for all 18 P. falciparum antigens (Figure 3A). A significant decrease following infection was observed in IgG3 for all antigens (p=<0.0001) except Rh5.1 (p=ns). IgG1 responses were more heterogeneous, showing mostly non-significant decreased, unchanged, or increased trends following infection (Figure 3A). IgG2 responses showed little or no change following infection (Supplementary Table 2). IgG4 responses showed minimal reduction for all antigens except GLURP RII (p=0.001) (Supplementary Table S2). There was no change in the tetanus toxoid responses following P. falciparum infection, confirming that these results were malaria specific. Taken together, the results demonstrated that waning total IgG responses following infection were consistently driven by decreases in IgG3 and occasionally by decreases in IgG1 responses (Supplementary Table S2).
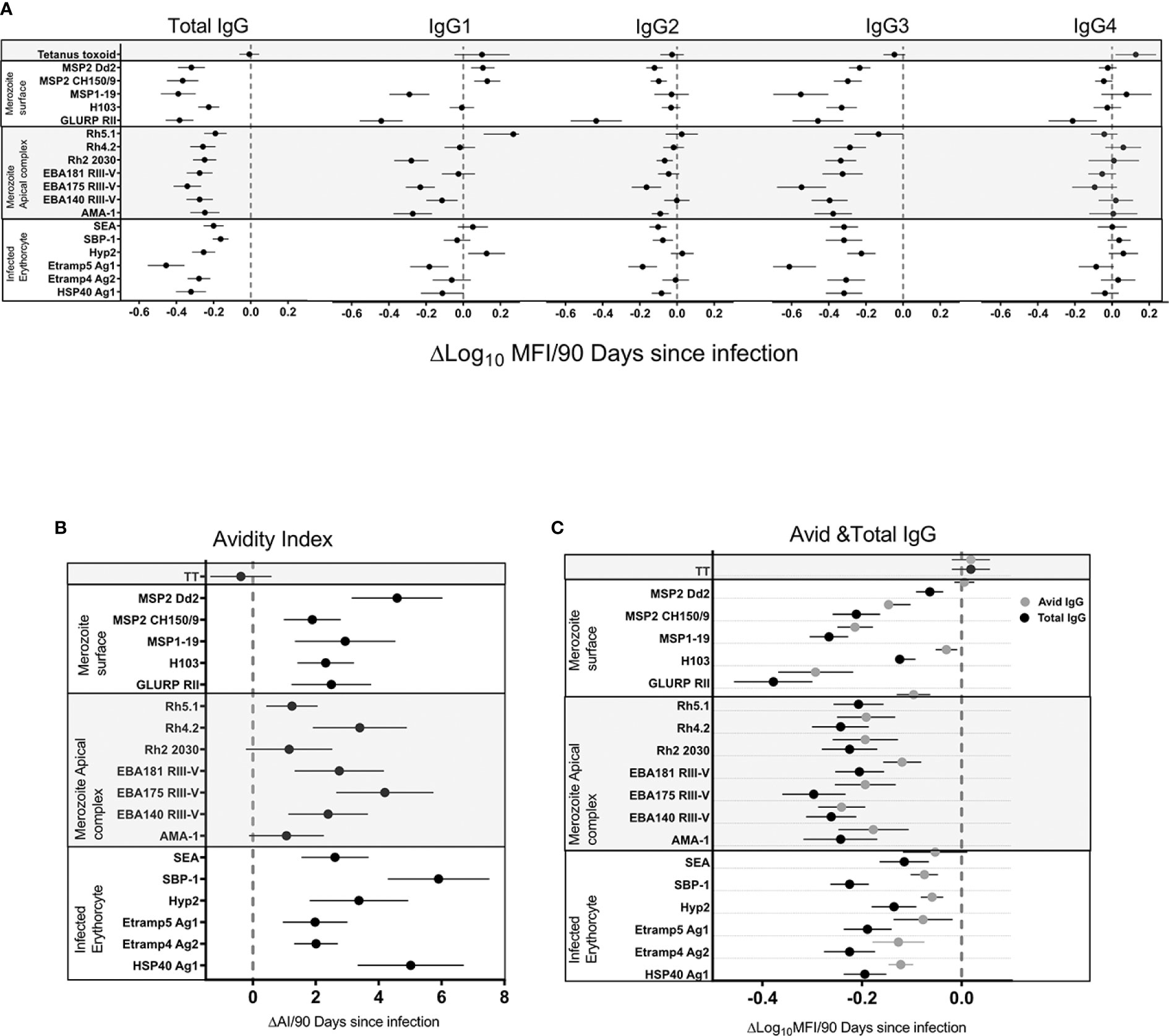
Figure 3 Association of total IgG, IgG1-4 (A), avidity index (B), avid, and total IgG (C) 90 days following infection. The black filled circle represents the mean with error bars showing 95% confidence interval of the regression coefficient. Right of the zero red line is an increase and left is a decrease 90 days following infection. Overlap of the zero red line is a non-significant association.
Increased P. falciparum Specific Antibody Avidity Index Following Infection Was Due to a Preferential Loss of Low Avidity Antibodies
We hypothesized that the population level increase in antibody avidity index following a decrease in malaria transmission was due to the differential waning of total verses high avidity antibodies in the absence of blood stage infection. To test this hypothesis and measure the rate of decline in total verses avid antibodies, and the rate of change in the avidity index, we related antibodies and avidity index at all four time points (T1–T4) to the duration of time since an individual’s last infection. Changes were estimated using log-linear or linear regression for antibody levels and avidity index respectively, i.e. assuming an exponential rate of change. The IgG avidity index increased significantly (p=<0.0001) following infection for all antigens except AMA-1 (Confidence Interval (CI) −0.001–0.025), Rh2_2030 (CI -0.002-0.028) and Rh5.1 (CI 0.004-0.022) (Figure 3B) (p=ns). The largest increase in avidity index following infection was seen for HSP40 ag1 (p=<0.0001, CI 0.037–0.074), SBP1 (p=<0.0001, CI 0.017–0.083) and MSP2_Dd2 (p=0.0001, CI 0.034–0.066) (Supplementary Table S3). There was no noticeable trend associated with the antigen categories of merozoite surface, merozoite apical complex and infected erythrocytes. As expected, the avidity index of IgG against tetanus toxoid did not change following P. falciparum infection, again confirming the specificity of the responses to the malarial antigens. In order to determine the driver of the increased avidity index in the absence of infection, the rate of total IgG decay was compared to avid IgG. The rate of decay following infection was higher in total compared to the avid IgG pool for all 18 antigens (Figure 3C). Because the avid IgG pool is a component of total IgG, the relative difference in the rate of decay following infection can be attributed to the non-avid pool. Thus, a preferential decay of non-avid antibodies is a likely explanation for the observed increase in avidity index.
The Rate of Antibody Decay Following Infection Declined With Age
Total IgG levels decayed faster in the younger (1–4 and 5–11 years) verses older (>18 years) age groups for the majority of the antigens (Figures 4A, C, and Supplementary Figure 2). The relative difference in the decay slopes was more pronounced in the first 200 days following infection, and in IgG3 compared to IgG1. There was little change in the rate of decay with age for IgG2 and 4 for most antigens (Figures 4A, C, and Supplementary Figure 2). The most pronounced changes across age category were observed for AMA-1, EBA175 RIII-V, EBA181 RIII-V, GLURP RII MSP2_Dd2, and MSP2_CH150/9. By contrast Etramp4 ag2, Etramp5 ag1 and HSP40 ag1 showed minimal changes across age. IgG1 and IgG3 had similar trends across the age categories for all the malaria antigens, with IgG3 showing more pronounced changes. The differences between ages were at maximum around 200 days following infection for total IgG, IgG1, and IgG3, driven by a rapid loss of antibodies associated with days since last infection in the 1–4 year age category compared to the older ages (5–11 and >18 years).
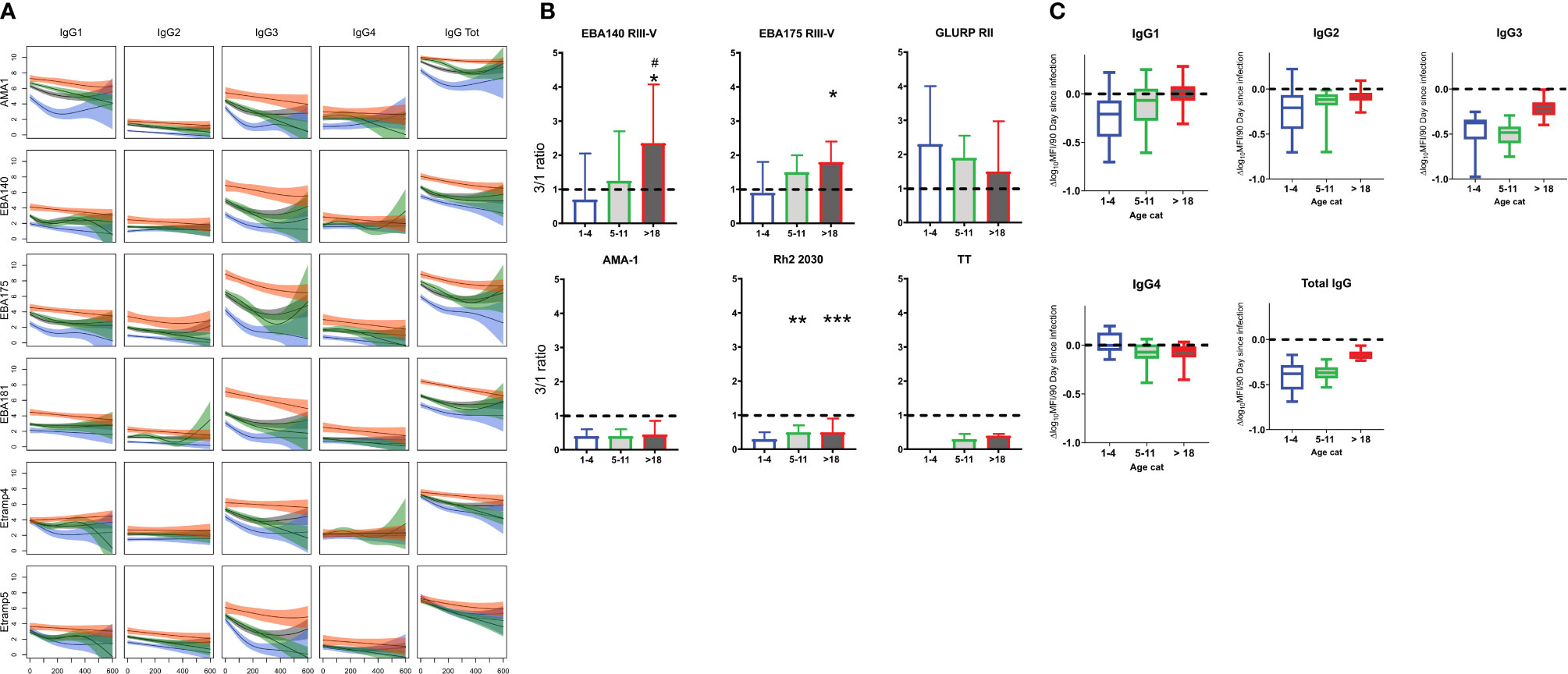
Figure 4 Changes in total IgG and subclasses 1–4 with age. (A) Representative plots of mean Log10 MFI against 90 days since last Infection, modeled using generalized additive models (GAMS), shaded areas represent 95% confidence interval. (B) Changes in IgG 3/1ratio with age. (C) Average rate (of 18 antigen) of decay following infection by age. Blue =1–4 years (N= 40), Green = 5–11 (N= 92) and Red = >18 years (N= 28). Gray = average (N=160) Ns, not significant, * = p= 0.05 – 0.01, **p = 0.009 – 0.0001 ***p > 0.00001.
To determine changes in the relative proportion of IgG1 and IgG3 with age, a ratio of Log10MFI IgG3 to IgG1 was determined and compared across the three age categories at T4 (to represent the antibody profile in the relative absence of recent infection). A ratio below 1 implied a higher proportion of IgG1 and a ratio above 1 a higher proportion of IgG3. For the 1–4 year age category the median IgG3/IgG1 ratio was below 1 for all antigens except for GLURP RII (Figure 4B and Supplementary Figure 3). Median IgG3/IgG1 ratio increased for ages 5–11 and >18 years, approaching or exceeding 1 for most antigens except for AMA-1, Rh2_2030, HSP40 ag1 and Rh5.1, for which the median ratio remained below 1 in all age categories. By contrast, the IgG3/IgG1 ratio of tetanus toxoid remained below 1 for all age categories.
High Avidity Antibodies Increased but the Avidity Index Decreased or Remained Unchanged With Age
There was no significant association between age and avidity index for most of the antigens, except for Etramp4 ag2 (1–4 vs 5–11, p=0.007, 5–11 vs >18, p =0.012), EBA181 RIII-V (1–4 vs >18, p=0.006, 5–11 vs >18, p =0.013), MSP2 Dd2 (1–4 vs >18, p<0.0001, 5–11 vs >18, p<0.0001), and AMA-1(1–4 vs 5–11, p=0.0004), for which avidity index was higher for age categories 1–4 compared with 5–11 and with >18 years (Figure 5). By contrast, there was significant increase in high avidity antibodies across all age categories for all antigens except H103, particularly between age categories 5–11 and >18 years. There was a significant decrease in avidity index for TT between age categories 1–4 and 5–11 years, and an increase in the >18year age category. A similar pattern was observed for the high avidity antibodies. The restoration of both avidity index and high avidity antibodies may be attributed to a possible TT vaccination boost.
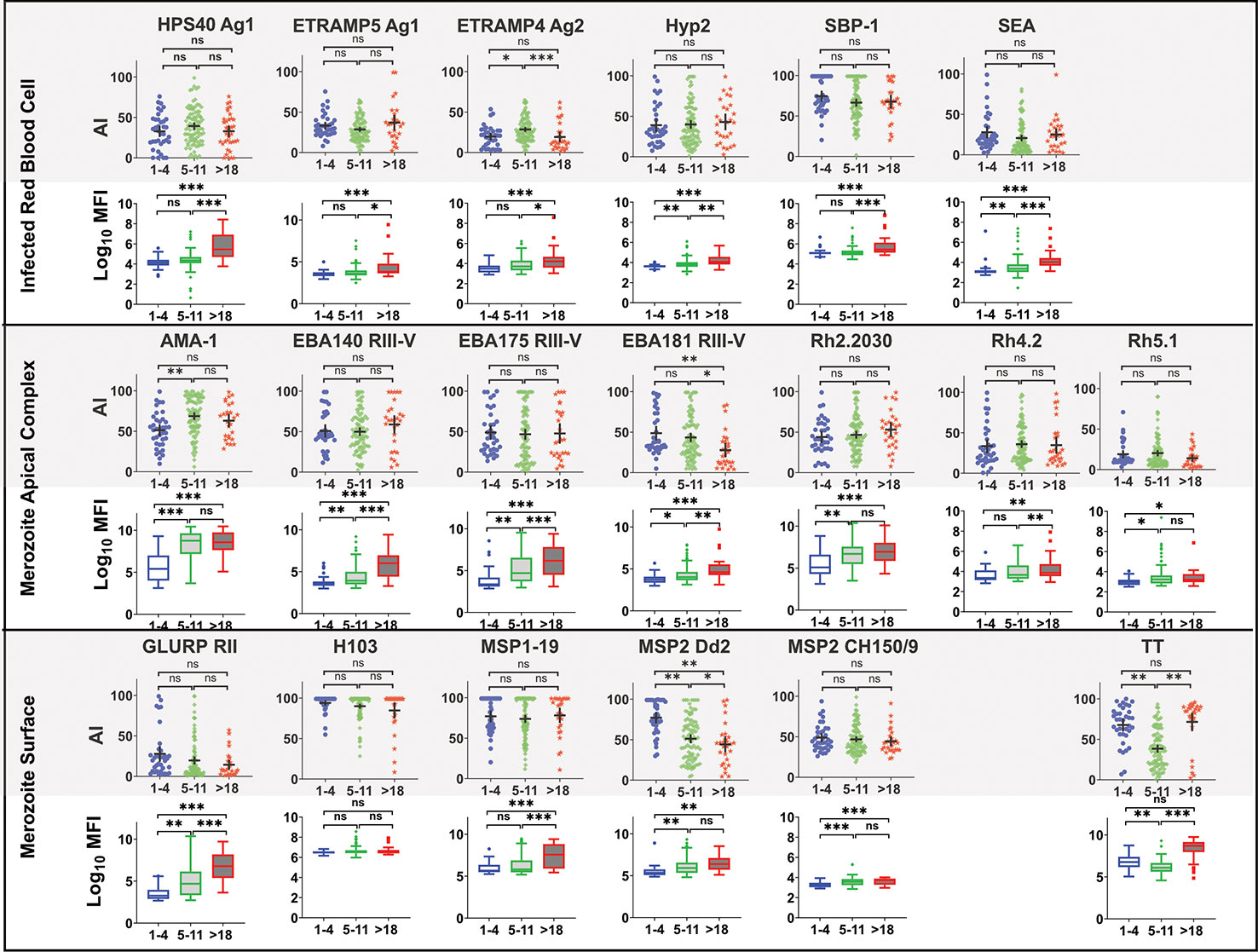
Figure 5 Comparison of Avidity index and high avidity antibodies across age category at T4. High avidity antibodies that remained binding after treatment with 2M GuHCl. Avidity index is the percentage of avid antibody of the total. Antibody was measure in a multiplex bead assay including 18 P. falciparum blood stage antigens and tetanus toxoid (TT). Age categories at T4 include 1–4 years (N= 40), 5–11 (N= 92) and >18 years (N= 28). Ns, not significant, * = p= 0.05 – 0.01, ** p = 0.009 – 0.0001 *** p>0.00001.
Discussion
We measured responses to a panel of 18 P. falciparum blood stage antigens to gain broad insight into the dynamics of antibody levels and avidity when malaria transmission is reduced.
The majority of the targets used were based on the 3D7 allelic sequence, all the targets were based largely on conserved or semi-conserved targets. All of which had been previously evaluated as important markers of prior exposure to infection (61, 63). The exceptions to this were MSP2, AMA1, and GLURP RII. MSP2 falls into two dimorphic types (Type A and B) represented here by the CH150/9 (type A) and Dd2 (type B) (52). Despite the level of repetition within the MSP2 proteins studies have used a single allele of MSP2 in combination with other recombinant targets to describe immunity to infection (64), or have opted for representative sequences from both major dimorphic types as has been done for this study. Lastly, the MSP2-C1 blood stage vaccine is also based on a single representative type from each of the dimorphic classifications (3D7 and Fc27) (65, 66). Although the importance of polymorphism in AMA1 has been demonstrated in protective immunity (67–69) in terms of measuring exposure to infection extensive studies have demonstrated that a single allele was sufficient to adequately capture exposure to infection (62, 70, 71), including in the establishment of a WHO reference reagent for anti-malaria human serum (72). In addition, Greenhouse and colleagues previously demonstrated a highly correlative antibody response between the 3D7 and FVO alleles of AMA1 (73). With MSP1-19, there is a high degree of conservation within P.falciparum (74). Further to this, Hill et al demonstrated strain transcending immunity between 15 different parasite strains, including 3D7, in antibody based opsonization assays (75). Finally, although the GLURP RII antigen is anchored within the carboxy terminal repeat region (54) and as such there is a potential for avidity responses may vary between antigen types. However, it has been demonstrated in a number of studies to be an important marker of prior exposure to infection (56, 76).
Consistent with previous studies, we showed that total IgG increased with age (10), that it substantially declined when exposure decreased, that IgG1 and IgG3 were the dominant subclasses, and that there was antigen specific bias of dominance to these subclasses (20, 21, 25). In addition to previous findings, we showed that the decrease in total IgG was consistently driven by the decrease in IgG3 and occasionally by a decrease in IgG1. We also showed that the proportion of IgG3 relative to IgG1 maintained in the absence of infection increased with age, and that the rate of antibody decay following infection declined with age.
We validated previous findings that the antimalarial antibody avidity index increased following infection and was not consistently associated with age (39, 49). In addition to previous work (46), we showed that the increase in avidity index following infection was likely due to the more rapid loss of the low avidity vs. high avidity antibodies.
Differences in individual antibody avidity is likely to be on a continuous spectrum. Use of GuHCl at one concentration to define avidity results in dichotomization of the data. Therefore, though useful, the designation of “avid” and “non-avid” is an over-simplification. We postulate that the low avidity antibodies are produced mainly by short-lived plasma cells (SLPC), and high avidity antibodies by long lived plasma cells (LLPC). Infection drives expansion of the SLPC and non-avid antibodies, and the rapid contraction of SLPCs following infection leads to rapid preferential decay of the low avidity antibodies. Therefore, the increase in avidity index we observed may indicate affinity maturation per se and/or the relative contributions of SLPCs and LLPCs following infection. A study conducted in the setting of ongoing malaria transmission and infection in Nigeria found that antibody avidity was stable among most individuals over time (77); this finding is largely consistent with our proposed model. In future studies, the direct quantification of high avidity antibodies—not performed here—may be a better indicator of affinity maturation compared to the avidity index. For example, we demonstrated that high avidity antibodies increased with age despite the lack of an increase in the avidity index with age.
The implication of early acquisition of the predominantly IgG1 response we observed is not well understood, however there is increasing evidence to suggest that IgG3 may be more protective than other subtypes against malaria. A stronger association between IgG3 and a reduced risk of malaria compared to IgG1 was reported against PfRH5 and PfRipr, (27). In addition IgG3 C1q fixing antibodies to MSP2 and EBA175 RIII-V were associated with parasite clearance following treatment (78). IgG3 has greater activity in promoting complement fixation and opsonic phagocytosis, which are mechanisms that appear to contribute to protective immunity (21, 23, 79). The observation that IgG3 decayed at a faster rate than IgG1 in the absence of infection, and was significantly slower in adults compared to infants, implies a gradual accumulation of IgG3+ LLPC with age and exposure.
The finding of a shift in dominance from IgG1 to IgG3 with age is intriguing, especially when it is considered that subclass switching generally proceeds in a single direction (IgG3, to IgG1, to IgG2 and IgG4) due to the nature of the CH domains in the Fc region (5). Therefore, memory B cells (MBC) that eventually give rise to IgG3+ LLPC cannot be the cells that switched to IgG1. Rather, naïve B cells, IgG3+ MBC, or IgM+ MBC are likely to be the source of IgG3+LLPC that gradually accumulate with age (80). Some studies have not found a major effect of age and exposure on the IgG subclass responses to antigens (33) suggesting the nature of exposure or specific epidemiological conditions may influence this effect.
IgG3 has the shortest intrinsic half-life, at 7 days, compared to ~21 days for the other subclasses (81). This implies that maintaining a large pool of IgG3 in the absence of infection requires a larger pool of LLPC compared to IgG1. We observed a slower decay or increased half-life with age of IgG1 and IgG3, but with a shift in relative proportion from predominantly IgG1 to IgG3. This may imply gradual expansion of preferentially IgG3+ LLPC with age. Previous studies have indicated a requirement of high antibody titers to maintain immunity to malaria (15). As IgG3 is the predominant IgG for most of the malaria antigens we evaluated, a larger pool of IgG3+ LLPC may be required to maintain high titers compared to IgG1 in the absence of infection. While IgG3 is suspected to be functionally more potent compared to IgG1 (82), being the dominant subclass poses a limitation of poor maintenance of protective levels in the absence of infection. This limitation poses a big challenge to malaria vaccine development, especially in endemic areas where existing memory B cells are inclined to switch to IgG3+ LLPC. A recent analysis of RTS,S vaccine responses in children found IgG3 responses had a higher decay rate than IgG1 (83). Antigens such as AMA-1 and Rh2_2030 elicited a predominantly IgG1 response across the age groups. In addition, these antigens had a relatively lower decay rate following infection compared to antigens that were predominantly IgG3. This observation implies that some P. falciparum antigens may have inherent features that influence IgG class switch bias. Therefore, we need to better understand the functional potency of IgG1 verses IgG3 and antigen features that lead to biased class switching to inform malaria vaccine designs.
Conclusions
In the setting of declining exposure to P. falciparum, IgG3 was the major driver of observed antibody decay rate and avidity index increased, likely due to preferential decay of the non-avid vs. avid antibodies. More direct quantification of avid antibodies, ascertainment of the cellular phenotype producing various antibodies over time, and functional studies of various aspects of antibody quality will be necessary to understand their protective roles in malarial immunity. This knowledge is critical for development and assessment of vaccine strategies that can improve longevity of malaria vaccine efficacy and in understanding the longevity of immunity to malaria as transmission declines.
Data Availability Statement
The original contributions presented in the study are included in the article/Supplementary Material. Further inquiries can be directed to the corresponding author.
Ethics Statement
The studies involving human participants were reviewed and approved by Makerere University School of Medicine Research and Ethics Committee (REC REF 2011-203), Uganda National Council for Science and Technology (HS 1074), LSHTM ethics committee (reference # 6012), University of California, San Francisco Committee on Human Research (reference 027911). Written informed consent to participate in this study was provided by the participants’ legal guardian/next of kin.
Author Contributions
GD, MK, PR, BG, and CD designed the surveys. JR, EA, and JN conducted the surveys. IR, BG, and HM-K contributed to data analysis and interpretation. KT and JB supplied antigen constructs. KT generated and supplied protein reagents. IS and KT designed the assays. KT, IS, BG, IR, and CD designed the study. IS wrote the first draft of the manuscript with support from KT, BG, and CD. All authors contributed to the article and approved the submitted version.
Funding
This study was supported by funds from the National Institutes of Health as part of the East African International Centers of Excellence in Malaria Research (ICMER) program (U19AI089674) and a Fogarty International Centre (FIC) Training in malaria research in Uganda award (D43TW7375). Additional support was provided by a Bloomsbury SET Award (Innovation Fellowship to KKAT; BSA14) under the UKRI Connecting Capabilities Fund (CCF).
Conflict of Interest
The authors declare that the research was conducted in the absence of any commercial or financial relationships that could be construed as a potential conflict of interest.
Supplementary Material
The Supplementary Material for this article can be found online at: https://www.frontiersin.org/articles/10.3389/fimmu.2020.576663/full#supplementary-material
Supplementary Figure 1 | Dot Plot of log10MFI of Merozoite apical complex. Antibody levels were measured using a MagPix (Luminex) multiplex assay including 18 malaria blood stage antigens and TT. Each dot represents a single sample from 160 participants at 4 time points. The horizontal bar represents the median and 95% CI.
Supplementary Figure 2 | Changes in total IgG and subclasses 1 – 4 with age. Total IgG and IgG1 – 4 for 19 P falciparum antigens and TT was measure in a multiplex bead array assay. Plots of mean Log10 MFI against days since last Infection, modeled using generalized additive models (GAMS), shaded areas represent 95% confidence interval.
Supplementary Figure 3 | Changes in total IgG3/IgG1 ratio with age. Total IgG and IgG1 – 4 for 19 P falciparum antigens and TT was measure in a multiplex bead array assay. Blue =1-4 years (N= 40), Green = 5-11 (N= 92) and Red = >18 years (N= 28). Gray = average (N=160) Ns = not significant, * = p= 0.05 – 0.01, ** p = 0.009 – 0.0001 *** p>0.0000.
Supplementary Table 1 | Summary of the P.falciparum Blood stage antigens.
Supplementary Table 2 | Generalised estimating equation (GEE) summary statistical data for Total IgG and IgG subclasses (1–4) for days since parasitemia.
Supplementary Table 3 | Generalised estimating equation (GEE) summary statistical data for the avidity index (AI) for days since parasitemia.
References
1. Wardemann H, Murugan R. From human antibody structure and function towards the design of a novel Plasmodium falciparum circumsporozoite protein malaria vaccine. Curr Opin Immunol (2018) 53:119–23. doi: 10.1016/j.coi.2018.04.023
2. Karush F. Affinity and the Immune Response*. Ann N Y Acad Sci (1970) 169(1):56–64. doi: 10.1111/j.1749-6632.1970.tb55970.x
3. Tijani MK, Reddy SB, Langer C, Beeson JG, Wahlgren M, Nwuba RI, et al. Factors influencing the induction of high affinity antibodies to Plasmodium falciparum merozoite antigens and how affinity changes over time. Sci Rep (2018) 8(1):9026–6. doi: 10.1038/s41598-018-27361-w
4. Vidarsson G, Dekkers G, Rispens T. IgG Subclasses and Allotypes: From Structure to Effector Functions. Front Immunol (2014) 5:520. doi: 10.3389/fimmu.2014.00520
5. Valenzuela NM, Schaub S. The Biology of Igg Subclasses and Their Clinical Relevance to Transplantation. Transplantation (2018) 102(1S Suppl 1):S7–13. doi: 10.1097/TP.0000000000001816
6. Gatto D, Brink R. The germinal center reaction. J Allergy Clin Immunol (2010) 126(5):898–907; quiz 908–9. doi: 10.1016/j.jaci.2010.09.007
7. DeFranco AL. The germinal center antibody response in health and disease. F1000Research (2016) 5 Suppl 1. doi: 10.12688/f1000research.7717.1
8. Cohen S, McGREGOR IA, Carrington S. Gamma-globulin and acquired immunity to human malaria. Nature (1961) 192:733–7. doi: 10.1038/192733a0
10. Tutterrow YL, Salanti A, Avril M, Smith JD, Pagano IS, Ako S, et al. High Avidity Antibodies to Full-Length VAR2CSA Correlate with Absence of Placental Malaria. PloS One (2012) 7(6):e40049. doi: 10.1371/journal.pone.0040049
11. Achtman AH, Bull PC, Stephens R, Langhorne J. Longevity of the immune response and memory to blood-stage malaria infection. Curr Top Microbiol Immunol (2005) 297:71–102. doi: 10.1007/3-540-29967-X_3
12. Kinyanjui SM, Conway DJ, Lanar DE, Marsh K. IgG antibody responses to Plasmodium falciparum merozoite antigens in Kenyan children have a short half-life. Malar J (2007) 6(1):82. doi: 10.1186/1475-2875-6-82
13. Akpogheneta OJ, Duah NO, Tetteh KKA, Dunyo S, Lanar DE, Pinder M, et al. Duration of Naturally Acquired Antibody Responses to Blood-Stage Plasmodium falciparum Is Age Dependent and Antigen Specific. Infect Immun (2008) 76(4):1748–55. doi: 10.1128/IAI.01333-07
14. Alonso PL, Sacarlal J, Aponte JJ, Leach A, Macete E, Aide P, et al. Duration of protection with RTS,S/AS02A malaria vaccine in prevention of Plasmodium falciparum disease in Mozambican children: single-blind extended follow-up of a randomised controlled trial. Lancet (2005) 366(9502):2012–8. doi: 10.1016/S0140-6736(05)67669-6
15. Osier FHA, Fegan G, Polley SD, Murungi L, Verra F, Tetteh KKA, et al. Breadth and Magnitude of Antibody Responses to Multiple Plasmodium falciparum Merozoite Antigens Are Associated with Protection from Clinical Malaria. Infect Immun (2008) 76(5):2240–8. doi: 10.1128/IAI.01585-07
16. Ferreira MU, Kimura EAS, Katzin AM, Santos-Neto LL, Ferrari JO, Villalobos JM, et al. The IgG-subclass distribution of naturally acquired antibodies to Plasmodium falciparum, in relation to malaria exposure and severity. Ann Trop Med Parasitol (1998) 92(3):245–56. doi: 10.1080/00034983.1998.11813287
17. Mugyenyi CK, Elliott SR, Yap XZ, Feng G, Boeuf P, Fegan G, et al. Declining Malaria Transmission Differentially Impacts the Maintenance of Humoral Immunity to Plasmodium falciparum in Children. J Infect Dis (2017) 216(7):887–98. doi: 10.1093/infdis/jix370
18. Fowkes FJ, McGready R, Cross NJ, Hommel M, Simpson JA, Elliott SR, et al. New Insights into Acquisition, Boosting, and Longevity of Immunity to Malaria in Pregnant Women. J Infect Dis (2012) 206(10):1612–21. doi: 10.1093/infdis/jis566
19. White MT, Bejon P, Olotu A, Griffin JT, Bojang K, Lusingu J, et al. A combined analysis of immunogenicity, antibody kinetics and vaccine efficacy from phase 2 trials of the RTS,S malaria vaccine. BMC Med (2014) 12(1):117. doi: 10.1186/s12916-014-0117-2
20. Biryukov S, Angov E, Landmesser ME, Spring MD, Ockenhouse CF, Stoute JA. Complement and Antibody-mediated Enhancement of Red Blood Cell Invasion and Growth of Malaria Parasites. EBioMedicine (2016) 9:207–16. doi: 10.1016/j.ebiom.2016.05.015
21. Osier FH, Feng G, Boyle MJ, Langer C, Zhou J, Richards JS, et al. Opsonic phagocytosis of Plasmodium falciparum merozoites: mechanism in human immunity and a correlate of protection against malaria. BMC Med (2014) 12:108. doi: 10.1186/1741-7015-12-108
22. Behet MC, Kurtovic L, van Gemert G-J, Haukes CM, Siebelink-Stoter R, Graumans W, et al. The complement system contributes to functional antibody-mediated responses induced by immunization with Plasmodium falciparum malaria sporozoites. Infect Immun (2018) 86(7):e00920–17. doi: 10.1128/IAI.00920-17
23. Boyle MJ, Reiling L, Feng G, Langer C, Osier FH, Aspeling-Jones H, et al. Human antibodies fix complement to inhibit Plasmodium falciparum invasion of erythrocytes and are associated with protection against malaria. Immunity (2015) 42(3):580–90. doi: 10.1016/j.immuni.2015.02.012
24. Cherif MK, Ouédraogo O, Sanou GS, Diarra A, Ouédraogo A, Tiono A, et al. Antibody responses to P. falciparum blood stage antigens and incidence of clinical malaria in children living in endemic area in Burkina Faso. BMC Res Notes (2017) 10(1):472. doi: 10.1186/s13104-017-2772-9
25. Saavedra-Langer R, Marapara J, Valle-Campos A, Durand S, Vásquez-Chasnamote ME, Silva H, et al. IgG subclass responses to excreted-secreted antigens of Plasmodium falciparum in a low-transmission malaria area of the Peruvian Amazon. Malar J (2018) 17(1):328. doi: 10.1186/s12936-018-2471-6
26. Dobaño C, Santano R, Vidal M, Jiménez A, Jairoce C, Ubillos I, et al. Differential Patterns of IgG Subclass Responses to Plasmodium falciparum Antigens in Relation to Malaria Protection and RTS,S Vaccination. Front Immunol (2019) 10:439. doi: 10.3389/fimmu.2019.00439
27. Weaver R, Reiling L, Feng G, Drew DR, Mueller I, Siba PM, et al. The association between naturally acquired IgG subclass specific antibodies to the PfRH5 invasion complex and protection from Plasmodium falciparum malaria. Sci Rep (2016) 086:33094. doi: 10.1038/srep33094
28. Oeuvray C, Theisen M, Rogier C, Trape JF, Jepsen S, Druilhe P. Cytophilic immunoglobulin responses to Plasmodium falciparum glutamate-rich protein are correlated with protection against clinical malaria in Dielmo, Senegal. Infect Immun (2000) 68(5):2617–20. doi: 10.1128/IAI.68.5.2617-2620.2000
29. Groux H, Gysin J. Opsonization as an effector mechanism in human protection against asexual blood stages of Plasmodium falciparum: Functional role of IgG subclasses. Res Immunol (1990) 141(5):529–42. doi: 10.1016/0923-2494(90)90021-P
30. Chaudhury S, Regules JA, Darko CA, Dutta S, Wallqvist A, Waters NC, et al. Delayed fractional dose regimen of the RTS,S/AS01 malaria vaccine candidate enhances an IgG4 response that inhibits serum opsonophagocytosis. Sci Rep (2017) 7(1):7998. doi: 10.1038/s41598-017-08526-5
31. Taylor RR, Allen SJ, Greenwood BM, Riley EM. IgG3 antibodies to Plasmodium falciparum merozoite surface protein 2 (MSP2): increasing prevalence with age and association with clinical immunity to malaria. Am J Trop Med Hyg (1998) 58(4):406–13. doi: 10.4269/ajtmh.1998.58.406
32. Metzger WG, Okenu DMN, Cavanagh DR, Robinson JV, Bojang KA, Weiss HA, et al. Serum IgG3 to the Plasmodium falciparum merozoite surface protein 2 is strongly associated with a reduced prospective risk of malaria. Parasite Immunol (2003) 25(6):307–12. doi: 10.1046/j.1365-3024.2003.00636.x
33. Stanisic DI, Richards JS, McCallum FJ, Michon P, King CL, Schoepflin S, et al. Immunoglobulin G subclass-specific responses against Plasmodium falciparum merozoite antigens are associated with control of parasitemia and protection from symptomatic illness. Infect Immun (2009) 77(3):1165–74. doi: 10.1128/IAI.01129-08
34. Richards JS, Stanisic DI, Fowkes FJI, Tavul L, Dabod E, Thompson JK, et al. Association between naturally acquired antibodies to erythrocyte-binding antigens of Plasmodium falciparum and protection from malaria and high-density parasitemia. Clin Infect Dis Off Publ Infect Dis Soc Am (2010) 51(8):e50–60. doi: 10.1086/656413
35. Tongren JE, Drakeley CJ, McDonald SLR, Reyburn HG, Manjurano A, Nkya WMM, et al. Target antigen, age, and duration of antigen exposure independently regulate immunoglobulin G subclass switching in malaria. Infect Immun (2006) 74(1):257–64. doi: 10.1128/IAI.74.1.257-264.2006
36. Hedman K, Rousseau SA. Measurement of avidity of specific IgG for verification of recent primary rubella. J Med Virol (1989) 27(4):288–92. doi: 10.1002/jmv.1890270406
37. Pour Abolghasem S, Bonyadi MR, Babaloo Z, Porhasan A, Nagili B, Gardashkhani OA, et al. IgG avidity test for the diagnosis of acute Toxoplasma gondii infection in early pregnancy. Iran J Immunol IJI (2011) 8(4):251–5.
38. Schlesinger Y, Granoff DM. Avidity and bactericidal activity of antibody elicited by different haemophilus influenzae type b conjugate vaccines. The Vaccine Study Group. JAMA (1992) 267(11):1489–94. doi: 10.1001/jama.267.11.1489
39. Cremers AJH, Lut J, Hermans PWM, Meis JF, de Jonge MI, Ferwerda G. Avidity of Antibodies against Infecting Pneumococcal Serotypes Increases with Age and Severity of Disease. Clin Vaccine Immunol (2014) 21(6):904–7. doi: 10.1128/CVI.00147-14
40. Goldblatt D, Vaz AR, Miller E. Antibody avidity as a surrogate marker of successful priming by Haemophilus influenzae type b conjugate vaccines following infant immunization. J Infect Dis (1998) 177(4):1112–5. doi: 10.1086/517407
41. Park DW, Nam M-H, Kim JY, Kim HJ, Sohn JW, Cho Y, et al. Mumps outbreak in a highly vaccinated school population: assessment of secondary vaccine failure using IgG avidity measurements. Vaccine (2007) 25(24):4665–70. doi: 10.1016/j.vaccine.2007.04.013
42. Sanz-Moreno JC, Limia-Sánchez A, García-Comas L, Mosquera-Gutiérrez MM, Echevarria-Mayo JE, Castellanos-Nadal A, et al. Detection of secondary mumps vaccine failure by means of avidity testing for specific immunoglobulin G. Vaccine (2005) 23(41):4921–5. doi: 10.1016/j.vaccine.2005.05.018
43. Ferreira MU, Kimura EA, De Souza JM, Katzin AM. The isotype composition and avidity of naturally acquired anti-Plasmodium falciparum antibodies: differential patterns in clinically immune Africans and Amazonian patients. Am J Trop Med Hyg (1996) 55(3):315–23. doi: 10.4269/ajtmh.1996.55.315
44. Leoratti FM, Durlacher RR, Lacerda MV, Alecrim MG, Ferreira AW, Sanchez MC, et al. Pattern of humoral immune response to Plasmodium falciparum blood stages in individuals presenting different clinical expressions of malaria. Malar J (2008) 7:186. doi: 10.1186/1475-2875-7-186
45. Reddy SB, Anders RF, Beeson JG, Färnert A, Kironde F, Berenzon SK, et al. High affinity antibodies to Plasmodium falciparum merozoite antigens are associated with protection from malaria. PloS One (2012) 7(2):e32242. doi: 10.1371/journal.pone.0032242
46. Ssewanyana I, Arinaitwe E, Nankabirwa JI, Yeka A, Sullivan R, Kamya MR, et al. Avidity of anti-malarial antibodies inversely related to transmission intensity at three sites in Uganda. Malar J (2017) 16(1):67. doi: 10.1186/s12936-017-1721-3
47. Kamya MR, Arinaitwe E, Wanzira H, Katureebe A, Barusya C, Kigozi SP, et al. Malaria Transmission, Infection, and Disease at Three Sites with Varied Transmission Intensity in Uganda: Implications for Malaria Control. Am J Trop Med Hyg (2015) 92(5):903–12. doi: 10.4269/ajtmh.14-0312
48. Kilama M, Smith DL, Hutchinson R, Kigozi R, Yeka A, Lavoy G, et al. Estimating the annual entomological inoculation rate for Plasmodium falciparum transmitted by Anopheles gambiae s.l. using three sampling methods in three sites in Uganda. Malar J (2014) 13:111. doi: 10.1186/1475-2875-13-111
49. Nankabirwa JI, Briggs J, Rek J, Arinaitwe E, Nayebare P, Katrak S, et al. Persistent Parasitemia Despite Dramatic Reduction in Malaria Incidence After 3 Rounds of Indoor Residual Spraying in Tororo, Uganda. J Infect Dis (2019) 219(7):1104–11. doi: 10.1093/infdis/jiy628
50. Collins CR, Withers-Martinez C, Hackett F, Blackman MJ. An Inhibitory Antibody Blocks Interactions between Components of the Malarial Invasion Machinery. PloS Pathog (2009) 5(1):e1000273. doi: 10.1371/journal.ppat.1000273
51. Jin J, Tarrant RD, Bolam EJ, Angell-Manning P, Soegaard M, Pattinson DJ, et al. Production, quality control, stability, and potency of cGMP-produced Plasmodium falciparum RH5.1 protein vaccine expressed in Drosophila S2 cells. NPJ Vaccines (2018) 3:32. doi: 10.1038/s41541-018-0071-7
52. Taylor RR, Smith DB, Robinson VJ, McBride JS, Riley EM. Human antibody response to Plasmodium falciparum merozoite surface protein 2 is serogroup specific and predominantly of the immunoglobulin G3 subclass. Infect Immun (1995) 63(11):4382–8. doi: 10.1128/IAI.63.11.4382-4388.1995
53. Burghaus PA, Holder AA. Expression of the 19-kilodalton carboxy-terminal fragment of the Plasmodium falciparum merozoite surface protein-1 in Escherichia coli as a correctly folded protein. Mol Biochem Parasitol (1994) 64(1):165–9. doi: 10.1016/0166-6851(94)90144-9
54. Theisen M, Vuust J, Gottschau A, Jepsen S, Høgh B. Antigenicity and immunogenicity of recombinant glutamate-rich protein of Plasmodium falciparum expressed in Escherichia coli. Clin Diagn Lab Immunol (1995) 2(1):30–4. doi: 10.1128/CDLI.2.1.30-34.1995
55. Wu L, Hall T, Ssewanyana I, Oulton T, Patterson C, Vasileva H, et al. Optimisation and standardisation of a multiplex immunoassay of diverse Plasmodium falciparum antigens to assess changes in malaria transmission using sero-epidemiology. Wellcome Open Res (2019) 4:26. doi: 10.12688/wellcomeopenres.14950.1
56. Kana IH, Singh SK, Garcia-Senosiain A, Dodoo D, Singh S, Adu B, et al. Breadth of functional antibodies is associated with Plasmodium falciparum merozoite phagocytosis and protection against febrile malaria. J Infect Dis (2019) 220(2):275–84. doi: 10.1093/infdis/jiz088/5367431
57. Varela ML, Mbengue B, Basse A, Loucoubar C, Vigan-Womas I, Dièye A, et al. Optimization of a magnetic bead-based assay (MAGPIX®-Luminex) for immune surveillance of exposure to malaria using multiple Plasmodium antigens and sera from different endemic settings. Malar J (2018) 17(1):324. doi: 10.1186/s12936-018-2465-4
58. Valmaseda A, Macete E, Nhabomba A, Guinovart C, Aide P, Bardají A, et al. Identifying Immune Correlates of Protection Against Plasmodium falciparum Through a Novel Approach to Account for Heterogeneity in Malaria Exposure. Clin Infect Dis (2018) 66(4):586–93. doi: 10.1093/cid/cix837
59. Cham GK, Kurtis J, Lusingu J, Theander TG, Jensen AT. Turner L. A semi-automated multiplex high-throughput assay for measuring IgG antibodies against Plasmodium falciparum erythrocyte membrane protein 1 (PfEMP1) domains in small volumes of plasma. Malar J (2008) 7(1):108. doi: 10.1186/1475-2875-7-108
60. Fernandez-Becerra C, Sanz S, Brucet M, Stanisic DI, Alves FP, Camargo EP, et al. Naturally-acquired humoral immune responses against the N- and C-termini of the Plasmodium vivax MSP1 protein in endemic regions of Brazil and Papua New Guinea using a multiplex assay. Malar J (2010) 9(1):29. doi: 10.1186/1475-2875-9-29
61. Gray JC, Corran PH, Mangia E, Gaunt MW, Li Q, Tetteh KKA, et al. Profiling the antibody immune response against blood stage malaria vaccine candidates. Clin Chem (2007) 53(7):1244–53. doi: 10.1373/clinchem.2006.081695
62. Helb DA, Tetteh KKA, Felgner PL, Skinner J, Hubbard A, Arinaitwe E, et al. Novel serologic biomarkers provide accurate estimates of recent Plasmodium falciparum exposure for individuals and communities. Proc Natl Acad Sci U S A (2015) 112(32):E4438–47. doi: 10.1073/pnas.1501705112
63. Richards JS, Arumugam TU, Reiling L, Healer J, Hodder AN, Fowkes FJI, et al. Identification and prioritization of merozoite antigens as targets of protective human immunity to Plasmodium falciparum malaria for vaccine and biomarker development. J Immunol Baltim Md (1950) 2013191(2):795–809. doi: 10.4049/jimmunol.1300778
64. Mbengue B, Fall MM, Varela M-L, Loucoubar C, Joos C, Fall B, et al. Analysis of antibody responses to selected Plasmodium falciparum merozoite surface antigens in mild and cerebral malaria and associations with clinical outcomes. Clin Exp Immunol (2019) 196(1):86–96. doi: 10.1111/cei.13254
65. McCarthy JS, Marjason J, Elliott S, Fahey P, Bang G, Malkin E, et al. A phase 1 trial of MSP2-C1, a blood-stage malaria vaccine containing 2 isoforms of MSP2 formulated with Montanide® ISA 720. PloS One (2011) 6(9):e24413. doi: 10.1371/journal.pone.0024413
66. Feng G, Boyle MJ, Cross N, Chan J-A, Reiling L, Osier F, et al. Human Immunization With a Polymorphic Malaria Vaccine Candidate Induced Antibodies to Conserved Epitopes That Promote Functional Antibodies to Multiple Parasite Strains. J Infect Dis (2018) 218(1):35–43. doi: 10.1093/infdis/jiy170
67. Ouattara A, Niangaly A, Adams M, Coulibaly D, Kone AK, Traore K, et al. Epitope-based sieve analysis of Plasmodium falciparum sequences from a FMP2.1/AS02A vaccine trial is consistent with differential vaccine efficacy against immunologically relevant AMA1 variants. Vaccine (2020) 38(35):5700–6. doi: 10.1016/j.vaccine.2020.06.035
68. Berry AA, Gottlieb ER, Kouriba B, Diarra I, Thera MA, Dutta S, et al. Immunoglobulin G subclass and antibody avidity responses in Malian children immunized with Plasmodium falciparum apical membrane antigen 1 vaccine candidate FMP2.1/AS02A. Malar J (2019) 18(1):13. doi: 10.1186/s12936-019-2637-x
69. Kamuyu G, Tuju J, Kimathi R, Mwai K, Mburu J, Kibinge N, et al. KILchip v1.0: A Novel Plasmodium falciparum Merozoite Protein Microarray to Facilitate Malaria Vaccine Candidate Prioritization. Front Immunol (2018) 9:2866. doi: 10.3389/fimmu.2018.02866
70. Assefa A, Ali Ahmed A, Deressa W, Sime H, Mohammed H, Kebede A, et al. Multiplex serology demonstrate cumulative prevalence and spatial distribution of malaria in Ethiopia. Malar J (2019) 18(1):246. doi: 10.1186/s12936-019-2874-z
71. Yman V, White MT, Asghar M, Sundling C, Sondén K, Draper SJ, et al. Antibody responses to merozoite antigens after natural Plasmodium falciparum infection: kinetics and longevity in absence of re-exposure. BMC Med (2019) 17(1):22. doi: 10.1186/s12916-019-1255-3
72. Bryan D, Silva N, Rigsby P, Dougall T, Corran P, Bowyer PW, et al. The establishment of a WHO Reference Reagent for anti-malaria (Plasmodium falciparum) human serum. Malar J (2017) 0516(1):314. doi: 10.1186/s12936-017-1958-x
73. Greenhouse B, Ho B, Hubbard A, Njama-Meya D, Narum DL, Lanar DE, et al. Antibodies to Plasmodium falciparum antigens predict a higher risk of malaria but protection from symptoms once parasitemic. J Infect Dis (2011) 204(1):19–26. doi: 10.1093/infdis/jir223
74. Miller LH, Roberts T, Shahabuddin M, McCutchan TF. Analysis of sequence diversity in the Plasmodium falciparum merozoite surface protein-1 (MSP-1). Mol Biochem Parasitol (1993) 59(1):1–14. doi: 10.1016/0166-6851(93)90002-F
75. Hill DL, Wilson DW, Sampaio NG, Eriksson EM, Ryg-Cornejo V, Harrison GLA, et al. Merozoite Antigens of Plasmodium falciparum Elicit Strain-Transcending Opsonizing Immunity. Infect Immun (2016) 84(8):2175–84. doi: 10.1128/IAI.00145-16
76. Adamou R, Dechavanne C, Sadissou I, d’Almeida T, Bouraima A, Sonon P, et al. Plasmodium falciparum merozoite surface antigen-specific cytophilic IgG and control of malaria infection in a Beninese birth cohort. Malar J (2019) 18(1):194. doi: 10.1186/s12936-019-2831-x
77. Tijani MK, Babalola OA, Odaibo AB, Anumudu CI, Asinobi AO, Morenikeji OA, et al. Acquisition, maintenance and adaptation of invasion inhibitory antibodies against Plasmodium falciparum invasion ligands involved in immune evasion. PloS One (2017) 12(8):e0182187. doi: 10.1371/journal.pone.0182187
78. O’Flaherty K, Ataíde R, Zaloumis SG, Ashley EA, Powell R, Feng G, et al. Contribution of Functional Antimalarial Immunity to Measures of Parasite Clearance in Therapeutic Efficacy Studies of Artemisinin Derivatives. J Infect Dis (2019) 220(7):1178–87. doi: 10.1093/infdis/jiz247
79. Joos C, Marrama L, Polson HEJ, Corre S, Diatta A-M, Diouf B, et al. Clinical protection from falciparum malaria correlates with neutrophil respiratory bursts induced by merozoites opsonized with human serum antibodies. PloS One (2010) 5(3):e9871. doi: 10.1371/journal.pone.0009871
80. Krishnamurty AT, Thouvenel CD, Portugal S, Keitany GJ, Kim KS, Holder A, et al. Somatically Hypermutated Plasmodium-Specific IgM(+) Memory B Cells Are Rapid, Plastic, Early Responders upon Malaria Rechallenge. Immunity (2016) 45(2):402–14. doi: 10.1016/j.immuni.2016.06.014
81. Mankarious S, Lee M, Fischer S, Pyun KH, Ochs HD, Oxelius VA, et al. The half-lives of IgG subclasses and specific antibodies in patients with primary immunodeficiency who are receiving intravenously administered immunoglobulin. J Lab Clin Med (1988) 112(5):634–40.
82. Irani V, Guy AJ, Andrew D, Beeson JG, Ramsland PA, Richards JS. Molecular properties of human IgG subclasses and their implications for designing therapeutic monoclonal antibodies against infectious diseases. Mol Immunol (2015) 67(2 Pt A):171–82. doi: 10.1016/j.molimm.2015.03.255
Keywords: antibody avidity, Plasmodium falciparum, immunity, IgG subclass antibodies, avidity index, malaria
Citation: Ssewanyana I, Rek J, Rodriguez I, Wu L, Arinaitwe E, Nankabirwa JI, Beeson JG, Mayanja-Kizza H, Rosenthal PJ, Dorsey G, Kamya MR, Drakeley C, Greenhouse B and Tetteh KKA (2021) Impact of a Rapid Decline in Malaria Transmission on Antimalarial IgG Subclasses and Avidity. Front. Immunol. 11:576663. doi: 10.3389/fimmu.2020.576663
Received: 13 July 2020; Accepted: 17 November 2020;
Published: 27 January 2021.
Edited by:
José Roberto Mineo, Federal University of Uberlandia, BrazilReviewed by:
V. Ann Stewart, Uniformed Services University of the Health Sciences, United StatesSunil Joshi, University of Miami, United States
Copyright © 2021 Ssewanyana, Rek, Rodriguez, Wu, Arinaitwe, Nankabirwa, Beeson, Mayanja-Kizza, Rosenthal, Dorsey, Kamya, Drakeley, Greenhouse and Tetteh. This is an open-access article distributed under the terms of the Creative Commons Attribution License (CC BY). The use, distribution or reproduction in other forums is permitted, provided the original author(s) and the copyright owner(s) are credited and that the original publication in this journal is cited, in accordance with accepted academic practice. No use, distribution or reproduction is permitted which does not comply with these terms.
*Correspondence: Kevin K. A. Tetteh, a2V2aW4uVGV0dGVoQGxzaHRtLmFjLnVr