- 1Department of Medical Physiology, Texas A&M University Health Science Center College of Medicine, Bryan, TX, United States
- 2Wellman Center for Photomedicine, Harvard Medical School, Massachusetts General Hospital, Boston, MA, United States
Mast cells (MCs) are abundant in almost all vascularized tissues. Furthermore, their anatomical proximity to lymphatic vessels and their ability to synthesize, store and release a large array of inflammatory and vasoactive mediators emphasize their significance in the regulation of the lymphatic vascular functions. As a major secretory cell of the innate immune system, MCs maintain their steady-state granule release under normal physiological conditions; however, the inflammatory response potentiates their ability to synthesize and secrete these mediators. Activation of MCs in response to inflammatory signals can trigger adaptive immune responses by dendritic cell-directed T cell activation. In addition, through the secretion of various mediators, cytokines and growth factors, MCs not only facilitate interaction and migration of immune cells, but also influence lymphatic permeability, contractility, and vascular remodeling as well as immune cell trafficking through the lymphatic vessels. In summary, the consequences of these events directly affect the lymphatic niche, influencing inflammation at multiple levels. In this review, we have summarized the recent advancements in our understanding of the MC biology in the context of the lymphatic vascular system. We have further highlighted the MC-lymphatic interaction axis from the standpoint of the tumor microenvironment.
Introduction
Since their first description by Paul Ehrlich in 1878 as “mastzellen,” mast cells (MCs) have been mostly viewed as effectors of allergy (1). Investigations from the recent past have shown that nearly all vascularized organs (including heart (2), lungs (3), kidneys (4), intestine (5, 6), and liver (7)), the brain side (8, 9) of the blood-brain barrier, as well as the interfaces of host and the external environment, such as skin (10), have a prevalence of MCs. However, it has only been in the last two decades that MCs have gained significant attention for their involvement in several physiological and pathological processes (11, 12).
Mast Cell Subsets and Tissue Specificity
MCs are hematopoietic in origin. Following egress from the bone marrow, MC progenitors circulate in the blood, enter various tissues and develop into mature MCs under the influence of local growth factors, such as stem cell factor (SCF) and interleukin 3 (IL3) (13). The hematopoietic development of MC is unique as their early lineage progenitors, known as MC progenitors, leave the bone marrow before they are detectable by any MC lineage-specific histochemical marker and undergo transendothelial migration. Then MCs according to tissue environment differentiate into two major subclasses: (1) connective tissue MCs, located by nerve endings and alongside the blood and lymphatic vasculature, and (2) mucosal MCs, located on mucosal surfaces such as gut and respiratory mucosa. The secretory elements and granule releasing potential of these MC phenotypes are mainly driven by the local factors in these tissue niches (14). In addition, the subpopulations of human mast cells from skin and lungs were initially classified as MCTC (mast cell tryptase and chymase) and MCT (mast cell tryptase) types. They were recognized on the basis of the protease composition of their secretory granules, with tryptase, chymase, carboxypeptidase A3, and cathepsin G in the former and only tryptase in the latter (15, 16). MCTC contribute to tissue remodeling and angiogenesis, whereas MCT have been shown to be associated with host defense and immune functions. Both of these two subtypes express FcεR1 (the high-affinity receptor for the Fc region of immunoglobulin E), enabling them to contribute to allergic and hypersensitivity reactions (17).
The density of MCs in tissues varies depending upon the species and the location. For example, in human skin MC density is 7,000–12,000, 20,000 per mm3 in the intestine and 500–4,000 per mm3 in the lungs (18). In addition, while MCs are prevalent in lymph nodes, their number is greatly increased in response to inflammation (19), where they actively contribute to the recruitment of immune cells to lymph nodes through secretion of cytokines and chemokines (20, 21).
Overview of Mechanisms of Mast Cell Activation
The cytoplasm of MCs carries secretory granules containing inflammatory mediators (such as histamine), heparin, and a number of cytokines among other mediators (Figures 1A,B). Upon activation, MCs release the contents of these pre-stored granules into their residing tissue microenvironment, initiating multiple physiological responses that are not limited to allergy, but are also involved in control of vascular tone and permeability, neovascularization, and defense against pathogen exposure (22–25) as well as influencing the trafficking of immune cells from the adjacent tissue niche. Interestingly, the recent findings of the close localization and high density of MCs near lymphatic vessels (LVs) and their regulated release of pre-stored as well as de novo synthesized vasoactive compounds has expanded the scope of MC biology in the context of lymphatic biology (6, 12, 26–28). Furthermore, recent studies also suggest MCs are immune sentinels, as they are able to present antigens via the expression of major histocompatibility complex II (MHC II) molecules and can regulate the function of innate and adaptive immune cells, including dendritic cells (DCs), macrophages, eosinophils, lymphocytes (T and B cells), and fibroblasts (23, 29–31).
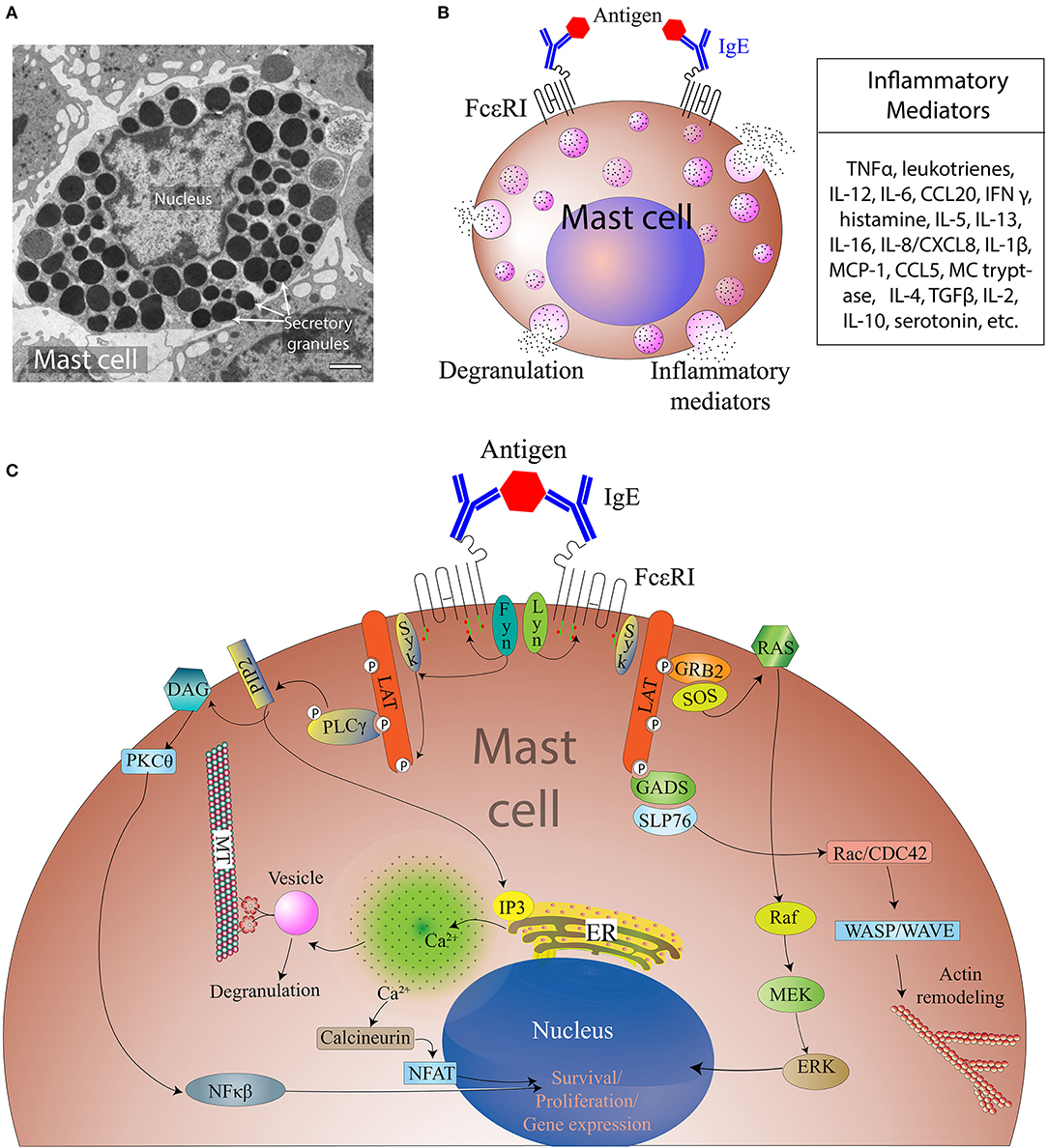
Figure 1. Overview of MC activation and degranulation mechanisms. (A) A transmission electron microscope image of an activated MC showing multiple secretory granules inside the cell. Adapted from Grujic et al. (25) and reproduced with written permission from the publisher. Copyright 2013, the American Association of Immunologists, Inc. (B) A schematic of a MC showing Immunoglobulin E (IgE)-mediated interaction with allergen and secretion of different inflammatory mediators. (C). Aggregation of the IgE Receptor (FcεRI) by multivalent antigen induces activation of tyrosine-protein kinase Lyn (Lyn), the Src kinase that phosphorylates immunoreceptor tyrosine-based activation motifs (ITAMs) of FcεRI β and γ subunits, followed by the association of the tyrosine-protein kinase Syk with the FcεRI via Syk-Src Homology domain 2 (SH2) within phosphorylated ITAMs. This clustering leads to activation of tyrosine-protein kinase Fyn that phosphorylates the adaptor growth factor receptor-bound protein 2 (Grb2). Activation of phospholipase C gamma 1 (PLC-γ1) results in the hydrolysis of phosphatidylinositol-4,5-bisphosphate (PIP2) into inositol 1, 4, 5-triphosphate (IP3) and diacylglycerol (DAG). IP3 production leads to increased intracellular free calcium (Ca2+) concentration, whereas DAG can activate both protein kinase C-θ (PKC-θ) and Ras. Tyrosine phosphorylated SLP76 also associates with the Rho-family guanine nucleotide exchange factor (GEF) Vav1 and the adaptor protein, Nck. Vav1 activates Rac and cell division control protein 42 (Cdc42), which initiate actin cytoskeletal rearrangement via activation of Wiskott-Aldrich syndrome protein (WASP). Cytoskeletal rearrangement is required for cell migration and microtubule-dependent degranulation of MCs.
As innate immune cells, MCs are equipped for early and rapid sensing of invading microorganisms such as bacteria, parasites, fungi, and viruses. The magnitude and nature of MC responses to different stimuli can be influenced by intrinsic as well as micro-environmental factors that can modulate the expression and functionality of MC surface receptors and/or signaling molecules contributing to these responses (31, 32). These pathogens display conserved molecular structures called pathogen-associated molecular patterns (PAMPs) that are recognized by pattern recognition receptors (PRRs), such as Toll-like receptors (TLRs), on the MC surface. MCs express TLRs 1 to 7 and 9, NOD-like receptors (NLRs), and retinoic acid-inducible gene-I (RIG-I). Signaling through TLRs on the MC surface activates myeloid differentiation primary response protein 88 (MyD88) and MyD88 adapter like protein/Toll/Interleukin-1 Receptor Domain-Containing Adapter Protein (MAL/TIRAP), which induces nuclear factor kappa-light-chain-enhancer of activated B cells (NF-κB) translocation to the nucleus resulting in the transcriptional initiation of several cytokines. MC-derived histamine is a necessary mediator involved in lipopolysaccharide- (LPS-) induced phosphorylation of NF-κB (33). TLR4 can be activated by LPS, subsequently stimulating MC/histamine/NF-κB-dependent production and release of multiple cytokines by MCs and surrounding tissues (33) as well as the release of preformed granules, whereas activation of TLR2 by peptidoglycan results in extensive degranulation (34, 35). Recent findings demonstrate that histamine, released by MCs, is able to bind to histamine receptors 1 and 2 on MCs and, as such, maintains or re-initiates further MC degranulation (12).
The most extensively investigated pathway for MC activation (schematically presented in Figure 1C) is mediated through antigen/IgE/FcϵRI cross-linking. The high affinity immunoglobulin E (IgE) receptor, FcϵRI, consists of an α-chain that binds to IgE, a β-chain that spans the cell membrane, and two γ chains. Tyrosine-protein kinase Lyn (Lyn) interacts and phosphorylates tyrosine in its immunoreceptor tyrosine-based activation motifs (ITAMs) on the β and γ chains of the FcϵRI, which further activates Syk tyrosine kinases that phosphorylate LAT1 and LAT2 (linkers for activation of T cells). Furthermore, downstream phosphorylated phospholipase Cγ1 (PLCγ1) hydrolyzes phosphatidylinositol-4,5-bisphosphate (PIP2) to make inositol-1,4,5-trisphosphate (IP3) and diacylglycerol (DAG), causing calcium (Ca2+) mobilization from the endoplasmic reticulum. The release of Ca2+ from the endoplasmic reticulum leads to stromal interaction molecule 1- (STIM1) mediated opening of the store-operated Ca2+ channel Orai1, leading to the influx of extracellular Ca2+. The influx of Ca2+ is accompanied by an additional mechanism that is mediated by transient receptor potential channel 1 (TRPC1). The increase in intracellular Ca2+ levels and the activation of PKC triggers the degranulation machinery. Calcium release also activates NF-κB translocation to the nucleus, which results in transcriptional initiation of several cytokines, such as IL6, tumor necrosis factor alpha (TNFα), and IL13. However, activation of FcϵRI also activates Fyn (Src kinase), complementary to the Lyn signaling pathway, which can also modulate MC degranulation. Fyn activates Syk which in a downstream cascade activates mammalian target of rapamycin (mTOR) in an Akt-dependent manner, and this can also induce MC chemotaxis and granule release (25, 36–39).
In addition to these mechanisms, MCs can be activated by a wide range of stimuli such as neuropeptides, cytokines, growth factors, vasoactive mediators such as non-MC-derived lymphatic-derived (40, 41) histamine, leukotrienes, toxins, certain lectins, basic compounds, complement proteins, immune complexes, certain drugs, as well as physical or mechanical stress and stretch. Recent investigations have shown that receptors for adenosine, complement C3A, chemokines, sphingosine-1-phosphate, substance P and SCF are also involved in MC activation (42–45). Activation of these receptors can also potentiate FcεRI-mediated activation (42–45).
An important aspect of the regulation of MC granule release is MC sensitization. When the host is exposed to pathogens, antigen-presenting cells are engaged. Subsequent activation of Th2 cells along with interleukin production causes B cells to undergo class-switching to form IgE antibody secreting plasma cells. IgE directly binds to the high affinity receptor FcεR1 expressed on MCs. In the process of B cell differentiation to IgE-secreting plasma cells, the MCs secrete interleukins-4,−5, and−10, all of which are crucial for this process. Thus, a MC-dependent MC sensitization loop can aggravate inflammatory pathophysiology. The precise mechanism by which this MC feedback regulation can be disrupted without inducing pathological outcomes is still not clearly understood, however repeated exposure to increasing doses of antigen or oral immune therapy (46) can potentially ameliorate or reduce the degree of MC sensitization (47) and thereby associated pathology.
Mast Cells as Effector Cells at the Interface of the Lymphatic and Immune Systems
Beyond the role of MCs in hypersensitivity reactions, as an immune sentinel MCs respond to a variety of pathogenic (and non-pathogenic) stimuli by releasing a host of vasoactive mediators, cytokines, growth factors, proteases, biogenic amines and interferon pre-stored within MC granules (see Table 1) (85). Release of granules can be broadly divided into two types: (1) degranulation, characterized by rapid release of pre-synthesized granules [often seen in allergic reactions], and (2) de novo mediator release, a comparatively slow process contributing to chronic responses in tissue remodeling, pathogen clearance and often involving engagement of innate as well as adaptive immune cells. MCs, as innate immune sentinels, preferentially reside in the interface of the host and its external environment (36, 86). Exposure of MCs to Gram-negative bacteria induces release of inflammatory mediators such as TNFα, IL6, and IL1β, and chemotactic migration of natural killer cells, eosinophils, and neutrophils, as well as upregulation of adhesion molecules and chemokine ligands in blood and lymphatic vasculatures (26). Activation of MCs also modulates both adaptive and innate immune responses (85, 87). For example, release of histamine by MCs has been shown to regulate T helper type 1 (Th1) and Th2 responses by inducing Th1-specific (IL4, IL10, IL13) and Th2-specific (IFNγ, IL2) (88) cytokines through differential activation of histamine receptors 1 and 2 (61). A recent study by Kambayashi et al. demonstrated that in certain inflammatory conditions MCs may express MHC II molecules and present antigens to CD4+ T cells and preferentially expand T regulatory cells (89). Furthermore, studies on the formation of MC-DC (12, 90) or MC-T cell (91) immune synapses reinforce the possibility of intercellular crosstalk and ability of MCs to recruit DCs or T cells to the site of inflammation, infection or injury. As such, MCs are endowed with the ability to not only modulate innate but also adaptive immune responses (28, 92). In addition, recent evidence suggests that MCs can increase their IL10 secretion in a T regulatory cell-dependent manner. This, in turn, contributes to the maintenance of graft tolerance (93). Therefore, while T cells require MHC-bound antigens on antigen-presenting cells to trigger T cell secretory pathways (94), MCs do not require any MHC-bound antigen to initiate the degranulation process. However, aberrant activation of MCs can cause MC degranulation, which can suppress T regulatory cell function, which essentially breaks down peripheral tolerance (95).
MCs are the major innate effector cell type localized close to LVs (6, 12, 26, 28) (Figure 2) and are able to release numerous inflammatory and vasoactive mediators (Tables 1, 2). These unique features make MCs a relevant and key player in the regulation of lymphatic immuno-physiology. The functional implication of such a lymphatic-oriented localization pattern of MCs is not completely understood. However, we believe that it is reasonable to speculate that as the major sensory arm of the innate immune response, activated and degranulated MCs can release a wide array of vascular and inflammatory mediators (135) that are able to diffuse to the adjacent LVs due to their proximity (see Table 2). These mediators can instantaneously interact with the lymphatic endothelium and promote expression of adhesion molecules, such as integrins, that promote the recruitment of circulating leukocytes as well as affecting the permeability of LVs (discussed in details in (33)). Furthermore, chemotactic migration of leukocytes from the tissue parenchyma facilitates LV-directed immune cell trafficking, advancing the resolution of a local inflammatory event (12, 28). MC mediators also influence lymphatic pumping by affecting lymphatic muscle cell contractility (33, 96), thus accelerating or slowing down delivery of pathogens and immune cells to draining lymph nodes. Many of these events are dysregulated due to age-related alterations in LVs and signaling pathways that lead to MC degranulation. In brief, aging alters structure (by increasing the size of zones with low muscle cell investiture) (136), ultrastructure (through loss of the glycocalyx), and proteome composition with a concomitant increase in permeability of aged lymphatic vessels (137). The contractile function of aged LVs is depleted (138–140) with the abolished role of nitric oxide and an increased role of lymphatic-born histamine in flow-dependent regulation of lymphatic phasic contractions and tone (40, 41). In addition, aging induces oxidative stress in LVs (141) and facilitates the spread of pathogens from these vessels into perilymphatic tissues (137). Aging causes the basal activation of perilymphatic MCs, which, in turn, restricts recruitment/activation of immune cells in perilymphatic tissues (6, 28). This aging-associated basal activation of MCs limits proper functioning of the MC/histamine/NF-κB axis that is essential for the regulation of LV transport and barrier functions as well as for both the interaction and trafficking of immune cells near and within lymphatic collecting vessels (33). Cumulatively, these aging-associated changes in MCs play important roles in the pathogenesis of alterations in inflammation and immunity associated with aging (33, 142, 143).
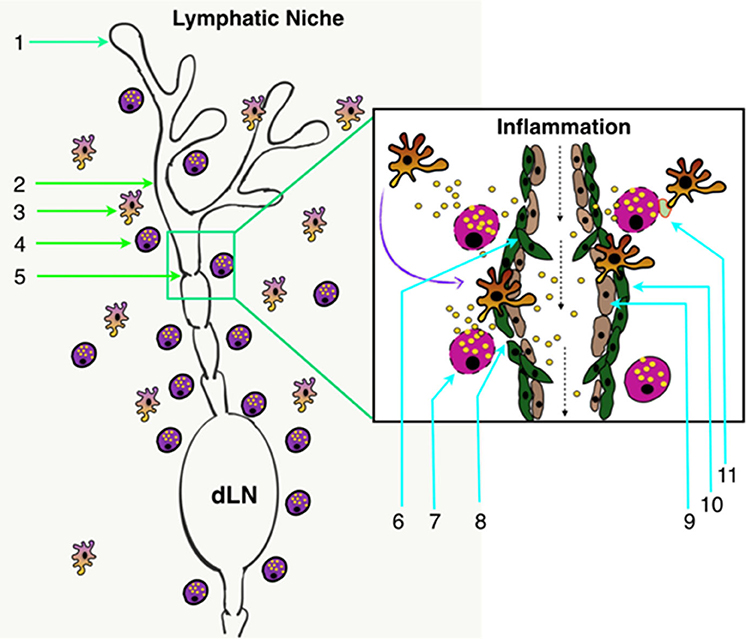
Figure 2. Lymphatic system architecture along with the lymphatic tissue niche. (1) Initial lymphatics (lymphatic capillaries) (2) Pre-collector LVs; (3) Perilymphatic antigen-presenting cells; (4) Perilymphatic MCs; (5) Collecting LVs; (6) Lymphatic valve in collecting LVs; (7) Degranulating MCs in response to inflammation; (8) Increased permeability of LVs in response to inflammation; (9) LECs; (10) Lymphatic muscle cells; and (11) Inflammation-induced MC-DC immune synapse formation in perilymphatic tissues. dLN, Draining lymph node.
Role of Lymphatic Vasculature-Associated Mast Cells in the Regulation of the Inflammatory Response
Local inflammation causes pathological outcome such as swelling, where chemotactic migration of leukocytes into the affected area is accompanied by the rapid influx of interstitial fluid. Such pathophysiological change increases the overall tissue interstitial pressure gradient. This pulls on the anchoring filaments attached to lymphatic capillaries (initial lymphatics), opening primary lymphatic valves and helping various immune cells to enter lymphatic network, to clear accumulated antigens and noxious tissue debris from the inflamed site (144–147). The phasic contractions of lymphangions in LVs support long-distance flow of lymph and immune cells and direct the immune cell trafficking from the affected site to the draining lymph nodes (146, 147). Furthermore, lymph nodes, as secondary lymphoid organs, are continuously perfused and presented with soluble foreign antigens by the lymph transported through afferent LVs. Continuous screening of lymph in the node is necessary for activation of node-resident naïve antigen-presenting cells and priming of naïve T and B cells in response to any given inflammatory stimuli.
In addition, novel studies suggest that inflammation-induced changes to the microenvironment can upregulate the expression of adhesion molecules such as ICAM-1 and VCAM-1, as well as chemokines such as CCL21 and CX3CL1 in lymphatic endothelial cells (LECs) (148, 149). This upregulation can enhance trafficking of antigen-presenting cells, such as DCs and macrophages, from the affected tissues through the walls of collecting LVs toward the draining lymph node, thus contributing to the resolution of tissue inflammation (145, 146, 150). In addition, molecules such as the chemokine scavenging receptor D6 expressed by LECs can selectively regulate the interaction between mature and immature DCs, which helps in the cellular trafficking and removal of inflammatory chemokines. At the same time, the expression of D6 is dependent on molecules such as IL6 and interferon gamma secreted by the MCs and present in the inflammatory microenvironment (151). In addition, inflammation can induce expansion of the lymphatic network through initiation of lymphangiogenic programs, driven by vascular endothelial growth factor-C (VEGF-C-) and VEGF-D-related signaling pathways. Their activation depends on molecules such as TNFα, fibroblast growth factor (FGF), platelet-derived growth factor (PDGF) and IL6 secreted by immune cells closely associated with LVs, such as perilymphatic MCs (Table 2), as well as tissue-resident or migratory macrophages. Although considerable progress has been made in understanding the basic mechanisms of lymphangiogenic programs, the question on how these regulatory factors induce inflammation-associated lymphangiogenesis warrants further investigation (152).
It has been suggested that lymphangiogenesis contributes to chronic inflammation-associated pathology or to the resolution and repair of damaged tissue. However, a chronic inflammatory environment can also influence not only lymphatic muscle and endothelial cells but also activate regulated release of granules by MCs. Overall, an inflammation-induced, MC-dependent dysfunction of LVs (33) may cause significant local stasis of lymph as well as diminished trafficking of lymphocytes to the draining lymph node causing overall immune suppression. The resultant altered tissue environment is manifested clinically, e.g., as lymphedema, local fibrosis, and secondary bacterial infections. These pathologies are strongly associated with diseases such as secondary lymphedema, often occurring following the surgical removal of lymph nodes to limit cancer progression or in cases of lymphatic filariasis or lipedema (153).
Roles of Mast Cells in the Modulation of Lymphatic Immuno-Physiology in Cancer
Genetic and epigenetic alteration of healthy as well as tumor-associated cells play a pivotal role in the development of tumor microenvironment and progression of tumor pathology. The presence of MCs in human tumors was known for more than a century (154), however their specific roles in cancer have just begun to be explored in recent decades. Several studies have shown an increased number of MCs in tumors, such as squamous cell carcinoma of the esophagus (155), pancreatic adenocarcinomas (156–158), prostate cancer (159), breast cancer (160, 161), and many hematological carcinomas (162–164). The studies on MCs in tumor sites suggest a potential role of MCs in tumor progression (165, 166). However, whether MCs play an anti-tumorigenic or pro-tumorigenic role remains controversial (165, 167) and has become an active area of research.
The cross-talk between MCs and other cells such as cancer-associated fibroblasts (CAFs), tumor cells, myeloid-derived suppressor cells, and immune cells in the tumor microenvironment is being explored by many research groups. MCs secrete several cytokines, depending on their activation status, which directly and indirectly impact the behavior of cancer cells and other components of the tumor microenvironment (Figure 3A). TNFα released by MCs helps DCs to mature and express more MHC Class I molecules and co-stimulatory receptors on MCs that activate CD8+ T cells in the tumor-draining lymph node (168, 169). Since activated CD8+ T cells play a critical role in eliminating cancerous cells from the body, it is important to explore to what extent MC-mediated activation of T cells contributes to cancer immunity (170). In one study, MCs were shown to be present in metastatic lymph nodes of cancer patients (171), suggesting a connection between the immune and lymphatic system in cancer. Interestingly, Stelekati et al. showed that MCs are capable of presenting antigens in the context of MHC class I and II molecules (168). In this latter study, MC-mediated antigen presentation was found to regulate cytotoxic T lymphocyte effector function. Another study by Nakae et al. reported that MCs express several co-stimulatory molecules and can activate T cells in a TNFα-dependent manner (169). MCs were also reported to release several cytokines that direct the functions of a subset of T cells (82). Thus, like other professional antigen presenting cells, MCs are also capable of providing signals to T cells: antigen presentation, expression of co-stimulatory molecules, and release of cytokines. However, these events happen in different contexts and in a poorly coordinated manner, and are not necessarily related to cancers. Antigen presentation by MCs has also been reported in the case of bacterial infections (172). Taken together, these findings broaden our understanding of the capabilities of MCs to serve as non-professional antigen presenting cells.
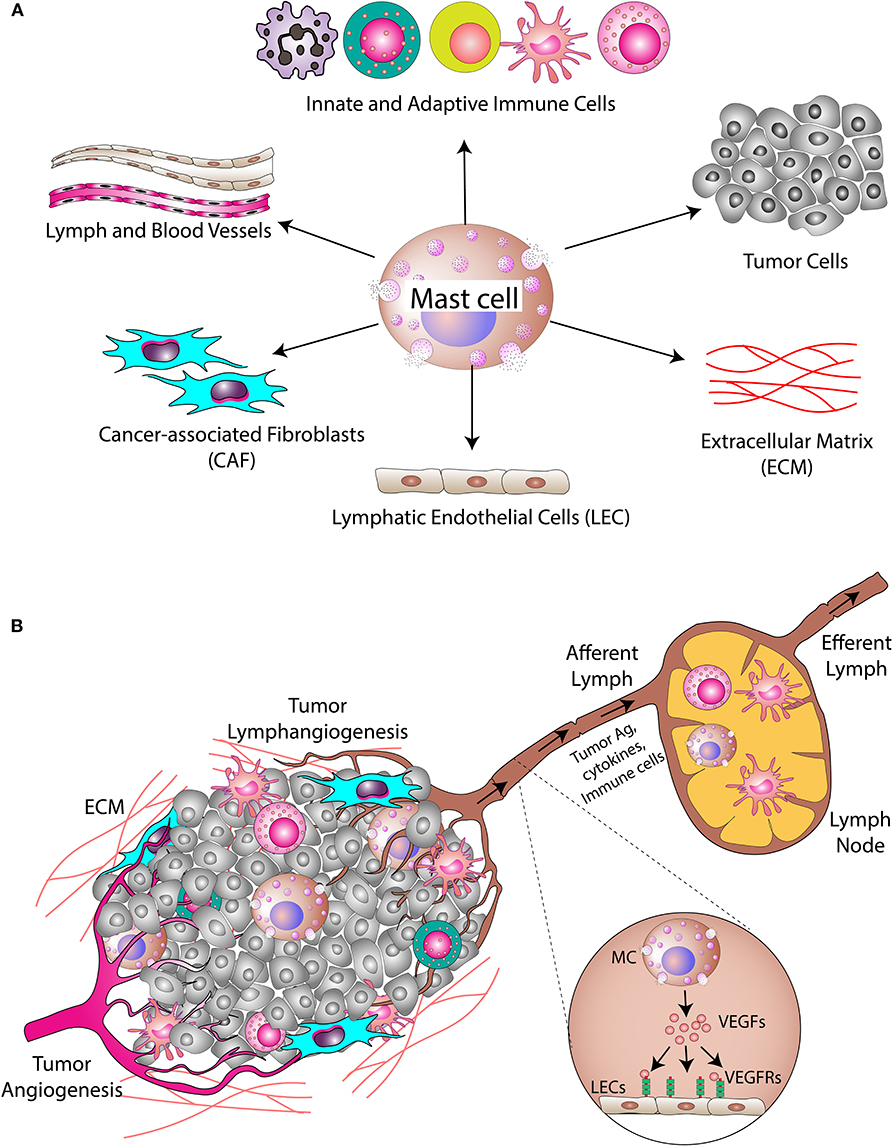
Figure 3. Mast cells in the tumor microenvironment. (A) MCs secrete different cytokines/inflammatory mediators into the tumor microenvironment. These cytokines/inflammatory mediators modulate cellular and acellular components of the tumor. The cellular components include cancer cells, cancer-associated fibroblasts, and different types of immune cells, while the acellular component is mainly the extracellular matrix (ECM). (B) MCs also modulate angiogenesis and lymphangiogenesis in the tumor. Tumor necrosis factor-alpha (TNFα) released by MCs induces migration of DCs into the draining lymph nodes where T cell activation takes place. MC-derived proteases induce modification of the ECM, which alters the microarchitecture leading to metastasis.
SCF is among the many inflammatory mediators produced and secreted by tumor cells in the tumor microenvironment. SCF is known to bind the c-kit receptor on the surface of MCs (43, 173). Activation of this signaling cascade is necessary for maturation, migration, and survival of MCs, which could explain why an increased accumulation of MCs is observed in the tumor microenvironment of many cancers. A study led by Zhang et al. (174) provided direct evidence that SCF released by tumor cells modulates tumor angiogenesis by regulating MCs. In this study, the researchers used sense or antisense SCF cDNA to overexpress or deplete SCF expression in rat mammary tumor cells. Depletion of SCF significantly decreased MC infiltration and vascularization in the tumor whereas the opposite effects were observed in SCF-overexpressing tumors. Thus, SCF should be considered as a therapeutic target to inhibit the progression of certain types of tumors. A later study by Huang et al. (175) suggested a role for SCF-activated MCs in remodeling of the tumor microenvironment and subsequent immunosuppression. The activated MCs were shown to release adenosine, which in turn increased T regulatory cell infiltration into the tumors.
Angiogenesis and lymphangiogenesis are very critical steps in the development of a tumor since their induction is necessary for the nourishment of growing tumors (176, 177). In addition, the tumor-associated lymphatic network also plays a pivotal role in the process of metastasis (178). Studies have shown that cancer cells leave their site of origin and migrate to tumor-draining lymph nodes (179). The presence of cancer cells in tumor-draining lymph nodes serves as a major prognostic indicator in many cancers, including breast, skin, and colon cancers. Thus, the lymphatic network within and around a solid tumor serves as a route for dissemination and metastasis of cancer cells (Figure 3B).
The evidence that MCs are involved in lymphangiogenesis comes from multiples studies. In a report by Raica et al. (180), MCs were identified as the key player in the development of tumor LVs in a certain subtype of breast cancer. The study was conducted by analyzing histopathological samples from human patients that showed a positive correlation between peritumoral MC density and lymphatic microvessel density. In contrast, Utrera-Barillas et al. (181) reported a direct correlation between MC density and blood vasculature, whereas macrophage density was directly correlated with lymphatic vasculature in certain stages of cervical carcinoma, suggesting tumor type-specific roles for MCs in neovascularization.
Poor prognosis, invasion, and metastasis of cancer are often associated with increased LV density and secretion of VEGF-C (182–184), a pro-lymphangiogenic cytokine known to be secreted by many cancer cells (182, 185, 186) as well as MCs (118). VEGF-C increases the rate of lymph flow to the tumor-draining lymph node (187), which could facilitate cancer metastasis. However, further studies are warranted to draw a concrete link between them. Tumor cells can also gain metastatic features as a result of epithelial-to-mesenchymal transition (EMT). EMT is associated with chemoresistance in many cancer types. MCs were reported to induce EMT and cancer stem cell signatures in human thyroid cancer through CXCL8/IL-8 pathways (188, 189).
MCs can remodel the tumor microenvironment not only by regulating the cells but also by changing the tumor matrix, commonly known as extracellular matrix (ECM). MCs secrete different matrix metalloproteinases (such as MMP9) (190) and proteases, including tryptase and chymase (77, 191). These enzymes digest tumor matrix favoring the expansion and migration of tumor and other cells in the microenvironment. Such matrix degradation also disrupts the physical contact between epithelial and stromal layers, leading to detachment of tumor cells and metastasis (192, 193).
In summary, the involvement of MCs in tumor progression is multi-faceted. Several studies of different cancers in human patients and experimental models indicate that MCs promote tumor growth by suppressing immunity and/or by promoting angiogenesis, while other studies indicate MCs are instrumental in inhibiting tumor growth. These studies have been summarized and discussed in multiple recent review articles (165, 167, 194). While the controversy remains as to whether MCs act as pro-tumorigenic or anti-tumorigenic cells, it is probably safe to assume that their functions are tumor type-specific in nature and cannot be generalized. Furthermore, it has long been debated whether inflammation is linked to cancer (195, 196) and to what extent MCs are involved in this process in order to develop new treatment lines and preventive measures. The precise mechanism by which MC-mediated inflammation leads to cancer progression, if any, is yet to be determined.
Conclusions and Perspectives
For many years, MCs have only been perceived as an integral part of the innate immune system. However, recent studies on MCs have tremendously changed our understanding of their roles in the development of adaptive immune responses as well as their close association with lymphatic vasculature. Evidence presented in this review suggests that MCs are intricately involved in the regulation of lymphatic functions, thus contributing to the convergence of the immune system with the lymphatic vascular system. Through the secretion of various mediators, cytokines and growth factors, MCs not only facilitate cellular interaction and migration but also influence lymphatic permeability, contractility, and vascular remodeling and immune cell trafficking toward and through the LVs. These MC-mediated physiological alterations on LVs are also critical to cancer progression. The signaling axis and the cross-talk between MCs and different cell types in the lymphatic niche of healthy and cancerous tissue remain poorly defined. Certainly, this lymphatic-MC-adaptive immune axis is greatly dependent upon the various inflammatory contexts as well as a tissue- or tumor-specific microenvironment. However, many of these pathways and the crosstalk between MCs and other cells have yet to be explored, warranting further studies to understand many diseases associated with inflammation and to determine further in depth how MCs could be approached as a potential therapeutic target for next-generation medicine.
Author Contributions
All authors contributed to manuscript writing, read, and approved the submitted version.
Funding
This Support was provided by the National Institutes of Health (NIH) grants 1R56DK099161 and 1R56AG061097-01, and by Texas A&M Health Science Center/Department of Medical Physiology.
Conflict of Interest
The authors declare that the research was conducted in the absence of any commercial or financial relationships that could be construed as a potential conflict of interest.
Acknowledgments
Authors acknowledge funding support as mentioned.
References
1. Theoharides TC, Valent P, Akin C. Mast cells mastocytosis, and related disorders. N Engl J Med. (2015) 373:163–72. doi: 10.1056/NEJMra1409760
2. Ngkelo A, Richart A, Kirk JA, Bonnin P, Vilar J, Lemitre M, et al. Mast cells regulate myofilament calcium sensitization and heart function after myocardial infarction. J Exp Med. (2016) 213:1353–74. doi: 10.1084/jem.20160081
3. Miller HR, Pemberton AD. Tissue-specific expression of mast cell granule serine proteinases and their role in inflammation in the lung and gut. Immunology. (2002) 105:375–90. doi: 10.1046/j.1365-2567.2002.01375.x
4. Ehara T, Shigematsu H. Mast cells in the kidney. Nephrology. (2003) 8:130–8. doi: 10.1046/j.1440-1797.2003.00153.x
5. Bischoff SC, Kramer S. Human mast cells, bacteria, and intestinal immunity. Immunol Rev. (2007) 217:329–37. doi: 10.1111/j.1600-065X.2007.00523.x
6. Chatterjee V, Gashev AA. Aging-associated shifts in functional status of mast cells located by adult and aged mesenteric lymphatic vessels. Am J Physiol Heart Circ Physiol. (2012) 303:H693–702. doi: 10.1152/ajpheart.00378.2012
7. Francis H, Meininger CJ. A review of mast cells and liver disease: what have we learned? Digest Liver Dis. (2010) 42:529–36. doi: 10.1016/j.dld.2010.02.016
8. Silver R, Silverman A-J, Vitković L, Lederhendler II. Mast cells in the brain: evidence and functional significance. Trends Neurosci. (1996) 19:25–31. doi: 10.1016/0166-2236(96)81863-7
9. Nautiyal KM, Ribeiro AC, Pfaff DW, Silver R. Brain mast cells link the immune system to anxiety-like behavior. Proc Natl Acad Sci USA. (2008) 105:18053–7. doi: 10.1073/pnas.0809479105
10. Weller K, Foitzik K, Paus R, Syska W, Maurer M, Weller K, et al. Mast cells are required for normal healing of skin wounds in mice. FASEB J. (2006) 20:2366–8. doi: 10.1096/fj.06-5837fje
11. Metcalfe DD. Mast cells and mastocytosis. Blood. (2008) 112:946–56. doi: 10.1182/blood-2007-11-078097
12. Pal S, Gasheva OY, Zawieja DC, Meininger CJ, Gashev AA. Histamine-mediated autocrine signaling in mesenteric perilymphatic mast cells. Am J Physiol Regul Integr Comp Physiol. (2020) 318:R590–604. doi: 10.1152/ajpregu.00255.2019
13. Gilfillan AM, Austin SJ, Metcalfe DD. Mast cell biology: introduction and overview. Adv Exp Med Biol. (2011) 716:2–12. doi: 10.1007/978-1-4419-9533-9_1
14. Gurish MF, Austen KF. Developmental origin and functional specialization of mast cell subsets. Immunity. (2012) 37:25–33. doi: 10.1016/j.immuni.2012.07.003
15. Xia HZ, Kepley CL, Sakai K, Chelliah J, Irani AM, Schwartz LB. Quantitation of tryptase, chymase, Fc epsilon RI alpha, and Fc epsilon RI gamma mRNAs in human mast cells and basophils by competitive reverse transcription-polymerase chain reaction. J Immunol. (1995) 154:5472–80.
16. Oskeritzian CA, Zhao W, Min HK, Xia HZ, Pozez A, Kiev J, et al. Surface CD88 functionally distinguishes the MCTC from the MCT type of human lung mast cell. J Allergy Clin Immunol. (2005) 115:1162–8. doi: 10.1016/j.jaci.2005.02.022
17. Church MK, Levi-Schaffer F. The human mast cell. J Allergy Clin Immunol. (1997) 99:155–60. doi: 10.1016/S0091-6749(97)70089-7
18. Abraham SN, Malaviya R. Mast cells in infection and immunity. Infect Immun. (1997) 65:3501–8. doi: 10.1128/IAI.65.9.3501-3508.1997
19. Sainte-Marie G, Peng FS. Mast cells and fibrosis in compartments of lymph nodes of normal, gnotobiotic, and athymic rats. Cell Tissue Res. (1990) 261:1–15. doi: 10.1007/BF00329433
20. Wang HW, Tedla N, Lloyd AR, Wakefield D, McNeil PH. Mast cell activation and migration to lymph nodes during induction of an immune response in mice. J Clin Invest. (1998) 102:1617–26. doi: 10.1172/JCI3704
21. Jawdat DM, Rowden G, Marshall JS. Mast cells have a pivotal role in TNF-independent lymph node hypertrophy and the mobilization of Langerhans cells in response to bacterial peptidoglycan. J Immunol. (2006) 177:1755–62. doi: 10.4049/jimmunol.177.3.1755
22. Kalesnikoff J, Galli SJ. New developments in mast cell biology. Nat Immunol. (2008) 9:1215–23. doi: 10.1038/ni.f.216
23. Abraham SN, St John AL. Mast cell-orchestrated immunity to pathogens. Nat Rev Immunol. (2010) 10:440–52. doi: 10.1038/nri2782
24. da Silva EZ, Jamur MC, Oliver C. Mast cell function: a new vision of an old cell. J Histochem Cytochem. (2014) 62:698–738. doi: 10.1369/0022155414545334
25. Krystel-Whittemore M, Dileepan KN, Wood JG. Mast cell: a multi-functional master cell. Front Immunol. (2015) 6:620. doi: 10.3389/fimmu.2015.00620
26. Kunder CA, St John AL, Abraham SN. Mast cell modulation of the vascular and lymphatic endothelium. Blood. (2011) 118:5383–93. doi: 10.1182/blood-2011-07-358432
27. Kunder CA, St John AL, Li G, Leong KW, Berwin B, Staats HF, et al. Mast cell-derived particles deliver peripheral signals to remote lymph nodes. J Exp Med. (2009) 206:2455–67. doi: 10.1084/jem.20090805
28. Chatterjee V, Gashev AA. Mast cell-directed recruitment of MHC class II positive cells and eosinophils towards mesenteric lymphatic vessels in adulthood and elderly. Lymphat Res Biol. (2014) 12:37–47. doi: 10.1089/lrb.2013.0031
29. Dudeck J, Medyukhina A, Frobel J, Svensson CM, Kotrba J, Gerlach M, et al. Mast cells acquire MHCII from dendritic cells during skin inflammation. J Exp Med. (2017) 214:3791–811. doi: 10.1084/jem.20160783
30. St John AL, Abraham SN. Innate immunity and its regulation by mast cells. J Immunol. (2013) 190:4458–63. doi: 10.4049/jimmunol.1203420
31. Urb M, Sheppard DC. The role of mast cells in the defence against pathogens. PLoS Pathog. (2012) 8:e1002619. doi: 10.1371/journal.ppat.1002619
32. Shelburne CP, Abraham SN. The mast cell in innate and adaptive immunity. Adv Exp Med Biol. (2011) 716:162–85. doi: 10.1007/978-1-4419-9533-9_10
33. Nizamutdinova IT, Dusio GF, Gasheva OY, Skoog H, Tobin R, Peddaboina C, et al. Mast cells and histamine are triggering the NF-kappaB-mediated reactions of adult and aged perilymphatic mesenteric tissues to acute inflammation. Aging. (2016) 8:3065–90. doi: 10.18632/aging.101113
34. Mekori YA, Metcalfe DD. Mast cells in innate immunity. Immunol Rev. (2000) 173:131–40. doi: 10.1034/j.1600-065X.2000.917305.x
35. Marshall JS, Jawdat DM. Mast cells in innate immunity. J Allergy Clin Immunol. (2004) 114:21–7. doi: 10.1016/j.jaci.2004.04.045
36. Wernersson S, Pejler G. Mast cell secretory granules: armed for battle. Nat Rev Immunol. (2014) 14:478–94. doi: 10.1038/nri3690
37. Sander LE, Frank SP, Bolat S, Blank U, Galli T, Bigalke H, et al. Vesicle associated membrane protein (VAMP)-7 and VAMP-8, but not VAMP-2 or VAMP-3, are required for activation-induced degranulation of mature human mast cells. Eur J Immunol. (2008) 38:855–63. doi: 10.1002/eji.200737634
38. Wajdner HE, Farrington J, Barnard C, Peachell PT, Schnackenberg CG, Marino JP, et al. Orai and TRPC channel characterization in fcepsilonri-mediated calcium signaling and mediator secretion in human mast cells. Physiol Rep. (2017) 5:e13166. doi: 10.14814/phy2.13166
39. Marquardt DL, Walker LL. Dependence of mast cell IgE-mediated cytokine production on nuclear factor-kappaB activity. J Allergy Clin Immunol. (2000) 105:500–5. doi: 10.1067/mai.2000.104942
40. Nizamutdinova IT, Maejima D, Nagai T, Bridenbaugh E, Thangaswamy S, Chatterjee V, et al. Involvement of histamine in endothelium-dependent relaxation of mesenteric lymphatic vessels. Microcirculation. (2014) 21:640–8. doi: 10.1111/micc.12143
41. Nizamutdinova IT, Maejima D, Nagai T, Meininger CJ, Gashev AA. Histamine as an endothelium-derived relaxing factor in aged mesenteric lymphatic vessels. Lymphat Res Biol. (2017) 15:136–45. doi: 10.1089/lrb.2016.0062
42. Schafer B, Piliponsky AM, Oka T, Song CH, Gerard NP, Gerard C, et al. Mast cell anaphylatoxin receptor expression can enhance IgE-dependent skin inflammation in mice. J Allergy Clin Immunol. (2013) 131:541–8.e1–9. doi: 10.1016/j.jaci.2012.05.009
43. Meininger CJ, Yano H, Rottapel R, Bernstein A, Zsebo KM, Zetter BR. The c-kit receptor ligand functions as a mast cell chemoattractant. Blood. (1992) 79:958–63. doi: 10.1182/blood.V79.4.958.bloodjournal794958
44. Saluja R, Kumar A, Jain M, Goel SK, Jain A. Role of sphingosine-1-phosphate in mast cell functions and asthma and its regulation by non-coding RNA. Front Immunol. (2017) 8:587. doi: 10.3389/fimmu.2017.00587
45. Li WW, Guo TZ, Liang DY, Sun Y, Kingery WS, Clark JD. Substance P signaling controls mast cell activation, degranulation, and nociceptive sensitization in a rat fracture model of complex regional pain syndrome. Anesthesiology. (2012) 116:882–95. doi: 10.1097/ALN.0b013e31824bb303
46. Tsai M, Mukai K, Chinthrajah RS, Nadeau KC, Galli SJ. Sustained successful peanut oral immunotherapy associated with low basophil activation peanut-specific IgE. J Allergy Clin Immunol. (2020) 145:885–96.e6. doi: 10.1016/j.jaci.2019.10.038
47. Ang WX, Church AM, Kulis M, Choi HW, Burks AW, Abraham SN. Mast cell desensitization inhibits calcium flux and aberrantly remodels actin. J Clin Invest. (2016) 126:4103–18. doi: 10.1172/JCI87492
48. Dudeck J, Ghouse SM, Lehmann CH, Hoppe A, Schubert N, Nedospasov SA, et al. Mast-cell-derived TNF amplifies CD8(+) dendritic cell functionality and CD8(+) T cell priming. Cell Rep. (2015) 13:399–411. doi: 10.1016/j.celrep.2015.08.078
49. Bischoff SC, Lorentz A, Schwengberg S, Weier G, Raab R, Manns MP. Mast cells are an important cellular source of tumour necrosis factor alpha in human intestinal tissue. Gut. (1999) 44:643–52. doi: 10.1136/gut.44.5.643
50. Brannan JD, Gulliksson M, Anderson SD, Chew N, Kumlin M. Evidence of mast cell activation and leukotriene release after mannitol inhalation. Eur Respir J. (2003) 22:491–6. doi: 10.1183/09031936.03.00113403
51. Malaviya R, Abraham SN. Role of mast cell leukotrienes in neutrophil recruitment and bacterial clearance in infectious peritonitis. J Leukoc Biol. (2000) 67:841–6. doi: 10.1002/jlb.67.6.841
52. Goldman G, Welbourn R, Klausner JM, Kobzik L, Valeri CR, Shepro D, et al. Mast cells and leukotrienes mediate neutrophil sequestration and lung edema after remote ischemia in rodents. Surgery. (1992) 112:578–86.
53. Nakano N, Nishiyama C, Kanada S, Niwa Y, Shimokawa N, Ushio H, et al. Involvement of mast cells in IL-12/23 p40 production is essential for survival from polymicrobial infections. Blood. (2007) 109:4846–55. doi: 10.1182/blood-2006-09-045641
54. Athie-Morales V, Smits HH, Cantrell DA, Hilkens CM. Sustained IL-12 signaling is required for Th1 development. J Immunol. (2004) 172:61–9. doi: 10.4049/jimmunol.172.1.61
55. Desai A, Jung MY, Olivera A, Gilfillan AM, Prussin C, Kirshenbaum AS, et al. IL-6 promotes an increase in human mast cell numbers and reactivity through suppression of suppressor of cytokine signaling 3. J Allergy Clin Immunol. (2016) 137:1863–71.e6. doi: 10.1016/j.jaci.2015.09.059
56. Kruger-Krasagakes S, Moller A, Kolde G, Lippert U, Weber M, Henz BM. Production of interleukin-6 by human mast cells and basophilic cells. J Invest Dermatol. (1996) 106:75–9. doi: 10.1111/1523-1747.ep12327815
57. Tanaka T, Narazaki M, Kishimoto T. IL-6 in inflammation, immunity, and disease. Cold Spring Harb Perspect Biol. (2014) 6:a016295. doi: 10.1101/cshperspect.a016295
58. Lin TJ, Maher LH, Gomi K, McCurdy JD, Garduno R, Marshall JS. Selective early production of CCL20, or macrophage inflammatory protein 3alpha, by human mast cells in response to Pseudomonas aeruginosa. Infect Immun. (2003) 71:365–73. doi: 10.1128/IAI.71.1.365-373.2003
59. Jain R, Tikoo S, Weninger W. Mast cell granules: modulating adaptive immune response remotely. J Allergy Clin Immunol. (2018) 143:1731–3. doi: 10.1016/j.jaci.2018.11.029
60. Gupta AA, Leal-Berumen I, Croitoru K, Marshall JS. Rat peritoneal mast cells produce IFN-gamma following IL-12 treatment but not in response to IgE-mediated activation. J Immunol. (1996) 157:2123–8.
61. Jutel M, Watanabe T, Klunker S, Akdis M, Thomet OA, Malolepszy J, et al. Histamine regulates T-cell and antibody responses by differential expression of H1 and H2 receptors. Nature. (2001) 413:420–5. doi: 10.1038/35096564
62. O'Mahony L, Akdis M, Akdis CA. Regulation of the immune response and inflammation by histamine and histamine receptors. J Allergy Clin Immunol. (2011) 128:1153–62. doi: 10.1016/j.jaci.2011.06.051
63. Arreola R, Becerril-Villanueva E, Cruz-Fuentes C, Velasco-Velazquez MA, Garces-Alvarez ME, Hurtado-Alvarado G, et al. Immunomodulatory effects mediated by serotonin. J Immunol Res. (2015) 2015:354957. doi: 10.1155/2015/354957
64. Herr N, Bode C, Duerschmied D. The effects of serotonin in immune cells. Front Cardiovasc Med. (2017) 4:48. doi: 10.3389/fcvm.2017.00048
65. Theoharides TC, Bondy PK, Tsakalos ND, Askenase PW. Differential release of serotonin and histamine from mast cells. Nature. (1982) 297:229–31. doi: 10.1038/297229a0
66. Masuda A, Yoshikai Y, Aiba K, Matsuguchi T. Th2 cytokine production from mast cells is directly induced by lipopolysaccharide and distinctly regulated by c-Jun N-terminal kinase and p38 pathways. J Immunol. (2002) 169:3801–10. doi: 10.4049/jimmunol.169.7.3801
67. Mukai K, Tsai M, Saito H, Galli SJ. Mast cells as sources of cytokines, chemokines, and growth factors. Immunol Rev. (2018) 282:121–50. doi: 10.1111/imr.12634
68. Rumsaeng V, Cruikshank WW, Foster B, Prussin C, Kirshenbaum AS, Davis TA, et al. Human mast cells produce the CD4+ T lymphocyte chemoattractant factor, IL-16. J Immunol. (1997) 159:2904–10.
69. Salamon P, Shoham NG, Gavrieli R, Wolach B, Mekori YA. Human mast cells release interleukin-8 and induce neutrophil chemotaxis on contact with activated T cells. Allergy. (2005) 60:1316–9. doi: 10.1111/j.1398-9995.2005.00886.x
70. Luft T, Jefford M, Luetjens P, Hochrein H, Masterman KA, Maliszewski C, et al. IL-1 beta enhances CD40 ligand-mediated cytokine secretion by human dendritic cells (DC): a mechanism for T cell-independent DC activation. J Immunol. (2002) 168:713–22. doi: 10.4049/jimmunol.168.2.713
71. Burd PR, Rogers HW, Gordon JR, Martin CA, Jayaraman S, Wilson SD, et al. Interleukin 3-dependent and -independent mast cells stimulated with IgE and antigen express multiple cytokines. J Exp Med. (1989) 170:245–57. doi: 10.1084/jem.170.1.245
72. Kim GY, Lee JW, Ryu HC, Wei JD, Seong CM, Kim JH. Proinflammatory cytokine IL-1beta stimulates IL-8 synthesis in mast cells via a leukotriene B4 receptor 2-linked pathway, contributing to angiogenesis. J Immunol. (2010) 184:3946–54. doi: 10.4049/jimmunol.0901735
73. Nakayama T, Mutsuga N, Yao L, Tosato G. Prostaglandin E2 promotes degranulation-independent release of MCP-1 from mast cells. J Leukoc Biol. (2006) 79:95–104. doi: 10.1189/jlb.0405226
74. Deshmane SL, Kremlev S, Amini S, Sawaya BE. Monocyte chemoattractant protein-1 (MCP-1): an overview. J Interferon Cytokine Res. (2009) 29:313–26. doi: 10.1089/jir.2008.0027
75. He S, Walls AF. Human mast cell chymase induces the accumulation of neutrophils, eosinophils and other inflammatory cells in vivo. Br J Pharmacol. (1998) 125:1491–500. doi: 10.1038/sj.bjp.0702223
76. Ebert S, Becker M, Lemmermann NA, Buttner JK, Michel A, Taube C, et al. Mast cells expedite control of pulmonary murine cytomegalovirus infection by enhancing the recruitment of protective CD8 T cells to the lungs. PLoS Pathog. (2014) 10:e1004100. doi: 10.1371/journal.ppat.1004100
77. Payne V, Kam PC. Mast cell tryptase: a review of its physiology and clinical significance. Anaesthesia. (2004) 59:695–703. doi: 10.1111/j.1365-2044.2004.03757.x
78. Weiss DL, Brown MA. Regulation of IL-4 production in mast cells: a paradigm for cell-type-specific gene expression. Immunol Rev. (2001) 179:35–47. doi: 10.1034/j.1600-065X.2001.790104.x
79. Mekori YA, Metcalfe DD. Mast cell-T cell interactions. J Allergy Clin Immunol. (1999) 104(Pt. 1):517–23. doi: 10.1016/S0091-6749(99)70316-7
80. Lebman DA, Edmiston JS. The role of TGF-beta in growth, differentiation, and maturation of B lymphocytes. Microbes Infect. (1999) 1:1297–304. doi: 10.1016/S1286-4579(99)00254-3
81. Lanconi G, Ravinal RC, Costa RS, Roselino AM. Mast cells and transforming growth factor-beta expression: a possible relationship in the development of porphyria cutanea tarda skin lesions. Int J Dermatol. (2008) 47:575–81. doi: 10.1111/j.1365-4632.2008.03607.x
82. Hershko AY, Suzuki R, Charles N, Alvarez-Errico D, Sargent JL, Laurence A, et al. Mast cell interleukin-2 production contributes to suppression of chronic allergic dermatitis. Immunity. (2011) 35:562–71. doi: 10.1016/j.immuni.2011.07.013
83. Hoyer KK, Dooms H, Barron L, Abbas AK. Interleukin-2 in the development and control of inflammatory disease. Immunol Rev. (2008) 226:19–28. doi: 10.1111/j.1600-065X.2008.00697.x
84. Chacon-Salinas R, Limon-Flores AY, Chavez-Blanco AD, Gonzalez-Estrada A, Ullrich SE. Mast cell-derived IL-10 suppresses germinal center formation by affecting T follicular helper cell function. J Immunol. (2011) 186:25–31. doi: 10.4049/jimmunol.1001657
85. Galli SJ, Maurer M, Lantz CS. Mast cells as sentinels of innate immunity. Curr Opin Immunol. (1999) 11:53–9. doi: 10.1016/S0952-7915(99)80010-7
86. Moon TC, Befus AD, Kulka M. Mast cell mediators: their differential release and the secretory pathways involved. Front Immunol. (2014) 5:569. doi: 10.3389/fimmu.2014.00569
87. Galli SJ, Nakae S, Tsai M. Mast cells in the development of adaptive immune responses. Nat Immunol. (2005) 6:135–42. doi: 10.1038/ni1158
88. Packard KA, Khan MM. Effects of histamine on Th1/Th2 cytokine balance. Int Immunopharmacol. (2003) 3:909–20. doi: 10.1016/S1567-5769(02)00235-7
89. Kambayashi T, Allenspach EJ, Chang JT, Zou T, Shoag JE, Reiner SL, et al. Inducible MHC class II expression by mast cells supports effector and regulatory T cell activation. J Immunol. (2009) 182:4686–95. doi: 10.4049/jimmunol.0803180
90. Carroll-Portillo A, Cannon JL, te Riet J, Holmes A, Kawakami Y, Kawakami T, et al. Mast cells and dendritic cells form synapses that facilitate antigen transfer for T cell activation. J Cell Biol. (2015) 210:851–64. doi: 10.1083/jcb.201412074
91. Mantri CK, St John AL. Immune synapses between mast cells and gammadelta T cells limit viral infection. J Clin Invest. (2019) 129:1094–108. doi: 10.1172/JCI122530
92. Shelburne CP, Nakano H, St John AL, Chan C, McLachlan JB, Gunn MD, et al. Mast cells augment adaptive immunity by orchestrating dendritic cell trafficking through infected tissues. Cell Host Microbe. (2009) 6:331–42. doi: 10.1016/j.chom.2009.09.004
93. Gan PY, Summers SA, Ooi JD, O'Sullivan KM, Tan DS, Muljadi RC, et al. Mast cells contribute to peripheral tolerance and attenuate autoimmune vasculitis. J Am Soc Nephrol. (2012) 23:1955–66. doi: 10.1681/ASN.2012060572
94. Nath S, Christian L, Tan SY, Ki S, Ehrlich LI, Poenie M. Dynein separately partners with NDE1 and dynactin to orchestrate T cell focused secretion. J Immunol. (2016) 197:2090–101. doi: 10.4049/jimmunol.1600180
95. de Vries VC, Wasiuk A, Bennett KA, Benson MJ, Elgueta R, Waldschmidt TJ, et al. Mast cell degranulation breaks peripheral tolerance. Am J Transplant. (2009) 9:2270–80. doi: 10.1111/j.1600-6143.2009.02755.x
96. Plaku KJ, von der Weid PY. Mast cell degranulation alters lymphatic contractile activity through action of histamine. Microcirculation. (2006) 13:219–27. doi: 10.1080/10739680600556902
97. Fox JL, von der Weid, PY. Effects of histamine on the contractile and electrical activity in isolated lymphatic vessels of the guinea-pig mesentery. Br J Pharmacol. (2002) 136:1210–8. doi: 10.1038/sj.bjp.0704820
98. Ferguson MK, Shahinian HK, Michelassi F. Lymphatic smooth muscle responses to leukotrienes, histamine and platelet activating factor. J Surg Res. (1988) 44:172–7. doi: 10.1016/0022-4804(88)90046-7
99. Mikelis CM, Simaan M, Ando K, Fukuhara S, Sakurai A, Amornphimoltham P, et al. RhoA and ROCK mediate histamine-induced vascular leakage and anaphylactic shock. Nat Commun. (2015) 6:6725. doi: 10.1038/ncomms7725
100. Sato M, Sasaki N, Ato M, Hirakawa S, Sato K, Sato K. Microcirculation-on-a-chip: a microfluidic platform for assaying blood- and lymphatic-vessel permeability. PLoS ONE. (2015) 10:e0137301. doi: 10.1371/journal.pone.0137301
101. von der Weid PY, Muthuchamy M. Regulatory mechanisms in lymphatic vessel contraction under normal and inflammatory conditions. Pathophysiology. (2010) 17:263–76. doi: 10.1016/j.pathophys.2009.10.005
102. Ohhashi T, Kawai Y, Azuma T. The response of lymphatic smooth muscles to vasoactive substances. Pflügers Archiv. (1978) 375:183–8. doi: 10.1007/BF00584242
103. Johnston MG, Kanalec A, Gordon JL. Effects of arachidonic acid and its cyclo-oxygenase and lipoxygenase products on lymphatic vessel contractility in vitro. Prostaglandins. (1983) 25:85–98. doi: 10.1016/0090-6980(83)90138-7
104. Orlov R, Lobov G. Ionic mechanisms of the electrical activity of the smooth-muscle cells of the lymphatic vessels. Fiziologicheskii Zhurnal SSSR Imeni IM Sechenova. (1984) 70:712–21.
105. Unthank JL, Hogan RD. The effect of vasoactive agents on the contractions of the initial lymphatics of the bat's wing. J Vasc Res. (1987) 24:31–44. doi: 10.1159/000158669
106. Watanabe N, Kawai Y, Ohhashi T. Dual effects of histamine on spontaneous activity in isolated bovine mesenteric lymphatics. Microvasc Res. (1988) 36:239–49. doi: 10.1016/0026-2862(88)90025-8
107. Dobbins D, Buehn M, Dabney J. Constriction of perfused lymphatics by acetylcholine, bradykinin and histamine. Microcirc Endothelium Lymphatics. (1990) 6:409–25.
108. Petunov S, Egorova A, Orlov R, Nikitina E. Effect of histamine on spontaneous contractions of mesenteric lymphatic vessels and lymph nodes of white rats: endothelium-dependent responses. Dokl Biol Sci. (2010) 432:176–80. doi: 10.1134/S0012496610030038
109. Pan'kova M, Lobov G, Chikhman V, Solnyshkin S. Effects of histamine on contractile activity of lymphatic node capsules. The NO role. Ross Fiziol Zh Im IM Sechenova. (2011) 97:633–40.
110. Breslin JW. ROCK and cAMP promote lymphatic endothelial cell barrier integrity and modulate histamine and thrombin-induced barrier dysfunction. Lymphat Res Biol. (2011) 9:3–11. doi: 10.1089/lrb.2010.0016
111. Jiang X, Nicolls MR, Tian W, Rockson SG. Lymphatic dysfunction, leukotrienes, and lymphedema. Annu Rev Physiol. (2018) 80:49–70. doi: 10.1146/annurev-physiol-022516-034008
112. Chan AK, von der Weid PY. 5-HT decreases contractile and electrical activities in lymphatic vessels of the guinea-pig mesentery: role of 5-HT 7-receptors. Br J Pharmacol. (2003) 139:243–54. doi: 10.1038/sj.bjp.0705264
113. Sawa Y, Sugimoto Y, Ueki T, Ishikawa H, Sato A, Nagato T, et al. Effects of TNF-alpha on leukocyte adhesion molecule expressions in cultured human lymphatic endothelium. J Histochem Cytochem. (2007) 55:721–33. doi: 10.1369/jhc.6A7171.2007
114. Hong H, Jiang L, Lin Y, He C, Zhu G, Du Q, et al. TNF-alpha promotes lymphangiogenesis and lymphatic metastasis of gallbladder cancer through the ERK1/2/AP-1/VEGF-D pathway. BMC Cancer. (2016) 16:240. doi: 10.1186/s12885-016-2259-4
115. Chen Y, Rehal S, Roizes S, Zhu HL, Cole WC, von der Weid PY. The pro-inflammatory cytokine TNF-alpha inhibits lymphatic pumping via activation of the NF-kappaB-iNOS signaling pathway. Microcirculation. (2017) 24:10.1111/micc.12364. doi: 10.1111/micc.12364
116. Baluk P, Yao LC, Feng J, Romano T, Jung SS, Schreiter JL, et al. TNF-alpha drives remodeling of blood vessels and lymphatics in sustained airway inflammation in mice. J Clin Invest. (2009) 119:2954–64. doi: 10.1172/JCI37626
117. Huang YH, Yang HY, Hsu YF, Chiu PT, Ou G, Hsu MJ. Src contributes to IL6-induced vascular endothelial growth factor-C expression in lymphatic endothelial cells. Angiogenesis. (2014) 17:407–18. doi: 10.1007/s10456-013-9386-1
118. Detoraki A, Staiano RI, Granata F, Giannattasio G, Prevete N, de Paulis A, et al. Vascular endothelial growth factors synthesized by human lung mast cells exert angiogenic effects. J Allergy Clin Immunol. (2009) 123:1142–9. 1149.e1–5. doi: 10.1016/j.jaci.2009.01.044
119. Lee KS, Kim SR, Park SJ, Min KH, Lee KY, Choe YH, et al. Mast cells can mediate vascular permeability through regulation of the PI3K-HIF-1alpha-VEGF axis. Am J Respir Crit Care Med. (2008) 178:787–97. doi: 10.1164/rccm.200801-008OC
120. Mullins RJ. Bradykinin causes a prolonged increase in skin microvascular permeability. J Surg Res. (1986) 40:540–9. doi: 10.1016/0022-4804(86)90096-X
121. Bell RD, Wainer BS. Effects of bradykinin on renal lymph flow and composition. Lymphology. (1983) 16:38–42.
122. Shi J, Li YJ, Yan B, Wei PK. Interleukin-8: a potent promoter of human lymphatic endothelial cell growth in gastric cancer. Oncol Rep. (2015) 33:2703–10. doi: 10.3892/or.2015.3916
123. Hastings NE, Feaver RE, Lee MY, Wamhoff BR, Blackman BR. Human IL-8 regulates smooth muscle cell VCAM-1 expression in response to endothelial cells exposed to atheroprone flow. Arterioscler Thromb Vasc Biol. (2009) 29:725–31. doi: 10.1161/ATVBAHA.109.184382
124. He A, Shi GP. Mast cell chymase and tryptase as targets for cardiovascular and metabolic diseases. Curr Pharm Des. (2013) 19:1114–25. doi: 10.2174/1381612811319060012
125. Compton SJ, Cairns JA, Holgate ST, Walls AF. The role of mast cell tryptase in regulating endothelial cell proliferation, cytokine release, and adhesion molecule expression: tryptase induces expression of mRNA for IL-1β and IL-8 and stimulates the selective release of IL-8 from human umbilical vein endothelial cells. J Immunol. (1998) 161:1939–46.
126. St John AL, Rathore AP, Raghavan B, Ng ML, Abraham SN. Contributions of mast cells and vasoactive products, leukotrienes and chymase, to dengue virus-induced vascular leakage. Elife. (2013) 2:e00481. doi: 10.7554/eLife.00481
127. Rehal S, Blanckaert P, Roizes S, von der Weid PY. Characterization of biosynthesis and modes of action of prostaglandin E2 and prostacyclin in guinea pig mesenteric lymphatic vessels. Br J Pharmacol. (2009) 158:1961–70. doi: 10.1111/j.1476-5381.2009.00493.x
128. Maejima D, Kawai Y, Ajima K, Ohhashi T. Platelet-derived growth factor (PDGF)-BB produces NO-mediated relaxation and PDGF receptor beta-dependent tonic contraction in murine iliac lymph vessels. Microcirculation. (2011) 18:474–86. doi: 10.1111/j.1549-8719.2011.00108.x
129. Liao CH, Akazawa H, Tamagawa M, Ito K, Yasuda N, Kudo Y, et al. Cardiac mast cells cause atrial fibrillation through PDGF-A-mediated fibrosis in pressure-overloaded mouse hearts. J Clin Invest. (2010) 120:242–53. doi: 10.1172/JCI39942
130. Cao R, Bjorndahl MA, Religa P, Clasper S, Garvin S, Galter D, et al. PDGF-BB induces intratumoral lymphangiogenesis and promotes lymphatic metastasis. Cancer Cell. (2004) 6:333–45. doi: 10.1016/j.ccr.2004.08.034
131. Artuc M, Steckelings UM, Henz BM. Mast cell-fibroblast interactions: human mast cells as source and inducers of fibroblast and epithelial growth factors. J Invest Dermatol. (2002) 118:391–5. doi: 10.1046/j.0022-202x.2001.01705.x
132. Chang LK, Garcia-Cardena G, Farnebo F, Fannon M, Chen EJ, Butterfield C, et al. Dose-dependent response of FGF-2 for lymphangiogenesis. Proc Natl Acad Sci USA. (2004) 101:11658–63. doi: 10.1073/pnas.0404272101
133. Cromer WE, Zawieja SD, Tharakan B, Childs EW, Newell MK, Zawieja DC. The effects of inflammatory cytokines on lymphatic endothelial barrier function. Angiogenesis. (2014) 17:395–406. doi: 10.1007/s10456-013-9393-2
134. Chrobak I, Lenna S, Stawski L, Trojanowska M. Interferon-γ promotes vascular remodeling in human microvascular endothelial cells by upregulating endothelin (ET)-1 and transforming growth factor (TGF) β2. J Cell Physiol. (2013) 228:1774–83. doi: 10.1002/jcp.24337
135. Gri G, Frossi B, D'Inca F, Danelli L, Betto E, Mion F, et al. Mast cell: an emerging partner in immune interaction. Front Immunol. (2012) 3:120. doi: 10.3389/fimmu.2012.00120
136. Bridenbaugh EA, Nizamutdinova IT, Jupiter D, Nagai T, Thangaswamy S, Chatterjee V, et al. Lymphatic muscle cells in rat mesenteric lymphatic vessels of various ages. Lymphat Res Biol. (2013) 11:35–42. doi: 10.1089/lrb.2012.0025
137. Zolla V, Nizamutdinova IT, Scharf B, Clement CC, Maejima D, Akl T, et al. Aging-related anatomical and biochemical changes in lymphatic collectors impair lymph transport, fluid homeostasis, and pathogen clearance. Aging Cell. (2015) 14:582–94. doi: 10.1111/acel.12330
138. Gasheva OY, Knippa K, Nepiushchikh ZV, Muthuchamy M, Gashev AA. Age-related alterations of active pumping mechanisms in rat thoracic duct. Microcirculation. (2007) 14:827–39. doi: 10.1080/10739680701444065
139. Nagai T, Bridenbaugh EA, Gashev AA. Aging-associated alterations in contractility of rat mesenteric lymphatic vessels. Microcirculation. (2011) 18:463–73. doi: 10.1111/j.1549-8719.2011.00107.x
140. Akl TJ, Nagai T, Cote GL, Gashev AA. Mesenteric lymph flow in adult and aged rats. Am J Physiol Heart Circ Physiol. (2011) 301:H1828–40. doi: 10.1152/ajpheart.00538.2011
141. Thangaswamy S, Bridenbaugh EA, Gashev AA. Evidence of increased oxidative stress in aged mesenteric lymphatic vessels. Lymphat Res Biol. (2012) 10:53–62. doi: 10.1089/lrb.2011.0022
142. Gashev AA, Chatterjee V. Aged lymphatic contractility: recent answers and new questions. Lymphat Res Biol. (2013) 11:2–13. doi: 10.1089/lrb.2013.0003
143. Pal S, Meininger C, Gashev A. aged lymphatic vessels and mast cells in perilymphatic tissues. Int J Mol Sci. (2017) 18:965. doi: 10.3390/ijms18050965
144. Breslin JW. Mechanical forces and lymphatic transport. Microvasc Res. (2014) 96:46–54. doi: 10.1016/j.mvr.2014.07.013
145. Randolph GJ, Angeli V, Swartz MA. Dendritic-cell trafficking to lymph nodes through lymphatic vessels. Nat Rev Immunol. (2005) 5:617–28. doi: 10.1038/nri1670
146. Hunter MC, Teijeira A, Montecchi R, Russo E, Runge P, Kiefer F, et al. Dendritic cells and T cells interact within murine afferent lymphatic capillaries. Front Immunol. (2019) 10:520. doi: 10.3389/fimmu.2019.00520
147. Kuan EL, Ivanov S, Bridenbaugh EA, Victora G, Wang W, Childs EW, et al. Collecting lymphatic vessel permeability facilitates adipose tissue inflammation and distribution of antigen to lymph node-homing adipose tissue dendritic cells. J Immunol. (2015) 194:5200–10. doi: 10.4049/jimmunol.1500221
148. Johnson LA, Clasper S, Holt AP, Lalor PF, Baban D, Jackson DG. An inflammation-induced mechanism for leukocyte transmigration across lymphatic vessel endothelium. J Exp Med. (2006) 203:2763–77. doi: 10.1084/jem.20051759
149. Miteva DO, Rutkowski JM, Dixon JB, Kilarski W, Shields JD, Swartz MA. Transmural flow modulates cell and fluid transport functions of lymphatic endothelium. Circ Res. (2010) 106:920–31. doi: 10.1161/CIRCRESAHA.109.207274
150. Kataru RP, Baik JE, Park HJ, Wiser I, Rehal S, Shin JY, et al. Regulation of immune function by the lymphatic system in lymphedema. Front Immunol. (2019) 10:470. doi: 10.3389/fimmu.2019.00470
151. McKimmie CS, Singh MD, Hewit K, Lopez-Franco O, Le Brocq M, Rose-John S, et al. An analysis of the function and expression of D6 on lymphatic endothelial cells. Blood. (2013) 121:3768–77. doi: 10.1182/blood-2012-04-425314
152. Kim H, Kataru RP, Koh GY. Inflammation-associated lymphangiogenesis: a double-edged sword? J Clin Invest. (2014) 124:936–42. doi: 10.1172/JCI71607
153. Mortimer PS, Rockson SG. New developments in clinical aspects of lymphatic disease. J Clin Invest. (2014) 124:915–21. doi: 10.1172/JCI71608
154. Ehrlich P. Beitrage zur theorie und praxis der histologischen färbung (thesis). University of Leipzig, Leipzig, Germany (1878).
155. Elpek GÖ, Gelen T, Aksoy N, Erdogan A, Dertsiz L, Demircan A, et al. The prognostic relevance of angiogenesis and mast cells in squamous cell carcinoma of the oesophagus. J Clin Pathol. (2001) 54:940–4. doi: 10.1136/jcp.54.12.940
156. Esposito I, Menicagli M, Funel N, Bergmann F, Boggi U, Mosca F, et al. Inflammatory cells contribute to the generation of an angiogenic phenotype in pancreatic ductal adenocarcinoma. J Clin Pathol. (2004) 57:630–6. doi: 10.1136/jcp.2003.014498
157. Strouch MJ, Cheon EC, Salabat MR, Krantz SB, Gounaris E, Melstrom LG, et al. Crosstalk between mast cells and pancreatic cancer cells contributes to pancreatic tumor progression. Clin Cancer Res. (2010) 16:2257–65. doi: 10.1158/1078-0432.CCR-09-1230
158. Cai S-W, Yang S-Z, Gao J, Pan K, Chen Y, Wang L, et al. Prognostic significance of mast cell count following curative resection for pancreatic ductal adenocarcinoma. Surgery. (2011) 149:576–84. doi: 10.1016/j.surg.2010.10.009
159. Johansson A, Rudolfsson S, Hammarsten P, Halin S, Pietras K, Jones J, et al. Mast cells are novel independent prognostic markers in prostate cancer and represent a target for therapy. Am J Pathol. (2010) 177:1031–41. doi: 10.2353/ajpath.2010.100070
160. Reddy SM, Reuben A, Barua S, Jiang H, Zhang S, Wang L, et al. Poor response to neoadjuvant chemotherapy correlates with mast cell infiltration in inflammatory breast cancer. Cancer Immunol Res. (2019) 7:1025–35. doi: 10.1158/2326-6066.CIR-18-0619
161. Ranieri G, Ammendola M, Patruno R, Celano G, Zito FA, Montemurro S, et al. Tryptase-positive mast cells correlate with angiogenesis in early breast cancer patients. Int J Oncol. (2009) 35:115–20. doi: 10.3892/ijo_00000319
162. Englund A, Molin D, Enblad G, Karlén J, Glimelius I, Ljungman G, et al. The role of tumour-infiltrating eosinophils, mast cells and macrophages in classical and nodular lymphocyte predominant hodgkin lymphoma in children. Eur J Haematol. (2016) 97:430–8. doi: 10.1111/ejh.12747
163. Parker L, Stepto NK, Shaw CS, Serpiello FR, Anderson M, Hare DL, et al. Acute high-intensity interval exercise-induced redox signaling is associated with enhanced insulin sensitivity in obese middle-aged men. Front Physiol. (2016) 7:411. doi: 10.3389/fphys.2016.00411
164. Molin D, Edström A, Glimelius I, Glimelius B, Nilsson G, Sundström C, et al. Mast cell infiltration correlates with poor prognosis in Hodgkin's lymphoma. Br J Haematol. (2002) 119:122–24. doi: 10.1046/j.1365-2141.2002.03768.x
165. Varricchi G, Galdiero MR, Loffredo S, Marone G, Iannone R, Marone G, et al. Are mast cells MASTers in cancer? Front Immunol. (2017) 8:424. doi: 10.3389/fimmu.2017.00424
166. Maciel TT, Moura IC, Hermine O. The role of mast cells in cancers. F1000Prime Rep. (2015) 7:09–09. doi: 10.12703/P7-09
167. Marichal T, Tsai M, Galli SJ. Mast cells: potential positive and negative roles in tumor biology. Cancer Immunol Res. (2013) 1:269–79. doi: 10.1158/2326-6066.CIR-13-0119
168. Stelekati E, Bahri R, D'Orlando O, Orinska Z H., -Mittrücker W, Langenhaun R, et al. Mast cell-mediated antigen presentation regulates CD8+ T cell effector functions. Immunity. (2009) 31:665–76. doi: 10.1016/j.immuni.2009.08.022
169. Nakae S, Suto H, Iikura M, Kakurai M, Sedgwick JD, Tsai M, et al. Mast cells enhance T cell activation: importance of mast cell costimulatory molecules and secreted TNF. J Immunol. (2006) 176:2238–48. doi: 10.4049/jimmunol.176.4.2238
170. Hiraoka N, Ino Y, Yamazaki-Itoh R. Tertiary lymphoid organs in cancer tissues. Front Immunol. (2016) 7:244. doi: 10.3389/fimmu.2016.00244
171. Bowers HM Jr, Mahapatro RC, Kennedy JW. Numbers of mast cells in the axillary lymph nodes of breast cancer patients. Cancer. (1979) 43:568–73.
172. Malaviya R, Twesten NJ, Ross EA, Abraham SN, Pfeifer JD. Mast cells process bacterial Ags through a phagocytic route for class I MHC presentation to T cells. J Immunol. (1996) 156:1490–6.
173. Zsebo KM, Williams DA, Geissler EN, Broudy VC, Martin FH, Atkins HL, et al. Stem cell factor is encoded at the Sl locus of the mouse and is the ligand for the c-kit tyrosine kinase receptor. Cell. (1990) 63:213–24. doi: 10.1016/0092-8674(90)90302-U
174. Zhang W, Stoica G, Tasca SI, Kelly KA, Meininger CJ. Modulation of tumor angiogenesis by stem cell factor. Cancer Res. (2000) 60:6757–62.
175. Huang B, Lei Z, Zhang GM, Li D, Song C, Li B, et al. SCF-mediated mast cell infiltration and activation exacerbate the inflammation and immunosuppression in tumor microenvironment. Blood. (2008) 112:1269–79. doi: 10.1182/blood-2008-03-147033
176. Stacker SA, Williams SP, Karnezis T, Shayan R, Fox SB, Achen MG. Lymphangiogenesis and lymphatic vessel remodelling in cancer. Nat Rev Cancer. (2014) 14:159. doi: 10.1038/nrc3677
177. Stacker SA, Achen MG, Jussila L, Baldwin ME, Alitalo K. Metastasis: lymphangiogenesis and cancer metastasis. Nat Rev Cancer. (2002) 2:573. doi: 10.1038/nrc863
178. Farnsworth RH, Achen MG, Stacker SA. The evolving role of lymphatics in cancer metastasis. Curr Opin Immunol. (2018) 53:64–73. doi: 10.1016/j.coi.2018.04.008
179. Ji RC. Lymph nodes and cancer metastasis: new perspectives on the role of intranodal lymphatic sinuses. Int J Mol Sci. (2016) 18:51. doi: 10.3390/ijms18010051
180. Raica M, Cimpean AM, Ceauşu R, Ribatti D, Gaje P. Interplay between mast cells and lymphatic vessels in different molecular types of breast cancer. Anticancer Res. (2013) 33:957–63.
181. Utrera-Barillas D, Castro-Manrreza M, Castellanos E, Gutierrez-Rodriguez M O., Arciniega-Ruiz de Esparza, Garcia-Cebada J, Velazquez JR, Flores-Resendiz D, Hernandez-Hernandez D, Benitez-Bribiesca L. The role of macrophages and mast cells in lymphangiogenesis and angiogenesis in cervical carcinogenesis. Exp Mol Pathol. (2010) 89:190–6. doi: 10.1016/j.yexmp.2010.06.002
182. Skobe M, Hawighorst T, Jackson DG, Prevo R, Janes L, Velasco P, et al. Induction of tumor lymphangiogenesis by VEGF-C promotes breast cancer metastasis. Nat Med. (2001) 7:192. doi: 10.1038/84643
183. Hirakawa S, Brown LF, Kodama S, Paavonen K, Alitalo K, Detmar M. VEGF-C-induced lymphangiogenesis in sentinel lymph nodes promotes tumor metastasis to distant sites. Blood. (2007) 109:1010–17. doi: 10.1182/blood-2006-05-021758
184. Karpanen T, Egeblad M, Karkkainen MJ, Kubo H, Ylä-Herttuala S, Jäättelä M, et al. Vascular endothelial growth factor C promotes tumor lymphangiogenesis and intralymphatic tumor growth. Cancer Res. (2001) 61:1786–90.
185. Akagi K, Ikeda Y, Miyazaki M, Abe T, Kinoshita J, Maehara Y, et al. Vascular endothelial growth factor-C (VEGF-C) expression in human colorectal cancer tissues. Br J Cancer. (2000) 83:887–91. doi: 10.1054/bjoc.2000.1396
186. Tang RF, Itakura J, Aikawa T, Matsuda K, Fujii H, Korc M, et al. Overexpression of lymphangiogenic growth factor VEGF-C in human pancreatic cancer. Pancreas. (2001) 22:285–92. doi: 10.1097/00006676-200104000-00010
187. Hoshida T, Isaka N, Hagendoorn J, di Tomaso E, Chen YL, Pytowski B, et al. Imaging steps of lymphatic metastasis reveals that vascular endothelial growth factor-C increases metastasis by increasing delivery of cancer cells to lymph nodes: therapeutic implications. Cancer Res. (2006) 66:8065–75. doi: 10.1158/0008-5472.CAN-06-1392
188. Visciano C, Prevete N, Liotti F, Marone G. Tumor-associated mast cells in thyroid cancer. Int J Endocrinol. (2015) 2015:705169. doi: 10.1155/2015/705169
189. Visciano C, Liotti F, Prevete N, Cali G, Franco R, Collina F, et al. Mast cells induce epithelial-to-mesenchymal transition and stem cell features in human thyroid cancer cells through an IL-8-Akt-Slug pathway. Oncogene. (2015) 34:5175–86. doi: 10.1038/onc.2014.441
190. Baram D, Vaday GG, Salamon P, Drucker I, Hershkoviz R, Mekori YA. Human mast cells release metalloproteinase-9 on contact with activated T cells: juxtacrine regulation by TNF-α. J Immunol. (2001) 167:4008–16. doi: 10.4049/jimmunol.167.7.4008
191. Schwartz L, Irani A, Roller K, Castells M, Schechter N. Quantitation of histamine, tryptase, and chymase in dispersed human T and TC mast cells. J Immunol. (1987) 138:2611–15.
192. Ammendola M, Sacco R, Donato G, Zuccalà V, Russo E, Luposella M, et al. Mast cell positivity to tryptase correlates with metastatic lymph nodes in gastrointestinal cancer patients treated surgically. Oncology. (2013) 85:111–16. doi: 10.1159/000351145
193. Yuan H, Hsiao Y-H, Zhang Y, Wang J, Yin C, Shen R, et al. Destructive impact of T-lymphocytes, NK and Mast cells on basal cell layers: implications for tumor invasion. BMC Cancer. (2013) 13:258. doi: 10.1186/1471-2407-13-258
194. Derakhshani A, Vahidian F, Alihasanzadeh M, Mokhtarzadeh A, Lotfi Nezhad P, Baradaran B. Mast cells: a double-edged sword in cancer. Immunol Lett. (2019) 209:28–35. doi: 10.1016/j.imlet.2019.03.011
Keywords: mast cells, lymphatic vessels, lymphatic system, immune response, cancer
Citation: Pal S, Nath S, Meininger CJ and Gashev AA (2020) Emerging Roles of Mast Cells in the Regulation of Lymphatic Immuno-Physiology. Front. Immunol. 11:1234. doi: 10.3389/fimmu.2020.01234
Received: 29 March 2020; Accepted: 18 May 2020;
Published: 17 June 2020.
Edited by:
Ursula Grohmann, University of Perugia, ItalyReviewed by:
Ilze Bot, Leiden University, NetherlandsJeffrey Louis Curtis, University of Michigan School of Medicine, United States
Copyright © 2020 Pal, Nath, Meininger and Gashev. This is an open-access article distributed under the terms of the Creative Commons Attribution License (CC BY). The use, distribution or reproduction in other forums is permitted, provided the original author(s) and the copyright owner(s) are credited and that the original publication in this journal is cited, in accordance with accepted academic practice. No use, distribution or reproduction is permitted which does not comply with these terms.
*Correspondence: Anatoliy A. Gashev, Z2FzaGV2QHRhbXUuZWR1
†These authors have contributed equally to this work