- 1Division of Immunology and Pathogenesis, Department of Molecular and Cell Biology, University of California, Berkeley, Berkeley, CA, United States
- 2Division of Cell and Developmental Biology, Department of Molecular and Cell Biology, University of California, Berkeley, Berkeley, CA, United States
- 3Division of Biological Sciences, University of California, San Diego, La Jolla, CA, United States
- 4Department of Pharmaceutical Sciences, University of Colorado School of Pharmacy, Aurora, CO, United States
- 5Department of Immunology and Microbiology, University of Colorado School of Medicine, Aurora, CO, United States
The CD8+ T cell response to the intracellular parasite Toxoplasma gondii varies dramatically between mouse strains, resulting in stark differences in control of the parasite. Protection in BALB/c mice can be attributed to an unusually strong and protective MHC-1 Ld-restricted CD8+ T cell response directed against a peptide derived from the parasite antigen GRA6. The MHC-1 Ld molecule has limited peptide binding compared to conventional MHC molecules such as Kb or Db, which correlates with polymorphisms associated with “elite control” of HIV in humans. To investigate the link between the unusual MHC-1 molecule Ld and the generation of “elite controller” CD8+ T cell responses, we compared the GRA6-Ld specific T cell response to the well-studied OVA-Kb specific response, and demonstrated that GRA6-Ld specific T cells are significantly more protective and resistant to exhaustion in chronic T. gondii infection. To further investigate the connection between limited peptide presentation and robust T cell responses, we used CRISPR/Cas9 to generate mice with a point mutation (W97R) in the peptide-binding groove of Ld that results in broader peptide binding. We investigated the effect of this Ld W97R mutation on another robust Ld-restricted response against the IE1 peptide during Murine Cytomegalovirus (MCMV) infection. This mutation leads to an increase in exhaustion markers in the IE1-Ld specific CD8+ T cell response. Our results indicate that limited peptide binding by MHC-1 Ld correlates with the development of robust and protective CD8+ T cell responses that may avoid exhaustion during chronic infection.
Introduction
Individuals differ dramatically in their ability to mount CD8+ T cell responses against intracellular infections, as exemplified by Human Immunodeficiency Virus (HIV) infected individuals who can control the virus without anti-retroviral therapy (1). HIV “elite control” is strongly correlated with polymorphisms in genes encoding the major histocompatibility complex (MHC) class I proteins (2). While the ability of elite control-associated alleles, such as Human Leukocyte Antigen (HLA)-B57 and B27, to induce strong CD8+ responses undoubtedly depends on their ability to bind particular viral peptides, these MHC alleles are also associated with autoimmunity and resistance to other infections, suggesting that more general properties of these molecules may underlie their ability to generate potent CD8+ T cell responses (3–5). Moreover, while potent CD8+ T cell responses are typically characterized by high affinity binding of T cell receptors (TCRs) for pathogen-derived peptides presented by MHC-1 proteins, other factors such as peptide affinity for MHC, T cell precursor frequency, and antigen density are also important (6, 7). Overall, the factors that determine the efficacy of CD8+ T cell responses, and why potent responses are linked to certain MHC-1 alleles, remains poorly understood.
Recent studies have revealed that the level of self-reactivity is another important factor in determining the strength of a T cell response. T cells undergo functional tuning in the thymus based on the strength of the self-pMHC signals they receive (8). CD5 is a key player in the tuning process, and also serves a robust marker of the degree of self-reactivity of naïve T cells (9–12). T cells with higher self-reactivity (CD5high) are generally superior in their responses to foreign antigens, with higher antigen-driven expansion, IL-2 production, and reactivity to cytokines compared to T cells with low self-reactivity (CD5low) (8, 10–14). In one study, a CD5low CD4+ T cell clone displayed resistance to activation-induced cell death and superior expansion during secondary challenge (13). This suggests that high vs. low self-reactivity T cells may be designed to fulfill distinct roles in different infection settings. However, the role of CD8+ T cells with low self-reactivity remains unknown.
While different MHC alleles vary in their ability to bind to particular antigenic peptides, there are indications that other properties of MHC-1 molecules may also play a role in shaping T cell responses. Human HLA-B alleles differ in several properties including their dependence on components of the antigen processing machinery, stability in absence of peptides, peptide loading efficiency, and the broadness of their specificity for peptides (15–18). It has been proposed that, while the majority of MHC-1 molecules are “generalists” able to bind a large set of peptides and produce adequate CD8+ responses to a large number of pathogens, certain MHC-1 molecules with distinctive peptide binding properties may serve as “specialists” that sacrifice broad coverage in exchange for the potential to make potent T cell responses to specific pathogens (17, 18). Notably, most mouse model studies of CD8+ T cell responses have been conducted on the C57BL/6 (B6) background, limiting studies to MHC-Kb and Db restricted responses. Therefore, our knowledge of CD8+ T cells restricted to other mouse MHC-1 molecules is limited.
The CD8+ T cell response to the intracellular parasite Toxoplasma gondii (T. gondii) differs dramatically between mouse strains, and determines whether or not mice eventually succumb to Toxoplasmic encephalitis. This difference is linked to MHC haplotype, with BALB/c (H-2d) mice being resistant and B6 (H-2b) mice being susceptible to infection. Resistance to T. gondii infection in H-2d mice is due to an unusually strong and protective CD8+ T cell response directed against a 10-mer peptide (HF10) derived from the parasite antigen GRA6, presented by the MHC-1 molecule Ld (19, 20). The GRA6-Ld specific CD8+ T cell response is maintained by a proliferative intermediate population that gives rise to large numbers of armed effector T cells throughout chronic infection, and shows no signs of functional exhaustion (21). In contrast, high levels of CD8+ T cell dysfunction have been reported in B6 mice after infection with T. gondii (22, 23). Interestingly, the MHC-1 Ld molecule shares key polymorphic residues with protective HLA-B alleles in HIV “elite controllers” which correlate with restricted peptide binding (17, 24, 25).
Here we investigated the link between the atypical MHC-1 molecule Ld and the generation of “elite controller” CD8+ T cell responses. First, we compared the GRA6-Ld specific CD8+ cell response to the OVA-Kb specific response restricted to the conventional MHC-1 Kb molecule in their ability to protect against T. gondii infection. We also investigated the effect of a point mutation in MHC-1 Ld which leads to broader peptide binding, on another robust Ld-restricted response during chronic Murine Cytomegalovirus (MCMV) infection (26). In both systems, T cells restricted to MHC-1 Ld exhibited resistance to exhaustion compared to T cells restricted to an MHC-1 with broader peptide binding. Overall, our results indicate that limited peptide binding by MHC correlates with the development of CD8+ T cell responses that resist exhaustion, and are consistent with a model in which limited peptide binding by MHC-1 and the corresponding low self-reactivity of CD8+ T cells favor protective responses during chronic infections.
Results
The CD8+ T Cell Response to GRA6-Ld Is Protective and Resistant to Exhaustion
We previously showed that the GRA6-Ld specific CD8+ T cell response to T. gondii infection is immunodominant and highly protective (20, 21). To further evaluate the protective capacity of this T cell response, we performed a direct comparison with the well-characterized and potent CD8+ T cell response to OVA-Kb (27–31). We used TCR transgenic mice expressing rearranged transgenes representative of either the GRA6-Ld (TG6) or OVA-Kb (OT-1) response (21, 32). Both TCRs exhibit similarly strong binding to their respective peptide-MHC complexes (Kd of 0.4–2 micromolar) [(33–35) and data not shown]. We also used a T. gondii strain in which both the model antigen OVA and the endogenous parasite antigen GRA6 are secreted from the parasite via dense granules (36).
We first compared the protective capacity of TG6 and OT-1 T cells during T. gondii infection. We transferred naïve splenic TG6 or OT-1 CD8+ T cells from TCR transgenic H-2b/d mice into T and B cell deficient H-2b/d (B6 Rag2−/− × BALB/c Rag2−/−) mice one day prior to infection, and monitored parasite load and T cell responses 6–8 weeks later (Figure 1A). Mice that received OT-1 T cells had ~30-fold higher parasite load in the brain compared to mice that received TG6 T cells (Figure 1B). T cell numbers in the brains of OT-1 transferred mice were higher than in TG6 transferred mice, implying that reduced protection was not due to reduced T cell expansion or trafficking to the brain Figure 1C. Interestingly, GRA6-Ld specific T cells could be readily detected by tetramer staining amongst donor T cells from OT-1 TCR transgenic mice (data not shown), suggesting that the outgrowth of GRA6-Ld specific T cells using endogenous TCR gene rearrangements could account for some protection in these mice. Consistent with this interpretation, we observed even more striking differences in cyst load, and a change in mouse survival, when splenocytes from TG6 Rag2−/− or OT-1 Rag2−/− mice were used as the donor populations (Supplementary Figure 1).
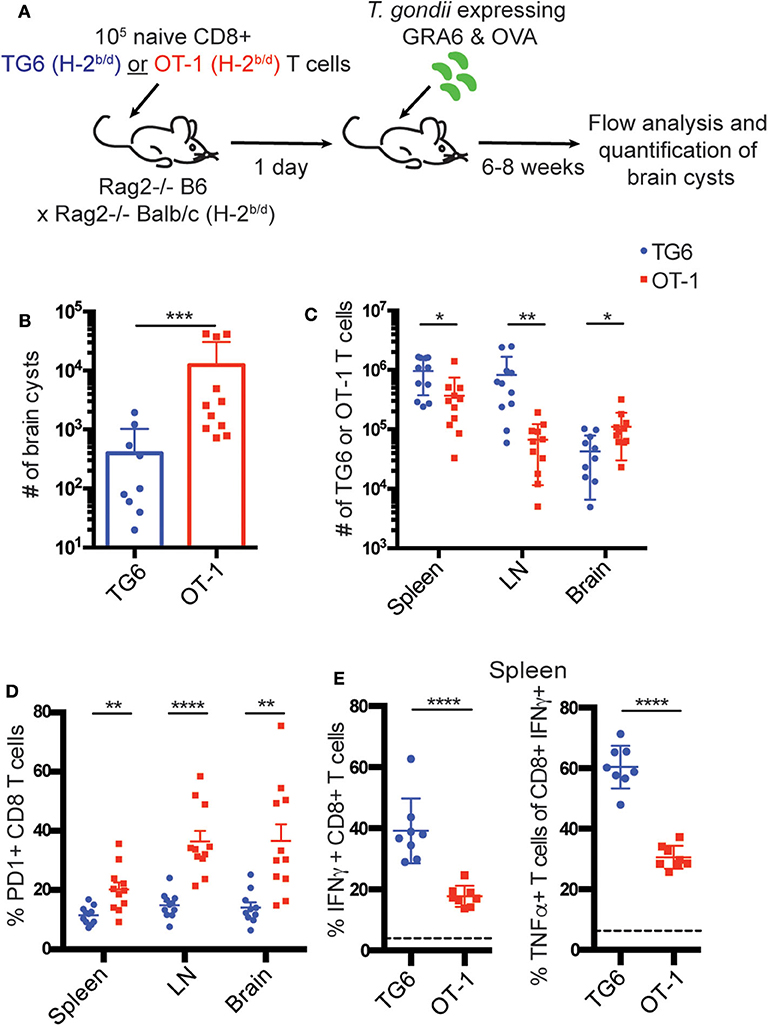
Figure 1. TG6 T cells are more protective and resistant to exhaustion compared to OT-1 T cells. (A) Experimental design to compare the protective capacity of TG6 or OT-1 T cells. B6 Rag2−/− × BALB/c Rag2−/− mice were injected intravenously (i.v.) with 105 naïve CD8+ T cells from TG6 (n = 11) or OT-1 (n = 11) TCR transgenic mice, infected 1 day later with 2,000 T. gondii Pru-OVA parasites, and analyzed at 6–8 weeks post infection. (B) Number of brain cysts in mice transferred with TG6 (avg. 397 cysts) or OT-1 (avg. 12,453 cysts) CD8+ T cells. (C) Numbers of TG6 or OT-1 T cells recovered in the spleen, lymph nodes and brain determined by flow cytometry. (D) Frequency of PD1 expression on TG6 or OT-1 CD8+ T cells. (E) Production of IFNγ and TNFα cytokines by TG6 or OT-1 CD8+ T cells isolated from the spleen (n = 8). Splenocytes were stimulated ex vivo with the HF10 (GRA6) or SIINFEKL (OVA) peptides in culture for 3 h, and cells were analyzed for cytokine production by flow cytometry. Values indicate the percentage of IFNγ+ CD8+ T cells (left) or the percentage of TNFα producing cells among the IFNγ+ CD8+ T cells (right), following stimulation with peptide. Dotted line indicates background cytokine staining in un-stimulated cells from the same mice. Graphs show the summary of two independent experiments. Statistical significance was measured by a Mann-Whitney test in (B) and a t-test in (C–E) (*p < 0.05, **p < 0.01, ***p < 0.001, and ****p < 0.0001).
We also observed that OT-1 T cells recovered from chronically infected mice were functionally impaired compared to TG6 T cells. OT-1 T cells in the spleen, lymph nodes and brain displayed significantly higher expression of the T cell exhaustion marker PD1 (Figure 1D). Additionally, OT-1 T cells in the spleen had a dramatically reduced capacity to produce the inflammatory cytokines IFNγ and TNFα compared to TG6 T cells (Figure 1E).
The increase in T cell exhaustion in mice transferred with OT-1 vs. TG6 T cells could be due differences in parasite load, and/or T cell intrinsic differences. To control for the effects of parasite load, we co-transferred both T cell populations into wild type H-2b/d mice before infection with T. gondii. Again, we observed an increase in PD1 expression on OT-1 T cells compared to TG6 T cells in the spleen and lymph nodes of infected recipient mice (Supplementary Figure 2). This difference was less dramatic than that seen in Rag2−/− mice, likely due to the effective parasite control by the GRA6-Ld specific T cells. These data suggest that T cell-intrinsic differences contribute to the difference in exhaustion between OT-1 and TG6 T cells.
To extend these observations to a polyclonal T cell response, we used fluorochrome-labeled GRA6-Ld and OVA-Kb tetramers to detect endogenous T cell responses in wild type H-2b/d mice infected with OVA-expressing T. gondii. Both T cell populations expanded robustly in the spleen and lymph nodes of infected mice, although the GRA6-Ld specific population exhibited slightly better expansion, especially in the spleen (Figures 2A–C). As expected, given the effective control of parasites in mice harboring the H-2d allele, inhibitory receptors remained generally low on CD8+ T cells in these mice. However, in approximately half of the mice, OVA-Kb specific T cells expressed significantly elevated levels of the inhibitory receptors PD1, Tim3, and Lag3, compared to GRA6-Ld specific T cells in both the spleen and lymph nodes (Figure 2D).
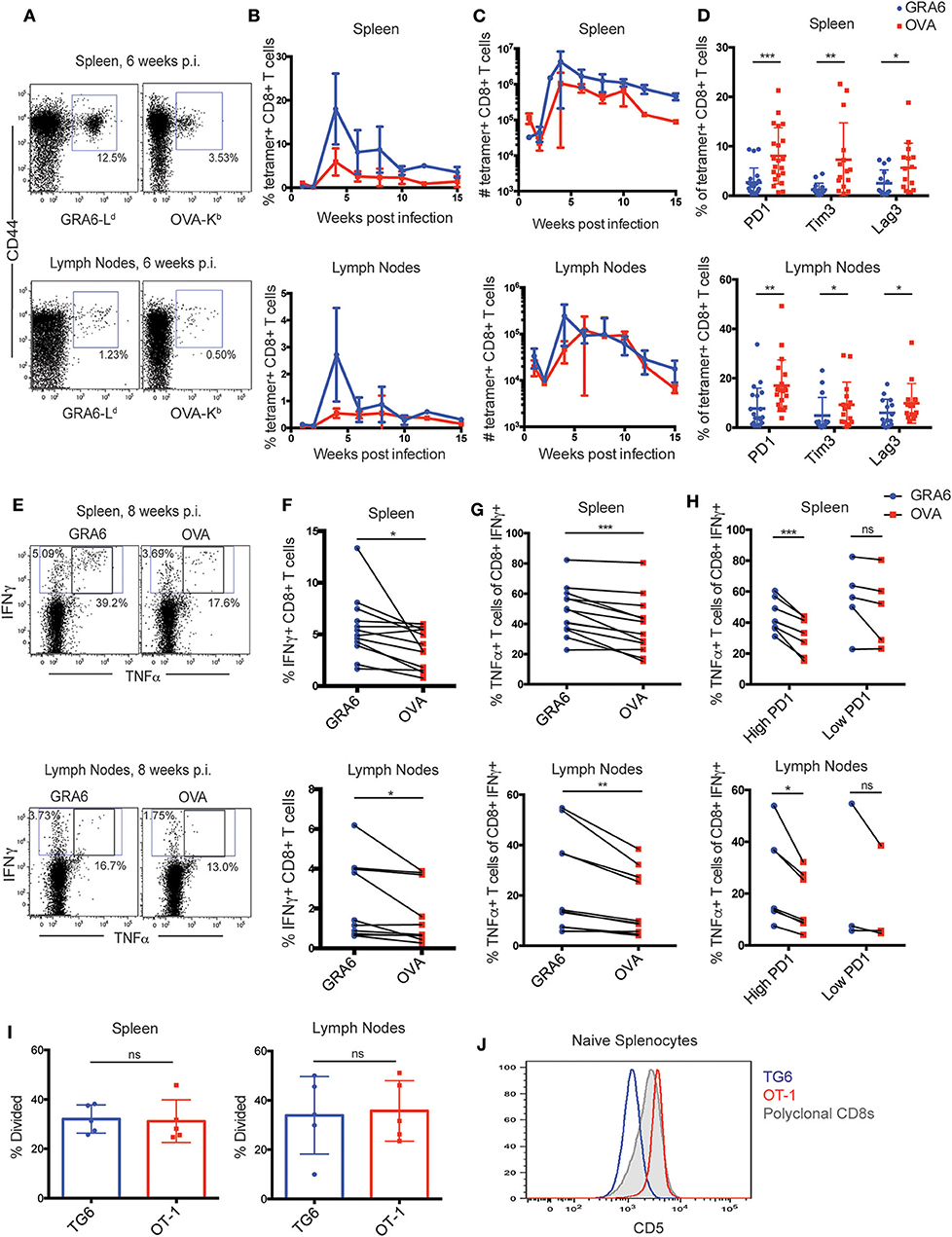
Figure 2. The OVA-Kb specific CD8+ T cell response to T. gondii infection shows signs of functional exhaustion. (A–H) H-2b/d mice were infected intra-peritoneally (i.p.) with 105 T. gondii Pru-OVA parasites and were sacrificed during the chronic infection (3–15 weeks). (A) Representative flow cytometry plots gated on live, singlet, CD8+ T cells and (B) compiled data showing percentage and (C) absolute numbers of GRA6-Ld (blue) and OVA-Kb (red) tetramer+ CD8+ T cells from the spleens and lymph nodes of infected mice. Graphs in (B,C) represent a total of 2–6 mice at each time point [n = 26 (spleen), n = 22 (lymph nodes)], and graphs display mean values with error bars depicting the SEM. (D) Expression of T cell exhaustion markers PD1, Tim3, and Lag3 on GRA6-Ld and OVA-Kb tetramer+ CD8+ T cells in the spleen and lymph nodes [n = 21 (PD1), n = 15 (Tim3/Lag3)]. (E–H) Production of IFNγ and TNFα cytokines by GRA6 or OVA specific T cells isolated from the spleens (n = 13) or lymph nodes (n = 10) of infected mice. Splenocytes or lymph node cells were stimulated ex vivo with the HF10 (GRA6) or SIINFEKL (OVA) peptides at 1 μM in culture for 3 h, and cells were analyzed for cytokine production by flow cytometry. (E) Representative flow cytometry plots of IFNγ and TNFα in CD8+ T cells from the spleen or lymph node. Compiled data showing the percentage of IFNγ+ cells among CD8+ T cells (F), or the percentage of TNFα producing cells among the IFNγ+ CD8+ T cells in the spleen and lymph nodes (G). (H) Data shown in (G) are separated based on PD1 expression in each mouse, with “high PD1” defined as >5% PD1+ in GRA6-Ld or OVA-Kb CD8+ T cells. Data points from the same animal are indicated by connecting lines. Graphs in (D) and (F–H) are the summary of >5 independent experiments, with data from all chronic time points (3–15 weeks) graphed together. (I) GRA6 and OVA antigen presentation in the spleens and lymph nodes of mice infected with T. gondii Pru-OVA. H-2b/d mice were infected i.p. with 105 T. gondii Pru-OVA parasites. At 6 weeks post infection, mice were injected i.v. with naïve CFSE-labeled splenocytes consisting of 106 TG6 and 106 OT-1 CD8+ T cells and analyzed by flow cytometry 2 days post T cell transfer. Transferred T cells were distinguished by a congenic marker together with Vβ2 (TG6) or Vβ5 (OT-1). Proliferation of TG6 and OT-1 CD8+ T cells was assessed by dilution of CFSE in the spleen and lymph nodes. Values are the percent proliferated cells out of the transferred T cell population. Graphs in (I) show data from one experiment (n = 5), representative of 5 independent experiments (see Supplementary Figure 3A). (J) CD5 expression on splenic CD8+ T cells from TG6 (blue) and OT-1 (red) TCR transgenic mice. CD5 expression on polyclonal CD8+ T cells is shown for comparison (gray). Histogram is representative of >5 independent experiments. Statistical significance was measured by a t-test in (D,I) and a paired t-test in (F–H) with data from the same mouse paired (*p < 0.05, **p < 0.01, and ***p < 0.001, ns is not significant).
To confirm that the expression of inhibitory receptors on OVA-Kb specific T cells correlates with reduced functionality of these cells, we analyzed cytokine production by GRA6-Ld and OVA-Kb specific T cells. We re-stimulated splenocytes or lymph node cells from infected mice ex vivo with GRA6 or OVA peptide and measured IFNγ and TNFα production by flow cytometry. Stimulation with the GRA6 peptide resulted in slightly higher proportion of IFNγ producing cells compared to the OVA peptide (Figures 2E,F), which is likely a reflection of the relative size of the antigen-specific population (Figure 2B). To assess the functionality of antigen-specific T cells on a per cell basis, we determined the percentage of IFNγ+ cells that also produced TNFα. This proportion was lower amongst OVA-Kb specific T cells, particularly in those mice with relatively high (>5%) PD1 expression on T cells (Figures 2G,H). Thus, high PD1 expression correlated with reduced cytokine production in OVA-Kb specific T cells, indicating that OVA-Kb specific T cells are less functional compared to GRA6-Ld specific T cells from the same infected mice.
T cell exhaustion can be caused by chronic exposure to high levels of inflammatory signals or antigen (37). Differences in inflammatory signals are unlikely to account for the differences observed between GRA6-Ld and OVA-Kb responses when compared in the same infected mice. Thus, we tested whether differences in antigen levels could account for these differences. While both GRA6 and OVA precursor proteins are secreted by dense granules during infection, differences in the expression levels or antigen processing efficiency could lead to differences in the level of peptide presentation (38–42). To test this possibility, we used an in vivo antigen presentation assay. We transferred equal numbers of naïve CFSE-labeled TG6 and OT-1 CD8+ T cells from TCR transgenic mice into chronically infected mice and assessed their proliferation by CFSE dilution 2 or 3 days after transfer. We observed a similar proportion of proliferating cells in TG6 and OT-1 T cells recovered from the spleen and lymph nodes of infected mice (Figure 2I, Supplementary Figure 3A). As an additional measure of antigen stimulation, we also examined expression of Nur77, a TCR target gene whose induction is proportional to the strength of TCR stimulus (43). We did not observe a significant difference in Nur77 expression between TG6 and OT-1 T cells at 2 or 3 days after transfer, suggesting TG6 and OT-1 T cells are receiving similar amounts of antigenic stimulation (Supplementary Figures 3B,C). The observation that OVA-Kb specific T cells show more exhaustion than GRA6-Ld specific T cells, in spite of exposure to similar levels of antigen and inflammatory signals and a similar affinity for peptide-MHC, suggested that there may be intrinsic differences between these two T cell populations that influence their susceptibility to functional exhaustion.
Limited Peptide Binding by Ld Correlates With the Selection of T Cells With Low Self-Reactivity
We considered that the unusual peptide binding characteristics of MHC-1 Ld might play a role in generating exhaustion-resistant T cell responses. Expression of Ld on the cell surface is 2- to 3-fold lower than that of other conventional MHC molecules, and several groups have reported that this is due to poor binding to endogenous (self) peptide ligands (25, 44, 45). Since Ld binds poorly to self-peptides, we might expect that positive selection on Ld would produce T cells with relatively low self-reactivity. To address this possibility, we examined expression of CD5, a robust surrogate marker for self-reactivity (9–11) on T cells from TG6 transgenic mice. We found that CD5 surface expression on naïve splenocytes from TG6 TCR transgenic mice is relatively low compared to polyclonal CD8+ T cells (Figure 2J), supporting that TG6 T cells are less self-reactive than polyclonal CD8+ T cells. On the other hand, splenocytes from OT-1 TCR transgenic mice, which are selected on the conventional MHC molecule Kb, have relatively high CD5 expression, consistent with previously published literature that OT-1 T cells have high self-reactivity (46) (Figure 2J).
The Ld-Restricted MCMV IE1 Specific CD8+ T Cell Response Has a Proliferative CXCR3+KLRG1+ Intermediate Population
Given the unusual properties of Ld, we considered whether other Ld restricted CD8+ T cell responses might also be particularly robust during chronic infection. The IE1-Ld specific CD8+ T cell response to MCMV is one of a handful of “inflationary” responses that expands during chronic infection and provides strong protection (26, 47). During T. gondii infection, the GRA6-Ld CD8+ T cell response is maintained by an intermediate population (Tint), defined by dual expression of CXCR3 and KLRG1, which is highly proliferative and replenishes a short-lived effector (Teff) population to allow for a long-lasting effector T cell response (21). Interestingly, we observed the same Tint population amongst IE1-Ld specific T cells in mice chronically infected with MCMV, whereas this population was less prominent in CD44+ tetramer- CD8+ T cells from the same mice (Figures 3A,B). The Tint population was also the most proliferative population within IE1-Ld specific T cells, as measured by Ki67 expression (Figure 3C), similar to what we previously observed in the GRA6-Ld specific response (21). Together, these data suggest that two robust Ld-restricted responses may be similarly maintained during chronic infection.
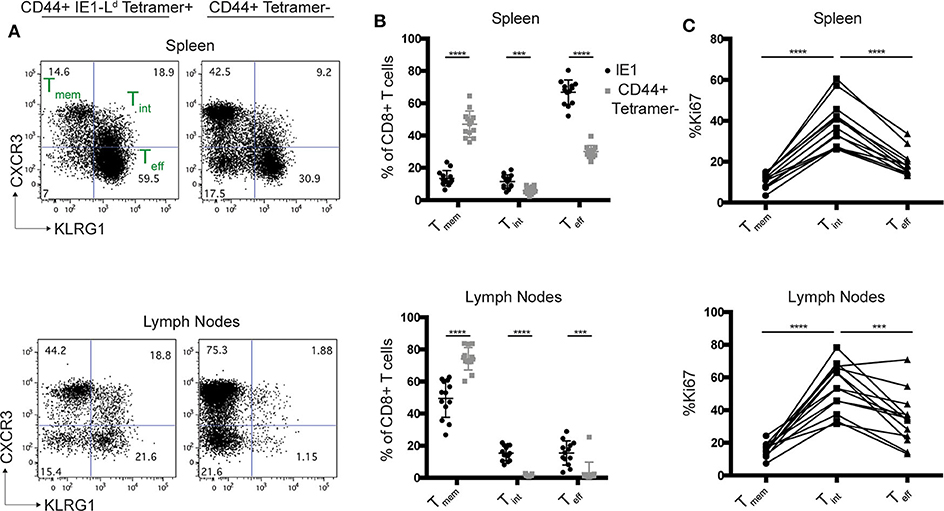
Figure 3. The Ld-restricted MCMV IE1 response has a proliferative intermediate population. H-2d/d mice were infected i.p. with 106 pfu MCMV and cells from the spleens and lymph nodes were analyzed by flow cytometry 11–16 weeks post infection. (A) Representative flow cytometry plots show CXCR3 and KLRG-1 expression within CD44+ IE1-Ld and CD44+ tetramer- CD8+ T cells in the spleens and lymph nodes of MCMV infected mice. (B) Percentage of CXCR3+KLRG1- (Tmem), CXCR3+KLRG-1+ (Tint), and CXCR3-KLRG-1+ (Teff) cells within IE1-Ld (black) and CD44+ tetramer- (gray) CD8+ T cells. (C) Percentage of Ki67+ cells within the indicated subset defined by CXCR3 and KLRG-1 expression in IE1-Ld CD8+ cells in the spleens and lymph nodes. Data points from the same animal are indicated by connecting lines. Graphs are the summary of 3 independent experiments (n = 13). Statistical significance was determined by a t-test in (B) and a one-way ANOVA in (C) ***p < 0.001 and ****p < 0.0001).
An H2-Ld Variant With Broader Peptide Binding Is Associated With Increased Expression of Exhaustion Markers by IE1-Ld Specific T Cells During MCMV Infection
We next wanted to investigate whether limited or broad peptide binding in the context of H2-L could contribute to the exhausted T cell phenotype. Previous studies in cell lines have shown that an HIV-controller associated polymorphism at amino acid position 97 in the mouse H2-L gene impacts the broadness of peptide binding (25, 48). To extend these studies to an in vivo setting, we used CRISPR-EZ (49) to generate mice in which the controller-associated tryptophan at position 97 of Ld is converted to a progressor-associated arginine residue (Ld W97R mutation) (Figure 4A) (24). As expected based on studies in cell lines (25), splenocytes from Ld 97R mice have higher surface expression compared to Ld 97W, consistent with the enhanced binding to self-peptides (Figure 4B). Additionally, while incubation with an antigenic peptide stabilized wild type (97W) Ld, this had little impact on the levels of 97R Ld (Figures 4D,E). In some cell types (e.g., CD4+ T cells) the levels of IE1 peptide-stabilized Ld 97W were equivalent to Ld 97R (Supplementary Figure 4). Thus, limited self-peptide binding by Ld 97W is reversed by the Ld W97R mutation, allowing for higher cell surface expression.
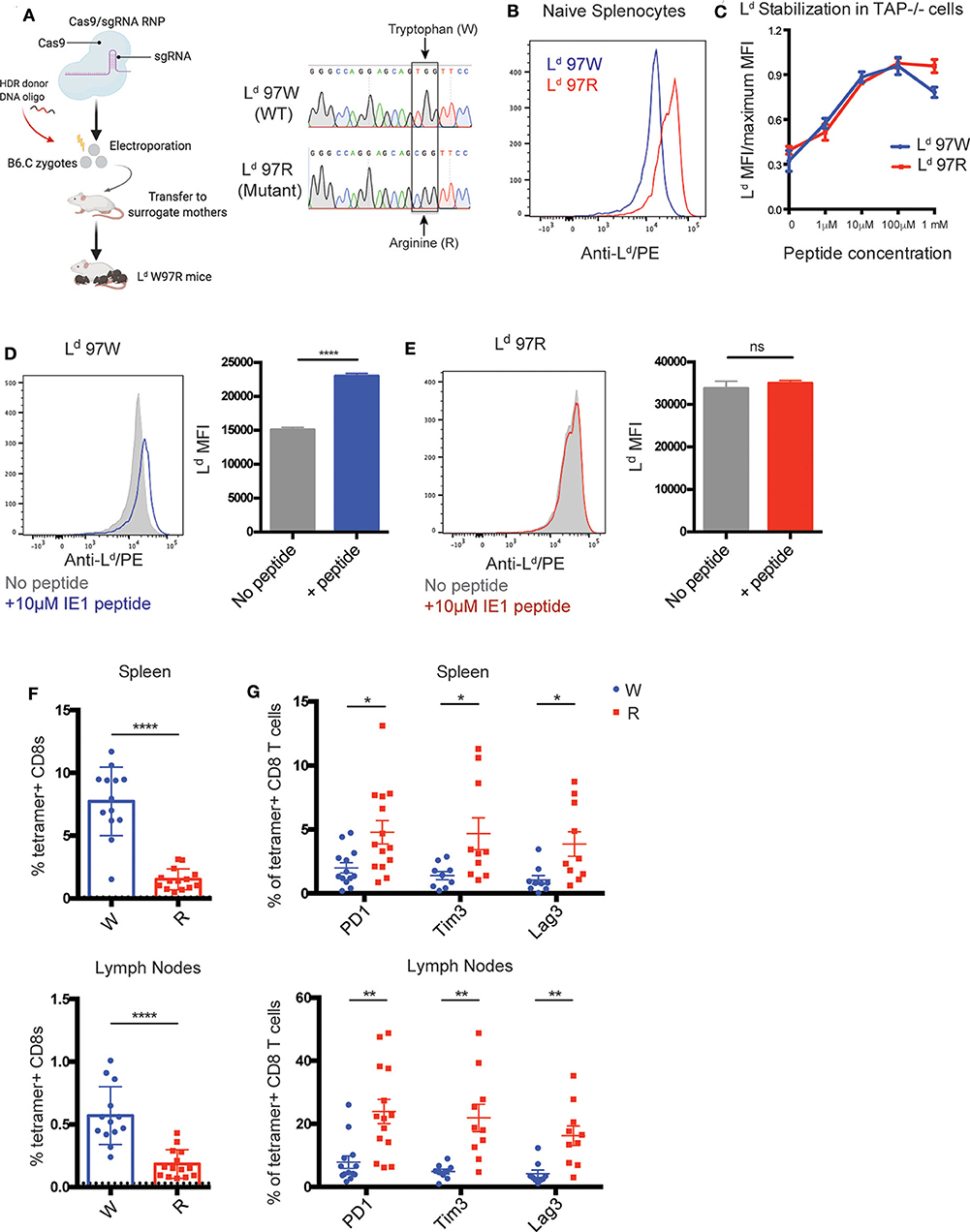
Figure 4. Polymorphism in H2-Ld increases self-peptide binding and correlates with increased expression of exhaustion markers on IE1-Ld specific CD8+ T cells. (A) Generation of Ld W97R mutant mouse by CRISPR-EZ (49, 50). Cas9-sgRNA ribonucleoprotein (RNP) was prepared and incorporated together with the HDR donor DNA oligo and electroporated into mouse zygotes from H-2d (B6.C) mice. A single TC point mutation that converts a tryptophan (TGG) to arginine (CGG) at position 97 in the Ld gene was generated. Sequences show an example of a wild-type B6.C mouse compared to an Ld W97R mutant mouse generated by sequencing DNA from the tail. (B) Surface expression of H2-Ld on naïve splenocytes from Ld W97 (blue) or Ld R97 mice (red). H2-Ld surface expression was measured by flow cytometry using an antibody that binds peptide-bound Ld. Data is representative of 3 independent experiments. (C) IE1 peptide binding strength to H-2Ld. Binding strength was measured using the ability of peptide to stabilize surface Ld expression on the TAP deficient RMA-S cell line expressing either the WT (97W) or mutated (97R) version of H2-Ld. The mean fluorescent intensity (MFI) of peptide-bound H2-Ld was measured after incubating the cells with increasing concentrations of peptide for 3 h. Graph shows representative data from 2 independent experiments. (D,E) Surface expression of H2-Ld on naïve splenocytes from Ld W97 (D) or Ld R97 (E) mice with (color) or without (gray) the addition of 10 μM IE1 peptide. Experimental groups consisted of 3 samples, and data is representative of 2 independent experiments. (F,G) Ld W97 (n = 13) or Ld R97 (n = 14) mice were infected with 106 pfu MCMV and sacrificed at 11–16 weeks post infection. (F) IE1-Ld tetramer+ CD8+ T cells were quantified in the spleen and lymph nodes of infected mice. Dotted line indicates background IE1-Ld tetramer staining in CD4+ T cells. (G) Expression of exhaustion markers PD1, Tim3, and Lag3 on IE1-Ld tetramer+ CD8+ T cells in the spleen and lymph nodes in Ld W97 and R97 mice. Graphs are pooled data from 3 independent experiments. Statistical significance was determined by a t-test (*p < 0.05, **p < 0.01, and ****p < 0.0001).
To further explore the connection between limited peptide binding by MHC and robust CD8+ T cell responses, we investigated the effect of the Ld W97R mutation in the setting of persistent infection. We focused on the MCMV IE1-Ld response, since this peptide, unlike the T. gondii GRA6 peptide, binds well to both 97R and 97W versions of Ld (Figure 4C and data not shown) (25). We infected Ld 97W and Ld 97R mice with MCMV and characterized the IE1-Ld specific CD8+ T cell response during chronic infection (11–16 weeks). IE1-Ld specific T cells expand robustly in Ld 97W mice, while expansion is weaker but still readily detectable in Ld 97R mice (Figure 4F). Furthermore, expression of the exhaustion markers PD1, Tim3, and Lag3 is significantly higher on IE1-Ld specific T cells in Ld 97R mice, in both the spleen and lymph nodes (Figure 4G). Interestingly, expression of exhaustion markers and the activation marker CD69 is markedly higher in the lymph nodes compared to the spleen in both Ld 97W and Ld 97R mice (Figure 4G, Supplementary Figure 5), although the IE1-Ld specific T cell population is smaller in the lymph nodes. This may be a result of higher antigen load in the lymph nodes during MCMV infection (51, 52), possibly pushing IE1-Ld specific T cells in the lymph nodes toward exhaustion and eventual deletion.
MCMV infected Ld 97W and Ld 97R mice have similar viral loads in the salivary glands (Supplementary Figure 6A), indicating that the difference in the IE1-Ld specific response is unlikely to be due to a difference in antigen load. To confirm that the changes in IE1-Ld specific T cells are a direct consequence of the W97R mutation, we examined another immunodominant response restricted to a different MHC-1 molecule (m164-Dd) (47). In contrast to IE1-Ld specific T cells, m164-Dd specific T cells do not exhibit an increase in expression of PD1, CD69, and Nur77 (Supplementary Figures 6B–D). Therefore, these data support our hypothesis that an intrinsic difference between T cells selected on MHCs with limited vs. broad peptide binding may contribute to resistance to exhaustion.
Discussion
While MHC-1 molecules with limited peptide binding are associated with HIV control in humans and T. gondii control in mice, the link between the peptide binding characteristics of MHC and effective CD8+ T cell responses has not been experimentally examined. We used two parallel approaches to address this issue. First, we compared two high affinity CD8+ T cell responses, one restricted to an MHC molecule with limited peptide binding (Ld), and another restricted to a typical MHC-1 molecule (Kb) in the same T. gondii-infected mice. Second, we compared T cell responses to the same viral peptide presented by either Ld, or a single amino acid variant of Ld with broad peptide binding, during chronic MCMV infection. In both cases, T cells restricted to MHC-1 Ld showed fewer signs of exhaustion compared to T cells restricted to MHC-1 with broader binding to self-peptides. These data suggest that MHC molecules with limited peptide binding allow for the development of T cells with the ability to persist during chronic infection without becoming exhausted.
At first glance, the association between limited peptide binding and highly protective CD8+ T cell responses appears contradictory. However, while elite control associated MHC molecules bind less broadly to peptides in general, they nevertheless bind strongly to certain antigenic peptides (17, 25, 53). Importantly, MHC-1 Ld binds poorly to self-peptides, leading to lower cell surface expression and reduced stability of surface peptide-MHC complexes (25, 44, 45). The inability of Ld to bind strongly to self-peptides may explain the unusually low self-reactivity of TG6, a public TCR representative of the CD8+ T cell response to the dominant T. gondii antigen GRA6 (35). These data suggest that the combination of low reactivity to self, coupled with high reactivity to a pathogen-derived peptide may be a key feature of elite controller CD8+ T cell responses.
These considerations support a model in which MHC-1 molecules with restricted peptide binding properties may be considered “specialists,” in contrast to the majority of “generalist” MHC-1 molecules with broad peptide binding (Supplementary Figure 7) (54). During thymic development, generalist MHC molecules select T cells with relatively high self-reactivity, whereas specialist MHC molecules select T cells with relatively low self-reactivity due to weak binding to self-peptides (Supplementary Figures 7A,B). While most infections favor expansion of a broad set of T cells with relatively high self-reactivity, in settings where pathogen-derived peptides are able to bind to, and stabilize, specialist MHC-1 molecules, T cells with high reactivity to a pathogen-derived peptide, but low reactivity to self can expand, giving rise to elite controller CD8+ T cell responses (Supplementary Figures 7C,D).
Why might low self-reactivity favor elite controller T cell responses? As suggested previously (17), T cells selected in the thymus on specialist MHC molecules may experience less stringent negative selection, allowing for the development of unusually high affinity T cells. Specifically, while negative selection primarily functions to remove highly cross-reactive thymocytes (55–57), modeling studies have predicted that limited self-peptide presentation by MHC allows for the selection of T cells with high cross-reactivity (17). In line with this prediction, an MHC-2 molecule presenting a single peptide selects T cells with highly cross-reactive TCRs by taking advantage of high affinity germline interactions, as a result of minimal negative selection (58). Strong germline interactions also contribute to high affinity of the TG6 TCR for GRA6-Ld (35), suggesting that enhanced germline-encoded TCR contacts due to limited negative selection may be a key feature of elite controller CD8+ T cell responses.
In addition to the selection of a distinctive TCR repertoire, specialist MHC molecules may also impact the functional tuning of T cells during their development in the thymus. Recent studies have shown that T cells tune their functional responsiveness based on their degree of self-reactivity, with higher self-reactivity (CD5high) T cells exhibiting greater IL-2 production, more rapid expansion during acute infection, and greater responsiveness to cytokines (8, 10–14). While CD5high T cells are generally superior in their responses to pathogens, in one study CD5low CD4+ T cells were relatively resistant to activation induced cell death, and responded better upon secondary challenge (13). It is tempting to speculate that the reduced responsiveness of CD5low T cells to inflammatory environments may allow them to better avoid exhaustion during chronic infection.
In addition to functional tuning during T cell selection in the thymus, the degree of self-reactivity may also impact the tonic TCR signals that T cells experience in the periphery. Tonic signals have been shown to contribute to inhibitory receptor upregulation and functional hyporesponsiveness in the absence of antigen-specific stimulation (59, 60). Recently, Duong et al., demonstrated that TCRs with higher affinity and higher CD5 expression were more susceptible to functional hyporesponsiveness after chronic exposure to self-peptide MHC (61). Higher tonic signaling may push CD5high T cells toward exhaustion, while CD5low T cells restricted to the specialist MHC-1 molecule Ld may experience lower levels of tonic signaling, contributing to their resistance to exhaustion during chronic infection.
While our model predicts that T cells restricted to specialist MHC molecules have the potential to generate high affinity responses to pathogen-derived peptides, it is unlikely that differences in antigenic stimulation alone can account for the lack of functional exhaustion in the Ld restricted responses examined in this study. TG6 and OT-1 TCRs have similarly high affinity for antigen-MHC [(33–35) and data not shown] and the infected mice in this study displayed similar levels of the two antigens. In addition, the greater expansion of IE1-Ld 97W compared to 97R specific T cells, is consistent with greater antigen stimulation for IE1-Ld 97W, which would be expected to lead to more, not less, exhaustion. Thus, it seems likely that differences in functional tuning and tonic signals contribute, at least in part, to the propensity of T cells to become exhausted.
In addition to the T cell intrinsic factors explored here, it is clear that extrinsic factors such as persistent inflammatory signals and high antigen levels also play a role in driving T cell exhaustion. In the T. gondii-mouse infection model, the highly protective GRA6-Ld specific T cell response may contribute to a feedback mechanism to prevent exhaustion of other T cell responses by keeping antigen and inflammation levels low. Thus, the OVA-Kb specific response exhibits less exhaustion in H-2b/d compared to H-2b mice due the ongoing GRA6-Ld specific response and reduced parasite load. On the other hand, MCMV is well-controlled in mice, even in the absence of a particularly protective T cell response, which may explain the overall low level of T cell exhaustion in this model. Finally, for infections where pathogen levels and inflammation remain high in spite of strong T cell responses, extrinsic factors may be able to overcome the intrinsic resistance of certain T cells to exhaustion. Consistent with this notion, the Ld-restricted CD8+ T cell response to NP peptide during mouse infection with LCMV clone 13 infection exhibited high levels of PD1 and functional exhaustion (data not shown).
In summary, we have used two experimental systems to demonstrate that limited peptide binding by MHC-1 Ld is associated with the generation of exhaustion-resistant CD8+ T cell responses. T cell intrinsic factors linked to the peptide binding properties of restricting MHC molecules may combine with T cell extrinsic factors, such as high antigen levels and persistent inflammatory signals, to determine the outcome of infection. Future studies should directly explore the role of TCR repertoire, T cell tuning, and tonic TCR signaling in T cell susceptibility to exhaustion. Furthermore, extensions to human studies may help to identify additional MHC-1 specialist molecules, and may provide insight into protective responses to HIV and other persistent infections, which would have valuable therapeutic implications.
Materials and Methods
Mice
B6 (C57BL/6), B6 Rag2−/− (B6-Cg-Rag2tm1.1Cgn/J), B6.C (B6.C-H2d/bByJ), BALB/c, BALB/c Rag2−/− [C.B6(Cg)-Rag2 tm1.1Cgn/J], and TCR transgenic mice specific for OVA-Kb [OT-1; C57BL/6-Tg(TcraTcrb)1100Mjb/J] were originally purchased from The Jackson Laboratory (Bar Harbor, ME, USA) and then bred in the AAALAC accredited animal facility at University of California, Berkeley. TCR transgenic mice specific for GRA6-Ld (TG6) were previously generated in our lab as described (21). F1 mice (B6xB6.C or B6 Rag2−/− × BALB/c Rag2−/−) were used for all T. gondii experiments in order to monitor multiple T. gondii epitopes. Ld W97R mutant mice were generated in our lab (described below). Mice were infected at 6–8 weeks old, and sacrificed at indicated times post infection. All mouse procedures were approved by the Animal Care and Use Committee (ACUC) of the University of California.
Infection
For T. gondii infections, C57BL/6 Rag2−/− × BALB/c Rag2−/− (H-2b/d) or intact C57BL/6 × B6.C (H-2b/d) mice were injected intraperitoneally (i.p.) with 2,000 or 105 live tachyzoites of the type II Prugniuad-tomato-OVA strain (Pru-OVA) T. gondii (36), respectively. T. gondii brain cysts were quantified by imaging of homogenized brain samples stained with fluorescein-conjugated Dolichos biflorus agglutinin (Vector Laboratories) to stain the cell wall with a protocol adapted from (62). For MCMV infections, Ld 97W or 97R B6.C (H-2d) mice were injected i.p. with 106 pfu Smith strain MCMV (63). MCMV viral titers were measured using quantitative PCR.
Flow Cytometry
Cells were stained with various fluorophore-labeled antibodies from BD Biosciences (San Diego, CA), Biolegend (San Diego, CA), or Tonbo Biosciences (San Diego, CA) at 4°C. For intracellular staining, cells were fixed and permeabilized using the eBioscience FoxP3 Fixation/permeabilization buffer kit (for Nur77 and Ki67) or the BD Biosciences Cytofix/Cytoperm kit (for cytokines) according to manufacturer protocols. Biotinylated peptide-MHC monomers were obtained from the NIH Tetramer Facility (Atlanta, GA). Tetramers were made by conjugating the biotin-labeled monomers with PE-labeled or APC-labeled streptavidin (Agilent) according to protocols from the NIH tetramer facility. A tetramer with the WR97 mutation in Ld was generated by the NIH Tetramer Facility and used for staining the IE1-Ld tetramer specific populations in 11/14 of the Ld 97R mice. No significant differences were observed when using the Ld 97W vs. 97R tetramer. Data were acquired on BD LSR Fortessa and analyzed using FlowJo software (Ashland, OR). Statistical analyses were conducted using GraphPad Prism (San Diego, CA).
Ex vivo Stimulation With Peptides for Quantification of Cytokine Production
Spleen or lymph node cells from infected mice were plated at a density of 1–3 × 105 cells per well in 96-well round-bottom tissue culture plates and incubated with 1 μM of the indicated peptide (Peptide 2.0 Inc.) in media for 3 h in the presence of protein transport inhibitor cocktail (eBioscience). Cells were then harvested for surface and intracellular antibody staining before flow cytometry analysis.
Antigen Presentation Detection in vivo
Lymph nodes and spleen of TG6 (Ly5.2/5.2) or OT-1 (Ly5.1/5.2) TCR transgenic (H-2b/d) mice were harvested and cells were labeled with the cell proliferation dye CFSE (5 μM). Cells were washed twice with RPMI and twice with PBS. Cells were then counted and mixed in equal proportions prior to transfer. 5 × 105 TG6 and 5 × 105 OT-1 cells were transferred by intravenous tail-vein injection into previously infected mice (Ly5.1/5.1) and proliferation dye dilution was examined 2 or 3 days after transfer by gating on congenic markers to identify each donor population. Relative antigen presentation is expressed as the percentage of donor TG6 or OT-1 cells that diluted the proliferation dye.
Generation of Ld W97R Mutant Mouse by CRISPR/Cas9
Single guide RNAs (sgRNAs) were designed using MIT's CRISPR RNA guide design tool (crispr.mit.edu). A homology directed repair (HDR) donor DNA oligo with the desired mutation was synthesized by Life Technologies Co., with a single TC point mutation that converts a tryptophan (TGG) to arginine (CGG) at position 97 in the Ld gene. Cas9-sgRNA RNP was prepared and incorporated together with the HDR donor DNA oligo and electroporated into mouse zygotes from H-2d (B6.C) mice as described (49, 50). Resulting mice were genotyped by sequencing. Mice were backcrossed to WT B6.C mice for several generations.
MHC-1 Stabilization Assay
RMA-S cells were infected with the VSV.G plasmid expressing the 97W or 97R version of Ld and sorted for Ld-positive cells using the Thy1.1 marker. The MHC-1 stabilization assay was performed as described (64). In brief, RMA-S.Ld (97W or 97R) cells or splenocytes from Ld 97W and 97R mice were incubated at room temperature overnight to increase surface MHC-1 expression. The next day, cells were washed with PBS and plated at 3 × 105 cells per well in a 96-well round-bottom tissue culture plate. Peptides of interest (Peptide 2.0 Inc.) were added to the cells at indicated concentrations. The cells were incubated for 1 h at room temperature and 3 h at 37°C. Cells were stained with the 30-5-7 antibody (specific for conformed, peptide-bound Ld) and a goat anti-mouse IgG phycoerythrin (PE)-conjugated secondary antibody and analyzed by flow cytometry.
Data Availability Statement
All datasets generated for this study are included in the article/Supplementary Material.
Ethics Statement
The animal study was reviewed and approved by Animal Care and Use Committee of University of CA, Berkeley.
Author Contributions
AT designed the research, conducted experiments, analyzed the data, and wrote the manuscript. ER designed the research and wrote the manuscript. DB, SC, KG, YW, and LL-B conducted experiments. AT, LL, and AM contributed to generation of the Ld W97R mutant mouse. EZ and SD designed the research. All authors contributed to the article and approved the submitted version.
Funding
Funding was provided by National Institutes of Health (RO1AI065537 and AI093132 to ER and RO1AI081923 to EZ) and a Cancer Research Coordinating Committee Fellowship (AT).
Conflict of Interest
The authors declare that the research was conducted in the absence of any commercial or financial relationships that could be construed as a potential conflict of interest.
Acknowledgments
We would like to thank Angus Lee and Harman Dhaliwal (UC Berkeley Cancer Research Lab, Gene Targeting Facility) for their help in generating the Ld W97R mutant mice.
Supplementary Material
The Supplementary Material for this article can be found online at: https://www.frontiersin.org/articles/10.3389/fimmu.2020.01464/full#supplementary-material
References
1. Deeks SG, Walker BD. Human immunodeficiency virus controllers: mechanisms of durable virus control in the absence of antiretroviral therapy. Immunity. (2007) 27:406–16. doi: 10.1016/j.immuni.2007.08.010
2. Goulder PJ, Walker BD. HIV and HLA class I: an evolving relationship. Immunity. (2012) 37:426–40. doi: 10.1016/j.immuni.2012.09.005
3. Bowness P. Hla B27. Annu Rev Immunol. (2015) 33:29–48. doi: 10.1146/annurev-immunol-032414-112110
4. Hraber P, Kuiken C, Yusim K. Evidence for human leukocyte antigen heterozygote advantage against hepatitis c virus infection. Hepatology. (2007) 46:1713–21. doi: 10.1002/hep.21889
5. Kim AY, Kuntzen T, Timm J, Nolan BE, Baca MA, Reyor LL, et al. Spontaneous control of HCV is associated with expression of HLA-B *57 and preservation of targeted epitopes. Gastroenterology. (2011) 140:686–96.e1. doi: 10.1053/j.gastro.2010.09.042
6. Tscharke DC, Croft NP, Doherty PC, La Gruta NL. Sizing up the key determinants of the CD8+ T cell response. Nat Rev Immunol. (2015) 15:705–16. doi: 10.1038/nri3905
7. Jenkins MK, Moon JJ. The role of naive T cell precursor frequency and recruitment in dictating immune response magnitude. J Immunol. (2015) 188:4135–40. doi: 10.4049/jimmunol.1102661
8. Hogquist KA, Jameson SC. The self-obsession of T cells: how TCR signaling thresholds affect fate ‘decisions’ and effector function. Nat Immunol. (2014) 15:815–23. doi: 10.1038/ni.2938
9. Azzam HS, DeJarnette JB, Huang K, Emmons R, Park CS, Sommers CL, et al. Fine tuning of TCR signaling by CD5. J Immunol. (2001) 166:5464–72. doi: 10.4049/jimmunol.166.9.5464
10. Persaud SP, Parker CR, Lo WL, Weber KS, Allen PM. Intrinsic CD4+ T cell sensitivity and response to a pathogen are set and sustained by avidity for thymic and peripheral complexes of self peptide and MHC. Nat Immunol. (2014) 15:266–74. doi: 10.1038/ni.2822
11. Fulton RB, Hamilton SE, Xing Y, Best JA, Goldrath AW, Hogquist KA, et al. The TCR's sensitivity to self peptide-MHC dictates the ability of naive CD8(+) T cells to respond to foreign antigens. Nat Immunol. (2015) 16:107–17. doi: 10.1038/ni.3043
12. Mandl JN, Monteiro JP, Vrisekoop N, Germain RN. T cell-positive selection uses self-ligand binding strength to optimize repertoire recognition of foreign antigens. Immunity. (2013) 38:263–74. doi: 10.1016/j.immuni.2012.09.011
13. Weber KS, Li QJ, Persaud SP, Campbell JD, Davis MM, Allen PM. Distinct CD4+ helper T cells involved in primary and secondary responses to infection. Proc Natl Acad Sci USA. (2012) 109:9511–6. doi: 10.1073/pnas.1202408109
14. Cho JH, Kim HO, Ju YJ, Kye YC, Lee GW, Lee SW, et al. CD45-mediated control of TCR tuning in naïve and memory CD8+T cells. Nat Commun. (2016) 7:1–15. doi: 10.1038/ncomms13373
15. Yarzabek B, Zaitouna AJ, Olson E, Silva GN, Geng J, Geretz A, et al. Variations in HLA-B cell surface expression, half-life and extracellular antigen receptivity. ELife. (2018) 7:1–33. doi: 10.7554/eLife.34961
16. Geng J, Zaitouna AJ, Raghavan M. Selected HLA-B allotypes are resistant to inhibition or deficiency of the transporter associated with antigen processing (TAP). PLoS Pathog. (2018) 14:1–22. doi: 10.1371/journal.ppat.1007171
17. Kosmrlj A, Read EL, Qi Y, Allen TM, Altfeld M, Deeks SG, et al. Effects of thymic selection of the T cell repertoire on HLA-class I associated control of HIV infection. Nature. (2010) 465:350–4. doi: 10.1038/nature08997
18. Chappell P, Meziane el K, Harrison M, Magiera Ł, Hermann C, Mears L, et al. Expression levels of Mhc class I molecules are inversely correlated with promiscuity of peptide binding. ELife. (2015) 2015:1–22. doi: 10.7554/eLife.05345
19. Brown CR, Hunter CA, Estes RG, Beckmann E, Forman J, David C, et al. Definitive identification of a gene that confers resistance against toxoplasma cyst burden and encephalitis. Immunology. (1995) 85:419–28.
20. Blanchard N, Gonzalez F, Schaeffer M, Joncker NT, Cheng T, Shastri AJ, et al. Immunodominant, Protective Response to the parasite Toxoplasma gondii requires antigen processing in the endoplasmic reticulum. Nat Immunol. (2008) 9:937–44. doi: 10.1038/ni.1629
21. Chu HH, Chan SW, Gosling JP, Blanchard N, Tsitsiklis A, Lythe G, et al. Continuous effector CD8+ T cell production in a controlled persistent infection is sustained by a proliferative intermediate population. Immunity. (2016) 45:159–71. doi: 10.1016/j.immuni.2016.06.013
22. Bhadra R, Gigley JP, Weiss LM, Khan IA. Control of Toxoplasma reactivation by rescue of dysfunctional CD8+ T-cell response via PD-1-PDL-1 blockade. Proc Natl Acad Sci USA. (2011) 108:9196–201. doi: 10.1073/pnas.1015298108
23. Bhadra R, Gigley JP, Khan IA. PD-1-mediated attrition of polyfunctional memory CD8+ T cells in chronic Toxoplasma infection. J Infect Dis. (2012) 206:125–34. doi: 10.1093/infdis/jis304
24. Pereyra F, Jia X, McLaren PJ, Kadie CM, Carlson JM, Heckerman D, et al. The major genetic determinants of HIV-1 control affect HLA Class I peptide presentation. Science. (2010) 330:1551–7. doi: 10.1126/science.1195271
25. Narayanan S, Kranz DM. The same major histocompatibility complex polymorphism involved in control of HIV influences peptide binding in the mouse H-2Ld system. J Biol Chem. (2013) 288:31784–94. doi: 10.1074/jbc.M113.478412
26. Pahl-Seibert MF, Juelch M, Podlech J, Thomas D, Deegen P, Reddehase MJ, et al. Highly protective in vivo function of cytomegalovirus IE1 epitope-specific memory CD8 T cells purified by T-cell receptor-based cell sorting. J Virol. (2005) 79:5400–13. doi: 10.1128/JVI.79.9.5400-5413.2005
27. Pope C, Kim SK, Marzo A, Masopust D, Williams K, Jiang J, et al. Organ-specific regulation of the CD8 T cell response to listeria monocytogenes infection. J Immunol. (2001) 166:3402–9. doi: 10.4049/jimmunol.166.5.3402
28. Kumar S, Tarleton RL. Antigen-specific Th1 but not Th2 cells provide protection from lethal Trypanosoma cruzi infection in mice. J Immunol. (2001) 166:4596–603. doi: 10.4049/jimmunol.166.7.4596
29. Radford KJ, Higgins DE, Pasquini S, Cheadle EJ, Carta L, Jackson AM, et al. A recombinant E. coli vaccine to promote MHC Class I-dependent antigen presentation: application to cancer immunotherapy Gene Ther. (2002) 9:1455–63. doi: 10.1038/sj.gt.3301812
30. Braaten DC, Sparks-Thissen RL, Kreher S, Speck SH, Virgin HW. An optimized CD8+ T-cell response controls productive and latent gammaherpesvirus infection. J Virol. (2005) 79:2573–83. doi: 10.1128/JVI.79.4.2573-2583.2005
31. Miyakoda M, Kimura D, Honma K, Kimura K, Yuda M, Yui K. Development of memory CD8 + T cells and their recall responses during blood-stage infection with plasmodium berghei ANKA. J Immunol. (2012) 189:4396–404. doi: 10.4049/jimmunol.1200781
32. Hogquist KA, Jameson SC, Heath WR, Howard JL, Bevan MJ, Carbone FR. T cell receptor antagonist peptides induce positive selection. Cell. (1994) 76:17–27. doi: 10.1016/0092-8674(94)90169-4
33. Alam SM, Davies GM, Lin CM, Zal T, Nasholds W, Jameson SC, et al. Qualitative and quantitative differences in T cell receptor binding of agonist and antagonist ligands. Immunity. (1999) 10:227–37. doi: 10.1016/S1074-7613(00)80023-0
34. Rosette C, Werlen G, Daniels MA, Holman PO, Alam SM, Travers PJ, et al. The impact of duration versus extent of TCR occupancy on T cell activation. Immunity. (2001) 15:59–70. doi: 10.1016/S1074-76130100173-X
35. Wang Y, Tsitsiklis A, Gao W, Chu HH, Zhang Y, Li W, et al. Novel Vb specific germline contacts shape an elite controller T cell response. bioRxiv. (2020). doi: 10.1101/2020.05.31.102566
36. Schaeffer M, Han SJ, Chtanova T, van Dooren GG, Herzmark P, Chen Y, et al. Dynamic imaging of T cell-parasite interactions in the brains of mice chronically infected with Toxoplasma gondii. J Immunol. (2009) 182:6379–93. doi: 10.4049/jimmunol.0804307
37. Wherry EJ, Kurachi M. Molecular and cellular insights into T cell exhaustion. Nat Rev Immunol. (2015) 15:486–99. doi: 10.1038/nri3862
38. Gubbels MJ, Striepen B, Shastri N, Turkoz M, Robey EA. Class I major histocompatibility complex presentation of antigens that escape from the parasitophorous vacuole of Toxoplasma gondii. Infect Immun. (2005) 73:703–11. doi: 10.1128/IAI.73.2.703-711.2005
39. Gregg B, Dzierszinski F, Tait E, Jordan KA, Hunter CA, Roos DS. Subcellular antigen location influences T-cell activation during acute infection with Toxoplasma gondii. PLoS ONE. (2011) 6:e22936. doi: 10.1371/journal.pone.0022936
40. Lopez J, Bittame A, Massera C, Vasseur V, Effantin G, Valat A, et al. Intravacuolar membranes regulate CD8 T cell recognition of membrane-bound Toxoplasma gondii protective antigen. Cell Rep. (2015) 13:2273–86. doi: 10.1016/j.celrep.2015.11.001
41. Buaillon C, Guerrero NA, Cebrian I, Blanié S, Lopez J, Bassot E, et al. MHC I presentation of Toxoplasma gondii immunodominant antigen does not require Sec22b and is regulated by antigen orientation at the vacuole membrane. Eur J Immunol. (2017) 47:1160–70. doi: 10.1002/eji.201646859
42. Tsitsiklis A, Bangs DJ, Robey EA. CD8+ T cell responses to Toxoplasma gondii: lessons from a successful parasite. Trends Parasitol. (2019) 35:887–98. doi: 10.1016/j.pt.2019.08.005
43. Moran AE, Holzapfel KL, Xing Y, Cunningham NR, Maltzman JS, Punt J, et al. T cell receptor signal strength in treg and INKT cell development demonstrated by a novel fluorescent reporter mouse. J Exp Med. (2011) 208:1279–89. doi: 10.1084/jem.20110308
44. Potter TA, Hansen TH, Habbersett R, Ozato K, Ahmed A. Flow microfluorometric analysis of H-2L expression. J Immunol. (1981) 127:580–4.
45. Lie WR, Myers NB, Connolly JM, Gorka J, Lee DR, Hansen TH. The specific binding of peptide ligand to Ld class I major histocompatibility complex molecules determines their antigenic structure. J Exp Med. (1991) 173:449–59. doi: 10.1084/jem.173.2.449
46. Ge Q, Bai A, Jones B, Eisen HN, Chen J. Competition for self-peptide-MHC complexes and cytokines between naive and memory CD8+ T cells expressing the same or different T cell receptors. Proc Natl Acad Sci USA. (2004) 101:3041–6. doi: 10.1073/pnas.0307339101
47. Holtappels R, Thomas D, Podlech J, Reddehase MJ. Two antigenic peptides from genes M123 and M164 of murine cytomegalovirus quantitatively dominate CD8 T-cell memory in the H-2d haplotype. J Virol. (2002) 76:151–64. doi: 10.1128/JVI.76.1.151-164.2002
48. Smith RA, Myers NB, Robinson M, Hansen TH, Lee DR. Polymorphism at position 97 in MHC class I molecules affects peptide specificity, cell surface stability, and affinity for beta2-microglobulin. J Immunol. (2002) 169:3105–11. doi: 10.4049/jimmunol.169.6.3105
49. Chen S, Lee B, Lee AY, Modzelewski AJ, He L. Highly efficient mouse genome editing by CRISPR ribonucleoprotein electroporation of zygotes. J Biol Chem. (2016) 291:14457–67. doi: 10.1074/jbc.M116.733154
50. Modzelewski AJ, Chen S, Willis BJ, Lloyd KCK, Wood JA, He L. Efficient mouse genome engineering by CRISPR-EZ Technology. Nat Protoc. (2018) 13:1253–74. doi: 10.1038/nprot.2018.012
51. Karrer U, Sierro S, Wagner M, Oxenius A, Hengel H, Koszinowski UH, et al. Memory inflation: continuous accumulation of antiviral CD8+ T cells over time. J Immunol. (2003) 170:2022–9. doi: 10.4049/jimmunol.170.4.2022
52. Torti N, Walton SM, Brocker T, Rülicke T, Oxenius A. Non-hematopoietic cells in lymph nodes drive memory CD8 T cell inflation during murine cytomegalovirus infection. PLoS Pathog. (2011) 7:e1002313. doi: 10.1371/journal.ppat.1002313
53. Schneidewind A, Brockman MA, Sidney J, Wang YE, Chen H, Suscovich TJ, et al. Structural and functional constraints limit options for cytotoxic T-lymphocyte escape in the immunodominant HLA-B27-restricted epitope in human immunodeficiency virus type 1 capsid. J Virol. (2008) 82:5594–605. doi: 10.1128/JVI.02356-07
54. Kaufman J. Generalists and specialists: a new view of how MHC class I molecules fight infectious pathogens. Trends Immunol. (2018) 39:367–79. doi: 10.1016/j.it.2018.01.001
55. Huseby ES, White J, Crawford F, Vass T, Becker D, Pinilla C, et al. How the T cell repertoire becomes peptide and MHC specific. Cell. (2005) 122:247–60. doi: 10.1016/j.cell.2005.05.013
56. Huseby ES, Crawford F, White J, Marrack P, Kappler JW. Interface-disrupting amino acids establish specificity between T cell receptors and complexes of major histocompatibility complex and peptide. Nat Immunol. (2006) 7:1191–9. doi: 10.1038/ni1401
57. McDonald BD, Bunker JJ, Erickson SA, Oh-Hora M, Bendelac A. Crossreactive? β T cell receptors are the predominant targets of thymocyte negative selection. Immunity. (2015) 43:859–69. doi: 10.1016/j.immuni.2015.09.009
58. Dai S, Huseby ES, Rubtsova K, Scott-Browne J, Crawford F, Macdonald WA, et al. Crossreactive T cells spotlight the germline rules for alphabeta T cell-receptor interactions with MHC molecules. Immunity. (2008) 28:324–34. doi: 10.1016/j.immuni.2008.01.008
59. Hsu LY, Cheng DA, Chen Y, Liang HE, Weiss A. Destabilizing the autoinhibitory conformation of Zap70 induces up-regulation of inhibitory receptors and T cell unresponsiveness. J Exp Med. (2017) 214:833–49. doi: 10.1084/jem.20161575
60. Ellestad KK, Lin J, Boon L, Anderson CC. PD-1 controls tonic signaling and lymphopenia-induced proliferation of T lymphocytes. Front Immunol. (2017) 8:1289. doi: 10.3389/fimmu.2017.01289
61. Duong MN, Erdes E, Hebeisen M, Rufer N. Chronic TCR-MHC (self)-interactions limit the functional potential of TCR affinity-increased CD8 T lymphocytes. J ImmunoTher Cancer. (2019) 7:1–17. doi: 10.1186/s40425-019-0773-z
62. Kim SK, Boothroyd JC. Stage-specific expression of surface antigens by Toxoplasma gondii as a mechanism to facilitate parasite persistence. J Immunol. (2005) 174:8038–48. doi: 10.4049/jimmunol.174.12.8038
63. Smith LM, McWhorter AR, Masters LL, Shellam GR, Redwood AJ. Laboratory strains of murine cytomegalovirus are genetically similar to but phenotypically distinct from wild strains of virus. J Virol. (2008) 82:6689–96. doi: 10.1128/JVI.00160-08
Keywords: CD8+ T cells, MHC, Toxoplasma gondii, exhaustion, chronic infection
Citation: Tsitsiklis A, Bangs DJ, Lutes LK, Chan SW, Geiger KM, Modzelewski AJ, Labarta-Bajo L, Wang Y, Zuniga EI, Dai S and Robey EA (2020) An Unusual MHC Molecule Generates Protective CD8+ T Cell Responses to Chronic Infection. Front. Immunol. 11:1464. doi: 10.3389/fimmu.2020.01464
Received: 22 January 2020; Accepted: 05 June 2020;
Published: 08 July 2020.
Edited by:
Remy Bosselut, National Cancer Institute (NCI), United StatesReviewed by:
Tim Hand, University of Pittsburgh, United StatesGeorge So Yap, Rutgers University, United States
Copyright © 2020 Tsitsiklis, Bangs, Lutes, Chan, Geiger, Modzelewski, Labarta-Bajo, Wang, Zuniga, Dai and Robey. This is an open-access article distributed under the terms of the Creative Commons Attribution License (CC BY). The use, distribution or reproduction in other forums is permitted, provided the original author(s) and the copyright owner(s) are credited and that the original publication in this journal is cited, in accordance with accepted academic practice. No use, distribution or reproduction is permitted which does not comply with these terms.
*Correspondence: Ellen A. Robey, ZXJvYmV5QGJlcmtlbGV5LmVkdQ==