- 1Program in Immunology, Clinical Research Division, Fred Hutchinson Cancer Research Center, Seattle, WA, United States
- 2Immunotherapy Integrated Research Center, Fred Hutchinson Cancer Research Center, Seattle, WA, United States
- 3Department of Immunology, University of Washington, Seattle, WA, United States
Even though the thymus is exquisitely sensitive to acute insults like infection, shock, or common cancer therapies such as cytoreductive chemo- or radiation-therapy, it also has a remarkable capacity for repair. This phenomenon of endogenous thymic regeneration has been known for longer even than its primary function to generate T cells, however, the underlying mechanisms controlling the process have been largely unstudied. Although there is likely continual thymic involution and regeneration in response to stress and infection in otherwise healthy people, acute and profound thymic damage such as that caused by common cancer cytoreductive therapies or the conditioning regimes as part of hematopoietic cell transplantation (HCT), leads to prolonged T cell deficiency; precipitating high morbidity and mortality from opportunistic infections and may even facilitate cancer relapse. Furthermore, this capacity for regeneration declines with age as a function of thymic involution; which even at steady state leads to reduced capacity to respond to new pathogens, vaccines, and immunotherapy. Consequently, there is a real clinical need for strategies that can boost thymic function and enhance T cell immunity. One approach to the development of such therapies is to exploit the processes of endogenous thymic regeneration into novel pharmacologic strategies to boost T cell reconstitution in clinical settings of immune depletion such as HCT. In this review, we will highlight recent work that has revealed the mechanisms by which the thymus is capable of repairing itself and how this knowledge is being used to develop novel therapies to boost immune function.
Introduction
Generation of a diverse but tolerant T cell repertoire, which is critical for adaptive immune function, is dependent on the development and maturation of T cell precursors in the thymus. The process of T cell development is reliant on the interactions with the stromal microenvironment, comprised of highly specialized thymic epithelial cells (TECs), endothelial cells (ECs), mesenchymal cells, dendritic cells (DCs) and macrophages. However, despite its importance for generating and maintaining T cells, thymic function is extremely sensitive to acute damage such as that caused by everyday insults like stress and infection, as well as more profound injuries such as that caused by cytoreductive therapies. Nevertheless, the thymus also has a remarkable capacity to regenerate itself from these acute injuries (1, 2), although until recently this phenomena has been largely unstudied. However, despite its crucial function, the ability of the thymus to facilitate efficient T cell generation deteriorates progressively with age (3, 4); which considerably hampers the ability of the thymus to respond to acute insults. Age-related thymic atrophy and immunosenescence are hallmarks of immune aging and ultimately lead to a constriction of the TCR repertoire (5), decreased naïve T cells and accumulation of memory T cells in the periphery; and chronic low-grade inflammation termed “inflamm-aging,” all conferring insufficient protective responses to pathogens and neoantigens (6–8). Together, these acute and chronic thymic injuries underlie prolonged immune deficiency associated with multiple conditions including the conditioning required for hematopoietic cell transplantation (HCT) and cytoreductive cancer treatments such as radio- and chemo-therapies.
Given the poor outcomes that are associated with deficient T cell immunity, there is a clear clinical need for therapies that can boost thymic function in periods of acute injury or reverse age-related thymic involution. In this review, we will outline what we know about how the thymus is damaged during different modalities of insult and the work that has been done to develop therapeutic strategies to boost thymic function; either ensuing acute insult such as following HCT, or in aged individuals to boost responses to vaccines or immunotherapy (Figure 1).
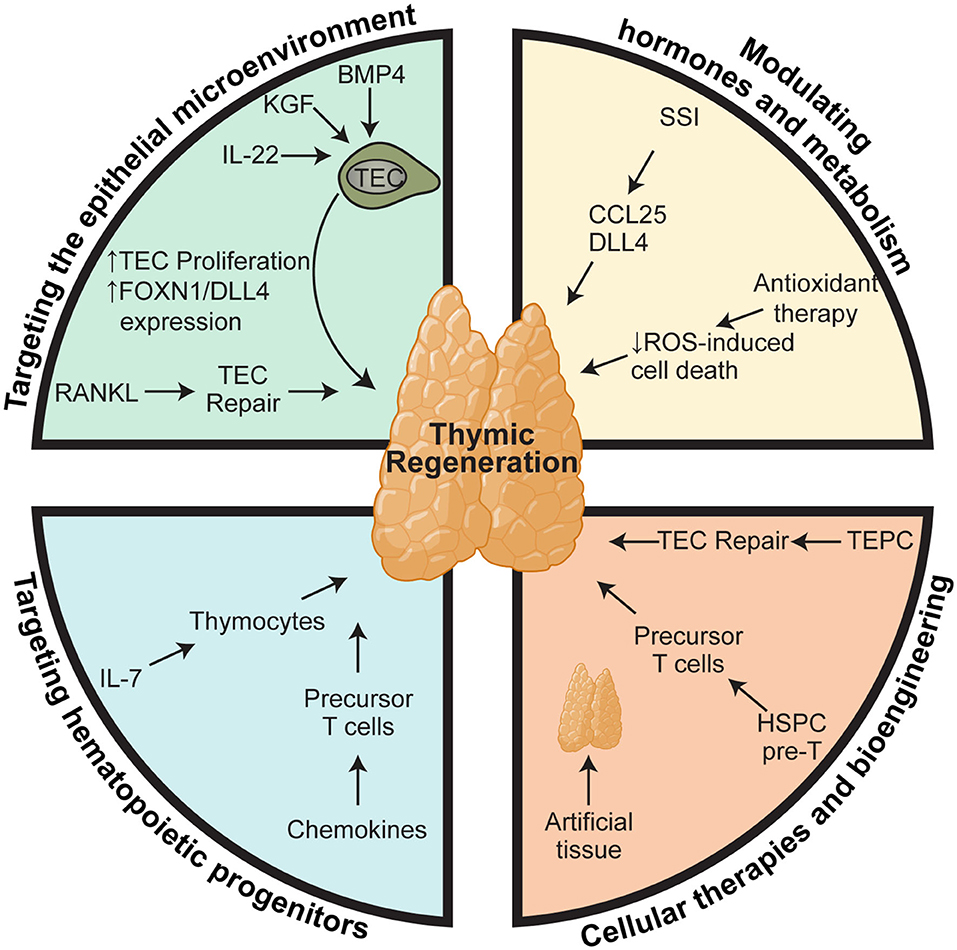
Figure 1. Therapeutic approaches for boosting thymus function. Regenerative therapies to boost thymic function after acute damage or to reverse age-related involution can be broadly stratified into four subgroups based on their cellular or molecular targets: (1) targeting the epithelial microenvironment that supports thymopoiesis; (2) targeting the precursors that provide the supply of developing thymocytes; (3) modulation of hormones and metabolism; and (4) cellular therapies and bioengineering. However, within each of these therapeutic modalities there are key nexus points at which they act mechanistically. One approach relies on stimulating TEC function, such as IL-22, BMP4, KGF, RANKL, SSI, which act by either promoting survival, proliferation, differentiation, or expression of key thymopoietic molecules like DLL4 and KITL. In contrast, approaches such as administration of exogenous IL-7 and chemokine therapies target T cell precursors to promote their migration, proliferation, and differentiation directly. Similarly, many of the bioengineering approaches have sought to recapitulate these same functions such as providing TEC signals or a ready supply of T cell precursors. Elements of the figure were generated using Biorender.com.
Acute Damage and Endogenous Regeneration in the Thymus
Everyday Insults: Stress and Infection
Thymic involution is a routine response to acute insult incurred by multiple triggers including emotional and physical distress, malnutrition, and opportunistic bacterial and viral infections. These can be modeled using approaches such as synthetic corticosteroid treatment, such as dexamethasone (9); nutrient depletion (10); sex steroid treatment (11); and several viral and bacterial infection models. While acute thymic involution results primarily from the loss of cortical thymocytes (12, 13); in cases of chronic atrophy, such as that induced by age-related thymic decline, thymocyte loss is preceded by the loss of Foxn1+ TECs, resulting in the functional decline the TEC compartment and the initiation of age-related thymic atrophy (14, 15).
Continuous export of naïve cells from the thymus, or recent thymic emigrants (RTEs), is essential for effective immune response to acute and chronic infections (16, 17). However, most acute bacterial or viral infections result in acute thymic atrophy, largely due to intense lymphocyte depletion as a result of increased apoptosis of thymocytes and interference with thymocyte development (18–21); which can, at least partially, be attributed to the increased induction of IFNγ from activated CD8+ T cells (22) and Natural Killer (NK)-driven responses (23). Most of the studies looking at viral infection-related thymic function have concentrated on HIV, which leads to several modes of thymic dysfunction including thymic atrophy, reduced thymic output, reduced export of immature thymocytes and disruption of the thymic microenvironment (24–26). Notably, effective response to anti-retroviral therapies was found to depend on competent thymic function, with enhanced function in HIV-infected children with higher basal levels of thymic function (27), in contrast with infected adults who have a reduced thymic output and output decreased CD4+ T cells (28, 29). Moreover, in addition to viral load, quantification of CD4+ RTE has long been known as a suitable marker for HIV disease progression, and a recent study has demonstrated the use of RTE CD4+ T cells as a marker of perinatal HIV infection in infants (30); further strengthening the link between viral infection, efficient thymic function, and therapeutic implications of thymic atrophy.
Although less well studied, bacterial infections also have negative effects on thymic function, primarily by enhancing thymocyte apoptosis. Streptococcus suis infection promotes thymic atrophy specifically by inducing increased activation of pro-apoptotic pathways and apoptotic cell death in thymocytes (31); while Mycobacterium tuberculosis infection also induces thymic atrophy (32), possibly by regulating glucocorticoid levels and in this way impact on homeostatic endocrine-immune communication (33). In fact, glucocorticoids are central to many acute forms of thymic involution (34, 35), directly inducing the apoptosis of CD4+CD8+ DP thymocytes, which preferentially express the glucocorticoid receptor (36).
Metabolic distress due to lack of nutrients, primarily glucose, leads to an attenuation of thymic function, with perturbed thymopoiesis in non-obese diabetic (NOD) mice (37), and reduced thymic atrophy with glucose supplementation in models of mitochondrial dysfunction (38).
Cytoreductive Therapies
Most therapies used in cancer treatments are cytoreductive, such as chemotherapy or radiation. One prominent example of this is that the pre-conditioning regimens required for successful HCT result in profound injury to the thymus, and, in contrast to the relatively early recovery of platelets, erythrocytes, and leukocytes involved in innate immunity, recipients of an HCT experience prolonged post-transplant deficiency in the recovery of adaptive immunity, especially T cell immunity. This delayed T cell reconstitution can last a year or more due to a delay in full recovery of function and T cell repertoire (39–41). Moreover, post-transplant T cell deficiency is associated with an increased risk of infections (39, 40, 42, 43), relapse of malignancy (44), and the development of secondary malignancies (45–50). In fact, infection and relapse account for >50% of mortality following allogeneic-HCT (allo-HCT) (51). T cell reconstitution after transplant is critically dependent on the thymus (39, 41, 47, 52–58) and thymic function pre-transplant can have a significant impact on clinical outcomes. Similarly, damage caused by cytoreductive chemotherapy results in significant thymic damage and can lead to a profoundly delayed recovery of T cells (45, 59). In mouse models of chemotherapy, in addition to almost complete depletion of thymocytes, there was also a severe depletion of TECs, most prominently MHCIIhi TECs (60); likely as they are the most highly proliferating TEC subset (61, 62). Specifically, genotoxic stress caused by chemotherapy leads to senescence in the thymic stromal compartment and the induction of an inflammatory environment in the thymus with endothelial cell secretion of IL-6, generating a chemoresistant niche that is cytoprotectant to certain cancer cells, such as lymphoma and melanoma (63, 64). Accompanying the damage caused by cytoreductive conditioning, the risk of further thymic damage caused by Graft-vs.-Host Disease (GVHD) is significant in the context of an allo-HCT. In fact, the thymus is a particularly sensitive GVHD target organ and presents pathological features even in the context of subclinical GVHD (65–67). Furthermore, there is likely a link between acute GVHD-mediated thymic damage and the formation of chronic GVHD, which may in part be a failure for tolerance induction (68–70).
Strategies of Boosting Thymic Function I: Targeting Non-Hematopoeitic Cells
Given the sensitivity of thymic function to negative stimuli, even everyday insults, a reparative capacity is crucially important for renewal of immune competence. In fact, this capacity of the thymus to regenerate itself has been known for longer than even the immunological function of the tissue was discovered (71, 72); however, until recently the mechanisms underlying this process have been poorly understood. One approach to developing therapies to enhance thymic function has come from exploiting these pathways of endogenous regeneration. Many of these pathways that mediate endogenous regeneration have been found to be effective for exogenous regeneration in periods of acute and profound injury such as that caused by cytoreductive chemotherapy and γ-radiation. Interestingly, many of these pathways specifically target TECs to mediate regeneration.
Interleukin-22
Although the phenomenon of endogenous thymic regeneration has been known for over almost a 100 years, it was not until recently that pathways mediating this regeneration have been described. The first of these was centered around the production of Interleukin-22 (IL-22), a member of the IL-10 family that typically targets non-hematopoietic cells such as epithelial cells and fibroblasts (73). In this regenerative network, acute damage to the thymus (and specifically the depletion of thymocytes) triggers the release of Interleukin-23 (IL-23) from dendritic cells, which induces the production of IL-22 by a group 3 innate lymphoid cells (2, 74–76). Expression of IL-22R in the thymus is lacking on thymocytes but detected in both cTECs and mTECs populations (2). IL-22 acts on TECs to mediate repair but the specific molecular mechanisms are not clear. In addition to the thymus, IL-22 also has a major role in the regeneration of epithelial cells in a diverse range of tissues including gut, lung, skin, breast, and kidney (77). The IL-22 receptor is a type 2 cytokine receptor, and a heterodimer formed of two subunits: IL-10 receptor 1 (IL-10R1) and IL-22 receptor A2 (IL-22RA2) (78). IL-22 receptor binding induces intracellular inactivation of the Jak1/Tyk2 complex which further allows downstream signaling and phosphorylation of Signal Transducer and Activator of Transcription (STATs) 1, 3, and 5, with a preference for STAT3 phosphorylation (79), including in TECs (2) which is consistent with the upregulation of Foxn1 concurrently with IL-22 in the thymus (76), and the importance of STAT3 for TEC maintenance (80). Furthermore, Ruxolitinib, a chemotherapeutic agent that inhibits Jak1 signaling also prevents thymic regeneration after injury (81).
Similar to other tissues (77), IL-22 is not required for the formation or maintenance of the thymus under steady-state physiological conditions; however, it has a key role in driving thymic regeneration after injury, by acting directly on TECs to induce survival and proliferation, potentially via regulation of Foxn1 expression (2, 76). Of note, both the numbers of innate lymphoid cells (ILC) 3 and IL-22 levels were decreased in the thymus and gut of mice with GVHD (74, 82), suggesting that ILCs are a target of alloreactive cells and this depletion likely causes a failure to repair after damage. Due to the diverse pathophysiological roles of IL-22, and the key role in epithelial cell regeneration, modulation of the IL-22-IL22R system is an attractive therapeutic target. In fact, a clinical trial is currently underway to assess the efficacy and safety of administration of IL-22 in combination of systemic corticosteroids to limit the effects of GVHD after hematopoietic stem cell transplantation, with secondary readouts to assess T cell reconstitution (NCT02406651).
Bone Morphogenic Protein 4
Although the role of thymic BMP4 and the endogenous BMP4R antagonist Noggin have been well-described in thymic development (83, 84), only recently has BMP4 been described as a regulator of thymic regeneration after acute injury (85). In the thymus, the source of BMP4 is fibroblasts and endothelial cells (ECs) (85). ECs are a highly radio-resistant population of cells in the thymus (85, 86) and are unique in their ability to induce BMP4 production in response to injury. Importantly, thymic expression of both Bmpr1a and Bmpr2 were identified on TEC populations, with a higher expression of the non-redundant Bmpr2 on cTECs (85); consistent with BMP4-induced expression of FOXN1 and its downstream target delta-like 4 (DLL4) specific to cTECs (85, 87). Although the importance for FOXN1 and DLL4 for the development of TECs and thymocytes, respectively, has been well studied (88, 89), recent findings have also highlighted their importance for thymic regeneration, with intrathymic concentration of DLL4 profoundly impacting on thymic size (90), and reports suggesting that the induction of FOXN1 can counteract age-associated thymic involution (91), acute damage (85), and thymic damage post-transplantation (92). While much of the role of BMP4 seems to be mediated by induction of the FOXN1/DLL4 axis, given the requirement for BMP4 in in vitro differentiation of TECs from multipotent progenitors (93–95), it is possible that an alternate mechanism may be by stimulating bipotent progenitors present in the adult thymus (96–99). Unfortunately, the preclinical studies assessing BMP4 have yet to successfully treat mice with recombinant protein, a therapeutic strategy has been developed that utilizes a technique of allowing for the propagation and expansion of tissue-specific ECs that can be transplanted and mediate regeneration across multiple tissues (85, 100–105). In the thymus, it was found that this therapeutic cellular strategy was dependent on the expression of BMP4 by transplanted ECs (85).
Keratinocyte Growth Factor
Keratinocyte growth factor (KGF, also known as FGF-7), is a fibroblast growth factor and acts as a mitogen targeting TECs, inducing epithelial proliferation in several organs (106–108). In the thymus, KGF is primarily produced by mature αβ+ thymocytes and feeds back to facilitate the proliferation and expansion of mTECs via the activation of p53 and NF-κB pathways (106, 108), preserving the thymic cytoarchitecture. Of note, KGF is also produced by thymic fibroblasts (108). Expression of the KGF receptor, fibroblast growth factor receptor-2 of the IIIb variant (FgfR2IIIb), is limited to TECs (109), and FgfR2-IIIb−/− mice have defective thymopoiesis and reduced cellularity, accounted for specifically by a reduction in TECs (110). KGF modulates TEC functionality by negatively regulating the levels several gatekeepers of positive selection, such as MHC-II invariant chain (Ii), and cathepsin L (CatL) (108), and acts on TECs to produce several cytokines that act directly on thymocytes to facilitate maturation, such as bone morphogenic protein 2 (BMP2), BMP4, Wnt5b, and Wnt10b (109).
Under normal physiological conditions, KGF can enhance thymic cellularity by increasing the number of early thymic progenitors (ETPs) equating to an enhanced number of engraftment niches, and increased TEC proliferation (109). Although it was shown that KGF is not essential in uninjured conditions (110, 111), studies using KGF−/− mice demonstrated the critical role of KGF on thymus function and immune reconstitution after insult, modeled by both syngeneic and allogeneic bone marrow transplant (112). The same study showed that exogenous administration of recombinant KGF enhanced thymopoiesis in young and middle-aged mice, and attenuated the negative effects of acute thymic injury, such as that caused by dexamethasone treatments, cyclophosphamide, and irradiation, highlighting an extremely attractive therapeutic approach to efficiently facilitating immunocompetence after damage. Additionally, exogenous KGF administration improved post-transplantation T cell reconstitution. Furthermore, pre-conditioning with KGF prior to bone marrow transplantation reduces GVHD in mouse models by protecting against epithelial injury (113). However, a recent clinical trial noted a reduction in thymopoiesis in lymphopenic patients following administration of KGF (114), highlighting that more studies need to be carried out before KGF can be used across the board as a therapeutic regulator of thymic regeneration.
RANKL
Receptor activator of nuclear factor kappa-B ligand (RANKL), a member of the Tumor necrosis factor (TNF) superfamily, is implicated in multiple physiological roles in the periphery, primarily in bone biology (115). RANKL has an essential role in the thymus as a potent inducer of epithelial cell differentiation by regulating the key mTEC transcription factor Aire (116). In this way, RANKL governs the maturation of Aire− mTECs to Aire+ mTECs which subsequently present MHC-II peptides that drive the elimination of self-reactive T cells during negative selection (117). RANKL is non-redundant for fetal Aire+ mTEC development, and is produced during development by ILCs, and subsequently by subsets of thymocytes (116, 118–120); although absence of RANKL postnatally can be compensated for by other factors (121). Importantly, RANKL is increased in CD4+ thymocytes and ILCs after injury from the cytoreductive conditioning required prior to HCT, suggesting that RANKL plays a role in endogenous regeneration of the thymus (2, 122).
The prominent role of RANKL in mTEC biology points to the ability of RANKL to modulate thymic regeneration and output. RANKL administration shows an enhancement of thymic function after bone marrow transplantation by boosting TEC subsets, including TEC progenitor niches (122). Moreover, systemic administration of recombinant soluble RANKL (sRANKL) improved thymic medullary architecture in RANKL deficient mice (123), and transgenic mice overexpressing human sRANKL, or mice lacking the soluble RANKL receptor OPG, have an enlarged thymic medulla with increased numbers of Aire+ mTECs (119, 124, 125), highlighting a therapeutic platform for the use of recombinant RANKL as a therapeutic for thymus regeneration.
Strategies of Boosting Thymic Function II: Targeting Hematopoeitic Cells
Given the fact that Cell development requires the input of hematopoietic progenitors, and the fact that the supply of those progenitors is severely limited after acute injury (126, 127), one approach to promoting thymic function is to directly stimulate precursor populations; either in the BM or thymus.
Bone Marrow Progenitors
Several approaches have been attempted that seeks to improve thymic function by stimulating the function of bone marrow hematopoietic progenitors. For instance, preclinical studies have shown that administration of Flt3L can also enhance both thymic dependent and independent T cell reconstitution (128, 129). The effects of Flt3L are predominantly due to an expansion in Flt3+ progenitors in the BM (130). However, increases in T cell reconstitution can be at the expense of B-lymphopoiesis which is significantly declined with exogenous Flt3L administration and, in particular, its effects on the EPLM subset of BM progenitors (131, 132).
Chemokines are key regulators of thymopoiesis, facilitating thymic population and intrathymic cell migration. Importantly, as the thymus does not contain long term progenitors that would enable self-renewal, repopulation of the thymus requires continuous recruitment of T cell progenitors (133). CCL25 (with its receptor CCR9) and CCL21 (with its receptor CCR7) play an important role in thymic colonization with hematopoietic progenitors (134). Interestingly, chemokine therapy, whereby bone marrow progenitors received CCL25 and CCL21 treatment prior to transplant, rescues thymic homing of progenitors which is otherwise suppressed in irradiated mice (86).
Thymic T Cell Precursors
While there are several approaches that have been postulated that target thymic precursor cells the most prominent and developed of these is with the lymphopoietic cytokine interleukin-7 (IL-7). IL-7 has a non-redundant role as a survival molecule in lymphoid tissues in mice and humans, most importantly in the thymus where IL-7 is critical for appropriate thymocyte development. An elegant study by Shitara et al. (135) showed that specific deletion of IL-7 in TECs resulted in the profound reduction in αβ and γδ T cells; and mice deficient for Il-7 have a peripheral loss of γδ T cells, a significant reduction in αβ T cells (136), an absence of innate lymphoid cell subsets (137), and disorganization of lymphoid tissue (138). Moreover, mice lacking Il-7 have a reduced number of DN2 or DN3 cells (139, 140), essentially creating a thymic block and limiting the progression thymocytes to maturity.
IL-7 is produced primarily by non-hematopoietic stromal cells such as TECs and signals by binding to the heterodimeric IL-7 receptor (IL-7R), comprised of IL-7R? (also known as CD127) and the cytokine receptor γ-chain (also known as CD132), and induces an anti-apoptotic pro-survival signaling cascade via the activation of phosphoinositide 3-kinase (PI3K) and the Janus Kinase (JAK)-STAT pathway. The expression of IL-7R? on developing thymocytes occurs in a cyclical pattern, with expression seemingly dependent on the fluctuating need for IL-7 signaling at different stages of thymocyte maturation (141), demonstrated by absence in the earliest T cell progenitors, expression at later DN stages, absence at the DP stage and re-expression in SP thymocytes.
These critical roles of IL-7 in both thymocyte development and in peripheral T cell homeostasis (142) reveal IL-7 as a strong therapeutic candidate to enhance T cell development and activation. Clear evidence exists for the therapeutic potential of IL-7 administration on thymic regeneration, centered on the beneficial effects of IL-7 on increasing progenitor T cells in the thymus and subsequently expanding circulating naïve T cells in viral infection setting (143); however, IL-7 therapy only transiently increased naïve T cells in the aged setting in rhesus macaques, with a more prominent and long lasting effect in the memory T cell compartment (144). Although recombinant IL-7 immunotherapy has had some success in clinical trials for treating septic shock (145), infection (146), and cancer remission (147), along with some early promise in the setting of HCT (148), further studies are necessary to identify a strategy for thymus-dependent IL-7 therapy.
Strategies of Boosting Thymic Function III: Modulation of Hormones and Metabolism
Given the impact of sex steroids on thymic function (149, 150), surgical or chemical ablation of sex steroids has been a well-studied means of boosting thymic function (58, 151). In fact, sex steroid inhibition (SSI) has been shown to promote thymic function in young as well as old mice, and enhances reconstitution after acute insult such as chemotherapy or HCT (60, 152–154). Furthermore, given that SSI is a standard and approved therapy for prostate cancer, thymic function has been assessed in prostate cancer and after HCT and significant improvement observed (155, 156). Although whole organismal ablation of sex steroids will understandably have systemic effects, and the specific means by which SSI improves thymic function are not yet clear, several putative mechanisms have been proposed. In particular, SSI has been shown to (1) promote lymphoid potential and overall function of hematopoietic stem and progenitor cells (2, 152, 157, 158) induce the expression of CCL25 (159), which promotes the importation of hematopoietic progenitors from the circulation (3, 134, 160) induces the expression of the Notch ligand DLL4 (90). Interestingly, KGF was not required for the beneficial effects of SSI on thymus (154), and in fact combination therapies have shown great promise, with the combined KGF administration and androgen blockage with Lupron, revealing reduced epithelial damage and enhanced T cell reconstitution after bone marrow transplant in mice (161). However, it has also been reported that regrowth of the thymus can result in an increase in autoreactive T cells in the periphery, particularly in models of castration, reflecting a lack of synergy between quality and quantity of thymopoiesis (162).
In addition to sex steroids, several other hormones and metabolic components have been implicated in thymic function and their modulation has been shown to improve thymopoiesis, particularly in the aged. Administration of the appetite stimulating hormone Ghrelin led to improved thymic cellularity and thymic output in aged mice (163), and similarly oral zinc supplementation increased thymic cellularity in aged mice (164). Targeting accumulating reactive oxygen species with antioxidants has proven to be beneficial in protecting against age-related thymic atrophy, whereby treatment with the mitochondrial antioxidant SkQ1 reduced age-associated thymic atrophy and increased the number of CD4+ and CD8+ thymocytes (165). Similarly, Leptin, a peptide hormone secreted from adipose tissue, has a protective effect on thymopoiesis in LPS-treated mice and mice that had been starved, primarily due to rescue from metabolic defects including increased corticosterone levels (10, 166).
Strategies of Thymic Regeneration IV: Cell Therapies and Bioengineering Approaches
Hematopoietic Precursors
In addition to the use of growth factors and hormone modulation, several groups have been working on cellular therapies that may enhance thymic function. Given that some of the delay in T cell reconstitution is due to the limited supply of BM-derived progenitors (126), in addition to the time taken for development into a naïve lymphocyte from a transplanted HSC, early studies that concentrated on providing hematopoietic cells found that lymphoid precursors isolated from donor bone marrow could be used to boost thymic function when infused into a recipient at the time of HCT, giving an early boost to T cell development (167). To overcome the limited number of hematopoietic progenitors in BM, an alternate approach of using precursor T cell populations that have been expanded using ex vivo culture systems that use Notch-1 stimulation of hematopoietic precursor cells has been demonstrated (168–173). Using this regimen, adoptive transfer of T cell precursors into lethally irradiated allogeneic HCT recipients caused a significant increase in thymic cellularity and chimerism, as well as enhanced peripheral T and NK cell reconstitution compared with recipients of allogeneic hematopoietic stem cells only (168, 174–178).
Thymic Epithelial Cells
In addition to the use of hematopoietic cells that can act as a boost of T cell precursors, another approach is to identify and isolate populations of thymic epithelial progenitor cells (TEPC). TEPC have been successfully isolated from fetal thymi and induced to generate a new thymus in athymic recipients (179–182), and neonatal TECs, or TECs derived from pluripotent progenitors can promote enhanced thymic function (183, 184). However, while there is evidence of a bipotent TEPC in the postnatal thymus (97–99), their capacity to self-organize as a whole organ like fetal TEPCs is limited. A TEC-like progenitor cell appropriate for this purpose has also been generated by direct conversion of embryonic fibroblasts by induced expression of the TEC transcription factor FOXN1 (185); although the efficacy of this therapy in a regeneration setting has not been investigated.
Artificial Thymic Niches
Finally, there are also several approaches that do not rely on the endogenous thymus at all, but rather concentrate on de novo formation of whole organs ex vivo that can be transplanted into patients as required (186, 187). Although in vivo evidence of their efficacy is still only limited, several approaches have been used to generate artificial thymuses ex vivo, including decellularizing the tissue, which has been performed in several tissues including the thymus, as well as generating synthetic matrices to support T cell development (188–190). Both of these approaches would require some cellular input to generate a functional thymus; namely the thymic epithelial microenvironment would need to be recapitulated with specific factors or, more likely, cells such as TECs derived from multipotent progenitors or reprogrammed, as above. Moreover, a recent report has demonstrated the efficacy of an artificial pre-thymic niche by implanting a scaffold with the Notch ligand DLL4 that acts as an intermediary between the BM and thymus (191). Although these approaches have shown some promise in preclinical mouse studies (192), further advances need to be made before this can be a viable therapeutic option.
Conclusion
Enhancing the regenerative capacity of the thymus and increasing thymic output, together with the expansion of the TCR repertoire has immensely beneficial clinical implications. Although there has been extensive progress in the development of multiple therapies targeting thymic regeneration and output, a deeper understanding of key endogenous molecular mechanisms that govern involution and regeneration of the thymus are needed to further the development of clinically translatable therapies.
Author Contributions
SK and JD wrote, drafted, and edited the manuscript. All authors contributed to the article and approved the submitted version.
Funding
This research was supported by National Institutes of Health award numbers R01-HL145276 (JD), Project 2 of P01-AG052359 (JD), and the NCI Cancer Center Support Grant P30-CA015704. Support was also received from a Scholar Award from the American Society of Hematology (JD), a Scholar Award from the Leukemia and Lymphoma Society (JD). SK was supported by a New investigator Award from the American Society for Transplantation and Cellular Therapy.
Conflict of Interest
The authors have patents and patent applications around potential therapeutics to promote thymus regeneration, including some listed in this review (IL-22 and BMP4) and others as yet unpublished.
References
1. van den Broek T, Delemarre EM, Janssen WJM, Nievelstein RAJ, Broen JC, Tesselaar K, et al. Neonatal thymectomy reveals differentiation and plasticity within human naive T cells. J Clin Invest. (2016) 126:1126–36. doi: 10.1172/JCI84997
2. Dudakov JA, Hanash AM, Jenq RR, Young LF, Ghosh A, Singer NV, et al. Interleukin-22 drives endogenous thymic regeneration in mice. Science. (2012) 336:91–5. doi: 10.1126/science.1218004
3. Chidgey A, Dudakov J, Seach N, Boyd R. Impact of niche aging on thymic regeneration and immune reconstitution. Semin Immunol. (2007) 19:331–40. doi: 10.1016/j.smim.2007.10.006
4. Nikolich-Zugich J. Ageing and life-long maintenance of T-cell subsets in the face of latent persistent infections. Nat Rev Immunol. (2008) 8:512–22. doi: 10.1038/nri2318
6. Thomas R, Wang W, Su DM. Contributions of age-related thymic involution to immunosenescence and inflammaging. Immun Ageing. (2020) 17:2. doi: 10.1186/s12979-020-0173-8
7. Clot J, Charmasson E, Brochier J. Age-dependent changes of human blood lymphocyte subpopulations. Clin Exp Immunol. (1978) 32:346–51.
8. Hulstaert F, Hannet I, Deneys V, Munhyeshuli V, Reichert T, De Bruyere M, et al. Age-related changes in human blood lymphocyte subpopulations. II. Varying kinetics of percentage and absolute count measurements. Clin Immunol Immunopathol. (1994) 70:152–8. doi: 10.1006/clin.1994.1023
9. Wyllie AH. Glucocorticoid-induced thymocyte apoptosis is associated with endogenous endonuclease activation. Nature. (1980) 284:555–6. doi: 10.1038/284555a0
10. Howard JK, Lord GM, Matarese G, Vendetti S, Ghatei MA, Ritter MA, et al. Leptin protects mice from starvation-induced lymphoid atrophy and increases thymic cellularity in ob/ob mice. J Clin Invest. (1999) 104:1051–9. doi: 10.1172/JCI6762
11. Greenstein BD, Fitzpatrick FT, Adcock IM, Kendall MD, Wheeler MJ. Reappearance of the thymus in old rats after orchidectomy: inhibition of regeneration by testosterone. J Endocrinol. (1986) 110:417–22. doi: 10.1677/joe.0.1100417
12. Wang SD, Huang KJ, Lin YS, Lei HY. Sepsis-induced apoptosis of the thymocytes in mice. J Immunol. (1994) 152:5014–21.
13. Khanam S, Sharma S, Pathak S. Lethal and nonlethal murine malarial infections differentially affect apoptosis, proliferation, and CD8 expression on thymic T cells. Parasite Immunol. (2015) 37:349–61. doi: 10.1111/pim.12197
14. Rode I. Martins VC, Küblbeck G, Maltry N, Tessmer C, Rodewald HR. Foxn1 protein expression in the developing, aging, regenerating thymus. J Immunol. (2015) 195:5678–87. doi: 10.4049/jimmunol.1502010
15. Ortman CL, Dittmar KA, Witte PL, Le PT. Molecular characterization of the mouse involuted thymus aberrations in expression of transcription regulators in thymocyte and epithelial compartments. Int Immunol. (2002) 14:813–22. doi: 10.1093/intimm/dxf042
16. Vezys V, Masopust D, Kemball CC, Barber DL, O'Mara LA, Larsen CP, et al. Continuous recruitment of naive T cells contributes to heterogeneity of antiviral CD8 T cells during persistent infection. J Exp Med. (2006) 203:2263–9. doi: 10.1084/jem.20060995
17. Miller NE, Bonczyk JR, Nakayama Y, Suresh M. Role of thymic output in regulating CD8 T-cell homeostasis during acute and chronic viral infection. J Virol. (2005) 79:9419–29. doi: 10.1128/JVI.79.15.9419-9429.2005
18. Vogel AB, Haasbach E, Reiling SJ, Droebner K, Klingel K, Planz O. Highly pathogenic influenza virus infection of the thymus interferes with T lymphocyte development. J Immunol. (2010) 185:4824–34. doi: 10.4049/jimmunol.0903631
19. Savino W. The thymus is a common target organ in infectious diseases. PLoS Pathog. (2006) 2:e62. doi: 10.1371/journal.ppat.0020062
20. Maines TR, Szretter KJ, Perrone L, Belser JA, Bright RA, Zeng H, et al. Pathogenesis of emerging avian influenza viruses in mammals and the host innate immune response. Immunol Rev. (2008) 225:68–84. doi: 10.1111/j.1600-065X.2008.00690.x
21. Cunha BA, Pherez FM, Schoch P. Diagnostic importance of relative lymphopenia as a marker of swine influenza (H1N1) in adults. Clin Infect Dis. (2009) 49:1454–6. doi: 10.1086/644496
22. Liu B, Zhang X, Deng W, Liu J, Li H, Wen M, et al. Severe influenza A(H1N1)pdm09 infection induces thymic atrophy through activating innate CD8(+)CD44(hi) T cells by upregulating IFN-γ. Cell Death Dis. (2014) 5:e1440. doi: 10.1038/cddis.2014.323
23. Duan X, Lu J, Zhou K, Wang J, Wu J, Fu Gao G, et al. NK-cells are involved in thymic atrophy induced by influenza A virus infection. J Gen Virol. (2015) 96:3223–3235. doi: 10.1099/jgv.0.000276
24. Su L, Kaneshima H, Bonyhadi M, Salimi S, Kraft D, Rabin L, et al. HIV-1-induced thymocyte depletion is associated with indirect cytopathogenicity and infection of progenitor cells in vivo. Immunity. (1995) 2:25–36. doi: 10.1016/1074-7613(95)90076-4
25. Calabro ML, Zanotto C, Calderazzo F, Crivellaro C, Del Mistro A, De Rossi A, et al. HIV-1 infection of the thymus. Evidence for a cytopathic and thymotropic viral variant in vivo. AIDS Res Hum Retroviruses. (1995) 11:11–9. doi: 10.1089/aid.1995.11.11
26. Li T, Wu N, Dai Y, Qiu Z, Han Y, Xie J, et al. Reduced thymic output is a major mechanism of immune reconstitution failure in HIV-infected patients after long-term antiretroviral therapy. Clin Infect Dis. (2011) 53:944–51. doi: 10.1093/cid/cir552
27. Sandgaard KS, Lewis J, Adams S, Klein N, Callard R. Antiretroviral therapy increases thymic output in children with HIV. AIDS. (2014) 28:209–14. doi: 10.1097/QAD.0000000000000063
28. Fernandez S, Nolan RC, Price P, Krueger R, Wood C, Cameron D, et al. Thymic function in severely immunodeficient HIV type 1-infected patients receiving stable and effective antiretroviral therapy. AIDS Res Hum Retroviruses. (2006) 22:163–70. doi: 10.1089/aid.2006.22.163
29. Rb-Silva R, Nobrega C, Azevedo C, Athayde E, Canto-Gomes J, Ferreira I, et al. Thymic function as a predictor of immune recovery in chronically HIV-infected patients initiating antiretroviral therapy. Front Immunol. (2019) 10:25. doi: 10.3389/fimmu.2019.00025
30. Zakhour R, Tran DQ, Degaffe G, Bell CS, Donnachie E, Zhang W, et al. Recent thymus emigrant CD4+ T cells predict HIV disease progression in patients with perinatally acquired HIV. Clin Infect Dis. (2016) 62:1029–1035. doi: 10.1093/cid/ciw030
31. Wang S, Lyu C, Duan G, Meng F, Yang Y, Yu Y, et al. Streptococcus suis serotype 2 infection causes host immunomodulation through induction of thymic atrophy. Infect Immun. (2020) 88:e00950–19. doi: 10.1128/IAI.00950-19
32. Reiley WW, Wittmer ST, Ryan LM, Eaton SM, Haynes L, Winslow GM, et al. Maintenance of peripheral T cell responses during Mycobacterium tuberculosis infection. J Immunol. (2012) 189:4451–8. doi: 10.4049/jimmunol.1201153
33. D'Attilio L, Santucci N, Bongiovanni B, Bay ML, Bottasso O. Tuberculosis, the disrupted immune-endocrine response and the potential thymic repercussion as a contributing factor to disease physiopathology. Front Endocrinol. (2018) 9:214. doi: 10.3389/fendo.2018.00214
34. Ashwell JD, Lu FW, Vacchio MS. Glucocorticoids in T cell development and function*. Annu Rev Immunol. (2000) 18:309–45. doi: 10.1146/annurev.immunol.18.1.309
35. Vacchio MS, Ashwell JD. Glucocorticoids and thymocyte development. Semin Immunol. (2000) 12:475–85. doi: 10.1006/smim.2000.0265
36. Purton JF, Monk JA, Liddicoat DR, Kyparissoudis K, Sakkal S, Richardson SJ, et al. Expression of the glucocorticoid receptor from the 1A promoter correlates with T lymphocyte sensitivity to glucocorticoid-induced cell death. J Immunol. (2004) 173:3816–24. doi: 10.4049/jimmunol.173.6.3816
37. Mendes-da-Cruz DA, Lemos JP, Passos GA, Savino W. Abnormal T-cell development in the thymus of non-obese diabetic mice possible relationship with the pathogenesis of type 1 autoimmune diabetes. Front Endocrinol (Lausanne). (2018) 9:381. doi: 10.3389/fendo.2018.00381
38. Missios P, Zhou Y, Guachalla LM, von Figura G, Wegner A, Chakkarappan SR, et al. Glucose substitution prolongs maintenance of energy homeostasis and lifespan of telomere dysfunctional mice. Nat Commun. (2014) 5:4924. doi: 10.1038/ncomms5924
39. Small TN, Papadopoulos EB, Boulad F, Black P, Castro-Malaspina H, Childs BH, et al. Comparison of immune reconstitution after unrelated and related T-cell-depleted bone marrow transplantation: effect of patient age and donor leukocyte infusions. Blood. (1999) 93:467–80. doi: 10.1182/blood.V93.2.467.402k22_467_480
40. Storek J, Joseph A, Espino G, Dawson MA, Douek DC, Sullivan KM, et al. Immunity of patients surviving 20 to 30 years after allogeneic or syngeneic bone marrow transplantation. Blood. (2001) 98:3505–12. doi: 10.1182/blood.V98.13.3505
41. Storek J, Witherspoon RP, Storb R. T cell reconstitution after bone marrow transplantation into adult patients does not resemble T cell development in early life. Bone Marrow Transplant. (1995) 16:413–25.
42. Maury S, Mary JY, Rabian C, Schwarzinger M, Toubert A, Scieux C, et al. Prolonged immune deficiency following allogeneic stem cell transplantation: risk factors and complications in adult patients. Br J Haematol. (2001) 115:630–41. doi: 10.1046/j.1365-2141.2001.03135.x
43. Storek J, Gooley T, Witherspoon RP, Sullivan KM, Storb R. Infectious morbidity in long-term survivors of allogeneic marrow transplantation is associated with low CD4 T cell counts. Am J Hematol. (1997) 54:131–8. doi: 10.1002/(SICI)1096-8652(199702)54:2<131::AID-AJH6>3.0.CO;2-Y
44. Maraninchi D, Gluckman E, Blaise D, Guyotat D, Rio B, Pico JL, et al. Impact of T-cell depletion on outcome of allogeneic bone-marrow transplantation for standard-risk leukaemias. Lancet. (1987) 2:175–8. doi: 10.1016/S0140-6736(87)90763-X
45. Mackall CL, Fleisher TA, Brown MR, Andrich MP, Chen CC, Feuerstein IM, et al. Age, thymopoiesis, and CD4+ T-lymphocyte regeneration after intensive chemotherapy. N Engl J Med. (1995) 332:143–9. doi: 10.1056/NEJM199501193320303
46. Parkman R, Weinberg KI. Immunological reconstitution following bone marrow transplantation. Immunol Rev. (1997) 157:73–8. doi: 10.1111/j.1600-065X.1997.tb00975.x
47. Weinberg K, Annett G, Kashyap A, Lenarsky C, Forman SJ, Parkman R. The effect of thymic function on immunocompetence following bone marrow transplantation. Biol Blood Marrow Transplant. (1995) 1:18–23.
48. Komanduri KV, St John LS, de Lima M, McMannis J, Rosinski S, McNiece I, et al. Delayed immune reconstitution after cord blood transplantation is characterized by impaired thymopoiesis and late memory T-cell skewing. Blood. (2007) 110:4543–51. doi: 10.1182/blood-2007-05-092130
49. Legrand N, Dontje W, van Lent AU, Spits H, Blom B. Human thymus regeneration and T cell reconstitution. Semin Immunol. (2007) 19:280–8. doi: 10.1016/j.smim.2007.10.001
50. Curtis RE, Rowlings PA, Deeg HJ, Shriner DA, Socie G, Travis LB, et al. Solid cancers after bone marrow transplantation. N Engl J Med. (1997) 336:897–904. doi: 10.1056/NEJM199703273361301
51. Jenq RR, van den Brink RM. Allogeneic haematopoietic stem cell transplantation: individualized stem cell and immune therapy of cancer. Nat Rev Cancer. (2010) 10:213–21. doi: 10.1038/nrc2825
52. Small TN, Avigan D, Dupont B, Smith K, Black P, Heller G, et al. Immune reconstitution following T-cell depleted bone marrow transplantation: effect of age and posttransplant graft rejection prophylaxis. Biol Blood Marrow Transplant. (1997) 3:65–75.
53. Atkinson K. Reconstruction of the haemopoietic and immune systems after marrow transplantation. Bone Marrow Transplant. (1990) 5:209–26.
54. Linch DC, Knott LJ, Thomas RM, Harper P, Goldstone AH, Davis EG, et al. T cell regeneration after allogeneic and autologous bone marrow transplantation. Br J Haematol. (1983) 53:451–8. doi: 10.1111/j.1365-2141.1983.tb02046.x
55. Keever CA, Small TN, Flomenberg N, Heller G, Pekle K, Black P, et al. Immune reconstitution following bone marrow transplantation: comparison of recipients of T-cell depleted marrow with recipients of conventional marrow grafts. Blood. (1989) 73:1340–50. doi: 10.1182/blood.V73.5.1340.bloodjournal7351340
56. Forman SJ, Nocker P, Gallagher M, Zaia J, Wright C, Bolen J, et al. Pattern of T cell reconstitution following allogeneic bone marrow transplantation for acute hematological malignancy. Transplantation. (1982) 34:96–8. doi: 10.1097/00007890-198208000-00007
57. Dudakov JA, Perales MA, van den Brink MRM. Chapter 2B: Immune reconstitution following hematopoietic cell transplantation. In: Forman S, Negrin RS, Antin JH, Appelbaum FA, editors. Thomas' Hematopoietic Cell Transplantation. Vol. 1. West Sussex: John Wiley and Sons, Ltd. (2016). p. 160–5.
58. Velardi E, Dudakov JA, Van den Brink MRM. Clinical strategies to enhance thymic recovery after allogeneic hematopoietic stem cell transplantation. Immunol Lett. (2013) 155:31–5. doi: 10.1016/j.imlet.2013.09.016
59. Mackall CL, Fleisher TA, Brown MR, Magrath IT, Shad AT, Horowitz ME. Lymphocyte depletion during treatment with intensive chemotherapy for cancer. Blood. (1994) 84:2221–8. doi: 10.1182/blood.V84.7.2221.bloodjournal8472221
60. Goldberg GL, Dudakov JA, Reiseger JJ, Seach N, Ueno T, Vlahos K, et al. Sex steroid ablation enhances immune reconstitution following cytotoxic antineoplastic therapy in young mice. J Immunol. (2010) 184:6014–24. doi: 10.4049/jimmunol.0802445
61. Gray D, Abramson J, Benoist C, Mathis D. Proliferative arrest and rapid turnover of thymic epithelial cells expressing Aire. J Exp Med. (2007) 204:2521–8. doi: 10.1084/jem.20070795
62. Gray DH, Seach N, Ueno T, Milton MK, Liston A, Lew AM, et al. Developmental kinetics, turnover, and stimulatory capacity of thymic epithelial cells. Blood. (2006) 108:3777–85. doi: 10.1182/blood-2006-02-004531
63. Gilbert LA, Hemann MT. DNA damage-mediated induction of a chemoresistant niche. Cell. (2010) 143:355–66. doi: 10.1016/j.cell.2010.09.043
64. Sizova O, Kuriatnikov D, Liu Y, Su DM. Atrophied thymus, a tumor reservoir for harboring melanoma cells. Mol Cancer Res. (2018) 16:1652–64. doi: 10.1158/1541-7786.MCR-18-0308
65. Krenger W, Hollander GA. The immunopathology of thymic GVHD. Semin Immunopathol. (2008) 30:439–56. doi: 10.1007/s00281-008-0131-6
66. Krenger W, Rossi S, Hollander GA. Apoptosis of thymocytes during acute graft-versus-host disease is independent of glucocorticoids. Transplantation. (2000) 69:2190–3. doi: 10.1097/00007890-200005270-00040
67. Na I-K, Lu SX, Yim NL, Goldberg GL, Tsai J, Rao U, et al. The cytolytic molecules Fas ligand and TRAIL are required for murine thymic graft-versus-host disease. J Clin Invest. (2010) 120:343–56. doi: 10.1172/JCI39395
68. Dertschnig S, Hauri-Hohl MM, Vollmer M, Holländer GA, Krenger W. Impaired thymic expression of tissue-restricted antigens licenses the de novo generation of autoreactive CD4+ T cells in acute GVHD. Blood. (2015) 125:2720–3. doi: 10.1182/blood-2014-08-597245
69. Hollander GA, Widmer B, Burakoff SJ. Loss of normal thymic repertoire selection and persistence of autoreactive T cells in graft vs host disease. J Immunol. (1994) 152:1609–17.
70. Wu T, Young JS, Johnston H, Ni X, Deng R, Racine J, et al. Thymic damage, impaired negative selection, and development of chronic graft-versus-host disease caused by donor CD4+ and CD8+ T cells. J Immunol. (2013) 191:488–99. doi: 10.4049/jimmunol.1300657
71. Jaffe HL. The influence of the suprarenal gland on the thymus: I. Regeneration of the thymus following double suprarenalectomy in the rat. J Exp Med. (1924) 40:325–42. doi: 10.1084/jem.40.3.325
72. Miller JF. Immunological function of the thymus. Lancet. (1961) 2:748–9. doi: 10.1016/S0140-6736(61)90693-6
73. Dumoutier L, Louahed J, Renauld JC. Cloning and characterization of IL-10-related T cell-derived inducible factor (IL-TIF), a novel cytokine structurally related to IL-10 and inducible by IL-9. J Immunol. (2000) 164:1814–9. doi: 10.4049/jimmunol.164.4.1814
74. Dudakov JA, Mertelsmann AM, O'Connor MH, Jenq RR, Velardi E, Young LF, et al. Loss of thymic innate lymphoid cells leads to impaired thymopoiesis in experimental graft-versus-host disease. Blood. (2017) 130:933–42. doi: 10.1182/blood-2017-01-762658
75. Pan B, Wang D, Li L, Shang L, Xia F, Zhang F, et al. Interleukin-22 accelerates thymus regeneration via Stat3/Mcl-1 and decreases chronic graft-versus-host disease in mice after allotransplants. Biol Blood Marrow Transplant. (2019) 25:1911–9. doi: 10.1016/j.bbmt.2019.06.002
76. Pan B, Liu J, Zhang Y, Sun Y, Wu Q, Zhao K, et al. Acute ablation of DP thymocytes induces up-regulation of IL-22 and Foxn1 in TECs. Clin Immunol. (2014) 150:101–8. doi: 10.1016/j.clim.2013.11.002
77. Dudakov JA, Hanash AM, van den Brink MRM. Interleukin-22: immunobiology and pathology. Annu Rev Immunol. (2015) 33:747–85. doi: 10.1146/annurev-immunol-032414-112123
78. Xie MH, Aggarwal S, Ho WH, Foster J, Zhang Z, Stinson J, et al. Interleukin (IL)-22, a novel human cytokine that signals through the interferon receptor-related proteins CRF2-4 and IL-22R. J Biol Chem. (2000) 275:31335–9. doi: 10.1074/jbc.M005304200
79. Lejeune D, Dumoutier L, Constantinescu S, Kruijer W, Schuringa JJ, Renauld JC. Interleukin-22 (IL-22) activates the JAK/STAT, ERK, JNK, and p38 MAP kinase pathways in a rat hepatoma cell line. Pathways that are shared with and distinct from IL-10. J Biol Chem. (2002) 277:33676–82. doi: 10.1074/jbc.M204204200
80. Sano S, Takahama Y, Sugawara T, Kosaka H, Itami S, Yoshikawa K, et al. Stat3 in thymic epithelial cells is essential for postnatal maintenance of thymic architecture and thymocyte survival. Immunity. (2001) 15:261–73. doi: 10.1016/S1074-7613(01)00180-7
81. Li L, Shang L, Gao J, Liu C, Xia F, Xu M, et al. Janus kinase inhibitor ruxolitinib blocks thymic regeneration after acute thymus injury. Biochem Pharmacol. (2020) 171:113712. doi: 10.1016/j.bcp.2019.113712
82. Hanash AM, Dudakov JA, Hua G, O,ÄôConnor MH, Young LF, Singer NV, et al. Interleukin-22 protects intestinal stem cells from immune-mediated tissue damage and regulates sensitivity to graft versus host disease. Immunity. (2012) 37:339–350. doi: 10.1016/j.immuni.2012.05.028
83. Bleul CC, Boehm T. BMP signaling is required for normal thymus development. J Immunol. (2005) 175:5213–21. doi: 10.4049/jimmunol.175.8.5213
84. Patel SR, Gordon J, Mahbub F, Blackburn CC, Manley NR. Bmp4 and Noggin expression during early thymus and parathyroid organogenesis. Gene Expr Patterns. (2006) 6:794–9. doi: 10.1016/j.modgep.2006.01.011
85. Wertheimer T, Velardi E, Tsai J, Cooper K, Xiao S, Kloss CC, et al. Production of BMP4 by endothelial cells is crucial for endogenous thymic regeneration. Sci Immunol. (2018) 3:eaal2736. doi: 10.1126/sciimmunol.aal2736
86. Zhang SL, Wang X, Manna S, Zlotoff DA, Bryson JL, Blazar BR, et al. Chemokine treatment rescues profound T-lineage progenitor homing defect after bone marrow transplant conditioning in mice. Blood. (2014) 124:296–304. doi: 10.1182/blood-2014-01-552794
87. Tsai PT, Lee RA, Wu H. BMP4 acts upstream of FGF in modulating thymic stroma and regulating thymopoiesis. Blood. (2003) 102:3947–53. doi: 10.1182/blood-2003-05-1657
88. Yuan JS, Kousis PC, Suliman S, Visan I, Guidos CJ. Functions of notch signaling in the immune system: consensus and controversies. Annu Rev Immunol. (2010) 28:343–65. doi: 10.1146/annurev.immunol.021908.132719
89. Takahama Y, Ohigashi I, Baik S, Anderson G. Generation of diversity in thymic epithelial cells. Nat Rev Immunol. (2017) 17:295–305. doi: 10.1038/nri.2017.12
90. Velardi E, Tsai JJ, Holland AM, Wertheimer T, Yu VW, Zakrzewski JL, et al. Sex steroid blockade enhances thymopoiesis by modulating Notch signaling. J Exp Med. (2014) 211:2341–9. doi: 10.1084/jem.20131289
91. Zook EC, Krishack PA, Zhang S, Zeleznik-Le NJ, Firulli AB, Witte PL, et al. Overexpression of Foxn1 attenuates age-associated thymic involution and prevents the expansion of peripheral CD4 memory T cells. Blood. (2011) 118:5723–31. doi: 10.1182/blood-2011-03-342097
92. Song Y, Su M, Zhu J, Di W, Liu Y, Hu R, et al. FOXN1 recombinant protein enhances T-cell regeneration after hematopoietic stem cell transplantation in mice. Eur J Immunol. (2016) 46:1518–28. doi: 10.1002/eji.201546196
93. Parent AV, Russ HA, Khan IS, LaFlam TN, Metzger TC, Anderson MS. Generation of functional thymic epithelium from human embryonic stem cells that supports host T cell development. Cell Stem Cell. (2013) 13:219–29. doi: 10.1016/j.stem.2013.04.004
94. Sun X, Xu J, Lu H, Liu W, Miao Z, Sui X, et al. Directed differentiation of human embryonic stem cells into thymic epithelial progenitor-like cells reconstitutes the thymic microenvironment in vivo. Cell Stem Cell. (2013) 13:230–6. doi: 10.1016/j.stem.2013.06.014
95. Soh C-L, Giudice A, Jenny RA, Elliott DA, Hatzistavrou T, Micallef SJ, et al. FOXN1GFP/w Reporter hESCs enable identification of integrin-β4, HLA-DR, and EpCAM as markers of human PSC-Derived FOXN1+ thymic epithelial progenitors. Stem Cell Rep. (2014) 2:925–37. doi: 10.1016/j.stemcr.2014.04.009
96. Dumont-Lagacé M, Gerbe H, Daouda T, Laverdure JP, Brochu S, Lemieux S, et al. Detection of quiescent radioresistant epithelial progenitors in the adult thymus. Front Immunol. (2017) 8:1717. doi: 10.3389/fimmu.2017.01717
97. Bleul CC, Corbeaux T, Reuter A, Fisch P, Monting JS, Boehm T. Formation of a functional thymus initiated by a postnatal epithelial progenitor cell. Nature. (2006) 441:992–6. doi: 10.1038/nature04850
98. Ulyanchenko S, O'Neill KE, Medley T, Farley AM, Vaidya HJ, Cook AM, et al. Identification of a bipotent epithelial progenitor population in the adult thymus. Cell Rep. (2016) 14:2819–32. doi: 10.1016/j.celrep.2016.02.080
99. Wong K, Lister NL, Barsanti M, Lim JM, Hammett MV, Khong DM, et al. Multilineage potential and self-renewal define an epithelial progenitor cell population in the adult thymus. Cell Rep. (2014) 8:1198–209. doi: 10.1016/j.celrep.2014.07.029
100. Ding BS, Nolan DJ, Guo P, Babazadeh AO, Cao Z, Rosenwaks Z, et al. Endothelial-derived angiocrine signals induce and sustain regenerative lung alveolarization. Cell. (2011) 147:539–53. doi: 10.1016/j.cell.2011.10.003
101. Nolan DJ, Ginsberg M, Israely E, Palikuqi B, Poulos MG, James D, et al. Molecular signatures of tissue-specific microvascular endothelial cell heterogeneity in organ maintenance and regeneration. Dev Cell. (2013) 26:204–19. doi: 10.1016/j.devcel.2013.06.017
102. Kobayashi H, Butler JM, O'Donnell R, Kobayashi M, Ding BS, Bonner B, et al. Angiocrine factors from Akt-activated endothelial cells balance self-renewal and differentiation of haematopoietic stem cells. Nat Cell Biol. (2010) 12:1046–56. doi: 10.1038/ncb2108
103. Ding BS, Cao Z, Lis R, Nolan DJ, Guo P, Simons M, et al. Divergent angiocrine signals from vascular niche balance liver regeneration and fibrosis. Nature. (2014) 505:97–102. doi: 10.1038/nature12681
104. Hooper AT, Butler JM, Nolan DJ, Kranz A, Iida K, Kobayashi M, et al. Engraftment and reconstitution of hematopoiesis is dependent on VEGFR2-mediated regeneration of sinusoidal endothelial cells. Cell Stem Cell. (2009) 4:263–74. doi: 10.1016/j.stem.2009.01.006
105. Butler JM, Nolan DJ, Vertes EL, Varnum-Finney B, Kobayashi H, Hooper AT, et al. Endothelial cells are essential for the self-renewal and repopulation of notch-dependent hematopoietic stem cells. Cell Stem Cell. (2010) 6:251–64. doi: 10.1016/j.stem.2010.02.001
106. Rossi SW, Jeker LT, Ueno T, Kuse S, Keller MP, Zuklys S, et al. Keratinocyte growth factor (KGF) enhances postnatal T-cell development via enhancements in proliferation and function of thymic epithelial cells. Blood. (2007) 109:3803–11. doi: 10.1182/blood-2006-10-049767
107. Danilenko DM. Preclinical and early clinical development of keratinocyte growth factor, an epithelial-specific tissue growth factor. Toxicol Pathol. (1999) 27:64–71. doi: 10.1177/019262339902700113
108. Erickson M, Morkowski S, Lehar S, Gillard G, Beers C, Dooley J, et al. Regulation of thymic epithelium by keratinocyte growth factor. Blood. (2002) 100:3269–78. doi: 10.1182/blood-2002-04-1036
109. Rossi S, Blazar BR, Farrell CL, Danilenko DM, Lacey DL, Weinberg KI, et al. Keratinocyte growth factor preserves normal thymopoiesis and thymic microenvironment during experimental graft-versus-host disease. Blood. (2002) 100:682–91. doi: 10.1182/blood.V100.2.682
110. Revest JM, Suniara RK, Kerr K, Owen JJ, Dickson C. Development of the thymus requires signaling through the fibroblast growth factor receptor R2-IIIb. J Immunol. (2001) 167:1954–61. doi: 10.4049/jimmunol.167.4.1954
111. Jenkinson WE, Jenkinson EJ, Anderson G. Differential requirement for mesenchyme in the proliferation and maturation of thymic epithelial progenitors. J Exp Med. (2003) 198:325–32. doi: 10.1084/jem.20022135
112. Alpdogan O, Hubbard VM, Smith OM, Patel N, Lu S, Goldberg GL, et al. Keratinocyte growth factor (KGF) is required for postnatal thymic regeneration. Blood. (2006) 107:2453–60. doi: 10.1182/blood-2005-07-2831
113. Panoskaltsis-Mortari A, Taylor PA, Rubin JS, Uren A, Welniak LA, Murphy WJ, et al. Keratinocyte growth factor facilitates alloengraftment and ameliorates graft-versus-host disease in mice by a mechanism independent of repair of conditioning-induced tissue injury. Blood. (2000) 96:4350–6. doi: 10.1182/blood.V96.13.4350.h8004350_4350_4356
114. Coles AJ, Azzopardi L, Kousin-Ezewu O, Mullay HK, Thompson SA, Jarvis L, et al. Keratinocyte growth factor impairs human thymic recovery from lymphopenia. JCI Insight. (2019) 5. doi: 10.1172/jci.insight.125377
115. Yasuda H, Shima N, Nakagawa N, Yamaguchi K, Kinosaki M, Mochizuki S, et al. Osteoclast differentiation factor is a ligand for osteoprotegerin/osteoclastogenesis-inhibitory factor and is identical to TRANCE/RANKL. Proc Natl Acad Sci U S A. (1998) 95:3597–602. doi: 10.1073/pnas.95.7.3597
116. Rossi SW, Kim MY, Leibbrandt A, Parnell SM, Jenkinson WE, Glanville SH, et al. RANK signals from CD4(+)3(-) inducer cells regulate development of Aire-expressing epithelial cells in the thymic medulla. J Exp Med. (2007) 204:1267–72. doi: 10.1084/jem.20062497
117. Akiyama T, Shinzawa M, Akiyama N. RANKL-RANK interaction in immune regulatory systems. World J Orthop. (2012) 3:142–50. doi: 10.5312/wjo.v3.i9.142
118. Desanti GE, Cowan JE, Baik S, Parnell SM, White AJ, Penninger JM, et al. Developmentally regulated availability of RANKL and CD40 ligand reveals distinct mechanisms of fetal and adult cross-talk in the thymus medulla. J Immunol. (2012) 189:5519–26. doi: 10.4049/jimmunol.1201815
119. Hikosaka Y, Nitta T, Ohigashi I, Yano K, Ishimaru N, Hayashi Y, et al. The cytokine RANKL produced by positively selected thymocytes fosters medullary thymic epithelial cells that express autoimmune regulator. Immunity. (2008) 29:438–50. doi: 10.1016/j.immuni.2008.06.018
120. Sobacchi C, Menale C, Villa A. The RANKL-RANK axis. A bone to thymus round trip. Front Immunol. (2019) 10:629. doi: 10.3389/fimmu.2019.00629
121. Akiyama T, Maeda S, Yamane S, Ogino K, Kasai M, Kajiura F, et al. Dependence of self-tolerance on TRAF6-directed development of thymic stroma. Science. (2005) 308:248–51. doi: 10.1126/science.1105677
122. Lopes N, Vachon H, Marie J, Irla M. Administration of RANKL boosts thymic regeneration upon bone marrow transplantation. EMBO Mol Med. (2017) 9:835–51. doi: 10.15252/emmm.201607176
123. Lo Iacono N, Blair HC, Poliani PL, Marrella V, Ficara F, Cassani B, Facchetti F, et al. Osteopetrosis rescue upon RANKL administration to Rankl−/− mice: a new therapy for human RANKL-dependent ARO. J Bone Miner Res. (2012) 27:2501–10. doi: 10.1002/jbmr.1712
124. Ohigashi I, Nitta T, Lkhagvasuren E, Yasuda H, Takahama Y. Effects of RANKL on the thymic medulla. Eur J Immunol. (2011) 41:1822–7. doi: 10.1002/eji.201141480
125. McCarthy NI, Cowan JE, Nakamura K, Bacon A, Baik S, White AJ, et al. Osteoprotegerin-mediated homeostasis of rank+ thymic epithelial cells does not limit Foxp3+ regulatory T cell development. J Immunol. (2015) 195:2675–82. doi: 10.4049/jimmunol.1501226
126. Zlotoff DA, Zhang SL, De Obaldia ME, Hess PR, Todd SP, Logan TD, et al. Delivery of progenitors to the thymus limits T-lineage reconstitution after bone marrow transplantation. Blood. (2011) 118:1962–70. doi: 10.1182/blood-2010-12-324954
127. Dudakov JA, Van den Brink MRM. Supply-side economics finds the thymus. Blood. (2011) 118:1715–6. doi: 10.1182/blood-2011-06-361337
128. Fry TJ, Sinha M, Milliron M, Chu YW, Kapoor V, Gress RE, et al. Flt3 ligand enhances thymic-dependent and thymic-independent immune reconstitution. Blood. (2004) 104:2794–800. doi: 10.1182/blood-2003-11-3789
129. Kenins L, Gill JW, Boyd RL, Hollander GA, Wodnar-Filipowicz A. Intrathymic expression of Flt3 ligand enhances thymic recovery after irradiation. J Exp Med. (2008) 205:523–31. doi: 10.1084/jem.20072065
130. Wils EJ, Braakman E, Verjans GM, Rombouts EJ, Broers AE, Niesters HG, et al. Flt3 ligand expands lymphoid progenitors prior to recovery of thymopoiesis and accelerates T cell reconstitution after bone marrow transplantation. J Immunol. (2007) 178:3551–7. doi: 10.4049/jimmunol.178.6.3551
131. Balciunaite G, Ceredig R, Massa S, Rolink AG. A B220+ CD117+ CD19– hematopoietic progenitor with potent lymphoid and myeloid developmental potential. Eur J Immunol. (2005) 35:2019–30. doi: 10.1002/eji.200526318
132. Ceredig R, Rauch M, Balciunaite G, Rolink AG. Increasing Flt3L availability alters composition of a novel bone marrow lymphoid progenitor compartment. Blood. (2006) 108:1216–22. doi: 10.1182/blood-2005-10-006643
133. Petrie HT. Cell migration and the control of post-natal T-cell lymphopoiesis in the thymus. Nat Rev Immunol. (2003) 3:859–66. doi: 10.1038/nri1223
134. Zlotoff DA, Sambandam A, Logan TD, Bell JJ, Schwarz BA, Bhandoola A. CCR7 and CCR9 together recruit hematopoietic progenitors to the adult thymus. Blood. (2010) 115:1897–905. doi: 10.1182/blood-2009-08-237784
135. Shitara S, Hara T, Liang B, Wagatsuma K, Zuklys S, Hollander GA, et al. IL-7 produced by thymic epithelial cells plays a major role in the development of thymocytes and TCRgammadelta+ intraepithelial lymphocytes. J Immunol. (2013) 190:6173–9. doi: 10.4049/jimmunol.1202573
136. Maki K, Sunaga S, Komagata Y, Kodaira Y, Mabuchi A, Karasuyama H, et al. Interleukin 7 receptor-deficient mice lack gammadelta T cells. Proc Natl Acad Sci U S A. (1996) 93:7172–7. doi: 10.1073/pnas.93.14.7172
137. Coles MC, Veiga-Fernandes H, Foster KE, Norton T, Pagakis SN, Seddon B, et al. Role of T and NK cells and IL7/IL7r interactions during neonatal maturation of lymph nodes. Proc Natl Acad Sci U S A. (2006) 103:13457–62. doi: 10.1073/pnas.0604183103
138. Yang J, Cornelissen F, Papazian N, Reijmers RM, Llorian M, Cupedo T, et al. IL-7-dependent maintenance of ILC3s is required for normal entry of lymphocytes into lymph nodes. J Exp Med. (2018) 215:1069–77. doi: 10.1084/jem.20170518
139. Peschon JJ, Morrissey PJ, Grabstein KH, Ramsdell FJ, Maraskovsky E, Gliniak BC, et al. Early lymphocyte expansion is severely impaired in interleukin 7 receptor-deficient mice. J Exp Med. (1994) 180:1955–60. doi: 10.1084/jem.180.5.1955
140. U. von Freeden-Jeffry Vieira P, Lucian LA, McNeil T, Burdach SE, Murray R. Lymphopenia in interleukin (IL)-7 gene-deleted mice identifies IL-7 as a nonredundant cytokine. J Exp Med. (1995) 181:1519–26. doi: 10.1084/jem.181.4.1519
141. Sudo T, Nishikawa S, Ohno N, Akiyama N, Tamakoshi M, Yoshida H, et al. Expression and function of the interleukin 7 receptor in murine lymphocytes. Proc Natl Acad Sci U S A. (1993) 90:9125–9. doi: 10.1073/pnas.90.19.9125
142. Fry TJ, Mackall CL. The many faces of IL-7: from lymphopoiesis to peripheral T cell maintenance. J Immunol. (2005) 174:6571–6. doi: 10.4049/jimmunol.174.11.6571
143. Beq S, Nugeyre MT, Fang RHT, Gautier D, Legrand R, Schmitt N, et al. IL-7 induces immunological improvement in SIV-infected rhesus macaques under antiviral therapy. J Immunol. (2006) 176:914–22. doi: 10.4049/jimmunol.176.2.914
144. Okoye AA, Rohankhedkar M, Konfe AL, Abana CO, Reyes MD, Clock JA, et al. Effect of IL-7 therapy on naive and memory T cell homeostasis in aged rhesus macaques. J Immunol. (2015) 195:4292–305. doi: 10.4049/jimmunol.1500609
145. Francois B, Jeannet R, Daix T, Walton AH, Shotwell MS, Unsinger J, et al. Interleukin-7 restores lymphocytes in septic shock: the IRIS-7 randomized clinical trial. JCI Insight. (2018) 3:e98960. doi: 10.1172/jci.insight.98960
146. Shindo Y, Fuchs AG, Davis CG, Eitas T, Unsinger J, Burnham CD, et al. Interleukin 7 immunotherapy improves host immunity and survival in a two-hit model of Pseudomonas aeruginosa pneumonia. J Leukoc Biol. (2017) 101:543–54. doi: 10.1189/jlb.4A1215-581R
147. Sportes C, Hakim FT, Memon SA, Zhang H, Chua KS, Brown MR, et al. Administration of rhIL-7 in humans increases in vivo TCR repertoire diversity by preferential expansion of naive T cell subsets. J Exp Med. (2008) 205:1701–14. doi: 10.1084/jem.20071681
148. Perales MA, Goldberg JD, Yuan J, Koehne G, Lechner L, Papadopoulos EB, et al. Recombinant human interleukin-7 (CYT107) promotes T-cell recovery after allogeneic stem cell transplantation. Blood. (2012) 120:4882–91. doi: 10.1182/blood-2012-06-437236
149. Olsen NJ, Kovacs WJ. Gonadal steroids and immunity. Endocr Rev. (1996) 17:369–84. doi: 10.1210/edrv-17-4-369
150. Olsen NJ, Kovacs WJ. Effects of androgens on T and B lymphocyte development. Immunol Res. (2001) 23:281–8. doi: 10.1385/IR:23:2-3:281
151. Hince M, Sakkal S, Vlahos K, Dudakov J, Boyd R, Chidgey A. The role of sex steroids and gonadectomy in the control of thymic involution. Cell Immunol. (2008) 252:122–38. doi: 10.1016/j.cellimm.2007.10.007
152. Dudakov JA, Goldberg GL, Reiseger JJ, Vlahos K, Chidgey AP, Boyd RL. Sex steroid ablation enhances hematopoietic recovery following cytotoxic antineoplastic therapy in aged mice. J Immunol. (2009) 183:7084–94. doi: 10.4049/jimmunol.0900196
153. Goldberg GL, King CG, Nejat RA, Suh DY, Smith OM, Bretz JC, et al. Luteinizing hormone-releasing hormone enhances T cell recovery following allogeneic bone marrow transplantation. J Immunol. (2009) 182:5846–54. doi: 10.4049/jimmunol.0801458
154. Goldberg GL, Alpdogan O, Muriglan SJ, Hammett MV, Milton MK, Eng JM, et al. Enhanced immune reconstitution by sex steroid ablation following allogeneic hemopoietic stem cell transplantation. J Immunol. (2007) 178:7473–84. doi: 10.4049/jimmunol.178.11.7473
155. Sutherland JS, Goldberg GL, Hammett MV, Uldrich AP, Berzins SP, Heng TS, et al. Activation of thymic regeneration in mice and humans following androgen blockade. J Immunol. (2005) 175:2741–53. doi: 10.4049/jimmunol.175.4.2741
156. Sutherland JS, Spyroglou L, Muirhead JL, Heng TS, Prieto-Hinojosa A, Prince HM, et al. Enhanced immune system regeneration in humans following allogeneic or autologous hemopoietic stem cell transplantation by temporary sex steroid blockade. Clin Cancer Res. (2008) 14:1138–49. doi: 10.1158/1078-0432.CCR-07-1784
157. Dudakov JA, Goldberg GL, Reiseger JJ, Chidgey AP, Boyd RL. Withdrawal of sex steroids reverses age- and chemotherapy-related defects in bone marrow lymphopoiesis. J Immunol. (2009) 182:6247–60. doi: 10.4049/jimmunol.0802446
158. Khong DM, Dudakov JA, Hammett MV, Jurblum MI, Khong SM, Goldberg GL, et al. Enhanced hematopoietic stem cell function mediates immune regeneration following sex steroid blockade. Stem Cell Rep. (2015) 4:445–58. doi: 10.1016/j.stemcr.2015.01.018
159. Williams KM, Lucas PJ, Bare CV, Wang J, Chu YW, Tayler E, et al. CCL25 increases thymopoiesis after androgen withdrawal. Blood. (2008) 112:3255–63. doi: 10.1182/blood-2008-04-153627
160. Krueger A, Willenzon S, Lyszkiewicz M, Kremmer E, Forster R. CC chemokine receptor 7 and 9 double-deficient hematopoietic progenitors are severely impaired in seeding the adult thymus. Blood. (2010) 115:1906–12. doi: 10.1182/blood-2009-07-235721
161. Kelly RM, Highfill SL, Panoskaltsis-Mortari A, Taylor PA, Boyd RL, Holländer GA, et al. Keratinocyte growth factor and androgen blockade work in concert to protect against conditioning regimen-induced thymic epithelial damage and enhance T-cell reconstitution after murine bone marrow transplantation. Blood. (2008) 111:5734–44. doi: 10.1182/blood-2008-01-136531
162. Griffith AV, Fallahi M, Venables T, Petrie HT. Persistent degenerative changes in thymic organ function revealed by an inducible model of organ regrowth. Aging Cell. (2012) 11:169–77. doi: 10.1111/j.1474-9726.2011.00773.x
163. Dixit VD, Yang H, Sun Y, Weeraratna AT, Youm YH, Smith RG, et al. Ghrelin promotes thymopoiesis during aging. J Clin Invest. (2007) 117:2778–90. doi: 10.1172/JCI30248
164. Mocchegiani E, Santarelli L, Muzzioli M, Fabris N. Reversibility of the thymic involution and of age-related peripheral immune dysfunctions by zinc supplementation in old mice. Int J Immunopharmacol. (1995) 17:703–18. doi: 10.1016/0192-0561(95)00059-B
165. Obukhova LA, Skulachev VP, Kolosova NG. Mitochondria-targeted antioxidant SkQ1 inhibits age-dependent involution of the thymus in normal and senescence-prone rats. Aging. (2009) 1:389–401. doi: 10.18632/aging.100043
166. Gruver AL, Sempowski GD. Cytokines, leptin, and stress-induced thymic atrophy. J Leukoc Biol. (2008) 84:915–23. doi: 10.1189/jlb.0108025
167. Arber C, BitMansour A, Sparer TE, Higgins JP, Mocarski ES, Weissman IL, et al. Common lymphoid progenitors rapidly engraft and protect against lethal murine cytomegalovirus infection after hematopoietic stem cell transplantation. Blood. (2003) 102:421–8. doi: 10.1182/blood-2002-12-3834
168. Zakrzewski JL, Kochman AA, Lu SX, Terwey TH, Kim TD, Hubbard VM, et al. Adoptive transfer of T-cell precursors enhances T-cell reconstitution after allogeneic hematopoietic stem cell transplantation. Nat Med. (2006) 12:1039–47. doi: 10.1016/j.bbmt.2005.11.255
169. Schmitt TM, Zuniga-Pflucker JC. Induction of T cell development from hematopoietic progenitor cells by delta-like-1 in vitro. Immunity. (2002) 17:749–56. doi: 10.1016/S1074-7613(02)00474-0
170. Awong G, Herer E, Surh CD, Dick JE, La Motte-Mohs RN, Zuniga-Pflucker JC. Characterization in vitro and engraftment potential in vivo of human progenitor T cells generated from hematopoietic stem cells. Blood. (2009) 114:972–82. doi: 10.1182/blood-2008-10-187013
171. Reimann C, Dal Cortivo L, Hacein-Bey-Abina S, Fischer A, Andre-Schmutz I, Cavazzana-Calvo M. Advances in adoptive immunotherapy to accelerate T-cellular immune reconstitution after HLA-incompatible hematopoietic stem cell transplantation. Immunotherapy. (2010) 2:481–96. doi: 10.2217/imt.10.36
172. Seet CS, He C, Bethune MT, Li S, Chick B, Gschweng EH, et al. Generation of mature T cells from human hematopoietic stem and progenitor cells in artificial thymic organoids. Nat Methods. (2017) 14:521–30. doi: 10.1038/nmeth.4237
173. Shukla S, Langley MA, Singh J, Edgar JM, Mohtashami M, Zuniga-Pflucker JC, et al. Progenitor T-cell differentiation from hematopoietic stem cells using Delta-like-4 and VCAM-1. Nat Methods. (2017) 14:531–8. doi: 10.1038/nmeth.4258
174. Holland AM, Zakrzewski JL, Goldberg GL, Ghosh A, van den Brink MRM. Adoptive precursor cell therapy to enhance immune reconstitution after hematopoietic stem cell transplantation in mouse and man. Semin Immunopathol. (2008) 30:479–87. doi: 10.1007/s00281-008-0138-z
175. Vago L, Oliveira G, Bondanza A, Noviello M, Soldati C, Ghio D, et al. T cell suicide gene therapy prompts thymic renewal in adults after hematopoietic stem cell transplantation. Blood. (2012) 120:1820–30. doi: 10.1182/blood-2012-01-405670
176. Smith MJ, Reichenbach DK, Parker SL, Riddle MJ, Mitchell J, Osum KC, et al. T cell progenitor therapy-facilitated thymopoiesis depends upon thymic input and continued thymic microenvironment interaction. JCI Insight. (2017) 2:e92056. doi: 10.1172/jci.insight.92056
177. Awong G, Singh J, Mohtashami M, Malm M, La Motte-Mohs RN, Benveniste P, et al. Human proT-cells generated in vitro facilitate hematopoietic stem cell-derived T-lymphopoiesis in vivo and restore thymic architecture. Blood. (2013) 122:4210–9. doi: 10.1182/blood-2012-12-472803
178. Zakrzewski JL, Suh D, Markley JC, Smith OM, King C, Goldberg GL, et al. Tumor immunotherapy across MHC barriers using allogeneic T-cell precursors. Nat Biotechnol. (2008) 26:453–61. doi: 10.1038/nbt1395
179. Gill J, Malin M, Hollander GA, Boyd R. Generation of a complete thymic microenvironment by MTS24(+) thymic epithelial cells. Nat Immunol. (2002) 3:635–42. doi: 10.1038/ni812
180. Bennett AR, Farley A, Blair NF, Gordon J, Sharp L, Blackburn CC. Identification and characterization of thymic epithelial progenitor cells. Immunity. (2002) 16:803–14. doi: 10.1016/S1074-7613(02)00321-7
181. Depreter MG, Blair NF, Gaskell TL, Nowell CS, Davern K, Pagliocca A, et al. Identification of Plet-1 as a specific marker of early thymic epithelial progenitor cells. Proc Natl Acad Sci U S A. (2008) 105:961–6. doi: 10.1073/pnas.0711170105
182. Rossi SW, Chidgey AP, Parnell SM, Jenkinson WE, Scott HS, Boyd RL, et al. Redefining epithelial progenitor potential in the developing thymus. Eur J Immunol. (2007) 37:2411–8. doi: 10.1002/eji.200737275
183. Kim M-J, Miller CM, Shadrach JL, Wagers AJ, Serwold T. Young proliferative thymic epithelial cells engraft and function in aging thymuses. J Immunol. (2015) 194:4784–95. doi: 10.4049/jimmunol.1403158
184. Lai L, Cui C, Jin J, Hao Z, Zheng Q, Ying M, et al. Mouse embryonic stem cell-derived thymic epithelial cell progenitors enhance T-cell reconstitution after allogeneic bone marrow transplantation. Blood. (2011) 118:3410–18. doi: 10.1182/blood-2011-03-340794
185. Bredenkamp N, Ulyanchenko S, O'Neill KE, Manley NR, Vaidya HJ, Blackburn CC. An organized and functional thymus generated from FOXN1-reprogrammed fibroblasts. Nat Cell Biol. (2014) 16:902–8. doi: 10.1038/ncb3023
186. Seach N, Layton D, Lim J, Chidgey A, Boyd R. Thymic generation and regeneration: a new paradigm for establishing clinical tolerance of stem cell-based therapies. Curr Opin Biotechnol. (2007) 18:441–7. doi: 10.1016/j.copbio.2007.07.001
187. Tajima A, Pradhan I, Trucco M, Fan Y. Restoration of thymus function with bioengineered thymus organoids. Curr Stem Cell Rep. (2016) 2:128–39. doi: 10.1007/s40778-016-0040-x
188. Ott HC, Clippinger B, Conrad C, Schuetz C, Pomerantseva I, Ikonomou L, et al. Regeneration and orthotopic transplantation of a bioartificial lung. Nat Med. (2010) 16:927–33. doi: 10.1038/nm.2193
189. Ott HC, Matthiesen TS, Goh S-K, Black LD, Kren SM, Netoff TI, et al. Perfusion-decellularized matrix: using nature's platform to engineer a bioartificial heart. Nat Med. (2008) 14:213–21. doi: 10.1038/nm1684
190. Tajima A, Pradhan I, Geng X, Trucco M, Fan Y. Construction of thymus organoids from decellularized thymus scaffolds. Methods Mol Biol. (2019) 1576:33–42. doi: 10.1007/7651_2016_9
191. Shah NJ, Mao AS, Shih TY, Kerr MD, Sharda A, Raimondo TM, et al. An injectable bone marrow-like scaffold enhances T cell immunity after hematopoietic stem cell transplantation. Nat Biotechnol. (2019) 37:293–302. doi: 10.1038/s41587-019-0017-2
Keywords: endogenous thymic regeneration, immune restoration, T cell reconstitution, thymic epithelial cells, BMP4, IL-22
Citation: Kinsella S and Dudakov JA (2020) When the Damage Is Done: Injury and Repair in Thymus Function. Front. Immunol. 11:1745. doi: 10.3389/fimmu.2020.01745
Received: 29 April 2020; Accepted: 30 June 2020;
Published: 12 August 2020.
Edited by:
Avinash Bhandoola, National Institutes of Health (NIH), United StatesReviewed by:
Dong-Ming Su, University of North Texas Health Science Center, United StatesTakeshi Nitta, The University of Tokyo, Japan
Jennifer Elizabeth Cowan, National Institutes of Health (NIH), United States
Copyright © 2020 Kinsella and Dudakov. This is an open-access article distributed under the terms of the Creative Commons Attribution License (CC BY). The use, distribution or reproduction in other forums is permitted, provided the original author(s) and the copyright owner(s) are credited and that the original publication in this journal is cited, in accordance with accepted academic practice. No use, distribution or reproduction is permitted which does not comply with these terms.
*Correspondence: Jarrod A. Dudakov, amR1ZGFrb3YmI3gwMDA0MDtmcmVkaHV0Y2gub3Jn