- 1Heidelberg University Children's Hospital, Department of Neonatology, Heidelberg, Germany
- 2Klinik für Kinderheilkunde und Jugendmedizin, Memmingen, Germany
Newborns are highly susceptible to infections and mainly rely on innate immune functions. Reduced reactivity, delayed activation and subsequent failure to resolve inflammation however makes the neonatal immune system a very volatile line of defense. Perinatal microbiota, nutrition and different extra-uterine factors are critical elements that define long-term outcomes and shape the immune system during the neonatal period. Neutrophils are first responders and represent a vital component of the immune system in newborns. They have long been regarded as merely executive immune cells, however this notion is beginning to shift. Neutrophils are shaped by their surrounding and adaptive elements have been described. The role of “innate immune memory” and the main triangle connection microbiome—neutrophil—adaptation will be discussed in this review.
The Role of Neutrophils and the Microbiome in Newborns
Neutrophil Function in Newborns
Neutrophils are innate immune cells which primarily act as first responders to invasive infections. They are the largest subgroup of polymorphonuclear cells (PMNs) and the most abundant type of immune cell in the peripheral blood. Typically, neutrophils constitute around 50% of bloodstream leukocytes in humans, however bacterial infections may trigger an increase of up to 80% in order to eliminate the invading pathogen (1). Neutrophils are terminally differentiated cells mainly characterized by a short lifespan (around 48 h after release into the circulation) (2, 3). They are involved in the defense against bacterial, fungal as well viral infections (4, 5). As first line responder cells, neutrophils harbor an armory of antimicrobial agents including hydrolytic enzymes (i.e., defensins), pro-inflammatory mediators and reactive oxygen species (ROS) (3). They possess the unique capacity to extrude a meshwork of chromatin fibers known as neutrophil extracellular traps (NETs) aiming to eliminate invading pathogens (6). Dysregulations of neutrophil recruitment, activation or survival are closely associated with the pathogenesis of various infectious as well inflammatory diseases (5, 7).
The neonatal immune system must continuously mount immune responses against external or internal factors whilst maturing (8). This immaturity partially accounts for the high mortality from opportunistic infections and renders the first year of life the deadliest until the age of 50 (9). Neutrophils as innate immune cells appear at low counts during gestational week (GW) 8 and reach the peak of expansion around birth (8, 10). The most vulnerable population of extremely premature infants are also born with an immature innate immune system which is less functional compared to term born neonates. Leukocyte recruitment is ontogenetically regulated, whereas extremely premature infants show heavily impaired recruitment, which gradually matures up to around 35 weeks of gestation (11–14). In particular, expression of adhesion molecules of both neutrophils and the endothelium are greatly suppressed in preterm infants as reviewed in detail previously (13). Further, preterm neutrophils show a lower phagocytic capacity toward both gram (+) and gram (–) bacteria compared to both term-born infants and adults, which may be due to reduced levels of opsonization factors such as maternal immunoglobulins (15–18). Neonatal neutrophils have long been considered as primitive and “dysfunctional,” however studies indicate that they show immunological plasticity by adapting in response to environmental cues (18). One such early influence for neutrophil adaptation is the rapidly shifting composition of the perinatal microbiota.
The Microbiota and the Neonatal Immune System
Early-life events such as the mode of delivery, maternal conditions, the use of antibiotics or pre-/probiotics, diet and many others drive the maturation of the immune system, especially of innate immune cells during infancy (19–22). Such perinatal circumstances also imprint themselves on microbiome composition and can be linked to favorable or adverse immunological outcomes (23). Proving causality between perinatal events, microbiome composition and immunity is difficult. Yet, the so-called “primitive” innate immune system of newborns may make disentangling such correlations a little more feasible.
The microbiota consists of many species including bacteria, viruses, fungi and protozoa. The cell number and the size of the genome of the human microbiota greatly exceeds the host (24). This “second genome” has a profound impact on the way we react to pathogens and how the immune system differentiates between friend and foe (25, 26). The perinatal period is marked as the most dramatic shift in both immune function and microbiome composition. The microbiota rapidly colonizes the newborn and represents a first challenge to the evolving immunity (27–29). Prenatally, a low immunological profile with a tolerogenic phenotype represents a protective intrauterine feature to prevent rejection of the semi-allogenic fetus by the maternal immune system (30–32). After birth, the neonatal immune system must undergo a rapid transition from immune evasion to immune defense. The neonatal immune system learns to “tolerate” its microbiota, however abnormal colonization (dysbiosis) may challenge this adaptation of the host. For example, the mode of delivery is known to prime the neonatal immune system through alterations in microbiome composition (33). Further, vaginal birth triggers the release of many stress hormones such as cortisol and catecholamines that have profound effects on the phenotype and function of a variety of innate immune cells like neutrophils, natural killer (NK) cells and monocytes (8, 22). The excessive use of antibiotics in the neonatal period is another example of microbiome-disruption with lasting consequences and has been linked to the development of chronic diseases (34, 35). Specifically, macrolide antibiotics are known to inhibit the proper activation and recruitment of neutrophils (34, 36). These alterations in the interplay of the microbiota and immune development can significantly impact neonatal morbidity and mortality. Gut dysbiosis is associated with the development of necrotizing enterocolitis (NEC), an often-fatal inflammatory disease of the preterm gut (37). Among very low birthweight infants (<1,500 grams), who are evolutionary not built to handle a postnatal microbiome or to deal with a magnitude of pathogens, alterations in microbiome composition precede the onset of NEC (38). Distortion of intestinal microbiome composition is also discussed as a contributing factor to the development of sepsis (39, 40). Outside immune priming, gut microbiome composition has been shown to influence growth in infants and to impact long-term health trajectories as reviewed in detail by Pflughoeft and Versalovic (41, 42).
The Interplay Between Microbiota and Neutrophils
Neutrophils as an essential part of the innate immune system are affected by different microbial components or metabolites of both resident microbiota and invading pathogens. Early investigations have shown that gut microbiota may alter the production of neutrophils through modulation of myelopoiesis in the bone-marrow (43–45). Later studies revealed that microbiota depletion leads to increased susceptibility to infections in neonates presumably caused by decreased numbers of neutrophils (40, 45, 46). Microbiome-derived mediators such as IL-17, IL-7, IL-6, stem cell factor (SCF) and thrombopoietin (THPO) affect the release of granulocyte colony-stimulating factor (G-CSF) (40, 47). Interestingly, microbiome alterations occurring by a high-fat diet prevail hematopoiesis by affecting the hematopoietic niche (48, 49). Apart from affecting neutrophil production, the microbiota also regulates neutrophil function. Short-chain fatty acids (SCFAs) as metabolites derived from the gut microbiome, suppress the activation and recruitment of neutrophils (50, 51) and promote the resolution of inflammation through induction of apoptosis in neutrophils and its efferocytosis by macrophages (52, 53). Further, the gut microbiota regulates bile acid metabolism, which have been shown to impact immune cells (54). Data from our lab indicates that tauroursodeoxycholate, a bile acid with chaperoning activity, directly suppresses neutrophil activation and recruitment to inflamed tissue (manuscript under submission). Inversely, neutrophils also have the capacity to modulate the microbiota by removing unwanted species (49). Despite such individual pathways or mediators that may facilitate cross talk between the microbiota and neutrophils, no systematic analysis has been performed for newborns or specifically preterm infants. Despite metabolites, another intriguing hypothesis is the crosstalk between the microbiota and immune cells via extracellular vesicles (EVs) or more specifically exosomes (small EVs with a diameter of 30–150 nm) (55). Whilst there is an ongoing debate as to whether EVs are active signaling components or mainly a “junk disposal system,” it is generally accepted that every living cell produces EVs. The cargo of bacterial EVs depends on the cellular component that they originate from: in Gram-negative bacteria they mainly originate from the outer membrane and contain periplasmic components (outer membrane vesicles, OMVs), whereas Gram-positive bacteria produce bacterial membrane vesicles (BMVs) (56). EVs may contain a variety of cargo including proteins, signaling components, receptors, mRNA and many others. Both OMVs and BMVs are used by bacteria for communication, for example for horizontal transfer of genes of resistance to antibiotics. Further, especially phagocytic immune cells like macrophages or neutrophils may incorporate BMVs or OMVs. For example, OMVs containing small RNA from P. aeruginosa can reduce inflammation of airway epithelial cells as well as neutrophil activation and infiltration in murine lungs (57). Also, BMVs from S. aureus were shown to exert a pro-inflammatory effect on endothelial cells by upregulating the expression of E-selectin, VCAM-1, ICAM-1 and IL-6 which lead to increased recruitment of monocytes (58). A direct communication of the microbiota with neutrophils via EVs would open the door for interventions or immune modulation using EVs from probiotics or commensal bacteria.
Assuming a direct influence of the microbiota on innate immune function and neutrophils, pre- or probiotics may be a feasible way to fine-tune the innate immune response, especially in preterm infants who are at greater risk for gut dysbiosis. The use of pre- and probiotics as perinatal supplements has been discussed as a possible way to improve the composition of microbiota resulting in favorable short and long-term outcomes (59–61). Specifically Lactobacillus and Bifidobacteria are reported to modulate immune responses acting either as immune activators or immune suppressors (62–65). Furthermore, the probiotic strain Bifidobacterium longum 51A has been reported to decline the pro-inflammatory response, as shown by decreased neutrophil recruitment and accumulation thus improving the ability of mice to deal with lung infections induced by Klebsiella pneumoniae (66, 67). Probiotics have been shown to improve neutrophil function and cytokine response in patients with alcoholic cirrhosis and Lactobacillus rhamnosus inhibits Staphylococcus aureus induced NET formation in mice (68, 69). However, specific reports on the effect of probiotics on neutrophils in newborns are missing. Looking at EVs from bacteria, it was shown that OMVs and BMVs derived from probiotics exert anti-inflammatory effects and promote immune tolerance (70). The advantage of EVs over probiotics could be their relative safety (no living bacteria used, especially in preterm infants), standardization and storage. It would also allow for individualization and gestational-age specific treatment. To date however, there have been no clinical trials for the use of bacteria-derived EVs in humans. As it harbors less implications, we are eager to develop this novel field of research.
Long-term Adaptation of Neutrophils
Despite direct effects of the microbiota on neutrophil function, the very limited lifespan raises the question whether such alterations by the microbiota have lasting effects on neutrophil populations. The notion of neutrophils as mere effector cells has been challenged and a growing body of evidence suggests that innate immunity is shaped by surrounding factors and displays adaptive elements (71). This new field of immunology is defined as “trained immunity” or “innate immune memory.” This section will explore, how the triangle connection microbiome—neutrophil—adaptation may promote plasticity in the neutrophil population.
Adaptation—A Twist to Innate Immunity
It has been established that innate immune cells continuously exposed to various pathogens are capable to develop long-term adaptive features manifested with an increase or decrease in their responsiveness. This feature of innate immune cells to mount nonspecific responses displaying thus adaptive characteristics has been defined as memory-like response (72–75). The ability to respond with memory-like (adaptive) behaviors resulting in increased, pro-inflammatory responses after re-challenge by conserved molecules known as pathogen-associated molecular patterns (PAMPs) has been termed as trained immunity or innate memory (74, 76). So, trained immunity has been defined as a nonspecific immunological memory triggered from long-term functional rewiring of the epigenetic program, evoked by different pathogenic insults that results in an altered response, specifically protection against secondary infections (71, 77). Furthermore, a set of host biomolecules known as danger-associated molecular patterns (DAMPs) have been identified to mount memory-like behaviors in macrophages (78). In contrast, exposure of innate immune cells to gram (–) bacterial lipopolysaccharide (LPS) has been reported to induce endotoxin tolerance (desensitization) characterized by decreased pro-inflammation and increased anti-inflammatory responses (79–82).
Initial reports disclosed a PAMP-specific development of innate memory, where priming by β-glucan and Bacillus Calmette-Guerin (BCG), after subsequent challenge by LPS, resulted in increased production of pro-inflammatory cytokines (i.e., IL-1β, TNF-α, IL-6) (83–85). Further studies displayed a pathogen dose-dependent induction of either innate memory by priming with low doses, or tolerance by high doses of sequential challenges with LPS, especially in macrophages and microglia (86–88). Moreover, cellular maturation is another important factor, influencing adaptive features through distinct signaling mechanisms (89, 90). Activation of the phosphoinositide 3-kinase (PI3K)/mechanistic target of rapamycin (mTOR) pathway is critical for the induction of trained immunity in macrophages leading to an increased inflammatory response (91). Contrary, suppression of the mTOR pathway drives immune tolerance, characterized by increased anti-inflammatory responses like IL-10 production and suppression of pro-inflammatory mediators (92). Interestingly, a study found that reduced synthesis of LPS-induced TNF-α is associated with increased activity of AMP-activated protein kinase (AMPK) (93). Several studies further highlighted, that both adaptive features are accompanied by epigenetic reprogramming, with resulting distinct changes in metabolism like increased glycolysis, glutaminolysis or accumulation of fumarate during trained immunity or increased itaconate production during tolerance (91, 94–97). A summary of the metabolic and cellular differences between trained immunity and tolerance is summarized in Table 1.
These opposing immuno-inflammatory responses shaped by different external and internal stressors aim at the reduction and elimination of pathogens—trained immunity as resistance mechanism, or are responsible for promoting maintenance and repairing activities in order to facilitate survival—tolerance as a persistence response (101). In newborns, which are largely reliant on their innate immune system, the development of trained immunity may be of crucial importance for the host survival by providing increased protection against pathogens. Figure 1 shows a schematic representation of the development of such adaptive responses in newborns. A recent study expressed the importance of maternal vaccination and the possible training effects of the innate immune system increasing the rate of survival, where children of BCG-vaccinated mothers had for around 35% less hospital admissions for infectious diseases and 41% lower mortality then control groups (111). The crucial role of trained immunity has also been highlighted in physiological processes during pregnancy where NK cells promote the vascular sprouting in the placenta and favor repeated pregnancies (112, 113). Moreover, NK cells have been observed to improve survival rates in BCG vaccinated mice lacking functional B and T cells, stressing the importance of innate memory in NK cells (84). Akin to BCG vaccinations, infants born to mothers with hepatitis B infection are prone to develop trained immunity in neutrophils or other haematopoietic phagocytic cells such as dendritic cells (DCs) (114). Yet, inappropriate induction of these opposing reactions (training vs. tolerance), might provoke maladaptive responses such as hyper-inflammation exhibiting a close relationship to overflowing resistance responses (trained immunity), or increased susceptibility to opportunistic infections (tolerance) (71, 115–117).
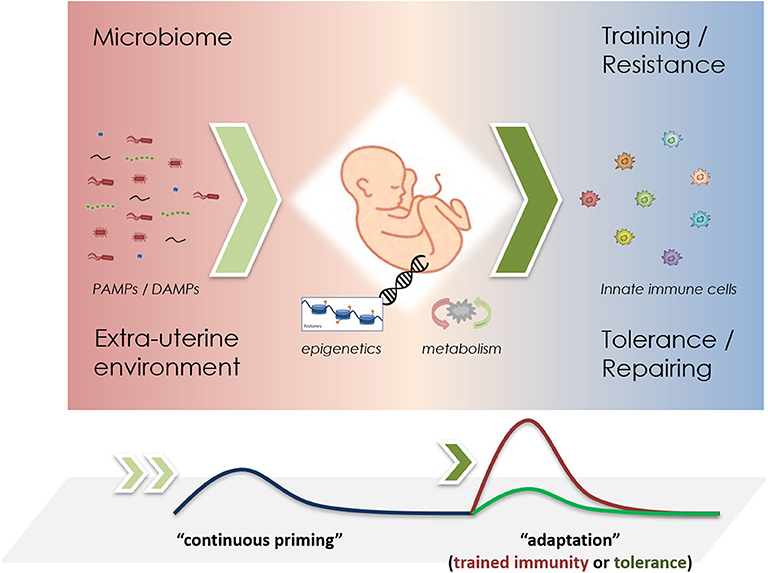
Figure 1. Schematic representation of priming mechanisms in innate immune cells during infancy. The microbiome and the perinatal environment challenge the innate immune system through PAMPs and DAMPs (left), driving epigenetic changes that promote metabolic reprogramming (middle). Upon re-challenge, either trained immunity or tolerance effects may be observed (right). In short-lived neutrophils, it remains unclear, whether “continuous priming” is necessary. If so, a stable microbiome could greatly influence these adaptive responses.
Adaptive Responses in Neutrophils—What Do We Know?
Neutrophils are among the first innate immune cells to enter the site of infection. They are closely shaped by different interactions with the microbiota or the extra-uterine environment (118–121). It was believed that due to their very short live-span neutrophils were unable to participate in enduring memory-like responses (122). However, early investigations showed that neutrophils are prone to be primed by different cytokines, especially IL-8 and TNF-α, improving the recruitment and killing activities of neutrophils in neonates (123, 124). As mentioned above, microbiota-derived mediators may similarly drive adaptive responses (125). For example, microbiome-derived metabolites promote production of antimicrobial peptides such as peptidoglycans that improve the killing capacity of neutrophils (126–128). In line, disruption of the neonatal microbiota by antibiotic exposure is linked to decreased numbers of bone-marrow and peripheral neutrophils, resulting from an impaired granulopoiesis (40).
It is well-known that the pro-inflammatory activity of various innate immune cells increases whilst aging (89, 129, 130). The pro-inflammatory activity of neutrophils characterized by increased tissue infiltration, phagocytosis and NET formation expands with age and is regulated particularly by the TLR4/MyD88 pathway (131). Depletion of the microbiota reduces the number of aged neutrophils and dampens inflammation. The study further confirmed that disruption of the microbiota composition affects the quantity and functional properties of neutrophils. This study outlines the crucial role of microbiota regulating different functional properties of neutrophils, that may drive the inflammatory state toward either resistance mechanisms or a tolerant phenotype. Moreover, microbiota-derived EVs may influence the functional properties of innate immune cells, in particular neutrophils (56, 57, 132, 133). EVs contain a variety of cargo including different proteins, phospholipids, glycolipids, nucleic acids and polysaccharides that are partially able to directly bind to pathogen recognition receptors (PRRs) (132, 134, 135). Furthermore, supplementation by pre- and probiotics may influence the development of adaptive features by neutrophils and other innate immune cells (136–138). Recently, a new concept called “microbiological memory” was introduced aiming to explain the role of microbiome regulating epigenetic rearrangements and their impact on different diseases (139).
A study from Mitroulis et al. showed that trained-immunity-induced effects modulate myeloid progenitors in the bone marrow, especially influencing the recovery of circulating neutrophils thus being of crucial importance for the protection during chemotherapy-induced myelosuppression (140). Furthermore, they revealed that these beneficial effects are closely associated with metabolic changes mainly promoted by altered epigenetic rearrangements. In line with this, another study showed that BCG-induced trained immunity triggers the increase of multipotent and hematopoietic progenitors leading to a sustained myeloid cell expansion (141).
So far it is known that septic reactions in infants are accompanied by a heightened immune activity driven by neutrophils, an energy-demanding process that is mainly promoted by a shift of metabolism toward aerobic glycolysis, a similar process that trained cells undergo (142–144). In line with this, accumulation or activation of several metabolic intermediate products have been shown to either promote the induction of trained immunity (e.g., accumulation of fumarate) or tolerance (i.e., itaconate pathway) thus affecting also main cellular actions of neutrophils that may affect the innate immune response during infancy (94, 97). Priming the niche of neutrophil progenitors may have lasting effects on long-term adaptation. However, the microbiota is a versatile source for innate immune priming, as it may also continuously prime peripheral neutrophils over time (Figure 1).
Taken together, the discovery of the ability of neutrophils to exhibit memory-like responses, open new perspectives for further research in order to understand the impact of trained or tolerized neutrophils in neonatal conditions. A better characterization of the relation duo microbiota—neutrophil could yield potential tailored interventions with probiotics. Here, we are excited to further explore the potential of probiotic-derived EVs as a potentially novel class of safe and standardized biologicals to modulate the immune response. We hope that the PRIMAL Consortium with a clinical trial under way to establish patterns and correlations between gut microbiome and immune profiling may yield answers in the near future (https://primal-studie.de/primal-consortium/).
Conclusions
Due to its immuno-compromised properties, the neonatal immune system is very susceptible to infectious diseases. Different perinatal factors shape the immunological response of innate immune cells including neutrophils. Through regulation of neutrophil production, function and apoptosis, the microbiota greatly influences the capacity of both initiation and resolution of inflammation. Priming and subsequent induction of trained immunity or tolerance introduces adaptive elements into the innate immune system and allows for immune memory. This concept has been described in detail for many innate immune cells, however only limited insight exists for immune memory in neutrophils and how the microbiota may act as a modulator. Their short lifespan makes neutrophils no easy target to study memory effects and leaves two options by which adaptation may occur: either by continuous priming or by modulating the niche of progenitors in the bone marrow. There is evidence for both, however more studies are needed to shed light on this novel aspect of innate immunity. A better understanding of microbiome—neutrophil interactions (for example through EVs) may support the design of tailored probiotic supplementation in newborns.
Author Contributions
TL and HH wrote the manuscript. DF and JP revised the manuscript. All authors contributed to the article and approved the submitted version.
Funding
This review was supported with funds by the German Federal Ministry of Education and Research (HH and DF) (BMBF, 01GL1746E). The sponsor had no role in the preparation of the manuscript.
Conflict of Interest
The authors declare that the research was conducted in the absence of any commercial or financial relationships that could be construed as a potential conflict of interest.
References
1. Rosales C. Neutrophil: a cell with many roles in inflammation or several cell types? Front Physiol. (2018) 9:113. doi: 10.3389/fphys.2018.00113
2. McCracken JM, Allen L-AH. Regulation of human neutrophil apoptosis and lifespan in health and disease. J Cell Death. (2014) 7:15–23. doi: 10.4137/JCD.S11038
3. Németh T, Sperandio M, Mócsai A. Neutrophils as emerging therapeutic targets. Nat Rev Drug Discov. (2020) 19:253–75. doi: 10.1038/s41573-019-0054-z
4. Drescher B, Bai F. Neutrophil in viral infections, friend or foe? Virus Res. (2013) 171:1–7. doi: 10.1016/j.virusres.2012.11.002
5. Hidalgo A, Chilvers ER, Summers C, Koenderman L. The neutrophil life cycle. Trends Immunol. (2019) 40:584–97. doi: 10.1016/j.it.2019.04.013
6. Kaplan MJ, Radic M. Neutrophil extracellular traps: double-edged swords of innate immunity. J Immunol. (2012) 189:2689–95. doi: 10.4049/jimmunol.1201719
7. Leliefeld PHC, Wessels CM, Leenen LPH, Koenderman L, Pillay J. The role of neutrophils in immune dysfunction during severe inflammation. Crit Care. (2016) 20:73. doi: 10.1186/s13054-016-1250-4
8. Gollwitzer ES, Marsland BJ. Impact of early-life exposures on immune maturation and susceptibility to disease. Trends Immunol. (2015) 36:684–96. doi: 10.1016/j.it.2015.09.009
10. Ygberg S, Nilsson A. The developing immune system - from foetus to toddler. Acta Paediatr. (2012) 101:120–7. doi: 10.1111/j.1651-2227.2011.02494.x
11. Nussbaum C, Gloning A, Pruenster M, Frommhold D, Bierschenk S, Genzel-Boroviczény O, et al. Neutrophil and endothelial adhesive function during human fetal ontogeny. J Leukoc Biol. (2013) 93:175–84. doi: 10.1189/jlb.0912468
12. Sperandio M, Quackenbush EJ, Sushkova N, Altstätter J, Nussbaum C, Schmid S, et al. Ontogenetic regulation of leukocyte recruitment in mouse yolk sac vessels. Blood. (2013) 121:e118–28. doi: 10.1182/blood-2012-07-447144
13. Karenberg K, Hudalla H, Frommhold D. Leukocyte recruitment in preterm and term infants. Mol Cell Pediatr. (2016) 3:35. doi: 10.1186/s40348-016-0063-5
14. Hudalla H, Karenberg K, Kuon R-J, Pöschl J, Tschada R, Frommhold D. LPS-induced maternal inflammation promotes fetal leukocyte recruitment and prenatal organ infiltration in mice. Pediatr Res. (2018) 84:757–64. doi: 10.1038/s41390-018-0030-z
15. Falconer AE, Carr R, Edwards SW. Impaired neutrophil phagocytosis in preterm neonates: lack of correlation with expression of immunoglobulin or complement receptors. Neonatology. (1995) 68:264–9. doi: 10.1159/000244245
16. Kallman J, Schollin J, Schalen C, Erlandsson A, Kihlstrom E. Impaired phagocytosis and opsonisation towards group Bstreptococci in preterm neonates. Arch Dis Child Fetal Neonatal Ed. (1998) 78:F46. doi: 10.1136/fn.78.1.F46
17. Filias A, Theodorou GL, Mouzopoulou S, Varvarigou AA, Mantagos S, Karakantza M. Phagocytic ability of neutrophils and monocytes in neonates. BMC Pediatr. (2011) 11:29. doi: 10.1186/1471-2431-11-29
18. Lawrence SM, Corriden R, Nizet V. Age-appropriate functions and dysfunctions of the neonatal neutrophil. Front Pediatr. (2017) 5:23. doi: 10.3389/fped.2017.00023
19. M'Rabet L, Vos AP, Boehm G, Garssen J. Breast-feeding and its role in early development of the immune system in infants: consequences for health later in life. J Nutr. (2008) 138:1782S−90S. doi: 10.1093/jn/138.9.1782S
20. Dominguez-Bello MG, Costello EK, Contreras M, Magris M, Hidalgo G, Fierer N, et al. Delivery mode shapes the acquisition and structure of the initial microbiota across multiple body habitats in newborns. Proc Natl Acad Sci USA. (2010) 107:11971–5. doi: 10.1073/pnas.1002601107
21. Loss G, Bitter S, Wohlgensinger J, Frei R, Roduit C, Genuneit J, et al. Prenatal and early-life exposures alter expression of innate immunity genes: the PASTURE cohort study. J Allergy Clin Immunol. (2012) 130:523–30.e9. doi: 10.1016/j.jaci.2012.05.049
22. Cho CE, Norman M. Cesarean section and development of the immune system in the offspring. Am J Obstet Gynecol. (2013) 208:249–54. doi: 10.1016/j.ajog.2012.08.009
23. Macpherson AJ, de Agüero MG, Ganal-Vonarburg SC. How nutrition and the maternal microbiota shape the neonatal immune system. Nat Rev Immunol. (2017) 17:508–17. doi: 10.1038/nri.2017.58
24. Ley RE, Peterson DA, Gordon JI. Ecological and evolutionary forces shaping microbial diversity in the human intestine. Cell. (2006) 124:837–48. doi: 10.1016/j.cell.2006.02.017
25. Bonder MJ, Kurilshikov A, Tigchelaar EF, Mujagic Z, Imhann F, Vila AV, et al. The effect of host genetics on the gut microbiome. Nat Genet. (2016) 48:1407–12. doi: 10.1038/ng.3663
26. Gupta N, Kumar R, Agrawal B. New players in immunity to tuberculosis: the host microbiome, lung epithelium, and innate immune cells. Front Immunol. (2018) 9:709. doi: 10.3389/fimmu.2018.00709
27. Francino MP. Early development of the gut microbiota and immune health. Pathogens. (2014) 3:769. doi: 10.3390/pathogens3030769
28. Milani C, Duranti S, Bottacini F, Casey E, Turroni F, Mahony J, et al. The first microbial colonizers of the human gut: composition, activities, and health implications of the infant gut microbiota. Microbiol Mol Biol Rev. (2017) 81:e00036–17. doi: 10.1128/MMBR.00036-17
29. Robertson RC, Manges AR, Finlay BB, Prendergast AJ. The human microbiome and child growth - first 1000 days and beyond. Trends Microbiol. (2019) 27:131–47. doi: 10.1016/j.tim.2018.09.008
30. PrabhuDas M, Bonney E, Caron K, Dey S, Erlebacher A, Fazleabas A, et al. Immune mechanisms at the maternal-fetal interface: perspectives and challenges. Nat Immunol. (2015) 16:328–34. doi: 10.1038/ni.3131
31. Mor G, Aldo P, Alvero AB. The unique immunological and microbial aspects of pregnancy. Nat Rev Immunol. (2017) 17:469–82. doi: 10.1038/nri.2017.64
32. van Well GTJ, Daalderop LA, Wolfs T, Kramer BW. Human perinatal immunity in physiological conditions and during infection. Mol Cell Pediatr. (2017) 4:4. doi: 10.1186/s40348-017-0070-1
33. Wampach L, Heintz-Buschart A, Fritz JV, Ramiro-Garcia J, Habier J, Herold M, et al. Birth mode is associated with earliest strain-conferred gut microbiome functions and immunostimulatory potential. Nat Commun. (2018) 9:5091. doi: 10.1038/s41467-018-07631-x
34. Kanoh S, Rubin BK. Mechanisms of action and clinical application of macrolides as immunomodulatory medications. Clin Microbiol Rev. (2010) 23:590–615. doi: 10.1128/CMR.00078-09
35. Zeissig S, Blumberg RS. Life at the beginning: perturbation of the microbiota by antibiotics in early life and its role in health and disease. Nat Immunol. (2014) 15:307–10. doi: 10.1038/ni.2847
36. Sugihara E. Effect of macrolide antibiotics on neutrophil function in human peripheral blood. Kansenshogaku Zasshi. (1997) 71:329–36. doi: 10.11150/kansenshogakuzasshi1970.71.329
37. Pammi M, Cope J, Tarr PI, Warner BB, Morrow AL, Mai V, et al. Intestinal dysbiosis in preterm infants preceding necrotizing enterocolitis: a systematic review and meta-analysis. Microbiome. (2017) 5:31. doi: 10.1186/s40168-017-0248-8
38. Warner BB, Deych E, Zhou Y, Hall-Moore C, Weinstock GM, Sodergren E, et al. Gut bacteria dysbiosis and necrotising enterocolitis in very low birthweight infants: a prospective case-control study. Lancet. (2016) 387:1928–36. doi: 10.1016/S0140-6736(16)00081-7
39. Mai V, Torrazza RM, Ukhanova M, Wang X, Sun Y, Li N, et al. Distortions in development of intestinal microbiota associated with late onset sepsis in preterm infants. PLoS ONE. (2013) 8:e52876. doi: 10.1371/journal.pone.0052876
40. Deshmukh HS, Liu Y, Menkiti OR, Mei J, Dai N, O'Leary CE, et al. The microbiota regulates neutrophil homeostasis and host resistance to Escherichia coli K1 sepsis in neonatal mice. Nat Med. (2014) 20:524–30. doi: 10.1038/nm.3542
41. Pflughoeft KJ, Versalovic J. Human microbiome in health and disease. Annu Rev Pathol. (2012) 7:99–122. doi: 10.1146/annurev-pathol-011811-132421
42. Marißen J, Haiß A, Meyer C, Van Rossum T, Bünte LM, Frommhold D, et al. Efficacy of Bifidobacterium longum, B. infantis and Lactobacillus acidophilus probiotics to prevent gut dysbiosis in preterm infants of 28+0-32+6 weeks of gestation: a randomised, placebo-controlled, double-blind, multicentre trial: the PRIMAL Clinical Study protocol. BMJ Open. (2019) 9:e032617. doi: 10.1136/bmjopen-2019-032617
43. Goris H, de Boer F, van der Waaij D. Myelopoiesis in experimentally contaminated specific-pathogen-free and germfree mice during oral administration of polymyxin. Infect Immun. (1985) 50:437–41. doi: 10.1128/IAI.50.2.437-441.1985
44. Tada T, Yamamura S, Kuwano Y, Abo T. Level of myelopoiesis in the bone marrow is influenced by intestinal flora. Cell Immunol. (1996) 173:155–61. doi: 10.1006/cimm.1996.0261
45. Khosravi A, Yáñez A, Price JG, Chow A, Merad M, Goodridge HS, et al. Gut microbiota promote hematopoiesis to control bacterial infection. Cell Host Microbe. (2014) 15:374–81. doi: 10.1016/j.chom.2014.02.006
46. Josefsdottir KS, Baldridge MT, Kadmon CS, King KY. Antibiotics impair murine hematopoiesis by depleting the intestinal microbiota. Blood. (2017) 129:729–39. doi: 10.1182/blood-2016-03-708594
47. Iwamura C, Bouladoux N, Belkaid Y, Sher A, Jankovic D. Sensing of the microbiota by NOD1 in mesenchymal stromal cells regulates murine hematopoiesis. Blood. (2017) 129:171–6. doi: 10.1182/blood-2016-06-723742
48. Luo Y, Chen G-L, Hannemann N, Ipseiz N, Krönke G, Bäuerle T, et al. Microbiota from obese mice regulate hematopoietic stem cell differentiation by altering the bone niche. Cell Metab. (2015) 22:886–94. doi: 10.1016/j.cmet.2015.08.020
49. Zhang D, Frenette PS. Cross talk between neutrophils and the microbiota. Blood. (2019) 133:2168–77. doi: 10.1182/blood-2018-11-844555
50. Vinolo MAR, Rodrigues HG, Hatanaka E, Hebeda CB, Farsky SHP, Curi R. Short-chain fatty acids stimulate the migration of neutrophils to inflammatory sites. Clin Sci. (2009) 117:331–8. doi: 10.1042/CS20080642
51. Koh A, De Vadder F, Kovatcheva-Datchary P, Bäckhed F. From dietary fiber to host physiology: short-chain fatty acids as key bacterial metabolites. Cell. (2016) 165:1332–45. doi: 10.1016/j.cell.2016.05.041
52. Aoyama M, Kotani J, Usami M. Butyrate and propionate induced activated or non-activated neutrophil apoptosis via HDAC inhibitor activity but without activating GPR-41/GPR-43 pathways. Nutrition. (2010) 26:653–61. doi: 10.1016/j.nut.2009.07.006
53. Vieira AT, Galvão I, Macia LM, Sernaglia ÉM, Vinolo MAR, Garcia CC, et al. Dietary fiber and the short-chain fatty acid acetate promote resolution of neutrophilic inflammation in a model of gout in mice. J Leukoc Biol. (2017) 101:275–84. doi: 10.1189/jlb.3A1015-453RRR
54. Guo C, Xie S, Chi Z, Zhang J, Liu Y, Zhang L, et al. Bile acids control inflammation and metabolic disorder through inhibition of NLRP3 inflammasome. Immunity. (2016) 45:802–16. doi: 10.1016/j.immuni.2016.09.008
55. Colombo M, Raposo G, Théry C. Biogenesis, secretion, and intercellular interactions of exosomes and other extracellular vesicles. Annu Rev Cell Dev Biol. (2014) 30:255–89. doi: 10.1146/annurev-cellbio-101512-122326
56. Macia L, Nanan R, Hosseini-Beheshti E, Grau GE. Host- and microbiota-derived extracellular vesicles, immune function, and disease development. Int J Mol Sci. (2019) 21:107. doi: 10.3390/ijms21010107
57. Koeppen K, Hampton TH, Jarek M, Scharfe M, Gerber SA, Mielcarz DW, et al. A novel mechanism of host-pathogen interaction through sRNA in bacterial outer membrane vesicles. PLOS Pathog. (2016) 12:e1005672. doi: 10.1371/journal.ppat.1005672
58. Kim J, Bin BH, Choi EJ, Lee HG, Lee TR, Cho EG. Staphylococcus aureus-derived extracellular vesicles induce monocyte recruitment by activating human dermal microvascular endothelial cells in vitro. Clin Exp Allergy. (2019) 49:68–81. doi: 10.1111/cea.13289
59. Vieira AT, Teixeira MM, Martins FS. The role of probiotics and prebiotics in inducing gut immunity. Front Immunol. (2013) 4:445. doi: 10.3389/fimmu.2013.00445
60. Sohn K, Underwood MA. Prenatal and postnatal administration of prebiotics and probiotics. Semin Fetal Neonatal Med. (2017) 22:284–9. doi: 10.1016/j.siny.2017.07.002
61. Swartwout B, Luo XM. Implications of probiotics on the maternal-neonatal interface: gut microbiota, immunomodulation, and autoimmunity. Front Immunol. (2018) 9:2840. doi: 10.3389/fimmu.2018.02840
62. Kim SW, Kim HM, Yang KM, Kim S-A, Kim S-K, An MJ, et al. Bifidobacterium lactis inhibits NF-kappaB in intestinal epithelial cells and prevents acute colitis and colitis-associated colon cancer in mice. Inflamm Bowel Dis. (2010) 16:1514–25. doi: 10.1002/ibd.21262
63. Tomosada Y, Villena J, Murata K, Chiba E, Shimazu T, Aso H, et al. Immunoregulatory effect of bifidobacteria strains in porcine intestinal epithelial cells through modulation of ubiquitin-editing enzyme A20 expression. PLoS ONE. (2013) 8:e59259. doi: 10.1371/journal.pone.0059259
64. Takanashi N, Tomosada Y, Villena J, Murata K, Takahashi T, Chiba E, et al. Advanced application of bovine intestinal epithelial cell line for evaluating regulatory effect of lactobacilli against heat-killed enterotoxigenic Escherichia coli-mediated inflammation. BMC Microbiol. (2013) 13:54. doi: 10.1186/1471-2180-13-54
65. Yan F, Liu L, Dempsey PJ, Tsai Y-H, Raines EW, Wilson CL, et al. A Lactobacillus rhamnosus GG-derived soluble protein, p40, stimulates ligand release from intestinal epithelial cells to transactivate epidermal growth factor receptor. J Biol Chem. (2013) 288:30742–51. doi: 10.1074/jbc.M113.492397
66. Vieira AT, Galvão I, Amaral FA, Teixeira MM, Nicoli JR, Martins FS. Oral treatment with Bifidobacterium longum 51A reduced inflammation in a murine experimental model of gout. Benef Microbes. (2015) 6:799–806. doi: 10.3920/BM2015.0015
67. Vieira AT, Rocha VM, Tavares L, Garcia CC, Teixeira MM, Oliveira SC, et al. Control of Klebsiella pneumoniae pulmonary infection and immunomodulation by oral treatment with the commensal probiotic Bifidobacterium longum 51A. Microbes Infect. (2016) 18:180–9. doi: 10.1016/j.micinf.2015.10.008
68. Stadlbauer V, Mookerjee RP, Hodges S, Wright GAK, Davies NA, Jalan R. Effect of probiotic treatment on deranged neutrophil function and cytokine responses in patients with compensated alcoholic cirrhosis. J Hepatol. (2008) 48:945–51. doi: 10.1016/j.jhep.2008.02.015
69. Vong L, Lorentz RJ, Assa A, Glogauer M, Sherman PM. Probiotic Lactobacillus rhamnosus inhibits the formation of neutrophil extracellular traps. J Immunol. (2014) 192:1870–7. doi: 10.4049/jimmunol.1302286
70. Fábrega MJ, Aguilera L, Giménez R, Varela E, Cañas MA, Antolín M, et al. Activation of immune and defense responses in the intestinal mucosa by outer membrane vesicles of commensal and probiotic Escherichia coli strains. Front Microbiol. (2016) 7:705. doi: 10.3389/fmicb.2016.00705
71. Netea MG, Joosten LAB, Latz E, Mills KHG, Natoli G, Stunnenberg HG, et al. Trained immunity: a program of innate immune memory in health and disease. Science (80-).(2016) 352:aaf1098. doi: 10.1126/science.aaf1098
72. Sun JC, Ugolini S, Vivier E. Immunological memory within the innate immune system. EMBO J. (2014) 33:1295–303. doi: 10.1002/embj.201387651
73. Kugelberg E. Macrophages: controlling innate immune memory. Nat Rev Immunol. (2015) 15:596. doi: 10.1038/nri3914
74. Netea MG, Latz E, Kingston HG, Mills LA, Neill JO'. Innate immune memory: a paradigm shift in understanding host defense. Nat Immunol. (2015) 16:675–9. doi: 10.1038/ni.3178
75. Yoshida K, Maekawa T, Zhu Y, Renard-Guillet C, Chatton B, Inoue K, et al. The transcription factor ATF7 mediates lipopolysaccharide-induced epigenetic changes in macrophages involved in innate immunological memory. Nat Immunol. (2015) 16:1034–43. doi: 10.1038/ni.3257
76. Netea MG, van der Meer JWM. Trained immunity: an ancient way of remembering. Cell Host Microbe. (2017) 21:297–300. doi: 10.1016/j.chom.2017.02.003
77. Netea MG, Domínguez-Andrés J, Barreiro LB, Chavakis T, Divangahi M, Fuchs E, et al. Defining trained immunity and its role in health and disease. Nat Rev Immunol. (2020) 20:375–88. doi: 10.1038/s41577-020-0285-6
78. van der Valk FM, Bekkering S, Kroon J, Yeang C, Van den Bossche J, van Buul JD, et al. Oxidized phospholipids on lipoprotein(a) elicit arterial wall inflammation and an inflammatory monocyte response in humans. Circulation. (2016) 134:611–24. doi: 10.1161/CIRCULATIONAHA.116.020838
79. West M, Heagy W. Endotoxin tolerance: a review. Crit Care Med. (2002) 30:S64–73. doi: 10.1097/00003246-200201001-00009
80. Beutler B, Rietschel ET. Innate immune sensing and its roots: the story of endotoxin. Nat Rev Immunol. (2003) 3:169–76. doi: 10.1038/nri1004
81. Sly LM, Rauh MJ, Kalesnikoff J, Song CH, Krystal G. LPS-induced upregulation of SHIP is essential for endotoxin tolerance. Immunity. (2004) 21:227–39. doi: 10.1016/j.immuni.2004.07.010
82. López-Collazo E, del Fresno C. Pathophysiology of endotoxin tolerance: mechanisms and clinical consequences. Crit Care. (2013) 17:242. doi: 10.1186/cc13110
83. Quintin J, Saeed S, Martens JHAA, Giamarellos-Bourboulis EJ, Ifrim DC, Logie C, et al. Candida albicans infection affords protection against reinfection via functional reprogramming of monocytes. Cell Host Microbe. (2012) 12:223–32. doi: 10.1016/j.chom.2012.06.006
84. Kleinnijenhuis J, Quintin J, Preijers F, Joosten LAB, Ifrim DC, Saeed S, et al. Bacille Calmette-Guerin induces NOD2-dependent nonspecific protection from reinfection via epigenetic reprogramming of monocytes. Proc Natl Acad Sci USA. (2012) 109:17537–42. doi: 10.1073/pnas.1202870109
85. Kleinnijenhuis J, Quintin J, Preijers F, Joosten LAB, Jacobs C, Xavier RJ, et al. BCG-induced trained immunity in NK cells: role for non-specific protection to infection. Clin Immunol. (2014) 155:213–9. doi: 10.1016/j.clim.2014.10.005
86. Yuan R, Geng S, Li L. Molecular mechanisms that underlie the dynamic adaptation of innate monocyte memory to varying stimulant strength of TLR ligands. Front Immunol. (2016) 7:497. doi: 10.3389/fimmu.2016.00497
87. Wendeln AC, Degenhardt K, Kaurani L, Gertig M, Ulas T, Jain G, et al. Innate immune memory in the brain shapes neurological disease hallmarks. Nature. (2018) 556:332–8. doi: 10.1038/s41586-018-0023-4
88. Lajqi T, Lang GP, Haas F, Williams DL, Hudalla H, Bauer M, et al. Memory-like inflammatory responses of microglia to rising doses of LPS: key role of PI3Kγ. Front Immunol. (2019) 10:2492. doi: 10.3389/fimmu.2019.02492
89. Lajqi T, Stojiljkovic M, Wetzker R. Toxin-induced hormesis may restrain aging. Biogerontology. (2019) 20:571–81. doi: 10.1007/s10522-019-09806-5
90. Namakula R, de Bree LCJA, Tvedt TH, Netea MG, Cose S, Hanevik K. Monocytes from neonates and adults have a similar capacity to adapt their cytokine production after previous exposure to BCG and β-glucan. PLoS ONE. (2020) 15:e0229287. doi: 10.1371/journal.pone.0229287
91. Cheng SC, Quintin J, Cramer RA, Shepardson KM, Saeed S, Kumar V, et al. mTOR- and HIF-1α-mediated aerobic glycolysis as metabolic basis for trained immunity. Science (80-).(2014) 345:1250684. doi: 10.1126/science.1250684
92. Ip WKE, Hoshi N, Shouval DS, Snapper S, Medzhitov R. Anti-inflammatory effect of IL-10 mediated by metabolic reprogramming of macrophages. Science (80-).(2017) 356:513–9. doi: 10.1126/science.aal3535
93. Kim J, Kwak HJ, Cha JY, Jeong YS, Rhee SD, Kim KR, et al. Metformin suppresses lipopolysaccharide (LPS)-induced inflammatory response in murine macrophages via Activating Transcription Factor-3 (ATF-3) induction. J Biol Chem. (2014) 289:23246–55. doi: 10.1074/jbc.M114.577908
94. Arts RJ, Novakovic B, ter Horst R, Carvalho A, Bekkering S, Lachmandas E, et al. Glutaminolysis and fumarate accumulation integrate immunometabolic and epigenetic programs in trained immunity. Cell Metab. (2016) 24:807–19. doi: 10.1016/j.cmet.2016.10.008
95. Arts RJW, Carvalho A, La Rocca C, Palma C, Rodrigues F, Silvestre R, et al. Immunometabolic pathways in BCG-induced trained immunity. Cell Rep. (2016) 17:2562–71. doi: 10.1016/j.celrep.2016.11.011
96. O'Neill LAJ, Artyomov MN. Itaconate: the poster child of metabolic reprogramming in macrophage function. Nat Rev Immunol. (2019) 19:273–81. doi: 10.1038/s41577-019-0128-5
97. Domínguez-Andrés J, Novakovic B, Li Y, Scicluna BP, Gresnigt MS, Arts RJ, et al. The itaconate pathway is a central regulatory node linking innate immune tolerance and trained immunity. Cell Metab. (2019) 29:211–20.e5. doi: 10.1016/j.cmet.2018.09.003
98. Ifrim DC, Quintin J, Joosten LAB, Jacobs C, Jansen T, Jacobs L, et al. Trained immunity or tolerance: opposing functional programs induced in human monocytes after engagement of various pattern recognition receptors. Clin Vaccine Immunol. (2014) 21:534–45. doi: 10.1128/CVI.00688-13
99. Maitra U, Deng H, Glaros T, Baker B, Capelluto DGS, Li Z, et al. Molecular mechanisms responsible for the selective and low-grade induction of proinflammatory mediators in murine macrophages by lipopolysaccharide. J Immunol. (2012) 189:1014–23. doi: 10.4049/jimmunol.1200857
100. Schaafsma W, Zhang X, van Zomeren KC, Jacobs S, Georgieva PB, Wolf SA, et al. Long-lasting pro-inflammatory suppression of microglia by LPS-preconditioning is mediated by RelB-dependent epigenetic silencing. Brain Behav Immun. (2015) 48:205–21. doi: 10.1016/j.bbi.2015.03.013
101. Bauer M, Weis S, Netea MG, Wetzker R. Remembering pathogen dose: long-term adaptation in innate immunity. Trends Immunol. (2018) 39:438–45. doi: 10.1016/j.it.2018.04.001
102. Jeon S-MM. Regulation and function of AMPK in physiology and diseases. Exp Mol Med. (2016) 48:e245. doi: 10.1038/emm.2016.81
103. Arts RJW, Joosten LAB, Netea MG. Immunometabolic circuits in trained immunity. Semin Immunol. (2016) 28:425–30. doi: 10.1016/j.smim.2016.09.002
104. Liu Y, Liang S, Ding R, Hou Y, Deng F, Ma X, et al. BCG-induced trained immunity in macrophage: reprograming of glucose metabolism. Int Rev Immunol. (2020) 39:83–96. doi: 10.1080/08830185.2020.1712379
105. Pena OM, Pistolic J, Raj D, Fjell CD, Hancock REW. Endotoxin tolerance represents a distinctive state of alternative polarization (M2) in human mononuclear cells. J Immunol. (2011) 186:7243–54. doi: 10.4049/jimmunol.1001952
106. Jeljeli M, Riccio LGC, Doridot L, Chêne C, Nicco C, Chouzenoux S, et al. Trained immunity modulates inflammation-induced fibrosis. Nat Commun. (2019) 10:5670. doi: 10.1038/s41467-019-13636-x
107. Chen X, El Gazzar M, Yoza BK, McCall CE. The NF-kB factor RelB and histone H3 lysine methyltransferase G9a directly interact to generate epigenetic silencing in endotoxin tolerance. J Biol Chem. (2009) 284:27857–65. doi: 10.1074/jbc.M109.000950
108. Saeed S, Quintin J, Kerstens HH, Rao NA, Aghajanirefah A, Matarese F, et al. Epigenetic programming of monocyte-to-macrophage differentiation and trained innate immunity. Science ((80-).(2014) 345:1251086. doi: 10.1126/science.1251086
109. Doxaki C, Kampranis SC, Eliopoulos AG, Spilianakis C, Tsatsanis C. Coordinated regulation of miR-155 and miR-146a genes during induction of endotoxin tolerance in macrophages. J Immunol. (2015) 195:5750–61. doi: 10.4049/jimmunol.1500615
110. Poplutz M, Levikova M, Lüscher-Firzlaff J, Lesina M, Algül H, Lüscher B, et al. Endotoxin tolerance in mast cells, its consequences for IgE-mediated signalling, and the effects of BCL3 deficiency. Sci Rep. (2017) 7:4534. doi: 10.1038/s41598-017-04890-4
111. Berendsen MLT, Øland CB, Bles P, Jensen AKG, Kofoed P-E, Whittle H, et al. Maternal priming: Bacillus Calmette-Guérin (BCG) vaccine scarring in mothers enhances the survival of their child with a BCG vaccine scar. J Pediatric Infect Dis Soc. (2020) 9:166–72. doi: 10.1093/jpids/piy142
112. Kuon RJ, Müller F, Vomstein K, Weber M, Hudalla H, Rösner S, et al. Pre-pregnancy levels of peripheral natural killer cells as markers for immunomodulatory treatment in patients with recurrent miscarriage. Arch Immunol Ther Exp. (2017) 65:339–46. doi: 10.1007/s00005-017-0457-7
113. Gamliel M, Goldman-Wohl D, Isaacson B, Gur C, Stein N, Yamin R, et al. Trained memory of human uterine NK cells enhances their function in subsequent pregnancies. Immunity. (2018) 48:951–62.e5. doi: 10.1016/j.immuni.2018.03.030
114. Hong M, Sandalova E, Low D, Gehring AJ, Fieni S, Amadei B, et al. Trained immunity in newborn infants of HBV-infected mothers. Nat Commun. (2015) 6:6588. doi: 10.1038/ncomms7588
115. Rajaiah R, Perkins DJ, Polumuri SK, Zhao A, Keegan AD, Vogel SN. Dissociation of endotoxin tolerance and differentiation of alternatively activated macrophages. J Immunol. (2013) 190:4763–72. doi: 10.4049/jimmunol.1202407
116. O'Carroll C, Fagan A, Shanahan F, Carmody RJ. Identification of a unique hybrid macrophage-polarization state following recovery from lipopolysaccharide tolerance. J Immunol. (2014) 192:427–36. doi: 10.4049/jimmunol.1301722
117. Bauer M, Wetzker R. The cellular basis of organ failure in sepsis-signaling during damage and repair processes. Med Klin Intens Notfallmedizin. (2020) 115:4–9. doi: 10.1007/s00063-020-00673-4
118. Basha S, Surendran N, Pichichero M. Immune responses in neonates. Expert Rev Clin Immunol. (2014) 10:1171–84. doi: 10.1586/1744666X.2014.942288
119. Hergott CB, Roche AM, Tamashiro E, Clarke TB, Bailey AG, Laughlin A, et al. Peptidoglycan from the gut microbiota governs the lifespan of circulating phagocytes at homeostasis. Blood. (2016) 127:2460–71. doi: 10.1182/blood-2015-10-675173
120. Witter AR, Okunnu BM, Berg RE. The essential role of neutrophils during infection with the intracellular bacterial pathogen listeria monocytogenes. J Immunol. (2016) 197:1557–65. doi: 10.4049/jimmunol.1600599
121. Dzidic M, Boix-Amorós A, Selma-Royo M, Mira A, Collado M. Gut microbiota and mucosal immunity in the neonate. Med Sci. (2018) 6:56. doi: 10.3390/medsci6030056
122. Netea MG. Training innate immunity: the changing concept of immunological memory in innate host defence. Eur J Clin Invest. (2013) 43:881–4. doi: 10.1111/eci.12132
123. Ferrante A. Tumor necrosis factor alpha potentiates neutrophil antimicrobial activity: increased fungicidal activity against Torulopsis glabrata and Candida albicans and associated increases in oxygen radical production and lysosomal enzyme release. Infect Immun. (1989) 57:2115–22. doi: 10.1128/IAI.57.7.2115-2122.1989
124. Yektaei-Karin E, Moshfegh A, Lundahl J, Berggren V, Hansson L-O, Marchini G. The stress of birth enhances in vitro spontaneous and IL-8-induced neutrophil chemotaxis in the human newborn. Pediatr Allergy Immunol. (2007) 18:643–51. doi: 10.1111/j.1399-3038.2007.00578.x
125. Schirmer M, Smeekens SP, Vlamakis H, Jaeger M, Oosting M, Franzosa EA, et al. Linking the human gut microbiome to inflammatory cytokine production capacity. Cell. (2016) 167:1125–36.e8. doi: 10.1016/j.cell.2016.10.020
126. Wikoff WR, Anfora AT, Liu J, Schultz PG, Lesley SA, Peters EC, et al. Metabolomics analysis reveals large effects of gut microflora on mammalian blood metabolites. Proc Natl Acad Sci USA. (2009) 106:3698–703. doi: 10.1073/pnas.0812874106
127. Clarke TB, Davis KM, Lysenko ES, Zhou AY, Yu Y, Weiser JN. Recognition of peptidoglycan from the microbiota by Nod1 enhances systemic innate immunity. Nat Med. (2010) 16:228–31. doi: 10.1038/nm.2087
128. Sridharan GV, Choi K, Klemashevich C, Wu C, Prabakaran D, Pan L, et al. Prediction and quantification of bioactive microbiota metabolites in the mouse gut. Nat Commun. (2014) 5:5492. doi: 10.1038/ncomms6492
129. Norden DM, Godbout JP. Review: microglia of the aged brain: primed to be activated and resistant to regulation. Neuropathol Appl Neurobiol. (2013) 39:19–34. doi: 10.1111/j.1365-2990.2012.01306.x
130. van Beek AA, Van den Bossche J, Mastroberardino PG, de Winther MPJ, Leenen PJM. Metabolic alterations in aging macrophages: ingredients for inflammaging? Trends Immunol. (2019) 40:113–27. doi: 10.1016/j.it.2018.12.007
131. Zhang D, Chen G, Manwani D, Mortha A, Xu C, Faith JJ, et al. Neutrophil ageing is regulated by the microbiome. Nature. (2015) 525:528–32. doi: 10.1038/nature15367
132. Ahmadi Badi S, Moshiri A, Fateh A, Rahimi Jamnani F, Sarshar M, Vaziri F, et al. Microbiota-derived extracellular vesicles as new systemic regulators. Front Microbiol. (2017) 8:1610. doi: 10.3389/fmicb.2017.01610
133. Sierro F, Grau GER. The ins and outs of cerebral malaria pathogenesis: immunopathology, extracellular vesicles, immunometabolism, and trained immunity. Front Immunol. (2019) 10:830. doi: 10.3389/fimmu.2019.00830
134. Bielig H, Dongre M, Zurek B, Wai SN, Kufer TA. A role for quorum sensing in regulating innate immune responses mediated by Vibrio cholerae outer membrane vesicles (OMVs). Gut Microbes. (2011) 2:274–9. doi: 10.4161/gmic.2.5.18091
135. Kaparakis-Liaskos M, Ferrero RL. Immune modulation by bacterial outer membrane vesicles. Nat Rev Immunol. (2015) 15:375–87. doi: 10.1038/nri3837
136. Hemarajata P, Versalovic J. Effects of probiotics on gut microbiota: mechanisms of intestinal immunomodulation and neuromodulation. Therap Adv Gastroenterol. (2013) 6:39–51. doi: 10.1177/1756283X12459294
137. Sanders ME, Merenstein DJ, Reid G, Gibson GR, Rastall RA. Probiotics and prebiotics in intestinal health and disease: from biology to the clinic. Nat Rev Gastroenterol Hepatol. (2019) 16:605–16. doi: 10.1038/s41575-019-0173-3
138. Enam F, Mansell TJ. Prebiotics: tools to manipulate the gut microbiome and metabolome. J Ind Microbiol Biotechnol. (2019) 46:1445–59. doi: 10.1007/s10295-019-02203-4
139. Devaux CA, Raoult D. The microbiological memory, an epigenetic regulator governing the balance between good health and metabolic disorders. Front Microbiol. (2018) 9:1379. doi: 10.3389/fmicb.2018.01379
140. Mitroulis I, Ruppova K, Wang B, Chen LS, Grzybek M, Grinenko T, et al. Modulation of myelopoiesis progenitors is an integral component of trained immunity. Cell. (2018) 172:147–61.e12. doi: 10.1016/j.cell.2017.11.034
141. Kaufmann E, Sanz J, Dunn JL, Khan N, Mendonça LE, Pacis A, et al. BCG educates hematopoietic stem cells to generate protective innate immunity against tuberculosis. Cell. (2018) 172:176–90.e19. doi: 10.1016/j.cell.2017.12.031
142. Pearce EL, Pearce EJ. Metabolic pathways in immune cell activation and quiescence. Immunity. (2013) 38:633–43. doi: 10.1016/j.immuni.2013.04.005
143. Cheng SC, Scicluna BP, Arts RJ, Gresnigt MS, Lachmandas E, Giamarellos-Bourboulis EJ, et al. Broad defects in the energy metabolism of leukocytes underlie immunoparalysis in sepsis. Nat Immunol. (2016) 17:406–13. doi: 10.1038/ni.3398
Keywords: microbiota, trained immunity, neutrophil (PMN), innate immunity, immune priming, newborn - immunology
Citation: Lajqi T, Pöschl J, Frommhold D and Hudalla H (2020) The Role of Microbiota in Neutrophil Regulation and Adaptation in Newborns. Front. Immunol. 11:568685. doi: 10.3389/fimmu.2020.568685
Received: 01 June 2020; Accepted: 31 August 2020;
Published: 29 September 2020.
Edited by:
David J. Dowling, Boston Children's Hospital, United StatesReviewed by:
Angelica Thomaz Vieira, Federal University of Minas Gerais, BrazilMihaela Gadjeva, Harvard Medical School, United States
Copyright © 2020 Lajqi, Pöschl, Frommhold and Hudalla. This is an open-access article distributed under the terms of the Creative Commons Attribution License (CC BY). The use, distribution or reproduction in other forums is permitted, provided the original author(s) and the copyright owner(s) are credited and that the original publication in this journal is cited, in accordance with accepted academic practice. No use, distribution or reproduction is permitted which does not comply with these terms.
*Correspondence: Hannes Hudalla, SGFubmVzLkh1ZGFsbGFAbWVkLnVuaS1oZWlkZWxiZXJnLmRl